- 1Laboratory of Medical Microbiology, Vaccine and Infectious Diseases Institute, University of Antwerp, Antwerp, Belgium
- 2Veterinary Epidemiology Unit, Department of Reproduction, Obstetrics and Herd Health, Faculty of Veterinary Medicine, Ghent University, Merelbeke, Belgium
- 3HIV/STI Unit, Department of Clinical Sciences, Institute of Tropical Medicine, Antwerp, Belgium
- 4Hospital Outbreak Support Team-HOST, ZNA Middelheim, Antwerp, Belgium
- 5Hospital Outbreak Support Team-HOST, GZA Ziekenhuizen, Wilrijk, Belgium
- 6BioMérieux SA, Sint-Martens-Latem, Belgium
- 7Department of Infection Control, Amphia Hospital, Breda, Netherlands
- 8Julius Center for Health Sciences and Primary Care, UMC Utrecht, University of Utrecht, CG Utrecht, Netherlands
- 9Amphia Academy Infectious Disease Foundation, Amphia Hospital, CK Breda, Netherlands
- 10Microvida Laboratory for Microbiology, Amphia Hospital, Breda, Netherlands
Background: The increasing number of infections caused by Escherichia coli resistant to clinically important antibiotics is a global concern for human and animal health. High overall levels of extended-spectrum beta-lactamase (ESBL)-producing and ciprofloxacin-resistant (ciproR) Escherichia coli in livestock are reported in Belgium. This cross-sectional study aimed to genotypically characterize and trace ESBL-and ciproR-E. coli of Belgian food-producing animals.
Methods: A total of 798 fecal samples were collected in a stratified-random sampling design from Belgian broilers and sows. Consequently, 77 ESBL-E. coli and 84 ciproR-E. coli were sequenced using Illumina MiSeq. Minimum inhibitory concentration (MIC) for fluoroquinolones and cephalosporins were determined. Molecular in silico typing, resistance and virulence gene determination, and plasmid identification was performed. Scaffolds harboring ESBL or plasmid-mediated quinolone resistance (PMQR) genes were analyzed to detect mobile genetic elements (MGEs) and plasmid origins. Core genome allelic distances were used to determine genetic relationships among isolates.
Results: A variety of E. coli sequence types (ST) (n = 63), resistance genes and virulence profiles was detected. ST10 was the most frequently encountered ST (8.1%, n = 13). The pandemic multidrug-resistant clone ST131 was not detected. Most farms harbored more than one ESBL type, with blaCTX-M-1 (41.6% of ESBL-E. coli) being the most prevalent and blaCTX M-15 (n = 3) being the least prevalent. PMQR genes (15.5%, n = 13) played a limited role in the occurrence of ciproR-E. coli. More importantly, sequential acquisition of mutations in quinolone resistance-determining regions (QRDR) of gyrA and parC led to increasing MICs for fluoroquinolones. GyrA S83L, D87N and ParC S80I mutations were strongly associated with high-level fluoroquinolone resistance. Genetically related isolates identified within the farms or among different farms highlight transmission of resistant E. coli or the presence of a common reservoir. IncI1-I(alpha) replicon type plasmids carried different ESBL genes (blaCTX-M-1, blaCTX-M-32 and blaTEM-52C). In addition, the detection of plasmid replicons with associated insertion sequence (IS) elements and ESBL/PMQR genes in different farms and among several STs (e.g., IncI1-I(alpha)/IncX3) underline that plasmid transmission could be another important contributor to transmission of resistance in these farms.
Conclusion: Our findings reveal a multifaceted narrative of transmission pathways. These findings could be relevant in understanding and battling the problem of antibiotic resistance in farms.
1. Introduction
Escherichia coli remains one of the most important pathogens for humans (Murray et al., 2022), as evidenced by its contribution to mortalities due to drug resistance. Fluoroquinolones and beta-lactam antibiotics are life savers in both human (World Health Organization Advisory Group on Integrated Surveillance of Antimicrobial Resistance, 2018) and animal healthcare (World Organization for Animal Health (OIE), 2018): these medications are essential for treating severe illnesses. Resistance to extended-spectrum cephalosporins and fluoroquinolones constitutes a major public health problem because this limits the treatment options for serious bacterial infections (World Health Organization Advisory Group on Integrated Surveillance of Antimicrobial Resistance, 2018) and drives the use of the last resort of antibiotic therapy, i.e., carbapenems. The gastrointestinal tract of animals serves as a reservoir of antimicrobial resistance (AMR), which can spread via MGEs (Moor et al., 2021). The presence of resistance genes on MGEs enables their dispersion, posing a great hazard to food safety (Partridge et al., 2018). Clinically significant ESBL genes, belonging to the blaCTX-M, blaTEM and blaSHV gene families, can successfully disseminate because they are commonly located on plasmids (IncA/C, IncF, IncHI1, IncHI2 IncI, IncK, IncN, IncX plasmids) (Rozwandowicz et al., 2018). In addition, three mechanisms of plasmid-mediated quinolone resistance (PMQR) are known: protection of DNA gyrase and topoisomerase IV from quinolone inhibition by qnr genes (ColE plasmids) (Tran et al., 2005), acetylation of quinolones by aminoglycoside acetyltransferase Aac(6′)-Ib-cr (Robicsek et al., 2006) and quinolone accumulation due to quinolone efflux pumps QepAB (Yamane et al., 2007) and OqxAB (ColE plasmids, IncX plasmids) (Hansen et al., 2007; Jacoby et al., 2015; Rozwandowicz et al., 2018). These mechanisms provide low-level resistance (ciprofloxacin MIC range: 0.06–0.25 mg/l); however, they are usually present on multidrug-resistant (MDR) plasmids and facilitate the selection of higher-level resistance making infections with PMQR-carrying pathogens harder to treat (Jacoby et al., 2015). Quinolone resistance in Gram-negative bacteria can also be caused by single amino acid changes in QRDRs in DNA gyrase (gyrA) and DNA topoisomerase IV (parC) (Karczmarczyk et al., 2011; Gordon and George, 2015). Another mechanism contributing to (fluoro)quinolone resistance is the increased expression of the AcrAB-TolC efflux pump which is regulated by repressor AcrR and other regulators of drug efflux MarAR and SoxRS as well as RNA polymerase RpoB (Amábile-cuevas and Demple, 1991; White et al., 1997; Oethinger et al., 1998; Lindgren et al., 2003; Pietsch et al., 2017) and the AcrB component of the efflux pump itself (White et al., 1997; Blair et al., 2015).
A previous study indicated a high occurrence of ESBL-producing and ciprofloxacin-resistant E. coli in fecal samples of broilers and pigs in Belgian farms (De Koster et al., 2021). Possible explanations for these observations include the dissemination of resistant E. coli vertically along the production chain from one generation to another (Dierikx et al., 2013; Zurfluh et al., 2014) and resistant E. coli residing in the farm environment (Blaak et al., 2015) along with the dissemination of resistant E. coli or their resistance genes between farm animals (Hayer et al., 2020). However, the research into the genetic diversity and antibiotic resistance of E. coli that colonize livestock in Belgian farms has been limited. Most studies of commensal E. coli in livestock, such as the Belgian Antimicrobial Consumption and Resistance in Animals (AMCRA) reports (FAVV-AFSCA, 2021), the European Food Safety Authority (EFSA) and European Centre for Disease Prevention and Control (ECDC) reports (European Food Safety Authority and European Centre for Disease Prevention and Control, 2021) rely on phenotypic AMR profiles. The lack of whole genome sequencing (WGS) to track MDR and high-risk clones was acknowledged in the latest BELMAP report, which aims to summarize monitoring programs in Belgium and recommends improving monitoring (FOD Volksgezondheid Veiligheid van de Voedselketen en Leefmilieu et al., 2021). An interdisciplinary One Health strategy is essential for tracking AMR’s spread between humans, animals and their shared environment. Data on E. coli found in food-producing animals should be utilized to identify potential pathways of transmission through which the risk may reach human populations through consumption. To investigate the molecular epidemiology of ESBL-E. coli and ciproR-E. coli, we used WGS to identify resistance genes, mutations and potential transmission pathways between and among farms.
2. Materials and methods
2.1. Setting, study period and sample/isolate collection
Within the framework of the i-4-1-Health project, a total of 798 fecal samples were collected in a stratified-random sampling design from conventional broiler (n = 15) and multiplier sow farms (n = 15) in Flanders, Belgium (September 2017–April 2018). When present, sampling was conducted in different units (broiler houses or rooms with weaned pigs) with a maximum of three units per farm. The farms were included based on the relative level of antibiotic use, meaning that antibiotic use was higher than average compared to the national benchmark value in the respective countries. Farm characteristics and antibiotic use were described previously (Caekebeke et al., 2020).
2.2. ESBL-producing and ciprofloxacin-resistant Escherichia coli
Isolation of ESBL- and ciproR-E. coli was performed as described by Kluytmans-van den Bergh et al. (2019). A total of 724 ESBL-E. coli and 467 ciproR-E. coli were isolated from the fecal samples. To investigate the molecular epidemiology, three ESBL-E. coli and three ciproR-E. coli from each farm were chosen for in-depth analysis including phenotypic characterization and whole genome sequencing. In particular, the first ESBL-E. coli and ciproR-E. coli isolated from each farm unit were selected. In farms with one sampled unit, three ESBL-E. coli and ciproR-E. coli with a distinct antibiotic susceptibility profile were selected from that unit. Using these selection criteria, 82 ESBL-E. coli [broiler (n = 45), pig (n = 37)] and 84 ciproR-E. coli [broiler (n = 45), pig (n = 39)] were selected for MIC determination and whole genome sequencing.
2.3. Whole genome sequencing
A single colony was inoculated in 4 ml Mueller Hinton broth and incubated overnight at 35–37°C. The MasterPure Complete DNA & RNA Purification kit (Epicentre, Madison, WI, USA) was used to extract genomic DNA. Libraries were prepared using the Nextera XT sample preparation kit (Illumina, San Diego, CA, USA) and sequenced with 2× 250 bp paired-end sequencing using the Illumina MiSeq platform (Illumina, San Diego, CA, USA). The sequencing data were submitted to NCBI under BioProject PRJNA905236. Supplementary Table 1 provides an overview of ESBL-E. coli and ciproR-E. coli sequences and their genetic characteristics used in this study.
2.4. De novo assembly, genotyping and phylogenetic analysis
Sequences were trimmed with TrimGalore v.0.4.41 and assembled de novo using SPAdes v.3.13.0 (Bankevich et al., 2012). Assembly quality was assessed with Quast (Gurevich et al., 2013). The assembled genome was annotated using Prokka v.1.12 (Seemann, 2014). Additional analysis was performed using BacPipe v1.2.6 (Xavier et al., 2020) including the PubMLST database (Achtman scheme) (Jolley et al., 2018), the CARD database (McArthur et al., 2013), ResFinder v4.1 (Bortolaia et al., 2020), VirulenceFinder v2.0.3 (Tetzschner and Lund, 2020) and PlasmidFinder v2.0 (Carattoli and Hasman, 2020). Serotype and pathotype were determined using BioNumerics v7.6.3 (Applied Maths NV, Sint-Martens-Latem, Belgium). The identification of pathotypes was performed according to the virulence factor database (VFDB) (Chen et al., 2016). In silico prediction of fimH type and H and O serotypes was performed using FimTyper 1.0 (Roer et al., 2017) and SeroTypeFinder (Joensen et al., 2015), respectively. Phylogroups were determined using ClermonTyping (Beghain et al., 2018). For core genome multilocus sequence typing (cgMLST), a gene-by-gene approach was employed by generating a study-specific scheme and analyzing allelic loci distances of cgMLST using ChewBBACA (Silva et al., 2018) and visualizing the tree using iTOL v6 (Letunic and Bork, 2021).
