- Department of Health Sciences, Carleton University, Ottawa, ON, Canada
The high pathogenicity of Pseudomonas aeruginosa is attributed to the production of many virulence factors and its resistance to several antimicrobials. Among them, sodium hypochlorite (NaOCl) is a widely used disinfectant due to its strong antimicrobial effect. However, bacteria develop many mechanisms to survive the damage caused by this agent. Therefore, this study aimed to identify novel mechanisms employed by P. aeruginosa to resist oxidative stress induced by the strong oxidizing agent NaOCl. We analyzed the growth of the P. aeruginosa mutants ΔkatA, ΔkatE, ΔahpC, ΔahpF, ΔmsrA at 1 μg/mL NaOCl, and showed that these known H2O2 resistance mechanisms are also important for the survival of P. aeruginosa under NaOCl stress. We then conducted a screening of the P. aeruginosa PA14 transposon insertion mutant library and identified 48 mutants with increased susceptibility toward NaOCl. Among them were 10 mutants with a disrupted nrdJa, bvlR, hcnA, orn, sucC, cysZ, nuoJ, PA4166, opmQ, or thiC gene, which also exhibited a significant growth defect in the presence of NaOCl. We focussed our follow-up experiments (i.e., growth analyzes and kill-kinetics) on mutants with defect in the synthesis of the secondary metabolite hydrogen cyanide (HCN). We showed that HCN produced by P. aeruginosa contributes to its resistance toward NaOCl as it acts as a scavenger molecule, quenching the toxic effects of NaOCl.
1. Introduction
Pseudomonas aeruginosa is an ubiquitous environmental, Gram-negative bacterium and a highly versatile opportunistic human pathogen that can be isolated from soil, water, plants, and animals (Ambreetha and Balachandar, 2022). In humans, it can cause severe and diverse infections of considerable medical importance, such as ventilator-associated pneumonia, endocarditis, urinary tract, and systemic infections, mainly in immunocompromised individuals (Bassetti et al., 2018). One remarkable characteristic of P. aeruginosa is its ability to adapt and survive under various and harsh environmental conditions due to a sophisticated network of stress responses, including cold, heat, and oxidative stress responses (Craig et al., 2021; Da Cruz Nizer et al., 2021).
Oxidizing agents are low-molecular-weight compounds that have an increased ability to oxidize other substances by removing electrons and, therefore, are considered potent antimicrobial agents (Finnegan et al., 2010). Among them, reactive oxygen (e.g., hydrogen peroxide, H2O2) and chlorine (e.g., hypochlorous acid, HOCl) species (ROS and RCS, respectively) are highly reactive molecules produced as by-products of the metabolism of oxygen of living organisms (endogenous production) or encountered by bacterial cells from exogenous sources, such as disinfectants (Bardaweel et al., 2018). In bacterial cells, these molecules oxidize several molecules and disrupt numerous cellular processes. For instance, they react with lipids, proteins, and nucleic acids resulting in membrane damage and affecting protein, DNA, RNA, and energy synthesis (Da Cruz Nizer et al., 2021). Due to their potent activity, ROS and RCS are widely used in many applications as disinfectants in domestic, industrial and hospital settings, water and wastewater treatment, cleaning of wounds, and as antiseptic agents (Da Cruz Nizer et al., 2020, 2021; Gold et al., 2020). Furthermore, H2O2 and HOCl are also produced by human immune cells as a defense against invading pathogens (Da Cruz Nizer et al., 2020). Overall, HOCl is a more potent oxidizing agent with a much faster antimicrobial effect than H2O2 (Peskin and Winterbourn, 2001; Winterbourn et al., 2016). It is the active ingredient of sodium hypochlorite (NaOCl; household bleach) and is considered the most commonly used chlorine-based disinfectant and oxidant in drinking water disinfection (Fukuzaki, 2006; Deborde and von Gunten, 2008).
Pseudomonas aeruginosa is constantly exposed to oxidative stress, either from endogenous production or exogenous sources, by the use of disinfectants. In this context, this bacterium has developed many mechanisms to survive the toxic effects of these agents. Although HOCl is a stronger oxidant than H2O2, most research has focused on the H2O2 responses adopted by P. aeruginosa, and only a few studies have identified and characterized specific stress responses and resistance mechanisms against HOCl (Da Cruz Nizer et al., 2020). However, most of these adaptive responses are not specific to a single agent but are part of a general oxidative stress response in P. aeruginosa. For instance, detoxifying enzymes, such as catalases, alkyl hydroperoxides, and protein repair systems, such as MrsR, are well-known to be involved in the resistance of P. aeruginosa against H2O2 (Panmanee and Hassett, 2009; Romsang et al., 2013). Furthermore, transcriptional regulators, mainly OxyR, are also crucial for the survival of P. aeruginosa under oxidizing conditions (Ochsner et al., 2000). Previous studies have used gene expression analyzes or screening for regulatory proteins to characterize the adaptation of P. aeruginosa to HOCl (Groitl et al., 2017; Farrant et al., 2020).
This study aims to identify novel genes involved in the resistance of P. aeruginosa to the strong oxidant NaOCl by a targeted screening of genes known to be involved in H2O2 resistance as well as screening the comprehensive P. aeruginosa PA14 mutant library (Liberati et al., 2006) for mutants with increased susceptibility to NaOCl.
2. Materials and methods
2.1. Bacterial strains, plasmids, and growth conditions
The bacterial strains used in this study included P. aeruginosa PA14 wild-type (PA14 WT) (Rahme et al., 1995), P. aeruginosa PAO1 WT (Stover et al., 2000) and the entire P. aeruginosa PA14 transposon mutant library from Harvard University (Liberati et al., 2006), which was used to screen susceptible mutants. After the selection of PA14 mutants, PAO1 homologs from the PAO1 comprehensive transposon mutant library (Jacobs et al., 2003) were also tested for further verification of our findings. The list of strains used in this study is shown in Supplementary Table S1. Unless otherwise stated, all strains were grown in Lysogeny broth (LB) overnight at 37°C under shaking conditions (220 rpm) and the experiments were conducted using cells in the stationary growth phase. Oxidative stress assays were conducted in BM2 minimal medium [7 mM (NH4)2SO4, 40 mM K2HPO4, 22 mM KH2PO, 0.4% (w/v) glucose, 0.5 mM MgSO4, 0.01 mM FeSO4, pH 7.0] (Overhage et al., 2008). For easier readability, we used the PAO1 gene numbers for our genes when the gene names were unknown.
The PA2194 PW4739 (ΔhcnB) mutant from the PAO1 transposon mutant library (Jacobs et al., 2003) was complemented by the transfer of the phcnBC plasmid expressing hcnBC (Létoffé et al., 2022), which was kindly provided by Dr. Jean-Marc Ghigo. The complemented mutant ΔhcnB-phcnBC was grown overnight in LB medium supplemented with 400 μg/mL kanamycin and 2 mM sodium benzoate for inducible gene expression (Létoffé et al., 2022).
2.2. Measurement of RCS
NaOCl aqueous solution was used to induce RCS stress. Free chlorine concentration of NaOCl aqueous solutions was determined weekly using DPD Free Chlorine Powder Packs (Thermo Scientific Orion), according to the manufacturer’s instructions. BM2 minimal medium was used to mitigate side reactions between NaOCl and growth medium components. Additionally, to confirm that the addition of NaOCl to BM2 did not reduce the amount of overall RCS, RCS concentration was measured as previously described (Ashby et al., 2020). In this context, addition of 1, 2, and 4 μg/mL NaOCl to BM2 minimal medium did not result in significant reduction in overall RCS levels. In contrast, NaOCl was completely quenched by LB in our control experiment.
2.3. Pre-liminary screening for susceptible mutants
To identify genes involved in P. aeruginosa stress resistance to NaOCl, we initially screened the P. aeruginosa PA14 transposon insertion mutant library (Liberati et al., 2006) for mutants with increased susceptibility to NaOCl. For this, frozen stocks of PA14 mutants arranged in 96-well microtiter plates were transferred to fresh LB medium and incubated for 24 h at 37°C. Then, the mutants were stamped with a 96-pin metal replicator into new 96-well microtiter plates containing NaOCl at 1 μg/mL (½ x MIC) in BM2 growth medium and incubated for 24 h at 37°C. Susceptible mutants (i.e., mutants that did not grow at 1 μg/mL NaOCl) were selected for further analysis.
2.4. Minimal inhibitory concentration assay
The MIC for NaOCl and H2O2 was determined by the standard broth microdilution method in BM2 minimal medium, as described previously (Wiegand et al., 2008). Briefly, P. aeruginosa strains were grown overnight in LB at 37°C and 220 rpm. Cells were collected by centrifugation (10,000 rpm for 2 min), washed twice with phosphate-buffered saline (PBS), and resuspended in BM2 medium. The optical density at 600 nm (OD600nm) was adjusted to 0.2 (2 × 108 CFU/mL), and 50 μL was mixed in 96-well plates with 50 μL of serial dilutions of NaOCl (0.125–128 μg/mL) or H2O2 (98–50,000 μg/mL) prepared in BM2 (final cell concentration of 1 × 108 CFU/mL). The plates were incubated for 24 h at 37°C, and the MIC was considered the lowest concentration of oxidizing agent that inhibits the visual growth of bacteria.
2.5. Growth curves
Pseudomonas aeruginosa overnight cultures grown at 37°C and 220 rpm in LB were washed twice with PBS, resuspended in BM2, and the OD600nm was adjusted to 0.2 (2 × 108 CFU/mL). Then, 50 μL of bacterial suspension and 50 μL of oxidizing agent were mixed in flat-bottom polystyrene 96-well microtiter plates, leading to a final concentration of NaOCl of 1 μg/mL and H2O2 of 400 μg/mL and the final cell concentration of 1 × 108 CFU/mL. The OD600nm was read every hour for 20 h at 37°C using the Epoch plate reader (Biotek, United States). Growth curves were statistically analyzed by measuring the area under the curve (AUC) using GraphPad Prism version 9.5.1 (San Diego, United States).
2.6. Semiquantitative analysis of Hydrogen cyanide (HCN) production
Volatile Hydrogen cyanide production was quantified by the semiquantitative method previously described (Castric, 1977; Létoffé et al., 2022). Briefly, 2 mL of overnight cultures of PAO1 WT, ΔhcnB, and ΔhcnB-phcnBC grown in LB were collected by centrifugation and washed twice with PBS. The cells were resuspended in 2 mL of LB and transferred to a small Petri dish (35 cm diameter) placed in the middle of a 100-mm diameter Petri dish. The small petri dish was covered with chromatography paper soaked in HCN detection reagent: 100 mg of copper (II) ethyl acetoacetate and 100 mg of 4,4′-methylenebis-(N,N-dimethylaniline) solubilized in 20 mL chloroform (Létoffé et al., 2022). The large petri dish was then closed and incubated at 37°C for 24 h under static conditions. HCN production was detected by blue color formation on the chromatography paper. Two mM sodium benzoate was added to ΔhcnB-phcnBC cells.
2.7. Time-kill kinetics experiments
Pseudomonas aeruginosa was grown overnight in LB at 37°C under shaking conditions, collected by centrifugation, washed twice, and resuspended in PBS. The OD600nm was adjusted to 0.1, and the bacterial suspensions were treated with NaOCl at 2 μg/mL for 5, 15, 30, and 60 min. For the experiments using the supernatant, PAO1 WT, ΔhcnB and ΔhcnB-phcnBC were grown in BM2 overnight. Then, PAO1 WT, ΔhcnB, or ΔhcnB-phcnBC culture supernatants were collected by centrifugation followed by sterile filtration using a 0.22 μm filter. On the other hand, ΔhcnB cells were collected by centrifugation, washed twice with PBS, and the OD600nm was adjusted to 0.1 by diluting the cells in PAO1 WT, ΔhcnB, or ΔhcnB-phcnBC supernatants. The bacterial suspensions were treated with 4 μg/mL NaOCl for 60 min.
After the treatments, 10 mM Na2S2O3 was added to the samples to quench NaOCl, and the cells were serially diluted and plated out on LB agar plates using the drop plate method previously described (Herigstad et al., 2001).
2.8. Statistical analysis
Statistical analyzes were performed using GraphPad Prism software version 9.5.1 (San Diego, United States). The Shapiro–Wilk test was used to confirm the normality of the data. Parametric data were analyzed by One Way ANOVA, followed by Tukey or Dunnett’s post-test for multiple comparisons or Student’s t-test for comparison between two groups. Non-parametric data were analyzed by the t-test and Mann–Whitney test for comparison between two groups. All experiments were performed in at least three independent experiments, and results were considered statistically significant when p < 0.05.
3. Results
3.1. H2O2 Detoxifying mechanisms also contribute to the NaOCl survival of Pseudomonas aeruginosa
Previous work on H2O2 has identified several detoxifying enzymes and oxidative stress repair systems in P. aeruginosa. To evaluate if these previously described genes involved in H2O2 adaptation also play a role in the adaptation of P. aeruginosa to NaOCl, we examined growth of the P. aeruginosa PAO1 and PA14 mutants ΔkatA and ΔkatE (catalases), ΔahpC and ΔahpF (alkyl hydroperoxide reductase), ΔmsrA (methionine sulfoxide reductase), and ΔohrR (organic hydroperoxide resistance protein) exposed to NaOCl at 1 μg/mL for 20 h at 37°C. This sub-lethal concentration was chosen based on the MIC of the WT strains (2 μg/mL). In accordance with Farrant et al. (2020), mutants were considered to possess a susceptibility phenotype when they presented an increased lag phase of >3 h compared to the WT strain. Furthermore, to statistically analyze the growth curves obtained, we measured the AUC. AUC, also known as growth potential (Todor et al., 2014), is a metric to quantify the cumulative effect of overall growth over time (Sprouffske and Wagner, 2016).
