- 1Department for Sustainability, Biotechnologies and Agroindustry Division, Italian National Agency for New Technologies, Energy and Sustainable Economic Development (ENEA), Rome, Italy
- 2Department of Agriculture and Forest Sciences, University of Tuscia, Viterbo, Italy
- 3I.L.P.A. Group, Valsamoggia, Italy
The prevalence of biofilm-associated microorganisms and the increasing use of ready-to-eat fresh products represent the current duality the food industry must address. Innovative and eco-friendly antibiofilm solutions and appropriate microbiological food control systems are urgently needed to improve food quality and safety. This study aimed to investigate the in vitro combined efficacy of carvacrol with a pre-formed biofilm monolayer of the probiotic Lactiplantibacillus plantarum DSM 20174. The antimicrobial activity of carvacrol against both planktonic and sessile cells of foodborne pathogens and spoilage microorganisms, alone or in the presence of the pre-formed biofilm of L. plantarum, was investigated by culture-based methods along with flow cytometry (FCM) to monitor cells' cultivability and viability. The synergistic action of carvacrol and the pre-formed biofilm of L. plantarum was evaluated in the 96-well plates. The results showed that L. plantarum pre-formed biofilm monolayer enhanced the antimicrobial effect of carvacrol determining a bactericidal action while the treatment alone induced the viable but not culturable (VBNC) cell state only. Furthermore, the great efficacy of the combined treatment allowed the application of a lower concentration of carvacrol (100 ppm) to achieve significant damage in cell viability. In conclusion, the incorporation of carvacrol into the L. plantarum pre-formed biofilm represents a promising alternative for an antimicrobial functionalized ready-to-eat packaging.
1 Introduction
The increasing focus on packaged and ready-to-eat fresh products has heightened the risks associated with the prevalence of biofilm-forming microorganisms and the limited effectiveness of current antimicrobial compounds, resulting in the urgent need to develop innovative and eco-friendly antibiofilm agents and appropriate food microbiological monitoring and control systems, combining concepts of quality, safety, sustainability, and consumer acceptability (Bondi et al., 2014; Di Carli et al., 2015; Tseng et al., 2022; Khodaei et al., 2023). Biofilms are formed by micro-structured communities of microorganisms embedded in a self-produced adhesive and protective extracellular polymeric substance (EPS), showing variations in growth rate and gene expression compared with their planktonic form (Asare et al., 2022). There is ample evidence from the scientific literature that bacteria in the biofilm phenotype are more resistant to antimicrobial agents than their planktonic counterparts due to the EPS matrix cell protection (Natan and Banin, 2017; Fulaz et al., 2019; Asare et al., 2022). In the food industry, microorganisms can adhere to packaging surfaces, form a biofilm, and compromise the shelf-life of food products (Galié et al., 2018; Carrascosa et al., 2021). In addition, studies show that bacteria in a biofilm state are more likely disposed to enter in the “viable but non-culturable” (VBNC) condition, where the cells are unable to grow and replicate on standard solid culture media, eluding detection by using conventional microbial culture-based techniques (Li and Zhao, 2020; Qi et al., 2022; Zhang et al., 2023). In this scenario, flow cytometry (FCM) emerges as a real-time technique for identifying the culture-uninvestigable cellular physiological state. The use of selected probes able to monitor several cellular parameters (i.e., membrane permeability, metabolic activity, and DNA/RNA content) enables obtaining a deeper knowledge of the mechanisms behind microbial inactivation and related subpopulation viability state response on both planktonic and sessile cells (March-Rosselló, 2017; Arioli et al., 2019; Truchado et al., 2020; Zand et al., 2021). Among new technological approaches in food packaging, the application of natural compounds as essential oils (EOs) and their components have been increasing in the last few years, to guarantee safety and preserve nutritional and organoleptic characteristics of food, in line with the “green and aware consumer” demand (Ojogbo et al., 2020; Abers et al., 2021; Cano et al., 2021; Angane et al., 2022). Particularly, essential oils of different Origanum species have exhibited antimicrobial effects against several bacteria, such as Escherichia coli, Pseudomonas spp., Staphylococcus aureus, and Salmonella spp. and fungi, including Candida albicans, and Aspergillus spp. Their efficacy can be attributed to the synergy of compounds such as carvacrol, thymol, and γ-terpinene, as well as cis- and trans-sabinene hydrate, with effective antimicrobial activity (Chouhan et al., 2017; Fikry et al., 2019; Angane et al., 2022). Biofilm control strategies are increasingly focused on the utilization of natural compounds and, particularly, carvacrol, for their capacity to selectively target the biofilm signaling pathways that regulate quorum sensing (QS), extracellular polymeric substance (EPS) synthesis, biofilm-related gene expression, microbial motility, adhesion, and dispersion. These findings are encouraging as they present a cost-effective, naturally derived anti-biofilm agent without inducing harmful side effects (Burt et al., 2014; Asma et al., 2022; Lu et al., 2022). Carvacrol, cymophenol (2-methyl-5-propan-2-yl-phenol), is a monoterpene phenolic compound with antimicrobial, antioxidant, anti-inflammatory, anti-cancer, antipyretic, and analgesic properties (Asadi et al., 2023). Therefore, carvacrol has received significant attention from researchers for food applications, notably due to its antibacterial and antibiofilm activities against a broad spectrum of food pathogenic and spoilage strains (Hyldgaard et al., 2012; Hajibonabi et al., 2023). These effects are attributed to the ability of carvacrol to impact the structural and functional characteristics of cytoplasmic membranes. It can expand and destabilize the outer membrane of bacterial strains, thereby increasing their fluidity and cytoplasmic permeability (Lambert et al., 2001; Burt, 2004; Chouhan et al., 2017).
Moreover, in the studies by Wijesundara et al. (2022), it was found that carvacrol induced the deactivation of extracellular polymeric substances (EPS), which were responsible for protecting the bacterial cell against toxic substances and ensuring its strength. Fang et al. (2019) and Luna et al. (2022) determined that the MIC values for carvacrol against Pseudomonas fluorescens ATCC 13525 and E. coli ATCC 25922 were 0.5 and 0.225 mg/ml, respectively, while Churklam et al. (2020) investigated carvacrol antibacterial activity against Listeria monocytogenes strains, including food isolates, with an MIC value of 250 μg/ml. Despite the significant antimicrobial properties of EOs and their compounds, they are not widely used as antimicrobials in the food industry because of their volatility, intense aromas, low solubility, and high susceptibility to oxidation (Hyldgaard et al., 2012; Bakry et al., 2016). Different techniques (e.g., encapsulation, spay drying, and solid lipid nanoparticles) can be used to counterbalance the limitations, ameliorating their biological activity and allowing a controlled release (Ribeiro-Santos et al., 2017; Cacciatore et al., 2022; Mo et al., 2022; Mukurumbira et al., 2022). A feasible approach to reducing this natural compound concentration could be the combination with other natural solutions. Lactic acid bacteria (LAB) represent one of the promising possibilities for the natural control of biofilms that are composed of high antimicrobial resistance and associated risks for foodborne disease spread (Turgis et al., 2012; Esposito and Turku, 2023). LAB can naturally produce antimicrobial compounds, such as organic acids (mainly, lactic acid and acetic acid), diacetyl, hydrogen peroxide, and bacteriocins such as nisin and natamycin, generally recognized as safe (GRAS) (Raman et al., 2022). Organic acid syntheses, such as lactic acid and acetic acid, are mainly responsible for their antagonistic activity against pathogens by acidifying intracellular pH and generating an unfavorable local microenvironment for pathogenic bacteria (Vieco-Saiz et al., 2019; Simons et al., 2020; Martín et al., 2022). In addition, due to its hydrophobic characteristic, the undissolved form of lactic acid and acetic acid enters the cell by passing through the cell membrane, causing alteration, death, and metabolic functions of pathogenic microorganisms (Surendran Nair et al., 2017; Sharma et al., 2022; Mgomi et al., 2023). It has been demonstrated that concentrations of 0.5% (v/v) lactic acid could completely disrupt the growth of pathogenic microorganisms, such as E. coli, L. monocytogenes, or Salmonella spp. (Wang et al., 2015). The reduction in pH due to the synthesis of LAB organic acids promotes the hydrophobicity of carvacrol, allowing it to explain the bactericidal activity (de Carvalho et al., 2018; Di Gregorio et al., 2022). However, few studies have focused on the synergistic effect of lactic acid bacteria and EOs or their components, showing that the combined treatment may have better antimicrobial activity than single ones and lower concentrations could also be utilized to minimize unwanted side effects (Govaris et al., 2010; Sharma et al., 2022; Esposito and Turku, 2023). Moreover, studies investigating the possibility of using a combination of a pre-formed LAB biofilm monolayer with sub-inhibitory concentrations of natural compounds to enhance whole antimicrobial action are very limited. The synergistic action of LAB monolayer biofilm and carvacrol can be investigated for prospective encapsulation in functional packaging, enabling biofilm formation inhibition and specific health and food biopreservation advantages (Hellebois et al., 2020; Ajlouni et al., 2021). Furthermore, an important aspect to highlight is that the development and application of highly effective natural anti-biofilm treatments for biofilm-related issues in food products and packaging are crucial, given the increasing microbial resistance to antibiotics (Burt, 2004; Asma et al., 2022). This is particularly noteworthy as it has been demonstrated that carvacrol can inhibit microbial growth and biofilm formation without promoting antimicrobial resistance (da Silva et al., 2023). Innovative approaches to assess the culturability and viability of food pathogens in complex matrices are needed to optimize the protocol used for food production and evaluate the effectiveness of preservation treatments (Fleischmann et al., 2021; Rubbens and Props, 2021). It is well-known that using traditional, culture-based microbiological approaches can lead to an overestimation of treatment efficacy (Di Gregorio et al., 2022). Cultivable methods coupled with FCM represent an important strategy for the study of antimicrobial treatment efficacy on both sessile and planktonic cells, providing a deeper understanding of the physiological state of microbial cells. In the present study, we aimed to exploit the in vitro bactericidal effect of carvacrol (100 and 250 ppm) enhanced by a pre-formed biofilm monolayer of Lactiplantibacillus plantarum against collection strains of foodborne pathogens (E. coli and L. monocytogenes) and spoilage microorganisms (P. fluorescens). The effect of LAB monolayer biofilm and carvacrol was evaluated by using a culture-based approach coupled with flow cytometry (FCM) for monitoring both cultivability and viability of the planktonic and sessile cells. Evaluating the antimicrobial action of bioactive compounds and microbial biofilm's inactivation and degradation activity is essential for developing innovative packaging.
2 Materials and methods
2.1 Bacterial strains and inoculum preparation
This study used four biofilm-forming strains, i.e., the foodborne pathogens E. coli ATCC 25922 and L. monocytogenes 56 LY, the food spoilage P. fluorescens ATCC 13525, and the probiotic microorganism L. plantarum subsp. plantarum DSM 20174. A loop of each microbial strain was picked up from glycerol stocks stored at −80°C and grown in 5 ml of culture medium in static condition as follows: E. coli and L. monocytogenes were grown overnight (o/n) at 37°C in brain heart infusion (BHI) broth (Merck KGaA, Darmstadt, Germany); P. fluorescens was grown o/n at 28°C in tryptone soy broth (TSB) (Merck KGaA, Darmstadt, Germany) at 180 rpm, and L. plantarum was grown o/n at 30°C in de Man, Rogosa, and Sharpe (MRS) broth (Merck KGaA, Darmstadt, Germany). Then, an aliquot of 1 ml was transferred to 9 ml of fresh medium. After o/n incubation, 1 ml was transferred to 30 ml of fresh sterile medium. Starting from the refreshed culture, the initial concentration of 6 Log CFU/ml (Colony Forming Unit: CFU) for each microorganism was applied for subsequent analysis (Di Gregorio et al., 2022).
2.2 Carvacrol solution
Carvacrol, with a purity of ≥98.5%, was purchased from Sigma–Aldrich (St. Louis, MO, United States, ref. W224511) and dissolved in ethanol (absolute, ≥99.8%) to reduce the hydrophobic characteristic. From the 100% stock, an intermediate 10% (v/v) was filtered through a 0.2-mm pore-size filter (Millipore, Bedford, MA, USA) to achieve the final tested concentrations of 50, 100, 250, and 500 ppm. Ethanol alone was used as control for each tested condition (Arioli et al., 2019).
