- 1Department of Biology, Duke University, Durham, NC, United States
- 2W. Szafer Institute of Botany, Polish Academy of Sciences (PAS), Kraków, Poland
Shifts in climate along elevation gradients structure mycobiont–photobiont associations in lichens. We obtained mycobiont (lecanoroid Lecanoraceae) and photobiont (Trebouxia alga) DNA sequences from 89 lichen thalli collected in Bolivia from a ca. 4,700 m elevation gradient encompassing diverse natural communities and environmental conditions. The molecular dataset included six mycobiont loci (ITS, nrLSU, mtSSU, RPB1, RPB2, and MCM7) and two photobiont loci (ITS, rbcL); we designed new primers to amplify Lecanoraceae RPB1 and RPB2 with a nested PCR approach. Mycobionts belonged to Lecanora s.lat., Bryonora, Myriolecis, Protoparmeliopsis, the “Lecanora” polytropa group, and the “L.” saligna group. All of these clades except for Lecanora s.lat. occurred only at high elevation. No single species of Lecanoraceae was present along the entire elevation gradient, and individual clades were restricted to a subset of the gradient. Most Lecanoraceae samples represent species which have not previously been sequenced. Trebouxia clade C, which has not previously been recorded in association with species of Lecanoraceae, predominates at low- to mid-elevation sites. Photobionts from Trebouxia clade I occur at the upper extent of mid-elevation forest and at some open, high-elevation sites, while Trebouxia clades A and S dominate open habitats at high elevation. We did not find Trebouxia clade D. Several putative new species were found in Trebouxia clades A, C, and I. These included one putative species in clade A associated with Myriolecis species growing on limestone at high elevation and a novel lineage sister to the rest of clade C associated with Lecanora on bark in low-elevation grassland. Three different kinds of photobiont switching were observed, with certain mycobiont species associating with Trebouxia from different major clades, species within a major clade, or haplotypes within a species. Lecanoraceae mycobionts and Trebouxia photobionts exhibit species turnover along the elevation gradient, but with each partner having a different elevation threshold at which the community shifts completely. A phylogenetically defined sampling of a single diverse family of lichen-forming fungi may be sufficient to document regional patterns of Trebouxia diversity and distribution.
Introduction
Alexander von Humboldt’s Essai sur La Géographie des Plantes was revolutionary for biogeography (Schrodt et al., 2019), but, with respect to lichens, Humboldt was limited by the nascent state of lichenology in the early 19th century. Drawing on observations from Europe and South America, Humboldt described lichens as “independent of the influence of the climates” (von Humboldt and Bonpland, 2009)—a statement that, with a modern understanding of lichen systematics and symbiosis, we now know is far from the truth. Climate does matter for lichens. There is turnover in lichenized fungi along elevation gradients, including those in the northern Andes (Wolf, 1993; Soto-Medina et al., 2019) that inspired Humboldt’s work. Furthermore, the mycobiont–photobiont interaction that defines the lichen symbiosis is affected by environmental conditions at micro and macro scales (e.g., James and Henssen, 1976; Peksa and Škaloud, 2011; Singh et al., 2017). What appears externally as the same lichen may actually represent a mycobiont species occurring with different photobiont species at different points in its range (Werth and Sork, 2014; Dal Grande et al., 2018).
Lichen photobiont biodiversity and patterns in the mycobiont–photobiont association have been most commonly studied at a regional, intra-biome scale at which climate is largely consistent [Hestmark et al., 2016; Jüriado et al., 2019; Yahr et al., 2004; but see Lu et al. (2018) for an intra-biome study along a climatic gradient] or at a global scale, at which climate may vary widely but regional patterns may not be apparent (Muggia et al., 2014; Nyati et al., 2014; Lutsak et al., 2016; Magain et al., 2017, 2018; Vančurová et al., 2018; Chagnon et al., 2019). Less commonly, regional photobiont turnover across adjacent biomes has been studied along gradients of latitude (Werth and Sork, 2014) or altitude (Dal Grande et al., 2018). In most studies, investigations of lichen photobionts have been structured around a specific mycobiont taxon, whether that taxon is a species (e.g., Blaha et al., 2006; Werth and Sork, 2014), genus (e.g., Vargas Castillo and Beck, 2012; Magain et al., 2017, 2018), or family (e.g., Helms, 2003; Nyati et al., 2014).
Lecanoraceae is one of the three largest families of lichenized fungi that associate with the green alga Trebouxia, the most common genus of lichen photobionts (Miadlikowska et al., 2006; Lücking et al., 2017; Muggia et al., 2018). The family is cosmopolitan and occurs on a range of substrates, typically with a crustose growth form (Brodo et al., 2001). Despite the family’s global distribution and diversity of corticolous species, the literature on the Lecanoraceae–Trebouxia association has mostly dealt with mycobiont taxa that are saxicolous, phylogenetically outside Lecanora s.str., and from Europe, North America, or Antarctica (Blaha et al., 2006; Guzow-Krzemińska, 2006; Pérez-Ortega et al., 2012; Ruprecht et al., 2012, 2020; Leavitt et al., 2015, 2016; Wagner et al., 2020). Photobiont studies that have included corticolous species of Lecanoraceae have generally done so in the context of sampling photobionts from across the lichen community of a small geographic area in the northern hemisphere (e.g., Singh et al., 2019).
Trebouxia is divided into five major clades: A, C, D, I, and S (Muggia et al., 2020; Xu et al., 2020). Clade D was recently identified and is known from Iceland, Svalbard, and Tierra del Fuego (Xu et al., 2020). Clade C is largely tropical, with a few temperate representatives. The remaining clades, A, I, and S, are cosmopolitan, including Antarctica. At the level of these major clades, lineages are distinguished by differences in pyrenoid structure (Muggia et al., 2020). The species-level classification of Trebouxia is still incompletely understood. Leavitt et al. (2015, 2016) and Muggia et al. (2020) implemented an alphanumeric classification system for putative Trebouxia species based on a so-called “barcode gap” species delimitation method (Puillandre et al., 2012). Although some of the species recognized by Muggia et al. (2020) correspond to named taxa, the majority have not been formally described.
In this paper, we investigate mycobiont–photobiont interactions in Lecanoraceae lichens from across an elevation gradient in Bolivia. Recent studies on lichen photobionts in Bolivia have uncovered putative new species of Trentepohlia and Asterochloris (Kosecka et al., 2020, 2021a), but the diversity of Trebouxia photobionts in Bolivia remains largely unexplored outside of one global study on photobionts of Cetraria aculeata (Lutsak et al., 2016). Our dataset includes corticolous and saxicolous species from Lecanora and other genera in Lecanoraceae and encompasses nearly 5,000 m of elevation change in the Andes mountains, south of where Humboldt developed his ideas on biogeography. We use this dataset to ask three main questions: (1) How is the biodiversity of Bolivian Lecanoraceae distributed, both in the phylogeny of this fungal family and along the elevation gradient? (2) What major clades and species of Trebouxia are associated with Lecanoraceae in Bolivia? (3) How do climate, substrate, and mycobiont host structure photobiont communities?
Materials and Methods
Field Sampling
Specimens of Bolivian lichens suspected of belonging to Lecanoraceae based on their morphology were collected from 2004 to 2019. Collection sites spanned over 4,700 m of elevation and included diverse natural communities across multiple biomes (Navarro and Maldonado, 2002; Navarro and Ferreira, 2007). Habitats ranged from patches of trees in Los Llanos de Moxos savanna below 200 m, to Yungas and Tucumano-Boliviano montane forests at mid-elevation sites, to dry, rocky grasslands of the high Andean Puna at about 4,000 m and subnival vegetation above 4,600 m (Figure 1). Voucher specimens are stored in KRAM and LPB.
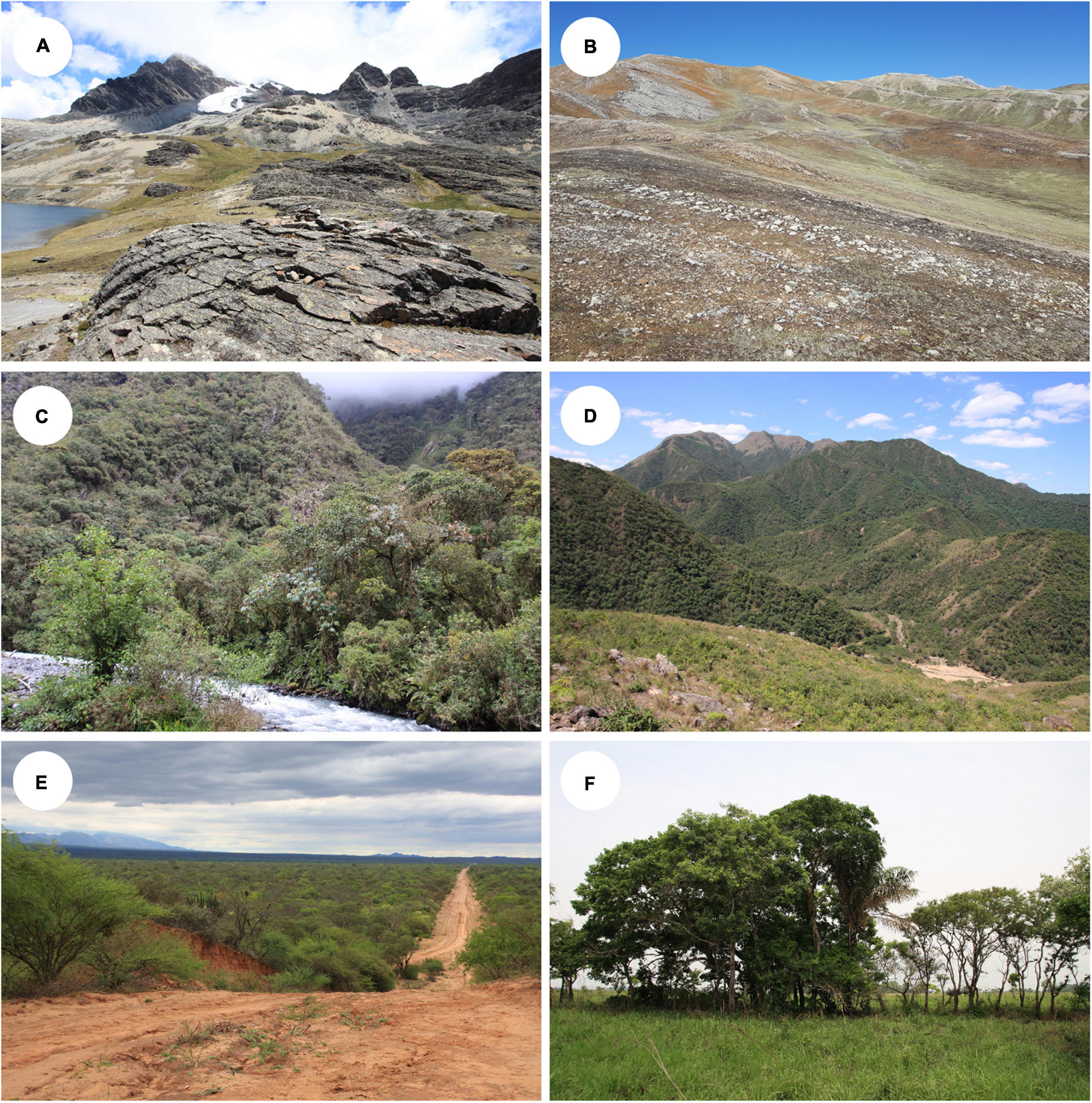
Figure 1. Habitats where Lecanoraceae lichens were collected. (A) La Paz, Bautista Saavedra, 14°48’9”S, 69°10’51”W, 4850 m, open high Andean vegetation with siliceous rocks. (B) La Paz, Bautista Saavedra, 15°15’0”S, 69°2’50”W, 4549 m, open high Andean area with limestone rocks. (C) La Paz, Franz Tamayo, 14°46’39”S, 69°0’35”W, 2550 m, Yungas montane cloud forest. (D) Tarija, Aniceto Arce, 21°41’36”S, 64°29’33”W, 2195 m, Tucumano-Boliviano montane forest. (E) Santa Cruz, Cordillera, 18°27’29”S, 61°23’1”W, 292 m, Chaqueño forest. (F) Beni, Yacuma, 14°51’7”S, 66°20’23”W, 175 m, Los Llanos de Moxos savanna. All photographs by AF.
Morphological Studies
Morphology and anatomy were studied by standard techniques. Cross sections of apothecia were mounted in water for observation of apothecium anatomy or in ca. 25% KOH for observation of ascospores. Crystals were observed under polarized light in a compound fluorescence microscope (Nikon Eclipse 80i) and their solubility was tested with KOH and 65% nitric acid. Lichen secondary metabolites were analyzed by thin-layer chromatography (TLC) in solvents A, B’, and C following Culberson and Kristinsson (1970) and Orange et al. (2001). For saxicolous samples, rocks were tested for carbonate minerals with 10% HCl.
DNA Extraction, PCR, and Sequencing
From a collection of approximately 550 specimens, 235 specimens representing diverse morphotypes and habitats were selected for molecular study. Note that the genus Lecidella, although part of Lecanoraceae, was not targeted in this sampling focused on lecanoroid species. Material collected in the years 2015–2018 was kept frozen at −20°C prior to DNA extraction, while collections from earlier years were stored under standard herbarium conditions. Specimens collected in 2019 were extracted when fresh. Apothecia excised from a single thallus—or soralia, if apothecia were absent—were cleaned in sterile, distilled water on a microscope slide. Visible contaminants (e.g., lichenicolous fungi) were removed with ultrathin tweezers and a razor blade. DNA was isolated using either the QIAamp DNA Investigator Kit or DNeasyTM Plant Mini Kit (Qiagen, Germany), depending on the amount of lichen tissue available, following the manufacturer’s instructions.
To conduct a multi-locus phylogenetic analysis of Lecanoraceae, we amplified the mycobiont nuclear ribosomal large subunit (nrLSU), internal transcribed spacer (ITS), mitochondrial small subunit (mtSSU), RNA polymerase II largest (RPB1) and second-largest (RPB2) subunits, and minichromosome maintenance factor 7 (MCM7). The ribosomal RNA (rRNA) loci ITS, mtSSU, and to some extent nrLSU have been frequently sequenced for phylogenetic studies of Lecanoraceae and represent most of the reference data for this family on GenBank. The ITS region, including the ITS1 spacer, 5.8S rRNA, and ITS2 spacer, was amplified with primers ITS1F (Gardes and Bruns, 1993) and either LR3 or ITS4 (Vilgalys and Hester, 1990; White et al., 1990); nrLSU was amplified with primers AL2R and LR6 (Vilgalys and Hester, 1990; Döring et al., 2000); and mtSSU was amplified with primers MSU1 and MSU7 (Zhou and Stanosz, 2001). Thermal cycler conditions for the three rRNA loci were 95°C for 3 min, followed by 35 cycles of 95°C for 40 s, 52°C for 40 s, and 72°C for 150 s, with a final extension at 72°C for 10 min.
