- 1Molecular Enzyme Technology and Biochemistry, Environmental Microbiology and Biotechnology, Centre for Water and Environmental Research, University of Duisburg-Essen, Essen, Germany
- 2Applied Analytical Chemistry, University of Duisburg-Essen, Essen, Germany
- 3Teaching and Research Center for Separation, University of Duisburg-Essen, Essen, Germany
- 4Aquatic Microbiology, Environmental Microbiology and Biotechnology, Centre for Water and Environmental Research, University of Duisburg-Essen, Essen, Germany
Extracellular polymeric substances (EPS) comprise mainly carbohydrates, proteins and extracellular DNA (eDNA) in biofilms formed by the thermoacidophilic Crenarchaeon Sulfolobus acidocaldarius. However, detailed information on the carbohydrates in the S. acidocaldarius biofilm EPS, i.e., the exopolysaccharides (PS), in terms of identity, composition and size were missing. In this study, a set of methods was developed and applied to study the PS in S. acidocaldarius biofilms. It was initially shown that addition of sugars, most significantly of glucose, to the basal N-Z-amine-based growth medium enhanced biofilm formation. For the generation of sufficient amounts of biomass suitable for chemical analyses, biofilm growth was established and optimized on the surface of membrane filters. EPS were isolated and the contents of carbohydrates, proteins and eDNA were determined. PS purification was achieved by enzymatic digestion of other EPS components (nucleic acids and proteins). After trifluoroacetic acid-mediated hydrolysis of the PS fraction, the monosaccharide composition was analyzed by reversed-phase liquid chromatography (RP-LC) coupled to mass spectrometry (MS). Main sugar constituents detected were mannose, glucose and ribose, as well as minor proportions of rhamnose, N-acetylglucosamine, glucosamine and galactosamine. Size exclusion chromatography (SEC) revealed the presence of one single PS fraction with a molecular mass of 4-9 × 104 Da. This study provides detailed information on the PS composition and size of S. acidocaldarius MW001 biofilms and methodological tools for future studies on PS biosynthesis and secretion.
Introduction
In nature, the majority of microorganisms is estimated to exist in a “continuum of aggregations” as flocs, sludges, or films, called biofilms (Moshynets and Spiers, 2016; Flemming and Wuertz, 2019). They are defined as aggregates of microorganisms that usually accumulate at interfaces or aggregate with themselves and that are encased in a self-produced matrix of hydrated extracellular polymeric substances (EPS) (Flemming and Wingender, 2010). EPS comprise biopolymers such as exopolysaccharides (PS), proteins, glycoproteins, nucleic acids, and lipids.
In total, the matrix of EPS builds the scaffold for the biofilm structure and enables surface adhesion, structural and mechanical stability and cohesion as well as the close proximity of cells. As a protecting “house of biofilm cells” (Flemming et al., 2007) it increases cells’ tolerance to antimicrobials and other environmental stresses, e.g., pH or temperature shifts, and the tolerance to organic solvents (Koerdt et al., 2010; Benninghoff et al., 2021). Each of the EPS components contributes to the functionality and stability of the matrix, and each of them is assigned to a set of distinct functions. PS are ubiquitous in the biofilm matrix. Bacterial PS have been reported to be homo- and more frequently heteropolysaccharides with molecular mass ranging from approximately 2.0 × 104 to 1.9 × 107 Da (Mata et al., 2006, 2008; Sutherland, 2017; Wang et al., 2019). PS display a high diversity in their composition of neutral and charged carbohydrate residues which can contain organic and inorganic substituents (Sutherland, 2017). Multiple functions of PS include adhesion of cells to interfaces (initial colonization and long-term attachment of biofilms to surfaces), cell aggregation, cohesion of biofilms by forming a hydrated polymer network determining structure and architecture of biofilms, and protection from diverse external stresses such as desiccation, antimicrobial compounds, host immune defenses or predation by protozoa (Flemming and Wingender, 2010; Limoli et al., 2015). EPS proteins share some functions with PS, and additionally exhibit unique properties, for instance, their enzymatic activities that enable modification of EPS and digestion of extracellular macromolecules for nutrient acquisition (Flemming and Wingender, 2010). eDNA supports adhesion, aggregation as well as cohesion (Whitchurch et al., 2002) and enables horizontal gene transfer (Flemming, 2016). Biofilm structure, integrity and biology depend not only on EPS composition, but also on functional interactions between PS, proteins and eDNA, sometimes in conjunction with other substances (e.g., signaling molecules, mutivalent inorganic ions) in the biofilm matrix. Functional interactions result, for example, in promoting cell aggregation, enhancing stability of the EPS matrix, or retaining and stabilizing extracellular matrix enzymes (Flemming and Wingender, 2010; Tielen et al., 2013; Sutherland, 2017; Reichhardt et al., 2020). Functional interactions essentially contribute to the generation of emergent properties of biofilms (Flemming et al., 2016).
Information on the molecular composition and chemical as well as physical properties of EPS is limited and mostly reported for bacterial monospecies biofilms of clinical relevance (Seviour et al., 2019). Here, the variety of EPS biopolymers and the difficulty in extracting and analyzing them, display challenging hurdles to overcome. Further, both the ratios of EPS polymers as well as their composition change depending on environmental conditions. Cells actively modify the excreted EPS of their matrix along the biofilm life cycle, remodeling their close environment as a function of time and environmental conditions (Flemming, 2011; Seviour et al., 2019).
While methods for high-throughput protein identification are well established, PS analysis methods lack mostly due to the high structural diversity of carbohydrates (Seviour et al., 2019). The complexity of PS analyses is increased by the fact that one strain may produce diverse PS molecules (Ryder et al., 2007). Initially, the EPS matrix composition is typically determined by biochemical, mostly colorimetric, assays that enable the calculation of component sum parameters and their ratios (Felz et al., 2019). The in-depth analysis of EPS components might require complex sample preparation and a significant quantity of a “sufficiently pure compound” (Seviour et al., 2019). For PS size determination gel permeation chromatography/size exclusion chromatography (GPC/SEC) (Simon et al., 2009; Ras et al., 2011) is typically applied. Determination of the composition requires cleavage of the PS into its monomers, which is usually accomplished by acid hydrolysis. A wide range of methods has been published, such as high-performance liquid chromatography (HPLC) (Qamar et al., 2021), high-performance anion-exchange chromatography (HPAEC) (Zhang et al., 2019), capillary electrophoresis (CE) (Gao et al., 2010), and GC after derivatization (Parra-Riofrío et al., 2020).
Compared to bacterial biofilms, substantially less information is available on the formation, regulation, EPS synthesis as well as composition of archaeal biofilms. Archaea were shown to be ubiquitous in nature and are reported to form biofilms as their predominant lifestyle (van Wolferen et al., 2018). Most cultivable archaeal species to date are extremophilic organisms that are adapted to extreme environmental conditions such as extremes of temperature, pH, salt, or combinations thereof. Aggregates of methanogenic archaea, Halobacterium, Haloferax and members of the Sulfolobales display the best-studied archaeal biofilms (van Wolferen et al., 2018). Sulfolobus acidocaldarius is adapted to both high temperature (75–80°C) and low pH values (pH 2–3), and forms complex, dense carpet-like biofilms with tower like structures (Koerdt et al., 2010). The extracellular proteome of S. acidocaldarius DSM639 biofilms was proven to comprise numerous enzyme classes, including proteases, lipases, esterases, phosphatases and glucosidases (Jachlewski et al., 2015). In contrast, limited information is available on S. acidocaldarius PS. Extracellular carbohydrates were visualized by confocal laser scanning microscopy (Koerdt et al., 2010; Benninghoff et al., 2021). Here, interactions of fluorophore-labeled lectins to PS structures indicated the presence of glucose and/or mannose, as well as lower amounts of galactose and/or N-acetyl-D-galactosamine residues. However, the complete and precise monomeric composition and size of the S. acidocaldarius PS were not studied so far by chemical analytical techniques.
This study fills the gap of knowledge on S. acidocaldarius EPS matrix composition with a special focus on the size of excreted PS as well as the identity of their constituent monosaccharides as a basis for the investigation of PS function for the development and integrity of archaeal biofilms.
Materials and methods
Strain and growth conditions
Sulfolobus acidocaldarius MW001 was used to generate biofilms. The strain is a deletion mutant of S. acidocaldarius DSM639, lacking 322 bp of pyrE (Wagner et al., 2012), enabling genetic selection and engineering via uracil auxotrophy. The strain was cultivated aerobically in basal Brock medium (Brock et al., 1972), supplemented with 0.1% (w/v) N-Z-amine (NZA; casein enzymatic hydrolysate N-Z-Amine® AS, Sigma-Aldrich, Darmstadt, Germany), 10 μg/mL uracil (min. 99%, Sigma-Aldrich) and different additional growth substrates at 76°C and pH 3.0 (adjusted with 50% (v/v) sulfuric acid): (i) 0.2% (w/v) D(+)-glucose (p.a., ACS, Carl Roth, Karlsruhe, Germany), (ii) 0.2% (w/v) D-xylose (min. 99%, Carl Roth), and (iii) 0.2% (w/v) D(+)-maltose (biochemical grade, Gerbu, Heidelberg, Germany). Cell growth was monitored by turbidity measurements at 600 nm.
Microtiter plate assay
To evaluate biofilm formation of S. acidocaldarius in dependency of different growth substrates, cells were grown in 24-well microtiter plates (MTP) (Cell+, flat bottom, polystyrene, Sarstedt, Nümbrecht, Germany). Each well was filled with 2 mL culture volume of an optical density at 600 nm (OD600nm) of 0.01. Plates were sealed with aluminum foil (aluminum sealing tape, pierceable, Greiner Bio-One, Frickenhausen, Germany) to limit medium evaporation. The foil was pierced once with a sterile needle for each well to ensure oxygen supply. Plates were cultivated for 1–5 days under static conditions at 76°C. After incubation, one set of MTP was used to determine total turbidity (OD600nm) values of biofilm and planktonic cells after extensive mixing of cultures (Biophotometer plus, Eppendorf, Hamburg, Germany). Another set of MTP was used to measure OD600nm values of planktonic cells only. The remaining biofilm biomass in each well was quantified by crystal violet (CV) staining. Briefly, wells were washed once with Brock medium (pH 3.0) to remove planktonic cells. Then, 2.5 mL of 0.1% (w/v) crystal violet solution were added into each cavity, incubated for 30 min at RT and washed five times with 2.5 mL dH2O. After air-drying, each well was filled with 2 mL of 95% (v/v) ethanol and the plates were incubated for 30 min at RT to release the dye from biofilm into solution. The absorbance of crystal violet was measured at 595 nm (Biophotometer plus, Eppendorf). Medium samples served as negative controls and CV values of medium controls were subtracted from CV values of S. acidocaldarius biofilms. Ratios of biofilm vs. planktonic cells were calculated by dividing the absorbance of crystal violet (OD595nm) by growth of planktonic cells (OD600nm values) (Koerdt et al., 2010). All experiments were performed in quadruplicates.
Determination of total cell counts
To determine total cell counts of S. acidocaldarius MW001 cultures and cell suspensions the correlation between total cell counts and OD600nm values was determined using the improved Neubauer counting chamber (0.0025 mm2, 0.020 mm depth, Brand, Wertheim, Germany) by phase-contrast microscopy (Leica DMLS bench microscope, Leica, Wetzlar, Germany). S. acidocaldarius MW001 liquid cultures were grown in Brock medium, pH 3.0, supplemented with 0.1% (w/v) NZA, 0.2% (w/v) D-glucose, 10 μg/mL uracil at 75°C and 180 rpm. Cultures were 10- to 40-fold diluted with 70% (v/v) sterile glycerol to enable a reliable counting of the cells under the microscope. For each dilution, cell numbers in six different squares were counted. Cell counts and corresponding OD600nm values were plotted.
