- 1Laboratorio de Biotecnología Molecular, Instituto de Biotecnología Misiones “Dra. María Ebe Reca”-InBioMis, Universidad Nacional de Misiones, Posadas, Misiones, Argentina
- 2Consejo Nacional de Investigaciones Científicas y Técnicas (CONICET), Buenos Aires, Argentina
- 3Facultad de Ciencias Agrarias y Forestales, Instituto de Fisiología Vegetal, Universidad Nacional de La Plata, La Plata, Buenos Aires, Argentina
- 4Facultad de Ciencias Naturales y Museo, Instituto de Botánica Carlos Spegazzini, Universidad Nacional de La Plata, La Plata, Buenos Aires, Argentina
- 5Cátedra de Microbiología Agrícola, Facultad de Ciencias Agrarias y Forestales, Universidad Nacional de La Plata, La Plata, Buenos Aires, Argentina
Introduction: Fungal mitogenomes exhibit remarkable variation in conformation, size, gene content, arrangement and expression, including their intergenic spacers and introns.
Methods: The complete mitochondrial genome sequence of the mycoparasitic fungus Trichoderma koningiopsis was determined using the Illumina next-generation sequencing technology. We used data from our recent Illumina NGS-based project of T. koningiopsis genome sequencing to study its mitochondrial genome. The mitogenome was assembled, annotated, and compared with other fungal mitogenomes.
Results: T. koningiopsis strain POS7 mitogenome is a circular molecule of 27,560 bp long with a GC content of 27.80%. It harbors the whole complement of the 14 conserved mitochondrial protein-coding genes (PCG) such as atp6, atp8, atp9, cox1, cox2, cox3, cob, nad1, nad2, nad3, nad4, nad4L, nad5, and nad6, also found in the same gene order to other Hypocreales. The mitogenome also contains 26 transfer RNA genes (tRNAs), 5 of them with more than one copy. Other genes also present in the assembled mitochondrial genome are a small rRNA subunit and a large rRNA subunit containing ribosomal protein S3 gene. Despite the small genome size, two introns were detected in the T. koningiopsis POS7 mitogenome, one of them in cox3 gene and the other in rnl gene, accounting 7.34% of this mitogenome with a total size of 2,024 bp. A phylogenetic analysis was done using the 14 PCGs genes of T. koningiopsis strain POS7 mitogenome to compare them with those from other fungi of the Subphyla Pezizomycotina and Saccharomycotina. T. koningiopsis strain POS7 was clustered together with other representatives of Trichoderma lineage, within the Hypocreales group, which is also supported by previous phylogenetic studies based on nuclear markers.
Discussion: The mitochondrial genome of T. koningiopsis POS7 will allow further investigations into the taxonomy, phylogenetics, conservation genetics, and evolutionary biology of this important genus as well as other closely related species.
1. Introduction
Mitochondria use electron transport coupled with oxidative phosphorylation to generate ATP and these organelles also participate in several other cellular important functions (Burger et al., 2003). Mitochondrial DNA or mitochondrial genome, also known as mitogenome, is made up of genes that encodes a small number of proteins, whose mRNAs are translated by a distinctive mitochondrial protein-synthesizing system (Burger et al., 2003; Kwak, 2020). Evolutionarily, mitogenomes exhibit remarkable variation in conformation, size, actual gene content, arrangement and expression, including their intergenic spacers and introns (Gray et al., 1999; Guha et al., 2018; Li et al., 2019). Within the fungi mitogenome can exist as either linear or circular molecules (Hausner, 2003; Medina et al., 2020).
Many fungi show similar morphological features, which are broadly dependent upon culture conditions, and so pleomorphic, which usually difficult their identification using only traditional morphological characters. Therefore, a fungal natural classification must be including phylogenetic tools. However, these taxonomic analyses of fungi using phylogenetic inferences need to be combined with several molecular markers, such as those from the mitogenome, which can be a good complement to explain their variation (Chen et al., 2019).
Mitochondria contain their own genome, and they may provide adequate information for phylogenetic studies based on their comparisons with ones from other reference taxa (Ghikas et al., 2006; Kanzi et al., 2016). However, fungal mitogenomes have been less studied than their animal or plant counterparts, limiting a complete understanding of their genetic characteristics and evolutionary histories (Aguileta et al., 2014).
Recently, development of high-throughput sequencing technologies and related bioinformatics tools have allowed considerably greater numbers of nucleotides to be characterized and the genome-scale data to be more easily assembled. Compared to the whole-genome data, mitogenomes are more readily sequenced with broader taxon sampling and a reasonable cost (Song et al., 2020). The number of complete filamentous fungal mitogenome sequences has significantly increased in the last years facilitating evolutionary and systematic studies (Mardanov et al., 2014; Megarioti and Kouvelis, 2020; Fatma et al., 2023). Fungal mitogenomes typically contain 15 standard protein-coding genes, two rRNA genes and a variable number of tRNA genes. These protein-coding genes are atp6, atp8, atp9, cob, cox1, cox2, cox3, nad1, nad2, nad3, nad4, nad4L, nad5, nad6, and rps3 (Lang, 2018), being some of them absent in certain fungal mitogenomes (Zhang and Zhang, 2019).
The independent inheritance from nuclear genomes, a high copy number, and several available molecular markers has made the mitogenomes widely used as a tool for studying evolution, phylogenetics, population genetics, and comparative or evolutionary genomics for some fungi (Van de Sande, 2012; Chen et al., 2019; Li et al., 2020; Wang et al., 2020).
Fungi belonging to the Trichoderma genus (Hypocreaceae, Hypocreales, Sordariomycetes, Pezizomycotina, Ascomycota) are ubiquitous inhabitants in different habitats, being dominate in a broad spectrum of soils and/or decaying organic matter. Species of this genus are economically important, in part because of their mycoparasitic ability, but also as they can stimulate plant resistance, and plant growth and development which increase crop production. Among these uses, T. koningiopsis was reported as a fungal species that can be used in biocontrol and other biotechnological applications (Samuels et al., 2006; Castrillo et al., 2015; Amerio et al., 2020; Castrillo et al., 2021a).
Trichoderma whole genome analyses have been reported previously (Halliwell and Griffin, 1973; Antal et al., 2002; Chambergo et al., 2002; Martinez et al., 2008; Kubicek et al., 2011; Studholme et al., 2013; Steindorff et al., 2014; Xie et al., 2014; Baroncelli et al., 2015, 2016; Shi-Kunne et al., 2015; Castrillo et al., 2017; Proctor et al., 2018). However, in spite of the extensive studies on biocontrol and nuclear genes of Trichoderma genus, information about its mitogenomes remains largely unknown. To date, even if Trichoderma genus has more than 300 known species, only 11 mitogenomes are available, including Trichoderma arundinaceum, Trichoderma asperellum, Trichoderma atroviride, Trichoderma cornu-damae, Trichoderma gamsii, Trichoderma hamatum, Trichoderma harzianum, Trichoderma pseudokoningii, Trichoderma reesei, Trichoderma simmonsii and Trichoderma virens (Kwak, 2020; Cai and Druzhinina, 2021). The characteristics of mitogenomes belonging to different representatives of Trichoderma genus are needed to elucidate the understanding of the evolution and potential technological use of specific isolates of this genus. The objectives of this work were to determine the complete mitogenome sequence of the T. koningiopsis POS7 strain and to compare its mitogenome organization with that of other known mitogenome from Subphylum Pezizomycotina.
2. Materials and methods
2.1. Strain
Trichoderma koningiopsis strain POS7 (Hypocreaceae) was isolated from soil samples (Posadas, Misiones, Argentina; Castrillo et al., 2017). Trichoderma koningiopsis POS7 was sequenced, NCBI ID: 337941, Bioproject PRJNA356137, Biosample SAMN06106985, WGS MRBD 00000000, ITS reference sequence KT030879, and was deposited at the Universidad Nacional de Misiones under accession number LBM116.
2.2. Mitochondrial genome assembly
In this study, the mitogenome of T. koningiopsis POS7 was determined from HiSeq Illumina reads data from whole genome sequencing of T. koningiopsis POS7 were used (Castrillo et al., 2017). All reads (7,773,936 reads) were used to map and assemble by comparing with mitochondrial DNA of other species of Trichoderma using the software Geneious 9.1.8 (Kearse et al., 2012). The reported mitogenomes of T. gamsii and T. asperellum (NC_030218 and NC_037075, respectively) were used as reference to map reads and assemble the mitogenome of T. koningiopsis. A total of 88,833 reads were retrieved from the complete genome sequencing project to assemble the mitogenome sequence. The contigs were manually curated into the final single genomic sequence. A single mitochondrial contig was extracted from the assembly for further analyses.