2.3 Experimental workflow
The effect of carvacrol, at the concentrations of 50, 100, 250, and 500 ppm, was tested against L. plantarum to estimate the probiotic tolerance vs. its planktonic and sessile fraction. Then, the antimicrobial effect of carvacrol (50, 100, 250, and 500 ppm) was assessed against planktonic and biofilm fractions of E. coli, P. fluorescens, and L. monocytogenes. Subsequently, the combined treatment with a pre-formed biofilm monolayer of the probiotic L. plantarum was assessed at the carvacrol concentrations of 100 and 250 ppm. Planktonic and sessile fractions were enumerated through culturability and viability analyses for all tested conditions. Culturability was investigated by using the standard plate count method, and the results were elaborated as logarithmic reduction values (Log CFU/ml) (see Section 2.4). Turbidity and crystal violet assays allowed us to evaluate the antimicrobial efficacy on culturable cells expressed as the percentage of inhibition and calculated as described by Bazargani and Rohloff (2016). The flow cytometry viability results were expressed as percentage values of the internal cell subpopulations, which were differentiated by membrane integrity (see Section 2.6). Three biological replicates from three independent experiments were considered for each tested condition.
2.4 Biofilm formation
An aliquot of bacterial suspension (0.05 ml) at the concentration of 6 Log cells/ml was added to sterile tubes containing 4.9 ml of sterile growth medium (BHI for E. coli and L. monocytogenes, TSB for P. fluorescens, and MRS for L. plantarum) and 0.05 ml of carvacrol solution at the final concentrations of 50, 100, 250, and 500 ppm. The experiment was performed in triplicate with a rapid microplate methodology using 96-well plates (Corning Costar 96-well, flat-bottom microplate) (Bragonzi et al., 2012). The four plates, one for each microorganism, were incubated overnight in static condition at 37°C for E. coli and L. monocytogenes, 28°C for P. fluorescens, and 30°C for L. plantarum. Uninoculated controls were present in each plate. After 24 h of incubation, the planktonic cell fractions were transferred to new microtiter plates (see below) while the attached cells were rinsed three times with 0.2 ml of phosphate-buffered saline (PBS, pH 7.4) to remove non-adherent and weakly adherent bacteria.
2.4.1 CV assay
The sessile cells were quantified using the crystal violet assay as described in the study by Bragonzi et al. (2012). In brief, plates were air-dried for 30 min at room temperature, and biofilms were stained with 0.2 ml of 1% (w/v) solution of crystal violet (CV) in water. After 20 min of staining at room temperature, the samples were washed thrice by dipping each sample in 200 μl of sterile PBS. The bound dye was dissolved by adding 0.2 ml of 95% (v/v) ethanol to de-stain the samples. The quantitative analysis of biofilm production was performed by measuring the absorbance at 595 nm with the Promega™ GloMax® automated reader. To compensate for background absorbance, OD readings from sterile medium, dye, and ethanol were averaged and subtracted from all test values.
2.4.2 Planktonic fraction
To correlate biofilm formation with the growth of planktonic cells in each well, the planktonic cell fractions, previously transferred to new microtiter plates as described above, were quantified by plating 0.1 ml of 10-fold bacterial suspension serial dilutions (NaCl 0.9% w/v) on BHI (for the enumeration of E. coli and L. monocytogenes), TSB (for the enumeration of P. fluorescens), and MRS (for the enumeration of L. plantarum) agar plates, following the procedure reported by Bragonzi et al. (2012). After 24 h of incubation at 37, 28, and 30°C, for E. coli, L. monocytogenes, P. fluorescens, and L. plantarum, respectively, colonies were counted. For the turbidity assay, microtiter plates were measured (OD 595 nm) by using a microplate automated reader (Promega™ GloMax®). The viability of planktonic cells was investigated through a flow cytometry approach (see Section 2.6).
2.4.3 Sessile fraction
To enumerate the sessile (adherent) cells of tested bacteria, the wells, after removing the planktonic fraction, were rinsed three times with 200 ml of PBS to remove non-adherent and weakly adherent bacteria. Then, the biofilm was removed by scraping the surface of each well with 1 ml of PBS, and the recovered cells were suspended by vortexing for 30 s. The number of sessile cells was determined by plating appropriate dilutions of biofilm samples on agar media, as described above. The viability of sessile cells was investigated through a flow cytometry approach (see Section 2.6).
2.5 L. plantarum pre-formed biofilm
The combined effect of carvacrol at concentrations of 100 and 250 ppm, with a pre-formed biofilm of L. plantarum, was investigated on E. coli, L. monocytogenes, and P. fluorescens. First, an aliquot of 0.2 ml of untreated L. plantarum (6 Log CFU/ml) was inoculated into 96-well plates, one for each microorganism, to avoid contaminations. After 24 h of incubation at 30°C, the L. plantarum planktonic fraction was removed, and each well was rinsed three times with 0.2 ml PBS to take off non-adherent and weakly adherent cells. In total, 0.2 ml of each bacterial strain (E. coli, L. monocytogenes, and P. fluorescens) at the concentration of 6 Log CFU/ml was added to the L. plantarum pre-formed biofilm wells. The experimental conditions for each microorganism were set up as follows:
i. L. plantarum preformed biofilm + microorganism (L.p.).
ii. L. plantarum preformed biofilm + carvacrol 100 ppm + microorganism (L.p.+100 ppm).
iii. L. plantarum preformed biofilm + carvacrol 250 ppm + microorganism (L.p.+250 ppm).
As a control, 0 ppm samples and the single treatments with carvacrol 100 ppm and carvacrol 250 ppm for each strain were used. Autoclaved bacterial suspension and uninoculated fresh sterile medium were added to each 96-well plate as a negative control. After 24 h of incubation, planktonic and sessile cells were analyzed to investigate the combined effect of L. plantarum pre-formed biofilm with carvacrol (see Section 2.4). Specifically, for the standard plate count method, samples obtained from the L. plantarum pre-formed biofilm test were also plated on MRS agar to subtract the CFU of L. plantarum from the whole colonies observed. The viability of cells was assessed by flow cytometry as described in Section 2.6.
2.6 Flow cytometry assay
E. coli, L. monocytogenes, P. fluorescens, and L. plantarum samples were diluted 100-fold in PBS, and a double staining SYTO24 (1x, ThermoFisher, USA)/Propidium Iodide (PI) (10 μg/ml) was performed. The stained cell suspensions were distributed in triplicate in 96-well plates and incubated for 15 min at 37°C before testing. Each sample utilized microspheres of 2.5 μm in diameter (Alignflow™ for Blue Lasers, Thermo Fisher Scientific Life Science Solutions, Milan, Italy) as internal reference standards. CytoFLEX S flow analyzer (Beckman Coulter, Flow Cytometry, Milan, Italy) was used by applying FSC 10,000 threshold settings. Each sample acquired 50,000 events at a slow flow rate (10 μl/min). Blue laser source (est. 488 nm) was used, and bandpass filters BP525/40 nm and BP675/50 nm were selected to collect fluorescence emission of SYTO24 and PI, respectively. The double staining method allowed us to distinguish three populations based on the three states of cell damage, namely, viable, injured, and dead cells, identified on a SYTO24 vs. PI dot plot according to the different ratio of green/red fluorescence signals from the positive and negative (autoclaved cells) control (Di Gregorio et al., 2022). The signals were measured as Total Fluorescent Units (TFUs), including all stained cells emitting fluorescence and embracing viable, dead, and injured cells. The ability to discriminate viable cells from damaged and dead cells allowed the numbering of viable cells as Active Fluorescent Units (AFU: total cell number minus damaged and dead ones) (Foglia et al., 2020; Di Gregorio et al., 2022). The parameters were acquired with a logarithmic scale and analyzed using CytExpert software v. 2.3 (Beckman Coulter Flow Cytometry, Milan, Italy). Cellular internal distribution results were expressed as percentage of alive, injured, and dead cells computed from the total FCM recorded events (50,000 events), excluding the background and standard bead signals. Each percentage was obtained as the average of three replicates from three independent experiments, and the standard deviation was always <5%.
2.7 Statistical analysis
The statistical analysis was performed by using ©GraphPad Prism software (version 9.5.1). Data are presented as mean ± standard deviations (SD) based on triplicates from at least three independent experiments. Data were compared using two-way ANOVA in Dunnett's multiple comparison tests with Tukey's pairwise test at p < 0.05 considered statistically significant (95% confidence interval).
3 Results
3.1 Carvacrol effect on L. plantarum DSM 20174 probiotic strain
3.1.1 Planktonic cells
Plate-count results showed a growth reduction that was proportional to the increasing concentration of carvacrol added. Significant results after carvacrol 250 and 500 ppm treatments were observed (Figure 1A). The highest carvacrol concentration (500 ppm) promoted a total loss of cultivability, while a logarithmic reduction of 4.62 was calculated after the application of carvacrol 250 ppm. However, the presence of carvacrol at concentrations of 50 and 100 ppm did not result in significant reductions in probiotic growth. A turbidimetric analysis also showed greater efficacy of 250 and 500 ppm carvacrol treatments. A higher inhibition percentage of 83.7% was observed when carvacrol at 500 ppm was used, confirming the loss of culturability detected using the culture-based methods (Figure 1B). Nevertheless, the FCM results showed that after carvacrol 500 ppm treatment, 42% of the cell population was still viable with no further ability to replicate on culture media, suggesting the transition of cells into the VBNC state treatment-induced (15% injured and 43% dead cells) (Figure 1C). Furthermore, FCM showed that after carvacrol 250 ppm treatment, the cells were mostly viable (63% alive, 16% damaged, and 21% dead).
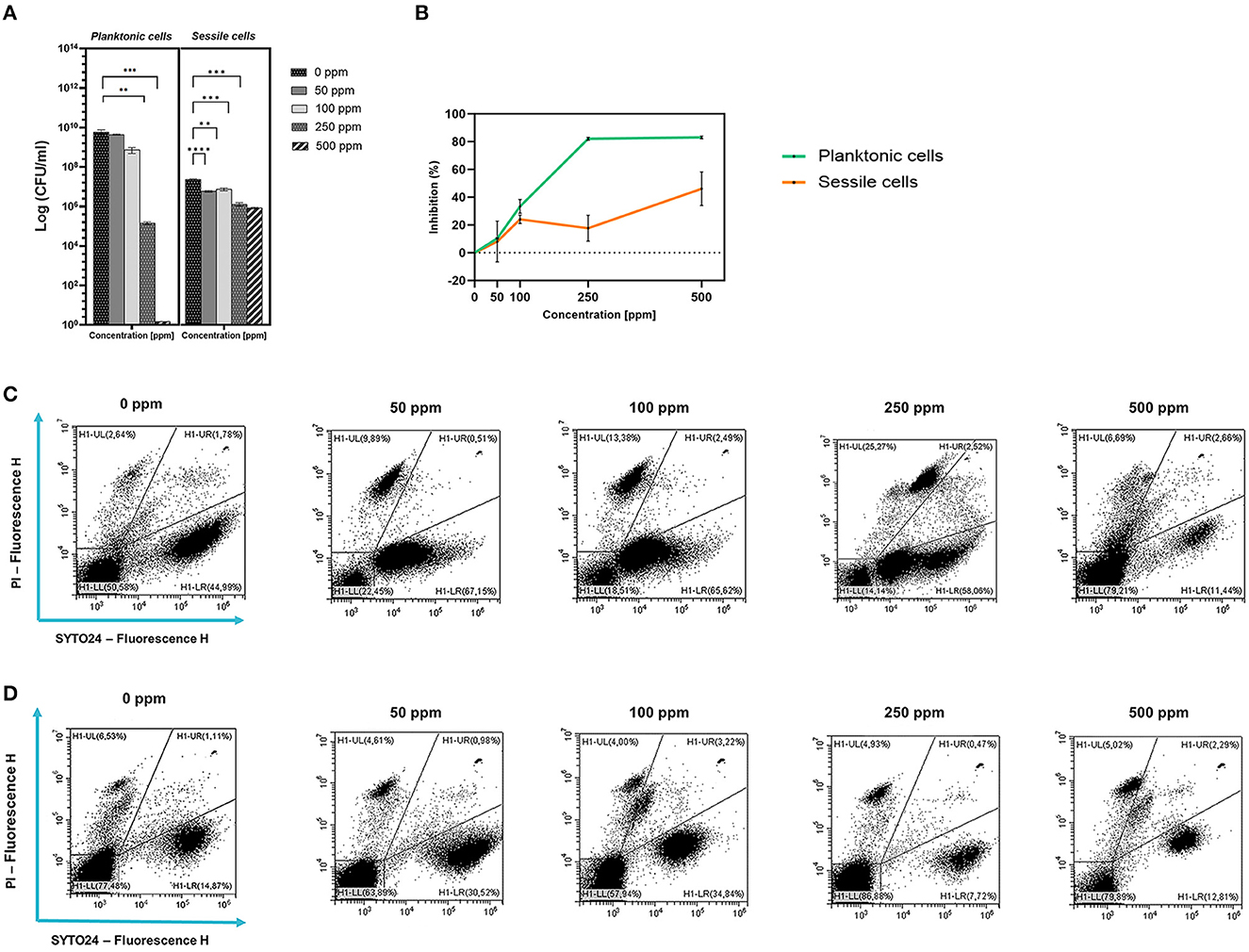
Figure 1. Effect of carvacrol against planktonic and sessile cells of L. plantarum DSM 20174 grown in MRS broth and plated on MRS agar plate. Histograms indicate the Log CFU/ml values after antimicrobial treatment with increasing concentration of carvacrol (50, 100, 250, and 500 ppm) compared to control (0 ppm) after 24 h of incubation. (*) p < 0.05, (**) p < 0.01, (***) p < 0.001, and (****) p < 0.0001 (A). Carvacrol antimicrobial treatment efficacy on L. plantarum DSM 20174 planktonic (turbidimetric analysis, OD 595 nm) and sessile cells (CV assay) grown in MRS broth. Graphs indicate the inhibition growth percentage values after antimicrobial treatments (carvacrol 50, 100, 250, and 500 ppm) compared with control (0 ppm) after 24 h of incubation (B). Data (A, B) are presented as mean ± standard deviations (SD) based on triplicates from three independent experiments. FCM double-staining (SYTO24 and PI) dot plot of L. plantarum DSM 20174 planktonic (C) and sessile cells (D) grown in MRS broth in the presence of carvacrol (50, 100, 250, and 500 ppm) and diluted in PBS (pH 7.4). The results were compared with control (0 ppm) after 24 h of incubation. H1-LL, unstained debris; H1-LR, intact cells/viable cells (SYTO24 positive); H1-UR, injured cell population; H1-UL, permeabilized/dead cells (PI positive).