Pseudomonas aeruginosa PAO1 and PA14 WT treated with 1 μg/mL NaOCl took approximately 5–6 h to reach an OD600nm of 0.2 (double the initial OD). Overall, all mutants presented reduced growth at 1 μg/mL NaOCl compared to the WT strains. Furthermore, the AUCs were statistically significant for both the PA14 and PAO1 mutant strains compared to the WT strains treated with 1 μg/mL NaOCl. An OhrR mutant, a transcriptional repressor involved in oxidative stress response in P. aeruginosa, was used as a control and presented growth compared to the untreated controls and WT strains (Figure 1; Table 1). Of note, the PA14 ΔkatA mutant (Figure 1A) required 11 h to reach an OD600nm of 0.2, nearly twice as long as the time needed for the PA14 WT strain, and the PAO1 mutant did not show any growth. Furthermore, the ΔkatA PA14 mutant exhibited an AUC approximately 2x smaller than that of the PA14 WT strain, whereas the ΔkatA PAO1 mutant had an AUC more than 4x smaller than the PAO1 WT strain.
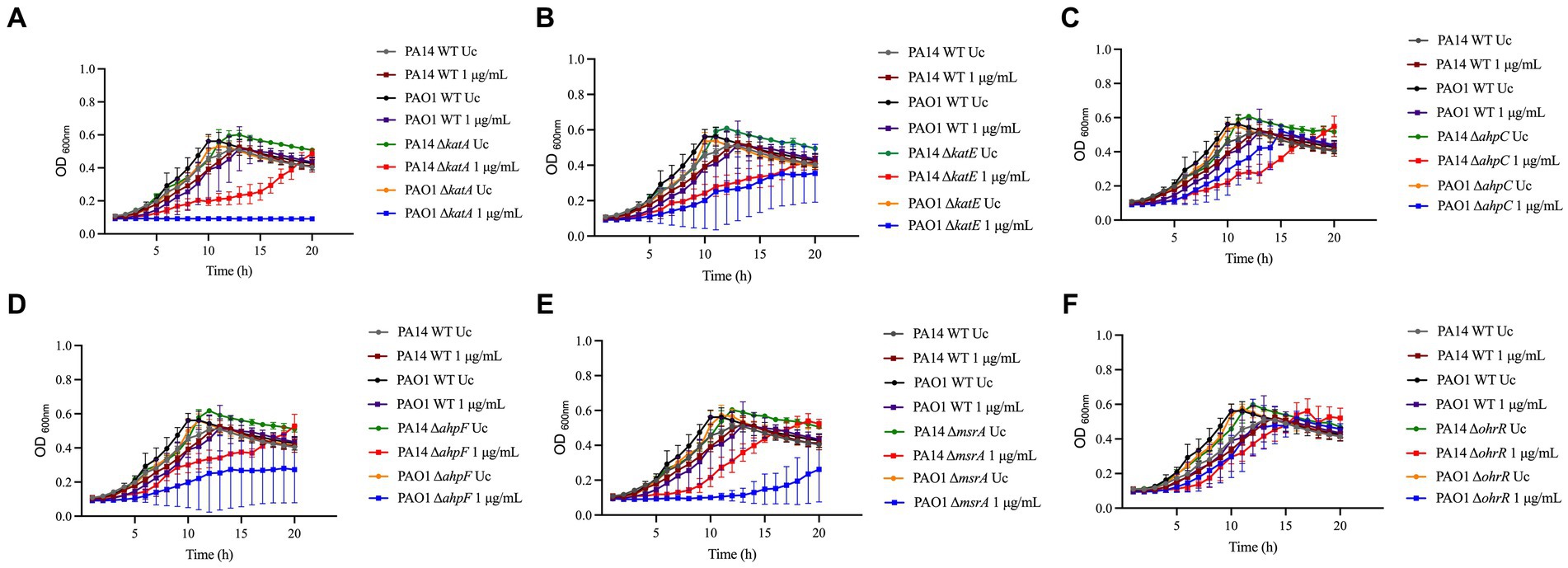
Figure 1. Susceptibility of known-H2O2 resistance mechanisms to NaOCl. Overnight cultures were grown in LB at 37°C and 220 rpm. Then, cells were washed twice with PBS, resuspended in BM2 minimal medium, and treated with NaOCl at 1 μg/mL (final cell concentration of 1 × 108 CFU/mL) for 20 h at 37°C. OD600nm was recorded every hour using an epoch plate reader. (A) ΔkatA; (B) ΔkatE; (C) ΔahpC; (D) ΔahpF; (E) ΔmsrA; (F) ΔohrR. WT: wild-type; Uc, untreated control.
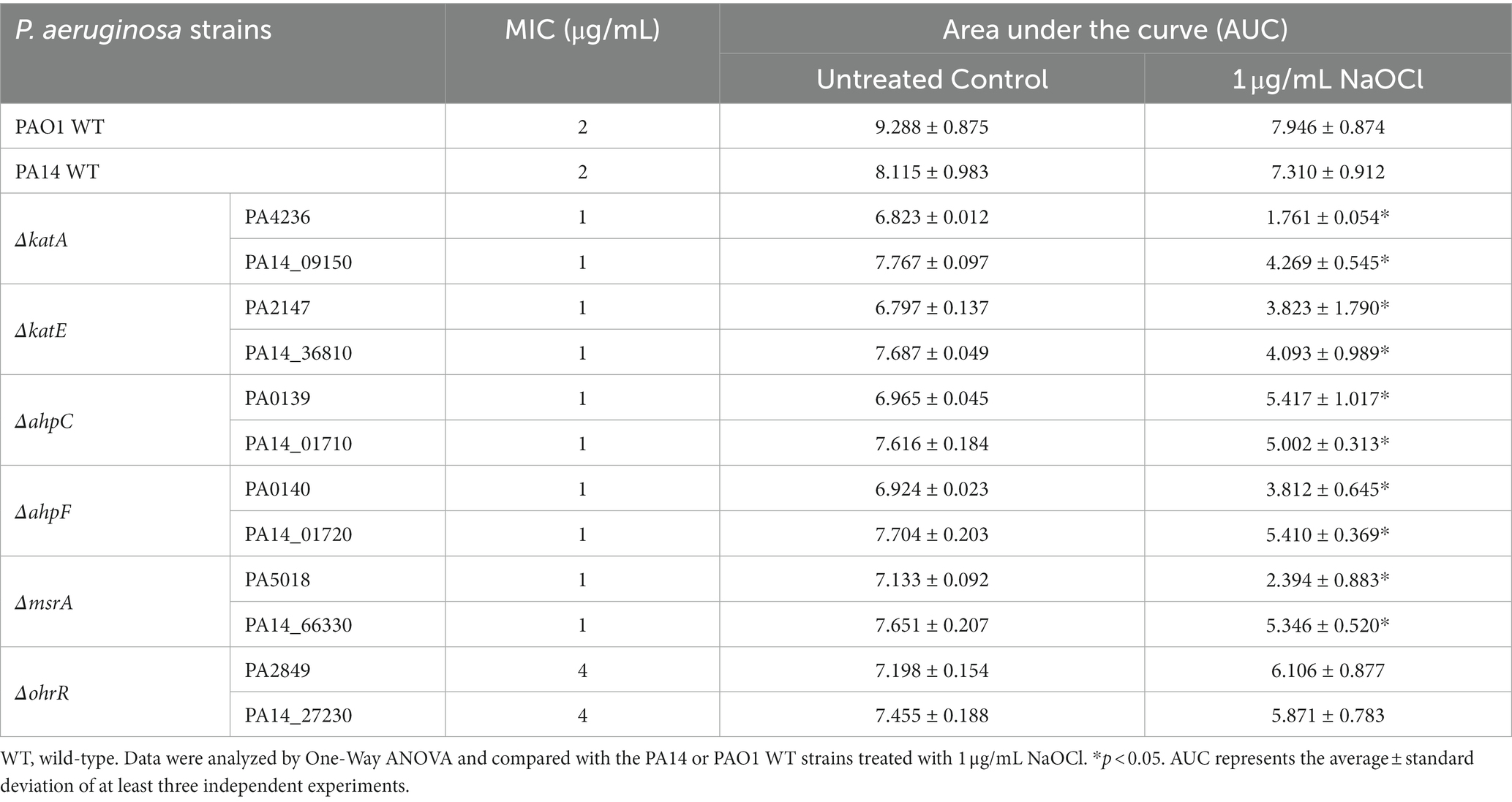
Table 1. MIC and area under the curve (AUC) of growth curves of Pseudomonas aeruginosa strains exposed to 1 μg/mL NaOCl.
In addition to the increased susceptibility in the growth analyzes, these mutants presented a 2-fold increase in susceptibility in MIC testing (MIC of 1 μg/mL; ½ x MIC of the WT), except for OhrR, which presented a MIC of 4 μg/mL (Table 1). These results demonstrate the importance of these repair systems in detoxifying toxic oxygen species, including NaOCl.
3.2. PA14 transposon mutant library screening for identification of novel genes involved in NaOCl resistance
To identify novel genes involved in NaOCl resistance, we screened the comprehensive Harvard PA14 transposon insertion mutant library (Liberati et al., 2006) for mutants with increased susceptibility to NaOCl. In the preliminary screening, we exposed the PA14 mutants to NaOCl at 1 μg/mL in BM2 minimal growth medium. Mutants not showing visual growth at this concentration were selected for further MIC testing to confirm their phenotypes. In total, 48 PA14 mutants with MIC of 0.5 and 1 μg/mL (¼ and ½ x MIC of PA14 WT) were identified and selected for further analysis (Figure 2A; Supplementary Table S2). Most of the mutants identified in this preliminary screening have a mutation in genes with unknown function (17/48), followed by genes involved in the transport of small molecules (8/48), such as ABC transporter, sulfate uptake protein, and major facilitator superfamily (MFS) transporter (Figure 2B). Furthermore, among the 48 mutants identified, 9 presented MIC values of 0.5 μg/mL [PA2077 (hypothetical protein), PA2193 (ΔhcnA, cyanide production), PA0846 (sulfate uptake protein), PA4110 (ΔampC, cephalosporinase), PA5446 (hypothetical protein), PA0040 (hemolysin activation/secretion protein), PA1046 (hypothetical protein), PA4973 (ΔthiC, thiamin biosynthesis protein ThiC), and PA1315 (transcriptional regulator)].
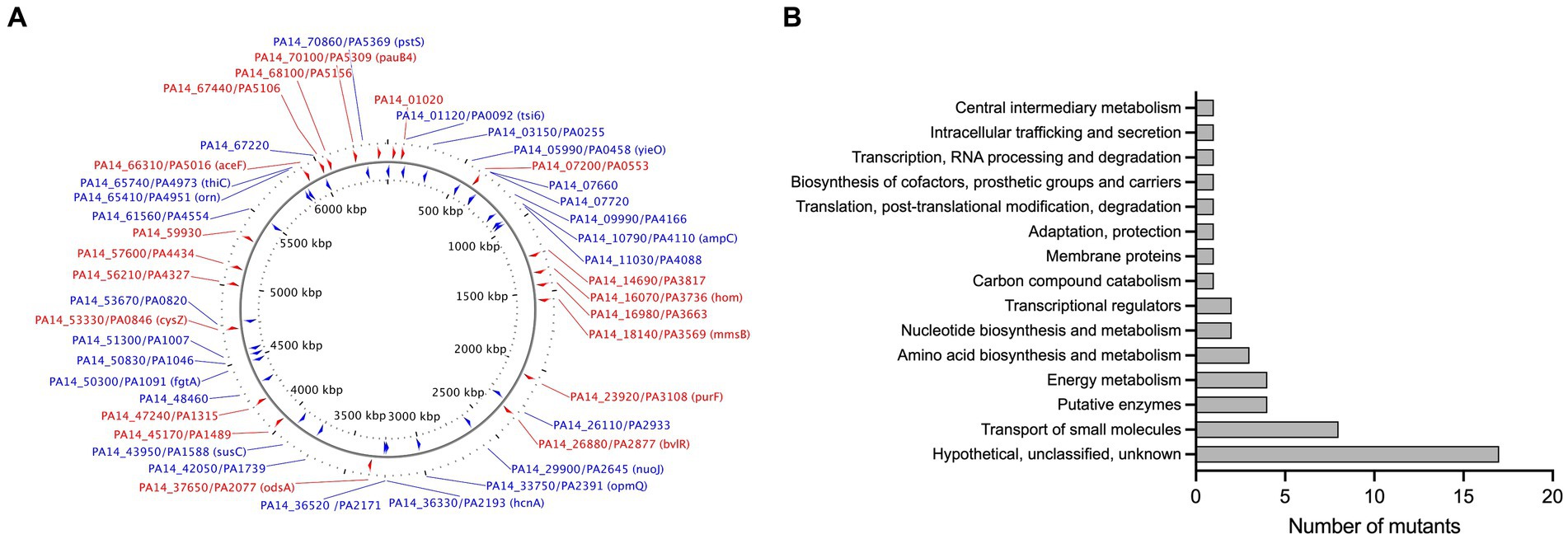
Figure 2. (A) Map of the P. aeruginosa genome with genes identified in the MIC screening. The image was generated by CGview (Stothard and Wishart, 2005). Red: Forward genes; Blue, Reverse genes. (B) Functional classification of genes identified in the MIC screening based on the P. aeruginosa genome classification.
3.3. Growth kinetic analyzes of mutants identified in the planktonic screening
To further characterize the susceptibility phenotype of the mutants identified in the MIC screening in more detail, we analyzed the growth of these 48 mutants in the presence of 1 μg/mL NaOCl for 20 h at 37°C in microtiter plates. Overall, 10 PA14 mutants identified in the library screening also presented a significant delay in growth in the presence of NaOCl compared to the WT-treated strains (i.e., more than 10 h to double the initial OD). Table 2 shows the 10 mutants identified in the PA14 mutant library screening, and Figure 3 illustrates their growth kinetics at 1 μg/mL NaOCl. Most of the mutants reached the OD600nm of 0.2 (double the initial cell concentration) after 11 h of incubation, while it took approximately 5–6 h for the WT strains to get to this OD. Among them, ΔbvlR, ΔhcnA, and ΔthiC presented overall reduced growth at 1 μg/mL NaOCl, reaching the maximum OD600nm of 0.364, 0.382, and 0.405, respectively, after 20 h compared to the PA14 WT (OD600nm of 0.6 after 20 h).