Due to the very small amount of DNA present in our extractions, the protein-coding loci RPB1, RPB2, and MCM7 were amplified with a nested PCR approach. For RPB1, the initial amplification was performed with primers RPB1-Af and either RPB1-Cr or RPB1-Dr (Stiller and Hall, 1997; Matheny et al., 2002). In the first round of PCR, the thermal cycler conditions were 94°C for 10 min, followed by 25 cycles of 94°C for 45 s, 50°C for 50 s, and 72°C for 100 s, with a final extension at 72°C for 10 min. The second round of PCR used forward and reverse primers lecRPB1-F and lecRPB1-R (Table 1), which we designed based on the Lecanoraceae RPB1 alignment from Zhao et al. (2016). Suitable priming sites were identified by visual inspection of alignments in Mesquite (Maddison and Maddison, 2018) and potential primer sequences were evaluated using online tools1,2. The thermal cycler conditions for the second round were 94°C for 5 min, followed by 35 cycles of 94°C for 45 s, 55°C for 60 s, and 72°C for 90 s, with a final extension at 72°C for 10 min. For RPB2, the first round of PCR used primers fRPB2-5F and fRPB2-7cR (Liu et al., 1999), and thermal cycler conditions were identical to the first-round program for RPB1. The second round used primers lecRPB2-6F and fRPB2-7cR. The newly designed forward primer lecRPB2-6F (Table 1) was modified from bRPB2-6F (Matheny, 2005) using Lecanoraceae sequences from Miadlikowska et al. (2014). Thermal cycler conditions for this round were the same as for the first round, except that the number of cycles was increased to 35 and the extension time was reduced to 85 sec per cycle. For MCM7, the first round of PCR used primers MCM7-709f and MCM7-1443r, while the second round used MCM7-709f and MCM7-1348r (Schmitt et al., 2009). The thermal cycler programs for both rounds followed Schmitt et al. (2009), except that the number of cycles in the first round was reduced to 25.
Sanger sequencing of PCR amplicons has been shown to reliably recover the dominant photobiont in Trebouxia-associated lichens even when other photobiont genotypes are present at low levels (Paul et al., 2018). Photobiont ITS was amplified with primers ITS1T and ITS4T (Kroken and Taylor, 2000) using the thermal cycler program described above for the mycobiont rRNA loci. For a subset of specimens, we also amplified a portion of the Rubisco large subunit (rbcL) with primers a-ch-rbcL-203-5’-MPN and a-ch-rbcL-991-3’-MPN (Nelsen et al., 2011). Thermal cycler conditions followed Nelsen et al. (2011). Selection of specimens for rbcL sequencing was based on the photobiont ITS results and focused on specimens that were: (1) outliers in the elevation distribution or host breadth of a photobiont clade, (2) photobionts that appeared to be new species-level lineages, (3) species-level photobiont lineages only found in a single specimen, or (4) previously recognized Trebouxia lineages for which rbcL data were lacking.
PCR amplicons were checked on 1% agarose gels to confirm the presence of a single fragment size and cleaned following an enzymatic cleanup protocol with exonuclease I and shrimp alkaline phosphatase (ThermoFisher Scientific, Waltham, MA, United States). Sanger sequencing was performed by Eurofins Genomics (Louisville, KY, United States). Sequencing reactions used PCR primers except in two cases. Primers mrSSU1 and mrSSU3R (Zoller et al., 1999) were used in place of MSU1 and MSU7 to sequence the mtSSU amplicons. A newly designed primer, lecRPB2-seq7R (Table 1) was used in place of fRPB2-7cR for sequencing the RPB2 amplicons. Forward and reverse sequence reads were assembled, trimmed, and checked for base-calling errors in SeqMan Pro (DNASTAR, Madison, WI, United States) or Geneious Prime 2021.0.33. When ITS and partial nrLSU were sequenced as one amplicon, they were split using the program ITSx (Bengtsson-Palme et al., 2013). Sequences were checked for contamination or specimen misidentification using two complementary methods: (1) BLAST with the NCBI nucleotide database and (2) placement in a phylogeny of Lecanoromycetes with T-BAS (Miadlikowska et al., 2014; Carbone et al., 2017, 2019). GenBank numbers for all newly obtained sequences are given in Supplementary Data Sheet 1.
Alignments and Phylogenetic Analyses
We compiled a reference dataset (Supplementary Data Sheet 2) of Lecanoraceae and outgroups based on taxa included in Miadlikowska et al. (2014) and Zhao et al. (2016). In general, reference taxa were only included if multiple loci, including protein-coding loci, were available. The families Parmeliaceae (represented by one species each of Protoparmelia and Letharia) and Gypsoplacaceae (represented by two species of Gypsoplaca) were used as outgroups. Our Lecanoraceae dataset included several species groups within Lecanora, Palicella, Lecidella, Protoparmeliopsis, Rhizoplaca, Myriolecis, the “Lecanora” polytropa group, and the “Lecanora” saligna group, as well as Haematomma, Ramboldia, and Miriquidica. Reference sequences were checked for specimen misidentifications or other metadata errors using BLAST with the NCBI nucleotide database.
The mycobiont rRNA loci (mycobiont ITS, nrLSU, and mtSSU) were initially aligned using the MAFFT online server4 with the G-INS-1 option (Katoh et al., 2019). The alignments were corrected by eye in Mesquite (Maddison and Maddison, 2018) and introns and ambiguously aligned regions were delimited manually and excluded from downstream analyses (Lutzoni et al., 2000). Protein-coding loci were aligned by translated amino acid in Mesquite. Introns were delimited manually and excluded from downstream analyses.
Each single-locus alignment was used as input for a maximum likelihood phylogenetic analysis in IQ-TREE version 2.1.2 (Nguyen et al., 2015; Chernomor et al., 2016) run on the CIPRES server (Miller et al., 2010). We performed 5,000 ultrafast bootstrap pseudoreplicates to calculate support for each single-locus tree (Hoang et al., 2018). We inspected the resulting trees for well-supported conflicts (≥95% ultrafast bootstrap; Hoang et al., 2018) among the six mycobiont loci, which were then concatenated to form a single dataset. The concatenated mycobiont alignment was used as input for a partitioned maximum likelihood analysis in IQ-TREE. We ran IQ-TREE with the -p and -m MFP + MERGE options so that ModelFinder (Kalyaanamoorthy et al., 2017) would both optimize the partitioning scheme and find the best-fitting substitution model for each partition. The input partitioning scheme divided the concatenated alignment by locus and by codon position for the protein-coding loci. The final partitioning schemes and substitution models used in this analysis are provided in Table 2. We performed 5,000 ultrafast bootstrap pseudoreplicates to calculate bipartition support for the tree topology.
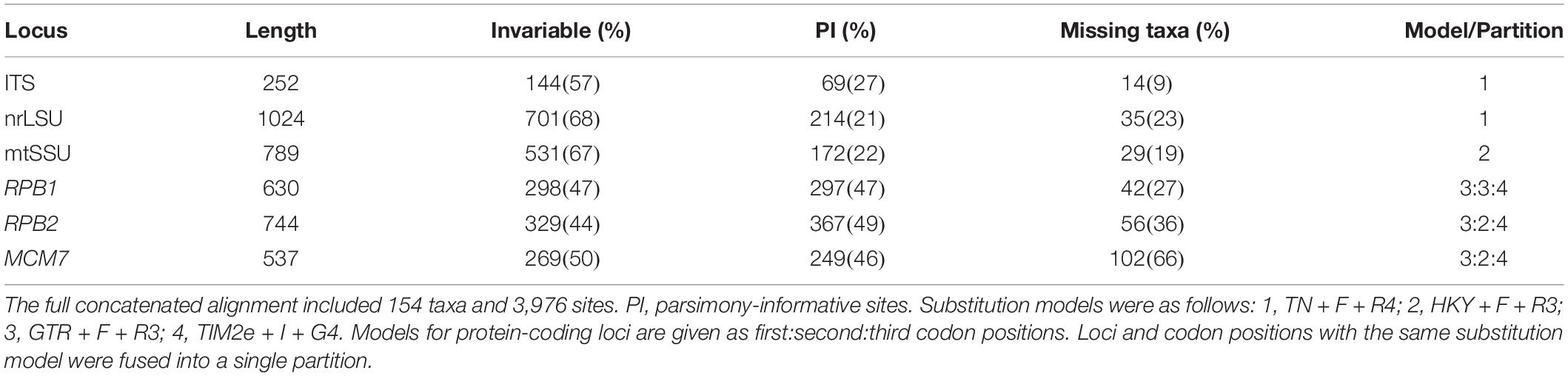
Table 2. Statistics on the alignments for Lecanoraceae (mycobiont) after the removal of introns and ambiguously aligned regions.
We performed two phylogenetic analyses with the photobiont data. First, all sequences were included in a global ITS-rbcL alignment for Trebouxia to assign each sample to one of the major Trebouxia clades. We used the ITS and rbcL sequences curated by Muggia et al. (2020) as a reference dataset, with slight modifications. These sequences are listed in the file “Supplementary Data 2” from Muggia et al. (2020). We removed sequence L138 from the Trebouxia clade S ITS alignment and sequences L1107, L1360, L1401, L1417, L1425, L1504, AJ007387, AJ969549, AJ249482, and 9493 from the Trebouxia clade A ITS alignment because they were missing a significant portion of ITS1 or ITS2. None of these ITS sequences had a corresponding rbcL sequence. L1107 and 9493 were the only representatives of the putative species A37 and A49, respectively, but preliminary analyses indicated that our new sequences were not closely related to these taxa. We also added all ITS and rbcL reference sequences for Trebouxia clade D from “Supplementary Table 5” of Xu et al. (2020). Representatives from three other genera of Trebouxiophyceae were used as outgroup taxa: Asterochloris, GenBank accession JN573844; Myrmecia, KM462861; and Vulcanochloris, KR952313. Only rbcL was included for the outgroup taxa because ITS was not alignable across the four genera.
ITS sequences were initially aligned with MAFFT using the G-INS-1 option and regions that were not conserved across Trebouxia were excluded by eye in Mesquite; rbcL sequences were aligned by translated amino acid in Mesquite. Phylogenies for each locus were inferred as described above for the mycobiont loci. The two alignments were concatenated after inspecting single-locus trees for well-supported conflicts. We used the concatenated dataset as input for a maximum likelihood phylogenetic analysis in IQ-TREE. Model selection, tree inference, and bootstrapping parameters were the same as described above for the concatenated mycobiont dataset, with the initial partitioning scheme dividing the alignment by locus and further splitting rbcL by codon position.
For the second set of analyses, we performed a separate phylogenetic analysis for each major clade of Trebouxia represented in our sampling. The genus-wide alignment allowed unknown sequences to be assigned to one of the major clades, but because variable regions of ITS1 and ITS2 had to be excluded, relationships within these clades were often poorly resolved. For each major clade, reference taxa and sample sequences were extracted from the global ITS and rbcL alignments. The ITS alignments were corrected by eye in Mesquite and ambiguous regions were re-delimited. One rbcL sequence from each of the other major clades of Trebouxia was retained for the outgroup. After checking for well-supported conflicts between the two loci as described above, we concatenated the ITS and rbcL alignments and performed a maximum-likelihood analysis in IQ-TREE. Model selection, tree inference, and bootstrapping parameters were the same as described above for the concatenated mycobiont dataset. The initial partitioning scheme divided the alignment by locus and further split the ITS by region (ITS1, 5.8S, and ITS2) and rbcL by codon position. The final partitioning schemes and substitution models used for these analyses are provided in Table 3.
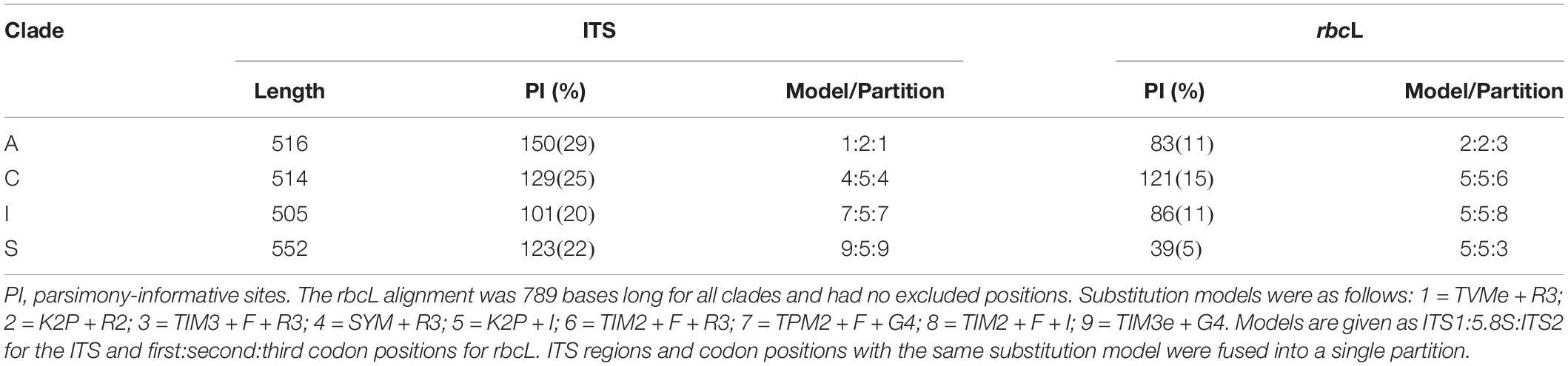
Table 3. Statistics on the alignments for Trebouxia (photobiont) after the removal of introns and ambiguously aligned regions.
To explore intraspecific diversity in a subset of putative Trebouxia species, we inferred haplotype networks in R version 3.6.1 (R Core Team, 2019) using the packages ape (Paradis and Schliep, 2019) and pegas (Paradis, 2010). Sites with ambiguous bases were excluded from the haplotype analysis.
Classification of Mycobionts and Photobionts
Our preliminary delimitation of mycobiont species was based on morphology, chemistry, and the inferred phylogeny. We used the program bPTP (Zhang et al., 2013) to inform our species delimitation, using the concatenated maximum likelihood phylogeny as input and running the Markov chain Monte Carlo (MCMC) analysis for 500,000 generations, sampling every 500 generations5. The first 25% of samples were discarded as burn-in.
Based on the ITS-rbcL phylogeny, photobiont sequences were assigned to a clade and putative species based on the framework from Muggia et al. (2020). Specimens that were phylogenetically distinct from any previously delimited species were considered to represent novel putative species.
Ecological Analyses
To better understand the ecological diversity across the elevation gradient, we extracted 19 bioclimatic variables from the WorldClim database (Fick and Hijmans, 2017) for each sampling locality. Bioclimatic variables were sampled at a resolution of 10 arc-minutes using the R packages sp (Pebesma and Bivand, 2005) and raster (Hijmans, 2021). We used the hclust function to perform a hierarchical clustering of the 19 bioclimatic variables, which was visualized as a dendrogram. Vegetation types for each site were classified with field locality descriptions and vegetation maps from Navarro and Ferreira (2007).
We analyzed patterns of species richness (α diversity) and turnover (β diversity) along the elevation gradient. From the original data for each lichen specimen (Supplementary Data Sheet 1), we prepared inferred presence/absence matrices for the mycobiont and photobiont species. The elevation gradient was split into 500 m bins starting at sea level and extending to 4,999 m. For each Lecanoraceae or Trebouxia species, we noted the lowest and highest elevations at which the species was found, and coded the species as “present” for all bins included in that range. For example, a Trebouxia species found at 2,400, 3,050, and 3,600 m would be coded as present from 2,000 to 3,999 m even though it was absent from the 2,500 to 2,999 m bin. The Sørensen index of dissimilarity was calculated for all pairwise comparisons between elevation bins using the vegdist function in the R package vegan (Oksanen et al., 2020).
