- 1Department of Endodontics, Stomatological Hospital, School of Stomatology, Southern Medical University, Guangzhou, Guangdong, China
- 2Department of Stomatology, Nanfang Hospital, Southern Medical University, Guangzhou, Guangdong, China
- 3Hainan General Hospital (Hainan Affiliated Hospital of Hainan Medical University), Haikou, Hainan, China
Accumulating evidence suggests that in various systems, not all bidirectional microbiota–host interactions involve direct cell contact. Bacterial extracellular vesicles (BEVs) may be key participants in this interkingdom crosstalk. BEVs mediate microbiota functions by delivering effector molecules that modulate host signaling pathways, thereby facilitating host–microbe interactions. BEV production during infections by both pathogens and probiotics has been observed in various host tissues. Therefore, these vesicles released by microbiota may have the ability to drive or inhibit disease pathogenesis in different systems within the host. Here, we review the current knowledge of BEVs and particularly emphasize their interactions with the host and the pathogenesis of systemic diseases.
1. Introduction
Bacterial extracellular vesicles (BEVs) are nanosized lipid vesicles with a particle size of 20–250 nm that are secreted by bacteria during growth. In 1963, the presence of BEVs was first observed in the cell wall of gram-negative bacteria by electron microscopy, and initial research primarily focused on BEV functions. Initially, they were viewed as cellular debris that occurred after dead cells degraded (Bishop and Work, 1965). Nonetheless, due to cargo analysis of BEVs and the discovery of their biogenesis mechanism, they are now regarded as contributors to physiological and pathological processes that lead to the occurrence and development of systemic diseases.
Although gram-negative and gram-positive bacteria have different vesicle secretory pathways due to different cell wall structures, as vectors of diverse bioactive compounds, BEVs participate in bacterial intraspecific and interspecific communication and interactions with hosts, including horizontal gene transfer, the killing of competing bacteria, phage neutralization and the delivery of virulence factors to host cells (Tzipilevich et al., 2017; Wei et al., 2022). In addition, components such as lipopolysaccharides (LPS) and peptidoglycan carried by vesicles are naturally immunogenic and can be recognized by host cell pathogen-recognition receptors (PRRs) to activate signaling pathways, induce cytokine production, and play physiological and pathological roles similar to those of parent bacteria (Dauros-Singorenko et al., 2020). Emerging studies have revealed that BEVs are involved in diseases of various systems of the human body, promoting bacterial infections and pro−/anti-inflammatory responses to drive the onset and progression of systemic diseases, such as autoimmune diseases, inflammatory bowel disease (IBD), liver diseases, allergic diseases, and metabolic syndromes such as diabetes.
In this review, we discuss the key functions of BEVs. Furthermore, we summarize the pathways of BEV synthesis and the mechanisms through which BEVs released by commensal and pathogenic bacteria are recognized by host PRRs to initiate inflammatory responses or mediate immunomodulation. Moreover, we highlight their biological role in microbiota-host interactions, in addition to their role in the pathogenesis of diseases of specific systems, namely, the nervous, digestive, circulatory, respiratory and motor systems.
2. Contents and biogenesis of bacterial extracellular vesicles
The structures of gram-positive bacteria and gram-negative bacteria have obvious differences, as do their released vesicles (Figure 1). Currently, there are different opinions on the mechanism of BEV biosynthesis; however, a consistent conclusion is that the formation and release of BEVs is not a random act but an ordered regulatory process (Schwechheimer and Kuehn, 2015).
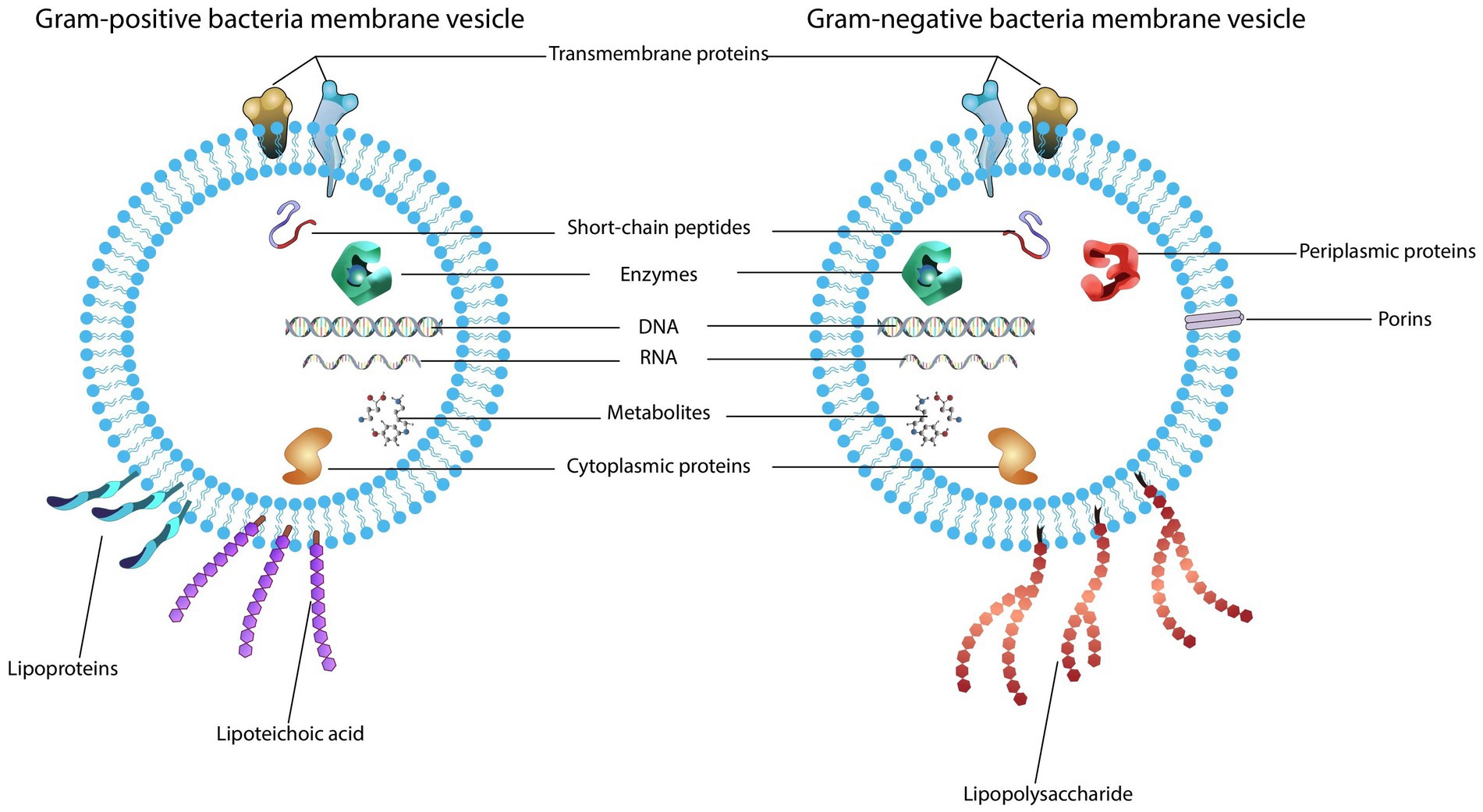
Figure 1. Structure and content of bacterial extracellular vesicles. (1) Gram-positive bacteria produce vesicles that carry bacterial cargo including transmembrane proteins, enzymes, toxins, peptidoglycan, lipoproteins, lipoteichoic acids and nucleic acids. (2) Outer membrane vesicles secreted by gram-negative bacteria also contain cargo composed of the parental bacterial components within a lipid membrane, such as proteins, lipids, peptidoglycan, and nucleic acids.
2.1. Contents and biogenesis of gram-negative bacterial outer membrane vesicles
The cell wall of gram-negative bacteria consists of an outer membrane, an inner membrane, and a peptidoglycan layer between them. Gram-negative bacteria release the outer membrane and cytoplasmic contents through the formation of a nanosized spherical structure with diameters in the range of 10–300 nm, whose composition is analogous to the outer membrane, so they are called outer membrane vesicles (OMVs). OMVs basically consist of proteins, virulence factors, lipids, and peptidoglycan (Santos et al., 2018; Toyofuku et al., 2019). Most of the proteins in OMVs are virulence-related proteins, and the lipids of OMVs are primarily LPS and phospholipids (Liu et al., 2019).
Gram-negative bacteria produce OMVs mainly in two ways: (1) through an imbalance in biosynthetic peptidoglycan or when hydrophobic molecules are embedded in the outer membrane, thereby causing cell membrane destabilization, which ultimately leads to the vesiculation of the outer membrane: the reduction of crosslink between peptidoglycan and the outer membrane induces the expansion of the outer membrane; the accumulation of bacterial cell wall peptidoglycan fragments increases peripheral pressure so that the outer membrane is bent to generate OMVs; and the accumulation of molecules that increase membrane curvature such as LPS can trigger membrane swelling (Arunmanee et al., 2016; Avila-Calderón et al., 2021); and (2) the generation of explosive outer membrane vesicles (EOMVs) and outer-inner membrane vesicles (OIMVs) can occur via cell lysis (Toyofuku et al., 2019). In recent years, a general mechanism has emerged that can explain how OMVs form in different environments, and the destruction of the highly conserved VacJ/Yrb ABC transport system may be a core mechanism involved in this process (Roier et al., 2016). These mechanisms are based on the common hypothesis that vesicles bulge from the outer membrane, and the destruction of the crosslink between peptidoglycan and the outer membrane or the increased extrusion pressure causes the outer membrane to separate from the peptidoglycan layer and release in the form of OMVs (Toyofuku et al., 2017).
2.2. Contents and biogenesis of gram-positive bacterial membrane vesicles
Gram-positive bacteria are a class of bacteria surrounded by a plasma membrane and a thick peptidoglycan layer. Due to the lack of an outer membrane and a thick cell wall, they were initially thought to be unable to produce and secrete EVs. In 2007, researchers isolated vesicles from mycobacteria and conducted extensive proteomic studies of their components, confirming that gram-positive bacteria also release EVs (Lee et al., 2009). Researchers then found that the vesicles of gram-positive bacteria are produced from the inner membrane and secreted through the peptidoglycan layer to the surrounding environment; therefore, these vesicles are usually called cytoplasmic membrane vesicles (CMVs) or membrane vesicles (MVs) (Brown et al., 2015). MVs contain cell membrane and cytoplasmic components, and periplasmic components are unique to MVs and not to OMVs (Toyofuku et al., 2017). MVs are approximately 20 ~ 400 nm in diameter and consist of membrane-associated proteins, cytoplasmic proteins, peptidoglycan, and lipoteichoic acid (Brown et al., 2015; Kopparapu et al., 2021).
The pathway by which gram-positive bacteria form MVs is primarily through endolysin-triggered death lysis, which is called bubbling cell death (Toyofuku et al., 2019). Studies have revealed that endolysin expressed by pro bacteriophages creates pores in the peptidoglycan layer of the cell wall; therefore, the material in the cytoplasm bulges outward and is released in the form of MVs; meanwhile, a few of them are secreted via encapsulation from the remaining peptidoglycan layer (Toyofuku et al., 2017; Jeong et al., 2022). Explosive cell lysis is another form of MV biogenesis (Jeong et al., 2022). Regarding the special type of EVs found in recent years, namely, the tubular membrane structure, local lysis of the cell wall may lead to blistering of the cytoplasmic membrane, which forms a nanotube structure as a bridge for material exchange between bacteria (Baidya et al., 2018). Although there is currently a lack of definitive evidence regarding how gram-positive bacteria bypass the thick cell wall to produce MVs, some underlying mechanisms can be investigated based on studies of vesicle composition, morphology, etc. (Kalra et al., 2016).
2.3. Conclusions on BEV biogenesis
Although the generation of BEVs is an energy-consuming process, this secretory mode has irreplaceable advantages in protecting cargo from degradation by extracellular proteases and triggering receptor-mediated signal transcriptional induction in host cells (Kaparakis-Liaskos and Ferrero, 2015). The biogenesis and composition of BEVs is dependent on the milieu to which the bacterium is exposed, and how vesicle formation and content shift in response to varying biological environments needs to be investigated to identify their specific functions.
3. The interaction between BEVs and host cells
BEVs can be released at all stages during bacterial growth as a secretory system that influences the communication and interaction between hosts and bacteria. Pathogen-associated molecular pattern (PAMP) contents of BEVs enable them to bind to PRRs on the membrane surface and in the cytoplasm of immune cells and nonimmune cells, thereby activating downstream inflammatory signaling pathways (Alvarez et al., 2016; Table 1). After BEVs enter host cells, they can transmit immunogenic protein components, DNA, and sRNAs into recipient cells to prime the host immune responses (Bitto et al., 2021; Figure 2).
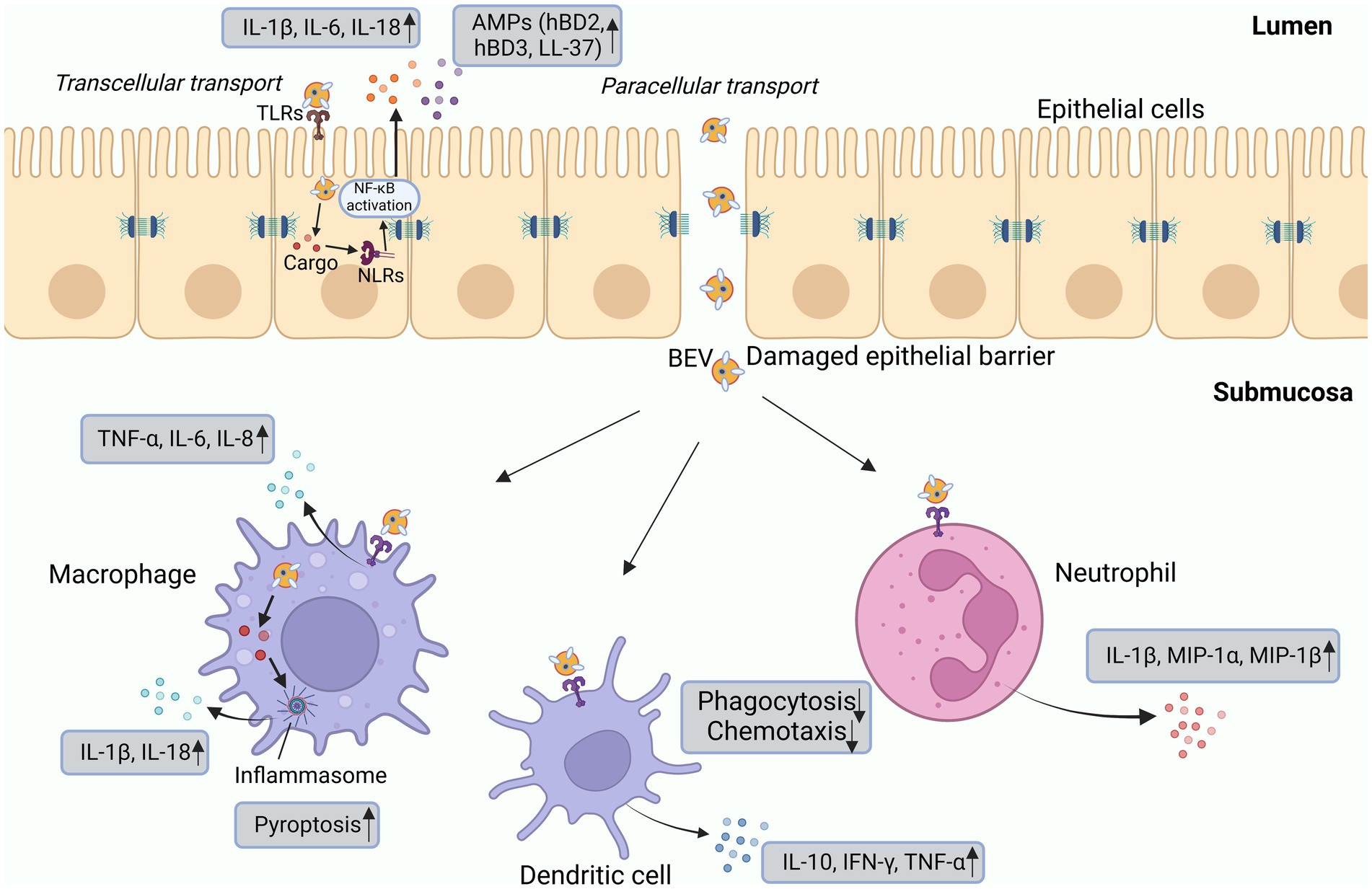
Figure 2. Interactions of BEVs with epithelial and innate immune cells. BEVs can directly interact with epithelial cells or PRRs to promote the production of IL-1β, IL-6, IL-8, and AMPs. Cargo delivered by BEVs is detected by intracellular NLRs and then activates signal transduction pathways. The interaction between BEVs and epithelial cells can damage tight junctions, facilitating the delivery of BEVs and the release of cargo components into the submucosa, where they can directly interact with immune cells. Macrophages produce inflammatory cytokines primarily in response to the activation of TLR2 and TLR4. Dendritic cells, upon stimulation by BEVs, enhance the release of IL-10, IFN-γ, and TNF-α. Neutrophils secrete proinflammatory cytokines such as IL-1β, MIP-1α, and MIP-1β. BEVs also promote pyroptosis and inhibit the chemotaxis and phagocytosis of immune cells. AMP, antimicrobial peptide; HBD, human β-defensin; MIP, macrophage inflammatory protein; IFN, interferon; IL, interleukin; LPS, lipopolysaccharide; NF-κB, nuclear factor-κB; TNF, tumor necrosis factor.
