- 1Instituto de Química y Bioquímica, Facultad de Ciencias, Universidad de Valparaíso, Valparaíso, Chile
- 2Escuela de Biología Marina, Facultad de Ciencias del Mar y de Recursos Naturales, Universidad de Valparaíso, Viña del Mar, Chile
- 3Laboratorio de Complejidad Microbiana y Ecología Funcional, Instituto de Antofagasta, Departamento de Biotecnología, Facultad de Ciencias del Mar y Recursos Biológicos, Universidad de Antofagasta, Antofagasta, Chile
- 4Centre for Biotechnology and Bioengineering, Universidad de Chile, Santiago, Chile
- 5Escuela de Ciencias del Mar e Instituto Milenio de Oceanografía, Pontificia Universidad Católica de Valparaíso, Valparaíso, Chile
- 6Laboratory of Aquatic Environmental Research, Centro de Estudios Avanzados, Universidad de Playa Ancha, Viña del Mar, Chile
- 7HUB Ambiental UPLA, Universidad de Playa Ancha, Valparaíso, Chile
- 8Departamento de Ciencias y Geografía, Facultad de Ciencias Naturales y Exactas, Universidad de Playa Ancha, Valparaíso, Chile
- 9Centro de Investigación Oceanográfica COPAS COASTAL, Universidad de Concepción, Concepción, Chile
The virus-to-prokaryote ratio (VPR) has been used in many ecosystems to study the relationship between viruses and their hosts. While high VPR values indicate a high rate of prokaryotes' cell lysis, low values are interpreted as a decrease in or absence of viral activity. Salar de Huasco is a high-altitude wetland characterized by a rich microbial diversity associated with aquatic sites like springs, ponds, streams and a lagoon with variable physicochemical conditions. Samples from two ponds, Poza Rosada (PR) and Poza Verde (PV), were analyzed by epifluorescence microscopy to determine variability of viral and prokaryotic abundance and to calculate the VPR in a dry season. In addition, to put Salar de Huasco results into perspective, a compilation of research articles on viral and prokaryotic abundance, VPR, and metadata from various Southern hemisphere ecosystems was revised. The ecosystems were grouped into six categories: high-altitude wetlands, Pacific, Atlantic, Indian, and Southern Oceans and Antarctic lakes. Salar de Huasco ponds recorded similar VPR values (an average of 7.4 and 1.7 at PR and PV, respectively), ranging from 3.22 to 15.99 in PR. The VPR variability was associated with VA and chlorophyll a, when considering all data available for this ecosystem. In general, high-altitude wetlands recorded the highest VPR average (53.22 ± 95.09), followed by the Oceans, Southern (21.91 ± 25.72), Atlantic (19.57 ± 15.77) and Indian (13.43 ± 16.12), then Antarctic lakes (11.37 ± 15.82) and the Pacific Ocean (6.34 ± 3.79). Physicochemical variables, i.e., temperature, conductivity, nutrients (nitrate, ammonium, and phosphate) and chlorophyll a as a biological variable, were found to drive the VPR in the ecosystems analyzed. Thus, the viral activity in the Wetland followed similar trends of previous reports based on larger sets of metadata analyses. In total, this study highlights the importance of including viruses as a biological variable to study microbial temporal dynamics in wetlands considering their crucial role in the carbon budgets of these understudied ecosystems in the southern hemisphere.
Introduction
Viruses are the most abundant components in the biosphere, far exceeding the number of bacteria and, anecdotally, even exceeding the number of stars in the visible universe (Griffin, 2013). Only in the oceans virus-like particles (VLP) reach between 104 to 108 per milliliter of seawater (Weinbauer, 2004; Clasen et al., 2008a; Payet et al., 2014) and 105 to 107 VLP mL−1 in others aquatic ecosystems such as wetlands (Jackson and Jackson, 2008). Viruses are dynamic and important components in many terrestrial and marine ecosystems (Srinivasiah et al., 2008). They can control the composition of communities, influence biogeochemical cycles, and induce horizontal gene transfer (Syvanen, 2012; Breitbart et al., 2018).
The virus to prokaryotic ratio (VPR) has been widely used by researchers (Wommack and Colwell, 2000; Weinbauer, 2004; Parikka et al., 2016) to study the relationship between viral communities and other microorganisms (whether bacteria or archaea) in the environment. This index determines the incidence that viruses have on their hosts, i.e., high values of VPR indicate that a high viral dynamic is taking place and, consequently, a high rate of prokaryotic cell lysis (Wommack and Colwell, 2000). In contrast, low values are interpreted as a decrease in or absence of viral activity (Wommack and Colwell, 2000; Weinbauer, 2004; Parikka et al., 2016). Despite the differences in the amount of VLPs in environments such as oceans, rivers, lakes, groundwater, soil, and marine sediments (Clasen et al., 2008b; Srinivasiah et al., 2008; Parikka et al., 2016), in most ecosystems the abundance of viruses far exceeds that of their prokaryotic hosts by at least an order of magnitude (Breitbart and Rohwer, 2005; Wigington et al., 2016). This can also be observed in the VPR averages of different ecosystems, being 20.7 in coastal marine environments, 38 in the open ocean, 11.3 in estuaries, and 18.8 in lakes (Parikka et al., 2016). These differences in VPR values may be caused by diverse biological (e.g., chlorophyll a concentration, and abundance of bacteria) (Clasen et al., 2008b) and physicochemical factors (e.g., temperature, salinity, and nutrients) (Tuomi et al., 1995; Eissler et al., 2010; Salter et al., 2011; Parikka et al., 2016). In eutrophic environments, viral production (VP), i.e. the number of viruses produced in a given volume and time span, is associated with prokaryotic production, which eventually reduces prokaryotes abundance due to viral lysis (Steward et al., 1996; Noble and Fuhrman, 2000; Wilhelm et al., 2002; Winget and Wommack, 2009). These top-down controls of virus activity on prokaryotes consequently result in an increase in virus abundance and VPR values (Wommack and Colwell, 2000; Parikka et al., 2016). In contrast, there is less prokaryotic production in oligotrophic environments, so viral production and VPR values are low (Parikka et al., 2016). In particular, the southern hemisphere concentrates conspicuous microbial diversity hotspots systems associated with trophic gradients, like the eutrophic coastal areas of the Eastern South Pacific an Eastern Upwelling Boundary System (EBUS) with prominent oxygen minimum zones (OMZ) and Anoxic Marine Zones in the waters (Ulloa et al., 2012). In this EBUS area, VPR values have been shown to decrease from the surface and then increase again in the sub-oxic or anoxic subsurface layer (Taylor et al., 2003; Chiang and Quiñones, 2007). High VPR values have been registered in coastal bay areas at EBUS (Kuznar et al., 2009; Eissler et al., 2010).
On the other hand, the southern hemisphere concentrates a significant number of extreme and poly extreme ecosystems understudied in terms of VPR dynamics, such as the highlands Andean wetlands. High-altitude wetlands, such as Salar de Huasco, have been considered a polyextreme environment, i.e., where multiple and/or simultaneous forms of stress determine diversity (Dorador et al., 2020). This ecosystem is located at 3,800 m above sea level at the Chilean Altiplano and covers an area of approximately 15,858 ha, with the presence of permanent brackish lagoons, ephemeral hypersaline ponds, and freshwater springs (Dorador et al., 2020). Regarding viral activity, a wide range of VPR values, from 2 to 351 (Eissler et al., 2019), have been found in this wetland. VPR values varied greatly associated with the high heterogeneity of aquatic environments within the Salar de Huasco, especially considering salinity ranging from fresh to mesosaline water (Eissler et al., 2019, 2020).
In this study, we aim to determine viral host dynamic relationships using VPR under different physical and chemical conditions in Salar de Huasco. Moreover, our results were compared with VPR variability in ecosystems characterized by contrasting origin and biogeochemical conditions from the southern hemisphere.
Materials and methods
Sampling sites
The sampling was carried out during the dry season in Salar de Huasco, Chile, (September 16-17, 2019). Samples were taken from two ponds located at site H3 (20° 17'01”S; 68° 53'21”W) (Figure 1A), referred to as “Poza Rosada” (PR) and “Poza Verde” (PV) due to their qualitative characteristics in relation to their respective predominant colors (Figures 1B–D). PR was sampled five times during 2 days and PV was sampled twice for 1 day.
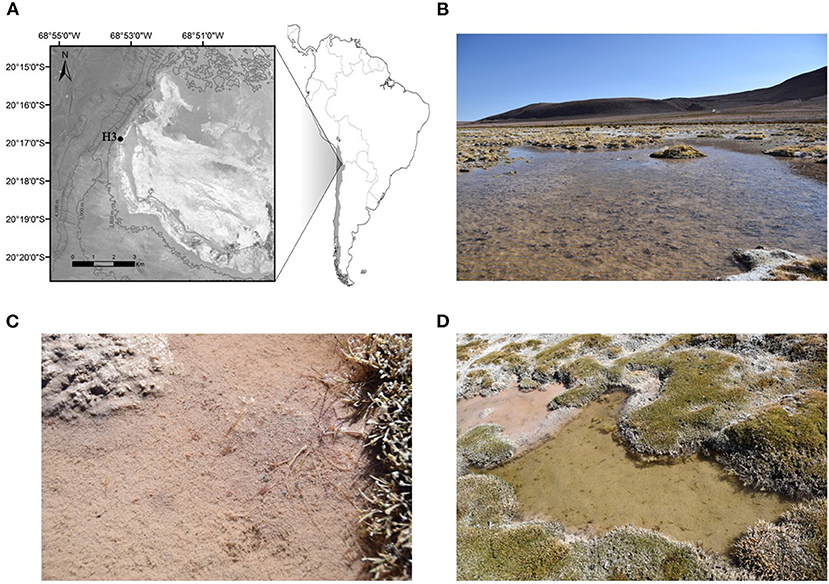
Figure 1. (A) Map showing the location and area of the site (H3) sampled during 16-17 September 2019, (B) Shows a general imagine of “Poza Rosada” (PR), (C) Shows a close up of microbial mats that are characterized by their pink color and (D) Shows “Poza Verde” (PV) and its green color in contrast with a near pond of pink color.
Measurement of physicochemical and biological variables
The physical and chemical parameters of these sites (i.e., temperature, conductivity and pH) were measured in situ with a Thermo Scientific Orion Star multiparameter device (model A329). To determine nitrate, nitrite, phosphate and silicic acid, water samples (60 mL) were taken in triplicate, filtered through a GF/F filter (Whatman®, Buckinghamshire, UK) and stored at −20°C. These nutrient samples were analyzed using colorimetric methods (Strickland and Parsons, 1972) with an automatic nutrient analyzer (Atlas et al., 1971). To determine chlorophyll-a concentration, 200 mL water samples were filtered through a GF/F filter (Whatman®, Buckinghamshire, UK). The filters were stored at −20°C to then be analyzed using the fluorometric method (Caspers, 1970) in a Turner A10 fluorometer.