Generation of Sulfolobus acidocaldarius MW001 biofilms: Membrane filtration and cultivation
To generate S. acidocaldarius biofilms, cells were pregrown for three passages in liquid Brock medium containing 0.1% (w/v) NZA, 10 μg/mL uracil and optionally 0.2% (w/v) D-glucose, D-xylose, or maltose, pH 3.0, at 180 rpm and 75°C. Cells from mid-exponential growth phase (OD600nm approx. 0.6-0.8) were then diluted with Brock medium yielding a total cell number of 5.45 × 104 cells/mL. Eleven mL of this culture dilution were filtered through filter membranes composed of different materials (summarized in Supplementary Table 1) using a stainless steel filtration system (Sartorius AG, Goettingen, Germany) connected to a vacuum pump (Liquiport, KNF Neuberger, Trenton, NJ, USA). Finally, highest biofilm yields were obtained with hydrophilic polytetrafluoroethylene (PTFE) membranes (PTFE polymer membrane bound to a high density polyethylene support, Omnipore membrane, 0.45 μm pore size, Ref.no. JHWP04700, Merck-Millipore, Darmstadt, Germany), which were further on used as standard membrane for biofilm cultivations. After filtration, the membranes were placed on the surface of 30 mL liquid Brock medium in Petri dishes (92 mm × 16 mm, polystyrene, without cams, Sarstedt) or on top of solidified Brock medium plates in initial experiments (Supplementary Table 1; 0.6% (w/v) gellan gum, Serva Electrophoresis, Heidelberg, Germany) supplemented with the different sugars as mentioned above. Petri dishes were statically incubated at 76°C for 4 days. Biofilms formed on the surface of membranes. For further EPS/PS extraction and analyses, the biofilm mass from 10 membranes was harvested and pooled. Negative controls for RP-LC-MS and SEC analyses were generated by the filtration of medium and subsequent incubation.
Isolation of extracellular polymeric substances
After cultivation, biofilms were harvested from membranes using a cell scraper (non-cytotoxic, non-pyrogenic, sterile, blade width: 1.70 cm, blade material: thermoplastic elastomers, Sarstedt). EPS extraction was performed using a cation exchange resin (CER, Dowex® Marathon® C sodium form, Sigma-Aldrich; according to Jachlewski et al., 2015). Briefly, biofilm cells were suspended in phosphate buffer (10 mg biofilm wet weight/mL buffer; 2 mM Na3PO4 × 12 H2O, 4 mM NaH2PO4 x 1 H2O, 9 mM NaCl, 1 mM KCl, pH 7.0). Ten milliliter biofilm suspension were treated with 2 g of hydrated CER for 20 min under shaking conditions. Centrifugation and sterile filtration of the supernatant (polyvinylidene fluoride (PVDF) filter Rotilabo, pore size 0.22 μm, Carl Roth) yielded the fraction of total extracellular material (TEM), containing cell-free low and high molecular weight compounds. To obtain the fraction of high molecular weight compounds only (EPS), the TEM sample was dialyzed against Milli-Q water using a membrane tube with a molecular weight cut-off (MWCO) of 3.5 kDa (Spectra/Por3, Standard RC Tubing Dialysis Membrane, ø11.5 mm, Spectrum Laboratories, Waltham, MA, USA) (Jachlewski et al., 2015). As negative controls for RP-LC-MS and SEC analyses medium samples from membrane filtration were used (see section “Generation of Sulfolobus acidocaldarius MW001 biofilms: Membrane filtration and cultivation”). Despite the absence of biofilms, the protocol for EPS isolation was followed as described for biofilm samples.
Quantification of EPS components
Carbohydrates and proteins were quantified in biofilm suspensions, TEM and EPS samples as described by Jachlewski et al. (2015). Proteins were quantified according to a modified Lowry assay (Peterson, 1977) using bovine serum albumin as standard (Serva Electrophoresis; calibration ranging from 0 to 100 μg/mL) (Wingender et al., 2001). Carbohydrates were quantified using the phenol/sulphuric acid method (Dubois et al., 1956) with D-glucose as standard (p.a., ACS, Carl Roth; calibration ranging from 0 to 200 μg/mL). eDNA was quantified using the Qubit™ dsDNA HS Assay Kit and the Qubit™ 3 Fluorometer (Invitrogen, Thermo Fisher Scientific, Waltham, Massachusetts, USA).
Spotting plates
Biofilms were grown on membrane filters for 4 days at 76°C. Then, biofilms were harvested from the filter using a cell scraper (Sarstedt) and resuspended in phosphate buffer as described in section “Isolation of extracellular polymeric substances”. Biofilm suspensions were diluted in a 10-fold dilution series (10–1–10–7) using Brock medium, pH 5.0–5.5. Ten microliter of biofilm suspensions and dilutions were spotted on Brock-gellan gum plates containing 0.6% (w/v) gellan gum (Gelzan, Sigma-Aldrich), 0.1% (w/v) NZA, 0.2% (w/v) D-glucose and 10 μg/mL uracil. Plates were incubated for 5 days at 76°C. Culturability was documented by camera.
Acid hydrolysis of exopolysaccharides
The hydrolysis method was adapted and modified from de Swaaf et al. (2001). Prior to hydrolysis, 1 mL of extracted EPS solution was evaporated to dryness in a vacuum centrifuge (Concentrator Plus, Eppendorf) at 45°C in vacuum. The dried EPS were dissolved in 150 μL of 2 M trifluoroacetic acid (TFA) (HPLC grade, AppliChem) and the solution was incubated in a heating cabinet at 95°C for 3 h. Afterward, samples were evaporated to dryness using a parallel evaporation concentrator (Shaker: Syncore; Pump: Vac V-500; Controller: Vacuum Controller V-800; Büchi Labortechnik, Essen, Germany) at 50°C, 100 mbar and 200 rpm. For neutralization and washing, 100 μL of 1 M NH4OH solution (Suprapur, Merck) were added and after vortexing for 10 s, the solution was evaporated to dryness as described above. Finally, samples were dissolved in 500 μL of ultrapure water and the solutions were vortexed for 10 s. Samples that were not directly analyzed, were stored at -20°C until further use.
Glycan labeling and fluorescence-assisted carbohydrate electrophoresis
Glycans were labeled at their reducing ends via reductive amination (Ruhaak et al., 2010) using 2-aminonaphthalene trisulfonic acid (ANTS; Invitrogen, Thermo Fisher Scientific). Solutions of 25 mM ANTS in Milli-Q water/acetic acid (17:3 (v/v); 99.8-100%, Bernd Kraft, Duisburg, Germany) and 1 M sodium cyanoborohydride (Sigma-Aldrich) in dimethyl sulfoxide (DMSO; 99.7%, Acros Organics, NJ, USA) were prepared. As glycan standards 0.15% (w/v) D(+)-glucose (p.a., ACS, Carl Roth), 0.15% (w/v) D(+)-maltose (biochemical grade, Gerbu) and 20% (w/v) dextrin (pure, Carl Roth) solutions were used. EPS and acid hydrolyzed EPS samples were concentrated 30-fold (SpeedVac, Concentrator Plus, Eppendorf). Twenty-five microliter of samples/glycan standard solutions or Milli-Q water (negative control) were mixed with 90 μL of 25 mM ANTS solution and 100 μL of 1 M sodium cyanoborohydride. Reaction mixtures were vortexed and incubated in the dark at 37°C overnight. ANTS-labeled glycans were visualized using fluorescence-assisted carbohydrate electrophoresis (FACE). Thirty% FACE gels were poured using Rotiphorese acrylamide/bisacrylamide solution 40 (37,5:1; Carl Roth), 10x Tris-acetate-EDTA buffer, 10% (w/v) ammonium peroxodisulfate (Carl Roth), TEMED (Carl Roth), and dH2O. DMSO was removed from labeling reaction mixtures using the SpeedVac (Concentrator Plus, Eppendorf) at 60°C for 4 h. The sample volumes were adjusted to 100 μL with Milli-Q water, then 10 μL of labeled sample and 90 μL of 50% (v/v) glycerol solution were mixed. Five microliter of each sample were applied to FACE. For electrophoresis (Mini-PROTEAN Tetra cell system, Bio-Rad) a constant electrical voltage of 300 V was applied for 30 min in TAE buffer. Based on the negative charge of ANTS, labeled glycans are separated according to their molecular size by electrophoresis. ANTS-mediated UV absorbance was visualized using the Molecular Imager® Gel Doc™ XR System (Bio-Rad).
Derivatization of monosaccharides
Prior to analysis by liquid chromatography, the monosaccharides from references and hydrolyzed PS samples were derivatized with 1-phenyl-3-methyl-5-pyrazolone (PMP). The derivatization method was adapted and modified from the ones originally reported by Honda et al. (1989) and Rühmann et al. (2015). As derivatization agent, a 0.1 M PMP solution (≥99%, Sigma-Aldrich) in methanol (LC-MS grade, VWR) and 0.4% ammonium hydroxide (Suprapur, Merck) were mixed in a volume ratio of 2:1. Twenty-five microliter of sample were mixed with 75 μL of derivatization agent, vortexed and centrifuged at 268 × g for 2 min at room temperature (MiniSpin Plus, Eppendorf). After that, the samples were incubated at 70°C for 100 min in a heating cabinet. Then, 25 μL of 0.5 M acetic acid (glacial, VWR) and 875 μL of ultrapure water were added. Each sample was filtered (pore size 0.2 μM, cellulose, CS—Chromatographie Service, Langerwehe, Germany) and transferred to LC vials. Samples which were not directly analyzed, were stored at -20°C until further use. For more details see Meyer et al. (2022).
Analysis of monosaccharide composition
The monosaccharide composition analysis in EPS from S. acidocaldarius biofilms has been carried out using reversed phase liquid chromatography (RP-LC) coupled to triple quadrupole (TQ) mass spectrometry (MS) in multiple reaction monitoring (MRM) mode. An Agilent 1290 Infinity II LC system was coupled to an Agilent Triple Quadrupole mass spectrometer. The LC/TQ system comprised a 1290 high speed pump (G7120A, Agilent, Santa Clara, CA, USA), a 1290 Vialsampler (G7167B, Agilent), a 1290 multicolumn thermostat (MCT G7116B, Agilent) and a LC/TQ (G6470B, Agilent). A Kinetex C18 (100 × 2.1 mm, 1.7 μm core shell particles) (Phenomenex, Torrance, USA) column was used and the separation was carried out at 50°C. The mobile phase contained a 5 mM ammonium acetate buffer, pH 5.6 (LiChropur LC-MS, Merck) with 15% (v/v) acetonitrile (mobile phase A) and pure acetonitrile (mobile phase B) (LC-MS grade, VWR). The optimized gradient time was 15 min at a constant flow rate of 0.5 mL/min. The gradient started at 0% B, to 1% B in 2 min, increased to 5% B in 5 min and held for 2 min; to 18% B in 1 min, further increased to 40% B in 0.3 min and held for 2 min. Prior to the next injection the column was equilibrated at initial conditions for 2.7 min.
Mass spectrometry was performed using an electrospray ionization source (ESI) operating in positive ion mode, with a 2.5 min cut off to remove the early eluting excess of PMP. Nitrogen was utilized as sheath gas, drying gas and collision gas. The sheath and drying gas flow rates were set to 8 and 6 L/min at 325°C, respectively. The nebulizer was set at 40 psi and the capillary voltage and nozzle voltage were set at 4000 and 500 V, respectively. The fragmentor and cell acceleration voltage (CAV) were set to 165 and 9 V, respectively. The acquisition mode was performed on MS/MS in MRM with transitions of [M-OH + 2PMP]+ to [PMP + H]+ at optimized collision energies (20 and 30 V) for each monosaccharide using a dwell time of 100 ms. The LC and MS system were controlled by the software Agilent Mass Hunter Workstation Data Acquisition (Version C.01.00), data evaluation was carried out using Agilent Mass Hunter Qualitative Analysis Navigator (Version B.08.00). A mixture of 15 separable monosaccharides was prepared and limit of detection (LOD) and quantification (LOQ) were determined by analyzing dilutions of this mixture in fifteen calibration levels as triplicates (100, 80, 60, 40, 20, 10, 5, 2, 1, 0.5, 0.2, 0.1, 0.05, 0.02, and 0.01 μmol/L for each monosaccharide). LOD of the RP-LC-MS method was calculated with minimum 3× and LOQ with minimum 9× signal-to-noise ratio. LODs and LOQs (as μg/L) for the separated monosaccharides, and additionally, coefficient of determination (r2), retention time (rt) and mass as derivatized compound [M-OH + 2PMP], are given in Supplementary Table 3. EPS samples were analyzed in triplicates in blocks by RP-LC. Between the blocks, one blank was injected and additionally, before each injection, the injection needle was washed thoroughly, to avoid carryover and cross-contamination of analytes from the different samples. For the correction of potential contamination effects and the prevention of a monomer overestimation in EPS samples, negative control samples were treated with hydrolysis and derivatization and were analyzed. Detected monosaccharides in negative controls were quantified and subtracted from quantification results of real biofilm EPS samples, to rule out false-positive assignment of monosaccharides not originating from S. acidocaldarius biofilms.
