- 1Laboratorio de Fisiología y Genética de Bacterias Lácticas, Instituto de Biología Molecular y Celular de Rosario (IBR), Sede Facultad de Ciencias Bioquímicas y Farmacéuticas (FBioyF), Universidad Nacional de Rosario (UNR), Consejo Nacional de Ciencia y Tecnología (CONICET), Rosario, Argentina
- 2Área Bioinformática, Departamento de Matemática y Estadística, Facultad de Ciencias Bioquímicas y Farmacéuticas, Universidad Nacional de Rosario, Rosario, Santa Fe, Argentina
- 3Department of General Microbiology, Georg August University, Göttingen, Germany
- 4Laboratorio de Biotecnología e Inocuidad de los Alimentos, Área de Biotecnología de los Alimentos, FBioyF, UNR–Municipalidad de Granadero Baigorria, Rosario, Argentina
Enterococcus is able to grow in media at pH from 5.0 to 9.0 and a high concentration of NaCl (8%). The ability to respond to these extreme conditions requires the rapid movement of three critical ions: proton (H+), sodium (Na+), and potassium (K+). The activity of the proton F0F1 ATPase and the sodium Na+ V0V1 type ATPase under acidic or alkaline conditions, respectively, is well established in these microorganisms. The potassium uptake transporters KtrI and KtrII were described in Enterococcus hirae, which were associated with growth in acidic and alkaline conditions, respectively. In Enterococcus faecalis, the presence of the Kdp (potassium ATPase) system was early established. However, the homeostasis of potassium in this microorganism is not completely explored. In this study, we demonstrate that Kup and KimA are high-affinity potassium transporters, and the inactivation of these genes in E. faecalis JH2-2 (a Kdp laboratory natural deficient strain) had no effect on the growth parameters. However, in KtrA defective strains (ΔktrA, ΔkupΔktrA) an impaired growth was observed under stress conditions, which was restored to wild type levels by external addition of K+ ions. Among the multiplicity of potassium transporters identify in the genus Enterococcus, Ktr channels (KtrAB and KtrAD), and Kup family symporters (Kup and KimA) are present and may contribute to the particular resistance of these microorganisms to different stress conditions. In addition, we found that the presence of the Kdp system in E. faecalis is strain-dependent, and this transporter is enriched in strains of clinical origin as compared to environmental, commensal, or food isolates.
1. Introduction
Enterococcus faecalis is a natural commensal bacterium of the animal gut that can be isolated from diverse niches (water, food, and vegetal; Giraffa, 2002; Byappanahalli et al., 2012). The physiology and genetics of this species are relevant due to its association with infections and diseases, such as bacteremia, endocarditis, urinary tract infections, and dental diseases (Murray, 1990; García-Solache and Rice, 2019). This group of microorganisms emerges as multi-resistant Gram-positive pathogens and, generally, is not regarded as safe (GRAS; Ogier and Serror, 2008). Despite this categorization, E. faecalis is present in fermented food productions, isolated mainly from traditional dairy products around the world where these bacteria contribute to the ripening process in favorable sensorial features (Giraffa, 2002; Franz et al., 2003). Finally, this microorganism is an important fecal contaminant frequently used as biological indicator for assessing recreational water quality (Boehm and Sassoubre, 2014). Like other members of the lactic acid bacteria, Enterococcus has the capability to persist, resist, and thrive in harsh conditions that include low and high pH, heat, and hyperosmotic stress. Members of this genus generally grow at temperatures between 10 and 45°C, in NaCl between 0 and 8% and pH values between 4.5 and 9.0 (Giraffa, 2002; Ogier and Serror, 2008; Byappanahalli et al., 2012).
The main biochemistry studies regarding potassium uptake in Enterococci were performed in Enterococcus hirae ATCC9790 (Kakinuma, 1998; Krulwich et al., 2011). In this strain, it was well established that the generation of proton-motive force at low pH is generated by proton expulsion via the F0F1 proton translocating ATPase (atpIABCDFE operon), extrusion of Na+ is catalyzed by NapA antiporter, and the accumulation of cytoplasmic K+ catalyzed by KtrI system (Kakinuma, 1998; Krulwich et al., 2011; Figure 1). In alkaline conditions, and in the presence of Na+, E. hirae induces the vacuolar V0V1 type sodium—ATPase (ntpFIKECGABDJ operon) which includes a K+ transporter KtrII system (last gene of the operon, ntpJ). Thus, Na+ is pumped outside the cells consuming ATP whereas K+ is accumulated via the K+/Na+ symporter (Kakinuma, 1998; Krulwich et al., 2011; Figure 1).
In the last decade, different K+ channels and transporters were characterized in pathogenic and nonpathogenic microorganisms (Stautz et al., 2021). In Bacillus subtilis, the KtrAB and KtrCD potassium channels as well as the KimA K+/H+ symporter were characterized (Gundlach et al., 2017). The KtrAB channel and the KimA transporter are high-affinity uptake systems whereas the KtrCD channel has a low affinity unless stimulated by glutamate (Krüger et al., 2020). In Staphylococcus aureus, the KdpFABC pump and KtrCB and KtrCD channels are involved in the response to osmotic shock (Gries et al., 2013, 2016; Price-Whelan et al., 2013). In Streptococcus pneumoniae, the trkH cabP operon encodes a member of the Trk/Ktr/HKT K+ channel family (Bai et al., 2014), and in Streptococcus mutans, coded by the trkAH operon (similar to described in S. pneumoniae), was defined as the main system involved in the K+ uptake in the response to acid stress (Binepal et al., 2016). In Streptococcus agalactiae, two members of the Trk/Ktr/HKT family are involved in the uptake of K+ (Devaux et al., 2018). In Streptococcus pyogenes, the KtrAB system have a main role in K+ uptake, while Kup and KimA may have functions under different growth conditions (Faozia et al., 2021). Finally, in Lactococcus, two copies of Kup (KupA and KupB) were found in the Lactococcus lactis IL1403 strain and only one in the Lactococcus cremoris MG1363 (KupB; Pham et al., 2018; Quintana et al., 2019).
The second messenger c-di-AMP was described as involved in the regulation of K+ homeostasis in Firmicutes (Corrigan and Gründling, 2013; Stülke and Krüger, 2020; Stautz et al., 2021). Specific interactions between potassium transporters and c-di-AMP were found, c-di-AMP binds to the interface of the KtrA dimer, and inactivates the KtrAB channel (Kim et al., 2015; Rocha et al., 2019). Also, direct interaction of c-di-AMP to KimA (Gibhardt et al., 2019; Gundlach et al., 2019), Kup (Quintana et al., 2019), and CabP (TrkH; Bai et al., 2014; Devaux et al., 2018) was found (Faozia et al., 2021). Additionally, it has been demonstrated that both c-di-AMP and the K+ transporters are directly and indirectly involved in the virulence of pathogenic microorganisms (Corrigan and Gründling, 2013; Gries et al., 2013; Stülke and Krüger, 2020; Kundra et al., 2021; Stautz et al., 2021). In E. faecalis inactivation of the gene encoding for CdaA (cdaA, diadenylate cyclase involved in the synthesis of c-di-AMP) or PDE phosphodiesterases (gdpP and dhh, encoding the enzymes that degrade c-di-AMP) result in the reduction of virulence and capacity to resist osmotic stress (Kundra et al., 2021).
Initial studies of potassium transport systems in E. faecalis V583 identified the kdpED operon, which encodes a two-component regulatory system (KdpD sensor kinase and KdpE transcriptional factor) widely distributed in bacteria, responsible for the transcriptional induction of the KdpFABC transporter complex (potassium-transporting ATPase; Hancock and Perego, 2004). It is also known that the transcriptional induction of kdp operon is coordinated with the V type ntp ATPase operon in response to the increased salt concentration, demonstrating the importance of the K+ uptake and Na+ extrusion in the ion homeostasis of the enterococcal cells (Solheim et al., 2014).
The purpose of this study was to study which K+ transporters are employed in the uptake of this ion in the kdp-defective E. faecalis JH2-2 strain and analyze the impact of the second messenger c-di-AMP on the activity of these proteins. Bioinformatics analyses were performed to show the distribution of four putative potassium transport systems identified in E. faecalis JH2-2: KtrAB, KtrAD, KimA, and Kup. In this study, we show that the E. faecalis Kup and KimA proteins are specific high affinity potassium transporters. We also demonstrate that Kup is inhibited by c-di-AMP in vivo. Moreover, genetic approaches were performed in the E. faecalis JH2-2 strain in order to characterize the multiple physiological roles of each potassium transporter system. We determined that KtrA is required to resist the growth at high pH and concentration of osmolites (NaCl, sorbitol). For the first time, we found evidence that Kup is also required to grow in complex medium at high pH under a limited K+ concentration and to resist osmotic stress.
2. Materials and methods
2.1. Bacterial strains and cultures
The bacterial strains used in this study are listed in Supplementary Table S1. Escherichia coli strain DH5α was used as an intermediate host for cloning, and E. coli EC101 was used as host for pBVGh constructs. Escherichia coli strains were grown at 37°C under aerobic conditions with vigorous shaking in Lysogeny Broth medium (LB). Potassium transporter deficient E. coli LB650 and LB2003 strains were cultivated in LB medium with the addition of 50 mM KCl or in minimal salts M9/M9mod medium supplemented with 50 mM KCl, unless otherwise stated. M9 medium contains 4 mM Na2HPO4, 22 mM KH2PO4, 18.5 mM NH4Cl, 1 mM MgSO4, 0.1 mM CaCl2, 0.5 μM FeCl3, 350 μM proline, 3 μM thiamine-di-chloride, 0.66% Casamino Acids, and 0.5% glucose or 0.2% glycerol as sources of carbon. Experiments with defined potassium concentrations were performed in M9mod, in which M9 potassium salts were replaced with equimolar quantities of sodium salts, and KCl was added as indicated. Enterococcus faecalis strains were routinely grown at 37°C without shaking in LB medium containing 0.5% w/v glucose (LBG). Alternatively, M17 medium (Oxoid) supplemented with 0.5% w/v glucose (M17G) and M17G supplemented with 0.5 M saccharose (SM17G) were employed when indicated. Experiments requiring low K+ medium were performed in mLBG, a modified LBG medium in which yeast extract was reduced from 0.5 to 0.025%; the K+ concentration of mLBG was less than 1 mM (Kawano et al., 2001). KCl, NaCl, and/or sorbitol were added to the growth media at the concentrations indicated in the figure legends. pH adjustments were made with addition of either HCl or NaOH. Suitable antibiotics were added to the media as selective agents when needed. For agar plates, 1.5% agar was added to the medium.