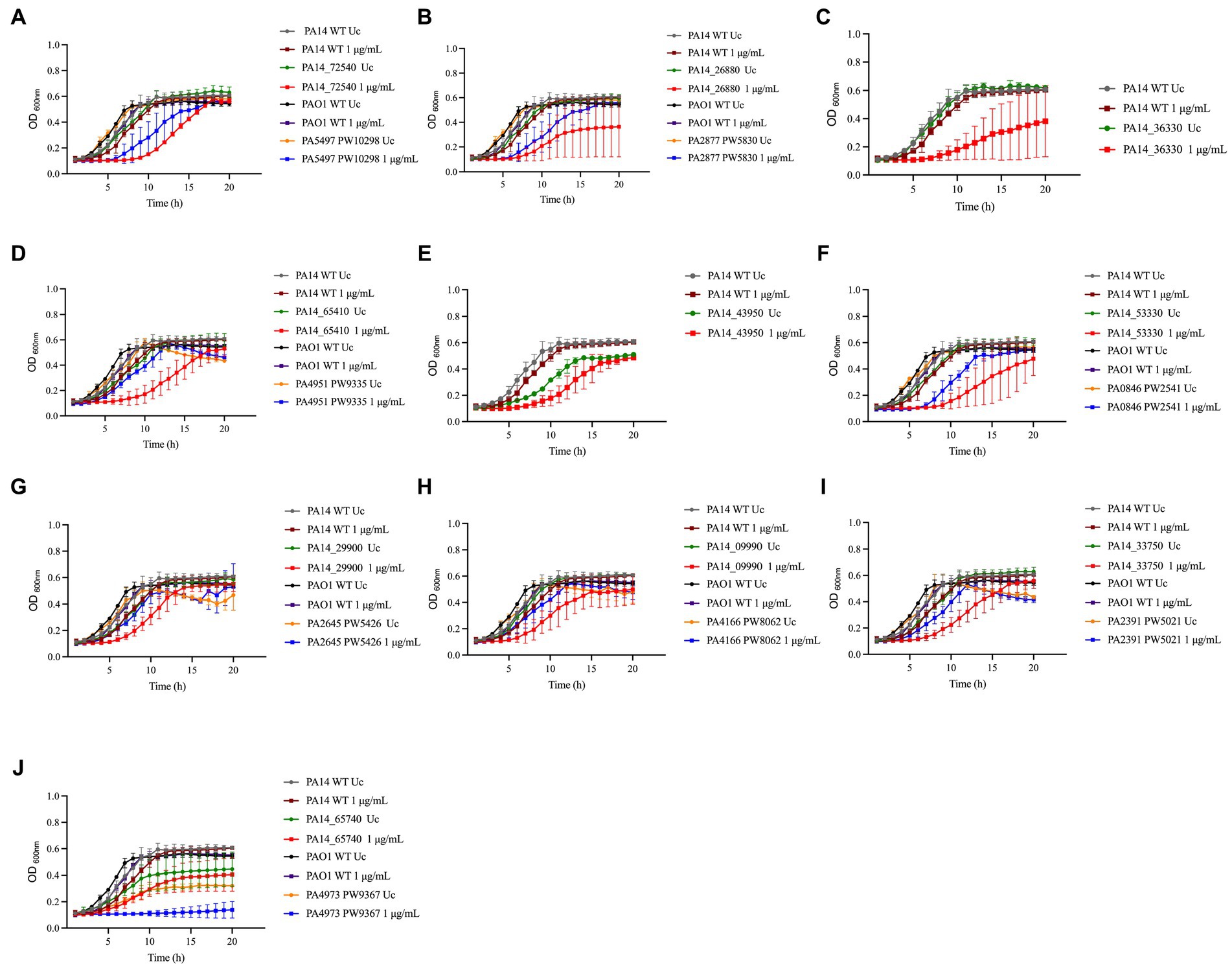
Figure 3. Susceptibility of PA14 mutants and PAO1 homologs identified in the screening to NaOCl. Overnight cultures were grown in LB at 37°C and 220 rpm. Then, cells were washed twice, resuspended in BM2 minimal medium, and treated with NaOCl at 1 μg/mL (final cell concentration of 1 × 108 CFU/mL) for 20 h at 37°C. OD600nm was recorded every hour using an epoch plate reader. (A) ΔnrdJa (PA14_72540/PA5497); (B) ΔbvlR (PA14_26880/PA2877); (C) ΔhcnA (PA14_36330); (D) Δorn (PA14_65410/PA4951); (E) ΔsucC (PA14_43950); (F) ΔcysZ (PA14_53330/PA0846); (G) ΔnuoJ (PA14_29900/PA2645); (H) PA14_09990/PA4166; (I) ΔopmQ (PA14_33750/PA2391); (J) ΔthiC (PA14_65740/PA4973). WT: wild-type; Uc: untreated control.
To evaluate if our findings are strain-specific, we also assessed the growth of the PAO1 mutant homologs from the PAO1 two-allele transposon mutant library from the University of Washington Genome Center (Jacobs et al., 2003) under the same experimental conditions over time (Figure 3). Moreover, to statistically analyze the growth curves obtained for the PA14 and PAO1 mutants, we calculated the AUC and compared the values obtained with the AUC of 1 μg/mL NaOCl-treated WT strains (Table 3). The mutants ΔnrdJa, ΔcysZ, ΔopmQ, and ΔthiC presented delayed growth (i.e., lag phase >3 h than the WT strains) and statistically different AUCs compared to the NaOCl-treated WT strains for both PA14 and PAO1 mutants, suggesting that the phenotype found is not strain specific. PAO1 homologs for the mutants ΔhcnA and ΔsucC were unavailable for testing.
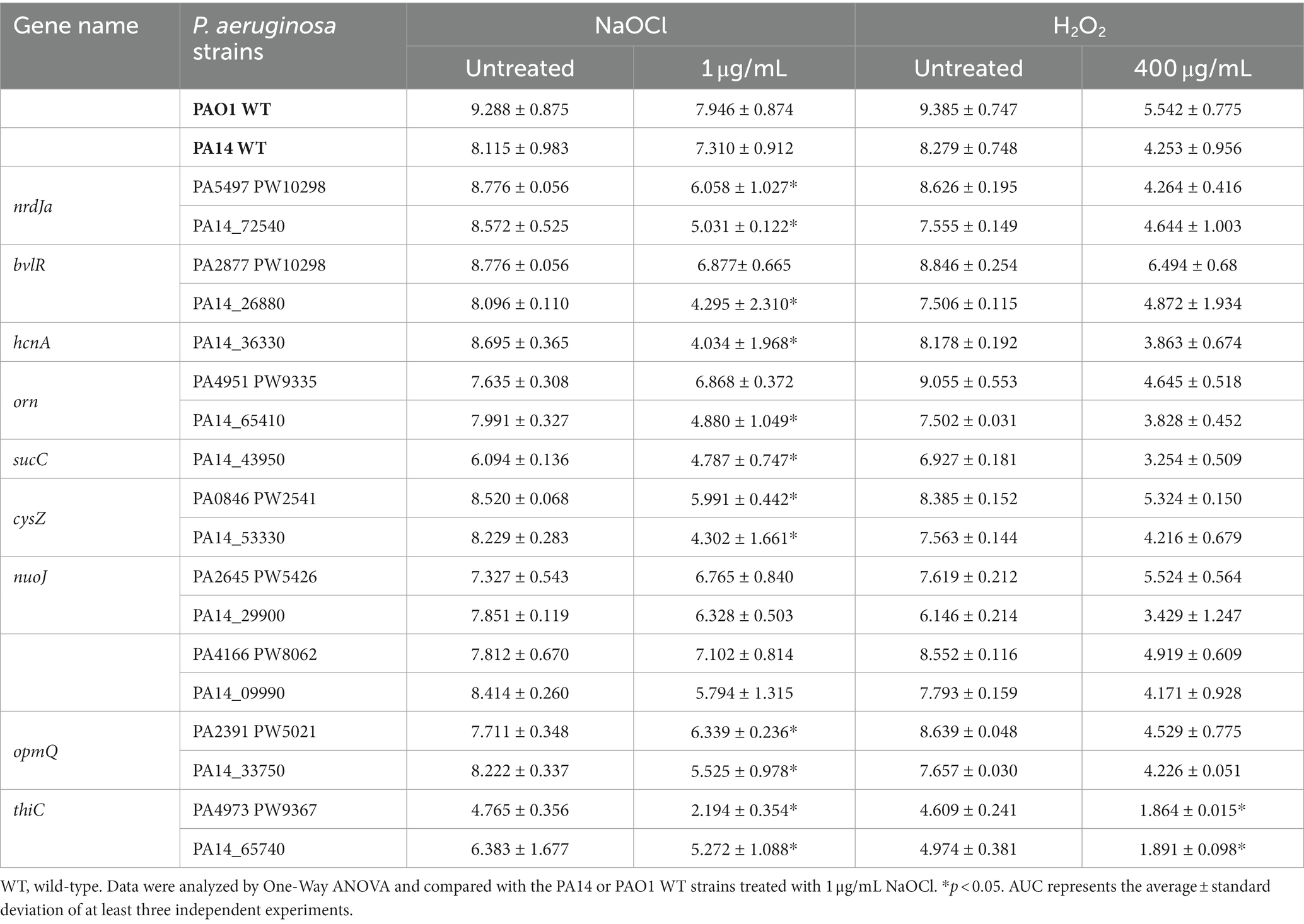
Table 3. Area under the curve (AUC) of NaOCl and H2O2 growth curves of NaOCl-susceptible Pseudomonas aeruginosa strains identified in the screening.
Most of the NaOCl responses previously reported in other studies are not specific to NaOCl but are rather employed by bacteria to survive the stress caused by different oxidizing agents (Da Cruz Nizer et al., 2021). Therefore, we conducted growth kinetics over time of the PA14 mutants identified and their PAO1 homologs to investigate if these genes are NaOCl-specific by exposing the mutants to 400 μg/mL H2O2. This concentration was chosen based on the growth curve of PA14 and PAO1 WT strains previously conducted (Supplementary Figure S1). As shown in the growth analyzes of Figure 4 and the AUC values in Table 3, the PA14 and PAO1 homologs tested were not susceptible to H2O2 under our experimental conditions, except for ΔthiC, which did not grow in the presence of 400 μg/mL H2O2. These results indicate that the susceptibility phenotypes found in our experiments are rather specific to NaOCl under our experimental conditions.
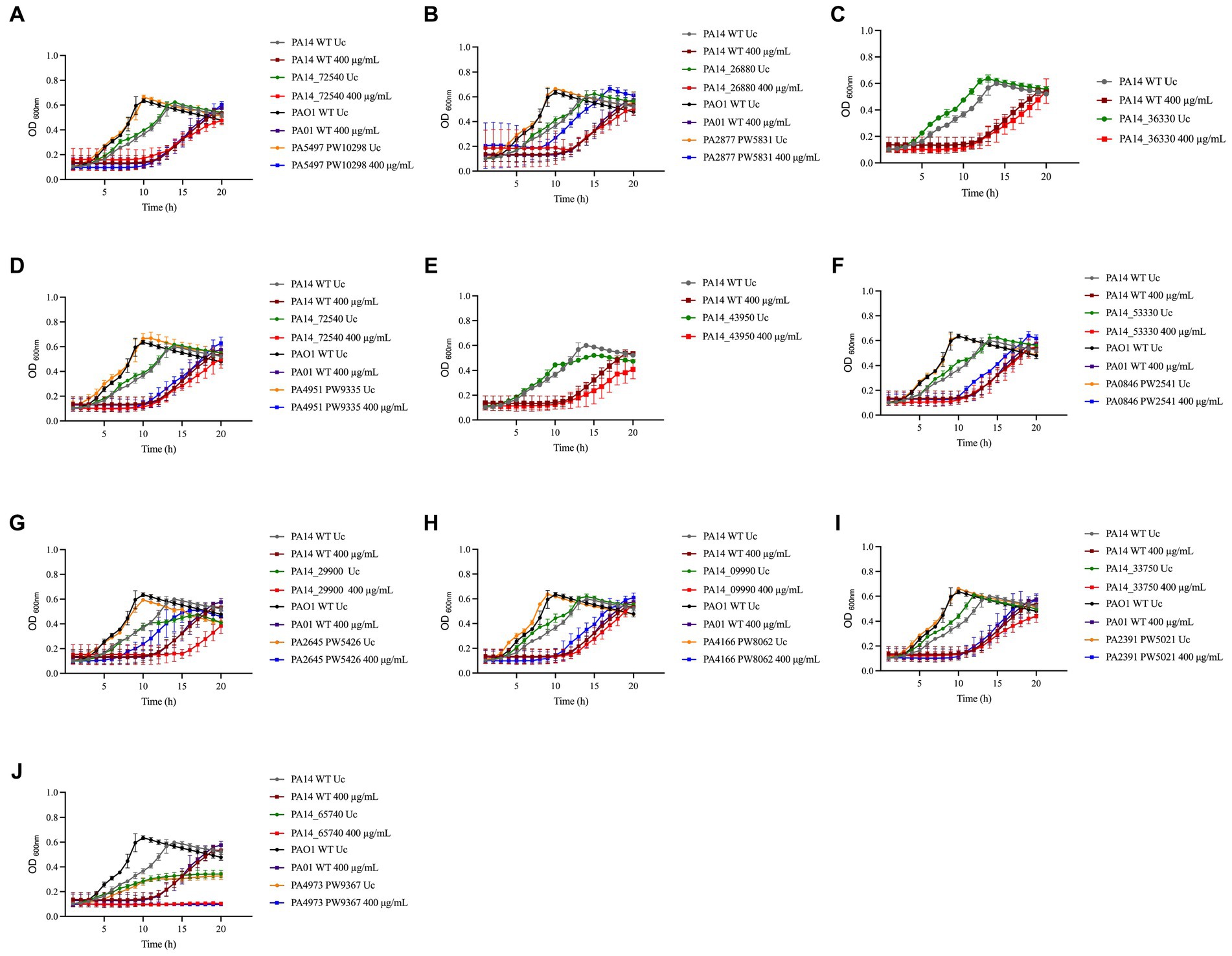
Figure 4. Susceptibility of PA14 mutants and PAO1 homologs identified in the screening to H2O2. Overnight cultures were grown in LB at 37°C and 220 rpm. Then, cells were washed twice, resuspended in BM2 minimal medium, and treated with H2O2 at 400 μg/mL (final cell concentration of 1 × 108 CFU/mL) for 20 h at 37°C. OD600nm was recorded every hour using an epoch plate reader. (A) ΔnrdJa (PA14_72540/PA5497); (B) ΔbvlR (PA14_26880/PA2877); (C) ΔhcnA (PA14_36330); (D) Δorn (PA14_65410/PA4951); (E) ΔsucC (PA14_43950); (F) ΔcysZ (PA14_53330/PA0846); (G) ΔnuoJ (PA14_29900/PA2645); (H) PA14_09990/PA4166; (I) ΔopmQ (PA14_33750/PA2391); (J) ΔthiC (PA14_65740/PA4973). WT: wild-type; Uc: untreated control.