RNaseA, DNaseI, and proteinase K treatment of TEM samples
To reduce the amounts of non-carbohydrate molecules in EPS samples other EPS components, namely extracellular nucleic acids and proteins, were digested. TEM fractions of S. acidocaldarius MW001 biofilms were treated with RNaseA to digest extracellular RNA (eRNA). Ten μg/mL RNaseA (Roche Diagnostics, Mannheim, Germany; stock solution of 10 mg/mL in 10 mM TrisHCl, 15 mM NaCl, pH 7.5) were added to TEM samples and incubated at 37°C overnight. This was followed by either further enzymatic treatments or by the dialysis of RNaseA treated TEM sample to generate the fraction of EPS molecules after RNaseA treatment (MWCO 3.5 kDa or 12–14 kDa; Spectrum Laboratories). DNaseI treatment was used to digest eDNA in the TEM fraction of S. acidocaldarius MW001 biofilms. Here, 10 μg/mL DNaseI (AppliChem, Darmstadt, Germany; stock solution of 10 mg/mL in 20 mM TrisHCl, 1 mM MgCl2, 50% (v/v) glycerol, pH 7.5) and 10 mM MgCl2 were added to TEM samples and incubated at 37°C for 1 h. To remove co-isolated (possibly glycosylated) EPS proteins as well as RNaseA and DNaseI, TEM samples were treated with proteinase K. About 100 μg/mL proteinase K (GeneOn, Ludwigshafen, Germany; 20 mg/mL) and 1 mM CaCl2 were added to TEM fractions of S. acidocaldarius biofilms. Incubation was performed at 37°C overnight. The enzymatically treated TEM sample was dialyzed against Milli-Q water using a MWCO of 3.5 kDa.
SDS-PAGE analyses of EPS proteins
To visualize EPS proteins EPS fractions were analyzed by SDS-PAGE. Samples treated with and without proteinase K were concentrated 20-fold by trichloroacetic acid (TCA) precipitation. One volume of 20% (w/v) TCA (ReagentPlus®, 99%, Sigma-Aldrich) was added to two volumes of sample, and the mixtures were incubated on ice for 20 min. After centrifugation (16,363 × g, 4°C, 30 min) the supernatant was discarded, the pellets were washed with a full volume of 80% (v/v) ice-cold acetone (analytical reagent grade, Fisher Chemical, Fisher Scientific) twice, air-dried for 30 min and resuspended in 50 mM TrisHCl buffer (pH 8.0) to yield 20-fold concentrated protein samples. Two microgram of EPS proteins were loaded on a 12% SDS-PAGE gel. After electrophoresis, EPS proteins were visualized using silver staining (Pierce Silver Stain Kit, Thermo Fisher Scientific) according to the manufacturer’s instructions. Gel documentation was performed using the Molecular Imager® Gel Doc ™ XR System (Bio-Rad, Hercules, CA, USA).
Size exclusion chromatography
Size exclusion chromatography for determination of molecular weight distribution of PS was performed using a HPLC system. An Agilent 1260 Infinity II SFC/UHPLC hybrid system operating in LC mode was coupled to an Agilent 1290 Infinity II Evaporating Light Scattering Detector (ELSD). The LC-ELSD system comprised a 1260 SFC binary pump (G4782A, Agilent), a 1260 SFC multisampler (G4767A, Agilent), a 1260 MCT (G7116A, Agilent), and a 1290 ELSD (G7102A, Agilent). The used columns were BioSep SEC-s2000 (300 mm × 7.8 mm, 5 μm particle size) and BioSep SEC-s4000 (300 mm × 7.8 mm, 5 μm particle size) (Phenomenex) in series, resulting in size fractionation between 1 and 1,500 kDa according to manufacturer information. Isocratic elution was carried out using water (ultrapure and desalted, resistivity 18.2 MΩ⋅cm, Sartorius) at constant 0.5 mL/min flow rate. Detector parameters were set to 80°C evaporator temperature, 90°C nebulizer temperature and 2.00 SLM (standard liter per minute) nitrogen gas flow rate. Calibration of the SEC columns was performed by injecting ten different size polysaccharide standards (Pullulan Standard Set 350-700,000, analytical standards, Supelco, St. Louis, MO, USA: 342 Da, 1,030 Da, 6,300 Da, 9,800 Da, 22,000 Da, 49,400 Da, 110,000 Da, 201,000 Da, 334,000 Da, 708,000 Da). Calibration resulted in following equation: log(MW) = –0.0004x3 + 0.0427x2 – 1.4439x + 21.425 with molecular weight (MW) expressed in Da and retention time x in minutes. The LC-ELSD system was controlled by Agilent OpenLAB CDS—Aquisition (Version 2.3), data evaluation was carried out using Agilent OpenLAB Data Analysis (Version 2.3). The calculation of the number of monomeric residues in the PS was performed assuming a linear polymer. The mass of the individual monosaccharide in a glycosidic bond was reduced by 18 atomic units (for water release) and the share of the different monosaccharides in the PS, which was previously determined in the LC-MS analyses, was incorporated in the calculation.
Results
Sulfolobus acidocaldarius biofilm formation in different growth media
Brock medium (Brock et al., 1972) with 0.1% (w/v) N-Z-amine (NZA) was used as complex basal medium for growth. Different sugars were added to this medium and their influence on biofilm formation and quantity was compared in 24-well plates. The sugars used in this study, D-glucose, D-xylose or maltose, had been shown to promote S. acidocaldarius growth in liquid cultures in previous studies (Choi et al., 2013; Wagner et al., 2014, 2018). Cultures were inoculated to an OD600nm value of 0.01. Cultivation was performed under static conditions for one to five days at 76°C. Each day (i) total turbidity (OD600nm) values of biofilm and planktonic cells, (ii) OD600nm values of planktonic cell suspensions, and (iii) biofilm amounts using crystal violet staining were determined (Supplementary Figure 1). Cultivation with 0.1% (w/v) NZA and 0.2% (w/v) D-glucose resulted in the highest amounts of firmly attached S. acidocaldarius biofilm mass after four days of static cultivation (91 h) (Supplementary Figures 1, 2). The highest ratio of biofilm to planktonic cells was detected by the supplementation of glucose, leading to an at least 1.85-fold higher ratio than observed for the other tested growth media (Supplementary Figure 2D). Hence, this medium composition was used predominantly in subsequent biofilm cultivation and PS analyses.
Optimization of Sulfolobus acidocaldarius biofilm cultivation
Up to now, archaeal biofilms were mostly generated in MTPs, dishes or on slides consisting of different types of polymers (e.g., polystyrene) or glass as substratum for biofilm formation (Benninghoff et al., 2017). While the cultivation of biofilms, e.g., in MTPs, is optimal to analyze biofilm properties on a high-throughput scale (see section “Sulfolobus acidocaldarius biofilm formation in different growth media”), it is suboptimal to receive high amounts of biofilm mass. The cultivation of S. acidocaldarius biofilms on gellan gum-solidified Brock medium plates, as reported by Jachlewski et al. (2015), bears the risk of co-isolation of the gelling agent, an anionic heteropolysaccharide (Jansson and Lindberg, 1983), during EPS extraction. Alternatively, synthetic filtration membranes have been used for the cultivation of biofilms (Vanysacker et al., 2013; Zhang et al., 2014; Waheed et al., 2022). We adapted and optimized this method for the cultivation of S. acidocaldarius biofilms. S. acidocaldarius MW001 cell suspensions were filtered on a membrane. The membrane was placed on the surface of liquid or gellan gum-solidified Brock medium and incubated statically at 76°C for 4 days. Media components were allowed to pass the pores of membranes and ensured nutrient availability for biofilm growth on top of the membranes. Ten different commercially available membranes were compared regarding their ability to ensure S. acidocaldarius biofilm formation. These included hydrophilic and hydrophobic round membranes of different chemical composition and pore sizes of 0.2–0.45 μm (Supplementary Table 1). The three best performing membranes regarding biofilm mass yield (Supplementary Table 1) were chosen for a more detailed comparison of S. acidocaldarius biofilm formation on liquid medium, comparing the generated biofilm wet weights per area, total cell counts, culturability and carbohydrate amounts in EPS fractions (Supplementary Figure 3). While heterogeneous biofilms were formed on the polycarbonate track etched filter or on the PTFE filter, a reproducibly homogeneous confluent biofilm was formed on the hydrophilic PTFE membrane (Omnipore, PTFE polymer membrane bound to a high density polyethylene support, 0.45 μm pore size, 47 mm diameter, Merck). Thus, this polymeric membrane was used for biofilm cultivation. Different inocula, in the range of 104–108 cells/membrane, were applied on the membrane and biofilm formation was compared after 4 days of static incubation at 76°C. The filtration of low cell numbers (104) resulted in the formation of macrocolonies after 4 days of cultivation, while the application of high cell numbers (107–108) resulted in the formation of a biofilm that was only loosely attached to the membrane (data not shown). An inoculum of 105–106 cells/membrane led to significant biofilm growth firmly attached to the membrane surface after 4 days of cultivation (Figure 1). In the optimized cultivation protocol, 6 × 105 S. acidocaldarius MW001 cells were filtered on the hydrophilic PTFE membrane (Omnipore) and incubated statically floating on liquid Brock medium (0.1% (w/v) NZA, 0.2% (w/v) glucose, 10 μg/mL uracil) at 76°C for 4 days.
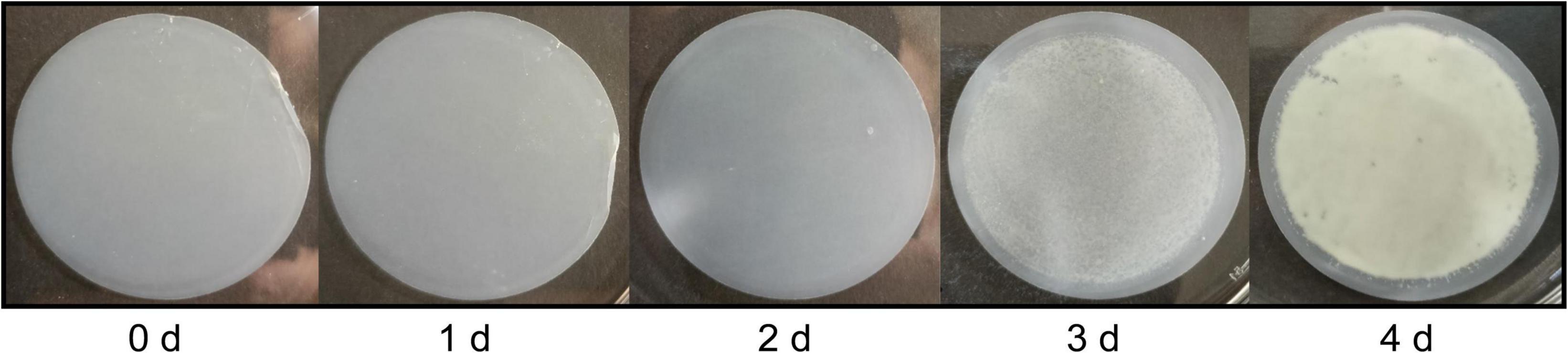
Figure 1. Time-dependent Sulfolobus acidocaldarius MW001 biofilm formation on the hydrophilic PTFE membrane. Membranes were inoculated with 6 × 105 cells/membrane, equivalent to a cell density of 3.5 × 104 cells/cm2. Cultivation was performed statically floating on liquid Brock medium (pH 3.0) supplemented with 0.1% (w/v) NZA, 0.2% (w/v) glucose, 10 μg/mL uracil, at 76°C. Biofilm formation was documented by camera every day. After 4 days of cultivation the confluent biofilm was harvested. A cell density of 3.4 × 109 cells/cm2 was determined after 4 d (Supplementary Figure 3D).
