- 1Laboratorio de Inmunobiología y Genética, Instituto Nacional de Enfermedades Respiratorias Ismael Cosío Villegas (INER), Mexico City, Mexico
- 2Tecnologico de Monterrey, Escuela de Medicina y Ciencias de la Salud, Mexico City, Mexico
- 3Red de Medicina para la Educación, el Desarrollo y la Investigación Científica de Iztacala, Facultad de Estudios Superiores Iztacala, Universidad Nacional Autónoma de México, Mexico City, Mexico
The role of the microbiome in asthma is highlighted, considering its influence on immune responses and its connection to alterations in asthmatic patients. In this context, we review the variables influencing asthma phenotypes from a microbiome perspective and provide insights into the microbiome’s role in asthma pathogenesis. Previous cohort studies in patients with asthma have shown that the presence of genera such as Bifidobacterium, Lactobacillus, Faecalibacterium, and Bacteroides in the gut microbiome has been associated with protection against the disease. While, the presence of other genera such as Haemophilus, Streptococcus, Staphylococcus, and Moraxella in the respiratory microbiome has been implicated in asthma pathogenesis, indicating a potential link between microbial dysbiosis and the development of asthma. Furthermore, respiratory infections have been demonstrated to impact the composition of the upper respiratory tract microbiota, increasing susceptibility to bacterial diseases and potentially triggering asthma exacerbations. By understanding the interplay between the microbiome and asthma, valuable insights into disease mechanisms can be gained, potentially leading to the development of novel therapeutic approaches.
1 Introduction
Asthma is a common respiratory disease that affects individuals of all ages. It is now recognized as a condition with several phenotypes and as a group of several distinct diseases, known as endotypes. Some asthma phenotypes that have been described include young individuals with allergies, overweight middle-aged individuals, and elderly individuals with unhealthy aging, among many others. However, their similarities give rise to a common syndrome characterized by reversible airway obstruction, nonspecific airway hyperresponsiveness, and chronic airway inflammation (Kuruvilla et al., 2019; Hizawa, 2023).
The underlying pathogenesis of asthma is extremely complex and diverse, with a significant economic impact due to the need for long-term treatment (Nurmagambetov et al., 2018) and a potential decrease in quality of life. Clinically, asthma is a chronic airways disease characterized by recurrent episodes of wheezing, coughing, thoracic oppression, and dyspnea (GINA Report, 2022). The immune system plays a central role in the pathophysiology of asthma, involving the inflammatory response and sensitivity to allergens (Bush, 2019). Furthermore, recent research has highlighted the importance of the microbiome in the development of the immune response, as it is educated and modified by microorganisms and metabolites of the microbiome (Zheng et al., 2020). On the other hand, respiratory diseases have been associated with decreased microbial diversity, termed dysbiosis, defined as deviation from a normal microbial composition, is associated with a number of adverse biological phenomena, sometimes with clinical consequences (Natalini et al., 2023). Respiratory and gut dysbiosis modifies immune system responses which influences inflammation in the lungs, leading to a potential role in asthma pathophysiology, phenotypes, and clinical outcomes (Ver Heul et al., 2019; Hufnagl et al., 2020). Typically, attention is usually focused on a single point, involving the analysis of microbiota from singular anatomical sites during specific developmental stages or, in certain instances, restricting the focus solely to pediatric and adult cohorts (Zimmermann et al., 2019; Losol et al., 2021; Aldriwesh et al., 2023). However, a noteworthy challenge arises when endeavoring to amalgamate shared findings from diverse studies. While certain commonalities have been identified, their respective implications vary depending upon the contextual framework (Barcik et al., 2020; Lupu et al., 2023; Zhao et al., 2023). Consequently, it has proven to be quite formidable to identify a specific taxonomic group that consistently influences or mitigates the risk factors or clinical presentations of asthma across all scenarios. Thus, we assert the significance of exploring the role of the microbiome within the context of its development, eschewing the presumption that a particular taxonomic group universally assumes an identical role in all circumstances. In this context, we have conducted a comprehensive review to explore the diverse roles of the microbiome in relation to the phenotypes and endotypes of asthma throughout human growth and development, encompassing prenatal factors, birth, childhood, adolescence, adulthood, and the elderly.
2 Microbiome
The microbiome encompasses the microbiota, their genetic material, metabolites, and the surrounding microenvironment (Berg et al., 2020). Each body site has its own distinct composition and complexity of microorganisms. When evaluating the microbiome based on sequencing data, two important terms are often employed: alpha diversity, which measures the number and abundance of microorganisms in a specific sample or site, and beta diversity, which quantifies the variation in microorganisms between different samples or sites (Finotello et al., 2018). Within the microbiome, a multitude of commensal microorganisms have undergone co-evolution with human cells; giving rise to complex, dynamic, interdependent, and context-dependent relationships essential for maintaining ecosystem balance within their respective communities, a state referred to as eubiosis (Iebba et al., 2016). Eubiosis and host-microbiome relationship influence various host functions, including metabolism, immunity, circadian rhythms, nutritional responses, and homeostasis (Zheng et al., 2020). In order to achieve its functions, human cells engage in intricate communication mechanisms through a system-system axis, enabling coordinated responses across different organs such as the gut-brain axis and gut-lung axis (Suganya and Koo, 2020; Ahlawat et al., 2021).
The gut-lung axis plays a significant role in respiratory pathologies as it establishes a bidirectional pathway for the transmission of internal and external factors, creating a signaling network that can influence systemic functions and responses (Enaud et al., 2020). The composition of the microbiome on mucosal surfaces, including the gastrointestinal and respiratory tracts, is highly dynamic. The gut microbiota consists of approximately 3,594 species, primarily classified under the phyla Actinobacteria, Bacteroidota, Firmicutes, and Proteobacteria (Leviatan et al., 2022). Contrary to previous beliefs, it is now known that the human lung harbors a distinct lung microbiota, mainly composed of genera such as Prevotella, Veillonella, and Streptococcus, thanks to advancements in sequencing techniques (Dickson et al., 2016; Yagi et al., 2021; Natalini et al., 2023). Molecular signals, including short-chain fatty acids (SCFAs) produced by Bifidobacterium, Lactobacillus, Faecalibacterium, and Ruminococcus (Tsukuda et al., 2021), facilitate communication between these organs through circulation or via the vagus nerve. Several species have also shown marked effects as neuromodulators and neurotransmitters such as monoamines, serotonin, and brain-derived neurotrophic factor (Suganya and Koo, 2020). Lactobacillus is strongly involved in both the gut-lung axis and the brain-gut axis (Rastogi and Singh, 2022), and can produce GABA and activate receptor expression, leading to cognitive enhancement via the vagus nerve (Breit et al., 2018). Oral ingestion of L. rhamnosus and L. murinus promotes migration of T Regulatory Cell (Treg) to the lungs and blocks the Th2 response (Zhang et al., 2018a; Han et al., 2021), thereby reducing respiratory inflammation (Figure 1). Wang et al. also prove that L. fermentum can reduce the expression of Toll-like Receptor 2 and Toll Like Receptor 4 in OVA mice model, with concurrent reduction in inflammatory cell infiltration and alveolar swelling (Wang et al., 2022). The composition and function of the microbiome in both the intestine and the lung are influenced by a variety of factors, including genetics, the immune system, pregnancy, birth conditions, age, dietary habits, pollution, antibiotics, and lifestyle (Martino et al., 2022; Figure 2).
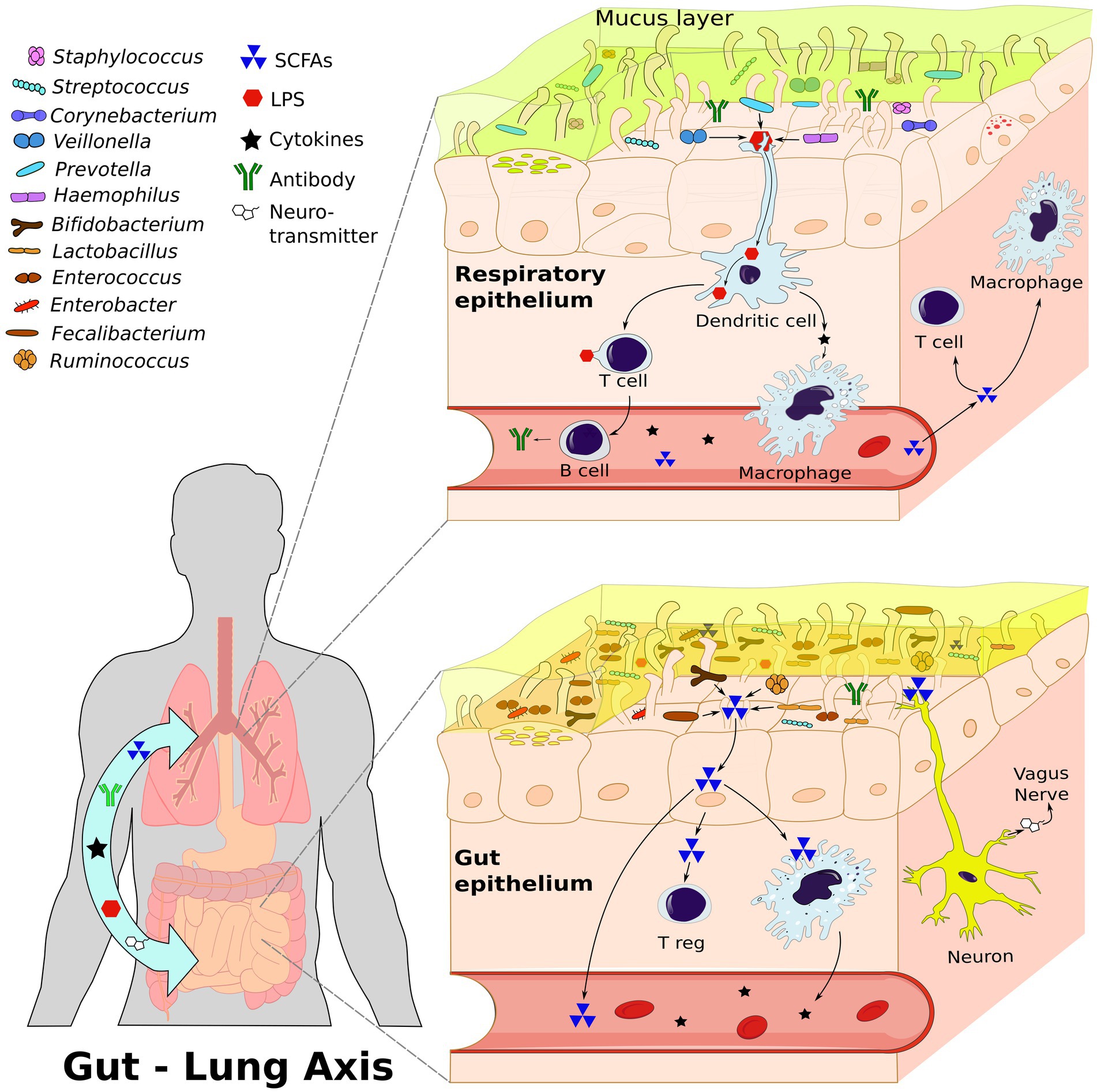
Figure 1. The Gut-Lung Axis: A bidirectional communication network between the gastrointestinal tract and the respiratory system involving interactions among immune cells, neurons, microbiota, and signaling molecules. This figure illustrates the composition of both lung and gut microbiota and the dynamic interactions within this axis; highlighting the role of microbiome dependent communication. This communication stimulates the immune cells through signal molecules, such as short-chain fatty acids (SCFAs), lipopolysaccharide (LPS), and cytokines. Additionally, through the vagus nerve, the microbiome stimulates the production of neurotransmitters. All these molecules can travel through the bloodstream to influence both the gut and lung systems. Created with inkscape.
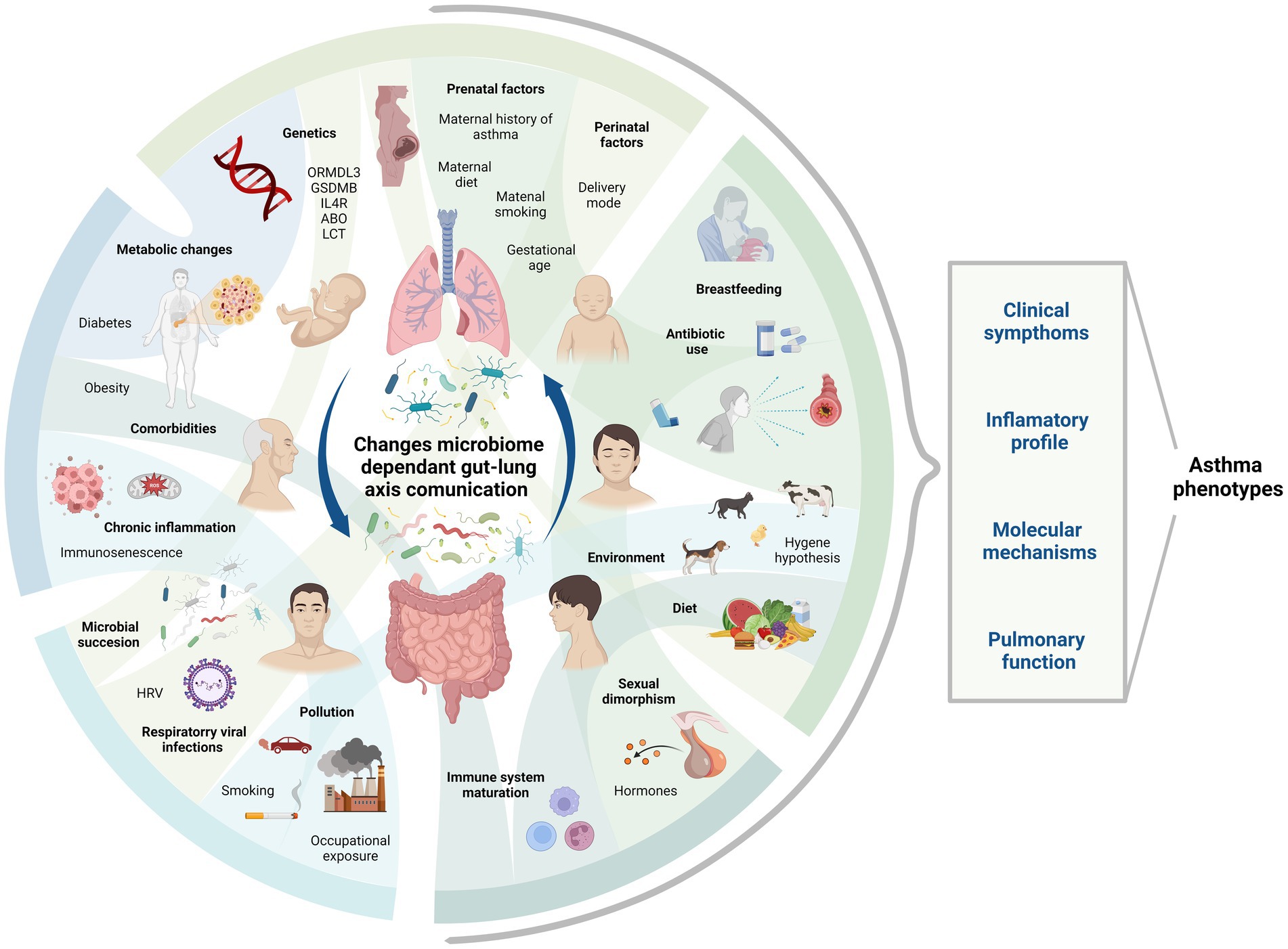
Figure 2. Asthma phenotype and factors determining microbiome through life stages. This figure shows some of the factors influencing asthma phenotype and microbiome composition during different life stages. Their complex interactions require study in order to comprehend their role in the context of gut-lung axis communication via microbiome and asthma development. Created with BioRender.com. Bio render agreement number: PT264MFOUQ.
3 Microbiome and asthma: the importance of the beginning
The journey of an individual begins with the intricate process of fertilization and genetic recombination, which sets the foundation for the expression of unique characteristics that will define their existence. However, the conditions of birth, growth, and development that an individual experience will play a crucial role in shaping their traits and creating the environment in which they thrive; shaping an individual’s life journey.
3.1 Genetics factors
Asthma is considered a chronic complex disease that is the consequence of an interaction between genetic and environmental factors. Over 100 genes have been associated with asthma and the features of the disease; however, there is marked variability in replication attempts in independent studies. Genetic variants on chromosome 17q21 near to ORMDL3/GSDMB locus have been associated with childhood-onset asthma (Ntontsi et al., 2021; Afzal et al., 2023). Kumar et al. highlighted the association between the variant rs1805011 in the IL-4 receptor gene and Th1/Th2 differentiation, which increases susceptibility to asthma (Kumar et al., 2015). This finding shed light on the intricate interplay between genetic factors and T-cell responses.
In this context, there has recently been growing interest in understanding the role of host genetics in shaping the gut microbiome, and several studies have shed light on this complex interplay. Boulund et al., identified significant correspondences in microbial taxa that are partly regulated by host genotype, with host genes associated with these taxa being related to secretion-metabolism, signaling-transport, and immunity (Boulund et al., 2022). Similarly, Lopera-Mayá et al., conducted a genome-wide association study to comprehensively characterize the effects of host genetics on the gut microbiome, discovering two study-wide significant signals near the Lactase and ABO genes with the Bifidobacterium and Collinsella genera, respectively (Lopera-Maya et al., 2022). Rühlemann et al., reported an association between the Prevotella genus in asthma and ABO blood groups (Rühlemann et al., 2021). Additionally, Ahluwalia et al. proposed that variations in the Fucosyltransferase 2 (FUT2) and ABO genes, along with epistatic effects, may contribute to an increased risk of early childhood asthma (Ahluwalia et al., 2020). They suggest that the expression of AB antigens in the respiratory epithelium and Streptococcus pneumoniae infection may be involved. Kurilshikov et al., conducted a genome-wide association study investigating human host genetic variation’s impact on microbial taxa. They identified 31 loci that influence the gut microbiome. Among these loci, the LCT gene stands out as being particularly significant for the Bifidobacterium genus (Kurilshikov et al., 2021). Regarding the gut microbiome, host genetics, and asthma, Li et al., described that, through a two-sample Mendelian randomization analysis, they predicted a positive correlation between the gut species Barnesiella and RuminococcaceaeUCG014 genera and the risk of asthma. Furthermore, they found that Akkermansia reduced the risk of adult-onset asthma (Li et al., 2023). Perez-Garcia et al., through a study of microbiome- genome wide association (mbGWAS) and microbiome quantitative trait loci (mbQTL) analysis, reported the identification of polymorphisms in the APOBEC3B-APOBEC3c, TRIM24, and TPST2 genes that are associated with asthma comorbidities. These polymorphisms were found to be mbQTLs related to Streptococcus, Tannerella, and Campylobacter in the upper airway (Perez-Garcia et al., 2020). Nevertheless, as proposed by Chen et al., it is suggested that risk variants on 17q12-21 and perturbations in the maturation of intestinal microbiota associate independently and exhibit additive effects on the risk of asthma development (Chen et al., 2023). Therefore, more studies are needed to define the role of host genetics in microbial diversity and asthma.