2.3. Mitochondrial genome annotation
Initial open reading frames were identified using ORF finder1 using the mold mitochondrial genetic code and Geneious 9.1.8 program. The protein-coding genes, rRNA, and tRNA genes of T. koningiopsis were predicted and curated manually based on comparisons with previously published Trichoderma mitogenome.
This initial annotation was helped using MFannot online tool2 based on Genetic Code 4 (Molds). The tRNAs genes were also identified using tRNAscan-SE 2.0 (Chan and Lowe, 2019). Putative functional assignments of genes were made based on sequence similarity to characterize fungal mitochondrial genes using BLASTN searches against NCBI databases (Altschul et al., 1990). Then manual examination was performed to rectify possible annotation errors. After confirming the presence of all the conserved mitochondrial genes in the assembled contig, the displayed overlaps at both ends were used to circularize the mitogenome.
Codon usage was determined using the online Sequence Manipulation Suite software (Stothard, 2000), and using the genetic code 4 for mold mitochondrial. The physical map of T. koningiopsis POS7 mitogenome was generated using the same software Geneious 9.1.8.
2.4. Phylogenetic analyses
Phylogenetic analyses were performed using the 14 conserved mitochondrial genes of a database constructed with selected taxa from the Subphylum Pezizomycotina (Cardona et al., 2018). In addition, one species belonging to the Subphylum Saccharomycotina, Wickerhamomyces canadensis was used as outgroups.
The complete mitogenomes of 22 species of Hypocreales and Sordariales order available in GenBank were retrieved, and two of them were annotated in this study (Table 1). The sequences of 14 conserved protein genes namely atp6, atp8, atp9, cox1, cox2, cox3, cob, nad1, nad2, nad3, nad4, nad4L, nad5, and nad6 from each species were concatenated and used for phylogenetic analyses.
Sequence alignment was performed using the Clustal W program of the MEGA 11package (Tamura et al., 2021) with parameters set to default. Phylogenetic reconstruction were inferred using the Maximum Likelihood (ML) and Neighbor Joining (NJ) methods using the Mega 11 package (Tamura et al., 2021) with parameters set to default. Boostrap values were determined using 1,000 replicates.
3. Results and discussion
3.1. General features of T. koningiopsis POS7 mitochondrial genome
The rapid development of next generation sequencing technologies has resulted in increasing the numbers of available mitogenomes from various organisms (Brankovics et al., 2017; Li et al., 2019). Fungal mitogenomes are among the most variable ones compared to others from several eukaryotic groups, both in size and organization (Hausner, 2003; Formey et al., 2012; Li et al., 2019; Lee et al., 2022).
So far, 2028 fungal mitochondrion NCBI Reference Sequences (RefSeq) are available in NCBI Database (https://www.ncbi.nlm.nih.gov/nuccore, January 2023). The overall average size of fungal mitogenomes sequenced is 50,512 bp (Jelen et al., 2016), though it can vary greatly, ranging from 11 kb in Hanseniaspora uvarum (Saccharomycetales, Pramateftaki et al., 2006; Megarioti and Kouvelis, 2020) to 236 kp in Rhizoctonia solani (Cantharellales from phylum Basidiomycota) or 272 kb in Morchella importuna (Pezizales, James et al., 2013; Losada et al., 2014; Li et al., 2020).
In this study, the mitogenome of the mycoparasitic fungus T. koningiopsis POS7 had a total size of 27,560 bp. The mtDNA sequence mean coverage in the assembly was 326X. This newly sequenced mitogenome was deposited in the GenBank database with the accession number MT816499. The size of T. koningiopsis POS7 mitogenome was the smallest genome among the 13 mitogenomes available in the Trichoderma genus (Table 1).
The GC content in organisms is considered to be influenced by mutational bias, selection, and biased recombination related to DNA repair, which could provide useful information regarding the evolution of different species (Li et al., 2019). The GC content of the T. koningiopsis POS7 mitogenome was 27.8%. This is in concordance with the other known Trichoderma mitogenomes evidencing no particular skew, ranging from 26.4% in T. reesei (NC_003388) to 28% in T. asperellum (NC_037075). These findings indicate that Trichoderma species may ultimately be subject to similar selection pressures; nevertheless, deeper studies with additional genomic regions are needed to confirm this.
Forty-three genes were detected into T. koningiopsis POS7 mitogenome, all of them being located on the sense strand (Table 2). The latter is in accordance with most Ascomycota mitogenomes where all genes are transcribed from the same DNA strand (Ghikas et al., 2006). However, in some Ascomycota some genes are located on the antisense strand as in Verticillium nonalfalfae (Glomerellales, Haridas and Gantt, 2010; Costa et al., 2016; Jelen et al., 2016).
The entire genome found in the mitochondrion of T. koningiopsis POS7 after its curated annotations is shown in Figure 1.
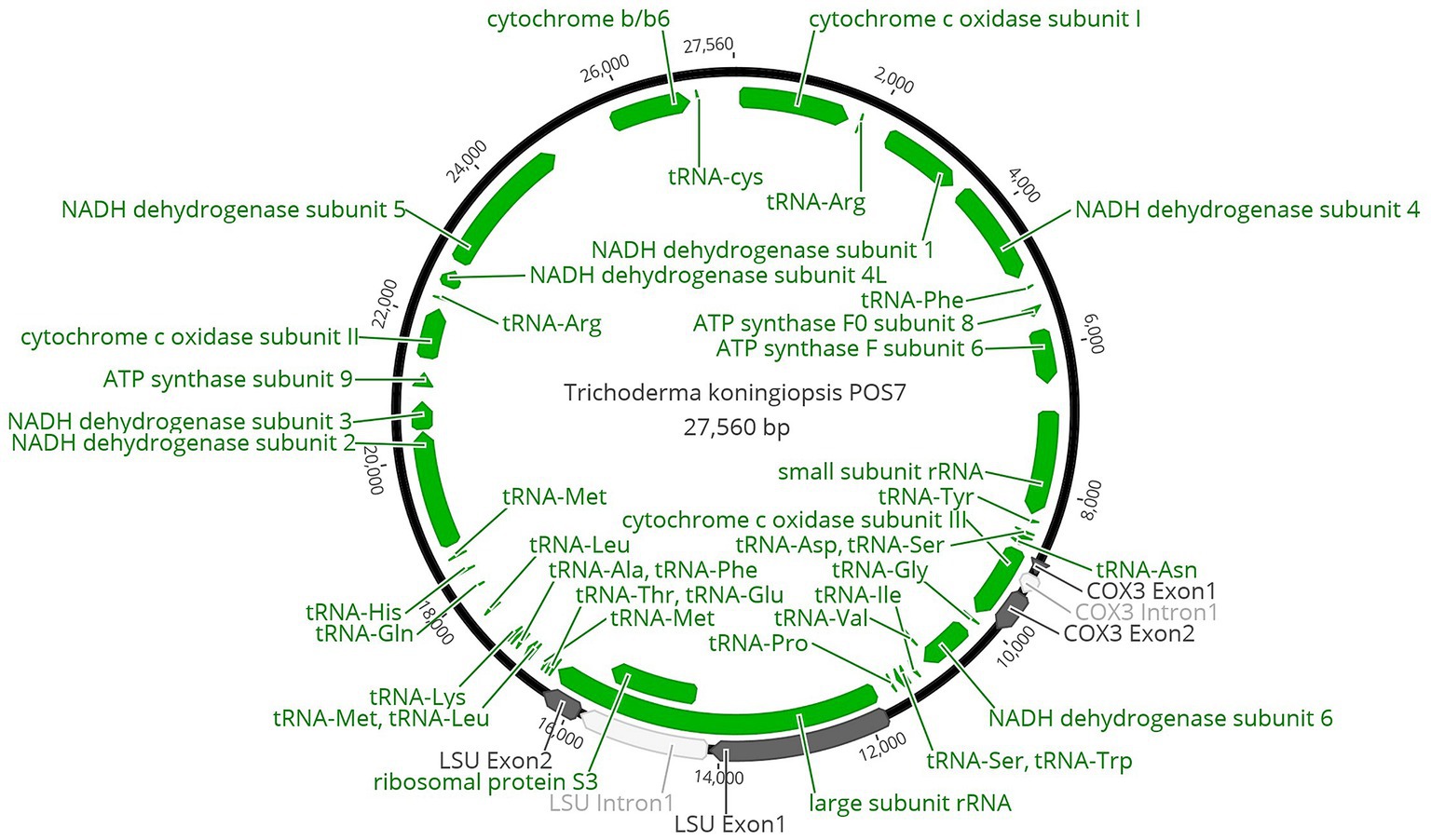
Figure 1. Circular map of Trichoderma koningiopsis POS7 mitochondrion. Proteins, rRNA and tRNA genes were indicated in green. Exons were indicated in dark grey and introns were indicated in white.