3.1. The entry of BEVs into mammalian host cells
3.1.1. The entry modes of BEVs into host cells
The entry of gram-negative bacterial OMVs into host cells via multiple pathways has been demonstrated, allowing their cargo to be detected by PRRs, and subsequently activating a series of signaling pathways. In phagocytic cells, phagocytosis is the major route to internalize OMVs (O'Donoghue and Krachler, 2016). In nonphagocytic cells, other endocytic routes affect the entry of OMVs, namely, clathrin-, caveolin-and lipid raft-mediated endocytosis and macropinocytosis, direct membrane fusion, and receptor-mediated uptake (O'Donoghue and Krachler, 2016). The biophysical properties inherent to OMVs including the surface lipid phase and membrane curvature might enable them to enter or fuse with early endosomes and then disintegrate and release their contents into the cytoplasm (Mulcahy et al., 2014; Behrens et al., 2021). Instead, early endosomes can transform into late endosomes and fuse with lysosomes, causing degradation of BEVs (Mulcahy et al., 2014). Subsequently, BEV contents released into the cytosolic space can activate signaling pathways and induce pro−/anti-inflammatory responses (O'Donoghue and Krachler, 2016).
There are relatively few studies on the internalization pathways of MVs derived from gram-positive bacteria. Recent studies have found that MVs enter host cells mainly via clathrin-dependent endocytosis, dynamin-dependent endocytosis, and membrane fusion (Bajic et al., 2020; Wang et al., 2020).
3.1.2. Factors that influence BEV uptake
Several factors seem to influence the mode and rate of BEV uptake, such as the size and composition of BEVs. For instance, smaller BEVs (20–100 nm) preferentially enter recipient cells via caveolin-mediated endocytosis, clathrin-mediated endocytosis can be utilized by BEVs with diameters ranging from 20 to 250 nm, and macropinocytosis appears to be effective for larger BEVs (90–450 nm). Clathrin-mediated endocytosis can be utilized by BEVs with diameters ranging from 20 to 250 nm (Weiner et al., 2016; Zhang et al., 2019). Toxins can serve as BEV adhesins and allow their internalization via ligand-receptor interactions. Additionally, BEVs could utilize complementary mechanisms to promote their entry. For example, the uptake of OMVs with O antigen is lipid raft-dependent, while OMVs lacking O antigen alternatively select clathrin-mediated endocytosis (O'Donoghue et al., 2017).
3.1.3. BEV uptake conclusion
Bacterial extracellular vesicles enter host cells with multiple uptake mechanisms among vesicles from different species of bacteria and even among that from the same bacterium. The quantifiable and dynamical assay of uptake pathways will be important in the illustration of bioprocesses that underlie the bacteria-host interactions, but also in the design of BEV-engineered delivery vectors and improvement of their treatment efficiency based on their entry into target cells.
3.2. Inflammatory responses triggered by BEVs
3.2.1. BEVs are sensed by PRRs on the cell membrane
PRRs present on the surface of immune cells can sense microbial-associated molecular patterns (MAMPs)/PAMPs carried by BEVs, activate signaling pathways, promote the release of proinflammatory cytokines, and trigger inflammatory responses (Cecil et al., 2017). For instance, Toll-like receptors (TLRs) of microglia and macrophages can recognize LPS, lipoproteins, flagellin and DNA carried by OMVs to release cytokines such as TNF-α and IL-10 (Balhuizen et al., 2022; Liu et al., 2022). BEVs can also be sensed by nonimmune cells. LPS carried by Pseudomonas aeruginosa OMVs triggers the immune response in epithelial cells through the MyD88-dependent TLR4 signaling pathway and promotes the expression of IL-8 in lung epithelial cells (Vitse and Devreese, 2020). DNA, RNA and peptidoglycan cargo in Staphylococcus aureus MVs activated several TLRs and nucleotide-binding oligomerization domain (NOD) 2 signaling and promoted cytokine and chemokine release by epithelial cells (Bitto et al., 2021). Upon stimulation by BEVs, cell surface PRRs also modulate antimicrobial peptide secretion, as evidenced by vesicles derived from Helicobacter pylori, P. aeruginosa, Neisseria gonorrhoeae and C. jejuni that induced the production of human β-defensins (hBD2, hBD3) and LL-37 by human gastric epithelial cells (Elmi et al., 2012). These in vitro studies revealed some of the mechanisms underlying bacteria-host interactions, whereas in the context of in vivo infections, we need to further clarify the mechanisms by which host cells detect BEVs to trigger immune responses.
3.2.2. BEVs are sensed by PRRs in the cytoplasm
Although most studies have reported that BEVs activate PRRs on the cell surface, PAMPs carried by BEVs can also be perceived and recognized by PRRs in the cytoplasm of host cells, thereby activating intracellular innate immunity and promoting the assemble assembly of inflammasomes (Vanaja et al., 2016).
3.2.2.1. Canonical inflammasome activation
Currently, four types of inflammasomes have been reported, namely, NLRP1, NLRP3, NLRC4, and AIM2, which eventually activate caspase-1 and induce the production of proinflammatory cytokines (Elizagaray et al., 2020; Johnston et al., 2021). The caspase-1-dependent process is called canonical inflammasome activation. Studies have shown that microbial DNA and flagellin carried by OMVs can activate inflammasome signaling in macrophages, as well as in in vivo models, inducing caspase-1-mediated pyroptosis and TNF-α, IL-1β, and IL-18 secretion (Elizagaray et al., 2020; Yang et al., 2020). Likewise, MVs produced by gram-positive bacteria delivering nucleic acids and peptidoglycan to epithelial cells can activate the NLRP3 inflammasome and caspase-1 and induce IL-1β and IL-18 production in macrophages (Wang et al., 2020).
3.2.2.2. Noncanonical inflammasome activation
The activation of the noncanonical inflammasome depends on caspase-11 (mice) or caspase 4/5 (human) (Elizagaray et al., 2020). OMVs transport LPS into host cells and activate caspase-11 via guanylate-binding proteins (Santos et al., 2018). Active caspase-11 enhances gasdermin D pore formation in the cell membrane of macrophages, causing NLRP3 inflammasome-mediated pyroptosis (Kayagaki et al., 2015; Vanaja et al., 2016). In human monocytes, P. aeruginosa OMVs activated noncanonical inflammasomes in a caspase-5-dependent manner (Bitto et al., 2018).
3.2.3. Conclusions on BEV PRRs
Bacterial extracellular vesicles are potent activators of PRRs in charge of regulating inflammatory responses that are correlated with pathogenesis in systemic diseases (Tiku and Tan, 2021). Additionally, pathogens release vesicles during infections to deliver virulence factors and evade immune defenses, whereas EVs from probiotics may exert a protective effect on LPS-mediated inflammation in the host (Hu et al., 2021). Thus, the balance between proinflammatory and anti-inflammatory signaling generated by PRRs upon BEV activation is crucial to understanding host–microbe interactions. Moreover, the functions of PRRs in complex disease conditions deserve in-depth studies to facilitate the design of PRR antagonists to restrict BEV-mediated inflammation in systemic diseases.
4. Physiological and pathological roles of BEVs in specific systems and diseases
In contrast to their parent bacteria, BEVs carry a higher concentration of virulence factors and insulate them during delivery to different organs and vascular-based tissue targets. These properties allow BEVs to travel long distances and access tissues that their parent bacteria cannot reach, strengthening the pathogenic functions of bacteria in both the local microenvironment and distant parts of the body and leading to the occurrence of Alzheimer’s disease (AD), metabolic diseases, cardiovascular disease (CVD), osteoporosis, etc.
4.1. Nervous system
4.1.1. BEV-related neurologic disorders
Recent research have revealed the role of microbiome on neuropsychiatric disorders (Jiang et al., 2015). Individuals suffering from stress response and depressive disorder tend to have lower abundance of beneficial intestinal bacteria with their functional impairment (Aizawa et al., 2016). EVs derived from microbiome cargo a range of bioactive compounds from bacteria to affect the central nervous system function. BEVs can enter the bloodstream and permeate the blood–brain barrier (BBB) to reach the brain, subsequently affecting the regulation of various signal transduction pathways and resulting in neurologic abnormalities (e.g., dementia, AD) (Han et al., 2019; Bittel et al., 2021; Xie et al., 2023; Figure 3). BEVs can compromise the integrity of tight junctions, the disruption of which facilitates the paracellular and/or transcellular pathways of endothelial cells and promotes the delivery of BEV contents to the circulation, as well as the vagus nerve (Stentz et al., 2018; Lee et al., 2020). Campylobacter jejuni OMVs have been reported to cleave occludin and E-cadherin, promoting intestinal penetrability and paracellular pathways (Elmi et al., 2016). Likewise, periodontal pathogen-derived EVs enriched in gingipains, LPS and small extracellular RNAs (exRNAs) can disrupt the tight junction zona occludens protein (ZO-1) in human brain microvascular endothelial cells and cross the BBB (Han et al., 2019; Pritchard et al., 2022).
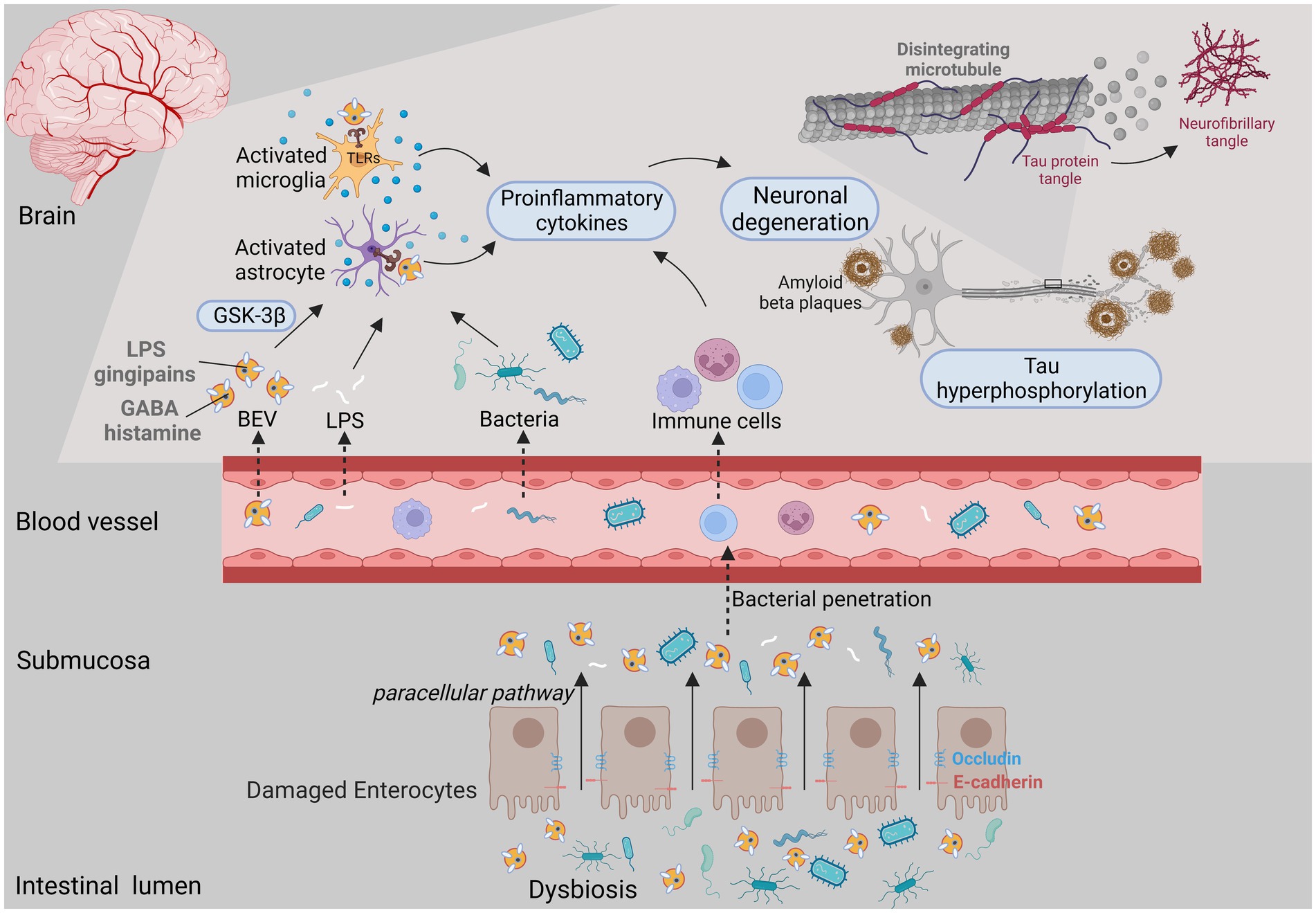
Figure 3. BEVs released under microecology dysbiosis could result in brain disorders. The intestinal epithelium is destroyed by both bacterial activity and the host immune response, which facilitates the penetrability and delivery of BEV cargo (e.g., LPS, gingipains, histamine and peptidoglycan) from the gut lumen to the circulation. When BEVs access the central nervous system, they potentially activate immune cells such as astrocytes and microglia through PRRs, thereby promoting proinflammatory cytokine secretion and neuronal damage and leading to neurological disorders.
