- 1Laboratory of Plant Biotechnology “Biotechnologies Végétales”, Faculty of Sciences, University Ibn Zohr (UIZ), Agadir, Morocco
- 2Unité de Recherche Résistance Induite et Bio Protection des Plantes, EA 4707 – USC INRAe1488, UFR Sciences Exactes et Naturelles, Moulin de la Housse, University of Reims Champagne-Ardenne, Reims, France
- 3Integrated Crop Production Research Unit, Regional Center of Agricultural Research of Agadir, National Institute of Agricultural Research, Rabat, Morocco
Nowadays, sustainable agriculture approaches are based on the use of biofertilizers and biopesticides. Tomato (Solanum lycopersicum L.) rhizosphere could provide rhizobacteria with biofertilizing and biopesticide properties. In this study, bacteria from the rhizosphere of tomato were evaluated in vitro for plant growth promotion (PGP) properties. Five Pseudomonas isolates (PsT-04c, PsT-94s, PsT-116, PsT-124, and PsT-130) and one Bacillus isolate (BaT-68s), with the highest ability to solubilize tricalcium phosphate (TCP) were selected for further molecular identification and characterization. Isolates showed phosphate solubilization up to 195.42 μg mL−1. All isolates showed phosphate solubilization by organic acid production. The six isolates improved seed germination and showed effective root colonization when tomato seeds were coated with isolates at 106 cfu g−1 in axenic soil conditions. Furthermore, the selected isolates were tested for beneficial effects on tomato growth and nutrient status in greenhouse experiments with natural rock phosphate (RP). The results showed that inoculated tomato plants in the presence of RP have a higher shoot and root lengths and weights compared with the control. After 60 days, significant increases in plant Ca, Na, P, protein, and sugar contents were also observed in inoculated seedlings. In addition, inoculated tomato seedlings showed an increase in foliar chlorophyll a and b and total chlorophyll, while no significant changes were observed in chlorophyll fluorescence. In greenhouse, two Pseudomonas isolates, PsT-04c and PsT-130, showed ability to trigger induced systemic resistance in inoculated tomato seedlings when subsequently challenged by Clavibacter michiganensis subsp. michiganensis, the causal agent of tomato bacterial canker. High protection rate (75%) was concomitant to an increase in the resistance indicators: total soluble phenolic compounds, phenylalanine-ammonia lyase, and H2O2. The results strongly demonstrated the effectiveness of phosphate-solubilizing bacteria adapted to rhizosphere as biofertilizers for tomato crops and biopesticides by inducing systemic resistance to the causal agent of tomato bacterial canker disease.
1 Introduction
The exponentially growing world population, which is estimated to reach 9.5 billion by 2050, will imply more food needs and more demand of agricultural productivity, particularly vegetable crops. Tomato (Solanum lycopersicum L.) is one of the most consumed and economically important vegetable crops in the world with a world production of over 186 million tons (FAOSTAT 2022). Plant nutrition is one of the major concerns for modern agriculture. Nitrogen (N), phosphorus (P), and potassium (K) are major growth-limiting macronutrients for plant growth. Sustainable agricultural practices should be encouraged, and development of new biological tools is needed. Application of beneficial soil microbia, either alone or combined with natural nutrient sources such as rock phosphate, represent a promising strategy. On the other hand, tomato is attacked by the gram-positive bacterium Clavibacter michiganensis subsp. michiganensis (Cmm), which causes bacterial canker on tomato stem, leaves and fruits. The disease is considered the major threat for tomato cultivation all over the world, as it can cause economic losses that can reach up to 100% of production (Chang et al., 1992; Wang et al., 2022). Nowadays, two main strategies are commonly adopted for enhancement of plant growth and disease management: (i) development of new cultivars with enhanced growth and nutritional qualities and resistance to phytopathogens (ii) use of chemical fertilizers and pesticides. However, for tomato bacterial canker, no resistant cultivars are available, and no chemical treatments are efficient to combat this disease.
Phosphorus is the second most important nutrient for the plant growth after nitrogen, which plays a crucial role in energy transfer, photosynthesis, signal transduction, and respiration (Ham et al., 2018; Bouizgarne, 2022). It accounts for between 0.2 and 0.8% of the dry weight of plants, and adequate availability of this nutrient is required in many physiological and biochemical plant activities. Phosphorus exists in two forms in the soil, organic and inorganic phosphates. Insoluble forms of iron, aluminum, and calcium as aluminum phosphate (Al3PO4) and ferrous phosphate (Fe3PO4) exist in acidic soils and as calcium phosphate (Ca3PO4)2 in calcareous soils. Natural rock phosphate (RP) is composed of calcium hydroxyapatite [Ca10 (PO4)6 OH], with only small fractions of phosphate anions, H2PO4−, HPO42−, and PO43−. In addition, soluble forms are rapidly converted into insoluble complex forms with iron, aluminum, or calcium. All these mechanisms render phosphorus into an insoluble form which is not directly available for plant uptake (Adnan et al., 2020). This conversion is also responsible for the loss of approximately 75% of chemical P fertilizer, added to soils each year, which rapidly becomes unavailable for plants. Consequently, although abundant in natural soils, P-availability in the soil solution is often insufficient as most soils are poor in phosphorus, which is considered a limiting factor for plant nutrition (Bouizgarne, 2022). Its deficiency severely restricts plant growth and yields. Under a limited P-availability, plant root system becomes concentrated in the topsoil, resulting in inhibited elongation of primary root and stimulation of lateral root initiation. In the other hand, direct application of rock phosphate as fertilizer is not effective in most soils as plants could not extract phosphorus from these forms, making increasing phosphorus use efficiency a major challenge in intensive agricultural production systems.
This issue could be addressed efficiently by using soil microorganisms, particularly those living in the vicinity of the roots, also called plant growth-promoting rhizobacteria (PGPR) in an eco-environmental context. PGPR have attracted more attention during last decades as they have the potential to contribute to acceptable crop yields while preserving soil health. They are crucial in maintaining agricultural soil function because they contribute to soil processes such as stabilizing soil aggregates, improving soil structure, breakdown of organic matter, and cycling of nutrients such as carbon, nitrogen, phosphorus, and sulfur. In addition, they act by providing growth compounds to plants, helping plants to uptake soil nutrients, or preventing them from being infected by phytopathogens (Bouizgarne, 2013; Riaz et al., 2021; Zhang et al., 2021).
Among PGPR, bacteria that are able to solubilize insoluble phosphate forms, named phosphate solubilizing bacteria (PSB), dwell in most soils and potentially represent 40% of the culturable population (Richardson, 2001). PSB have been considered important for making soluble phosphorus forms available to plants by solubilizing insoluble inorganic phosphates or mineralizing organic phosphate compounds into soluble forms (Gyaneshwar et al., 2002; Rodríguez et al., 2006). They also prevent the released P from being immobilized again (Richardson et al., 2011). The most known mechanism by which PSB act is by generating organic acids that are able to solubilize complex mineral phosphates, including calcium phosphates in alkaline soils and RP (Alori et al., 2017; Bargaz et al., 2021). The mechanisms of PGPR-mediated enhancement of plant growth and yield of many crops are not yet fully understood. However, some PSB might actively contribute to the plant health by other mechanisms such as producing phytohormones or active substances against some specific plant pathogens or reducing ethylene synthesis (Bouizgarne, 2013). Bacterial strains belonging to genera Bacillus, Pseudomonas, and Serratia were reported for their ability to solubilize insoluble mineral phosphate compounds, such as dicalcium and tricalcium phosphate and rock phosphate (Ahmad et al., 2022; Bouizgarne et al., 2023). In particular, bacteria belonging to Pseudomonas species have frequently been isolated from the plant rhizosphere, and several have been reported as plant growth-promoting rhizobacteria (PGPR), considering their ability to increase P-availability in plants, thus helping to sustain crop health and productivity (Bouizgarne, 2013). In addition, the opportunity of improving the P uptake of crops by artificial inoculation with P-solubilizing rhizobacteria has been shown as an attractive plan for research (Azaroual et al., 2020).
The demand for chemical-free food is rising among consumers and sparked the research for environmentally safe techniques in order to reduce the reliance on chemicals. It is therefore recommended to search for alternative methods such as using phosphate solubilizing PGPR adapted to the rhizosphere of plant roots combined with natural rock phosphate in order to enhance the effectiveness of rock phosphate as fertilizer. The application of phosphate solubilizing bacterial strains from Moroccan rock phosphate, for tomato growth promotion has been reported (Bouizgarne et al., 2023). This is the first report of the use of Pseudomonas or Bacillus, combined with rock phosphate, for tomato growth promotion and protection against the causal agent of bacterial canker, Clavibacter michiganensis subsp. michiganensis, by improving host resistance mechanisms.
Thus, the present study aims (i) to screen for efficient phosphate, potash, and zinc solubilizing bacteria from tomato rhizosphere soil in addition to indole acetic acid (IAA) production, (ii) investigate the beneficial effects of their combination with natural rock phosphate (RP) on tomato growth and nutrient uptake in greenhouse experiments, and (iii) biocontrol of tomato bacterial canker caused by Cmm through triggering induced systemic resistance in inoculated tomato seedlings.
2 Materials and methods
2.1 Isolation of bacteria and screening for P, K, and Zn solubilizing activity
2.1.1 Screening for phosphate solubilizing bacteria
Bacterial strains were isolated from two soil areas in the Souss-Massa region, Morocco. Soil samples were collected from the root system of five healthy tomato plants. Soil dilutions were performed, and 0.1 mL was spread on the King B medium (per liter: proteose peptone 20 g; K2HPO4 1.5 g; MgSO4.7H2O 1.5 g; pH 7.2). After incubation at 28°C for 48 h, bacteria were stained with the Gram staining method and checked for cytochrome-C oxidase (oxidase test). Bacteria showing fluorescence under UV at 365 nm were considered as fluorescent pseudomonads. The capacity of isolates to solubilize inorganic phosphate was tested on National Botanical Research Institute’s phosphate (NBRIP) growth medium [per liter: 10 g glucose, 0.1 g (NH4)2SO4, 0.2 g KCl, 0.25 g MgSO4, 7H2O, 5 g MgCl2, 6H2O, and 5 g tri-calcium phosphate (TCP)] (Nautiyal, 1999). Appearance of halos around colonies reflected tri-calcium phosphate solubilization. The experiment was performed in four replicates. Halo diameter around colony and the colony diameter were measured, and the solubilization index (PSI) was calculated according to the following formula:
Quantitative estimation of phosphate solubilization was performed in liquid NBRIP medium; 20 mL of NBRIP containing TCP was inoculated with 100 μL of bacterial suspension from a 24-h preculture in King B medium (KB) at a final concentration of 106 cfu mL−1. The flasks were incubated at 28°C in the dark under shaking at 180 rpm in an incubator shaker (KS 3000 IC, IKA® Werke Staufen, Germany). After 48 h, phosphate solubilization was monitored by colorimetry in the culture supernatant at 600 nm, according to the Fiske and Subbarow (1925) method. A calibration curve was plotted using KH2PO4, and the pH variation was estimated by recording pH value at starting conditions and after 48 h of culture. The experiment was performed in six replicates, and the amount of P in the medium was expressed as mg L−1.
2.1.2 Identification of organic acid by HPLC
High-performance liquid chromatography (HPLC) (Ultimate 3000 Dionex), coupled with mass spectrometry (Exactive Plus de Thermo Scientific) and equipped with a BDS Hypersil C18 (150 × 4.6 mm × 5 μm) HPLC Column, was used to identify and quantify organic acids. Flasks containing 20 mL of liquid NBRIP with TCP as P source were inoculated with 100 μL of standardized bacterial suspension at a final concentration of 106 cfu mL−1 and incubated at 28°C in the dark under shaking at 180 rpm. After 48 h, samples were collected, filtered through a 0.22 μm cellulose membrane, and injected into the chromatographic column. Detection of organic acids was performed using the following organic acids (Supelco/Sigma–Aldrich) as analytical standards with typical retention time means as follows: 2-ketogluconic acid (2.10 min), phytic acid (3.25 min), gluconic acid (3.75 min), tartaric acid (3.93 min), quinic acid (4.03 min), oxalic acid (4.25 min), succinic acid (4.85 min), DL-iso-citric acid (4.86 min), ascorbic acid (5.05 min), maleic acid (5.56 min), citric acid (7.51 min), fumaric acid (8.10 min), shikimic acid (8.71 min), propionic acid (9.00 min), malonic acid (9.27 min), and benzoic acid (12.5 min). The mobile phase was 0.1% H3PO4 (pH 1.81) with a flow rate of 0.5 mL min−1 and a 100 μL injection per sample, according to Marra et al. (2015). The acquisition time of the chromatograms was estimated to be 30 min with 30 min of intervals between runs. Detection was performed by UV at 210 nm with a diode array detector (DAD).
2.1.3 Screening for potassium solubilization
Potassium solubilization was carried out by bacterial isolates on Aleksandrow agar medium (Rajawat et al., 2014) containing 5.0 g/L glucose, 0.5 g/L MgSO4, 7H2O, 0.1 g/L CaCO3, 0.006 g/L FeCl3, 2.0 g/L Na2 HPO4, and feldspar (2.0 g/L) as sole K source. The plates were then incubated for 2 days at 28°C. Appearance of yellow halos around colonies reflected potassium solubilization. The experiment was performed in four replicates. Halo diameter around each colony and the colony diameter were measured. Potassium mineral solubilizing index (KSI) was calculated as follows:
2.1.4 Screening for zinc solubilization
The zinc solubilization was investigated in Bunt and Rovira medium (Bunt and Rovira, 1955) (glucose: 20.0 g, peptone: 1.0 g; yeast extract: 1.0 g, (NH4)2 SO4: 0.50 g; K2 HPO4: 0.40 g; MgCl2: 0.10 g, FeCl3: 0.01 g, distilled water 1.000 mL, and adjusted pH 6.7) containing 0.1% insoluble zinc oxide. Fresh cultures of bacteria were spot inoculated at the center of the plate in triplicates with toothpicks and incubated for 72 h in the dark at 26°C. The experiment was performed in four replicates. The appearance of halo zones around colonies reflected zinc solubilization. Halo diameter around each colony and the colony diameter were measured. The zinc solubilizing index (ZSI) was calculated as follows:
2.2 Screening for indole-3-acetic-acid production
Production of IAA was demonstrated according to Bric et al. (1991). Isolates were cultivated in Luria–Bertani (LB) medium (per liter: tryptone 10 g; yeast extract 5 g; NaCl 5 g; pH 7.2) and incubated at 28°C for 2 days, and then, positive colonies were revealed by Salkowski reagent. For quantitative measurement, liquid LB medium containing 0.1 g/L of L-Trp was seeded with isolates and incubated for 3 days at 28°C. Amount of IAA was determined according to Gravel et al. (2007). A calibration curve was obtained by synthetic IAA (Sigma–Aldrich). The experiment was performed in six replicates, and amounts of IAA were expressed in μg mL−1.
2.3 Effect of bacterial isolates on tomato seed germination
Bacterial isolates were cultivated for 48 h days in King B broth and incubated at 28°C on a rotating shaker at 180 rpm in an incubator shaker (KS 3000 IC, IKA® Werke Staufen/Germany). Tomato seeds (cultivar calvi) were surface sterilized using 2% sodium hypochlorite and subsequently washed with distilled water. Then, 50 tomato seeds were placed in sterile Petri dishes containing wet sterile paper filter. Seeds were coated with 1 mL of a mixture containing culture suspension from each of the six bacteria at a concentration of 106 cfu mL−1, 0.5% carboxymethyl cellulose (CMC), and 0.1% RP. The composition of RP was evaluated by using scanning electron microscopy (SEM–EDX, JSM IT-100, JEOL, USA) as follows: C: 7.1%, O: 48.6%, F: 11.0%, Na: 1.8%, Al: 1.7%; Si: 3.5%, P: 8.9%, and Ca: 17.1%. RP contains particles with an average size of 377 μm. pH of H2O (1.1) was 6.86 and electrical conductivity was 488 mmho cm−1. It contains approximately 5% total phosphorus and low amount of assimilable phosphorus (P-Olsen) (P2O5 < 18 mg/Kg). It contains low amount of organic carbon (0.23%) and organic matter (0.40%) and 99.5% of mineral matter with 5.72% total limestone. The C/N ratio was 3.55. RP contains low amount of nitrogen (0.012%), calcium (CaO) 1.93 g/kg, and potassium (0.01 g/kg). RP magnesium (MgO) content was 0.34 g/kg. The mixture was added to seeds under shaking condition until a fine coating appeared on seeds. The plates were placed in a germination chamber at 28 ± 2°C for 12 days. Germinated seeds of negative control were coated with bacteria-free CMC. The percent of germinated seeds was determined after 15 days (International Seed Testing Association, 2009). The experiment was performed with three replicates.