3.2 Prenatal factors
Prenatal factors have been associated with increased susceptibility to asthma, as demonstrated by cross-sectional studies and systematic reviews (Castro-Rodriguez et al., 2016; Arif and Veri, 2019; Esmeraldino et al., 2022). These factors include maternal smoking (Moradzadeh et al., 2018), maternal history of asthma (García-Serna et al., 2021), antibiotic use during pregnancy (Alhasan et al., 2020), maternal diet (Gray et al., 2017), and maternal stress (Van De Loo et al., 2016; Douros et al., 2017), among others.
Furthermore, the human fetal immune system initiates its development during the first four weeks of gestation (Park et al., 2020). Early exposures to metabolites from the maternal microbiota contribute to establishing a functional immune response at birth (Donald and Finlay, 2023). In a murine model, the transfer of antigen-specific IgG during fetal development provides protection to the offspring against allergic airway inflammation (Nakata et al., 2010). This suggests that antibodies play a dual role by not only safeguarding against particular pathogens but also contributing to the establishment of tolerance and recognition of commensal bacteria that will colonize the newborn (Koch et al., 2016; Macpherson et al., 2017; Mimoun et al., 2020). Additionally, the maternal microbiota prepares the newborn for host-microbial mutualism, which results from microbial metabolite transfer. By transiently colonizing pregnant female mice, with E. coli HA107, the maternal microbiota shapes the immune system of the offspring. Maternal microbial metabolites increases intestinal group 3 innate lymphoid cells and F4/80 + CD11c (Gomez de Agüero et al., 2016). Regarding the translocation of maternal microorganisms for fetal colonization, Jimenez et al. designed an experiment using a labeled strain of Enterococcus faecium. They orally inoculated pregnant mice and successfully recovered the microorganism from the meconium and amniotic fluid of the cesarean-born animals (Jiménez et al., 2008). However, a systematic review reports that the meconium microbiota in humans begins to develop after birth (Turunen et al., 2023).
On the other hand, the administration of antibiotics closer to parturition has been shown to have a significant impact on the diversity of both neonatal and maternal microbiota. Specifically, it has been found to increase the abundance of the phylum Proteobacteria in neonates exposed to antibiotics. In contrast, unexposed neonates tend to have a dominance of phylum Firmicutes with families such as Streptococcaceae and Lactobacillaceae (Stiemsma and Michels, 2017).
Furthermore, antibiotics can disrupt the maternal microbiome in the vagina. For example, the administration of antibiotics to the mother during the intrapartum period before birth, as well as the duration of rupture of membranes (ROM), have been found to be significantly associated with a decreased transmission rate of Lactobacillus-dominant mixed flora to neonates (Keski-Nisula et al., 2013). Considering all of the above, we believe it is necessary to continue investigating prenatal factors in the development of the newborn’s immune system and microbiome.
3.3 Perinatal factors
The process of birth represents a complex series of changes, including the first interaction between the newborn and the microbiome along with the onset of immune system training. In the beginning, we were born with an immature immune system mainly dependent on the innate immune system. Neonatal dendritic cells (DCs) exhibit adult levels of the immunoregulatory cytokine IL-10 when stimulated with lipopolysaccharides (LPS) from the maternal microbiome, but they are less proficient in promoting T helper 1 (Th1) cell differentiation due to delayed IL-12 production. Instead, the neonatal immune system tends to favor immunoregulatory and Th2 cell responses. This serves as a protective mechanism to prevent excessive inflammatory responses to novel antigens found in the environment and commensal microorganisms of their own microbiota (Donald and Finlay, 2023).
Hygiene hypothesis proposes that during early life, exposure to exogenous determinants such as breastfeeding, environment and microbiome plays a protective role against allergic diseases by facilitating the maturation of the immune system. This critical period of exposure spans from perinatal life until school-age (Liu, 2007; Garn et al., 2021; Pfefferle et al., 2021). In essence, it suggests that a lack of exposure to microorganisms may result in impaired immune tolerance development.
The initial colonization of microorganisms is closely linked to the mode of delivery and gestational age at birth (Castro-Rodriguez et al., 2016). When a baby is born vaginally, they are immediately exposed to microorganisms primarily inhabiting the maternal gut lumen and vagina, such as Lactobacillus, Bacteroides, and Bifidobacterium genera (Kalbermatter et al., 2021). Consequently, the gut microbiota of newborns born vaginally tends to be similar to that of their mothers (Song et al., 2021; Yao et al., 2021). Within the first days after birth, Clostridiaceae, Enterococcaceae, and Streptococcaceae families are observed, followed by the appearance of Bacteroides and Bifidobacterium in the guts of 40% of infants after the third day (Yao et al., 2021). In contrast, the colonization of the upper respiratory tract initiates with Staphylococcus and Streptococcus followed by the proliferation of Moraxella, Corynebacterium, Dolosigranulum, and/or Haemophilus species (Bosch et al., 2016), which are associated with reduced risk of respiratory symptoms (Biesbroek et al., 2014; Teo et al., 2015).
However, the colonization process can be interrupted for several reasons, mainly Cesarean section (C-section) delivery. Compared to babies born vaginally, infants delivered via C-section share roughly 30% fewer bacterial species with their mothers (Kalbermatter et al., 2021). In the upper respiratory tract Bosh et al., reported a lower abundance of Corynebacterium and Dolosigranulum, especially in the first months of life (Bosch et al., 2016). In gut microbiota, these newborns primarily harbor microbes from the maternal skin and antibiotic-resistant bacteria from the hospital environment, including Staphylococcus, Streptococcus, and Clostridium, which can alter its maturation (Rutayisire et al., 2016).
In fact, evidence suggests that alteration of microbiota by C-section, especially the elective one, is associated with alterations in the immune system, increasing the risk for developing asthma, allergies, type I diabetes mellitus, and celiac disease (Salas Garcia et al., 2018; Ferllini Montealegre et al., 2019; Kumbhare et al., 2019). Słabuszewska-Jóźwiak et al. in a meta-analysis study for C-section delivery and asthma in offspring, reported an odds ratio of 1.23 (95%CI 1.14–1.33, p < 0.00001) and a higher frequency of asthma in the C-section delivered children (Słabuszewska-Jóźwiak et al., 2020). Another cohort study revealed a relative risk of 1.11 (95% CI 1.00 to 1.25) in children with partially controlled asthma which increased to 1.8 (95% CI 1.00 to 1.39) in children with uncontrolled asthma (Moore et al., 2023). This risk could be explained because children who maintain a microbiota associated with C-section display a different immune response during respiratory symptom episodes, with lower levels of TNF-a, IL-4, IL-13, or IL-1b. Additionally, infants delivered by C-section to long-term have high levels of IgE making them susceptible to asthma or the development of allergies (Stokholm et al., 2018).
C-section delivery is more common in premature babies (infants born before 37 weeks of gestation) and has been associated with the development of various health problems, including asthma. Also, preterm can lead to delayed and reduced gut colonization by beneficial bacteria like Bacteroides, Bifidobacterium, and Lactobacillus species. This creates an opportunity for other bacteria, such as family Clostridiaceae, to colonize gut lumen, potentially contributing to the development of asthma and allergic disorders (Zimmermann et al., 2019). Zhang et al., through a meta-analysis reported that prematures have up to a 36% higher risk of asthma compared to infants born at term estimated (Zhang et al., 2018b). Furthermore, formula feeding in premature babies reduces overall gut microbial diversity and reduces Bifidobacterium levels (Healy et al., 2022). A Swiss cohort study that followed 4, millions of births up to 46 years of age, described that the risk of developing asthma increases as the age of the infant at birth decreases, with a probability 1.5 to 2.5 times greater than that of a full-term newborn (Crump et al., 2023). Moreover, premature infants often experience reduced lung function and structural alterations in the lungs, leading to airflow problems (Arroyas et al., 2020).
Furthermore, preterm infants exhibit elevated rates of hospitalization related to Respiratory Syncytial Virus (RSV), admissions to the intensive care unit, the need for mechanical ventilation, and extended hospital stays when contrasted with full-term infants (Anderson et al., 2017). In a multicenter prospective cohort study involving 221 infants affected by RSV bronchiolitis, Raita et al. identified an endotype characterized by several distinctive features. This endotype included a high prevalence of parental asthma, IgE sensitization, and concurrent rhinovirus (HRV) infection. Notably, the co-dominance of Streptococcus pneumoniae and Moraxella catarrhalis in the nasopharynx, along with an elevated IFN-α and -γ response, were also prominent characteristics. This particular endotype was significantly associated with an increased risk of developing childhood asthma. It’s worth noting that among these patients, 22.2% were born prematurely (Raita et al., 2021). Importantly, infection with HRV-C has been linked to more severe asthma exacerbations compared to HRV-A and HRV-B (Bizzintino et al., 2011).
These infections reduce Th1 and IFN-γ responses, leading to an increase in Th2 responses, which promote inflammation and bronchoconstriction (Pinto et al., 2006). Additionally, premature infants often have elevated levels of IL-17 from Th17 cells, which further exacerbates inflammation, resulting in characteristics of both eosinophilic asthma (IL-4 and IL-13) and neutrophilic asthma (IL-17), leading to a combined type of asthma (Chesné et al., 2014; Anderson et al., 2023).
While progress has been made in the study of the aforementioned factors, additional long-term longitudinal studies are still needed to understand the effects of changes in the microbiome during the perinatal period.
4 Infancy and childhood, imprint development
Childhood asthma is a prevalent and complex respiratory condition that affects millions of children worldwide, posing significant health challenges and burdens. According to literature review, early-life microbiota disturbances can lead to immune alterations, including T-reg cells proliferation, Th17 response, and IgE response in humans (Lee and Kim, 2017). Furthermore, the gut and airway microbiota in the first year of life has been reported to induce T-reg cells that enhance tolerogenic immunity in infants at high risk for asthma (Busse and Rosenwasser, 2003). Multiple investigations have shown differences between the lung and gut microbiome of individuals with established asthma vs. healthy subjects, being the population with asthma the ones with lower bacterial diversity (Marsland et al., 2015; Carr et al., 2019; Liu et al., 2020). Case–control studies have associated gut dysbiosis with a reduction in the specific genera Faecalibacterium, Bifidobacterium, Lachnospira, Veillonella, and Rothia (Yap et al., 2014; Arrieta et al., 2015). Therefore, systematic review highlights the importance of establishing a healthy microbiota during the first years of life. Notably, breastfeeding (Doherty et al., 2018) and supplementation with Lactobacillus (Durack et al., 2018; Alliet et al., 2022), have long-lasting potential for immune development and reduce the risk of developing asthma and allergic conditions.
4.1 Breastfeeding and changes in diet
Evidence suggests that the microbiome in children is influenced to a greater extent by first feeding method and dietary intake. Human milk, contains immunomodulators and anti-inflammatory agents such as alpha-tocopherol, beta-casomorphins, prolactin, lactoferrin, lysozyme, antioxidants, cytokines, and secretory IgA (Miliku and Azad, 2018), which promote the proper development of both mucosal and systemic immune systems, playing an important role in shaping a more robust immune system compared to the immune system of formula fed infants (Munblit et al., 2017; Domenici and Vierucci, 2022).
The presence of certain bacterial genera, including Staphylococcus, Streptococcus, Lactobacillus, and Bifidobacterium, has been consistently reported in human milk (Boquien, 2018; Lyons et al., 2020). According to a randomized double-blind trial in Sweden, the richness of bacterial species in breast milk appears to be critical in preventing the overgrowth of potentially harmful species associated with asthma development like Enterococcus (Dzidic et al., 2020). Moreover, Bifidobacterium, a common genus in breast milk, has demonstrated anti-inflammatory properties by promoting the production of anti-inflammatory cytokines, inhibiting Th2 immune responses, and suppressing IgE production, which further supports the notion that breast milk contributes to reducing asthma risk (Eslami et al., 2020). In contrast, a cohort made in Korea shows that infants who are formula-fed demonstrate early diversification of their gut microbiota, accompanied by decreased levels of Bifidobacteria and increased abundance of Escherichia, Veillonella, and Enterococcus (Lee et al., 2015). Compared with direct breastfeeding, any other mode of infant feeding (including formula) was associated with a higher occurrence of opportunistic pathogens, antibiotic resistance, and an increased risk of asthma (Klopp et al., 2017; Pärnänen et al., 2022).
While all these suggest breastfeeding is protective against asthma in children (Ahmadizar et al., 2017), others have found no significant association at all (Elliott et al., 2009). These discrepancies may be attributed to several factors, including differences in study design and confounding variables such as breast milk composition, which can vary across populations, potentially explaining the observed variations in breastfeeding effects of breastfeeding across studies (Miliku and Azad, 2018). It is important to continue research efforts to better understand the complex relationship between breastfeeding and asthma, taking into account microbiome immune training.
An important event during infancy that impacts the development of the gut microbiome is the introduction of solid food and the cessation of breastfeeding. This shift results in an increase in Lachnospiraceae and Ruminococcaceae, while causing a decrease in Bifidobacterium, Enterobacteriaceae, Enterococcaceae, Lactobacillaceae, Veillonellaceae, Clostridiaceae, and an overall increase in microbial diversity (Laursen et al., 2017). This transition is both necessary and advantageous. It fosters the development of a microbial community better suited to extract energy and process a diet that is no longer reliant on milk, transitioning to a diet rich in fiber and protein (Dong and Gupta, 2019).
Dietary intake has been shown to influence systemic inflammation. The Western diet is characterized by a lack of antioxidants and high levels of fatty acids. This diet has been associated with the promotion of oxidative stress and the activation of inflammatory cascades through receptors such as Toll-like receptor 4 (TLR4), leading to a pro-inflammatory environment, according to a cohort study which compares asthmatic patients vs. healthy controls (Wood et al., 2015). Additionally, the consumption of high-fat mixed meals has been found to increase sputum neutrophils in patients with asthma, particularly observed 4 h after the meal (Wood et al., 2011). Schroeder et al. conducted a study using a mouse model fed a Western diet, which resulted in a reduction in the populations of Bifidobacterium, Sutturella, and Akkermansia genus. Simultaneously, there was an increase in the Clostridiales order and the Lactobacillus and Oscillospira genus. Notably, the decrease in Bifidobacterium taxa coincided with the onset of mucus defects in this model (Schroeder et al., 2018). Moreover, a Western diet can lead to endotoxemia, contributing to intestinal barrier impairment and increased levels of bacterial lipopolysaccharides (LPS), consequently leading to heightened inflammatory signaling (Pendyala et al., 2012).
Conversely, the Mediterranean diet characterized by a diverse range of fruits, olive oil, vegetables, and whole grain cereals is believed to create an anti-inflammatory environment. This is attributed to the presence of dietary fiber, unsaturated fatty acids, such as monounsaturated fatty acids and Omega-3, as well as the abundance of antioxidants. In a meta-analysis conducted by Garcia-Marcos et al., it was found that the Mediterranean diet was significantly associated with a reduced prevalence of asthma (OR: 0.86; 95% CI 0.78–0.95; p = 0.004) (Garcia-Marcos et al., 2013). Similarly, a meta-analysis by Zhang et al., reported protective effects of a Mediterranean diet on asthma (OR = 0.88; 95% CI: 0.79–0.97; p = 0.014) (Zhang et al., 2023). However, further research is required to gain a deeper understanding of the specific role of the microbiome in individuals with asthma who adhere to a Mediterranean diet. Moreover, high-fiber diets have been associated with an increase in the colonic Bacteroidetes and Actinobacteria phyla, while the Firmicutes and Proteobacteria phyla decreased. These shifts in microbial composition provide protection against allergic responses, as demonstrated in mouse models (Zhang et al., 2016). Dietary fibers, such as pectin, are fermented by commensal gut bacteria, which produce metabolites including SCFAs, which mediate anti-inflammatory responses (Blanco-Pérez et al., 2021). Ketogenic diet during pregnancy, lactation, and early childhood have been related to low risk of developing asthma due to changes in epigenetic markers. For instance, Bifidobacterium and Lactobacillus species produce SCFAs, which exert anti-inflammatory effects on immune cells through an epigenetic mechanism that involves the inhibition of histone deacetylases associated with butyrate. This modulation of the immune response leads to a reduced risk of asthma (Alsharairi, 2020).
4.2 Environment influences on children’s development and growth
Numerous epidemiological studies have shown that living on a farm during early childhood is associated with a reduced risk of developing asthma in childhood (Stein et al., 2016; House et al., 2017; Depner et al., 2020). Under these circumstances, Bifidobacterium and Lactobacillus species produce SCFAs, which exert anti-inflammatory effects on immune cells through an epigenetic mechanism involving the inhibition of histone deacetylases associated with butyrate. In particular, a study that compares the Amish and Hutterites populations, who had similar genetic backgrounds but different farming practices, found that the Amish, who followed traditional farming practices involving high microbial exposures to animals, had a lower risk of childhood asthma. In contrast, the Hutterites, who practiced industrialized farming, did not show the same protective effect (Stein et al., 2016).