3.2. Protein-coding genes and codon usage
The T. koningiopsis POS7 mitogenome had the conserved set of 14 mitochondrial protein-coding genes: the ATP synthase subunits (atp6, atp8, and atp9), the cytochrome oxidase subunits (cox1, cox2, and cox3), the reduced nicotinamide adenine dinucleotide ubiquinone oxidoreductase subunits (nad1, nad2, nad3, nad4, nad4L, nad5, and nad6), and the apocytochrome b (cob). All these genes were located in the same order in the other species of Trichoderma genus (Supplementary Table S1). Remarkably, in the T. koningiopsis mitogenome, inside the cox3 gene an intron of 274 bp was located. Similarly, the mitogenomes of T. hamatum (MF287973) and T. asperellum (NC_037075) had an intron located also into the cox3 gene (Table 3).
Codon usage analyses indicated that the most frequently used codons in T. koningiopsis POS7 mitogenome were ATT (for isoleucine; Ile), TTT (for phenylalanine; Phe), and ATA (for isoleucine; Ile). The high frequency of AT content of these codons (72.2%) is in agreement to the high AT content of the other12 Trichoderma analyzed mitogenomes [from 72.1% in T. cornu-damae (MW525445) to 73.6% in T. reesei (NC_003388)].
Genes were also analyzed with respect to their start and stop codons. Among the predicted genes, the “AUG” initiation codon was mostly prevalent, with only the gene rps3 using the alternative “AUU” initiation codon (Deng et al., 2016). In all cases the stop codon was “UAA” (Table 2).
3.3. Ribosomal protein, transfer RNA, and ribosomal RNA genes
In T. koningiopsis POS7 mitogenome the mitochondrial ribosomal protein S3 (rps3) gene was found with a sequence length of 1,401 bp. This gene was also found in the mitogenomes of T. atroviride (1,389 bp—MN125601), T. hamatum (1,350 bp—MF287973), T. harzianum (1,368 bp—MN564945), T. lixii (1,386 bp—NC_052832), T. simmonsii (1,368 bp—NC_063562), T. cornu-damae (1,428 bp—MW525445), and T. afroharzianum (1,383 bp—NC_065768). Furthermore, in all of these mitogenomes, except T. cornu-damae, the rps3 gene is located into the large subunit ribosomal RNA (LSU rRNA; Supplementary Table S1). The rps3 gene of T. koningiopsis had elevated identity (> 91%) with the other rps3 gene of the Trichoderma genus, specifically with that belonging to T. atroviride (98.07%).
Across the fungi, the rps3 gene is probably implicated in the assembly of the mitochondrial ribosomal subunit and is extremely diverse in location and organization (Korovesi et al., 2018). Some versions of this gene have been incorporated into a group I intron, others appear to have gained large insertions, microsatellite expansions, or have been invaded by homing endonucleases (Sethuraman et al., 2009; Wai et al., 2019). Among these ones, the rps3 gene of T. afroharzianum is located into the LSU rRNA, together the LAGLIDADG, one of the two types of homing endonucleases known in fungal mitogenomes (Megarioti and Kouvelis, 2020), and the rps3 gene of T. lixii is located into an intron IA with 1,634 bp (Supplementary Table S1).
Also, rps3 gene, that is most likely native to mitogenome, can be lost, likely due to the presences of a nuclear-encoded analog or homolog that could complement for a missing mitochondrial-encoded rps3 gene (Wai et al., 2019). Analyzing mitogenomes from Trichoderma representatives, the rps3 gene seems have been in T. gamsii (NC_030218), T. pseudokoningii (OW971927) and T. asperellum (NC_037075) but was lost. These events may be resulted of a remnant of a mechanism that led to Organellar Gene Transfer (OGT) of the mt genes to the nucleus or to extinction, because the rps3 gene is an ancient gene whose evolutionary history may reflect the evolution of the fungal mitogenomes (Korovesi et al., 2018). Interestingly, in T. virens (1,411 bp, CP071122) and T. reesei (1,425 bp, NC_003388) a rps5 gene with similar length to the rps3 gene is found. Furthermore, this rps5 gene in the latter two mitogenomes is located into the LSU rRNA too (Supplementary Table S1).
A total of 26 tRNA genes were identified on T. koningiopsis POS7 mitogenome using tRNAscan. Among the tRNAs, all the 20 for standard amino-acids were accounted for, but for some amino-acids multiple tRNAs were found. There were found two copies of the tRNAs genes for arginine, phenylalanine, serine, and leucine, and three copies of the tRNA gene for methionine. No introns have been found in the tRNA genes detected. The number of tRNA genes reported in the 12 other Trichoderma mitogenomes ranged from 22 in T. afroharzianum (NC_065768) to 27 in T. atroviride (MN125601), and the tRNA genes with more than one copy, in general, are the same of the reported in T. koningiopsis POS7 mitogenome (Table 3; Figure 2).
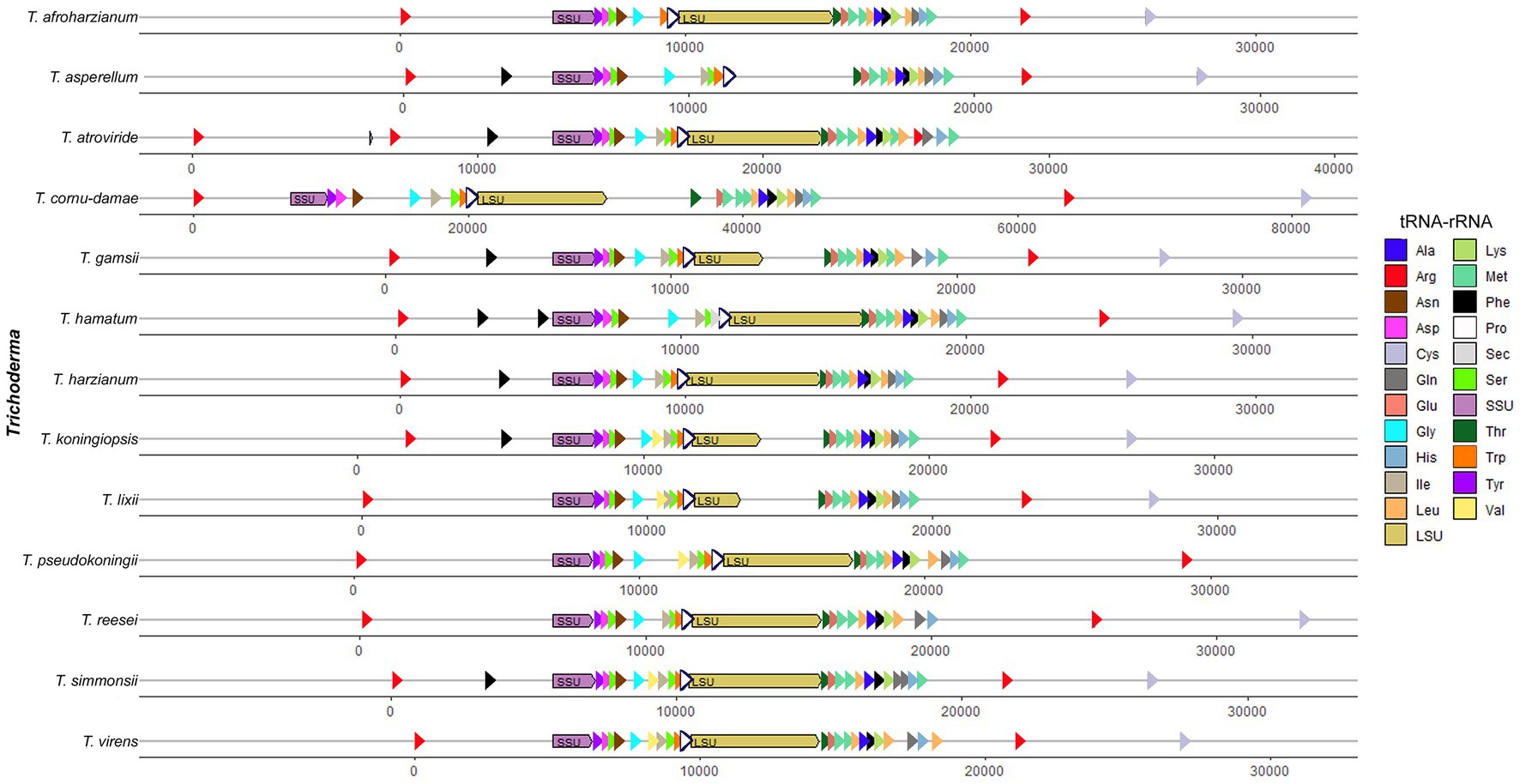
Figure 2. Annotated tRNA and rRNA genes order from analyzed Trichoderma mitogenomes. All tRNA and rRNA genes in their mitogenomes were arbitrarily organized starting immediately after the cox1 gene.
tRNA genes are an important nexus between mRNAs and proteins, as they are a fundamental component of the translation machinery in that they deliver amino acids to the ribosome to translate the genetic information in a mRNA (Kirchner and Ignatova, 2015). Mitochondrial tRNA mutations have been demonstrated to be associated with metabolism and various diseases in humans; however, little research has been done on tRNA mutations in fungal mitogenomes related to the growth and pathogenicity of pathogenic fungi (Chen et al., 2019).