Viruses and prokaryotes abundance
Samples were taken with 15 mL centrifuge tubes, fixed with 1% glutaraldehyde and immediately frozen until analysis. To count virioplankton, the viral samples were pre-filtered using a syringe and a 0.22 μm polyethersulfone filter unit (Millex GP, Millipore®, Darmstadt, Germany), followed by filtration through an Anodisc membrane filter (Whatman®, Buckinghamshire, UK) of 0.02 μm pore size, applying 130 mm Hg of pressure. Each filter was placed in a Petri dish in the dark and stained for 15 min with 100 μl of SYBRTM-Gold at a concentration of 2x (10000X stock solution; Invitrogen, Carlsbad, CA, USA), which was prepared from a 50x intermediate solution with molecular biology grade water previously filtered by 0.025 μm MFTM membrane filters (Millipore®, Darmstadt, Germany). Once the filters were stained, the excess solution was removed with Kimwipe® tissues (Kimtechscience, Little Rock, AR, USA) and they were allowed to dry for approximately 10 min on a filter paper in the dark. Finally, each filter was mounted on a slide for later observation. To estimate the abundance of prokaryotes, the same procedures described for the viral samples were conducted, omitting the pre-filtering of the sample, and using an Anodisc membrane filter (Whatman®, Buckinghamshire, UK) of 0.2 μm pore size to mount the samples (Chen et al., 2001). VLPs and prokaryotes were observed at 1000x magnification through an epifluorescence microscope (Olympus BX60F-3, equipped with a WIB long-pass fluorescence cube, excitation wavelength 460-490 nm, DM 505 beam splitter, emission BA 515IF) fitted with a 50 W HBO mercury lamp, where a total of at least 100 viral particles and cells were counted in 10 to 20 randomly chosen fields.
Data processing
Literature review of articles on microbial communities of the Salar de Huasco and other aquatic ecosystems of the Southern hemisphere was carried out. Research articles with the original viral abundance and prokaryote data available in tables, either in results or Supplementary Files, were selected. Articles with graphical representation of results had to be excluded. The articles were obtained from different databases (ScienceDirect, SciELO, PubMed and Google Scholar). The search was carried out by entering the following keywords: “virus-to-prokaryote ratio”, “virus-to-bacteria ratio”, “virus-to-cell ratio”, “Virus-to-host ratio”, “VPR”, “VBR”, “Salar de Huasco”, “viral abundance” and “viral activity”, applying the same criteria used by Parikka et al. (2016). Two of the articles found did not present the data in a tabular format, for which reason the authors were contacted for data details (Chiang and Quiñones, 2007 and Eissler et al., 2010). When VPR was not available in the article, viral and prokaryotic abundances were used to calculate this index. Units had to be converted, when necessary, to enable statistical analyses. In the case of salinity, practical salinity units and parts-per-thousand (‰) were considered as equivalent. Salinity was transformed to conductivity using the salinity conversion calculator available at: https://www.hamzasreef.com/Contents/Calculators/SalinityConversion.php, following Wagner et al. (2006). These data were classified on the basis of habitat (e.g., ponds, springs, marine, freshwater) and ecosystems type (e.g., high-altitude wetlands, oceans, and lakes) and are available, along with the physicochemical and biological variables, in Supplementary Table S1.
Data and statistical analysis
First, Shapiro-Wilk, Kolmogorov-Smirnov and Lilliefors tests were conducted to check the normality of all data. Data distribution was not normal (Supplementary Table S2). Therefore, statistical analysis to compare VPR values of the different ecosystems were carried out with a non-parametric test like Kruskal Wallis and Tukey test (Statistica version 7.0). To determine relationships between the VPR values and the biological and environmental parameters of the Salar de Huasco and all ecosystems, a non-parametric Spearman's rank correlation was performed (Statistica version 7.0).
VPR distribution of the different ecosystems analyzed, considering the physicochemical conditions such as temperature, conductivity and depth, were visualized using bubble plots with ggplot2 and geom_jitter in R studio (R Core Team, 2021).
Principal component analysis (PCA) was performed with the environmental data normalized by using scale function in R studio, to visualize the physicochemical variability in different habitats of Salar de Huasco, i.e., ponds, springs, and lagoon (database n = 16) and ecosystems, i.e., high-altitude wetlands, Pacific Ocean, Atlantic Ocean, and Southern Ocean (database n = 74), then the envfit function (library vegan) was used to overlay viral, prokaryotic abundances and VPR and determine their potential association with the ordination axes (R Core Team, 2021). SigmaPlot version 14.0 program was used to plot the variation of viral and prokaryotic abundances and other environmental variables studied from PV and PR in Salar de Huasco, and was also used to compare VPR values of the studied ecosystems.
Results
Data
A total of 15 reports on microbial abundances, including viruses and environmental parameters from 19 areas of different ecosystems in the Southern hemisphere, were considered for comparison (Figure 2; Table 1). Together, these publications account for a total of 1198 viral and prokaryotic abundance data with their respective VPR (Supplementary Table S1). The following physical, chemical and biological conditions, i.e., dissolved oxygen, pH, salinity, concentrations of nitrate, nitrite, ammonium, phosphate, silicate, chlorophyll, carbon dioxide, methane, nitrous oxide, when available (Supplementary Table S1), were listed. The areas were divided on the basis of the large ecosystems of origin (i.e., high-altitude wetlands, Pacific, Atlantic, Indian and Southern Oceans and Antarctic Lakes, Figure 2).
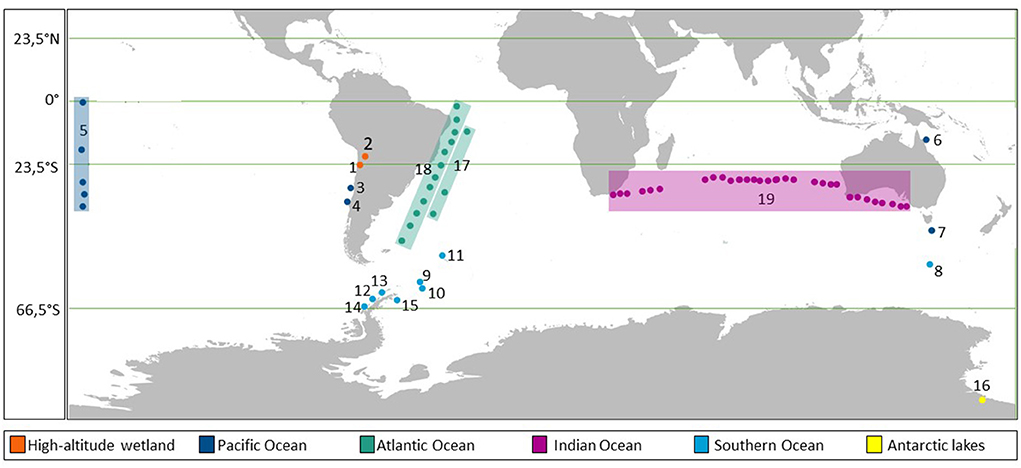
Figure 2. Map showing the areas and sampling sites analyzed in this work from the Southern hemisphere. The numbers (ID) show the areas sampled and colored squares indicate sampled transects.
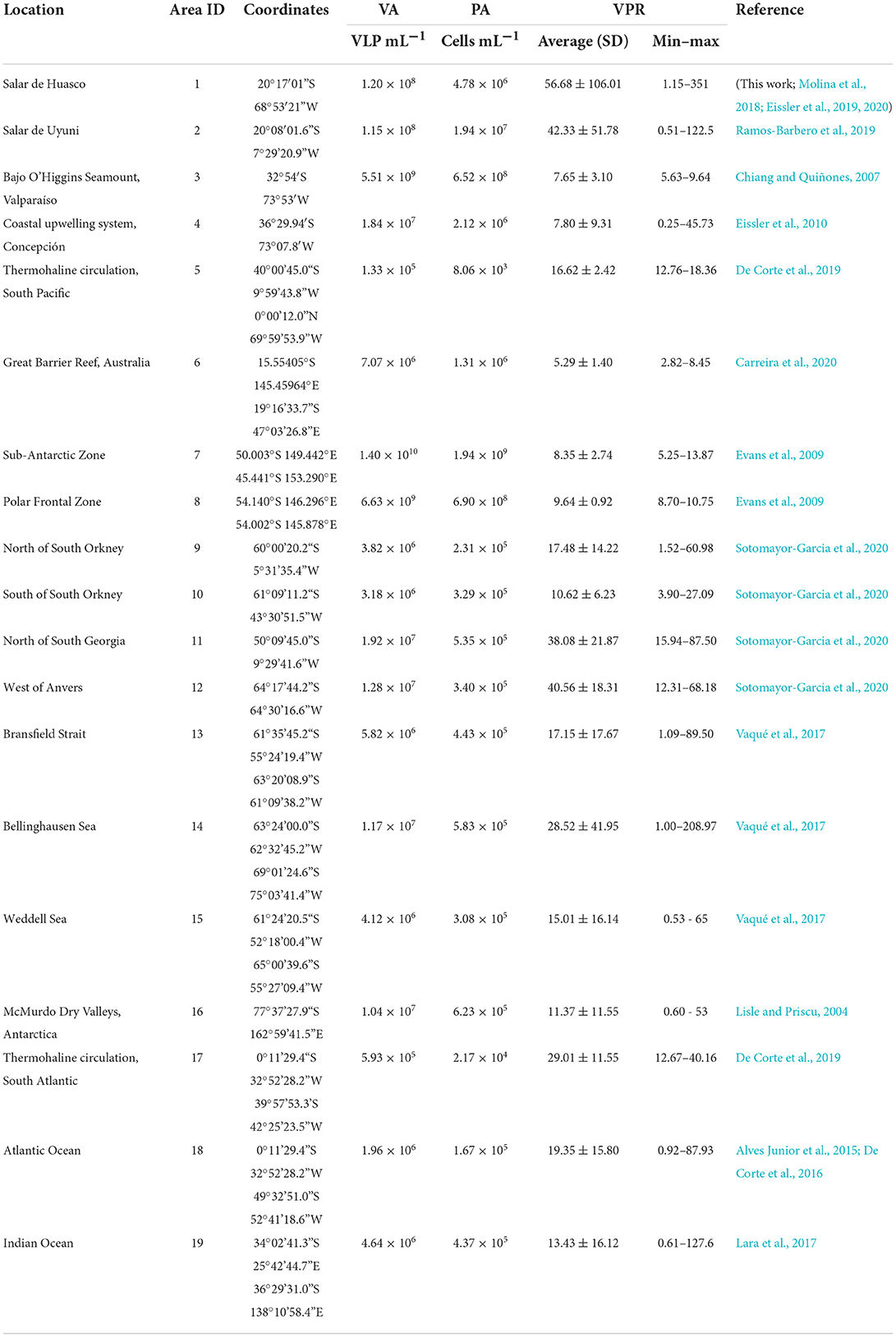
Table 1. Mean values of viral (VA) and prokaryotic abundance (PA); average, standard deviation (SD) and range (minimum to maximum) of VPR in aquatic ecosystems of South hemisphere.