3.1.2 Sessile cells
Despite the loss of planktonic cell culturability, L. plantarum sessile fraction was less sensitive to carvacrol antimicrobial treatment (1.27 and 1.46 log reduction for 250 and 500 ppm, respectively). However, the reduction values were significant at 50 ppm concentration. A different behavior was observed when samples were analyzed using the CV assay (Figure 1). The highest percentage of inhibition was found after carvacrol 500 ppm treatment (46%), while carvacrol 250 ppm treatment resulted in 17.7%. An FCM analysis confirmed the resistance of L. plantarum sessile cells, showing a higher degree of damage at the carvacrol 100 and 500 ppm concentrations (29 and 21%, respectively), and a high percentage of alive cells was maintained at all tested concentrations (72, 53, 61, and 64% for 50, 100, 250, and 500 ppm, respectively).
3.2 Carvacrol effect on E. coli, P. fluorescens, and L. monocytogenes planktonic cells
Carvacrol treatment had a significant antimicrobial action on E. coli, P. fluorescens, and L. monocytogenes planktonic cells for all tested concentrations (p < 0.05) compared with the control (Figure 2). Overall, 250 and 500 ppm concentrations promoted a total loss of culturability in each strain. A high logarithmic reduction in P. fluorescens cells compared with the control was observed at 2.27 and 3.20 when 50 and 100 ppm concentrations of carvacrol were applied. For the same concentrations, the E. coli cell growth was reduced to 1.10 and 2.29 and to 0.86 and 1.61 in L. monocytogenes, respectively. According to the plate-count results, after the addition of carvacrol 250 and 500 ppm, more than 90% of inhibition in E. coli e P. fluorescens was calculated by turbidimetric analysis, and 82 and 78% of inhibition were estimated in L. monocytogenes (Figures 3A–C). In all tested strains, 250 ppm was the minimum concentration which was able to inhibit at least 80% of the cell population. In P. fluorescens, inhibition of 37% was observed already after 50 ppm treatment, compared with 25 and 12% in E. coli and L. monocytogenes for the same concentration, respectively. L. monocytogenes was shown to be the least susceptible strain to carvacrol antimicrobial action (Figures 3A–C). The dot plot obtained from FCM analysis showed different microorganisms' responses to the treatments (Figure 4): Planktonic cells of E. coli exhibited a proportional damage degree up to the 250 ppm carvacrol concentration, while after 500 ppm treatment, part of the cells recovered viability resulting in 46% viable cells. Therefore, the FCM analysis suggested that the cells were unculturable but still viable (VBNC) (Figure 4A). Samples treated with 50 ppm maintained a viable cell fraction of 84% compared with 87% alive cells of the control. Using the 100 ppm concentration, injured and dead cells increased to 12 and 23%, respectively, while at 250 ppm concentration, 61% were damaged and only 27 and 12% were viable and dead, respectively. However, in P. fluorescens and L. monocytogenes, the percentage of viable cells remained almost unchanged after treatments: in P. fluorescens, viable cells were 52, 48, 51, and 56% for carvacrol at 50, 100, 250, and 500 ppm concentrations, respectively, while in L. monocytogenes, they were 71, 68, 59, and 43% for the same increasing concentrations of carvacrol, respectively (Figures 4B, C).
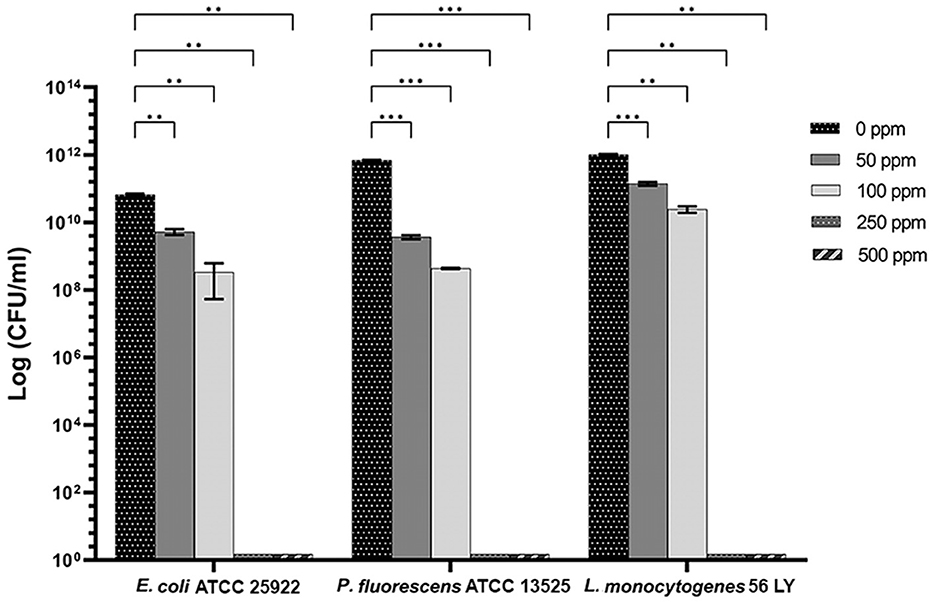
Figure 2. Effect of carvacrol against planktonic cells of E. coli ATCC 25922, P. fluorescens ATCC 13525, and L. monocytogenes 56 LY, grown in BHI broth (E. coli and L. monocytogenes) and TSB broth (P. fluorescens) and plated on BHI agar (E. coli and L. monocytogenes) and TSA agar (P. fluorescens). Histograms indicate the Log CFU/ml values after antimicrobial treatment with increasing concentrations of carvacrol (50, 100, 250, and 500 ppm) compared with control (0 ppm) after 24 h of incubation. Data are presented as mean ± standard deviations (SD) based on triplicates from three independent experiments. (*) p < 0.05, (**) p < 0.01, (***) p < 0.001, and (****) p < 0.0001.
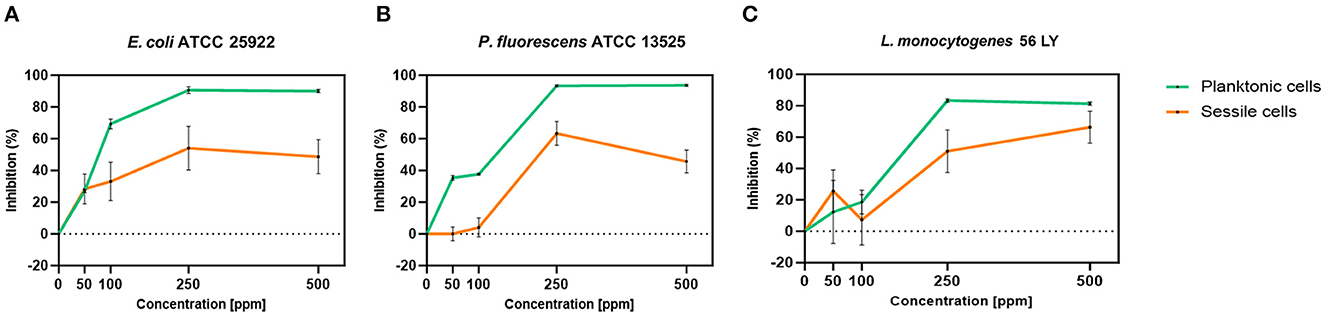
Figure 3. Carvacrol antimicrobial treatment efficacy on E. coli ATCC 25922 (A), P. fluorescens ATCC 13525 (B), and L. monocytogenes 56 LY (C) planktonic (turbidimetric analysis, OD 595 nm) and sessile cells (CV assay), grown in BHI broth (E. coli and L. monocytogenes) and TSB broth (P. fluorescens). Graphs indicate the inhibition growth percentage values after antimicrobial treatments (carvacrol 50, 100, 250, and 500 ppm) compared with control (0 ppm), after 24 h of incubation. Data are presented as mean ± standard deviations (SD) based on triplicates from three independent experiments.
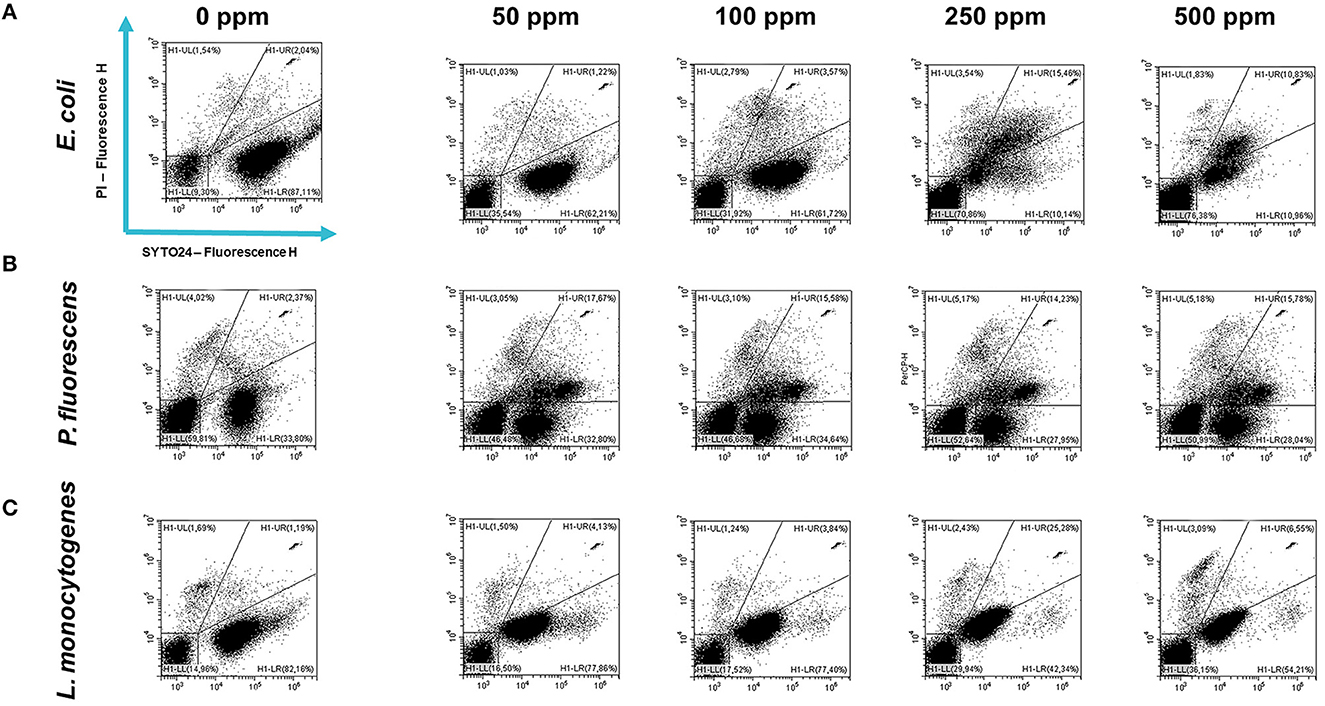
Figure 4. Double-staining dot plot of E. coli ATCC 25922 (A), P. fluorescens ATCC 13525 (B), and L. monocytogenes 56 LY (C) planktonic cells grown in BHI broth (E. coli and L. monocytogenes) and TSB broth (P. fluorescens) in the presence of carvacrol (50, 100, 250, and 500 ppm) and diluted in PBS (pH 7.4). The results were compared with 0 ppm (control) after 24 h of incubation. Cells were stained with SYTO24 and PI simultaneously. H1-LL, unstained debris; H1-LR, intact cells/viable cells (SYTO24); H1-UR, injured cell population; H1-UL, permeabilized/dead cells (PI).