Quantification of carbohydrates, proteins and eDNA in EPS fractions of Sulfolobus acidocaldarius MW001 biofilms
Extracellular polymeric substances were isolated from biofilm suspensions with a cation exchange resin (CER) as reported by Jachlewski et al. (2015). After CER treatment, the sterile-filtered extract contained total extracellular material (TEM) of low- and high-molecular-weight compounds. The EPS fraction was obtained by dialysis of the TEM sample, removing low-molecular-weight compounds (MWCO 3.5 kDa). The amounts of carbohydrates, proteins and eDNA per cell were quantified for the biofilm suspension (BF) and the fractions of TEM and EPS of 4 days old biofilms (Figure 2).
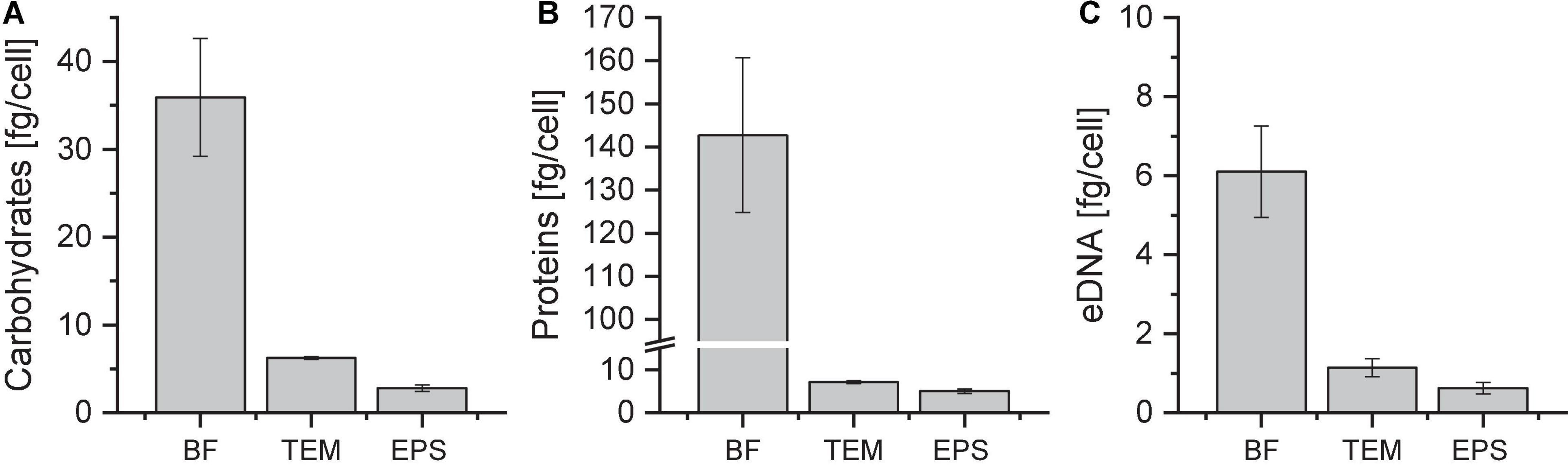
Figure 2. Amounts of carbohydrates (A), proteins (B), and eDNA (C) in different Sulfolobus acidocaldarius MW001 biofilm fractions. Biofilms were grown on hydrophilic PTFE membranes at 76°C for 4 days (liquid Brock medium, pH 3.0, 0.1% (w/v) NZA, 0.2% (w/v) glucose, 10 μg/mL uracil). EPS isolation was performed using CER. Carbohydrate, protein, and eDNA concentrations were determined using the phenol/sulfuric acid method, the modified Lowry assay and the Qubit dsDNA assay, respectively. BF, biofilm suspension; TEM, total extracellular material; EPS, extracellular polymeric substances (MWCO 3.5 kDa) (n = 3).
With an amount of 5.0 ± 0.5 fg/cell proteins were most abundant in the EPS fraction of S. acidocaldarius MW001 biofilms grown on membranes, followed by carbohydrates with an amount of 2.8 ± 0.6 fg/cell and eDNA with 0.6 ± 0.1 fg/cell (Figure 2). The mass ratios of proteins:carbohydrates:eDNA were approx. 8:4:1 under the chosen growth conditions.
Analysis of exopolysaccharide monomeric composition
To unravel the monosaccharide composition in S. acidocaldarius EPS, samples of S. acidocaldarius MW001 biofilms were subjected to acid hydrolysis. In preliminary experiments, three different hydrolysis methods were compared, using trifluoroacetic acid (TFA), sulfuric acid or hydrochloric acid, and the corresponding monosaccharide concentration was determined (Supplementary Figure 4A and Supplementary Table 2). Highest carbohydrate concentrations in EPS hydrolysates were observed using TFA for acid hydrolysis. In addition, the method required less extensive neutralization steps and an overall lower sample preparation time and was thus less prone to losses of analytes throughout the procedure. Lower concentrations of individual sugars, as well as overall lower yields for the carbohydrate fraction were determined using sulfuric acid or hydrochloric acid. The success of PS hydrolysis by TFA, meaning the formation of monosaccharides, was also visualized by ANTS carbohydrate labeling and FACE (Supplementary Figure 4B). While the sensitivity of the method did not allow the visualization of PS, TFA hydrolysis led to an increased availability of reducing ends for the labeling reaction and the occurrence of monosaccharide signals in FACE. After 2 h of hydrolysis, a strong monosaccharide signal was present in hydrolyzed EPS samples. The monosaccharide signal intensity did not increase any further after 3 h of hydrolysis (Supplementary Figure 4B).
For the analysis of the monomeric PS composition, reversed-phase liquid chromatography (RP-LC) coupled to mass spectrometry was chosen as an advanced method for analysis. To determine the suitability of the RP-LC method for analysis of the PS composition, single component standards out of a set of 15 monosaccharides, which were selected as they are among the most common naturally occurring monosaccharides, were derivatized with 1-phenyl-3-methyl-5-pyrazolone (PMP) and retention times for each component were assigned. All monosaccharides could be separated either by retention time or mass differences (Supplementary Figure 5; Supplementary Table 3; Meyer et al., 2022).
EPS samples from S. acidocaldarius MW001 biofilms were processed by TFA hydrolysis and PMP derivatization. Besides FACE analysis, successful hydrolysis and exclusion of remaining poly- and oligosaccharide structures was confirmed by LC-MS in full scan mode with a larger m/z (mass-to-charge ratio) range. No signals were detected above m/z = 600, hence, all PS structures were hydrolyzed to monosaccharides (data not shown). Analysis of the hydrolyzed EPS fraction of biofilm samples resulted in the detection of mannose (Man), ribose (Rib), glucosamine (GlcN), rhamnose (Rha), N-acetylglucosamine (GlcNAc), glucose (Glc), and galactosamine (GalN) (Figure 3).
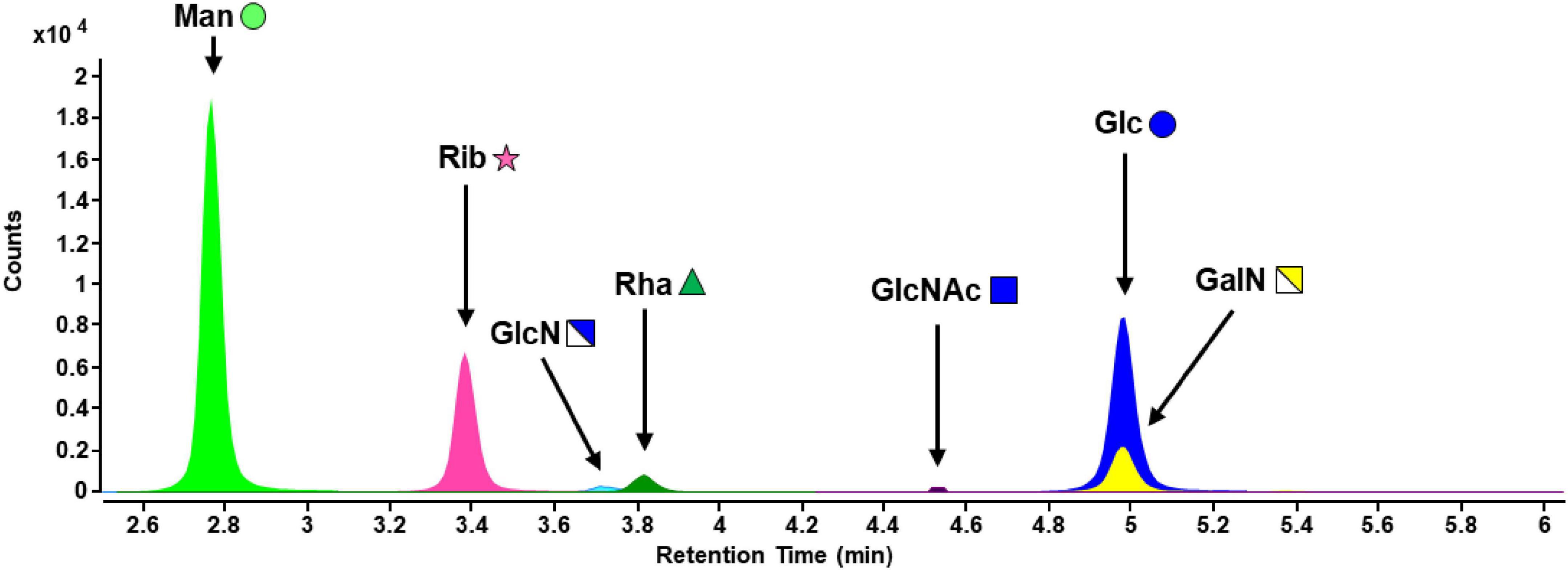
Figure 3. MRM chromatogram of carbohydrates of hydrolyzed EPS from S acidocaldarius MW001 biofilms cultivated on hydrophilic PTFE membranes at 76°C for 4 days (liquid Brock medium, pH 3.0, 0.1% (w/v) NZA, 0.2% (w/v) glucose, 10 μg/mL uracil). Monosaccharide symbols were used according to the glycan nomenclature of NCBI (Man, mannose; Rib, ribose; GlcN, glucosamine; Rha, rhamnose; GlcNAc, N-acetylglucosamine; Glc, glucose; GalN, galactosamine). Further information on the analytical performance of the RP-LC-MS method can be found in Supplementary Table 3.
Major monosaccharide components detected in EPS were mannose (35%), glucose (29%), and ribose (26%), making up more than 90% of the monosaccharidic composition in terms of molar ratios (Figure 4). Minor components were glucosamine (5%) and rhamnose (3%). Galactosamine and N-acetylglucosamine, with a fraction of around 1% each regarding the total concentration, were detected above the limit of quantification (LOQ) and were therefore quantifiable. Due to the fact that acetylated carbohydrate species are usually mostly deacetylated during acid hydrolysis, some share of the galactosamine and glucosamine fraction might originate from N-acetylated species such as N-acetylgalactosamine and N-acetylglucosamine, respectively. Investigation of deacetylation of N-acetylgalactosamine and N-acetylglucosamine under the same acid hydrolysis conditions used for EPS resulted in rates of 100% deacetylation for GalNAc and 98% for GlcNAc (data not shown). An unknown compound from the uronic acid group was also found in S. acidocaldarius EPS extracts, which was not included in the developed RP-LC-MS method (no retention time matching) and could not be identified so far.