Viral abundance, VPR and environmental variables in the Salar de Huasco
During the daily cycle studied in PR, an increase in viral abundance was observed in the evening. A minimum value (5.73 × 107 ± 7.08 × 106 VLP mL−1) was observed around midday, at 11:30 h, while a maximum value of 9.01 × 107 ± 4.06 × 106 VLP mL−1 was registered at 6:30 h the next day, (Figure 3A; Supplementary Table S3). Prokaryotic abundance peaked in the afternoon (2.25 × 107 ± 4.22 × 106 cells mL−1), at 17:30 h, and reached its lowest value (5.64 × 106 ± 3.54 × 105 cells mL−1) at 6:30 h, which is consistent with the time at which the maximum viral abundance was found (Figure 3A; Supplementary Table S3). The VPR values during the daily cycle ranged from 3.17 to 15.99, with the minimum being observed at 15:30 h and the maximum at 6:30 h of the following sampling day (Figure 3A; Supplementary Table S3). Similar to PR, the viral abundance for PV was higher in the morning than at midday (1.18 × 107 ± 1.46 × 106 VLP mL−1, 7:30 h, 9.39 × 106 ± 1.03 × 106 VLP mL 11:30 h) (Figure 3B; Supplementary Table S3). In contrast, prokaryotic abundance was lower at noon (Figure 3B), thus, the higher VPR value (2.38) was registered at 7:30 h (Figure 3B; Supplementary Table S3).
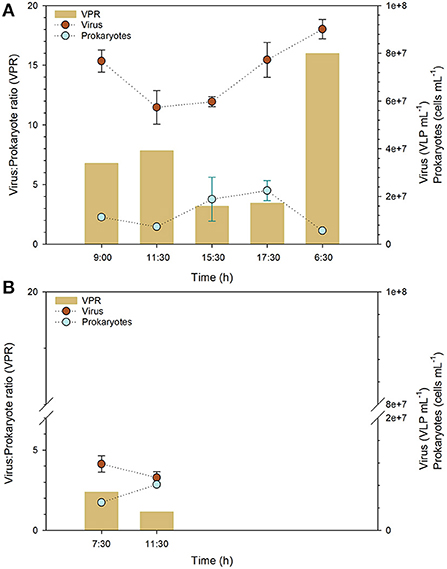
Figure 3. Viral and prokaryotic abundance and virus to prokaryote ratios (VPR) in two ponds sampled in Salar de Huasco (September 16-17th, 2019), (A) PR and (B) PV. Error bars represent the standard deviation.
Generally, there was not a significant nutrient variation over time; however, a variation in temperature and chlorophyll a concentrations, which were inversely related to viral abundance and VPR (Figures 3, 4), was recorded at PR. Regardless of the little data available, when relationships were examined, temperature presented no significant correlations with any of the variables, and chlorophyll a only showed a significant relation (r = −0.89) with conductivity (Supplementary Table S4). Viruses showed a significant correlation with Nitrite (r = 0.767) and Silicic acid (r = 0.821) and VPR with conductivity, pH (both r = 0.9) and chlorophyll a (r = 0.893); conversely, no relationships were found for prokaryotic abundance (Supplementary Table S4).
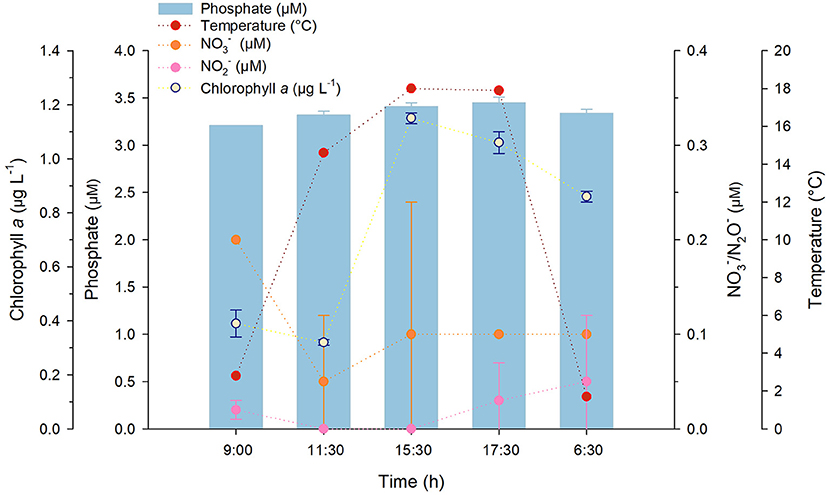
Figure 4. Temperature, nitrate, nitrite phosphate and chlorophyll a concentration changes in PR during sampling in Salar de Huasco (September 16–17th, 2019). Error bars represent the standard deviation.
When all data available up to now for Salar de Huasco was correlated, significant relationships were found between viral abundance and prokaryotic abundance, VPR, conductivity, pH and Silicic acid (Supplementary Table S5). Prokaryotic abundance was significantly correlated with conductivity and nitrate, and VPR was significantly correlated with VA and chlorophyll a concentration Supplementary Table S5). On the other hand, the PCA ordination axes accounted for > 67%, of the variability associated with the environmental conditions of the different aquatic areas of Salar de Huasco, i.e, ponds, springs and lagoons as categories (Figure 5). Ponds were the habitat exhibiting a higher variability related with conductivity, pH and phosphate. Viral and prokaryotic abundance were positively correlated (r = 0.73 and 0.998, P ≥ 0.07), whereas VPR was negatively correlated (r = −0.88, P = 0.92) to PC1, axis accounting 37% of the aquatic environments' variability.
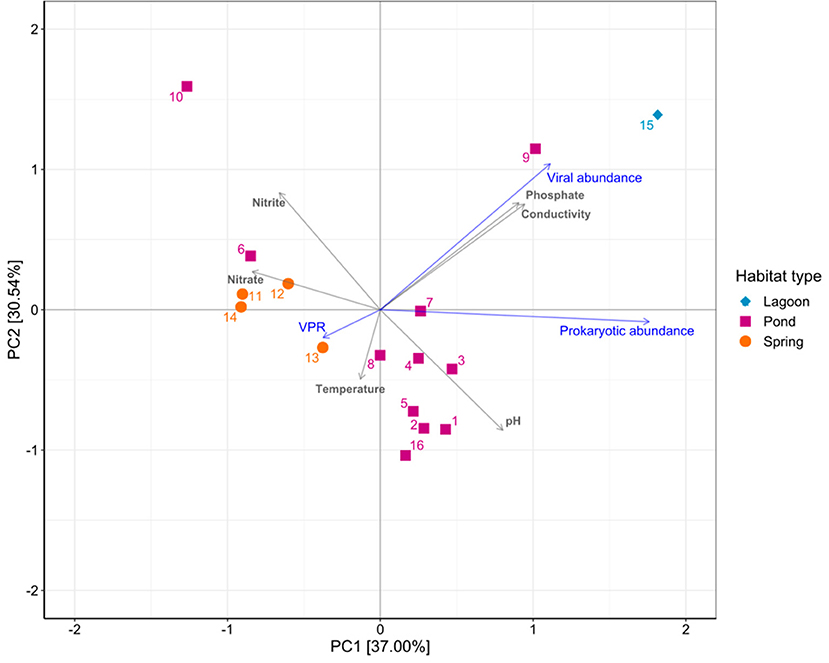
Figure 5. Principal component analysis showing the variability of environmental variables and VPR values associated with the different habitats studied in Salar de Huasco (data from this study, Molina et al., 2018; Eissler et al., 2019, 2020).
VPR in different ecosystems of the southern hemisphere
A wide range of VPR values were found among all the ecosystems studied, varying from 0.25 in coastal upwelling system, Concepción to a maximum value of 351 in Salar de Huasco (Table 1), showing a difference of more than three orders of magnitude.
The high-altitude wetlands (i.e., Salar de Huasco and Salar de Uyuni) recorded the highest average VPR value (53.22 ± 95.09), followed by the Southern (21.91 ± 25.72), Atlantic (19.57 ± 15.77) and Indian (13.43 ± 16.12) Oceans (Figure 6). On the other hand, the Antarctic lakes and the Pacific Ocean recorded the lowest average VPR values, being 11.37 ± 15.82 and 6.34 ± 3.79, respectively (Figure 6).
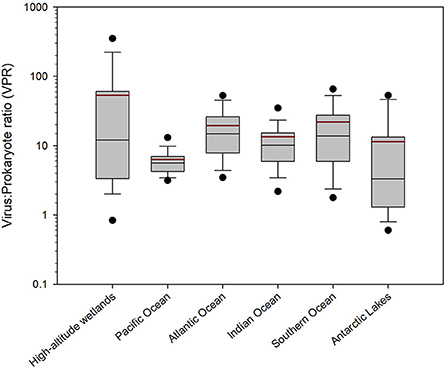
Figure 6. Boxplot graph of the VPR values of different aquatic ecosystems in the Southern hemisphere. The area of the boxes shows the 25th and 75th percentiles and the whiskers represent 5 and 95% of the data. The thin black line is the median and the thick dark red line is the average. Outliers are shown in black circles.
The Kruskal-Wallis H test showed significant differences among the ecosystems' VPR values (H = 241.8; P < 0.001). Particularly, a Tukey test comparison showed that VPR values for the Pacific Ocean were significantly different from all the other ecosystems (P < 0.05), except for the Antarctic lakes (Table 2). The high-altitude wetlands showed significant differences with the Pacific Ocean and Antarctic lakes (P < 0.05), while the Atlantic Ocean showed significant differences with the Indian Ocean and Antarctic lakes (P < 0.05) (Table 2). Finally, the Indian Ocean and the Antarctic lakes showed significant differences with the Southern Ocean (P < 0.05) (Table 2).
Significant and positive correlations using the Spearman Rank test were found for VPR values and viral abundance, nitrate, ammonium, phosphate, and chlorophyll a (P < 0.05) (Table 3). In addition, VPR values presented a significant and negative correlation with prokaryotic abundance, temperature, and conductivity (P < 0.05) (Table 3). While viral abundance of the ecosystems was positive and significantly correlated with the abundance of prokaryotes, VPR, temperature, nitrate, and phosphate, it was negatively correlated with silicic acid and chlorophyll a (P < 0.05; Table 3). Finally, prokaryotic abundance was significant and positively correlated with viral abundance, temperature and conductivity and negatively related to VPR values, nitrate, ammonium, silicic acid, and chlorophyll a (P < 0.05) (Table 3).