3.4. HCN affects NaOCl resistance in Pseudomonas aeruginosa
Among the mutants identified in our screening and follow-up MIC and growth analyzes (Table 2) was the ΔhcnA mutant, which presented increased susceptibility to NaOCl. For instance, it took approximately 11 h for the ΔhcnA mutant to reach an OD600nm of 0.2, while the PA14 WT strain grew to an OD600nm of 0.2 in only 5–6 h. After 20 h of growth, the ΔhcnA mutant exhibited an OD600nm of 0.382, while the PA14 WT showed an OD600nm of 0.6. Furthermore, the ΔhcnA mutant presented a MIC of 0.5 μg/mL, which was ¼ x MIC of PA14 WT. Given this pronounced increase in susceptibility, we focused the following analyzes on HCN and its contribution to NaOCl resistance.
Since HCN is produced by the hcnABC gene cluster in P. aeruginosa (Gilchrist et al., 2011), we evaluated if the absence of hcnB and hcnC also affects the susceptibility of P. aeruginosa to NaOCl by analyzing the growth of the corresponding PA14 and PAO1 ΔhcnB and ΔhcnC mutants at 1 μg/mL NaOCl for 20 h at 37°C. Like the ΔhcnA PA14 mutant, the corresponding PA14 and PAO1 ΔhcnB and ΔhcnC mutants showed an increase in susceptibility to NaOCl, presenting extended lag phase and reduced AUC compared to the WT strains at 1 μg/mL NaOCl. This phenotype seemed specific to NaOCl since these mutants did not present altered susceptibility to H2O2 at a final concentration of 400 μg/mL compared to WT strains (Figure 5; Table 4). These results confirm the importance of HCN production for P. aeruginosa survival under NaOCl stress conditions.
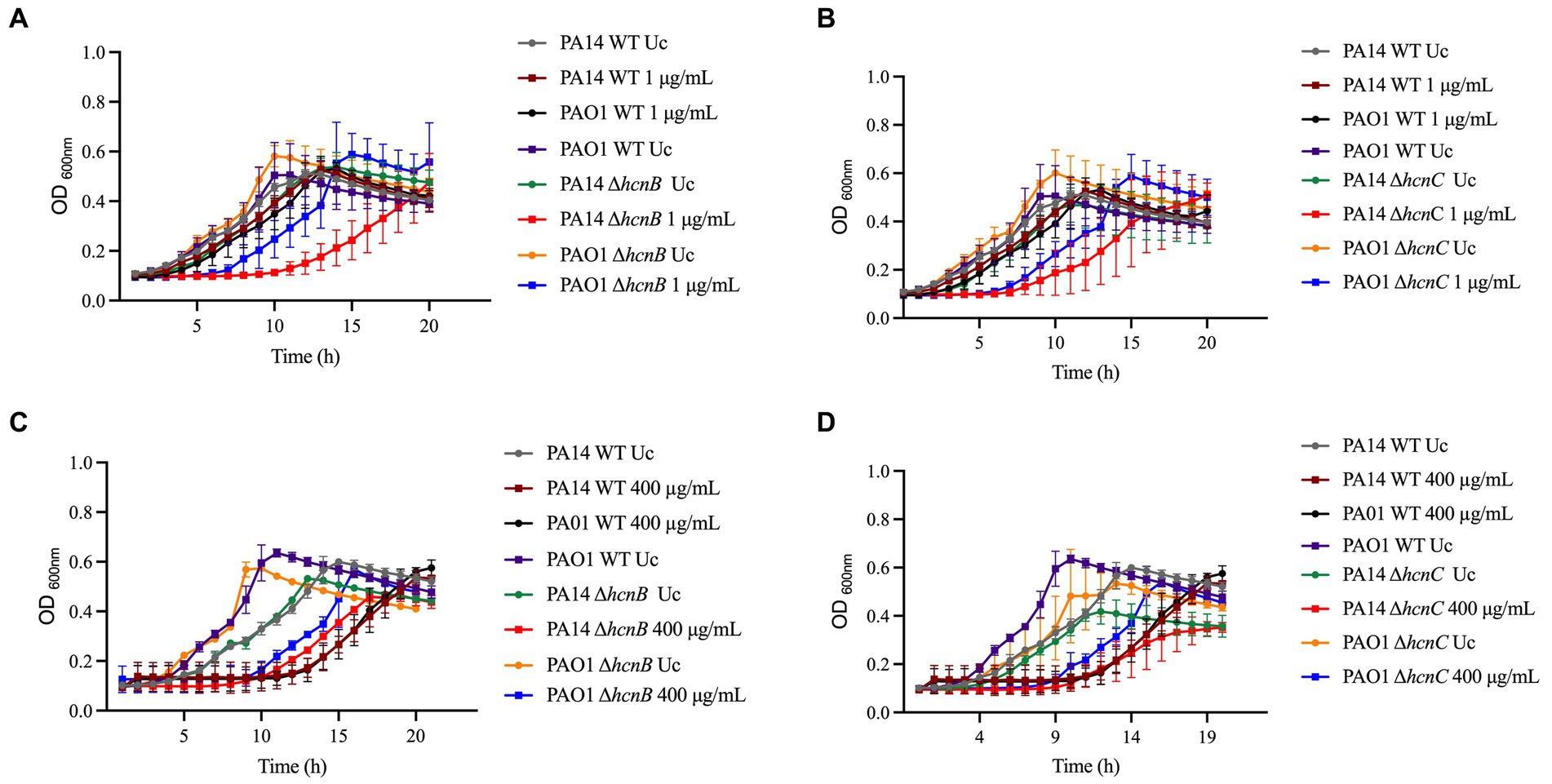
Figure 5. Susceptibility of hcn PA14 and PAO1 mutants to NaOCl and H2O2. Susceptibility to NaOCl of (A) ΔhcnB and (B) ΔhcnC PA14 and PAO1 mutants. Susceptibility to H2O2 of (C) ΔhcnB and (D) ΔhcnC PA14 and PAO1 mutants. Overnight cultures were grown in LB at 37°C and 220 rpm. Then, cells were washed twice, resuspended in BM2 minimal medium, and treated with NaOCl at 1 μg/mL or H2O2 at 400 μg/mL (final cell concentration of 1 × 108 CFU/mL) for 20 h at 37°C. OD600nm was recorded every hour using an epoch plate reader. WT: wild-type; Uc: untreated control.
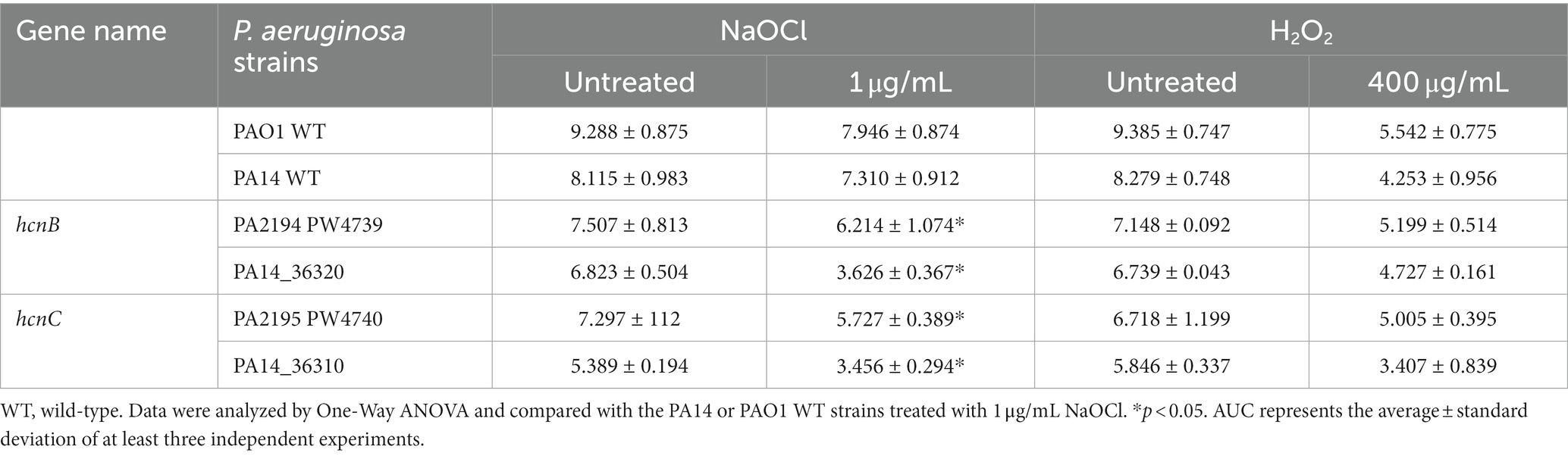
Table 4. Area under the curve (AUC) of NaOCl and H2O2 growth curves of hcn Pseudomonas aeruginosa mutants.
To test whether the increased susceptibility of the hcn mutants was due to the absence of HCN, we complemented the PAO1 mutant PA2194 PW4739 (ΔhcnB) by the transfer of the phcnBC plasmid, which expresses the genes hcnBC. Since both PA14 and PAO1 mutants presented increased susceptibility to NaOCl, we focused our analyzes on the PAO1 ΔhcnB mutant since this mutant has been characterized in a recent study (Létoffé et al., 2022). We assessed if the ΔhcnB mutant could be complemented by evaluating the release of HCN by our PAO1 strains using a semiquantitative method for HCN detection (Létoffé et al., 2022). When grown in LB for 24 h, both PAO1 WT and the ΔhcnB-phcnBC produced a detectable amount of HCN, while the ΔhcnB mutant did not produce HCN (Figure 6A).
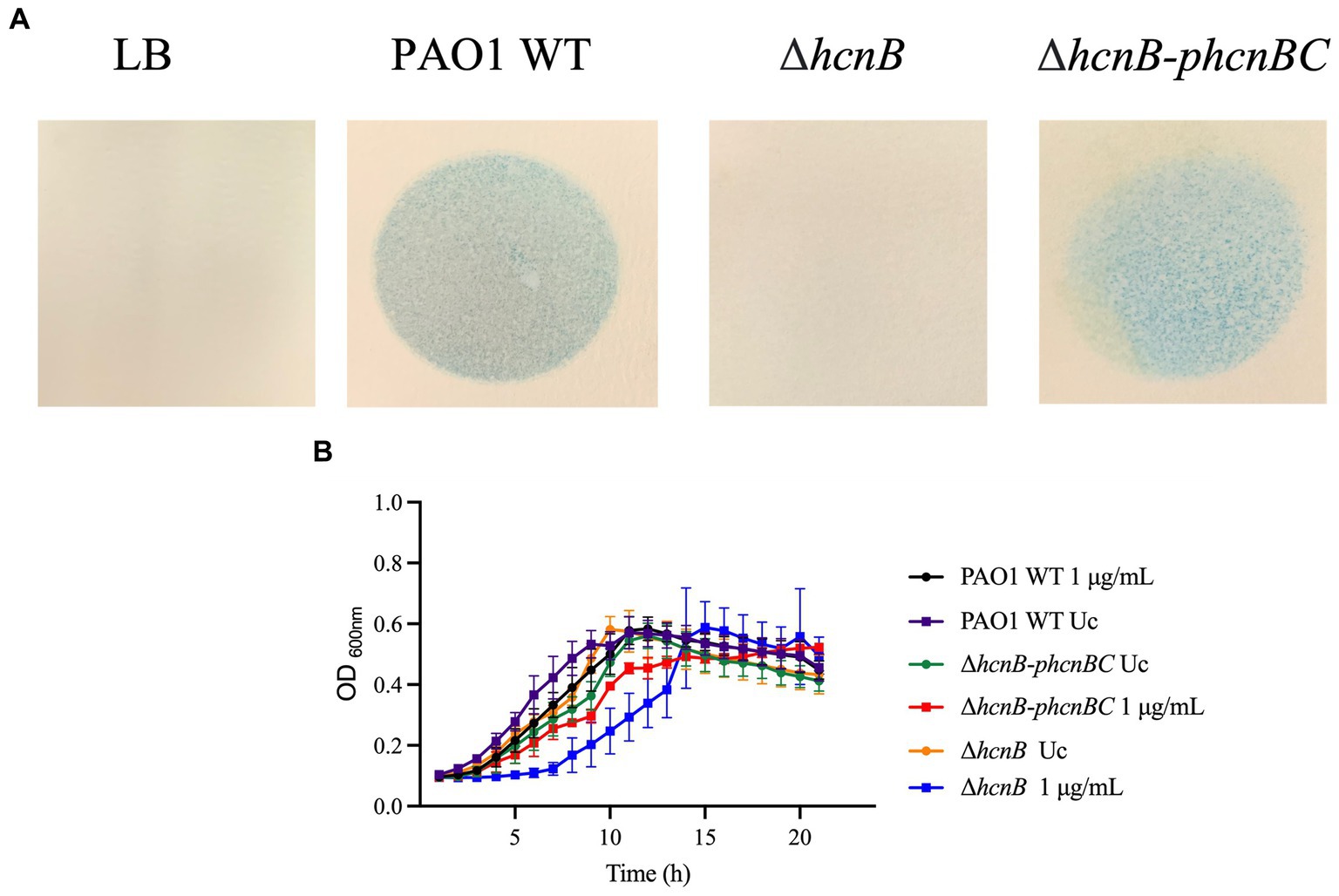
Figure 6. (A) Semiquantitative detection of HCN production by P. aeruginosa strains. cultures were grown in LB at 37°C and 220 rpm. Then, cells were washed twice and resuspended in LB. Two mL of overnight cultures were transferred to a small petri dish, which was covered by chromatography paper soaked in HCN detection reagent composed of copper (II) ethyl acetoacetate and 4,4′-methylenebis-(N,N-dimethylaniline) solubilized in chloroform. The small plates were placed in larger petri dish plates, which were covered. Production of volatile HCN was measured as the formation of blue color in the chromatography paper. The figures are representative of at least two independent experiments. (B) Susceptibility ΔhcnB-phcnBC complemented strain to NaOCl compared to PAO1 WT and PAO1 ΔhcnB. Overnight cultures were grown in LB at 37°C and 220 rpm. Then, cells were washed twice, resuspended in BM2 minimal medium, and treated with NaOCl at 1 μg/mL or (final cell concentration of 1 × 108 CFU/mL) for 20 h at 37°C. OD600nm was recorded every hour using an epoch plate reader. WT, wild-type; Uc, untreated control.