2.5. Phenotypic and genotypic antibiotic resistance determination
ESBL production was phenotypically confirmed using the combination disk diffusion method. Ciprofloxacin resistance was confirmed by ciprofloxacin MIC determination using VITEK® MS system (bioMérieux, Marcy l’Etoile, France). In addition, MICs for amoxicillin-clavulanic acid, ampicillin, cefuroxim, cefotaxime, ceftazidime, cefoxitin, fosfomycin, gentamicin, imipenem, meropenem, nitrofurantoin, piperacillin-tazobactam, tobramycin, trimethoprim were determined using VITEK® MS system (bioMérieux, Marcy l’Etoile, France). Furthermore, ciprofloxacin, enrofloxacin, levofloxacin and moxifloxacin were tested for 106 E. coli of which 18 were ciprofloxacin-susceptible E. coli and 88 were ciprofloxacin non-susceptible E. coli using E-tests (bioMérieux, Marcy l’Etoile, France) to identify genome-wide associations between genetic markers and fluoroquinolone resistance levels. Results were interpreted using the EUCAST breakpoint tables v12.0 (The European Committee on Antimicrobial Susceptibility Testing, 2022) and an enrofloxacin breakpoint of MIC≤0.25 mg/l (Hao et al., 2013). After sequencing, known ESBL genes could not be detected in five phenotypic ESBL-E. coli (5/82, 6%) (from broiler farms one, four and eight and pig farms three and fifteen); therefore, these isolates were excluded, resulting in 77 ESBL-E. coli for further analysis. QRDRs were investigated for mutations conferring resistance within gyrase gyrA and gyrB and topoisomerases IV parC and parE. In addition, mutations in acrB, acrR, marA, marR, rpoB, soxR, soxS were considered. Mutations and predicted amino acid changes were aligned using clustalw, inbuilt within the CLC genomics workbench v.9.5.3 (CLC bio, Denmark). Prediction of whether amino acid changes affect protein function was performed by Sorting Intolerant From Tolerant (SIFT) (Ng and Henikoff, 2003). Scaffolds containing ESBL or PMQR genes were analyzed using MGEFinder v1.0.3 (Durrant et al., 2020), and ISFinder (Siguier et al., 2006) to detect MGEs and replicon types of plasmids. Scaffolds containing ESBL genes or PMQR represent plasmid sequences were analyzed further on NCBI using blastn search with default settings to the blast database v5. Resistance genes were classified as Rank I (human-associated, mobile ARGs, in ESKAPE pathogens, current threats) or Rank II (human-associated, mobile ARGs emerging from non-pathogens, future threats) (Zhang et al., 2021; Supplementary Table 2).
2.6. Statistical tests and visualization
Statistical tests and visualization of the presence of resistance genes, virulence genes and plasmids were performed using R version 4.2.0 (R Core Team, 2020). Differences in the presence of genes were tested using a One-way ANOVA and TukeyHSD test in case of equal variances or a Welch ANOVA and the Games-Howell test in case of unequal variances (mean ± standard deviation and p-values are shown). Associations of genetic markers with a phenotype were examined using phi and chi-squared test.
3. Results
3.1. ESBL and PMQR genes in ESBL-producing and ciprofloxacin-resistant Escherichia coli
The most abundant ESBL genes detected in E. coli isolated from broilers were blaCTX-M-1 (40.5%, n = 17) followed by blaSHV-12 (31.0%, n = 13). Other ESBL genes detected in broiler isolates were blaCTX-M-32 (2.4%), blaCTX-M-55 (2.4%), blaSHV-2 (2.4%), blaTEM-15 (2.4%), blaTEM-52B (4.8%) and blaTEM-52C (7.1%). Three isolates (7.1%) from different broiler farms harbored blaCTX-M-1 and blaSHV-12. BlaCTX-M-1 was also the most common in E. coli from pigs (34.3%, n = 12), followed by blaCTX-M-32 (22.9%), blaTEM-52C (11.4%), blaCTX-M-3 (8.6%), blaCTX-M-14 (8.6%), blaCTX-M-15 (5.7%), blaSHV-2 (5.7%), blaTEM-52B (2.9%) in pig isolates (Figure 1A). Eight of the ciproR-E. coli also harbored blaCTX-M-1 (n = 2), blaCTX-M-32 (n = 2), blaCTX-M-15 (n = 1), blaSHV-12 (n = 2) and one isolate with both blaCTX-M-1 and blaSHV-12. PMQR genes were found in a relatively low number of ciproR-isolates (14.3%, n = 12) (Figure 1B). Of the 84 ciproR-E. coli, 12 isolates harbored qnrS1 (8.9% of the broiler isolates and 15.4% of the pig isolates). Two pig isolates (5.1%) additionally contained the efflux pump OqxAB. A total of 9.5% of the ESBL-E. coli from broilers and 8.6% of the ESBL isolates from pigs harbored qnrS1. Also, qnrB19 was detected in 5.7% of porcine ESBL-E. coli.
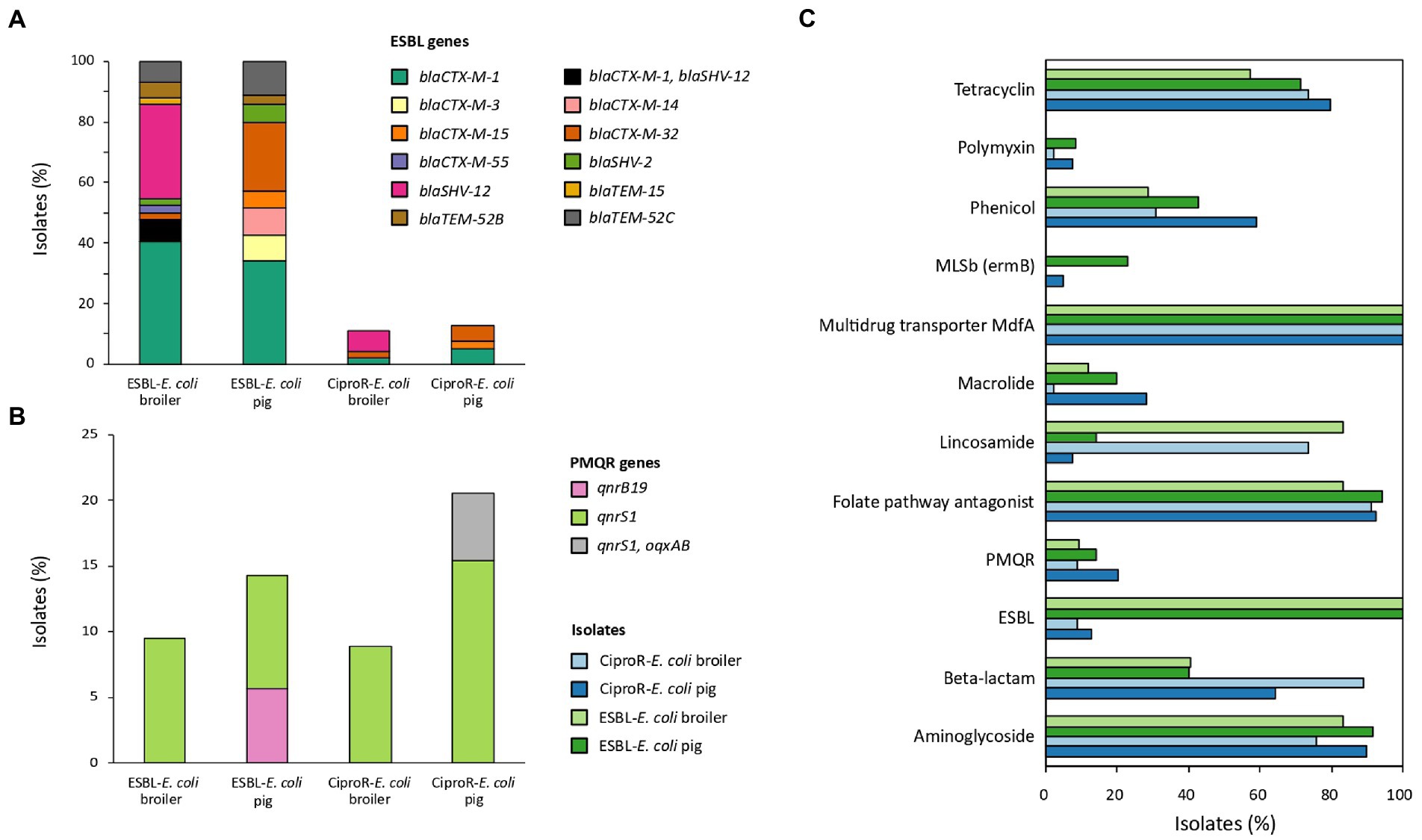
Figure 1. The percentage of isolates carrying ESBL genes (A), plasmid-mediated quinolone resistance (PMQR) genes (B) and genes conferring resistance to other antibiotic classes (C).
3.2. Other resistance genes, virulence genes and plasmids
In total, 95.8% of the isolates were MDR (i.e., resistant to at least 3 antibiotic classes (Magiorakos et al., 2012)). Genes conferring resistance to aminoglycosides were abundant (overall in 84.5% of the isolates), folate pathway antagonists were present in 90.1% of the isolates, and all isolates harbored multidrug transporter MdfA. Lincosamide resistance was often detected in broiler isolates (ciproR-E. coli: 73.3%, ESBL-E. coli 83.3%) and beta-lactam resistance was often detected in ciproR-E. coli (pig: 64.1%, broiler: 88.9%) (Figure 1C). Plasmid-mediated colistin resistance was found in three pig farms [mcr-1.1 (n = 1), mcr-2.1 (n = 2), mcr-9 (n = 1)] and in one broiler farm [mcr-9 (n = 1)]. Both mcr-9- containing isolates did not have the complete qseC-qseB two-component system to induce colistin resistance. Highly diverse resistance gene profiles (131 different profiles among 161 isolates) were detected within the same farm and between farms.
The mean number of resistance genes was significantly higher (p < 0.05) in ciproR-E. coli from pigs (9.44 ± 4.01) compared to ciproR-E. coli from broilers (7.51 ± 2.85) (Figure 2A). Resistance genes that are a current threat to public health, referred to as Rank I resistance genes, were more abundantly present in ciproR-E. coli compared to ESBL-E. coli and more in pig isolates (4.6 ± 2.4 Rank I resistance genes) compared to broiler isolates (2.8 ± 1.4 Rank I resistance genes) (p < 0.01) (Figure 2B). Similar observations can be made for Rank II resistance genes (considered future threats) which were present in higher numbers in porcine ciproR-E. coli compared to ESBL-E. coli from both broilers and pigs (p < 0.05) (Figure 2C). On the other hand, broiler isolates contain a higher number of virulence genes (ciproR-E. coli: 4.62 ± 2.23; ESBL-E. coli: 5.45 ± 2.60) compared to pig isolates (ciproR-E. coli: 3.10 ± 2.25; ESBL-E. coli: 3.97 ± 2.81) (Figure 2D). This divergence of resistance and virulence was observed in the higher number of virulence genes (up to 12 genes) and lower number of Rank I resistance genes in ESBL-E. coli, while the opposite was seen for most ciproR-E. coli, which can carry a higher number of Rank I resistance genes (up to 10 Rank I resistance genes) (Supplementary Figure 1). Fourteen isolates showed a convergence of virulence and resistance (at least 3 Rank I resistance genes and more than six virulence genes) which belonged to ST117, ST189 (n = 2), ST648, ST88, ST1011, ST75, ST624, ST115 (n = 3), ST48 and ST350 (n = 2). Overall, a large diversity was seen in the number of virulence and Rank I resistance genes ranging from lower-risk (one resistance gene and one virulence gene) to high-risk isolates (five Rank I resistance genes and nine virulence genes) (Supplementary Figure 1). On average, four plasmids were detected per isolate and no significant differences in the number of plasmids between the isolates of different origins were detected (Figure 2E). The most common replicon markers (>10% in one or more categories) were IncFIB (52.9%), IncI1-I (gamma) (38.2%), Col (MG828) (30.1%), IncFII (27.7%), IncX1 (25.6%), IncFIC(FII) (23.6%) and p0111 (18.9%). Plasmid replicon IncB/O/K/Z was exclusively detected in broiler isolates (in 23.0% of ciproR-E. coli and in 28.9% of ESBL-E. coli) (Supplementary Figure 2). Most virulence genes were involved in adherence and invasion (Supplementary Figure 3). The most prevalent virulence genes were iss (75%), gad (57%), lpfA (37%) and iroN (37%). A total of 120 different virulence profiles were detected within farms.