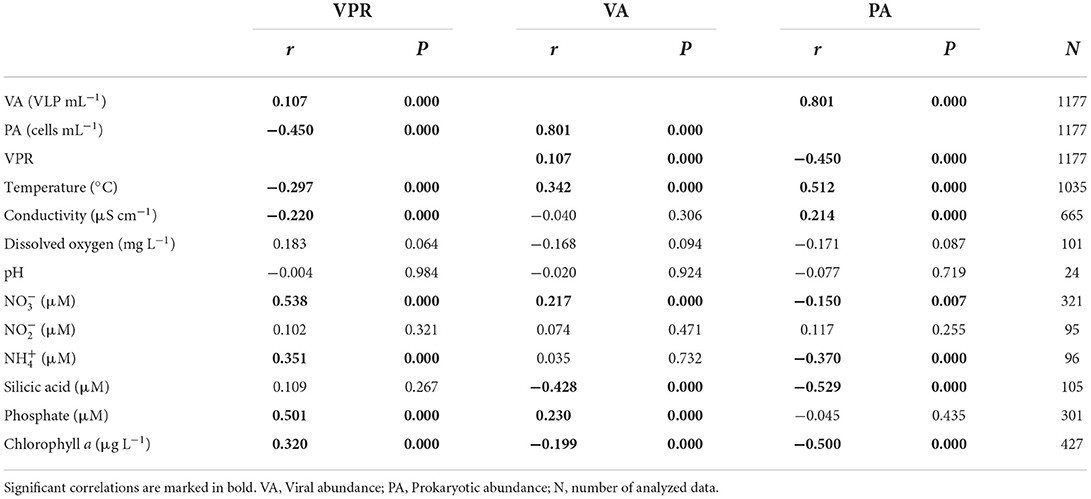
Table 3. Spearman's rank correlations (r) between viral and prokaryotic abundance, VPR and environmental variables in all studied ecosystems of South hemisphere.
Overall, bubble plots showed a high VPR variability associated with temperature, conductivity, and depth conditions (Figures 7A–C). Extreme environments, such as high-altitude wetlands and Antarctic lakes, presented the highest VPR variability compared with the oceanic ecosystems. However, when comparing the oceans, some trends were evidenced in the databases characterized by a higher VPR variability from the Southern, Indian, Atlantic compared with the Pacific Ocean. For seawater environments, the lowest and highest VPR values were recorded in the Pacific Ocean and Southern Ocean, respectively (Figures 7A–C). Most of the VPR data available was associated with the upper 1,000 m water depth for all marine systems and surface waters in terrestrial aquatic sites. Higher VPR values were concentrated at the surface compared with deep and cold waters in the Southern Ocean, whereas the opposite was observed in the Pacific Ocean Supplementary Figure S1). The highest VPR magnitudes were registered in high-altitude wetlands (Figure 7).
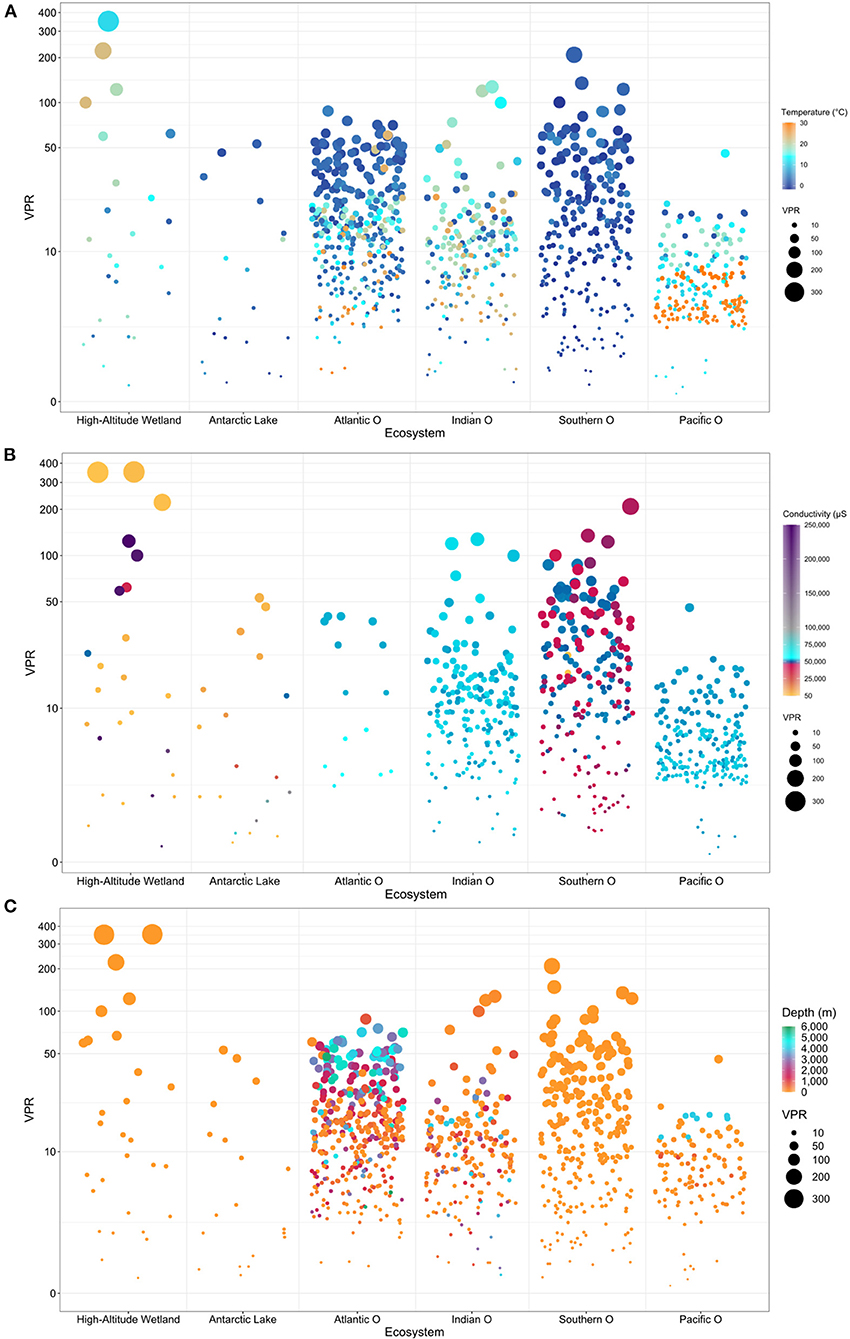
Figure 7. Bubble plots analysis (A) Temperature (B) Conductivity and (C) Depth, showing the relationship between environmental variables and VPR values potentially relevant in the different ecosystems analyzed.
PCA analysis of environmental conditions, considering Atlantic, Pacific and Southern Ocean and high-altitude wetlands (Salar de Huasco and Salar de Uyuni), accounted for 41.68% in PC1 and 24.11% PC2 of the variability (Figure 8). The ordination showed that the aquatic systems were clearly differentiated on the PC1-axis, associated mainly with temperature, conductivity and nitrate; whereas depth and ammonium, on the PC2-axis. Viral and prokaryotic abundances and VPR were significantly related with the variability represented in PC1-axis, showing r = −0.89, −0.998 and −0.73, respectively (P ≤ 0.01) (Figure 8). The variability was mostly associated with the differentiation between high-altitude wetland compared with the other ecosystems.
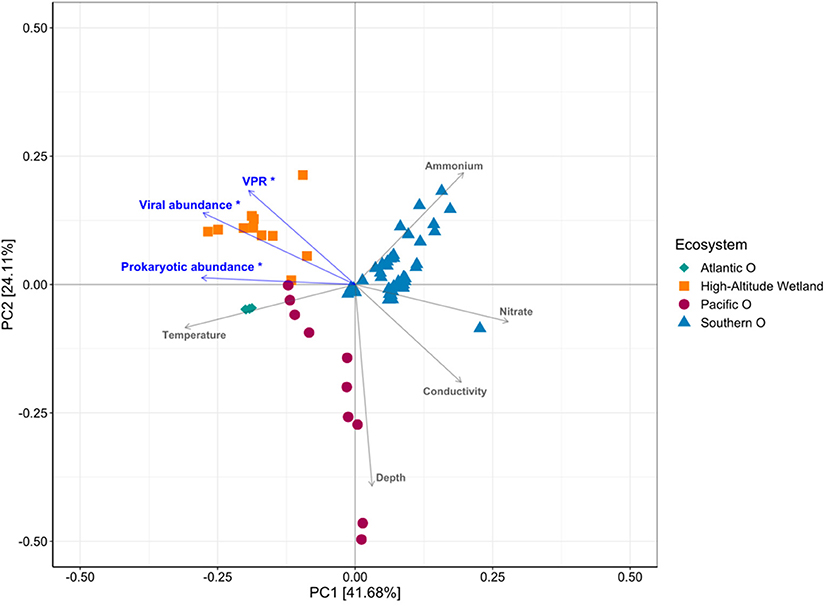
Figure 8. Principal component analysis showing the relationship between environmental variables and VPR values potentially relevant in the different ecosystems analyzed.
Discussion
In this study, we analyzed the virus-to-prokaryote ratio (VPR) values from two ponds of Salar de Huasco, including the latest available data for this ecosystem to explore the relationships between biological and physicochemical variables. In addition, to investigate general patterns in the relationship between VPR and environmental variables, metadata from other aquatic ecosystems from the Southern hemisphere was analyzed.
VPR and environmental conditions in Salar de Huasco
Viral and prokaryotic abundance found in the PR and PV pond sites of Salar de Huasco were within the range found in previous studies, i.e., 7.78 × 105 to 4.78 × 108 VLP mL−1 for viral (VA) and 1.10 × 104 to 1.83 × 107 cells mL−1 for prokaryotes (PA) (Supplementary Table S1, Molina et al., 2018; Eissler et al., 2019, 2020). However, the VPR ranges (1.15–15.99) were lower than those reported for the study area (67–351), measured at similar aquatic sites but during the austral summer wet season in December–February (Eissler et al., 2019). Moreover, these VPR values were in the higher range found for most ecosystems (Wommack and Colwell, 2000; Parikka et al., 2016). Our results were consistent with the VPR ranges determined in other high-altitude wetlands such as Salar de Uyuni (0.51–122.5, Ramos-Barbero et al., 2019).
