- 1Department of Microbiology and Biotechnology, University of Hamburg, Hamburg, Germany
- 2Molecular Microbiology, Institute for General Microbiology, Kiel University, Kiel, Germany
- 3Center for Structural Studies, Heinrich-Heine-University, Düsseldorf, Germany
- 4Department of Microbiology and Immunology, University of British Columbia, Vancouver, BC, Canada
- 5Department of Biochemistry, University of Bayreuth, Bayreuth, Germany
- 6Institute of Biochemistry and Technical Biochemistry, University of Stuttgart, Stuttgart, Germany
- 7European Bioinformatics Institute (EMBL-EBI), Hinxton, United Kingdom
- 8Wellcome Sanger Institute, Hinxton, United Kingdom
- 9Graduate Program in Bioinformatics, University of British Columbia, Vancouver, BC, Canada
- 10Genome Science and Technology Program, University of British Columbia, Vancouver, BC, Canada
- 11Life Sciences Institute, University of British Columbia, Vancouver, BC, Canada
- 12ECOSCOPE Training Program, University of British Columbia, Vancouver, BC, Canada
- 13Institute of Biochemistry, Heinrich-Heine-University, Düsseldorf, Germany
Certain members of the Actinobacteria and Proteobacteria are known to degrade polyethylene terephthalate (PET). Here, we describe the first functional PET-active enzymes from the Bacteroidetes phylum. Using a PETase-specific Hidden-Markov-Model- (HMM-) based search algorithm, we identified several PETase candidates from Flavobacteriaceae and Porphyromonadaceae. Among them, two promiscuous and cold-active esterases derived from Aequorivita sp. (PET27) and Kaistella jeonii (PET30) showed depolymerizing activity on polycaprolactone (PCL), amorphous PET foil and on the polyester polyurethane Impranil® DLN. PET27 is a 37.8 kDa enzyme that released an average of 174.4 nmol terephthalic acid (TPA) after 120 h at 30°C from a 7 mg PET foil platelet in a 200 μl reaction volume, 38-times more than PET30 (37.4 kDa) released under the same conditions. The crystal structure of PET30 without its C-terminal Por-domain (PET30ΔPorC) was solved at 2.1 Å and displays high structural similarity to the IsPETase. PET30 shows a Phe-Met-Tyr substrate binding motif, which seems to be a unique feature, as IsPETase, LCC and PET2 all contain Tyr-Met-Trp binding residues, while PET27 possesses a Phe-Met-Trp motif that is identical to Cut190. Microscopic analyses showed that K. jeonii cells are indeed able to bind on and colonize PET surfaces after a few days of incubation. Homologs of PET27 and PET30 were detected in metagenomes, predominantly aquatic habitats, encompassing a wide range of different global climate zones and suggesting a hitherto unknown influence of this bacterial phylum on man-made polymer degradation.
Introduction
PET is one of the major plastic pollutants found in landfills, oceans and other environments (Jambeck et al., 2015; Geyer et al., 2017). Our knowledge of microbial degradation of most plastics is rather limited, but recent research has demonstrated that some bacteria are able to degrade PET (Yoshida et al., 2016). Although it is unclear if larger crystalline fibers are degraded by bacteria, it is well known that some cutinases (EC 3.1.1.74), lipases (EC 3.1.1.3) and carboxylesterases (EC 3.1.1.1) can act on amorphous and low crystalline PET. These enzymes, often referred to as “PETases,” cleave the ester bond of the polymer to either produce bis-(2-hydroxyethyl) terephthalate (BHET), mono-hydroxyethyl terephthalate (MHET) or they complete degradation to terephthalic acid (TPA) and ethylene glycol (EG). TPA monomers can be further degraded via cleavage of the aromatic ring structure using known aryl pathways and can then enter the β-ketoadipate pathway (Wei and Zimmermann, 2017; Danso et al., 2019; Wright et al., 2021).
To date, only a limited number of bacterial and fungal species have been identified that are capable of breaking down PET to either its oligomers or monomers, TPA and EG. Most bacterial isolates with verified enzymatic PET-degrading activity are affiliated with the Gram-positive phylum Actinobacteria (Acero et al., 2011). The best characterized examples belong to the genera Thermobifida or Thermomonospora (Kleeberg et al., 1998; Chen et al., 2008; Hu et al., 2010; Acero et al., 2011; Ribitsch et al., 2012; Wei et al., 2014). Further, the leaf compost-derived cutinase LCC is closely related to Actinobacterial enzymes and is currently one of the best described and most active PETases (Sulaiman et al., 2012, 2014). Regarding Proteobacteria, the Gram-negative Betaproteobacterium Ideonella sakaiensis 201-F6 is capable of using amorphous PET as a major energy and carbon source (Yoshida et al., 2016). I. sakaiensis’ genome also encodes a tannase which is designated MHETase as it is capable of degrading MHET. Besides, a number of other PETases affiliated with the Proteobacteria have been identified originating from e.g., Pseudomonas aestusnigri and Vibrio gazogenes (Ronkvist et al., 2009; Haernvall et al., 2017; Danso et al., 2018; Bollinger et al., 2020).
In a previous study, we identified potential PET esterases affiliated with the Bacteroidetes phylum using HMM profile database searches (Danso et al., 2018, 2019). These enzyme hits mainly occurred in metagenomes and genomes from marine environments and were annotated solely on the basis of homology. However, their enzymatic function and environmental distributions have not been studied within that framework, and we target these questions in the present study. Bacteroidetes representatives can be found in nearly all ecological niches including soils, oceans and fresh water and are part of the microbiome of many animals, especially as inhabitants of the intestinal tract (Wexler, 2007; Krieg et al., 2015; Hahnke et al., 2016; Munoz et al., 2016). The Bacteroidetes phylum, however, is highly heterogeneous and contains at least four classes of bacteria (e.g., Bacteroidia, Flavobacteria, Sphingobacteria, and Cytophagia), with each class having several thousand described species. The phylum contains non-spore forming and rod shaped microorganisms, some aerobic, but often anerobic, with an enormous metabolic diversity (Krieg et al., 2015). The global distribution of Bacteroidetes representatives is likely due to their ability to decompose a very wide variety of bio-based polymers such as cellulose, chitin or algal cell walls. In particular, the decomposition of polysaccharides (cellulose and hemicellulose) by Bacteroidetes inhabiting the intestinal tract of humans and animals has been well-studied in gut microbiome research (Thomas et al., 2011).
Here, we provide the first experimental evidence that different Bacteroidetes representatives have evolved promiscuous esterases that degrade the PET polymer. We show that at least two Bacteroidetes genera, Aequorivita and Kaistella (formerly Chryseobacterium), harbor PET-active enzymes and elucidated the crystal structure of PET30. These enzymes have relatively low turnover rates, indicating that PET hydrolysis may be a side reaction. Still, given their abundance and diversity, we speculate that the described bacteroidetal PET-active enzymes could have considerable impact on long-term degradation of PET in the marine environment.
Materials and Methods
Bacterial Strains, Plasmids, and Primers
Bacterial strains, plasmids and primers used in this study are listed in Supplementary Tables 1, 2. If not mentioned otherwise, Escherichia coli clones were grown in LB medium (1% tryptone/peptone, 0.5% yeast extract, 1% NaCl) supplemented with appropriate antibiotics (25 μg/ml kanamycin, or 100 μg/ml ampicillin) at 37°C for 18 h.
Databases Used in This Study and Bioinformatic Analyses
Nucleotide and amino acid sequences of putative and confirmed PETases were acquired from databases integrated into the NCBI1, UniProt2 and IMG (JGI)3 servers (Markowitz et al., 2012; NCBI Resource Coordinators, 2017; The UniProt Consortium, 2017). Human gut sequences were retrieved from the Unified Human Gastrointestinal Protein (UHGP) catalog (PMID: 32690973). Sequences were compared to others deposited in the NCBI databases using BLAST alignment tools (Agarwala et al., 2016). Amino acid sequence HMM search was carried out using the HMMER4 webpage or a local version of the software (v3.1b2) (Mistry et al., 2013) with downloaded datasets. Structural information on the enzymes was retrieved from the RCSB-PDB (Berman et al., 2000) database.
Sequence data were processed and analyzed using ChromasPro 2.1.8 (Technelysium, Brisbaine Australia) or SnapGene (GSL Biotech LLC, San Diego CA, United States). Amino acid alignment was constructed using structural alignments with T-Coffee (Notredame et al., 2000) and was further visualized with Bioedit (Hall, 1999). The model structures of bacteroidetal PETase-candidates were modeled with the Robetta server (Kim et al., 2004) using the IsPETase crystal structure (6EQE) as a backbone. A phylogenetic tree was constructed using the RAxML-NG autoMRE algorithm (Kozlov et al., 2019) with the treesapp create command implemented in TreeSAPP (Morgan-Lang et al., 2020) with maximum bootstraps set at 1,000. RAxML-MG has recently been shown to return the best scoring tree for highest number of datasets when compared against other fast maximum likelihood (ML) methods (Kozlov et al., 2019), allowing a large number of maximum bootstraps to be used to produce as conservative a tree as possible. Sequences were assigned NCBI lineages according to source organisms listed in Table 1 and Supplementary Table 3, and colors were assigned to the tree at the phylum level using the treesapp color command. The tree was visualized in iTOL (Letunic and Bork, 2019).
Scanning IMG/M was completed on 19/November/2020 for PET30 and on 14/January/2021 for PET27. Geo locations were used as provided whenever available. In case no Geo location was available, whenever possible, information about isolation source/location/city/country were used to look up Geo coordinates on GeoHack.5 The map representing the frequency and geographical distribution of PET hydrolases in metagenomes (Figure 1) was constructed using QGis Desktop 2.18.56.