2.2. DNA manipulation
Plasmid DNA from E. coli cells was prepared with a Wizard Plus Minipreps DNA purification system (Promega). Chromosomal DNA of E. faecalis was extracted using Microbial DNA Kit (Macherey-Nagel). Treatment of DNA with restriction enzymes, T4 DNA ligase, and DNA polymerases was performed as recommended by the suppliers. DNA fragments were purified using the Wizard SV Gel and PCR Clean-Up System (Promega). DNA sequences were checked by sequencing in the World Meridian Venture Center, Macrogen’s sequencing service (Seoul, Korea). Escherichia coli cells were transformed using standard procedures (Sambrook et al., 1989). Enterococcus faecalis transformation was performed by electroporation according to the procedure of Dornan and Collins (1990).
2.3. Plasmid construction
Heterologous expression of the putative potassium transporter proteins in E. coli was approached as follows: the kup and kimA genes from E. faecalis JH2-2 were amplified using the oligonucleotide pairs GA1/GA2 and GA3/GA4, respectively. The PCR products were cloned between the BamHI and SalI sites of plasmid pWH844 to allow IPTG-inducible expression. The resulting plasmids were designated as pWH-kup and pWH-kimA. The pBAD33 and pWH844 expression vectors have compatible selection markers and origin of replications allowing the co-expression of potassium transporter genes (from pWH844) and the cdaA variants (from pBAD33; Gibhardt et al., 2019; Quintana et al., 2019).
2.4. Growth of Escherichia coli in minimal salt medium
Strains derived from E. coli LB650 (ΔkdpABC5, ΔtrkD1, ΔtrkH,ΔktrG, Km50, and Cm30, see Supplementary Table S1) were propagated twice from −80°C stocks in M9mod medium supplemented with 1% glucose, the corresponding antibiotics, and 50 mM KCl. The overnight culture obtained was used to inoculate fresh M9mod medium supplemented with antibiotics, and 10 mM KCl for strains expressing the Kup and KimA proteins or 100 mM KCl for the strain expressing the empty vector control. At an OD600 of 0.5, cells were harvested. The pellet was re-suspended in the initial volume of fresh medium without KCl addition and incubated for 1 h at 37°C. Cultures were harvested again and washed three times with fresh medium. These samples were then used to inoculate fresh M9mod medium in microplates supplemented with 1% glucose, antibiotics, and different KCl concentrations. Microplates were incubated at 37°C with continuous orbital shaking and culture growth was monitored by measuring OD600 in 15-min intervals in a PowerWave™ XS Microplate reader (BioTek, BioTek Instrument Inc., Vermont, United States).
2.5. Co-expression of potassium transporters and diadenylate cyclases
Strain E. coli LB2003 (ΔkdpABC5, kupD1, and ΔtrkA), a clean deficient in the gene encoding potassium transporter but sensible to antibiotic which allow the introduction of plasmids encoding kanamycin or chloramphenicol resistance determinants was co-transformed with pWH844 or derivatives and the pBAD33 derivatives (see Supplementary Table S1). This strain was inoculated from −80°C stocks in M9mod medium supplemented with 0.2% glycerol as the carbon source, corresponding antibiotics, and 10 mM KCl and propagated twice. At an OD600 of 0.5, cultures were harvested and re-suspended in same volume of M9mod medium containing 0.2% glycerol (no KCl added). Samples were incubated at 37°C for 1 h, after which two wash steps were performed. These washed samples were used for microplate inoculation supplemented with 0.075 mM KCl, and 0.05% L-arabinose when indicated. Antibiotics were added as appropriate. Microplates were incubated at 37°C with continuous orbital shaking and culture growth was monitored by measuring OD600 in 15-min intervals in a PowerWave™ XS Microplate reader (BioTek Instrument Inc., Vermont, United States).
2.6. Construction of Enterococcus faecalis potassium transporter defective strains and complementation of the mutants
Deletion of kup, kimA, and ktrA from E. faecalis JH2-2 was carried out using the thermosensitive vector pBVGh (Blancato and Magni, 2010). The oligonucleotides used for the amplification of upstream and downstream fragments of kup, kimA, and ktrA genes are indicated in Supplementary Table S1. Fragments were purified, digested, and ligated into the corresponding sites of the pBVGh vector. Cloned fragments were checked by sequencing. Finally, the protocol to generate the chromosomal deletion in E. faecalis was followed as previously described (Blancato and Magni, 2010). The Δkup ΔkimA, Δkup ΔktrA, and ΔkimA ΔktrA double mutants were obtained by eletroporating the pBVGh-kimA plasmid in the Δkup and ΔktrA single mutants, and the pBVGh-ktrA plasmid in the Δkup single mutant. All gene deletions were confirmed by PCR sequencing using a pair of primers hybridizing in the adjacent up and downstream genes (Supplementary Table S1). The full-length ktrA gene was amplified by PCR using chromosomal DNA extracted from E. facealis JH2-2 as template and the pair of primers GA19/GA20 (Supplementary Table S1). The resulting fragment was purified, digested with the appropriate restriction enzymes, and ligated into the pBV153 vector giving rise to plasmid pBV-ktrA. The ΔkupΔktrA and ΔkimAΔktrA double mutants were complemented by the plasmid pBV-ktrA. Vector pBV153 was also transformed into the wild-type and the mutant strains as a control of any possible effect of plasmid transformation alone.
2.7. Growth of Enterococcus faecalis strains under stress conditions
In order to compare the growth curves of wild-type and the different potassium transporter mutant strains, E. faecalis was cultivated in microplates at 37°C. For this, overnight cultures grown in LBG medium were used to inoculate fresh LBG medium adjusted to different initial pH values, as indicated. The inoculums were diluted to an initial OD600 of 0.1. After the cell culture had reached the exponential growth phase, the cells were harvested and washed twice with fresh medium. These samples were then diluted to an initial OD600 of 0.1 in LBG or mLBG medium at different initial pH, and supplemented with 10 mM KCl when indicated. The OD600 was registered every 20 min in a PowerWave™ XS Microplate reader.
For osmotolerance assays, cells were cultured as described above except with the addition of either NaCl or sorbitol, and KCl when indicated, at the specified concentrations. The growth rates were calculated and plotted against the NaCl concentrations, which ranged between 0 and 8%.
Growth in urine or blood was analyzed as described previously (Martino et al., 2018). Infection and survival experiments in Galleria mellonella were carried out as described (Terán et al., 2022).
2.8. Bioinformatic analysis
Genomic sequence data sets as well as predicted coding sequences of E. faecalis strains were retrieved from GenBank1 using Download Genomes tool.2 Datasets were composed of sequences submitted until 10 October 2020. Maximum likelihood phylogenetic trees were constructed as reported (Espariz et al., 2016). To counter-select potential paralogues, coverage and identity percentage cut-offs were set at 70% using GeM-Pro tool (Torres Manno et al., 2019). Metadata from NCBI biosample were obtained via ad hoc R script.3 Strains were classified based on these metadata in the categories such as clinical, commensal, environmental, or food sample.
For presence/absence analysis, the sequences of KimA, KupA, KtrA, KtrB, KtrD, KdpFABC, KdpDE, TrkHA, AtpIABCDFE, NtpFIKECGABDJ, and NapA proteins were used as query in TBLASTN (Blast +2.9.0) searches against E. faecalis genomes using as thresholds coverages > = 70% and identities > = 50%. In order to determine associations between protein-coding genes and metadata-assigned categories, enrichment statistical analyses were performed with PhyloLM V2.6 (Levy et al., 2018) using “logistic_IG10” method. The multiple comparisons for the statistical tests were corrected with Benjamini–Hochberg approach (Benjamini and Hochberg, 1995).
3. Results
3.1. KimA and Kup of Enterococcus faecalis encode high affinity potassium transporters
Potassium transport systems present in E. faecalis JH2-2 (a natural deficient in the Kdp system strain) were identified based on homology with the proteins present in strain V583. Strain JH2-2 codes for the KtrAB and KtrAD channels and two members of the Kup family: Kup and KimA (Table 1). To test the functional role of the enterococcal proteins KimA and Kup, the E. faecalis JH2-2 kup and kimA genes were individually cloned in the pWH844 vector (Figure 2A). The resulting pWH-kup (kup) and pWH-kimA (kimA) plasmids were introduced in the E. coli strain LB650 that lacks the main potassium transport systems KdpABC, Kup (formerly TrkD, TrkH, and TrkG and is unable to grow at low K+ concentrations; Schlosser et al., 1995; Supplementary Table S1). The transformants were used to evaluate whether the expression of these enterococcal proteins could restore growth in minimal salt media when no K+ salts are added. As shown in Figure 2B, strain LB650 harboring the empty vector pWH844 was unable to grow in M9 minimal salt medium plates (M9mod), in which potassium salts were replaced by equimolar quantities of the respective sodium salts (see the section Materials and methods), without or in the presence of both 1 and 5 mM of KCl. Colonies of the triple mutant LB650 transformed with the vector pWH844 appear on the plates only when the concentration of KCl added reaches 50 mM (Figure 2B, colonies number 1 in each plate). Escherichia coli strain LB650/pWH-kup grows in M9mod independent of the addition of external K+ salt (Figure 2B, colonies number 2 in each plate), whereas strain LB650/pWH-kimA recovered ability to grow in M9mod in the presence of 1 mM KCl in solid medium (Figure 2B, colonies number 3 in each plate). In all cases, the cells were propagated in M9mod plates without IPTG induction. Thus, the basal expression of each of the enterococcal genes, due to readthrough of the lac promoter present in the vector pWH844, is already sufficient to allow potassium uptake and, consequently, growth of the complemented mutant strains.