The aquatic areas of the ponds in Salar de Huasco are characterized by high variability in their environmental conditions associated with physicochemical changes such as conductivity, pH and nutrients (Figure 5). Salar de Huasco has been described as a hyper arid environment having different microhabitats with extreme differences in salinity (Risacher et al., 2003), which is a relevant environmental factor for microbial community composition (e.g., Dorador et al., 2020). Previous studies indicate that ponds are aquatic systems covering large surface areas of Salar de Huasco, which have a highly diverse and conspicuous microbial life contributing significantly with biogeochemical recycling processes (Aguilar et al., 2016; Hernández et al., 2016; Molina et al., 2020). During our study, the ponds analyzed presented a particular coloration (Figure 1) due to the presence of microbial mats with the predominance of bacterial communities, probably from the Roseobacter group in PR and Cyanobacteria in the case of PV, which have been previously identified in the Salar de Huasco (Dorador et al., 2008, 2013; Aguilar et al., 2016).
In addition, VA and PA cycled during the day in the PR pond, showed a maximum VPR value early in the morning compared to the afternoon, a trend supported in the PV pond comparing two sampling points (Figure 3). Extreme changes in the environmental conditions in this extreme ecosystem, including solar radiation, thermal and wind stress, have been found to shape microbial communities, influencing the nutrient and greenhouse gases reservoirs of the ponds of Salar de Huasco (Molina et al., 2020). In the current study, physicochemical (conductivity and pH) and biological (chlorophyll a) variables were correlated with VPR shifts (Supplementary Table S4). On the other hand, considering all the available data on this high-altitude wetland, significant correlations support the role of physicochemical conditions in shaping viral and prokaryotic abundance, including nutrients (Supplementary Table S5). The relationships found between viral dynamics and environmental conditions are consistent with other natural wetland types, such as tidal, mangrove, freshwater, forested, riparian and constructed wetlands, which are understudied land systems in comparison to marine systems (Jackson and Jackson, 2008), especially in the southern hemisphere. In the Salar de Huasco wetland, higher nutrient availability, mainly during the wet season, has been related with microbial activity and the different microhabitats, such as ponds, springs, and the lagoon (Eissler et al., 2019, 2020). Several authors have pointed out the importance of viruses in the cycling of nutrients, especially regarding the carbon cycle in aquatic ecosystems (e.g., Fuhrman, 1999; Wommack and Colwell, 2000; Weinbauer, 2004; Suttle, 2005, 2007; Breitbart et al., 2018). Recently, Gao et al. (2022), calculated that for wetlands from tundra ecosystems the contribution rate of carbon release by viral lysis of bacteria to the ecosystem's dissolved organic carbon ranged from 0.8 to 4.4, with an average of 2.6 ‰, representing a significant amount compared with marine ecosystems. In some wetlands, rewatering and water level conditions could change viral lysis rates which may vary the emissions of greenhouse gases in these systems as experimentally explored (Bonetti et al., 2021). So far, a high carbon release by viral lysis is expected in the high-altitude wetlands during the wet season and, particularly, at specific hours of the day, such as in the early morning periods, where greater dissolved organic carbon, nutrient and CH4 accumulation have been registered in diel cycling at pond sites (Molina et al., 2020).
Comparison of viral dynamics in different ecosystems of the southern hemisphere
The ecosystems compared in this study presented large physicochemical and biological differences, in terms of salinity, nutrients (i.e., nitrate, nitrite, phosphate and silicate), temperature, dissolved oxygen, pH and chlorophyll (Supplementary Table S1; Figure 8), which can influence and regulate viral dynamics, virus-host interactions and, therefore, the VPR values in the ecosystems studied (Wommack and Colwell, 2000; Parikka et al., 2016). The high-altitude wetlands, located in the Andes where Chile, Bolivia and Argentina meet, are extreme environments presenting saline lakes fed by waters from the Andes mountains, characterized by high evaporation and low rainfall (Risacher et al., 2003; Risacher and Fritz, 2009). As mentioned before, Salar de Huasco is characterized by being a diverse and changing environment, with permanent brackish lagoons, ephemeral hypersaline or freshwater ponds (de la Fuente and Niño, 2010; Bull and Asenjo, 2013; Carrasco-Lagos et al., 2015). On the other hand, Salar de Uyuni (Ramos-Barbero et al., 2019; Figure 2, ID 2) stands out for being one of the world's largest and most hypersaline high-altitude wetlands (Rettig et al., 1980; Risacher and Fritz, 2009). In both wetlands, samples were collected from different habitats: ponds and lakes in the Salar de Huasco; ponds, pits, groundwater, and lagoon in the Salar de Uyuni (Ramos-Barbero et al., 2019). These extreme ecosystems exhibit the wider range of VPR magnitude compared with other environments in our database (Figure 6). This is consistent with Parikka et al. (2016) study showing that saline environments, including marine areas, presented a high range of VPR values, significantly higher compared to freshwater environments, groundwater and hot springs.
Particularly, despite high-altitude, wetlands presented vastly different environmental characteristics in comparison with the other ecosystems analyzed in our study. The viral activity measured using the VPR was not significantly different, except when compared with the Pacific Ocean VPR values (Table 2). The Pacific Ocean recorded the low average VPR values (7.65, 7.80 and 5.29, respectively) compared with the data registered in the Atlantic, Indian and Southern oceans (Figure 6; Table 1). The Pacific Ocean VPR variability could be associated with the temporal-spatial distribution of the data analyzed, such as, an oxygen minimum zone (OMZ) 135 miles west off Valparaíso, Chile (Chiang and Quiñones, 2007; Figure 2, ID 3), an upwelling system 18 and 40 miles west off Concepción, Chile (Eissler et al., 2010; Figure 2, ID 4) and surface waters in the Great Barrier Reef (Carreira et al., 2020; Figure 2, ID 6). The oxygen level related to depth in the Pacific Ocean also influences the distribution of VPR values, which decreased from the well-oxygenated surface part of the water column (from 12.56 on the surface to 4.3 at 100 m) (Chiang and Quiñones, 2007). Additionally, when hypoxic conditions were observed, a slight increase was detected (7.05 at 150 m) (Chiang and Quiñones, 2007). These variations in the VPR values in the oxic and anoxic layer were the result of different microbial assemblages that inhabit these strata (Chiang and Quiñones, 2007). Likewise, low VPR values observed in anoxic environments could be accounted for a reduction in viral production due to the decrease in the host communities or to a high loss of free-living viruses (Cassman et al., 2012). In contrast, despite the low VPR average observed off Concepción, the values ranged from 0.25 to 45.73, indicating a high viruses and prokaryotes dynamic and that during upwelling conditions VPR values were in fact higher (Eissler et al., 2010). In addition, Wommack and Colwell (2000) indicated that VPR values generally vary between 3 and 10, and that higher values are associated with highly productive environments (Wommack and Colwell, 2000; Weinbauer, 2004; Parikka et al., 2016), which has been also associated with upwelling environments of the Eastern Pacific (Kuznar et al., 2009; Letelier et al., 2009; Eissler et al., 2010). In two of these areas (Chiang and Quiñones, 2007; Carreira et al., 2020) nutrient availability was relatively low, especially in the Great Barrier Reef (Carreira et al., 2020). As an example, HPO average was 0.09 ± 0.02 mmoL μL−1, therefore, this could have contributed to the overall lower VPR average and range observed.
Specifically, the VPR values of the Antarctic lakes showed significant differences from those of the Southern Ocean and high-altitude wetlands (Table 2). The McMurdo dry valleys in Antarctica (Lisle and Priscu, 2004; Figure 2, ID 16) are some of the most extreme ecosystems on the planet. Its frozen lakes are characterized by their perennial ice covers, consequently, they have poor nutrient input (McKay et al., 1985; Chinn, 1993). According to Lisle and Priscu (2004), this lack of nutrients (especially the shortage of phosphorus) would produce low viral activity and induce the lysogenic cycle. In addition, it would induce the formation of microbial aggregates around the available nutrients, thus allowing nutrients recycling (such as phosphate) within these aggregates, which would be promoted by viral lysis (Lisle and Priscu, 2004). On the other hand, the Southern Ocean presents a greater availability of nutrients that supports an increase in primary production (Tréguer and Jacques, 1992; Smith Jr et al., 2000), and, therefore, an increase in VPR values. Furthermore, Vaqué et al. (2017) and Sotomayor-Garcia et al. (2020) indicate that viral activity in Antarctic waters is more sensitive to temperature and prokaryotic abundance than to any other environmental factors. In contrast, high-altitude wetlands present a larger nutrient input, allowing a greater development of microbial and viral communities (Márquez-García et al., 2009; Haferburg et al., 2017; Eissler et al., 2019; Ramos-Barbero et al., 2019).
Influence of environmental variables on the viral dynamics in the southern hemisphere ecosystems
Regardless of the methods used to count viruses and prokaryotes, 4 out of the 15 studies here use epifluorescence microscopy and the rest use flow cytometry (Supplementary Table S1). The tendencies of the relationships between VPR values and physicochemical variables found here were consistent with previous studies that analyzed a larger data set from a wider spatial-temporal scale (Wommack and Colwell, 2000; Parikka et al., 2016). First, as expected by solving the mathematical relationship between viral and prokaryotic abundance, using VPR (VPR = Viral abundance/Prokaryotic abundance) (Heldal and Bratbak, 1991; Parikka et al., 2016), VPR values were positively related to the viral abundance and negatively to the prokaryotic abundance, since there is an increase in the ratio when the viral abundance increases in relation to the prokaryotic abundance.
In the ecosystems studied, VPR was significantly positively correlated with chlorophyll a concentration (Table 3). These correlations were in agreement with Parikka et al. (2016) results in aquatic habitats and sediment-related ecosystems where VPR was positively correlated with VA, chlorophyll a and other microbial variables associated with infected cells (e.g., visibly infected cells and burst size). This positive correlation may suggest a viral infection on phytoplankton since chlorophyll a has been largely used as an indicator of biomass of photoautotrophic organisms and this relationship has numerous examples in literature as well as in seawater and in freshwater environments (Wommack and Colwell, 2000; Mojica and Brussaard, 2014; Zhong et al., 2014; Parikka et al., 2016; Flynn et al., 2022). Moreover, it has been suggested that this relationship indicates that viral activity regulates the structure of the phytoplankton community and the primary productivity of the marine ecosystems studied (Suttle, 1994; Boyer et al., 2009; Weitz et al., 2015).
Both temperature and conductivity in the ecosystems analyzed showed a significant negative relationship with VPR values, as has been reported by Parikka et al. (2016). In aquatic environments with high temperatures, the structural conformation of the viral lipid membranes and viral capsid proteins can be affected, which makes viruses and their host more susceptible to this environmental factor (Baudoux and Brussaard, 2005; Mojica and Brussaard, 2014). In contrast, low temperatures in polar environments do not inhibit viral activity and its potential to infect microbial communities (Anesio and Bellas, 2011). It has been observed that in Antarctic and Sub-Antarctic marine environments viral abundance is primarily related to temperature (Sotomayor-Garcia et al., 2020; Figure 2, ID 9-12). Furthermore, in areas surrounding the Antarctic peninsula (Vaqué et al., 2017; Figure 2, ID 13-15) viruses rather than grazers are more important agents of prokaryotic mortality. In addition, in our database, the relationship between VPR and temperature may be influenced by the data obtained from the areas studied in the Southern Ocean (Vaqué et al., 2017; Sotomayor-Garcia et al., 2020), where viral activity and prokaryotic production is more affected by temperature than by any other environmental factors. It is worth mentioning that viruses are resilient to low temperatures, since when weather conditions are unfavorable for prokaryotes growth, viruses change their lytic life strategy for a lysogenic one (Laybourn-Parry et al., 2007; Anesio and Bellas, 2011; Brum et al., 2017).