Results
Sequenced Specimens
We obtained DNA sequence data from approximately half of the 235 specimens for which extractions were performed. Most of the specimens that failed to yield any sequence data were those collected before 2015 and stored under standard herbarium conditions. Of the specimens from which we obtained sequence data, approximately ten were not Lecanoraceae and were excluded from further analysis. A further ca. 20 specimens yielded only mycobiont or only photobiont sequences; the sequences from specimens without paired mycobiont–photobiont data were not used for the present paper. Finally, five specimens were excluded from the analysis because of well-supported conflicts between loci or discordance between molecular and morphological data. A total of 89 specimens with both mycobiont and photobiont data were used for the remaining analyses. We generated over 500 new DNA sequences from this group of specimens: 83 mycobiont ITS, 68 nrLSU, 79 mtSSU, 70 RPB1, 64 RPB2, 22 MCM7, 89 photobiont ITS, and 27 rbcL.
Alignments
Summary statistics for the Lecanoraceae and Trebouxia alignments are presented in Tables 2, 3, respectively. For the mycobiont, almost all of ITS1 and a large fraction of ITS2 could not be aligned across Lecanoraceae and were excluded from the phylogenetic analysis. The three protein-coding loci contained the greatest number of parsimony-informative sites in absolute terms and relative to their length (Table 2). For the Trebouxia sequences, most of ITS1 and ITS2 could be aligned within each major clade. ITS provided most of the parsimony-informative sites for all four Trebouxia single-clade alignments, while rbcL varied from being nearly as informative as ITS in Trebouxia clade C to being minimally informative in Trebouxia clade S (Table 3). Complete alignments with excluded regions delimited are available in the supplementary materials (Supplementary Data Sheets 3–8).
Lecanoraceae and Trebouxia Phylogeny and Biodiversity
Photobionts from members of the Lecanoraceae collected in Bolivia represented approximately 21 putative species in four of the five major clades within Trebouxia (Figure 2 and Supplementary Figure 1). The majority of photobionts were phylogenetically nested within previously delimited species, but six lineages appear to represent new species-level taxa in Trebouxia clades A, C, and I (Supplementary Figures 2–4). All samples from Trebouxia clade S corresponded to previously delimited species (Supplementary Figure 5). No samples were from Trebouxia group D (Supplementary Figure 1).
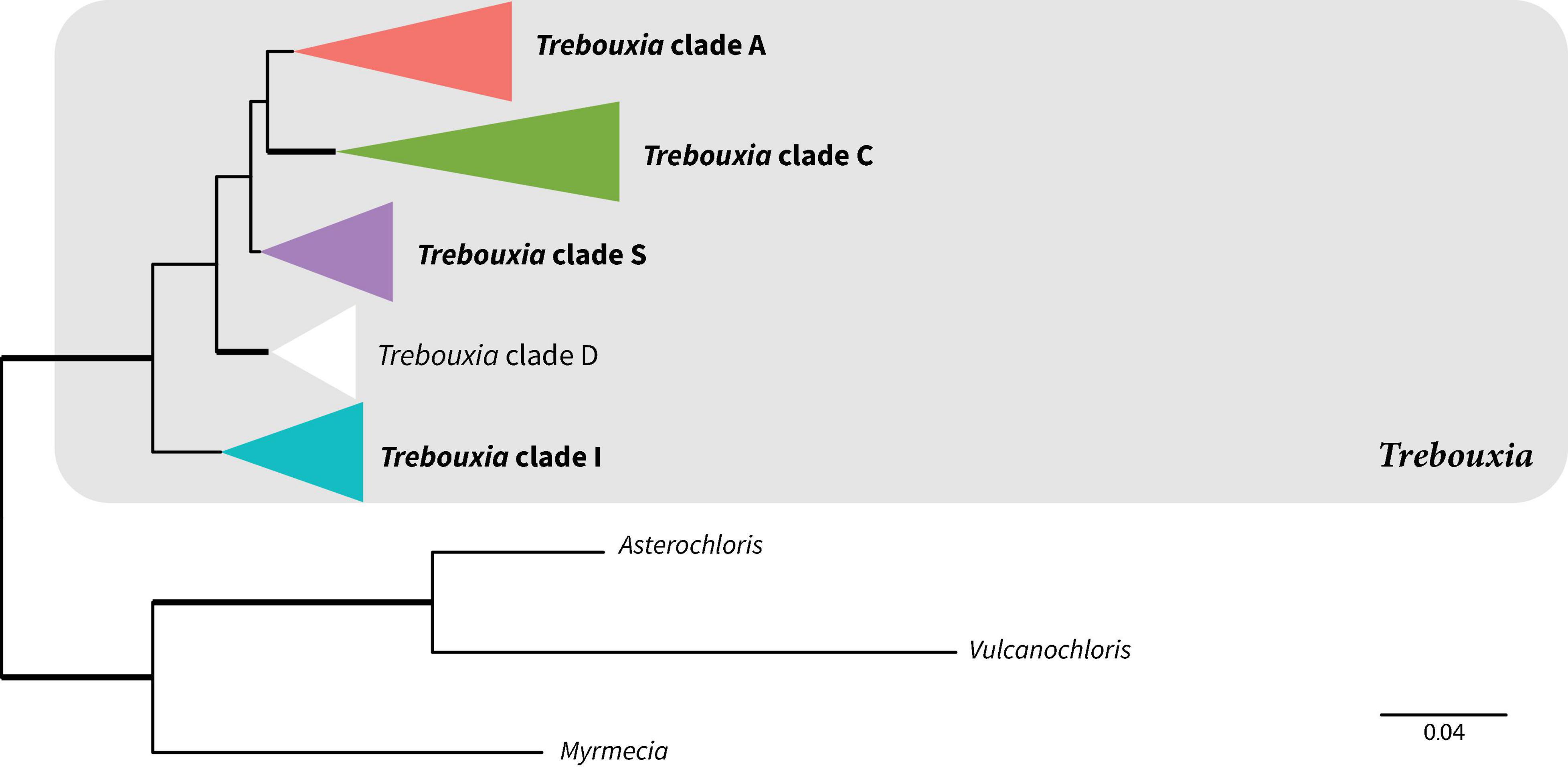
Figure 2. Summary phylogeny showing relationships among major clades of Trebouxia based on maximum likelihood analysis of the ITS-rbcL dataset. Bold clade names indicate clades represented in our Bolivian sampling. Bold branches indicate UFboot2 support ≥ 95. Scale represents substitutions per site. The color scheme introduced here will be used for these Trebouxia clades throughout the rest of the paper. For the full tree with all tip names and bootstrap values, see Supplementary Figure 1.
The single-locus trees for the mycobiont ITS, nrLSU, and mtSSU had some well-supported nodes near the tips, but deeper relationships were for the most part poorly supported (Supplementary Figures 6–8). RPB1, RPB2, and MCM7 were better able to resolve deeper relationships in Lecanoraceae, with RPB1 yielding the best-supported tree of any single locus (Supplementary Figures 9–11). The maximum likelihood tree from the concatenated six-locus dataset (Figure 3 and Supplementary Figure 12) included strong support for many putative genus- and subgenus-level clades and some relationships among those clades, but the backbone was poorly supported in several regions of the tree. For example, the relationships among Haematomma, Miriquidica, Ramboldia, and the rest of the family were not well supported, nor was the position of Lecidella. However, none of our mycobiont specimens belonged to these genera.
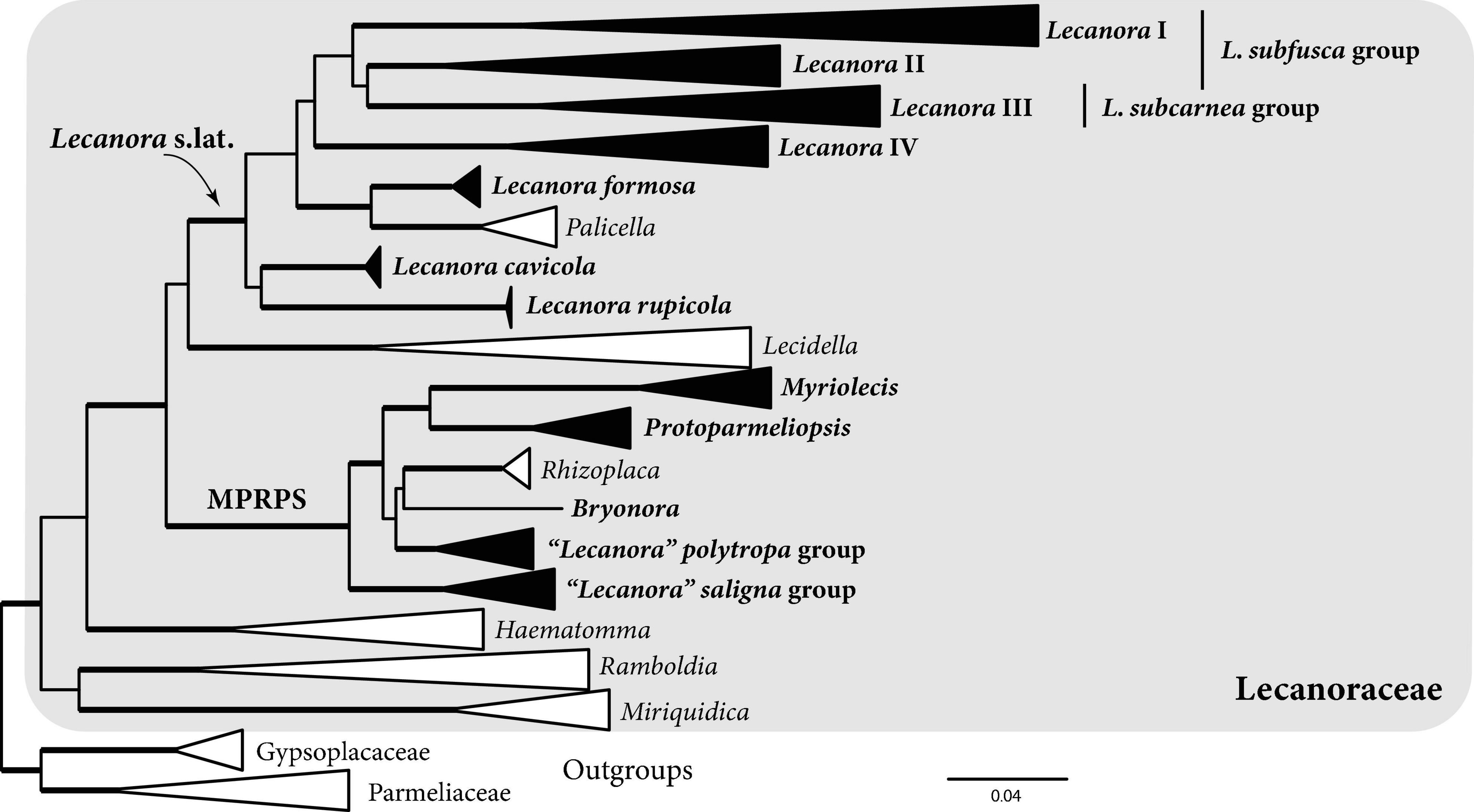
Figure 3. Summary phylogeny showing relationships within Lecanoraceae based on maximum likelihood analysis of the six-locus dataset. The clade names Lecanora I–IV, Lecanora s.lat., and MPRPS (Myriolecis, Protoparmeliopsis, Rhizoplaca, “L.” polytropa, “L.” saligna) will be used throughout the paper in the sense shown here. Bold clade names and filled triangles indicate clades represented in our Bolivian sampling. Bold branches indicate UFboot2 support ≥ 95. Scale represents substitutions per site. For the full tree with all tip names and bootstrap values, see Supplementary Figure 12.
Sampled mycobionts belong to two well-supported clades within Lecanoraceae (Figure 3). The first clade, which we call Lecanora s.lat., includes the Lecanora subfusca group (Lecanora clades I and II), L. subcarnea group (Lecanora clade III), a group we call Lecanora clade IV, L. formosa and Palicella, L. cavicola, and L. rupicola. None of the relationships among these clades were well supported. Species of Lecanora s.lat. were found predominantly on bark at low- to mid-elevation sites, but some collections were from rock at high elevation (Figure 4). The high-elevation, saxicolous specimens mostly belonged to L. rupicola, L. cavicola, and L. formosa, but also included taxa from Lecanora clades II and III (Figures 4, 5A).
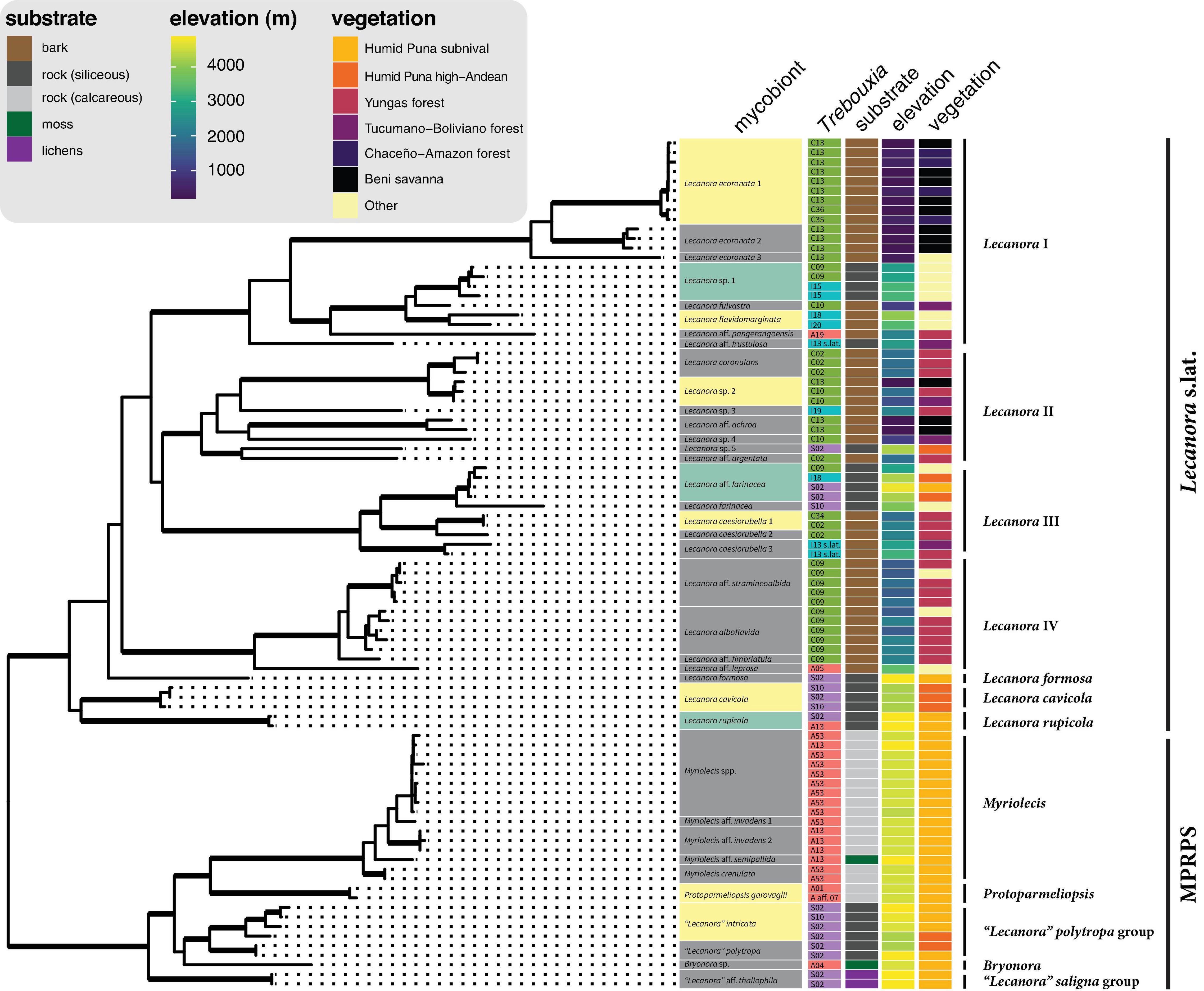
Figure 4. Photobiont association, substrate, elevation, and vegetation type in the context of the mycobiont phylogeny. The tree is from the same six-locus analysis as Figure 3 and Supplementary Figure 12; reference taxa were removed in R with the drop.tip function in ape and data were added to the tree with the package ggtree (Yu et al., 2017). Bold branches indicate clades with UFboot2 support ≥ 95 in Supplementary Figure 12. Lecanoraceae species highlighted in green were found with photobionts from more than one major clade of Trebouxia. Lecanoraceae species highlighted in yellow were found with multiple Trebouxia species from the same clade. Colors used for Trebouxia clades are the same as in Figure 2. Species-level relationships within Myriolecis are not well-resolved in this analysis.