Then, we conducted growth kinetic analyzes to determine whether the production of HCN by P. aeruginosa increases its resistance to NaOCl. As shown in Figure 6B, it took 6 h for the complemented strain ΔhcnB-phcnBC to reach the OD600nm of 0.2, similar to the PAO1 WT strain. Furthermore, no statistical difference in growth was found for the AUC of ΔhcnB-phcnBC and PAO1 WT when treated with 1 μg/mL NaOCl (Table 5). Together, these results indicate that growth delay in response to NaOCl in the PAO1 ΔhcnB mutant could be complemented by the insertion of the hcnBC-producing plasmid phcnBC, demonstrating that HCN plays a role in the resistance of P. aeruginosa to NaOCl.
3.5. Characterization of HCN-mediated resistance to NaOCl in Pseudomonas aeruginosa
In order to provide further insight into the underlying mechanism of the HCN phenotype found in this study, we formulated two hypotheses: (i) HCN-related NaOCl resistance is mediated by cellular effects caused by HCN, or (ii) HCN acts as an extracellular metabolite, directly reacting with NaOCl and quenching its antimicrobial effect. In a previous study, Frangipani et al. (2014) investigated the effect of endogenously produced HCN on P. aeruginosa by transcriptomic analysis. The authors identified four P. aeruginosa genes that were repressed in response to endogenously produced HCN and 12 genes induced in response to HCN. To test if any of these HCN-controlled genes are involved in NaOCl resistance, we conducted growth analyzes by exposing P. aeruginosa PAO1 strains with mutations on the identified genes to 1 μg/mL NaOCl and measured their growth for 20 h. Among the mutants tested, only the mutant with a mutation in the PA4134 gene, which synthesizes a hypothetical protein with unknown function, presented statistically different AUC compared to the PAO1 WT strain treated with NaOCl (Supplementary Figure S2; Table 6). This mutant strain presented an AUC (5.785 ± 3.059) similar to ΔhcnB (6.214 ± 1.074). The PA4134 gene forms a gene cluster together with PA4133, with PA4133 being located upstream of PA4134;1 however, the PA4133 mutant did not show any significant difference in NaOCl susceptibility.
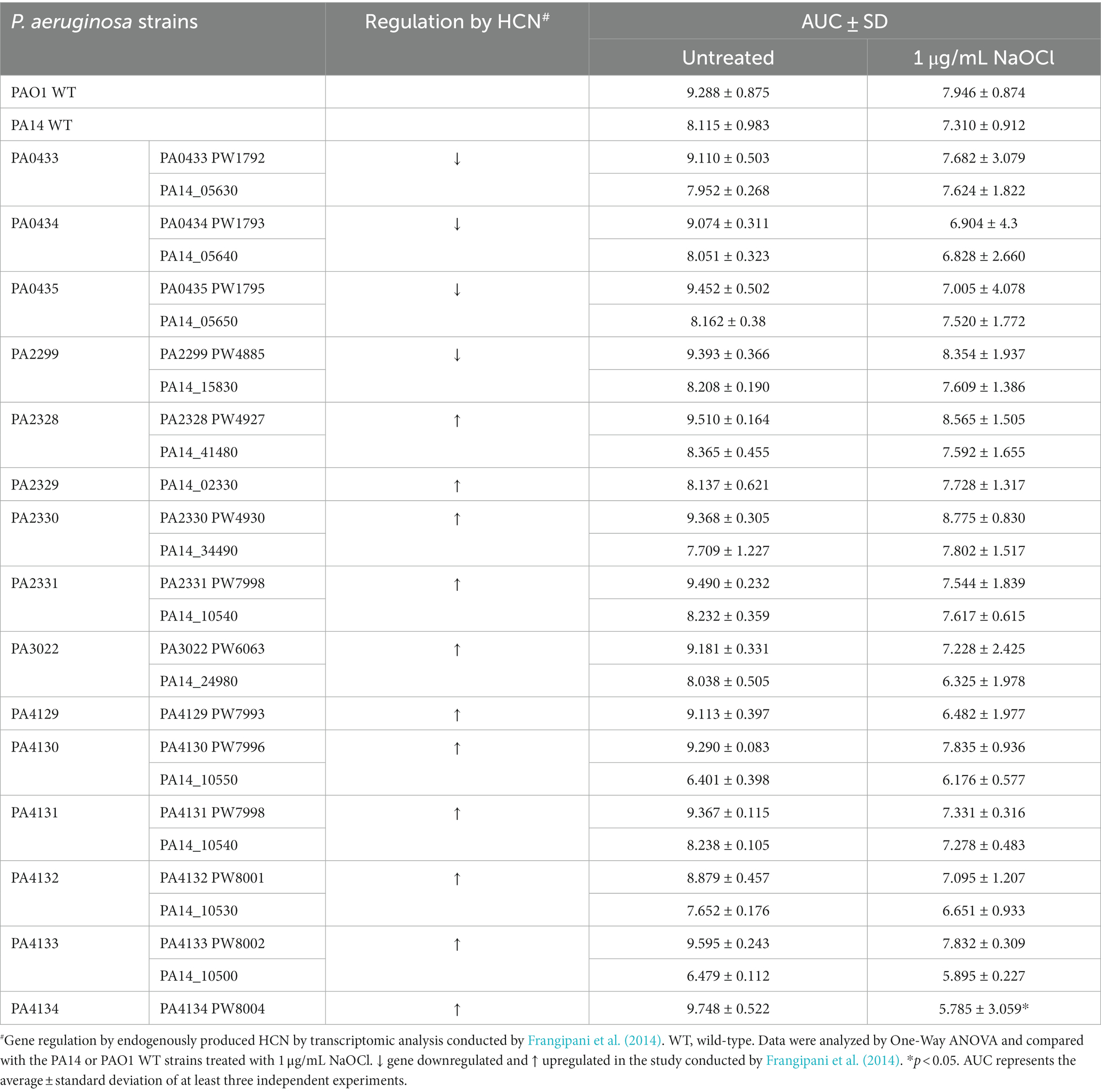
Table 6. Area under the curve (AUC) of NaOCl growth curves of Pseudomonas aeruginosa PAO1 and PA14 strains.
We then tested if the metabolite HCN itself would directly react with NaOCl and quench its toxic effect. For this, we first analyzed if removing HCN from the medium would change the susceptibility of ΔhcnB and PAO1 WT strains by evaluating the kill kinetics in response to NaOCl. Cells grown overnight were washed twice, resuspended in PBS to remove the HCN from the medium, and NaOCl was added to a final concentration of 2 μg/mL. The absence of HCN in the medium did not provoke a difference in the NaOCl susceptibility of PAO1 WT and ΔhcnB (Figure 7A), in which no statistical difference was found for the cell concentration over time for both strains. Overall, the growth analyzes and kill-kinetic results suggested that the susceptibility phenotype found for ΔhcnB is likely not due to a cellular effect.
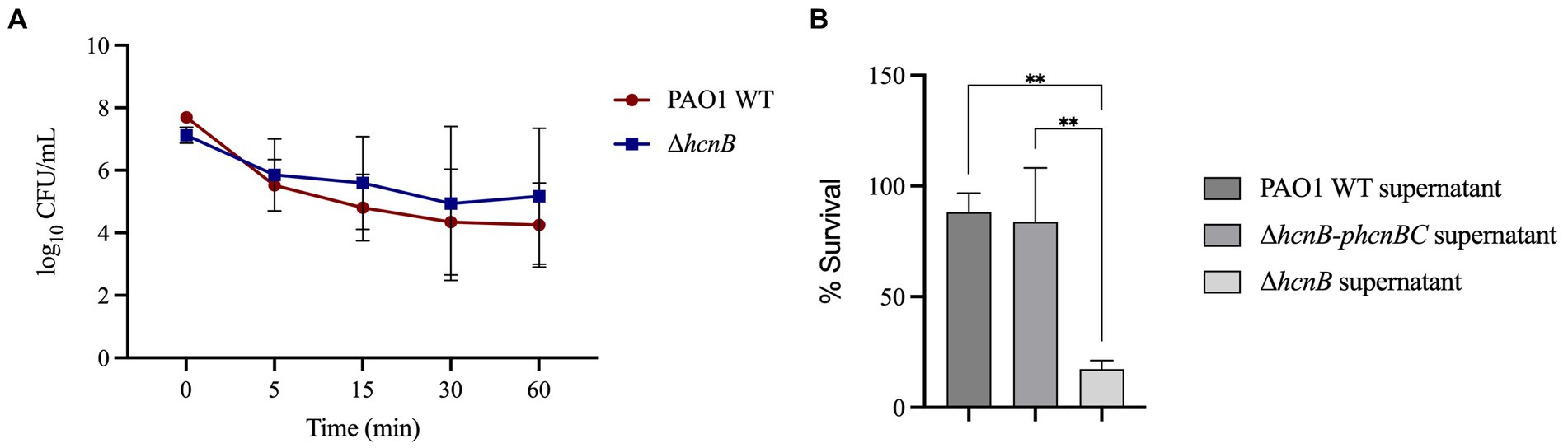
Figure 7. Effect of exogenous HCN on the susceptibility of P. aeruginosa to NaOCl. (A) PAO1 WT and ΔhcnB were grown overnight, washed twice, and resuspended in PBS. The OD600nm was adjusted to 0.1, and the cell suspension was treated with 2 μg/mL NaOCl for 5, 15, 30, and 60 min. Then, CFU counts were determined by the drop plate method. (B) ΔhcnB, PAO1 WT, and ΔhcnB-phcnBC were grown overnight in BM2, and the supernatant was collected by centrifugation followed by filtration in a 0.22 μm filter. ΔhcnB overnight cells were resuspended in each supernatant to an OD600nm of 0.1, treated with NaOCl at 4 μg/mL, and the CFU counts were determined by the drop plate method. ** p < 0.01.
Next, we aimed to complement the susceptibility phenotype of ΔhcnB with the supernatant from PAO1 WT and the complemented strain ΔhcnB-phcnBC. We used the culture supernatant of HCN-producing strains instead of the pure chemical HCN due to the high toxicity of HCN and its related forms [e.g., sodium cyanide (NaCN) and potassium cyanide (KCN)]. For this, we resuspended overnight ΔhcnB cells in the supernatants of ΔhcnB, PAO1 WT, and ΔhcnB-phcnBC grown in BM2 minimal medium. Then, the bacterial suspensions were treated with NaOCl at 4 μg/mL for 60 min. Figure 7B shows that the addition of PAO1 WT and ΔhcnB-phcnBC supernatants to cells of ΔhcnB complemented the ΔhcnB phenotype, and ΔhcnB cells showed increased resistance to NaOCl (percentage survival of 88 and 83%, respectively) compared to the ΔhcnB cells resuspended in ΔhcnB supernatant (17% survival). These results suggest that HCN reacts with NaOCl, quenching its lethal effect.
4. Discussion
Bacteria have developed several mechanisms to mitigate the harmful and often irreversible damage caused by oxidizing agents. Most of these resistance mechanisms are not specific but rather provide a general defense against a broad range of oxidizing agents. In this context, the knowledge of HOCl-specific responses is still limited (Gray et al., 2013; da Cruz Nizer et al., 2020). Among the HOCl responses described so far, ATP-independent chaperones are considered the immediate response against HOCl since they are readily activated by the oxidation of amino acid residues. These enzymes are essential for HOCl response since they do not require the expression of sensor mechanisms, which takes a long time compared to the fast action of HOCl on proteins (Goemans and Collet, 2019; Sultana et al., 2020). Other mechanisms include the activation of the transcriptional regulators HypT, NemR, and RclR and the formation of biofilms (Gray et al., 2013; Strempel et al., 2017; Da Cruz Nizer et al., 2020).
In this study, we demonstrated that the loss of KatA, KatE, AhpC, AhpF, and MsrA increases the susceptibility of P. aeruginosa PAO1 and PA14 to NaOCl compared to the WT strains, showing that these H2O2 responses are employed by this bacterium as a general response against oxidizing agents. We then identified 48 mutants with increased susceptibility to NaOCl and characterized 10 mutants in more detail. Among them, we found that HCN acts as a scavenger molecule and increases the survival of P. aeruginosa in the presence of NaOCl.