A study conducted across 14 countries compared the effects of farm environments and inner city environments on the development of allergic diseases (Campbell et al., 2017). The findings revealed that exposure to a farm environment was associated with a protective effect against allergic diseases. One of the key factors identified by other authors in this protective effect was the consumption of farm milk, which has been reported to contain higher levels of gram-negative bacteria such as Escherichia coli, Pseudomonas, and Klebsiella, as well as lipopolysaccharides (LPS) (Garedew et al., 2012; Gehring et al., 2020). Endotoxins as LPS, most commonly studied in combination with dust, induce a Th2 response in mice and exacerbate lung eosinophilia via TLR4 pathways, which can result protective against allergic diseases, such as asthma (Ren et al., 2019). A low dose but continuous exposure to an endotoxin, was protective in a mouse model of asthma. This suggests that by the time children reach school age, they will exhibit a marked suppression of the capacity of a Th2 response as a consequence of long-term exposure to environmental endotoxins (Braun-Fahrländer et al., 2002).
Furthermore, it has been considered that growing up with pets and siblings exerts complex changes in microbiota composition, a longitudinal study comparing fecal microbiota composition in infants reports that subjects exposed to both pets and siblings tended to have low relative abundance of family Bifidobacteriaceae and other bacterias (Azad et al., 2013). These changes in gut microbiota result in the development of a healthy immune system that can end up being protective against asthma and allergy development. Siblings are another of the most important determinants in the development of microbiota during early childhood, due to its impact on alpha and beta diversity (Christensen et al., 2022). However, there is also a risk of exacerbating asthma if the child has a pet-specific allergic sensitization (Pinot de Moira et al., 2022). Moreover, at least 56 bacterial genera were significantly more abundant in homes with dogs, such as Prevotella, Porphyromonas, Moraxella, and Bacteroides, which were found in the mouth and feces of the animals and also in their owners (Barberán et al., 2015; Hufnagl et al., 2020).
The relationship between hygiene hypothesis and its effect at immunology level can be explained by different mechanisms. First, exposure to a larger diversity of bacterial species enables the development of a balanced immune response. Second, these exposures might serve as the starting point for the development of the infant gastrointestinal microbiome. Third, dietary transitions that facilitate immune tolerance to food nutrients. Fourth, the environment in which one grows. Lack of appropriate microbial and diet exposure might be related with allergic diseases such as asthma (Penders et al., 2006; Fujimura et al., 2010; Garn et al., 2021; Pfefferle et al., 2021).
4.3 Antibiotics: use and abuse
Nowadays, antibiotics play an important role in modern medicine and have improved the prognosis of an endless number of patients, however, we must not forget they have several adverse effects to take into consideration, such as antibiotic resistance and microbiome alteration (Ni et al., 2019).
Several studies have shown that the use of antibiotics in early childhood increases the risk for developing asthma up to 2.3 times (Kim et al., 2018; Lin et al., 2020; Li et al., 2023), these risks increase even more with the number of antibiotics courses prescribed. In infants (<1 year) antibiotic use is associated with a 24% higher incidence of asthma for every 10% increase in prescriptions (Patrick et al., 2020). Among the most prescribed are B-lactams such as amoxicillin (2-fold), followed by macrolides, second-generation cephalosporins (2.7 fold) and sulfonamides (Marra et al., 2009; Li et al., 2023).
A cohort of 697 children demonstrates that the exposure to 2 or more antibiotics from B-lactam group, during the first year of life was related with an increment in the risk of asthma together with longitudinal changes in the nasal microbiome, being the most significant change Moraxella sparsity (Toivonen et al., 2021). In another experimental study where mice were exposed to antibiotic treatment there was an increase in Phylum Proteobacteria, and a decrease in Bacteroidetes and Firmicutes, in the same model, 2 weeks after antibiotic-free period Firmicutes and Bacteroidetes returned to dominance, however Proteobacteria turned up to be relatively increased, compared to controls (Antonopoulos et al., 2009), change that has been found in fecal samples from both allergic and non-allergic asthmatic subjects compared to healthy ones (Zheng et al., 2022).
Specifically, regarding amoxicillin antibiotic, a clinical trial in humans demonstrates that Clostridia and Firmicutes part of gut microbiome after cessation of treatment with this antibiotic were decreased in abundance. In fact, the use of amoxicillin for long periods of time was related with significant depletion of SCFAs bacterial species even after antibiotic therapy completion (Dhariwal et al., 2023). As mentioned before SCFAs mediate anti-inflammatory responses, explaining their protective effects against asthma and other allergic diseases (Blanco-Pérez et al., 2021).
An experimental study using mouse models demonstrated that the disturbance of the microbiota following antibiotic exposure leads to an elevated presence of fungal microbiota, particularly Candida albicans. These fungi are known to produce molecules with immunomodulatory functions, such as prostaglandin-like oxilipin protein (Noverr et al., 2004). Furthermore, the same study reported that the disruption of the microbiota resulted in an upregulation of the Th2 immune response to spores and ovalbumin, indicating a consistent allergic reaction (Noverr et al., 2004).
The complex interplay between antibiotics, microbiome changes, and the development of asthma calls for further research. However, it is true that antibiotics are essential for treating bacterial infections and that due to their impact on human microbiome and the risk they represent for the development of asthma and other allergic diseases their excessive or inappropriate use must be approached with caution, especially in children.
5 Adolescence, why does everything change?
Changes are a natural process in the course of life. Adolescence is an important transitioning phase of maturation from childhood to adulthood, comprising the 10th to 19th years according to the WHO. Adolescents experience rapid metabolic, immune, sexual, and psychosocial changes among many others. Adolescent development significantly influences the shift in the prevalence of diseases from childhood to adulthood, including asthma.
5.1 Adolescence, sexual dimorphism, and hormones
In puberty, significant changes occur in the metabolic, immune, and hormonal responses, which have lasting effects into adulthood. This crucial period shapes the pattern of immune reactions and hormonal regulation, setting the foundation for future responses. Analyses of gene expression and epigenetic modifications have revealed interactions between genotype and puberty on the expression of B cell (IGKV1-27 in males) and T cell (TRBV30 in females) antigen-recognition proteins, with the influence of genetic factors on gene expression to tend to diminish as puberty progresses. Genes associated with pulmonary function exhibit an upregulation, suggesting potential improvements in respiratory capacity during this stage. However, in females, changes in gene expression related to puberty demonstrate a positive correlation with asthma symptoms and an inverse correlation with pulmonary function. With a notable shift in the immune response from a predominantly innate to a more adaptive pattern in females (Resztak et al., 2023).
Hormones are involved in growth and development, especially during adolescence. Sex hormones, in turn, play a key role in the maturation of many tissues. Male and female endocrine patterns differ, resulting in what is known as sexual dimorphism, which has been studied in multiple etiologies. Recently, many research groups have investigated how this phenomenon affects the immune system and its relation with the microbiome (Ucciferri and Dunn, 2022).
The sex-specific prevalence of asthma changes throughout life. Boys have a higher prevalence of asthma than girls (CDC and Prevention. Asthma data, statistics, and Surveillance, 2020) and are twice as likely to be hospitalized for an asthma exacerbation (Kynyk et al., 2011). This pattern can be explained in part by the fact that they have increased allergic inflammation, elevated serum IgE levels (Borish et al., 2005), and dysanapsis, described as a reduction in airway diameter relative to lung volume (Pagtakhan et al., 1984).
In teenagers (around 11 to 16 years), asthma prevalence decreases in males but increases in females (Genuneit, 2014). It has also been observed that adult women are three times more likely to be hospitalized for an exacerbation than men and that this difference decreases after menopause (Troisi et al., 1995). Fu et al., studied this phenomenon and associated symptom progression in girls with the onset of puberty, at the time when it decreases in boys (Fu et al., 2014). During adolescence hormonal differences between males and females modify physiological aspects as well as abiotic (pH, oxygen levels, nutrition) and biotic factors (immune surveillance, signaling molecules), creating numerous niches that allow for the appearance of microbiome differences with further implications for the host. The best-studied example to date is the intestinal microbiome, which has been strongly implicated in numerous sex-specific physiological processes and diseases (Fuseini and Newcomb, 2017). Also, many studies have shown that alpha diversity tends to be higher in adult females than males, with less in older adults (de la Cuesta-Zuluaga et al., 2019; Takagi et al., 2019). Furthermore, studies have provided evidence supporting the presence of sexual dimorphism in the adult gut microbiome (Shin et al., 2019). In a mouse model, it was observed that androgens had a significant impact on the modulation of the gut microbiome and the glutamine/glutamate ratio (Gao et al., 2021). A study conducted on human dizygotic twins revealed that while male and female infant twins displayed conserved beta diversity of the gut microbiome, differentiation between the sexes became more apparent during puberty (Yatsunenko et al., 2012). Additionally, the beta diversity of the gut microbiomes in pubertal males and females became increasingly similar to the adult microbiomes of their respective sexes as they progressed further into puberty (Yuan et al., 2020).
Since both innate and adaptive immunity shapes many of the interactions in the gut microbiome and vice versa, the sexually dimorphic nature of immune systems shows us an association between gut microbiome allergy and autoimmune disorders, as it seems for Intestinal Bowel Disease (Sisk-Hackworth et al., 2023). Currently, it is unclear how immunity and microbiome dimorphism modify the natural history of asthma, but further research may enlighten it.
6 Adult, new adaptation
Asthma incidence is lower in adults compared to children, but adult asthma complications have a higher mortality rate (Dharmage et al., 2019). Adults with early-onset current asthma were more prone to atopic conditions and had a higher occurrence of asthma attacks, adult-onset asthma represents a unique phenotype primarily associated with environmental risk factors (Tan et al., 2015). However, He et al., reported through a prospective cohort study that adult-onset asthma has higher all-cause and cardiovascular mortality (He et al., 2021). Determining asthma prevalence in adults is challenging due to reliance on self-reporting and varied approaches in studies. Globally, doctor-diagnosed asthma, clinical/treated asthma, and wheezing prevalence in adults is 4.3, 4.5, and 8.6%, respectively, (To et al., 2012). Recent prevalence ranges from 5.4 to 17.9% depending on the definition and region (Song et al., 2016).
According to the phenotype, Wang et al. aimed to characterize the inflammatory response in acute and stable asthma in adults and children. They found that paucigranulocytic inflammation was the most common phenotype in children and adults with stable asthma. However, in acute asthma, neutrophilic inflammation was more prevalent in adults, while eosinophilic inflammation was more prevalent in children (Wang et al., 2017).
In healthy adults, the most common bacterial phyla in the lungs are Bacteroidetes, Firmicutes, and Proteobacteria. Dominant genera found in bronchoalveolar lavage (BAL) from healthy adults include Prevotella, Veillonella, Pseudomonas, Fusobacteria, and Streptococcus (Wang et al., 2017; Leviatan et al., 2022). Wang et al., compared the gut microbiota of 185 controls and 36 asthmatic adults in the UK and found Faecalibacterium prausnitzii, Sutterella wadsworthensis, and Bacteroides stercoris were depleted in cases, while Clostridium with Eggerthella were over-represented in individuals with asthma (Wang et al., 2018). Concerning the respiratory microbiome in adults with established asthma has been reported an increased abundance of the genera Streptococcus, Haemophilus, and Moraxella while a decrease of Prevotella and Corynebacterium, which is associated with proinflammatory response, associated with severe airway obstruction and airway neutrophilia, through activation of a Th2 response (Hufnagl et al., 2020).
Overall, changes in the microbiome that take place during adulthood can be more reliably linked to health and disease compared to younger individuals. Specific taxa have been found to have associations with both health and disease, indicating the importance of preserving potentially beneficial symbionts (Ghosh et al., 2022).
6.1 Environment pollution and microbiome in asthma adulthood
Asthma is a complex condition influenced by several factors, including the immune system, allergens, environmental triggers, and epigenetics. Within this intricate interplay, environmental factors play a significant role. These factors encompass a wide range of biomolecules, such as pollutants, household cleaners, microplastics, nanoparticles, and tobacco smoke.
A study conducted by Gehring et al., provided evidence of a notable increase in asthma occurrence among individuals exposed to pollutants during early life, which can have long-term consequences in adulthood (Gehring et al., 2020). In industrialized countries, where people spend most of their day indoors, the composition of indoor air is affected by various factors, including outdoor pollutants, ventilation quality and quantity, indoor allergens, and activities such as smoking, heating, and cooking. Microorganisms present in indoor air, particularly certain fungal species such as Aspergillus, Penicillium, and Cladosporium, have been associated with an increased risk of asthma in both children and adults (Sharpe et al., 2015).
Cigarette smoke contains nicotine, aldehydes, and polycyclic aromatic compounds, which can decrease endogenous antioxidants, increase lipid peroxidation, and induce oxidative stress. Furthermore, these substances can contribute to intestinal dysbiosis. Animal models have shown that cigarette smoking significantly reduces the concentrations of organic acids, such as acetic acid, propionic acid, butyric acid, and valeric acid, as well as the population of Bifidobacterium in the cecum, indicating the presence of intestinal dysbiosis (Tomoda et al., 2011). In a study by Pfeiffer et al., Prevotella, Veillonella, Streptococcus, and Actinomyces were found to be the most abundant genera in the respiratory tract of smokers (Pfeiffer et al., 2021). Furthermore, when evaluating smoking patterns, they observed a negative correlation between the prevalence of Corynebacterium and Dolosigranulum in nasal samples and the maximum number of cigarettes smoked daily. Simpson et al. examined asthma patients and characterized their sputum microbiota. Their findings showed that ex-smokers had a greater occurrence of the Fusobacteria phylum, as well as higher levels of Firmicutes and Bacteroidetes, while they had lower levels of Proteobacteria when compared to individuals who had never smoked. Additionally, they discovered a connection between smoking and increased bacterial diversity (Simpson et al., 2016). In contrast, Munk et al. report no significant changes in the microbiome of smoking asthmatic patients compared to those who have quit smoking (Munck et al., 2016). Hence, further research is needed to elucidate the connection between smoking and the microbiome in individuals with asthma.
6.2 Microbiome and asthma in occupationally exposed workers
Occupational asthma, which accounts for 10 to 25% of asthma cases in adulthood, is the most common form of occupational lung disease. It can be classified into different types based on its etiology, including work-exacerbated asthma, irritant-induced asthma, and immunologic occupational asthma. Low molecular weight isocyanates are particularly prevalent among the compounds responsible for occupational asthma (Kenyon et al., 2012; Maestrelli et al., 2020). Isocyanates have also been linked to dysbiosis observed in other chronic inflammatory diseases such as atopic dermatitis, as they disrupt the symbiotic pathways between Roseomonas mucosa and Staphylococcal species present on the skin (Zeldin et al., 2023).
Another study conducted by Ahmed et al., focused on ceramics industry workers in a major industrial Egyptian city compared to individuals from a rural village. They found a significant increase in the relative abundance of the Proteobacteria phylum in the industrial group (p = 0.02). The industrial group was predominantly populated by Staphylococcus, Sphingomonas, and Moraxella, leading to the conclusion that environmental pollution may alter the nasal microbiome and disrupt its community structure (Ahmed et al., 2019). While the changes in the microbiota resulting from occupational exposure are well-documented, as are their associations with other inflammatory diseases, establishing a direct link between these changes and the prevalence of asthma remains challenging based on current evidence. However, considering that occupational exposure is part of our life, several occupations could represent a risk factor for the development and exacerbation of asthma through diverse mechanisms.
6.3 Viral infections associated with the development of asthma
Respiratory viral infections play a crucial role in the development of asthma and are significant contributors to asthma exacerbations (Hofstra et al., 2015). Among viral infections, HRV infections are particularly common, as this pathogen circulates widely within the community. In adults, viral infections, especially HRV, are responsible for 50–80% of asthma exacerbations, with HRV being detected in up to 83% of adult cases (Jartti et al., 2020; Ojanguren et al., 2022).
In a study involving 88 adults hospitalized for asthma exacerbation, respiratory viruses were detected in 50% of the patients. HRV was the most frequently identified virus (77%), followed by human coronavirus (16%), parainfluenza virus (5%), and human metapneumovirus (2%). Six of these patients also had bacterial coinfections (Bjerregaard et al., 2017). Voraphani et al., reports that individuals who are active smokers and have a history of respiratory syncytial virus infections during the first 3 years are 1.7 times more likely to have current asthma as adults (Voraphani et al., 2014).
Interestingly, in a study of the virome in the sputum of asthma patients, Choi et al. reported an increase in the abundance of Cytomegalovirus (CMV) and Epstein–Barr virus (EBV). Additionally, there was a decrease in Streptococcus phage in patients who experienced exacerbations, which was correlated with more severe disease (Choi et al., 2021).
The precise mechanisms underlying virus-related asthma are still under investigation. However, deficient interferon-γ and interleukin-10 responses, along with an increase in Th2 cytokines such as interleukin-4, interleukin-5, and interleukin-13, have been strongly associated with poor clinical outcomes in the context of viral infections (Busse et al., 2010).
Moreover, respiratory virus infections have been found to induce changes in the composition of the upper respiratory tract microbiota. Infections with rhinovirus, for instance, can predispose individuals to bacterial diseases such as otitis media, sinusitis, and pneumonia (Hofstra et al., 2015). These infections have been linked to an increase in the relative abundance of bacteria such as Haemophilus, Neisseria, Streptococcus, and Moraxella. These alterations in bacterial composition have been associated with a neutrophilic airway phenotype and persistent asthma that is resistant to treatment (Ver Heul et al., 2019).
That is why it is necessary to continue investigating the complex interplay between environmental exposures, viral infections, dysbiosis, and asthma. Through this exploration we can gain valuable insights into the mechanisms driving asthma exacerbations and potentially develop new strategies for prevention and treatment.
7 Elderly, everything has changed
Aging refers to all natural and progressive physiological changes that lead to cellular senescence and a gradual decline in the organism’s biological functions and metabolic stress adaptability. Certain biomarkers help determine aging, such as bone density, frailty, muscle mass, cognitive function, cardiovascular health, some blood biometrics and chemistry parameters, and telomere length (López-Otín et al., 2013), and the microbiome (Partridge et al., 2018). For this reason, chronic systemic inflammation, immunosenescence, and microbiome changes are important for understanding aging diseases such as asthma.