It was reported a characteristic clustering of the trn genes in the mtDNA to all Pezizomycotina, just with minor differences, especially the cluster of 12 trn genes, located between rnl and nad2 genes in all Sordariomycetes remained almost unchanged both in gene content and order (Ghikas et al., 2006; Aguileta et al., 2014). In T. koningiopsis mitogenome, this cluster of 12 trn genes was identical to the cluster reported for T. pseudokoningii mitogenome, and it only had one less trnM gene between the third and the fourth trn genes compared to the cluster found in the T. lixii (NC_052832) and T. hamatum (MF287973) mitogenomes (Supplementary Table S1; Figure 2).
Two mitochondrial rRNA genes were identified in the T. koningiopsis POS7 mitogenome, namely the large subunit ribosomal RNA (rnl) and the small subunit ribosomal RNA (rns) genes. These genes were similar to the others compared Trichoderma fungal mitogenomes (Table 3; Figure 2), with one exception, in the case of T. asperellum (NC_037075) which had only one mitochondrial rRNA gene reported (Kwak, 2020). The rnl gene in the T. koningiopsis POS7 mitogenome was 4,702 bp in length with an intronic sequence of 1,750 bp containing the rps3 gene. The rns gene was a complete gene sequence of 1,503 bp without any intronic spacer identified. The rns genes of T. reesei (NC_003388), T. virens (CP071122), T. afroharzianum (NC_065768), T. pseudokoningii (OW971927) and T. cornu-damae (MW525445) had a similar intronic sequence (Table 3; Supplementary Table S1). Furthermore, the rnl gene of T. koningiopsis had a high identity (> 94%) with the other rns genes of the Trichoderma genus, particularly this gene had the highest identity (98.41%) with the rnl gene of T. atroviride.
3.4. Intronic and intergenic regions
Mitochondrial introns are divided into two groups (I and II) based on their secondary structure and splicing mechanism (Zhang and Zhang, 2019). Group I introns are considered to be mobile genetic elements which interrupt protein-coding and RNA genes in all domains of life (Edgell et al., 2011). In fungi, introns are inserted in many different mitochondrial genes, with a strong preference for protein-coding genes, most frequently cox and cob (Paquin et al., 1997; Megarioti and Kouvelis, 2020). However, despite the small genome size, two introns were detected in the T. koningiopsis POS7 mitogenome.
The intron of 274 bp in cox3 gene had a high identity (BLAST) with the intron 1 in cox3 gene of Cordyceps militaris (OM203116 and MW387534, 96.3% 1e–40) and Cordyceps cicadae (NC_041489, MK110677, and MH922223, 91.59% 4e–31), which are an intron Group IB. In addition, the another intron found in T. koningiopsis POS7 mitogenome had a size of 1,750 bp was detected in the rnl gene. The latter intron had high identity (BLAST; 97.6%, 1e-0) with the mitogenomes of T. atroviride (NC_048477 and MN125601), T. gamsii (NC_030218 and KU687109) and T. hamatum (MF287973 and NC_036144).
Related to the other species in the genus, T. asperellum (NC_037075) has one intron of 1,118 bp into cox3 gene reported in its mitogenome (3.73%), and T. hamatum (MF287973) has four introns reported with a total size of 4,825 bp (14.73%), one of these is located into cox3 gene with a total size of 1,112 bp. Related to introns into rnl gene, T. reesei (NC_003388) has nine introns reported with a total size of 10,963 bp (26.02%), and one of these is located into rnl gene with a total size of 1,535 bp. T. virens (CP071122) has three introns reported with a total size of 2,720 bp (8.75%), and one of these is located also into rnl gene with a total size of 1,520 bp. T. pseudokoningii (OW971927) has eight introns reported with a total size of 8,805 bp (19.52%), and one of these is located into rnl gene with a total size of 1,539 bp, and the mitogenomes of T. afroharzianum (NC_065768, total size of 4,000 bp—13.55%) and T. cornu-damae (MW525445, total size of 45,522 bp—48.12%) have many identified introns into their genes, and particularly have two and four introns into their rnl genes, respectively (Table 3). So, the existence of only two intronic sequences in T. koningiopsis POS7 mitogenome might constitute an intermediate condition in the continuum reported for the representatives of Trichoderma genus, accounting for 7.34% of this mitogenome with a total size of 2,024 bp.
The high evolution rate of mitogenomes makes that amount and localization of their introns can lead to explain variability found among different strains within the same genus or even within a species (Deng et al., 2016). Related to introns, one evolutive hypothesis suggests that within any particular host lineage, a dynamic cycle of invasion of them might be the result of several processes, including horizontal transmission, degeneration or eventual loss followed by reinvasion (Goddard and Burt, 1999; Mardanov et al., 2014). According to other authors, intron variation can be explained evolutionarily using theories proposed for all organisms. One theory, called “Early Intron,” proposes that introns were abundant in ancestral genes, but a general evolutionary process led to the loss of introns over time (Goddard and Burt, 1999; Gonzalez et al., 1999). Another theory, known as the “Late Intron,” suggests that intron mobility allows for expansion within genes due to events of horizontal transfer, even between phylogenetically distant species (Vaughn et al., 1995; Gonzalez et al., 1998). A third hypothesis called “aenaon” combines features of the first two models (Megarioti and Kouvelis, 2020). On the basis of the number of introns, sequence identities, and the locations of them in related species, we could hypothesize that the variation of introns in T. koningiopsis was related to this third theory.
All this information could suggests that the number of introns in mitogenomes might contribute to the variability such as it was inferred here where T. cornu-damae (94,608 bp—MW525445) had the largest mitogenome with 48.12% of introns, versus the shortest mitogenome of T. koningiopsis with 7.34% (Hausner, 2003; Deng et al., 2016; Kanzi et al., 2016; Fan et al., 2019; Lee et al., 2022). However, on the basis of available studies about mitochondrial gene order, Aguileta et al. (2014) did not found a significant correlation between rearrangements of the fungal mitogenomes and the proportions of introns and intronic ORF.
Other authors indicated that the length of intergenic regions is also other key contributor to mitochondrial genomic size variation in fungi (Lin et al., 2015). In the T. koningiopsis POS7 mitogenome, the smallest mitogenome in the genus, the intergenic regions accounted for a total of 5,960 bp (21.63% of the total mitgenome), with lengths ranging from 4 to 890 bp. The largest intergenic region was between nad5 and cob genes. Meanwhile, in all of the other analyzed mitogenomes, the length of intergenic regions was similar to T. koningiopsis, with the exception of the species with the largest mitogenome in the genus T. cornu-damae (MW525445; Kwak, 2020; Lee et al., 2022). So, in Trichoderma, intergenic regions are not proposed as a key force modeling mitochondrial genomic size variation.
3.5. Phylogenetic analyses
The most common approach for phylogenetic studies of fungi with mitochondrial sequences is using a set of conserved proteins from their annotated sequences. Since mitogenomes corresponding to the evaluated species are considered to be circular molecules, the alignment start position was arbitrarily decided as the cox1 gene (Jelen et al., 2016).
Mitogenomes of T. koningiopsis POS7 and of 23 other selected fungi (Table 1) were used to construct ML and NJ phylogenetic trees. Both algorithms yielded single trees with the same branch topology (for clarity only the NJ tree is presented in Figure 3).