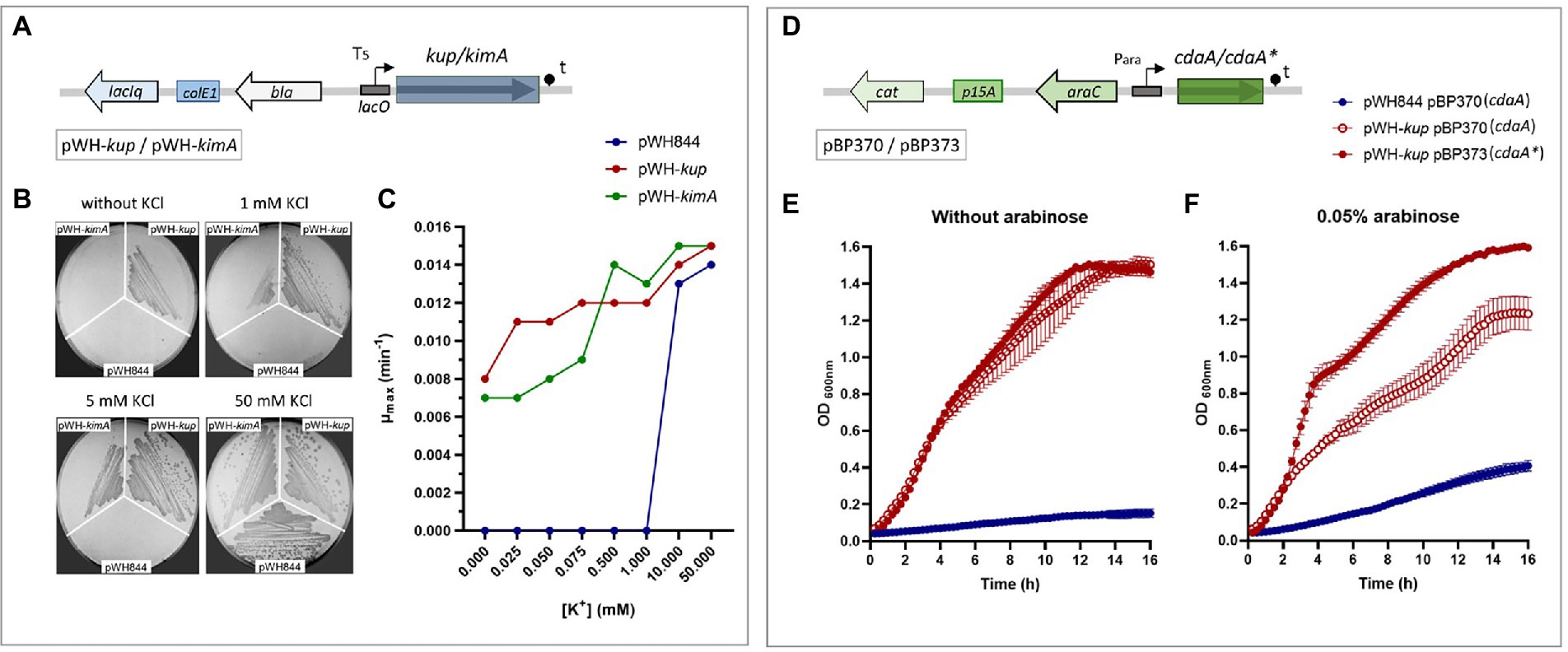
Figure 2. KimA and Kup of Enterococcus faecalis are potassium transporters. (A) pWH844 derived carrying full copy of Kup (pWH-kup) or KimA (pWH-kimA) used in this study. (B) Escherichia coli LB650 strains harboring plasmids pWH844, pWH-kup, and pWH-kimA were grown in M9-mod solid medium with or without supplementation of KCl (1, 5, or 50 mM). (C) Growth rate parameters determined at different KCl concentrations of LB650 derived strains, pWH844 (blue dot), pWH-kup (red dot), and pWH-kimA (green dot). Inhibition of Kup activity by c-di-AMP (D) pBAD derived carrying a copy of cdaAlmo (pBP370) or cdaAlmo* (pBP373) used in this study. (E,F) Escherichia coli LB2003 harboring the plasmid combinations pWH844/pBP370 (blue dot), pWH-kup/pBP370 (empty red dot), and pWH-kup/pBP373 (full red dot), was cultivated in minimal salt M9mod medium, and supplemented without arabinose (E) or with arabinose 0.05% (F).
In addition, growth curves were determined in liquid M9mod medium with external additions of KCl to reach final concentrations between 0.025 and 50 mM. The growth parameters maximum optical density (ODmax) and μmax (Supplementary Table S2) were determined for the strains LB650/pWH844, LB650/pWH-kup, and LB650/pWH-kimA. Then, the μmax were plotted against the potassium concentrations added to the medium (Figure 2C). The strains carrying the full copies of each enterococcal gene, kup or kimA, showed similar patterns in the variation of the values of μmax vs. the K+ concentration. Both transporters allowed growth even at very low concentrations of KCl and, furthermore, it can be seen as μmax increases as the concentration of K+ in the medium increases. However, the latter is not consistent with the ODmax values, as this parameter remains constant throughout the curve (Supplementary Table S2). The addition of the inducer IPTG in the medium produces a defect on the growth of the cells containing kup or kimA genes, that could be because overexpression of membrane proteins is often toxic and interferes with essential functions or because of an accumulation of toxic levels of potassium ions inside the cell (data not shown).
Recent studies have demonstrated that several members of the Kup transporter family (KimA from B. subtilis and Listeria monocytogenes, KupA and KupB from L. lactis) are negatively regulated by direct interaction with the nucleotide second messenger c-di-AMP (Gibhardt et al., 2019; Gundlach et al., 2019; Quintana et al., 2019). To test whether c-di-AMP impacts on the potassium transport activity of Kup and KimA, a co-expression system was established in the defective potassium transporter E. coli strain LB2003. Like E. coli LB650, this strain is deficient in the Trk, Kup, and Kdp potassium transport systems, hence is unable to grow in low K+ medium without complementation of a potassium transporter coding gene. A relevant fact is that E. coli lacks c-di-AMP synthesizing enzymes; therefore, the co-expression system to assess the phenotypic effect of c-di-AMP on the potassium transport systems KimA and Kup, can be carried out without interference of host-synthesized c-di-AMP. To control c-di-AMP recombinant production in E. coli, the diadenylate cyclase CdaA from L. monocytogenes was used. The CdaALmo (carrying the wild type gene of cdaA) and the inactive CdaALmo*variant D171N are encoded by the plasmids pBP370 and pBP373, respectively (Rosenberg et al., 2015). These plasmids are pBAD33 derivatives, allowing expression of genes under control of the arabinose Para promoter (Figure 2D), and compatible with pWH844 derivatives that were used to express the potassium transporters (Figure 2A). For the co-expression assays, the E. coli LB2003 derivative strains carrying pWH844 or derivatives as well as either of the two diadenylate cyclases encoding plasmids were grown in M9mod supplemented with KCl, and L-arabinose when indicated, at the specified concentrations (see the section Materials and methods).
Since in our previous growth experiments performed in E. coli LB2003 harboring a copy of kimA or kup, addition of 0.5 mM KCl to M9mod medium was sufficient for the strains to grow, this concentration was selected for the later co-expression assays. It is important to mention that at this potassium concentration, strain LB2003 harboring empty vector pWH844 was not able to grow (Figure 2E, blue circle). The growth patterns of the strains synthesizing Kup protein, carrying either the plasmid encoding the active CdaALmo (Figure 2E, empty red circle) or the inactive CdaALmo* (Figure 2E, fill red circle) proteins were similar in the absence of the inducer (arabinose). By contrast, in the presence of 0.05% arabinose the growth of the strain synthesizing Kup was reduced when the functional diadenylate cyclase CdaALmo protein was co-produced, and thus in the presence of c-di-AMP (Figure 2F, empty red circle); however, no effect on the growth of the strain was observed when the inactive CdaALmo* protein was co-produced, and thus in the absence of c-di-AMP (Figure 2F, fill red circle).
To test the possibility that high intracellular c-di-AMP concentrations or expression of the heterologous diadenylate cyclase affect growth of the strain co-producing Kup and the active CdaALmo , similar growth curves of E. coli LB2003 harboring the empty vector pWH844 and plasmid pBP370 or pBP373 were performed. In this assays, 50 mM KCl was added to the growth media, condition that allows growth of the bacteria. The growth of the strain carrying pWH844 as well as either of the two CdaA encoding plasmids was not reduced both in absence and presence of the inducer arabinose, and both strains exhibited similar growth phenotypes, as observed for the strain carrying the empty vector pWH844.
Similar experiments were performed to assess the effect of c-di-AMP on KimA. Despite different amounts of arabinose were added to de culture medium, the results obtained were unable to show evidence of the specific interaction between the second messenger and the transport system under study (data not shown).