When BEVs access the central nervous system, they not only affect the reactivity of glial cells to facilitate neuroinflammation, but also exacerbate neuronal dysfunction and tau hyperphosphorylation, accelerating cognitive decline (Cheon and Lee, 2021; Park and Tsunoda, 2022). Gingipain-positive P. gingivalis OMVs can reach the cerebral ventricle, promote intracerebral inflammation via complement activation, trigger the NLRP3 inflammasome, and increase the expression of amyloid beta (Aβ) and neurofibrillary tangles (Han et al., 2019; Gong et al., 2022; Yoshida et al., 2022). Likewise, H. pylori OMVs led to the activation and migration of microglia and astrocytes, which induced neuronal damage via complement component 3 (C3)-C3a receptor (C3aR) signaling, ultimately leading to aggravated Aβ pathology (Park and Tsunoda, 2022; Xie et al., 2023). In vitro experiments have shown that exRNAs delivered via BEVs increased the expression of IL-6 in brain monocytes/microglia by activating the NF-κB pathway (Han et al., 2019; Ha et al., 2020). BEVs are also able to transport their cargo such as neurotransmitters [e.g., histamine and gamma-amino-butyric acid (GABA)], from the gut to the brain, suggesting their potential effects on brain functions (Zakharzhevskaya et al., 2017; Bittel et al., 2021). Furthermore, oral gavage of EVs derived from Paenalcaligenes hominis reduced brain-derived neurotrophic factor (BDNF) expression in hippocampal neurons while increasing IL-1β expression in the blood (Lee et al., 2020).
Therefore, it could be speculated that the export of BEVs to the brain may contribute to infection at any place in the body, which could result in immune responses in the brain and related neurological disorders.
4.1.2. BEVs and psychiatric disease therapy
Emerging evidence confirms that pathogenic BEVs exert harmful effects on the brain function, whereas probiotic BEVs show beneficial effects on peripheral tissues. After the induction of depression symptoms by glucocorticoid (GC) treatment, Lactobacillus plantarum-derived EVs enhanced the expression of BDNF in the hippocampus and exerted antidepressive-like effects (Choi et al., 2019). In chronic restraint stress (CRS)-treated mice, parenterally injected EVs from L. plantarum, Bacillus subtilis, and A. muciniphila exhibited antidepressant-like effects and reversed stress-induced decreases in the expression of Bdnf, Nt3, and/or Nt4/5 in HT22 cells and in the hippocampus (Choi et al., 2022). Moreover, A. muciniphila EVs increased 5-HT synthesis by increasing Tph2 expression in the brain and produced a stronger effect than the parent bacterium on the reuptake and clearance of serotonin (Yaghoubfar et al., 2020). These results indicate that EVs derived from probiotics may act on neuronal cells, promote the transcription of neurotrophic factors, and produce antidepressant-like effects, which have the potential to be applied to the design of neuropsychiatric treatment such as drug delivery vehicles and vaccines.
4.2. Digestive system
4.2.1. Oral cavity
4.2.1.1. Streptococcus mutans MVs and caries
Since S. mutans MVs can disseminate over long distances, their local exploitation of nutrient substances such as sucrose to produce extracellular polysaccharides (EPS) on the hydroxyapatite surface could facilitate bacterial colonization and biofilm formation associated with cariogenicity (Nakamura et al., 2020). S. mutans MVs have been found to package metabolic enzymes associated with carbohydrate metabolism, such as glucosyltransferase (Gtf), glucan-binding proteins and dextranase (DexA) (Cao et al., 2020). Moreover, S. mutans MVs containing Gtfs increase EPS formation in C. albicans biofilms, and genes of C. albicans related to mannan and glucan synthesis increased upon exposure to S. mutans MVs, indicating that S. mutans MVs facilitate cariogenic bacterial carbohydrate metabolism (Wu et al., 2020).
MVs also promote the cariogenic ability of bacteria even at low pH values. Intriguingly, the initial pH value affects various characteristics of S. mutans MVs, including biofilm quantity (Nakamura et al., 2020; Iwabuchi et al., 2021). Under low pH conditions, S. mutans released more MVs to deliver proteins related to cariogenesis, and several important enzymes carried by MVs, such as the shock heat proteins, lactate dehydrogenase, DexA and Gtfs, still possessed enzyme activity (Cao et al., 2020). These may be new mechanisms of MV biogenesis and could underlie the acid resistance of S. mutans; furthermore, these data are helpful to develop biofilm formation inhibitors targeting BEVs to prevent dental caries.
4.2.1.2. Periodontitis
Once released, periodontopathogen-derived EVs, enriched in virulence factors such as muramic acid, LPS, fimbriae, dentilisin, outer membrane proteins and gingipains, may act as representatives of parent bacteria to communicate with other oral bacteria and host cells and adhere to the tooth surface (Inagaki et al., 2006). For instance, P. gingivalis EVs alone can promote the aggregation of a broad range of Streptococcus spp., Fusobacterium nucleatum, Treponema denticola, Actinomyces viscosus, Actinomyces naeslundii, and Lachnoanaerobaculum saburreum in oral biofilms (Hiratsuka et al., 1992; Kamaguchi et al., 2003; Inagaki et al., 2006; Grenier, 2013). P. gingivalis EVs aggregate other oral bacteria primarily depending on OMV-related gingipain proteases (Ito et al., 2010). Additionally, other species present in oral biofilms, such as T. forsythia, can also release OMVs related to biofilm formation (Friedrich et al., 2015). BEVs also protect other organisms from complement activities to accelerate the progression of periodontitis. Consistent with this, Actinobacillus actinomycetes EVs can serve as decoys to activate complement in an LPS-dependent manner and deplete complement to defend against serum-susceptible bacteria (Lindholm et al., 2020). Moreover, P. gingivalis OMVs induced selective TNF deficiency that suppressed microbial recognition by macrophages/monocytes (Waller et al., 2016). Apart from escaping the surveillance of innate immune cells, BEVs also evade adaptive immune cells. For instance, small RNAs carried by OMVs derived from A. actinomycetemcomitans, P. gingivalis, and T. denticola inhibited the release of IL-13 and IL-5 by Jurkat T cells (Choi et al., 2017). This evidence indicates the contribution of periodontal pathogenic EVs to bacterial survival and aggregation, favoring the pathogenic process of periodontitis.
Bacterial extracellular vesicles can activate the first guard against bacterial infections, the oral mucosal epithelium, in multiple ways. P. gingivalis EVs can be internalized into epithelial and endothelial cells via lipid raft-mediated endocytosis and facilitate the invasion of other pathogens, such as Tannerella forsythia (Furuta et al., 2009). After invasion, BEVs inhibit oral epithelial migration and proliferation, leading to cell dysfunction in periodontal tissues (Furuta et al., 2009). For example, P. gingivalis OMVs lead to apoptosis after their uptake by human periodontal ligament cells and cause pyroptosis by activating inflammasomes both in vitro and in vivo (Cecil et al., 2017; Fan et al., 2023).
After their evasion of the oral epithelial barrier, BEVs enter submucosal tissues, where they interact directly with host innate or adaptive immune cells. OMVs released from P. gingivalis, T. forsythia and T. denticola, activate PRRs on macrophages and monocytes, and increase the production of TNF-α, IL-1β, and IL-8 (Cecil et al., 2016). Similarly, OMVs from A. actinomycetemcomitans activated NOD1-dependent nuclear factor kappa-B (NF-κB) in monocytes (Thay et al., 2014). OMVs from F. nucleatum also facilitated the differentiation of macrophages toward the proinflammatory phenotype (Chen et al., 2022). Moreover, OMVs may be a second route through which neutrophils in the oral cavity may encounter bacterial virulence factors and hinder neutrophil chemotaxis and phagocytosis (Jones et al., 2019). It is possible that the inflammatory milieu induced by BEVs can further exacerbate their toxicity to gingival fibroblasts and periodontal tissue destruction.
Bacterial extracellular vesicles can deliver toxic payloads to susceptible cells in the periodontium and aggravate alveolar bone loss, thereby causing periodontal tissue destruction. A. actinomycetemcomitans OMVs were found to promote damage in the sulcular/junctional epithelium via the delivery of cytolethal distending toxin into human gingival fibroblasts (Rompikuntal et al., 2012). A recent study reported that EVs from both oral commensal bacteria and periodontal pathogens can provoke osteoclastogenic activity through TLR2 activation (Kim et al., 2022). Intracellular delivery of prostaglandin (PG) via A. actinomycetemcomitans OMVs could directly trigger alveolar bone loss (Jiao et al., 2013). Likewise, F. nucleatum BEVs increased osteoclast numbers, and inflammatory factor (IL-1β, IL-6, and TNF-α) production, and accelerated periodontal bone loss in a periodontitis mouse model (Chen et al., 2022).
In conclusion, periodontopathogen-derived vesicles can activate or degrade bioactive substances in host cells, hinder cell proliferation, facilitate cell death, and induce inflammatory cytokine release, thereby promoting the establishment of an inflammatory microenvironment in periodontal tissues and subsequent alveolar bone destruction.
4.2.2. Liver
4.2.2.1. BEVs and diabetes mellitus
Bacterial extracellular vesicles have been recently considered a critical mediator facilitating the pathogenic process of the endocrine system disease type 2 diabetes mellitus (T2DM), and they can also be applied to the diagnosis and treatment of T2DM and its complications (Figure 4). A significantly higher concentration of BEVs was observed in patients with T2DM than in the healthy population among stool, serum, and urine (Nah et al., 2019). In diabetes animal model, intestinal microbiota-derived OMVs are also increased (Chen et al., 2023). Furthermore, gut microbe-derived EVs were reported to permeate the intestinal barrier and enter the bloodstream followed by distribution to distant metabolic organs (e.g., adipose tissue, liver, and skeletal muscle), where they trigger insulin resistance and damage glucose metabolism (Choi et al., 2015; Nah et al., 2019; Bittel et al., 2021). For instance, P. panacis OMVs can block insulin signaling in adipose and skeletal tissue, and induce a diabetic phenotype in mice (Choi et al., 2015). Gingipain-positive cells were found in the liver sinuses of mice injected with P. gingivalis OMVs, suggesting that the hepatic cells were exposed to gingipains delivered by OMVs (Seyama et al., 2020). In these mice, gingipains in P. gingivalis OMVs weakened glycogen synthesis and insulin sensitivity through the activated protein kinase B (Akt)/glycogen synthase kinase-3 beta (GSK-3β) signaling pathways (Nakayama et al., 2015; Seyama et al., 2020). Moreover, obese BEVs enriched in microbial DNA notably lowered the number of liver CRIg+ and islet Vsig4+ macrophages, causing the dissemination of BEVs to insulin-responsive tissues and subsequently aggravating the inflammation and insulin resistance of hepatocytes through the activation of cGAS/STING signaling (Luo et al., 2021; Gao et al., 2022). These studies emphasized that BEVs either from the oral or intestinal microbiota, as participants in insulin resistance, are correlated with obesity and an increased incidence of T2DM.
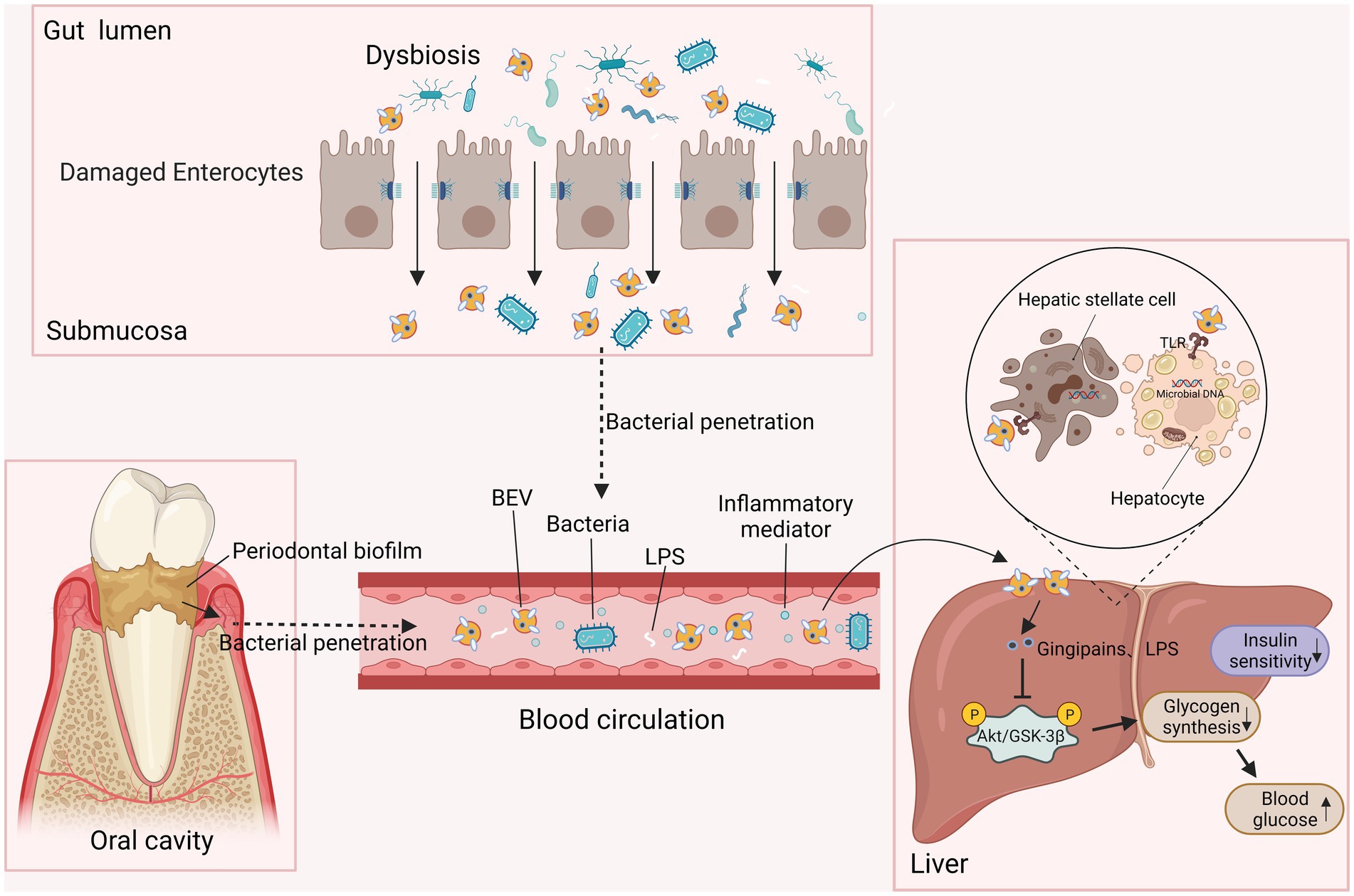
Figure 4. BEVs participate in insulin resistance and result in T2DM. EVs from periodontal biofilms and dysfunctional intestinal microbiota deliver toxins such as gingipains and LPS to the liver through the circulation. When they access the liver, BEVs induce insulin resistance in hepatic stellate cells and hepatocytes by inhibiting AKT/GSK-3β signaling, decreasing hepatic glycogen synthesis, and ultimately increasing the level of blood glucose.