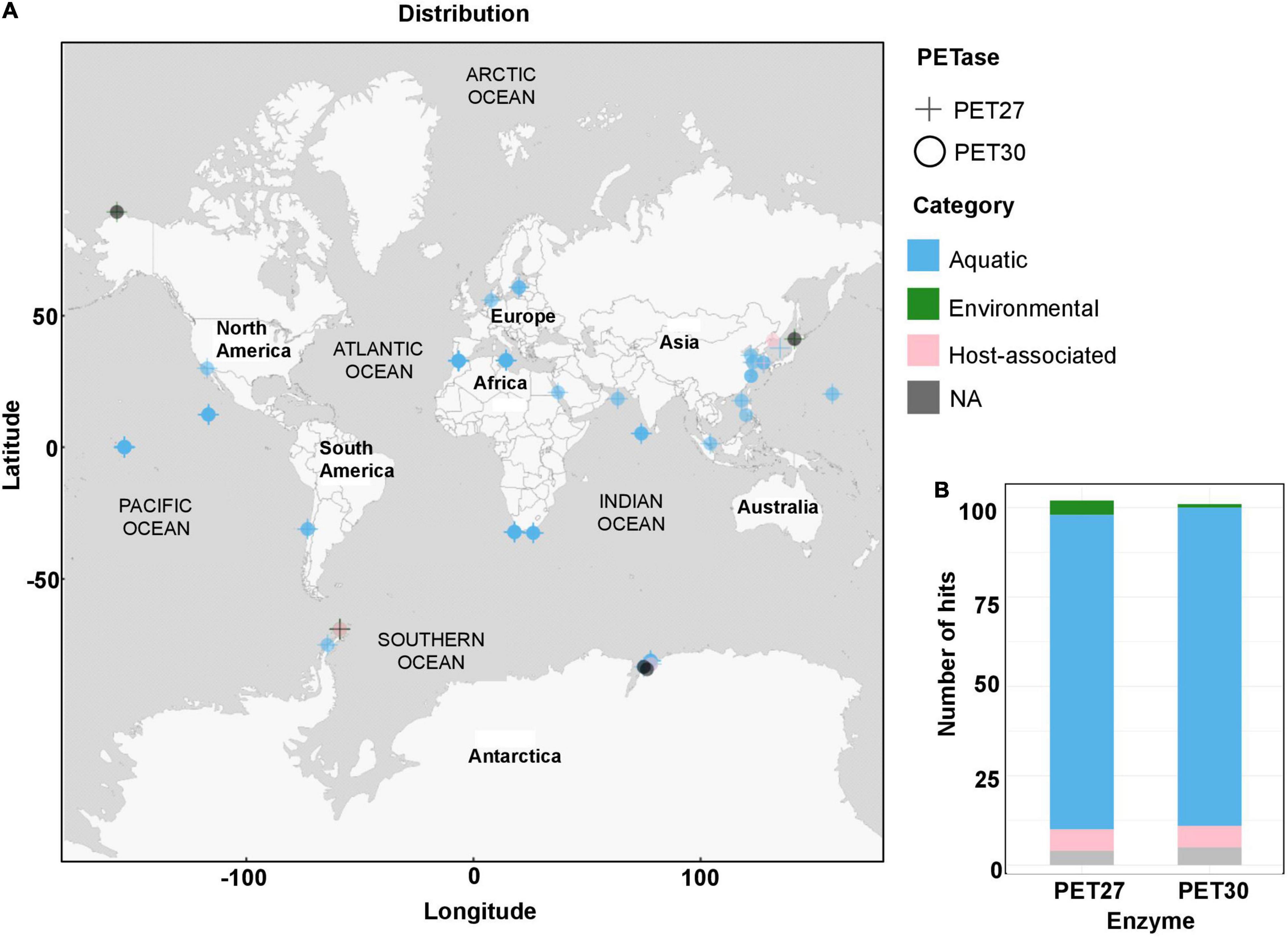
Figure 1. Global distribution of PET27 and PET30 homologs. (A) PET27 and PET30 homologs containing metagenomes were visualized on a world map containing circles for the different metagenomes. The cut-off in the similarity searches was set to 50%. Data depicted include only hits to bacteria affiliated with the Flavobacteria-Cytophaga-Bacteroidetes (FCB) group. The metagenomes searched and included in this figure are listed in Supplementary Table 2. (B) Number of hits observed in the same global metagenomes. Color code indicates the type of habitat: air, aquatic, terrestrial host-associated and/or engineered.
Heterologous Expression of Putative Polyethylene Terephthalate Esterase Genes in Escherichia coli BL21 (DE3)
The putative PETases were extracted from metagenomic datasets (Table 1), therefore the gene sequences were optimized for expression in E. coli and synthesized into pET21a(+) vector at Biomatik (Wilmington, United States). The obtained constructs were sequenced at Microsynth Seqlab GmbH (Goettingen, Germany) and checked for correctness by comparing to the original sequences. E. coli T7-Shuffle or E. coli BL21 (DE3) cells were used for heterologous expression of possible PETases. The IsPETase gene in pMAL-p4x was expressed in E. coli BL21 and purified by its maltose-binding tag. The cultures were grown aerobically in auto-induction medium (ZYM-5052) (Studier, 2005) containing 100 μg/ml ampicillin at 37°C until they reached an OD600 of 1.0. The proteins were expressed afterward at 22°C for 16–20 h. The cells were harvested and lysed with pressure using a French press. Afterward, the proteins harboring a sixfold C-terminal histidine tag were purified with nickel-ion affinity chromatography using Ni-NTA agarose (Qiagen, Hilden, Germany) and analyzed by SDS-PAGE. The elution buffer was exchanged against 0.1 mM potassium phosphate buffer pH 8.0 in a 10 kDa Amicon Tube (GE Health Care, Solingen, Germany).
Biochemical Characterization of PET27 and PET30
For activity tests, both enzymes were assayed using purified recombinant protein. bis-(2-hydroxyethyl) terephthalate (BHET) and polycaprolactone (PCL) agar plates were prepared as described elsewhere (Pérez-García et al., 2021). The polyester polyurethane Impranil DLN containing LB agar plates were prepared according to Molitor et al. (2020) with LB medium. For the pNP-assay, unless otherwise indicated, a total amount of 0.1–1 μg of the enzymes were added to a substrate solution containing 190 μl of either 0.2 M sodium phosphate buffer or 0.1 M potassium phosphate with a defined pH between 7 and 8 and 10 μl of 0.1 mM pNP-substrate dissolved in isopropanol. After incubating the samples for 10 min, the assay was stopped by adding 200 mM of Na2CO3. Afterward, the samples were centrifuged at 4°C, 13,000 rpm for 3 min. As substrates, pNP-esters with chain lengths of C4, C6, C8, C10, C12, C14, C16 and C18 were tested. After incubation at defined temperatures, the color change from colorless to yellow was measured at 405 nm in a plate reader (Biotek, Winooski, United States). All samples were measured in triplicate. To determine the optimal temperature, samples were incubated between 10 and 90°C for 10 min. The influence of pH conditions on the activity of each enzyme was measured in citrate phosphate (pH 3.0, 4.0, and 5.0), potassium phosphate (pH 6.0, 7.0, and 8.0) and carbonate bicarbonate buffer (pH 9.2 and 10.2). The impact of cofactors, solvents, detergents, and inhibitors was assayed at different concentration levels. The possible cofactors Ca2+, Co2+, Cu2+, Fe3+, Mg2+, Mn2+, Rb2+, and Zn2+ with a final concentration of 1 and 10 mM were used. Detergent stability was assayed with sodium dodecyl sulfate (SDS), Triton X-100 and Tween 80 at 1 and 5% (w/v, v/v) concentration. The inhibitory effect of ethylenediaminetetraacetic acid (EDTA), dithiothreitol (DTT) and phenylmethanesulfonyl fluoride (PMSF) was tested at 1 and 10 mM concentration. After 1 h incubation in the presence of these substances, the residual activity was determined after 10 min incubation at the optimal temperature with para-nitrophenol- (pNP-) C6 and at the optimal pH.
For the verification of enzymatic PET hydrolysis, a 7 mg platelet (Ø 5 mm) of low-crystallinity PET film (Goodfellow GmbH, Bad Nauheim, Germany), which corresponds to 36.4 μmol of the terephthalic acid-ethylene glycol (TPA-EG) unit, was folded in half and used as substrate together with 200 μg of enzyme in 200 μl of 100 mM potassium phosphate buffer at pH 8.0. Incubation was carried out under continuous shaking at 400 rpm in 1.5 ml microcentrifuge tubes at 30°C, if not stated otherwise.
Analysis of breakdown products was performed with an UltiMate™ 3000 UHPLC system from Thermo Fisher Scientific (Waltham, MA, United States) using a Triart C18 column (YMC Europe GmbH, Dinslaken, Germany) with a dimension of 100 × 2.0 mm containing particles with 1.9 μm diameter. Isocratic elution was performed using a mobile phase consisting of 20:80 (v/v) acetonitrile and water (acidified with 0.1% vol trifluoroacetic acid) at a flowrate of 0.4 ml min–1. UHPLC samples were prepared by mixing 50 μl of incubation supernatant with 200 μl acetonitrile (acidified with 1% vol trifluoroacetic acid), followed by centrifugation at 10,000 × g for 3 min and transferring 200 μl of the supernatant into 600 μl water. Fifteen microliter of sample were injected per measurement and detection was performed at 254 nm with a VWD-3400 detector from Thermo Scientific (Waltham, MA, United States). The UHPLC profiles were plotted and edited using the software MATLAB version R2020b [The MathWorks, Inc., Natick, MA, United States (Matlab, 2012)]. Quantification of peak areas was performed using data analysis software supplied with the Compass HyStar software package from Bruker (Billerica, MA, United States).
Crystallization and Data Collection
Crystallization of PET30ΔPorC was achieved by sitting-drop vapor-diffusion at 12°C. 0.2 μl of 10.2 mg/ml PET30ΔPorC in 100 mM phosphate buffer pH 8.0 and 0.1 μl reservoir solution consisting of 0.1 M sodium aetate pH 4.6 and 25% (w/v) PEG 4000 were mixed. This drop was equilibrated against reservoir solution and crystals formed after several weeks. Crystallization drops were overlayed with mineral oil and the crystals were dragged through it for cryoprotection, flash frozen and diffraction data were collected at beamline P13 (DESY, Hamburg, Germany). The PET30ΔPorC crystals had the space group P 43 21 2 and diffracted to 2.1 Å resolution.
Structure Determination
A complete data set of the PET30ΔPorC was collected at beamline P13 (DESY, EMBL, Hamburg, Germany) at 100 K and wavelength 0.9795 Å up to 2.1 Å resolution. All data were processed using the automated pipeline at the EMBL HAMBURG and reprocessed afterward using XDS (Kabsch, 2014). The above obtained model for PET30ΔPorC by TOPMODEL was successfully used to phase the 2.1 Å data set of PET30ΔPorC using the PHASER program from the PHENIX program suite (Afonine et al., 2012; Mulnaes et al., 2020). The structure was then refined in iterative cycles of manual building and refinement in coot followed by software-based refinements using the program suite Phenix (Emsley and Cowtan, 2004; Liebschner et al., 2019). All residues were in the preferred and additionally allowed regions of the Ramachandran plot. The data collection and refinement statistics are listed in Supplementary Table 4. The images of the models were prepared using PyMOL (DeLano, 2002) and UCSF Chimera X.7 The structure was deposited at the worldwide protein data bank under the accession code 7PZJ.
Confocal Laser Scanning Microscopy of Polyethylene Terephthalate Foil Platelets
The starter culture of K. jeonii was grown in R2A medium at 22°C and 130 rpm to a cell density of 0.2. 1% of the starter culture was inoculated into 30 ml fresh R2A medium and PET foil platelets were put into the cultures. PET platelets were removed after 5-7 days, washed three times with PBS and subsequently given into μ-Slide 8 wells plates from ibidi GmbH (Martinsried, Germany). Cells were stained using 100 μl of LIVE/DEAD stain BacLight Viability Kit (Thermo Fisher Scientific). The stain is composed of propidium iodide (PI) dying dead cells with a damaged membrane and causing red fluorescence and green fluorescence SYTO 9™ dying all bacterial membranes of living cells. Therefore, 10 μl PI and 10 μl SYTO 9™ were mixed. 15 μl of the nucleic acid-binding stains were pipetted into 5 ml PBS. The PET platelets were incubated for 1 h in the dark at room temperature. Afterward, the samples were investigated under the microscope Axio Observer Z1/7, LSM 800 using objective C-Apochromat 63x/1.20 W Korr UV VisIR (both Carl Zeiss Microscopy GmbH, Jena, Germany) using the Channels Syto 09 (528/20 nm emission wavelength) and PI (645/20 nm emission wavelength).
Results
Profile Hidden Markov Model Searches Identify Potential Bacteroidetal PETases
Protein sequences from both genomes and metagenomes were screened using the previously described Hidden Markov Model (HMM) (Danso et al., 2018) to enrich the diversity of PET-active enzymes from Bacteroidetes. The global searches were performed in publicly available datasets of NCBI GenBank and additionally in several private datasets harboring human-associated and environmental Bacteroidetes sequences (Table 1). Searches were conducted from January until March 2019. This global search initially resulted in the identification of 37 potential PETase sequences from Bacteroidetes with a bit score above 298.7. After sequence comparison, nine distinct hits were chosen. These candidates belonged to bacteroidetal genomes originating from either Seaweed (Li et al., 2017), Antarctic moss (Li et al., 2017), river sediment (Woyke et al., 2011), an aquaculture (own unpublished dataset) or human gut microbiomes (Mitchell et al., 2019; Almeida et al., 2021; Table 1). Most of these candidates were affiliated with the Flavobacteriaceae genus Aequorivita sp. (PET27-29, PET31 and PET53). PET29 and PET31 were highly similar on amino acid level (< 98% identity) but differed in the length of their sequence by 10 amino acids (aa). PET30, annotated as a potential lipase, was derived from the published genome sequence of Kaistella jeonii NCTC 13459. The predicted PETases PET57-59 were derived from bacteria affiliated with the genus Porphyromonas sp. (Porphyromonadaceae), while the predicted enzyme PET38 was derived from the species Fluviicola taffensis (Cryomorphaceae).