3.2. Inactivation of genes coding for potassium transporter systems belonging to the Kup family in Enterococcus faecalis JH2-2
To investigate whether kup and kimA genes products are involved in K+ uptake in E. faecalis, simple Δkup and ΔkimA, and double ΔkupΔkimA mutants were generated using the chimeric vector pBVGh (Blancato and Magni, 2010). Enterococcus faecalis JH2-2 strain is able to grow in LBG or low K+ medium mLBG (Kawano et al., 2001) at broad pH values (initial pH 9.0, 7.0, and 5.0 units). In LBG medium, when the initial pH was fixed to 9.0, enterococcal cells reached the maximal OD600 and growth rate (1.22 OD and 0.81 h−1, respectively; Supplementary Figure S1). At this pH, in mLBG a decrease of the growth parameters was observed (0.67 OD and 0.46 h−1, respectively; Supplementary Figure S1). At neutral initial pH (7 unit), similar patterns of growth were observed in both media, LB (0.63 OD and 0.88 h−1, respectively) and mLB (0.54 OD and 0.61 h−1, respectively). The reduction of the biomass generation could be due to the rapid acidification of the medium in a batch culture (Supplementary Figure S1). At acidic condition (pH 5.0), a significative reduction in the growth parameters was observed in LB and mLB media (OD = 0.53, growth rate 0.49 h−1 and OD = 0.36, growth rate 0.19 h−1, respectively; Supplementary Figure S1). The growth parameters of each enterococcal mutant strain (Δkup and ΔkimA, and Δkup ΔkimA) were analyzed by testing growth in both LBG or mLBG, and at the same pH values (9.0, 7.0, and 5.0). No differences were observed in the values of biomass and growth rate of the single and double mutants compared to the wild-type strain E. faecalis JH2-2 (Supplementary Figure S2).
3.3. Effect of Kup and KimA gene deletion on deficient strain in the Ktr channel systems
Taking into account the results presented above and the pivotal role of the Ktr channel systems in E. faecalis, different Ktr deficient strains were generated. Since previous studies in other related microorganisms have demonstrated that one ion-transporting protein (KtrB or KtrD) and the KtrA regulator function together in K+ uptake (Holtmann et al., 2003; Gries et al., 2013), the suspected major function of Ktr channel was tested by generating a deletion of the ktrA gene. A series of different K+-transporter systems deficient strains was constructed (ΔktrA, ΔktrA Δkup, and ΔktrA ΔkimA). The rates of growth of the wild type and the ktrA-derived mutants were compared in LB, and mLB at different pH values (Figure 3). In LB medium, only the double mutant Δkup ΔktrA strain shows reduced growth at alkaline pH (Figure 3A, yellow circle), whereas no other mutant showed phenotype at the other pHs in this medium (Figure 3D, pH 7.0 and 2G, pH 5.0). When the concentration of K+ in the complex medium was reduced (mLB), delayed growth was observed at alkaline conditions (pH 9.0) for all ktrA mutants, which was more severe for the double mutant Δkup ΔktrA (Figure 3B, yellow circle). In the same medium at neutral pH, this growth defect was observed only for the Δkup ΔktrA double mutant (Figure 3E, yellow circle). At acidic pH 5.0 in mLB, no growth alterations were observed for the mutant strains as compared to the JH2-2 parent strain (Figure 3H). Growth deficiencies of the KtrA mutants were restored to wild type phenotype by the addition of potassium (10 mM) to the growth medium (Figures 3C,F), strongly suggesting the inability of the mutant strains to concentrate K+ ions inside the cell.
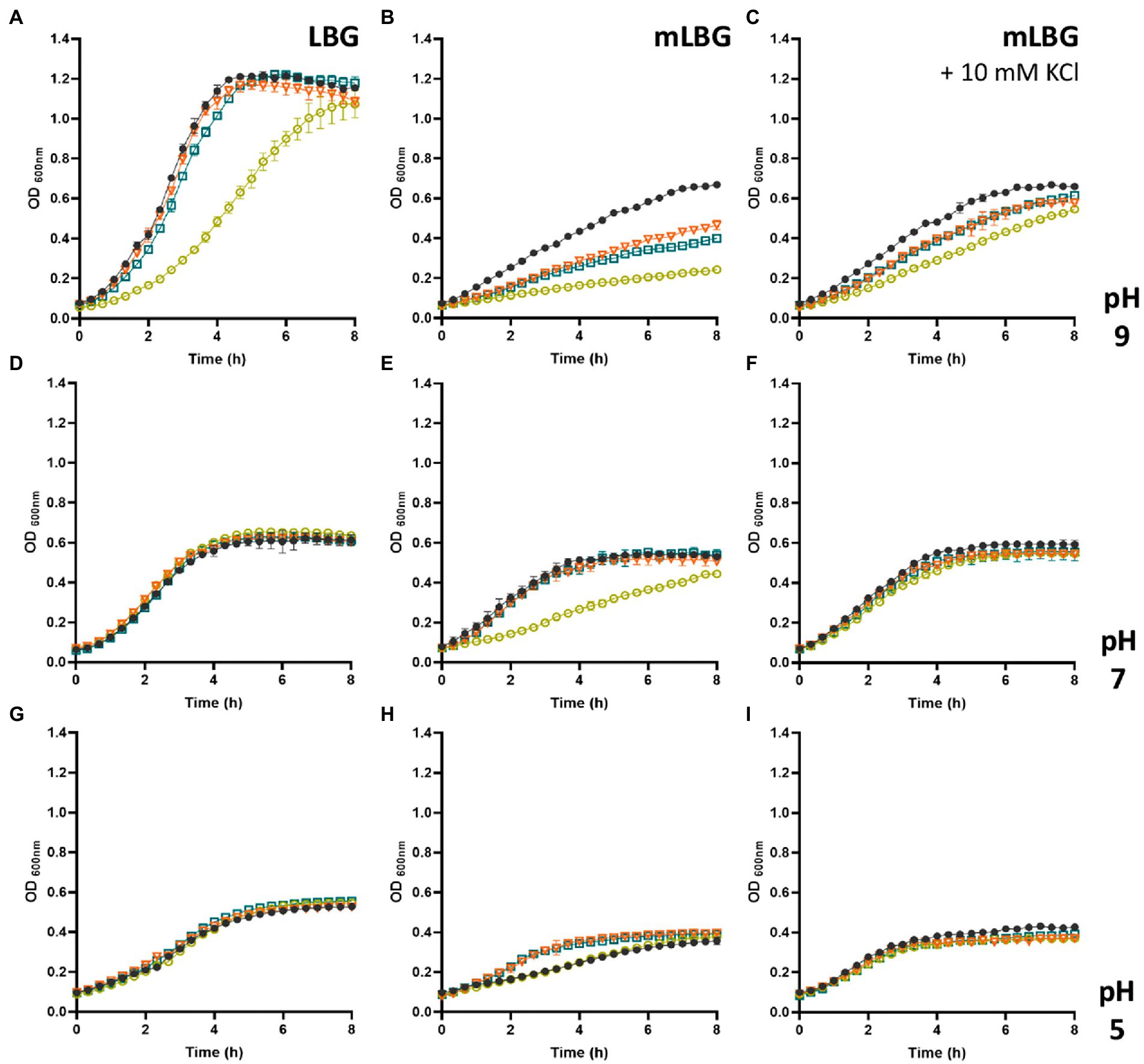
Figure 3. Growth curves of Enterococcus faecalis JH2-2 mutants under different conditions. Wild type (black dot), ΔktrA (light blue square), ΔkupΔktrA (yellow circle), and ΔktimAΔktrA (orange triangle) strains were grown in LBG (A,D,G), mLBG (B,E,H), or mLGB medium supplemented with 10 mM KCl (C,F,I). Initial pH was fixed at 9.0 units (A–C), 7.0 (D–F), or 5.0 (G–I).
3.4. Comparison of osmotic response of the Enterococcus faecalis potassium transporter deficient strains
As mentioned above, E. faecalis is able to resist high concentrations of NaCl. The first response to cope with hyperosmotic stress is K+ accumulation via massive uptake (Stülke and Krüger, 2020). The effect of salt compound on the growth of the parental and derived potassium transporters deficient strains of E. faecalis JH2-2 was analyzed in LBG and mLBG at different starting pH (9.0, 7.0, and 5.0) in the presence of up to 8% NaCl. As shown in Figure 4, during growth under alkaline stress conditions (pH 9.0), the toxic effect of NaCl addition is observed in both media, LB and LBm, for all ktrA mutants (ΔktrA, Δkup ΔktrA, and ΔkimA ΔktrA). Particularly, in LBG, the double mutant strain Δkup ΔktrA exhibited a more pronounced defect in growth as compared to wild type JH2-2 strain (Figure 4A, yellow circle). When the content of K+ in the medium is reduced but the pH remained the same, all defective strains were unable to grow at the lowest amount of the salt added (3% NaCl; Figures 4B, 5A), while the JH2-2 strain resisted up to 6% NaCl. As expected, these defects in the growth of all ktrA mutant strains were restored by addition of 10 mM KCl (Figure 5B). Growth in LB medium at neutral conditions (pH 7.0) with the addition of increasing concentrations of NaCl (from 0 to 8%), had similar effects for the wild type and the ΔktrA and ΔkimA ΔktrA mutant strains, however, a clear inhibition was observed for the double mutant Δkup ΔktrA (Figure 4C). When strains were grown in mLBG and neutral pH, again all ktrA mutants exhibited defective growth or sensitivity to the osmotic stressor NaCl, and normal growth was observed with the addition of 10 mM KCl (data not shown). Finally, at low pH condition (5.0), the treatment with NaCl led to drastically reduced growth parameters for all the tested strains; thus, the osmotic effect was not evident (data not shown).