4.2.2.2. Probiotic-derived EVs improve metabolic function
Detrimental BEV characteristics are counterbalanced with their beneficial characteristics under physiological and pathological circumstances given that probiotic-derived EVs prevent adverse processes that induce obesity-related diseases. For instance, Akkermansia muciniphila-derived EVs enhanced the expression of tight junction proteins in Caco-2 cells and eventually increased gut barrier integrity in an HFD-induced diabetic model through AMPK activation (Ashrafian et al., 2021). Treatment with A. muciniphila OMVs markedly ameliorated lipid metabolism and reduced inflammatory cytokine release in adipose tissues (Ashrafian et al., 2019). A. muciniphila and its OMVs could also regulate energy balance and improve blood parameters, such as lipid profiles and glucose levels (Ashrafian et al., 2019). The aforementioned data indicate that probiotic-derived EVs can potentially be used to improve intestinal penetrability and metabolic functions such as glucose and lipid metabolism, while more investigation related to the underlying mechanism is needed to treat obesity-related diseases.
4.2.2.3. BEVs and nonalcoholic fatty liver disease
BEVs may be involved in several mechanisms, such as intestinal barrier disruption and systemic inflammation, associated with the onset and progression toward nonalcoholic fatty liver disease (NAFLD) or nonalcoholic steatohepatitis (NASH)-related liver abnormalities. Intragastrically administered feces-derived EVs (fEVs) entered the liver and increased proinflammatory cytokines and chemokines from hepatic sinusoidal endothelial cells via TLR4 action through LPS and activated profibrotic and proinflammatory protein production in hepatic stellate cells (Fizanne et al., 2023). Likewise, LPS-positive P. gingivalis EVs provoked Kupffer cell (KC) activation through TLR4 and subsequent liver inflammation, glycogen synthesis reduction and progression toward steatohepatitis (Miura et al., 2010). H. pylori OMVs increased the level of liver fibrosis markers in hepatocytes, and exosomes derived from OMV-treated cells activated hepatic stellate cells (HSCs) and induced liver fibrosis (Zahmatkesh et al., 2022). Furthermore, the accumulation of microbial DNA may be a mechanism involved in NAFLD progression. Intestinal BEV translocation promoted bacterial DNA accumulation in HSCs and hepatocytes, which induced hepatocyte inflammation and HSC fibrosis via the activation of cGAS/STING (Luo et al., 2022). Collectively, BEVs may be a critical moiety in the pathogenesis of NAFLD by facilitating liver inflammation and hepatic steatosis and fibrosis by delivering toxic payloads into liver cells.
4.2.2.4. The antifibrotic effects of BEVs
Probiotic-derived vesicles exhibit beneficial effects on the prevention of liver inflammation and liver fibrosis. Research has shown that A. muciniphila EVs could efficiently enhance the regression of activated HSCs (Keshavarz Azizi Raftar et al., 2021). In the HFD/carbon tetrachloride-induced liver injury model, A. muciniphila OMV treatment substantially attenuated fibrosis and inflammatory biomarkers and ameliorated liver and colon damage (Keshavarz Azizi Raftar et al., 2021). Therefore, EVs from probiotics may have anti-inflammatory and antifibrotic effects and protect against liver injury.
4.2.3. Gut
4.2.3.1. BEVs and inflammatory bowel disease
Bacterial extracellular vesicles exhibit regulatory effects on intestinal immunity and homeostasis, as evidenced by stool BEVs from an IBD mouse model showing severe dysbiosis compared to that of the normal controls (Kang et al., 2013). BEVs were also implicated in barrier damage in IBD, HIV and cancer therapy-induced intestinal mucositis, leading to an intestinal and systemic inflammatory environment in these diseases (Liu et al., 2021).
Bacterial extracellular vesicles released into the intestinal lumen can pass through the mucus layer and interact with intestinal epithelial cells and immune cells, regulating immunomodulation and corresponding signaling pathways in the pathogenesis of IBD (Gul et al., 2022). In colonic epithelial cells and human colonoid (organoid) monolayers, F. nucleatum-derived OMVs activate TLR4, leading to inflammatory cytokine release (Engevik et al., 2021). Studies that focused on the effects of BEVs on the mucosal immune system showed that the uptake of B. fragilis OMVs by dendritic cells (DCs) induced regulatory T cells (Tregs), and Bacteroides thetaiotaomicron OMVs also exerted an effect on T-cell functions (Chu et al., 2016; Wegorzewska et al., 2019). Likewise, OMVs from specific strains of E. coli activated DCs and derived CD4+ T-cell responses (Diaz-Garrido et al., 2022). In patients with UC and CD, a lack of regulatory IL-10 response by DCs to B. thetaiotaomicron OMVs was observed (Durant et al., 2020). Efficient BEV internalization by mucosal phagocytic cells both in vitro and in vivo occurred and pronounced BEV-induced inflammatory responses in these macrophages were observed (Bittel et al., 2021). The regulation of immunomodulatory miRNAs by BEVs may partly underlie several specific effects (Díaz-Garrido et al., 2020). For instance, B. thetaiotaomicron EVs have been found to harbor microbial helicases specifically targeting the human polymerase protein PAPD5, a negative regulator of miR-21, the targets of which are genes that participate in immune responses and the pathogenesis of IBD (Gul et al., 2022).
In general, these studies illustrate that gut microbe-derived EVs distributed in serum or other tissues could be an effective marker for intestinal integrity and could promote inflammation in the gut and even in distant organs through the leaky intestinal barrier (Nah et al., 2019). Further research is needed to elucidate the systemic functions of circulating BEVs and to determine and associate their taxonomy with the metabolic activity of the gut microbiota.
4.2.3.2. BEVs and intestinal viral infection
The significance of BEVs is further consolidated by the capability of enteric viruses to utilize these vesicles to facilitate viral infection. EVs from commensal Enterobacter cloacae, B. thetaiotaomicron, and Lactobacillus acidophilus cross the intestinal epithelium and enter the lamina propria in which the prime targets of acute norovirus infection, namely, immune cells, reside (Mosby et al., 2022, 2023). Similarly, P. gingivalis OMVs promoted HIV translocation from mucosal surfaces to subcutaneous tissues and reached HIV permissive cells, such as DCs and T cells, and nonpermissive cells, such as human oral keratinocytes; this may also serve as a mechanism for cell-free HIV transcytosis through the intestine (Dong et al., 2018). Moreover, virus interaction with commensal bacteria changes the size, yield, cargo and content of BEVs, suggesting that viral binding may alter the mechanism of BEV biogenesis (Mosby et al., 2022, 2023). Therefore, BEVs potentially offer a mechanistic basis for the bacterial promotion of viral infection by facilitating virus entry into target cells and regulating host immune responses; meanwhile, the relevant mechanism deserves further exploration for a better understanding of bacteria-virus infections and to develop beneficial therapeutic strategies.
4.2.3.3. BEVs and cancer development
Several studies have demonstrated that BEVs can penetrate the intestinal epithelial barrier, selectively accumulate near intestinal tumor cells, change the tumor microenvironment (TME), and participate in the progression of gastrointestinal cancer. H. pylori-derived OMVs were upregulated in the gastric juice of gastric cancer patients compared to healthy controls, and could penetrate and remain in the mouse stomach for an extended period of time (Choi et al., 2017). Intravenous injection of E. coli OMVs specifically accumulated near the tumor tissues of BALB/c mice with CT26 tumors (Kim et al., 2017). These vesicles attract T cells and natural killer cells, and induce the production of TNF-α, IL-6, and IL-1β by macrophages and IL-8 by gastric epithelial cells (Choi et al., 2017; Kim et al., 2017). EVs from E. coli could be internalized into the Caco-2 cell line and promote carcinogenesis in intestinal epithelial cells (Tyrer et al., 2014). Likewise, OMVs from E. coli and Vibrio cholerae were involved in enhancing cell differentiation in colon cancer cells (Vdovikova et al., 2018). H. pylori OMVs were found to contain CagA and VacA proteins, which were correlated with the induction of apoptosis in the adenocarcinoma gastric cell line (AGS) and an increase in ATP affinity to H1 histone proteins in chromosomes (Turkina et al., 2015). Moreover, BEVs can increase the release of proinflammatory cytokines and activate a series of abnormal signaling pathways, leading to the occurrence of cancer (Choi et al., 2017). Therefore, BEVs can not only access the TME efficiently but also alter the TME by producing or inducing the release of oncogenic metabolites. Furthermore, the composition of intestinal microbe-derived EVs in colorectal cancer exhibited discrepancies compared to that of healthy controls, indicating that BEVs may be harnessed as a marker for detecting cancer and predicting cancer prognosis (Park et al., 2021).
4.2.3.4. BEVs help maintain intestinal homeostasis
Bacterial extracellular vesicles released by probiotic and commensal bacteria have been indicated to activate the immune system and maintain gut homeostasis in multiple ways (Table 2). BEVs can regulate the interaction with host cells by regulating PRRs. For instance, OMVs derived from B. fragilis modified the gene expression of TLR2 and TLR4 in epithelial cells and increased the secretion of IL-10 by CD4+ T cells (Ahmadi Badi et al., 2019). DCs sense OMV-associated polysaccharides through TLR2, resulting in an increase in Tregs and anti-inflammatory cytokine production (Shen et al., 2012). Furthermore, epithelial cells could sense OMVs derived from commensal E. coli strains ECOR12 and Nissle 1917 in a NOD1-dependent manner and regulate cytokine production (Cañas et al., 2018).
In mouse models, EVs from Bifidobacterium longum and Bifidobacterium bifidum dampened allergy-related diarrhea by inducing mast cell apoptosis and Treg production, respectively (López et al., 2012; Kim et al., 2016). Additionally, oral treatment with MVs from Lactobacillus rhamnosus promoted the expression of IL-10 and heme oxygenase-1 in bone marrow-derived DCs and then triggered Tregs in Peyer’s patches and mouse mesenteric lymph nodes (Al-Nedawi et al., 2015). Likewise, B. thetaiotaomicron OMVs mediated monocyte activation and IL-10 production through TLR2 activation and alleviated acute intestinal inflammation in dextran sodium sulfate (DSS)-treated mice (Fonseca et al., 2022). Bacteroides vulgatus and B. fragilis OMVs have also been reported to elicit a tolerogenic phenotype in DCs and enhance Treg production, respectively (Shen et al., 2012; Maerz et al., 2018). These studies indicate the potential utilization of BEVs to reinduce tolerance and rebuild immune homeostasis in IBD.
Regarding the protective effects on restoring the integrity of the physicochemical barrier, OMVs released by E. coli Nissle 1917 could reduce inflammation in DSS-treated mice and increase IL-22 in colonic explants (Alvarez et al., 2016; Fábrega et al., 2017). A. muciniphila OMVs also decreased inflammatory cell recruitment to the colon wall in DSS-induced colitis and restored epithelial stability by promoting the expression of tight junctions and mucus in epithelial cells (Kang et al., 2013; Wang et al., 2023). In 2,4,6-trinitrobenzenesulfonic acid (TNBS)-induced IBD, MVs from several Lactobacillus species, namely, kefir, kefirgranum, and kefiranofaceins, were demonstrated to reduce the release of proinflammatory cytokines (Seo et al., 2018). A. muciniphila OMVs also suppressed HFD-induced colonic inflammation, increased AMPK phosphorylation and prevented LPS-induced intestinal barrier damage (Chelakkot et al., 2018; Ashrafian et al., 2021). Moreover, MVs from Lactobacillus sakei and A. muciniphila promoted the production of IgA in the intestine and improved epithelial barrier function (Yamasaki-Yashiki et al., 2019; Wang et al., 2023). Furthermore, BEV-mediated modulation of the intestinal microbiota might involve selective cross-talk with specific commensal species, as indicated by A. muciniphila OMV-mediated increase in the abundance of beneficial commensal Firmicutes and Bacteroidetes and decrease in the abundance of potentially pathogenic taxa in the phylum Proteobacteria (Wang et al., 2023).
Collectively, the multifunctional role of BEVs in modulating intestinal homeostasis may occur through reciprocal and complementary mechanisms that regulate mucosal immunity, physicochemical barriers, and the gut microbiota. Thus, enteric microbiota-derived EVs may provide insight into therapeutic strategies against diseases implicated in inflammation and barrier dysfunction, such as T2DM.
4.3. Circulatory system
Bacterial extracellular vesicle is a hazard factor for CVD and coronary heart diseases such as atherosclerosis, among which endothelial dysfunction and calcium deposition play a key role in the development of atherosclerosis. Nanoscale BEVs can lead to proteolytic damage in blood vessels that cannot be accessed by bacteria, making them analogous to parent bacteria in the pathogenesis of CVD (Farrugia et al., 2020).
Studies have shown the role that BEVs play in causing endothelial injury to promote vascular permeability and cause disease phenotypes both in vitro and in vivo (Zhang et al., 2020). P. gingivalis OMVs can increase vascular permeability probably through proteolytic cleavage of endothelial cell–cell adhesins such as PECAM-1 (Farrugia et al., 2020). P. gingivalis OMVs can also activate Rho kinase (ROCK) in human umbilical vein endothelial cells, causing endothelial dysfunction (Jia et al., 2015). In addition, stimulation with OMVs from CagA-enriched H. pylori facilitated atherosclerotic plaque formation via endothelium injury in vivo and promoted apoptosis in human umbilical vein endothelial cells (Wang et al., 2021). Additionally, P. gingivalis OMVs induced the calcification of vascular smooth muscle cells by activating the ERK1/2-RUNX2 pathway (Miyakawa et al., 2004; Yang et al., 2016).
Bacterial extracellular vesicles facilitate cardiac tissue inflammation to cause related diseases. A recent study revealed that gut BEVs containing microbial DNA led to obesity-associated adrenomedullary inflammation and catecholamine production (Gao et al., 2022). Additionally, EVs from a uropathogenic E. coli strain exerted a direct effect on cardiomyocytes and induced cardiac tissue inflammation and injury (Svennerholm et al., 2017).
These studies highlight the idea that the entry of BEVs into circulation potentially initiates atherosclerosis and cardiac tissue inflammation and may contribute to the disruption of the vascular system, resulting in the occurrence of CVD.
4.4. Respiratory system
The existence of BEVs in the lungs of patients with severe pulmonary infections and the fact that BEVs can transport virulence factors may indicate their role in the process of infection (Bomberger et al., 2009). The mechanism mediated by BEVs may inhibit the host’s immune response to bacteria. For example, the delivery of Cif through P. aeruginosa OMVs to the cytoplasm of host cells hampered CTRF chloride production and thus dampened the ability to clear respiratory pathogens through mucus cilia (Bomberger et al., 2011). P. aeruginosa OMVs also evade the host immune response by altering DNA methylation in human lung macrophages (Kyung Lee et al., 2021). Streptococcus pneumoniae MVs delivered vesicle-associated proteins into human monocyte-derived dendritic cells, induced proinflammatory cytokines, and exposed targets for complement factors in serum, thereby promoting pneumococcal evasion of humoral host defense (Codemo et al., 2018).