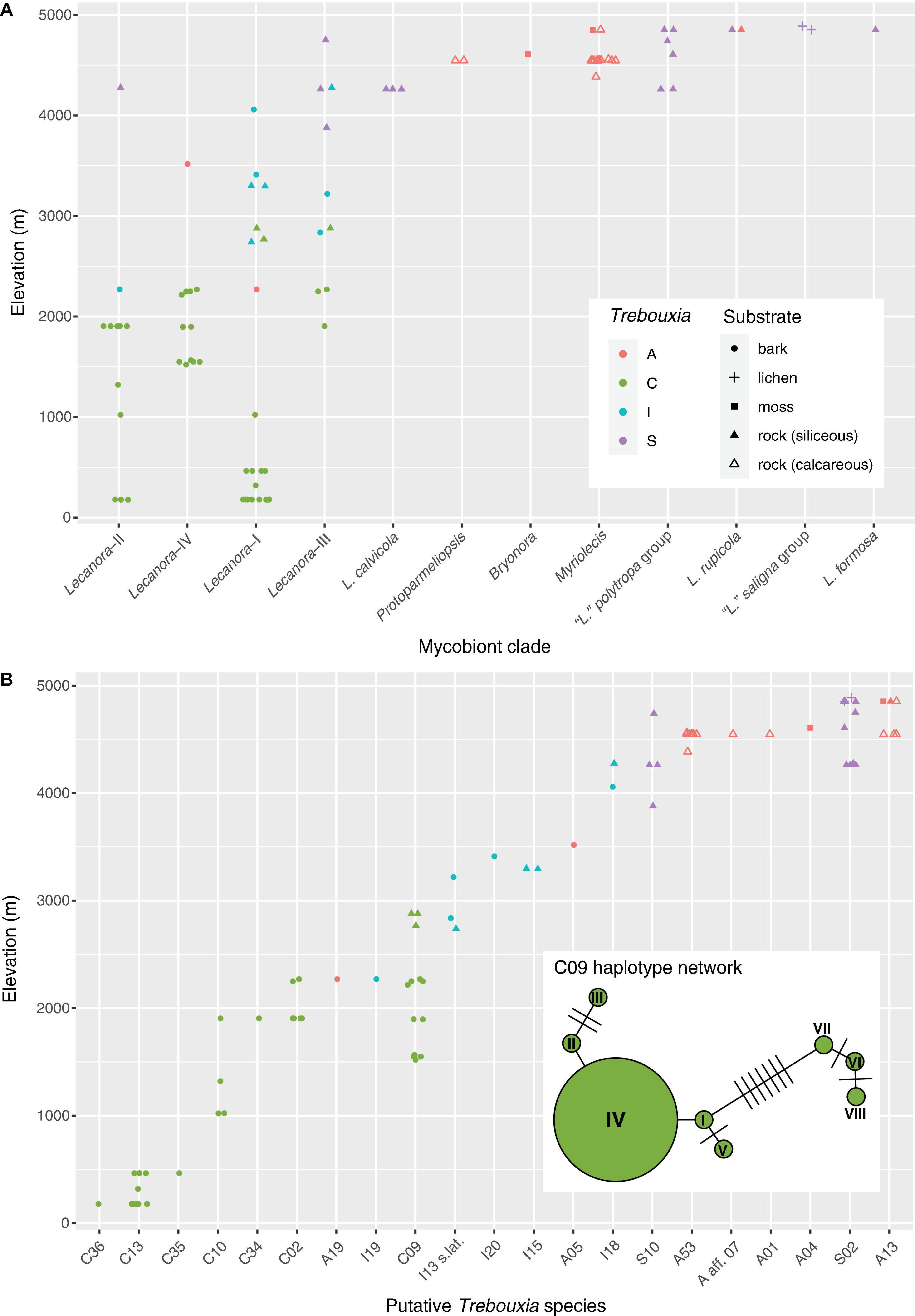
Figure 5. Elevation distribution of Lecanoraceae mycobionts and their Trebouxia photobionts. (A) Elevation distribution of the major mycobiont clades, showing Trebouxia clades and substrates. Note that photobiont turnover across the elevation gradient is apparent for Lecanoraceae as a whole and within individual mycobiont clades. There are relatively few samples from the 3,000 to 4,000 m range because specimens for molecular study were originally selected for a taxonomic study of Lecanoraceae. Although we have many collections from this altitudinal range, they were not particularly diverse morphologically and therefore were not sampled heavily in our sequencing. (B) Elevation distribution of putative Trebouxia species. A single photobiont species typically occupies 500–1,000 m of the elevation gradient. Inset shows haplotype network for Trebouxia C09, which occupies 1,500 m of the gradient. The haplotypes clustered on the left occur across the elevation range of this species, while those clustered on the right only occur at the bottom of the range.
The second major clade, which we call the MPRPS clade for lack of a formal name, included high-elevation collections from Myriolecis, Protoparmeliopsis, the “Lecanora” polytropa group, Bryonora, and the “Lecanora” saligna group. MPRPS also includes Rhizoplaca, although none of our collections belong to that genus (Figure 3). Myriolecis and Protoparmeliopsis form a well-supported clade, and the “L.” saligna group is a well-supported sister to the rest of the MPRPS clade, but the phylogenetic placements of the “L.” polytropa group, Rhizoplaca, and Bryonora are uncertain. Except for the single specimen of Bryonora and one Myriolecis specimen that were found on moss and two specimens of the “L.” saligna group that were lichenicolous lichens, species in the MPRPS clade occurred on rock (Figure 4).
Based on morphology, chemistry, and the molecular phylogeny, our sampling included approximately 27 species in Lecanora s.lat. and approximately 10 species in the MPRPS clade (Figure 4). The tree topology and species delimitation analysis suggest that several of the morphospecies contain multiple cryptic species. For example, there are three well-supported lineages on long branches in the morphospecies Lecanora ecoronata (Lecanora clade I), and the specimens identified as L. caesiorubella were distributed across three lineages in Lecanora clade III that did not form a monophyletic group (Figure 4).
Photobiont Ecology and Mycobiont Associations
The distribution of Trebouxia species was structured by elevation, substrate, and mycobiont identity (Figures 4, 5). Species of the MPRPS clade were always found with photobionts from Trebouxia clades A and S. Myriolecis, Protoparmeliopsis, and the single specimen of Bryonora were exclusively associated with Trebouxia clade A, while the “Lecanora” polytropa and “L.” saligna groups occurred only with Trebouxia clade S. All of these mycobiont genera were restricted to high elevations (Figure 5A). This pattern is also related to substrate chemistry: Saxicolous species of Myriolecis and Protoparmeliopsis grew on calcareous rock, while species of the “L.” polytropa group and the lichen hosts of members of the “L.” saligna group occurred on siliceous rock (Figure 4). All of these mycobiont genera were restricted to high-elevation sites (Figure 5A).
Species of the Lecanora s.lat. clade were found in association with Trebouxia clades A, C, I, and S. There was a pronounced turnover in photobiont clade along the elevation gradient, with Trebouxia clade C at low to mid-elevations (up to ca. 3,000 m), clade I mostly at upper mid elevations (ca. 2,000–3,500 m), and clade S at high elevations (above 3,500 m) (Figure 5A). Trebouxia clade A was only rarely associated with Lecanora s.lat., always above 2,000 m (Figure 5A).
Clades within Lecanora s.lat. occupied varying portions of the total elevation gradient (Figure 5A). Lecanora clade I was only absent from the highest elevations, while Lecanora clade III was missing at low elevations; Lecanora clade IV occupied intermediate elevations. Lecanora clade II was primarily at low elevations, except for a single high elevation species. Lecanora clades I–IV were each associated with photobionts from at least two, and often three, Trebouxia clades. The same elevational turnover of photobiont clades that is apparent for Lecanoraceae as a whole also occurs within these smaller clades; see, for example, Lecanora clades I and III (Figure 5A).
At the level of putative Trebouxia species, there is considerable variation in the level of specificity exhibited toward habitat and mycobiont (Figures 4, 5B). Most Trebouxia species occupied a 500–1,000 m subset of the elevation gradient (Figure 5B). Some taxa occurred only with a single mycobiont clade in that narrow elevation range. For example, Trebouxia C13 was only found below 500 m with species of Lecanora clade I, while Trebouxia A53 only occurred with species of Myriolecis at about 4,500 m (Figure 4). Other photobionts were generalists with respect to mycobiont but were only found in a specific habitat. Trebouxia A13, S02, and S10 occurred with mycobiont species from both the Lecanora s.lat. and MPRPS clades, but nearly always above 4,000 m (Figure 4). On the other hand, some photobionts exhibited substantial niche breadth for both mycobiont host and elevation. Trebouxia C09, the most broadly distributed photobiont species in our sampling, was associated with species from Lecanora clades I–IV and occurred on both bark and rock from 1,500 to 3,000 m (Figures 4, 5B).
Numbers of mycobiont and photobiont species were tightly linked across the elevation gradient, with the number of mycobiont species nearly always greater; both Lecanoraceae and Trebouxia had the greatest number of species at high elevation (Figure 6A). Species turnover was consistently high across the elevation gradient for both partners (Figure 6B). Each partner had a mid- to mid-high-elevation threshold across which no species were shared, but this threshold differed between Lecanoraceae (2,500 m) and Trebouxia (3,500 m) (Figure 6B).
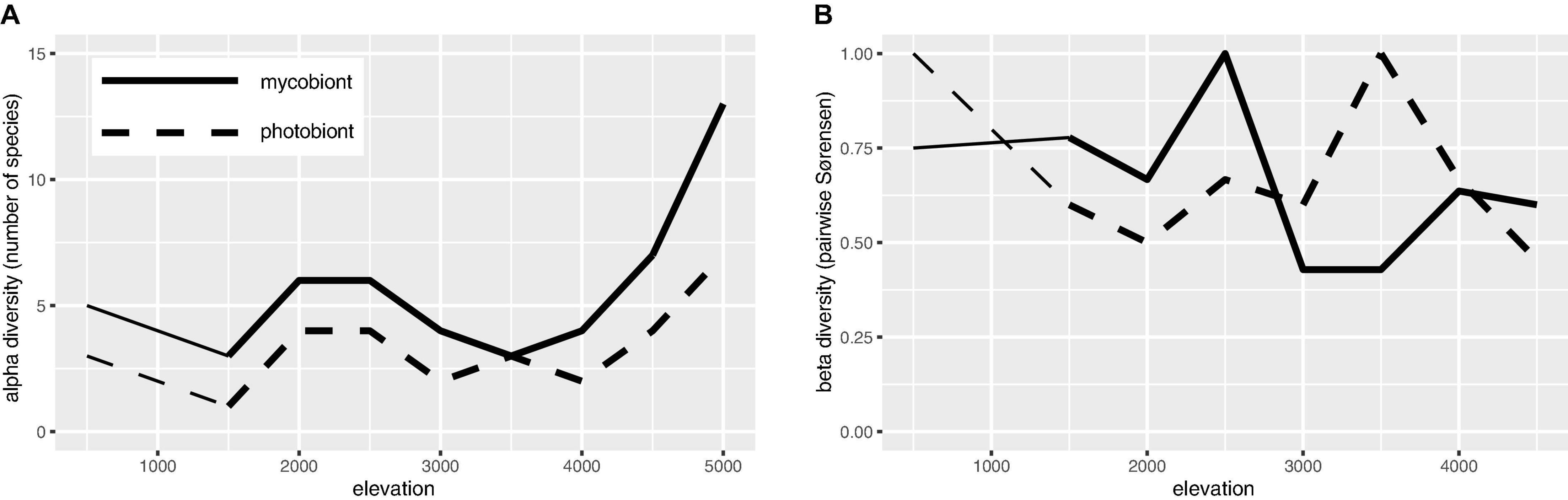
Figure 6. Species richness and β-diversity of Lecanoraceae and Trebouxia along the elevation gradient. (A) Number of Lecanoraceae (solid line) and Trebouxia (dashed line) species observed and inferred for 500 m elevation bins. (B) Pairwise comparisons of 500 m elevation bins by the Sørensen index of dissimilarity, where 0 means all species are shared and 1 means no species are shared. Note that each partner has a mid- to mid-high-elevation threshold not spanned by any species, but this threshold is not the same for mycobiont and photobiont. Comparisons below 1,500 m (reduced line weight) may be unreliable because of the absence of samples from the 500–999 m elevation band.
Photobiont Switching
Three mycobiont species were associated with photobionts from two or more Trebouxia clades (Figure 4). Lecanora sp. 1 (n = 4) occurred on sandstone in open areas between 2,700 and 3,300 m. Below 3,000 m, this species was found with Trebouxia C09, while above 3,000 m it was found with Trebouxia I15. Lecanora rupicola (n = 2) was found in association with Trebouxia A13 and S02 at a single high-elevation site, where it grew on siliceous rocks. Lecanora aff. farinacea (n = 4) occurred with Trebouxia C09 at ca. 2,900 m and with Trebouxia I18 and S02 at ca. 4,250 m. All four collections were from siliceous rocks, either sandstone at the lower site or schist at the upper sites.