2.4 Greenhouse experiments
2.4.1 Rhizosphere colonization by selected bacteria
To investigate the ability of the selected isolates to colonize the roots of tomato plants, six tomato seeds (cv. calvi) were first surface disinfected with 2.5% sodium hypochlorite solution for 3 min and then coated with 1 mL of a mixture containing culture suspension from each of six bacteria at a concentration of 106 cfu mL−1, 0.5% carboxymethyl cellulose (CMC), and 0.1% RP and germinated in Petri dishes. After germination, seedlings were transferred in a mixture of soil and peat (2,1 w,w) and cultured in axenic conditions. Soil characteristics were as follows: silt (6.70%), clay (4.15%), sand (87.32%), pH (H2O): 8.57, and electrical conductivity (EC) at 25°C (0.28 mS cm−1). Water retention capacity was 30.71%, and total organic carbon was 0.4%. Rhizosphere colonization was determined after 15 days by uprooting seedlings and removing root adhering soil by washing three times. The homogenate was then stirred in 9 mL sterile water for 5 min. Then, serial decimal dilutions were carried out in sterile physiological water. Overall, 0.1 mL of each dilution was spread on King B agar medium and incubated at 28°C for 24 h, and the growth of the bacterial isolates was estimated by counting colonies. Rhizosphere colonization was expressed as colony forming unit (cfu) g−1 soil. Each experiment was performed in triplicate.
2.4.2 Effects on plant growth parameters
In addition, after germination, seedlings were transferred to disinfected pots (one seedling per pot) containing 5 kg of a mixture of soil and peat (2,1 w,w) amended with RP at 1 g/kg mixture. Plants were placed in an experimental greenhouse where the average temperature was 35°C during the day and 10–18°C at night, a photoperiod of 16 h day/8 h night, and an average relative humidity of 67.5%. Seedlings were watered daily with sterilized water. To check for the ability of bacteria to promote the growth in tomato, the following experiments were carried out: (i) control seedlings: tomato seedlings were grown in the mixture of soil and peat and amended with RP (1 g/kg) as a source of phosphorus and (ii) bacterized seedlings: tomato seedlings were grown in the mixture of soil and peat and amended with RP (1 g/kg) and inoculated at plant rhizosphere with 20 mL of bacterial suspension from each selected isolate at 106 mL−1. Each month, the treatment with the bacteria at plant rhizosphere was renewed. Thirty seedlings were used for each experiment in three replicates. Tomato plants were harvested after 60 days, and then, the length of the seedling shoots and roots and the shoot and root fresh and dry weight were measured. In addition, the following physiological parameters were measured:
2.4.3 Effects on chlorophyll content and chlorophyll fluorescence
The effect of the different treatments on total chlorophyll content was measured in youngest fully expanded leaves, which were detached from tomato plants immediately after harvesting. Measurements were performed according to Arnon (1949). Four repetitions were performed per treatment, and chlorophyll contents were expressed in mg g−1 DM. The chlorophyll fluorescence (Fv/Fm) (Maxwell and Johnson, 2000) was measured using a portable fluorometer (Handy PEA fluorimeter, Hansatech instruments, Norfolk, UK). This parameter was measured on well-developed leaves of the same rank (seven repetitions per treatment). Clamps were placed on the upper side of the leaves for 20 min, to keep the leaves in the dark before measurement.
2.4.4 Effects on proteins and total soluble sugars
The extract was prepared by mixing 100 mg of the aerial part (stem+ leaves) of each plant sample with 4 mL of 0.1 M phosphate buffer pH 6 containing 5% insoluble polyvinylpyrrolidone and 0.1 mM ethylenediaminetetraacetic acid (EDTA). The protein content in the supernatant was measured according to Bradford (1976). Total soluble sugar was determined according to Dubois et al. (1956). The results were expressed in mg g−1 of fresh matter.
2.4.5 Effects on mineral elements contents
Sodium (Na+), potassium (K+), calcium (Ca2+), and phosphorus (P) were measured after mineralization of the plant shoot and root material. Sodium (Na+), potassium (K+), and calcium (Ca2+) cations were measured by flame photometer PFP7 (Jenway, United Kingdom), according to Tuna et al. (2007). To measure phosphorus in leaves, the phospho-molybdate blue method was used (Olsen and Sommers, 1982). In brief, an aliquot of the solution (1 mL) was added to 5 mL of the following reagent mixture: ammonium molybdate (2.5%), hydrazine sulfate (0.15%), and 4 mL of distilled water. After heating in a water bath at 70°C for 10 min and cooling for 5 min, optical density of the samples was measured at λ = 820 nm with a UV/VIS spectrophotometer (UV-3100PC, VWR®, United States).
2.5 Biocontrol of tomato bacterial canker by induced systemic resistance
2.5.1 Ability of bacteria to grow inside root tissues and on root exudates
Tomato seedlings were used to test the growth of six bacterial isolates, PsT-04c, PsT-94s, BaT-86s, PsT-116, PsT-124, and PsT-130, on root tissues and crude root exudates. Tomato seedlings (15 days old) were removed carefully from pots containing the mixture of soil and peat and washed three times with sterilized water. The roots were carefully cleaned with cotton soaked in alcohol, surface disinfected with 0.4% sodium hypochlorite, and rinsed extensively with sterile deionized water. 10 μL of each bacterial suspension at a concentration of 106 bacteria mL−1 was injected into the main roots of six tomato seedlings by using a Hamilton micro syringe. Seedlings were then transferred to aseptic flasks containing 450 mL of sterile distilled water, inoculated with 106 mL−1 of each of the six bacteria, and hydroponically grown in a growth chamber at 25 ± 2°C in natural light with 16-h photoperiod for 15 days. The control consisted of six seedlings injected only with sterile water and transferred to uninoculated flasks under the same conditions. To check for the ability of isolates to grow in root tissues, after 15 days, roots were ground in a mortar at 4°C; 1 g of the homogenate was stirred in 9 mL of sterile water for 5 min. Then, serial decimal dilutions were carried out in sterile physiological water. In total, 0.1 mL of each dilution was spread on nutrient agar plates and amended with cycloheximide (40 μg mL−1) to inhibit the fungal growth. Three replicates were carried out by dilution. The agar plates were then incubated at 28°C for 48 h. Colonies of bacteria grown from root extracts were identified by their phenotypic characteristics, and representative colonies were identified by 16S rRNA sequencing. Colonies were counted, and their growth was estimated as colony forming units (cfu) per gram roots.
For growth on root exudates, exudates from control seedlings were extracted and sterilized by filtration using a 0.22 μm Millipore filter. In total, 20 mL exudates were added to 80 mL sterilized distilled water and 1.5 g agar in order to prepare a solid medium (exudates agar). For each isolate, a serial dilution of non-sterilized exudate was performed, and 0.1 mL of each dilution was spread on the exudate agar, amended with cycloheximide, and incubated for 48 h at 28°C. Control consisted of root exudate-free medium. Three replicates were performed for each isolate. Colonies of bacteria grown on root exudates were counted, roots were weighed, and growth was estimated as colony forming units (cfu) per gram roots. No growth was recorded in the control without root exudates.
2.5.2 Ability of bacteria to protect tomato against Cmm and induce systemic resistance
2.5.2.1 Bacterial strains: Pseudomonas strains and the pathogen Cmm
A virulent strain of Clavibacter michiganensis subsp. michiganensis (Cmm) and the two selected Pseudomonas isolates, PsT-04c and PsT-130, were used for biocontrol experiments. Cmm colonies were cultivated on a slightly modified BCT medium (Ftayeh et al., 2011) containing per liter of deionized water: 2.5 g mannitol, 2.0 g yeast extract, 1.0 g K2HPO4, 0.1 g KH2PO4, 0.05 g NaCl, 0.1 g MgSO4. 7H2O, 0.015 g MnSO4. H2O, 0.015 g FeSO4. 7H2O, 0.6 g H3BO3 (pH 7.1), and 15 g agar. After autoclaving and cooling at 50°C under stirring, nalidixic acid (20 mg/L), trimethoprim (100 mg/L), and 50 μL of a solution of penconazole (80 g/L) were added to the medium. To confirm the pathogenicity of the Cmm isolate, tomato seedlings were infected with a suspension of Cmm at 106 bacteria mL−1 by depositing 50 μL droplets on the surface of the leaves and injecting 10 μL droplets into roots. After 15–20 days, Cmm was reisolated from infected seedlings, showing symptoms of bacterial canker.
2.5.2.2 Protection of tomato seedlings against bacterial canker
For biocontrol experiments in greenhouse experiments, 1-month-old tomato seedlings obtained from seed germination were treated by either Cmm or Pseudomonas + Cmm and transplanted into the mixture containing soil and peat (2: 1). Treatments were carried out as follows:
• Negative control: seedlings for which roots were soaked only in sterilized water for 8 h and transplanted into pots.
• Positive control: seedlings infected by soaking in 10 mL of Cmm suspension at 106 bacteria mL−1 for 8 h and transplanted into pots.
• Bacterial treatment: seedlings for which roots have been soaked in a 10 mL suspension of each of the two Pseudomonas strains PsT-04c and PsT-130 and subsequently infected by Cmm. Treatments were performed as follows: The first treatment was carried out with Pseudomonas (1st immunization) by soaking seedlings in 10 mL of each Pseudomonas isolate at 106 bacteria mL−1 for 8 h before transplanted into pots. After 1 week, seedlings were infected by inoculating 10 mL of Cmm suspension at 106 bacteria mL−1 at root level. Finally, after 20 days, the second bacterial treatment, by inoculating 10 mL of each of the two Pseudomonas isolates at 106 bacteria mL−1 at root level, was performed (2nd immunization).
Each treatment consisted of 32 seedlings arranged in a complete random block. After 120 days of culture in greenhouse, symptoms were observed, and the mortality rate was calculated as follows:
In addition, the following parameters were measured:
2.5.2.3 Total soluble phenolic compounds
After 1 month of the second bacterization, control tomato seedlings, Cmm-infected seedlings, and seedlings treated with the two isolates PsT-04c and PsT-130 were used for the evaluation of the root-soluble phenolic compound contents, which is carried out according to the protocol described by Macheix et al. (1990). Roots (200 mg) were ground in an ice bath with 2 mL of 80% methanol before stirring for 15 min and centrifugation at 7,000 g for 3 min. The extraction operation was repeated three times, and the recovered supernatants were stored in the freezer (−20°C). The supernatants were used for quantitative determination of the total soluble phenolic compounds by the Folin–Ciocalteu reagent. The reaction mixture consisted of 50 μL of phenolic extracts diluted in 2 mL of distilled water and 0.5 mL of the Folin–Ciocalteu reagent (10%, v/v). After shaking, 0.5 mL of a Na2CO3 solution (20%) was added, and the mixture was incubated at 40°C for 30 min. For the colorimetric measurement, blank was prepared with 0.5 mL of Folin–Ciocalteu reagent (10%, v/v) and 0.5 mL of 20% Na2CO3. The results were read on a UV/VIS spectrophotometer (UV-3100PC, VWR®, United States) at 765 nm. The experiment was performed with three replicates, and total soluble phenolic contents were expressed in mg g−1 of fresh material by using a standard curve of caffeic acid.
2.5.2.4 Phenylalanine ammonia-lyase activity
After 1 month of the second bacterization, the phenylalanine ammonia-lyase activity was determined according to Mozzetti et al. (1995). Tomato roots were ground in 100 mmol/L potassium borate buffer, pH 8.8, with 14 mmol/L 2-mercaptoethanol. The homogenate was then centrifuged at 13,000 g for 30 min, and 100 μL of the supernatant (enzymatic extract) was added to 1 mL of 100 mmol/L potassium borate buffer, pH 8.8, with 200 μL of 100 mmol/L L-phenylalanine. After incubation at 40°C for 60 min, the reaction was stopped by the addition of 250 μL of 5 N HCl, and the absorbance was determined at 290 nm. Cinnamic acid was used to perform a standard curve, and the protein content was determined according to Bradford (1976). The experiment was performed with three replicates, and L-phenylalanine was expressed as μg cinnamic acid mL−1 enzymatic extract.
2.5.2.5 Hydrogen peroxide (H2O2) content
One month after the second bacterization, the H2O2 assay was performed according to Velikova et al. (2000). The fresh plant material (500 mg) was homogenized in 2 mL of 1 g/L solution of trichloroacetic acid (TCA). After centrifugation at 10,000 g for 15 min, 0.5 mL of the supernatant was added to the reaction mixture containing 0.5 mL of 10 mM potassium phosphate buffer (pH 7) and 1 mL of a 1 M solution of KI. The absorbance was measured at 390 nm. The experiment was performed with three replicates, and the H2O2 content was expressed in μg g−1 of fresh weight using a standard curve of H2O2, which was established under the same conditions.
2.6 Identification of bacterial isolates
The characterization of bacterial isolates was performed by observing phenotypic traits such as colony morphology, Gram staining, and fluorescence under UV light (254 nm). Furthermore, oxidase, tween, and Levan tests were assayed according to the methods described by Lelliott et al. (1966). Other traits were determined by using API 20NE strips (Biomérieux-France). All tests were performed in triplicate. Molecular identification of the six isolates was performed by using 16S rRNA gene sequences as follows: the selected bacteria were grown on King B broth on a rotary shaker at 180 rpm in an incubator shaker KS 3000 IC (IKA® Werke Staufen/Germany) at 28°C for 48 h. After centrifugation, total DNA was extracted with the “MagPurix Bacterial DNA extraction kit” using the MagPurix extractor robot. Purified bacterial DNA was estimated by Nanodrop 8,000 (Thermo Fisher Scientific, USA). The bacterial 16S rRNA gene was amplified by using the universal 16S rRNA primers FD1 (5′- AGAGTTTGATCCTGGCTCAG-3′) and RP2 (5’-TACGGCTACCTTGTTACGACTT- 3′) (Weisburg et al., 1991). The PCR was carried out by using an ABI “Veriti, Applied Biosystems™” thermal cycler in a mixture containing 10 μL of PCR Master Mix (2X) (HS MyTaq DNA polymerase kit from Bioline), 1.5 μL of each primer, 130 ng of DNA, and purified water in a final volume of 25 μL, and the PCR program is as follows: 95°C, 2 min; (95°C, 30 s; 52°C, 30 s; and 72°C, 30 s) 35x; 72°C, 3 min. In total, 8 μL of PCR products was deposited on 1% agarose gel in the presence of the ladder of 1 kb molecular weight, and the gel was visualized by the “G Box” documentation system. Cleaned PCR products were used as a template for the cycle sequencing. Sequencing was performed using the 3130XL Dye Terminator Cycle Sequencing (DTCS) Quick Start kit (Applied Biosystems). The thermocycling conditions were as follows: 25 cycles of 96°C for 1 min, 96°C for 10 s, 50°C for 5 s, and 60°C for 4 min, followed by a 4°C infinite hold. The Sephadex G50 superfine (Sigma–Aldrich) was used to remove unincorporated dye terminators by washing the column with 300 μL of distilled water and centrifuging at 1,500 g for 3 min before applying the sample to the column. The sequencing reaction was performed using the forward and reverse primers FD1 and RP2. Forward and reverse sequencing were performed using Big Dye Terminator version 3.1 cycle sequencing kit (Applied Biosystems, Foster City, CA). Sequence trimming and assembly were performed using DNA baser. Then, the sequences were submitted to Basic Local Alignment Search Tool (BLAST) (Altschul et al., 1990) and ClustalW was used for multiple alignment with available 16S rRNA sequences in GenBank (National Center for Biotechnology Information NCBI, Bethesda, MD, USA). Phylogenic relationships were performed by Mega 11 software (Stecher et al., 2020; Tamura et al., 2021). The phylogenetic tree was built using the neighbor-joining method (Saitou and Nei, 1987) with statistic method of maximum likelihood, taking into account the correction of Jukes and Cantor (1969). Tree topology was evaluated by bootstrap analyses (1,000 replicates) (Felsenstein, 1985). The cutoff for species identification was 98.8%. The sequence data were deposited at the GenBank database under the following accession numbers PsT-04c (OP677775), PsT-94s (OP677776), PsT-116 (OP677777), PsT-124 (OP677861), PsT-130 (OP677782), and BaT-68s (OP677783).
2.7 Data analysis
Values are means and standard deviation of three or more than three replicates. Statistical analyses were conducted using SPSS software package version 22 for Microsoft Windows. The data obtained were examined through analysis of variance (ANOVA), to test the statistical differences. Means were compared at the 5% significance level using Tukey’s post-hoc test, to detect statistically significant differences between treatments. Principal component analysis (PCA) was performed using GraphPad Prism 9 software.
3 Results
3.1 Isolation of bacteria and screening for P, K, and Zn solubilizing activity and IAA production
In the present study, we screened isolated bacteria for effective phosphate solubilization, and we investigated in vitro PGP traits and their potential as tomato plant growth promoters. The primary isolation of bacteria from tomato rhizosphere soil was performed on King B medium. In total, 100 different bacterial strains were isolated. Among these bacteria, those which developed yellow-green fluorescent pigment under UV light (365 nm) are putatively considered, belonging to the fluorescent pseudomonads. The isolates were further screened for phosphate solubilizing ability. Out of 100 isolates tested on NBRIP agar medium, 16 isolates (PsT-04c, PsT-11b, PsT-35b, PsT-63k, BaT-68s, PsT-77, PsT-82k, PsT-88k, BaT-91k, PsT-92k, PsT-94s, PsT-96k, PsT-98k, PsT-116, PsT-124, and PsT-130) were able to solubilize phosphate on NBRIP (Table 1). These isolates exhibited clear halo zones on NBRIP after 24–72 h of incubation at 28°C (Figure 1). In NBRIP broth, the growth of these isolates was accompanied with increased amount of released P ranging from 73.22 to 195.42 mg mL−1. The maximum amount was showed by isolate PsT-94s, while the lowest amount was detected in isolate PsT-63k. All isolates lowered pH of the medium compared with control. All isolates brought the pH value of the medium below 6. The pH of the medium at initial conditions ranged between 6.7 and 6.9 and dropped to 3.22 as the lowest value for the isolate PsT-130 after 48 h, suggesting acidification as the main mechanism for solubilization. Organic acids were detected by HPLC/MS in NBRIP cultures of six bacterial isolates (Figure 2 and Supplementary Figure S1), showing highest phosphate solubilization (isolates PsT-04c, BaT-68s, PsT-94s, PsT-116, PsT-124, and PsT-130). Retention times for all isolates were compared with those of standards. The main organic acids detected were gluconic acid, maleic acid, and citric acid (Figure 2). The rest of peaks were not determined in this investigation.