Recombinant PET27 and PET30 Hydrolyze Polycaprolactone, Impranil®-DLN and Polyethylene Terephthalate Foil
The nine candidate genes of the predicted PETases were synthesized, cloned into the expression vector pET21a(+) (Biomatik, Wilmington, DA, United States) and transformed in E. coli BL21 and T7-Shuffle cells (Supplementary Table 1). Initial tests using recombinant purified proteins and tributyrin (TBT)-containing agar plates indicated that the genes PET27-30 coded for active esterases. The remaining enzymes PET38, PET53, PET57, and PET58 were inactive and were either produced as insoluble proteins and/or only at very low amounts (Table 1). Because of these obvious difficulties facing their expression, these four predicted enzymes were not further characterized. Additional tests with PET27-PET30 indicated that these enzymes hydrolyzed the esters pNP-hexanoate (C6), and pNP-decanoate (C10, Table 1). All four recombinant enzymes were able to hydrolyze the polymeric polycaprolactone (PCL), the PET-constituent BHET, and the polyester polyurethane Impranil® DLN (Covestro AG, Leverkusen, Germany) (Table 1 and Figure 2A). The enzymes produced clear halos on agar plates containing these substrates after overnight incubation at 30°C (Figure 2A and Table 1). PET-hydrolyzing activities were confirmed for the enzymes PET27 and PET30 on amorphous PET foil as substrate in a 200 μl reaction volume by UHPLC analyses. In these tests, 1 mg ml–1 PET27 released 871.8 ± 200.4 μM (corresponds to 174.4 ± 40.0 nmol in 200 μl reaction volume) of TPA in 120 h at 30°C from a 7 mg PET platelet. PET foil (7 mg) corresponds to 36.4 μmol of a TPA-EG monomer unit (Figure 2B and Table 2). Surprisingly, under the same conditions, PET30 released only 15.9 ± 9.5 μM TPA (corresponds to 3.2 ± 1.9 nmol; Figure 2C and Table 2). These results were directly benchmarked with recombinant IsPETase, of which 1 mg ml–1 released under the same conditions 4,055.7 ± 516.9 μM of TPA (corresponds to 811.1 ± 103.4 nmol). Thus, IsPETase is 4.7-fold more active compared to PET27 and approximately 253-fold more active compared to PET30. Because of the relatively low turnover rates observed for PET27 and PET30 on PET foil, it can be assumed that PET is not the preferred substrate of both enzymes.
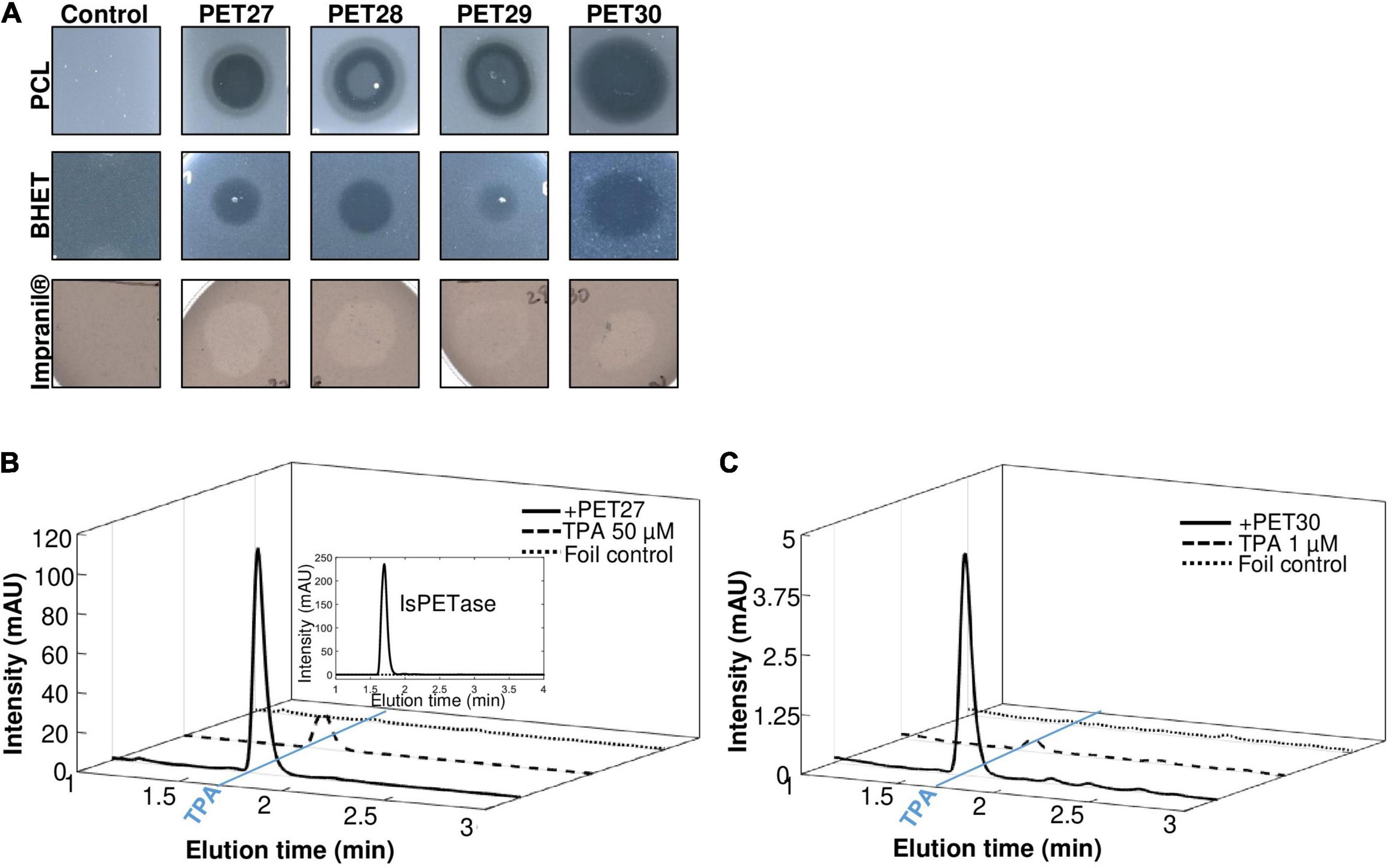
Figure 2. Hydrolytic activities of PET27-PET30 on PCL, BHET, Impranil® DLN and PET foil. Activities on PCL, BHET and Impranil® DLN were visible by halo-formation on agar plates (A). 10 μl of purified enzyme (10-100 μg) were applied to agar plates containing either 500 mg/l PCL, 5 mM BHET or 1% Impranil® DLN. Clearing zones were observed after 12 h at 30°C. Control indicates plates without enzymes but treated with 10 μl buffer. UHPLC profiles of PET27 (B) and PET30 (C) after incubation on PET foil for 120 h. Two hundred microliter of recombinant and purified enzymes (1 mg/ml) were applied to amorphous foil and incubated over 120 h at 30°C. The IsPETase was included for reasons of benchmarking in (B) at 1 mg/assay (upper right corner). Graphs shown are representative graphs and were repeated at least three times.
Biochemical Characterization and Activity of PET27 and PET30 on Esterase Substrates
Both recombinant enzymes were characterized in more detail with pNP-esters. A substrate spectrum was recorded with pNP-esters, which had acyl chain lengths of 4–18 C-atoms. PET27 and PET30 revealed a relatively narrow spectrum of substrates they could hydrolyze. The highest activities were observed with pNP-hexanoate (-C6) for PET30 and pNP-octanoate (-C8) for PET27 (Figure 3A). The optimal temperature of PET30 is 30°C, but 80% activity was observed at 20°C and between 40 and 50°C (Figure 3B). In contrast to that, PET27 shows a better activity at higher temperatures with an optimum at 40°C and even 45% activity at 90°C. Surprisingly, at 10°C, both enzymes still showed a relative activity of 65% (PET30) and 73% (PET27). PET30 remained active at 4°C showing a relative activity of 42% on pNP-C6. Concerning the optimal pH, PET27 was most active between pH 7–8 and PET30 between pH 6-8 when tested in 0.1 M potassium phosphate (Figure 3C).
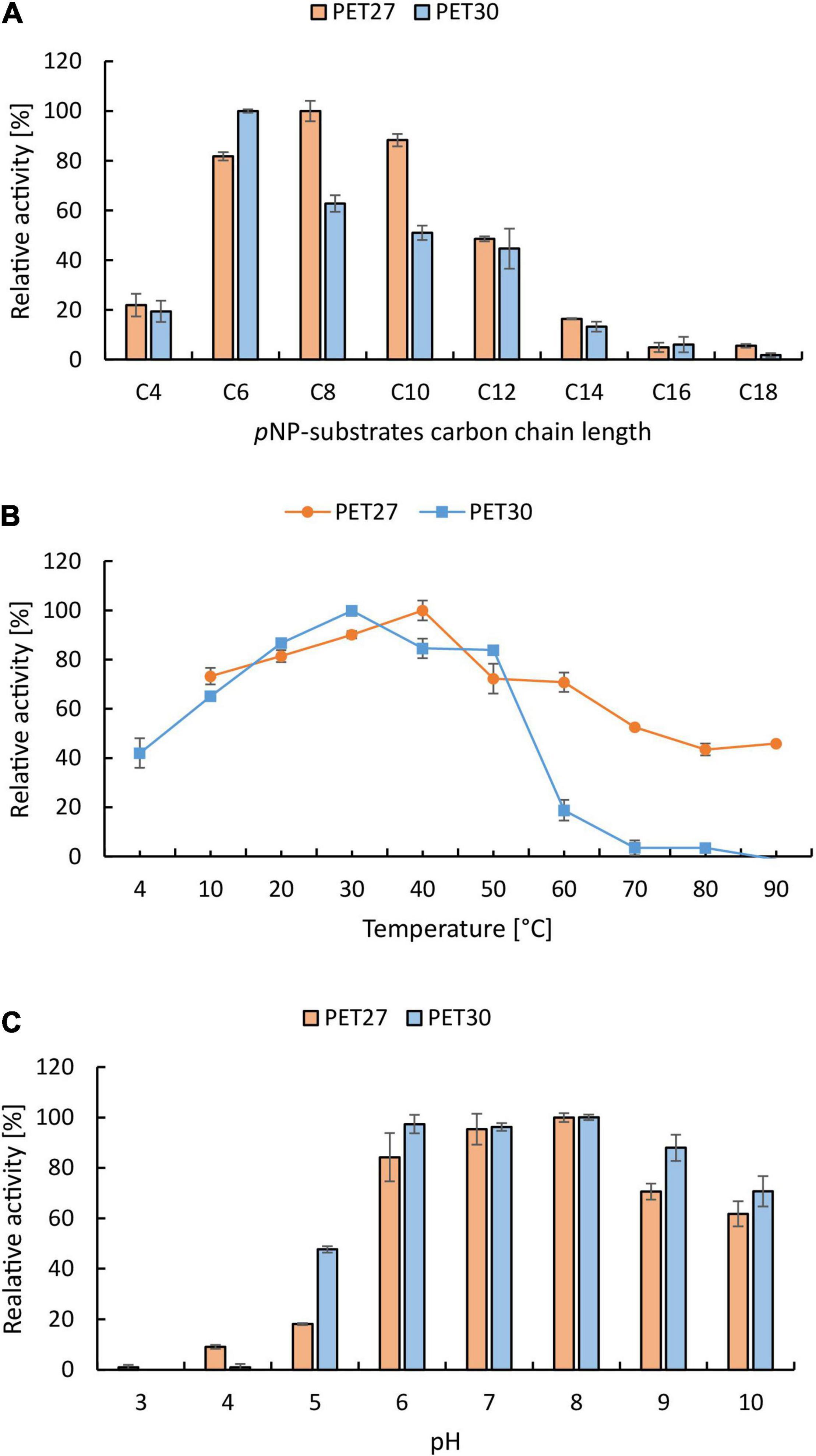
Figure 3. Biochemical characterization of PET27 and PET30 using pNP-substrates. Data represent mean values of at least three independent samples. Substrate preference (A) was tested with pNP-butyrate (-C4) to –stearate (-C18). Temperatures (B) and pH (C) were tested with pNP-octanoate (-C8) for PET27 and with pNP-hexanoate (-C6) for PET30. All assays except B were conducted at 40°C for PET27 and at 30°C for PET30. Both enzymes have their highest activity at cold to moderate temperatures (B) and at slightly acidic to alkaline pH (C).