A larger number of mycobiont species associated with multiple photobionts within one of the major Trebouxia clades (Figure 4). “Lecanora” intricata (n = 4) and L. cavicola (n = 3) each associated with Trebouxia S02 and S10. At one site at ca. 4,260 m, L. cavicola was found with both photobionts. All specimens of these two species were collected from siliceous rocks in open vegetation above 4,000 m. Protoparmeliopsis garovaglii (n = 2), which was only collected at a single site at ca. 4,550 m, on limestone, occurred with Trebouxia A01 and A aff. 07. Lecanora caesiorubella 1 (n = 2) occurred with Trebouxia C34 at ca. 1,900 m and Trebouxia C02 at 2,250 m; both sites were in Yungas forest. Lecanora ecoronata 1 (n = 9) was predominantly associated with Trebouxia C13, but at the same savanna sites also occurred with Trebouxia C35 and C36. Lecanora sp. 2 (n = 3) occurred with Trebouxia C13 at the bottom of its range and C10 at the top of its range. Lecanora flavidomarginata (n = 2) occurred with Trebouxia I18 and I20, at ca. 4,000 m and ca. 3,400 m, respectively.
Haplotypes of Trebouxia C09 fell into two clusters (Figure 5B). One cluster spanned the entire elevation range of this putative species, while the other occurred only in the lower portion of its range. Lecanora aff. stramineoalbida was associated with both haplotype clusters.
Discussion
Mycobiont Phylogeny
Phylogenetic studies of Lecanoraceae have long been bedeviled by a poorly supported backbone (Grube et al., 2004; Pérez-Ortega et al., 2010; Zhao et al., 2016; Yakovchenko et al., 2019; Davydov et al., 2021). Most of these studies, including recent publications, have used ITS and mtSSU to infer the phylogeny of Lecanoraceae, but those loci are most useful for resolving relationships within genera or species groups and cannot resolve deeper relationships across the entire family. Zhao et al. (2016) obtained better support with a six-locus dataset, but their analysis excluded many morphologically distinct species groups for which the protein-coding loci were lacking. In the present study, we used the same six loci as Zhao et al. (2016): ITS, nrLSU, mtSSU, RPB1, RPB2, and MCM7. We expanded their taxon sampling, bringing in additional clades that were missing from their six-locus analysis: Bryonora, Lecanora IV, Lecanora cavicola, Lecanora rupicola, and the “Lecanora” saligna group. Despite this expanded sampling, we found that several regions of the phylogeny remain poorly supported.
One particularly difficult problem in Lecanoraceae systematics is the delimitation of Lecanora s.str. In the present paper, we have sidestepped this issue by referring to a well-supported Lecanora s.lat. and well-supported clades nested within it, without making a taxonomic judgment about what should be included in Lecanora s.str. If morphologically distinctive groups such as Palicella, Pulvinora, and the Lecanora rupicola group are to be excluded from Lecanora s.str., as various authors have proposed (Grube et al., 2004; Rodriguez Flakus and Printzen, 2014; Davydov et al., 2021), Lecanora s.str. should ideally be tied to a well-supported node within our Lecanora s.lat. Our analysis recovered no such node deeper than the species groups delimited in Figure 3. For example, the Lecanora subfusca group as traditionally circumscribed (Lecanora clades I and II; Zhao et al., 2016) was not well supported in our analysis (Figure 3 and Supplementary Figure 12). Further research, potentially with genomic data, will be required to stabilize the genus-level nomenclature of Lecanoraceae.
Based on our results and the existing literature, we recommend sequencing at a minimum ITS, mtSSU, and RPB1 in order to place a specimen within the phylogeny of Lecanoraceae. The new primers and nested PCR protocols we describe in this paper can be used to sequence RPB1 and RPB2 even from extractions with a very small quantity of DNA. In this study, we have substantially increased the number of RPB1, RPB2, and MCM7 sequences available for Lecanoraceae, which should facilitate the use of these loci in future phylogenetic studies of this family. The inclusion of Bryonora in our multilocus dataset illustrates how the protein-coding loci can clarify relationships in Lecanoraceae. Bryonora was previously represented on GenBank by a single ITS sequence from Grube et al. (2004), and its phylogenetic position was unknown. The phylogenetic analysis of Grube et al. (2004) placed it outside Lecanoraceae, albeit without support. Our results, which included ITS, nrLSU, mtSSU, RPB2, and MCM7 sequences for a specimen of Bryonora, strongly support Bryonora as a distinct genus belonging to the MPRPS clade.
Although there have been morphological studies on Lecanoraceae in Bolivia (Śliwa et al., 2013, 2014; to a minor extent, Guderley, 1999), ours is the first study to generate molecular data for Bolivian Lecanoraceae and one of the only molecular phylogenetic studies of tropical Lecanoraceae (Kirika et al., 2012; Papong et al., 2013). These data will be used in forthcoming publications on the systematics of Lecanoraceae. As a resource for the community, we have made our Lecanoraceae and Trebouxia trees and alignments available on the T-BAS online portal6 to facilitate the phylogenetic placement of specimens from these clades (Carbone et al., 2017, 2019).
Trebouxia Diversity and Ecology
We found Lecanoraceae mycobionts from Bolivia in association with four of the five major clades of Trebouxia, with only Trebouxia clade D absent from our sampling. Clades A, I, and S have been recorded with Lecanoraceae in numerous previous studies (Beck, 1999; Blaha et al., 2006; Guzow-Krzemińska, 2006; Hauck et al., 2007; Pérez-Ortega et al., 2012; Ruprecht et al., 2012, 2020; Leavitt et al., 2015, 2016; Voytsekhovich and Beck, 2016; Singh et al., 2019; Muggia et al., 2020; Wagner et al., 2020). Lecanoraceae species have not previously been reported to associate with Trebouxia clade C. Our results show that species of Lecanora s.lat.—specifically Lecanora clades I–IV—frequently associate with Trebouxia clade C photobionts in low to mid-elevation habitats (Figure 5A). That this association has not been seen before is a result of the dearth of molecular phylogenetic studies on tropical Lecanora. Our results reinforce the importance of Trebouxia clade C photobionts in tropical lichens. The prevalence of Trebouxia clade C photobionts in the tropics has been recognized for nearly two decades (Helms, 2003; Cordeiro et al., 2005), but it was not until recently that the species richness of this clade was well understood (Muggia et al., 2020).
Several Trebouxia ecological patterns seen in other regions and with other mycobiont taxa are evident in our results. These include the association of Trebouxia clade A with calcareous rock (Helms, 2003) and open, intermittently humid Andean vegetation (Vargas Castillo and Beck, 2012), as well as the association of Trebouxia clade S with high elevation (Blaha et al., 2006; Muggia et al., 2008). In an analysis of over 6,000 publicly available sequences of lichenized Trebouxia, originally sampled from around the world and associated with diverse mycobiont hosts, Nelsen et al. (2021) found that Trebouxia clade I photobionts occupy a bioclimatic space intermediate between clade C and clade S, while clade A—the most speciose lineage in Trebouxia—overlaps with all three. Our results confirm this pattern (Figures 5A,B) and show that it can be observed on a regional scale within the symbionts of a single mycobiont family.
Along an elevation gradient from ca. 700–2,100 m in Europe, Dal Grande et al. (2018) found that Trebouxia photobionts of the mycobiont genus Lasallia had larger altitudinal ranges at higher elevations. We saw no evidence for this pattern across the entire elevation range represented in our sampling (Figure 5B). It is possible that greater inter-biome variation in climate and vegetation along our nearly 5,000 m elevation gradient leads to species turnover that overrides any such pattern.
Our photobiont data included putative new species of Trebouxia in clades A, C, and I (Supplementary Figures 2–4). In clade S, which is globally less diverse than the other three clades (Muggia et al., 2020), all of our specimens belonged to previously delimited species (Supplementary Figure 5). Most of the putative novel species were recovered from a single specimen (C34, C35, C36, I19, and I20) (Figure 5B). The exception is A53, which we found in 11 specimens of Myriolecis across four localities in the humid puna subnival vegetation (Figures 4, 5). A53 was always found in lichen thalli growing on exposed, calcareous rock (Figure 4).
Several Trebouxia species we found in our sampling were previously known primarily, or exclusively, from east Africa (see below). Shared Trebouxia genotypes between Bolivia and Kenya have been previously reported (Lutsak et al., 2016), highlighting that long-distance dispersal probably plays a major role in the distribution of lichenized algae (Rolshausen et al., 2020).
Notes on Selected Trebouxia Species
A01 — We found this species associated with a single thallus of Protoparmeliopsis garovaglli. Guzow-Krzemińska (2006) recorded this photobiont in association with P. muralis in Europe, and Leavitt et al. (2015) found it associated with species of Protoparmeliopsis, Lecanora, Rhizoplaca, and Xanthoparmelia.
A04 — We recovered Trebouxia A04 from a single specimen of Bryonora. This is the first photobiont DNA sequence obtained from a lichen thallus of this mycobiont genus.
A05 — This species was found in association with a species of Lecanora clade IV from ca. 3,500 m. It has otherwise been reported from various species of Parmeliaceae: Hypotrachyna and Punctelia in Kenya (Muggia et al., 2020) and Oropogon in Central and South America (Leavitt et al., 2015).
A13 — This species is globally distributed (De los Ríos et al., 2002; Blaha et al., 2006; Guzow-Krzemińska, 2006; Muggia et al., 2008, 2014; Nyati et al., 2014; Leavitt et al., 2015; Voytsekhovich and Beck, 2016).
A19 — We provide the first rbcL sequence from this previously delimited species (Muggia et al., 2020).
A53 — This is the most common novel putative species we recovered in our sampling (Figure 4). It was always found in association with species of Myriolecis growing on calcareous rock.
C02 — We provide the first rbcL sequence from this previously delimited species (Muggia et al., 2020).
C09 — This species was previously reported from multiple genera of Parmeliaceae in Kenya and from Tephromela in Kenya, Russia, and Peru (Muggia et al., 2014, 2020). In our sampling, Trebouxia C09 was found in association with species of Lecanora s.lat. It is clearly a generalist with respect to mycobiont host and substrate, occurring with species of Lecanora clade IV on bark at the lower end of its altitudinal range, then with species from clades I, II, and III, on bark and on rock, at the upper end of its range (Figures 4, 5B).
C10 — This species was previously reported from multiple genera of Parmeliaceae in Kenya and Parmotrema tinctorum in Japan (Ohmura et al., 2006; Muggia et al., 2020). In our sampling, Trebouxia C10 was only found in association with species of Lecanora clades I and II (i.e., the Lecanora subfusca group) (Figure 4).
C13 — This species is known from three specimens of Parmotrema and Canoparmelia from Kenya (Muggia et al., 2020). In our sampling, Trebouxia C13 was only found in association with species of Lecanora clades I and II (i.e., the Lecanora subfusca group) (Figure 4).
C34 — This novel putative species was also found by Kosecka et al. (2021b). See discussion below.
C35 and C36 — These OTUs form a novel lineage sister to the rest of Trebouxia clade C (Supplementary Figure 3). They were both found in association with Lecanora ecoronata 1 in the low-elevation savanna. The phylogenetic position of these species is supported by both ITS and rbcL, but additional investigations of the ultrastructure of these species should be conducted to evaluate whether they would be better treated as a new major clade (i.e., if they do not have a corticola-type pyrenoid).
I13 s.lat. — We found several specimens associated with Trebouxia I13 but, despite having both ITS and rbcL data, the clade is not well supported (Supplementary Figure 4). This region of the Trebouxia clade I tree, which also includes I17, I18, I19, and I20, appears to be primarily tropical (Muggia et al., 2020) and requires further phylogenetic study.
I15 — We provide the first rbcL sequence from this previously delimited species. Trebouxia I15 was reported from Punctelia and Hypotrachyna in Kenya (Leavitt et al., 2015; Muggia et al., 2020).
I18 — This species was reported from Hypotrachyna in Peru and Kenya and Tephromela in Peru (Muggia et al., 2014, 2020).
I19 and I20 — These novel putative species were also found by Kosecka et al. (2021b). See discussion below.
S02 — This species is a generalist with respect to mycobiont host, associating with species of multiple genera within both the Lecanora s.lat. and MPRPS clades.
S10 — This OTU corresponds to the formally described species Trebouxia simplex (Tschermak-Woess, 1978), the type of which was originally isolated from Chaenotheca in Europe, and has also been reported from Bryoria in North America and Europe (Lindgren et al., 2014) and Lasallia in Europe (Sadowska-Deś et al., 2014). Our results confirm that S10/T. simplex is a generalist with respect to mycobiont host, associating with species from both the Lecanora s.lat and MPRPS clades.
Multiple Photobionts in a Single Thallus
Although intra-thalline genetic diversity has been documented in lichenized Trebouxia (e.g., Mansournia et al., 2012; Dal Grande et al., 2014), Sanger sequencing of PCR amplicons has been shown to consistently identify the most abundant photobiont genotype (Paul et al., 2018). Furthermore, when multiple photobiont genotypes are present, they are generally closely related, belonging to the same species (Mansournia et al., 2012; Dal Grande et al., 2014), sister taxa (Casano et al., 2011), or at least the same group within Trebouxia (Paul et al., 2018).
For one specimen (Flakus 29597), Sanger sequencing recovered two different ITS sequences with the forward and reverse primers, corresponding to the photobionts I18 and S02. Repeated PCR and sequencing of this specimen generated the same result: two clean chromatograms with different sequences. The rbcL sequence obtained from this specimen was consistent with I18, so we used that photobiont in the figures and analyses, although S02 is also consistent with the ecology of this specimen. A technique such as fluorescence in situ hybridization (FISH) would be required to conclusively demonstrate that both photobionts occur in the same lichen thallus.
Specimens for which the photobiont ITS PCR was successful (as determined by a single clear band on an agarose gel) but sequencing failed because the chromatograms were unusable due to double peaks might plausibly represent cases where multiple algal species were present in the thallus. Likewise, cases where isolated double peaks occurred in otherwise high-quality chromatograms may indicate the presence of multiple algal haplotypes in a single thallus. Further investigation with next-generation sequencing would be required to verify these conjectures.
Photobiont Switching Within a Mycobiont Species
Photobiont switching within a mycobiont species can occur at multiple phylogenetic levels: between photobiont genera (Ertz et al., 2018; Vančurová et al., 2018), between major clades within a photobiont genus (Blaha et al., 2006), between photobiont species within a subgeneric clade (Magain et al., 2017, 2018; Dal Grande et al., 2018), or between haplotypes within a photobiont species (Gasulla et al., 2020). We found no evidence of switching at the genus level, reaffirming that Lecanoraceae mycobionts are strict specialists on Trebouxia (Miadlikowska et al., 2006). Conversely, we found evidence for all three of the intrageneric types of switching, suggesting that the specificity of these mycobionts for particular algal species may be lower than commonly thought. It should be emphasized, however, that our sampling included few thalli from any one mycobiont species and is therefore not ideal for studying photobiont interactions within a mycobiont species. The conclusions drawn from the instances of switching we did observe should be considered preliminary and should be tested with a more focused sampling.
One of the mycobiont species we found with photobionts from two different Trebouxia clades was Lecanora rupicola (Figure 4). Blaha et al. (2006) sequenced the photobiont for specimens of L. rupicola from across Europe. They found that this species associated with diverse photobionts from three of the main clades of Trebouxia, including in some cases multiple photobiont species at a single site. The mycobiont was not sequenced in that study, so we do not know what role population structure or cryptic diversity may have played in the observed patterns, although the authors noted that there was no correlation between variation in secondary chemistry and photobiont identity.