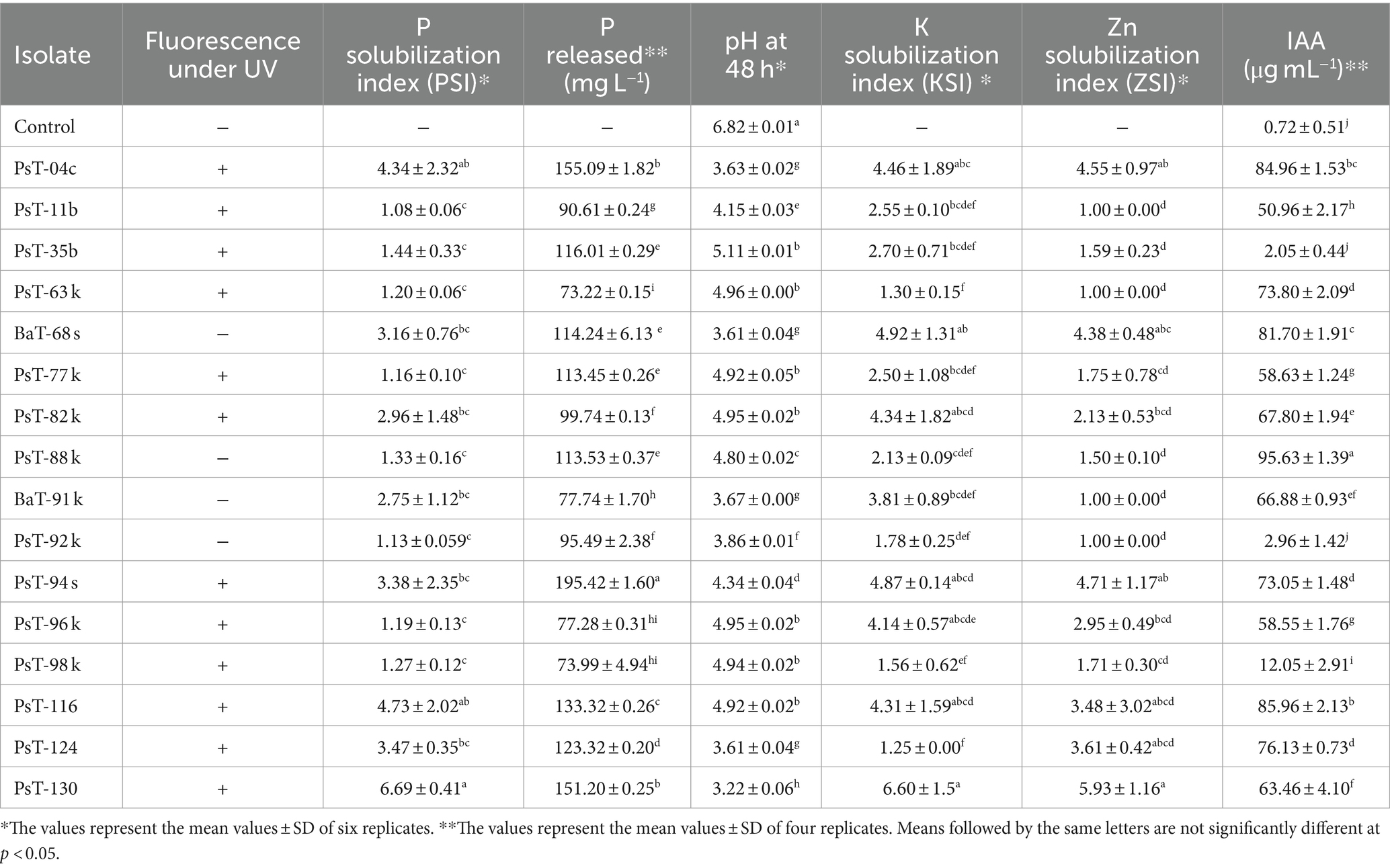
Table 1. Fluorescence under UV, phosphate, potash, and zinc solubilization and IAA production by 16 bacterial isolates.
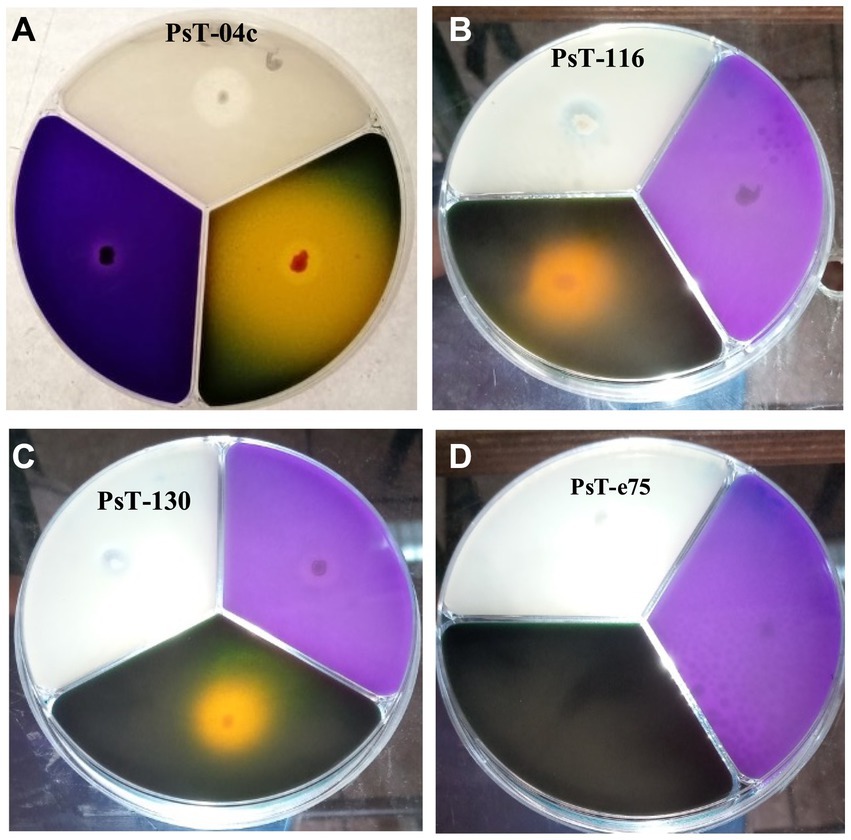
Figure 1. Solubilization of phosphorus, potassium, and zinc insoluble forms on NBRIP (purple medium), Alexandrov (green medium), and Bunt and Rovira media (translucid medium) respectively by three solubilizing strains PsT-04c (A), PsT-116 (B), and PsT-130 (C) compared with a non- solubilizing strain PsT-e75 (D).
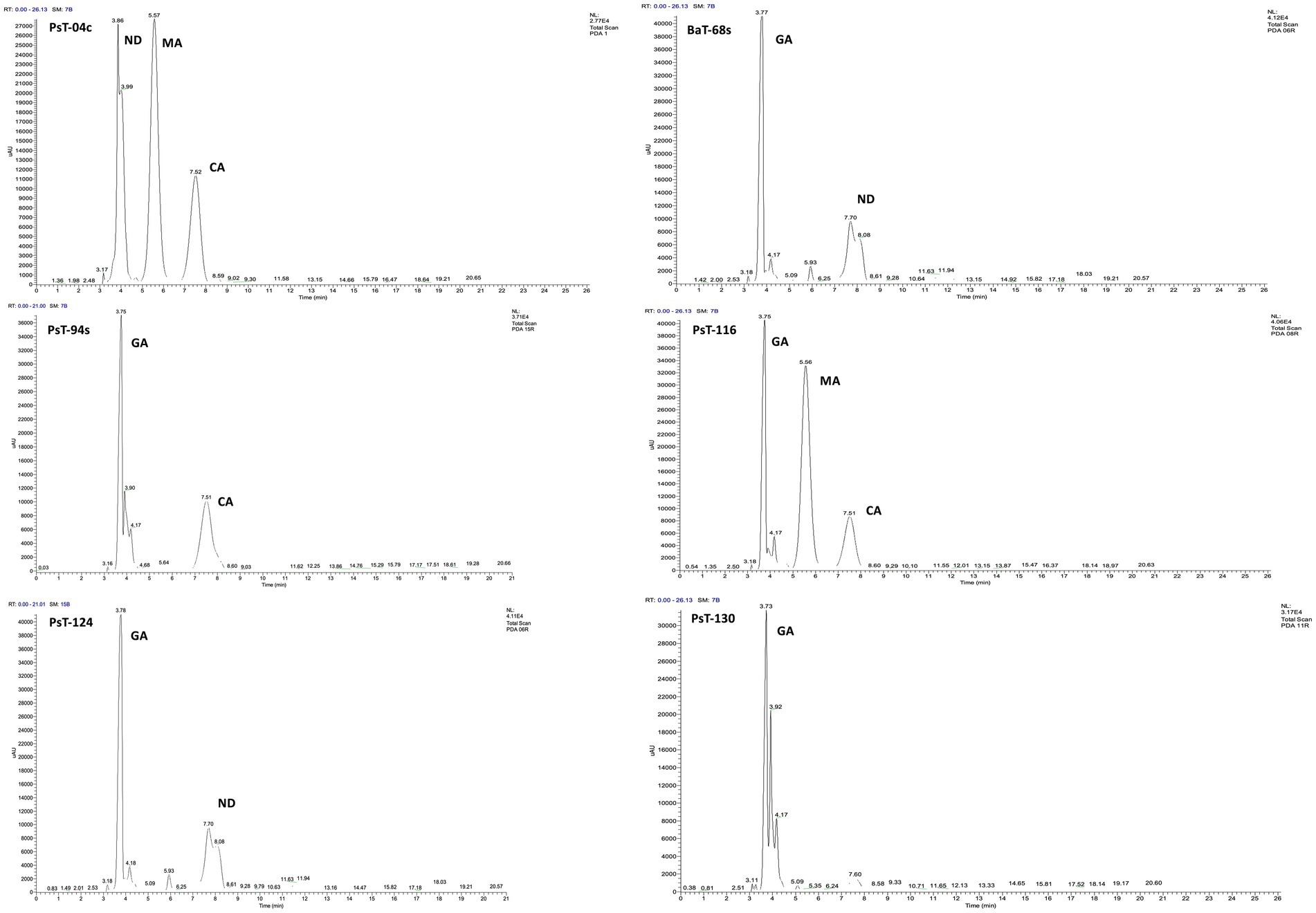
Figure 2. C18-HPLC profiles of the organic acids secreted by the six selected bacterial isolates PsT-04c, BaT-68s, PsT-94s, PsT-116, PsT-124, and PsT-130. GA, gluconic acid; CA, citric acid; MA, maleic acid; ND, not determined.
Most of the selected bacterial isolates for phosphate solubilizing ability also solubilized insoluble forms of potash and zinc and produced IAA (Figure 1 and Table 1). The isolates PsT-11b, PsT-63k, BaT-91k, and PsT-92k did not solubilize insoluble form of zinc. Isolates PsT-04c, PsT-94s, BaT-68s, and PsT-130 showed the highest potassium solubilization with index values of 4.46, 4.87, 4.92, and 6.60, respectively. The selected bacterial isolates showed significant levels of hormone IAA production (Table 1). The IAA amounts exceeded 80 μg mL−1 for several isolates. The six isolates PsT-88 k, PsT-116, PsT-04c, BaT-68s, PsT-124, PsT-63 k, and PsT-94s produced 95.63, 85.96, 84.96, 81.70, 76.13, 73.80, and 73.05 μg mL−1 of IAA, respectively, while only less amount was produced by isolates PsT-98 k (12.05 μg mL−1), PsT-92 k (2.96 μg mL−1), and PsT-35b (2.05 μg mL−1).
3.2 Plant growth-promoting effects on tomato
3.2.1 Effects on growth parameters
The six bacterial isolates, which showed the highest phosphate solubilization and IAA production abilities (isolates PsT-04c, BaT-68s, PsT-94s, PsT-116, PsT-124, and PsT-130), were selected for further experiments in order to test their abilities to show positive effects on seed germination and successfully colonize tomato rhizosphere (Table 2). The results showed that the germination rate of the seeds was improved in the presence of the isolates. Results of the effect of selected isolates combined with RP on seed germination showed that all treatments with bacterial isolates and RP had a significant positive effect on the germination rate at p < 0.05 compared with the controls consisting of seeds treated only with RP (RP amended, bacteria-free seeds). The maximum increase was obtained by the isolates PsT-04c and PsT-130 (Table 2). Greenhouse experiments were conducted by using these six isolates, and shoot and root length and fresh and dry weight were measured at the end of the experiment (Table 3). The maximum effect on shoot length and weight was showed by the isolates PsT-04c, BaT-68s, and PsT-94s, while the maximum positive effect on roots was showed by the isolates PsT-116 and BaT-68s. The results showed that the shoot length was increased by 45.51 and 43.25 % when seedlings were treated by the isolates PsT-04c and PsT-94s, respectively, while the root length reached up to 54.52% by the isolate PsT-116. Shoot and root fresh weight increased up to 63.68 and 72.96 %, respectively, while dry weight increased by 56.26 and 78.13%, respectively.
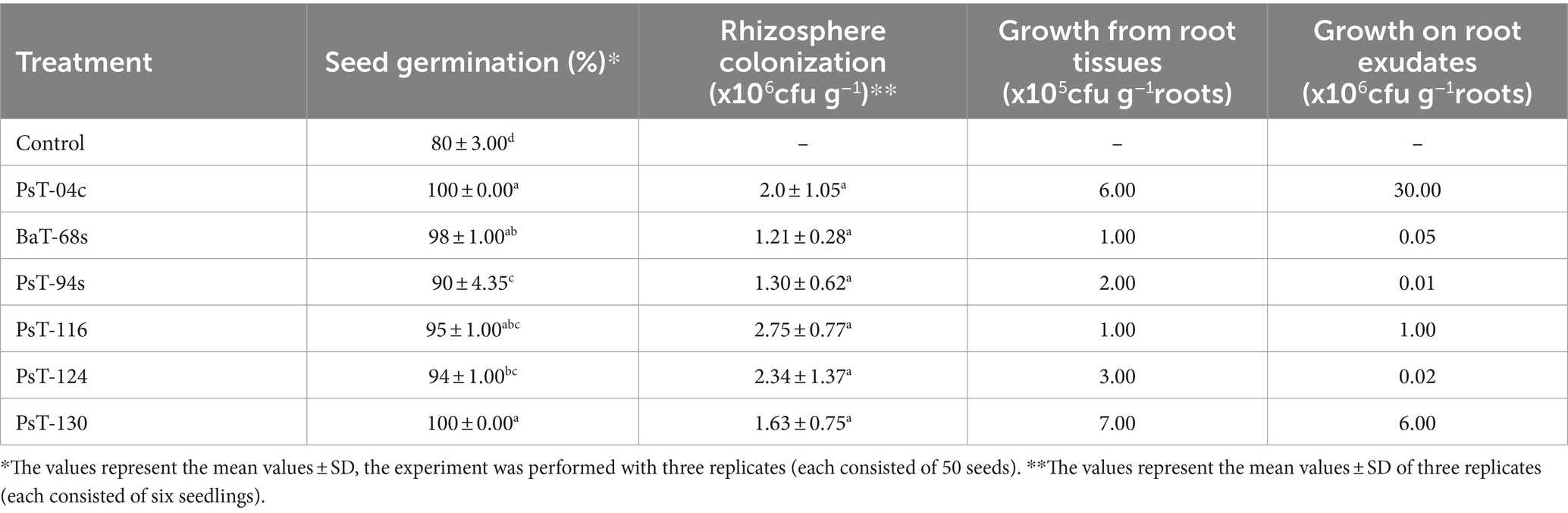
Table 2. Seed germination, rhizosphere colonization, growth from root tissues, and on root exudates displayed by the six bacterial isolates.