3.3 Carvacrol effect on E. coli, P. fluorescens, and L. monocytogenes sessile cells
Carvacrol antimicrobial action had a significant effect on P. fluorescens sessile fraction when 250 and 500 ppm concentrations were applied (1.95 and 0.65 log reduction, respectively) (p < 0.05) and on L. monocytogenes after 100, 250, and 500 ppm treatments (0.82, 2.49, and 2.49, respectively) (p < 0.05). For E. coli, a logarithmic reduction of 3.10, 3.09, 4.82, and 3.66 after carvacrol 50, 100, 250, and 500 ppm treatments was found, respectively (p > 0.05) (Figure 5). Based on the culture-based results, carvacrol 250 ppm proved to be the most effective minimum concentration in all tested strains, whereas increasing to 500 ppm did not enhance the antimicrobial effect (Figure 5). Unlike the planktonic fraction, no loss of culturability in any strain was observed; indeed, the minimum growth level after treatment was 104 Log CFU/ml (E. coli, carvacrol 250 ppm) (Figures 2, 5). The CV test showed a percentage of inhibition of 52 and 63% for E. coli and P. fluorescens, respectively, while in L. monocytogenes, the higher efficacy was obtained after carvacrol 500 ppm (65% reduction compared with 48% inhibition related to carvacrol 250 ppm) (Figure 3C). FCM analysis confirmed a greater carvacrol effect at 250 ppm concentration in E. coli e P. fluorescens. E. coli viability fraction was down to 4% in favor of a highly damaged state, which represented 73% of cells. Nevertheless, after the carvacrol 500 ppm treatment, 36% of cells persisted viable, and 27 and 37% were injured and dead cells, respectively (Figure 6A). Overall, 50 ppm concentration increased the damaged state (33 %) compared with the 100 ppm concentration, where 43% of cells were viable, 21% were injured, and 36% were dead cells. P. fluorescens displayed an increase in damaged cells as early as 50 ppm concentration (51% compared with 22% of the control), resulting in a bactericidal effect when the 250 ppm concentration was applied: 23% alive, 31% injured, and 46% dead cells (Figure 6B). The most notable percentages of dead cells were observed at concentrations of 50 and 250 ppm (31 and 46%); indeed, after 100 ppm treatment, a percentage of 42% was still viable. Although 500 ppm was the highest concentration used, this resulted in a high fraction of viable cells after treatment (68%), and only 15 and 17% were damaged and dead, respectively. On the other hand, in L. monocytogenes, minor reductions in viable cells were observed (68, 61, 57, and 54% at 50, 100, 250, and 500 ppm, respectively) (Figure 6C).
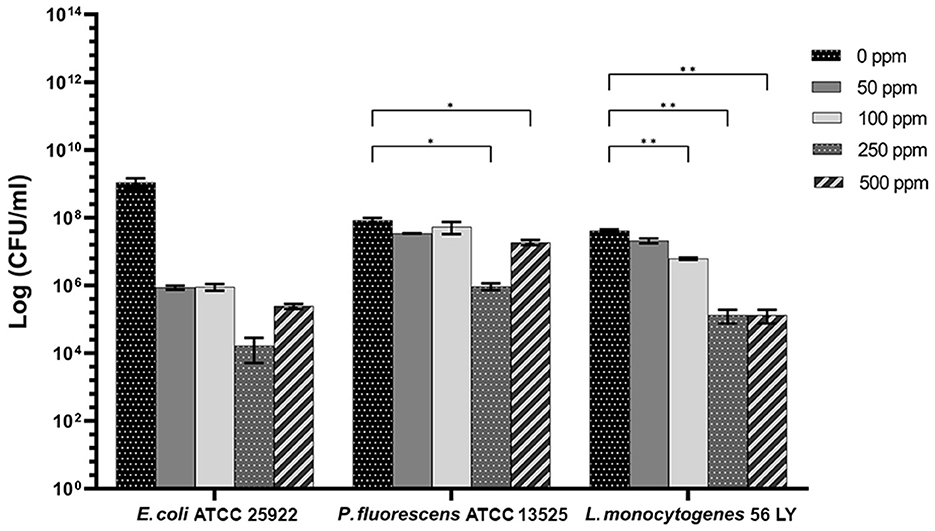
Figure 5. Effect of carvacrol against sessile cells of E. coli ATCC 25922, P. fluorescens ATCC 13525, and L. monocytogenes 56 LY grown in BHI broth (E. coli and L. monocytogenes) and TSB broth (P. fluorescens) and plated on BHI agar (E. coli and L. monocytogenes) and TSA agar (P. fluorescens). Histograms indicate the Log CFU/ml values after antimicrobial treatment with increasing concentrations of carvacrol (50, 100, 250, and 500 ppm) compared with control (0 ppm) after 24 h of incubation. Data are presented as mean ± standard deviations (SD) based on triplicates from three independent experiments. (*) p < 0.05, (**) p < 0.01, (***) p < 0.001, and (****) p < 0.0001.
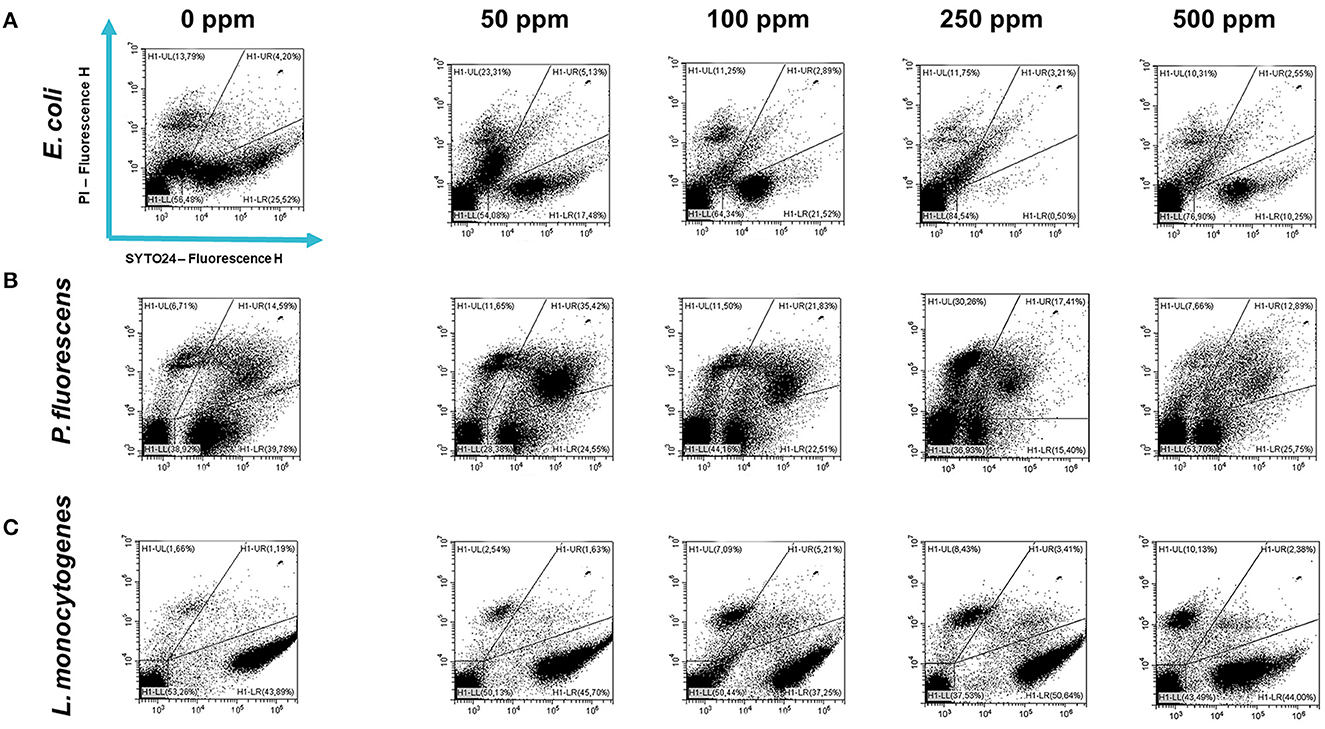
Figure 6. Double-staining dot plot of E. coli ATCC 25922 (A), P. fluorescens ATCC 13525 (B), and L. monocytogenes 56 LY (C) sessile cells grown in BHI broth (E. coli and L. monocytogenes) and TSB broth (P. fluorescens) in the presence of carvacrol (50, 100, 250, and 500 ppm) and diluted in PBS (pH 7.4). The results were compared with control (0 ppm) after 24 h of incubation. Cells were stained with SYTO24 and PI simultaneously. H1-LL, unstained debris; H1-LR, intact cells/viable cells (SYTO24); H1-UR, injured cell population; H1-UL, permeabilized/dead cells (PI).
3.4 Pre-formed L. plantarum biofilm combined with carvacrol against E. coli, P. fluorescens, and L. monocytogenes
3.4.1 Planktonic cells
The presence of pre-formed biofilm evaluated by the culture-based methods determined a significant growth reduction in P. fluorescens (2.82) (p < 0.05) (Figure 7). The combined effect of L. plantarum +100 ppm caused a significant reduction in all tested strains (p < 0.05) compared with carvacrol treatment alone: L. plantarum + 100 ppm promoted a reduction of 2.54 in E. coli, 6.22 in P. fluorescens, and 3.64 in L. monocytogenes. Moreover, the turbidity assay showed a growth inhibition of 58, 43, and 32% in E. coli, P. fluorescens, and L. monocytogenes, respectively (Figures 8A–C). A total loss of culturability in all samples that were treated with only carvacrol 250 ppm and L. plantarum + 250 ppm was observed (Figure 7). The logarithmic reduction detected by plate count was confirmed by the turbidity assay, where more than 80% of inhibition was detected (Figures 7, 8). FCM analysis emphasized the difference between the bactericidal effect of combined L. plantarum + 250 ppm treatment and the bacteriostatic action of carvacrol 250 ppm alone (69% damaged cells) against E. coli (Figure 9A). The presence of pre-formed biofilm induced a strong cellular impairment, leading to an increase in the background signal caused by the presence of debris, while the combined treatment (L. plantarum + 100 ppm) showed no substantial differences from the single one (100 ppm) (Figure 9A). In P. fluorescens, the antimicrobial action of single treatment with pre-formed biofilm (L.p.) was already evident in terms of cellular damage and death (26 and 44%, respectively) compared with the 100 ppm carvacrol treatment alone (42 and 13%, respectively). Moreover, P. fluorescens samples treated with carvacrol 100 ppm showed a reduction in viable fraction from the single (45%) to the combined treatment (26%). The L. plantarum + 250 treatment compromised a cellular membrane with a subsequent increase in the background signal, although a viable fraction of 32% persisted but was still lower than the 250 ppm single treatment (48%) (Figure 9B). Finally, the internal population distribution of L. monocytogenes was not significantly changed (Figure 9C).