To further characterize the effects of metal ions, different ions (Ca2+, Co2+, Cu2+, Fe3+, Mg2+, Mn2+, Ni2+, and Zn2+) were added to the assays at 1 and 10 mM final concentrations. Metal ions have a minor influence on PET27. Addition of Ca2+ resulted in a 1.4-fold increase of the activity (Supplementary Figure 1A). In case of PET30, addition of Zn2+, Ni2+, and Co2+ resulted in an up to threefold increase of activity.
The kinetic parameters for PET27 and PET30 were determined with pNP-C6 at 30°C and pH 8 according to Michaelis-Menten. Thereby, PET27 revealed a vmax of 4.9 nmol min–1, a kcat of 19.08 s–1, a Km of 1.37 mM and a kcat/Km value of 13,859.27 M–1 s–1. For PET30, we calculated a vmax of 2.3 nmol min–1, a kcat of 8.9 s–1, a Km of 0.3 mM and a kcat/Km value of 26,136.11 M–1 s–1.
Further, PET30 was investigated in more detail. To assess thermostability, the enzyme was incubated at 50 and 60°C for 3 h, after which the enzyme retained only 23 and 5% of its original activity, respectively (Supplementary Figure 1B). As inhibiting substances, EDTA, DTT and PMSF were applied in final concentrations of 1 and 10 mM (Supplementary Figure 1C). The presence of DTT and PMSF (1 and 10 mM) inactivated PET30 almost completely, whereas EDTA at 1 and 10 mM had no large impact on the enzyme’s activity. A concentration of 1 and 5 % of the detergents Triton X-100, Tween 80 and SDS decreased PET30’s activities (Supplementary Figure 1D).
As both enzymes were active at lower temperatures, PET foil degradation was assayed at 4°C. Over a time of 30 days in a 200 μl reaction volume, TPA release was measured. Under these conditions, 1 mg ml–1 of PET30 released an average of 6.1 μM of TPA (corresponds to 1.2 nmol). Interestingly, IsPETase released under the same conditions a similar amount (5.9 μM TPA corresponds to 1.2 nmol). Notably, under these conditions, no detectable amounts of TPA were released with PET27 after 30 days.
Amino Acid Sequence and Structural Analyses Identify Unique Traits of Bacteroidetal Polyethylene Terephthalate-Hydrolyzing Enzymes
While all four enzymes PET27-PET30 were able to hydrolyze PCL, BHET and Impranil® DLN, only PET27 and PET30 were able to depolymerize PET. To identify the key differences that confer this activity on PET, all predicted PETases were studied on sequence and structural level. With an average of 330 aa, the predicted molecular weights of the enzymes ranged from 36 to 48 kDa. Each candidate contained a C-terminal signal domain for protein transport to the periplasm as predicted with SignalP 5.0 (Almagro Armenteros et al., 2019), supporting the notion that these are secreted proteins (Table 3). Remarkably, the predicted PETases PET27-PET30 and PET38 showed a type IX secretion system (T9SS)/PorC-type sorting domain-containing part at the C-terminus. It has been described earlier by a profile HMM from the TIGRFAM database (TIGR04183). T9SS sorting domains are involved in protein transport across the bacterial outer membrane and have so far been described as a Bacteroidetes-specific secretion system (Sato et al., 2010; Shoji et al., 2011; de Diego et al., 2016). The predicted domain encompassed 62–64 aa in the cases of PET27-PET30 and PET38. PET57 and 58 carried truncated sorting domains of 42 and 55 aa in length. This observation also implies that these enzymes are most likely exoenzymes (Table 3 and Supplementary Figures 3, 4). To ensure that this C-terminus does not affect catalytic activity, a deletion mutant designated PET30ΔPorC was created that lacked the sorting sequence between the amino acids 300–366. Activity tests confirmed that it was not affected in its activities using pNP-C6 or PET foil (Supplementary Figure 1E and Table 2). The enzyme released similar amounts of TPA as it was observed for the native PET30 (Table 2).
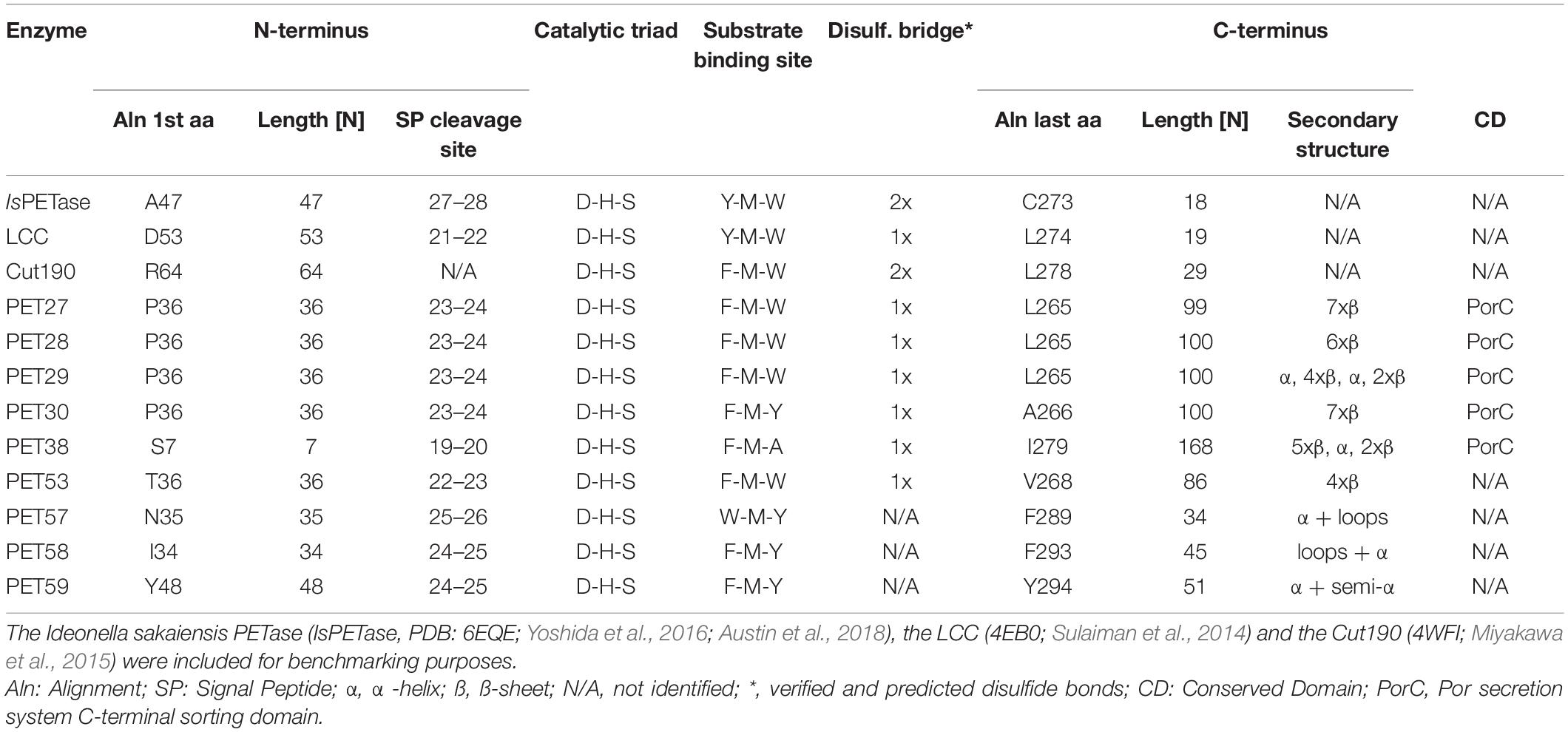
Table 3. Conserved motifs and structural features identified in the predicted bacteroidetal PET-hydrolyzing esterases.
Further analyses of the amino acid sequences identified a G-x-S-x-G motif which is typical for α/β serine hydrolases (Ollis et al., 1992) and a catalytic triad that consists of the residues Asp-His-Ser (Figure 4 and Supplementary Figure 4). Potential substrate binding sites in all bacteroidetal enzymes were identified containing the aa Phe-Met-(Trp/Tyr/Ala). The latter differed from the known IsPETase, the LCC and PET2 binding sites, in which a Tyr-Met-Trp motif is present (Table 3). PET57 is the only exception with a Trp-Met-Tyr binding site. PET27, however, has the Tyr replaced with a Phe that is identical to Cut190, while PET30 has in addition the Trp in position 3 replaced with a Tyr (Table 3). This single amino acid substitution in the predicted substrate binding pocket of PET30, however, is not solely responsible for PET-degrading activity. The mutants PET30_F80Y and PET30_Y178W as well as a version containing both of these point mutations even lost BHET- and PET-degrading activity (data can be shown upon request).