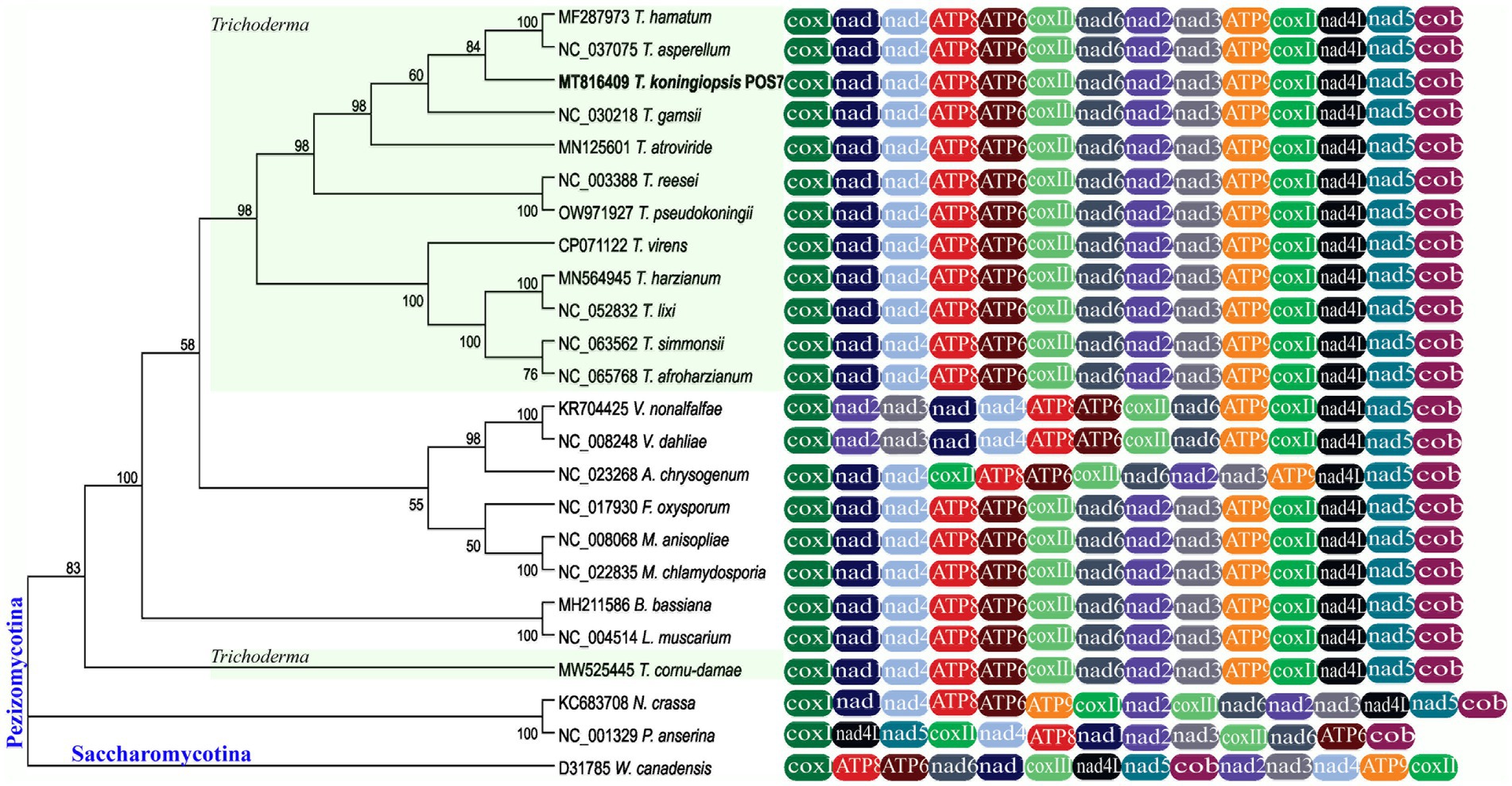
Figure 3. The Trichoderma koningiopsis POS7 phylogenetic analysis of 14 mitochondrial protein-encoding genes through ML. One species from the Saccharomycotina subphylum (Wickerhamomyces Canadensis D31785) were used as outgroup. The mtDNA T. koningiopsis POS7 is indicated in bold letters. Numbers on branches correspond to bootstrap values obtained with 1,000 replicates. Nodes with Bootstraps values below 50% were collapsed.
Based on the phylogenetic analysis of the 14 conserved genes used, the 24 Ascomycota species could be divided into two major clades corresponding to the subphyla Pezizomycotina and Saccharomycotina (this latter used as outgroup). Most nodes in this tree had high bootstrap values which indicate the robustness of the phylogenetic tree inferred.
In the Pezizomycotina clade, mitogenomes of the orders Hypocreales and Glomerellales were clustered including a big clade containing all Trichoderma mitogenomes (support value 98%), with the exception of the largest Trichoderma mitogenome, T. cornu-damae (MW525445).
A similar pattern of phylogeny branching was obtained for fungi of Ascomycota by Ghikas et al. (2006), for selected Basidiomycota and Ascomycota by Aguileta et al. (2014), for Pezizomycotina by Cardona et al. (2018), and for Hypocreales by Wang et al. (2017) and Lee et al. (2022). Therefore, the combination of 14 mitochondrial gene set analyzed in this work may be a useful strategy for some fungal taxa identification and phylogenetic analysis.
Even it has been reported that the mitochondrial gene order in fungi varied greatly among different species, even among strains from the same species (Li et al., 2019). In the present study, the mitochondrial gene order of T. koningiopsis was identical to all other Trichoderma mitogenomes reported (Supplementary Table S1), so we did not find that a gene rearrangement occurred among Trichoderma species including other closely related fungal genus involving mitochondrial core genes encoding proteins.
Related to the gene order comparison, 18 of the 19 compared species of the order Hypocreales analyzed have complete synteny in gene order. In contrast, the gene order in the mitogenome of Acremonium chrysogenum differs only in the location of the gene cox2, between nad4 and atp8 genes. Lin et al. (2015) evaluated the mitochondrial gene order of different members belonging to Hypocreales and obtained similar results. Also, it was observed that V. nonalfalfae and V. dahliae, from the Glomerellales order, were clustered together with our evaluated sequences of the Hypocreales order, but it was observed that the nad2 and nad3 genes shifted in their gene order between the cox1 and nad1 genes.
Even the fungal mitogenome organization is apparently quite diverse; certain features appear to be conserved in the mitogenome organization of some related fungi like the overlapping of nad4L/nad5 so that the last base of the first gene was also the first base of the second gene, a feature that is underlined as common in mitogenomes of Sordariomycetes (Kouvelis et al., 2004). It was noted that in general the nad4L/nad5 and nad2/nad3 genes, tend to be next to each other, and that in these two pairs of genes existed an overlap of the stop and initiation nucleotides (Aguileta et al., 2014).
Both mitochondrial and nuclear gene sequences have been employed in efforts to reconstruct phylogenetic relationships among fungi. Also, similar phylogeny branching could be obtained using ITS genes for the same Trichoderma species (Castrillo, 2015; Castrillo et al., 2021b). But in other groups of organisms the mitochondrial data alone have less resolving power that nuclear genes where in contrast phylogenies from nuclear data is generally well-supported (Springer et al., 2001; Wiens et al., 2010).
Mitochondrial sequences have been found to be useful to determine and/or confirm phylogenetic relationships, especially in cases where nuclear genes are not reliable molecular timers to clarify conflicting phylogenies (Ghikas et al., 2010; Kanzi et al., 2016). Aguileta et al. (2014) argued that although mitochondrial genes tend to be conserved due to their universal role in cellular metabolism, fungal mitochondrial gene order is relatively free to vary, and that this variation is probably largely due to recombination. It is worth mentioning that it was reported that mitochondrial intergenic regions can serve as information for phylogenetic analysis and for the development of tools for intra-and inter-specific discrimination of fungi (Ghikas et al., 2006, 2010).
Other sources of fungal mitogenome variation are presence of repeats, variable numbers of ORFs with an unknown function and variability in the mitochondrial gene order (Jelen et al., 2016). The arrangement of mitochondrial genes has been widely employed to understand the phylogenetic status of species (Ghikas et al., 2006; Li et al., 2019; Chen et al., 2019).
In many cases, it has been shown that a single gene may not always represent the history of the genome containing it, and analyses based on this single gene may lead to wrong conclusions about the phylogenetic relationship of a fungus (Franco et al., 2016). The independent nature of mitochondrial genomes from nuclear genomes presents a promising and alternative source of data for phylogenetic analysis. Thus, the sequence of the entire mitogenome, the set of their 14 conserved protein coding-genes, the set of their tRNA and rRNA sequences, or even only their intergenic sequences, may be considered a promising alternative for phylogenetic analyses. All this can provide an approach to evaluate genetic divergences in related taxons, or offer a thorough view of the placement and types of various genes, as well as any connected introns that may reveal ancestral linkages to study the origin and evolution of eukaryotes, as in the case of fungi (Chen et al., 2019). This might be the case also for inferring phylogenetic uncertainties found in the Hypocreales group. In the last years different Trichoderma mitochondrial genome were reported, and 12 were included and analyzed. Therefore, the mitochondrial genome of T. koningiopsis POS7 will allow further investigations into evolutionary biology of this important genus as well as other closely related species. Since Medina et al. (2020) reported that both conservative mitochondrial genes and highly variable regions in the fungal mitogenomes are involved in the phenotypic plasticity of hosts, future studies analyzing other isolates of T. koningiopsis that differ in their mycoparasitic ability might give information about the contribution of the mitogenome to the behavior of these strains in biocontrol of phytopathogens and for the selection for other biotechnological uses.