Most oxidative stress responses have been characterized for H2O2 and other ROS, while their roles in RCS and NaOCl resistance remain mostly unknown. Therefore, in the first part of this study, we showed by growth kinetics analyzes that well-known H2O2 responses are also involved in the survival of P. aeruginosa to NaOCl. Catalases and peroxidases are specialized enzymes that convert H2O2 into less toxic species (i.e., H2O + O2 and alcohol + H2O, respectively; Cavinato et al., 2020). These enzymes are widely distributed among bacteria (Yuan et al., 2021) and are the primary response against oxidative stress (Romsang et al., 2013). P. aeruginosa has three catalases (katA, katB, and katE) and four alkyl hydroperoxide reductases (ahpA, ahpB, ahpCF, and ohr), in which KatA is considered the main catalase and its expression is controlled by various systems, such as OxyR, quorum sensing, and ANR (anaerobic regulator; Heo et al., 2010; Su et al., 2014). KatA and AhpA are continually expressed during bacterial growth, implying their importance as a defense not only during harmful conditions but also against endogenously produced ROS (Ochsner et al., 2000; Lee et al., 2005). Many studies have shown that the production of these detoxifying systems is upregulated by oxidizing agents in P. aeruginosa, such as H2O2 (Salunkhe et al., 2002; Palma et al., 2004; Chang et al., 2005; Small et al., 2007a,b), NaOCl (Small et al., 2007a,b; Groitl et al., 2017), hypobromous acid (Groitl et al., 2017), hypothiocyaous acid (Groitl et al., 2017) and peracetic acid (Chang et al., 2005). Furthermore, P. aeruginosa katA, katB, ahpB, and oxyR mutant strains were consistently more susceptible to H2O2 than the WT strains (Ochsner et al., 2000; Lee et al., 2005), corroborating our results. This susceptibility phenotype of kat and ahp mutants was also reported for other bacterial strains, such as Vibrio cholerae (Wang et al., 2012) and Stenotrophomonas maltophilia (Li et al., 2020). In the context of HOCl resistance, little has been explored on the roles of these enzymes in the resistance of P. aeruginosa toward this oxidant. The importance of detoxifying enzymes such as catalases has been described for Escherichia coli and Helicobacter pylori (Dukan and Touati, 1996; Benoit and Maier, 2016), in which catalases are considered a ubiquitous enzymes with quenching ability toward oxidizing agents in general (Benoit and Maier, 2016), corroborating our findings for P. aeruginosa. Considering that proteins are the main target of HOCl, another important stress response mechanism is the protein repair system Msr. P. aeruginosa and most bacterial species have two highly conserved Msr systems: MsrA and MsrB (Romsang et al., 2013). As for KatA and AhpA, MsrA is expressed during all growth phases, while MsrB is overproduced under oxidative stress conditions (Romsang et al., 2013). In accordance with our PA14 susceptibility results, PAO1 msrA and msrB mutants presented increased susceptibility to H2O2 and NaOCl in the study by Romsang et al. (2013). Hence, our findings report the involvement of kat, ahp, and msr genes in NaOCl resistance, adding to the previously described function of these response mechanisms.
We then performed a genome-wide mutant library screening of the PA14 transposon mutant library (Liberati et al., 2006) to find mutants with increased susceptibility to NaOCl. We were able to identify 48 genes with reduced MIC values toward NaOCl compared to PA14 WT, and we characterized 10 mutants (disrupted nrdJa, bvlR, hcnA, orn, sucC, cysZ, nuoJ, PA4166, opmQ, and thiC gene, respectively) in more detail (Table 2). Library screenings allow access to a large number of mutants carrying specific genetic alterations; therefore, researchers can simultaneously screen multiple mutants for the phenotype of interest in a short period. This approach is valuable for uncovering novel genes and pathways that contribute to bacterial resistance (Moser et al., 2014). Among them, two mutant strains, ΔcysZ and ΔopmQ, lacked genes involved in the transport of small molecules. The first one (ΔcysZ) has a mutation in a sulfate uptake protein. Proteins, mainly the sulfur-containing ones, are the main target of HOCl in the cells (Da Cruz Nizer et al., 2020); therefore, due to the reduction of the amount of sulfur in the cells due to its reaction with HOCl, the transport of this compound to the cells seems to be necessary (Farrant et al., 2020). The upregulation of transport and metabolism of sulfur genes by HOCl has also been described for E. coli and Salmonella enterica Serovars Enteritidis (Wang et al., 2009, 2010). Overexpression of genes involved in the transport of small molecules (Small et al., 2007a,b) has been detected by transcriptomic studies and has been implicated in the need of cells to allow the entry or exit of metabolites, such as toxic HOCl-by-products and compounds needed for cell metabolism (Albrich et al., 1986).
In accordance with previous reports about the oxidation of DNA by HOCl (Prütz, 1996), we found the nrdJa gene, which encodes for a ribonucleotide reductase and was previously reported to be involved in DNA repair (Torrents et al., 2005). NrdJa is crucial for growth under anaerobic conditions (Wu et al., 2005; Filiatrault et al., 2013) and was upregulated after ciprofloxacin exposure (Cirz et al., 2006). Furthermore, Crespo et al. (2017) also found increased transcription of nrdJ under H2O2 stress, supporting our findings (Crespo et al., 2017). Ribonucleotide reductases have also been shown to be used by many other bacteria, such as Bifidobacterium longum (Zuo et al., 2018), Bacillus subtilis (Castro-Cerritos et al., 2017) and E. coli (Monje-Casas et al., 2001) as a response mechanism to oxidative stress, mainly H2O2.
Considering the high metabolic diversity of P. aeruginosa, many genes and pathways remain to be explored regarding their secondary effects and possible roles in resistance. In this context, we identified two genes involved in energy metabolism (i.e., sucC and nuoJ) and one implicated in thiamine biosynthesis (thiC). Thiamine, for example, has been explored as a target in the development of antibiotics (Lünse et al., 2014; Kim et al., 2020), while energy metabolism genes, such as sucC and nuoH, nuoM, and nuoN have been shown to be downregulated in response to tobramycin in A. baumannii (Kashyap et al., 2022). Furthermore, transcriptomic studies have shown the downregulation of energy production genes in cells under HOCl (Small et al., 2007a,b). However, their contribution to oxidative stress remains to be elucidated.
In our screening, we also identified genes involved in the pathogenicity of P. aeruginosa by controlling virulence factors and resistance production (bvlR and orn). BvlR is a transcriptional repressor that belongs to the LysR-type transcriptional regulator (LTTR) family. It is upregulated during exposure to epithelial cells (Frisk et al., 2004) and controls several virulence factors in P. aeruginosa, indicating a role in the pathogenicity of this bacterium. BvlR represses the expression of the type 3 secretion system (T3SS), cupA-associated fimbrial-based surface attachment, and toxin A. Furthermore, it was shown to promote tight microcolony formation, which is associated with the formation of biofilms in the lung of cystic fibrosis (CF) patients (McCarthy et al., 2014). The oligorribonuclease Orn also affects T3SS production (Chen et al., 2016) and contributes to bacterial resistance to fluoroquinolones by a pyocin-mediated mechanism (Chen et al., 2017) and aminoglycosides, β-lactams and oxidative stress by influencing the translation of katA mRNA (Xia et al., 2019).
Another finding in our screening was a mutant with a disrupted hcnA gene. The absence of HCN in our P. aeruginosa mutant strains increased their susceptibility to NaOCl, while the complementation of ΔhcnB with an HCN-producing plasmid recovered the phenotype in our growth kinetic analyzes. HCN is a toxic volatile secondary metabolite as it has no apparent function in primary metabolism and is produced at later stages during the exponential phase and offers an advantage for the producing strain, which is tolerant to it (Blumer and Haas, 2000). It is synthesized by many bacterial genera, including Alcaligenes, Aeromonas, Bacillus, Pseudomonas, and Rhizobium (Blumer and Haas, 2000; Abd El-Rahman et al., 2019). In P. aeruginosa, HCN is synthesized by the HCN synthase, encoded by the hcnABC operon, and regulated by quorum sensing and the ANR regulator (Pessi and Haas, 2000). P. aeruginosa cultures produce up to 300 μM of HCN by the decarboxylation of glycine, and the hcnABC operon is induced by low oxygen (Blumer and Haas, 2000) and high cell density, with maximum production at the end of the exponential phase (Blier et al., 2012). The toxic effect of HCN is due to the inhibition of cytochrome c oxidase, impairing cell oxygen consumption and energy production (Zuhra and Szabo, 2022). P. aeruginosa has two systems to avoid HCN intoxication. One involves a cyanide-insensitive terminal oxidase, CioAB, which allows aerobic respiration in the presence of cyanide (Cunningham et al., 1997) and the other system is the enzyme rhodonase, which forms thiocyanate by the reaction with HCN (Cipollone et al., 2007).
HCN has been detected in the breath (Enderby et al., 2009; Smith et al., 2013) and the sputum and lung of CF patients (Ryall et al., 2008; Sanderson et al., 2008), suggesting that P. aeruginosa could also employ this metabolite as a virulence factor to increase its pathogenicity. Due to its high toxicity, HCN also exerts a toxic effect on non-producing strains (Zdor, 2015; Biswas and Götz, 2022). In this context, HCN produced by P. aeruginosa has been shown to control the growth of S. aureus, contributing to P. aeruginosa competition (Létoffé et al., 2022). Here we show that HCN also contributes to the survival of P. aeruginosa to the strong antioxidant NaOCl. Recently, studies have shown the effect of signaling molecules on the resistance profile of bacterial species (Li et al., 2022). Therefore, to investigate the HCN-mediated response to NaOCl in P. aeruginosa, we first tested the hypothesis that the production of HCN induces cellular mechanisms that, in turn, activate resistance mechanisms. For instance, indole produced by bacterial species induces bacterial resistance endogenously and exogenously by many mechanisms, including efflux pump regulation, biofilm formation, and induction of the persister state (Li et al., 2022). We then tested a list of P. aeruginosa genes that were up or down-regulated under endogenously produced HCN (Frangipani et al., 2014). Except for the gene PA4134, no NaOCl susceptibility was found for the mutant strains tested, suggesting that the NaOCl phenotype found in this study is likely not due to cellular regulation.
We then assessed if the NaOCl susceptibility found was due to the reaction of HCN with NaOCl and found evidence supporting the scavenger effect of HCN in the presence of NaOCl, quenching the toxic effect of this oxidizing agent. Due to its high reactivity, HOCl is known to react rapidly with sulfur- and nitrogen-containing compounds, producing chlorinated derivatives with impaired functions (Winterbourn, 1985; Pattison et al., 2012). In this context, the reaction between HCN and HOCl, the active ingredient of NaOCl in aqueous solution (Fukuzaki, 2006), forms CO2, N2, and HCl. However, due to its toxicity, we could not evaluate if adding HCN to P. aeruginosa would rescue the NaOCl-susceptibility phenotype found for the ΔhcnB mutant, and we used different supernatants from WT and HCN-deficient strains as an alternative approach.
It is believed that the production of HCN by bacteria serves various purposes, including defense mechanisms, helping the bacteria compete with other microorganisms for resources, and antimicrobial effect, inhibiting the growth of other microorganisms. Here, we have identified a new role for HCN produced by pathogenic bacteria as a NaOCl scavenger molecule, contributing to bacterial resistance under NaOCl stress conditions. We hypothesize that HCN is produced in the context of P. aeruginosa infection, such as wound infection in which the environment presents low oxygen levels, favoring anaerobic microorganisms (Versey et al., 2021), and helping in the fight against oxidative stress produced by the immune system or from exogenous sources. Many bacteria produce a wide array of virulence factors and secreted metabolites. Many of these secreted molecules have been shown to quench and neutralize the toxic effect of oxidizing agents. For example, melanin produced by bacteria is a free radical scavenger (Sichel et al., 1991; Agodi et al., 1996) that protects bacterial cells against oxidative stress (Rodríguez-Rojas et al., 2009; Thippakorn et al., 2018). The search for molecules that act as scavengers for antimicrobial agents or immune system factors and understanding these metabolites help develop new strategies to eradicate P. aeruginosa and fight infections and the spread of this bacterium.
Of note, due to the high reactivity of NaOCl and its active ingredient, HOCl, the chemistry behind the formation and consumption of RCS in media is complex (Peskin et al., 2005; Pattison and Davies, 2006). For instance, rich media such as LB have been shown to completely quench the oxidizing effect of HOCl, while in some buffers such as PBS, no change in the levels of RCS was detected (Ashby et al., 2020). In this context, in minimal growth media containing amine, chloramines can be formed by the chlorination of amino groups by the chlorine present in HOCl (Pál Fehér et al., 2019). Therefore, the amount of HOCl and other RCS will depend on the type of media used and their ability to quench HOCl as well as to produce other RCS.
5. Conclusion
Although much effort has been made to uncover the mechanisms employed by bacteria to resist oxidative stress, most of the studies have focused on H2O2, and the knowledge on adaption to RCS, including NaOCl, is still in its infancy. Our PA14 mutant library screening identified 48 genes and showed that P. aeruginosa relies on diverse mechanisms to survive the potent and often irreversible stress caused by NaOCl. Among them, we identified the hcnA gene and showed that HCN contributes to the resistance of P. aeruginosa by quenching the toxic effect of NaOCl. To our knowledge, this is the first study reporting the roles of HCN in NaOCl resistance.
Data availability statement
The original contributions presented in the study are included in the article/Supplementary material, further inquiries can be directed to the corresponding author.
Author contributions
WSdCN: Conceptualization, Formal analysis, Investigation, Methodology, Project administration, Visualization, Writing – original draft, Writing – review & editing. MEA: Formal analysis, Investigation, Methodology, Writing – original draft. VI: Formal analysis, Investigation, Methodology, Writing – original draft. CB: Methodology, Writing – original draft, Investigation. JO: Methodology, Writing – original draft, Conceptualization, Funding acquisition, Project administration, Resources, Supervision, Writing – review & editing.
Funding
The author(s) declare financial support was received for the research, authorship, and/or publication of this article. This research was funded by Carleton University and the Natural Sciences and Engineering Research Council of Canada (NSERC, RGPIN-2019-06335).
Acknowledgments
We would like to thank Jean-Marc Ghigo for providing the phcnBC plasmid used in this study.
Conflict of interest
The authors declare that the research was conducted in the absence of any commercial or financial relationships that could be construed as a potential conflict of interest.
Publisher’s note
All claims expressed in this article are solely those of the authors and do not necessarily represent those of their affiliated organizations, or those of the publisher, the editors and the reviewers. Any product that may be evaluated in this article, or claim that may be made by its manufacturer, is not guaranteed or endorsed by the publisher.