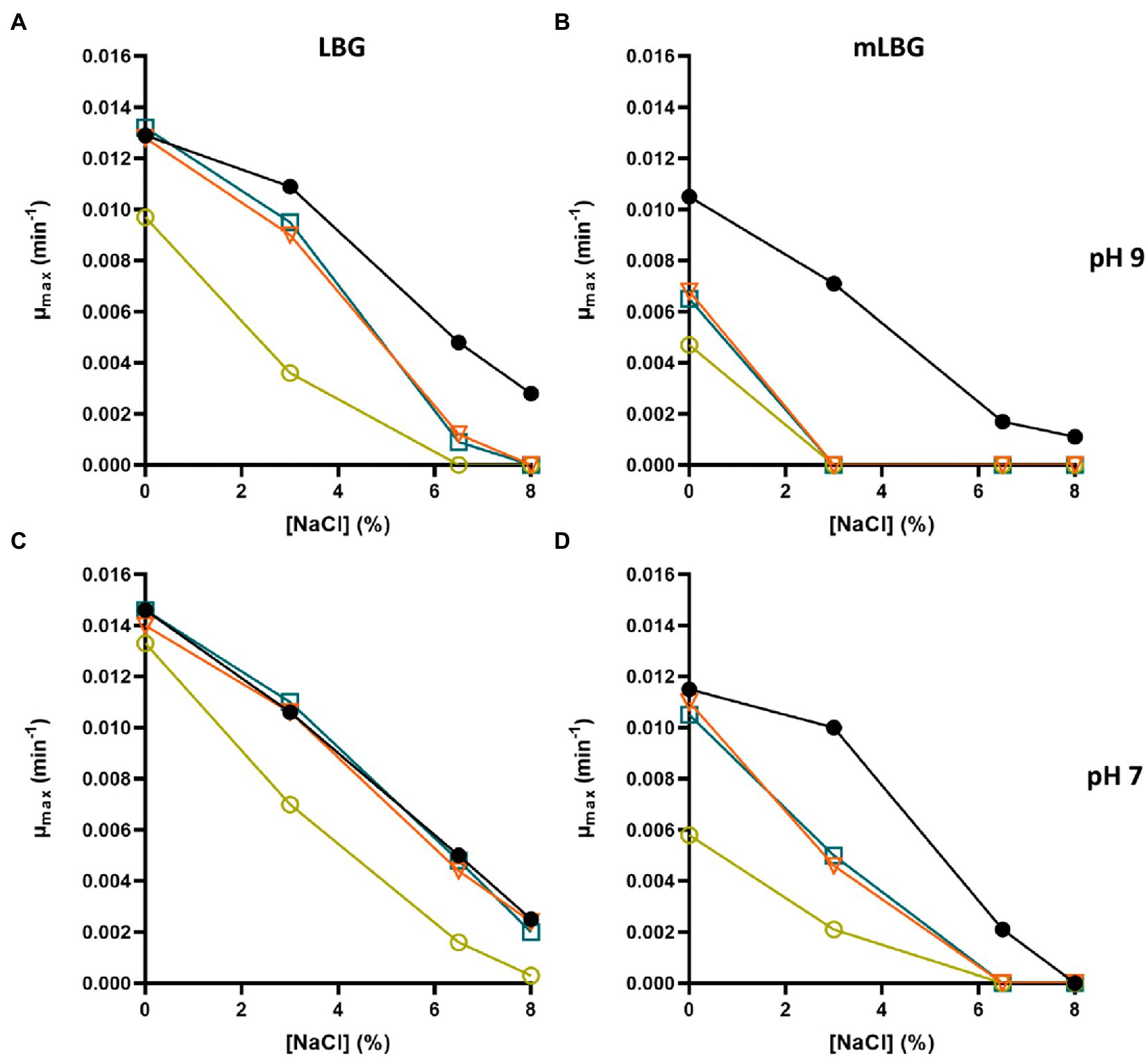
Figure 4. Inhibition of growth rate of Enterococcus faecalis JH2-2 mutants by NaCl. Wild type (black dot), ΔktrA (light blue square), ΔkupΔktrA (yellow circle), and ΔktimAΔktrA (orange triangle) strains were grown in LBG (A,C) and mLBG medium (B,D) at initial pH 9.0 (top) or 7.0 (low) in the presence of increasing concentrations of NaCl.
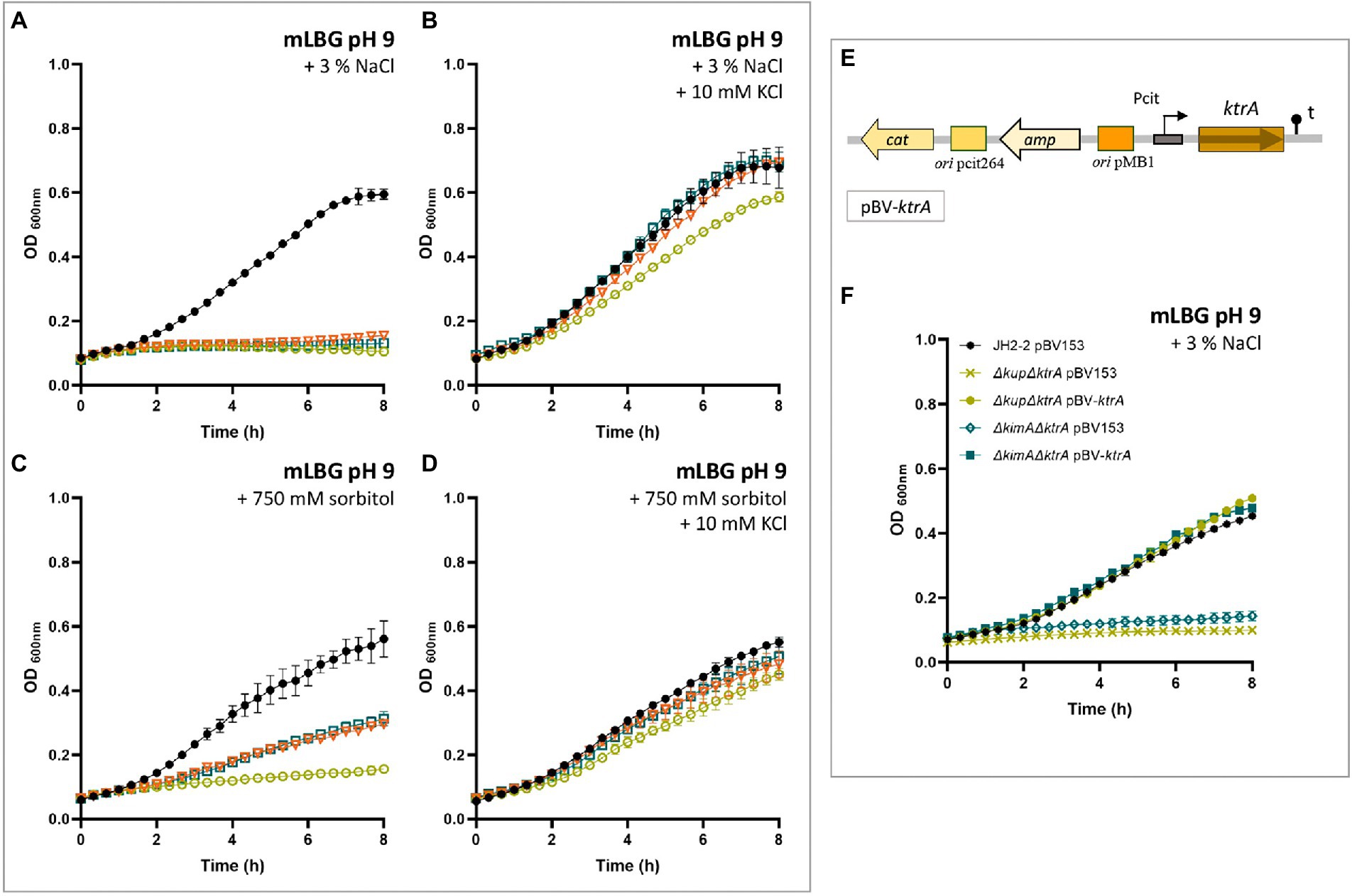
Figure 5. KtrA contributes to the osmotic response in Enterococcus faecalis. Wild type (black dot), ΔktrA (light blue square), ΔkupΔktrA (yellow circle), and ΔktimAΔktrA (orange triangle) strains were grown in mLBG at alkaline initial pH and supplemented with 3% NaCl (A) or 750 mM sorbitol (C). In both growth conditions, the addition of 10 mM KCl (B and D, respectively) completely restored defective strains growth to near wild-type levels. (E) Schematic representation of the plasmid pBV-ktrA used for complementation of a full copy of the wild type ktrA gene. (F) Expression of ktrA from a pBV153 fully complemented the growth defects of the KtrA-mutant strains in mLBG at alkaline initial pH and supplemented with 3% NaCl, and in all conditions tested in this study (data not shown).
Additionally, the ability of the mutant strains to grow in low K+ medium mLB supplemented with a low molecular weight osmolyte, different than NaCl, was tested to guarantee that the NaCl-induced effects on growth reported here were due to an osmotic effect and not to an ionic one. Thus, the growth of ktrA deficient mutant strains of E. facecalis was examined at high concentrations of sorbitol. Both in neutral and alkaline conditions, the addition of 750 mM sorbitol to mLB medium had a severe impact on growth of the ΔktrA, ΔkimA ΔktrA, and Δkup ΔktrA strains as compared to the wild type strain (Figure 5C). Similar to the growth in presence of 3% NaCl, addition of 10 mM KCl to the culture medium restored growth of the mutant strains (Figure 5D).