Data availability statement
The datasets presented in this study can be found in online repositories. The names of the repository/repositories and accession number(s) can be found at: https://www.ncbi.nlm.nih.gov/genbank/, MT816499.1.
Author contributions
MC, GB, NA, MB, PZ, MS, and LV participated in the design of the study. MC, GB, NA, and MB performed the experiments. MC and GB analyzed the data and wrote the paper. All authors contributed to the critical revision of the manuscript and have seen and approved the final draft. All authors read and approved the final manuscript.
Acknowledgments
The authors would also like to thank the Consejo Nacional de Investigaciones Científicas y Técnicas (CONICET, Argentina). NA and MB are a postgraduate CONICET fellowship holder. MC, GB, PZ, and MS are researchers in CONICET, Argentina. The authors would also like to thank the Agencia Nacional de Promoción Científica y Tecnológica (PICT-2021-GRF-TI-00528).
Conflict of interest
The authors declare that the research was conducted in the absence of any commercial or financial relationships that could be construed as a potential conflict of interest.
Publisher’s note
All claims expressed in this article are solely those of the authors and do not necessarily represent those of their affiliated organizations, or those of the publisher, the editors and the reviewers. Any product that may be evaluated in this article, or claim that may be made by its manufacturer, is not guaranteed or endorsed by the publisher.
Supplementary material
The Supplementary material for this article can be found online at: https://www.frontiersin.org/articles/10.3389/fmicb.2023.1141087/full#supplementary-material
Footnotes
1. ^https://www.ncbi.nlm.nih.gov/orffinder/
2. ^https://megasun.bch.umontreal.ca/cgi-bin/dev_mfa/mfannotInterface.pl
References
Aguileta, G., De Vienne, D. M., Ross, O. N., Hood, M. E., Giraud, T., and Petit, E. (2014). High variability of mitochondrial gene order among fungi. Genome Biol. Evol. 6, 451–465. doi: 10.1093/gbe/evu028
Altschul, S. F., Gish, W., Miller, W., Myers, E. W., and Lipman, D. J. (1990). Basic local alignment search tool. J. Mol. Biol. 215, 403–410. doi: 10.1016/S0022-2836(05)80360-2
Amerio, N. S., Castrillo, M. L., Bich, G. A., Zapata, P. D., and Villalba, L. L. (2020). Trichoderma en la Argentina: Estado del arte. Ecol. Austral 30, 113–124. doi: 10.25260/EA.20.30.1.0.945
Antal, Z., Manczinger, L., Kredics, L., Kevei, F., and Nagy, E. (2002). Complete DNA sequence and analysis of a mitochondrial plasmid in the mycoparasitic Trichoderma harzianum strain T95. Plasmid 47, 148–152. doi: 10.1006/plas.2001.1559
Baroncelli, R., Piaggeschi, G., Fiorini, L., Bertolini, E., Zapparata, A., Enrico Pè, M., et al. (2015). Draft whole-genome sequence of the biocontrol agent Trichoderma harzianum T6776. Genome Announc. 3, 00647–00615. doi: 10.1128/genomeA.00647-15
Baroncelli, R., Zapparata, A., Piaggeschi, G., Sarrocco, S., and Vannacci, G. (2016). Draft whole-genome sequence of Trichoderma gamsii T6085, a promising biocontrol agent of Fusarium head blight on wheat. Genome Announc. 4, 01747–01715. doi: 10.1128/genomeA.01747-15
Brankovics, B., van Dam, P., Rep, M., de Hoog, G. S., et al. (2017). Mitochondrial genomes reveal recombination in the presumed asexual Fusarium oxysporum species complex. BMC Genom. 18:735. doi: 10.1186/s12864-017-4116-5
Burger, G., Gray, M. W., and Lang, B. F. (2003). Mitochondrial genomes: anything goes. Trends Genet. 19, 709–716. doi: 10.1016/j.tig.2003.10.012
Cai, F., and Druzhinina, I. S. (2021). In honor of John Bissett: authoritative guidelines on molecular identification of Trichoderma. Fungal Divers. 107, 1–69. doi: 10.1007/s13225-020-00464-4
Cardona, N. L., Franco-Sierra, N. D., and Correa, A. J. (2018). Complete mitogenome of the biocontroller fungus Purpureocillium sp. (Ascomycota, Ophiocordycipitaceae, Hypocreales). Mitochondrial DNA Part B 3, 1158–1160. doi: 10.1080/23802359.2018.1522982
Castrillo, M. L . (2015). Caracterización de celulasas secretadas por aislamientos de Trichoderma, nativos de la provincia de Misiones (Argentina) aplicables en la etapa de sacarificación. doctoral thesis. Universidad Nacional de Misiones, Misiones, Argentina.
Castrillo, M. L., Bich, G. A., Amerio, N. S., Rodriguez, M. D., Zapata, P. D., and Villalba, L. L. (2021b). Assessment of cellulase complex secretory capacity of Trichoderma strains and morphological and molecular identification of the isolate with the highest enzymatic secretion capacity. J. Microbiol. Biotechnol. Food Sci. 10:e1357. doi: 10.15414/jmbfs.1357
Castrillo, M. L., Bich, G. A., Kramer, G. R., Velazquez, J. E., Rodriguez, M., Zapata, P., et al. (2015). Utilization of synthetic and semi-synthetic culture media for endo-1,4-β-glucanases secretion by Trichoderma koningiopsis. Procedia Materials Science 8, 786–792. doi: 10.1016/j.mspro.2015.04.136
Castrillo, M. L., Bich, G. Á., Modenutti, C., Turjanski, A., Zapata, P. D., and Villalba, L. L. (2017). First whole-genome shotgun sequence of a promising cellulase secretor, Trichoderma koningiopsis strain POS7. Genome Announc. 5, 00823–00817. doi: 10.1128/genomeA.00823-17
Castrillo, M. L., Bich, G. A., Sioli, G. A., Zapata, P. D., and Villalba, L. L. (2021a). Capacidad biocontroladora de aislamientos nativos de Trichoderma sp. contra el hongo fitopatógeno Alternaria alternata, aislado de yerba mate (Ilex paraguariensis Saint Hil.). Chilean J. Agric. Anim. Sci. 37, 244–256. doi: 10.29393/chjaas37-26cbml50026
Chambergo, F. S., Bonaccorsi, E. D., Ferreira, A. J., Ramos, A. S. P., Ferreira, J. R., Abrahao-Neto, J., et al. (2002). Elucidation of the metabolic fate of glucose in the filamentous fungus Trichoderma reesei using expressed sequence tag (EST) analysis and cDNA microarrays. J. Biol. Chem. 277, 13983–13988. doi: 10.1074/jbc.M107651200
Chan, P. P., and Lowe, T. M. (2019). tRNAscan-SE: searching for tRNA genes in genomic sequences. Methods Mol. Biol. 1962, 1–14. doi: 10.1007/978-1-4939-9173-0_1
Chen, C., Li, Q., Fu, R., Wang, J., Xiong, C., Fan, Z., et al. (2019). Characterization of the mitochondrial genome of the pathogenic fungus Scytalidium auriculariicola (Leotiomycetes) and insights into its phylogenetics. Sci. Rep. 9, 17447–17412. doi: 10.1038/s41598-019-53941-5
Costa, G. G., Cabrera, O. G., Tiburcio, R. A., Medrano, F. J., Carazzolle, M. F., Thomazella, D. P., et al. (2016). The mitochondrial genome of Moniliophthora roreri, the frosty pod rot pathogen of cacao. Fungal Biol-UK 116, 551–562. doi: 10.1016/j.funbio.2012.01.008
Deng, Y., Zhang, Q., Ming, R., Lin, L., Lin, X., Lin, Y., et al. (2016). Analysis of the mitochondrial genome in Hypomyces aurantius reveals a novel two intron complex in fungi. Int. J. Mol. Sci. 17:1049. doi: 10.3390/ijms17071049
Edgell, D. R., Chalamcharla, V. R., and Belfort, M. (2011). Learning to live together: mutualism between self-splicing introns and their hosts. BMC Biol. 9:22. doi: 10.1186/1741-7007-9-22
Fan, W. W., Zhang, S., and Zhang, Y. J. (2019). The complete mitochondrial genome of the Chan-hua fungus Isaria cicadae: a tale of intron evolution in Cordycipitaceae. Environ. Microbiol. 21, 864–879. doi: 10.1111/1462-2920.14522
Fatma, T., Khan, H. A., Ahmed, A., Adnan, F., Virk, N., and Bhatti, M. F. (2023). Functional annotation and comparative analysis of four Botrytis cinerea mitogenomes reported from Punjab, Pakistan, Saudi. J. Biol. Sci. 30:103605. doi: 10.1016/j.sjbs.2023.103605
Formey, D., Molès, M., Haouy, A., Savelli, B., et al. (2012). Comparative analysis of mitochondrial genomes of Rhizophagus irregularis–syn. Glomus irregulare–reveals a polymorphism induced by variability generating elements. New Phytol. 196, 1217–1227. doi: 10.1111/j.1469-8137.2012.04283-x
Franco, M. E. E., López, S. M. Y., Saparrat, M. C. N., and Balatti, P. A. (2016). Mitochondrial and nuclear gene sequences to infer the phylogeny of Pezizomycotina (Ascomycota). In American Phytopathological Society Annual Meeting.