Supplementary material
The Supplementary material for this article can be found online at: https://www.frontiersin.org/articles/10.3389/fmicb.2023.1294518/full#supplementary-material
Footnotes
References
Abd El-Rahman, A. F., Shaheen, H. A., Abd El-Aziz, R. M., and Ibrahim, D. S. S. (2019). Influence of hydrogen cyanide-producing rhizobacteria in controlling the crown gall and root-knot nematode, Meloidogyne incognita. Egypt. J. Biol. Pest Control 29:41. doi: 10.1186/s41938-019-0143-7
Agodi, A., Stefani, S., Corsaro, C., Campanile, F., Gribaldo, S., and Sichel, G. (1996). Study of a melanic pigment of Proteus mirabilis. Res. Microbiol. 147, 167–174. doi: 10.1016/0923-2508(96)80216-6
Albrich, J. M., Gilbaugh, J. H., Callahan, K. B., and Hurst, J. K. (1986). Effects of the putative neutrophil-generated toxin, hypochlorous acid, on membrane permeability and transport systems of Escherichia coli. J. Clin. Invest. 78, 177–184. doi: 10.1172/JCI112548
Ambreetha, S., and Balachandar, D. (2022). Pathogenesis of plant-associated Pseudomonas aeruginosa in Caenorhabditis elegans model. BMC Microbiol. 22:269. doi: 10.1186/s12866-022-02682-z
Ashby, L. V., Springer, R., Hampton, M. B., Kettle, A. J., and Winterbourn, C. C. (2020). Evaluating the bactericidal action of hypochlorous acid in culture media. Free Radic. Biol. Med. 159, 119–124. doi: 10.1016/j.freeradbiomed.2020.07.033
Bardaweel, S. K., Gul, M., Alzweiri, M., Ishaqat, A., ALSalamat, H. A., and Bashatwah, R. M. (2018). Reactive oxygen species: the dual role in physiological and pathological conditions of the human body. Eurasian J Med 50, 193–201. doi: 10.5152/eurasianjmed.2018.17397
Bassetti, M., Vena, A., Croxatto, A., Righi, E., and Guery, B. (2018). How to manage Pseudomonas aeruginosa infections. Drugs Context 7:212527, 1–18. doi: 10.7573/dic.212527
Benoit, S. L., and Maier, R. J. (2016). Helicobacter catalase devoid of catalytic activity protects the bacterium against oxidative stress. J. Biol. Chem. 291, 23366–23373. doi: 10.1074/jbc.M116.747881
Biswas, L., and Götz, F. (2022). Molecular mechanisms of Staphylococcus and Pseudomonas interactions in cystic fibrosis. Front. Cell. Infect. Microbiol. 11:4042. doi: 10.3389/fcimb.2021.824042
Blier, A.-S., Vieillard, J., Gerault, E., Dagorn, A., Varacavoudin, T., Le Derf, F., et al. (2012). Quantification of Pseudomonas aeruginosa hydrogen cyanide production by a polarographic approach. J. Microbiol. Methods 90, 20–24. doi: 10.1016/j.mimet.2012.04.005
Blumer, C., and Haas, D. (2000). Mechanism, regulation, and ecological role of bacterial cyanide biosynthesis. Arch. Microbiol. 173, 170–177. doi: 10.1007/s002039900127
Castric, P. A. (1977). Glycine metabolism by Pseudomonas aeruginosa: hydrogen cyanide biosynthesis. J. Bacteriol. 130, 826–831. doi: 10.1128/jb.130.2.826-831.1977
Castro-Cerritos, K. V., Yasbin, R. E., Robleto, E. A., and Pedraza-Reyes, M. (2017). Role of ribonucleotide reductase in Bacillus subtilis stress-associated mutagenesis. J. Bacteriol. 199, e00715–e00716. doi: 10.1128/JB.00715-16
Cavinato, L., Genise, E., Luly, F. R., Di Domenico, E. G., Del Porto, P., and Ascenzioni, F. (2020). Escaping the phagocytic oxidative burst: the role of SODB in the survival of Pseudomonas aeruginosa within macrophages. Front. Microbiol. 11:326. doi: 10.3389/fmicb.2020.00326
Chang, W., Small, D. A., Toghrol, F., and Bentley, W. E. (2005). Microarray analysis of Pseudomonas aeruginosa reveals induction of pyocin genes in response to hydrogen peroxide. BMC Genomics 6:115. doi: 10.1186/1471-2164-6-115
Chen, F., Chen, G., Liu, Y., Jin, Y., Cheng, Z., Liu, Y., et al. (2017). Pseudomonas aeruginosa Oligoribonuclease contributes to tolerance to ciprofloxacin by regulating Pyocin biosynthesis. Antimicrob. Agents Chemother. 61, e02256–e02216. doi: 10.1128/AAC.02256-16
Chen, G., Zhao, Q., Zhu, F., Chen, R., Jin, Y., Liu, C., et al. (2016). Oligoribonuclease is required for the type III secretion system and pathogenesis of Pseudomonas aeruginosa. Microbiol. Res. 188-189, 90–96. doi: 10.1016/j.micres.2016.05.002
Cipollone, R., Frangipani, E., Tiburzi, F., Imperi, F., Ascenzi, P., and Visca, P. (2007). Involvement of Pseudomonas aeruginosa Rhodanese in protection from cyanide toxicity. Appl. Environ. Microbiol. 73, 390–398. doi: 10.1128/AEM.02143-06
Cirz, R. T., O’Neill, B. M., Hammond, J. A., Head, S. R., and Romesberg, F. E. (2006). Defining the Pseudomonas aeruginosa SOS response and its role in the global response to the antibiotic ciprofloxacin. J. Bacteriol. 188, 7101–7110. doi: 10.1128/JB.00807-06
Craig, K., Johnson, B. R., and Grunden, A. (2021). Leveraging Pseudomonas stress response mechanisms for industrial applications. Front. Microbiol. 12:134. doi: 10.3389/fmicb.2021.660134
Crespo, A., Pedraz, L., Van Der Hofstadt, M., Gomila, G., and Torrents, E. (2017). Regulation of ribonucleotide synthesis by the Pseudomonas aeruginosa two-component system AlgR in response to oxidative stress. Sci. Rep. 7, 17892–17815. doi: 10.1038/s41598-017-17917-7
Cunningham, L., Pitt, M., and Williams, H. D. (1997). The cioAB genes from Pseudomonas aeruginosa code for a novel cyanide-insensitive terminal oxidase related to the cytochrome bd quinol oxidases. Mol. Microbiol. 24, 579–591. doi: 10.1046/j.1365-2958.1997.3561728.x
da Cruz Nizer, W. S., Inkovskiy, V., and Overhage, J. (2020). Surviving reactive chlorine stress: responses of gram-negative Bacteria to Hypochlorous acid. Microorganisms 8:1220. doi: 10.3390/microorganisms8081220
Da Cruz Nizer, W. S., Inkovskiy, V., Versey, Z., Strempel, N., Cassol, E., and Overhage, J. (2021). Oxidative stress response in Pseudomonas aeruginosa. Pathogens 10:1187. doi: 10.3390/pathogens10091187
Deborde, M., and von Gunten, U. (2008). Reactions of chlorine with inorganic and organic compounds during water treatment—kinetics and mechanisms: a critical review. Water Res. 42, 13–51. doi: 10.1016/j.watres.2007.07.025
Dukan, S., and Touati, D. (1996). Hypochlorous acid stress in Escherichia coli: resistance, DNA damage, and comparison with hydrogen peroxide stress. J. Bacteriol. 178, 6145–6150. doi: 10.1128/jb.178.21.6145-6150.1996
Enderby, B., Smith, D., Carroll, W., and Lenney, W. (2009). Hydrogen cyanide as a biomarker for Pseudomonas aeruginosa in the breath of children with cystic fibrosis. Pediatr. Pulmonol. 44, 142–147. doi: 10.1002/ppul.20963
Farrant, K. V., Spiga, L., Davies, J. C., and Williams, H. D. (2020). Response of Pseudomonas aeruginosa to the innate immune system-derived oxidants Hypochlorous acid and Hypothiocyanous acid. J. Bacteriol. 203, e00300–e00320. doi: 10.1128/JB.00300-20
Filiatrault, M. J., Tombline, G., Wagner, V. E., Alst, N. V., Rumbaugh, K., Sokol, P., et al. (2013). Pseudomonas aeruginosa PA1006, which plays a role in molybdenum homeostasis, is required for nitrate utilization, biofilm formation, and virulence. PLoS One 8:e55594. doi: 10.1371/journal.pone.0055594
Finnegan, M., Linley, E., Denyer, S. P., McDonnell, G., Simons, C., and Maillard, J.-Y. (2010). Mode of action of hydrogen peroxide and other oxidizing agents: differences between liquid and gas forms. J. Antimicrob. Chemother. 65, 2108–2115. doi: 10.1093/jac/dkq308
Frangipani, E., Pérez-Martínez, I., Williams, H. D., Cherbuin, G., and Haas, D. (2014). A novel cyanide-inducible gene cluster helps protect Pseudomonas aeruginosa from cyanide. Environ. Microbiol. Rep. 6, 28–34. doi: 10.1111/1758-2229.12105
Frisk, A., Schurr, J. R., Wang, G., Bertucci, D. C., Marrero, L., Hwang, S. H., et al. (2004). Transcriptome analysis of Pseudomonas aeruginosa after interaction with human airway epithelial cells. Infect. Immun. 72, 5433–5438. doi: 10.1128/iai.72.9.5433-5438.2004
Fukuzaki, S. (2006). Mechanisms of actions of sodium hypochlorite in cleaning and disinfection processes. Biocontrol Sci. 11, 147–157. doi: 10.4265/bio.11.147
Gilchrist, F. J., Alcock, A., Belcher, J., Brady, M., Jones, A., Smith, D., et al. (2011). Variation in hydrogen cyanide production between different strains of Pseudomonas aeruginosa. Eur. Respir. J. 38, 409–414. doi: 10.1183/09031936.00166510
Goemans, C. V., and Collet, J.-F. (2019). Stress-induced chaperones: a first line of defense against the powerful oxidant hypochlorous acid. F1000Res 8:1678. doi: 10.12688/f1000research.19517.1
Gold, M. H., Andriessen, A., Bhatia, A. C., Bitter, P. Jr., Chilukuri, S., Cohen, J. L., et al. (2020). Topical stabilized hypochlorous acid: the future gold standard for wound care and scar management in dermatologic and plastic surgery procedures. J. Cosmet. Dermatol. 19, 270–277. doi: 10.1111/jocd.13280
Gray, M. J., Wholey, W.-Y., and Jakob, U. (2013). Bacterial responses to reactive chlorine species. Annu. Rev. Microbiol. 67, 141–160. doi: 10.1146/annurev-micro-102912-142520
Groitl, B., Dahl, J.-U., Schroeder, J. W., and Jakob, U. (2017). Pseudomonas aeruginosa defense systems against microbicidal oxidants. Mol. Microbiol. 106, 335–350. doi: 10.1111/mmi.13768
Heo, Y.-J., Chung, I.-Y., Cho, W.-J., Lee, B.-Y., Kim, J.-H., Choi, K.-H., et al. (2010). The major catalase gene (katA) of Pseudomonas aeruginosa PA14 is under both positive and negative control of the global Transactivator OxyR in response to hydrogen peroxide. J. Bacteriol. 192, 381–390. doi: 10.1128/JB.00980-09
Herigstad, B., Hamilton, M., and Heersink, J. (2001). How to optimize the drop plate method for enumerating bacteria. J. Microbiol. Methods 44, 121–129. doi: 10.1016/s0167-7012(00)00241-4
Jacobs, M. A., Alwood, A., Thaipisuttikul, I., Spencer, D., Haugen, E., Ernst, S., et al. (2003). Comprehensive transposon mutant library of Pseudomonas aeruginosa. Proc. Natl. Acad. Sci. 100, 14339–14344. doi: 10.1073/pnas.2036282100
Kashyap, S., Sharma, P., and Capalash, N. (2022). Tobramycin stress induced differential gene expression in Acinetobacter baumannii. Curr. Microbiol. 79:88. doi: 10.1007/s00284-022-02788-7
Kim, H. J., Lee, H., Lee, Y., Choi, I., Ko, Y., Lee, S., et al. (2020). The ThiL enzyme is a valid antibacterial target essential for both thiamine biosynthesis and salvage pathways in Pseudomonas aeruginosa. J. Biol. Chem. 295, 10081–10091. doi: 10.1074/jbc.RA120.013295
Lee, J.-S., Heo, Y.-J., Lee, J. K., and Cho, Y.-H. (2005). KatA, the major catalase, is critical for Osmoprotection and virulence in Pseudomonas aeruginosa PA14. Infect. Immun. 73, 4399–4403. doi: 10.1128/IAI.73.7.4399-4403.2005
Létoffé, S., Wu, Y., Darch, S. E., Beloin, C., Whiteley, M., Touqui, L., et al. (2022). Pseudomonas aeruginosa production of hydrogen cyanide leads to airborne control of Staphylococcus aureus growth in biofilm and in vivo lung. Environments 13:e0215422. doi: 10.1128/mbio.02154-22
Li, Y., Feng, T., and Wang, Y. (2022). The role of bacterial signaling networks in antibiotics response and resistance regulation. Mar Life Sci Technol 4, 163–178. doi: 10.1007/s42995-022-00126-1
Li, L.-H., Shih, Y.-L., Huang, J.-Y., Wu, C.-J., Huang, Y.-W., Huang, H.-H., et al. (2020). Protection from hydrogen peroxide stress relies mainly on AhpCF and KatA2 in Stenotrophomonas maltophilia. J. Biomed. Sci. 27:37. doi: 10.1186/s12929-020-00631-4
Liberati, N. T., Urbach, J. M., Miyata, S., Lee, D. G., Drenkard, E., Wu, G., et al. (2006). An ordered, nonredundant library of Pseudomonas aeruginosa strain PA14 transposon insertion mutants. Proc. Natl. Acad. Sci. 103, 2833–2838. doi: 10.1073/pnas.0511100103
Lünse, C. E., Schüller, A., and Mayer, G. (2014). The promise of riboswitches as potential antibacterial drug targets. Int. J. Med. Microbiol. 304, 79–92. doi: 10.1016/j.ijmm.2013.09.002
McCarthy, R. R., Mooij, M. J., Reen, F. J., Lesouhaitier, O., and O’Gara, F. (2014). A new regulator of pathogenicity (bvlR) is required for full virulence and tight microcolony formation in Pseudomonas aeruginosa. Microbiology 160, 1488–1500. doi: 10.1099/mic.0.075291-0