It has been recently shown that the majority of host proinflammatory responses induced by PAMPs are mediated by BEVs. MVs produced by S. aureus also fuse in a cholesterol-dependent manner with the plasma membrane of host cells, causing the delivery of α-hemolysin (HIa), which can trigger apoptosis in T-lymphocytes (Thay et al., 2013). Klebsiella pneumoniae OMVs increased the proinflammatory cytokines IL-1β, IL-8 and TNF-α in human epithelial cells, mast cells and macrophages (You et al., 2019). Likewise, OMVs secreted by respiratory pathogens induced a strong proinflammatory response in immature THP-1 macrophages (Volgers et al., 2017). L. pneumophila OMVs can activate macrophages via TLR2 and cause tissue damage in human lung tissue explants (Jäger et al., 2015; Jung et al., 2016). Furthermore, peptidoglycan-containing OMVs were internalized into epithelial cells via lipid rafts to trigger NOD1-dependent responses both in vitro and in vivo (Kaparakis et al., 2010). Additionally, intratracheal exposure to K. pneumoniae OMVs caused severe lung pathology in neutropenic mice similar to bacterial infection (Lee et al., 2012). OMVs from P. aeruginosa and A. baumannii provoked pulmonary inflammation in vivo, partly modulated by the TLR2 and TLR4 pathways (Park et al., 2013; Marion et al., 2019). Furthermore, BEVs can promote the development of airway hypersensitivity to inhaled allergens. Repeated airway treatment with S. aureus MVs provoked Th1 and Th17 neutrophilic pulmonary inflammation, primarily through TLR2 signaling (Kim et al., 2012).
In addition, BEVs can promote bacterial colonization in the respiratory tract and the maintenance of biofilms. For instance, EVs from several common respiratory pathogens including, Haemophilus influenzae, M. catarrhalis, S. pneumoniae, and P. aeruginosa, promoted the adherence and aggregation of intracellular bacteria (Volgers et al., 2017). The changes induced by P. aeruginosa OMVs resulted in an increase in the Psl/biomass ratio in the early biofilm matrix, which helped to protect growing colonies from the harmful effects of antimicrobial agents (Esoda and Kuehn, 2019). Additionally, C. albicans biofilm EVs participated in matrix polysaccharide formation and decreased sensitivity to the antifungal drug fluconazole (Zarnowski et al., 2018). Furthermore, a proteomics study of P. aeruginosa biofilms identified that the proteins related to OMVs consist of more than 20% of the total matrix proteome (Couto et al., 2015). Many proteins associated with virulence are exclusively secreted via L. pneumophila OMVs, such as intracellular survival and replication (ProA1), invasion (IcmK), persistence and spreading in the lung (fliC) (Galka et al., 2008). Furthermore, after exposure to OMVs, vitronectin increased both in vivo and in vitro, and the increase in vitronectin in the bronchoalveolar space helped evade complement-mediated clearance (Paulsson et al., 2018).
In summary, by targeting the BEV-related contents involved in the interaction between these vesicles and human lung cells or immune cells, new treatments for pulmonary infections may emerge, such as vaccines or drugs, that protect patients from bacterial invasion.
4.5. Motor system
4.5.1. The osteoclastic effects of BEVs
Studies have revealed that microbes or their released vesicles can induce inflammatory responses to initiate osteoclast activity and dampen osteoblast activity, leading to bone loss. Some citrullinated proteins were confirmed in OMVs from P. gingivalis, which implied a correlation between BEVs and rheumatoid arthritis (RA) (Larsen et al., 2020). Peptidylarginine deiminase (PPAD), which is correlated with the occurrence of RA, was also abundantly present in secreted BEVs (Gabarrini et al., 2018a,b). Moreover, human osteoblasts and synovial cells can internalize Kingella kingae OMVs, and the levels of granulocyte-macrophage colony-stimulating factor (GM-CSF) and IL-6 increase in RA synovial fluid upon interaction with OMVs, promoting signal transduction in infected joints and damaging bone tissues during bacterial infection (Maldonado et al., 2011). BEVs also aggravate joint damage by promoting bacterial evasion. After exposure to P. gingivalis OMVs, S. aureus accumulated in a gingipain-and PPAD-dependent manner, which promoted the uptake of Staphylococcus by human neutrophils and facilitated bacterial entry into the bloodstream (du Teil Espina et al., 2022). Therefore, the role of BEVs could potentially explain why RA patients show higher levels of disease severity or complications such as osteoarticular infection.
To investigate the association between BEVs and osteoporosis, an in vivo model of MAMP-induced inflammatory bone loss in mice was established, and Filifactor alocis EVs triggered systemic bone loss and osteoclastogenesis through TLR2 activation (Song et al., 2020; Kim et al., 2021). These studies provide new insight into the effects of pathogen-derived EVs in systemic bone loss.
4.5.2. The osteoprotective effects of BEVs
In contrast, probiotic-derived vesicles exhibit osteoprotective effects. After oral administration to GC-treated mice, Lactobacillus animalis EVs could access the femoral head and improve trabecular bone microarchitecture (Chen et al., 2022). EVs produced by A. muciniphila and the gut microbiota from children can access and accumulate in bone tissues to ameliorate ovariectomy-induced osteoporotic phenotypes by enhancing osteogenic activity and dampening osteoclast formation (Liu et al., 2021). Likewise, Proteus mirabilis OMVs inhibited osteoclast differentiation and caused mitochondria-dependent apoptosis (Wang et al., 2022). In the same study, treatment with OMVs restored bone loss in experimental osteoporosis and collagen-induced arthritis (Wang et al., 2022). Intriguingly, BEVs counteracted bacteria-mediated osteoclastogenic pathways. For example, K. kingae OMVs decreased osteoclastogenesis in a dose-dependent manner and inhibited proinflammatory cytokine production by infected macrophages (Pesce Viglietti et al., 2021). Therefore, BEVs exhibit advantages in bone health, and these studies offer a mechanistic basis for BEV-mediated osteoprotective functions.
5. Conclusion
In recent decades, our knowledge of the physiological and pathological effects of EVs derived from gram-negative and gram-positive bacteria has improved unprecedentedly. BEVs are now commonly recognized as a delivery system that consolidate bacterial roles in bacterial survival, inflammation and pathogenesis in diverse biological milieu, and bacteria can modulate the biogenesis and content of BEVs in a tailored manner as needed. Moreover, recent advances in BEV science have attempted to address the question of how BEV-host interactions contribute to systemic diseases from different perspectives. To expound the intricate mechanisms underlying the role that BEVs play in infection and anti-infection activities in almost every system, we hope to explore novel therapeutic interventions.
Author contributions
YW: Writing – original draft. XL: Supervision, Writing – review & editing. XX: Writing – review & editing, Visualization. CH: Writing – review & editing, Validation. DM: Writing – review & editing.
Funding
The author(s) declare financial support was received for the research, authorship, and/or publication of this article. This work was supported by National Natural Science Foundation of China (81970930) and Hainan Province Science and Technology Special Fund (ZDYF2022SHFZ017).
Acknowledgments
Figures were created with BioRender software (https://biorender.com/). Space limitations preclude covering all the research in this field, and we sincerely apologize to colleagues whose exceptional work could not be included in this review.
Conflict of interest
The authors declare that the research was conducted in the absence of any commercial or financial relationships that could be construed as a potential conflict of interest.
Publisher’s note
All claims expressed in this article are solely those of the authors and do not necessarily represent those of their affiliated organizations, or those of the publisher, the editors and the reviewers. Any product that may be evaluated in this article, or claim that may be made by its manufacturer, is not guaranteed or endorsed by the publisher.
References
Ahmadi Badi, S., Khatami, S. H., Irani, S. H., and Siadat, S. D. (2019). Induction effects of Bacteroides fragilis derived outer membrane vesicles on toll like receptor 2, toll like receptor 4 genes expression and cytokines concentration in human intestinal epithelial cells. Cell J. 21, 57–61. doi: 10.22074/cellj.2019.5750
Aizawa, E., Tsuji, H., Asahara, T., Takahashi, T., Teraishi, T., Yoshida, S., et al. (2016). Possible association of Bifidobacterium and Lactobacillus in the gut microbiota of patients with major depressive disorder. J. Affect. Disord. 202, 254–257. doi: 10.1016/j.jad.2016.05.038
Al-Nedawi, K., Mian, M. F., Hossain, N., Karimi, K., Mao, Y. K., Forsythe, P., et al. (2015). Gut commensal microvesicles reproduce parent bacterial signals to host immune and enteric nervous systems. FASEB J. 29, 684–695. doi: 10.1096/fj.14-259721
Alvarez, C. S., Badia, J., Bosch, M., Giménez, R., and Baldomà, L. (2016). Outer membrane vesicles and soluble factors released by probiotic Escherichia coli Nissle 1917 and commensal ECOR63 enhance barrier function by regulating expression of tight junction proteins in intestinal epithelial cells. Front. Microbiol. 7:1981. doi: 10.3389/fmicb.2016.01981
Arunmanee, W., Pathania, M., Solovyova, A. S., Le Brun, A. P., Ridley, H., Baslé, A., et al. (2016). Gram-negative trimeric porins have specific LPS binding sites that are essential for porin biogenesis. Proc. Natl. Acad. Sci. U. S. A. 113, E5034–E5043. doi: 10.1073/pnas.1602382113
Ashrafian, F., Keshavarz Azizi Raftar, S., Lari, A., Shahryari, A., Abdollahiyan, S., Moradi, H. R., et al. (2021). Extracellular vesicles and pasteurized cells derived from Akkermansia muciniphila protect against high-fat induced obesity in mice. Microb. Cell Factories 20:219. doi: 10.1186/s12934-021-01709-w
Ashrafian, F., Shahriary, A., Behrouzi, A., Moradi, H. R., Keshavarz Azizi Raftar, S., Lari, A., et al. (2019). Akkermansia muciniphila-derived extracellular vesicles as a mucosal delivery vector for amelioration of obesity in mice. Front. Microbiol. 10:2155. doi: 10.3389/fmicb.2019.02155
Avila-Calderón, E. D., Ruiz-Palma, M. D. S., Aguilera-Arreola, M. G., Velázquez-Guadarrama, N., Ruiz, E. A., Gomez-Lunar, Z., et al. (2021). Outer membrane vesicles of gram-negative Bacteria: An outlook on biogenesis. Front. Microbiol. 12:557902. doi: 10.3389/fmicb.2021.557902
Baidya, A. K., Bhattacharya, S., Dubey, G. P., Mamou, G., and Ben-Yehuda, S. (2018). Bacterial nanotubes: a conduit for intercellular molecular trade. Curr. Opin. Microbiol. 42, 1–6. doi: 10.1016/j.mib.2017.08.006
Bajic, S. S., Cañas, M. A., Tolinacki, M., Badia, J., Sánchez, B., Golic, N., et al. (2020). Proteomic profile of extracellular vesicles released by Lactiplantibacillus plantarum BGAN8 and their internalization by non-polarized HT29 cell line. Sci. Rep. 10:21829. doi: 10.1038/s41598-020-78920-z
Balhuizen, M. D., Versluis, C. M., van Grondelle, M. O., Veldhuizen, E. J. A., and Haagsman, H. P. (2022). Modulation of outer membrane vesicle-based immune responses by cathelicidins. Vaccine 40, 2399–2408. doi: 10.1016/j.vaccine.2022.03.015
Behrens, F., Funk-Hilsdorf, T. C., Kuebler, W. M., and Simmons, S. (2021). Bacterial membrane vesicles in pneumonia: from mediators of virulence to innovative vaccine candidates. Int. J. Mol. Sci. 22:3858 doi: 10.3390/ijms22083858
Bishop, D. G., and Work, E. (1965). An extracellular glycolipid produced by Escherichia coli grown under lysine-limiting conditions. Biochem. J. 96, 567–576. doi: 10.1042/bj0960567
Bittel, M., Reichert, P., Sarfati, I., Dressel, A., Leikam, S., Uderhardt, S., et al. (2021). Visualizing transfer of microbial biomolecules by outer membrane vesicles in microbe-host-communication in vivo. J Extracell Vesicles 10:e12159. doi: 10.1002/jev2.12159
Bitto, N. J., Baker, P. J., Dowling, J. K., Wray-McCann, G., De Paoli, A., Tran, L. S., et al. (2018). Membrane vesicles from Pseudomonas aeruginosa activate the noncanonical inflammasome through caspase-5 in human monocytes. Immunol. Cell Biol. 96, 1120–1130. doi: 10.1111/imcb.12190
Bitto, N. J., Cheng, L., Johnston, E. L., Pathirana, R., Phan, T. K., Poon, I. K. H., et al. (2021). Staphylococcus aureus membrane vesicles contain immunostimulatory DNA, RNA and peptidoglycan that activate innate immune receptors and induce autophagy. J Extracell Vesicles 10:e12080. doi: 10.1002/jev2.12080
Bomberger, J. M., Maceachran, D. P., Coutermarsh, B. A., Ye, S., O'Toole, G. A., and Stanton, B. A. (2009). Long-distance delivery of bacterial virulence factors by Pseudomonas aeruginosa outer membrane vesicles. PLoS Pathog. 5:e1000382. doi: 10.1371/journal.ppat.1000382