Ghikas, D. V., Kouvelis, V. N., and Typas, M. A. (2006). The complete mitochondrial genome of the entomopathogenic fungus Metarhizium anisopliae var. anisopliae: gene order and trn gene clusters reveal a common evolutionary course for all Sordariomycetes, while intergenic regions show variation. Arch. Microbiol. 185:393. doi: 10.1007/s00203-006-0104-x
Ghikas, D. V., Kouvelis, V. N., and Typas, M. A. (2010). Phylogenetic and biogeographic implications inferred by mitochondrial intergenic region analyses and ITS1-5.8 S-ITS2 of the entomopathogenic fungi Beauveria bassiana and B. brongniartii. BMC Microbiol. 10, 1–15. doi: 10.1186/1471-2180-10-174
Goddard, M. R., and Burt, A. (1999). Recurrent invasion and extinction of a selfish gene. Proc. Natl. Acad. Sci. USA 96, 13880–13885. doi: 10.1073/pnas.96.24.13880
Gonzalez, P., Barroso, G., and Labarere, J. (1998). Molecular analysis of the split cox1 gene from the Basidiomycota Agrocybe aegerita: relationship of its introns with homologous Ascomycota introns and divergence levels from common ancestral copies. Gene 220, 45–53. doi: 10.1016/s0378-1119(98)00421-1
Gonzalez, P., Barroso, G., and Labarere, J. (1999). Molecular gene organisation and secondary structure of the mitochondrial large subunit ribosomal RNA from the cultivated Basidiomycota Agrocybe aegerita: a 13 kb gene possessing six unusual nucleotide extensions and eight introns. Nucleic Acids Res 27, 1754–1761. doi: 10.1093/nar/27.7.1754
Gray, M. W., Burger, G., and Lang, B. F. (1999). Mitochondrial evolution. Science 283, 1476–1481. doi: 10.1126/science.283.5407.1476
Guha, T. K., Wai, A., Mullineux, S. T., and Hausner, G. (2018). The intron landscape of the mtDNA cytb gene among the Ascomycota: introns and intron-encoded open reading frames. Mitochondrial DNA part A., 29 1015–1024. doi: 10.1080/24701394.2017.1404042
Halliwell, G., and Griffin, M. (1973). The nature and mode of action of the cellulolytic component C1 of Trichoderma koningii on native cellulose. Biochem. J. 135, 587–594. doi: 10.1042/bj1350587
Haridas, S., and Gantt, J. S. (2010). The mitochondrial genome of the wood-degrading basidiomycete Trametes cingulata. FEMS Microbiol. Lett. 308, 29–34. doi: 10.1111/j.1574-6968.2010.01979.x
Hausner, G. (2003). Fungal Mitochondrial Genomes. Fungal. Genomics 3:101. doi: 10.1016/S1874-5334(03)80009-6
James, T. Y., Pelin, A., Bonen, L., Ahrendt, S., Sain, D., Corradi, N., et al. (2013). Shared signatures of parasitism and phylogenomics unite Cryptomycota and microsporidia. Curr. Biol. 23, 1548–1553. doi: 10.1016/j.cub.2013.06.057
Jelen, V., de Jonge, R., van de Peer, Y., Javornik, B., and Jakše, J. (2016). Complete mitochondrial genome of the Verticillium-wilt causing plant pathogen Verticillium nonalfalfae. PLoS One 11:e0148525. doi: 10.1371/journal.pone.0148525
Kanzi, A. M., Wingfield, B. D., Steenkamp, E. T., Naidoo, S., and van der Merwe, N. A. (2016). Intron derived size polymorphism in the mitochondrial genomes of closely related Chrysoporthe species. PLoS One 11:e0156104. doi: 10.1371/journal.pone.0156104
Kearse, M., Moir, R., Wilson, A., Stones-Havas, S., Cheung, M., Sturrock, S., et al. (2012). Geneious basic: an integrated and extendable desktop software platform for the organization and analysis of sequence data. Bioinformatics 28, 1647–1649. doi: 10.1093/bioinformatics/bts199
Kirchner, S., and Ignatova, Z. (2015). Emerging roles of tRNA in adaptive translation, signalling dynamics and disease. Nat. Rev. Genet. 16, 98–112. doi: 10.1038/nrg3861
Korovesi, A. G., Ntertilis, M., and Kouvelis, V. N. (2018). Mt-rps3 is an ancient gene which provides insight into the evolution of fungal mitochondrial genomes. Mol. Phylogenet. Evol. 127, 74–86. doi: 10.1016/j.ympev.2018.04.037
Kouvelis, V. N., Ghikas, D. V., and Typas, M. A. (2004). The analysis of the complete mitochondrial genome of Lecanicillium muscarium (synonym Verticillium lecanii) suggests a minimum common gene organization in mtDNAs of Sordariomycetes: phylogenetic implications. Fungal Genet. Biol. 41, 930–940. doi: 10.1016/j.fgb.2004.07.003
Kubicek, C. P., Herrera-Estrella, A., Seidl-Seiboth, V., Martinez, D. A., Druzhinina, I. S., Thon, M., et al. (2011). Comparative genome sequence analysis underscores mycoparasitism as the ancestral life style of Trichoderma. Genome Biol. 12:R40. doi: 10.1186/gb-2011-12-4-r40
Kwak, Y. (2020). Complete mitochondrial genome of the fungal biocontrol agent Trichoderma atroviride: genomic features, comparative analysis and insight into the mitochondrial evolution in Trichoderma. Front. Microbiol, 11, 1–16. doi: 10.3389/fmicb.2020.00785
Lang, B. F. (2018). “Mitochondrial genome in Fung” in Molecular Life Sciences. eds. R. D. Wells, J. S. Bond, J. Klinman, and B. S. S. Masters (New York: Springer), 722–728.