7.1 Metabolic changes and comorbidities
Comorbidities are a fundamental factor when studying asthma. They can occur at all ages, however, from adulthood onwards they become more important. Yañez et al., showed that of a total of 152 elderly people with asthma, 36% had three or more comorbidities (Yáñez et al., 2018). Obesity, metabolic syndrome (MetS), and diabetes are among the most common metabolic disorders related to asthma, due to their high prevalence (Park et al., 2018). Furthermore, adipose tissue mass is positively related to high levels of proinflammatory molecules like leptin, IL-6, and TNF-a, and negatively related to anti-inflammatory markers such as adiponectin. Similarly, inflamed adipose tissue releases adipokines that circulate to the lungs and contribute to hyperresponsiveness (Shore, 2010; Park et al., 2018; Palma et al., 2022).
A connection has been established between dysbiosis and obesity, characterized by a decrease in the diversity of bacterial genera that constitute the microbiota (Kim et al., 2021). A study by Fu et al. reported that the richness of bacterial microbiota correlates negatively with body mass index and serum triglyceride levels, while positively correlating with serum High-density lipoprotein levels (Fu et al., 2015). Likewise, it has been reported that individuals with obesity and severe asthma showed an increase in taxa belonging to family Prevotellaceae, Mycoplasmataceae, Lachnospiraceae, and Spirochaetaceae (Huang et al., 2015).
The relationship between intestinal microbiota alterations and the improvement of asthma symptoms remains poorly understood. However, it is speculated that increased production of SCFAs, particularly butyrate and propionate, reduces pro-inflammatory cytokines and/or increased immunoregulatory cytokines (Kim et al., 2021). Nevertheless, these associations have been primarily described within the context of the intestinal microbiota, highlighting the need for further investigation of the relationship between asthma, obesity, and pulmonary microbiota dysbiosis.
7.2 Microbial succession in the final stage
Late succession has become very important as a subject of study because of the direct relationship between it and healthy aging. Over time, the alpha diversity of the microbiota decreases, and beta diversity increases, making older adults more susceptible to infection by opportunistic bacteria (Martino et al., 2022). In 2016, Biagi et al. showed that the gut microbiota of elderly people is dominated by the families Bacteroidaceae, Lachnospiraceae, and Ruminococcaceae (Biagi et al., 2016). However, other families such as Prevotellaceae, Enterococcaceae, Lactobacillaceae, Turicibacteraceae, Christensenellaceae, Clostridiaceae, Enterobacteriaceae, Bifidobacteriaceae, Porphyromonadaceae, Peptostreptococcaceae, and Coriobacteriaceae were also found. It has been described that the elderly generally has a gut microbiota composed predominantly of Firmicutes, Tenericutes, Actinobacteria, Lachnospira, and Proteobacteria.
Nevertheless, a decline in taxa such as Prevotella, Eubacterium, Bifidobacterium, Faecalibacterium, Coprococcus, and Roseburia is observed with increasing age. In contrast, Akkermansia, Odoribacter, Butyricimonas, Butyrivibrio, Oscillospira, Christensenellaceae, and Barnesiellaceae have been found to increase in abundance in older adults, which has been associated with healthy aging (Biagi et al., 2010; Claesson et al., 2012; Park et al., 2015; Biagi et al., 2016; Effendi et al., 2022). The gradual decline of some microorganisms directly affects systemic inflammation and disease development in the elderly. Additionally, some conformational changes are also associated with unhealthy aging, such as an increase in pathogenic microorganisms like Eggerthella, Bacteroides, Desulfovibrio, Enterobacteriaceae, Campylobacter, Streptococcus, Actinomyces, and Clostridium species.
Even though the gut microbiome is the best studied, organisms in the respiratory tract are also important when studying respiratory diseases such as asthma. A study reported by Lee et al., examined the composition of airway microbiota in young adults and elderly individuals, comparing those with and without asthma. The dominant phyla in young adults and elderly groups were Actinobacteria, Firmicutes, Proteobacteria, and Bacteroidetes, but their relative abundances differed significantly. Additionally, the research noted a higher prevalence of Moraxella in elderly individuals without asthma compared to their asthmatic counterparts (Lee et al., 2019). Recently, centenarian gut microbiota have been found to undergo new compositional changes despite differences or similarities between different populations. This has sparked interest in their study, as an increase in the abundance of genera such as Akkermansia, Bifidobacterium, Christensenellaceae, and other species associated with healthy aging has been described (Biagi et al., 2016; Kato et al., 2017). However, further studies need to be conducted to understand these relatively recent findings.
7.3 Immunosenescence and chronic inflammation
Immunosenescence is a multifactorial phenomenon in which both innate and acquired immunity are affected over time, impairing the effective immune response against pathogens, pathobionts and antigens (Van Den Munckhof et al., 2020). It is thought to result from a combination of three factors: Autoimmunity, Immunodeficiency, and Immune dysregulation (Chotirmall and Burke, 2015). The increase in pro-inflammatory cells leads to a chronic low-grade inflammatory state known as “inflammaging.” This inflammaging is a synergistic process between immunosenescence, chronic disease, and the microbiome in which older adults become vulnerable to potentially dangerous bacteria and increase the risk for diseases such as diabetes, obesity, heart disease, and asthma (Chotirmall and Burke, 2015; Huang et al., 2020).
Chronic inflammation is also mediated by the abundance of certain microorganisms. Short-chain fat-producing genera such as Faecalibacterium, Roseburia, Lachnospira, Eubacterium, Coprococcus, Butyricimonas, and Butyrivibrio have been studied to maintain immune homeostasis by downregulating proinflammatory mediators (Serrano-Villar et al., 2017; Effendi et al., 2022; Singh et al., 2023). Unfortunately, the progressive decrease of these genera leads to a deficiency of SCFA, which increases the permeability of the intestinal mucosa. For this reason, genera such as Akkermansia become more important as they are acetate producers (Bodogai et al., 2018; Wu et al., 2021). In contrast to the above genera, the increase of Bacteroides is associated with low-grade inflammation, as shown in a study conducted in Korea by Lim et al. (2021). This study also showed that increases in C. hathewayi positively correlate with activation of proinflammatory Th17 cells. Similarly, some Campylobacter strains produce cytolysin toxins that induce hyperinflammatory proteins, and Desulfovibrio oxidizes butyrate (Callahan et al., 2021).
Some microorganisms help regulate the immune system. Enterococcus faecalis is a ROS-producing species involved in oxidative metabolism. However, its progressive increase contributes to inflammation, increased apoptosis, and contributes to oxidative damage to mitochondrial and nuclear DNA (Hemachandra Reddy, 2011; Bullone and Lavoie, 2017). Bacteroides fragilis produces PSA, a polysaccharide that binds to B cells inducing CD4+ and CD8+ regulatory T cells, thereby secreting IL-10 (Ramakrishna et al., 2019). It has also been suggested that this species may stimulate and differentiate Treg cells and thus participate in immune regulation (Troy and Kasper, 2010; Johnson et al., 2015; Wu et al., 2021). A study by Li et al. (2023), showed that species such as Lactobacillus fermentum and Bacteroides fragilis play a role as probiotic strains. Their combined use in senescent mice improved neuronal cell necrosis, antioxidant capacity, and reduced inflammation levels (Li et al., 2023).
7.4 Microbiome and asthma in elderly people
Asthma is a heterogeneous phenotypic disease that has not been fully characterized in the elderly (Liu et al., 2020). However, it is known that reversible obstruction, hyperresponsiveness, and chronic airway inflammation are representative features of this disease. The deterioration of the immune system, systemic inflammation, impaired lung function, different phenotypes, airway remodeling, comorbidities and late onset of this disease complicate its investigation and treatment (Zhang and Huang, 2021). Allergens, tobacco, pollution, and diet are also directly involved in the development of the disease. With age, microbiome alterations in the elderly lead to opportunistic microorganisms colonizing the lungs as environmental conditions are optimal for their development and the immune system is less effective in eliminating them (Santacroce et al., 2020).
A study by Lee et al. analyzed the composition and functional profile of the microbiota in asthmatic and non-asthmatic elderly in Seoul (Lee et al., 2019). The genera Burkholderia and Psychrobacter were positively correlated with lower forced expiratory volume (FEV1). Therefore, the authors suggested that the low abundance of these microorganisms might be related to asthmatic features. It was also suggested that the increased abundance of Corynebacterium might be related to the development of asthma, as this genus has been described in other respiratory diseases such as rhinosinusitis.
Although asthma directly affects the airways, it has been discovered that the gut microbiota can be associated with lung function and asthma. Begley et al., showed that the gut microbiota of older adults in Michigan is certainly dominated by some Prevotella species and that they are associated with chronic inflammatory diseases (Begley et al., 2018). Staphylococcus aureus is a microorganism of the microbiota involved in the pathophysiology of airway diseases, including asthma. A study by Song et al., showed that staphylococcal enterotoxin IgE (SE-IgE) is significantly associated with asthma in the elderly, particularly with late-onset asthma (Song et al., 2016). This species has also been shown to produce staphylococcal enterotoxin B (SEB) which can induce Th2 polarization, inflammation and corticosteroid resistance and can inhibit regulatory T-cell functions in humans (Hauk et al., 2000; Cardona et al., 2006).
The elderly are a highly vulnerable sector of the population, diminished by the effects of aging and various diseases. For this reason, the study of the microbiota in the elderly has become a useful and fundamental tool to understand pathologies, find new treatments, and create an adequate culture of prevention. Research on the microbiota and its relationship with diseases such as asthma has been limited; however, understanding this relationship may lead to useful insights for people of other ages.
8 Effects of asthma treatment on the composition of microbial diversity
International Asthma guidelines define corticosteroids as the key asthma treatment (Reddel et al., 2022). These anti-inflammatory molecules inhibit the recruitment of immune cells in the airway by suppressing the production of IL-1B, IL-6, GM-CSF, ICAM-1, induce eosinophils apoptosis, and diminish the survival of T-lymphocytes and mast cells (Barnes, 2010). Usually inhaled corticosteroids (ICS) are enough to control symptoms and reduce complications, but during asthma exacerbations, higher doses are needed and sometimes Oral Corticosteroids (OCS) therapy is required, with increased adverse reactions because of their systemic effects (Perez-Garcia et al., 2020).
Zhou et al., conducted a longitudinal study to identify changes in nasal microbiota related to the risk of asthma exacerbations despite ICS therapy (Zhou et al., 2019). Nasal swabs were collected among 214 European children with mild–moderate asthma, at the time of well-controlled and during the first loss-asthma-control episode. Patients with nasal microbiome dominated by Corynebacterium and Dolosigranulum had fewer episodes of exacerbation and longer time between them compared to those with predominant Staphylococcus, Streptococcus, or Moraxella genera. Bacterial richness increased during exacerbations compared with well-controlled asthma. Furthermore, a higher relative abundance of Corynebacterium was associated with a lower risk of asthma exacerbations requiring OCS use, whereas Moraxella was associated with a higher risk of requiring OCS (Zhou et al., 2019).
Immune modulatory effects of corticosteroids might change the respiratory microbiome. Huang et al. evaluate the effect of ICS on microbiome composition, they studied asthma patients over 9 months, sampling before dosage, three months later, and nine months after the start of treatment. Genera Streptococcus, Rothia, Actinomyces, Leptotrichia, and Neisseria were identified as the predominant in all samples without significant differences between them or in alpha diversity during the study. More than two-fold decrease the percentage of Wallemia, Cladosporium, Penicillium and Alternia genera compared with baseline, concurrently with decrease in bacteria-fungus intra and inter-kingdom networks after ICS therapy (Huang et al., 2022). Martin et al., compared low- and high-dose ICS groups in sputum microbiome composition without significant differences in bacterial load or overall community (Martin et al., 2020). However, Streptococcus genera showed significantly higher relative abundance in subjects taking low-dose ICS and Haemophilus parainfluenzae was significantly more abundant in subjects on high-dose fluticasone propionate than those on high-dose budesonide over a 2-week period. Denner et al., studied the bronchial microbiome and correlated OCS use with a decrease in the relative abundance of Bacteroidetes and Fusobacteria with an increase in Proteobacteria phyla, generalized linear models on brush samples demonstrated OCS usage influence the relative abundance of Pseudomonas, Rickettsia, Lactobacillus and Streptococcus genera, significantly enriched in asthmatic patients sample (Denner et al., 2016). In addition, α-diversity in brush samples from asthmatic subjects was correlated with lowest FEV1 levels, a clinical parameter of airway obstruction.
Goleva et al., showed that asthma patients resistant to corticosteroid treatment occur due to the expansion of specific gram-negative bacteria in the airways, like Haemophilus parainfluenzae, the LPS of item interact with Toll-like receptor 4 and activate transforming growth factor-b-associated kinase-1 (TAK1), by MyD88 pathway resulting in the p38 MAPK activation and Nuclear Factor-kappa Beta (NF-kb), increasing the production of proinflammatory cytokines like IL-8, also activation of TAK1 inhibits the production of MKP-1 mediated by glucocorticoid receptor, this results in reduced cellular responses to corticosteroids and reduction of sensitivity to them (Goleva et al., 2013).
These studies show the complex correlation between microbiome and corticosteroids, enlightening the need for more research to better understand the phenomena and its implications for better and more reasonable treatment of asthma patients.
9 New perspective for asthma treatment: probiotics
The adaptive immune system provides versatile defense against infectious agents but faces the challenge of potential autoimmune inflammation due to T cell self-reactivity. Tregs play an important role in preventing autoimmunity. Tregs can reverse fatal autoimmunity, tissue pathology, and offer long-term protection (Hu et al., 2021).
Probiotics have the potential to modulate various types of immune cells, including T helper (Th)-1, Th2, Th17, Treg cells, and B cells, which play an important role in human health and the development of immune-related disorders. The use of probiotics has been associated with the modulation of the severity of allergic inflammation (Dargahi et al., 2019). The beneficial effect induced by probiotics is based on their ability to act as an “on/off” switch to control immune responses in a strain-dependent manner at the mucosal level. In allergic asthma, they protect the immune system’s homeostasis by regulating the balance between Th1 and Th2 cells, reducing the inflammatory response, modulating the gut microbiota, and increasing the number of Tregs (Huang et al., 2021).
In a study conducted by Wu et al., a probiotic formulation comprising Lactobacillus acidophilus, Lactobacillus rhamnosus, and Bifidobacterium animalis demonstrated the ability to regulate peribronchial inflammation and control the expression of the PI3K gene in individuals with allergic asthma (Wu et al., 2022). A systematic review conducted by Lin et al., revealed that supplementation with probiotics may reduce the number of asthma episodes (Lin et al., 2018). However, no significant improvements were observed in terms of symptoms during the daytime or nighttime, as well as pulmonary function measures such as FEV1 and PEF. Nevertheless, the authors emphasize the importance of further well-designed randomized controlled trials with larger sample sizes to fully evaluate the effects of probiotics in children with asthma.
10 Conclusion
The interplay between the host microbiome and asthma exhibits significant variations across diverse contexts, and whether a microbiome is considered healthy or disease-associated depends on the context (Table 1). A comprehensive understanding of these intricate interactions among variables and the microbiome is essential for unveiling the underlying mechanisms of asthma phenotypes and for developing precise interventions for prevention and treatment.
The microbiome has been shown to play a significant role in early-life immune development and modulation. It’s crucial to note that this interaction can be influenced by genetic factors, the mode of birth (vaginal delivery or cesarean section), first feeding method (breast or formula), upbringing environment (rural or urban, presence of older siblings or pets), among others. In early-life, it has been reported that certain genus in the gut microbiome, including Bifidobacterium, Lactobacillus, Faecalibacterium, and Bacteroides, play a protective role against asthma. During adolescence, changes in the microbiome occur, partially influenced by the maturation of the immune system and hormonal changes during this stage. As individuals reach adulthood, the impact of the microbiome on health and disease becomes more apparent, contributing to the development of chronic conditions that can lead to comorbidities and proinflammatory states that predispose individuals to asthma and other diseases in old age. In adults, there is evidence indicating an increase in the presence of Clostridium and Eggerthella in the gut microbiome of individuals with asthma. Moreover, genus such as Haemophilus, Streptococcus, Staphylococcus, and Moraxella in the respiratory microbiome have emerged as significant contributors to the pathogenesis of asthma. Additionally, the reduction of the genus Corynebacterium in the respiratory tract during early-life and adult stages has been associated with proinflammatory responses in specific contexts. However, this genus may also be linked to the development of asthma, particularly among the elderly population.
To illustrate this concept, let us consider a forest with its plant and animal species. The population and composition of these organisms can vary significantly based on whether the forest is in a temperate, equatorial, or Mediterranean climate. Moreover, the roles these organisms play are influenced by seasonal changes, creating distinct contexts. Much like this ecological example, the microbiome’s impact on various stages of human growth and development also demonstrates dynamic variations, including its relevance to asthma.
Our review has some limitations; many studies are confined to cohort designs, lacking long-term longitudinal data on individual changes. Furthermore, obtaining samples from the lower respiratory tract to investigate this microbiome remains challenging, and much of the information is derived from the gut microbiome. Moreover, a substantial portion of these studies relies on the sequencing and analysis of the 16S ribosomal gene, limiting the scope to bacterial aspects and gender-based analyses. Consequently, a comprehensive understanding of the virome and mycobiome is still needed. Therefore, further research and long-term follow-up studies are necessary to fully elucidate the mechanisms underlying these interactions and explore potential interventions, such as probiotics, that can modulate immune responses and improve health outcomes in diverse populations worldwide.
Author contributions
DG-C: Conceptualization, Data curation, Formal analysis, Investigation, Methodology, Project administration, Validation, Visualization, Writing – original draft, Writing – review & editing. IG-G: Formal analysis, Investigation, Writing – original draft, Writing – review & editing. KL-S: Formal analysis, Investigation, Writing – original draft, Writing – review & editing. VI-M: Formal analysis, Investigation, Writing – original draft, Writing – review & editing. FJ-J: Formal analysis, Investigation, Writing – original draft, Writing – review & editing. AT-G: Data curation, Formal analysis, Investigation, Writing – original draft, Writing – review & editing. CB-C: Formal analysis, Investigation, Validation, Writing – original draft, Writing – review & editing. MM-M: Formal analysis, Investigation, Visualization, Writing – original draft, Writing – review & editing. LJ-A: Formal analysis, Investigation, Visualization, Writing – original draft, Writing – review & editing. JZ: Conceptualization, Formal analysis, Funding acquisition, Investigation, Project administration, Supervision, Visualization, Writing – original draft, Writing – review & editing. AC: Conceptualization, Data curation, Formal analysis, Funding acquisition, Investigation, Project administration, Resources, Supervision, Validation, Visualization, Writing – original draft, Writing – review & editing.