Bomberger, J. M., Ye, S., Maceachran, D. P., Koeppen, K., Barnaby, R. L., O'Toole, G. A., et al. (2011). A Pseudomonas aeruginosa toxin that hijacks the host ubiquitin proteolytic system. PLoS Pathog. 7:e1001325. doi: 10.1371/journal.ppat.1001325
Brown, L., Wolf, J. M., Prados-Rosales, R., and Casadevall, A. (2015). Through the wall: extracellular vesicles in gram-positive bacteria, mycobacteria and fungi. Nat. Rev. Microbiol. 13, 620–630. doi: 10.1038/nrmicro3480
Cañas, M. A., Fábrega, M. J., Giménez, R., Badia, J., and Baldomà, L. (2018). Outer membrane vesicles from probiotic and commensal Escherichia coli activate NOD1-mediated immune responses in intestinal epithelial cells. Front. Microbiol. 9:498. doi: 10.3389/fmicb.2018.00498
Cao, Y., Zhou, Y., Chen, D., Wu, R., Guo, L., and Lin, H. (2020). Proteomic and metabolic characterization of membrane vesicles derived from Streptococcus mutans at different pH values. Appl. Microbiol. Biotechnol. 104, 9733–9748. doi: 10.1007/s00253-020-10563-6
Cecil, J. D., O'Brien-Simpson, N. M., Lenzo, J. C., Holden, J. A., Chen, Y. Y., Singleton, W., et al. (2016). Differential responses of pattern recognition receptors to outer membrane vesicles of three periodontal pathogens. PLoS One 11:e0151967. doi: 10.1371/journal.pone.0151967
Cecil, J. D., O'Brien-Simpson, N. M., Lenzo, J. C., Holden, J. A., Singleton, W., Perez-Gonzalez, A., et al. (2017). Outer membrane vesicles prime and activate macrophage inflammasomes and cytokine secretion in vitro and in vivo. Front. Immunol. 8:1017. doi: 10.3389/fimmu.2017.01017
Chelakkot, C., Choi, Y., Kim, D. K., Park, H. T., Ghim, J., Kwon, Y., et al. (2018). Akkermansia muciniphila-derived extracellular vesicles influence gut permeability through the regulation of tight junctions. Exp. Mol. Med. 50:e450. doi: 10.1038/emm.2017.282
Chen, C. Y., Rao, S. S., Yue, T., Tan, Y. J., Yin, H., Chen, L. J., et al. (2022). Glucocorticoid-induced loss of beneficial gut bacterial extracellular vesicles is associated with the pathogenesis of osteonecrosis. Sci. Adv. 8:eabg8335. doi: 10.1126/sciadv.abg8335
Chen, G., Sun, Q., Cai, Q., and Zhou, H. (2022). Outer membrane vesicles from Fusobacterium nucleatum switch M0-like macrophages toward the M1 phenotype to destroy periodontal tissues in mice. Front. Microbiol. 13:815638. doi: 10.3389/fmicb.2022.815638
Chen, P. P., Zhang, J. X., Li, X. Q., Li, L., Wu, Q. Y., Liu, L., et al. (2023). Outer membrane vesicles derived from gut microbiota mediate tubulointerstitial inflammation: a potential new mechanism for diabetic kidney disease. Theranostics 13, 3988–4003. doi: 10.7150/thno.84650
Cheon, S. Y., and Lee, J. E. (2021). Extracellular vesicles and immune system in ageing and immune diseases. Exp Neurobiol 30, 32–47. doi: 10.5607/en20059
Choi, H. I., Choi, J. P., Seo, J., Kim, B. J., Rho, M., Han, J. K., et al. (2017). Helicobacter pylori-derived extracellular vesicles increased in the gastric juices of gastric adenocarcinoma patients and induced inflammation mainly via specific targeting of gastric epithelial cells. Exp. Mol. Med. 49:e330. doi: 10.1038/emm.2017.47
Choi, J., Kim, Y. K., and Han, P. L. (2019). Extracellular vesicles derived from Lactobacillus plantarum increase BDNF expression in cultured hippocampal neurons and produce antidepressant-like effects in mice. Exp Neurobiol 28, 158–171. doi: 10.5607/en.2019.28.2.158
Choi, J. W., Kim, S. C., Hong, S. H., and Lee, H. J. (2017). Secretable small RNAs via outer membrane vesicles in periodontal pathogens. J. Dent. Res. 96, 458–466. doi: 10.1177/0022034516685071
Choi, J., Kwon, H., Kim, Y. K., and Han, P. L. (2022). Extracellular vesicles from gram-positive and gram-negative probiotics remediate stress-induced depressive behavior in mice. Mol. Neurobiol. 59, 2715–2728. doi: 10.1007/s12035-021-02655-9
Choi, Y., Kwon, Y., Kim, D. K., Jeon, J., Jang, S. C., Wang, T., et al. (2015). Gut microbe-derived extracellular vesicles induce insulin resistance, thereby impairing glucose metabolism in skeletal muscle. Sci. Rep. 5:15878. doi: 10.1038/srep15878
Chu, H., Khosravi, A., Kusumawardhani, I. P., Kwon, A. H., Vasconcelos, A. C., Cunha, L. D., et al. (2016). Gene-microbiota interactions contribute to the pathogenesis of inflammatory bowel disease. Science 352, 1116–1120. doi: 10.1126/science.aad9948
Codemo, M., Muschiol, S., Iovino, F., Nannapaneni, P., Plant, L., Wai, S. N., et al. (2018). Immunomodulatory effects of pneumococcal extracellular vesicles on cellular and humoral host defenses. MBio 9:e00559–18 doi: 10.1128/mBio.00559-18
Couto, N., Schooling, S. R., Dutcher, J. R., and Barber, J. (2015). Proteome profiles of outer membrane vesicles and extracellular matrix of Pseudomonas aeruginosa biofilms. J. Proteome Res. 14, 4207–4222. doi: 10.1021/acs.jproteome.5b00312
Dauros-Singorenko, P., Hong, J., Swift, S., Phillips, A., and Blenkiron, C. (2020). Effect of the extracellular vesicle RNA cargo from Uropathogenic Escherichia coli on bladder cells. Front. Mol. Biosci. 7:580913. doi: 10.3389/fmolb.2020.580913
Diaz-Garrido, N., Badia, J., and Baldomà, L. (2022). Modulation of dendritic cells by microbiota extracellular vesicles influences the cytokine profile and exosome cargo. Nutrients 14:344 doi: 10.3390/nu14020344
Díaz-Garrido, N., Bonnin, S., Riera, M., Gíménez, R., Badia, J., and Baldomà, L. (2020). Transcriptomic microRNA profiling of dendritic cells in response to gut microbiota-secreted vesicles. Cells 9:1534 doi: 10.3390/cells9061534
Dong, X. H., Ho, M. H., Liu, B., Hildreth, J., Dash, C., Goodwin, J. S., et al. (2018). Role of Porphyromonas gingivalis outer membrane vesicles in oral mucosal transmission of HIV. Sci. Rep. 8:8812. doi: 10.1038/s41598-018-27284-6
du Teil Espina, M., Haider Rubio, A., Fu, Y., López-Álvarez, M., Gabarrini, G., and van Dijl, J. M. (2022). Outer membrane vesicles of the oral pathogen Porphyromonas gingivalis promote aggregation and phagocytosis of Staphylococcus aureus. Front Oral Health 3:948524. doi: 10.3389/froh.2022.948524
Durant, L., Stentz, R., Noble, A., Brooks, J., Gicheva, N., Reddi, D., et al. (2020). Bacteroides thetaiotaomicron-derived outer membrane vesicles promote regulatory dendritic cell responses in health but not in inflammatory bowel disease. Microbiome 8:88. doi: 10.1186/s40168-020-00868-z
Elizagaray, M. L., Gomes, M. T. R., Guimaraes, E. S., Rumbo, M., Hozbor, D. F., Oliveira, S. C., et al. (2020). Canonical and non-canonical Inflammasome activation by outer membrane vesicles derived from Bordetella pertussis. Front. Immunol. 11:1879. doi: 10.3389/fimmu.2020.01879
Elmi, A., Nasher, F., Jagatia, H., Gundogdu, O., Bajaj-Elliott, M., Wren, B., et al. (2016). Campylobacter jejuni outer membrane vesicle-associated proteolytic activity promotes bacterial invasion by mediating cleavage of intestinal epithelial cell E-cadherin and occludin. Cell. Microbiol. 18, 561–572. doi: 10.1111/cmi.12534
Elmi, A., Watson, E., Sandu, P., Gundogdu, O., Mills, D. C., Inglis, N. F., et al. (2012). Campylobacter jejuni outer membrane vesicles play an important role in bacterial interactions with human intestinal epithelial cells. Infect. Immun. 80, 4089–4098. doi: 10.1128/IAI.00161-12
Engevik, M. A., Danhof, H. A., Ruan, W., Engevik, A. C., Chang-Graham, A. L., Engevik, K. A., et al. (2021). Fusobacterium nucleatum secretes outer membrane vesicles and promotes intestinal inflammation. MBio 12. doi: 10.1128/mBio.02706-20
Esoda, C. N., and Kuehn, M. J. (2019). Pseudomonas aeruginosa leucine aminopeptidase influences early biofilm composition and structure via vesicle-associated Antibiofilm activity. MBio 10:e02548–19 doi: 10.1128/mBio.02548-19
Fábrega, M. J., Rodríguez-Nogales, A., Garrido-Mesa, J., Algieri, F., Badía, J., Giménez, R., et al. (2017). Intestinal anti-inflammatory effects of outer membrane vesicles from Escherichia coli Nissle 1917 in DSS-experimental colitis in mice. Front. Microbiol. 8:1274. doi: 10.3389/fmicb.2017.01274
Fan, R., Zhou, Y., Chen, X., Zhong, X., He, F., Peng, W., et al. (2023). Porphyromonas gingivalis outer membrane vesicles promote apoptosis via msRNA-regulated DNA methylation in periodontitis. Microbiol Spectr 11:e0328822. doi: 10.1128/spectrum.03288-22
Farrugia, C., Stafford, G. P., and Murdoch, C. (2020). Porphyromonas gingivalis outer membrane vesicles increase vascular permeability. J. Dent. Res. 99, 1494–1501. doi: 10.1177/0022034520943187
Fizanne, L., Villard, A., Benabbou, N., Recoquillon, S., Soleti, R., Delage, E., et al. (2023). Faeces-derived extracellular vesicles participate in the onset of barrier dysfunction leading to liver diseases. J Extracell Vesicles 12:e12303. doi: 10.1002/jev2.12303
Fonseca, S., Carvalho, A. L., Miquel-Clopés, A., Jones, E. J., Juodeikis, R., Stentz, R., et al. (2022). Extracellular vesicles produced by the human gut commensal bacterium Bacteroides thetaiotaomicron elicit anti-inflammatory responses from innate immune cells. Front. Microbiol. 13:1050271. doi: 10.3389/fmicb.2022.1050271
Friedrich, V., Gruber, C., Nimeth, I., Pabinger, S., Sekot, G., Posch, G., et al. (2015). Outer membrane vesicles of Tannerella forsythia: biogenesis, composition, and virulence. Mol Oral Microbiol 30, 451–473. doi: 10.1111/omi.12104
Furuta, N., Takeuchi, H., and Amano, A. (2009). Entry of Porphyromonas gingivalis outer membrane vesicles into epithelial cells causes cellular functional impairment. Infect. Immun. 77, 4761–4770. doi: 10.1128/IAI.00841-09
Furuta, N., Tsuda, K., Omori, H., Yoshimori, T., Yoshimura, F., and Amano, A. (2009). Porphyromonas gingivalis outer membrane vesicles enter human epithelial cells via an endocytic pathway and are sorted to lysosomal compartments. Infect. Immun. 77, 4187–4196. doi: 10.1128/IAI.00009-09
Gabarrini, G., Heida, R., van Ieperen, N., Curtis, M. A., van Winkelhoff, A. J., and van Dijl, J. M. (2018a). Dropping anchor: attachment of peptidylarginine deiminase via A-LPS to secreted outer membrane vesicles of Porphyromonas gingivalis. Sci. Rep. 8:8949. doi: 10.1038/s41598-018-27223-5
Gabarrini, G., Palma Medina, L. M., Stobernack, T., Prins, R. C., du Teil Espina, M., Kuipers, J., et al. (2018b). There's no place like OM: vesicular sorting and secretion of the peptidylarginine deiminase of Porphyromonas gingivalis. Virulence 9, 456–464. doi: 10.1080/21505594.2017.1421827
Galka, F., Wai, S. N., Kusch, H., Engelmann, S., Hecker, M., Schmeck, B., et al. (2008). Proteomic characterization of the whole secretome of legionella pneumophila and functional analysis of outer membrane vesicles. Infect. Immun. 76, 1825–1836. doi: 10.1128/IAI.01396-07
Gao, H., Jin, Z., Tang, K., Ji, Y., Suarez, J., Suarez, J. A., et al. (2022). Microbial DNA enrichment promotes Adrenomedullary inflammation, catecholamine secretion, and hypertension in obese mice. J. Am. Heart Assoc. 11:e024561. doi: 10.1161/JAHA.121.024561
Gao, H., Luo, Z., Ji, Y., Tang, K., Jin, Z., Ly, C., et al. (2022). Accumulation of microbial DNAs promotes to islet inflammation and β cell abnormalities in obesity in mice. Nat. Commun. 13:565. doi: 10.1038/s41467-022-28239-2
Gong, T., Chen, Q., Mao, H., Zhang, Y., Ren, H., Xu, M., et al. (2022). Outer membrane vesicles of Porphyromonas gingivalis trigger NLRP3 inflammasome and induce neuroinflammation, tau phosphorylation, and memory dysfunction in mice. Front. Cell. Infect. Microbiol. 12:925435. doi: 10.3389/fcimb.2022.925435
Grenier, D. (2013). Porphyromonas gingivalis outer membrane vesicles mediate Coaggregation and piggybacking of Treponema denticola and Lachnoanaerobaculum saburreum. Int J Dent 2013:305476. doi: 10.1155/2013/305476
Gul, L., Modos, D., Fonseca, S., Madgwick, M., Thomas, J. P., Sudhakar, P., et al. (2022). Extracellular vesicles produced by the human commensal gut bacterium Bacteroides thetaiotaomicron affect host immune pathways in a cell-type specific manner that are altered in inflammatory bowel disease. J Extracell Vesicles 11:e12189. doi: 10.1002/jev2.12189
Ha, J. Y., Choi, S. Y., Lee, J. H., Hong, S. H., and Lee, H. J. (2020). Delivery of Periodontopathogenic extracellular vesicles to brain monocytes and microglial IL-6 promotion by RNA cargo. Front. Mol. Biosci. 7:596366. doi: 10.3389/fmolb.2020.596366
Han, E. C., Choi, S. Y., Lee, Y., Park, J. W., Hong, S. H., and Lee, H. J. (2019). Extracellular RNAs in periodontopathogenic outer membrane vesicles promote TNF-α production in human macrophages and cross the blood-brain barrier in mice. FASEB J. 33, 13412–13422. doi: 10.1096/fj.201901575R
Hiratsuka, K., Abiko, Y., Hayakawa, M., Ito, T., Sasahara, H., and Takiguchi, H. (1992). Role of Porphyromonas gingivalis 40-kDa outer membrane protein in the aggregation of P. gingivalis vesicles and Actinomyces viscosus. Arch. Oral Biol. 37, 717–724. doi: 10.1016/0003-9969(92)90078-M
Hu, R., Lin, H., Wang, M., Zhao, Y., Liu, H., Min, Y., et al. (2021). Lactobacillus reuteri-derived extracellular vesicles maintain intestinal immune homeostasis against lipopolysaccharide-induced inflammatory responses in broilers. J Anim Sci Biotechnol 12:25. doi: 10.1186/s40104-020-00532-4