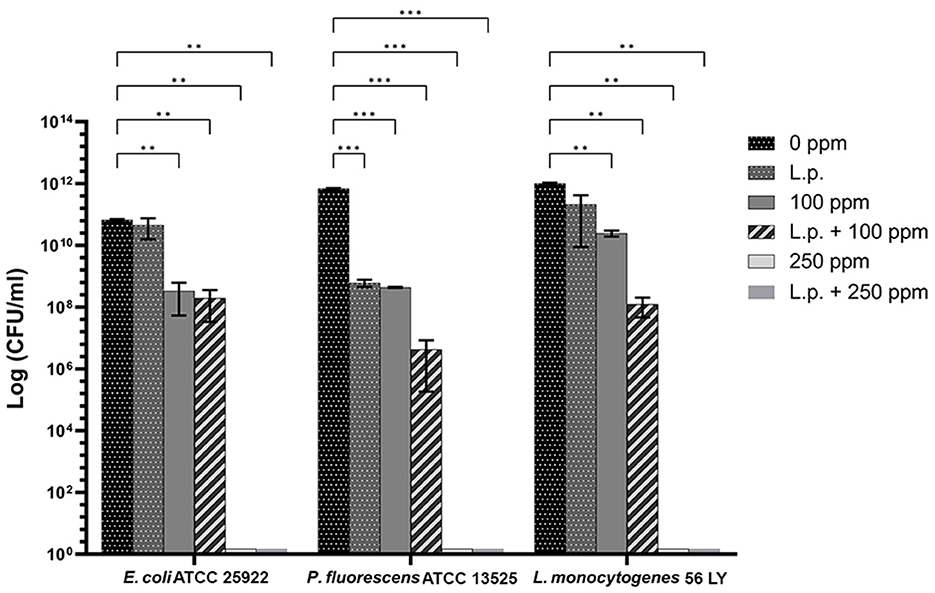
Figure 7. Effect of L. plantarum pre-formed biofilm against planktonic cells of E. coli ATCC 25922, P. fluorescens ATCC 13525, and L. monocytogenes 56 LY grown in BHI broth (E. coli and L. monocytogenes) and TSB broth (P. fluorescens), and plated on BHI agar (E. coli and L. monocytogenes) and TSA agar (P. fluorescens). Histograms indicate the Log CFU/mL values after antimicrobial treatments: single treatments with L. plantarum pre-formed biofilm (L.p.), carvacrol 100 ppm, and carvacrol 250 ppm against each strain, and combined treatments with carvacrol 100 ppm (L.p. + 100 ppm) and 250 ppm (L.p. + 250 ppm), compared to control (0 ppm) after 24 h of incubation. Data are presented as mean ± standard deviations (SD) based on triplicates from three independent experiments. (*) p < 0.05, (**) p < 0.01, (***) p < 0.001, (****) p < 0.0001.
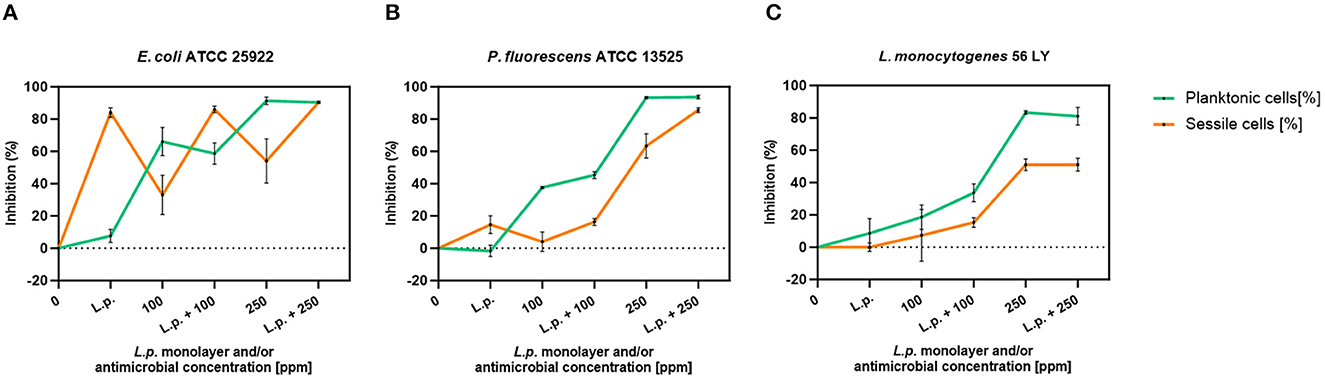
Figure 8. L. plantarum pre-formed biofilm efficacy on E. coli ATCC 25922 (A), P. fluorescens ATCC 13525 (B), and L. monocytogenes 56 LY (C) planktonic (turbidimetric analysis, OD 595 nm) and sessile cells (CV assay) grown in BHI broth (E. coli and L. monocytogenes) and TSB broth (P. fluorescens). Graphs indicate the inhibition growth percentage values after antimicrobial treatments: single treatments with L. plantarum pre-formed biofilm alone (L.p.), carvacrol 100 and 250 ppm, and combined treatments with carvacrol 100 ppm (L.p. + 100 ppm) and 250 ppm (L.p. + 250 ppm), compared with control (0 ppm) after 24 h of incubation. Data are presented as mean ± standard deviations (SD) based on triplicates from three independent experiments.
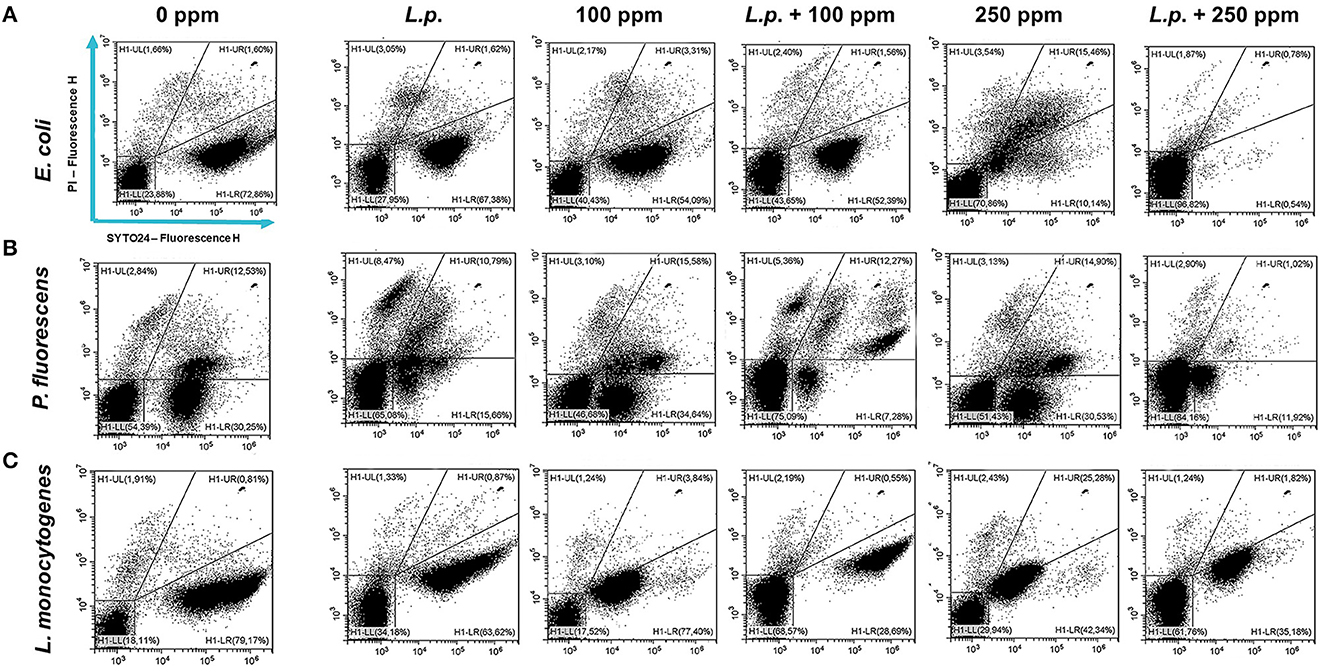
Figure 9. Double-staining dot plot of E. coli ATCC 25922 (A), P. fluorescens ATCC 13525 (B), and L. monocytogenes 56 LY (C) planktonic cells grown in the presence of L. plantarum pre-formed biofilm and diluted in PBS (pH 7.4). The addition of L. plantarum pre-formed biofilm (L.p.) to tested strains and combined treatments with carvacrol 100 ppm (L.p. + 100 ppm) and 250 ppm (L.p. + 250 ppm), compared with control (0 ppm), carvacrol 100 and 250 ppm single treatments, after 24 h of incubation. Cells were stained with SYTO24 and PI simultaneously. H1-LL, unstained debris; H1-LR, intact cells/viable cells (SYTO24); H1-UR, injured cell population; H1-UL, permeabilized/dead cells (PI).
3.4.2 Sessile cells
A total loss of culturability in E. coli was detected after the pre-formed biofilm L. plantarum monolayer application, showing an enhanced antibiofilm efficacy when the probiotic action was completed. In addition, the absence of growth was observed when L. plantarum +100 ppm and L. plantarum + 250 ppm treatments were applied. P. fluorescens displayed a significant reduction of 1.52 with the single pre-formed L. plantarum treatment (p < 0.05), remaining comparable to the combined L. plantarum + 100 ppm one (1.43). However, P. fluorescens cells lost their ability to grow on agar medium after combined L. plantarum + 250 ppm treatment. L. monocytogenes responded with lower sensitivity to treatments than the other strains. L. plantarum pre-formed biofilm alone did not result in significant logarithmic reduction, instead a significant reduction of 0.6 and 2.82 was observed in L. plantarum + 100 ppm (p < 0.05) and L. plantarum + 250 ppm (p < 0.05), respectively, compared with the control (Figure 10). The results obtained with the CV assay confirmed the culturable ones: a reduction over 80% corresponded to a loss of culturability, while a reduced antimicrobial effect on L. monocytogenes was detected; nevertheless, the inhibition was still proportional to the intensity of the combined treatment applied (18 and 53% inhibition in L. plantarum +100 ppm and L. plantarum + 250 ppm, respectively) (Figures 8A–C). The FCM analysis allowed us to study the efficacy of different treatments (Figure 11); as for the planktonic fraction, the combined treatment caused a bactericidal effect unlike the carvacrol single one. In E. coli, L. plantarum pre-formed single treatment induced cellular death which was represented by 28%, while 57% of cells were injured. A smaller fraction of 15% was still viable, even though the culture-based methods showed a complete loss of culturability. The 100 ppm single treatment induced no significant bactericidal effect (46% viable), while the combined L. plantarum + 100 ppm treatment displayed a significant one (68% dead cells and 29% damaged cells), expressed by a complete loss of cell viability. When comparing the combined L. plantarum + 250 ppm treatment with carvacrol 250 ppm alone, a substantial difference was highlighted: in the single treatment, 64% of the cells were mainly damaged, whereas, after L. plantarum + 250 ppm treatment, only dead cells (91%) and an increased background signal with the absence of cell viability were recorded (Figure 11A). In P. fluorescens, a considerable effect of the combined treatment was found. The presence of L. plantarum pre-formed caused 76% cell death compared with the control. When carvacrol was added to the L. plantarum pre-formed at the lowest concentration of 100 ppm, the bactericidal action resulted in 74% dead and 22% damaged cells. Increasing the carvacrol concentration in the combined treatment, an enhanced background signal, due to cellular debris, was observed (<1% viable cells) (Figure 11B). Although L. monocytogenes explicated a lower sensitivity to the treatments, an enhanced bactericidal effect on the combined treatment was quantified. The L. plantarum pre-formed biofilm resulted in the following distribution of cell populations: 34% alive, 38% injured, and 28% dead, whereas in L. plantarum + 100 ppm and L. plantarum + 250 ppm, 41 and 36% cells represented dead population, respectively (Figure 11C).