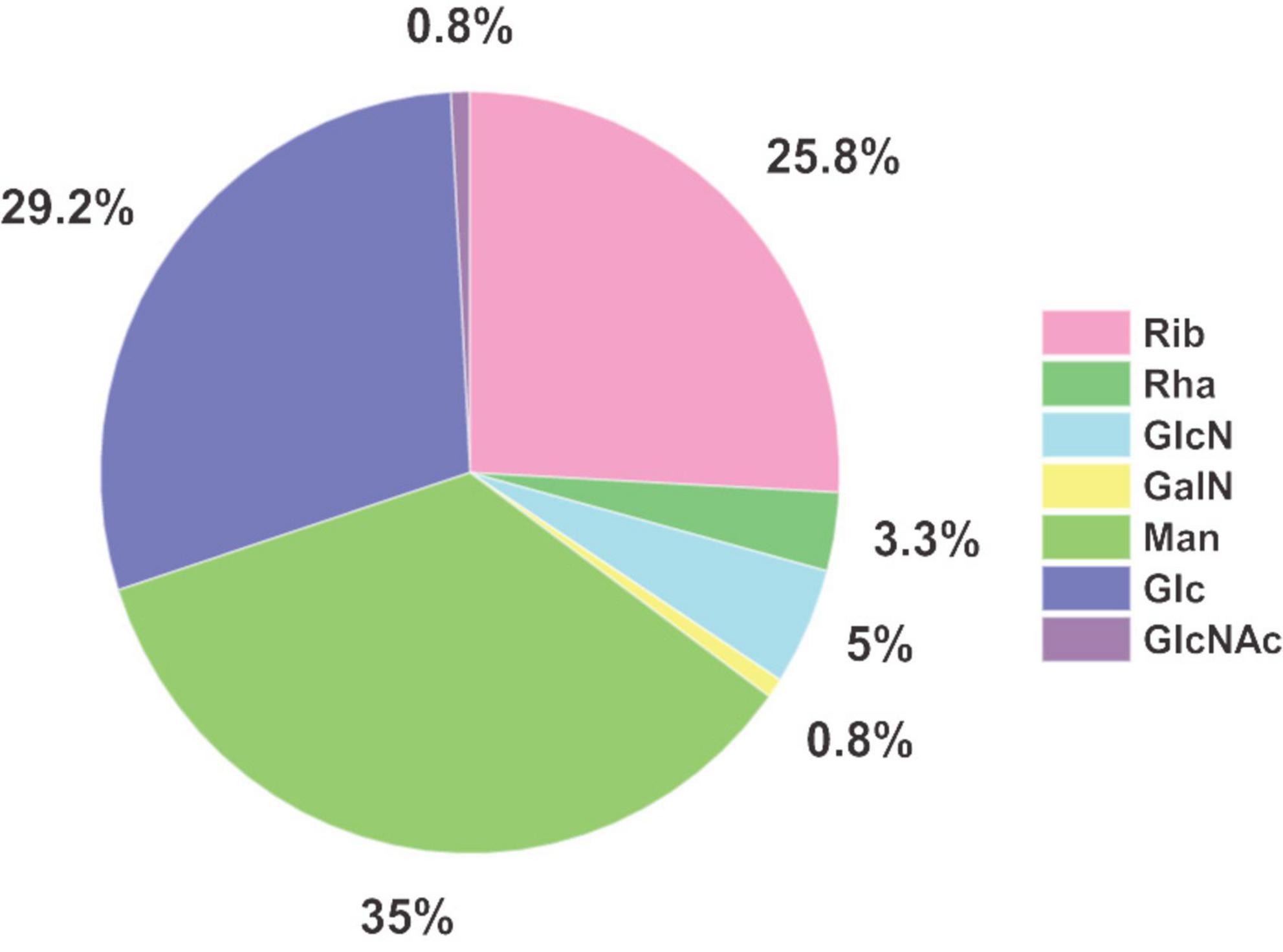
Figure 4. Molar ratios of monosaccharides identified in EPS of Sulfolobus acidocaldarius MW001 biofilm cultivated on hydrophilic PTFE membranes at 76°C for 4 days (liquid Brock medium, pH 3.0, 0.1% (w/v) NZA, 0.2% (w/v) glucose, 10 μg/mL uracil) (n = 3). Values and standard deviations of the biological replicates are given in Supplementary Table 5.
Initial experiments indicated the influence of growth substrates on S. acidocaldarius MW001 biofilm formation (see section “Sulfolobus acidocaldarius biofilm formation in different growth media”; Supplementary Figures 1, 2). Therefore, we also examined the EPS and monosaccharide composition in dependency of different growth substrates. Brock medium with 0.1% (w/v) NZA served as basal medium and was compared to medium additionally supplemented with either 0.2% (w/v) D-glucose D-xylose, or maltose. Our data indicate that the ratios of EPS components (i.e., carbohydrate, protein and eDNA) change depending on the presence of different sugars in the growth medium (Supplementary Figure 6 and Supplementary Table 4). Highest amounts of carbohydrates were detected upon the addition of D-glucose to the medium. In general, the same sugar monomer species were identified in biofilm EPS samples of all tested cultivation conditions, while the molar ratios of single constituents changed slightly depending on the added carbohydrate substrates (Supplementary Figure 7 and Supplementary Table 5).
Reduction of RNA, DNA, and protein amounts in EPS fractions by enzymatic treatments
A relatively high proportion of ribose (26%) was detected in the hydrolyzed EPS sample of S. acidocaldarius MW001 biofilms (Figure 4), raising the question of its origin. In general, next to eDNA also eRNA is regarded as relevant structural nucleic acid in microbial EPS matrices (Karygianni et al., 2020). To examine whether the detected ribose moieties derived from eRNA molecules, TEM samples were subjected to RNaseA treatment. Afterward, samples were dialyzed to remove low-molecular-weight ribose molecules (MWCO 3.5 kDa and 12–14 kDa). No change in the proportion of ribose to the monosaccharide composition was found after RNaseA treatment in comparison to no treatment under the tested conditions (data not shown). Furthermore, proteins are a major compound of the S. acidocaldarius EPS matrix (Figure 2; Jachlewski et al., 2015). Many extracellular proteins of S. acidocaldarius, for example FlaB (subunit of the archaellum filament) and S-layer proteins SlaA and SlaB are known to be glycosylated (hexasaccharide composed of Glc1Man2GlcNAc2 plus 6-sulfoquinovose) (Peyfoon et al., 2010; Guan et al., 2016). To exclude the influence of glycosylated EPS proteins on S. acidocaldarius PS analyses, proteins were digested by proteinase K and the EPS protein-free sample was analyzed by RP-LC-MS (Supplementary Figure 8). Proteinase K treated EPS samples of S. acidocaldarius showed the same carbohydrate concentrations and monosaccharide compositions as non-treated samples. Thus, glycolsylated EPS proteins had no impact on the applied PS composition analysis.
Determination of the exopolysaccharide size
In order to determine the PS size an enzymatic pretreatment of the EPS fraction with RNaseA, followed bv DNaseI and proteinase K, for the degradation of eRNA, eDNA and proteins, respectively, was established to avoid interference of other polymers. RP-LC-MS analyses confirmed no changes in monosaccharide identity and composition due to the enzymatic treatments of EPS samples (data not shown). Non-treated and enzymatically treated EPS fractions (RNaseA, DNaseI and proteinase K) were compared by size exclusion chromatography (Figure 5). As carbohydrate reference for size determination a set of pullulan standards was used (Supplementary Table 6).
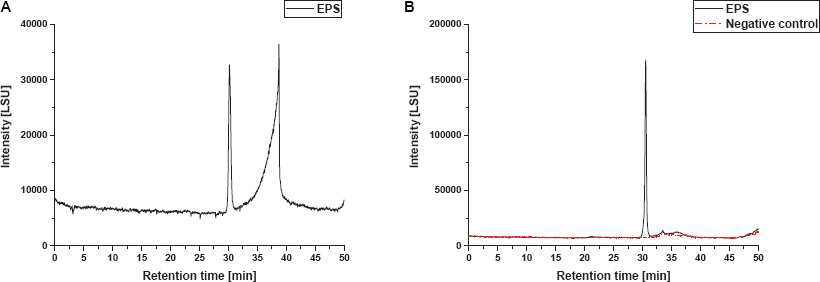
Figure 5. SEC-ELSD size distribution profiles of EPS fractions from S. acidocaldarius MW001 biofilms. (A) Non-treated EPS extract and (B) enzymatically treated EPS extract and corresponding negative control sample are shown. Both EPS sample and negative control were subjected to RNaseA, DNaseI, and proteinase K treatment for the removal of macromolecular interferences.
Chromatographic profiles obtained from EPS extracts in the non-treated sample displayed one peak at a retention time of approximately 30 min and subsequent one additional strongly fronting signal between 31 and 40 min (Figure 5A). The chromatogram of the enzymatically treated sample (RNaseA, DNaseI, proteinase K) shows only one peak with a narrow size distribution (Figure 5B, black). Thus, it appears plausible to suppose that the second signal in the non-treated sample resulted from macromolecular interference, such as proteins or eDNA, and shows a strong fronting behavior due to the non-optimized SEC method for this particular substance class. Therefore, enzymatic treatment was crucial for the size determination of the PS fraction by SEC. A distinct PS peak occurred between 29.6 to 31.4 min retention time, with the peak apex at 30.5 min, which corresponds to an elution volume of 15.3 mL and a molecular mass of ∼44 kDa for the exemplary biological replicate shown in Figure 5B. Hence, the mean PS fraction of this replicate comprised approx. 287 monosaccharide residues (with regard to the molar fraction of the different identified monosaccharides). Furthermore, four additional biological replicates of S. acidocaldarius MW001 biofilm PS samples were analyzed by SEC and showed a biological variance in the size of the excreted PS, ranging between ∼44 and ∼92 kDa (approx. 290—600 monosaccharide residues), resulting in a mean of approximately 71 kDa (approx. 460 monosaccharides; n = 5). For all replicates, only a single narrowly defined size fraction of PS was found in the SEC chromatograms.
In order to exclude an effect of the enzymatic pretreatment on PS size determination, a medium/buffer control was equivalently enzymatically treated and analyzed by SEC (negative control, Figure 5B, red). Since the samples were treated with different enzymes to remove macromolecular interferences, the used enzymes themselves and their molecular weights had to be taken into account. According to manufacturer’s information DNAseI, proteinase K and RNAseA have molecular weights of 30, 28.9, and 13.7 kDa, respectively. Thus, the mass fraction found in SEC analysis does not coincide with any molecular mass of the enzymes used; in correspondence the equally enzymatically treated negative control did not show any relevant signal in SEC analysis (Figure 5B, red).
Discussion
Here, we report the development of a workflow for the generation of high biofilm mass, EPS isolation and chemical analyses of the monomeric composition and size of the exopolysaccharide from EPS produced by S. acidocaldarius biofilms.
Compared to other published work on S. acidocaldarius biofilms, using MTPs or dishes for cultivation (Koerdt et al., 2010; Benninghoff et al., 2017), an alternative cultivation method was established to generate high amounts of biomass necessary for subsequent EPS analysis. Different polymeric membranes were tested for their suitability to form homogeneous, thick biofilms. Cellulose-based membranes were less suitable for biofilm generation and the usage of a polycarbonate membrane resulted in the formation of visually observed macrocolonies, rather than a homogeneous biofilm. Highest yields of biofilm (5.25 mg/cm2) were generated with the fibrous PTFE polymer membrane, bound to a polyethylene support (Omnipore membrane, Merck), yielding a reproducibly homogeneous confluent biofilm. Compared to the cultivation of submersed biofilm at the bottom of petri dishes, a 48-fold amount of biofilm wet weight was harvested from membranes (0.11 mg/cm2 according to Benninghoff et al., 2021). Similar to our study, also bacterial biofilm attachment and maturation was reported to increase on fibrous membranes, such as PTFE membranes (Waheed et al., 2022).
In general, S. acidocaldarius MW001 biofilm amounts were increased by the addition of sugars to the growth medium, most significantly by the addition of glucose (in combination with NZA, after 4 days of cultivation in 24-well plates). In agreement with the increased biofilm amount in the presence of glucose, highest carbohydrate amounts were detected in S. acidocaldarius EPS fractions under this condition. Hence, the addition of glucose was beneficial to generate plenty of EPS for further PS analyses. Similar correlations between the presence of glucose in the growth medium and the production of PS or EPS were also reported for Haloferax volcanii biofilms (Fröls et al., 2012), Ferroplasma acidiphilum biofilms (Zhang et al., 2014), Haloferax mediterranei (Antón et al., 1988), and Haloterrigena turkmenica (Squillaci et al., 2016), respectively. Next to the obvious function of glucose as energy source, it may serve as sugar precursor for S. acidocaldarius PS synthesis. Glucose may either be activated and integrated into the exopolysaccharide (29% of glucose in PS) or converted to other PS sugar monomers, particularly mannose as a main constituent of S. acidocaldarius PS (35%). Although mannose is a primary component of both PS and protein N-glycosylation (Peyfoon et al., 2010), its synthesis pathway is yet unknown in S. acidocaldarius.