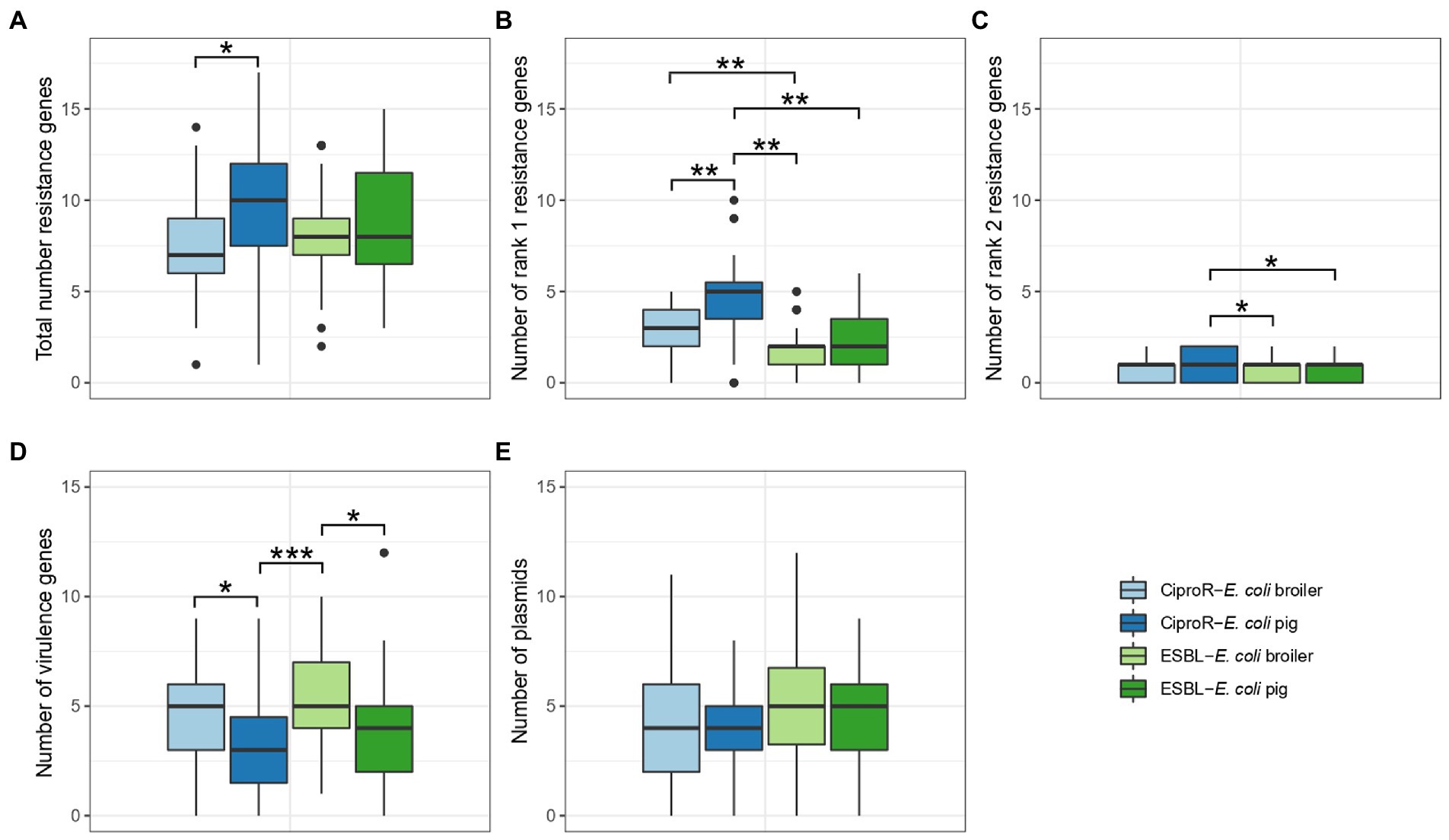
Figure 2. Number of resistance genes (A–C), virulence genes (D) and plasmids (E) in ESBL-Escherichia coli and CiproR-E. coli isolated from broilers and pigs. Statistically significant differences are indicated according to the level of significance: * (p < 0.05), ** (p < 0.01), *** (p < 0.001) (ANOVA with TukeyHSD or Games-Howell post-hoc tests).
3.3. Genotype–phenotype correlations for resistance in ESBL-Escherichia coli and ciproR-Escherichia coli
More than one type of ESBL gene was detected in most of the sampled farms (73.3%; 22/30 farms). All ESBL genes were associated with very high ampicillin (MIC ≥32 mg/l) and cefotaxime (MIC 8 to ≥64 mg/l) resistance levels (p < 0.001), except for two blaSHV-2-harboring porcine isolates which showed cefotaxime MICs below breakpoint (MIC ≤1 mg/l). Strong levels of agreement between ESBL genotype and phenotype were detected for cefuroxime (89.44%, phi coefficient: 0.76), and ceftazidime (86.96%, phi coefficient: 0.77) and an almost perfect level of agreement was detected for cefotaxime (98.14%, phi coefficient: 0.96) (Table 1).
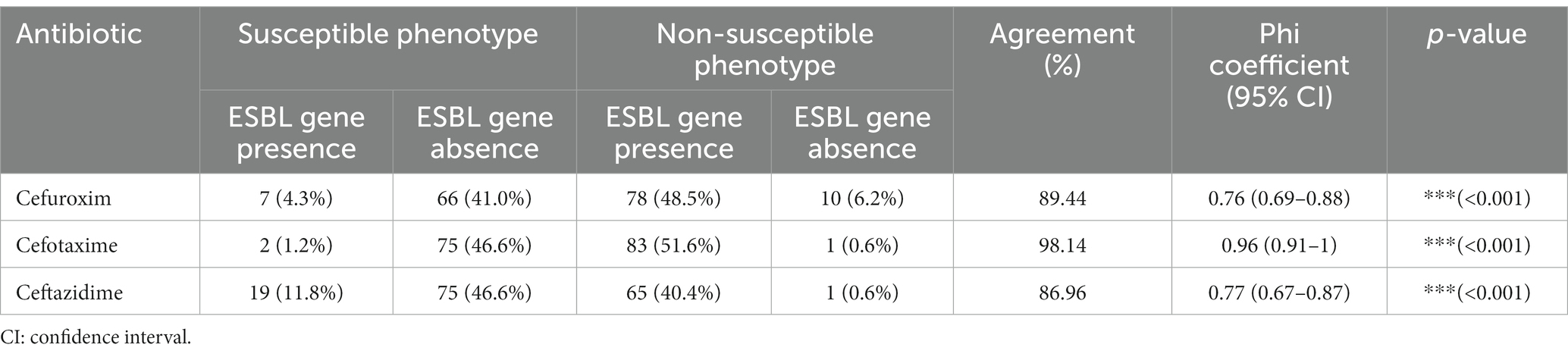
Table 1. Concordance between ESBL genotypes and cephalosporin phenotypes in Escherichia coli isolates from livestock.
Mutations in QRDR of gyrA and parC were found in all ciproR-E. coli. Sequential acquisition of individual mutations in QRDR of gyrA and parC led to increasing MICs for all tested fluoroquinolone antibiotics. Predicted amino acid change S83L in GyrA caused low-level resistance to enrofloxacin and moxifloxacin, but not to ciprofloxacin and levofloxacin. Triple or quadruple mutations in QRDR caused high-level fluoroquinolone resistance (MIC>4 mg/l). QnrS1 or QnrB19 alone leads to low-level resistance to enrofloxacin and moxifloxacin and a sensitive/intermediate phenotype for ciprofloxacin and levofloxacin. The presence of both oqxAB and qnrS1 genes lead to a non-susceptible phenotype for all four fluoroquinolones (Figure 3).
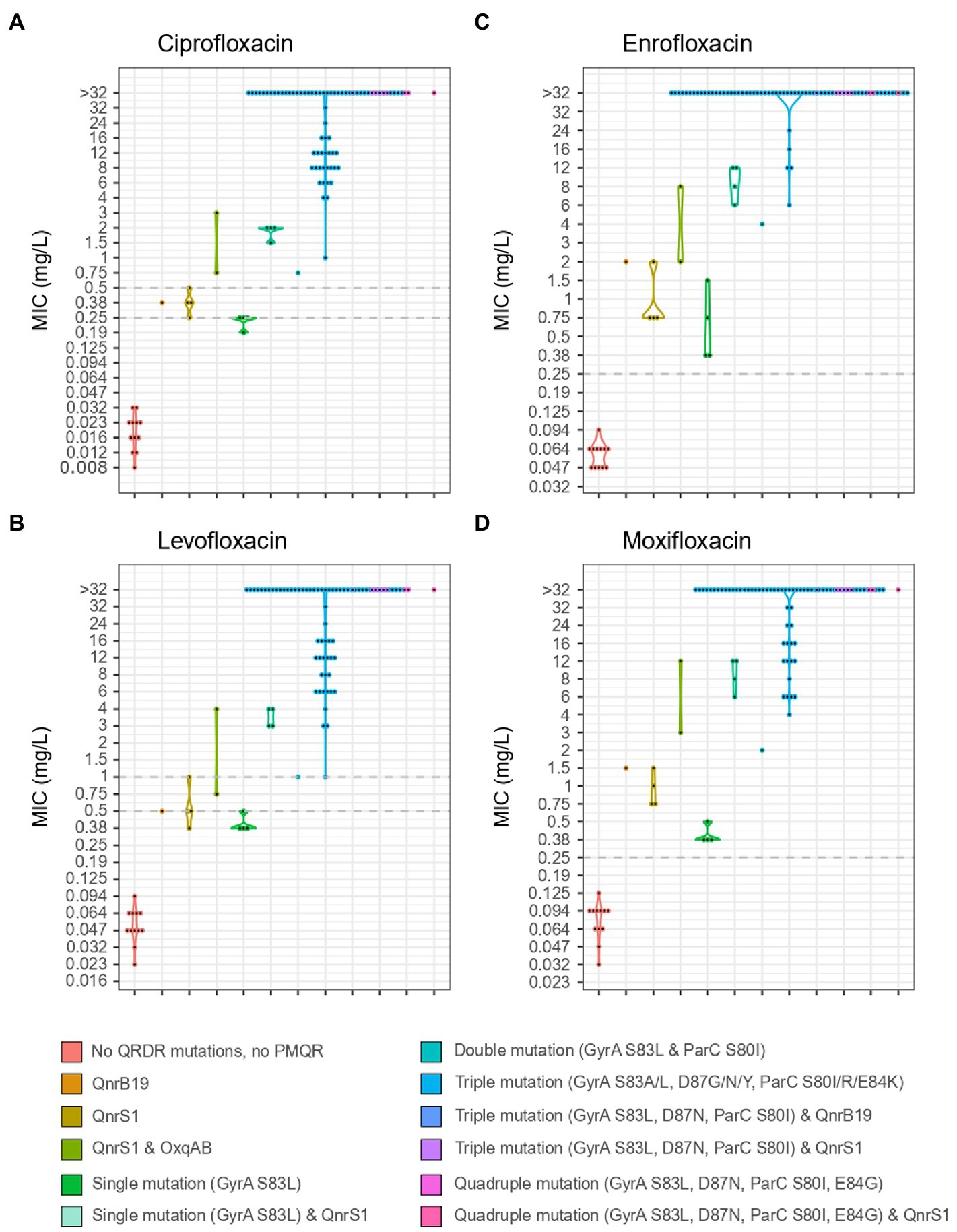
Figure 3. MIC values for ciprofloxacin (A), levofloxacin (B), enrofloxacin (C) and moxifloxacin (D) of 106 isolates from Belgian broilers and pigs in association with the mutations in QRDR regions of GyrA and ParC and the presence of PMQR genes. EUCAST breakpoints are indicated with a horizontal, dotted grey line.
GyrA S83L, D87N and ParC S80I were strongly and significantly associated with resistance to fluoroquinolones. Triple mutations in gyrA (S83L and D87N/Y/G) and parC (S80I/R or E84K) were detected in 88% of the ciproR-E. coli and confer resistance to all tested fluoroquinolones. Two isolates contained a fourth mutation (GyrA S83L and D87N, ParC S80I and E84G) and one isolate additionally contained the qnrS1 gene that showed MIC>32 mg/l for all fluoroquinolones. Outside of the QRDR in gyrA and parC, other mutations were detected in gyrA, parC, gyrB, parE, acrB, acrR, marR, rpoB, soxR and soxS, yet, were not positively associated with fluoroquinolone resistance (Figure 4). No mutations were detected in marA.