Finally, defective phenotypes found in the ΔktrA-containing mutants were analyzed by complementation with a full copy of the wild type ktrA gene, by use of the plasmid vector pBV153 (Figure 5E). Since the phenotype of the single mutant in KtrA and the double mutant in KtrA and KimA was the same in all the conditions examined, the complementation was performed only with the ktrA gene on the double mutant strains. Expression of ktrA rescued the growth defect of ΔkimA ΔktrA and Δkup ΔktrA mutants to wild-type levels in mLBG under alkaline constitions (pH 9.0) and in the presence of 3% NaCl (Figure 5F). This observation demonstrates that the KtrA protein is indeed required to allow growth of E. faecalis under alkaline conditions or in the presence of NaCl.
3.5. Diversity of K+ transporters in Enterococcus species
Orthologs of the Kdp pump, Ktr/Trk channels, and Kup symporter family (Stülke and Krüger, 2020; Tascón et al., 2020) were collected from the species with finished whole sequence genomes (NCBI) of the family that include the genus Enterococcus (44 species). As shown in the Figure 6A, distribution of the kdp operon is limited to 24 of the 44 species of Enterococcus analyzed. kup was found in 42/44 species studied of Enterococcus (mutated in Enterococcus massiliensis), whereas kimA is present in 38/44 species of Enterococcus. In the case of ktr genes, both genes encoding for the cytosolic component KtrA and for the membrane component of the system, KtrB, were found encoded in all 44 species analyzed. The ktrD gene encoding for the membrane component was found in 41 species. On the other hand, the trkAH operon, encoding the cytoplasmatic component TrkA (455 residues) and the membrane component TrkH (479 residues), was found disseminated in only a few species (Enterococcus asini, Enterococcus avium, Enterococcus diestrammaneae, Enterococcus raffinosus, Enterococcus pseudoavium, and Enterococcus xiangfangensis).
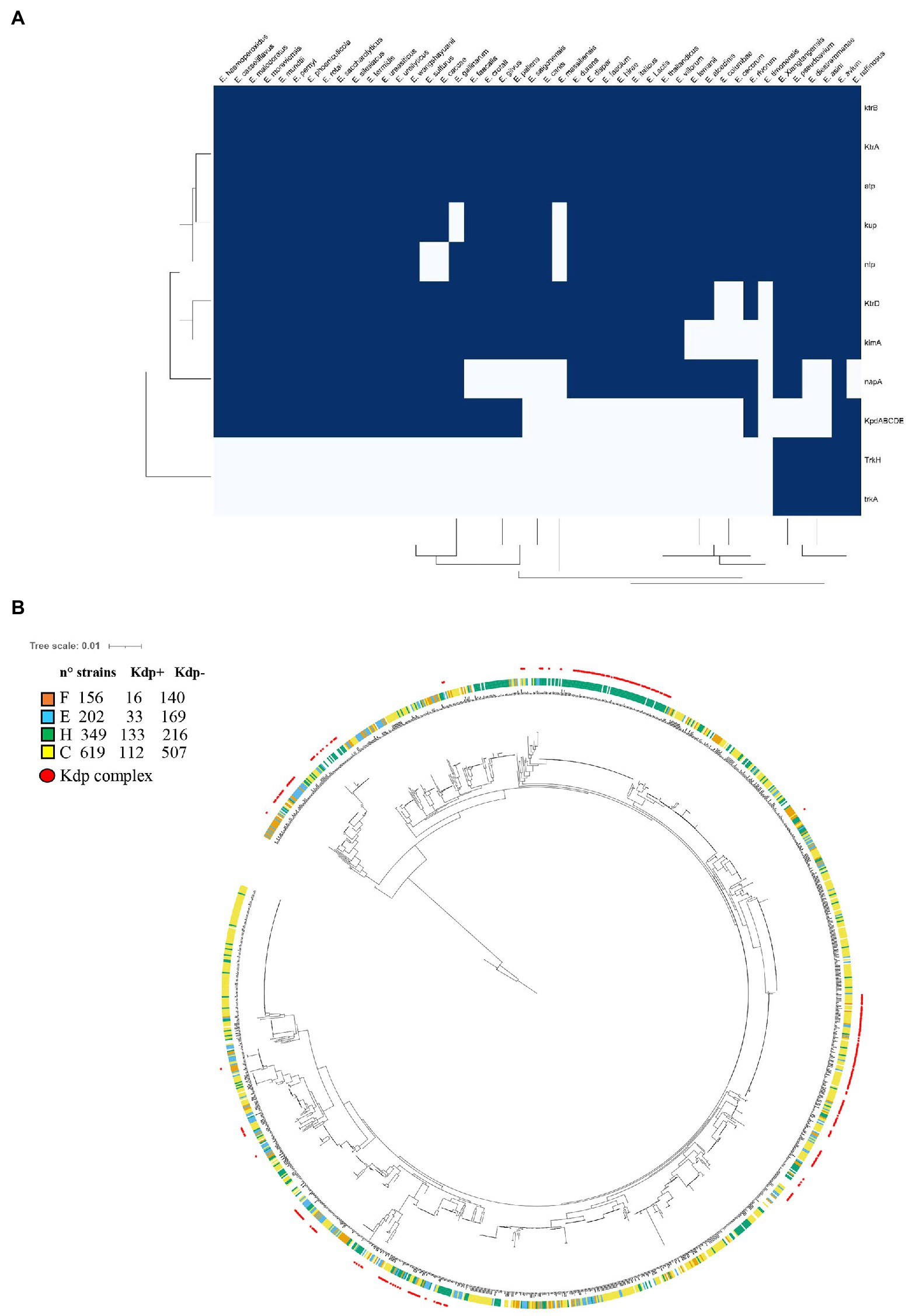
Figure 6. (A) Heatmap showing the presence of K+ transporters and related ATPases in Enterococcus species. A presence-absence matrix was constructed and Distance-based clustering was applied. Dark blue indicates presence of the genes analyzed. (B) Analysis of the presence of kdp genes and the phylogenomic relationships among E. faecalis members. 34 common ancestral genes were individually aligned, concatenated, and trimmed resulting in a final alignment containing a total of 4,726 residues. The evolutionary history of the 1,510 E. faecalis members was inferred with RAxML algorithm (Stamatakis, 2014) and displayed and annotated using iTOL (Letunic and Bork, 2011). The inner circles colors indicate the source of isolation; F, food (orange); C, commensal (yellow); E, environmental (light blue); and H, clinical (green). The red outside circles indicate the presence of the kdp genes within the strains.
In addition to the K+ transporters, other transporters associated to ion fluxes were sought in Enterococcus. Such is the case of the F0F1-ATPase complex, a well conserved proton translocator which has an essential function in the establishment of the proton motive force (PFM) and, therefore, it is related to the mechanisms of resistance to low pH. Also, the presence of Na+ transporters, which are involved in the alkaline and osmotic resistance, was identified. The results showed that the ntp operon, which encodes for the F0F1-ATPase complex, is widespread in Enterococcus species (41/44), with few exceptions (Enterococcus canis, E. massilensis, and Enterococcus sulfureus), while napA, encoding the Na+ symporter NapA, is present in 33 species of the 44 analyzed.
Finally, considering that the expression of the Kdp system has been studied in E. faecalis V583, and that the induction of this potassium transporter under alkaline and NaCl stress conditions was reported (Solheim et al., 2014), we hypothesized that the requirement of the Kdp system could be involved in the pathogenesis of clinical strain. Though KimA, Kup, and Ktr transporters are homogeneously distributed in nearly all E. faecalis strains, the KdpFABC complex and the regulatory KdpDE system were found in only 319 strains out of a total of 1,510 complete genome sequences analyzed (21%) of these species (Figure 6B). The latter indicates that, as in other species, these genes do not belong to the gene core in E. faecalis. Then, we classified the strains and tested the hypothesis that the presence of kdp genes is correlated with pathogenicity. The phylogenetic tree depicted in Figure 6B showed the existence of clusters of strains with high phylogenetic signal (very related phylogenetically) that have similar kdp occurrence. Nevertheless, using phylogeny-aware methods, we observed that the presence of kdp genes was significantly positively correlated with E. faecalis of clinical origin (p value of 2.76 E−11). Taking into account the relevance of the robust physiology of E. faecalis, which allows it to persist and thrive in harsh environments that include acid, oxidative and hyperosmotic stress, for its pathogenic potential (Solheim et al., 2014), we investigated whether K+ transporter systems contribute to the pathogenesis of the natural Kdp defective strain JH2-2. Notably, deletion of the potassium transport systems Kup, KimA, or Ktr in E. faecalis JH2-2 did not affect growth and survival in animal fluids associated to common diseases caused by this bacterium (blood and urine) nor the infection and virulence in Galleria Mellonella model (Supplementary Figures S3, S4).