The isolation of water-soluble EPS was performed using the CER extraction method as described by Jachlewski et al. (2015). Compared to other extraction methods, the CER method is best suited for S. acidocaldarius biofilms with respect to EPS yield, impact on cell viability and its compatibility with subsequent (bio)chemical analyses (Jachlewski et al., 2015). The EPS fraction from S. acidocaldarius MW001 biofilms grown on membranes was dominated by 5.0 ± 0.5 fg/cell protein, followed by carbohydrates of 2.8 ± 0.6 fg/cell and 0.6 ± 0.1 fg/cell eDNA (0.1% (w/v) NZA, 0.2% (w/v) Glc medium). Beside the total biofilm formation, also the EPS composition and the ratios of protein, eDNA and carbohydrate amounts changed in response to the supplementation of different sugars to the growth medium.
Available information on the PS composition of archaeal biofilms is dominated by non-destructive analysis methods, using confocal laser scanning microscopy (CLSM) in combination with fluorophore-labeled lectins to visualize biofilm architecture and sugar moieties in the EPS matrix. Comprehensive fluorescence lectin-binding analyses are available for Sulfolobus metallicus DSM 6482T (Zhang R. et al., 2015) and Acidianus sp. DSM 29099 (Zhang R.Y. et al., 2015), while only two fluorophore-labeled lectins, Concanavalin A (ConA) from Canavalia ensiformis binding to Glc or Man and Isolectin IB4 from Griffonia simplicifolia (GS-IB4) binding to Gal or GalNAc residues, were applied for the visualization of extracellular sugars in S. acidocaldarius biofilms (Henche et al., 2012; Orell et al., 2013, 2018; Recalde et al., 2021). CLSM analysis can serve as a qualitative indication for the presence of certain extracellular carbohydrates, but it cannot offer any information on the total sugar composition as well as quantitative amounts and ratios. In addition to that, unexpected lectin binding was reported for ConA, which was reported to interact with bacterial alginate composed of (1→4)-linked β-D-mannuronate and α-L-guluronate residues (Strathmann et al., 2002). A workflow including destroying biofilm integrity necessary for EPS isolation and advanced chemical analysis methods are beneficial for a full analysis of the extracellular monosaccharide composition in EPS.
Acid hydrolysis followed by high-performance anion exchange chromatography with pulsed amperometric detection (HPAEC-PAD) or gas chromatography coupled to MS (GC-MS) were used for the analysis of archaeal PS composition before (Lü et al., 2017; Zhang et al., 2019). Recently, a comparative study was performed in which different chromatographic methods for the analysis of monosaccharides from biological samples were assessed (Meyer et al., 2022). This study demonstrated that RP-LC-MS after monosaccharide derivatization is superior to other methods such as supercritical fluid chromatography (SFC), hydrophilic interaction liquid chromatography (HILIC), and GC in terms of separation performance, sensitivity and method standard deviation. Compared to the application of HPAEC, advantages in RP-LC-MS are also evident in terms of sensitivity (Anders et al., 2015; Squillaci et al., 2016). Furthermore, RP-LC-MS provides mass spectrometric information, which allows an assignment of unknown species to a substance class, e.g., hexoses, pentoses, uronic acids. RP-LC after derivatization has already been applied for the analysis of bacterial EPS (Rühmann et al., 2015; Qamar et al., 2021) and shows its strength in our study with regard to the detailed analysis of the PS composition of S. acidocaldarius biofilm.
Compared to Bacteria, information on the monomeric composition of archaeal PS is limited and only a few crenarchaeal and euryarchaeal examples were studied yet. Regarding Crenarchaea, studies are available for Acidianus sp. DSM 29099, S. metallicus DSM 6482T (Zhang et al., 2019) and Sulfolobus solfataricus MT3 and MT4 biofilms (Nicolaus et al., 1993). For instance, S. solfataricus MT4 was reported to produce an exopolysaccharide from Glc/Man/GlcN/Gal in a ratio of 1.2:1.0:0.18:0.13 when grown with 0.3% (w/v) glucose, as detected by HPAEC-PAD and TLC (Nicolaus et al., 1993). Slightly different ratios of monosaccharides were identified in PS of S. solfactarius MT3 with Glc/Man/GlcN/Gal in a ratio of 1.2:1.0:0.77:0.73. A distinction was made between colloidal and capsular EPS for Acidianus sp. DSM 29099 and S. metallicus DSM6482T biofilms (Zhang et al., 2019). Here, colloidal EPS were defined as soluble EPS fraction, present in the supernatant after EDTA extraction, while capsular EPS were bound to the microbial cell pellet, extracted and analyzed separately. Acid hydrolysis with TFA and HPAEC-PAD analysis of PS (>3.5 kDa) identified Glc and Man (74.4 and 25.6%) in colloidal EPS and GalN:Gal:GlcN:Glc:Xyl:Man [0.9:2.1:0.2:49.8:2.9:44.2 (%)] in capsular EPS of Acidianus sp. DSM 29099 (grown on S0). For S. metallicus DSM6482T Gal:Glc:Man were detected in the colloidal EPS in ratios of 8.7:63.4:25.1 (%), and in the capsular EPS in ratios of 1.0:50.7:48.3 (%) (grown on S0) (Zhang et al., 2019).
In this study, pentoses (Rib), hexoses (Man, Glc), amino sugars (GalN, GlcN), deoxyhexoses (Rha), and acetylated species (GlcNAc) were identified in S. acidocaldarius EPS samples by RP-LC-MS analysis (Man/Glc/Rib/GlcN/Rha/GlcNAc/GalN in a ratio of 42:35:31:6:4:1:1). The molar proportions of mannose, glucose and ribose were the highest, making up around 90% of the detected monosaccharides. The identified amino sugars (GalN and GlcN) could originate from originally N-acetylated species that were deacetylated during PS hydrolysis. This is a well-known mechanism, which has been frequently studied and discussed in the literature (Einbu et al., 2007; Einbu and Varum, 2008; Knirel et al., 2019). Based on our data, observing deacetlyation rates of 100 and 98% for sugar standards of GalNAc and GlcNAc, respectively, we cannot exclude that the amino sugars found in S. acidocaldarius PS originate from N-acetylated species. Compared to CLSM analyses of S. acidocaldarius biofilms, using fluorophore-labeled lectins, several additional monosaccharides were identified in EPS by RP-LC-MS. Comparing the monomeric PS compositions published for different crenarchaeal species, it is obvious that glucose and mannose are the dominant monomer species detected in crenarcheal EPS samples, including that of S. acidocaldarius MW001. The occurrence of ribose in PS samples was also reported for bacterial EPS, including Hahella chejuensis (Lee et al., 2001), a bacterial/archaeal co-culture of Thermotoga maritima and Methanococcus jannaschii (Johnson et al., 2005) as well as archaeal EPS of Halorubrum sp. TBZ112 (HPAEC-PAD) (Hamidi et al., 2019) and Methanosarcina mazeii DSM6300 (GC-MS) (Veiga et al., 1997).
PS from bacterial biofilms are reported to be very long molecules with proposed molecular mass of approx. 104–106 Da (Mata et al., 2006, 2008; Sutherland, 2017; Wang et al., 2019). Molecular masses of euryarchaeal biofilm PS were also in the range of 104–106 Da, as determined by size-exclusion chromatography (SEC) for PS of Halorubrum sp. TBZ112 with 0.5 × 104Da (Hamidi et al., 2019), Thermococcus litoralis with 4.1 × 104Da (Rinker and Kelly, 1996), Haloferax mucosum with 7.65 × 104 -1.52 × 105Da (López-Ortega et al., 2020) and Haloarcula hispanica with 1.1 × 106Da (Lü et al., 2017). For Haloterrigena turkmenica, three size fractions with significantly different molecular weights of 80.1, 20.6 and 3.8 × 104Da were determined (Squillaci et al., 2016). A molecular mass of one PS with a mean of 7.1 × 104 Da was identified by SEC for the crenarchaeal strain S. acidocaldarius MW001 in this study. This mass is comparable to reported euryarchaeal exopolysaccharide sizes. Combined with the results from the quantitative determination of the monomers, S. acidocaldarius PS consists of approx. 460 monomeric residues on average, assuming a linear PS structure. In order to determine a possible branching, further analyses, such as FT-IR and NMR spectroscopy, and GC-MS after methanolysis (Parolis et al., 1996, 1999; Paramonov et al., 1998; Li et al., 2018) may be performed.
Regarding the exopolysaccharide synthesis pathway in S. acidocaldarius, the presence of a membrane-associated synthesis, secretion and polymerization mechanism is likely. Here, especially the regulation of PS size seems to be a well-organized and controlled process, resulting in the secretion of biopolymers of a well-defined length. Previous studies observed the influence of a Lrs14-like transcriptional regulator on S. acidocaldarius biofilm formation and cell motility [Archaeal Biofilm Regulator 1 (abfR1)] (Orell et al., 2013). The corresponding protein (Saci_0446) was described as biofilm transcriptional repressor, controlling the expression of the archaellum operon, the archaeal adhesive pili operon and an additional gene cluster (saci_1904-saci_1927), encoding several glycosyltransferases and membrane proteins (Orell et al., 2013; van Wolferen et al., 2018). This gene cluster may encode proteins involved in S. acidocaldarius PS synthesis and excretion. In response to changing environmental conditions and nutrient availability, different glycosyltransferases may be involved in the synthesis of the PS, resulting in varying ratios of the monomers in dependency of nutrient availability (i.e., growth medium composition).
In summary, this study provides deeper insights into the EPS composition and dynamics of S. acidocaldarius MW001 biofilms in response to energy and carbon sources with a special focus on the monosaccharide composition and PS size. We established a protocol for the cultivation of S. acidocaldarius biofilm on a filter membrane as well as PS composition analyses and size determination. The presented method set displays the basis for further research on the function of PS in the EPS matrix as well as its synthesis and export by S. acidocaldarius.
Data availability statement
The original contributions presented in this study are included in the article/Supplementary material, further inquiries can be directed to the corresponding authors.
Author contributions
LK performed microbial, microscopic as well as biochemical assays and glycan labeling experiments and performed biofilm cultivation and EPS isolation. LK and MM performed biochemical assays for the quantification of EPS components. MM performed detailed analytical analyses, including sample preparation (acid hydrolyses), RP-LC-MS and SEC. CB, JW, OS, and BS acquired funding and supervised the study. LK and MM wrote the manuscript under supervision of CB, JW, OS, and BS. All authors contributed to the article and approved the submitted version.
Funding
This work received funding from the German Research Foundation (DFG, SCHM 1699/26-1 | SI 642/13-1 | WI 831/4-1; project number: 393406057).
Acknowledgments
We thank Benjamin Meyer for supporting this manuscript with critical discussions and Astrid Dannehl for her experimental assistance.
Conflict of interest
The authors declare that the research was conducted in the absence of any commercial or financial relationships that could be construed as a potential conflict of interest.
Publisher’s note
All claims expressed in this article are solely those of the authors and do not necessarily represent those of their affiliated organizations, or those of the publisher, the editors and the reviewers. Any product that may be evaluated in this article, or claim that may be made by its manufacturer, is not guaranteed or endorsed by the publisher.
Supplementary material
The Supplementary Material for this article can be found online at: https://www.frontiersin.org/articles/10.3389/fmicb.2022.982745/full#supplementary-material
Abbreviations
2dGlc, 2-deoxyglucose; ANTS, 2-aminonaphthalene trisulfonic acid; Ara, arabinose; BF, biofilm suspension; CAV, cell acceleration voltage; CE, capillary electrophoresis; CER, cation exchange resin; CLSM, confocal laser scanning microscopy; ConA, Concanavalin A from Canavalia ensiformis; CV, crystal violet; Dex, dextrin; DMSO, dimethyl sulfoxide; dRib, 2-deoxyribose; dsDNA, double-stranded deoxyribonucleic acid; eDNA, extracellular DNA; ELSD, evaporative light scattering detector; EPS, extracellular polymeric substances; eRNA, extracellular RNA; ESI, electrospray ionization; FACE, fluorescence-assisted carbohydrate electrophoresis; Fuc, fucose; Gal, galactose; GalA, galacturonic acid; GalN, galactosamine; GalNAc, N-acetylgalactosamine; GC, gas chromatography; Glc, glucose; GlcA, glucuronic acid; GlcN, glucosamine; GlcNAc, N-acetylglucosamine; GPC, gel permeation chromatography; GS-IB4, Isolectin IB4 from Griffonia simplicifolia; HILIC, hydrophilic interaction liquid chromatography; HPAEC, high-performance anion-exchange chromatography; (HP)LC, (high-performance) liquid chromatography; LOD, limit of detection; LOQ, limit of quantification; Mal, maltose; Man, mannose; MRM, multiple reaction monitoring; MS, mass spectrometry; MTP, microtiter plates; MWCO, molecular weight cut-off; m/z, mass-to-charge ratio; NMR, nuclear magnetic resonance; NZA, N-Z -amine; OD, optical density; PAD, pulsed amperometric detection; PMP, 1-phenyl-3-methyl-5-pyrazolone; ProtK, proteinase K; PS, exopolysaccharides; PTFE, polytetrafluoroethylene; Rha, rhamnose; Rib, ribose; RP-LC, reversed-phase liquid chromatography; SDS-PAGE, sodium dodecylsulfate polyacrylamide gel electrophoresis; SEC, size exclusion chromatography; SFC, supercritical fluid chromatography; TCA, trichloroacetic acid; TEM, total extracellular material; TFA, trifluoroacetic acid; TQ, triple quadrupole; Xyl, xylose.