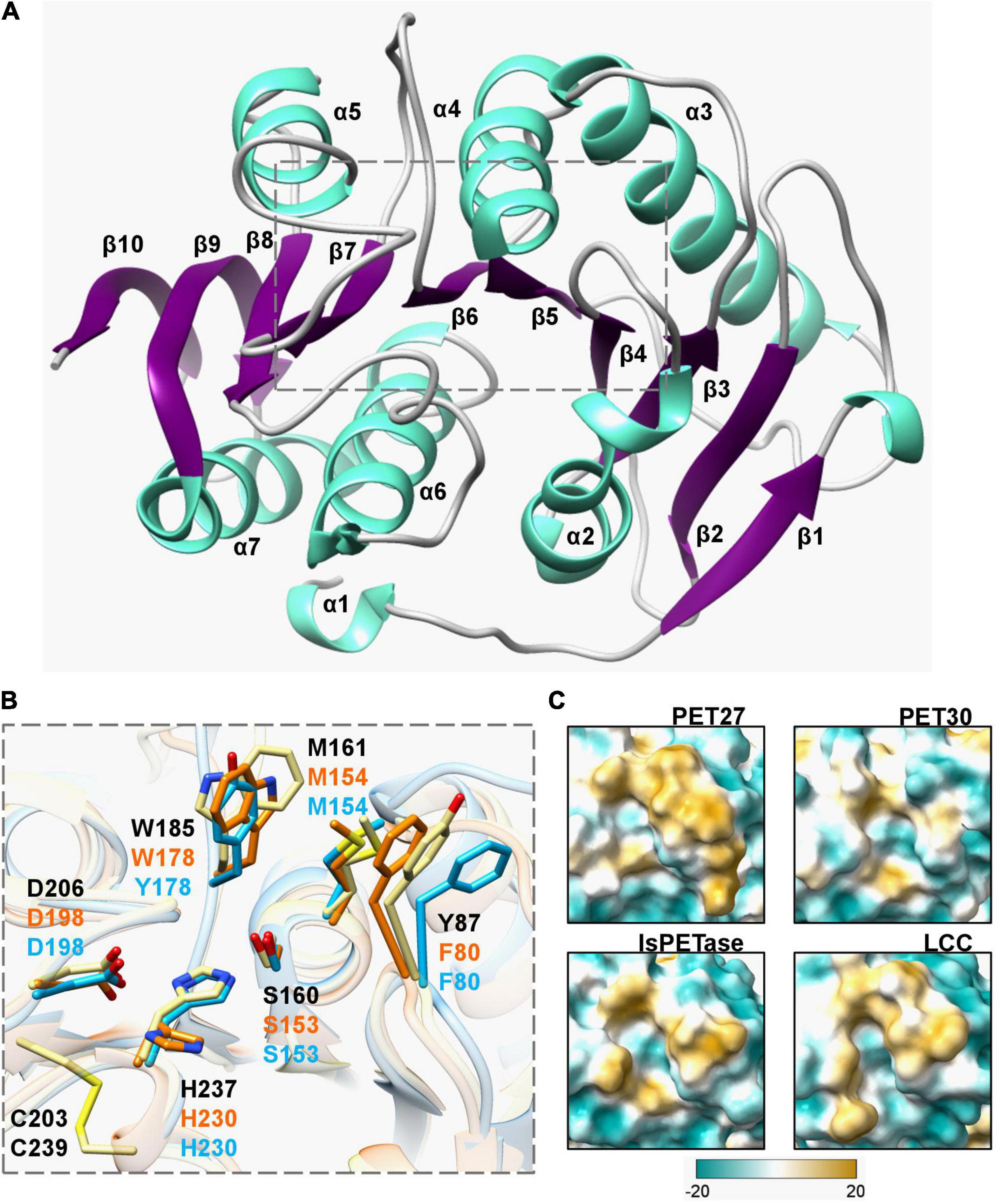
Figure 4. Crystal structure of the PET-hydrolyzing bacteroidetal PET30ΔPorC including active site and hydrophobicity comparison. (A) Overall structure of PET30ΔPorC. The structure was solved by X-ray crystallography to a resolution of 2.1 Å and is shown as cartoon representation. Helices are colored in light turquoise and the beta-sheets are shown in purple. The number of the secondary structure elements are numbered according to the occurrence in the sequence. (B) Comparison of active site residues. All three enzymes PET30ΔPorC (light blue), PET27 (orange) and IsPETase (light yellow) have the typical residues of Ser-hydrolases at the catalytically active positions (Ser, His, and Asp), but PET27 and PET30ΔPorC differ in some of the amino acids associated with PET-binding. The residues of IsPETase are indicated in black. PET30 and PET27 lack a disulfide bridge in the proximity of a catalytic loop. Supplementary Figure 4 provides the positions of these residues in details on the amino acids level. The 3D structure of PET27 was modeled using the Robetta server (Kim et al., 2004) using the IsPETase crystal structure (6EQE) as a backbone. (C) Surface hydrophobicity around the tunnel leading to the active site of four PET-degrading enzymes. Hydrophilic regions are displayed in turquoise and hydrophobic in gold.
Structure Determination of PET30 and Structural Modeling of the Other Putative PETases
For structural insights into the mechanism of polymer degradation, the structures of all predicted PETases, except PET30, were modeled using the IsPETase (PDB code 6EQE) as template. Crystallization was conducted with purified PET30ΔPorC and X-ray structure determination using a model of PET30 for molecular replacement. The structure was solved at 2.1 Å resolution, containing one monomer in the asymmetric unit, with 16.2% for Rwork and 21.9% for Rfree. Data collection and structure refinement statistics are given in Supplementary Table 4. Coordinates of PET30ΔPorC were deposited under the PDB accession code 7PZJ. The PET30ΔPorC protein shows a canonical α/β-fold consisting of a central twisted β-sheet composed of 9 β-strands flanked by 7 α-helices on both sides (Figure 4A), as already reported for homologous structures, i.e., PETases and cutinases (Han et al., 2017; Numoto et al., 2018). The structure of PET30ΔPorC revealed an extra β-sheet (β10) which is located at the C-terminus and connects the PorC domain which has been deleted in this construct. PET30ΔPorC represents the overall fold of several PETases as revealed by a similarity search performed by PDBeFold.8 Here, especially the known structures of the PETase from Ideonella sakaiensis (for example PDB code 5XH3; Han et al., 2017), show high consistency indicated by the rmsd of 1.3-1.5 Å. Also, high structural similarity can be seen with the structure of PE-H from Pseudomonas aestusnigri (PDB code 6SCD rmsd 1.3) and the cutinase from a member of the Burkhoderiales bacteria family (PDB code 7CWQ, rmsd of 1.32 (Bollinger et al., 2020; Chen et al., 2021). One disulfide bond is present in PET30ΔPorC (C262-C285), a common feature for Type II PET-degrading enzymes (Joo et al., 2018). In the PETase from I. sakaiensis (PDB code 5YNS), a second disulfide bond is present which is located closely to the active site. Mutational analysis revealed that this disulfide bond plays a crucial role in activity since mutation of the involved cysteine to serine completely abolished activity, likely due to a destabilizing effect on the active site. The sequence in PET30ΔPorC at this position deviates, and here, G195 and V232 are present. In the PE-H structure, also no disulfide bridge can be found, although one cysteine residue remained (G195 and C251). From the many structures of the PETase from I. sakaiensis, one was solved in complex with 2-hydroxyethyl methyl terephthalate (HEMT; PDB code 5XH3). Here, HEMT is bound via interactions with W156, I179, H208, A131, W130, Y58, and M132. We looked into the active site and overlaid the HEMT molecule with our PET30ΔPorC structure (Supplementary Figure 2). Here similar interactions of the HEMT molecule by the PET30ΔPorC protein can be deduced mediated by Y178, T200, H230, W152, F80, and M154 (Figure 4B).
The largest differences can be seen in the C-terminal part affiliated with the T9SS-domain. It differed largely from the IsPETase and consisted of up to seven predicted β-sheets and, occasionally, a few α-helices (Supplementary Figure 3A and Table 3). Another difference between PET27, PET30, IsPETase and LCC is the surface hydrophobicity of the region channeling the substrates to the active site (Figure 4C). PET30 shows a less hydrophobic surrounding of the catalytic pocket when compared to the structures of Type I and Type II PET-degrading enzymes (Joo et al., 2018). PET27 contains a bulkier hydrophobic domain on one of the sides of the channel. This feature might influence accommodation of the substrate and activity on the polymers. A similar catalytic pocket was predicted for PET28 and PET29 (Supplementary Figure 3). Although active on BHET and PCL, these enzymes showed no measurable activity on PET. The enzymes derived from the human gut present a larger hydrophobic surface around the catalytic site, especially PET58 and PET59 (Supplementary Figure 3B).
Bacteroidetal Polyethylene Terephthalate-Degrading Esterases Forming Two Phylogenetic Subclusters Are Globally Occurring Enzymes
Using the amino acid sequences of published and functionally verified PETases and employing the RAxML-NG autoMRE algorithm via TreeSAPP (Morgan-Lang et al., 2020), aphylogenetic analysis was performed. Multiple phylogenetic clusters formed that roughly corresponded to Actinobacteria, Proteobacteria, Firmicutes and Ascomycota (Figure 5A). While the putative and now confirmed Bacteroidetal PET-degrading hydrolases appear to be polyphyletic when added into the tree, distinct subclusters were formed. Notably, the two enzymes PET27 and PET30, shown to be active on PET foil, were grouped as part of subcluster that consisted of predicted and functional enzymes affiliated with genera Aequorivita and Kaistella. Furthermore, the predicted but functionally not verified enzymes from the genus Porphyromonas (PET57-PET59) formed a separate subcluster. Interestingly, these two subclusters harbored only sequences of aquatic and environmental origin orgut-affiliated sequences, respectively. Overall, sequences derived from Bacteroidetes seem to group by both taxonomy and environment, though low bootstrap values do not show a high degree of confidence. However, the interleaving of Bacteroidetal sequences from both a Firmicute, B. subtilis, and the eukaryotic Ascomycota sequences may suggest that PET-active enzymes are widely distributed phylogenetically, and further characterization studies resulting in additions to the tree are likely to provide better phylogenetic resolution. Additionally, the pairwise distance on the level computed in MEGAX with the p-distance model (Figure 5B) confirmed these groupings, with the highest similarity, as indicated by low pairwise distanced, occurring within the subclusters described. This analysis also indicated rather low similarity between the putative bacteroidetal PETases and the known PETases (IsPETase, LCC, PE-H, and PET2), mounting further evidence for the wide phylogenetic distribution of these enzymes.