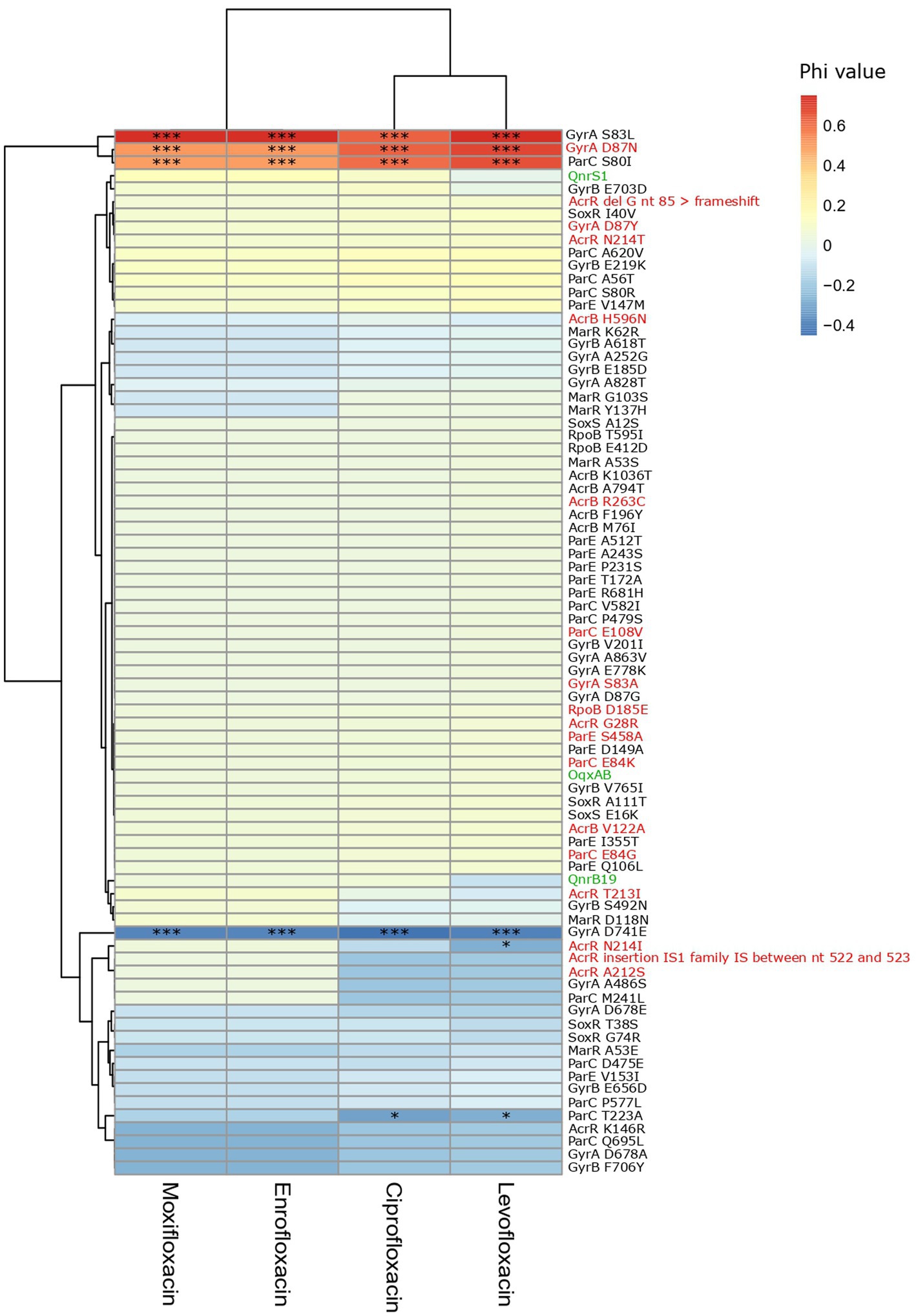
Figure 4. Heatmap of the association between the presence of PMQR genes and mutations and fluoroquinolone non-susceptibility. Colors represent the phi values. Negative phi values represent negative associations, positive values represent positive associations between the genes/mutations and the non-susceptibility to the fluoroquinolone antibiotics. PMQR genes are indicated in green, predicted amino acid changes that are likely deleterious for the protein function according to SIFT are indicated in red. *(p < 0.05), *** (p < 0.001) (Chi-squared test). IS: insertion sequence, nt: nucleotide.
3.4. Genetic context of ESBL genes and PMQR in ESBL-producing and ciprofloxacin-resistant Escherichia coli
The specific genetic context of ESBL and PMQR genes (closest MGE and, if possible, identification of plasmid origin or replication) could be identified for 66 isolates (Figure 5). MGEs tended to be present at a fixed distance from the resistance gene. ESBL gene blaCTX-M-1 was commonly found in association with ISEcp1 upstream of the gene (n = 30) and was always detected on plasmids (Figure 5A). The plasmid IncI1-I(alpha) could be detected in 12 blaCTX-M-1-producing strains and, using pMLST, six of the IncI1-I(alpha) plasmids showed ST3, clonal complex 3. Evidently, this particular MGE circulates in six pig and 13 broiler farms amongst various E. coli genotypes, showcasing the remarkable distribution reach of this blaCTX-M-1 harboring plasmid (Figure 5B). Other resistance genes detected on a subset of the blaCTX-M-1-containing sequences are: aadA5 (n = 2), dfrA17 (n = 2), mdtG (n = 1), mdtH (n = 1), mexA (n = 1), mexB (n = 1), qnrS1 (n = 1), sul2 (n = 6) and tetA (n = 1), as well as virulence gene cib (n = 10). One porcine isolate harbored blaCTX-M-1 associated with IS5 on an IncI1-I(alpha), ST3, CC3 plasmid. The IncI1-I(alpha) plasmid origin of replication could also be detected in association with other ESBL genes, such as blaTEM-52C (n = 3) and blaCTX-M-32 (n = 1). The blaSHV-12 gene was detected on an IncN plasmid, without any association of IS elements in four broiler isolates from four different farms or in association with IS26 137 bp upstream of the blaSHV-12 gene on an IncB/O/K/Z plasmid in two isolates from a broiler farm. A composite transposon IS26 surrounded the blaSHV-2 gene in isolates (n = 2) from a pig farm. Most ESBL genes were located on a plasmid. However, seven ESBL genes [blaCTX-M-3 (n = 3) associated with ISEcp1, blaCTX-M-14 associated with IS903 (n = 1), blaCTX-M-15 (n = 1) and blaCTX-M-32 (n = 2)] were predicted to be located on the chromosome. Different IS elements/transposons flanked the blaCTX-M-32 gene (upstream ISKpn26 (n = 2) on an IncX plasmid (n = 1) or downstream ISSbo1 on an IncI1-I(alpha) plasmid (n = 1) or upstream ISVas3 (n = 1)) and the blaTEM-52C [upstream ISSbo1 (n = 2), upstream Tn2 (n = 1), downstream ISRor2 (n = 2)] in different isolates. The blaTEM-52B gene was flanked by Tn2 in one porcine isolate and was located on an IncX1 plasmid. Co-localization of QnrS1 with blaCTX-M-15 (n = 1) or blaCTX-M-55 (n = 1) on a predicted plasmid contig was detected (Supplementary Figure 4). In 14 out of 75 isolates (18.7%), co-localization of virulence factor colicin Ib (cib gene, polypeptide toxins against E. coli and closely related bacteria) with an ESBL gene was detected.
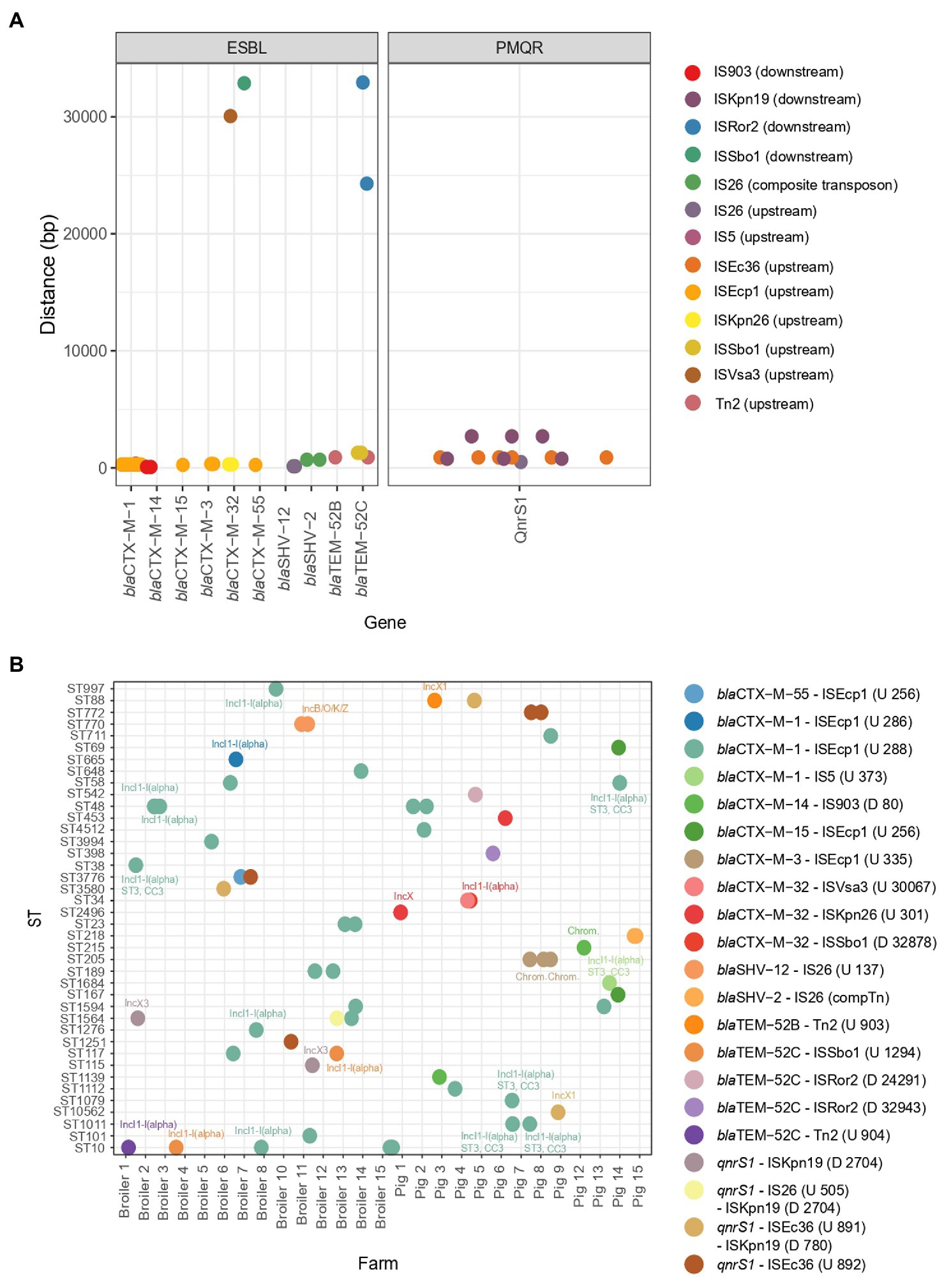
Figure 5. Mobile genetic elements and their association to ESBL genes and PMQR genes. (A) Distance of mobile genetic elements to the ESBL or PMQR gene. (B) The combination of the ESBL/PMQR gene with the closest mobile genetic element for every farm and ST element. The plasmid origins of replication are indicated in the figure. The distance and upstream (U)/downstream (D) location of the mobile genetic element are indicated in the figure legend.
The PMQR gene qnrS1 was flanked by downstream ISKnp19 (n = 6) and upstream either by ISEc36 (n = 7) or by IS26 (n = 1). For one porcine isolate, the plasmid replicon could be identified as IncX1 harbouring blaTEM-1B. For two broiler isolates from two different farms, QnrS1 could be located on an IncX3 plasmid (Figure 5B). QnrB19 was found to be located on a Col(pHAD) plasmid (n = 2); however, no IS elements flanking the gene could be identified. Also, no flanking MGEs could be identified for oqxAB genes.
3.5. Typing and possible transmission events of resistant Escherichia coli within and between farms
A highly diverse population of E. coli was isolated from broiler and pig farms (Figure 6). Overall, 63 different E. coli STs were detected with ST10 being the most abundant (13 out of 161 isolates, 8.1%). Phylogroup A was most common in ESBL-E. coli from broilers (47.6%) and pigs (57.1%) and in ciproR-E. coli from pigs (53.8%), where phylogroup B1 was most common among ciproR-E. coli from broilers (31.1%). Phylogroup A was most common among ESBL-E. coli from pigs (57.1%) and broilers (47.6%), and ciproR-E. coli from pigs (53.8%), while B1 was most common among ciproR-E. coli from broilers (31.1%). The number of virulence genes in phylogroups A and B1 was lower compared to phylogroups D and G (Supplementary Figure 5). FimH54 was the most common among ESBL-E. coli from broilers (16.7%) and pigs (40.0%) and ciproR-E. coli from pigs (41.0%), and fimH32 was most common among ciproR-E. coli from broilers (22.2%). With 85 different serotypes among 161 isolates, serotypes were widely diverse.