Funding
The author(s) declare that no financial support was received for the research, authorship, and/or publication of this article.
Acknowledgments
This paper and the work behind it, would not have been possible without the lectures and support of the UNAM and Red MEDICI program.
Conflict of interest
The authors declare that the research was conducted in the absence of any commercial or financial relationships that could be construed as a potential conflict of interest.
Publisher’s note
All claims expressed in this article are solely those of the authors and do not necessarily represent those of their affiliated organizations, or those of the publisher, the editors and the reviewers. Any product that may be evaluated in this article, or claim that may be made by its manufacturer, is not guaranteed or endorsed by the publisher.
Abbreviations
WHO, World Health Organization; IL, Interleukins; TNF, Tumor Necrosis Factor; IFN, Interferon; Tregs, T Regulatory Cells; Th, T Helper Cells; GM-CSF, Granulocyte-Macrophage Colony-Stimulating Factor; ICAM, Intercellular Adhesion Molecule; NF-kB, Nuclear Factor-kappa B; TAK1, Transforming Growth Factor Beta-Activated Kinase 1; MAPK, Mitogen-Activated Protein Kinase; SCFAs, Short-Chain Fatty Acids; FEV1, Forced Expiratory Volume in 1 s; mbGWAS, microbiome- genome wide association; mbQTL, microbiome quantitative trait loci; PEF, Peak Expiratory Flow; C-section, Cesarean section; RSV, Respiratory Syncytial Virus; HRV, Human Rhinovirus; LPS, Lipopolysaccharide; TLR, Toll-Like Receptors; GABA, Gamma-Aminobutyric Acid; OVA, Ovalbumin; CDKIs, Cyclin-Dependent Kinase Inhibitors; SA- beta gal, Senescence-Associated Beta-Galactosidase; ROS, Reactive Oxygen Species; MetS, metabolic syndrome; ICS, Inhalated corticosteroids; OCS, Oral Corticosteroid.
References
Afzal, S., Ramzan, K., Ullah, S., Jamal, A., Basit, S., AlKattan, K. M., et al. (2023). Association between 17q21 variants and asthma predisposition in Pashtun population from Pakistan. J. Asthma 60, 63–75. doi: 10.1080/02770903.2021.2025391
Ahlawat, S., Asha,, and Sharma, K. K. (2021). Gut–organ axis: a microbial outreach and networking. Lett. Appl. Microbiol. 72, 636–668. doi: 10.1111/lam.13333
Ahluwalia, T. S., Eliasen, A. U., Sevelsted, A., Pedersen, C. T., Stokholm, J., Chawes, B., et al. (2020). ‘FUT2–ABO epistasis increases the risk of early childhood asthma and Streptococcus pneumoniae respiratory illnesses’, nature. Communications 11, 1–12. doi: 10.1038/s41467-020-19814-6
Ahmadizar, F., Vijverberg, S. J. H., Arets, H. G. M., de Boer, A., Garssen, J., Kraneveld, A. D., et al. (2017). Breastfeeding is associated with a decreased risk of childhood asthma exacerbations later in life. Pediatr. Allergy Immunol. 28, 649–654. doi: 10.1111/pai.12760
Ahmed, N., Mahmoud, N. F., Solyman, S., and Hanora, A. (2019). Human nasal microbiome as characterized by metagenomics differs markedly between rural and industrial communities in Egypt. OMICS J. Integr. Biol. 23, 573–582. doi: 10.1089/omi.2019.0144
Aldriwesh, M. G., al-Mutairi, A. M., Alharbi, A. S., Aljohani, H. Y., Alzahrani, N. A., Ajina, R., et al. (2023). Paediatric asthma and the microbiome: a systematic review. Microorganisms 11, 1–29. doi: 10.3390/microorganisms11040939
Alhasan, M. M., Cait, A. M., Heimesaat, M. M., Blaut, M., Klopfleisch, R., Wedel, A., et al. (2020). ‘Antibiotic use during pregnancy increases offspring asthma severity in a dose-dependent manner’, allergy: European. J. Allergy Clin. Immunol. 75, 1979–1990. doi: 10.1111/all.14234
Alliet, P., Vandenplas, Y., Roggero, P., Jespers, S. N. J., Peeters, S., Stalens, J. P., et al. (2022). Safety and efficacy of a probiotic-containing infant formula supplemented with 2’-fucosyllactose: a double-blind randomized controlled trial. Nutr. J. 21, 11–16. doi: 10.1186/s12937-022-00764-2
Alsharairi, N. A. (2020). The role of short-chain fatty acids in the interplay between a very low-calorie ketogenic diet and the infant gut microbiota and its therapeutic implications for reducing asthma. Int. J. Mol. Sci. 21, 1–21. doi: 10.3390/ijms21249580
Anderson, E. J., Carbonell-Estrany, X., Blanken, M., Lanari, M., Sheridan-Pereira, M., Rodgers-Gray, B., et al. (2017). Burden of severe respiratory syncytial virus disease among 33-35 weeks’ gestational age infants born during multiple respiratory syncytial virus seasons. Pediatr. Infect. Dis. J. 36, 160–167. doi: 10.1097/INF.0000000000001377
Anderson, J., do, L. A. H., Wurzel, D., and Licciardi, P. V. (2023). ‘Understanding the increased susceptibility to asthma development in preterm infants’, allergy: European. J. Allergy Clin. Immunol. 78, 928–939. doi: 10.1111/all.15662
Antonopoulos, D. A., Huse, S. M., Morrison, H. G., Schmidt, T. M., Sogin, M. L., and Young, V. B. (2009). Reproducible community dynamics of the gastrointestinal microbiota following antibiotic perturbation. Infect. Immun. 77, 2367–2375. doi: 10.1128/IAI.01520-08
Arif, A. A., and Veri, S. D. (2019). The association of prenatal risk factors with childhood asthma. J. Asthma 56, 1056–1061. doi: 10.1080/02770903.2018.1515224
Arrieta, M. C., Stiemsma, L. T., Dimitriu, P. A., Thorson, L., Russell, S., Yurist-Doutsch, S., et al. (2015). Early infancy microbial and metabolic alterations affect risk of childhood asthma. Sci. Transl. Med. 7:307ra152. doi: 10.1126/scitranslmed.aab2271
Arroyas, M., Calvo, C., Rueda, S., Esquivias, M., Gonzalez-Menchen, C., Gonzalez-Carrasco, E., et al. (2020). Asthma prevalence, lung and cardiovascular function in adolescents born preterm. Sci. Rep. 10, 19616–19610. doi: 10.1038/s41598-020-76614-0
Azad, M. B., Konya, T., Maughan, H., Guttman, D. S., Field, C. J., Sears, M. R., et al. (2013). Infant gut microbiota and the hygiene hypothesis of allergic disease: impact of household pets and siblings on microbiota composition and diversity. Allergy Asthma Clin. Immunol. 9, 1–9. doi: 10.1186/1710-1492-9-15
Barberán, A., Dunn, R. R., Reich, B. J., Pacifici, K., Laber, E. B., Menninger, H. L., et al. (2015). The ecology of microscopic life in household dust. Proc. Biol. Sci. 282:20151139. doi: 10.1098/rspb.2015.1139
Barcik, W., Boutin, R. C. T., Sokolowska, M., and Finlay, B. B. (2020). The role of lung and gut microbiota in the pathology of asthma. Immunity 52, 241–255. doi: 10.1016/j.immuni.2020.01.007
Begley, L., Madapoosi, S., Opron, K., Ndum, O., Baptist, A., Rysso, K., et al. (2018). ‘Gut microbiota relationships to lung function and adult asthma phenotype: a pilot study’, BMJ open. Respir. Res. 5:e000324. doi: 10.1136/bmjresp-2018-000324
Berg, G., et al. (2020). Microbiome definition re-visited: old concepts and new challenges. Microbiome. 2020;8(1):1–22. Definition re-visited: old concepts and new challenges. Microbiome 8, 1–22.
Biagi, E., Franceschi, C., Rampelli, S., Severgnini, M., Ostan, R., Turroni, S., et al. (2016). Gut microbiota and extreme longevity. Curr. Biol. 26, 1480–1485. doi: 10.1016/j.cub.2016.04.016
Biagi, E., Nylund, L., Candela, M., Ostan, R., Bucci, L., Pini, E., et al. (2010). Through ageing, and beyond: gut microbiota and inflammatory status in seniors and centenarians. PLoS One 5:e10667. doi: 10.1371/journal.pone.0010667
Biesbroek, G., Bosch, A. A. T. M., Wang, X., Keijser, B. J. F., Veenhoven, R. H., Sanders, E. A. M., et al. (2014). The impact of breastfeeding on nasopharyngeal microbial communities in infants. Am. J. Respir. Crit. Care Med. 190, 298–308. doi: 10.1164/rccm.201401-0073OC
Bisgaard, H., Hermansen, M. N., Buchvald, F., Loland, L., Halkjaer, L. B., Bønnelykke, K., et al. (2007). Childhood asthma after bacterial colonization of the airway in neonates. N. Engl. J. Med. 357, 1487–1495. doi: 10.1056/NEJMoa052632
Bizzintino, J., Lee, W. M., Laing, I. A., Vang, F., Pappas, T., Zhang, G., et al. (2011). Association between human rhinovirus C and severity of acute asthma in children. Eur. Respir. J. 37, 1037–1042. doi: 10.1183/09031936.00092410
Bjerregaard, A., Laing, I. A., Poulsen, N., Backer, V., Sverrild, A., Fally, M., et al. (2017). Characteristics associated with clinical severity and inflammatory phenotype of naturally occurring virus-induced exacerbations of asthma in adults. Respir. Med. 123, 34–41. doi: 10.1016/j.rmed.2016.12.010
Blanco-Pérez, F., Steigerwald, H., Schülke, S., Vieths, S., Toda, M., and Scheurer, S. (2021). The dietary Fiber pectin: health benefits and potential for the treatment of allergies by modulation of gut microbiota. Curr Allergy Asthma Rep 21:43. doi: 10.1007/s11882-021-01020-z
Bodogai, M., O’Connell, J., Kim, K., Kim, Y., Moritoh, K., Chen, C., et al. (2018). Commensal bacteria contribute to insulin resistance in aging by activating innate B1a cells. Sci. Transl. Med. 10, 1–26. doi: 10.1126/scitranslmed.aat4271
Boquien, C. Y. (2018). Human milk: an ideal food for nutrition of preterm newborn. Front. Pediatr. 6, 1–9. doi: 10.3389/fped.2018.00295
Borish, L., Chipps, B., Deniz, Y., Gujrathi, S., Zheng, B., and Dolan, C. M. (2005). Total serum IgE levels in a large cohort of patients with severe or difficult-to-treat asthma. Ann. Allergy Asthma Immunol. 95, 247–253. doi: 10.1016/S1081-1206(10)61221-5
Bosch, A. A. T. M., Levin, E., van Houten, M. A., Hasrat, R., Kalkman, G., Biesbroek, G., et al. (2016). Development of upper respiratory tract microbiota in infancy is affected by mode of delivery. EBioMedicine 9, 336–345. doi: 10.1016/j.ebiom.2016.05.031
Boulund, U., Bastos, D. M., Ferwerda, B., van den Born, B. J., Pinto-Sietsma, S. J., Galenkamp, H., et al. (2022). Gut microbiome associations with host genotype vary across ethnicities and potentially influence cardiometabolic traits. Cell Host Microbe 30, 1464–1480.e6. doi: 10.1016/j.chom.2022.08.013
Braun-Fahrländer, C., Riedler, J., Herz, U., Eder, W., Waser, M., Grize, L., et al. (2002). Environmental exposure to endotoxin and its relation to asthma in school-aged children. N. Engl. J. Med. 347:869. doi: 10.1056/NEJMoa020057
Breit, S., Kupferberg, A., Rogler, G., and Hasler, G. (2018). Vagus nerve as modulator of the brain-gut axis in psychiatric and inflammatory disorders. Front. Psych. 9:e0044. doi: 10.3389/fpsyt.2018.00044
Bullone, M., and Lavoie, J. P. (2017). The contribution of oxidative stress and inflamm-aging in human and equine asthma. Int. J. Mol. Sci. 18, 1–23. doi: 10.3390/ijms18122612
Bush, A. (2019). Pathophysiological mechanisms of asthma. Front. Pediatr. 7, 1–17. doi: 10.3389/fped.2019.00068
Busse, W., Lemanske, R., and Gern, J. (2010). Role of viral respiratory infections in asthma and asthma exacerbations. Lancet 376, 826–834. doi: 10.1016/S0140-6736(10)61380-3
Busse, W. W., and Rosenwasser, L. J. (2003). Mechanisms of asthma. J. Allergy Clin. Immunol. 111, S799–S804. doi: 10.1067/mai.2003.158
Callahan, S. M., Dolislager, C. G., and Johnson, J. G. (2021). The host cellular immune response to infection by campylobacter s and its role in disease. Infect. Immun. 89, e0011621–e0011617. doi: 10.1128/IAI.00116-21
Campbell, B., Raherison, C., Lodge, C. J., Lowe, A. J., Gislason, T., Heinrich, J., et al. (2017). The effects of growing up on a farm on adult lung function and allergic phenotypes: an international population-based study. Thorax 72, 236–244. doi: 10.1136/thoraxjnl-2015-208154
Cardona, I. D., Goleva, E., Ou, L. S., and Leung, D. Y. M. (2006). Staphylococcal enterotoxin B inhibits regulatory T cells by inducing glucocorticoid-induced TNF receptor-related protein ligand on monocytes. J. Allergy Clin. Immunol. 117, 688–695. doi: 10.1016/j.jaci.2005.11.037
Carr, T. F., Alkatib, R., and Kraft, M. (2019). Microbiome in mechanisms of asthma. Clin. Chest Med. 40, 87–96. doi: 10.1016/j.ccm.2018.10.006
Castro-Nallar, E., Shen, Y., Freishtat, R. J., Pérez-Losada, M., Manimaran, S., Liu, G., et al. (2015). Integrating microbial and host transcriptomics to characterize asthma-associated microbial communities. BMC Med. Genet. 8, 50–59. doi: 10.1186/s12920-015-0121-1
Castro-Rodriguez, J. A., Forno, E., Rodriguez-Martinez, C. E., and Celedón, J. C. (2016). ‘Risk and protective factors for childhood asthma: what is the evidence?’, Journal of allergy and clinical immunology. In Pract. 4, 1111–1122. doi: 10.1016/j.jaip.2016.05.003
CDC and Prevention. Asthma data, statistics, and Surveillance (2020). Available at: https://www.cdc.gov/asthma/most_recent_national_asthma_data.htm (accessed May 2023).