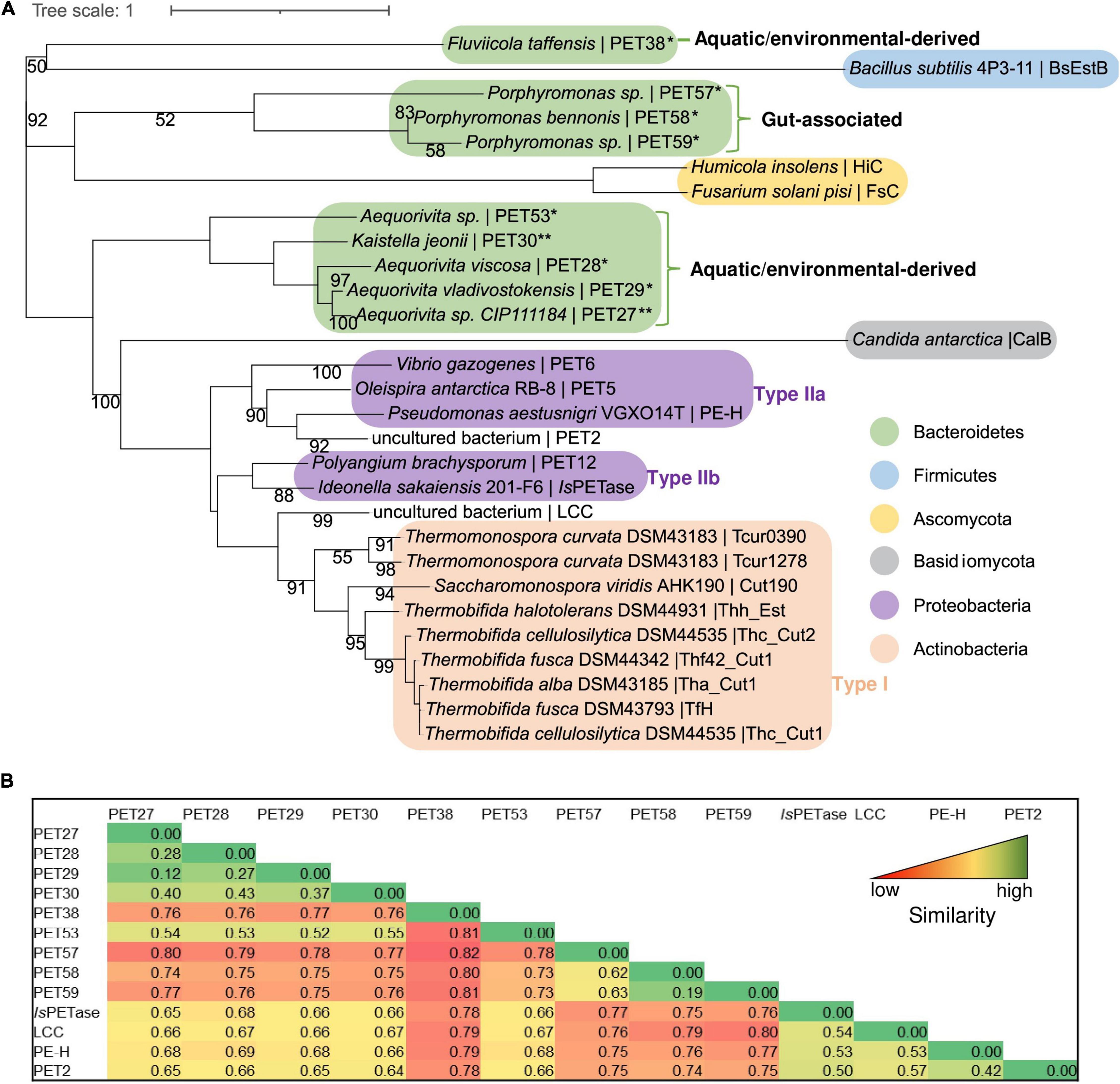
Figure 5. Phylogenetic tree and pairwise comparison of known PETases and bacteroidetal PET-hydrolyzing enzymes. (A) The tree was constructed using the RAxML-NG autoMRE algorithm (Kozlov et al., 2019) with the treesapp create command implemented in TreeSAPP (Morgan-Lang et al., 2020) with maximum bootstraps set at 1,000. GenBank entries of the putative and verified PET-active enzymes affiliated with the Bacteroidetes phylum are listed in Table 1; the entries of all other PETases included in the tree are indicated in Supplementary Table 3. The term “uncultured bacterium” refers to a not further specified microorganism derived from a metagenome or a mixed microbial consortium. (B) Heatmap representation of pairwise similarities between all enzymes affiliated with the Bacteroidetes phylum in comparison with four known PETases (IsPETase, LCC, PE-H, and PET2). The pairwise comparison was performed in MEGAX with the p-distance model. High values indicating low similarity are colored in red, low values indicating high similarity are colored in green.
The diversity of bacteroidetal enzymes acting on PET raised the question to what extent these enzymes could impact plastic degradation in the environment. To address this question in part, we analyzed the global distribution of PET27 and PET30 and their homologs. The protein sequences of PET27 and PET30 were analyzed for their occurrence and frequency in global databases available in IMG/M ER (Woyke et al., 2011; Mukherjee et al., 2020). Using both enzymes for a BLASTp-based search (cutoffs 50% identity; 80% coverage), we were initially able to identify very few (<10) possible homologs in the global databases analyzed and affiliated with the genera Aequorivita and Kaistella (Figure 1A). Interestingly, when we extended our search to the Flavobacterium-Cytophaga-Bacteroidetes (FCB), we were able to identify 98 possible homologs in our global searches including single-cell amplified genomes (SAGs) from the Baltic Sea (Supplementary Table 5). 47 hits were affiliated with the genus of the Marinimicrobia (candidate phylum of the FCB group), indicating a potential role for these ubiquitous and abundant marine microorganisms in PET-degradation. Others were more closely associated with Bacteroidetes (Austin et al., 2018). As expected, the majority of these homologs were associated with marine and aquatic samples (Figure 1B).
Kaistella jeonii Is Able to Colonize Polyethylene Terephthalate Surfaces
These metagenomic analyses showed that K. jeonii, the organism from which PET30 originates, exists primarily in aquatic habitats. To illustrate that it not only exists there, but is also able to actively colonize PET surfaces, a culture of this organism was incubated with PET foil platelets. The foil sections were incubated for up to 1 week in R2A medium with K. jeonii, removed from the culture, and washed three times with buffer before staining with LIVE/DEAD staining solution. Samples were investigated under the confocal laser scanning microscope Axio Observer Z1/7, LSM 800 by Carl Zeiss Microscopy GmbH (Jena, Germany). Cells visible in Supplementary Figure 5 resisted repeated washing, indicating that they adhere under these laboratory conditions to the surface and an increasing number of cells was visible on the amorphous PET-foil after 5–7 days. The exact mechanism of attachment is not known. Nonetheless, it can be speculated that SusD binding modules, which are crucial for sugar polymer binding, could be involved. They can be found in both genomes of K. jeonii (GenBank acc. no. WP_039349586.1; RagB/SusD family nutrient uptake outer membrane protein) and Aequorivita sp. CIP111184 (GenBank acc. no. WP_111879847.1; SusD/RagB family nutrient-binding outer membrane lipoprotein).
Discussion
Currently, only a handful of known bacterial phyla are known to produce active PET-esterases (Figure 5 and Supplementary Table 3). Here, we have identified and partially characterized two novel functional PET-hydrolyzing enzymes affiliated with the Kaistella and Aequorivita genera within the Bacteroidetes phylum. Bacteria belonging to the genus Kaistella are globally occurring aerobic organisms colonizing a wide range of different habitats including plants, soil, fish, the human gut, and sea water. Within the genus Kaistella, over one hundred species have been described of which few are pathogens, but many are beneficial and host-associated (Bernardet et al., 2005; Loch and Faisal, 2015). Only a few species have been identified within the genus Aequorivita, mainly belonging to marine or fresh-water organisms that are mostly psychrotolerant and aerobic (Bowman and Nichols, 2002). Notably, Bacteroidetes have been described as very potent degraders of polymers, and they harbor a multitude of hydrolases and binding modules (Dodd et al., 2011; Thomas et al., 2011; Foley et al., 2016).
The enzymes PET27 and PET30 characterized here are both typical esterases (i.e., serine hydrolases) belonging to the EC 3.1. Both appear to be secreted enzymes as they carry an C-terminal secretion signal linked to the transport into the periplasm (Desvaux et al., 2009) and one secretion PorC-like motif which is related to the type IX secretion system (T9SS) (Sato et al., 2010; de Diego et al., 2016). The T9SS is composed of several outer membrane, periplasmic and inner membrane proteins, whereby it is responsible for the secretion of pathogenicity factors, hydrolases and also for gliding motility in the Bacteroidetes phylum (Sato et al., 2010; de Diego et al., 2016).
PET27 and PET30 were active on PET foil, but differed strongly in their overall activities. PET27 contains a Phe-Met-Trp motif and PET30 a Phe-Met-Tyr. The most active enzymes such as LCC and IsPETase both carry a Tyr-Met-Trp consensus binding motif while the non-active enzymes PET38, PET53, PET57 PET58 and PET59 revealed either a Phe-Met-Ala, a Phe-Met-Trp or a Trp-Met-Tyr substrate binding motif. PET30 mutants, in which the amino acids were adjusted according to the active PETases, did not show the expected increase in activity. Therefore, we assume that not only individual amino acids are decisive, but rather that an interplay of hydrophobicity, location and accessibility of the catalytic triad is crucial for whether polymers can be degraded (Figure 4).
Benchmarking activities of polymer active enzymes with literature values is not trivial since most studies use different types of foils with different degrees of crystallinity and distinct assay conditions. To partially overcome this challenge, we produced our own recombinant wildtype enzymes of the IsPETase and compared its activities with PET27 and PET30. As expected, IsPETase was 4.7-fold more active at 30°C than PET27 and up to 253-fold more active than PET30. With respect to the overall activity of the IsPETase, however, our data are in line with published data for this enzyme (Son et al., 2019). The observation here that the activities of the PET27 and PET30 enzymes are relatively low compared to the IsPETase and certainly with respect to the published values of the even more active LCC imply that PET27 and PET30 are not PET esterases in sensu strictu. However, our data imply that both are short-chain fatty acid acting esterases revealing promiscuity in their substrate profile (Table 3 and Figure 3).
Intriguingly, the observation that both enzymes were catalytically active on PET foil could imply a wider role in the degradation of PET and especially PET nanoparticles. Because of the significant activities even at 4°C, these enzymes may in fact play a yet unknown role in long-term degradation of PET microparticles in cold environments. This hypothesis is supported by our observations that homologs of both enzymes can be found on a global level covering a wide range of climate zones (Figure 1) and that at least the cultivable K. jeonii is able to attach to PET surfaces.
In summary, our biochemical results significantly extend the knowledge of PET-degrading enzymes and provide promising candidates for biotechnological applications at low temperatures. Furthermore, the data presented here will help to advance our knowledge on the ecological role of the Bacteroidetes in the decomposition of marine PET litter and enable the development of an expanded phylogenetic framework for identifying the diversity of putative PETases in diverse marine microbial groups throughout the global ocean.
Data Availability Statement
The original contributions presented in the study are included in the article/Supplementary Material, further inquiries can be directed to the corresponding author/s.
Author Contributions
WS, JC, and PP-G designed the study, contributed to manuscript writing and designing bioassays. HZ and RD conducted experiments and contributed to planning and writing. PP-G, JC, and HZ were involved in enzyme structural work, bioinformatics, and initial phylogenetic analyses. VA, JS, and SSm conducted crystallization and structure determination. CS and JP contributed to planning and corrections. RS and CC were involved in global data base searches. DD performed HMM searches. SW and BH delivered clone producing the IsPETase. LP did microscopic analyses. AA was involved in gut microbiome data mining. SSt and SH were involved in phylogenetic analysis. All authors contributed to manuscript writing and editing.
Funding
This work was in part supported by the BMBF within the programs MarBiotech (031B0562A) MetagenLig (031B0571B), MethanoPEP (031B0851B), LipoBiocat (031B0837B), PlastiSea (031B867B), and MetagenLig (031B0571A) at the Universities of Hamburg, Kiel, Düsseldorf and Stuttgart. The support for the University of Bayreuth was given by the Deutsche Forschungsgemeinschaft (DFG, German Research Foundation)—Project Number 391977956—SFB 1357. Further funding came from the US Department of Energy (DOE) Joint Genome Institute, an Office of Science User Facility, supported by the Office of Science of the U.S. Department of Energy under Contract DE-AC02-05CH11231, the Natural Sciences and Engineering Research Council (NSERC) of Canada, the G. Unger Vetlesen and Ambrose Monell Foundations, the Canada Foundation for Innovation (CFI) and Compute Canada through grants awarded to SH. AA was funded by EMBL core funds. The Center for Structural Studies was funded by the Deutsche Forschungsgemeinschaft (DFG Grant number 417919780; INST 208/740-1 FUGG; INST 208/761-1 FUGG).
Conflict of Interest
SH is a co-founder of Koonkie Inc., a bioinformatics consulting company that designs and provides scalable algorithmic and data analytics solutions in the cloud. The remaining authors declare that the research was conducted in the absence of any commercial or financial relationships that could be construed as a potential conflict of interest.
Publisher’s Note
All claims expressed in this article are solely those of the authors and do not necessarily represent those of their affiliated organizations, or those of the publisher, the editors and the reviewers. Any product that may be evaluated in this article, or claim that may be made by its manufacturer, is not guaranteed or endorsed by the publisher.
Acknowledgments
The synchrotron MX data were collected at beamline P13 operated by EMBL Hamburg at the PETRA III storage ring (DESY, Hamburg, Germany). We thank Dr. Michael Agthe for the assistance in using the beamline. We also thank the staff of the ID23-eh1 at the ESRF, Grenoble for help with initial screening of the crystal quality.