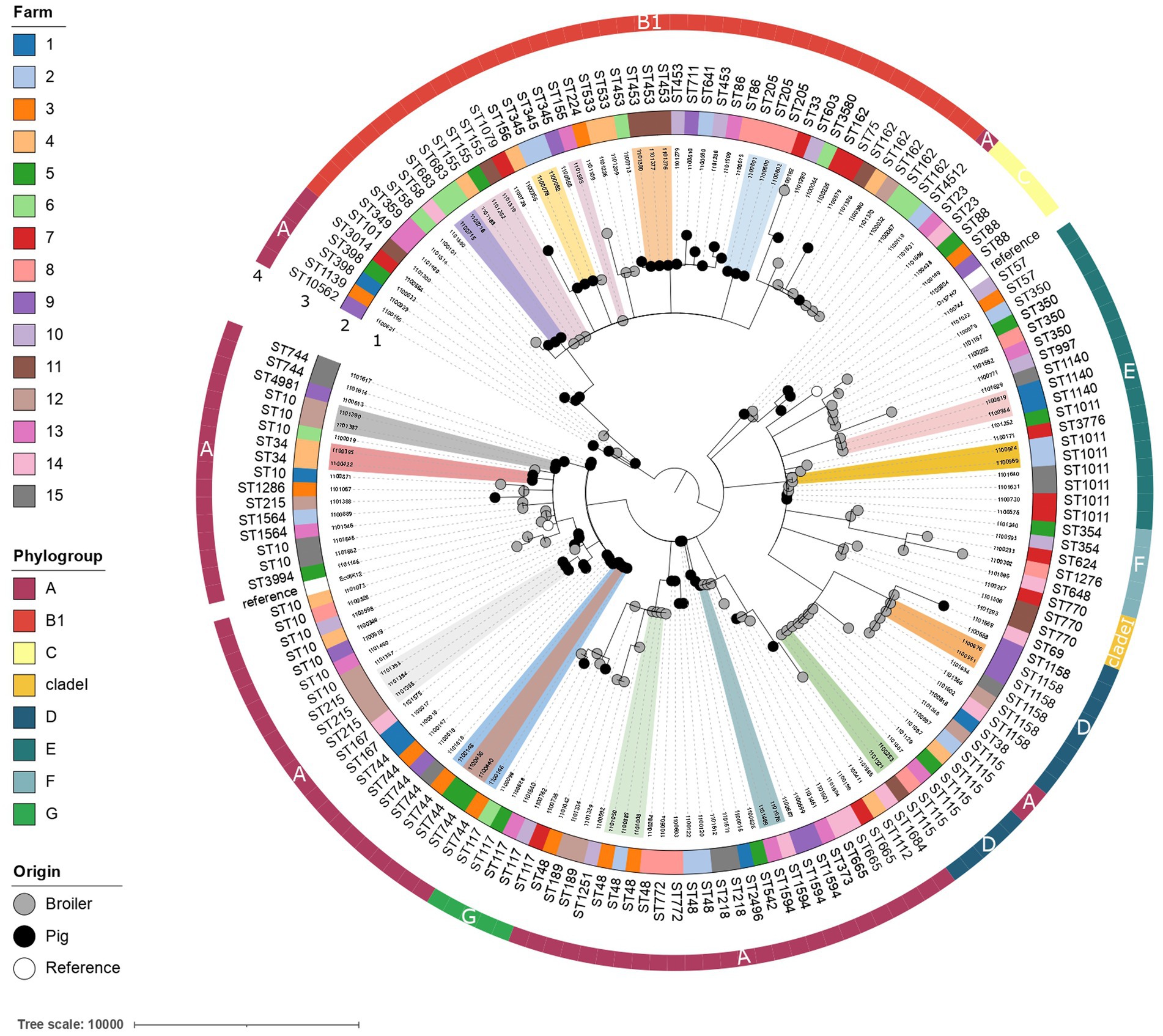
Figure 6. Phylogenetic tree of ciprofloxacin-resistant and ESBL-producing E. coli from broilers and pigs. The minimum spanning tree is distance-based and was generated by iTOL using cgMLST profile data (3,012 loci). Colored clusters indicate genetically related isolates with ≤10 allelic differences from different broilers/pigs. The isolate IDs are shown in the first ring. The farm is indicated in colored strips in the second ring. Achtmann ST and phylogroups are indicated in rings three and four, respectively. The origin of the isolate is indicated with black (pig), grey (broiler) or white (E. coli K12 and E. coli O157-H7 reference strains) nodes.
To determine the genetic relatedness of the isolates, a study specific cgMLST scheme with 3,012 loci was developed. Genetically linked bacterial clusters, with a maximal difference of 10 alleles among them (Schürch et al., 2018; Van Hoek et al., 2020), were identified on several pig (n = 8) and broiler farms (n = 3) (ST10, ST34, ST205, ST215, ST345, ST453, ST683, ST744, ST1011, ST1140, ST1158). Moreover, the presence of genetically similar resistant bacteria was detected between different broiler farms (n = 5) (ST115, ST48, ST155). These results suggest either transmission or a common reservoir between broiler farms. Transmission of E. coli ST1594 has likely occurred between a broiler farm and a pig farm as an allelic difference of 3 loci was shown between the two isolates (Figure 6).
3.6. Pathotypes detected in Belgian farm animals: ESBL-producing and ciprofloxacin-resistant ETEC and ESBL-producing EPEC
Most of the E. coli isolates were non-pathogenic. However, 12 pathogenic E. coli (7.45%) were detected in five pig farms and two broiler farms. ESBL-producing enterotoxigenic E. coli (ETEC) were detected in pig farms six (n = 2; phylogroup B1, CTX-M-32-producing) and 15 (n = 2 from the same pig; phylogroup A; SHV-2-producing) and ciprofloxacin-resistant ETEC were detected in pig farms eight (n = 2, ST772, phylogroup A, FimH54) and nine (n = 1, ST10, phylogroup A, FimH54). Enterotoxins sta and stb were present in 4 ETEC strains, sta was present in one ETEC strain and stb was present in two ETEC strains. The stb-containing contigs of the ETEC strains from pig farm 15 also contained the astA gene encoding the heat-stable enterotoxin (EAST1) and IS100, an IS21 family insertion element.
ESBL-producing enteropathogenic E. coli (EPEC) were detected in pig farm two (n = 2) and broiler farms four (n = 1) and 12 (n = 2). All EPEC strains were atypical because of the lack of bundle-forming pili (BFP). All EPEC strains were fimH54 belonging to phylogroup A; two were ST48 and CTX-M-1-producing strains, one was ST10 and TEM-52C producing strain and two were ST189 and CTX-M-1-producing strain. The latter two contained the IS256 composite transposon to mobilize the cassette of pathogenic virulence genes (eae, espA, espB, espF, astA, tir).
4. Discussion
The study showed that livestock is a reservoir for a large variety of antimicrobial resistance genes, virulence genes and plasmids. More than one type of ESBL gene was detected in most farms and E. coli belonging to a variety of STs was found in Belgian broilers and pigs.
The large collection of STs and serotypes of commensal E. coli in animals was described before (Ahmed et al., 2017; Duggett et al., 2020; Kaspersen et al., 2020; Massella et al., 2020; Zingali et al., 2020; Leekitcharoenphon et al., 2021). However, the pandemic multidrug-resistant clone ST131 commonly associated with human infections was not detected and blaCTX M-15 was rarely found [n = 3 from two pig farms (ST4981, ST69, ST167)]. Escherichia coli ST131 was also not detected in pig farms in Switzerland during a longitudinal study (Moor et al., 2021). The spread of blaCTX-M-15 in human-associated E. coli is globally linked to IncFII plasmids in ST131 (Rozwandowicz et al., 2018). IncFII plasmids were commonly detected (27.7% of the isolates) in this study but could not be linked to blaCTX-M-15 or ST131. Instead, CTX-M-1 predominates in E. coli from food-producing animals and food in Europe (Ceccarelli et al., 2019; Duggett et al., 2020). We found that the most common ESBL genes were blaCTX-M-1 and blaSHV-12 and ST10 was the most abundant sequence type. This is in line with other reports (Smet et al., 2008; Ahmed et al., 2017; Reid et al., 2017; van Damme et al., 2017; Ceccarelli et al., 2019; Stubberfield et al., 2019; Duggett et al., 2020; Zingali et al., 2020; Leekitcharoenphon et al., 2021; Moor et al., 2021). ST10 has been found in both humans, animals, retail meat and the environment (Oteo et al., 2009; Leverstein-van Hall et al., 2011; Toval et al., 2014; Manges et al., 2015; Reid et al., 2017), is associated with ESBL production (Oteo et al., 2009; Moor et al., 2021), and has been reported as an emerging extra-intestinal pathogen in humans, pigs and broilers (Riley, 2014; Manges and Johnson, 2015; Bojesen et al., 2022). The results from our study combined with published data confirm that ST10 is a potential dominant clonal group of commensal E. coli in food-producing animals globally. Other high-risk lineages (ST69, ST117, ST23, ST58, ST648, ST744) of E. coli were identified among our isolates. A total of 12 (7.45%) pathogenic E. coli strains were detected (ETEC and atypical EPEC), one ST10 TEM-52C-producing strain and two ST189 CTX-M-1-producing strains which contained an IS256 composite transposon to mobilize the cassette of pathogenic virulence genes (eae, espA, espB, espF, astA, tir). These composite transposons can move as a single unit to move these pathogenic virulence genes and disseminate them among bacteria.
The spread of ESBL genes is highly linked to epidemic and highly transmissible plasmids (Rozwandowicz et al., 2018; Kurittu et al., 2021). Most ESBL genes were predicted to be located on plasmids (91%) and were in the proximity of an IS element or transposon that was usually located at a fixed distance from the ESBL gene. The blaCTX-M-1 gene was often associated with ISEcp1 and IncI1-I(alpha)-ST3 in several broiler and pig farms, as described before (de Been et al., 2014; Zurfluh et al., 2014; Partridge et al., 2018; Rozwandowicz et al., 2018; Ceccarelli et al., 2019; Bernreiter-hofer et al., 2021). ISEcp1 is known to be associated with ESBL genes. Genes downstream of this IS element can be mobilized through transposition (including chromosomal integration) and are able to enhance ESBL gene expression under its own promotor (Poirel et al., 2003; Ceccarelli et al., 2019; Massella et al., 2020). In our study, the IncI1-I(alpha) plasmid was also found to carry other ESBL genes (blaCTX-M-32 and blaTEM-52C). These results indicate that the IncI1-I(alpha) plasmid is a major plasmid type contributing to the spread of ESBLs in Belgian farms. Other ESBL-plasmid origin-of-replication combinations were: blaSHV-12 on an IncN plasmid or IncB/O/K/Z plasmid, blaCTX-M-32 on an IncX plasmid and blaTEM-52B on an IncX1 plasmid. QnrS1 seems to be flanked by different IS elements and was located on IncX1 in a pig farm or IncX3 plasmids in two broiler farms. IncX plasmids were described as widely distributed and to be associated with fluoroquinolone resistance (Dobiasova and Dolejska, 2016). The presence of QnrS1 on IncX1 or IncX3 plasmids was shown before in Germany’s pork and beef production chain (Juraschek et al., 2021). QnrB19 could be located on a Col(pHAD) plasmid in two isolates in our study, which was also the case in Salmonella spp. from poultry in Nigeria (Jibril et al., 2021).
Co-localization of ESBL genes with virulence factor cib was detected in 14/75 isolates (18.7%) and co-localization with other resistance genes (such as aadA genes, dfrA genes, aph(3′)-Id, aph(6)-Id, blaTEM-1B, cmlA1, sul genes, tetA, and qnrS) was detected. PMQR and ESBL genes localized on the same presumed plasmid contig [qnrS1 with blaCTX-M-15 (n = 1) or blaCTX-M-55 (n = 1)] is concerning. Plasmids co-harboring multiple resistance determinants to critically important antibiotics for human medicine limit treatment options for severe infections and are a threat to public health.