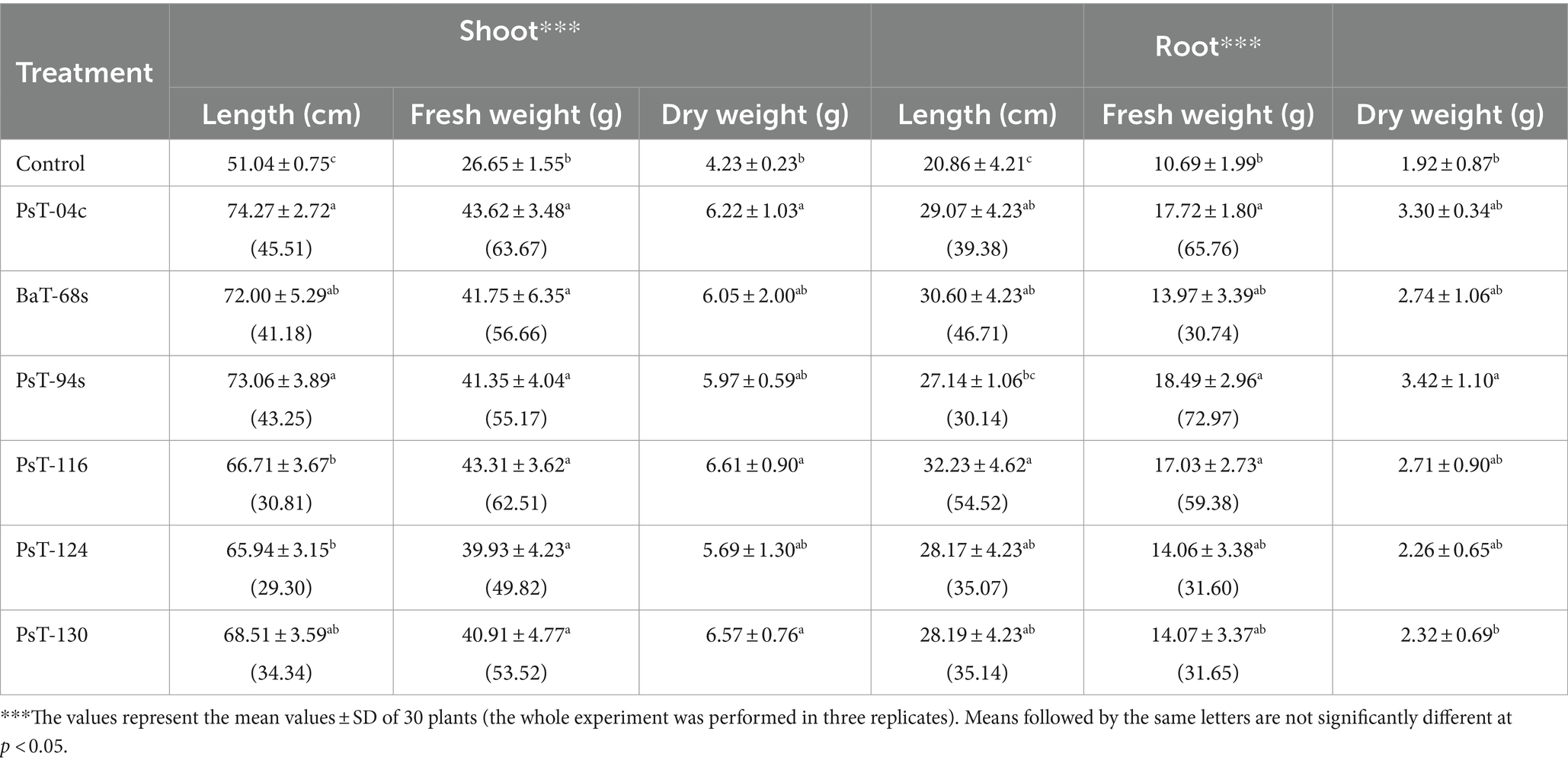
Table 3. Plant growth promotion displayed by selected bacterial isolates: on tomato (calvi cv.): effects on shoot and root length and fresh and dry weight, percentage between parenthesis correspond to increase (%) in shoot and root length and fresh weight.
3.2.2 Effects on mineral, sugars, proteins, and chlorophyll contents and chlorophyll fluorescence
The bacterial isolates were further investigated for their abilities to enhance nutrient contents of protein, sugars, calcium (Ca), sodium (Na), potassium (K), phosphorus (P), and chlorophyll. Sugar contents enhanced by up to three-fold with the isolate PsT-116, while protein contents enhanced by 47.77, 46.90, and 45.55%, respectively, by isolates PsT-04c, PsT-94s, and PsT-130 (Figure 3). In comparison to the control, all bacterial isolates showed significant increases in K, Ca, and Na contents of the shoot and root, with the maximum increase by isolate BaT-68s for root K, and PsT-94s for root Na and Ca (Table 4). The maximum amounts of shoot Ca and K were detected following treatment with strain PsT-124 while the maximum amount of Na was obtained by isolates PsT-124 and PsT-130. The results also showed a significant increase in P content in leaves by up to three-fold in seedlings treated with PsT-94s, in comparison to non-inoculated control. Total chlorophyll content increased by up to 43–47% in seedlings treated with the isolates PsT-04c and PsT-94s, respectively, while Chl a and Chl b increased by 40 and 55%, following treatment by the isolate PsT-94s. The maximum increases in Chl a and Chl b were shown respectively by the isolate BaT-68s (47%) and PsT-04c (76%). No significant changes in chlorophyll fluorescence (photosynthetic quantum yield: Fv/Fm) were observed after 60 days of culture (Table 5).
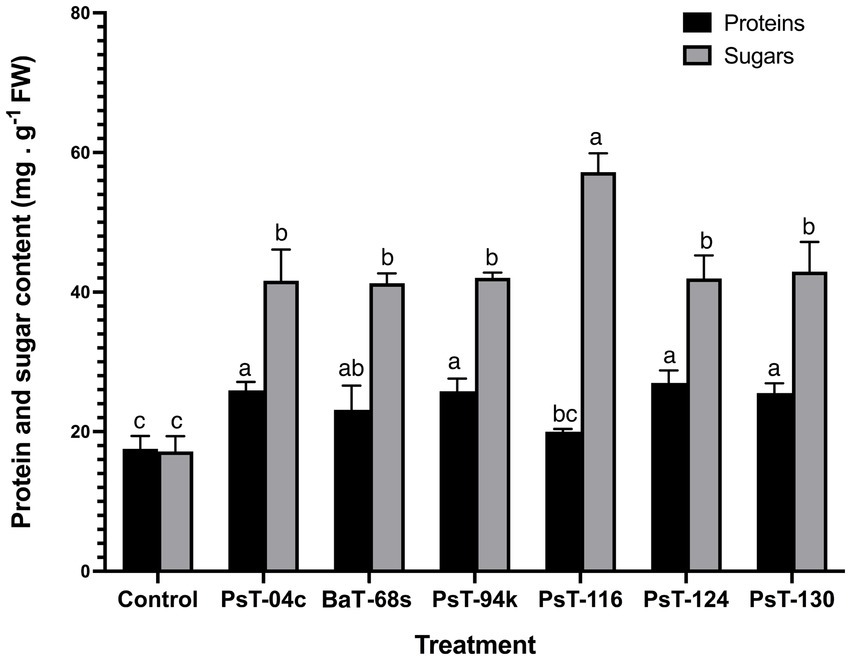
Figure 3. Effect of treatments by selected bacterial isolates on protein and sugar contents of tomato seedlings after 60 days. Different letters above the bars indicate the differences are significant at P < 0.05.
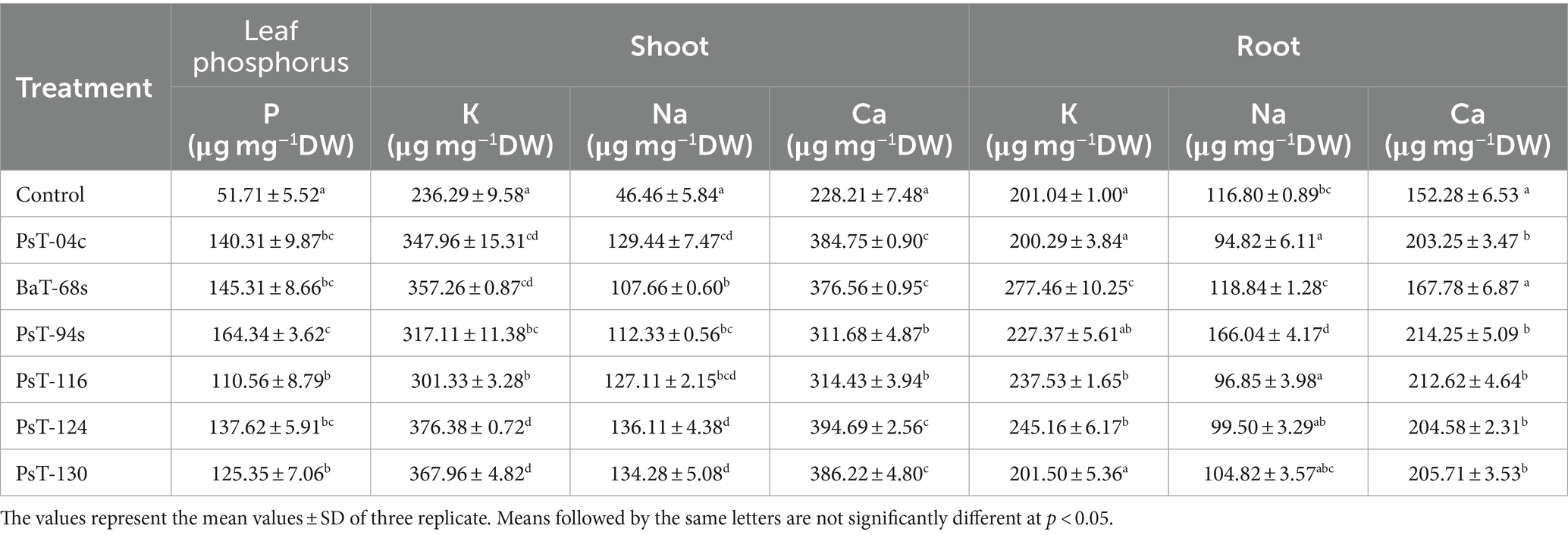
Table 4. Effect of inoculation by selected bacterial isolates on phosphorus content of tomato leaves and shoot and root cation element contents: potassium (K), calcium (Ca), and sodium (Na) after 60 days.
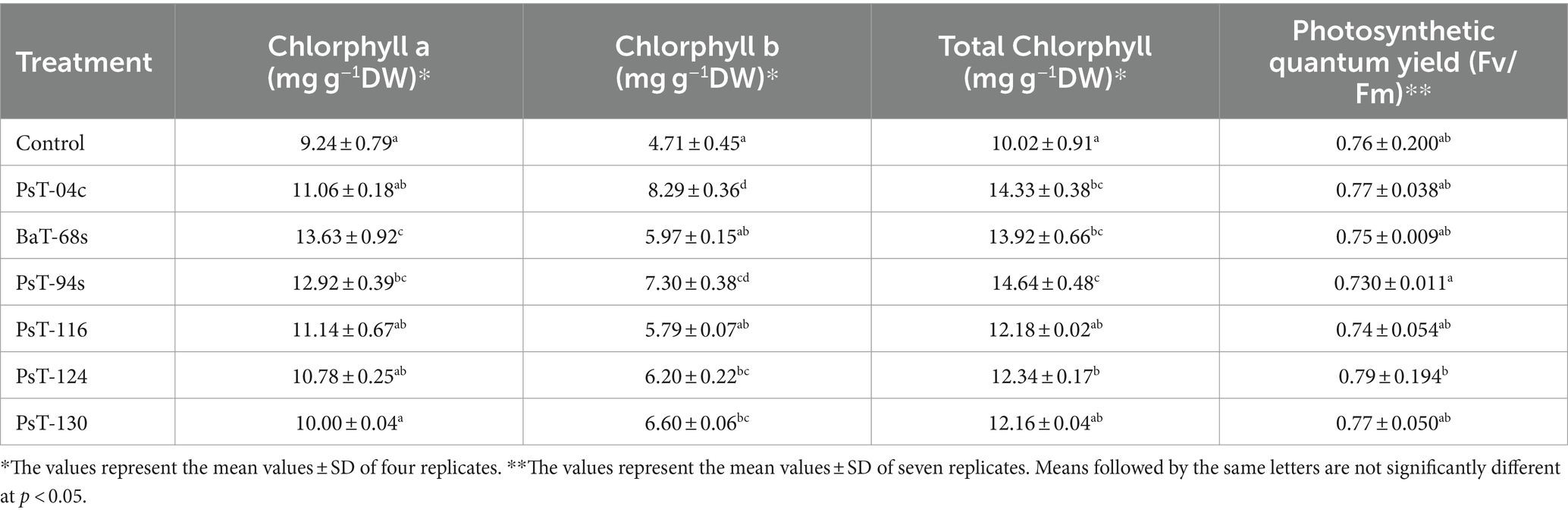
Table 5. Effect of inoculation by selected bacteria on the chlorophyll content of tomato leaves (expressed as mg/dry weight) and chlorophyll fluorescence (Fv/Fm).
3.2.3 Principal component analysis
To assess the contributions of each parameter to phosphate solubilizing bacteria-treated tomato plants compared with control plants, we performed a principal component analysis using plant growth and physiological parameters. The principal component analysis showed that bacterial treatments (red) and variables (blue) were associated with two top PCs, accounting for 78.50% of the total variation in traits under greenhouse conditions (Figure 4). PC1 explained 62.18% of the total variation and was strongly influenced by morphological and biochemical parameters, while PC2 accounted for 16.32% of the total variation and was strongly associated with physiological photosynthetic parameters. The PCA showed a positive correlation between applied phosphate solubilizing bacteria and growth parameters, photosynthetic pigments, and protein and sugar contents, which were positively correlated with each other and confirmed the positive impact of the used bacteria on growth, physiology, biochemistry, and nutrition parameters. In addition, PCA showed that all applied treatments were separated from their controls. Agro-morphological (shoot fresh weight and root fresh weight), physio-biochemical (total soluble sugar, protein, chlorophyll a, chlorophyll b and total chlorophyll contents), shoot and root minerals (Na, K, and Ca) and leaf phosphorus parameters grouped the applied treatments into three main groups; the best responses in terms of higher growth and physio-biochemical parameters were shown by treatments with strains PsT-04c, PsT-116, PsT-124, and PsT-130 (right side of the first axis PC1, upper panel). In addition, treatments with strains PsT-04c and BaT-68s (right side of the first axis, lower panel) resulted in better efficient photosynthetic system and phosphorus nutrition. In contrast, the control treatment without inoculation showed lower growth in both aboveground and root parts, in addition to lower mineral nutrition.
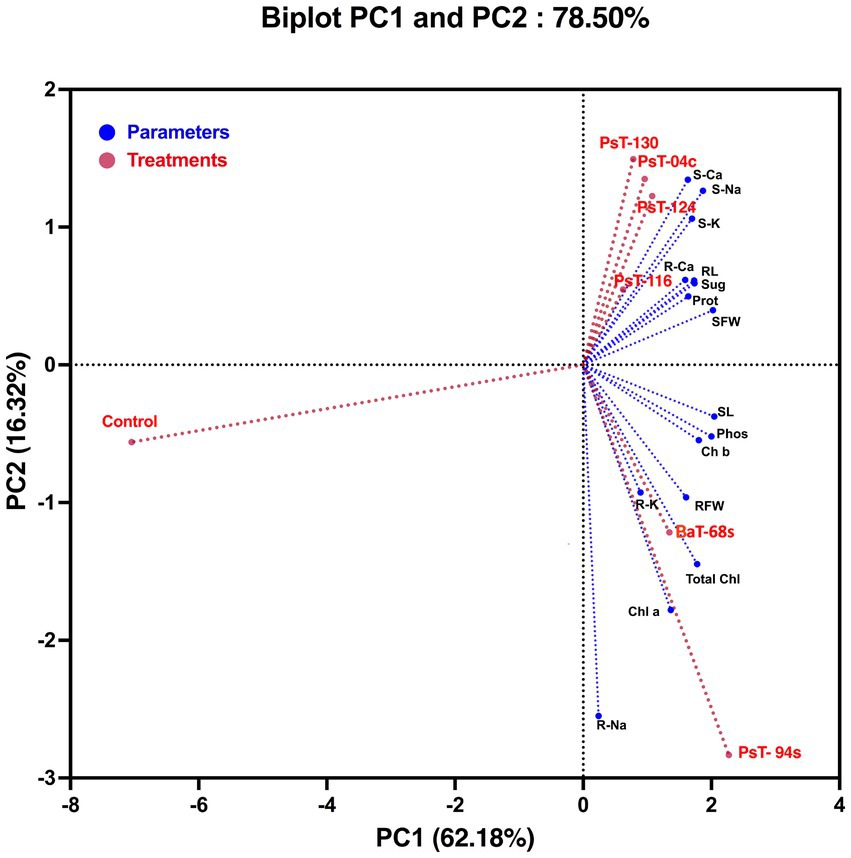
Figure 4. Principal component analysis (PCA) of tomato plants subjected to phosphate solubilizing bacteria. The variables (agro-morphological, physio-biochemical, and minerals including phosphorus) are presented in blue while bacterial treatments are presented in red. Control: absence of the phosphate solubilizing bacteria, PsT-04c, PsT-94s, PsT-116, PsT-124, and PsT-130: treatments with phosphate solubilizing Pseudomonas sp. isolates. BaT-68s: treatment with phosphate solubilizing Bacillus isolate. SL, shoot Lenght; RL, root length; SFW, shoot fresh weight; RFW, root fresh weight; S-Ca, shoot calcium content; S-Na, shoot sodium content; S-K, shoot potassium content; R-Ca, root calcium content; R-Na, root sodium content; R-K, root potassium content; Phos, plant leaf phosphorus; Sug, total soluble sugar content; Prot, protein content; Chl a, chlorophyll a; Chl b, chlorophyll b; Total Chl, total chlorophyll.