Chen, Y. C., Chen, Y., Lasky-Su, J., Kelly, R. S., Stokholm, J., Bisgaard, H., et al. (2023). Environmental and genetic associations with aberrant early-life gut microbial maturation in childhood asthma. J. Allergy Clin. Immunol. 151, 1494–1502.e14. doi: 10.1016/j.jaci.2023.01.006
Chesné, J., Braza, F., Mahay, G., Brouard, S., Aronica, M., and Magnan, A. (2014). IL-17 in severe asthma: where do we stand? Am. J. Respir. Crit. Care Med. 190, 1094–1101. doi: 10.1164/rccm.201405-0859PP
Choi, S., Sohn, K. H., Jung, J. W., Kang, M. G., Yang, M. S., Kim, S., et al. (2021). Lung virome: new potential biomarkers for asthma severity and exacerbation. J. Allergy Clin. Immunol. 148, 1007–1015.e9. doi: 10.1016/j.jaci.2021.03.017
Chotirmall, S. H., and Burke, C. M. (2015). Aging and the microbiome: implications for asthma in the elderly? Expert Rev. Respir. Med. 9, 125–128. doi: 10.1586/17476348.2015.1002473
Christensen, E. D., Hjelmsø, M. H., Thorsen, J., Shah, S., Redgwell, T., Poulsen, C. E., et al. (2022). The developing airway and gut microbiota in early life is influenced by age of older siblings. Microbiome 10, 106–115. doi: 10.1186/s40168-022-01305-z
Claesson, M. J., Jeffery, I. B., Conde, S., Power, S. E., O’Connor, E. M., Cusack, S., et al. (2012). Gut microbiota composition correlates with diet and health in the elderly. Nature 488, 178–184. doi: 10.1038/nature11319
Crump, C., Sundquist, J., and Sundquist, K. (2023). Preterm or early term birth and long-term risk of asthma into midadulthood: a national cohort and cosibling study. Thorax 78, 653–660. doi: 10.1136/thorax-2022-218931
Dargahi, N., Johnson, J., Donkor, O., Vasiljevic, T., and Apostolopoulos, V. (2019). Immunomodulatory effects of probiotics: can they be used to treat allergies and autoimmune diseases? Maturitas 119, 25–38. doi: 10.1016/j.maturitas.2018.11.002
de la Cuesta-Zuluaga, J., Kelley, S. T., Chen, Y., Escobar, J. S., Mueller, N. T., Ley, R. E., et al. (2019). Age- and sex-dependent patterns of gut microbial diversity in human adults. mSystems 4:e00261–19. doi: 10.1128/msystems.00261-19
Denner, D. R., Sangwan, N., Becker, J. B., Hogarth, D. K., Oldham, J., Castillo, J., et al. (2016). Corticosteroid therapy and airflow obstruction influence the bronchial microbiome, which is distinct from that of bronchoalveolar lavage in asthmatic airways. J. Allergy Clin. Immunol. 137, 1398–1405.e3. doi: 10.1016/j.jaci.2015.10.017
Depner, M., Taft, D. H., Kirjavainen, P. V., Kalanetra, K. M., Karvonen, A. M., Peschel, S., et al. (2020). Maturation of the gut microbiome during the first year of life contributes to the protective farm effect on childhood asthma. Nat. Med. 26, 1766–1775. doi: 10.1038/s41591-020-1095-x
Dhariwal, A., Haugli Bråten, L. C., Sturød, K., Salvadori, G., Bargheet, A., Åmdal, H., et al. (2023). Differential response to prolonged amoxicillin treatment: long-term resilience of the microbiome versus long-lasting perturbations in the gut resistome. Gut Microbes 15, 1–22. doi: 10.1080/19490976.2022.2157200
Dharmage, S. C., Perret, J. L., and Custovic, A. (2019). ‘Epidemiology of asthma in children and adults’, Frontiers. Pediatrics 7, 1–15. doi: 10.3389/fped.2019.00246
Dickson, R. P., Erb-Downward, J. R., Martinez, F. J., and Huffnagle, G. B. (2016). The microbiome and the respiratory tract. Annu. Rev. Physiol. 78, 481–504. doi: 10.1146/annurev-physiol-021115-105238
Doherty, A. M., Lodge, C. J., Dharmage, S. C., Dai, X., Bode, L., and Lowe, A. J. (2018). ‘Human milk oligosaccharides and associations with immune-mediated disease and infection in childhood: a systematic review’, Frontiers. Pediatrics 6, 1–8. doi: 10.3389/fped.2018.00091
Domenici, R., and Vierucci, F. (2022). Exclusive breastfeeding and vitamin D supplementation: a positive synergistic effect on prevention of childhood infections? Int. J. Environ. Res. Public Health 19, 1–29. doi: 10.3390/ijerph19052973
Donald, K., and Finlay, B. B. (2023). Early-life interactions between the microbiota and immune system: impact on immune system development and atopic disease. Nat. Rev. Immunol. 23, 735–748. doi: 10.1038/s41577-023-00874-w
Dong, T. S., and Gupta, A. (2019). Influence of early life, diet, and the environment on the microbiome. Clin. Gastroenterol. Hepatol. 17, 231–242. doi: 10.1016/j.cgh.2018.08.067
Douros, K., Moustaki, M., Tsabouri, S., Papadopoulou, A., Papadopoulos, M., and Priftis, K. N. (2017). Prenatal maternal stress and the risk of asthma in children. Front. Pediatr. 5, 1–9. doi: 10.3389/fped.2017.00202
Durack, J., Kimes, N. E., Lin, D. L., Rauch, M., McKean, M., McCauley, K., et al. (2018). ‘Delayed gut microbiota development in high-risk for asthma infants is temporarily modifiable by Lactobacillus supplementation’, nature. Communications 9, 1–9. doi: 10.1038/s41467-018-03157-4
Dzidic, M., Mira, A., Artacho, A., Abrahamsson, T. R., Jenmalm, M. C., and Collado, M. C. (2020). Allergy development is associated with consumption of breastmilk with a reduced microbial richness in the first month of life. Pediatr. Allergy Immunol. 31, 250–257. doi: 10.1111/pai.13176
Effendi, R. M. R. A., Anshory, M., Kalim, H., Dwiyana, R. F., Suwarsa, O., Pardo, L. M., et al. (2022). Akkermansia muciniphila and Faecalibacterium prausnitzii in immune-related diseases. Microorganisms 10, 1–16. doi: 10.3390/microorganisms10122382
Elliott, L., et al. (2009). Prospective study of breastfeeding in relation to wheeze, atopy, and bronchial hyperresponsiveness in the Avon longitudinal study of parents and children (ALSPAC). Genomics 94, 20–31. doi: 10.1016/j.jaci.2008.04.001.Prospective
Enaud, R., Prevel, R., Ciarlo, E., Beaufils, F., Wieërs, G., Guery, B., et al. (2020). The gut-lung Axis in health and respiratory diseases: a place for inter-organ and inter-kingdom Crosstalks. Front. Cell. Infect. Microbiol. 10, 1–11. doi: 10.3389/fcimb.2020.00009
Eslami, M., Bahar, A., Keikha, M., Karbalaei, M., Kobyliak, N. M., and Yousefi, B. (2020). Probiotics function and modulation of the immune system in allergic diseases. Allergol. Immunopathol. 48, 771–788. doi: 10.1016/j.aller.2020.04.005
Esmeraldino, L., Traebert, E., Nunes, R. D., and Traebert, J. (2022). Association between prenatal and neonatal factors and occurrence of asthma symptoms in six-year-old children. Ciencia e Saude Coletiva 27, 545–554. doi: 10.1590/1413-81232022272.44892020
Fazlollahi, M., Lee, T. D., Andrade, J., Oguntuyo, K., Chun, Y., Grishina, G., et al. (2018). The nasal microbiome in asthma. J. Allergy Clin. Immunol. 142, 834–843.e2. doi: 10.1016/j.jaci.2018.02.020
Ferllini Montealegre, S. M., Miranda Muñoz, M. F., and Vindas Vargas, J. F. (2019). Revista Medica Sinergia 4, e266. doi: 10.31434/rms.v4i9.266,
Finotello, F., Mastrorilli, E., and Di Camillo, B. (2018). Measuring the diversity of the human microbiota with targeted next-generation sequencing. Brief. Bioinform. 19, bbw119–bbw692. doi: 10.1093/bib/bbw119
Fu, J., Bonder, M. J., Cenit, M. C., Tigchelaar, E. F., Maatman, A., Dekens, J. A. M., et al. (2015). The gut microbiome contributes to a substantial proportion of the variation in blood lipids. Circ. Res. 117, 817–824. doi: 10.1161/CIRCRESAHA.115.306807
Fu, L., Freishtat, R. J., Gordish-Dressman, H., Teach, S. J., Resca, L., Hoffman, E. P., et al. (2014). Natural progression of childhood asthma symptoms and strong influence of sex and puberty. Ann. Am. Thorac. Soc. 11, 939–944. doi: 10.1513/AnnalsATS.201402-084OC
Fujimura, K. E., Johnson, C. C., Ownby, D. R., Cox, M. J., Brodie, E. L., Havstad, S. L., et al. (2010). Man’s best friend? The effect of pet ownership on house dust microbial communities. J. Allergy Clin. Immunol. 126, 8–13. doi: 10.1016/j.jaci.2010.05.042
Fuseini, H., and Newcomb, D. C. (2017). Mechanisms Driving Gender Differences in Asthma. Curr Allergy Asthma Rep 17:19. doi: 10.1007/s11882-017-0686-1
Gao, A., Su, J., Liu, R., Zhao, S., Li, W., Xu, X., et al. (2021). ‘Sexual dimorphism in glucose metabolism is shaped by androgen-driven gut microbiome’, nature. Communications 12, 1–14. doi: 10.1038/s41467-021-27187-7
Garcia-Marcos, L., Castro-Rodriguez, J. A., Weinmayr, G., Panagiotakos, D. B., Priftis, K. N., and Nagel, G. (2013). Influence of Mediterranean diet on asthma in children: a systematic review and meta-analysis. Pediatr. Allergy Immunol. 24, 330–338. doi: 10.1111/pai.12071
García-Serna, A. M., Martín-Orozco, E., Hernández-Caselles, T., and Morales, E. (2021). Prenatal and perinatal environmental influences shaping the neonatal immune system: a focus on asthma and allergy origins. Int. J. Environ. Res. Public Health 18, 1–24. doi: 10.3390/ijerph18083962
Garedew, L., Berhanu, A., Mengesha, D., and Tsegay, G. (2012). Identification of gram-negative bacteria from critical control points of raw and pasteurized cow milk consumed at Gondar town and its suburbs, Ethiopia. BMC Public Health 12:1. doi: 10.1186/1471-2458-12-950
Garn, H., Potaczek, D. P., and Pfefferle, P. I. (2021). The hygiene hypothesis and new perspectives—current challenges meeting an old postulate. Front. Immunol. 12, 1–7. doi: 10.3389/fimmu.2021.637087
Gehring, U., Wijga, A. H., Koppelman, G. H., Vonk, J. M., Smit, H. A., and Brunekreef, B. (2020). Air pollution and the development of asthma from birth until young adulthood. Eur. Respir. J. 56:2000147. doi: 10.1183/13993003.00147-2020
Genuneit, J. (2014). Sex-specific development of asthma differs between farm and nonfarm children: a cohort study. Am. J. Respir. Crit. Care Med. 5, 588–590. doi: 10.1164/rccm.201403-0428LE
Ghosh, T. S., Shanahan, F., and O’Toole, P. W. (2022). Toward an improved definition of a healthy microbiome for healthy aging. Nat. Aging 2, 1054–1069. doi: 10.1038/s43587-022-00306-9
Goleva, E., Jackson, L. P., Harris, J. K., Robertson, C. E., Sutherland, E. R., Hall, C. F., et al. (2013). The effects of airway microbiome on corticosteroid responsiveness in asthma. Am. J. Respir. Crit. Care Med. 188, 1193–1201. doi: 10.1164/rccm.201304-0775OC
Gomez de Agüero, M., Ganal-Vonarburg, S. C., Fuhrer, T., Rupp, S., Uchimura, Y., Li, H., et al. (2016). The maternal microbiota drives early postnatal innate immune development. Science 351, 1296–1302. doi: 10.1126/science.aad2571
Gray, L. E. K., O’Hely, M., Ranganathan, S., Sly, P. D., and Vuillermin, P. (2017). The maternal diet, gut bacteria, and bacterial metabolites during pregnancy influence offspring asthma. Front. Immunol. 8, 1–13. doi: 10.3389/fimmu.2017.00365
Ham, J., Kim, J., Choi, S., Park, J., Baek, M. G., Kim, Y. C., et al. (2021). Interactions between ncr+ilc3s and the microbiome in the airways shape asthma severity. Immune Network 21, 1–16. doi: 10.4110/in.2021.21.e25
Han, W., Tang, C., Baba, S., Hamada, T., Shimazu, T., and Iwakura, Y. (2021). Ovalbumin-induced airway inflammation is ameliorated in Dectin-1–deficient mice, in which pulmonary regulatory T cells are expanded through modification of intestinal commensal Bacteria. J. Immunol. 206, 1991–2000. doi: 10.4049/jimmunol.2001337
Hauk, P. J., Hamid, Q. A., Chrousos, G. P., and Leung, D. Y. M. (2000). Induction of corticosteroid insensitivity in human PBMCS by microbial superantigens. J. Allergy Clin. Immunol. 105, 782–787. doi: 10.1067/mai.2000.105807
He, X., Cheng, G. S., He, L., Liao, B., du, Y. J., Xie, X., et al. (2021). Adults with current asthma but not former asthma have higher all-cause and cardiovascular mortality: a population-based prospective cohort study. Sci. Rep. 11:1329. doi: 10.1038/s41598-020-79264-4
Healy, D. B., Ryan, C. A., Ross, R. P., Stanton, C., and Dempsey, E. M. (2022). Clinical implications of preterm infant gut microbiome development. Nat. Microbiol. 7, 22–33. doi: 10.1038/s41564-021-01025-4
Hemachandra Reddy, P. (2011). Mitochondrial dysfunction and oxidative stress in asthma: implications for mitochondria-targeted antioxidant therapeutics. Pharmaceuticals 4, 429–456. doi: 10.3390/ph4030429
Hilty, M., Burke, C., Pedro, H., Cardenas, P., Bush, A., Bossley, C., et al. (2010). Disordered microbial communities in asthmatic airways. PLoS One 5:e8578. doi: 10.1371/journal.pone.0008578
Hizawa, N. (2023). The understanding of asthma pathogenesis in the era of precision medicine. Allergol. Int. 72, 3–10. doi: 10.1016/j.alit.2022.09.001
Hofstra, J. J., Matamoros, S., van de Pol, M. A., de Wever, B., Tanck, M. W., Wendt-Knol, H., et al. (2015). Changes in microbiota during experimental human rhinovirus infection. BMC Infect. Dis. 15, 336–339. doi: 10.1186/s12879-015-1081-y
House, J. S., Wyss, A. B., Hoppin, J. A., Richards, M., Long, S., Umbach, D. M., et al. (2017). Early-life farm exposures and adult asthma and atopy in the agricultural lung health study. J. Allergy Clin. Immunol. 140, 249–256.e14. doi: 10.1016/j.jaci.2016.09.036
Hu, W., Wang, Z. M., Feng, Y., Schizas, M., Hoyos, B. E., van der Veeken, J., et al. (2021). Regulatory T cells function in established systemic inflammation and reverse fatal autoimmunity. Nat. Immunol. 22, 1163–1174. doi: 10.1038/s41590-021-01001-4
Huang, Y. J., Nariya, S., Harris, J. M., Lynch, S. V., Choy, D. F., Arron, J. R., et al. (2015). The airway microbiome in patients with severe asthma: associations with disease features and severity. J. Allergy Clin. Immunol. 136, 874–884. doi: 10.1016/j.jaci.2015.05.044
Huang, C., Ni, Y., du, W., and Shi, G. (2022). Effect of inhaled corticosteroids on microbiome and microbial correlations in asthma over a 9-month period. Clin. Transl. Sci. 15, 1723–1736. doi: 10.1111/cts.13288
Huang, C., Wang, Y., Li, X., Ren, L., Zhao, J., Hu, Y., et al. (2020). Clinical features of patients infected with 2019 novel coronavirus in Wuhan, China. Lancet 395, 497–506. doi: 10.1016/S0140-6736(20)30183-5
Huang, J., Yang, Z., Li, Y., Chai, X., Liang, Y., Lin, B., et al. (2021). Lactobacillus paracasei R3 protects against dextran sulfate sodium (DSS)-induced colitis in mice via regulating Th17/Treg cell balance. J. Transl. Med. 19, 1–13. doi: 10.1186/s12967-021-02943-x
Hufnagl, K., Pali-Schöll, I., Roth-Walter, F., and Jensen-Jarolim, E. (2020). Dysbiosis of the gut and lung microbiome has a role in asthma. Semin. Immunopathol. 42, 75–93. doi: 10.1007/s00281-019-00775-y
Iebba, V., Totino, V., Gagliardi, A., Santangelo, F., Cacciotti, F., Trancassini, M., et al. (2016). Eubiosis and dysbiosis: the two sides of the microbiota. New Microbiol. 39, 1–12.