Inagaki, S., Onishi, S., Kuramitsu, H. K., and Sharma, A. (2006). Porphyromonas gingivalis vesicles enhance attachment, and the leucine-rich repeat BspA protein is required for invasion of epithelial cells by "Tannerella forsythia". Infect. Immun. 74, 5023–5028. doi: 10.1128/IAI.00062-06
Ito, R., Ishihara, K., Shoji, M., Nakayama, K., and Okuda, K. (2010). Hemagglutinin/Adhesin domains of Porphyromonas gingivalis play key roles in coaggregation with Treponema denticola. FEMS Immunol. Med. Microbiol. 60, 251–260. doi: 10.1111/j.1574-695X.2010.00737.x
Iwabuchi, Y., Nakamura, T., Kusumoto, Y., Nakao, R., Iwamoto, T., Shinozuka, O., et al. (2021). Effects of pH on the properties of membrane vesicles including glucosyltransferase in Streptococcus mutans. Microorganisms 9:2308 doi: 10.3390/microorganisms9112308
Jäger, J., Keese, S., Roessle, M., Steinert, M., and Schromm, A. B. (2015). Fusion of Legionella pneumophila outer membrane vesicles with eukaryotic membrane systems is a mechanism to deliver pathogen factors to host cell membranes. Cell. Microbiol. 17, 607–620. doi: 10.1111/cmi.12392
Jeong, D., Kim, M. J., Park, Y., Chung, J., Kweon, H. S., Kang, N. G., et al. (2022). Visualizing extracellular vesicle biogenesis in gram-positive bacteria using super-resolution microscopy. BMC Biol. 20:270. doi: 10.1186/s12915-022-01472-3
Jia, Y., Guo, B., Yang, W., Zhao, Q., Jia, W., and Wu, Y. (2015). Rho kinase mediates Porphyromonas gingivalis outer membrane vesicle-induced suppression of endothelial nitric oxide synthase through ERK1/2 and p38 MAPK. Arch. Oral Biol. 60, 488–495. doi: 10.1016/j.archoralbio.2014.12.009
Jiang, H., Ling, Z., Zhang, Y., Mao, H., Ma, Z., Yin, Y., et al. (2015). Altered fecal microbiota composition in patients with major depressive disorder. Brain Behav. Immun. 48, 186–194. doi: 10.1016/j.bbi.2015.03.016
Jiao, Y., Darzi, Y., Tawaratsumida, K., Marchesan, J. T., Hasegawa, M., Moon, H., et al. (2013). Induction of bone loss by pathobiont-mediated Nod1 signaling in the oral cavity. Cell Host Microbe 13, 595–601. doi: 10.1016/j.chom.2013.04.005
Johnston, E. L., Heras, B., Kufer, T. A., and Kaparakis-Liaskos, M. (2021). Detection of bacterial membrane vesicles by NOD-like receptors. Int. J. Mol. Sci. 22. doi: 10.3390/ijms22031005
Jones, M. M., Vanyo, S. T., and Visser, M. B. (2019). The Msp protein of Treponema denticola interrupts activity of phosphoinositide processing in neutrophils. Infect. Immun. 87. doi: 10.1128/IAI.00553-19
Jung, A. L., Stoiber, C., Herkt, C. E., Schulz, C., Bertrams, W., and Schmeck, B. (2016). Legionella pneumophila-derived outer membrane vesicles promote bacterial replication in macrophages. PLoS Pathog. 12:e1005592. doi: 10.1371/journal.ppat.1005592
Kalra, H., Drummen, G. P., and Mathivanan, S. (2016). Focus on extracellular vesicles: introducing the next small big thing. Int. J. Mol. Sci. 17:170. doi: 10.3390/ijms17020170
Kamaguchi, A., Nakayama, K., Ichiyama, S., Nakamura, R., Watanabe, T., Ohta, M., et al. (2003). Effect of Porphyromonas gingivalis vesicles on coaggregation of Staphylococcus aureus to oral microorganisms. Curr. Microbiol. 47, 485–491. doi: 10.1007/s00284-003-4069-6
Kang, C. S., Ban, M., Choi, E. J., Moon, H. G., Jeon, J. S., Kim, D. K., et al. (2013). Extracellular vesicles derived from gut microbiota, especially Akkermansia muciniphila, protect the progression of dextran sulfate sodium-induced colitis. PLoS One 8:e76520. doi: 10.1371/journal.pone.0076520
Kaparakis, M., Turnbull, L., Carneiro, L., Firth, S., Coleman, H. A., Parkington, H. C., et al. (2010). Bacterial membrane vesicles deliver peptidoglycan to NOD1 in epithelial cells. Cell. Microbiol. 12, 372–385. doi: 10.1111/j.1462-5822.2009.01404.x
Kaparakis-Liaskos, M., and Ferrero, R. L. (2015). Immune modulation by bacterial outer membrane vesicles. Nat. Rev. Immunol. 15, 375–387. doi: 10.1038/nri3837
Kayagaki, N., Stowe, I. B., Lee, B. L., O'Rourke, K., Anderson, K., Warming, S., et al. (2015). Caspase-11 cleaves gasdermin D for non-canonical inflammasome signalling. Nature 526, 666–671. doi: 10.1038/nature15541
Keshavarz Azizi Raftar, S., Ashrafian, F., Yadegar, A., Lari, A., Moradi, H. R., Shahriary, A., et al. (2021). The protective effects of live and pasteurized Akkermansia muciniphila and its extracellular vesicles against HFD/CCl4-induced liver injury. Microbiol Spectr 9:e0048421. doi: 10.1128/Spectrum.00484-21
Kim, M. R., Hong, S. W., Choi, E. B., Lee, W. H., Kim, Y. S., Jeon, S. G., et al. (2012). Staphylococcus aureus-derived extracellular vesicles induce neutrophilic pulmonary inflammation via both Th1 and Th17 cell responses. Allergy 67, 1271–1281. doi: 10.1111/all.12001
Kim, J. H., Jeun, E. J., Hong, C. P., Kim, S. H., Jang, M. S., Lee, E. J., et al. (2016). Extracellular vesicle-derived protein from Bifidobacterium longum alleviates food allergy through mast cell suppression. J. Allergy Clin. Immunol. 137, 507–516.e508. doi: 10.1016/j.jaci.2015.08.016
Kim, O. Y., Park, H. T., Dinh, N. T. H., Choi, S. J., Lee, J., Kim, J. H., et al. (2017). Bacterial outer membrane vesicles suppress tumor by interferon-γ-mediated antitumor response. Nat. Commun. 8:626. doi: 10.1038/s41467-017-00729-8
Kim, H. Y., Song, M. K., Gho, Y. S., Kim, H. H., and Choi, B. K. (2021). Extracellular vesicles derived from the periodontal pathogen Filifactor alocis induce systemic bone loss through toll-like receptor 2. J Extracell Vesicles 10:e12157. doi: 10.1002/jev2.12157
Kim, H. Y., Song, M. K., Lim, Y., Jang, J. S., An, S. J., Kim, H. H., et al. (2022). Effects of extracellular vesicles derived from oral bacteria on osteoclast differentiation and activation. Sci. Rep. 12:14239. doi: 10.1038/s41598-022-18412-4
Kopparapu, P. K., Deshmukh, M., Hu, Z., Mohammad, M., Maugeri, M., Götz, F., et al. (2021). Lipoproteins are responsible for the pro-inflammatory property of Staphylococcus aureus extracellular vesicles. Int. J. Mol. Sci. 22. doi: 10.3390/ijms22137099
Kyung Lee, M., Armstrong, D. A., Hazlett, H. F., Dessaint, J. A., Mellinger, D. L., Aridgides, D. S., et al. (2021). Exposure to extracellular vesicles from Pseudomonas aeruginosa result in loss of DNA methylation at enhancer and DNase hypersensitive site regions in lung macrophages. Epigenetics 16, 1187–1200. doi: 10.1080/15592294.2020.1853318
Larsen, D. N., Mikkelsen, C. E., Kierkegaard, M., Bereta, G. P., Nowakowska, Z., Kaczmarek, J. Z., et al. (2020). Citrullinome of Porphyromonas gingivalis outer membrane vesicles: confident identification of Citrullinated peptides. Mol. Cell. Proteomics 19, 167–180. doi: 10.1074/mcp.RA119.001700
Lee, E. Y., Choi, D. Y., Kim, D. K., Kim, J. W., Park, J. O., Kim, S., et al. (2009). Gram-positive bacteria produce membrane vesicles: proteomics-based characterization of Staphylococcus aureus-derived membrane vesicles. Proteomics 9, 5425–5436. doi: 10.1002/pmic.200900338
Lee, K. E., Kim, J. K., Han, S. K., Lee, D. Y., Lee, H. J., Yim, S. V., et al. (2020). The extracellular vesicle of gut microbial Paenalcaligenes hominis is a risk factor for vagus nerve-mediated cognitive impairment. Microbiome 8:107. doi: 10.1186/s40168-020-00881-2
Lee, J. C., Lee, E. J., Lee, J. H., Jun, S. H., Choi, C. W., Kim, S. I., et al. (2012). Klebsiella pneumoniae secretes outer membrane vesicles that induce the innate immune response. FEMS Microbiol. Lett. 331, 17–24. doi: 10.1111/j.1574-6968.2012.02549.x
Lindholm, M., Metsäniitty, M., Granström, E., and Oscarsson, J. (2020). Outer membrane vesicle-mediated serum protection in Aggregatibacter actinomycetemcomitans. J. Oral Microbiol. 12:1747857. doi: 10.1080/20002297.2020.1747857
Liu, J. H., Chen, C. Y., Liu, Z. Z., Luo, Z. W., Rao, S. S., Jin, L., et al. (2021). Extracellular vesicles from child gut microbiota enter into bone to preserve bone mass and strength. Adv Sci (Weinh) 8:2004831. doi: 10.1002/advs.202004831
Liu, J., Hsieh, C. L., Gelincik, O., Devolder, B., Sei, S., Zhang, S., et al. (2019). Proteomic characterization of outer membrane vesicles from gut mucosa-derived fusobacterium nucleatum. J. Proteome 195, 125–137. doi: 10.1016/j.jprot.2018.12.029
Liu, L., Liang, L., Yang, C., Zhou, Y., and Chen, Y. (2021). Extracellular vesicles of Fusobacterium nucleatum compromise intestinal barrier through targeting RIPK1-mediated cell death pathway. Gut Microbes 13, 1–20. doi: 10.1080/19490976.2021.1902718
Liu, P., Wang, X., Yang, Q., Yan, X., Fan, Y., Zhang, S., et al. (2022). Collaborative action of microglia and astrocytes mediates neutrophil recruitment to the CNS to defend against Escherichia coli K1 infection. Int. J. Mol. Sci. 23. doi: 10.3390/ijms23126540
López, P., González-Rodríguez, I., Sánchez, B., Gueimonde, M., Margolles, A., and Suárez, A. (2012). Treg-inducing membrane vesicles from Bifidobacterium bifidum LMG13195 as potential adjuvants in immunotherapy. Vaccine 30, 825–829. doi: 10.1016/j.vaccine.2011.11.115
Luo, Z., Ji, Y., Gao, H., Gomes Dos Reis, F. C., Bandyopadhyay, G., Jin, Z., et al. (2021). CRIg(+) macrophages prevent gut microbial DNA-containing extracellular vesicle-induced tissue inflammation and insulin resistance. Gastroenterology 160, 863–874. doi: 10.1053/j.gastro.2020.10.042
Luo, Z., Ji, Y., Zhang, D., Gao, H., Jin, Z., Yang, M., et al. (2022). Microbial DNA enrichment promotes liver steatosis and fibrosis in the course of non-alcoholic steatohepatitis. Acta Physiol (Oxf.) 235:e13827. doi: 10.1111/apha.13827
Maerz, J. K., Steimle, A., Lange, A., Bender, A., Fehrenbacher, B., and Frick, J. S. (2018). Outer membrane vesicles blebbing contributes to B. vulgatus mpk-mediated immune response silencing. Gut Microbes 9, 1–12. doi: 10.1080/19490976.2017.1344810
Maldonado, R., Wei, R., Kachlany, S. C., Kazi, M., and Balashova, N. V. (2011). Cytotoxic effects of Kingella kingae outer membrane vesicles on human cells. Microb. Pathog. 51, 22–30. doi: 10.1016/j.micpath.2011.03.005
Marion, C. R., Lee, J., Sharma, L., Park, K. S., Lee, C., Liu, W., et al. (2019). Toll-like receptors 2 and 4 modulate pulmonary inflammation and host factors mediated by outer membrane vesicles derived from Acinetobacter baumannii. Infect. Immun. 87. doi: 10.1128/IAI.00243-19
Miura, K., Seki, E., Ohnishi, H., and Brenner, D. A. (2010). Role of toll-like receptors and their downstream molecules in the development of nonalcoholic fatty liver disease. Gastroenterol. Res. Pract. 2010:362847. doi: 10.1155/2010/362847
Miyakawa, H., Honma, K., Qi, M., and Kuramitsu, H. K. (2004). Interaction of Porphyromonas gingivalis with low-density lipoproteins: implications for a role for periodontitis in atherosclerosis. J. Periodontal Res. 39, 1–9. doi: 10.1111/j.1600-0765.2004.00697.x
Mosby, C. A., Bhar, S., Phillips, M. B., Edelmann, M. J., and Jones, M. K. (2022). Interaction with mammalian enteric viruses alters outer membrane vesicle production and content by commensal bacteria. J Extracell Vesicles 11:e12172. doi: 10.1002/jev2.12172
Mosby, C. A., Edelmann, M. J., and Jones, M. K. (2023). Murine norovirus interaction with Enterobacter cloacae leads to changes in membrane stability and packaging of lipid and metabolite vesicle content. Microbiol Spectr 11:e0469122. doi: 10.1128/spectrum.04691-22
Mulcahy, L. A., Pink, R. C., and Carter, D. R. (2014). Routes and mechanisms of extracellular vesicle uptake. J Extracell Vesicles 3. doi: 10.3402/jev.v3.24641
Nah, G., Park, S. C., Kim, K., Kim, S., Park, J., Lee, S., et al. (2019). Type-2 diabetics reduces spatial variation of microbiome based on extracellur vesicles from gut microbes across human body. Sci. Rep. 9:20136. doi: 10.1038/s41598-019-56662-x
Nakamura, T., Iwabuchi, Y., Hirayama, S., Narisawa, N., Takenaga, F., Nakao, R., et al. (2020). Roles of membrane vesicles from Streptococcus mutans for the induction of antibodies to glucosyltransferase in mucosal immunity. Microb. Pathog. 149:104260. doi: 10.1016/j.micpath.2020.104260
Nakayama, M., Inoue, T., Naito, M., Nakayama, K., and Ohara, N. (2015). Attenuation of the phosphatidylinositol 3-kinase/Akt signaling pathway by Porphyromonas gingivalis gingipains RgpA, RgpB, and Kgp. J. Biol. Chem. 290, 5190–5202. doi: 10.1074/jbc.M114.591610
O'Donoghue, E. J., and Krachler, A. M. (2016). Mechanisms of outer membrane vesicle entry into host cells. Cell. Microbiol. 18, 1508–1517. doi: 10.1111/cmi.12655
O'Donoghue, E. J., Sirisaengtaksin, N., Browning, D. F., Bielska, E., Hadis, M., Fernandez-Trillo, F., et al. (2017). Lipopolysaccharide structure impacts the entry kinetics of bacterial outer membrane vesicles into host cells. PLoS Pathog. 13:e1006760. doi: 10.1371/journal.ppat.1006760
Park, J., Kim, N. E., Yoon, H., Shin, C. M., Kim, N., Lee, D. H., et al. (2021). Fecal microbiota and gut microbe-derived extracellular vesicles in colorectal Cancer. Front. Oncol. 11:650026. doi: 10.3389/fonc.2021.650026