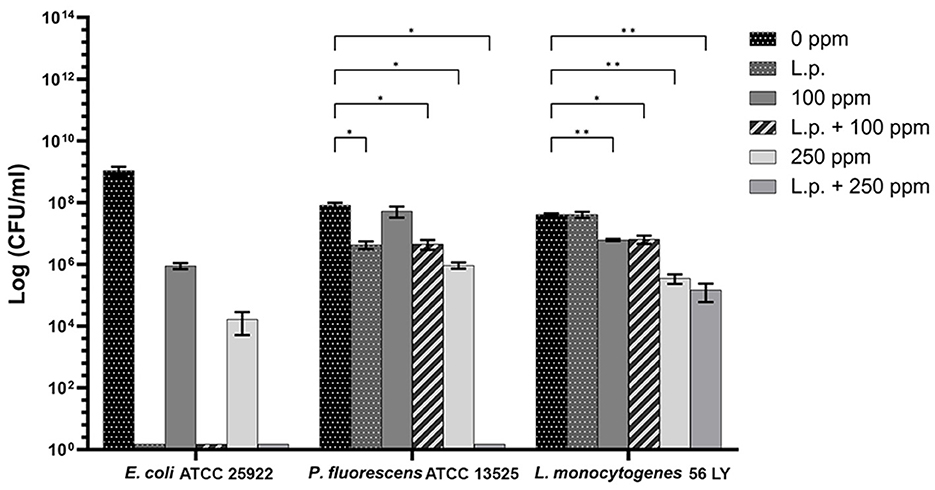
Figure 10. Effect of L. plantarum pre-formed biofilm against sessile cells of E. coli ATCC 25922, P. fluorescens ATCC 13525, and L. monocytogenes 56 LY grown in BHI broth (E. coli and L. monocytogenes) and TSB broth (P. fluorescens) and plated on BHI agar (E. coli and L. monocytogenes) and TSA agar (P. fluorescens). Histograms indicate the Log CFU/ml values after antimicrobial treatments: single treatments with L. plantarum pre-formed biofilm (L.p.), carvacrol 100 ppm, and carvacrol 250 ppm against each strain, and combined treatments with carvacrol 100 ppm (L.p. + 100 ppm) and 250 ppm (L.p. + 250 ppm), compared with control (0 ppm) after 24 h of incubation. Data are presented as mean ± standard deviations (SD) based on triplicates from three independent experiments. (*) p < 0.05, (**) p < 0.01, (***) p < 0.001, and (****) p < 0.0001.
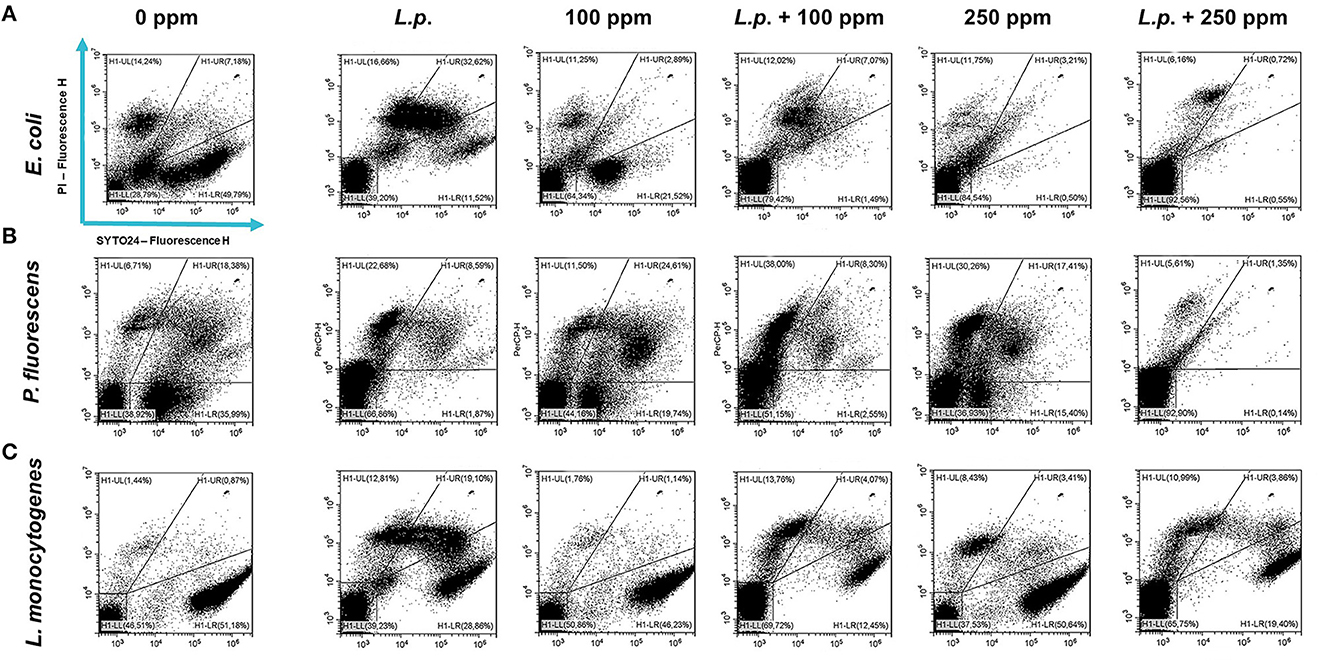
Figure 11. Double-staining dot plot of E. coli ATCC 25922 (A), P. fluorescens ATCC 13525 (B), and L. monocytogenes 56 LY (C) sessile cells grown in the presence of L. plantarum pre-formed biofilm, diluted in PBS (pH 7.4). The addition of L. plantarum pre-formed biofilm to tested strains (L.p.) and combined treatments with carvacrol 100 ppm (L.p. + 100 ppm) and 250 ppm (L.p. + 250 ppm), compared with control (0 ppm), carvacrol 100 and 250 ppm single treatments, after 24 h of incubation. Cells were stained with SYTO24 and PI simultaneously. H1-LL, unstained debris; H1-LR, intact cells/viable cells (SYTO24); H1-UR, injured cell population; H1-UL, permeabilized/dead cells (PI).
4 Discussion
Foodborne biofilms are of particular concern in the food packaging industry, with the prevalence of some pathogens such as L. monocytogenes, E. coli, or spoilage (e.g., P. fluorescens) microbes, all found to be predominantly adherent resilient formers of biofilms on foods and food preparation and surfaces (Olanbiwoninu and Popoola, 2023). In this study, the combined bactericidal effect of carvacrol (100 and 250 ppm) and pre-formed biofilm monolayer of L. plantarum against foodborne pathogens (E. coli and L. monocytogenes) and spoilage (P. fluorescens) microorganisms, in vitro, was assessed. Primarily, the impact of carvacrol on L. plantarum was investigated to assess the carvacrol viability effect on the L. plantarum monolayer. The first results showed that the probiotic L. plantarum demonstrated strong tolerance to carvacrol. The L. plantarum planktonic fraction persisted viable up to 250 ppm carvacrol concentration, while the sessile fraction showed greater resistance to the antimicrobial treatment, preserving cell viability even after the 500 ppm treatment. Further tests involving carvacrol alone against E. coli, P. fluorescens, and L. monocytogenes were performed, enabling the fulfillment of two objectives: selecting the most effective carvacrol concentration against all target strains and, simultaneously, preserving the L. plantarum monolayer viability to enhance the overall antimicrobial effect. Additionally, the culture-based methods overestimated the effectiveness of carvacrol treatment against E. coli, P. fluorescens, and L. monocytogenes, showing a loss of culturability when the cells were still viable. FCM allows the identification of VBNC cells after using carvacrol concentrations of 250 and 500 ppm: in this state, the cells may regain culturable when favorable conditions are restored, posing a potential risk to the consumer (Jayeola et al., 2022). Moreover, the 250 ppm carvacrol concentration showed higher efficacy in inhibiting biofilm-forming adherent cells across all tested strains, while the increase in concentration (500 ppm) did not significantly enhance the antimicrobial effect. Furthermore, the lowest concentration of 100 ppm was selected to evaluate if a reduced amount of carvacrol could be utilized in a combined treatment with L. plantarum pre-formed biofilm, to counteract undesirable organoleptic impact and control foodborne pathogens and food spoilage biofilm (Hellebois et al., 2020; Pateiro et al., 2021). Indeed, antimicrobial resistance of biofilm-forming cells is a severe threat to the food industry (Esposito and Turku, 2023; Olanbiwoninu and Popoola, 2023). It is well-known that Lactobacillus species produce different exometabolites and biosurfactants with antibiofilm activity and are able to compete with pathogens for nutrients and space with different mechanisms of action (Barzegari et al., 2020). LAB can form biofilms on biotic and abiotic surfaces, acting as antagonistic effectors against various foodborne pathogens in either planktonic or biofilm mode of growth (Tatsaporn and Kornkanok, 2020; Tomé et al., 2023). Here, we found that when LAB and EOs or their components are examined individually, they exploit different effects, suggesting that their combined use can enhance the antimicrobial and antibiofilm action, improving their functioning. It is well-known that the high tolerance of LAB to lowering pH is caused by self-produced organic acids and, at low pH, the antimicrobial activity of carvacrol is enhanced due to its increased hydrophobicity, allowing it to dissolve freely in the cell membrane lipids of target bacteria (Jara et al., 2020; Cisneros et al., 2021; Sornsenee et al., 2021; Abiola et al., 2022). In addition, antimicrobial LAB-bacteriocins, which have been widely studied for their preservative properties in the food industry, improve the antimicrobial effect by reducing the carvacrol sensory impact on the food matrix (Kim et al., 2019; Simons et al., 2020; Gumienna and Górna, 2021). The combined treatment showed a more marked impact, compared with the single treatment, in the planktonic fractions and, most notably, in the sessile fractions of the tested strains. Both in E. coli and P. fluorescens, a strong growth inhibition with the combined treatment was observed. The L. plantarum biofilm monolayer action allowed the use of lower concentrations of carvacrol (100 ppm) to achieve substantial damage to bacterial physiology; pre-formed biofilm induced severe cellular impairment. Recognizing the cellular damage degree after treatment is crucial to avoid the resuscitation of viable but not culturable cells that could recover full pathogenicity, comparable to untreated bacterial cells in the log phase (Kan et al., 2019; Jayeola et al., 2022). This study displayed that the combined treatment produced a bactericidal effect which was not detectable by single treatments, showing mainly a bacteriostatic action. Furthermore, L. monocytogenes exhibited the most negligible efficacy among all strains, being the microorganism most resistant to pH changes and able to adapt in environments unfavorable to its growth (Barker and Park, 2001). Considering FCM results, it may be concluded that using carvacrol alone can determine, especially at high concentrations, stress and VBNC state induction. Nevertheless, turbidimetric assays on both planktonic and sessile cells were in agreement with the culture-based methods, underlying the need to integrate conventional microbiology methods based on culturability with advanced real-time techniques that can provide information on bacterial physiology (Fleischmann et al., 2021; Zand et al., 2021; Özel Duygan and van der Meer, 2022). Therefore, based on the findings from FCM analysis indicating that carvacrol primarily causes cellular damage rather than exerting direct bactericidal effects, the synergistic action of an L. plantarum monolayer combined with carvacrol presents a natural alternative to enhance the efficacy of carvacrol. This approach enables the achievement of bactericidal action, specifically against biofilm-forming cells, providing a promising alternative to be incorporated in a functionalized packaging.
5 Conclusion
The results of the present study confirm that the application of lactic acid bacteria (LAB) and carvacrol represents a promising solution for the natural control of food packaging pathogenic and spoilage foodborne biofilms. L. plantarum biofilm monolayer and carvacrol together showed an enhanced antibiofilm action, acting on the adhesion process. Indeed, the combined treatment with LAB, which create an acidic environment, fostered the interaction and dissolution of carvacrol in the cell membrane lipids of target bacteria, thus allowing its use at sub-inhibitory concentrations and achieving an increased efficacy. The FCM analysis enabled a comprehensive investigation of cell “sub-populations” distribution based on the physiological state, providing information on viability vs. culturability and highlighting the overestimation of the treatment success if considering the culture-based approaches only. Further studies are needed to test the antibiofilm combination against other pathogenic bacteria. Furthermore, it is crucial to perform in vivo analysis, evaluating the impact of LAB biofilm monolayer plus carvacrol on product shelf-life and characteristics, by enclosing them within a matrix or inert material (e.g., polymers). Considering the expansion of the functional food market over the decades due to increased “green consumer” demand for natural, nutritional, and healthy food products, it will be essential to implement food packaging and design successful delivery systems for such bioactive compounds.
Data availability statement
The raw data supporting the conclusions of this article will be made available by the authors, without undue reservation.
Author contributions
VP: Conceptualization, Data curation, Formal analysis, Investigation, Methodology, Software, Visualization, Writing – original draft, Writing – review & editing. LD: Conceptualization, Data curation, Formal analysis, Investigation, Methodology, Software, Visualization, Writing – original draft, Writing – review & editing. MC: Conceptualization, Investigation, Methodology, Writing – review & editing. CN: Methodology, Writing – review & editing. RB: Methodology, Validation, Writing – review & editing. LG: Funding acquisition, Writing – review & editing. AB: Conceptualization, Funding acquisition, Investigation, Methodology, Project administration, Resources, Supervision, Validation, Visualization, Writing – original draft, Writing – review & editing.