All of the mycobiont species associated with photobionts from multiple Trebouxia clades occurred on siliceous rocks in open areas at mid-high to high elevation (Figure 4). The same was true for many of the mycobiont species that partnered with multiple photobionts from a single Trebouxia clade (Figure 4). This is consistent with previous work that has shown that mycobiont–photobiont associations in lichens are more specialized in warmer climates (Singh et al., 2017). One hypothesis to explain this pattern is that there are simply more photobiont species, and therefore greater opportunity for partner switching, at high elevation (Figure 6A). However, many of the instances of switching we observed were related to elevation change (Figure 5), lending support to the alternate hypothesis that mycobionts switch between locally-adapted photobionts along their range (Muggia et al., 2014; Dal Grande et al., 2018).
Turnover of Mycobionts and Photobiont Communities
Our results add to a growing body of literature showing that elevation gradients have a substantial effect on the composition of lichen photobiont communities (Dal Grande et al., 2018; Devkota et al., 2019; Gasulla et al., 2020; Rolshausen et al., 2020; Wagner et al., 2020). This literature is part of a broader realization that microbial diversity is subject to some of the same biogeographic influences as macroscopic organisms (e.g., Nottingham et al., 2018; but see Bryant et al., 2008). For example, various taxa of free-living cyanobacteria and green algae in the soil may vary in their abundance along elevation gradients (Řeháková et al., 2011; Stewart et al., 2021). The elevation difference between the lowest and highest sites in our dataset—nearly 5,000 m, greater than any other study of this type—presents a unique opportunity to study mycobiont and photobiont turnover accompanying the transition between geographically close but ecologically distant biomes (Figure 7). In contrast to previous studies where turnover of mycobiont and photobiont communities occurred at different spatial scales (Dal Grande et al., 2018; Lu et al., 2018; Gasulla et al., 2020; Rolshausen et al., 2020), we observed turnover in Lecanoraceae communities and their associated Trebouxia photobionts at roughly equivalent rates (Figures 5, 6). However, because there are no mycobiont species that occur along the entire length of the gradient, we cannot tease apart the impact of substrate, climate, and mycobiont host on photobiont distribution to the degree possible in other studies (e.g., Dal Grande et al., 2018). For example, species of Myriolecis are almost always found on calcareous rock at high elevation with Trebouxia clade A.
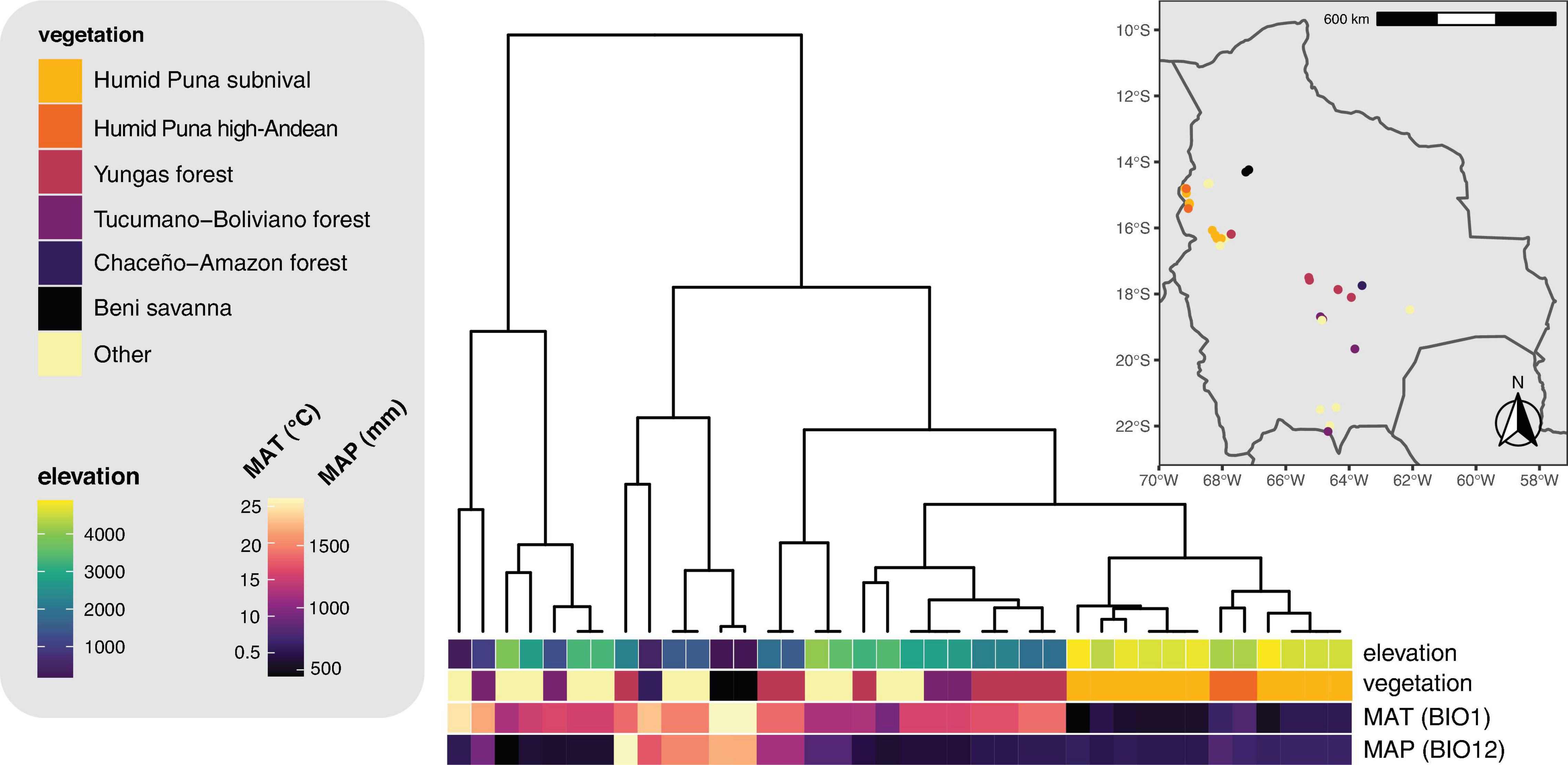
Figure 7. Hierarchical clustering dendrogram of 19 bioclimatic variables for the sampling localities. Sampling locations and vegetation types are displayed on the inset map. MAT, mean annual temperature (WorldClim variable BIO1). MAP, mean annual precipitation (WorldClim variable BIO12).
Our findings on species richness and β-diversity along the elevation gradient are consistent with previously observed patterns and also suggest phenomena which should be investigated in a larger sampling replicated across multiple transects. The finding that, within each elevation bin, Trebouxia species were less diverse than Lecanoraceae (Figure 6A) recalls the conventional wisdom that natural communities contain more mycobiont species than photobiont species (e.g., Singh et al., 2019; Wagner et al., 2020). The one exception at 3,500 m, where the number of mycobiont and photobiont species are equal, corresponds to a poorly-sampled elevation band and may be an artifact (Figure 5).
Our results for β-diversity suggest an interesting phenomenon: Along an elevation gradient, species turnover occurs for both partners in a mutualistic interaction, but the elevation thresholds at which community composition shifts most dramatically are different for the two partners (Figure 6B). This pattern has not been seen in similar studies of smaller elevation gradients and fewer mycobiont taxa (e.g., Dal Grande et al., 2018). These thresholds may be equivalent to the “symbiont turnover zones” described by Rolshausen et al. (2020), but while those authors found a turnover zone for one partner, we show turnover zones for both partners and, crucially, these are at widely spaced elevations. No Lecanoraceae species are found both above and below 2,500 m, while no Trebouxia species are found on both sides of 3,500 m. The former elevation is the threshold at which rock begins to appear as a substrate for Lecanoraceae, and is also the transition point between aseasonal Yungas forest and seasonal Tucumano-Boliviano forest (Figures 4, 7). The latter elevation corresponds to the transition from Trebouxia clades C and I to Trebouxia clades A and S (Figure 5B); this is also the threshold at which open, rocky habitats fully replace forest vegetation (Figure 7). Perhaps not coincidentally, 3,500 m is also very close to a similar threshold for woody plant ranges in the Bolivian Andes (Tello et al., 2015).
These findings suggest two alternative hypotheses. One possibility is that mycobiont and photobiont ranges are responding to different biotic or abiotic factors (e.g., substrate versus temperature; rainfall versus the presence of pathogens). Another possibility is that both thresholds are a response to the same factor, but the two partners are responding differently. Lu et al. (2018) found that ranges of Peltigera species and Nostoc phylogroups did not have the same response to climate shifts along a latitudinal gradient, but their study was at an intra-biome scale and lacked the sharp community discontinuities we see here. While our sampling is not adequate to distinguish between these hypotheses, it provides guidance as to an ideal region and study design that would have the power to resolve this question.
Phylogenetically Versus Ecologically Designed Sampling to Study Photobiont Communities
Kosecka et al. (2021b) examined the community of Trebouxia photobionts along a largely identical elevation gradient in Bolivia. That study included a four- to five-fold larger sample size from multiple families of Trebouxia-associated Lecanoromycetes, including some Lecanoraceae, but only dealt with the photobiont sequences from these specimens. The results of Kosecka et al. (2021b) in terms of Trebouxia diversity and distribution are largely consistent with our own findings, from the broad pattern of turnover among major clades of Trebouxia along the elevation gradient to many of the individual Trebouxia species found. Our study recovered several novel lineages not found by Kosecka et al. (2021b)—A53, C35, and C36; conversely, and commensurate with their larger sample size, Kosecka et al. (2021b) found several putative Trebouxia species which were missing from our sampling. Nevertheless, the close correspondence between the two studies suggests that dense sampling of a single mycobiont family may be sufficient to reveal regional patterns of biodiversity in lichenized Trebouxia. This stands in contrast to the situation with cyanolichens, where the Nostoc community will look very different if, for example, one samples from only Collemataceae, Peltigeraceae, or Lobariaceae in the same geographic area (Magain et al., 2018). Studies of Trebouxia photobionts from a single mycobiont clade are the norm in the literature, but it has not been clear whether their results can be generalized to represent the entire Trebouxia community of a region. Our results suggest that the answer may be yes, as long as the focal mycobiont clade occurs in a sufficiently diverse range of micro- and macrohabitats.
Data Availability Statement
The datasets presented in this study can be found in online repositories. New sequence data generated for this study are available on GenBank with accession numbers OK665489–OK665671, OL603980–OL604141, OL625025–OL625113, and OL663852–OL663919. Other data are included in the online Supplementary Material for this article.
Author Contributions
FL, JM, and LŚ contributed to the conception and design of the study and supervised Ph.D. students. LŚ and JM secured funding. AF and PR-F collected the specimens and ecological data in the field. EM and LŚ studied specimen morphology and delimited morphospecies. EM and EC prepared DNA extractions. IM designed primers for PCR and sequencing and generated the figures. EM, IM, and CP-DH collected the molecular data. IM and EM curated, validated, and analyzed the data and wrote the original draft of the manuscript. All authors participated in review and editing of the final manuscript and approved the submitted version.
Funding
Funding for this research was provided by the National Science Centre, Poland, under a grant to LŚ (project no. 2016/21/B/NZ8/02463). IM was supported by a US National Science Foundation Graduate Research Fellowship (DGE 1644868).
Conflict of Interest
The authors declare that the research was conducted in the absence of any commercial or financial relationships that could be construed as a potential conflict of interest.
Publisher’s Note
All claims expressed in this article are solely those of the authors and do not necessarily represent those of their affiliated organizations, or those of the publisher, the editors and the reviewers. Any product that may be evaluated in this article, or claim that may be made by its manufacturer, is not guaranteed or endorsed by the publisher.
Acknowledgments
AF and PR-F are greatly indebted to the staff of the Herbario Nacional de Bolivia, Instituto de Ecología, Universidad Mayor de San Andrés, La Paz, for their cooperation and to the Servicio Nacional de Áreas Protegidas (SERNAP) and all protected area staff for providing permits for scientific studies, as well as assistance and logistical support during field work. We thank the two reviewers for their helpful feedback on the manuscript.
Supplementary Material
The Supplementary Material for this article can be found online at: https://www.frontiersin.org/articles/10.3389/fmicb.2021.774839/full#supplementary-material
Footnotes
- ^ https://eurofinsgenomics.eu/en/ecom/tools/pcr-primer-design/ (accessed June 24, 2021).
- ^ https://www.thermofisher.com/us/en/home/brands/thermo-scientific/molecular-biology/molecular-biology-learning-center/molecular-biologyresource-library/thermo-scientific-web-tools/multiple-primer-analyzer.html (accessed June 24, 2021).
- ^ https://www.geneious.com
- ^ https://mafft.cbrc.jp/alignment/server/
- ^ https://species.h-its.org/
- ^ https://decifr.hpc.ncsu.edu/index.php
References
Beck, A. (1999). Photobiont inventory of a lichen community growing on heavy-metal-rich rock. Lichenologist 31, 501–510. doi: 10.1006/lich.1999.0232
Bengtsson-Palme, J., Ryberg, M., Hartmann, M., Branco, S., Wang, Z., Godhe, A., et al. (2013). Improved software detection and extraction of ITS1 and ITS2 from ribosomal ITS sequences of fungi and other eukaryotes for analysis of environmental sequencing data. Methods Ecol. Evol. 4, 914–919. doi: 10.1111/2041-210X.12073
Blaha, J., Baloch, E., and Grube, M. (2006). High photobiont diversity associated with the euryoecious lichen-forming ascomycete Lecanora rupicola (Lecanoraceae, Ascomycota). Biol. J. Linn. Soc. 88, 283–293. doi: 10.1111/j.1095-8312.2006.00640.x
Brodo, I. M., Sharnoff, S. D., and Sharnoff, S. (2001). Lichens of North America. New Haven, CT: Yale University Press.