References
Anders, N., Humann, H., Langhans, B., and Spieß, A. C. (2015). Simultaneous determination of acid-soluble biomass-derived compounds using high performance anion exchange chromatography coupled with pulsed amperometric detection. Anal. Methods 7, 7866–7873. doi: 10.1039/C5AY01371B
Antón, J., Meseguer, I., and Rodriguez-Valera, F. (1988). Production of an extracellular polysaccharide by Haloferax mediterranei. Appl. Environ. Microbiol. 54, 2381–2386. doi: 10.1128/aem.54.10.2381-2386.1988
Benninghoff, J. C., Kuschmierz, L., Zhou, X., Albersmeier, A., Pham, T. K., Busche, T., et al. (2021). Exposure to 1-butanol exemplifies the response of the thermoacidophilic Archaeon Sulfolobus acidocaldarius to solvent stress. Appl. Environ. Microbiol. 87:e02988–20. doi: 10.1128/AEM.02988-20
Benninghoff, J. C., Wingender, J., Flemming, H.-C., and Siebers, B. (2017). “Biofilms X-treme: composition of extracellular polymeric substances and exopolysaccharide synthesis in Archaea,” in The Perfect Slime: Microbial Extracellular Polymeric Substances, eds H.-C. Flemming, T. R. Neu, and J. Wingender (Berlin: Springer International), 301–317.
Brock, T. D., Brock, K. M., Belly, R. T., and Weiss, R. L. (1972). Sulfolobus: A new genus of sulfur-oxidizing bacteria living at low pH and high temperature. Arch. Microbiol. 84, 54–68. doi: 10.1007/BF00408082
Choi, K. H., Hwang, S., and Cha, J. (2013). Identification and characterization of MalA in the maltose/maltodextrin operon of Sulfolobus acidocaldarius DSM639. J. Bacteriol. 195, 1789–1799. doi: 10.1128/JB.01713-12
De Swaaf, M. E., Grobben, G. J., Eggink, G., De Rijk, T. C., Van Der Meer, P., and Sijtsma, L. (2001). Characterisation of extracellular polysaccharides produced by Crypthecodinium cohnii. Appl. Microbiol. Biotechnol. 57, 395–400. doi: 10.1007/s002530100788
Dubois, M., Gilles, K. A., Hamilton, J. K., Rebers, P. A., and Smith, F. (1956). Colorimetric method for determination of sugars and related substances. Anal. Chem. 28, 350–356. doi: 10.1021/ac60111a017
Einbu, A., Grasdalen, H., and Varum, K. M. (2007). Kinetics of hydrolysis of chitin/chitosan oligomers in concentrated hydrochloric acid. Carbohydr. Res. 342, 1055–1062. doi: 10.1016/j.carres.2007.02.022
Einbu, A., and Varum, K. M. (2008). Characterization of chitin and its hydrolysis to GlcNAc and GlcN. Biomacromolecules 9, 1870–1875. doi: 10.1021/bm8001123
Felz, S., Vermeulen, P., Van Loosdrecht, M. C. M., and Lin, Y. M. (2019). Chemical characterization methods for the analysis of structural extracellular polymeric substances (EPS). Water Res. 157, 201–208. doi: 10.1016/j.watres.2019.03.068
Flemming, H. C. (2011). The perfect slime. Colloids Surf. B 86, 251–259. doi: 10.1016/j.colsurfb.2011.04.025
Flemming, H. C., Neu, T. R., and Wozniak, D. J. (2007). The EPS matrix: The “house of biofilm cells”. J. Bacteriol. 189, 7945–7947. doi: 10.1128/JB.00858-07
Flemming, H. C., and Wingender, J. (2010). The biofilm matrix. Nat. Rev. Microbiol. 8, 623–633. doi: 10.1038/nrmicro2415
Flemming, H. C., Wingender, J., Szewzyk, U., Steinberg, P., Rice, S. A., and Kjelleberg, S. (2016). Biofilms: An emergent form of bacterial life. Nat. Rev. Microbiol. 14, 563–575. doi: 10.1038/nrmicro.2016.94
Flemming, H. C., and Wuertz, S. (2019). Bacteria and archaea on Earth and their abundance in biofilms. Nat. Rev. Microbiol. 17, 247–260. doi: 10.1038/s41579-019-0158-9
Fröls, S., Dyall-Smith, M., and Pfeifer, F. (2012). Biofilm formation by haloarchaea. Environ. Microbiol. 14, 3159–3174. doi: 10.1111/j.1462-2920.2012.02895.x
Gao, Q., Araia, M., Leck, C., and Emmer, A. (2010). Characterization of exopolysaccharides in marine colloids by capillary electrophoresis with indirect UV detection. Anal. Chim. Acta 662, 193–199. doi: 10.1016/j.aca.2010.01.008
Guan, Z., Delago, A., Nussbaum, P., Meyer, B., Albers, S. V., and Eichler, J. (2016). N-glycosylation in the thermoacidophilic archaeon Sulfolobus acidocaldarius involves a short dolichol pyrophosphate carrier. FEBS Lett. 590, 3168–3178. doi: 10.1002/1873-3468.12341
Hamidi, M., Mirzaei, R., Delattre, C., Khanaki, K., Pierre, G., Gardarin, C., et al. (2019). Characterization of a new exopolysaccharide produced by Halorubrum sp. TBZ112 and evaluation of its anti-proliferative effect on gastric cancer cells. 3 Biotech 9:1. doi: 10.1007/s13205-018-1515-5
Henche, A. L., Koerdt, A., Ghosh, A., and Albers, S. V. (2012). Influence of cell surface structures on crenarchaeal biofilm formation using a thermostable green fluorescent protein. Environ. Microbiol. 14, 779–793. doi: 10.1111/j.1462-2920.2011.02638.x
Honda, S., Akao, E., Suzuki, S., Okuda, M., Kakehi, K., and Nakamura, J. (1989). High-performance liquid chromatography of reducing carbohydrates as strongly ultraviolet-absorbing and electrochemically sensitive 1-phenyl-3-methyl-5-pyrazolone derivatives. Anal. Biochem. 180, 351–357. doi: 10.1016/0003-2697(89)90444-2
Jachlewski, S., Jachlewski, W. D., Linne, U., Bräsen, C., Wingender, J., and Siebers, B. (2015). Isolation of extracellular polymeric substances from biofilms of the thermoacidophilic Archaeon Sulfolobus acidocaldarius. Front. Bioeng. Biotechnol. 3:123. doi: 10.3389/fbioe.2015.00123
Jansson, P.-E., and Lindberg, B. (1983). Structural studies of gellan gum, an extracellular polysaccharide elaborated by Pseudomonas elodea. Carbohydr. Res. 124, 135–139. doi: 10.1016/0008-6215(83)88361-X
Johnson, M. R., Montero, C. I., Conners, S. B., Shockley, K. R., Bridger, S. L., and Kelly, R. M. (2005). Population density-dependent regulation of exopolysaccharide formation in the hyperthermophilic bacterium Thermotoga maritima. Mol. Microbiol. 55, 664–674. doi: 10.1111/j.1365-2958.2004.04419.x
Karygianni, L., Ren, Z., Koo, H., and Thurnheer, T. (2020). Biofilm matrixome: Extracellular components in structured microbial communities. Trends Microbiol. 28, 668–681. doi: 10.1016/j.tim.2020.03.016
Knirel, Y. A., Naumenko, O. I., Senchenkova, S. N., and Perepelov, A. V. (2019). Chemical methods for selective cleavage of glycosidic bonds in the structural analysis of bacterial polysaccharides. Russ. Chem. Rev. 88, 406–424. doi: 10.1070/RCR4856
Koerdt, A., Gödeke, J., Berger, J., Thormann, K. M., and Albers, S.-V. (2010). Crenarchaeal biofilm formation under extreme conditions. PLoS One 5:e14104. doi: 10.1371/journal.pone.0014104
Lee, H. K., Chun, J., Moon, E. Y., Ko, S.-H., Lee, D.-S., Lee, H. S., et al. (2001). Hahella chejuensis gen. nov., sp. nov., an extracellular-polysaccharide-producing marine bacterium. Int. J. Syst. Evol. Microbiol. 51, 661–666. doi: 10.1099/00207713-51-2-661
Li, B., Zhang, N., Wang, D. X., Jiao, L., Tan, Y., Wang, J., et al. (2018). Structural analysis and antioxidant activities of neutral polysaccharide isolated from Epimedium koreanum Nakai. Carbohydr. Polym. 196, 246–253. doi: 10.1016/j.carbpol.2018.05.037
Limoli, D. H., Jones, C. J., and Wozniak, D. J. (2015). Bacterial extracellular polysaccharides in biofilm formation and function. Microbiol. Spectr. 3, 233–247. doi: 10.1128/9781555817466.ch11
López-Ortega, M. A., Rodríguez-Hernández, A. I., Camacho-Ruíz, R. M., Córdova, J., López-Cuellar, M. D. R., Chavarría-Hernández, N., et al. (2020). Physicochemical characterization and emulsifying properties of a novel exopolysaccharide produced by haloarchaeon Haloferax mucosum. Int. J. Biol. Macromol. 142, 152–162. doi: 10.1016/j.ijbiomac.2019.09.087
Lü, Y., Lu, H., Wang, S., Han, J., Xiang, H., and Jin, C. (2017). An acidic exopolysaccharide from Haloarcula hispanica ATCC33960 and two genes responsible for its synthesis. Archaea 2017:5842958. doi: 10.1155/2017/5842958
Mata, J. A., Bejar, V., Bressollier, P., Tallon, R., Urdaci, M. C., Quesada, E., et al. (2008). Characterization of exopolysaccharides produced by three moderately halophilic bacteria belonging to the family Alteromonadaceae. J. Appl. Microbiol. 105, 521–528. doi: 10.1111/j.1365-2672.2008.03789.x
Mata, J. A., Bejar, V., Llamas, I., Arias, S., Bressollier, P., Tallon, R., et al. (2006). Exopolysaccharides produced by the recently described halophilic bacteria Halomonas ventosae and Halomonas anticariensis. Res. Microbiol. 157, 827–835. doi: 10.1016/j.resmic.2006.06.004
Meyer, M., Montero, L., Meckelmann, S. W., and Schmitz, O. J. (2022). Comparative study for analysis of carbohydrates in biological samples. Anal. Bioanal. Chem. 414, 2117–2130. doi: 10.1007/s00216-021-03845-z
Moshynets, O. V., and Spiers, A. J. (2016). “Viewing biofilms within the larger context of bacterial aggregations,” in Microbial Biofilms - Importance and Applications, eds D. Dhanasekaran and N. Thajuddin (London: InTech). doi: 10.5772/62912
Nicolaus, B., Manca, M. C., Romano, I., and Lama, L. (1993). Production of an exopolysaccharide from two thermophilic archaea beloning to the genus Sulfolobus. FEMS Microbiol. Lett. 109, 203–206. doi: 10.1111/j.1574-6968.1993.tb06168.x
Orell, A., Peeters, E., Vassen, V., Jachlewski, S., Schalles, S., Siebers, B., et al. (2013). Lrs14 transcriptional regulators influence biofilm formation and cell motility of Crenarchaea. ISME J. 7, 1886–1898. doi: 10.1038/ismej.2013.68
Orell, A., Tripp, V., Aliaga-Tobar, V., Albers, S. V., Maracaja-Coutinho, V., and Randau, L. (2018). A regulatory RNA is involved in RNA duplex formation and biofilm regulation in Sulfolobus acidocaldarius. Nucleic Acids Res. 46, 4794–4806. doi: 10.1093/nar/gky144
Paramonov, N. A., Parolis, L. A. S., Parolis, H., Boan, I. F., Antón, J., and Rodriguez-Valera, F. (1998). The structure of the exocellular polysaccharide produced by the Archaeon Haloferax gibbonsii (ATCC 33959). Carbohydr. Res. 309, 89–94. doi: 10.1016/S0008-6215(98)00102-5