3.3 Protection of tomato against Clavibacter michiganensis subsp. michiganensis (Cmm) by induced systemic resistance
3.3.1 Ability of bacteria to grow inside root tissues and on root exudates
Bacterial growth in the rhizosphere depends on their ability to positively interact with the root system, since rhizosphere represents the contact point of plant with major soil microorganisms. In this study, all the six isolates showed abilities to develop in root tissues and on root exudates. In root tissues, their numbers were: PsT-04c: 6.105, BaT-68s: 105, PsT-94s: 2. 105, PsT-116: 105, PsT-124: 3.105, and PsT-130: 7.105 cfu g−1 roots. Moreover, the two isolates Pst-04c and Pst-130 showed the highest ability to grow on tomato root exudates: 3.107 and 6.106 cfu g−1 roots, respectively (Table 2). Hence, the isolates PsT-04c and PsT-130 were selected and used in greenhouse experiments for application as biocontrol agents against the causal agent of bacterial canker, Clavibacter michiganensis subsp. michiganensis (Cmm), by enhanced induced resistance.
3.3.2 Protection against tomato bacterial canker
For biocontrol experiments by resistance induction, we used an aggressive isolate of Cmm and the two isolates PsT-04c and PsT-130 in greenhouse, and we performed the following experiments (i) non-bacterized, uninfected control seedlings, (ii) seedlings infected with Cmm, and (iii) bacterized seedlings with the Pseudomonas isolates PsT-04c and PsT-130 and infected with Cmm 1 week after the first bacterization and bacterized again 20 days after Cmm inoculation.
Figure 5 shows symptoms on infected seedlings which resulted in plant death compared with control and bacterized seedlings. Artificial infection of tomato seedlings by the pathogen Cmm led to a high mortality rate of approximately 80% compared with the control. Treatments with Pseudomonas isolates PsT-04c and PsT-130 led to a significant reduction in the mortality of tomato plants infected with Cmm (Figure 6). At the end of experiment (120 days), no mortality was detected in the negative control, which received sterilized water. In the bacterized seedlings by Pst-04c PsT-130, bacterial canker symptoms were very limited to the leaves. Moreover, appearance of symptoms in the Cmm-infected seedlings was detected after 40 days to 2 months, while appearance of the first symptoms was delayed by one month in the bacterized seedlings by PsT-130 and 40 days in those bacterized by PsT-04c. After 120 days, bacterized seedlings resulted in a protection rate of 75% by PsT-04c and 64% by PsT-130 (Figure 6).
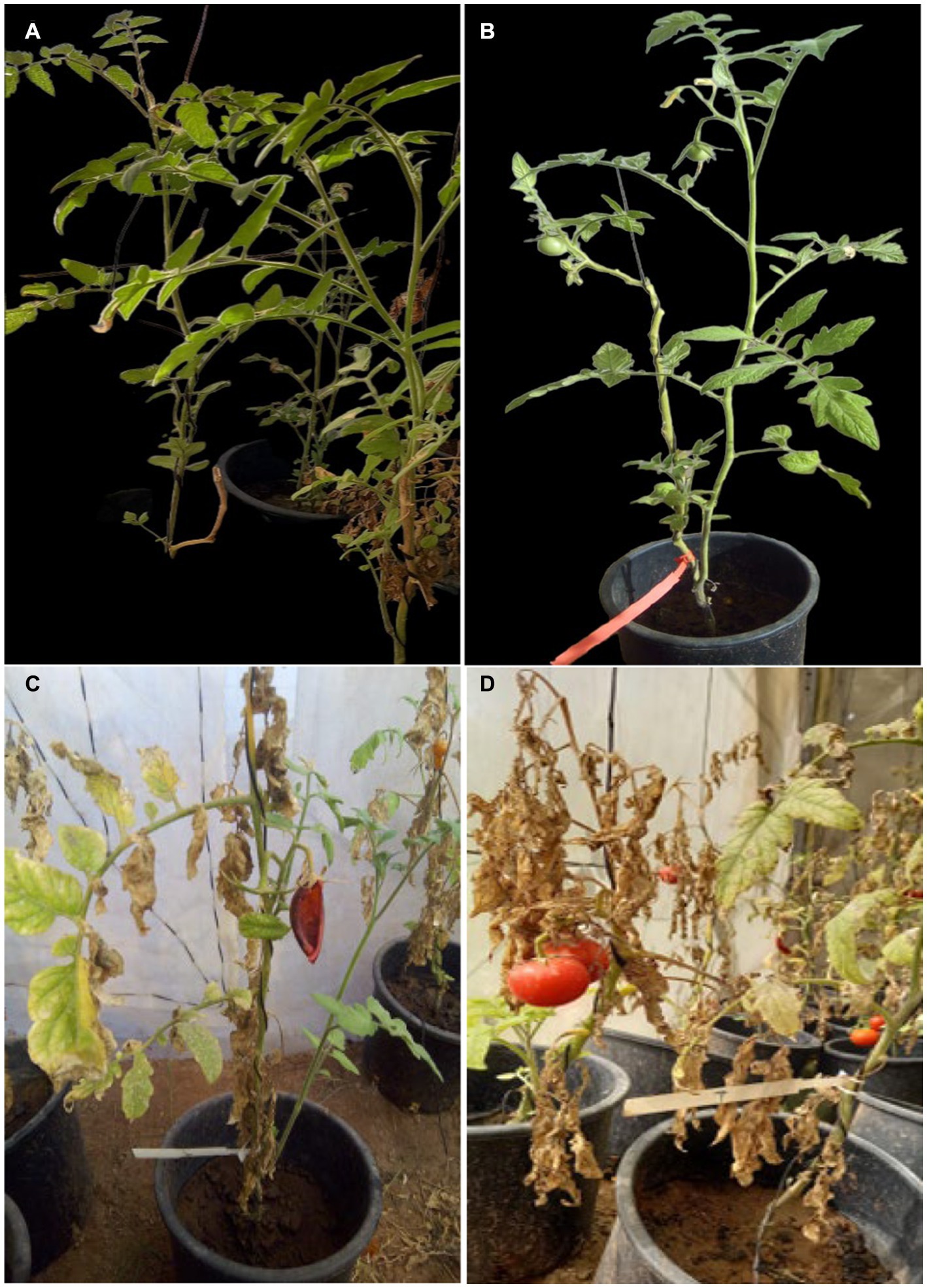
Figure 5. Representative pictures showing biocontrol of tomato bacterial canker after 60 days. (A) Control tomato seedling, (B) Tomato seedlings inoculated with the Pseudomonas isolate PsT-04c and susbesquently infected with Clavibacter michiganensis, (C,D) Decaying tomato seedlings infected with Cmm showing bacterial canker symptoms.
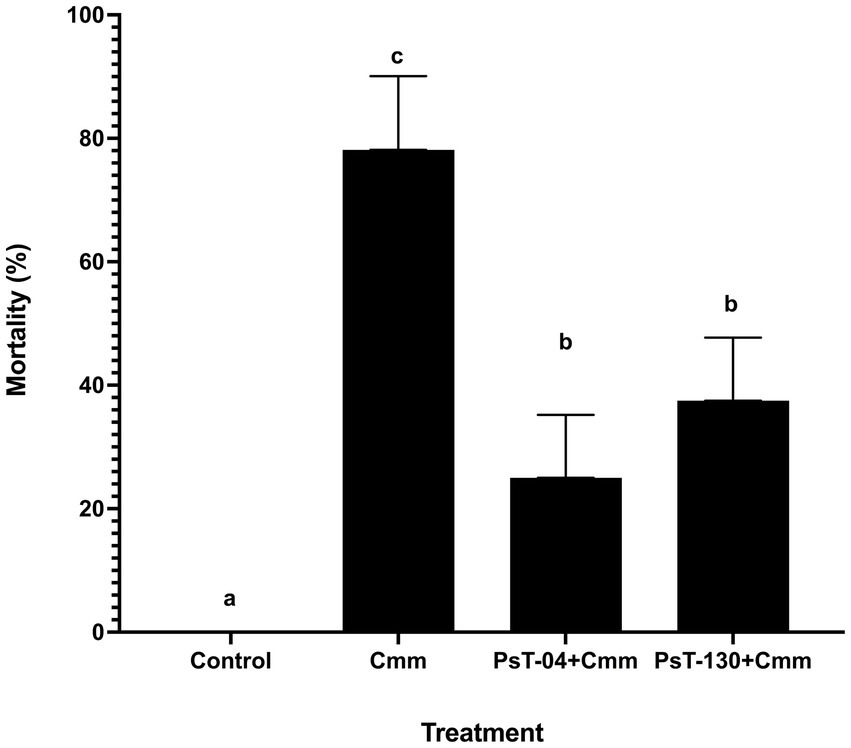
Figure 6. The mortality rate recorded after 120 days in tomato seedling treated with water (negative control), in tomato seedlings artificially infected with Cmm (positive control), and in tomato seedlings inoculated with isolates PsT-04c and PsT-130 before infection by Cmm. Different letters above the bars indicate that the differences are significant at p < 0.05.
3.3.3 Ability to induce systemic resistance mechanisms
3.3.3.1 Total soluble phenolic compounds
After 43 days of infection by Cmm, corresponding to the appearance of bacterial canker symptoms in infected plants, soluble phenolic compounds were measured in Pseudomonas-treated seedlings compared with the control and Cmm-infected seedlings. The treatment with Pseudomonas resulted in an increase in the total root-soluble phenolic compounds of the treated tomato (Figure 7A). Content of total soluble phenolic compounds was 6.81 mg g−1 FW in the untreated plants. No significant differences were found in Cmm-infected plants (6.79 mg g−1 FW) compared with the control. Their content increased in bacteria-treated plants and reached 16.11 and 13.53 mg g−1 FW, respectively, by strains PsT-04c and PsT-130. These levels represented 5.86 times for PsT-04c and 4.42 times for PsT-130 in comparison to the control.
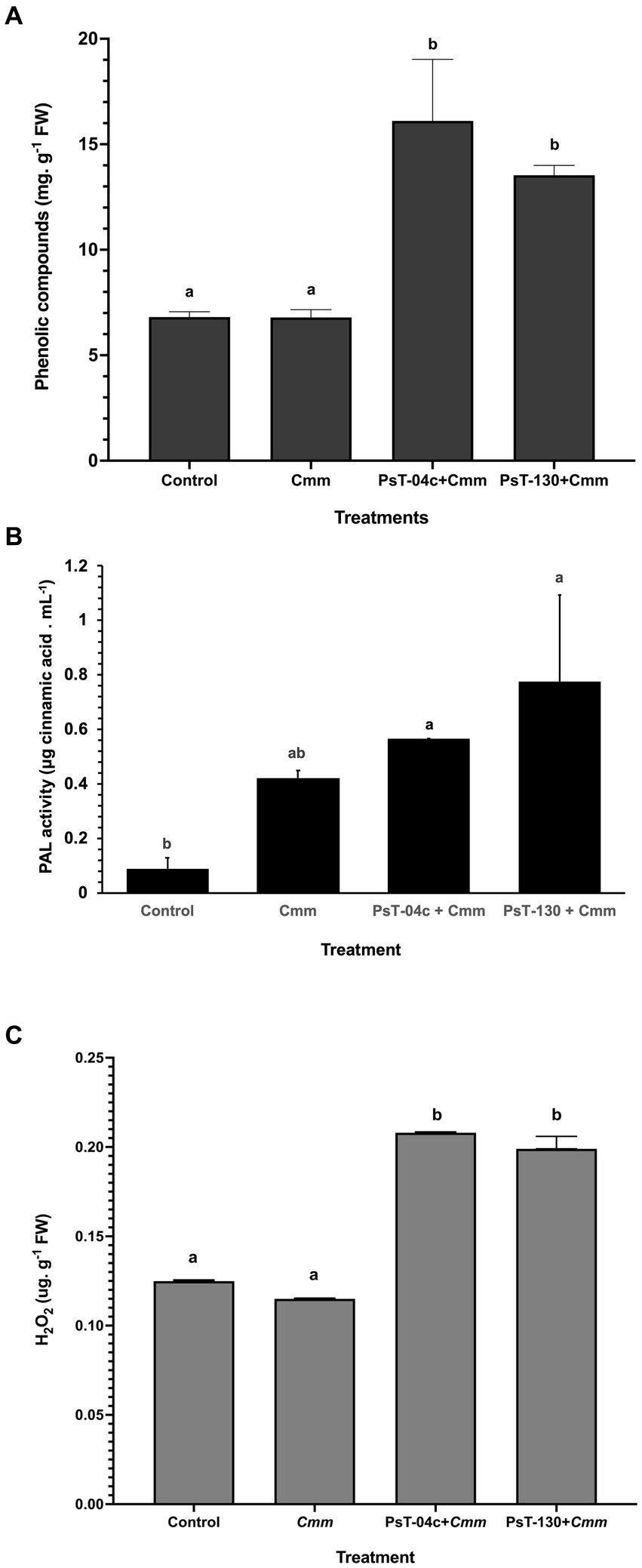
Figure 7. Mechanisms of defense induced in bacterized tomato seedlings by the two Pseudomonas isolates PsT-04c and PsT-130 compared with negative control and Cmm-infected seedlings. (A) Phenolic compounds contents, (B) Phenylalanine ammonia-lyase (PAL) activity, and (C) H2O2 content. Cmm: Clavibacter michiganensis positive control, Cmm + PsT-04c, and Cmm + PsT-130: seedlings treated, respectively, with PsT-04c and PsT-130 and post challenged with Cmm. Different letters above the bars indicate that the differences are significant at p < 0.05.
3.3.3.2 Phenylalanine ammonia-lyase
After 43 days of infection by Cmm, corresponding to the appearance of bacterial canker symptoms in infected plants, phenylalanine ammonia-lyase (PAL) contents were measured in Pseudomonas-treated seedlings compared with the control and Cmm-infected seedlings. The results of phenylalanine ammonia-lyase (PAL) analysis showed that isolates PsT-04c and PsT-130 enhanced PAL activity. After one month of the second bacterization, seedlings treated by PsT-04c and PsT-130 and subsequently infected by Cmm showed higher PAL amount (respectively 0.563 and 0.773 μg mL−1) in comparison to the control (0.088 μg mL−1). PAL activities in PsT-04c and PsT-130 treated seedlings were 6.37 and 8.76 times higher than the control. Cmm-infected tomato plants showed only 0.42 μg mL−1 representing an increase by 4.75 times in comparison to the control (Figure 7B).
3.3.3.3 Hydrogen peroxide (H2O2) content
After 43 days of infection by Cmm, corresponding to the appearance of bacterial canker symptoms in infected plants, hydrogen peroxide (H2O2) contents were measured in Pseudomonas-treated seedlings compared with the control and Cmm-infected seedlings. Treatments with Pseudomonas isolates PsT-04c and PsT-130 led to a significant enhancement of H2O2 in the tomato plants infected with Cmm compared with the control, 1month after the second bacterization (Figure 7C). Isolates PsT-04c showed the highest amount of H2O2 of 0.208 μg g−1 FW compared with the control (0.115 μg g−1 FW), while no significant changes were detected in plants infected with Cmm (0.125 μg g−1 FW) compared with the control.
3.4 Molecular identification of the bacterial isolates
The six isolates were identified using phenotypic and genotypic techniques (Bossis et al., 2000). API 20NE identification system and other biochemical traits showed that all the strains were different (Supplementary Table S1). All isolates were gram-negative except BaT-68s, unicellular bacilli with circular white to yellow colonies. All the five fluorescent isolates PsT-04c, PsT-94s, PsT-116, PsT-124, and PsT-130 were oxidase-positive, suggesting their belonging to the fluorescent pseudomonad group. They were also positive for Levan, except for PsT-94s, and tween, except for PsT-124. All strains were neither able to produce H2S nor to assimilate citrate. Furthermore, they were able to hydrolyze gelatin, except for PsT-124. Based on 16SrRNA gene sequences, the molecular identification revealed that all isolates shared high sequence similarity (>99%) with species from the genus Pseudomonas. Moreover, the phylogenetic analysis constructed using MEGA 11 with the neighbor-joining method supported by a 1,000% bootstrap value showed that all strains belong to Pseudomonas. Isolates PsT-04c, PsT-94s, and PsT-116 shared >99% sequence identity with the type strains of Pseudomonas aeruginosa. Isolate PsT-124 shared >99% sequence identity with P. qingdaonensis. Isolate PsT-130 showed high similarity level to P. azotoformans (Figure 8A), and isolate BaT-68s shared >99% sequence identity with Bacillus paramycoides (Figure 8B).