Monje-Casas, F., Jurado, J., Prieto-Alamo, M. J., Holmgren, A., and Pueyo, C. (2001). Expression analysis of the nrdHIEF operon from Escherichia coli. Conditions that trigger the transcript level in vivo. J. Biol. Chem. 276, 18031–18037. doi: 10.1074/jbc.M011728200
Moser, S., Chileveru, H. R., Tomaras, J., and Nolan, E. M. (2014). A bacterial mutant library as a tool to study the attack of a Defensin peptide. Chembiochem 15, 2684–2688. doi: 10.1002/cbic.201402354
Ochsner, U. A., Vasil, M. L., Alsabbagh, E., Parvatiyar, K., and Hassett, D. J. (2000). Role of the Pseudomonas aeruginosa oxyR-recG operon in oxidative stress defense and DNA repair: OxyR-dependent regulation of katB-ankB, ahpB, and ahpC-ahpF. J. Bacteriol. 182, 4533–4544. doi: 10.1128/JB.182.16.4533-4544.2000
Overhage, J., Bains, M., Brazas, M. D., and Hancock, R. E. W. (2008). Swarming of Pseudomonas aeruginosa is a complex adaptation leading to increased production of virulence factors and antibiotic resistance. J. Bacteriol. 190, 2671–2679. doi: 10.1128/jb.01659-07
Pál Fehér, P., Purgel, M., Lengyel, A., Stirling, A., and Fábián, I. (2019). The mechanism of monochloramine disproportionation under acidic conditions. Dalton Trans. 48, 16713–16721. doi: 10.1039/C9DT03789F
Palma, M., DeLuca, D., Worgall, S., and Quadri, L. E. N. (2004). Transcriptome analysis of the response of Pseudomonas aeruginosa to hydrogen peroxide. J. Bacteriol. 186, 248–252. doi: 10.1128/JB.186.1.248-252.2004
Panmanee, W., and Hassett, D. J. (2009). Differential roles of OxyR-controlled antioxidant enzymes alkyl hydroperoxide reductase (AhpCF) and catalase (KatB) in the protection of Pseudomonas aeruginosa against hydrogen peroxide in biofilm vs. planktonic culture. FEMS Microbiol. Lett. 295, 238–244. doi: 10.1111/j.1574-6968.2009.01605.x
Pattison, D. I., and Davies, M. J. (2006). Reactions of myeloperoxidase-derived oxidants with biological substrates: gaining chemical insight into human inflammatory diseases. Curr. Med. Chem. 13, 3271–3290. doi: 10.2174/092986706778773095
Pattison, D. I., Davies, M. J., and Hawkins, C. L. (2012). Reactions and reactivity of myeloperoxidase-derived oxidants: differential biological effects of hypochlorous and hypothiocyanous acids. Free Radic. Res. 46, 975–995. doi: 10.3109/10715762.2012.667566
Peskin, A. V., Midwinter, R. G., Harwood, D. T., and Winterbourn, C. C. (2005). Chlorine transfer between glycine, taurine, and histamine: reaction rates and impact on cellular reactivity. Free Radic. Biol. Med. 38, 397–405. doi: 10.1016/j.freeradbiomed.2004.11.006
Peskin, A. V., and Winterbourn, C. C. (2001). Kinetics of the reactions of hypochlorous acid and amino acid chloramines with thiols, methionine, and ascorbate. Free Radic. Biol. Med. 30, 572–579. doi: 10.1016/S0891-5849(00)00506-2
Pessi, G., and Haas, D. (2000). Transcriptional control of the hydrogen cyanide biosynthetic genes hcnABC by the anaerobic regulator ANR and the quorum-sensing regulators LasR and RhlR In Pseudomonas aeruginosa. J. Bacteriol. 182, 6940–6949. doi: 10.1128/jb.182.24.6940-6949.2000
Prütz, W. A. (1996). Hypochlorous acid interactions with thiols, nucleotides, DNA, and other biological substrates. Arch. Biochem. Biophys. 332, 110–120. doi: 10.1006/abbi.1996.0322
Rahme, L. G., Stevens, E. J., Wolfort, S. F., Shao, J., Tompkins, R. G., and Ausubel, F. M. (1995). Common virulence factors for bacterial pathogenicity in plants and animals. Science 268, 1899–1902. doi: 10.1126/science.7604262
Rodríguez-Rojas, A., Mena, A., Martín, S., Borrell, N., Oliver, A., and Blázquez, J. (2009). Inactivation of the hmgA gene of Pseudomonas aeruginosa leads to pyomelanin hyperproduction, stress resistance and increased persistence in chronic lung infection. Microbiology 155, 1050–1057. doi: 10.1099/mic.0.024745-0
Romsang, A., Atichartpongkul, S., Trinachartvanit, W., Vattanaviboon, P., and Mongkolsuk, S. (2013). Gene expression and physiological role of Pseudomonas aeruginosa methionine sulfoxide reductases during oxidative stress. J. Bacteriol. 195, 3299–3308. doi: 10.1128/JB.00167-13
Ryall, B., Davies, J. C., Wilson, R., Shoemark, A., and Williams, H. D. (2008). Pseudomonas aeruginosa, cyanide accumulation and lung function in CF and non-CF bronchiectasis patients. Eur. Respir. J. 32, 740–747. doi: 10.1183/09031936.00159607
Salunkhe, P., von Götz, F., Wiehlmann, L., Lauber, J., Buer, J., and Tümmler, B. (2002). GeneChip expression analysis of the response of Pseudomonas aeruginosa to Paraquat-induced superoxide stress. Genome Lett 1, 165–174. doi: 10.1166/gl.2002.019
Sanderson, K., Wescombe, L., Kirov, S. M., Champion, A., and Reid, D. W. (2008). Bacterial cyanogenesis occurs in the cystic fibrosis lung. Eur. Respir. J. 32, 329–333. doi: 10.1183/09031936.00152407
Sichel, G., Corsaro, C., Scalia, M., Di Bilio, A. J., and Bonomo, R. P. (1991). In vitro scavenger activity of some flavonoids and melanins against O2-(.). Free Radic. Biol. Med. 11, 1–8. doi: 10.1016/0891-5849(91)90181-2
Small, D. A., Chang, W., Toghrol, F., and Bentley, W. E. (2007a). Comparative global transcription analysis of sodium hypochlorite, peracetic acid, and hydrogen peroxide on Pseudomonas aeruginosa. Appl. Microbiol. Biotechnol. 76, 1093–1105. doi: 10.1007/s00253-007-1072-z
Small, D. A., Chang, W., Toghrol, F., and Bentley, W. E. (2007b). Toxicogenomic analysis of sodium hypochlorite antimicrobial mechanisms in Pseudomonas aeruginosa. Appl. Microbiol. Biotechnol. 74, 176–185. doi: 10.1007/s00253-006-0644-7
Smith, D., Spaněl, P., Gilchrist, F. J., and Lenney, W. (2013). Hydrogen cyanide, a volatile biomarker of Pseudomonas aeruginosa infection. J. Breath Res. 7:044001. doi: 10.1088/1752-7155/7/4/044001
Sprouffske, K., and Wagner, A. (2016). Growthcurver: an R package for obtaining interpretable metrics from microbial growth curves. BMC Bioinformatics 17:172. doi: 10.1186/s12859-016-1016-7
Stothard, P., and Wishart, D. S. (2005). Circular genome visualization and exploration using CGView. Bioinformatics 21, 537–539. doi: 10.1093/bioinformatics/bti054
Stover, C. K., Pham, X. Q., Erwin, A. L., Mizoguchi, S. D., Warrener, P., Hickey, M. J., et al. (2000). Complete genome sequence of Pseudomonas aeruginosa PAO1, an opportunistic pathogen. Nature 406, 959–964. doi: 10.1038/35023079
Strempel, N., Nusser, M., Neidig, A., Brenner-Weiss, G., and Overhage, J. (2017). The oxidative stress agent hypochlorite stimulates c-di-GMP synthesis and biofilm formation in Pseudomonas aeruginosa. Front. Microbiol. 8:2311. doi: 10.3389/fmicb.2017.02311
Su, S., Panmanee, W., Wilson, J. J., Mahtani, H. K., Li, Q., VanderWielen, B. D., et al. (2014). Catalase (KatA) plays a role in protection against anaerobic nitric oxide in Pseudomonas aeruginosa. PLoS One 9:e91813. doi: 10.1371/journal.pone.0091813
Sultana, S., Foti, A., and Dahl, J.-U. (2020). Bacterial defense systems against the neutrophilic oxidant Hypochlorous acid. Infect. Immun. 88, e00964–e00919. doi: 10.1128/IAI.00964-19
Thippakorn, C., Isarankura-Na-Ayudhya, C., Pannengpetch, S., Isarankura-Na-Ayudhya, P., Schaduangrat, N., Nantasenamat, C., et al. (2018). Oxidative responses and defense mechanism of hyperpigmented P. aeruginosa as characterized by proteomics and metabolomics. EXCLI J. 17, 544–562. doi: 10.17179/excli2018-1238
Todor, H., Dulmage, K., Gillum, N., Bain, J. R., Muehlbauer, M. J., and Schmid, A. K. (2014). A transcription factor links growth rate and metabolism in the hypersaline adapted archaeon Halobacterium salinarum. Mol. Microbiol. 93, 1172–1182. doi: 10.1111/mmi.12726
Torrents, E., Poplawski, A., and Sjöberg, B.-M. (2005). Two proteins mediate class II ribonucleotide reductase activity in Pseudomonas aeruginosa: expression and transcriptional analysis of the aerobic enzymes. J. Biol. Chem. 280, 16571–16578. doi: 10.1074/jbc.M501322200
Versey, Z., Da Cruz Nizer, W. S., Russell, E., Zigic, S., DeZeeuw, K. G., Marek, J. E., et al. (2021). Biofilm-innate immune Interface: contribution to chronic wound formation. Front. Immunol. 12:648554. doi: 10.3389/fimmu.2021.648554
Wang, H., Chen, S., Zhang, J., Rothenbacher, F. P., Jiang, T., Kan, B., et al. (2012). Catalases promote resistance of oxidative stress in Vibrio cholerae. PLoS One 7:e53383. doi: 10.1371/journal.pone.0053383
Wang, S., Deng, K., Zaremba, S., Deng, X., Lin, C., Wang, Q., et al. (2009). Transcriptomic response of Escherichia coli O157:H7 to oxidative stress. Appl. Environ. Microbiol. 75, 6110–6123. doi: 10.1128/AEM.00914-09
Wang, S., Phillippy, A. M., Deng, K., Rui, X., Li, Z., Tortorello, M. L., et al. (2010). Transcriptomic responses of Salmonella enterica Serovars Enteritidis and typhimurium to chlorine-based oxidative stress. Appl. Environ. Microbiol. 76, 5013–5024. doi: 10.1128/AEM.00823-10
Wiegand, I., Hilpert, K., and Hancock, R. E. W. (2008). Agar and broth dilution methods to determine the minimal inhibitory concentration (MIC) of antimicrobial substances. Nat. Protoc. 3, 163–175. doi: 10.1038/nprot.2007.521
Winterbourn, C. C. (1985). Comparative reactivities of various biological compounds with myeloperoxidase-hydrogen peroxide-chloride, and similarity of oxidant to hypochlorite. Biochim. Biophys. Acta Gen. Subj. 840, 204–210. doi: 10.1016/0304-4165(85)90120-5
Winterbourn, C. C., Kettle, A. J., and Hampton, M. B. (2016). Reactive oxygen species and neutrophil function. Annu. Rev. Biochem. 85, 765–792. doi: 10.1146/annurev-biochem-060815-014442
Wu, M., Guina, T., Brittnacher, M., Nguyen, H., Eng, J., and Miller, S. I. (2005). The Pseudomonas aeruginosa proteome during anaerobic growth. J. Bacteriol. 187, 8185–8190. doi: 10.1128/JB.187.23.8185-8190.2005
Xia, B., Li, M., Tian, Z., Chen, G., Liu, C., Xia, Y., et al. (2019). Oligoribonuclease contributes to tolerance to aminoglycoside and β-lactam antibiotics by regulating KatA in Pseudomonas aeruginosa. Antimicrob. Agents Chemother. 63, e00212–e00219. doi: 10.1128/AAC.00212-19
Yuan, F., Yin, S., Xu, Y., Xiang, L., Wang, H., Li, Z., et al. (2021). The richness and diversity of catalases in Bacteria. Front. Microbiol. 12:477. doi: 10.3389/fmicb.2021.645477
Zdor, R. E. (2015). Bacterial cyanogenesis: impact on biotic interactions. J. Appl. Microbiol. 118, 267–274. doi: 10.1111/jam.12697
Zuhra, K., and Szabo, C. (2022). The two faces of cyanide: an environmental toxin and a potential novel mammalian gasotransmitter. FEBS J. 289, 2481–2515. doi: 10.1111/febs.16135
Keywords: Pseudomonas aeruginosa, oxidative stress, sodium hypochlorite, hydrogen cyanide, reactive chlorine species, oxidative stress response, antimicrobial resistance
Citation: da Cruz Nizer WS, Adams ME, Inkovskiy V, Beaulieu C and Overhage J (2023) The secondary metabolite hydrogen cyanide protects Pseudomonas aeruginosa against sodium hypochlorite-induced oxidative stress. Front. Microbiol. 14:1294518. doi: 10.3389/fmicb.2023.1294518
Edited by:
Hector Alex Saka, CONICET, ArgentinaReviewed by:
Anne J. Anderson, Utah State University, United StatesSamina Mehnaz, Forman Christian College, Pakistan
Copyright © 2023 da Cruz Nizer, Adams, Inkovskiy, Beaulieu and Overhage. This is an open-access article distributed under the terms of the Creative Commons Attribution License (CC BY). The use, distribution or reproduction in other forums is permitted, provided the original author(s) and the copyright owner(s) are credited and that the original publication in this journal is cited, in accordance with accepted academic practice. No use, distribution or reproduction is permitted which does not comply with these terms.
*Correspondence: Joerg Overhage, am9lcmdvdmVyaGFnZUBjdW5ldC5jYXJsZXRvbi5jYQ==