Park, K. S., Lee, J., Jang, S. C., Kim, S. R., Jang, M. H., Lötvall, J., et al. (2013). Pulmonary inflammation induced by bacteria-free outer membrane vesicles from Pseudomonas aeruginosa. Am. J. Respir. Cell Mol. Biol. 49, 637–645. doi: 10.1165/rcmb.2012-0370OC
Park, A. M., and Tsunoda, I. (2022). Helicobacter pylori infection in the stomach induces neuroinflammation: the potential roles of bacterial outer membrane vesicles in an animal model of Alzheimer's disease. Inflamm Regen 42:39. doi: 10.1186/s41232-022-00224-8
Paulsson, M., Che, K. F., Ahl, J., Tham, J., Sandblad, L., Smith, M. E., et al. (2018). Bacterial outer membrane vesicles induce Vitronectin release into the Bronchoalveolar space conferring protection from complement-mediated killing. Front. Microbiol. 9:1559. doi: 10.3389/fmicb.2018.01559
Pesce Viglietti, A. I., Sviercz, F. A., López, C. A. M., Freiberger, R. N., Quarleri, J., and Delpino, M. V. (2021). Proinflammatory microenvironment during Kingella kingae infection modulates Osteoclastogenesis. Front. Immunol. 12:757827. doi: 10.3389/fimmu.2021.757827
Pritchard, A. B., Fabian, Z., Lawrence, C. L., Morton, G., Crean, S., and Alder, J. E. (2022). An investigation into the effects of outer membrane vesicles and lipopolysaccharide of Porphyromonas gingivalis on blood-brain barrier integrity, permeability, and disruption of scaffolding proteins in a human in vitro model. J. Alzheimers Dis. 86, 343–364. doi: 10.3233/JAD-215054
Roier, S., Zingl, F. G., Cakar, F., and Schild, S. (2016). Bacterial outer membrane vesicle biogenesis: a new mechanism and its implications. Microb Cell 3, 257–259. doi: 10.15698/mic2016.06.508
Rompikuntal, P. K., Thay, B., Khan, M. K., Alanko, J., Penttinen, A. M., Asikainen, S., et al. (2012). Perinuclear localization of internalized outer membrane vesicles carrying active cytolethal distending toxin from Aggregatibacter actinomycetemcomitans. Infect. Immun. 80, 31–42. doi: 10.1128/IAI.06069-11
Santos, J. C., Dick, M. S., Lagrange, B., Degrandi, D., Pfeffer, K., Yamamoto, M., et al. (2018). LPS targets host guanylate-binding proteins to the bacterial outer membrane for non-canonical inflammasome activation. EMBO J. 37:e98089 doi: 10.15252/embj.201798089
Schwechheimer, C., and Kuehn, M. J. (2015). Outer-membrane vesicles from gram-negative bacteria: biogenesis and functions. Nat. Rev. Microbiol. 13, 605–619. doi: 10.1038/nrmicro3525
Seo, M. K., Park, E. J., Ko, S. Y., Choi, E. W., and Kim, S. (2018). Therapeutic effects of kefir grain Lactobacillus-derived extracellular vesicles in mice with 2,4,6-trinitrobenzene sulfonic acid-induced inflammatory bowel disease. J. Dairy Sci. 101, 8662–8671. doi: 10.3168/jds.2018-15014
Seyama, M., Yoshida, K., Yoshida, K., Fujiwara, N., Ono, K., Eguchi, T., et al. (2020). Outer membrane vesicles of Porphyromonas gingivalis attenuate insulin sensitivity by delivering gingipains to the liver. Biochim. Biophys. Acta Mol. basis Dis. 1866:165731. doi: 10.1016/j.bbadis.2020.165731
Shen, Y., Giardino Torchia, M. L., Lawson, G. W., Karp, C. L., Ashwell, J. D., and Mazmanian, S. K. (2012). Outer membrane vesicles of a human commensal mediate immune regulation and disease protection. Cell Host Microbe 12, 509–520. doi: 10.1016/j.chom.2012.08.004
Song, M. K., Kim, H. Y., Choi, B. K., and Kim, H. H. (2020). Filifactor alocis-derived extracellular vesicles inhibit osteogenesis through TLR2 signaling. Mol Oral Microbiol 35, 202–210. doi: 10.1111/omi.12307
Stentz, R., Carvalho, A. L., Jones, E. J., and Carding, S. R. (2018). Fantastic voyage: the journey of intestinal microbiota-derived microvesicles through the body. Biochem. Soc. Trans. 46, 1021–1027. doi: 10.1042/BST20180114
Svennerholm, K., Park, K. S., Wikström, J., Lässer, C., Crescitelli, R., Shelke, G. V., et al. (2017). Escherichia coli outer membrane vesicles can contribute to sepsis induced cardiac dysfunction. Sci. Rep. 7:17434. doi: 10.1038/s41598-017-16363-9
Thay, B., Damm, A., Kufer, T. A., Wai, S. N., and Oscarsson, J. (2014). Aggregatibacter actinomycetemcomitans outer membrane vesicles are internalized in human host cells and trigger NOD1-and NOD2-dependent NF-κB activation. Infect. Immun. 82, 4034–4046. doi: 10.1128/IAI.01980-14
Thay, B., Wai, S. N., and Oscarsson, J. (2013). Staphylococcus aureus α-toxin-dependent induction of host cell death by membrane-derived vesicles. PLoS One 8:e54661. doi: 10.1371/journal.pone.0054661
Tiku, V., and Tan, M. W. (2021). Host immunity and cellular responses to bacterial outer membrane vesicles. Trends Immunol. 42, 1024–1036. doi: 10.1016/j.it.2021.09.006
Toyofuku, M., Cárcamo-Oyarce, G., Yamamoto, T., Eisenstein, F., Hsiao, C. C., Kurosawa, M., et al. (2017). Prophage-triggered membrane vesicle formation through peptidoglycan damage in Bacillus subtilis. Nat. Commun. 8:481. doi: 10.1038/s41467-017-00492-w
Toyofuku, M., Nomura, N., and Eberl, L. (2019). Types and origins of bacterial membrane vesicles. Nat. Rev. Microbiol. 17, 13–24. doi: 10.1038/s41579-018-0112-2
Turkina, M. V., Olofsson, A., Magnusson, K. E., Arnqvist, A., and Vikström, E. (2015). Helicobacter pylori vesicles carrying CagA localize in the vicinity of cell-cell contacts and induce histone H1 binding to ATP in epithelial cells. FEMS Microbiol. Lett. 362. doi: 10.1093/femsle/fnv076
Tyrer, P. C., Frizelle, F. A., and Keenan, J. I. (2014). Escherichia coli-derived outer membrane vesicles are genotoxic to human enterocyte-like cells. Infect Agent Cancer 9:2. doi: 10.1186/1750-9378-9-2
Tzipilevich, E., Habusha, M., and Ben-Yehuda, S. (2017). Acquisition of Phage Sensitivity by Bacteria through exchange of phage receptors. Cells 168, 186–199.e112. doi: 10.1016/j.cell.2016.12.003
Vanaja, S. K., Russo, A. J., Behl, B., Banerjee, I., Yankova, M., Deshmukh, S. D., et al. (2016). Bacterial outer membrane vesicles mediate cytosolic localization of LPS and Caspase-11 activation. Cells 165, 1106–1119. doi: 10.1016/j.cell.2016.04.015
Vdovikova, S., Gilfillan, S., Wang, S., Dongre, M., Wai, S. N., and Hurtado, A. (2018). Modulation of gene transcription and epigenetics of colon carcinoma cells by bacterial membrane vesicles. Sci. Rep. 8:7434. doi: 10.1038/s41598-018-25308-9
Vitse, J., and Devreese, B. (2020). The contribution of membrane vesicles to bacterial pathogenicity in cystic fibrosis infections and healthcare associated pneumonia. Front. Microbiol. 11:630. doi: 10.3389/fmicb.2020.00630
Volgers, C., Benedikter, B. J., Grauls, G. E., Savelkoul, P. H. M., and Stassen, F. R. M. (2017). Immunomodulatory role for membrane vesicles released by THP-1 macrophages and respiratory pathogens during macrophage infection. BMC Microbiol. 17:216. doi: 10.1186/s12866-017-1122-3
Waller, T., Kesper, L., Hirschfeld, J., Dommisch, H., Kölpin, J., Oldenburg, J., et al. (2016). Porphyromonas gingivalis outer membrane vesicles induce selective tumor necrosis factor tolerance in a toll-like receptor 4-and mTOR-dependent manner. Infect. Immun. 84, 1194–1204. doi: 10.1128/IAI.01390-15
Wang, X., Eagen, W. J., and Lee, J. C. (2020). Orchestration of human macrophage NLRP3 inflammasome activation by Staphylococcus aureus extracellular vesicles. Proc. Natl. Acad. Sci. U. S. A. 117, 3174–3184. doi: 10.1073/pnas.1915829117
Wang, X., Lin, S., Wang, L., Cao, Z., Zhang, M., Zhang, Y., et al. (2023). Versatility of bacterial outer membrane vesicles in regulating intestinal homeostasis. Sci. Adv. 9:eade5079. doi: 10.1126/sciadv.ade5079
Wang, T., Mo, L., Ou, J., Fang, Q., Wu, H., Wu, Y., et al. (2022). Proteus mirabilis vesicles induce mitochondrial apoptosis by regulating miR96-5p/Abca1 to inhibit Osteoclastogenesis and bone loss. Front. Immunol. 13:833040. doi: 10.3389/fimmu.2022.833040
Wang, N., Zhou, F., Chen, C., Luo, H., Guo, J., Wang, W., et al. (2021). Role of outer membrane vesicles from Helicobacter pylori in atherosclerosis. Front. Cell Dev. Biol. 9:673993. doi: 10.3389/fcell.2021.673993
Wegorzewska, M. M., Glowacki, R. W. P., Hsieh, S. A., Donermeyer, D. L., Hickey, C. A., Horvath, S. C., et al. (2019). Diet modulates colonic T cell responses by regulating the expression of a Bacteroides thetaiotaomicron antigen. Sci Immunol 4. doi: 10.1126/sciimmunol.aau9079
Wei, S., Li, X., Wang, J., Wang, Y., Zhang, C., Dai, S., et al. (2022). Outer membrane vesicles secreted by Helicobacter pylori transmitting gastric pathogenic virulence factors. ACS Omega 7, 240–258. doi: 10.1021/acsomega.1c04549
Weiner, A., Mellouk, N., Lopez-Montero, N., Chang, Y. Y., Souque, C., Schmitt, C., et al. (2016). Macropinosomes are key players in early Shigella invasion and vacuolar escape in epithelial cells. PLoS Pathog. 12:e1005602. doi: 10.1371/journal.ppat.1005602
Wu, R., Tao, Y., Cao, Y., Zhou, Y., and Lin, H. (2020). Streptococcus mutans membrane vesicles harboring glucosyltransferases augment Candida albicans biofilm development. Front. Microbiol. 11:581184. doi: 10.3389/fmicb.2020.581184
Xie, J., Cools, L., Van Imschoot, G., Van Wonterghem, E., Pauwels, M. J., Vlaeminck, I., et al. (2023). Helicobacter pylori-derived outer membrane vesicles contribute to Alzheimer's disease pathogenesis via C3-C3aR signalling. J Extracell Vesicles 12:e12306. doi: 10.1002/jev2.12306
Yaghoubfar, R., Behrouzi, A., Ashrafian, F., Shahryari, A., Moradi, H. R., Choopani, S., et al. (2020). Modulation of serotonin signaling/metabolism by Akkermansia muciniphila and its extracellular vesicles through the gut-brain axis in mice. Sci. Rep. 10:22119. doi: 10.1038/s41598-020-79171-8
Yamasaki-Yashiki, S., Miyoshi, Y., Nakayama, T., Kunisawa, J., and Katakura, Y. (2019). IgA-enhancing effects of membrane vesicles derived from Lactobacillus sakei subsp. sakei NBRC15893. Biosci Microbiota Food Health 38, 23–29. doi: 10.12938/bmfh.18-015
Yang, W. W., Guo, B., Jia, W. Y., and Jia, Y. (2016). Porphyromonas gingivalis-derived outer membrane vesicles promote calcification of vascular smooth muscle cells through ERK1/2-RUNX2. FEBS Open Bio 6, 1310–1319. doi: 10.1002/2211-5463.12151
Yang, J., Hwang, I., Lee, E., Shin, S. J., Lee, E. J., Rhee, J. H., et al. (2020). Bacterial outer membrane vesicle-mediated cytosolic delivery of Flagellin triggers host NLRC4 canonical Inflammasome signaling. Front. Immunol. 11:581165. doi: 10.3389/fimmu.2020.581165
Yoshida, K., Yoshida, K., Seyama, M., Hiroshima, Y., Mekata, M., Fujiwara, N., et al. (2022). Porphyromonas gingivalis outer membrane vesicles in cerebral ventricles activate microglia in mice. Oral Dis. doi: 10.1111/odi.14413
You, H. S., Ok, Y. J., Lee, S. H., Kang, S. S., and Hyun, S. H. (2019). Changes in gene expression in human epithelial and mast cells in response to vesicles from Klebsiella pneumonia ATCC 13883. Indian J. Microbiol. 59, 241–245. doi: 10.1007/s12088-018-0774-5
Zahmatkesh, M. E., Jahanbakhsh, M., Hoseini, N., Shegefti, S., Peymani, A., Dabin, H., et al. (2022). Effects of exosomes derived from Helicobacter pylori outer membrane vesicle-infected hepatocytes on hepatic stellate cell activation and liver fibrosis induction. Front. Cell. Infect. Microbiol. 12:857570. doi: 10.3389/fcimb.2022.857570
Zakharzhevskaya, N. B., Vanyushkina, A. A., Altukhov, I. A., Shavarda, A. L., Butenko, I. O., Rakitina, D. V., et al. (2017). Outer membrane vesicles secreted by pathogenic and nonpathogenic Bacteroides fragilis represent different metabolic activities. Sci. Rep. 7:5008. doi: 10.1038/s41598-017-05264-6
Zarnowski, R., Sanchez, H., Covelli, A. S., Dominguez, E., Jaromin, A., Bernhardt, J., et al. (2018). Candida albicans biofilm-induced vesicles confer drug resistance through matrix biogenesis. PLoS Biol. 16:e2006872. doi: 10.1371/journal.pbio.2006872
Zhang, Y., Fang, Z., Li, R., Huang, X., and Liu, Q. (2019). Design of outer membrane vesicles as cancer vaccines: a new toolkit for cancer therapy. Cancers (Basel) 11:1314 doi: 10.3390/cancers11091314
Keywords: bacterial extracellular vesicles, outer membrane vesicles, membrane vesicles, interaction, pathogenesis, systemic diseases
Citation: Wang Y, Luo X, Xiang X, Hao C and Ma D (2023) Roles of bacterial extracellular vesicles in systemic diseases. Front. Microbiol. 14:1258860. doi: 10.3389/fmicb.2023.1258860
Edited by:
Michal Letek, University of León, SpainReviewed by:
Dhiman Sankar Pal, Johns Hopkins University, United StatesMartina Bielaszewska, National Institute of Public Health (NIPH), Czechia
Medicharla Venkata Jagannadham, University of Hyderabad, India
Copyright © 2023 Wang, Luo, Xiang, Hao and Ma. This is an open-access article distributed under the terms of the Creative Commons Attribution License (CC BY). The use, distribution or reproduction in other forums is permitted, provided the original author(s) and the copyright owner(s) are credited and that the original publication in this journal is cited, in accordance with accepted academic practice. No use, distribution or reproduction is permitted which does not comply with these terms.
*Correspondence: Dandan Ma, bWRkQHNtdS5lZHUuY24=
†ORCID: Dandan Ma, https://orcid.org/0000-0003-4484-9455