Regarding conductivity, the ecosystems examined in this study showed that albeit viral abundance had a non-significant relationship with conductivity, VPR values and PA had a significant negative and positive relationship, respectively (Table 3). Viruses and prokaryotic hosts are conditioned to their own tolerance to the habitat's salinity. In other words, high salinities can affect the permeability and structure of the prokaryotic cell wall, preventing the coupling of viruses to cells (Brown, 1964), thus reducing viral activity. Nonetheless, it has been observed that bacteriophages and archaeoviruses seem to be more tolerant to strong ionic changes, showing adaptations to saline environments (Kukkaro and Bamford, 2009). In high-altitude wetlands, viral groups belonging to Caudovirales, which mainly include viruses infecting prokaryotes, are predominant (Ramos-Barbero et al., 2019; Eissler et al., 2020) and probably highly adapted to extreme physicochemical conditions, such as significant variations in conductivity and temperatures, common to these ecosystems (Dorador et al., 2020).
In the ecosystems studied, the VPR values were also positively correlated with the concentrations of nitrate, ammonium and phosphate (Table 3). According to Weinbauer (2004), the availability of inorganic nutrients indirectly influences viral activity, through host metabolism. For example, it has been observed that adding phosphate to a microcosm experiment results in an increase of biomass, indirectly generating an increase in VPR values (Tuomi et al., 1995). Therefore, nitrate, nitrite, and phosphate assimilated by the prokaryotic communities for their metabolic processes, indirectly favors the proliferation of viruses (Breitbart et al., 2018). To support the aforementioned, an increase in VPR values has been related to the trophic gradient, finding higher values in nutrient-rich environments (Wommack and Colwell, 2000; Parikka et al., 2016). In marine environments, the high concentration of organic substrates and nutrients such as during upwelling seasons, favors the rapid development of prokaryotic communities, in turn, favoring a higher viral dynamic (Culley and Welschmeyer, 2002; Kuznar et al., 2009; Eissler et al., 2010). Conversely, it has been determined that the unavailability of nutrients results in a decrease in the VPR values in oligotrophic environments (Wommack and Colwell, 2000; Parikka et al., 2016). This has been observed in Antarctic lakes, where the lack of phosphate and other nutrients causes changes in the life strategy of viral communities, with the lysogenic cycle prevailing over the lytic cycle (Lisle and Priscu, 2004; Säwström et al., 2007), and for microorganisms inhabiting the deep-sea (Weinbauer et al., 2003; Williamson et al., 2008; Anderson et al., 2011). Consequently, among other responses, viral life strategy change is directly related to the host physiological capacity to increase their metabolism and adapt to the lack of nutrient availability (Mojica and Brussaard, 2014).
Despite the fact that our database is biased because of the predominance of marine environments, the incorporation of high-altitude wetlands and other extreme ecosystems support previously reported factors known to account for VA and VPR variability in the southern hemisphere. Conductivity and temperature gradients, as well as nutrients prevail as the main factors known to influence viral – host activity globally (Wells and Deming, 2006; Mojica and Brussaard, 2014; Finke et al., 2017).
Conclusions
In Salar de Huasco, ponds with different characteristics have a variable microbial dynamic associated with viral and prokaryotic abundance that is reflected in the VPR values that variated with the time of the day and which is probably linked to the ponds' nutrient reservoir. The high-altitude wetlands, in comparison with other ecosystems with different conditions, showed a greater range of VPR values, as a consequence of the highly diverse microbial communities hosting viruses and the physicochemical gradients that structure aquatic life conditions, generating diverse niches such as permanent brackish lagoons, ephemeral hypersaline or freshwater ponds. Metadata analyses of southern ecosystems indicate that virus and prokaryotic dynamics were understudied in wetlands and in extreme ecosystems; thus, more studies to deeply characterize the role of viruses in these types of environments are required. In addition, the comparison that we have conducted provides information on key environmental factors shaping microbial community dynamics. In general, temperature, salinity and nutrients were identified to account for viral dynamics of the different ecosystems analyzed in this study. These findings confirm the relevance of the viral dynamics in the high-altitude wetlands potentially influencing nutrient and carbon dynamics which needs to be further explored in this extreme ecosystem.
Data availability statement
The original contributions presented in the study are included in the article/Supplementary material, further inquiries can be directed to the corresponding authors.
Author contributions
YE and VM designed the study. VM, YE, PC-P, CD, and MC-D'O organized the sampling and collected samples. AC-R, YE, and VM managed data mining and analyses, interpreted the results, and wrote the manuscript. PC-P, MC-D'O, and PA contributed to the data analyses. All authors contributed substantially to manuscript revisions and read and approved the final manuscript.
Funding
This research was funded by FONDECYT, Grants Numbers 1181773 and 1211977.
Acknowledgments
We are grateful to all the participants of the field trip and particularly to Pedro and Margarita Luca for their hospitality at Salar de Huasco Shelter Facilities.
Conflict of interest
The authors declare that the research was conducted in the absence of any commercial or financial relationships that could be construed as a potential conflict of interest.
Publisher's note
All claims expressed in this article are solely those of the authors and do not necessarily represent those of their affiliated organizations, or those of the publisher, the editors and the reviewers. Any product that may be evaluated in this article, or claim that may be made by its manufacturer, is not guaranteed or endorsed by the publisher.
Supplementary material
The Supplementary Material for this article can be found online at: https://www.frontiersin.org/articles/10.3389/fmicb.2022.938066/full#supplementary-material
Supplementary Table S1. All data used in this work to obtain and compare VPR values with physicochemical and biological variables from different ecosystems in the Southern hemisphere.
Supplementary Table S2. Normality test of biological and physicochemical variables for all ecosystems of Southern Hemisphere. Data that did not comply with the assumption of normality are presented in bold (P < 0.05). K-S, Kolmogórov-Smirnov Test; W, Shapiro-Wilk Test. P, probability and N, Number of analyzed data; VPR, viral to prokaryote ratio.
Supplementary Table S3. Values of viral (VA) and prokaryotic abundance (PA), VPR (viral to prokaryote ratio) and environmental parameters (Temp, water temperature; Cond, conductivity) for PR (“Poza Rosada”) and PV (“Poza Verde”) in Salar de Huasco. SD; standard deviation.
Supplementary Table S4. Spearman rank order correlations (r) matrix for Salar de Huasco variables. Significant correlations (P < 0.05) are marked in bold, N = 7. VA, viral abundance; PA, prokaryotic abundance, VPR, viral to prokaryote ratio, Temp, temperature, Cond, conductivity.
Supplementary Table S5. Spearman rank order correlations (r) matrix for Salar de Huasco variables using all data available (Molina et al., 2018; Eissler et al., 2019, 2020). Significant correlations (P < 0.05) are marked in bold. VA, viral abundance; PA, prokaryotic abundance; VPR, viral to prokaryote ratio; Temp, temperature; Cond, conductivity. VA, PA, VPR, Nitrite, Phosphate N = 21, Cond, pH, Nitrate N = 19, Ammonium, Dissolved Oxygen N = 14, Chlorophyll a N = 7.
Supplementary Table S6. Mean values of physicochemical parameters and nutrients in different ecosystems studied. Temp, temperature; Cond, conductivity; DO, Dissolve Oxygen; Ch-a, Chlorophyll a; NA, data not available.
Supplementary Figure S1. Bubble plots analysis showing VPR distribution with depth in the different ecosystems analyzed, (A) depth from 1–1,000 m, (B) depth from 1,000–6,000 m.
References
Aguilar, P., Acosta, E., Dorador, C., and Sommaruga, R. (2016). Large differences in bacterial community composition among three nearby extreme waterbodies of the high Andean plateau. Front. Microbiol. 7, 976. doi: 10.3389/fmicb.2016.00976
Alves Junior, N., Meirelles, P. M., de Oliveira Santos, E., Dutilh, B., Silva, G. G. Z., Paranhos, R., et al. (2015). Microbial community diversity and physical–chemical features of the Southwestern Atlantic Ocean. Arch. Microbiol. 197, 165–179. doi: 10.1007/s00203-014-1035-6
Anderson, R. E., Brazelton, W. J., and Baross, J. A. (2011). Is the genetic landscape of the deep subsurface biosphere affected by viruses? Front. Microbiol. 2, 219. doi: 10.3389/fmicb.2011.00219
Anesio, A. M., and Bellas, C. M. (2011). Are low temperature habitats hot spots of microbial evolution driven by viruses? Trends Microbiol. 19, 52–57. doi: 10.1016/j.tim.2010.11.002
Atlas, E. L., Gordon, L. I., Hager, S. W., and Park, P. K. (1971). A practical manual for use of the Technicon AutoAnalyzer in seawater nutrient analyses (revised). Corvallis, OR: Tech. Rep. Department of Oceanography, School of Science, Oregon State University.
Baudoux, A. C., and Brussaard, C. P. D. (2005). Characterization of different viruses infecting the marine harmful algal bloom species Phaeocystis globosa. Virology 341, 80–90. doi: 10.1016/j.virol.2005.07.002
Bonetti, G., Trevathan-Tackett, S. M., Carnell, P., and El Macreadie, P.I. (2021). The potential of viruses to influence the magnitude of greenhouse gas emissions in an inland wetland. Water Res. 193, 116875. doi: 10.1016/j.watres.2021.116875
Boyer, J. N., Kelble, C. R., Ortner, P. B., and Rudnick, D. T. (2009). Phytoplankton bloom status: Chlorophyll a biomass as an indicator of water quality condition in the southern estuaries of Florida, USA. Ecol. Indic. 9, S56–S67. doi: 10.1016/j.ecolind.2008.11.013
Breitbart, M., Bonnain, C., Malki, K., and Sawaya, N. A. (2018). Phage puppet masters of the marine microbial realm. Nat. Microbiol. 3, 754–766. doi: 10.1038/s41564-018-0166-y
Breitbart, M., and Rohwer, F. (2005). Here a virus, there a virus, everywhere the same virus? Trends Microbiol. 13, 278–284. doi: 10.1016/j.tim.2005.04.003
Brown, A. D. (1964). Aspects of bacterial response to the ionic environment. Bacteriol. Rev. 28, 296–329. doi: 10.1128/br.28.3.296-329.1964
Brum, J. R., Hurwitz, B. L., Schofield, O., Ducklow, H. W., and Sullivan, M. B. (2017). Seasonal time bombs: dominant temperate viruses affect Southern Ocean microbial dynamics. ISME J. 11, 588. doi: 10.1038/ismej.2016.126
Bull, A. T., and Asenjo, J. A. (2013). Microbiology of hyper-arid environments: recent insights from the Atacama Desert, Chile. Antonie van Leeuwenhoek, Int. J. Gen. Mol. Microbiol. 103, 1173–1179. doi: 10.1007/s10482-013-9911-7
Carrasco-Lagos, P., Moreno, R. A., Figueroa, A., and Espoz y C de la Maza, C. (2015). Sitios Ramsar de Chile. Seremi del Medio Ambiente Región Metropolitana de Santiago, Universidad Santo Tomás, Universidad de Chile y CONAF. p. 56.