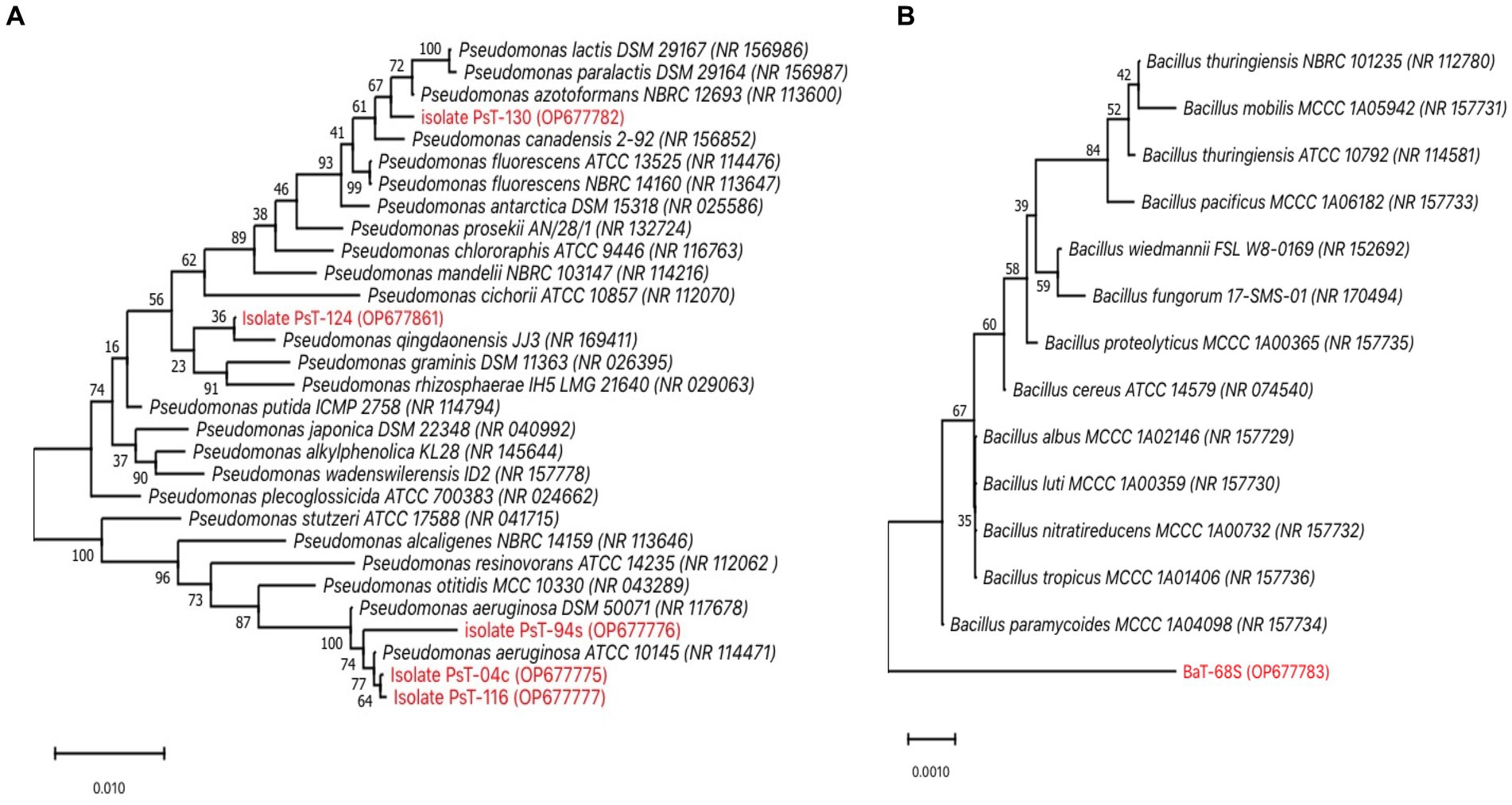
Figure 8. Unrooted consensus phylogenetic trees reconstructed by the neighbor-joining method (Saitou and Nei, 1987) based on almost-complete 16S rRNA gene sequences (1,500 bp). (A) phylogenetic relationships between five fluorescent Pseudomonad isolates (PsT-04c, PsT-94s, PsT-116, PsT-124, and PsT-130) and 25 closely related Pseudomonas type species, (B) phylogenetic relationships between BaT-68s and 13 closely related Bacillus species. Closest species were derived using NCBI BLAST search tool (Altschul et al., 1990). The GenBank accession numbers of species are placed in parenthesis. Sequences were aligned using Muscle, and Evolutionary analyses were conducted in MEGA 11 software (Stecher et al., 2020; Tamura et al., 2021). Bootstrap support values: percentage of replicate trees in which the associated taxa clustered together in the bootstrap test (1,000 replicates) are shown above the branches (Felsenstein, 1985). The evolutionary distances are computed using the Maximum Composite Likelihood method (Tamura et al., 2004) and are in the units of the number of base substitutions per site. The scale bar represents nucleotide substitutions per site. There were 1,558 positions for Pseudomonas and 1,580 for Bacillus in the final dataset. Isolates PsT-04c, PsT-94s, and PsT-116 shared >99% sequence identity with the type strains of Pseudomonas aeruginosa. Isolate PsT-124 shared >99% sequence identity with P. qingdaonensis. Isolate PsT-130 showed high similarity level to P. azotoformans, isolate BaT-68s shared >99% sequence identity with Bacillus paramycoides.
4 Discussion
Tomato rhizosphere could represent an interesting source of well-adapted PGPR with phosphate solubilizing abilities and biocontrol abilities against phytopathogens. We isolated 100 bacteria from tomato rhizosphere soil using King B medium. King B is a non-selective medium and hence may support the growth of bacteria, belonging to diverse taxa including Pseudomonas, Bacillus, and Paenibacillus (Nam et al., 2016; Bouizgarne et al., 2023; Koo et al., 2023). Furthermore, bacteria were subsequently screened for their ability to solubilize TCP on NBRIP agar medium. Selected highly efficient phosphate solubilizing bacteria (PSB) combined with rock phosphate from Moroccan phosphate mines were used to enhance tomato growth. Among these bacteria, those showing the highest ability to survive inside tomato roots and grow on root exudates were used in order to protect tomato seedlings against Cmm by induced systemic resistance. Nautiyal (1999) showed that the NBRIP medium is three times more efficient in terms of demonstrating solubilization compared with Pikovskaya medium (Pikovskaya, 1948). Thus, NBRIP medium used in this investigation was adopted to demonstrate solubilization by the isolates as halos are easy to detect by color change around the colony. In addition, it was demonstrated that solubilization obtained with TCP as sole P source in the medium was significantly greater than in the presence of rock phosphate (Azaroual et al., 2020; Ma et al., 2023). Thus, we used TCP as P source in our experiments. However, qualitative assay on solid medium only allows to detect bacteria, which exhibits solubilization by the production of organic acids. Thus, solubilizing isolates acting by chelating mechanisms could not be detected by this assay. In addition, the importance of the solubilization halo does not necessarily reflect their ability to solubilize in a liquid medium or in the presence of the plant. The decrease in pH during solubilization is probably due to the release of organic acids. The six isolates, PsT-04c, BaT-68s, PsT-94s, PsT-116, PsT-124, and PsT-130, were selected as they showed maximum solubilization ability, in addition to IAA production, and were investigated for the production of organic acids during phosphate solubilization, mainly gluconic acid, maleic acid, and citric acid.
Most microorganisms, particularly Pseudmonads and Bacillus, set up mechanisms for the solubilization of phosphates based essentially on secreting organic acids and protons (Illmer and Schinner, 1995; Chen et al., 2006; Yahya et al., 2021; Ahmad et al., 2022) or producing phosphatases (Richardson, 2001). During phosphate solubilization, bacteria acidify the periplasmic space by releasing various organic acids of low molecular weight, including lactic acid, gluconic acid, isobutyric acid, acetic acid, glycolic acid, oxalic acid, malonic acid, succinic acid, and 2-ketogluconic acid. Among released organic acids, gluconic acid is the most common in fluorescent Pseudomonas spp. (Khan et al., 2009), and it was shown to be involved in the conversion of insoluble phosphate forms into soluble forms, easily assimilated by plants. Consequently, organic acid release leads to an acidification of the external environment of the bacterial cells and a decrease of its pH. As for P solubilization, the major mechanism of K solubilization is conducted by the production of organic and inorganic acids and protons (Meena et al., 2015).
Molecular identification revealed that five strains PsT-04c, PsT-94s, PsT-116, PsT-124, and PsT-130 belong to Pseudomonas, while BaT-68s belongs to Bacillus. Although PsT-04c, PsT-94s, and PsT-116 are close neighbors of P. aeruginosa according to pairwise distance calculated following 16S rRNA gene sequences, they are phenotypically different by their physiological and PGPR traits, suggesting that they represent different strains of the same species. PsT-124 was close to P. qingdaonesis and PsT-130 was close to P. azotoformans, while BaT-68s was close to B. paramycoides. Pseudomonas and Bacillus were reported to be powerful phosphate solubilizers (Banerjee et al., 2006; Bouizgarne, 2013; Bouizgarne et al., 2023). Pseudomonas are gram-negative bacteria, which inhabit a wide range of environments and exhibit a great metabolic diversity (Stanier et al., 1966). Opposite to Pseudomonas, Bacillus are gram-positive spore-forming bacteria that allow them to overcome harsh conditions and survive for extended periods (Bouizgarne, 2013). Both species are well documented as plant growth-promoting rhizobacteria (PGPR). Search for microorganisms with multiple PGPR traits is a promising strategy. Phosphate solubilizing bacteria can promote and improve plant growth parameters (Gamalero et al., 2004; Yahya et al., 2021; Bouizgarne et al., 2023). Beneficial effects of Pseudomonas might be due to combined effects of phosphate solubilization, phytohormone production, or biosynthesis of antifungal and antibacterial agents, and these effects usually affects plant size and/or fruit yields positively (Bouizgarne, 2013; Yahya et al., 2021; Bouizgarne et al., 2023). Members of Bacillus could trigger plant growth promotion by auxin production or increasing uptake availability of phosphorus (Deepa et al., 2010). The direct effect of Pseudomonas spp. and Bacillus on plant growth promotion was demonstrated to be due to various mechanisms such as nitrogen fixation, phosphate and iron solubilization, phytohormone modulation, and increased abiotic stress tolerance (Robin et al., 2008; Lugtenberg and Kamilova, 2009; Richardson et al., 2009; Bouizgarne, 2013; Misra and Chauhan, 2020). Thus, six bacterial isolates, which showed maximum solubilization (PsT-04c, BaT-68s, PsT-94s, PsT-116, PsT-124, and PsT-130), were screened in further experiments to test their abilities to trigger other PGPR traits. In addition to phosphate solubilization phosphate, these bacteria showed abilities to solubilize potash and zinc in vitro, produce significant amount of the IAA phytohormone, and positively affect the growth of seedlings in the greenhouse when they were applied to tomato. The fact that the selected isolates were able to solubilize phosphate, potash and zinc allow to consider them as potent biofertilizers for tomato. The use of phosphate, potash, and zinc solubilizing bacteria combined with natural phosphate is a promising tool in an eco-friendly strategy, leading to a potential reduction in the application of chemical fertilizers. Different species of Pseudomonas fluorescens have been reported as PGPR, and strains belonging to this genus are predominantly found inside the P. fluorescens complex (Bouizgarne, 2013; Garrido-Sanz et al., 2016). The application of Pseudomonas fluorescens B16 bacteria led to an increase in height, number of flowers, number of fruits, and total fruit weight of tomato plants (Mirza et al., 2001). In addition to phosphate, selected isolates in this investigation showed interesting ability to solubilize insoluble potassium and zinc forms. After nitrogen and phosphorus, potassium is the third important plant nutrient that has a key role in the growth, metabolism, and development of plants. It is involved in increasing plant resistance to biotic and abiotic stresses and is required to activate most different enzymes involved in plant processes (Ahmad et al., 2016). As for phosphorus, potassium is relatively unavailable for plant growth as 90-98% of soil potassium is present as insoluble mineral form (feldspar and mica), and only 2% is available to plants as free soluble form, directly absorbed by plants (Etesami et al., 2017; Jain et al., 2022). Potassium solubilizing bacteria (KSM) can solubilize insoluble potassium minerals such as biotite, feldspar, muscovite, vermiculite, smectite, orthoclase, and mica to soluble forms which could be available to plants (Ahmad et al., 2016; Jain et al., 2022). Species from the genera including Bacillus, Paenibacillus, Pseudomonas, Burkholderia, and Serratia have been reported to possess the ability of K solubilization (Ahmad et al., 2016; Sun et al., 2020). As for phosphorus, potassium is a macronutrient whose concentration in the soil might limit plant growth (Parmar and Sindhu, 2013), and our isolates could probably also enhance its solubilization or uptake from soil. Zinc is among the essential micronutrients for plant growth, and inorganic zinc in soil is unavailable form for plant assimilation. Thus, the selected bacteria could be involved in making the insoluble inorganic zinc into biologically available form for plants. Zinc plays an essential role in the biosynthesis of IAA through the formation of its precursor, tryptophan. In addition, zinc is involved in many other physiological functions: cell division, elongation, fruit development, and control of gene expression, stabilizing RNA and DNA structure and maintaining the activity of RNA-degrading enzymes (Brown et al., 1993). Although not measured in Bunt and Rovira liquid medium in this investigation like for NBRIP, it is suggested that zinc solubilization could be due to a drop in pH and organic acid production, potentially involved in K solubilization (Fasim et al., 2002; Ahmad et al., 2016). Moreover, as for phosphorus, it has been reported that inoculation with potassium and zinc solubilizing-bacteria led to beneficial effects on the growth of different plants (Bakhshandeh et al., 2017; Ali et al., 2020; Batool et al., 2021). In addition, indole-3-acetic acid is the most common auxin phytohormone, involved in cell division, root elongation, fruit development, and senescence, and it is the most important auxin produced by bacteria, plants, and fungi (Bouizgarne, 2013; Lesueur et al., 2016). Its role in the observed positive beneficial effects of the six isolates is probably crucial. Chu et al. (2020), reported positive effects of Pseudomonas PS01 on development of the root system by triggering lateral root and root hair formation, due to the production of IAA by the bacterium. All those potential beneficial effects demonstrated in vitro, make them good candidates for application as inoculants for tomato growth promotion. In this investigation, treatment by the six bacteria led to an improvement in growth parameters: shoot and root length of seedlings and their fresh and dry weight.
Photosynthesis plays a key role in metabolic processes by which plants grow. The selected bacteria increased the chlorophyll content in plant leave tissues, which could be involved in enhanced photosynthesis. In this investigation, some isolates showed a positive effect on chlorophyll a and b and total chlorophyll. Research studies, aiming to test the effectiveness of PGPR on nutrient parameters, demonstrated that their application resulted in a significant increased total chlorophyll, sugar, and protein contents (Pirlak and Köse, 2009; Khan et al., 2019). On the other hand, the absence of any changes in chlorophyll fluorescence after 60 days of culture suggests that our isolates did not induce any biotic stress to plants. The inoculation by selected bacterial isolates resulted in positive effects on the mineral element contents of tomato seedlings after 60 days. Indeed, following application of the bacterial strains, an increase in leaves P content and K, Na and Ca contents in shoot was recorded for all the isolates. Enhancement in exchangeable cations was shown in many plants, following treatments with PGPR bacteria. Increased mineral nutrition, improved water and nutrient translocation, efficient nutrient uptake and utilization, increased photosynthesis, and the ability to enhance N, Ca, Mg, and K uptake by plants are important beneficial effects of the plant growth-promoting rhizobacteria (Abd-Allah et al., 2018; Kour et al., 2020). A study by Ipek et al. (2014) demonstrated an increase in plant tissue nutrients, such as nitrogen, phosphorus, potassium, calcium, iron, copper, manganese, and boron by PGPR treatments. Those effects could be displayed even under harsh environmental conditions (Jha and Subramanian, 2018; Khan et al., 2019). High total sugars and proteins contents shown in treated plants with the phosphate solubilizing bacteria, compared to control, may be due to increased leaf biomass, to increased availability of assimilates due to increased photosynthesis and availability of nutrients to the plants following the application of plant growth promoting bacteria.