4. Discussion
In this study two members of the KUP family, Kup and KimA, present in E. faecalis were identified and characterized. Our work demonstrates that both transporters possess a high affinity for the K+ ion, similar to family members described in L. lactis or B. subtilis (Gundlach et al., 2019; Quintana et al., 2019). The inhibition of K+ transport by the second messenger c-di-AMP was demonstrated, by in vivo studies in a c-di-AMP producing E. coli strain (Figure 2F), for the Kup protein, however, it could not be demonstrated for KimA. In Firmicutes, as mentioned above, regulation of potassium transport by the second messenger c-di-AMP was demonstrated for several members of this phylum; this is the case for the control of the activity of Kup from L. lactis, KimA from B. subtilis and L. monocytogenes, and KtrA/C from B. subtilis, L. monocytogenes, S. aureus, S. pneumoniae, and S. mutans. Considering the high similarity among orthologous transporters in this group of related bacteria, it is strongly suggested that K+ homeostasis in E. faecalis could be controlled by the intracellular c-di-AMP concentration (Figure 1). Also, as stated above, disruptions in both the potassium uptake and the c-di-AMP homeostasis can affect the virulence potential of bacterial pathogens. Recent studies with E. faecalis strain OG1RF (Kdp+ strain) revealed that variations in the intracellular levels of c-di-AMP modify its pathogenic potential (Kundra et al., 2021). In our study, no differences concerning the growth either in animal fluids or in the G. mellonella insect model were detected by using simple and double mutants in the different K+ transporters (Kup, KimA, and Ktr systems) present in E. faecalis JH2-2. This observation differs to findings in other opportunistic pathogen such S. aureus, where a KtrA deficient strain showed a deficiency in the capacity to infect mice (Gries et al., 2013). Here, it is important to mention that, in this study we were unable to obtain a K+ transporter-free triple mutant (Δkup ΔkimA ΔktrA) using our gene deletion methodology. Therefore, although further studies will be required, this impossibility suggests that at least one potassium transport system is necessary and sufficient to satisfy the demand for this ion in the cellular interior, in the media and conditions studied. On the other hand, a detailed analysis of the distribution of transport systems in clinical and non-clinical isolates of E. faecalis showed that the kdp genes were enriched significantly in clinical isolate samples.
Given that the robust nature of E. faecalis facilitates its tolerance to hostile environments, and the fact that bacterial cells are known to import K+ to help resist and survive under various stressful conditions, growth of simple and double potassium transport mutants derived from E. faecalis JH2-2 parental strain was assayed under different conditions. Interestingly, the inactivation of KtrA - dependent K+ channels contribute to the sensitivity to both alkaline growth condition and osmotic stress. These phenotypes were increased in a low K+ medium, and recovered by the external addition of potassium salt or by the introduction of a plasmid carrying a full copy of the wild type ktrA gene. We also found that inactivation of the K+ transporter Kup, but not KimA, in the KtrA—deficient mutant strain increases both the sensitivity to alkaline stress and the toxicity by NaCl. From the latter data, we propose that the Ktr system plays an important role in the ability of E. faecalis to adapt to hyperosmotic stress, and that Kup functions secondarily to Ktr. However, altogether the growth assays of the potassium transporter defective strains indicate that E. faecalis uses different transporters systems with capacity to replace one to the others in different stress conditions, such as low K+, extreme pH, and salt concentrations.
Based on the results of this study, we propose a general mechanism of Enterococcus resistance, which as shown in Figure 1 involves the expression of different ATPases for the movement of the essential ions. Proton extrusion is carried out by the F0F1 ATPase complex, which is functional at neutral and acidic pH. Sodium ions are pumped outside cells by the V0V1 ATPase complex, which is active at low H+ concentrations. Concerning to the homeostasis of K+, multiple channels and transporters are present in E. faecalis, but the specific physiological roles of each transporter are poorly studied. Diversity analysis of the potassium transporters performed in the Enterococcus species revealed that the channels KtrA/B is the most widely disseminated of the K+ transporters. The two members of the H+/K+ symporter KUP family, Kup and KimA, described here, are widely disseminated in the Enterococcus genera. Taking into account how the ion flux of H+, Na+, and K+ involved in alkaline stress occurs in this microorganism (Figure 1), and the results obtained in this work with respect to the mutant strains in potassium transport, we hypothesize that the KtrAB system is involved in the symport of K+ and Na+, and function together, under alkaline pH growth, with the Na+ ATPase, responsible to expel out the Na+. In addition, we found that Kup could mediate the intracellular import of K+ and H+, which could contribute to not only the homeostasis of potassium, but also to hold protons inside the cells. This participation of the Kup transporter in alkaline conditions is highly relevant, and its contribution could be that this protein can transport K+ ions with H+, thus contributing not only to K+ uptake but also to the acidification of the cytoplasm in extreme alkaline conditions.
5. Conclusion
Enterococcus faecalis is able to resist and grow in different conditions. By using genetic techniques, we conclude that KtrA is required for the functionality of the uptake system in alkaline and high osmolarity, in this condition also Kup increase the resistance to this stress conditions to the concentration of K+. Finally, simple or double mutans in the K+ transporter did not produce significative modification in the virulence of the strains.
Data availability statement
The original contributions presented in the study are included in the article/Supplementary material, further inquiries can be directed to the corresponding author.
Author contributions
GA done experimental design and performed experiments. FG performed experiments. MT contributed to bioinformatic analysis. ME contributed to experimental design and bioinformatic analysis. VB designed and performed experiments and bioinformatic analysis. JS designed experiments. CM designed experiments and performed bioinformatic analysis. All authors contributed to the article and approved the submitted version.
Funding
This work was supported by Ministerio de Ciencia y Tecnologia PICT 2020–3227 (Prest BID) and PIP 11220200101356CO, CONICET.
Conflict of interest
The authors declare that the research was conducted in the absence of any commercial or financial relationships that could be construed as a potential conflict of interest.
Publisher’s note
All claims expressed in this article are solely those of the authors and do not necessarily represent those of their affiliated organizations, or those of the publisher, the editors and the reviewers. Any product that may be evaluated in this article, or claim that may be made by its manufacturer, is not guaranteed or endorsed by the publisher.
Supplementary material
The Supplementary material for this article can be found online at: https://www.frontiersin.org/articles/10.3389/fmicb.2023.1117684/full#supplementary-material
Footnotes
References
Bai, Y., Yang, J., Zarrella, T. M., Zhang, Y., Metzger, D. W., and Bai, G. (2014). Cyclic Di-AMP impairs potassium uptake mediated by a cyclic Di-AMP binding protein in streptococcus pneumoniae. J. Bacteriol. 196, 614–623. doi: 10.1128/JB.01041-13
Benjamini, Y., and Hochberg, Y. (1995). Controlling the false discovery rate: a practical and powerful approach to multiple testing. J. R. Stat. Soc. Ser. B 57, 289–300.
Binepal, G., Gill, K., Crowley, P., Cordova, M., Brady, J. L., Senadheera, D. B., et al. (2016). Trk2 potassium transport system in Streptococcus mutans and its role in potassium homeostasis, biofilm formation, and stress tolerance. J. Bacteriol. 198, 1087–1100. doi: 10.1128/JB.00813-15
Blancato, V. S., and Magni, C. (2010). A chimeric vector for efficient chromosomal modification in enterococcus faecalis and other lactic acid bacteria. Lett. Appl. Microbiol. 50, 542–546. doi: 10.1111/j.1472-765X.2010.02815.x
Boehm, A. B., and Sassoubre, L. M. (2014). “Enterococci as indicators of environmental fecal contamination,” in Enterococci: From Commensals to Leading Causes of Drug Resistant Infection [Internet]. eds. M. S. Gilmore, D. B. Clewell, Y. Ike and N. Shankar (Boston: Massachusetts Eye and Ear Infirmary).