Funding
The author(s) declare financial support was received for the research, authorship, and/or publication of this article. This research was supported by ILIP S.r.l and funded by the European Commission—NextGenerationEU programme under the National Recovery and Resilience Plan (NRRP), Mission 4 “Education and Research,” Component 2 “From research to business:” Project ON Foods—Research and innovation network on food and nutrition Sustainability, Safety and Security—Working ON Foods, Project code PE00000003, CUP I83C22001790001, Investment 1.3 creation of “Partnerships extended to universities, research centers, companies for the financing of basic research projects,” and Project SUS-MIRRI.IT “Strengthening the MIRRI Italian Research Infrastructure for Sustainable Bioscience and Bioeconomy,” Project code IR0000005, CUP D13C22001390001, Investment 3.1 Fund for the realisation of an integrated system of research and innovation infrastructures, Action 3.1.1 “Creation of new research infrastructures strengthening of existing ones and their networking for Scientific Excellence under Horizon Europe.”
Acknowledgments
The authors are grateful to Dr. Sergio Lucretti (ENEA) for the helpful discussion. Their special thanks are extended to Dr. Massimo Iannetta, Director of ENEA Biotechnologies and Agroindustry Division, and Dr. Claudia Zoani (ENEA). The authors gratefully acknowledge the highly valuable technical and administrative support of Stefano Rinaldi and Matteo Magaudda (ENEA). The authors are grateful for the support of the ENEA microbial collection and the Microbial Resource Research Infrastructure—Italian Joint Research Unit (MIRRI-IT) for providing data related to microbial collections.
Conflict of interest
LG was employed by I.L.P.A. Group.
The remaining authors declare that the research was conducted in the absence of any commercial or financial relationships that could be construed as a potential conflict of interest.
The author(s) declared that they were an editorial board member of Frontiers, at the time of submission. This had no impact on the peer review process and the final decision.
Publisher's note
All claims expressed in this article are solely those of the authors and do not necessarily represent those of their affiliated organizations, or those of the publisher, the editors and the reviewers. Any product that may be evaluated in this article, or claim that may be made by its manufacturer, is not guaranteed or endorsed by the publisher.
Supplementary material
The Supplementary Material for this article can be found online at: https://www.frontiersin.org/articles/10.3389/fmicb.2023.1296608/full#supplementary-material
References
Abers, M., Schroeder, S., Goelz, L., Sulser, A., St. Rose, T., Puchalski, K., et al. (2021). Antimicrobial activity of the volatile substances from essential oils. BMC Comp. Med. Ther. 21, 1–14. doi: 10.1186/s12906-021-03285-3
Abiola, R. R., Okoro, E. K., and Sokunbi, O. (2022). Lactic acid bacteria and the food industry - a comprehensive review. Int. J. Heal. Sci. Res. 12, 128–142. doi: 10.52403/ijhsr.20220516
Ajlouni, S., Ranadheera, C. S., and Chua, E. L. (2021). Encapsulation increases the in vitro bioaccessibility of probiotics in yoghurt. Int. J. Dairy Technol. 74, 118–127. doi: 10.1111/1471-0307.12746
Angane, M., Swift, S., Huang, K., Butts, C. A., and Quek, S. Y. (2022). Essential oils and their major components: an updated review on antimicrobial activities, mechanism of action and their potential application in the food industry. Foods 11, 464. doi: 10.3390/foods11030464
Arioli, S., Montanari, C., Magnani, M., Tabanelli, G., Patrignani, F., Lanciotti, R., et al. (2019). Modelling of Listeria monocytogenes Scott A after a mild heat treatment in the presence of thymol and carvacrol: effects on culturability and viability. J. Food Eng. 240, 73–82. doi: 10.1016/j.jfoodeng.2018.07.014
Asadi, S., Nayeri-Fasaei, B., Zahraei-Salehi, T., Yahya-Rayat, R., Shams, N., and Sharifi, A. (2023). Antibacterial and anti-biofilm properties of carvacrol alone and in combination with cefixime against Escherichia coli. BMC Microbiol. 23, 1–10. doi: 10.1186/s12866-023-02797-x
Asare, E. O., Mun, E. A., Marsili, E., and Paunov, V. N. (2022). Nanotechnologies for control of pathogenic microbial biofilms. J. Mater. Chem. B 10, 5129–5153. doi: 10.1039/D2TB00233G
Asma, S. T., Imre, K., Morar, A., Herman, V., Acaroz, U., Mukhtar, H., et al. (2022). An overview of biofilm formation–combating strategies and mechanisms of action of antibiofilm agents. Life 12, 1–31. doi: 10.3390/life12081110
Bakry, A. M., Abbas, S., Ali, B., Majeed, H., Abouelwafa, M. Y., Mousa, A., et al. (2016). Microencapsulation of oils: a comprehensive review of benefits, techniques, and applications. Compr. Rev. Food Sci. Food Saf. 15, 143182. doi: 10.1111/1541-4337.12179
Barker, C., and Park, S. F. (2001). Sensitization of Listeria monocytogenes to low pH, organic acids, and osmotic stress by ethanol. Appl. Environ. Microbiol. 67, 1594–1600. doi: 10.1128/AEM.67.4.1594-1600.2001
Barzegari, A., Kheyrolahzadeh, K., Mahdi, S., Khatibi, H., Sharifi, S., Memar, M. Y., et al. (2020). The battle of probiotics and their derivatives against biofilms. Infect. Drug Resist. 13, 659–672. doi: 10.2147/IDR.S232982
Bazargani, M. M., and Rohloff, J. (2016). Antibiofilm activity of essential oils and plant extracts against Staphylococcus aureus and Escherichia coli biofilms. Food Control. 61, 156–164. doi: 10.1016/j.foodcont.2015.09.036
Bondi, M., Messi, P., Halami, P. M., Papadopoulou, C., and De Niederhausern, S. (2014). Emerging microbial concerns in food safety and new control measures. Biomed Res. Int. 2014, 251512. doi: 10.1155/2014/251512
Bragonzi, A., Farulla, I., Paroni, M., Twomey, K. B., Pirone, L., Lor,è, N. I., et al. (2012). Modelling co-infection of the cystic fibrosis lung by Pseudomonas aeruginosa and Burkholderia cenocepacia reveals influences on biofilm formation and host response. PLoS ONE 7, e0052330. doi: 10.1371/journal.pone.0052330
Burt, S. (2004). Essential oils: their antibacterial properties and potential applications in foods—a review. Int. J. Food Microbiol. 94, 223–253. doi: 10.1016/j.ijfoodmicro.2004.03.022
Burt, S. A., Ojo-Fakunle, V. T. A., Woertman, J., and Veldhuizen, E. J. A. (2014). The natural antimicrobial carvacrol inhibits quorum sensing in Chromobacterium violaceum and reduces bacterial biofilm formation at sub-lethal concentrations. PLoS ONE 9, e0093414. doi: 10.1371/journal.pone.0093414
Cacciatore, F. A., Maders, C., Alexandre, B., Mauricio, C., Pinilla, B., Brandelli, A., et al. (2022). Carvacrol encapsulation into nanoparticles produced from chia and flaxseed mucilage: characterization, stability and antimicrobial activity against Salmonella and Listeria monocytogenes. Food Microbiol. 108, 104116. doi: 10.1016/j.fm.2022.104116
Cano, A., Contreras, C., Chiralt, A., and González-Martínez, C. (2021). Using tannins as active compounds to develop antioxidant and antimicrobial chitosan and cellulose based films. Carbohydr. Polym. Technol. Appl. 2, 100156. doi: 10.1016/j.carpta.2021.100156
Carrascosa, C., Raheem, D., Ramos, F., Saraiva, A., and Raposo, A. (2021). Microbial biofilms in the food industry—a comprehensive review. Int. J. Environ. Res. Public Health 18, 1–31. doi: 10.3390/ijerph18042014
Chouhan, S., Sharma, K., and Guleria, S. (2017). Antimicrobial activity of some essential oils—present status and future perspectives. Medicines 4, 58. doi: 10.3390/medicines4030058
Churklam, W., Chaturongakul, S., Ngamwongsatit, B., and Aunpad, R. (2020). The mechanisms of action of carvacrol and its synergism with nisin against Listeria monocytogenes on sliced Bologna sausage. Food Control 108, 106864. doi: 10.1016/j.foodcont.2019.106864
Cisneros, L., Cattelan, N., Villalba, M. I., Rodriguez, C., Serra, D. O., Yantorno, O., et al. (2021). Lactic acid bacteria biofilms and their ability to mitigate Escherichia coli O157:H7 surface colonization. Lett. Appl. Microbiol. 73, 247–256. doi: 10.1111/lam.13509
da Silva, A. R. P., do Costa, M. S., Araújo, N. J. S., de Freitas, T. S., dos Santos, A. T. L., Gonçalves, S. A., et al. (2023). Antibacterial activity and antibiotic-modifying action of carvacrol against multidrug-resistant bacteria. Adv. Sample Prep. 7, 100072. doi: 10.1016/j.sampre.2023.100072
de Carvalho, R. J., de Souza, G. T., Pagán, E., García-Gonzalo, D., Magnani, M., and Pagán, R. (2018). Nanoemulsions of Mentha piperita L. essential oil in combination with mild heat, pulsed electric fields (PEF) and high hydrostatic pressure (HHP) as an alternative to inactivate Escherichia coli O157: H7 in fruit juices. Innov. Food Sci. Emerg. Technol. 48, 219–227. doi: 10.1016/j.ifset.2018.07.004
Di Carli, M., De Rossi, P., Paganin, P., Del Fiore, A., Lecce, F., Capodicasa, C., et al. (2015). Bacterial community and proteome analysis of fresh-cut lettuce as affected by packaging. FEMS Microbiol. Lett. 363, 1–7. doi: 10.1093/femsle/fnv209
Di Gregorio, L., Tchuenchieu, A., Poscente, V., Arioli, S., Del Fiore, A., Costanzo, M., et al. (2022). Synergistic action of mild heat and essential oil treatments on culturability and viability of Escherichia coli ATCC 25922 tested in vitro and in fruit juice. Foods 11, 1615. doi: 10.3390/foods11111615
Esposito, M. M., and Turku, S. (2023). The use of natural methods to control foodborne biofilms. Pathogens 12, 1–18. doi: 10.3390/pathogens12010045
Fang, S., Zhou, Q., Hu, Y., Liu, F., and Mei, J. X. (2019). Carvacrol antimicrobien incorporé dans les films actifs de gomme de lin et d'alginate de sodium pour améliorer les attributs de qualité du bar de Chine (Lateolabrax maculatus) pendant la conservation au froid. Molecules 24, 1–17. doi: 10.3390/molecules24183292
Fikry, S., Khalil, N., and Salama, O. (2019). Chemical profiling, biostatic and biocidal dynamics of Origanum vulgare L. essential oil. AMB Exp. 9, 41. doi: 10.1186/s13568-019-0764-y
Fleischmann, S., Robben, C., Alter, T., Rossmanith, P., and Mester, P. (2021). How to evaluate non-growing cells—current strategies for determining antimicrobial resistance of VBNC bacteria. Antibiotics 10, 1–24. doi: 10.3390/antibiotics10020115
Foglia, C., Allesina, S., Amoruso, A., De Prisco, A., and Pane, M. (2020). New insights in enumeration methodologies of probiotic cells in finished products. J. Microbiol. Methods 175, 105993. doi: 10.1016/j.mimet.2020.105993
Fulaz, S., Vitale, S., Quinn, L., and Casey, E. (2019). Nanoparticle–biofilm interactions: the role of the eps matrix. Trends Microbiol. 27, 915–926. doi: 10.1016/j.tim.2019.07.004
Galié, S., García-Gutiérrez, C., Miguélez, E. M., Villar, C. J., and Lombó, F. (2018). Biofilms in the food industry: health aspects and control methods. Front. Microbiol. 9, 1–18. doi: 10.3389/fmicb.2018.00898
Govaris, A., Solomakos, N., Pexara, A., and Chatzopoulou, P. S. (2010). The antimicrobial effect of oregano essential oil, nisin and their combination against Salmonella Enteritidis in minced sheep meat during refrigerated storage. Int. J. Food Microbiol. 137, 175–180. doi: 10.1016/j.ijfoodmicro.2009.12.017