Due to their various antagonistic properties against phytopathogens, fluorescent Pseudomonas and Bacillus are the most studied bacteria regarding their usefulness as biocontrol agents of phytopathogens (Lemanceau et al., 2007; Bouizgarne, 2013; Bouizgarne and Ouhdouch, 2017). Another indirect mechanism is known as induced systemic resistance (ISR) which allows plants to protect themselves against fungal and bacterial disease agents (Chen et al., 2020; Yahya et al., 2021; Abdelaziz et al., 2023; Bellotti et al., 2023). Bacteria belonging to Pseudomonas and Bacillus strains were described as resistance inducers in tomato phytopathogens (Gautam et al., 2019; Bellotti et al., 2023). Thus, the selection of bacterial isolates which could induce resistance could be adopted as a strategy for biocontrol of bacterial canker. In this study, we selected two Pseudomonas isolates PsT-04c and PsT-130 as ISR inducers against an aggressive isolate of Cmm. The bacteria selected in this study showed ability to grow on root tissues and root exudates. Root exudates are carbon-rich rhizodeposits containing primary and secondary low molecular weight metabolites containing multiple carbohydrates (amino acids, organic acids, and soluble phenolic compounds), which are secreted by plant roots. The role of the root exudates in plant–microbe interactions and plant responses to various phytopathogens is well established (Kandel et al., 2017; Mavrodi et al., 2021; Yahya et al., 2021). It was demonstrated that root exudates initiate the communication between soil bacteria and host plants, leading to bacterial adhesion to the root surfaces. Root exudates also modulate rhizosphere microbial regulation of phosphorus uptake (Sasse et al., 2018) and stimulate beneficial microbial agents including biocontrol agents of plant pathogens (Zhang et al., 2014; Wang et al., 2019; Wen et al., 2021). Thus, we selected the best growing isolates on root tissues and root exudates, PsT-04c and PsT-130 for root treatments in greenhouse resistance induction trials. Treatments with these bacteria provided protection against bacterial canker disease and increased H2O2 levels. Treatments allowed a significant increase in the levels of root soluble phenolic compounds, for which the role as defense molecules of plants against phytopathogens is well established. An increase was also noticed in phenylalanine ammonia-lyase (PAL) activity, explaining the increase in soluble phenolic compounds. Indeed, PAL is the key enzyme involved in the phenylpropanoid pathway of the phenolic compound synthesis. The role of PAL in tomato resistance to bacterial canker was reported by Umesha (2006). The results of protection of tomato seedlings against the causative agent of bacterial canker can be explained by the marked increase in the content of soluble phenolic compounds and hydrogen peroxide (H2O2). While infection of the plant with Cmm did not significantly increase the H2O2 level (0.125 μg g−1 FW) compared with the control seedlings (0.115 μg g−1 FW), this level was almost doubled during the PsT-04c and PsT-130 treatments with approximately 0.200 μg g−1 FW. Accumulation of H2O2 was reported as the mechanism of induced systemic resistance, following treatment by Pseudomonas biocontrol agents (De Vleesschauwer and Hoefte, 2006; Bellameche et al., 2021). H2O2 plays a central role in plant resistance to pathogens as it can activate several defense reactions including the strengthening of physical barriers and development of the hypersensitivity reaction. Phenolic compounds are secondary metabolites that are commonly present in plant tissues and are known for their intervention in the defense of plants against phytopathogens. We suggest that these bacteria act by inducing systemic resistance in tomatoes, allowing protection against the pathogen. The involvement of other enzymes, such as catalase, peroxidase, polyphenol oxidase, or superoxide dismutase (Yahya et al., 2021), is not excluded and still to be investigated. The induction of systemic resistance (ISR) by the two Pseudomonas bacteria is an excellent way to boost the defense of the tomato against a post challenge with Cmm. On the other hand, those two isolates showed antagonistic activity against Cmm growth in vitro (unpublished data) by the production of antibiotics (Raaijmakers et al., 2002), and it is not excluded that this activity could also contribute to the protective effect against Cmm at ground level, which is found in our experience.
In conclusion, isolated fluorescent pseudomonad and Bacillus sp. have PGPR effect that improves growth parameters. In this study, we isolate phosphate, potash, and zinc-solubilizing bacteria including five fluorescent pseudomonads and one Bacillus. The application of these bacteria in combination with Moroccan rock phosphate led to an increase in tomato shoot and root length and their fresh and dry weights. Phosphate solubilizing bacteria showing beneficial effects on tomato growth parameters showed abilities to improve to different extents, the measured physiological parameters. The triggering of defense mechanisms of tomato by two Pseudomonas strains suggests that they are interesting as bio primers for biocontrol of bacterial canker disease. Research on these two areas will help widen the alternative options available for tomato crop fertilization and managing bacterial canker disease. Experiments, aiming to use the two inducing bacteria and other Pseudomonas and Serratia strains, showing in vitro antibiosis against Cmm (Bouizgarne et al., 2023; Bouizgarne and Bakki, 2024), are in progress, as well as bioformulations of most efficient bacteria in combination with rock phosphate are applied as biofertilizers and biopesticides.
Data availability statement
The datasets presented in this study can be found in online repositories. The names of the repository/repositories and accession number(s) can be found at: https://www.ncbi.nlm.nih.gov/genbank/, OP677775, OP677776, OP677777, OP677861, OP677782, and OP677783.
Author contributions
MB: Conceptualization, Investigation, Methodology, Visualization, Software, Data curation, Writing – original draft. BaB: Formal analysis, Software, Visualization, Investigation, Methodology, Writing – review & editing. OM: Investigation, Methodology, Writing – review & editing. QE: Writing – review & editing, Software. AH: Resources, Writing – review & editing. EB: Writing – review & editing. KA: Resources, Writing – review & editing. BrB: Conceptualization, Data curation, Funding acquisition, Investigation, Methodology, Project administration, Resources, Software, Supervision, Validation, Visualization, Writing – original draft, Writing – review & editing.
Funding
The author(s) declare financial support was received for the research, authorship, and/or publication of this article. The authors would like to express appreciation for the support of the sponsors through the R&D Initiative—Appel à projets autour des phosphates APPHOS—sponsored by OCP (OCP Foundation, R&D OCP, Mohammed VI Polytechnic University, National Center of Scientific and technical Research CNRST, Ministry of Higher Education, Scientific Research and Professional Training of Morocco MESRSFC) under the project entitled *Bioformulations d’un consortium de microorganismes solubilisateurs du phosphate: effets bénéfiques sur la croissance et la protection des plantes *, project ID * BIO-BIZ-01/2017.
Acknowledgments
The authors acknowledge UATRS, CNRST, Rabat, specially Elmostafa El Fahime, Safaa Rhoulam, and Taha Chouati for DNA sequencing. The authors also Acknowledge Jaber Boujemâa, Hind Chakchak, and Hanae Ouaddari for HPLC/MS assays.
Conflict of interest
The authors declare that the research was conducted in the absence of any commercial or financial relationships that could be construed as a potential conflict of interest.
The author(s) declared that they were an editorial board member of Frontiers E. Ait Barka, at the time of submission. This had no impact on the peer review process and the final decision.
Publisher’s note
All claims expressed in this article are solely those of the authors and do not necessarily represent those of their affiliated organizations, or those of the publisher, the editors and the reviewers. Any product that may be evaluated in this article, or claim that may be made by its manufacturer, is not guaranteed or endorsed by the publisher.
Supplementary material
The Supplementary material for this article can be found online at: https://www.frontiersin.org/articles/10.3389/fmicb.2024.1289466/full#supplementary-material
References
Abd-Allah, E. F., Alqarawi, A. A., Hashem, A., Radhakrishnan, R., Al-Huqail, A. A., Al-Otibi, F. O. N., et al. (2018). Endophytic bacterium Bacillus subtilis (BERA 71) improves salt tolerance in chickpea plants by regulating the plant defense mechanisms. J. Plant Interact. 13, 37–44. doi: 10.1080/17429145.2017.1414321
Abdelaziz, S., Belal, E. E., Al-Quwaie, D. A., Ashkan, M. F., Alqahtani, F. S., El-Tarabily, K. A., et al. (2023). Extremophilic bacterial strains as plant growth promoters and biocontrol agents against Pythium ultimum and Rhizocotnia solani. J. Plant Pathol. 105, 1347–1369. doi: 10.1007/s42161-023-01460-8
Adnan, M., Fahad, S., Zamin, M., Shah, S., Mian, I. A., Danish, S., et al. (2020). Coupling phosphate-solubilizing bacteria with phosphorus supplements improve maize phosphorus acquisition and growth under lime induced salinity stress. Plan. Theory 9:900. doi: 10.3390/plants9070900
Ahmad, A., Moin, S. F., Liaqat, I., Saleem, S., Muhammad, F., Mujahid, T., et al. (2022). Isolation, solubilization of inorganic phosphate, and production of organic acids by individual and co-inoculated microorganisms. Geomicrobiol J. 40, 111–121. doi: 10.1080/01490451.2022.2124329
Ahmad, M., Nadeem, S. M., Naveed, M., and Zahir, Z. A. (2016). “Potassium-solubilizing bacteria and their application in agriculture” in Potassium solubilizing microorganisms for sustainable agriculture. eds. V. Meena, B. Maurya, J. Verma, and R. Meena (New Delhi: Springer). 293–313. doi: 10.1007/978-81-322-2776-2_21
Ali, A. M., Awad, M. Y. M., Hegab, S. A., Gawad, A. M. A. E., and Eissa, M. A. (2020). Effect of potassium solubilizing bacteria (Bacillus cereus) on growth and yield of potato. J. Plant Nutr. 44, 411–420. doi: 10.1080/01904167.2020.1822
Alori, E. T., Glick, B. R., and Babalola, O. O. (2017). Microbial phosphorus solubilization and its potential for use in sustainable agriculture. Front. Microbiol. 8:971. doi: 10.3389/fmicb.2017.00971
Altschul, S. F., Gish, W., Miller, W., Myers, E. W., and Lipman, D. J. (1990). Basic local alignment search tool. J. Mol. Biol. 215, 403–410. doi: 10.1016/S0022-2836(05)80360-2
Arnon, D. I. (1949). Copper enzymes in isolated chloroplasts. Polyphenoloxidase in Beta vulgaris. Plant Physiol. 24, 1–15. doi: 10.1104/pp.24.1.1
Azaroual, S. E., Hzzoumi, Z., El Mernissi, N., Aasfar, A., Kadmiri, I. M., and Bouizgarne, B. (2020). Role of inorganic phosphate solubilizing bacilli isolated from Moroccan phosphate rock mine and rhizosphere soils in wheat (Triticum aestivum L) phosphorus uptake. Curr. Microbiol. 77, 2391–2404. doi: 10.1007/s00284-020-02046-8
Bakhshandeh, E., Pirdashti, H., and Lendeh, K. S. (2017). Phosphate and potassium-solubilizing bacteria effect on the growth of rice. Ecol. Eng. 103, 164–169. doi: 10.1016/j.ecoleng.2017.03.008
Banerjee, M. R., Yesmin, L., and Vessey, J. K. (2006). “Plant growth promoting rhizobacteria as biofertilizers and biopesticides” in Handbook of microbial biofertilizers. ed. M. Rai (New York: Haworth Press), 137–181.
Bargaz, A., Elhaissoufi, W., Khourchi, S., Benmrid, B., Borden, K. A., and Rchiad, Z. (2021). Benefits of phosphate solubilizing bacteria on belowground crop performance for improved crop acquisition of phosphorus. Microbiol. Res. 252:126842. doi: 10.1016/j.micres.2021.126842
Batool, S., Asghar, H. N., Shehzad, M. A., Yasin, S., Sohaib, M., Nawaz, F., et al. (2021). Zinc solubilizing bacteria-mediated enzymatic and physiological regulations confer zinc biofortification in chickpea (Cicer arietinum L.). J. Soil Sci. Plant Nutr. 21, 2456–2471. doi: 10.1007/s42729-021-00537-6
Bellameche, F., Jasim, M. A., Mauch-Mani, B., and Mascher, F. (2021). Histopathological aspects of resistance in wheat to Puccinia triticina, induced by Pseudomonas protegens CHA0 and β-aminobutyric acid. Phytopathol. Mediterr. 60, 441–453. doi: 10.36253/phyto-13123
Bellotti, G., Guerrieri, M. C., Giorni, P., Bulla, G., Fiorini, A., Bertuzzi, T., et al. (2023). Enhancing plant defense using rhizobacteria in processing tomatoes: a bioprospecting approach to overcoming early blight and Alternaria toxins. Front. Microbiol. 14:1221633. doi: 10.3389/fmicb.2023.1221633
Bossis, E., Lemanceau, P., Latour, X., and Gardan, L. (2000). The taxonomy of Pseudomonas fluorescens and Pseudomonas putida: current status and need for revision. Agronomie 20, 51–63. doi: 10.1051/agro:2000112
Bouizgarne, B. (2013). “Bacteria for plant growth promotion and disease management” in Bacteria in agrobiology: Disease management. ed. D. Maheshwari (Berlin: Springer). doi: 10.1007/978-3-642-33639-3_2
Bouizgarne, B. (2022). “Phosphate-solubilizing Actinomycetes as biofertilizers and biopesticides: bioformulations for sustainable agriculture” in Microbial BioTechnology for sustainable agriculture. eds. N. K. Arora and B. Bouizgarne, vol. 1 (Singapore: Springer). doi: 10.1007/978-981-16-4843-4_13
Bouizgarne, B., and Bakki, M. (2024). “Tomato bacterial canker disease management: molecular interactions and role of biocontrol agents (BCAs) against Clavibacter michiganensis” in Microbial BioTechnology for sustainable agriculture. eds. N. K. Arora and B. Bouizgarne, vol. 2 (Singapore: Springer).
Bouizgarne, B., Bakki, M., Boutasknit, A., Banane, B., El Ouarrat, H., Ait El Maalem, S., et al. (2023). Phosphate and potash solubilizing bacteria from Moroccan phosphate mine showing antagonism of bacterial canker agent and inducing effective tomato growth promotion. Front. Plant Sci. 14:970382. doi: 10.3389/fpls.2023.970382
Bouizgarne, B., and Ouhdouch, Y. (2017). “Management of tomato foot and root rot (TFRR) by biocontrol agents with emphasis on factors affecting its effectiveness” in Probiotics and plant health. eds. V. Kumar, et al. (Springer, Singapore Pte Ltd.), 1–19. doi: 10.1007/978-981-10-3473-2_1
Bradford, M. M. (1976). A rapid and sensitive method for the quantitation of microgram quantities of protein utilizing the principle of protein-dye binding. Anal. Biochem. 72, 248–254. doi: 10.1006/abio.1976.9999
Bric, J. M., Bostock, R. M., and Silverstone, S. E. (1991). Rapid in situ assay for indoleacetic acid production by bacteria immobilized on a nitrocellulose membrane. Appl. Environ. Microbiol. 57, 535–538. doi: 10.1128/aem.57.2.535-538.1991
Brown, P. H., Cakmak, I., and Zhang, Q. (1993). “Form and function of zinc in soils and plants” in Zinc in soils and plants. ed. A. D. Robson (Dordrecht: Kluwer Acadamic Publishers), 90–106.
Bunt, J. S., and Rovira, A. D. (1955). Microbiological studies of some subantartic soils. J. Soil Sci. 6, 119–128. doi: 10.1111/j.1365-2389.1955.tb00836.x
Chang, R. J., Ries, S. M., and Pataky, J. K. (1992). Reductions in yield of processing tomatoes and incidence of bacterial canker. Ecol. Epidemiol 76, 805–560. doi: 10.1094/PD-76-0805
Chen, Y. P., Rekha, P. D., Arun, A. B., Shen, F. T., Lai, W.-A., and Young, C. C. (2006). Phosphate solubilizing bacteria from subtropical soil and their tricalcium phosphate solubilizing abilities. Appl. Soil Ecol. 34, 33–41. doi: 10.1016/j.apsoil.2005.12.002
Chen, L., Wang, X., Ma, Q., Bian, L., Liu, X., Xu, Y., et al. (2020). Bacillus velezensis CLA178-Induced systemic resistance of Rosa multiflora against crown gall disease. Front. Microbiol. 11:587667. doi: 10.3389/fmicb.2020.587667
Chu, T. N., Bui, L., and Van Hoang, M. T. T. (2020). Pseudomonas PS01 isolated from maize rhizosphere alters root system architecture and promotes plant growth. Microorganisms 8:471. doi: 10.3390/microorganisms8040471
De Vleesschauwer, D., and Hoefte, P. M. (2006). Redox-active pyocyanin secreted by Pseudomonas aeruginosa 7NSK2 triggers systemic resistance to Magnaporthe grisea but enhances Rhizoctonia solani susceptibility in rice. Mol. Plant-Microbe Interact. 19, 1406–1419. doi: 10.1094/MPMI-19-1406
Deepa, C. K., Dastager, S. G., and Pandey, A. (2010). Plant growth-promoting activity in newly isolated Bacillus thioparus (NII-0902) from Western Ghat forest, India. World J. Microbiol. Biotechnol. 26, 2277–2283. doi: 10.1007/s11274-010-0418-3
Dubois, M., Gilles, K. A., Hamilton, J. K., Rebers, P. A., and Smith, F. (1956). Colorimetric method for determination of sugars and related substances. Anal. Chem. 28, 350–356. doi: 10.1021/ac60111a017
Etesami, H., Emami, S., and Likhani, H. A. (2017). Potassium solubilizing bacteria (KSB): mechanisms, promotion of plant growth, and future prospects - a review. J. Soil Sci. Plant Nutr. 17, 897–911. doi: 10.4067/S0718-95162017000400005
FAOSTAT, Crops and livestock products, Tomatoes. (2022). Available at: http://www.fao.org/faostat/en/#data/QCL/visualize (Accessed November 1, 2022).