Supplementary Material
The Supplementary Material for this article can be found online at: https://www.frontiersin.org/articles/10.3389/fmicb.2021.803896/full#supplementary-material
Supplementary Figure 1 | Further biochemical characterization of PET27 and PET30 using pNP-substrates. Cofactor requirements were tested for PET27 with pNP-C8 at 40°C and for PET30 with pNP-C6 at 30°C (A). For PET30, all other tests were carried out with pNP-C6 and all assays except (B) were conducted at 30°C. Thermostability of PET30 was assessed at 50 and 60°C over 3 h with pNP-C6 (B). Inhibitors (C) and detergents (D) generally decrease the activity of PET30, particularly with higher concentrations. Activity of PET30 and PET30ΔPorC were compared under the same conditions using pNP-C6 (E). Data represent mean values of at least three independent measurements.
Supplementary Figure 2 | Zoom into the active site of PET30ΔPorC. Overlaid are the structures of PET30ΔPorC (blue) with the PETase from I. sakaiensis (light yellow, black labeling) in complex with HEMT (purple; PDB code 5XH3). The residues involved in binding are highlighted and numbered according to their structure. *The structure 5XH3 is a mutant where the catalytic Serine was mutated to an Alanine.
Supplementary Figure 3 | Structure prediction models of verified and predicted PETases affiliated with the phylum of the Bacteroidetes. (A) 3D structures were modeled using the Robetta server using the IsPETase crystal structure (light yellow, 6EQE) as a backbone. For PET30, the crystal structure was shown. (B) Surface hydrophobicity around the tunnel leading to the active site of putative bacteroidetal PETases and functionally verified PET-degrading enzymes. Hydrophilic regions are displayed in turquoise and hydrophobic in gold.
Supplementary Figure 4 | Amino acid alignment of 9 potential PETases affiliated with the Bacteroidetes phylum. The original sequences were used for the structural alignment and the alignment was constructed with T-Coffee. Alignment was visualized with Bioedit version 7.0.5. The IsPETase was included for reasons of benchmarking. Blue arrows indicate the start and the end of the active PET27 and PET30 clones. We introduced a methionine as the first aa of the protein sequences. The signal peptide deleted version of the enzymes was functionally verified. The red arrow labeled with CS indicates the predicted cleavage site of C-terminal PorC domain (Lasica et al., 2017). The gray arrow indicates the C-terminus of the truncated version of PET30 (PET30Δ300-366), number indicates the position of amino acid). PET30Δ300-366 was active on PCL and BHET.
Supplementary Figure 5 | Confocal microscopic pictures of K. jeonii colonizing PET foil. The pictures were taken after 5–7 days of incubation of K. jeonii in R2A medium. Cells are dyed with LIVE/DEAD™ stain. Green fluorescence shows living cells, red fluorescence indicates dead cells. 2D front pictures were taken with Axio Observer Z1/7, LSM 800 (Carl Zeiss, Jena, Germany) of a 3D Z-stack image.
Footnotes
- ^ https://www.ncbi.nlm.nih.gov/
- ^ http://www.uniprot.org/
- ^ http://jgi.doe.gov/
- ^ http://hmmer.org
- ^ https://geohack.toolforge.org
- ^ http://www.qgis.org
- ^ www.cgl.ucsf.edu/chimera
- ^ www.ebi.ac.uk/msd-srv/ssm/
References
Acero, E. H., Ribitsch, D., Steinkellner, G., Gruber, K., Greimel, K., Eiteljoerg, I., et al. (2011). Enzymatic surface hydrolysis of PET: effect of structural diversity on kinetic properties of cutinases from Thermobifida. Macromolecules 44, 4632–4640. doi: 10.1021/ma200949p
Afonine, P. V., Grosse-Kunstleve, R. W., Echols, N., Headd, J. J., Moriarty, N. W., Mustyakimov, M., et al. (2012). Towards automated crystallographic structure refinement with phenix. refine. Acta Crystallogr. D Biol. Crystallogr. 68, 352–367. doi: 10.1107/S0907444912001308
Agarwala, R., Barrett, T., Beck, J., Benson, D. A., Bollin, C., Bolton, E., et al. (2016). Database resources of the national center for biotechnology information. Nucleic Acids Res. 44, D7–D19.
Almagro Armenteros, J. J., Tsirigos, K. D., Sønderby, C. K., Petersen, T. N., Winther, O., Brunak, S., et al. (2019). SignalP 5.0 improves signal peptide predictions using deep neural networks. Nat. Biotechnol. 37, 420–423. doi: 10.1038/s41587-019-0036-z
Almeida, A., Nayfach, S., Boland, M., Strozzi, F., Beracochea, M., Shi, Z. J., et al. (2021). A unified catalog of 204,938 reference genomes from the human gut microbiome. Nat. Biotechnol. 39, 105–114. doi: 10.1038/s41587-020-0603-3
Austin, H. P., Allen, M. D., Donohoe, B. S., Rorrer, N. A., Kearns, F. L., Silveira, R. L., et al. (2018). Characterization and engineering of a plastic-degrading aromatic polyesterase. Proc. Natl. Acad. Sci. U.S.A. 115, E4350–E4357. doi: 10.1073/pnas.1718804115
Berman, H. M., Westbrook, J., Feng, Z., Gilliland, G., Bhat, T. N., and Weissig, H. I., et al. (2000). The protein data bank. Nucleic Acids Res. 28, 235–242.
Bernardet, J. F., Vancanneyt, M., Matte-Tailliez, O., Grisez, L., Tailliez, P., Bizet, C., et al. (2005). Polyphasic study of Chryseobacterium strains isolated from diseased aquatic animals. Syst. Appl. Microbiol. 28, 640–660. doi: 10.1016/j.syapm.2005.03.016
Bollinger, A., Thies, S., Knieps-Grunhagen, E., Gertzen, C., Kobus, S., Hoppner, A., et al. (2020). A novel polyester hydrolase from the marine bacterium Pseudomonas aestusnigri–structural and functional insights. Front. Microbiol. 11:114. doi: 10.3389/fmicb.2020.00114
Bowman, J. P., and Nichols, D. S. (2002). Aequorivita gen. nov., a member of the family Flavobacteriaceae isolated from terrestrial and marine Antarctic habitats. Int. J. Syst. Evol. Microbiol. 52, 1533–1541. doi: 10.1099/00207713-52-5-1533
Chen, C.-C., Han, X., Li, X., Jiang, P., Niu, D., Ma, L., et al. (2021). General features to enhance enzymatic activity of poly (ethylene terephthalate) hydrolysis. Nat. Catal. 4, 425–430. doi: 10.1038/s41929-021-00616-y
Chen, S., Tong, X., Woodard, R. W., Du, G., Wu, J., and Chen, J. (2008). Identification and characterization of bacterial cutinase. J. Biol. Chem. 283, 25854–25862. doi: 10.1074/jbc.m800848200
NCBI Resource Coordinators (2017). Database resources of the national center for biotechnology information. Nucleic Acids Res. 45, D12–D17.
Danso, D., Chow, J., and Streit, W. R. (2019). Plastics: microbial degradation, environmental and biotechnological perspectives. Appl. Environ. Microbiol. 85, 1–14. doi: 10.1007/978-3-030-48973-1_1
Danso, D., Schmeisser, C., Chow, J., Zimmermann, W., Wei, R., Leggewie, C., et al. (2018). New insights into the function and global distribution of polyethylene terephthalate (PET)-degrading bacteria and enzymes in marine and terrestrial metagenomes. Appl. Environ. Microbiol. 84:e02773-17. doi: 10.1128/AEM.02773-17
de Diego, I., Ksiazek, M., Mizgalska, D., Koneru, L., Golik, P., Szmigielski, B., et al. (2016). The outer-membrane export signal of Porphyromonas gingivalis type IX secretion system (T9SS) is a conserved C-terminal β-sandwich domain. Sci. Rep. 6:23123. doi: 10.1038/srep23123
DeLano, W. L. (2002). Pymol: an open-source molecular graphics tool. CCP4 Newslett. Protein Crystallogr. 40, 82–92.
Desvaux, M., Hébraud, M., Talon, R., and Henderson, I. R. (2009). Outer membrane translocation: numerical protein secretion nomenclature in question in mycobacteria. Trends Microbiol. 17, 338–340. doi: 10.1016/j.tim.2009.05.008
Dodd, D., Mackie, R. I., and Cann, I. K. O. (2011). Xylan degradation, a metabolic property shared by rumen and human colonic Bacteroidetes. Mol. Microbiol. 79, 292–304. doi: 10.1111/j.1365-2958.2010.07473.x
Emsley, P., and Cowtan, K. (2004). Coot: model-building tools for molecular graphics. Acta Crystallogr. D Biol. Crystallogr. 60, 2126–2132. doi: 10.1107/s0907444904019158
Foley, M. H., Cockburn, D. W., and Koropatkin, N. M. (2016). The Sus operon: a model system for starch uptake by the human gut Bacteroidetes. Cell. Mol. Life Sci. 73, 2603–2617. doi: 10.1007/s00018-016-2242-x
Geyer, R., Jambeck, J. R., and Law, K. L. (2017). Production, use, and fate of all plastics ever made. Sci. Adv. 3:e1700782. doi: 10.1126/sciadv.1700782
Haernvall, K., Zitzenbacher, S., Amer, H., Zumstein, M. T., Sander, M., McNeill, K., et al. (2017). Polyol structure influences enzymatic hydrolysis of bio-based 2,5-furandicarboxylic acid (FDCA) polyesters. Biotechnol. J. 12:1600741. doi: 10.1002/biot.201600741
Hahnke, R. L., Meier-Kolthoff, J. P., García-López, M., Mukherjee, S., Huntemann, M., Ivanova, N. N., et al. (2016). Genome-based taxonomic classification of bacteroidetes. Front. Microbiol. 7:2003. doi: 10.3389/fmicb.2016.02003
Hall, T. A. (1999). BioEdit: A User-Friendly Biological Sequence Alignment Editor and Analysis Program for Windows 95/98/NT. Nucleic Acids Symposium Series. London: Information Retrieval Ltd., c1979–c2000.
Han, X., Liu, W., Huang, J. W., Ma, J., Zheng, Y., Ko, T. P., et al. (2017). Structural insight into catalytic mechanism of PET hydrolase. Nat. Commun. 8:2106.
Hu, X., Thumarat, U., Zhang, X., Tang, M., and Kawai, F. (2010). Diversity of polyester-degrading bacteria in compost and molecular analysis of a thermoactive esterase from Thermobifida alba AHK119. Appl. Microbiol. Biotechnol. 87, 771–779. doi: 10.1007/s00253-010-2555-x
Jambeck, J. R., Geyer, R., Wilcox, C., Siegler, T. R., Perryman, M., Andrady, A., et al. (2015). Plastic waste inputs from land into the ocean. Science 347, 768–771. doi: 10.1126/science.1260352
Joo, S., Cho, I. J., Seo, H., Son, H. F., Sagong, H. Y., Shin, T. J., et al. (2018). Structural insight into molecular mechanism of poly(ethylene terephthalate) degradation. Nat. Commun. 9:382.
Kabsch, W. (2014). Processing of X-ray snapshots from crystals in random orientations. Acta Crystallogr. D Biol. Crystallogr. 70, 2204–2216. doi: 10.1107/S1399004714013534
Kim, D. E., Chivian, D., and Baker, D. (2004). Protein structure prediction and analysis using the Robetta server. Nucleic Acids Res. 32, W526–W531.