Gumienna, M., and Górna, B. (2021). Antimicrobial food packaging with biodegradable polymers and bacteriocins. Molecules 26, 3735. doi: 10.3390/molecules26123735
Hajibonabi, A., Yekani, M., Sharifi, S., Nahad, J. S., Dizaj, S. M., and Memar, M. Y. (2023). Antimicrobial activity of nanoformulations of carvacrol and thymol: new trend and applications. OpenNano 13, 100170. doi: 10.1016/j.onano.2023.100170
Hellebois, T., Tsevdou, M., and Soukoulis, C. (2020). Functionalizing and bio-preserving processed food products via probiotic and synbiotic edible films and coatings. Adv. Food Nutr. Res. 94, 161–221. doi: 10.1016/bs.afnr.2020.06.004
Hyldgaard, M., Mygind, T., and Meyer, R. L. (2012). Essential oils in food preservation: mode of action, synergies, and interactions with food matrix components. Front. Microbiol. 3, 1–24. doi: 10.3389/fmicb.2012.00012
Jara, J., Pérez-Ramos, A., del Solar, G., Rodríguez, J. M., Fernández, L., and Orgaz, B. (2020). Role of Lactobacillus biofilms in Listeria monocytogenes adhesion to glass surfaces. Int. J. Food Microbiol. 334, 108804. doi: 10.1016/j.ijfoodmicro.2020.108804
Jayeola, V., Farber, J. M., and Kathariou, S. (2022). Induction of the viable-but-nonculturable state in Salmonella contaminating dried fruit. Appl. Environ. Microbiol. 88, e0173321. doi: 10.1128/AEM.01733-21
Kan, Y., Jiang, N., Xu, X., Lyu, Q., Gopalakrishnan, V., Walcott, R., et al. (2019). Induction and resuscitation of the viable but non-culturable (VBNC) state in Acidovorax citrulli, the causal agent of bacterial fruit blotch of Cucurbitaceous crops. Front. Microbiol. 10, 1–13. doi: 10.3389/fmicb.2019.01081
Khodaei, S. M., Gholami-Ahangaran, M., Karimi Sani, I., Esfandiari, Z., and Eghbaljoo, H. (2023). Application of intelligent packaging for meat products: a systematic review. Vet. Med. Sci. 9, 481–493. doi: 10.1002/vms3.1017
Kim, N. N., Kim, W. J., and Kang, S. S. (2019). Anti-biofilm effect of crude bacteriocin derived from Lactobacillus brevis DF01 on Escherichia coli and Salmonella Typhimurium. Food Control 98, 274–280. doi: 10.1016/j.foodcont.2018.11.004
Lambert, R. J. W., Skandamis, P. N., Coote, P. J., and Nychas, G. J. (2001). A study of the minimum inhibitory concentration and mode of action of oregano essential oil, thymol and carvacrol. J. Appl. Microbiol. 91, 453–462. doi: 10.1046/j.1365-2672.2001.01428.x
Li, J., and Zhao, X. (2020). Effects of quorum sensing on the biofilm formation and viable but non-culturable state. Food Res. Int. 137, 109742. doi: 10.1016/j.foodres.2020.109742
Lu, J., Xuechao, H., and Ren, L. (2022). Biofilm control strategies in food industry: inhibition and utilization. Trends Food Sci. Technol. 123, 103–113. doi: 10.1016/j.tifs.2022.03.007
Luna, M., Beltran, O., Encinas-Basurto, D. A., Ballesteros-Monrreal, M. G., Topete, A., Hassan, N., et al. (2022). High antibacterial performance of hydrophobic chitosan-based nanoparticles loaded with carvacrol. Colloids Surf. B Biointer. 209, 112191. doi: 10.1016/j.colsurfb.2021.112191
March-Rosselló, G. A. (2017). Rapid methods for detection of bacterial resistance to antibiotics. Enferm. Infecc. Microbiol. Clin. 35, 182–188. doi: 10.1016/j.eimce.2017.02.007
Martín, I., Rodríguez, A., Delgado, J., and Córdoba, J. J. (2022). Strategies for biocontrol of Listeria monocytogenes using lactic dairy-ripened products. Foods 11, 1–18. doi: 10.3390/foods11040542
Mgomi, F. C., Yang, Y.-r., Cheng, G., and Yang, Z.-q. (2023). Lactic acid bacteria biofilms and their antimicrobial potential against pathogenic microorganisms. Biofilm 5, 100118. doi: 10.1016/j.bioflm.2023.100118
Mo, K., Li, J., Liu, F., Xu, Y., Huang, X., and Ni, H. (2022). Superiority of microencapsulated essential oils compared with common essential oils and antibiotics: effects on the intestinal health and gut microbiota of weaning piglet. Front. Nutr. 8, 1–14. doi: 10.3389/fnut.2021.808106
Mukurumbira, A. R., Shellie, R. A., Keast, R., Palombo, E. A., and Jadhav, S. R. (2022). Encapsulation of essential oils and their application in antimicrobial active packaging. Food Control 136, 108883. doi: 10.1016/j.foodcont.2022.108883
Natan, M., and Banin, E. (2017). From nano to micro: using nanotechnology to combat microorganisms and their multidrug resistance. FEMS Microbiol. Rev. 41, 302–322. doi: 10.1093/femsre/fux003
Ojogbo, E., Ward, V., and Mekonnen, T. H. (2020). Functionalized starch microparticles for contact-active antimicrobial polymer surfaces. Carbohydr. Polym. 229, 115422. doi: 10.1016/j.carbpol.2019.115422
Olanbiwoninu, A. A., and Popoola, B. M. (2023). Biofilms and their impact on the food industry. Saudi J. Biol. Sci. 30, 103523. doi: 10.1016/j.sjbs.2022.103523
Özel Duygan, B. D., and van der Meer, J. R. (2022). Recent advances in microbial community analysis from machine learning of multiparametric flow cytometry data. Curr. Opin. Biotechnol. 75, 102688. doi: 10.1016/j.copbio.2022.102688
Pateiro, M., Gómez, B., Munekata, P. E. S., Barba, F. J., Putnik, P., Kovačević, D. B., et al. (2021). Nanoencapsulation of promising bioactive compounds to improve their absorption, stability, functionality and the appearance of the final food products. Molecules 26, 1547. doi: 10.3390/molecules26061547
Qi, Z., Huang, Z., and Liu, C. (2022). Science of the total environment metabolism differences of biofilm and planktonic Pseudomonas aeruginosa in viable but nonculturable state induced by chlorine stress. Sci. Total Environ. 821, 153374. doi: 10.1016/j.scitotenv.2022.153374
Raman, J., Kim, J. S., Choi, K. R., Eun, H., Yang, D., Ko, Y. J., et al. (2022). Application of lactic acid bacteria (LAB) in sustainable agriculture: advantages and limitations. Int. J. Mol. Sci. 23, 7784. doi: 10.3390/ijms23147784
Ribeiro-Santos, R., Andrade, M., and Sanches-Silva, A. (2017). Application of encapsulated essential oils as antimicrobial agents in food packaging. Curr. Opin. Food Sci. 14, 78–84. doi: 10.1016/j.cofs.2017.01.012
Rubbens, P., and Props, R. (2021). Computational analysis of microbial flow cytometry data. mSystems 6, e00895–20. doi: 10.1128/mSystems00895-20
Sharma, H., Fidan, H., Özogul, F., and Rocha, J. M. (2022). Recent development in the preservation effect of lactic acid bacteria and essential oils on chicken and seafood products. Front. Microbiol. 13, 1092248. doi: 10.3389/fmicb.2022.1092248
Simons, A., Alhanout, K., and Duval, R. E. (2020). Bacteriocins, antimicrobial peptides from bacterial origin: overview of their biology and their impact against multidrug-resistant bacteria. Microorganisms 8, 639. doi: 10.3390/microorganisms8050639
Sornsenee, P., Chatatikun, M., Mitsuwan, W., Kongpol, K., Kooltheat, N., Sohbenalee, S., et al. (2021). Lyophilized cell-free supernatants of Lactobacillus isolates exhibited antibiofilm, antioxidant, and reduces nitric oxide activity in lipopolysaccharide- stimulated RAW 264.7 cells. PeerJ 9, e12586. doi: 10.7717/peerj.12586
Surendran Nair, M., Amalaradjou, M. A., and Venkitanarayanan, K. (2017). Antivirulence properties of probiotics in combating microbial pathogenesis. Adv. Appl. Microbiol. 98, 1–29. doi: 10.1016/bs.aambs.2016.12.001
Tatsaporn, T., and Kornkanok, K. (2020). Using potential lactic acid bacteria biofilms and their compounds to control biofilms of foodborne pathogens. Biotechnol. Rep. 26, e00477. doi: 10.1016/j.btre.2020.e00477
Tomé, A. R., Carvalho, F. M., Teixeira-Santos, R., Burmølle, M., Mergulhão, F. J. M., and Gomes, L. C. (2023). Use of probiotics to control biofilm formation in food industries. Antibiotics 12, 1–17. doi: 10.3390/antibiotics12040754
Truchado, P., Gil, M. I., Larrosa, M., and Allende, A. (2020). Detection and quantification methods for viable but non-culturable (VBNC) cells in process wash water of fresh-cut produce: industrial validation. Front. Microbiol. 11, 673. doi: 10.3389/fmicb.2020.00673
Tseng, Y. H., Barbosa, J., de Carvalho, T. B., and Teixeira, P. (2022). Microbiological safety of cut melons sold in portuguese retail markets: a pilot study. Foods 11, 4010. doi: 10.3390/foods11244010
Turgis, M., Vu, K. D., Dupont, C., and Lacroix, M. (2012). Combined antimicrobial effect of essential oils and bacteriocins against foodborne pathogens and food spoilage bacteria. Food Res. Int. 48, 696–702. doi: 10.1016/j.foodres.2012.06.016
Vieco-Saiz, N., Belguesmia, Y., Raspoet, R., Auclair, E., Gancel, F., Kempf, I., et al. (2019). Benefits and inputs from lactic acid bacteria and their bacteriocins as alternatives to antibiotic growth promoters during food-animal production. Front. Microbiol. 10, 57. doi: 10.3389/fmicb.2019.00057
Wang, C., Chang, T., Yang, H., and Cui, M. (2015). Antibacterial mechanism of lactic acid on physiological and morphological properties of Salmonella Enteritidis, Escherichia coli and Listeria monocytogenes. Food Control 47, 231–236. doi: 10.1016/j.foodcont.2014.06.034
Wijesundara, N. M., Lee, S. F., and Rupasinghe, H. V. (2022). Carvacrol inhibits Streptococcus pyogenes biofilms by suppressing the expression of genes associated with quorum-sensing and reducing cell surface hydrophobicity. Microb. Pathog. 169, 105684. doi: 10.1016/j.micpath.2022.105684
Zand, E., Froehling, A., Schoenher, C., Zunabovic-Pichler, M., Schlueter, O., and Jaeger, H. (2021). Potential of flow cytometric approaches for rapid microbial detection and characterization in the food industry-a review. Foods 10, 3112. doi: 10.3390/foods10123112
Keywords: biofilm control, carvacrol, pre-formed biofilm, probiotics, flow cytometry, food spoilage, foodborne pathogens, food packaging
Citation: Poscente V, Di Gregorio L, Costanzo M, Nobili C, Bernini R, Garavaglia L and Bevivino A (2023) Lactiplantibacillus plantarum monolayer enhanced bactericidal action of carvacrol: biofilm inhibition of viable foodborne pathogens and spoilage microorganisms. Front. Microbiol. 14:1296608. doi: 10.3389/fmicb.2023.1296608
Received: 18 September 2023; Accepted: 23 October 2023;
Published: 22 November 2023.
Edited by:
Lizziane Kretli Winkelströter, University of Western São Paulo, BrazilReviewed by:
Pantu Kumar Roy, Gyeongsang National University, Republic of KoreaAdriana Morar, Banat University of Agricultural Sciences and Veterinary Medicine, Romania
Copyright © 2023 Poscente, Di Gregorio, Costanzo, Nobili, Bernini, Garavaglia and Bevivino. This is an open-access article distributed under the terms of the Creative Commons Attribution License (CC BY). The use, distribution or reproduction in other forums is permitted, provided the original author(s) and the copyright owner(s) are credited and that the original publication in this journal is cited, in accordance with accepted academic practice. No use, distribution or reproduction is permitted which does not comply with these terms.
*Correspondence: Luciana Di Gregorio, luciana.digregorio@enea.it; Annamaria Bevivino, annamaria.bevivino@enea.it