Carreira, C., Carvalho, J. P. S., Talbot, S., Pereira, I., and Lønborg, C. (2020). Small-scale distribution of microbes and biogeochemistry in the Great Barrier Reef. PeerJ. 8, 1–24. doi: 10.7717/peerj.10049
Caspers, H. (1970). J. D. H. Strickland and T. R. Parsons: a practical handbook of seawater analysis. Ottawa: Fisheries Research Board of Canada, Bulletin 167, 1968. 293 pp. $ 7.50. Int. Rev. der gesamten Hydrobiol. und Hydrogr. 55, 167. doi: 10.1002/iroh.19700550118
Cassman, N., Prieto-Davó, A., Walsh, K., Silva, G. G. Z., Angly, F., Akhter, S., et al. (2012). Oxygen minimum zones harbour novel viral communities with low diversity. Environ. Microbiol. 14, 3043–3065. doi: 10.1111/j.1462-2920.2012.02891.x
Chen, F., Lu, J.-R., Binder, B. J., Liu, Y.-C., and Hodson, R. E. (2001). Application of digital image analysis and flow cytometry to enumerate marine viruses stained with SYBR gold application of digital image analysis and flow cytometry to enumerate marine viruses stained with SYBR gold. Appl. Environ. Microbiol. 67, 539–545. doi: 10.1128/AEM.67.2.539-545.2001
Chiang, O. E., and Quiñones, R. A. (2007). Relationship between viral and prokaryotic abundance on the Bajo O'Higgins 1 Seamount (Humboldt Current System off Chile). Sci. Mar. 71, 37–46. doi: 10.3989/scimar.2007.71n137
Chinn, T. J. (1993). Physical hydrology of the dry valley lakes. Phys. Biogeochem. Process. Antarct. lakes 59, 1–51. doi: 10.1029/AR059p0001
Clasen, J. L., Brigden, S. M., Payet, J. P., and Suttle, C. A. (2008a). Evidence that viral abundance across oceans and lakes is driven by different biological factors. Freshw. Biol. 53, 1090–1100.
Clasen, J. L., Brigden, S. M., Payet, J. P., and Suttle, C. A. (2008b). Evidence that viral abundance across oceans and lakes is driven by different biological factors. Freshw. Biol. 53, 1090–1100. doi: 10.1111/j.1365-2427.2008.01992.x
Culley, A. I., and Welschmeyer, N. A. (2002). The abundance, distribution, and correlation of viruses, phytoplankton, and prokaryotes along a Pacific Ocean transect. Limnol. Oceanogr. 47, 1508–1513. doi: 10.4319/lo.2002.47.5.1508
De Corte, D., Martínez, J. M., Cretoiu, M. S., Takaki, Y., Nunoura, T., Sintes, E., et al. (2019). Viral communities in the global deep ocean conveyor belt assessed by targeted viromics. Front. Microbiol. 10, 1–14. doi: 10.3389/fmicb.2019.01801
De Corte, D., Sintes, E., Yokokawa, T., Lekunberri, I., and Herndl, G. J. (2016). Large-scale distribution of microbial and viral populations in the South Atlantic Ocean. Environ. Microbiol. Rep. 8, 305–315. doi: 10.1111/1758-2229.12381
de la Fuente, A., and Niño, Y. (2010). Temporal and spatial features of the thermohydrodynamics of shallow salty lagoons in northern Chile. Limnol. Oceanogr. 55, 279–288. doi: 10.4319/lo.2010.55.1.0279
Dorador, C., Molina, V., Hengst, M., Eissler, Y., Cornejo, M., Fernández, C., et al. (2020). Microbial Communities composition, activity, and dynamics at salar de huasco: a polyextreme environment in the chilean altiplano. In: Farías, M. E., editor. Microbial Ecosystems in Central Andes Extreme Environments. New York, NY: Springer International Publishing. p. 123–139.
Dorador, C., Vila, I., Imhoff, J. F., and Witzel, K. P. (2008). Cyanobacterial diversity in Salar de Huasco, a high altitude saline wetland in northern Chile: an example of geographical dispersion? FEMS Microbiol. Ecol. 64, 419–432. doi: 10.1111/j.1574-6941.2008.00483.x
Dorador, C., Vila, I., Witzel, K.-P., and Imhoff, J. F. (2013). Bacterial and archaeal diversity in high altitude wetlands of the Chilean Altiplano. Fundam. Appl. Limnol. 182, 135–159. doi: 10.1127/1863-9135/2013/0393
Eissler, Y., Dorador, C., Kieft, B., Molina, V., and Hengst, M. (2020). Virus and potential host microbes from viral-enriched metagenomic characterization in the high-altitude wetland, Salar de Huasco, Chile. Microorganisms 8, 1–15. doi: 10.3390/microorganisms8071077
Eissler, Y., Gálvez, M. J., Dorador, C., Hengst, M., and Molina, V. (2019). Active microbiome structure and its association with environmental factors and viruses at different aquatic sites of a high-altitude wetland. Microbiologyopen 8, 1–13. doi: 10.1002/mbo3.667
Eissler, Y., Letelier, J., Cuevas, L. A., Morales, C. E., and Escribano, R. (2010). The microbial community in the coastal upwelling system off Concepción, Chile, 36°S, 2002-2003 period. Rev. Biol. Mar. Oceanogr. 45, 1–18. doi: 10.4067/S0718-19572010000100001
Evans, C., Pearce, I., and Brussaard, C. P. D. (2009). Viral-mediated lysis of microbes and carbon release in the sub-Antarctic and polar frontal zones of the Australian Southern Ocean. Environ. Microbiol. 11, 2924–2934. doi: 10.1111/j.1462-2920.2009.02050.x
Finke, J. F., Hunt, B. P. V., Winter, C., Carmack, E. C., and Suttle, C. A. (2017). Nutrients and other environmental factors influence virus abundances across oxic and hypoxic marine environments. Viruses 9, 152. doi: 10.3390/v9060152
Flynn, K. J., Mitra, A., Wilson, W. H., Kimmance, S. A., Clark, D. R., Pelusi, A., et al. (2022). ‘Boom-and-busted' dynamics of phytoplankton–virus interactions explain the paradox of the plankton. New Phytol. 234, 990–1002. doi: 10.1111/nph.18042
Fuhrman, J. A. (1999). Marine viruses and their biogeochemical and ecological effects. Nature 399, 1–8.
Gao, Y., Lu, Y., Dungait, J. A. J., Liu, J., Lin, S., Jia, J., et al. (2022). The “Regulator” function of viruses on ecosystem carbon cycling in the anthropocene. Front. Public Heal. 10, 1–14. doi: 10.3389/fpubh.2022.858615
Griffin, D. W. (2013). The quest for extraterrestrial life: what about the viruses? Astrobiology 13, 774–783. doi: 10.1089/ast.2012.0959
Haferburg, G., Gröning, J. A. D., Schmidt, N., Kummer, N.-A., Erquicia, J. C., and Schlömann, M. (2017). Microbial diversity of the hypersaline and lithium-rich Salar de Uyuni, Bolivia. Microbiol. Res. 199, 19–28. doi: 10.1016/j.micres.2017.02.007
Heldal, M., and Bratbak, G. (1991). Production and decay of viruses in aquatic environments. Mar. Ecol. Prog. Ser. 72, 205–212. doi: 10.3354/meps072205
Hernández, K. L., Yannicelli, B., Olsen, L. M., Dorador, C., Menschel, E. J., Molina, V., et al. (2016). Microbial activity response to solar radiation across contrasting environmental conditions in Salar de Huasco, northern Chilean altiplano. Front. Microbiol. 7, 1–13. doi: 10.3389/fmicb.2016.01857
Jackson, E. F., and Jackson, C. R. (2008). Viruses in wetland ecosystems. Freshw. Biol. 53, 1214–1227. doi: 10.1111/j.1365-2427.2007.01929.x
Kukkaro, P., and Bamford, D. H. (2009). Virus–host interactions in environments with a wide range of ionic strengths. Environ. Microbiol. Rep. 1, 71–77. doi: 10.1111/j.1758-2229.2008.00007.x
Kuznar, J., Espinoza, J. C., Avaria, S., Valenzuela, M., and Pavez, C. (2009). Efectos microbiológicos producidos por un evento de surgencia costera en Chile central. Rev. Biol. Mar. Oceanogr. 44, 189–196. doi: 10.4067/S0718-19572009000100019
Lara, E., Vaqué, D., Sà, E. L., Boras, J. A., Gomes, A., Borrull, E., et al. (2017). Unveiling the role and life strategies of viruses from the surface to the dark ocean. Sci. Adv. 3:e1602565. doi: 10.1126/sciadv.1602565
Laybourn-Parry, J., Marshall, W. A., and Madan, N. J. (2007). Viral dynamics and patterns of lysogeny in saline Antarctic lakes. Polar Biol. 30, 351–358. doi: 10.1007/s00300-006-0191-9
Letelier, J., Pizarro, O., and Nuñez, S. (2009). Seasonal variability of coastal upwelling and the upwelling front off central Chile. J. Geophys. Res. Ocean. 114, C12009. doi: 10.1029/2008JC005171
Lisle, J. T., and Priscu, J. C. (2004). The occurrence of lysogenic bacteria and microbial aggregates in the lakes of the McMurdo Dry Valleys, Antarctica. Microb. Ecol. 47, 427–439. doi: 10.1007/s00248-003-1007-x
Márquez-García, M., Vila, I., Hinojosa, L. F., Méndez, M. A., Carvajal, J. L., and Sabando, M. C. (2009). Distribution and seasonal fluctuations in the aquatic biodiversity of the southern Altiplano. Limnologica 39, 314–318. doi: 10.1016/j.limno.2009.06.007
McKay, C. P., Clow, G. D., Wharton, R. A., and Squyres, S. W. (1985). Thickness of ice on perennially frozen lakes. Nature 313, 561–562. doi: 10.1038/313561a0
Mojica, K. D. A., and Brussaard, C. P. D. (2014). Factors affecting virus dynamics and microbial host-virus interactions in marine environments. FEMS Microbiol. Ecol. 89, 495–515. doi: 10.1111/1574-6941.12343
Molina, V., Eissler, Y., Cornejo, M., Galand, P. E., Dorador, C., Hengst, M., et al. (2018). Distribution of greenhouse gases in hyper-arid and arid areas of northern Chile and the contribution of the high altitude wetland microbiome (Salar de Huasco, Chile). Antonie van Leeuwenhoek, Int. J. Gen. Mol. Microbiol. 111, 1421–1432. doi: 10.1007/s10482-018-1078-9
Molina, V., Eissler, Y., Fernandez, C., Cornejo-D'Ottone, M., Dorador, C., Bebout, B. M., et al. (2020). Greenhouse gases and biogeochemical diel fluctuations in a high-altitude wetland. Sci. Total Environ. 768, 144370. doi: 10.1016/j.scitotenv.2020.144370
Noble, R. T., and Fuhrman, J. A. (2000). Rapid virus production and removal as measured with fluorescently labeled viruses as tracers. Appl. Environ. Microbiol. 66, 3790–3797. doi: 10.1128/AEM.66.9.3790-3797.2000
Parikka, K. J., Le Romancer, M., Wauters, N., and Jacquet, S. (2016). Deciphering the virus-to-prokaryote ratio (VPR): insights into virus–host relationships in a variety of ecosystems. Biol. Rev. 92, 1081–1100. doi: 10.1111/brv.12271
Payet, J. P., McMinds, R., Burkepile, D. E., and Vega Thurber, R. L. (2014). Unprecedented evidence for high viral abundance and lytic activity in coral reef waters of the South Pacific Ocean. Front. Microbiol. 5, 493. doi: 10.3389/fmicb.2014.00493
R Core Team (2021). R: A Language and Environment for Statistical Computing. Vienna: R Foundation for Statistical Computing.