Bryant, J. A., Lamanna, C., Morlon, H., Kerkhoff, A. J., Enquist, B. J., and Green, J. L. (2008). Microbes on mountainsides: contrasting elevational patterns of bacterial and plant diversity. Proc. Natl. Acad. Sci. U.S.A. 105(Suppl. 1) 11505–11511. doi: 10.1073/pnas.0801920105
Carbone, I., White, J. B., Miadlikowska, J., Arnold, A. E., Miller, M. A., Kauff, F., et al. (2017). T-BAS: Tree-Based Alignment Selector toolkit for phylogenetic-based placement, alignment downloads, and metadata visualization: an example with the Pezizomycotina tree of life. Bioinformatics 33, 1160–1168. doi: 10.1093/bioinformatics/btw808
Carbone, I., White, J. B., Miadlikowska, J., Arnold, A. E., Miller, M. A., Magain, N., et al. (2019). T-BAS version 2.1: Tree-Based Alignment Selector toolkit for evolutionary placement and viewing of alignments and metadata on curated and custom trees. Microbiol. Resour. Announce. 8:e00328-19. doi: 10.1128/MRA.00328-19
Casano, L. M., del Campo, E. M., García-Breijo, F. J., Reig-Armiñana, J., Gasulla, F., del Hoyo, A., et al. (2011). Two Trebouxia algae with different physiological performances are ever-present in lichen thalli of Ramalina farinacea. Coexistence versus competition? Environ. Microbiol. 13, 806–818. doi: 10.1111/j.1462-2920.2010.02386.x
Chagnon, P. L., Magain, N., Miadlikowska, J., and Lutzoni, F. (2019). Species diversification and phylogenetically constrained symbiont switching generated high modularity in the lichen genus Peltigera. J. Ecol. 107, 1645–1661. doi: 10.1111/1365-2745.13207
Chernomor, O., Von Haeseler, A., and Minh, B. Q. (2016). Terrace aware data structure for phylogenomic inference from supermatrices. Syst. Biol. 65, 997–1008. doi: 10.1093/sysbio/syw037
Cordeiro, L. M. C., Reis, R. A., Cruz, L. M., Stocker-Wörgötter, E., Grube, M., and Iacomini, M. (2005). Molecular studies of photobionts of selected lichens from the coastal vegetation of Brazil. FEMS Microbiol. Ecol. 54, 381–390. doi: 10.1016/j.femsec.2005.05.003
Culberson, C. F., and Kristinsson, H. (1970). A standardized method for the identification of lichen products. J. Chromatogr. 46, 85–93. doi: 10.1016/S0021-9673(00)83967-9
Dal Grande, F., Alors, D., Divakar, P. K., Bálint, M., Crespo, A., and Schmitt, I. (2014). Insights into intrathalline genetic diversity of the cosmopolitan lichen symbiotic green alga Trebouxia decolorans Ahmadjian using microsatellite markers. Mol. Phylogenet. Evol. 72, 54–60. doi: 10.1016/j.ympev.2013.12.010
Dal Grande, F., Rolshausen, G., Divakar, P. K., Crespo, A., Otte, J., Schleuning, M., et al. (2018). Environment and host identity structure communities of green algal symbionts in lichens. New Phytol. 217, 277–289. doi: 10.1111/nph.14770
Davydov, E. A., Yakovchenko, L. S., Hollinger, J., Bungartz, F., Parrinello, C., and Printzen, C. (2021). The new genus Pulvinora (Lecanoraceae) for species of the ‘Lecanora pringlei’ group, including the new species Pulvinora stereothallina. Bryologist 124, 242–256. doi: 10.1639/0007-2745-124.2.242
De los Ríos, A., Ascaso, C., and Grube, M. (2002). An ultrastructural, anatomical and molecular study of the lichenicolous lichen Rimularia insularis. Mycol. Res. 106, 946–953. doi: 10.1017/S0953756202006238
Devkota, S., Chaudhary, R. P., Werth, S., and Scheidegger, C. (2019). Genetic diversity and structure of the epiphytic foliose lichen Lobaria pindarensis in the Himalayas depends on elevation. Fungal Ecol. 41, 245–255. doi: 10.1016/j.funeco.2019.07.002
Döring, H., Clerc, P., Grube, M., and Wedin, M. (2000). Mycobiont-specific PCR primers for the amplification of nuclear ITS and LSU rDNA from lichenized ascomycetes. Lichenologist 32, 200–204. doi: 10.1006/lich.1999.0250
Ertz, D., Guzow-Krzemińska, B., Thor, G., Łubek, A., and Kukwa, M. (2018). Photobiont switching causes changes in the reproduction strategy and phenotypic dimorphism in the Arthoniomycetes. Sci. Rep. 8:4952. doi: 10.1038/s41598-018-23219-3
Fick, S. E., and Hijmans, R. J. (2017). WorldClim 2: new 1-km spatial resolution climate surfaces for global land areas. Int. J. Climatol. 37, 4302–4315. doi: 10.1002/joc.5086
Gardes, M., and Bruns, T. D. (1993). ITS primers with enhanced specificity for basidiomycetes—application to the identification of mycorrhizae and rusts. Mol. Ecol. 2, 113–118. doi: 10.1111/j.1365-294X.1993.tb00005.x
Gasulla, F., Barrasa, J. M., Casano, L. M., and del Campo, E. M. (2020). Symbiont composition of the basidiolichen Lichenomphalia meridionalis varies with altitude in the Iberian Peninsula. Lichenologist 52, 17–26. doi: 10.1017/S002428291900046X
Grube, M., Baloch, E., and Arup, U. (2004). A phylogenetic study of the Lecanora rupicola group (Lecanoraceae, Ascomycota). Mycol. Res. 108, 506–514. doi: 10.1017/S0953756204009888
Guderley, R. (1999). Die Lecanora subfusca-gruppe in Süd-und Mittelamerika. J. Hattori Bot. Lab. 87, 131–257.
Guzow-Krzemińska, B. (2006). Photobiont flexibility in the lichen Protoparmeliopsis muralis as revealed by ITS rDNA analyses. Lichenologist 38, 469–476. doi: 10.1017/S0024282906005068
Hauck, M., Helms, G., and Friedl, T. (2007). Photobiont selectivity in the epiphytic lichens Hypogymnia physodes and Lecanora conizaeoides. Lichenologist 39, 195–204. doi: 10.1017/S0024282907006639
Helms, G. (2003). Taxonomy and Symbiosis in Associations of Physciaceae and Trebouxia. Ph.D. dissertation. Göttingen: Georg-August Universität Göttingen.
Hestmark, G., Lutzoni, F., and Miadlikowska, J. (2016). Photobiont associations in co-occurring umbilicate lichens with contrasting modes of reproduction in coastal Norway. Lichenologist 48, 545–557. doi: 10.1017/S0024282916000232
Hijmans, R. J. (2021). raster: Geographic Data Analysis and Modeling. R Package Version 3.4-13. Available online at: https://CRAN.R-project.org/package=raster
Hoang, D. T., Chernomor, O., Von Haeseler, A., Minh, B. Q., and Vinh, L. S. (2018). UFBoot2: improving the ultrafast Bootstrap approximation. Mol. Biol. Evol. 35, 518–522. doi: 10.1093/molbev/msx281
James, P. W., and Henssen, A. (1976). “The morphological and taxonomical significance of cephalodia,” in Lichenology: Progress and Problems, eds D. H. Brown, D. L. Hawksworth, and R. H. Bailey (London: Academic Press), 27–77.
Jüriado, I., Kaasalainen, U., Jylhä, M., and Rikkinen, J. (2019). Relationships between mycobiont identity, photobiont specificity and ecological preferences in the lichen genus Peltigera (Ascomycota) in Estonia (northeastern Europe). Fungal Ecol. 39, 45–54. doi: 10.1016/j.funeco.2018.11.005
Kalyaanamoorthy, S., Minh, B. Q., Wong, T. K., Von Haeseler, A., and Jermiin, L. S. (2017). ModelFinder: fast model selection for accurate phylogenetic estimates. Nat. Methods 14, 587–589. doi: 10.1038/nmeth.4285
Katoh, K., Rozewicki, J., and Yamada, K. D. (2019). MAFFT online service: multiple sequence alignment, interactive sequence choice and visualization. Brief. Bioinform. 20, 1160–1166. doi: 10.1093/bib/bbx108
Kirika, P., Parnmen, S., and Lumbsch, T. (2012). Two new species of Lecanora sensu stricto (Lecanoraceae, Ascomycota) from east Africa. MycoKeys 3, 37–47. doi: 10.3897/mycokeys.3.3201
Kosecka, M., Guzow-Krzemińska, B., Černajová, I., Škaloud, P., Jabłońska, A., and Kukwa, M. (2021a). New lineages of photobionts in Bolivian lichens expand our knowledge on habitat preferences and distribution of Asterochloris algae. Sci. Rep. 11:8701. doi: 10.1038/s41598-021-88110-0
Kosecka, M., Guzow-Krzemińska, B., Jabłońska, A., Flakus, A., Rodriguez-Flakus, P., and Kukwa, M. (2021b). “Adaptation strategies of Bolivian lichens: biodiversity and ecology of Trebouxia photobionts,” in Proceedings of the IAL9 Program and Abstract Book, 82.
Kosecka, M., Jabłońska, A., Flakus, A., Rodriguez-Flakus, P., Kukwa, M., and Guzow-Krzemińska, B. (2020). Trentepohlialean algae (Trentepohliales, Ulvophyceae) show preference to selected mycobiont lineages in lichen symbioses. J. Phycol. 56, 979–993. doi: 10.1111/jpy.12994
Kroken, S., and Taylor, J. W. (2000). Phylogenetic species, reproductive mode, and specificity of the green alga Trebouxia forming lichens with the fungal genus Letharia. Bryologist 103, 645–660.
Leavitt, S. D., Kraichak, E., Nelsen, M. P., Altermann, S., Divakar, P. K., Alors, D., et al. (2015). Fungal specificity and selectivity for algae play a major role in determining lichen partnerships across diverse ecogeographic regions in the lichen-forming family Parmeliaceae (Ascomycota). Mol. Ecol. 24, 3779–3797. doi: 10.1111/mec.13271
Leavitt, S. D., Kraichak, E., Vondrak, J., Nelsen, M. P., Sohrabi, M., Perez-Ortega, S., et al. (2016). Cryptic diversity and symbiont interactions in rock-posy lichens. Mol. Phylogenet. Evol. 99, 261–274. doi: 10.1016/j.ympev.2016.03.030
Lindgren, H., Velmala, S., Hognabba, F., Goward, T., Holien, H., and Myllys, L. (2014). High fungal selectivity for algal symbionts in the genus Bryoria. Lichenologist 46, 681–695. doi: 10.1017/S0024282914000279
Liu, Y. J., Whelen, S., and Hall, B. D. (1999). Phylogenetic relationships among ascomycetes: evidence from an RNA polymerase II subunit. Mol. Biol. Evol. 16, 1799–1808. doi: 10.1093/oxfordjournals.molbev.a026092
Lu, J., Magain, N., Miadlikowska, J., Coyle, J. R., Truong, C., and Lutzoni, F. (2018). Bioclimatic factors at an intrabiome scale are more limiting than cyanobiont availability for the lichen-forming genus Peltigera. Am. J. Bot. 105, 1198–1211. doi: 10.1002/ajb2.1119
Lücking, R., Hodkinson, B. P., and Leavitt, S. D. (2017). The 2016 classification of lichenized fungi in the Ascomycota and Basidiomycota–approaching one thousand genera. Bryologist 119, 361–416. doi: 10.1639/0007-2745-119.4.361
Lutsak, T., Fernández-Mendoza, F., Kirika, P., Wondafrash, M., and Printzen, C. (2016). Mycobiont-photobiont interactions of the lichen Cetraria aculeata in high alpine regions of East Africa and South America. Symbiosis 68, 25–37. doi: 10.1007/s13199-015-0351-1
Lutzoni, F., Wagner, P., Reeb, V., and Zoller, S. (2000). Integrating ambiguously aligned regions of DNA sequences in phylogenetic analyses without violating positional homology. Syst. Biol. 49, 628–651. doi: 10.1080/106351500750049743
Maddison, W. P., and Maddison, D. R. (2018). Mesquite: A Modular System for Evolutionary Analysis. Version 3.51. Available online at: http://www.mesquiteproject.org
Magain, N., Miadlikowska, J., Goffinet, B., Sérusiaux, E., and Lutzoni, F. (2017). Macroevolution of specificity in cyanolichens of the genus Peltigera section Polydactylon (Lecanoromycetes, Ascomycota). Syst. Biol. 66, 74–99. doi: 10.1093/sysbio/syw065
Magain, N., Truong, C., Goward, T., Niu, D., Goffinet, B., Sérusiaux, E., et al. (2018). Species delimitation at a global scale reveals high species richness with complex biogeography and patterns of symbiont association in Peltigera section Peltigera (lichenized Ascomycota: Lecanoromycetes). Taxon 67, 836–870. doi: 10.12705/675.3
Mansournia, M. R., Bingyun, W. U., Matsushita, N., and Hogetsu, T. (2012). Genotypic analysis of the foliose lichen Parmotrema tinctorum using microsatellite markers: association of mycobiont and photobiont, and their reproductive modes. Lichenologist 44, 419–440. doi: 10.1017/S0024282911000909
Matheny, P. B. (2005). Improving phylogenetic inference of mushrooms with RPB1 and RPB2 nucleotide sequences (Inocybe; Agaricales). Mol. Phylogenet. Evol. 35, 1–20. doi: 10.1016/j.ympev.2004.11.014
Matheny, P. B., Liu, Y. J., Ammirati, J. F., and Hall, B. D. (2002). Using RPB1 sequences to improve phylogenetic inference among mushrooms (Inocybe, Agaricales). Am. J. Bot. 89, 688–698. doi: 10.3732/ajb.89.4.688
Miadlikowska, J., Kauff, F., Hofstetter, V., Fraker, E., Grube, M., Hafellner, J., et al. (2006). New insights into classification and evolution of the Lecanoromycetes (Pezizomycotina, Ascomycota) from phylogenetic analyses of three ribosomal RNA-and two protein-coding genes. Mycologia 98, 1088–1103. doi: 10.1080/15572536.2006.11832636
Miadlikowska, J., Kauff, F., Högnabba, F., Oliver, J. C., Molnár, K., Fraker, E., et al. (2014). Multigene phylogenetic synthesis for the class Lecanoromycetes (Ascomycota): 1307 fungi representing 1139 infrageneric taxa, 312 genera and 66 families. Mol. Phylogenet. Evol. 79, 132–168. doi: 10.1016/j.ympev.2014.04.003
Miller, M. A., Pfeiffer, W., and Schwartz, T. (2010). “Creating the CIPRES science gateway for inference of large phylogenetic trees,” in Proceedings of the Gateway Computing Environments Workshop (GCE), 14 Nov. 2010, New Orleans, LA, 1–8.
Muggia, L., Grube, M., and Tretiach, M. (2008). Genetic diversity and photobiont associations in selected taxa of the Tephromela atra group (Lecanorales, lichenised Ascomycota). Mycol. Prog. 7, 147–160. doi: 10.1007/s11557-008-0560-6
Muggia, L., Leavitt, S. D., and Barreno, E. (2018). The hidden diversity of lichenised Trebouxiophyceae (Chlorophyta). Phycologia 57, 503–524. doi: 10.2216/17-134.1
Muggia, L., Nelsen, M. P., Kirika, P. M., Barreno, E., Beck, A., Lindgren, H., et al. (2020). Formally described species woefully underrepresent phylogenetic diversity in the common lichen photobiont genus Trebouxia (Trebouxiophyceae, Chlorophyta): an impetus for developing an integrated taxonomy. Mol. Phylogenet. Evol. 149:106821. doi: 10.1016/j.ympev.2020.106821
Muggia, L., Pérez-Ortega, S., Kopun, T., Zellnig, G., and Grube, M. (2014). Photobiont selectivity leads to ecological tolerance and evolutionary divergence in a polymorphic complex of lichenized fungi. Ann. Bot. 114, 463–475. doi: 10.1093/aob/mcu146
Navarro, G., and Ferreira, W. (2007). Mapa de Vegetación de Bolivia, Escala 1: 250000. Edición CD-ROM. RUMBOL SRL. Depósito Legal 2-7-116-11. Edición auspiciada por The Nature Conservancy (TNC), CONDESAN, The Natureserve.
Navarro, G., and Maldonado, M. (2002). Geografía Ecológica de Bolivia: Vegetación y Ambientes Acuáticos. Cochabamba: Centro de Ecología Simón I. Patiño, Departamento de Difusión.