Jartti, T., Bønnelykke, K., Elenius, V., and Feleszko, W. (2020). Role of viruses in asthma. Semin. Immunopathol. 42, 61–74. doi: 10.1007/s00281-020-00781-5
Jiménez, E., Marín, M. L., Martín, R., Odriozola, J. M., Olivares, M., Xaus, J., et al. (2008). Is meconium from healthy newborns actually sterile? Res. Microbiol. 159, 187–193. doi: 10.1016/j.resmic.2007.12.007
Johnson, J. L., Jones, M. B., and Cobb, B. A. (2015). Polysaccharide a from the capsule of Bacteroides fragilis induces clonal CD4+ T cell expansion. J. Biol. Chem. 290, 5007–5014. doi: 10.1074/jbc.M114.621771
Kalbermatter, C., Fernandez Trigo, N., Christensen, S., and Ganal-Vonarburg, S. C. (2021). Maternal microbiota, early life colonization and breast Milk drive immune development in the newborn. Front. Immunol. 12, 1–22. doi: 10.3389/fimmu.2021.683022
Kato, K., Odamaki, T., Mitsuyama, E., Sugahara, H., Xiao, J. Z., and Osawa, R. (2017). Age-related changes in the composition of gut Bifidobacterium species. Curr. Microbiol. 74, 987–995. doi: 10.1007/s00284-017-1272-4
Kenyon, N. J., Morrissey, B. M., Schivo, M., and Albertson, T. E. (2012). Occupational asthma. Clin. Rev. Allergy Immunol. 43, 3–13. doi: 10.1007/s12016-011-8272-0
Keski-Nisula, L., Kyynäräinen, H. R., Kärkkäinen, U., Karhukorpi, J., Heinonen, S., and Pekkanen, J. (2013). Maternal intrapartum antibiotics and decreased vertical transmission of Lactobacillus to neonates during birth Acta Paediatrica. Int. J. Paediatr. 102, 480–485. doi: 10.1111/apa.12186
Kim, D. H., Han, K., and Kim, S. W. (2018). Effects of antibiotics on the development of asthma and other allergic diseases in children and adolescents. Allergy Asthma Immunol. Res. 10, 457–465. doi: 10.4168/aair.2018.10.5.457
Kim, Y., Womble, J. T., Gunsch, C. K., and Ingram, J. L. (2021). The gut/lung microbiome Axis in obesity, asthma and bariatric surgery: a literature review. Yeon. Obesity 29, 636–644. doi: 10.1002/oby.23107
Klopp, A., Vehling, L., Becker, A. B., Subbarao, P., Mandhane, P. J., Turvey, S. E., et al. (2017). Modes of infant feeding and the risk of childhood asthma: a prospective birth cohort study. J. Pediatr. 190:e2, 192–199.e2. doi: 10.1016/j.jpeds.2017.07.012.29144244
Koch, M. A., Reiner, G. L., Lugo, K. A., Kreuk, L. S. M., Stanbery, A. G., Ansaldo, E., et al. (2016). Maternal IgG and IgA antibodies dampen mucosal T helper cell responses in early life. Cells 165, 827–841. doi: 10.1016/j.cell.2016.04.055
Kumar, A., das, S., Agrawal, A., Mukhopadhyay, I., and Ghosh, B. (2015). Genetic association of key Th1/Th2 pathway candidate genes, IRF2, IL6, IFNGR2, STAT4 and IL4RA, with atopic asthma in the Indian population. J. Hum. Genet. 60, 443–448. doi: 10.1038/jhg.2015.45
Kumbhare, S. V., Patangia, D. V., Patil, R. H., Shouche, Y. S., and Patil, N. P. (2019). Factors influencing the gut microbiome in children: from infancy to childhood. J. Biosci. 44, 1–19. doi: 10.1007/s12038-019-9860-z
Kurilshikov, A., Medina-Gomez, C., Bacigalupe, R., Radjabzadeh, D., Wang, J., Demirkan, A., et al. (2021). Large-scale association analyses identify host factors influencing human gut microbiome composition. Nat. Genet. 53, 156–165. doi: 10.1038/s41588-020-00763-1
Kuruvilla, M. E., Lee, F. E. H., and Lee, G. B. (2019). Understanding asthma phenotypes, Endotypes, and mechanisms of disease. Clin. Rev. Allergy Immunol. 56, 219–233. doi: 10.1007/s12016-018-8712-1
Kynyk, J. A., Mastronarde, J. G., and McCallister, J. W. (2011). Asthma, the sex difference. Curr. Opin. Pulm. Med. 17, 6–11. doi: 10.1097/MCP.0b013e3283410038
Laursen, M. F., Bahl, M. I., Michaelsen, K. F., and Licht, T. R. (2017). First foods and gut microbes. Front. Microbiol. 8, 1–8. doi: 10.3389/fmicb.2017.00356
Lee, N., and Kim, W. U. (2017). Microbiota in T-cell homeostasis and inflammatory diseases. Exp. Mol. Med. 49:e340. doi: 10.1038/emm.2017.36
Lee, J. J., Kim, S. H., Lee, M. J., Kim, B. K., Song, W. J., Park, H. W., et al. (2019). ‘Different upper airway microbiome and their functional genes associated with asthma in young adults and elderly individuals’, allergy: European. J. Allergy Clin. Immunol. 74, 709–719. doi: 10.1111/all.13608
Lee, S. A., Lim, J. Y., Kim, B. S., Cho, S. J., Kim, N. Y., Kim, O. B., et al. (2015). Comparison of the gut microbiota profile in breast-fed and formula-fed Korean infants using pyrosequencing. Nutr. Res. Pract. 9, 242–248. doi: 10.4162/nrp.2015.9.3.242
Leviatan, S., Shoer, S., Rothschild, D., Gorodetski, M., and Segal, E. (2022). An expanded reference map of the human gut microbiome reveals hundreds of previously unknown species. Nat. Commun. 13, 3863–3814. doi: 10.1038/s41467-022-31502-1
Li, R., Guo, Q., Zhao, J., Kang, W., Lu, R., Long, Z., et al. (2023). Assessing causal relationships between gut microbiota and asthma: evidence from two sample Mendelian randomization analysis. Front. Immunol. 14:684. doi: 10.3389/fimmu.2023.1148684
Li, R., Zheng, W. X., Zhang, Q. R., Song, Y., Liao, Y. T., Shi, F. C., et al. (2023). Longevity-associated Core gut microbiota mining and effect of mediated probiotic combinations on aging mice: case study of a long-lived population in Guangxi, China. Nutrients 15, 1–22. doi: 10.3390/nu15071609
Lim, M. Y., Hong, S., Kim, J. H., and Nam, Y. D. (2021). Association between gut microbiome and frailty in the older adult population in Korea. J. Gerontol. Ser. A Biol. Sci. Med. Sci. 76, 1362–1368. doi: 10.1093/gerona/glaa319
Lin, Y. C., Chen, Y. C., Kuo, C. H., Chang, Y. H., Huang, H. Y., Yeh, W. J., et al. (2020). Antibiotic exposure and asthma development in children with allergic rhinitis. J. Microbiol. Immunol. Infect. 53, 803–811. doi: 10.1016/j.jmii.2019.02.003
Lin, J., Zhang, Y., He, C., and Dai, J. (2018). Probiotics supplementation in children with asthma: a systematic review and meta-analysis. J. Paediatr. Child Health 54, 953–961. doi: 10.1111/jpc.14126
Liu, A. H. (2007). Hygiene theory and allergy and asthma prevention. Paediatr. Perinat. Epidemiol. 21, 2–7. doi: 10.1111/j.1365-3016.2007.00878.x
Liu, Q. H., Kan, X., Wang, Y. B., Liu, K. X., and Zeng, D. (2020). Differences in the clinical characteristics of early- and late-onset asthma in elderly patients. Biomed. Res. Int. 2020, 1–7. doi: 10.1155/2020/2940296
Liu, C., Makrinioti, H., Saglani, S., Bowman, M., Lin, L. L., Camargo, C. A., et al. (2022). Microbial dysbiosis and childhood asthma development: Integrated role of the airway and gut microbiome, environmental exposures, and host metabolism and immune response. Front. Immunol. 12, 1–9. doi: 10.3389/fimmu.2022.1028209
Lopera-Maya, E. A., Kurilshikov, A., van der Graaf, A., Hu, S., Andreu-Sánchez, S., Chen, L., et al. (2022). Effect of host genetics on the gut microbiome in 7,738 participants of the Dutch microbiome project. Nat. Genet. 54, 143–151. doi: 10.1038/s41588-021-00992-y
López-Otín, C., Blasco, M. A., Partridge, L., Serrano, M., and Kroemer, G. (2013). The hallmarks of aging Europe PMC funders group. Cells 153, 1194–1217. doi: 10.1016/j.cell.2013.05.039
Losol, P., Choi, J. P., Kim, S. H., and Chang, Y. S. (2021). The role of upper airway microbiome in the development of adult asthma. Immune Network 21, 1–18. doi: 10.4110/in.2021.21.e19
Lu, Y., Wang, Y., Wang, J., Lowe, A. J., Grzeskowiak, L. E., and Hu, Y. J. (2023). Early-life antibiotic exposure and childhood asthma trajectories: a National Population-Based Birth Cohort. Antibiotics 12, 1–15. doi: 10.3390/antibiotics12020314
Lupu, A., Jechel, E., Mihai, C. M., Mitrofan, E. C., Fotea, S., Starcea, I. M., et al. (2023). The footprint of microbiome in pediatric asthma-a complex puzzle for a balanced development. Nutrients 15, 1–18. doi: 10.3390/nu15143278
Lyons, K. E., Ryan, C. A., Dempsey, E. M., Ross, R. P., and Stanton, C. (2020). Breast milk, a source of beneficial microbes and associated benefits for infant health. Nutrients 12, 1–30. doi: 10.3390/nu12041039
Macpherson, A. J., De Agüero, M. G., and Ganal-Vonarburg, S. C. (2017). How nutrition and the maternal microbiota shape the neonatal immune system. Nat. Rev. Immunol. 17, 508–517. doi: 10.1038/nri.2017.58
Maestrelli, P., Henneberger, P. K., Tarlo, S., Mason, P., and Boschetto, P. (2020). Causes and phenotypes of work-related asthma. Int. J. Environ. Res. Public Health 17, 1–10. doi: 10.3390/ijerph17134713
Marra, F., Marra, C. A., Richardson, K., Lynd, L. D., Kozyrskyj, A., Patrick, D. M., et al. (2009). Antibiotic use in children is associated with increased risk of asthma. Pediatrics 123, 1003–1010. doi: 10.1542/peds.2008-1146
Marsland, B. J., Trompette, A., and Gollwitzer, E. S. (2015). The gut-lung axis in respiratory disease. Ann. Am. Thorac. Soc. 12, S150–S156. doi: 10.1513/AnnalsATS.201503-133AW
Martin, M. J., Zain, N. M. M., Hearson, G., Rivett, D. W., Koller, G., Wooldridge, D. J., et al. (2020). The airways microbiome of individuals with asthma treated with high and low doses of inhaled corticosteroids. PLoS One 15, e0244681–e0244614. doi: 10.1371/journal.pone.0244681
Martino, C., Dilmore, A. H., Burcham, Z. M., Metcalf, J. L., Jeste, D., and Knight, R. (2022). Microbiota succession throughout life from the cradle to the grave. Nat. Rev. Microbiol. 20, 707–720. doi: 10.1038/s41579-022-00768-z
Miliku, K., and Azad, M. B. (2018). Breastfeeding and the developmental origins of asthma: current evidence, possible mechanisms, and future research priorities. Nutrients 10, 1–15. doi: 10.3390/nu10080995
Mimoun, A., Delignat, S., Peyron, I., Daventure, V., Lecerf, M., Dimitrov, J. D., et al. (2020). Relevance of the Materno-fetal Interface for the induction of antigen-specific immune tolerance. Front. Immunol. 11, 1–16. doi: 10.3389/fimmu.2020.00810
Moore, L. E., Serrano-Lomelin, J., Rosychuk, R. J., Kozyrskyj, A. L., Chari, R., Crawford, S., et al. (2023). Perinatal and early life factors and asthma control among preschoolers: a population-based retrospective cohort study. BMJ Open Respir. Res. 10, e001928–e001929. doi: 10.1136/bmjresp-2023-001928
Moradzadeh, R., Mansournia, M. A., Baghfalaki, T., Nadrian, H., Gustafson, P., and McCandless, L. C. (2018). The impact of maternal smoking during pregnancy on childhood asthma: adjusted for exposure misclassification; results from the National Health and nutrition examination survey, 2011–2012. Ann. Epidemiol. 28, 697–703. doi: 10.1016/j.annepidem.2018.07.011
Munblit, D., Peroni, D., Boix-Amorós, A., Hsu, P., Land, B., Gay, M., et al. (2017). Human milk and allergic diseases: an unsolved puzzle. Nutrients 9, 1–43. doi: 10.3390/nu9080894
Munck, C., Helby, J., Westergaard, C. G., Porsbjerg, C., Backer, V., and Hansen, L. H. (2016). Smoking cessation and the microbiome in induced sputum samples from cigarette smoking asthma patients. PLoS One 11, e0158622–e0158611. doi: 10.1371/journal.pone.0158622
Nakata, K., Kobayashi, K., Ishikawa, Y., Yamamoto, M., Funada, Y., Kotani, Y., et al. (2010). The transfer of maternal antigen-specific IgG regulates the development of allergic airway inflammation early in life in an FcRn-dependent manner. Biochem. Biophys. Res. Commun. 395, 238–243. doi: 10.1016/j.bbrc.2010.03.170
Natalini, J. G., Singh, S., and Segal, L. N. (2023). The dynamic lung microbiome in health and disease. Nat. Rev. Microbiol. 21, 222–235. doi: 10.1038/s41579-022-00821-x
Ni, J., Friedman, H., Boyd, B. C., McGurn, A., Babinski, P., Markossian, T., et al. (2019). Early antibiotic exposure and development of asthma and allergic rhinitis in childhood. BMC Pediatr. 19, 225–228. doi: 10.1186/s12887-019-1594-4
Noverr, M. C., Noggle, R. M., Toews, G. B., and Huffnagle, G. B. (2004). Role of antibiotics and fungal microbiota in driving pulmonary allergic responses. Infect. Immun. 72, 4996–5003. doi: 10.1128/IAI.72.9.4996-5003.2004
Ntontsi, P., Photiades, A., Zervas, E., Xanthou, G., and Samitas, K. (2021). Genetics and epigenetics in asthma. Int. J. Mol. Sci. 22, 1–14. doi: 10.3390/ijms22052412
Nurmagambetov, T., Kuwahara, R., and Garbe, P. (2018). The economic burden of asthma in the United States, 2008-2013. Ann. Am. Thorac. Soc. 15, 348–356. doi: 10.1513/AnnalsATS.201703-259OC
Ojanguren, I., Satia, I., and Usmani, O. S. (2022). The role of viral infections on severe asthma exacerbations: present and future. Archivos de Bronconeumologia 58, 632–634. doi: 10.1016/j.arbres.2021.10.007
Pagtakhan, R. D., Bjelland, J. C., Landau, L. I., Loughlin, G., Kaltenborn, W., Seeley, G., et al. (1984). Sex differences in growth patterns of the airways and lung parenchyma in children. J. Appl. Physiol. Res. Environ. Exerc. Physiol. 56, 1204–1210. doi: 10.1152/jappl.1984.56.5.1204
Palma, G., Sorice, G. P., Genchi, V. A., Giordano, F., Caccioppoli, C., D’Oria, R., et al. (2022). Adipose tissue inflammation and pulmonary dysfunction in obesity. Int. J. Mol. Sci. 23, 10–12. doi: 10.3390/ijms23137349
Park, S., Choi, N. K., Kim, S., and Lee, C. H. (2018). The relationship between metabolic syndrome and asthma in the elderly. Sci. Rep. 8:9378. doi: 10.1038/s41598-018-26621-z
Park, J. E., Jardine, L., Gottgens, B., Teichmann, S. A., and Haniffa, M. (2020). Prenatal development of human immunity. Science 368, 600–603. doi: 10.1126/science.aaz9330
Park, S. H., Kim, K. A., Ahn, Y. T., Jeong, J. J., Huh, C. S., and Kim, D. H. (2015). Comparative analysis of gut microbiota in elderly people of urbanized towns and longevity villages. BMC Microbiol. 15:49. doi: 10.1186/s12866-015-0386-8
Park, H. K., Shin, J. W., Park, S. G., and Kim, W. (2014). Microbial communities in the upper respiratory tract of patients with asthma and chronic obstructive pulmonary disease. PLoS One 9:e109710. doi: 10.1371/journal.pone.0109710
Pärnänen, K. M. M., Hultman, J., Markkanen, M., Satokari, R., Rautava, S., Lamendella, R., et al. (2022). Early-life formula feeding is associated with infant gut microbiota alterations and an increased antibiotic resistance load. Am. J. Clin. Nutr. 115, 407–421. doi: 10.1093/ajcn/nqab353
Partridge, L., Deelen, J., and Slagboom, P. E. (2018). Facing up to the global challenges of ageing. Nature 561, 45–56. doi: 10.1038/s41586-018-0457-8
Patrick, D. M., Sbihi, H., Dai, D. L. Y., al Mamun, A., Rasali, D., Rose, C., et al. (2020). Decreasing antibiotic use, the gut microbiota, and asthma incidence in children: evidence from population-based and prospective cohort studies. Lancet Respir. Med. 8, 1094–1105. doi: 10.1016/S2213-2600(20)30052-7
Penders, J., Stobberingh, E. E., Thijs, C., Adams, H., Vink, C., van Ree, R., et al. (2006). Molecular fingerprinting of the intestinal microbiota of infants in whom atopic eczema was or was not developing. Clin. Exp. Allergy 36, 1602–1608. doi: 10.1111/j.1365-2222.2006.02599.x
Pendyala, S., Walker, J. M., and Holt, P. R. (2012). A high-fat diet is associated with endotoxemia that originates from the gut. Gastroenterology 142, 1100–1101.e2. doi: 10.1053/j.gastro.2012.01.034
Perez-Garcia, J., Herrera-Luis, E., Lorenzo-Diaz, F., González, M., Sardón, O., Villar, J., et al. (2020). Precision medicine in childhood asthma: Omic studies of treatment response. Int. J. Mol. Sci. 21, 1–15. doi: 10.3390/ijms21082908
Pfefferle, P. I., Keber, C. U., Cohen, R. M., and Garn, H. (2021). The hygiene hypothesis – learning from but not living in the past. Front. Immunol. 12, 1–6. doi: 10.3389/fimmu.2021.635935
Pfeiffer, S., Herzmann, C., Gaede, K. I., Kovacevic, D., Krauss-Etschmann, S., and Schloter, M. (2021). Different responses of the oral, nasal and lung microbiomes to cigarette smoke. Thorax 77, 191–195. doi: 10.1136/thoraxjnl-2020-216153
Pinot de Moira, A., Strandberg-Larsen, K., Bishop, T., Pedersen, M., Avraam, D., Cadman, T., et al. (2022). Associations of early-life pet ownership with asthma and allergic sensitization: a meta-analysis of more than 77,000 children from the EU child cohort network. J. Allergy Clin. Immunol. 150, 82–92. doi: 10.1016/j.jaci.2022.01.023
Pinto, R. A., Arredondo, S. M., Bono, M. R., Gaggero, A. A., and Díaz, P. V. (2006). T helper 1/T helper 2 cytokine imbalance in respiratory syncytial virus infection is associated with increased endogenous plasma cortisol. Pediatrics 117, e878–e886. doi: 10.1542/peds.2005-2119
Raita, Y., Pérez-Losada, M., Freishtat, R. J., Harmon, B., Mansbach, J. M., Piedra, P. A., et al. (2021). ‘Integrated omics endotyping of infants with respiratory syncytial virus bronchiolitis and risk of childhood asthma’, nature. Communications 12, 1–13. doi: 10.1038/s41467-021-23859-6
Ramakrishna, C., Kujawski, M., Chu, H., Li, L., Mazmanian, S. K., and Cantin, E. M. (2019). Bacteroides fragilis polysaccharide a induces IL-10 secreting B and T cells that prevent viral encephalitis. Nat. Commun. 10, 1–13. doi: 10.1038/s41467-019-09884-6
Rastogi, S., and Singh, A. (2022). Gut microbiome and human health: exploring how the probiotic genus Lactobacillus modulate immune responses. Front. Pharmacol. 13, 1–17. doi: 10.3389/fphar.2022.1042189