PMQR genes were found in a remarkably low number of isolates and play a limited role in the occurrence of ciproR-E. coli in Belgian farms. Ciprofloxacin resistance was caused by mutations in the QRDR region of gyrA and parC in all ciproR-E. coli, of which most showed triple mutations (GyrA S83L and D87N and ParC S80I) significantly associated with high-level fluoroquinolone resistance. In contrast, QnrS1 or QnrB19 alone leads to low-level resistance to enrofloxacin and moxifloxacin and a sensitive/intermediate phenotype for ciprofloxacin and levofloxacin. Despite strong negative correlations between the presence of qnr genes and gyrA mutations shown previously and the hypothesis that Qnr proteins have a protective effect on quinolone targets (Kaspersen et al., 2020), the presence of QnrS1 combined with GyrA S83L amino acid change was almost always detected in our study. Only two porcine ciproR-E. coli isolates did not contain any mutations in the QRDR of gyrA and parC, instead harbored two PMQR (OqxAB and QnrS1). Although PMQR mechanisms provide low-level resistance (Jacoby et al., 2015), the combination of OqxAB and QnrS1 was sufficient to result in fluoroquinolone resistance above breakpoint.
Pig isolates showed a higher mean number of resistance genes, especially for porcine ciproR-E. coli, which could reflect the higher use of antibiotics in pigs compared to broilers (FOD Volksgezondheid Veiligheid van de Voedselketen en Leefmilieu et al., 2021). In contrast, virulence genes were more abundantly present in broiler isolates. Most virulence genes were involved in adherence and invasion (most prevalent virulence genes were iss, gad, lpfA), which can contribute to successful colonization and enhanced survival in the gut and the environment (Projahn et al., 2018). Also, the presence of ExPEC-associated virulence factors (such as astA, iss, iha, and iroN) is an indication that these commensal E. coli in Belgian farms may have pathogenic potential (Mo et al., 2016). Phylogroups A and B1 were the most common and are associated with commensal phenotypes (Johnson and Stell, 2000). In line with this, phylogroups A and B1 carried a lower number of virulence genes compared to phylogroups D and G. However, the pathogenic E. coli (ETEC and EPEC) detected in this study belonged to phylogroups A and B1 showing that these phylogroups also have the potential to cause extraintestinal infections.
We identified multiple genetically related clones in different animals of the same farm and of distinct farms. The presence of clonally-related bacteria in different poultry farms suggests a common reservoir or transmission of resistant bacteria. The vertical spread of resistant bacteria from the top to the bottom of the broiler production pyramid (Dierikx et al., 2013; Zurfluh et al., 2014) and resistant E. coli residing in the farm environment (Blaak et al., 2015) were previously identified as important transmission routes of resistant bacteria. The diverse profiles of resistance genes, virulence genes and plasmid profiles reflect complex epidemiology. In addition, the detection of plasmid replicons with associated IS elements and ESBL/PMQR genes in different farms and among several STs (such as IncI1-I(alpha) and IncX3) underline that plasmid transmission could be another important contributor to the transmission of resistance.
Our data show the complex epidemiology of ESBL-production and ciprofloxacin resistance in E. coli from livestock, suggesting the spread of these resistances involves both dissemination of resistant clones and horizontal transmission of plasmids. This emphasizes how critical it is to curtail the unnecessary use of antibiotics across all levels of the livestock production chain to preserve antibiotic effectiveness. Additionally, further research into plasmid involvement should include sequencing over longer reads to better understand its circulation on farms. The study supports that commensal E. coli in livestock should be monitored using WGS. Although not all resistance genes could be associated with MGEs or plasmids and we only sequenced a sub-selection of the resistant strains per farm, we gained valuable information on the genetic characteristics of ESBL-E. coli and ciproR-E. coli and the transmission of clones and resistance genes in Belgian farms using genomic data.
5. Conclusion
Our research uncovers a multifaceted landscape of ESBL production and ciprofloxacin resistance in Belgian farms. The complex epidemiology with diverse combinations of ESBL genes, ST types, antibiotic resistance and virulence profiles makes it difficult to translate these findings to the impact on human health. Nevertheless, WGS provides detailed information and should be utilized to properly track this complex situation which poses an urgent challenge for preventing the spread of antimicrobial resistance in the broiler and pig production chain.
Data availability statement
The datasets presented in this study can be found in online repositories. The names of the repository/repositories and accession number(s) can be found in the article/Supplementary material.
Ethics statement
Ethical review and approval was not required for the animal study because the procedure to collect fresh fecal droppings is considered to cause no discomfort, and animals were neither handled nor sacrificed during the study (EC Directive 2010/63). Written informed consent was obtained from the owners for the participation of their animals in this study.
I-4-1-Health Study Group
Lieke van Alphen, Maastricht University Medical Center +, Maastricht, the Netherlands; Nicole van den Braak, Avans University of Applied Sciences, Breda, the Netherlands; Caroline Broucke, Agency for Care and Health, Brussels, Belgiu; Anton Buiting, Elisabeth-TweeSteden Ziekenhuis, Tilburg, the Netherlands; Liselotte Coorevits, Ghent University Hospital, Ghent, Belgium; Sara Dequeker, Agency for Care and Health, Brussels, Belgium; Jeroen Dewulf, Ghent University, Ghent, Belgium; Wouter Dhaeze, Agency for Care and Health, Brussels, Belgium; Bram Diederen, ZorgSaam Hospital, Terneuzen, the Netherlands; Helen Ewalts, GGD Hart voor Brabant, Tilburg, the Netherlands; Herman Goossens, University of Antwerp, Antwerp, Belgium and Antwerp University Hospital, Antwerp, Belgium; Inge Gyssens, Hasselt University, Hasselt, Belgium; Casper den Heijer, GGD Zuid-Limburg, Heerlen, the Netherlands; Christian Hoebe, Maastricht University Medical Center+, Maastricht, the Netherlands and GGD Zuid-Limburg, Heerlen, the Netherlands; Casper Jamin, Maastricht University Medical Center+, Maastricht, the Netherlands; Patricia Jansingh, GGD Limburg Noord, Venlo, the Netherlands; Jan Kluytmans, Amphia Hospital, Breda, the Netherlands and University Medical Center Utrecht, Utrecht University, Utrecht, the Netherlands; Marjolein Kluytmans–van den Bergh, Amphia Hospital, Breda, the Netherlands and University Medical Center Utrecht, Utrecht University, Utrecht, the Netherlands; Stefanie van Koeveringe, Antwerp University Hospital, Antwerp, Belgium; Sien De Koster, University of Antwerp, Antwerp, Belgium; Christine Lammens, University of Antwerp, Antwerp, Belgium; Isabel Leroux, Ghent University Hospital, Ghent, Belgium; Hanna Masson, Agency for Care and Health, Brussel, Belgium; Ellen Nieuwkoop, Elisabeth-TweeSteden Ziekenhuis, Tilburg, the Netherlands; Anita van Oosten, Admiraal de Ruyter Hospital, Goes, the Netherlands; Natascha Perales Selva, Antwerp University Hospital, Antwerp, Belgium; Merel Postma, Ghent University, Ghent, Belgium; Stijn Raven, GGD West-Brabant, Breda, the Netherlands; Paul Savelkoul, Maastricht University Medical Center+, Maastricht, the Netherlands; Annette Schuermans; University Hospitals Leuven, Leuven, Belgium; Nathalie Sleeckx, Proefbedrijf Pluimveehouderij VZW, Geel, Belgium; Krista van der Slikke, GGD Zeeland, Goes, the Netherlands; Arjan Stegeman, Utrecht University, Utrecht, the Netherlands; Tijs Tobias, Utrecht University, Utrecht, the Netherlands; Paulien Tolsma, GGD Brabant Zuid-Oost, Hertogenbosch, the Netherlands; Jacobien Veenemans, Admiraal de Ruyter Hospital, Goes, the Netherlands; Dewi van der Vegt, PAMM Laboratory for pathology and medical microbiology, Veldhoven, the Netherlands; Martine Verelst, University Hospitals Leuven, Leuven, Belgium; Carlo Verhulst, Amphia Hospital, Breda, the Netherlands; Pascal De Waegemaeker, Ghent University Hospital, Ghent, Belgium; Veronica Weterings, Amphia Hospital, Breda, the Netherlands and Radboud University Medical Center, Nijmegen, the Netherlands; Clementine Wijkmans; GGD Hart voor Brabant, Tilburg, the Netherlands; Patricia Willemse–Smits, Elkerliek Ziekenhuis, Geldrop, the Netherlands; Ina Willemsen, Amphia Hospital, Breda, the Netherlands.
Author contributions
SK: original draft writing. MR, BX, CL, DC, KB, KM, JD, MK, JK, and HG: review and editing. SK and MR: data collection. SK, BX, DC, KB, and KM: data analysis. HG and JK: funding acquisition. MB, JK, and HG: project administration. HG, CL, MK, and JK: supervision. SK and MK: data curation. All authors contributed to the article and approved the submitted version.
Funding
The i-4-1-Health project was financed by the Interreg V Flanders-The Netherlands program, the cross-border cooperation program with financial support from the European Regional Development Fund (ERDF) (0215). Additional financial support was received from the Dutch Ministry of Health, Welfare and Sport (325911), the Dutch Ministry of Economic Affairs (DGNR-RRE/14191181), the Province of Noord-Brabant (PROJ-00715/PROJ-01018/PROJ-00758), the Belgian Department of Agriculture and Fisheries (no reference), the Province of Antwerp (1564470690117/1564470610014) and the Province of East-Flanders (E01/subsidie/VLNL/i-4-1-Health). The authors are free to publish the results from the project without interference from the funding bodies. Selective and non-selective agar plates, Etests and Vitek2 AST cards were provided by bioMérieux; FecalSwabs and tryptic soy broths were provided by Copan. The authors were free to publish the results from the project without interference by bioMérieux or Copan.
Acknowledgments
We are grateful to the farmers, the veterinarians and all collaborators in the participating farms for their contribution to the collection of the epidemiological data. We are grateful to the microbiology technicians in the participating laboratories for their contribution to the collection of the microbiological data.
Conflict of interest
The authors declare that the research was conducted in the absence of any commercial or financial relationships that could be construed as a potential conflict of interest.
Publisher’s note
All claims expressed in this article are solely those of the authors and do not necessarily represent those of their affiliated organizations, or those of the publisher, the editors and the reviewers. Any product that may be evaluated in this article, or claim that may be made by its manufacturer, is not guaranteed or endorsed by the publisher.