Nelsen, M. P., Leavitt, S. D., Heller, K., Muggia, L., and Lumbsch, H. T. (2021). Macroecological diversification and convergence in a clade of keystone symbionts. FEMS Microbiol. Ecol. 97:fiab072. doi: 10.1093/femsec/fiab072
Nelsen, M. P., Rivas Plata, E., Andrew, C. J., Lücking, R., and Lumbsch, H. T. (2011). Phylogenetic diversity of Trentepohlialean algae associated with lichen-forming fungi. J. Phycol. 47, 282–290.
Nguyen, L. T., Schmidt, H. A., Von Haeseler, A., and Minh, B. Q. (2015). IQ-TREE: a fast and effective stochastic algorithm for estimating maximum-likelihood phylogenies. Mol. Biol. Evol. 32, 268–274. doi: 10.1093/molbev/msu300
Nottingham, A. T., Fierer, N., Turner, B. L., Whitaker, J., Ostle, N. J., McNamara, N. P., et al. (2018). Microbes follow Humboldt: temperature drives plant and soil microbial diversity patterns from the Amazon to the Andes. Ecology 99, 2455–2466. doi: 10.1002/ecy.2482
Nyati, S., Scherrer, S., Werth, S., and Honegger, R. (2014). Green-algal photobiont diversity (Trebouxia spp.) in representatives of Teloschistaceae (Lecanoromycetes, lichen-forming ascomycetes). Lichenologist 46, 189–212. doi: 10.1017/S0024282913000819
Ohmura, Y., Kawachi, M., Kasai, F., Watanabe, M. M., and Takeshita, S. (2006). Genetic combinations of symbionts in a vegetatively reproducing lichen, Parmotrema tinctorum, based on ITS rDNA sequences. Bryologist 109, 43–59.
Oksanen, J., Blanchet, F. G., Friendly, M., Kindt, R., Legendre, P., McGlinn, D., et al. (2020). vegan: Community Ecology Package. R Package Version 2.5-7. Available online at: https://CRAN.R-project.org/package=vegan
Orange, A., James, P. W., and White, F. J. (2001). Microchemical Methods for the Identification of Lichens. London: British Lichen Society.
Papong, K., Boonpragob, K., Parnmen, S., and Lumbsch, H. T. (2013). Molecular phylogenetic studies on tropical species of Lecanora sensu stricto (Lecanoraceae, Ascomycota). Nova Hedwigia 96, 1–13. doi: 10.1127/0029-5035/2012/0072
Paradis, E. (2010). pegas: an R package for population genetics with an integrated–modular approach. Bioinformatics 26, 419–420. doi: 10.1093/bioinformatics/btp696
Paradis, E., and Schliep, K. (2019). ape 5.0: an environment for modern phylogenetics and evolutionary analyses in R. Bioinformatics 35, 526–528. doi: 10.1093/bioinformatics/bty633
Paul, F., Otte, J., Schmitt, I., and Dal Grande, F. (2018). Comparing Sanger sequencing and high-throughput metabarcoding for inferring photobiont diversity in lichens. Sci. Rep. 8:8624. doi: 10.1038/s41598-018-26947-8
Pebesma, E. J., and Bivand, R. S. (2005). Classes and methods for spatial data in R. R News 5, 9–13.
Peksa, O., and Škaloud, P. (2011). Do photobionts influence the ecology of lichens? A case study of environmental preferences in symbiotic green alga Asterochloris (Trebouxiophyceae). Mol. Ecol. 20, 3936–3948. doi: 10.1111/j.1365-294X.2011.05168.x
Pérez-Ortega, S., Ortiz-Álvarez, R., Allan Green, T. G., and de Los Ríos, A. (2012). Lichen myco-and photobiont diversity and their relationships at the edge of life (McMurdo Dry Valleys, Antarctica). FEMS Microbiol. Ecol. 82, 429–448. doi: 10.1111/j.1574-6941.2012.01422.x
Pérez-Ortega, S., Spribille, T., Palice, Z., Elix, J. A., and Printzen, C. (2010). A molecular phylogeny of the Lecanora varia group, including a new species from western North America. Mycol. Prog. 9, 523–535. doi: 10.1007/s11557-010-0660-y
Puillandre, N., Lambert, A., Brouillet, S., and Achaz, G. (2012). ABGD, Automatic Barcode Gap Discovery for primary species delimitation. Mol. Ecol. 21, 1864–1877. doi: 10.1111/j.1365-294X.2011.05239.x
R Core Team (2019). R: A Language and Environment for Statistical Computing. Vienna: R Foundation for Statistical Computing.
Řeháková, K., Chlumská, Z., and Doležal, J. (2011). Soil cyanobacterial and microalgal diversity in dry mountains of Ladakh, NW Himalaya, as related to site, altitude, and vegetation. Microb. Ecol. 62, 337–346. doi: 10.1007/s00248-011-9878-8
Rodriguez Flakus, P., and Printzen, C. (2014). Palicella, a new genus of lichenized fungi and its phylogenetic position within Lecanoraceae. Lichenologist 46, 535–552. doi: 10.1017/S0024282914000127
Rolshausen, G., Hallman, U., Dal Grande, F., Otte, J., Knudsen, K., and Schmitt, I. (2020). Expanding the mutualistic niche: parallel symbiont turnover along climatic gradients. Proc. R. Soc. B 287:20192311. doi: 10.1098/rspb.2019.2311
Ruprecht, U., Brunauer, G., and Printzen, C. (2012). Genetic diversity of photobionts in Antarctic lecideoid lichens from an ecological view point. Lichenologist 44, 661–678. doi: 10.1017/S0024282912000291
Ruprecht, U., Fernández-Mendoza, F., Türk, R., and Fryday, A. M. (2020). High levels of endemism and local differentiation in the fungal and algal symbionts of saxicolous lecideoid lichens along a latitudinal gradient in southern South America. Lichenologist 52, 287–303. doi: 10.1017/S0024282920000225
Sadowska-Deś, A. D., Dal Grande, F., Lumbsch, H. T., Beck, A., Otte, J., Hur, J. S., et al. (2014). Integrating coalescent and phylogenetic approaches to delimit species in the lichen photobiont Trebouxia. Mol. Phylogenet. Evol. 76, 202–210. doi: 10.1016/j.ympev.2014.03.020
Schmitt, I., Crespo, A., Divakar, P. K., Fankhauser, J. D., Herman-Sackett, E., Kalb, K., et al. (2009). New primers for promising single-copy genes in fungal phylogenetics and systematics. Persoonia 23, 35–40. doi: 10.3767/003158509X470602
Schrodt, F., Santos, M. J., Bailey, J. J., and Field, R. (2019). Challenges and opportunities for biogeography—what can we still learn from von Humboldt? J. Biogeogr. 46, 1631–1642. doi: 10.1111/jbi.13616
Singh, G., Dal Grande, F., Divakar, P. K., Otte, J., Crespo, A., and Schmitt, I. (2017). Fungal–algal association patterns in lichen symbiosis linked to macroclimate. New Phytol. 214, 317–329. doi: 10.1111/nph.14366
Singh, G., Kukwa, M., Dal Grande, F., Łubek, A., Otte, J., and Schmitt, I. (2019). A glimpse into genetic diversity and symbiont interaction patterns in lichen communities from areas with different disturbance histories in Białowieża forest, Poland. Microorganisms 7:335. doi: 10.3390/microorganisms7090335
Śliwa, L., Rodriguez Flakus, P., Wilk, K., and Flakus, A. (2014). New records of Lecanora for Bolivia. II. Pol. Bot. J. 59, 97–103. doi: 10.2478/pbj-2014-0021
Śliwa, L., Wilk, K., Rodriguez Flakus, P., and Flakus, A. (2013). New records of Lecanora for Bolivia. Mycotaxon 121, 385–392. doi: 10.5248/121.385
Soto-Medina, E., Lücking, R., Silverstone-Sopkin, P. A., and Torres, A. M. (2019). Changes in functional and taxonomic diversity and composition of corticolous lichens in an altitudinal gradient in Colombia. Cryptogam. Mycol. 40, 97–115. doi: 10.5252/cryptogamie-mycologie2019v40a6
Stewart, A., Rioux, D., Boyer, F., Gielly, L., Pompanon, F., Saillard, A., et al. (2021). Altitudinal zonation of green algae biodiversity in the French Alps. Front. Plant Sci. 12:679428. doi: 10.3389/fpls.2021.679428
Stiller, J. W., and Hall, B. D. (1997). The origin of red algae: implications for plastid evolution. Proc. Natl. Acad. Sci. U.S.A. 94, 4520–4525.
Tello, J. S., Myers, J. A., Macía, M. J., Fuentes, A. F., Cayola, L., Arellano, G., et al. (2015). Elevational gradients in β-diversity reflect variation in the strength of local community assembly mechanisms across spatial scales. PLoS One 10:e0121458. doi: 10.1371/journal.pone.0121458
Tschermak-Woess, E. (1978). Über die phycobionten der sektion Cystophora von Chaenotheca, insbesondere Dictyochloropsis splendida und Trebouxia simplex, spec. nova. Plant Syst. Evol. 129, 185–208. doi: 10.1007/BF00990760
Vančurová, L., Muggia, L., Peksa, O., Řídká, T., and Škaloud, P. (2018). The complexity of symbiotic interactions influences the ecological amplitude of the host: a case study in Stereocaulon (lichenized Ascomycota). Mol. Ecol. 27, 3016–3033. doi: 10.1111/mec.14764
Vargas Castillo, R., and Beck, A. (2012). Photobiont selectivity and specificity in Caloplaca species in a fog-induced community in the Atacama Desert, northern Chile. Fungal Biol. 116, 665–676. doi: 10.1016/j.funbio.2012.04.001
Vilgalys, R., and Hester, M. (1990). Rapid genetic identification and mapping of enzymatically amplified ribosomal DNA from several Cryptococcus species. J. Bacteriol. 172, 4238–4246. doi: 10.1128/jb.172.8.4238-4246.1990
von Humboldt, A., and Bonpland, A. (2009). Essay on the Geography of Plants, ed. S. T. Jackson, trans. S. Romanowski (Chicago, IL: University of Chicago Press).
Voytsekhovich, A., and Beck, A. (2016). Lichen photobionts of the rocky outcrops of Karadag massif (Crimean Peninsula). Symbiosis 68, 9–24. doi: 10.1007/s13199-015-0346-y
Wagner, M., Bathke, A. C., Cary, S. C., Green, T. A., Junker, R. R., Trutschnig, W., et al. (2020). Myco-and photobiont associations in crustose lichens in the McMurdo Dry Valleys (Antarctica) reveal high differentiation along an elevational gradient. Polar Biol. 43, 1967–1983. doi: 10.1007/s00300-020-02754-8
Werth, S., and Sork, V. L. (2014). Ecological specialization in Trebouxia (Trebouxiophyceae) photobionts of Ramalina menziesii (Ramalinaceae) across six range-covering ecoregions of western North America. Am. J. Bot. 101, 1127–1140. doi: 10.3732/ajb.1400025
White, T. J., Bruns, T., Lee, S., and Taylor, J. (1990). “Amplification and direct sequencing of fungal ribosomal RNA genes for phylogenetics,” in PCR Protocols: A Guide to Methods and Applications, eds M. A. Innis, D. H. Gelfand, J. J. Sninsky, and T. J. White (London: Academic Press), 315–322.
Wolf, J. H. (1993). Diversity patterns and biomass of epiphytic bryophytes and lichens along an altitudinal gradient in the northern Andes. Ann. Mo. Bot. Gard. 80, 928–960.
Xu, M., De Boer, H., Olafsdottir, E. S., Omarsdottir, S., and Heidmarsson, S. (2020). Phylogenetic diversity of the lichenized algal genus Trebouxia (Trebouxiophyceae, Chlorophyta): a new lineage and novel insights from fungal-algal association patterns of Icelandic cetrarioid lichens (Parmeliaceae, Ascomycota). Bot. J. Linn. Soc. 194, 460–468. doi: 10.1093/botlinnean/boaa050
Yahr, R., Vilgalys, R., and DePriest, P. T. (2004). Strong fungal specificity and selectivity for algal symbionts in Florida scrub Cladonia lichens. Mol. Ecol. 13, 3367–3378. doi: 10.1111/j.1365-294X.2004.02350.x
Yakovchenko, L. S., Davydov, E. A., Ohmura, Y., and Printzen, C. (2019). The phylogenetic position of species of Lecanora sl containing calycin and usnic acid, with the description of Lecanora solaris Yakovchenko & Davydov sp. nov. Lichenologist 51, 147–156. doi: 10.1017/S0024282919000045
Yu, G., Smith, D. K., Zhu, H., Guan, Y., and Lam, T. T. Y. (2017). ggtree: an R package for visualization and annotation of phylogenetic trees with their covariates and other associated data. Methods Ecol. Evol. 8, 28–36. doi: 10.1111/2041-210X.12628
Zhang, J., Kapli, P., Pavlidis, P., and Stamatakis, A. (2013). A general species delimitation method with applications to phylogenetic placements. Bioinformatics 29, 2869–2876. doi: 10.1093/bioinformatics/btt499
Zhao, X., Leavitt, S. D., Zhao, Z. T., Zhang, L. L., Arup, U., Grube, M., et al. (2016). Towards a revised generic classification of lecanoroid lichens (Lecanoraceae, Ascomycota) based on molecular, morphological and chemical evidence. Fungal Divers. 78, 293–304. doi: 10.1007/s13225-015-0354-5
Zhou, S., and Stanosz, G. R. (2001). Primers for amplification of mt SSU rDNA, and a phylogenetic study of Botryosphaeria and associated anamorphic fungi. Mycol. Res. 105, 1033–1044. doi: 10.1016/S0953-7562(08)61965-6
Keywords: elevation gradients, systematics, symbiosis, Andes mountains, new PCR primer, Lecanoromycetes, Trebouxiophyceae, lichen biogeography
Citation: Medeiros ID, Mazur E, Miadlikowska J, Flakus A, Rodriguez-Flakus P, Pardo-De la Hoz CJ, Cieślak E, Śliwa L and Lutzoni F (2021) Turnover of Lecanoroid Mycobionts and Their Trebouxia Photobionts Along an Elevation Gradient in Bolivia Highlights the Role of Environment in Structuring the Lichen Symbiosis. Front. Microbiol. 12:774839. doi: 10.3389/fmicb.2021.774839
Received: 13 September 2021; Accepted: 19 November 2021;
Published: 20 December 2021.
Edited by:
Lucia Muggia, University of Trieste, ItalyReviewed by:
Gábor M. Kovács, Eötvös Loránd University, HungaryJulieta Orlando, University of Chile, Chile
Copyright © 2021 Medeiros, Mazur, Miadlikowska, Flakus, Rodriguez-Flakus, Pardo-De la Hoz, Cieślak, Śliwa and Lutzoni. This is an open-access article distributed under the terms of the Creative Commons Attribution License (CC BY). The use, distribution or reproduction in other forums is permitted, provided the original author(s) and the copyright owner(s) are credited and that the original publication in this journal is cited, in accordance with accepted academic practice. No use, distribution or reproduction is permitted which does not comply with these terms.
*Correspondence: Jolanta Miadlikowska, am9sYW50YW1AZHVrZS5lZHU=
†These authors have contributed equally to this work and share first authorship
‡These authors share senior authorship