Ramos-Barbero, M. D., Martínez, J. M., Almansa, C., Rodríguez, N., Villamor, J., Gomariz, M., et al. (2019). Prokaryotic and viral community structure in the singular chaotropic salt lake Salar de Uyuni. Environ. Microbiol. 21, 2029–2042. doi: 10.1111/1462-2920.14549
Rettig, S. L., Jones, B. F., and Risacher, F. (1980). Geochemical evolution of brines in the Salar of Uyuni, Bolivia. Chem. Geol. 30, 57–79. doi: 10.1016/0009-2541(80)90116-3
Risacher, F., Alonso, H., and Salazar, C. (2003). The origin of brines and salts in Chilean salars: A hydrochemical review. Earth-Science Rev. 63, 249–293. doi: 10.1016/S0012-8252(03)00037-0
Risacher, F., and Fritz, B. (2009). Origin of salts and brine evolution of bolivian and chilean salars. Aquat. Geochemistry 15, 123–157. doi: 10.1007/s10498-008-9056-x
Salter, I., Böttjer, D., and Christaki, U. (2011). The effect of inorganic particle concentration on bacteria–virus–nanoflagellate dynamics. Environ. Microbiol. 13, 2768–2777. doi: 10.1111/j.1462-2920.2011.02547.x
Säwström, C., Anesio, M. A., Granéli, W., and Laybourn-Parry, J. (2007). Seasonal viral loop dynamics in two large ultraoligotrophic Antarctic freshwater lakes. Microb. Ecol. 53, 1–11. doi: 10.1007/s00248-006-9146-5
Smith Jr, W. O., Marra, J., Hiscock, M. R., and Barber, R. T. (2000). The seasonal cycle of phytoplankton biomass and primary productivity in the Ross Sea, Antarctica. Deep Sea Res. Part II Top. Stud. Oceanogr. 47, 3119–3140. doi: 10.1016/S0967-0645(00)00061-8
Sotomayor-Garcia, A., Sala, M. M., Ferrera, I., Estrada, M., Vázquez-Domínguez, E., Emelianov, M., et al. (2020). Assessing viral abundance and community composition in four contrasting regions of the Southern Ocean. Life 10, 1–20. doi: 10.3390/life10070107
Srinivasiah, S., Bhavsar, J., Thapar, K., Liles, M., Schoenfeld, T., and Wommack, K. E. (2008). Phages across the biosphere: contrasts of viruses in soil and aquatic environments. Res. Microbiol. 159, 349–357. doi: 10.1016/j.resmic.2008.04.010
Steward, G. F., Smith, D. C., and Azam, F. (1996). Abundance and production of bacteria and viruses in the Bering and Chukchi Seas. Mar. Ecol. Prog. Ser. 131, 287–300. doi: 10.3354/meps131287
Strickland, J. D. H., and Parsons, T. R. (1972). A Practical Handbook of Seawater Analysis. Nanaimo, BC: Fisheries research board of Canada.
Suttle, C. A. (1994). The significance of viruses to mortality in aquatic microbial communities. Microb. Ecol. 28, 237–243. doi: 10.1007/BF00166813
Suttle, C. A. (2007). Marine viruses-major players in the global ecosystem. Nat. Rev. Microbiol. 5, 801–812. doi: 10.1038/nrmicro1750
Syvanen, M. (2012). Evolutionary implications of horizontal gene transfer. Annu. Rev. Genet. 46, 341–358. doi: 10.1146/annurev-genet-110711-155529
Taylor, G. T., Hein, C., and Iabichella, M. (2003). Temporal variations in viral distributions in the anoxic Cariaco Basin. Aquat. Microb. Ecol. 30, 103–116. doi: 10.3354/ame030103
Tréguer, P., and Jacques, G. (1992). Review Dynamics of nutrients and phytoplankton, and fluxes of carbon, nitrogen and silicon in the Antarctic Ocean. In: Weddell Sea Ecology. Ney York: Springer. p. 149–162.
Tuomi, P., Fagerbakke, K. M., Bratbak, G., and Heldal, M. (1995). Nutritional enrichment of a microbial community: the effects on activity, elemental composition, community structure and virus production. FEMS Microbiol. Ecol. 16, 123–134. doi: 10.1111/j.1574-6941.1995.tb00276.x
Ulloa, O., Canfield, D. E., DeLong, E. F., Letelier, R. M., and Stewart, F. J. (2012). Microbial oceanography of anoxic oxygen minimum zones. Proc. Natl. Acad. Sci. USA. 109, 15996–16003. doi: 10.1073/pnas.1205009109
Vaqué, D., Boras, J. A., Torrent-Llagostera, F., Agustí, S., Arrieta, J. M., Lara, E., et al. (2017). Viruses and protists induced-mortality of prokaryotes around the Antarctic peninsula during the Austral summer. Front. Microbiol. 8, 1–12. doi: 10.3389/fmicb.2017.00241
Wagner, R., Boulger, R., Oblinger, C., and Smith, B. (2006). Guidelines and Standard Procedures for Continuous Water-Quality Monitors: Station Operation, Record Computation, and Data Reporting. Techniques and Methods 1–D3. Report, USGS Numbered Series. p. 51. doi: 10.3133/tm1D3
Weinbauer, M. G. (2004). Ecology of prokaryotic viruses. FEMS Microbiol. Rev. 28, 127–181. doi: 10.1016/j.femsre.2003.08.001
Weinbauer, M. G., Brettar, I., and Höfle, M. G. (2003). Lysogeny and virus-induced mortality of bacterioplankton in surface, deep, and anoxic marine waters. Limnol. Oceanogr. 48, 1457–1465. doi: 10.4319/lo.2003.48.4.1457
Weitz, J. S., Stock, C. A., Wilhelm, S. W., Bourouiba, L., Coleman, M. L., Buchan, A., et al. (2015). A multitrophic model to quantify the effects of marine viruses on microbial food webs and ecosystem processes. Isme J. 9, 1352. doi: 10.1038/ismej.2014.220
Wells, L. E., and Deming, J. W. (2006). Effects of temperature, salinity and clay particles on inactivation and decay of cold-active marine Bacteriophage 9A. Aquat. Microb. Ecol. 45, 31–39. doi: 10.3354/ame045031
Wigington, C. H., Sonderegger, D., Brussaard, C. P. D., Buchan, A., Finke, J. F., Fuhrman, J. A., et al. (2016). Re-examination of the relationship between marine virus and microbial cell abundances. Nat. Microbiol. 1. doi: 10.1038/nmicrobiol.2015.24
Wilhelm, S. W., Brigden, S. M., and Suttle, C. A. (2002). A dilution technique for the direct measurement of viral production: a comparison in stratified and tidally mixed coastal waters. Microb. Ecol. 43, 168–173. doi: 10.1007/s00248-001-1021-9
Williamson, S. J., Cary, S. C., Williamson, K. E., Helton, R. R., Bench, S. R., Winget, D., et al. (2008). Lysogenic virus–host interactions predominate at deep-sea diffuse-flow hydrothermal vents. ISME J. 2, 1112–1121. doi: 10.1038/ismej.2008.73
Winget, D. M., and Wommack, K. E. (2009). Diel and daily fluctuations in virioplankton production in coastal ecosystems. Environ. Microbiol. 11, 2904–2914. doi: 10.1111/j.1462-2920.2009.02038.x
Wommack, K. E., and Colwell, R. R. (2000). Virioplankton: viruses in aquatic ecosystems. Microbiol. Mol. Biol. Rev. 64, 69–114. doi: 10.1128/MMBR.64.1.69-114.2000
Keywords: virus, bacteria, prokaryote, relationships, aquatic ecosystems, VBR, high-altitude wetland, VPR
Citation: Eissler Y, Castillo-Reyes A, Dorador C, Cornejo-D'Ottone M, Celis-Plá PSM, Aguilar P and Molina V (2022) Virus-to-prokaryote ratio in the Salar de Huasco and different ecosystems of the Southern hemisphere and its relationship with physicochemical and biological parameters. Front. Microbiol. 13:938066. doi: 10.3389/fmicb.2022.938066
Received: 06 May 2022; Accepted: 31 July 2022;
Published: 18 August 2022.
Edited by:
Matthew John Wade, UK Health Security Agency (UKHSA), United KingdomReviewed by:
Pavla Debeljak, University of Vienna, AustriaVarada Khot, University of Calgary, Canada
Copyright © 2022 Eissler, Castillo-Reyes, Dorador, Cornejo-D'Ottone, Celis-Plá, Aguilar and Molina. This is an open-access article distributed under the terms of the Creative Commons Attribution License (CC BY). The use, distribution or reproduction in other forums is permitted, provided the original author(s) and the copyright owner(s) are credited and that the original publication in this journal is cited, in accordance with accepted academic practice. No use, distribution or reproduction is permitted which does not comply with these terms.
*Correspondence: Yoanna Eissler, yoanna.eissler@uv.cl; Verónica Molina, veronica.molina@upla.cl