Supplementary material
The Supplementary material for this article can be found online at: https://www.frontiersin.org/articles/10.3389/fmicb.2023.1150470/full#supplementary-material
Footnotes
References
Ahmed, S., Olsen, J. E., and Herrero-Fresno, A. (2017). The genetic diversity of commensal Escherichia coli strains isolated from nonantimicrobial treated pigs varies according to age group. PLoS One 12, 1–18. doi: 10.1371/journal.pone.0178623
Amábile-cuevas, C. F., and Demple, B. (1991). Molecular characterization of the soxRS genes of Escherichia coli: two genes control a superoxide stress regulon. Nucleic Acids Res. 19, 4479–4484. doi: 10.1093/nar/19.16.4479
Bankevich, A., Nurk, S., Antipov, D., Gurevich, A. A., Dvorkin, M., Kulikov, A. S., et al. (2012). SPAdes: a new genome assembly algorithm and its applications to single-cell sequencing. J. Comput. Biol. 19, 455–477. doi: 10.1089/cmb.2012.0021
Beghain, J., Bridier-Nahmias, A., Nagard, H.Le, Denamur, E., and Clermont, O. (2018). ClermonTyping: an easy-to-use and accurate in silico method for Escherichia genus strain phylotyping. Microb Genom 4, 1–8. doi: 10.1099/mgen.0.000192
Bernreiter-Hofer, T., Schwarz, L., Müller, E., Cabal-Rosel, A., Korus, M., Misic, D., et al. (2021). The pheno- and genotypic characterization of porcine Escherichia coli isolates. Microorganisms 9, 1–21. doi: 10.3390/microorganisms9081676
Blaak, H., van Hoek, A. H. A. M., Hamidjaja, R. A., van der Plaats, R. Q. J., Kerkhof-de Heer, L., de Roda Husman, A. M., et al. (2015). Distribution, numbers, and diversity of ESBL-Producing E. coli in the poultry farm environment. PLoS One 10:e0135402. doi: 10.1371/journal.pone.0135402
Blair, J. M. A., Bavro, V. N., Ricci, V., Modi, N., Cacciotto, P., Kleinekathӧfer, U., et al. (2015). AcrB drug-binding pocket substitution confers clinically relevant resistance and altered substrate specificity. Proc. Natl. Acad. Sci. U. S. A. 112, 3511–3516. doi: 10.1073/pnas.1419939112
Bojesen, A. M., Ahmed, U., Skaarup, H., and Espinosa-Gongora, C. (2022). Recurring outbreaks by the same Escherichia coli ST10 clone in a broiler unit during 18 months. Vet. Res. 53:2. doi: 10.1186/s13567-021-01017-6
Bortolaia, V., Kaas, R. S., Ruppe, E., Roberts, M. C., Schwarz, S., Cattoir, V., et al. (2020). ResFinder 4.0 for predictions of phenotypes from genotypes. J. Antimicrob. Chemother. 75, 3491–3500. doi: 10.1093/jac/dkaa345
Caekebeke, N., Jonquiere, F. J., Ringenier, M., Tobias, T. J., Postma, M., van den Hoogen, A., et al. (2020). Comparing farm biosecurity and antimicrobial use in high-antimicrobial-consuming broiler and pig farms in the Belgian–Dutch border region, Comparing farm biosecurity and antimicrobial use in high-antimicrobial-consuming broiler and pig farms in the Belgian-Dutch border region. Front Vet Sci 7:558455. doi: 10.3389/fvets.2020.558455
Carattoli, A., and Hasman, H. (2020). PlasmidFinder and in silico pMLST: identification and typing of plasmid replicons in whole-genome sequencing (WGS). Methods Mol. Biol. 2075, 3895–903. doi: 10.1007/978-1-4939-9877-7_20
Ceccarelli, D., Kant, A., van Essen-Zandbergen, A., Dierikx, C., Hordijk, J., Wit, B., et al. (2019). Diversity of plasmids and genes encoding resistance to extended spectrum cephalosporins in commensal Escherichia coli from Dutch livestock in 2007-2017. Front. Microbiol. 10, 1–9. doi: 10.3389/fmicb.2019.00076
Chen, L., Zheng, D., Liu, B., Yang, J., and Jin, Q. (2016). VFDB 2016: hierarchical and refined dataset for big data analysis - 10 years on. Nucleic Acids Res. 44, D694–D697. doi: 10.1093/nar/gkv1239
de Been, M., Lanza, V. F., de Toro, M., Scharringa, J., Dohmen, W., du, Y., et al. (2014). Dissemination of cephalosporin resistance genes between Escherichia coli strains from farm animals and humans by specific plasmid lineages. PLoS Genet. 10:e1004776. doi: 10.1371/journal.pgen.1004776
De Koster, S., Ringenier, M., Lammens, C., Stegeman, A., Tobias, T., Velkers, F., et al. (2021). ESBL-producing, carbapenem-and ciprofloxacin-resistant Escherichia coli in Belgian and Dutch broiler and pig farms: a cross-sectional and cross-border study. Antibiotics 10, 1–14. doi: 10.3390/antibiotics10080945
Dierikx, C. M., Van Der Goot, J. A., Smith, H. E., Kant, A., and Mevius, D. J. (2013). Presence of ESBL/AmpC -producing Escherichia coli in the broiler production pyramid: a descriptive study. PLoS One 8:e79005. doi: 10.1371/journal.pone.0079005
Dobiasova, H., and Dolejska, M. (2016). Prevalence and diversity of IncX plasmids carrying fluoroquinolone and β-lactam resistance genes in Escherichia coli originating from diverse sources and geographical areas. J. Antimicrob. Chemother. 71, 2118–2124. doi: 10.1093/jac/dkw144
Duggett, N., AbuOun, M., Randall, L., Horton, R., Lemma, F., Rogers, J., et al. (2020). The importance of using whole genome sequencing and extended spectrum beta-lactamase selective media when monitoring antimicrobial resistance. Sci. Rep. 10, 19880–19810. doi: 10.1038/s41598-020-76877-7
Durrant, M. G., Li, M. M., Siranosian, B. A., Montgomery, S. B., and Bhatt, A. S. (2020). A Bioinformatic analysis of integrative Mobile genetic elements highlights their role in bacterial adaptation. Cell Host Microbe 27, 140–153.e9. doi: 10.1016/j.chom.2019.10.022
European Food Safety Authority and European Centre for Disease Prevention and Control (2021). The European Union summary report on antimicrobial resistance in zoonotic and indicator bacteria from humans, animals and food in 2018/2019. EFSA J. 19:e06490. doi: 10.2903/j.efsa.2021.6490
FAVV-AFSCA (2021). Activities and achievements regarding the reduction in the use of antibiotics and antimicrobial resistance in veterinary medicine in Belgium in 2016-2020. Available at: https://www.favv-afsca.be/professionnals/publications/reportconvenantAB/_documents/2018-06-27-Publiekrapport_EN_Internet2.pdf (Accessed January 18, 2023).
FOD Volksgezondheid Veiligheid van de Voedselketen en Leefmilieu, Federaal Agentschap van de Geneesmiddelen en Gezondheidsproducten, Federaal Agentschap van de Veiligheid van de Voedselketen, Rijksinstituut voor Ziekte- en Invaliditeitsverzekering, and Sciensano (2021). BELMAP: one Health report on antibiotic use and resistance 2011-2020. Available at: https://www.health.belgium.be/sites/default/files/uploads/fields/fpshealth_theme_file/rapport_annuel_amr_2021.pdf (Accessed December 22, 2022).
Gordon, D., and George, J. (2015). Mechanisms of drug resistance: quinolone resistance. Ann. N. Y. Acad. Sci. 176, 139–148. doi: 10.1111/nyas.12830.Mechanisms
Gurevich, A., Saveliev, V., Vyahhi, N., and Tesler, G. (2013). QUAST: quality assessment tool for genome assemblies. Bioinformatics 29, 1072–1075. doi: 10.1093/bioinformatics/btt086
Hansen, L. H., Jensen, L. B., Sørensen, H. I., and Sørensen, S. J. (2007). Substrate specificity of the OqxAB multidrug resistance pump in Escherichia coli and selected enteric bacteria. J. Antimicrob. Chemother. 60, 145–147. doi: 10.1093/jac/dkm167
Hao, H., Pan, H., Ahmad, I., Cheng, G., Wang, Y., Dai, M., et al. (2013). Susceptibility breakpoint for enrofloxacin against swine salmonella spp. J. Clin. Microbiol. 51, 3070–3072. doi: 10.1128/JCM.01096-13
Hayer, S. S., Lim, S., Hong, S., Elnekave, E., Johnson, T., and Rovira, A. (2020). Genetic determinants of resistance to extended-Spectrum cephalosporin and fluoroquinolone in Escherichia coli isolated from diseased pigs in the United States 5, e00990–e00920. doi: 10.1128/mSphere.00990-20
Jacoby, G. A., Strahilevitz, J., and Hooper, D. C. (2015). Plasmid-mediated quinolone resistance, 2. doi: 10.1128/microbiolspec.PLAS-0006-2013.Plasmid-mediated
Jibril, A. H., Okeke, I. N., Dalsgaard, A., Menéndez, V. G., and Olsen, J. E. (2021). Genomic analysis of antimicrobial resistance and resistance plasmids in salmonella serovars from poultry in Nigeria. Antibiotics 10, 1–22. doi: 10.3390/antibiotics10020099
Joensen, K. G., Tetzschner, A. M. M., Iguchi, A., Aarestrup, F. M., and Scheutz, F. (2015). Rapid and easy in silico serotyping of Escherichia coli isolates by use of whole-genome sequencing data. J. Clin. Microbiol. 53, 2410–2426. doi: 10.1128/JCM.00008-15
Johnson, J. R., and Stell, A. L. (2000). Extended virulence genotypes of Escherichia coli strains from patients with Urosepsis in relation to phylogeny and host compromise. J. Infect. Dis. 181, 261–272. doi: 10.1086/315217
Jolley, K. A., Bray, J. E., and Maiden, M. C. J. (2018). Open-access bacterial population genomics: BIGSdb software, the PubMLST.org website and their applications. Wellcome Open Res 3, 124–120. doi: 10.12688/wellcomeopenres.14826.1
Juraschek, K., Käsbohrer, A., Malorny, B., Schwarz, S., Meemken, D., and Hammerl, J. A. (2021). Dissection of highly prevalent qnrS1-carrying IncX plasmid types in commensal Escherichia coli from German food and livestock. Antibiotics 10:1236. doi: 10.3390/antibiotics10101236
Karczmarczyk, M., Martins, M., Quinn, T., Leonard, N., and Fanning, S. (2011). Mechanisms of fluoroquinolone resistance in Escherichia coli isolates from food-producing animals. Appl. Environ. Microbiol. 77, 7113–7120. doi: 10.1128/AEM.00600-11
Kaspersen, H., Sekse, C., Fiskebeck, Z., Slettemeås, S., and Simm, R. (2020). Dissemination of quinolone-resistant Escherichia coli in the Norwegian broiler and pig production chains and possible persistence in the broiler production environment. Appl. Environ. Microbiol. 86, e02769–e02719. doi: 10.1128/AEM.02769-19
Kluytmans-van den Bergh, M., Lammens, C., Perales Selva, N., Buiting, A., Leroux-roels, I., Saegeman, V., et al. (2019). Microbiological methods to detect intestinal carriage of highly-resistant microorganisms (HRMO) in humans and livestock in the i-4-1-Health Dutch- Belgian cross-border project [Preprint]. doi: 10.20944/preprints201912.0216.v1
Kurittu, P., Khakipoor, B., Aarnio, M., Nykäsenoja, S., Brouwer, M., Myllyniemi, A. L., et al. (2021). Plasmid-borne and chromosomal ESBL/AmpC genes in Escherichia coli and Klebsiella pneumoniae in global food products. Front. Microbiol. 12, 1–19. doi: 10.3389/fmicb.2021.592291
Leekitcharoenphon, P., Johansson, M. H. K., Munk, P., Malorny, B., Skarżyńska, M., Wadepohl, K., et al. (2021). Genomic evolution of antimicrobial resistance in Escherichia coli. Sci. Rep. 11, 15108–15112. doi: 10.1038/s41598-021-93970-7
Letunic, I., and Bork, P. (2021). Interactive tree of life (iTOL) v5: an online tool for phylogenetic tree display and annotation. Nucleic Acids Res. 49, W293–W296. doi: 10.1093/nar/gkab301
Leverstein-van Hall, M. A., Dierikx, C. M., Cohen Stuart, J., Voets, G. M., van den Munckhof, M. P., van Essen-Zandbergen, A., et al. (2011). Dutch patients, retail chicken meat and poultry share the same ESBL genes, plasmids and strains. Clin. Microbiol. Infect. 17, 873–880. doi: 10.1111/j.1469-0691.2011.03497.x
Lindgren, P. K., Karlsson, Å., and Hughes, D. (2003). Mutation rate and evolution of fluoroquinolone resistance in Escherichia coli isolates from patients with urinary tract infections. Antimicrob. Agents Chemother. 47, 3222–3232. doi: 10.1128/AAC.47.10.3222-3232.2003
Magiorakos, A. P., Srinivasan, A., Carey, R. B., Carmeli, Y., Falagas, M. E., Giske, C. G., et al. (2012). Multidrug-resistant, extensively drug-resistant and pandrug-resistant bacteria: an international expert proposal for interim standard definitions for acquired resistance. Clin. Microbiol. Infect. 18, 268–281. doi: 10.1111/j.1469-0691.2011.03570.x
Manges, A. R., Harel, J., Masson, L., Edens, T. J., Portt, A., Reid-Smith, R. J., et al. (2015). Multilocus sequence typing and virulence gene profiles associated with Escherichia coli from human and animal sources. Foodborne Pathog. Dis. 12, 302–310. doi: 10.1089/fpd.2014.1860
Manges, A. R., and Johnson, J. R. (2015). Reservoirs of Extraintestinal pathogenic Escherichia coli. Microbiol Spectr 3:UTI-0006-2012. doi: 10.1128/microbiolspec.uti-0006-2012
Massella, E., Reid, C. J., Cummins, M. L., Anantanawat, K., Zingali, T., Serraino, A., et al. (2020). Snapshot study of whole genome sequences of Escherichia coli from healthy companion animals, livestock, wildlife, humans and food in Italy. Antibiotics 9, 1–22. doi: 10.3390/antibiotics9110782
McArthur, A. G., Waglechner, N., Nizam, F., Yan, A., Azad, M. A., Baylay, A. J., et al. (2013). The comprehensive antibiotic resistance database. Antimicrob. Agents Chemother. 57, 3348–3357. doi: 10.1128/AAC.00419-13
Mo, S. S., Slettemeås, J. S., Berg, E. S., Norström, M., and Sunde, M. (2016). Plasmid and host strain characteristics of Escherichia coli resistant to extended-spectrum cephalosporins in the norwegian broiler production. PLoS One 11, 1–14. doi: 10.1371/journal.pone.0154019
Moor, J., Aebi, S., Rickli, S., Mostacci, N., Overesch, G., Oppliger, A., et al. (2021). Dynamics of extended-spectrum cephalosporin-resistant Escherichia coli in pig farms: a longitudinal study. Int. J. Antimicrob. Agents 58:106382. doi: 10.1016/j.ijantimicag.2021.106382
Murray, C. J., Ikuta, K. S., Sharara, F., Swetschinski, L., Robles Aguilar, G., Gray, A., et al. (2022). Global burden of bacterial antimicrobial resistance in 2019: a systematic analysis. Lancet 399, 629–655. doi: 10.1016/s0140-6736(21)02724-0
Ng, P. C., and Henikoff, S. (2003). SIFT: predicting amino acid changes that affect protein function. Nucleic Acids Res. 31, 3812–3814. doi: 10.1093/nar/gkg509
Oethinger, M., Podglajen, I., Kern, W. V., and Levy, S. B. (1998). Overexpression of the marA or soxS regulatory gene in clinical topoisomerase mutants of Escherichia coli. Antimicrob. Agents Chemother. 42, 2089–2094. doi: 10.1128/aac.42.8.2089
Oteo, J., Diestra, K., Juan, C., Bautista, V., Novais, Â., Pérez-Vázquez, M., et al. (2009). Extended-spectrum β-lactamase-producing Escherichia coli in Spain belong to a large variety of multilocus sequence typing types, including ST10 complex/a, ST23 complex/a and ST131/B2. Int. J. Antimicrob. Agents 34, 173–176. doi: 10.1016/j.ijantimicag.2009.03.006
Partridge, S. R., Kwong, S. M., Firth, N., and Jensen, S. O. (2018). Mobile genetic elements associated with antimicrobial resistance. Clin. Microbiol. Rev. 31, 1–61. doi: 10.1128/CMR.00088-17
Pietsch, F., Bergman, J. M., Brandis, G., Marcusson, L. L., Zorzet, A., Huseby, D. L., et al. (2017). Ciprofloxacin selects for RNA polymerase mutations with pleiotropic antibiotic resistance effects. J. Antimicrob. Chemother. 72, 75–84. doi: 10.1093/jac/dkw364
Poirel, L., Decousser, J. W., and Nordmann, P. (2003). Insertion sequence ISEcp1B is involved in expression and mobilization of a blaCTX-M β-lactamase gene. Antimicrob. Agents Chemother. 47, 2938–2945. doi: 10.1128/AAC.47.9.2938-2945.2003
Projahn, M., Daehre, K., Semmler, T., Guenther, S., Roesler, U., and Friese, A. (2018). Environmental adaptation and vertical dissemination of ESBL-/pAmpC-producing Escherichia coli in an integrated broiler production chain in the absence of an antibiotic treatment. Microb. Biotechnol. 11, 1017–1026. doi: 10.1111/1751-7915.13040
R Core Team (2020). R: a language and environment for statistical computing. Vienna, Austria: R Foundation for Statistical Computing. Available at: https://www.r-project.org/.