Byappanahalli, M. N., Nevers, M. B., Korajkic, A., Staley, Z. R., and Harwood, V. J. (2012). Enterococci in the environment. Microbiol. Mol. Biol. Rev. 76, 685–706. doi: 10.1128/MMBR.00023-12
Corrigan, R. M., and Gründling, A. (2013). Cyclic di-AMP: another second messenger enters the fray. Nat. Rev. Microbiol. 11, 513–524. doi: 10.1038/nrmicro3069
Devaux, L., Sleiman, D., Mazzuoli, M. V., Gominet, M., Lanotte, P., Trieu-Cuot, P., et al. (2018). Cyclic di-AMP regulation of osmotic homeostasis is essential in group B streptococcus. PLoS Genet. 14, e1007342–e1007353. doi: 10.1371/journal.pgen.1007342
Dornan, S., and Collins, M. A. (1990). High efficiency electroporation of Lactococcus lactis subsp. lactis LM0230 with plasmid pGB301. Lett. Appl. Microbiol. 11, 62–64. doi: 10.1111/j.1472-765X.1990.tb01275.x
Espariz, M., Zuljan, F. A., Esteban, L., and Magni, C. (2016). Taxonomic identity resolution of highly phylogenetically related strains and selection of phylogenetic markers by using genome-scale methods: the bacillus pumilus group case. PLoS One 11:e0163098. doi: 10.1371/journal.pone.0163098
Faozia, S., Fahmi, T., Port, G. C., and Cho, K. H. (2021). C-di-AMP-regulated K+ importer KtrAB affects biofilm formation, stress response, and SpeB expression in Streptococcus pyogenes. Infect. Immun. 89, e00317–e00320. doi: 10.1128/IAI.00317-20
Franz, C. M. A. P., Stiles, M. E., Schleifer, K. H., and Holzapfel, W. H. (2003). Enterococci in foods—a conundrum for food safety. Int. J. Food Microbiol. 88, 105–122. doi: 10.1016/S0168-1605(03)00174-0
García-Solache, M., and Rice, L. B. (2019). The enterococcus: a model of adaptability to its environment. Clin. Microbiol. Rev. 32, 1–28. doi: 10.1128/CMR.00058-18
Gibhardt, J., Hoffmann, G., Turdiev, A., Wang, M., Lee, V. T., and Commichau, F. M. (2019). C-di-AMP assists osmoadaptation by regulating the listeria monocytogenes potassium transporters KimA and KtrCD. J. Biol. Chem. 294, 16020–16033. doi: 10.1074/jbc.RA119.010046
Giraffa, G. (2002). Enterococci from foods. FEMS Microbiol. Rev. 26, 163–171. doi: 10.1111/j.1574-6976.2002.tb00608.x
Gries, C. M., Bose, J. L., Nuxoll, A. S., Fey, P. D., and Bayles, K. W. (2013). The Ktr potassium transport system in Staphylococcus aureus and its role in cell physiology, antimicrobial resistance and pathogenesis. Mol. Microbiol. 89, 760–773. doi: 10.1111/mmi.12312
Gries, C. M., Sadykov, M. R., Bulock, L. L., Chaudhari, S. S., Thomas, V. C., Bose, J. L., et al. (2016). Potassium uptake modulates Staphylococcus aureus metabolism. mSphere :1. doi: 10.1128/msphere.00125-16
Gundlach, J., Herzberg, C., Kaever, V., Gunka, K., Hoffmann, T., Weiß, M., et al. (2017). Control of potassium homeostasis is an essential function of the second messenger cyclic di-AMP in Bacillus subtilis. Sci. Signal. 10, 1–10. doi: 10.1126/scisignal.aal3011
Gundlach, J., Krüger, L., Herzberg, C., Turdiev, A., Poehlein, A., Tascón, I., et al. (2019). Sustained sensing in potassium homeostasis: cyclic di-AMP controls potassium uptake by KimA at the levels of expression and activity. J. Biol. Chem. 294, 9605–9614. doi: 10.1074/jbc.RA119.008774
Hancock, L. E., and Perego, M. (2004). Systematic inactivation and phenotypic characterization of two-component signal transduction systems of enterococcus faecalis V583. J. Bacteriol. 186, 7951–7958. doi: 10.1128/JB.186.23.7951-7958.2004
Holtmann, G., Bakker, E. P., Uozumi, N., and Bremer, E. (2003). KtrAB and KtrCD: two K. Society 185, 1289–1298. doi: 10.1128/JB.185.4.1289
Kakinuma, Y. (1998). Inorganic cation transport and energy transduction in enterococcus hirae and other streptococci. Microbiol. Mol. Biol. Rev. 62, 1021–1045. doi: 10.1128/MMBR.62.4.1021-1045.1998
Kawano, M., Abuki, R., Igarashi, K., and Kakinuma, Y. (2001). Potassium uptake with low affinity and high rate in enterococcus hirae at alkaline pH. Arch. Microbiol. 175, 41–45. doi: 10.1007/s002030000234
Kim, H., Youn, S. J., Kim, S. O., Ko, J., Lee, J. O., and Choi, B. S. (2015). Structural studies of potassium transport protein KtrA regulator of conductance of K+ (RCK) C domain in complex with cyclic diadenosine monophosphate (c-di-AMP). J. Biol. Chem. 290, 16393–16402. doi: 10.1074/jbc.M115.641340
Krüger, L., Herzberg, C., Warneke, R., Poehlein, A., Stautz, J., Weiß, M., et al. (2020). Two ways to convert a low-affinity potassium channel to high affinity: control of Bacillus subtilis KtrCD by glutamate. J. Bacteriol. 202, e00138–e00158. doi: 10.1128/JB.00138-20
Krulwich, T. A., Sachs, G., and Padan, E. (2011). Molecular aspects of bacterial pH sensing and homeostasis. Nat. Rev. Microbiol. 9, 330–343. doi: 10.1038/nrmicro2549
Kundra, S., Lam, L. N., Kajfasz, J. K., Casella, L. G., Andersen, M. J., Abranches, J., et al. (2021). C-di-AMP is essential for the virulence of Enterococcus faecalis. Infect. Immun. 89:e0036521. doi: 10.1128/IAI.00365-21
Letunic, I., and Bork, P. (2011). Interactive tree of life v2: online annotation and display of phylogenetic trees made easy. Nucleic Acids Res. 39, W475–W478. doi: 10.1093/nar/gkr201
Levy, A., Salas Gonzalez, I., Mittelviefhaus, M., Clingenpeel, S., Herrera Paredes, S., Miao, J., et al. (2018). Genomic features of bacterial adaptation to plants. Nat. Genet. 50, 138–150. doi: 10.1038/s41588-017-0012-9
Martino, G. P., Perez, C. E., Magni, C., and Blancato, V. S. (2018). Implications of the expression of enterococcus faecalis citrate fermentation genes during infection. PLoS One 13:e0205787. doi: 10.1371/journal.pone.0205787
Murray, B. E. (1990). The life and times of the enterococcus. Clin. Microbiol. Rev. 3, 46–65. doi: 10.1128/CMR.3.1.46
Ogier, J. C., and Serror, P. (2008). Safety assessment of dairy microorganisms: the enterococcus genus. Int. J. Food Microbiol. 126, 291–301. doi: 10.1016/j.ijfoodmicro.2007.08.017
Pham, H. T., Nhiep, N. T. H., Vu, T. N. M., Huynh, T. A. N., Zhu, Y., Huynh, A. L. D., et al. (2018). Enhanced uptake of potassium or glycine betaine or export of cyclic-di-AMP restores osmoresistance in a high cyclic-di-AMP Lactococcus lactis mutant. PLoS Genet. 14:e1007574. doi: 10.1371/journal.pgen.1007574
Price-Whelan, A., Poon, C. K., Benson, M. A., Eidem, T. T., Roux, C. M., Boyd, J. M., et al. (2013). Tanscriptional profiling of staphylococcus aureus during growth in 2 M NaCl leads to clarification of physiological roles for Kdp and Ktr K+ uptake systems. MBio 4, e00407–e00413. doi: 10.1128/mBio.00407-13
Quintana, I. M., Gibhardt, J., Turdiev, A., Hammer, E., Commichau, F. M., Lee, V. T., et al. (2019). The KupA and KupB proteins of Lactococcus lactis IL1403 are novel c-di-AMP receptor proteins responsible for potassium uptake. J. Bacteriol. 201, 1–13. doi: 10.1128/JB.00028-19
Rocha, R., Teixeira-Duarte, C. M., Jorge, J. M. P., and Morais-Cabral, J. H. (2019). Characterization of the molecular properties of KtrC, a second RCK domain that regulates a Ktr channel in Bacillus subtilis. J. Struct. Biol. 205, 34–43. doi: 10.1016/j.jsb.2019.02.002
Rosenberg, J., Dickmanns, A., Neumann, P., Gunka, K., Arens, J., Kaever, V., et al. (2015). Structural and biochemical analysis of the essential diadenylate cyclase CdaA from listeria monocytogenes. J. Biol. Chem. 290, 6596–6606. doi: 10.1074/jbc.M114.630418
Sambrook, J., Fritsch, E. F., and Maniatis, T. (1989). Molecular Cloning: A Laboratory Manual. 2nd Edn. Cold Spring Harbor, NY: Cold Spring Harbor Laboratory Press.
Schlosser, A., Meldorf, M., Stumpe, S., Bakker, E. P., and Epstein, W. (1995). TrkH and its homolog, TrkG, determine the specificity and kinetics of cation transport by the Trk system of Escherichia coli. J. Bacteriol. 177, 1908–1910. doi: 10.1128/jb.177.7.1908-1910.1995
Solheim, M., La Rosa, S. L., Mathisen, T., Snipen, L. G., Nes, I. F., and Brede, D. A. (2014). Transcriptomic and functional analysis of NaCl-induced stress in enterococcus faecalis. PLoS One 9, 1–13. doi: 10.1371/journal.pone.0094571
Stamatakis, A. (2014). RAxML version 8: a tool for phylogenetic analysis and post-analysis of large phylogenies. Bioinformatics 30, 1312–1313. doi: 10.1093/bioinformatics/btu033
Stautz, J., Hellmich, Y., Fuss, M. F., Silberberg, J. M., Devlin, J. R., Stockbridge, R. B., et al. (2021). Molecular mechanisms for bacterial potassium homeostasis. J. Mol. Biol. 433:166968. doi: 10.1016/j.jmb.2021.166968
Stülke, J., and Krüger, L. (2020). Cyclic di-AMP signaling in bacteria. Annu. Rev. Microbiol. 74, 159–179. doi: 10.1146/annurev-micro-020518-115943
Tascón, I., Sousa, J. S., Corey, R. A., Mills, D. J., Griwatz, D., Aumüller, N., et al. (2020). Structural basis of proton-coupled potassium transport in the KUP family. Nat. Commun. 11:626. doi: 10.1038/s41467-020-14441-7
Terán, L. C., Mortera, P., Tubio, G., Alarcón, S. H., Blancato, V. S., Espariz, M., et al. (2022). Genomic analysis revealed conserved acid tolerance mechanisms from native micro-organisms in fermented feed. J. Appl. Microbiol. 132, 1152–1165. doi: 10.1111/jam.15292
Keywords: Enterococcus faecalis, potassium transport, KUP/HAK/KT K+ transporters, Ktr family, Kdp system
Citation: Acciarri G, Gizzi FO, Torres Manno MA, Stülke J, Espariz M, Blancato VS and Magni C (2023) Redundant potassium transporter systems guarantee the survival of Enterococcus faecalis under stress conditions. Front. Microbiol. 14:1117684. doi: 10.3389/fmicb.2023.1117684
Edited by:
Paloma López, Spanish National Research Council (CSIC), SpainReviewed by:
Victor Ladero, Spanish National Research Council (CSIC), SpainTimothy Meredith, The Pennsylvania State University, United States
Copyright © 2023 Acciarri, Gizzi, Torres Manno, Stülke, Espariz, Blancato and Magni. This is an open-access article distributed under the terms of the Creative Commons Attribution License (CC BY). The use, distribution or reproduction in other forums is permitted, provided the original author(s) and the copyright owner(s) are credited and that the original publication in this journal is cited, in accordance with accepted academic practice. No use, distribution or reproduction is permitted which does not comply with these terms.
*Correspondence: Christian Magni, ✉ bWFnbmlAaWJyLWNvbmljZXQuZ292LmFy
†ORCID: Christian Magni https://orcid.org/0000-0002-5179-9700