Fasim, F., Ahmed, N., Parsons, R., and Gadd, G. M. (2002). Solubilization of zinc salts by bacterium isolated from the air environment of a tannery. FEMS Microbiol. Lett. 213, 1–6. doi: 10.1111/j.1574-6968.2002.tb11277.x
Felsenstein, J. (1985). Confidence limits on phylogenies: an approach using the bootstrap. Evolution 39, 783–791. doi: 10.1111/j.1558-5646.1985.tb00420.x
Fiske, C., and Subbarow, Y. (1925). The colorimetric determination of phosphorus. Biol. Chem. 66, 375–400. doi: 10.1016/S0021-9258(18)84756-1
Ftayeh, R. M., von Tiedemann, A., and Rudolph, K. W. (2011). A new selective medium for isolation of Clavibacter michiganensis subsp. michiganensis from tomato plants and seed. Phytopathology 101, 1355–1364. doi: 10.1094/PHYTO-02-11-0045
Gamalero, E., Trotta, A., Massa, N., Copetta, A., Martinotti, M. G., and Berta, G. (2004). Impact of two fluorescent pseudomonads and an arbuscular mycorrhizal fungus on tomato plant growth, root architecture and P acquisition. Mycorrhiza 14, 185–192. doi: 10.1007/s00572-003-0256-3
Garrido-Sanz, D., Meier-Kolthoff, J. P., Göker, M., Martín, M., Rivilla, R., and Redondo-Nieto, M. (2016). Genomic and genetic diversity within the Pseudomonas fluorescens complex. PLoS One 11:e0150183. doi: 10.1371/journal.pone.0150183
Gautam, S., Chauhan, A., Sharma, R., Sehgal, R., and Shirkot, C. K. (2019). Potential of Bacillus amyloliquefaciens for biocontrol of bacterial canker of tomato incited by Clavibacter michiganensis ssp. michiganensis. Microb. Pathog. 130, 196–203. doi: 10.1016/j.micpath.2019.03.006
Gravel, V., Antoun, H., and Tweddell, R. J. (2007). Growth stimulation and fruit yield improvement of greenhouse tomato plants by inoculation with Pseudomonas putida or Trichoderma atroviride: possible role of indole acetic acid (IAA). Soil Biol. Biochem. 39, 1968–1977. doi: 10.1016/j.soilbio.2007.02.015
Gyaneshwar, P., Kumar, G. N., Parekh, L. J., and Poole, P. S. (2002). Role of soil microorganisms in improving P nutrition of plants. Plant Soil 245, 83–93. doi: 10.1023/A:1020663916259
Ham, B. K., Chen, J., Yan, Y., and Lucas, W. J. (2018). Insights into plant phosphate sensing and signaling. Curr. Opin. Biotechnol. 49, 1–9. doi: 10.1016/j.copbio.2017.07.005
Illmer, P., and Schinner, F. (1995). Solubilization of inorganic calcium phosphates solubilization mechanisms. Soil Biol. Biochem. 27, 257–263. doi: 10.1016/0038-0717(94)00190-C
Ipek, M., Pirlak, L., Esitken, A., Figen Dönmez, M., Turan, M., and Sahin, F. (2014). Plant growth-promoting rhizobacteria (PGPR) increase yield, growth and nutrition of strawberry under high-calcareous soil conditions. J. Plant Nutr. 37, 990–1001. doi: 10.1080/01904167.2014.881
Jain, D., Saheewala, H., Sanadhaya, S., Joshi, A., Bhojiya, A. A., Verma, A. K., et al. (2022). “Potassium solubilizing microorganisms as soil health engineers: an insight into molecular mechanism” in Rhizosphere engineering. eds. R. C. Dubey and P. Kumar (USA: Academic Press), 199–214. doi: 10.1016/C2020-0-03385-2
Jha, Y., and Subramanian, R. B. (2018). Effect of root-associated bacteria on soluble sugar metabolism in plant under environmental stress. in Plant metabolites and regulation under environmental stress. Ed. A. Parvaiz. Amsterdam, The Netherlands: Elsevier, 231–240. doi: 10.1016/B978-0-12-812689-9.00012-1
Jukes, T. H., and Cantor, C. R. (1969). “Evolution of protein molecules” in Mammalian Protein Metabolism. Ed. H. N. Munro, vol. 3 (New York: Academic Press), 21–132.
Kandel, S. L., Joubert, P. M., and Doty, S. L. (2017). Bacterial endophyte colonization and distribution within plants. Microorganisms 5:E77. doi: 10.3390/microorganisms5040077
Khan, N., Bano, A., and Babar, M. D. A. (2019). The stimulatory effects of plant growth promoting rhizobacteria and plant growth regulators on wheat physiology grown in sandy soil. Arch. Microbiol. 201, 769–785. doi: 10.1007/s00203-019-01644-w
Khan, A. A., Jilani, G., Akhtar, M. S., Naqvi, S., and Rasheed, M. (2009). Phosphorus solubilizing bacteria: occurrence, mechanisms and their role in crop production. J. Agric. Biol. Sci. 1, 48–58.
Koo, Y. M., Heo, A. Y., and Choi, H. W. (2023). Isolation and identification antagonistic bacterium Paenibacillus tianmuensis YM002 against Acidovorax citrulli. Front. Plant Sci. 14:1173695. doi: 10.3389/fpls.2023.1173695
Kour, D., Rana, K. L., Yadav, A. N., Yadav, N., Kumar, M., Kumar, V., et al. (2020). Microbial biofertilizers: Bioresources and eco-friendly technologies for agricultural and environmental sustainability. Biocatal. Agric. Biotechnol. 23, 101487. doi: 10.1016/j.bcab.2019.101487
Lelliott, R. A., Billing, E., and Hayward, A. C. (1966). A determinative scheme for the fluorescent plant pathogenic pseudomonads. J Appl Bacteriol. 29,470–89. doi: 10.1111/j.1365-2672.1966.tb03499.x
Lemanceau, P., Robin, A., Mazurier, S., and Vansuyt, G. (2007). “Implication of pyoverdines in the interactions of fluorescent pseudomonads with soil microflora and plant in the rhizosphere” in Microbial Siderophores. Soil biology. eds. A. Varma and S. B. Chincholkar, vol. 12 (Berlin, Heidelberg: Springer).
Lesueur, D., Deaker, R., Herrmann, L., Bräu, L., and Jansa, J. (2016). “The production and potential of biofertilizers to improve crop yields” in Bioformulations: for sustainable agriculture. eds. N. Arora, S. Mehnaz, and R. Balestrini (New Delhi: Springer).
Lugtenberg, B., and Kamilova, F. (2009). Plant-growth-promoting rhizobacteria. Ann. Rev. Microbiol. 63, 541–556. doi: 10.1146/annurev.micro.62.081307.162918
Ma, Q., He, S., Wang, X., Rengel, Z., Chen, L., Wang, X., et al. (2023). Isolation and characterization of phosphate-solubilizing bacterium Pantoea rhizosphaerae sp. nov. from Acer truncatum rhizosphere soil and its effect on Acer truncatum growth. Front. Plant Sci. 14:1218445. doi: 10.3389/fpls.2023.1218445
Marra, L. M., de Oliveira-Longatti, S. M., Soares, C. R., de Lima, J. M., Olivares, F. L., and Moreira, F. M. (2015). Initial pH of medium affects organic acids production but do not affect phosphate solubilization. Braz. J. Microbiol. 46, 367–375. doi: 10.1590/S1517-838246246220131102
Mavrodi, O. V., McWilliams, J. R., Peter, J. O., Berim, A., Hassan, K. A., Elbourne, L. D. H., et al. (2021). Root exudates alter the expression of diverse metabolic, transport, regulatory, and stress response genes in rhizosphere Pseudomonas. Front. Microbiol. 12:651282. doi: 10.3389/fmicb.2021.651282
Maxwell, K., and Johnson, G. N. (2000). Chlorophyll fluorescence-a practical guide. J. Exp. Bot. 51, 659–668. doi: 10.1093/jxb/51.345.659
Meena, V. S., Maurya, B. R., Verma, J. P., Aeron, A., Kumar, A., Kim, K., et al. (2015). Potassium solubilizing rhizobacteria (KSR): isolation, identification, and K-release dynamics from waste mica. Ecol. Eng. 81, 340–347. doi: 10.1016/j.ecoleng.2015.04.065
Mirza, M. S., Ahmad, W., Latif, F., Haurat, J., Bally, R., Normand, P., et al. (2001). Isolation, partial characterization, and the effect of plant growth-promoting bacteria (PGPB) on micro-propagated sugarcane in vitro. Plant Soil 237, 47–54. doi: 10.1023/A:1013388619231
Misra, S., and Chauhan, P. S. (2020). ACC deaminase-producing rhizosphere competent Bacillus spp. mitigate salt stress and promote Zea mays growth by modulating ethylene metabolism. 3 Biotech 10, 119–114. doi: 10.1007/s13205-020-2104-y
Mozzetti, C., Ferraris, L., Tamietti, G., and Matta, A. (1995). Variation in enzyme activities in leaves and cell suspensions as markers of incompatibility in different Phytophthora–pepper interactions. Physiol. Mol. Plant Pathol. 46, 95–107. doi: 10.1006/pmpp.1995.1008
Nam, H. S., Yang, H. J., Oh, B. J., Anderson, A. J., and Kim, Y. C. (2016). Biological control potential of Bacillus amyloliquefaciens KB3 isolated from the feces of Allomyrina dichotoma larvae. Plant Pathol. J. 32, 273–280. doi: 10.5423/PPJ.NT.12.2015.0274
Nautiyal, C. S. (1999). An efficient microbiological growth medium for screening phosphate solubilizing microorganisms. FEMS Microbiol. Lett. 170, 265–270. doi: 10.1111/j.1574-6968.1999.tb13383.x
Olsen, S. R., and Sommers, L. E. (1982). “Phosphorus” in Methods of soil analysis part 2. Chemical and microbiological properties. eds. A. L. Page, et al. (Madison: American Society of Agronomy, Soil Science Society of America), 403–430. doi: 10.2134/agronmonogr9.2.2ed
Parmar, P., and Sindhu, S. S. (2013). Potassium solubilization by rhizosphere bacteria: influence of nutritional and environmental conditions. J. Microbiol. Res. 3, 25–31. doi: 10.5923/j.microbiology.20130301.04
Pikovskaya, R. I. (1948). Mobilization of phosphorus in soil connection with the vital activity of some microbial species. Microbiol 17, 362–370.
Pirlak, L., and Köse, M. (2009). Effects of plant growth promoting rhizobacteria on yield and some fruit properties of strawberry. J. Plant Nutr. 32, 1173–1184. doi: 10.1080/01904160902943197
Raaijmakers, J. M., Vlami, M., and De Souza, J. T. (2002). Antibiotic production by bacterial biocontrol agents. Antonie Van Leeuwenhoek 81, 537–547. doi: 10.1023/a:1020501420831
Rajawat, M. V., Singh, S., and Saxena, A. K. (2014). A new spectrophotometric method for quantification of potassium solubilized by bacterial cultures. Indian J. Exp. Biol. 52, 261–266.
Riaz, U., Murtaza, G., Anum, W., Samreen, T., Sarfraz, M., and Nazir, M. Z. (2021). “Plant growth-promoting rhizobacteria (PGPR) as biofertilizers and biopesticides” in Microbiota and biofertilizers. eds. K. R. Hakeem, G. H. Dar, M. A. Mehmood, and R. A. Bhat (Cham: Springer).
Richardson, A. E. (2001). Prospects for using soil microorganisms to improve the acquisition of phosphorus by plants. Funct. Plant Biol. 28, 897–906. doi: 10.1071/PP01093
Richardson, A. E., Barea, J.-M., McNeill, A. M., and Prigent-Combaret, C. (2009). Acquisition of phosphorus and nitrogen in the rhizosphere and plant growth promotion by microorganisms. Plant Soil 321, 305–339. doi: 10.1007/s11104-009-9895-2
Richardson, A. E., Lynch, J. P., Ryan, P. R., Delhaize, E., Smith, F. A., Smith, S. E., et al. (2011). Plant and microbial strategies to improve the phosphorus efficiency of agriculture. Plant Soil 349, 121–156. doi: 10.1007/s11104-011-0950-4
Robin, A., Vansuyt, G., Hinsinger, P., Meyer, J. M., Briat, J. F., and Lemanceau, P. (2008). “Iron dynamics in the rhizosphere: consequences for plant health and nutrition” in Advances in agronomy. ed. D. L. Sparks, (London, UK: Academic Press). vol. 99, 183–225.
Rodríguez, H., Fraga, R., Gonzalez, T., and Bashan, T. (2006). Genetics of phosphate solubilization and its potential applications for improving plant growth-promoting bacteria. Plant Soil 287, 15–21. doi: 10.1007/s11104-006-9056-9
Saitou, N., and Nei, M. (1987). The neighbor-joining method: a new method for reconstructing phylogenetic trees. Molec. Biol. Evol. 4, 406–425. doi: 10.1093/oxfordjournals.molbev.a040454
Sasse, J., Martinoia, E., and Northen, T. (2018). Feed your friends: do plant exudates shape the root microbiome? Trends Plant Sci. 23, 25–41. doi: 10.1016/j.tplants.2017.09.003
Stanier, R. Y., Palleroni, N. J., and Doudoroff, M. (1966). The aerobic pseudomonads a taxonomic study. J. Gen. Microbiol. 43, 159–271. doi: 10.1099/00221287-43-2-159
Stecher, G., Tamura, K., and Kumar, S. (2020). Molecular evolutionary genetics analysis (MEGA) for macOS. Mol. Biol. Evol. 37, 1237–1239. doi: 10.1093/molbev/msz312
Sun, F., Ou, Q., Wang, N., Guo, Z. X., Ou, Y., Li, N., et al. (2020). Isolation and identification of potassium-solubilizing bacteria from Mikania micrantha rhizospheric soil and their effect on M. micrantha plants. Glob. Ecol. Conserv. 23:e01141. doi: 10.1016/j.gecco.2020
Tamura, K., Nei, M., and Kumar, S. (2004). Prospects for inferring very large phylogenies by using the neighbor-joining method. Proc. Natl. Acad. Sci. USA 101, 11030–11035. doi: 10.1073/pnas.0404206101
Tamura, K., Stecher, G., and Kumar, S. (2021). MEGA 11: molecular evolutionary genetics analysis version 11. Mol. Biol. Evol. 38, 3022–3027. doi: 10.1093/molbev/msab120
Tuna, A. L., Kaya, C., Ashraf, M., Altunlu, H., Yokas, I., and Yagmur, B. (2007). The effects of calcium sulphate on growth, membrane stability and nutrient uptake of tomato plants grown under salt stress. Environ. Exp. Bot. 59, 173–178. doi: 10.1016/j.envexpbot.2005.12.007
Umesha, S. (2006). Phenylalanine ammonia-lyase activity in tomato seedlings and its relationship to bacterial canker disease resistance. Phytoparasitica 34, 68–71. doi: 10.1007/BF02981341
Velikova, V., Yordanov, I., and Edereva, A. (2000). Oxidative stress and some antioxidant systems in acid rain-treated bean plants. Protective role of exogenous polyamines. Plant Sci. 151, 59–66. doi: 10.1016/S0168-9452(99)00197-1
Wang, Y., Deng, S., Li, Z., and Yang, W. (2022). Advances in the characterization of the mechanism underlying bacterial canker development and tomato plant resistance. Horticulturae 8:209. doi: 10.3390/horticulturae8030209
Wang, N., Wang, L., Zhu, K., Hou, S., Chen, L., Mi, D., et al. (2019). Plant root exudates are involved in Bacillus cereus AR156 mediated biocontrol against Ralstonia solanacearum. Front. Microbiol. 10:98. doi: 10.3389/fmicb.2019.00098
Weisburg, W. G., Barns, S. M., Pelletier, D. A., and Lane, D. J. (1991). 16S ribosomal DNA amplification for phylogenetic study. J. Bacteriol. 173, 697–703. doi: 10.1128/jb.173.2.697-703.1991
Wen, T., Zhao, M., Yuan, J., Kowalchuk, G. A., and Shen, Q. (2021). Root exudates mediate plant defense against foliar pathogens by recruiting beneficial microbes. Soil Ecol. Lett. 3, 42–51. doi: 10.1007/s42832-020-0057-z
Yahya, M., Islam, E. U., Rasul, M., Farooq, I., Mahreen, N., Tawab, A., et al. (2021). Differential root exudation and architecture for improved growth of wheat mediated by phosphate solubilizing bacteria. Front. Microbiol. 12:744094. doi: 10.3389/fmicb.2021.744094
Zhang, N., Wang, D., Liu, Y., Li, S., Shen, Q., and Zhang, R. (2014). Effects of different plant root exudates and their organic acid components on chemotaxis, biofilm formation and colonization by beneficial rhizosphere-associated bacterial strains. Plant Soil 374, 689–700. doi: 10.1007/s11104-013-1915-6
Keywords: Bacillus , biofertilization, rock phosphate, plant growth promotion, Pseudomonas , solubilization, induced systemic resistance
Citation: Bakki M, Banane B, Marhane O, Esmaeel Q, Hatimi A, Barka EA, Azim K and Bouizgarne B (2024) Phosphate solubilizing Pseudomonas and Bacillus combined with rock phosphates promoting tomato growth and reducing bacterial canker disease. Front. Microbiol. 15:1289466. doi: 10.3389/fmicb.2024.1289466
Edited by:
Puneet Singh Chauhan, National Botanical Research Institute (CSIR), IndiaReviewed by:
Satyendra Pratap Singh, Agricultural Research Organization (ARO), IsraelKrishnamoorthy Ramasamy, Tamil Nadu Agricultural University, India
Copyright © 2024 Bakki, Banane, Marhane, Esmaeel, Hatimi, Barka, Azim and Bouizgarne. This is an open-access article distributed under the terms of the Creative Commons Attribution License (CC BY). The use, distribution or reproduction in other forums is permitted, provided the original author(s) and the copyright owner(s) are credited and that the original publication in this journal is cited, in accordance with accepted academic practice. No use, distribution or reproduction is permitted which does not comply with these terms.
*Correspondence: Brahim Bouizgarne, Yi5ib3Vpemdhcm5lQHVpei5hYy5tYQ==