Lee, H. Y., Jo, J. W., Kwak, Y. N., Lee, H., Ryu, H., and Chung, J. W. (2022). The complete mitochondrial genome of the poisonous mushroom Trichoderma cornu-damae (Hypocreaceae). Mitochondrial DNA Part B 7, 1899–1901. doi: 10.1080/23802359.2022.2135393
Li, Q., Wang, Q., Jin, X., Chen, Z., Xiong, C., Li, P., et al. (2019). Characterization and comparative analysis of six complete mitochondrial genomes from ectomycorrhizal fungi of the Lactarius genus and phylogenetic analysis of the Agaricomycetes. Int. J. Biol. Macromol. 121, 249–260. doi: 10.1016/j.ijbiomac.2018.10.029
Li, Q., Yang, L., Xiang, D., Wan, Y., Wu, Q., Huang, W., et al. (2020). The complete mitochondrial genomes of two model ectomycorrhizal fungi (Laccaria): features, intron dynamics and phylogenetic implications. Int. J. Biol. Macromol. 145, 974–984. doi: 10.1016/j.ijbiomac.2019.09.188
Lin, R., Liu, C., Shen, B., Bai, M., Ling, J., Chen, G., et al. (2015). Analysis of the complete mitochondrial genome of Pochonia chlamydosporia suggests a close relationship to the invertebrate-pathogenic fungi in Hypocreales. BMC Microbiol. 15, 1–15. doi: 10.1186/s12866-015-0341-8
Losada, L., Pakala, S. B., Fedorova, N. D., Joardar, V., Shabalina, S. A., Hostetler, J., et al. (2014). Mobile elements and mitochondrial genome expansion in the soil fungus and potato pathogen Rhizoctonia solani AG-3. FEMS Microbiol. Lett. 352, 165–173. doi: 10.1111/1574-6968.12387
Mardanov, A. V., Beletsky, A. V., Kadnikov, V. V., Ignatov, A. N., and Ravin, N. V. (2014). The 203 kbp mitochondrial genome of the phytopathogenic fungus Sclerotinia borealis reveals multiple invasions of introns and genomic duplications. PLoS One 9:e107536. doi: 10.1371/journal.pone.0107536
Martinez, D., Berka, R. M., Henrissat, B., Saloheimo, M., et al. (2008). Genome sequencing and analysis of the biomass-degrading fungus Trichoderma reesei (syn. Hypocrea jecorina). Nat. Biotechnol. 26, 553–560. doi: 10.1038/nbt1403
Medina, R., Franco, M. E. E., Bartel, L. C., Martinez Alcántara, V., Saparrat, M. C. N., and Balatti, P. A. (2020). Fungal Mitogenomes: relevant features to Pplanning plant disease management. Front. Microbiol. 11:978. doi: 10.3389/fmicb.2020.00978
Megarioti, A. H., and Kouvelis, V. N. (2020). The coevolution of fungal mitochondrial introns and their homing endonucleases (GIY-YIG and LAGLIDADG). Genome Biol. Evol. 12, 1337–1354. doi: 10.1093/gbe/evaa126
Paquin, B., Laforest, M. J., Forget, L., Roewer, I., Wang, Z., Longcore, J., et al. (1997). The fungal mitochondrial genome project: evolution of fungal mitochondrial genomes and their gene expression. Curr. Genet. 31, 380–395. doi: 10.1007/s002940050220
Pramateftaki, P. V., Kouvelis, V. N., Lanaridis, P., and Typas, M. A. (2006). The mitochondrial genome of the wine yeast Hanseniaspora uvarum: a unique genome organization among yeast/fungal counterparts. FEMS Yeast Res. 6, 77–90. doi: 10.1111/j.1567-1364.2005.00018.x
Proctor, R. H., McCormick, S. P., Kim, H. S., Cardoza, R. E., Stanley, A. M., Lindo, L., et al. (2018). Evolution of structural diversity of trichothecenes, a family of toxins produced by plant pathogenic and entomopathogenic fungi. PLoS Pathog. 14:e1006946. doi: 10.1371/journal.ppat.1006946
Samuels, G. J., Dodd, S. L., Lu, B. S., Petrini, O., Schroers, H. J., Druzhinina, I. S., et al. (2006). The Trichoderma koningii aggregate species. Stud. Mycol. 56, 67–133. doi: 10.3114/sim.2006.56.03
Sethuraman, J., Majer, A., Iranpour, M., and Hausner, G. (2009). Molecular evolution of the mtDNA encoded rps3 gene among filamentous ascomycetes fungi with an emphasis on the ophiostomatoid fungi. J. Mol. Evol. 69, 372–385. doi: 10.1007/s00239-009-9291-9
Shi-Kunne, X., Seidl, M. F., Faino, L., and Thomma, B. P. (2015). Draft genome sequence of a strain of cosmopolitan fungus Trichoderma atroviride. Genome Announc. 3, e00287–e00215. doi: 10.1128/genomeA.00287-15
Song, N., Geng, Y., and Li, X. (2020). The mitochondrial genome of the phytopathogenic fungus Bipolaris sorokiniana and the utility of mitochondrial genome to infer phylogeny of Dothideomycetes. Front. Microbiol. 11:863. doi: 10.3389/fmicb.2020.00863
Springer, M. S., DeBry, R. W., Douady, C., Amrine, H. M., Madsen, O., de Jong, W. W., et al. (2001). Mitochondrial versus nuclear gene sequences in deep-level mammalian phylogeny reconstruction. Mol. Biol. Evol. 18, 132–143. doi: 10.1093/oxfordjournals.molbev.a003787
Steindorff, A. S., Ramada, M. H., Coelho, A. S., Miller, R. N., Pappas, G. J. Júnior, Ulhoa, C. J., et al. (2014). Identification of mycoparasitism-related genes against the phytopathogen Sclerotinia sclerotiorum through transcriptome and expression profile analysis in Trichoderma harzianum. BMC Genomics 15:204. doi: 10.1186/1471-2164-15-204
Stothard, P. (2000). The sequence manipulation suite: Java script programs for analyzing and formatting protein and DNA sequences. BioTechniques 28, 1102–1104. doi: 10.2144/00286ir01
Studholme, D. J., Harris, B., le Cocq, K., Winsbury, R., Perera, V., Ryder, L., et al. (2013). Investigating the beneficial traits of Trichoderma hamatum GD12 for sustainable agriculture-insights from genomics. Front. Plant Sci. 4:258. doi: 10.3389/fpls.2013.00258
Tamura, K., Stecher, G., and Kumar, S. (2021). MEGA11: molecular evolutionary genetics analysis version 11. Mol. Biol. Evol. 38, 3022–3027. doi: 10.1093/molbev/msab120
Van de Sande, W. W. (2012). Phylogenetic analysis of the complete mitochondrial genome of Madurella mycetomatis confirms its taxonomic position within the order Sordariales. PLoS One 7:e38654. doi: 10.1371/journal.pone.0038654
Vaughn, J. C., Mason, M. T., Sper-Whitis, G. L., Kuhlman, P., and Palmer, J. D. (1995). Fungal origin by horizontal transfer of a plant mitochondrial group I intron in the chimeric CoxI gene of Peperomia J. Mol. Evol. 41, 563–572. doi: 10.1007/BF00175814
Wai, A., Shen, C., Carta, A., Dansen, A., Crous, P. W., and Hausner, G. (2019). Intron-encoded ribosomal proteins and N-acetyltransferases within the mitochondrial genomes of fungi: here today, gone tomorrow? Mitochondrial DNA Part A 30, 573–584. doi: 10.1080/24701394.2019.1580272
Wang, X., Wang, Y., Yao, W., Shen, J., Chen, M., Gao, M., et al. (2020). The 256 kb mitochondrial genome of Clavaria fumosa is the largest among phylum Basidiomycota and is rich in introns and intronic ORFs. IMA Fungus. 11:1–14. doi: 10.1186/s43008-020-00047-7
Wang, X. C., Zeng, Z. Q., and Zhuang, W. Y. (2017). The complete mitochondrial genome of the important mycoparasite Clonostachys rosea (Hypocreales, Ascomycota). Mitochondrial DNA Part B 2, 180–181. doi: 10.1080/23802359.2017.1303344
Wiens, J. J., Kuczynski, C. A., and Stephens, P. R. (2010). Discordant mitochondrial and nuclear gene phylogenies in emydid turtles: implications for speciation and conservation. Biol. J. Linn. Soc. 99, 445–461. doi: 10.1111/j.1095-8312.2009.01342-x
Xie, B. B., Qin, Q. L., Shi, M., Chen, L. L., Shu, Y. L., Luo, Y., et al. (2014). Comparative genomics provide insights into evolution of Trichoderma nutrition style. Genome Biol. Evol. 6, 379–390. doi: 10.1093/gbe/evu018
Keywords: biocontrol agent, mitochondrial, genome, next generation sequencing, phylogenetic relationships
Citation: Castrillo ML, Bich GÁ, Amerio NS, Barengo MP, Zapata PD, Saparrat MCN and Villalba LL (2023) Trichoderma koningiopsis (Hypocreaceae) has the smallest mitogenome of the genus Trichoderma. Front. Microbiol. 14:1141087. doi: 10.3389/fmicb.2023.1141087
Edited by:
Anne D. Van Diepeningen, Wageningen Plant Research, Wageningen University and Research, NetherlandsReviewed by:
Kamil Steczkiewicz, Polish Academy of Sciences, PolandVassili N. Kouvelis, National and Kapodistrian University of Athens, Greece
Copyright © 2023 Castrillo, Bich, Amerio, Barengo, Zapata, Saparrat and Villalba. This is an open-access article distributed under the terms of the Creative Commons Attribution License (CC BY). The use, distribution or reproduction in other forums is permitted, provided the original author(s) and the copyright owner(s) are credited and that the original publication in this journal is cited, in accordance with accepted academic practice. No use, distribution or reproduction is permitted which does not comply with these terms.
*Correspondence: María Lorena Castrillo, bWxjXzgyN0Bob3RtYWlsLmNvbQ==
†These authors have contributed equally to this work