Reid, C. J., Wyrsch, E. R., Chowdhury, P. R., Zingali, T., Liu, M., Darling, A. E., et al. (2017). Porcine commensal Escherichia coli: a reservoir for class 1 integrons associated with IS26. Microb Genom 3:e000143. doi: 10.1099/mgen.0.000143
Riley, L. W. (2014). Pandemic lineages of extraintestinal pathogenic Escherichia coli. Clin. Microbiol. Infect. 20, 380–390. doi: 10.1111/1469-0691.12646
Robicsek, A., Strahilevitz, J., Jacoby, G. A., Macielag, M., Abbanat, D., Chi, H. P., et al. (2006). Fluoroquinolone-modifying enzyme: a new adaptation of a common aminoglycoside acetyltransferase. Nat. Med. 12, 83–88. doi: 10.1038/nm1347
Roer, L., Tchesnokova, V., Allesøe, R., Muradova, M., Chattopadhyay, S., Ahrenfeldt, J., et al. (2017). Development of a web tool for Escherichia coli subtyping based on fimH alleles. J. Clin. Microbiol. 55, 2538–2543. doi: 10.1128/JCM.00737-17
Rozwandowicz, M., Brouwer, M. S. M., Fischer, J., Wagenaar, J. A., Gonzalez-Zorn, B., Guerra, B., et al. (2018). Plasmids carrying antimicrobial resistance genes in Enterobacteriaceae. J. Antimicrob. Chemother. 73, 1121–1137. doi: 10.1093/jac/dkx488
Schürch, A. C., Arredondo-Alonso, S., Willems, R. J. L., and Goering, R. V. (2018). Whole genome sequencing options for bacterial strain typing and epidemiologic analysis based on single nucleotide polymorphism versus gene-by-gene–based approaches. Clin. Microbiol. Infect. 24, 350–354. doi: 10.1016/j.cmi.2017.12.016
Seemann, T. (2014). Prokka: rapid prokaryotic genome annotation. Bioinformatics 30, 2068–2069. doi: 10.1093/bioinformatics/btu153
Siguier, P., Perochon, J., Lestrade, L., Mahillon, J., and Chandler, M. (2006). ISfinder: the reference Centre for bacterial insertion sequences. Nucleic Acids Res. 34, D32–D36. doi: 10.1093/nar/gkj014
Silva, M., Machado, M. P., Silva, D. N., Rossi, M., Moran-Gilad, J., Santos, S., et al. (2018). chewBBACA: a complete suite for gene-by-gene schema creation and strain identification. Microb. Genom. 4, 1–7. doi: 10.1099/mgen.0.000166
Smet, A., Martel, A., Persoons, D., Dewulf, J., Heyndrickx, M., Catry, B., et al. (2008). Diversity of extended-Spectrum -lactamases and class C -lactamases among cloacal Escherichia coli isolates in Belgian broiler farms. Antimicrob. Agents Chemother. 52, 1238–1243. doi: 10.1128/AAC.01285-07
Stubberfield, E., AbuOun, M., Sayers, E., O’Connor, H. M., Card, R. M., and Anjum, M. F. (2019). Use of whole genome sequencing of commensal Escherichia coli in pigs for antimicrobial resistance surveillance, United Kingdom, 2018. Eur. Secur. 24:1900136. doi: 10.2807/1560-7917.ES.2019.24.50.1900136
Tetzschner, A. M. M., and Lund, O. (2020). In silico genotyping of Escherichia coli isolates for sequencing data. J. Clin. Microbiol. 58, 1–13. doi: 10.1128/JCM.01269-20
The European Committee on Antimicrobial Susceptibility Testing (2022). The European Committe on antimicrobial susceptibility testing. Breakpoint tables for interpretation of MICs and zone diameters. Version 12.0. Available at: https://www.eucast.org/fileadmin/src/media/PDFs/EUCAST_files/Breakpoint_tables/v_12.0_Breakpoint_Tables.pdf (Accessed January 18, 2022).
Toval, F., Köhler, C. D., Vogel, U., Wagenlehner, F., Mellmann, A., Fruth, A., et al. (2014). Characterization of Escherichia coli isolates from hospital inpatients or outpatients with urinary tract infection. J. Clin. Microbiol. 52, 407–418. doi: 10.1128/JCM.02069-13
Tran, J. H., Jacoby, G. A., and Hooper, D. C. (2005). Interaction of the plasmid-encoded quinolone resistance protein QnrA with Escherichia coli topoisomerase IV. Antimicrob. Agents Chemother. 49, 3050–3052. doi: 10.1128/AAC.49.7.3050-3052.2005
van Damme, I., Garcia-Graells, C., Biasino, W., Gowda, T., Botteldoorn, N., and de Zutter, L. (2017). High abundance and diversity of extended-spectrum beta-lactamase (ESBL)-producing Escherichia coli in faeces and tonsils of pigs at slaughter. Vet. Microbiol. 208, 190–194. doi: 10.1016/j.vetmic.2017.08.009
Van Hoek, A. H. A. M., Dierikx, C., Bosch, T., Schouls, L., Van Duijkeren, E., and Visser, M. (2020). Transmission of ESBL-producing Escherichia coli between broilers and humans on broiler farms. J. Antimicrob. Chemother. 75, 543–549. doi: 10.1093/jac/dkz507
White, D. G., Goldman, J. D., Demple, B., and Levy, S. B. (1997). Role of the acrAB locus in organic solvent tolerance mediated by expression of marA, soxS, or robA in Escherichia coli. J. Bacteriol. 179, 6122–6126. doi: 10.1128/jb.179.19.6122-6126.1997
World Health Organization Advisory Group on Integrated Surveillance of Antimicrobial Resistance (2018). Critically important antimicrobials for human medicine: ranking of medically important antimicrobial for risk management of antimicrobial resistance due to non-human use- 6th revision. Switzerland. Available at: https://apps.who.int/iris/bitstream/handle/10665/312266/9789241515528-eng.pdf.
World Organization for Animal Health (OIE) (2018). OIE list of antimicrobial agents of veterinary importance. Available at: https://www.oie.int/fileadmin/home/eng/our_scientific_expertise/docs/pdf/amr/a_oie_list_antimicrobials_may2018.pdf (Accessed July 5, 2022).
Xavier, B. B., Mysara, M., Bolzan, M., Ribeiro-Gonçalves, B., Alako, B. T. F., Harrison, P., et al. (2020). BacPipe: a rapid, user-friendly whole-genome sequencing pipeline for clinical diagnostic bacteriology. iScience 23:100769. doi: 10.1016/j.isci.2019.100769
Yamane, K., Wachino, J. I., Suzuki, S., Kimura, K., Shibata, N., Kato, H., et al. (2007). New plasmid-mediated fluoroquinolone efflux pump, QepA, found in an Escherichia coli clinical isolate. Antimicrob. Agents Chemother. 51, 3354–3360. doi: 10.1128/AAC.00339-07
Zhang, A. N., Gaston, J. M., Dai, C. L., Zhao, S., Poyet, M., Groussin, M., et al. (2021). An omics-based framework for assessing the health risk of antimicrobial resistance genes. Nat. Commun. 12, 4765–4712. doi: 10.1038/s41467-021-25096-3
Zingali, T., Reid, C. J., Chapman, T. A., Gaio, D., Liu, M., Darling, A. E., et al. (2020). Whole genome sequencing analysis of porcine faecal commensal Escherichia coli carrying class 1 integrons from sows and their offspring. Microorganisms 8, 1–17. doi: 10.3390/microorganisms8060843
Zurfluh, K., Wang, J., Klumpp, J., Nüesch-Inderbinen, M., Fanning, S., and Stephan, R. (2014). Vertical transmission of highly similar blaCTX-M-1-harbouring IncI1 plasmids in Escherichia coli with different MLST types in the poultry production pyramid. Front. Microbiol. 5, 1–7. doi: 10.3389/fmicb.2014.00519
Keywords: ESBL, ciprofloxacin, Escherichia coli, livestock, WGS
Citation: De Koster S, Ringenier M, Xavier BB, Lammens C, De Coninck D, De Bruyne K, Mensaert K, Kluytmans-van den Bergh M, Kluytmans J, Dewulf J, Goossens H and on behalf of the i-4-1-Health Study Group (2023) Genetic characterization of ESBL-producing and ciprofloxacin-resistant Escherichia coli from Belgian broilers and pigs. Front. Microbiol. 14:1150470. doi: 10.3389/fmicb.2023.1150470
Edited by:
Qixia Luo, Zhejiang University, ChinaReviewed by:
Asghar Ali, Jamia Hamdard University, IndiaTam Tran, University of Science and Technology of Hanoi (USTH), Vietnam
Copyright © 2023 De Koster, Ringenier, Xavier, Lammens, De Coninck, De Bruyne, Mensaert, Kluytmans-van den Bergh, Dewulf, Goossens and on behalf of the i-4-1-Health Study Group. This is an open-access article distributed under the terms of the Creative Commons Attribution License (CC BY). The use, distribution or reproduction in other forums is permitted, provided the original author(s) and the copyright owner(s) are credited and that the original publication in this journal is cited, in accordance with accepted academic practice. No use, distribution or reproduction is permitted which does not comply with these terms.
*Correspondence: Herman Goossens, aGVybWFuLmdvb3NzZW5zQHV6YS5iZQ==