Kleeberg, I., Hetz, C., Kroppenstedt, R. M., Muller, R. J., and Deckwer, W. D. (1998). Biodegradation of aliphatic-aromatic copolyesters by Thermomonospora fusca and other thermophilic compost isolates. Appl. Environ. Microbiol. 64, 1731–1735. doi: 10.1128/AEM.64.5.1731-1735.1998
Kozlov, A. M., Darriba, D., Flouri, T., Morel, B., and Stamatakis, A. (2019). RAxML-NG: a fast, scalable and user-friendly tool for maximum likelihood phylogenetic inference. Bioinformatics 35, 4453–4455. doi: 10.1093/bioinformatics/btz305
Krieg, N., Ludwig, W., Euzéby, J., and Whitman, W. (2015). “Bacteroidetes phyl. nov,” in Bergey’s Manual of Systematics of Archaea and Bacteria, eds M. E. Trujillo, S. Dedysh, P. DeVos, et al. (Hoboken, NJ: John Wiley & Sons, Inc.), 1–2. doi: 10.1002/9781118960608.pbm00004
Lasica, A. M., Ksiazek, M., Madej, M., and Potempa, J. (2017). The type IX secretion system (T9SS): highlights and recent insights into its structure and function. Front. Cell. Infect. Microbiol. 7:215. doi: 10.3389/fcimb.2017.00215
Letunic, I., and Bork, P. (2019). Interactive tree of life (iTOL) v4: recent updates and new developments. Nucleic Acids Res. 47, W256–W259. doi: 10.1093/nar/gkz239
Li, N., Zhu, Y., LaFrentz, B. R., Evenhuis, J. P., Hunnicutt, D. W., Conrad, R. A., et al. (2017). The type IX secretion system is required for virulence of the fish pathogen Flavobacterium columnare. Appl. Environ. Microbiol. 83:e01769-17.
Liebschner, D., Afonine, P. V., Baker, M. L., Bunkóczi, G., Chen, V. B., Croll, T. I., et al. (2019). Macromolecular structure determination using X-rays, neutrons and electrons: recent developments in Phenix. Acta Crystallogr. D Struct. Biol. 75, 861–877. doi: 10.1107/S2059798319011471
Loch, T. P., and Faisal, M. (2015). Emerging flavobacterial infections in fish: a review. J. Adv. Res. 6, 283–300. doi: 10.1016/j.jare.2014.10.009
Markowitz, V. M., Chen, I. M., Palaniappan, K., Chu, K., Szeto, E., Grechkin, Y., et al. (2012). IMG: the integrated microbial genomes database and comparative analysis system. Nucleic Acids Res. 40, D115–D122.
Mistry, J., Finn, R. D., Eddy, S. R., Bateman, A., and Punta, M. (2013). Challenges in homology search: HMMER3 and convergent evolution of coiled-coil regions. Nucleic Acids Res. 41:e121. doi: 10.1093/nar/gkt263
Mitchell, A. L., Almeida, A., Beracochea, M., Boland, M., Burgin, J., Cochrane, G., et al. (2019). MGnify: the microbiome analysis resource in 2020. Nucleic Acids Res. 48, D570–D578. doi: 10.1093/nar/gkz1035
Miyakawa, T., Mizushima, H., Ohtsuka, J., Oda, M., Kawai, F., and Tanokura, M. (2015). Structural basis for the Ca 2+-enhanced thermostability and activity of PET-degrading cutinase-like enzyme from Saccharomonospora viridis AHK190. Appl. Microbiol. Biotechnol. 99, 4297–4307. doi: 10.1007/s00253-014-6272-8
Molitor, R., Bollinger, A., Kubicki, S., Loeschcke, A., Jaeger, K. E., and Thies, S. (2020). Agar plate-based screening methods for the identification of polyester hydrolysis by Pseudomonas species. Microb. Biotechnol. 13, 274–284. doi: 10.1111/1751-7915.13418
Morgan-Lang, C., McLaughlin, R., Armstrong, Z., Zhang, G., Chan, K., and Hallam, S. J. (2020). TreeSAPP: the tree-based sensitive and accurate phylogenetic profiler. Bioinformatics 36, 4706–4713. doi: 10.1093/bioinformatics/btaa588
Mukherjee, S., Stamatis, D., Bertsch, J., Ovchinnikova, G., Sundaramurthi, J. C., Lee, J., et al. (2020). Genomes OnLine Database (GOLD) v.8: overview and updates. Nucleic Acids Res. 49, D723–D733. doi: 10.1093/nar/gkaa983
Mulnaes, D., Porta, N., Clemens, R., Apanasenko, I., Reiners, J., Gremer, L., et al. (2020). TopModel: template-based protein structure prediction at low sequence identity using top-down consensus and deep neural networks. J. Chem. Theory Comput. 16, 1953–1967. doi: 10.1021/acs.jctc.9b00825
Munoz, R., Rosselló-Móra, R., and Amann, R. (2016). Revised phylogeny of Bacteroidetes and proposal of sixteen new taxa and two new combinations including Rhodothermaeota phyl. nov. Syst. Appl. Microbiol. 39, 281–296. doi: 10.1016/j.syapm.2016.04.004
Notredame, C., Higgins, D. G., and Heringa, J. (2000). T-Coffee: a novel method for fast and accurate multiple sequence alignment. J. Mol. Biol. 302, 205–217.
Numoto, N., Kamiya, N., Bekker, G.-J., Yamagami, Y., Inaba, S., Ishii, K., et al. (2018). Structural dynamics of the PET-degrading cutinase-like enzyme from Saccharomonospora viridis AHK190 in substrate-bound states elucidates the Ca2+-driven catalytic cycle. Biochemistry 57, 5289–5300. doi: 10.1021/acs.biochem.8b00624
Ollis, D. L., Cheah, E., Cygler, M., Dijkstra, B., Frolow, F., Franken, S. M., et al. (1992). The alpha/beta hydrolase fold. Protein Eng. 5, 197–211.
Pérez-García, P., Danso, D., Zhang, H., Chow, J., and Streit, W. R. (2021). Exploring the global metagenome for plastic-degrading enzymes. Methods Enzymol. 648, 137–157. doi: 10.1016/bs.mie.2020.12.022
Ribitsch, D., Herrero Acero, E., Greimel, K., Dellacher, A., Zitzenbacher, S., Marold, A., et al. (2012). A new esterase from Thermobifida halotolerans hydrolyses polyethylene terephthalate (PET) and polylactic acid (PLA). Polymers 4, 617–629. doi: 10.3390/polym4010617
Ronkvist, ÅM., Xie, W., Lu, W., and Gross, R. A. (2009). Cutinase-catalyzed hydrolysis of poly(ethylene terephthalate). Macromolecules 42, 5128–5138.
Sato, K., Naito, M., Yukitake, H., Hirakawa, H., Shoji, M., McBride, M. J., et al. (2010). A protein secretion system linked to bacteroidete gliding motility and pathogenesis. Proc. Natl. Acad. Sci. U.S.A. 107, 276–281. doi: 10.1073/pnas.0912010107
Shoji, M., Sato, K., Yukitake, H., Kondo, Y., Narita, Y., Kadowaki, T., et al. (2011). Por secretion system-dependent secretion and glycosylation of Porphyromonas gingivalis hemin-binding protein 35. PLoS One 6:e21372. doi: 10.1371/journal.pone.0021372
Son, H. F., Cho, I. J., Joo, S., Seo, H., Sagong, H.-Y., Choi, S. Y., et al. (2019). Rational protein engineering of thermo-stable PETase from Ideonella sakaiensis for highly efficient PET degradation. ACS Catal. 9, 3519–3526.
Studier, F. W. (2005). Protein production by auto-induction in high-density shaking cultures. Protein Exp. Purif. 41, 207–234.
Sulaiman, S., Yamato, S., Kanaya, E., Kim, J. J., Koga, Y., Takano, K., et al. (2012). Isolation of a novel cutinase homolog with polyethylene terephthalate-degrading activity from leaf-branch compost by using a metagenomic approach. Appl. Environ. Microbiol. 78, 1556–1562. doi: 10.1128/AEM.06725-11
Sulaiman, S., You, D. J., Kanaya, E., Koga, Y., and Kanaya, S. (2014). Crystal structure and thermodynamic and kinetic stability of metagenome-derived LC-cutinase. Biochemistry 53, 1858–1869. doi: 10.1021/bi401561p
The UniProt Consortium (2017). UniProt: the universal protein knowledgebase. Nucleic Acids Res. 45, D158–D169.
Thomas, F., Hehemann, J. H., Rebuffet, E., Czjzek, M., and Michel, G. (2011). Environmental and gut Bacteroidetes: the food connection. Front. Microbiol. 2:16. doi: 10.3389/fmicb.2011.00093
Wei, R., Oeser, T., and Zimmermann, W. (2014). Synthetic polyester-hydrolyzing enzymes from thermophilic actinomycetes. Adv. Appl. Microbiol. 89, 267–305. doi: 10.1016/B978-0-12-800259-9.00007-X
Wei, R., and Zimmermann, W. (2017). Microbial enzymes for the recycling of recalcitrant petroleum-based plastics: how far are we? Microb. Biotechnol. 10, 1308–1322. doi: 10.1111/1751-7915.12710
Wexler, H. M. (2007). Bacteroides: the good, the bad, and the nitty-gritty. Clin. Microbiol. Rev. 20, 593–621. doi: 10.1128/CMR.00008-07
Woyke, T., Chertkov, O., Lapidus, A., Nolan, M., Lucas, S., Del Rio, T. G., et al. (2011). Complete genome sequence of the gliding freshwater bacterium Fluviicola taffensis type strain (RW262). Stand. Genomic Sci. 5, 21–29. doi: 10.4056/sigs.2124912
Wright, R. J., Bosch, R., Langille, M. G., Gibson, M. I., and Christie-Oleza, J. A. (2021). A multi-OMIC characterisation of biodegradation and microbial community succession within the PET plastisphere. Microbiome 9, 1–22. doi: 10.1155/2021/6620574
Keywords: metagenomics, metagenomic screening, PET degradation, polyethylene terephthalate (PET), PETase, Bacteroidetes, Flavobacteriaceae
Citation: Zhang H, Perez-Garcia P, Dierkes RF, Applegate V, Schumacher J, Chibani CM, Sternagel S, Preuss L, Weigert S, Schmeisser C, Danso D, Pleiss J, Almeida A, Höcker B, Hallam SJ, Schmitz RA, Smits SHJ, Chow J and Streit WR (2022) The Bacteroidetes Aequorivita sp. and Kaistella jeonii Produce Promiscuous Esterases With PET-Hydrolyzing Activity. Front. Microbiol. 12:803896. doi: 10.3389/fmicb.2021.803896
Received: 28 October 2021; Accepted: 22 November 2021;
Published: 05 January 2022.
Edited by:
Kian Mau Goh, University of Technology Malaysia, MalaysiaReviewed by:
Fusako Kawai, Okayama University Japan, JapanLukasz Jaroszewski, University of California, Riverside, United States
Alessandro Pellis, University of Genoa, Italy
Copyright © 2022 Zhang, Perez-Garcia, Dierkes, Applegate, Schumacher, Chibani, Sternagel, Preuss, Weigert, Schmeisser, Danso, Pleiss, Almeida, Höcker, Hallam, Schmitz, Smits, Chow and Streit. This is an open-access article distributed under the terms of the Creative Commons Attribution License (CC BY). The use, distribution or reproduction in other forums is permitted, provided the original author(s) and the copyright owner(s) are credited and that the original publication in this journal is cited, in accordance with accepted academic practice. No use, distribution or reproduction is permitted which does not comply with these terms.
*Correspondence: Wolfgang R. Streit, d29sZmdhbmcuc3RyZWl0QHVuaS1oYW1idXJnLmRl