Parolis, H., Parolis, L. A. S., Boan, I. F., Rodriguez-Valera, F., Widmalm, G., Manca, M. C., et al. (1996). The structure of the exopolysaccharide produced by the halophilic Archaeon Haloferax mediterranei strain R4 (ATCC 33500). Carbohydr. Res. 295, 147–156. doi: 10.1016/S0008-6215(96)90134-2
Parolis, L. A. S., Parolis, H., Paramonov, N. A., Boan, I. F., Antón, J., and Rodriguez-Valera, F. (1999). Structural studies on the acidic exopolysaccharide from Haloferax denitrificans ATCC 35960. Carbohydr. Res. 319, 133–140. doi: 10.1016/S0008-6215(99)00111-1
Parra-Riofrío, G., García-Márquez, J., Casas-Arrojo, V., Uribe-Tapia, E., and Abdala-Díaz, R. T. (2020). Antioxidant and cytotoxic effects on tumor cells of exopolysaccharides from Tetraselmis suecica (Kylin) butcher grown under autotrophic and heterotrophic conditions. Mar. Drugs 18:534. doi: 10.3390/md18110534
Peterson, G. L. (1977). A simplification of the protein assay method of Lowry et al. which is more generally applicable. Anal. Biochem. 83, 346–356. doi: 10.1016/0003-2697(77)90043-4
Peyfoon, E., Meyer, B., Hitchen, P. G., Panico, M., Morris, H. R., Haslam, S. M., et al. (2010). The S-layer glycoprotein of the crenarchaeote Sulfolobus acidocaldarius is glycosylated at multiple sites with chitobiose-linked N-glycans. Archaea 2010:754101. doi: 10.1155/2010/754101
Qamar, S. A., Asgher, M., and Bilal, M. (2021). Sustainable production, optimization, and partial characterization of exopolysaccharides by Macrococcus brunensis. Waste Biomass Valor. 12, 6847–6859. doi: 10.1007/s12649-021-01479-x
Ras, M., Lefebvre, D., Derlon, N., Paul, E., and Girbal-Neuhauser, E. (2011). Extracellular polymeric substances diversity of biofilms grown under contrasted environmental conditions. Water Res. 45, 1529–1538. doi: 10.1016/j.watres.2010.11.021
Recalde, A., Van Wolferen, M., Sivabalasarma, S., Albers, S. V., Navarro, C. A., and Jerez, C. A. (2021). The role of polyphosphate in motility, adhesion, and biofilm formation in Sulfolobales. Microorganisms 9:193. doi: 10.3390/microorganisms9010193
Reichhardt, C., Jacobs, H. M., Matwichuk, M., Wong, C., Wozniak, D. J., and Parsek, M. R. (2020). The versatile Pseudomonas aeruginosa biofilm matrix protein CdrA promotes aggregation through different extracellular exopolysaccharide interactions. J. Bacteriol. 202:e00216–e00220. doi: 10.1128/JB.00216-20
Rinker, K. D., and Kelly, R. M. (1996). Growth physiology of the hyperthermophilic Archaeon Thermococcus litoralis: Development of a sulphur-free defined medium, characterization of an exopolysaccharide, and evidence of biofilm formation. Appl. Environ. Microbiol. 62, 4478–4485. doi: 10.1128/aem.62.12.4478-4485.1996
Ruhaak, L. R., Zauner, G., Huhn, C., Bruggink, C., Deelder, A. M., and Wuhrer, M. (2010). Glycan labeling strategies and their use in identification and quantification. Anal. Bioanal. Chem. 397, 3457–3481. doi: 10.1007/s00216-010-3532-z
Rühmann, B., Schmid, J., and Sieber, V. (2015). High throughput exopolysaccharide screening platform: From strain cultivation to monosaccharide composition and carbohydrate fingerprinting in one day. Carbohydr. Polym. 122, 212–220. doi: 10.1016/j.carbpol.2014.12.021
Ryder, C., Byrd, M., and Wozniak, D. J. (2007). Role of polysaccharides in Pseudomonas aeruginosa biofilm development. Curr. Opin. Microbiol. 10, 644–648. doi: 10.1016/j.mib.2007.09.010
Seviour, T., Derlon, N., Dueholm, M. S., Flemming, H.-C., Girbal-Neuhauser, E., Horn, H., et al. (2019). Extracellular polymeric substances of biofilms: Suffering from an identity crisis. Water Res. 151, 1–7. doi: 10.1016/j.watres.2018.11.020
Simon, S., Pairo, B., Villain, M., D’abzac, P., Van Hullebusch, E., Lens, P., et al. (2009). Evaluation of size exclusion chromatography (SEC) for the characterization of extracellular polymeric substances (EPS) in anaerobic granular sludges. Bioresour. Technol. 100, 6258–6268. doi: 10.1016/j.biortech.2009.07.013
Squillaci, G., Finamore, R., Diana, P., Restaino, O. F., Schiraldi, C., Arbucci, S., et al. (2016). Production and properties of an exopolysaccharide synthesized by the extreme halophilic archaeon Haloterrigena turkmenica. Appl. Microbiol. Biotechnol. 100, 613–623. doi: 10.1007/s00253-015-6991-5
Strathmann, M., Wingender, J., and Flemming, H. C. (2002). Application of fluorescently labelled lectins for the visualization and biochemical characterization of polysaccharides in biofilms of Pseudomonas aeruginosa. J. Microbiol. Methods 50, 237–248. doi: 10.1016/S0167-7012(02)00032-5
Sutherland, I. W. (2017). “EPS - a complex mixture,” in The Perfect Slime: Microbial Extracellular Polymeric Substances, eds H.-C. Flemming, T. R. Neu, and J. Wingender (Berlin: Springer International), 15–24. doi: 10.2166/9781780407425
Tielen, P., Kuhn, H., Jaeger, K. E., Flemming, H. C., and Wingender, J. (2013). Interaction between the extracellular lipase LipA and the polysaccharide alginate of Pseudomonas aeruginosa. BMC Microbiol. 13:159. doi: 10.1186/1471-2180-13-159
Van Wolferen, M., Orell, A., and Albers, S.-V. (2018). Archaeal biofilm formation. Nat. Rev. Microbiol. 16, 699–713. doi: 10.1038/s41579-018-0058-4
Vanysacker, L., Denis, C., Declerck, P., Piasecka, A., and Vankelecom, I. F. (2013). Microbial adhesion and biofilm formation on microfiltration membranes: A detailed characterization using model organisms with increasing complexity. Biomed Res. Int. 2013:470867. doi: 10.1155/2013/470867
Veiga, M. C., Jain, M. K., Wu, W.-M., Hollingsworth, R. I., and Zeikus, J. G. (1997). Composition and role of extracellular polymers in methanogenic granules. Appl. Environ. Microbiol. 63, 403–407. doi: 10.1128/aem.63.2.403-407.1997
Wagner, M., Shen, L., Albersmeier, A., Van Der Kolk, N., Kim, S., Cha, J., et al. (2018). Sulfolobus acidocaldarius transports pentoses via a carbohydrate uptake transporter 2 (CUT2)-type ABC transporter and metabolizes them through the aldolase-independent Weimberg pathway. Appl. Environ. Microbiol. 17:e01273–17. doi: 10.1128/AEM.01273-17
Wagner, M., Van Wolferen, M., Wagner, A., Lassak, K., Meyer, B. H., Reimann, J., et al. (2012). Versatile genetic tool box for the crenarchaeote Sulfolobus acidocaldarius. Front. Microbiol. 3:214. doi: 10.3389/fmicb.2012.00214
Wagner, M., Wagner, A., Ma, X., Kort, J. C., Ghosh, A., Rauch, B., et al. (2014). Investigation of the malE promoter and MalR, a positive regulator of the maltose regulon, for an improved expression system in Sulfolobus acidocaldarius. Appl. Environ. Microbiol. 80, 1072–1081. doi: 10.1128/AEM.03050-13
Waheed, H., Mehmood, C. T., Yang, Y., Tan, W., Fu, S., and Xiao, Y. (2022). Dynamics of biofilms on different polymeric membranes – A comparative study using five physiologically and genetically distinct bacteria. J. Membr. Sci. 642:120000. doi: 10.1016/j.memsci.2021.120000
Wang, J., Salem, D. R., and Sani, R. K. (2019). Extremophilic exopolysaccharides: A review and new perspectives on engineering strategies and applications. Carbohydr. Polym. 205, 8–26. doi: 10.1016/j.carbpol.2018.10.011
Whitchurch, C. B., Tolker-Nielsen, T., Ragas, P. C., and Mattick, J. S. (2002). Extracellular DNA required for bacterial biofilm formation. Science 295:1487. doi: 10.1126/science.295.5559.1487
Wingender, J., Strathmann, M., Rode, A., Leis, A., and Flemming, H. C. (2001). Isolation and biochemical characterization of extracellular polymeric substances from Pseudomonas aeruginosa. Methods Enzymol. 336, 302–314. doi: 10.1016/S0076-6879(01)36597-7
Zhang, R., Bellenberg, S., Castro, L., Neu, T. R., Sand, W., and Vera, M. (2014). Colonization and biofilm formation of the extremely acidophilic archaeon Ferroplasma acidiphilum. Hydrometallurgy 150, 245–252. doi: 10.1016/j.hydromet.2014.07.001
Zhang, R., Neu, T. R., Li, Q., Blanchard, V., Zhang, Y., Schippers, A., et al. (2019). Insight into interactions of thermoacidophilic Archaea with elemental sulfur: Biofilm dynamics and EPS analysis. Front. Microbiol. 10:896. doi: 10.3389/fmicb.2019.00896
Zhang, R., Neu, T. R., Zhang, Y., Bellenberg, S., Kuhlicke, U., Li, Q., et al. (2015). Visualization and analysis of EPS glycoconjugates of the thermoacidophilic archaeon Sulfolobus metallicus. Appl. Microbiol. Biotechnol. 99, 7343–7356. doi: 10.1007/s00253-015-6775-y
Keywords: Sulfolobus acidocaldarius, Archaea, biofilm, extracellular polymeric substances, exopolysaccharide, reversed-phase liquid chromatography, size exclusion chromatography
Citation: Kuschmierz L, Meyer M, Bräsen C, Wingender J, Schmitz OJ and Siebers B (2022) Exopolysaccharide composition and size in Sulfolobus acidocaldarius biofilms. Front. Microbiol. 13:982745. doi: 10.3389/fmicb.2022.982745
Received: 30 June 2022; Accepted: 30 August 2022;
Published: 26 September 2022.
Edited by:
Dennis W. Grogan, University of Cincinnati, United StatesReviewed by:
Andrea Koerdt, Federal Institute for Materials Research and Testing (BAM), GermanyMaria Michela Corsaro, University of Naples Federico II, Italy
Copyright © 2022 Kuschmierz, Meyer, Bräsen, Wingender, Schmitz and Siebers. This is an open-access article distributed under the terms of the Creative Commons Attribution License (CC BY). The use, distribution or reproduction in other forums is permitted, provided the original author(s) and the copyright owner(s) are credited and that the original publication in this journal is cited, in accordance with accepted academic practice. No use, distribution or reproduction is permitted which does not comply with these terms.
*Correspondence: Bettina Siebers, YmV0dGluYS5zaWViZXJzQHVuaS1kdWUuZGU=; Oliver J. Schmitz, b2xpdmVyLnNjaG1pdHpAdW5pLWR1ZS5kZQ==
†These authors have contributed equally to this work and share first authorship
‡ORCID: Martin Meyer, orcid.org/0000-0002-7360-1849; Jost Wingender, orcid.org/0000-0003-3143-4760; Oliver J. Schmitz, orcid.org/0000-0003-2184-1207; Bettina Siebers, orcid.org/0000-0002-9905-541X