Reddel, H. K., Bacharier, L. B., Bateman, E. D., Brightling, C. E., Brusselle, G. G., Buhl, R., et al. (2022). Global initiative for asthma strategy 2021: executive summary and rationale for key changes. EurRespirJ 59:2102730. doi: 10.1183/13993003.02730-2021
Ren, Y., Ichinose, T., He, M., Youshida, S., Nishikawa, M., and Sun, G. (2019). Co-exposure to lipopolysaccharide and desert dust causes exacerbation of ovalbumin-induced allergic lung inflammation in mice via TLR4/MyD88-dependent and -independent pathways. Allergy Asthma Clin. Immunol. 15, 1–12. doi: 10.1186/s13223-019-0396-4
GINA Report (2022). Global strategy for asthma management and prevention. Available at: https://ginasthma.org/gina-reports/
Resztak, J. A., Choe, J., Nirmalan, S., Wei, J., Bruinsma, J., Houpt, R., et al. (2023). Analysis of transcriptional changes in the immune system associated with pubertal development in a longitudinal cohort of children with asthma. Nat. Commun. 14, 230–214. doi: 10.1038/s41467-022-35742-z
Rühlemann, M. C., Hermes, B. M., Bang, C., Doms, S., Moitinho-Silva, L., Thingholm, L. B., et al. (2021). Genome-wide association study in 8,956 German individuals identifies influence of ABO histo-blood groups on gut microbiome. Nat. Genet. 53, 147–155. doi: 10.1038/s41588-020-00747-1
Rutayisire, E., Huang, K., Liu, Y., and Tao, F. (2016). The mode of delivery affects the diversity and colonization pattern of the gut microbiota during the first year of infants’ life: a systematic review. BMC Gastroenterol. 16, 1–12. doi: 10.1186/s12876-016-0498-0
Salas Garcia, M. C., Yee, A. L., Gilbert, J. A., and Dsouza, M. (2018). Dysbiosis in children born by caesarean section. Ann. Nutr. Metab. 73, 24–32. doi: 10.1159/000492168
Santacroce, L., Charitos, I. A., Ballini, A., Inchingolo, F., Luperto, P., de Nitto, E., et al. (2020). The human respiratory system and its microbiome at a glimpse. Biology 9, 1–16. doi: 10.3390/biology9100318
Schroeder, B. O., Birchenough, G. M. H., Ståhlman, M., Arike, L., Johansson, M. E. V., Hansson, G. C., et al. (2018). Bifidobacteria or Fiber protects against diet-induced microbiota-mediated colonic mucus deterioration. Cell Host and Microbe 23, 27–40.e7. doi: 10.1016/j.chom.2017.11.004
Serrano-Villar, S., Vázquez-Castellanos, J. F., Vallejo, A., Latorre, A., Sainz, T., Ferrando-Martínez, S., et al. (2017). The effects of prebiotics on microbial dysbiosis, butyrate production and immunity in HIV-infected subjects. Mucosal Immunol. 10, 1279–1293. doi: 10.1038/mi.2016.122
Sharpe, R. A., Bearman, N., Thornton, C. R., Husk, K., and Osborne, N. J. (2015). Indoor fungal diversity and asthma: a meta-analysis and systematic review of risk factors. J. Allergy Clin. Immunol. 135, 110–122. doi: 10.1016/j.jaci.2014.07.002
Shin, J. H., Park, Y. H., Sim, M., Kim, S. A., Joung, H., and Shin, D. M. (2019). Serum level of sex steroid hormone is associated with diversity and profiles of human gut microbiome. Res. Microbiol. 170, 192–201. doi: 10.1016/j.resmic.2019.03.003
Shore, S. A. (2010). Obesity, airway hyperresponsiveness, and inflammation. J. Appl. Physiol. 108, 735–743. doi: 10.1152/japplphysiol.00749.2009
Simpson, J. L., Daly, J., Baines, K. J., Yang, I. A., Upham, J. W., Reynolds, P. N., et al. (2016). Airway dysbiosis: Haemophilus influenza and Tropheryma in poorly controlled asthma. Eur. Respir. J. 47, 792–800. doi: 10.1183/13993003.00405-2015
Singh, V., Lee, G. D., Son, H. W., Koh, H., Kim, E. S., Unno, T., et al. (2023). Butyrate producers, “the sentinel of gut”: their intestinal significance with and beyond butyrate, and prospective use as microbial therapeutics. Front. Microbiol. 13, 1–16. doi: 10.3389/fmicb.2022.1103836
Sisk-Hackworth, L., Kelley, S. T., and Thackray, V. G. (2023). Sex, puberty, and the gut microbiome. Soc. Reprod. Fertility 165, R61–R74. doi: 10.1530/REP-22-0303
Słabuszewska-Jóźwiak, A., Szymański, J. K., Ciebiera, M., Sarecka-Hujar, B., and Jakiel, G. (2020). Pediatrics consequences of caesarean section—a systematic review and meta-analysis. Int. J. Environ. Res. Public Health 17, 1–17. doi: 10.3390/ijerph17218031
Song, W. J., Sintobin, I., Sohn, K. H., Kang, M. G., Park, H. K., Jo, E. J., et al. (2016). Staphylococcal enterotoxin IgE sensitization in late-onset severe eosinophilic asthma in the elderly. Clin. Exp. Allergy 46, 411–421. doi: 10.1111/cea.12652
Song, S. J., Wang, J., Martino, C., Jiang, L., Thompson, W. K., Shenhav, L., et al. (2021). Naturalization of the microbiota developmental trajectory of cesarean-born neonates after vaginal seeding. Med 2, 951–964.e5. doi: 10.1016/j.medj.2021.05.003
Stein, M., Hrusch, C. L., Gozdz, J., Igartua, C., Pivniouk, V., Murray, S. E., et al. (2016). Innate immunity and asthma risk in amish and hutterite farm children. N. Engl. J. Med. 375, 411–421. doi: 10.1056/nejmoa1508749
Stiemsma, L. T., and Michels, K. B. (2017). The role of the microbiome in the developmental origins of health and disease. Pediatrics 141:e20172437
Stokholm, J., Blaser, M. J., Thorsen, J., Rasmussen, M. A., Waage, J., Vinding, R. K., et al. (2018). ‘Maturation of the gut microbiome and risk of asthma in childhood’, nature. Communications 9, 1–10. doi: 10.1038/s41467-017-02573-2
Suganya, K., and Koo, B. S. (2020). Gut–brain axis: role of gut microbiota on neurological disorders and how probiotics/prebiotics beneficially modulate microbial and immune pathways to improve brain functions. Int. J. Mol. Sci. 21, 1–29. doi: 10.3390/ijms21207551
Takagi, T., Naito, Y., Inoue, R., Kashiwagi, S., Uchiyama, K., Mizushima, K., et al. (2019). Differences in gut microbiota associated with age, sex, and stool consistency in healthy Japanese subjects. J. Gastroenterol. 54, 53–63. doi: 10.1007/s00535-018-1488-5
Tan, D. J., Walters, E. H., Perret, J. L., Lodge, C. J., Lowe, A. J., Matheson, M. C., et al. (2015). Age-of-asthma onset as a determinant of different asthma phenotypes in adults: a systematic review and meta-analysis of the literature. Expert Rev. Respir. Med. 9, 109–123. doi: 10.1586/17476348.2015.1000311
Taylor, S. L., Leong, L. E. X., Choo, J. M., Wesselingh, S., Yang, I. A., Upham, J. W., et al. (2018). Inflammatory phenotypes in patients with severe asthma are associated with distinct airway microbiology. J. Allergy Clin. Immunol. 141, 94–103.e15. doi: 10.1016/j.jaci.2017.03.044
Teo, S. M., Mok, D., Pham, K., Kusel, M., Serralha, M., Troy, N., et al. (2015). The infant nasopharyngeal microbiome impacts severity of lower respiratory infection and risk of asthma development. Cell Host Microbe 17, 704–715. doi: 10.1016/j.chom.2015.03.008
Thorsen, J., Stokholm, J., Rasmussen, M. A., Mortensen, M. S., Brejnrod, A. D., Hjelmsø, M., et al. (2021). The airway microbiota modulates effect of azithromycin treatment for episodes of recurrent asthma-like symptoms in preschool children a randomized clinical trial. Am. J. Respir. Crit. Care Med. 204, 149–158. doi: 10.1164/rccm.202008-3226OC
To, T., Stanojevic, S., Moores, G., Gershon, A. S., Bateman, E. D., Cruz, A. A., et al. (2012). Global asthma prevalence in adults: findings from the cross-sectional world health survey. BMC Public Health 12:204. doi: 10.1186/1471-2458-12-204
Toivonen, L., Schuez-Havupalo, L., Karppinen, S., Waris, M., Hoffman, K. L., Camargo, C. A., et al. (2021). Antibiotic treatments during infancy, changes in nasal microbiota, and asthma development: population-based cohort study. Clin. Infect. Dis. 72, 1546–1554. doi: 10.1093/cid/ciaa262
Tomoda, K., Kubo, K., Asahara, T., Andoh, A., Nomoto, K., Nishii, Y., et al. (2011). Cigarette smoke decreases organic acids levels and population of bifidobacterium in the caecum of rats. J. Toxicol. Sci. 36, 261–266. doi: 10.2131/jts.36.261
Troisi, R. J., Speizer, F. E., Willett, W. C., Trichopoulos, D., and Rosner, B. (1995). Menopause, postmenopausal estrogen preparations, and the risk of adult-onset asthma. Am. J. Respir. Crit. Care Med. 152, 1183–1188. doi: 10.1164/ajrccm.152.4.7551368
Troy, E. B., and Kasper, D. L. (2010). Beneficial effects of Bacteroides fragilis polysaccharides on the immune system. Front. Biosci. 15, 25–34. doi: 10.2741/3603
Tsukuda, N., Yahagi, K., Hara, T., Watanabe, Y., Matsumoto, H., Mori, H., et al. (2021). Key bacterial taxa and metabolic pathways affecting gut short-chain fatty acid profiles in early life. ISME J. 15, 2574–2590. doi: 10.1038/s41396-021-00937-7
Turunen, J., Tejesvi, M. V., Paalanne, N., Pokka, T., Amatya, S. B., Mishra, S., et al. (2023). Investigating prenatal and perinatal factors on meconium microbiota: a systematic review and cohort study. Pediatr. Res. 14, 1–11. doi: 10.1038/s41390-023-02783-z
Ucciferri, C. C., and Dunn, S. E. (2022). ‘Effect of puberty on the immune system: relevance to multiple sclerosis’, Frontiers. Pediatrics 10, 1–20. doi: 10.3389/fped.2022.1059083
van de Loo, K., van Gelder, M., Roukema, J., Roeleveld, N., Merkus, P. J., and Verhaak, C. M. (2016). Prenatal maternal psychological stress and childhood asthma and wheezing: a meta-analysis. Eur. Respir. J. 47, 133–146. doi: 10.1183/13993003.00299-2015
van den Munckhof, E. H. A., Hafkamp, H. C., de Kluijver, J., Kuijper, E. J., de Koning, M. N. C., Quint, W. G. V., et al. (2020). Nasal microbiota dominated by Moraxella s is associated with respiratory health in the elderly population: a case control study. Respir. Res. 21, 181–189. doi: 10.1186/s12931-020-01443-8
Ver Heul, A., Planer, J., and Kau, A. (2019). The human microbiota and asthma. Clin. Rev. Allergy Immunol. 57, 350–363. doi: 10.1007/s12016-018-8719-7
Voraphani, N., Stern, D. A., Wright, A. L., Guerra, S., Morgan, W. J., and Martinez, F. D. (2014). Risk of current asthma among adult smokers with respiratory syncytial virus illnesses in early life. Am. J. Respir. Crit. Care Med. 190, 392–398. doi: 10.1164/rccm.201311-2095OC
Wang, W., Li, Y., Han, G., Li, A., and Kong, X. (2022). Lactobacillus fermentum CECT5716 alleviates the inflammatory response in asthma by regulating TLR2/TLR4 expression. Front. Nutr. 9, 1–12. doi: 10.3389/fnut.2022.931427
Wang, Q., Li, F., Liang, B., Liang, Y., Chen, S., Mo, X., et al. (2018). A metagenome-wide association study of gut microbiota in asthma in UK adults. BMC Microbiol. 18, 114–117. doi: 10.1186/s12866-018-1257-x
Wang, J., Li, F., and Tian, Z. (2017). Role of microbiota on lung homeostasis and diseases. Sci. China Life Sci. 60, 1407–1415. doi: 10.1007/s11427-017-9151-1
Wood, L. G., Garg, M. L., and Gibson, P. G. (2011). A high-fat challenge increases airway inflammation and impairs bronchodilator recovery in asthma. J. Allergy Clin. Immunol. 127, 1133–1140. doi: 10.1016/j.jaci.2011.01.036
Wood, L. G., Shivappa, N., Berthon, B. S., Gibson, P. G., and Hebert, J. R. (2015). Dietary inflammatory index is related to asthma risk, lung function and systemic inflammation in asthma. Clin. Exp. Allergy 45, 177–183. doi: 10.1111/cea.12323
Wu, Z., Mehrabi Nasab, E., Arora, P., and Athari, S. S. (2022). Study effect of probiotics and prebiotics on treatment of OVA-LPS-induced of allergic asthma inflammation and pneumonia by regulating the TLR4/NF-kB signaling pathway. J. Transl. Med. 20, 130–114. doi: 10.1186/s12967-022-03337-3
Wu, Y. L., Xu, J., Rong, X. Y., Wang, F., Wang, H. J., and Zhao, C. (2021). Gut microbiota alterations and health status in aging adults: from correlation to causation. Aging Med. 4, 206–213. doi: 10.1002/agm2.12167
Yagi, K., Huffnagle, G. B., Lukacs, N. W., and Asai, N. (2021). The lung microbiome during health and disease. Int. J. Mol. Sci. 22, 1–13. doi: 10.3390/ijms221910872
Yáñez, A., Soria, M., de Barayazarra, S., Recuero, N., Rovira, F., Jares, E., et al. (2018). Clinical characteristics and comorbidities of elderly asthmatics who attend allergy clinics. Asthma Res. Pract. 4, 1–7. doi: 10.1186/s40733-018-0041-x
Yao, Y., Cai, X., Ye, Y., Wang, F., Chen, F., and Zheng, C. (2021). The role of microbiota in infant health: from early life to adulthood. Front. Immunol. 12, 1–21. doi: 10.3389/fimmu.2021.708472
Yap, G. C., Loo, E. X., Aw, M., Lu, Q., Shek, L. P., and Lee, B. W. (2014). Molecular analysis of infant fecal microbiota in an Asian at-risk cohort-correlates with infant and childhood eczema. BMC. Res. Notes 7, 1–6. doi: 10.1186/1756-0500-7-166
Yatsunenko, T., Rey, F. E., Manary, M. J., Trehan, I., Dominguez-Bello, M. G., Contreras, M., et al. (2012). Human gut microbiome viewed across age and geography. Nature 486, 222–227. doi: 10.1038/nature11053
Yuan, X., Chen, R., Zhang, Y., Lin, X., and Yang, X. (2020). Sexual dimorphism of gut microbiota at different pubertal status. Microb. Cell Factories 19, 152–116. doi: 10.1186/s12934-020-01412-2
Zeldin, J., Chaudhary, P. P., Spathies, J., Yadav, M., D’Souza, B. N., Alishahedani, M. E., et al. (2023). Exposure to isocyanates predicts atopic dermatitis prevalence and disrupts therapeutic pathways in commensal bacteria. Sci. Adv. 9, eade8898–eade8820. doi: 10.1126/sciadv.ade8898
Zhang, J., He, M., Yu, Q., Xiao, F., Zhang, Y., and Liang, C. (2023). The effects of a healthy diet on asthma and wheezing in children and adolescents: a systematic review and Meta-analysis. J. Asthma Aller. 6, 1007–1024. doi: 10.2147/JAA.S423884
Zhang, Y., and Huang, L. (2021). Characteristics of older adult hospitalized patients with bronchial asthma: a retrospective study. Allergy Asthma Clin. Immunol. 17, 122–127. doi: 10.1186/s13223-021-00628-0
Zhang, J., Ma, J.-y., Li, Q.-h., Su, H., and Sun, X. (2018a). Lactobacillus rhamnosus GG induced protective effect on allergic airway inflammation is associated with gut microbiota. Cell. Immunol. 332, 77–84. doi: 10.1016/j.cellimm.2018.08.002
Zhang, J., Ma, C., Yang, A., Zhang, R., Gong, J., and Mo, F. (2018b). Is preterm birth associated with asthma among children from birth to 17 years old? -a study based on 2011-2012 US National Survey of Children’s health. Ital. J. Pediatr. 44, 151–159. doi: 10.1186/s13052-018-0583-9
Zhang, Z., Shi, L., Pang, W., Liu, W., Li, J., Wang, H., et al. (2016). Dietary fiber intake regulates intestinal microflora and inhibits ovalbumin-induced allergic airway inflammation in a mouse model. PLoS One 11, 1–16. doi: 10.1371/journal.pone.0147778
Zhao, X., Hu, M., Zhou, H., Yang, Y., Shen, S., You, Y., et al. (2023). The role of gut microbiome in the complex relationship between respiratory tract infection and asthma. Front. Microbiol. 14, 1–13. doi: 10.3389/fmicb.2023.1219942
Zheng, D., Liwinski, T., and Elinav, E. (2020). Interaction between microbiota and immunity in health and disease. Cell Res. 30, 492–506. doi: 10.1038/s41422-020-0332-7
Zheng, P., Zhang, K., Lv, X., Liu, C., Wang, Q., and Bai, X. (2022). Gut microbiome and metabolomics profiles of allergic and non-allergic childhood asthma. J. Asthma Aller. 15, 419–435. doi: 10.2147/JAA.S354870
Zhou, Y., Jackson, D., Bacharier, L. B., Mauger, D., Boushey, H., Castro, M., et al. (2019). The upper-airway microbiota and loss of asthma control among asthmatic children. Nat. Commun. 10, 5714–5710. doi: 10.1038/s41467-019-13698-x
Zimmermann, P., Messina, N., Mohn, W. W., Finlay, B. B., and Curtis, N. (2019). Association between the intestinal microbiota and allergic sensitization, eczema, and asthma: a systematic review. J. Allergy Clin. Immunol. 143, 467–485. doi: 10.1016/j.jaci.2018.09.025
Keywords: asthma, microbiota, exacerbations, gut-lung axis, diversity, environmental factors, asthma phenotypes
Citation: Galeana-Cadena D, Gómez-García IA, Lopez-Salinas KG, Irineo-Moreno V, Jiménez-Juárez F, Tapia-García AR, Boyzo-Cortes CA, Matías-Martínez MB, Jiménez-Alvarez L, Zúñiga J and Camarena A (2023) Winds of change a tale of: asthma and microbiome. Front. Microbiol. 14:1295215. doi: 10.3389/fmicb.2023.1295215
Edited by:
Chun-Hsing Liao, National Yang Ming Chiao Tung University, TaiwanReviewed by:
Yen-Chu Huang, Taichung Veterans General Hospital, TaiwanNaser Alsharairi, Griffith University, Australia
Wendy Fonseca, University of Michigan, United States
Copyright © 2023 Galeana-Cadena, Gómez-García, Lopez-Salinas, Irineo-Moreno, Jiménez-Juárez, Tapia-García, Boyzo-Cortes, Matías-Martínez, Jiménez-Alvarez, Zúñiga and Camarena. This is an open-access article distributed under the terms of the Creative Commons Attribution License (CC BY). The use, distribution or reproduction in other forums is permitted, provided the original author(s) and the copyright owner(s) are credited and that the original publication in this journal is cited, in accordance with accepted academic practice. No use, distribution or reproduction is permitted which does not comply with these terms.
*Correspondence: Joaquín Zúñiga, joazu@yahoo.com; Angel Camarena, ang_edco@yahoo.com.mx