- Programa de Ingeniería Genómica, Centro de Ciencias Genómicas, Universidad Nacional Autónoma de México, Cuernavaca, Mexico
Double-strand breaks (DSBs) are the most dangerous injuries for a genome. When unrepaired, death quickly ensues. In most bacterial systems, DSBs are repaired through homologous recombination. Nearly one-quarter of bacterial species harbor a second system, allowing direct ligation of broken ends, known as Non-Homologous End Joining (NHEJ). The relative role of both systems in DSBs repair in bacteria has been explored only in a few cases. To evaluate this in the bacterium Rhizobium etli, we used a modified version of the symbiotic plasmid (264 kb), containing a single copy of the nifH gene. In this plasmid, we inserted an integrative plasmid harboring a modified nifH gene fragment containing an I-SceI site. DSBs were easily inflicted in vivo by conjugating a small, replicative plasmid that expresses the I-SceI nuclease into the appropriate strains. Repair of a DSB may be achieved through homologous recombination (either between adjacent or distant repeats) or NHEJ. Characterization of the derivatives that repaired DSB in different configurations, revealed that in most cases (74%), homologous recombination was the prevalent mechanism responsible for repair, with a relatively minor contribution of NHEJ (23%). Inactivation of the I-SceI gene was detected in 3% of the cases. Sequence analysis of repaired derivatives showed the operation of NHEJ. To enhance the number of derivatives repaired through NHEJ, we repeated these experiments in a recA mutant background. Derivatives showing NHEJ were readily obtained when the DSB occurred on a small, artificial plasmid in a recA mutant. However, attempts to deliver a DSB on the symbiotic plasmid in a recA background failed, due to the accumulation of mutations that inactivated the I-SceI gene. This result, coupled with the absence of derivatives that lost the nonessential symbiotic plasmid, may be due to an unusual stability of the symbiotic plasmid, possibly caused by the presence of multiple toxin-antitoxin modules.
Introduction
Double-strand breaks (DSB) are a common, yet deadly form of DNA damage; for that reason, bacteria have developed multiple mechanisms for repairing them (Baharoglu and Mazel, 2014). In the presence of either homologous DNA or repeated sequences, most bacteria can repair a DSB faithfully by homologous recombination through a variety of mechanisms (Ayora et al., 2011; Michel and Leach, 2012; Glickman, 2014; Kowalczykowski, 2015; Verma and Greenberg, 2016), depicted in Figure 1. After a DSB (Figure 1A), a helicase-nuclease protein complex resects damaged DNA exposing a 3’ DNA overhang to which the RecA protein binds (Figure 1B). According to double-strand break repair model (DSBR), the RecA protein stimulates the pairing of the 3′ single strand DNA to its homologous sequence and, through DNA polymerization, causes the displacement of the complementary strand (Figure 1C). This strand displacement allows the other 3′ end to anneal to its homolog and polymerize too. This combination of processes ends in the formation of a double Holliday Junction (Figure 1D, DSBR). Resolution of both Holliday Junctions (Figure 1E) generates two possible outcomes: crossover (CO, Figure 1E) or gene conversion (GC, Figure 1E). A variation of this process, called synthesis-dependent strand annealing (SDSA), could also repair a DSB without needing a Holliday Junction. According to this model, DNA resection exposes 3′ overhangs, RecA pairs to the homologous sequences and DNA polymerization, using the homologous strand as a template, effectively bridges the region affected by the DSB (Figures 1A–C). At this point, the polymerized strand stops binding to its homolog and relocates to the other end of the damaged DNA (Figure 1D, SDSA). Since the other end of the damaged DNA has an exposed 3′ end, it can anneal with the newly synthesized DNA. DNA repair terminates with the polymerization of the complementary DNA (Figure 1E, SDSA). An alternative mode of repair, called single-strand annealing (SSA), may operate when a DSB occurs in the interval between two repeated sequences (Tomso and Kreuzer, 2000; Bzymek and Lovett, 2001; Glickman, 2014; Ithurbide et al., 2015). Resection of DNA on both sides of the DSB exposing 3′ ends could leave two single-strand DNA fragments within the repeated sequence (Figure 1C, SSA). Complementarity between the single-stranded repeats allows annealing (Figure 1C, SSA). Repairing the DSB concludes with trimming any single-strand DNA that does not complement each other, thus causing a deletion (Figure 1C). SSA is most prevalent with repeats of small size (< 300 bp) and its operation is reduced upon increasing distance between the repeats (Bzymek and Lovett, 2001, and references therein).
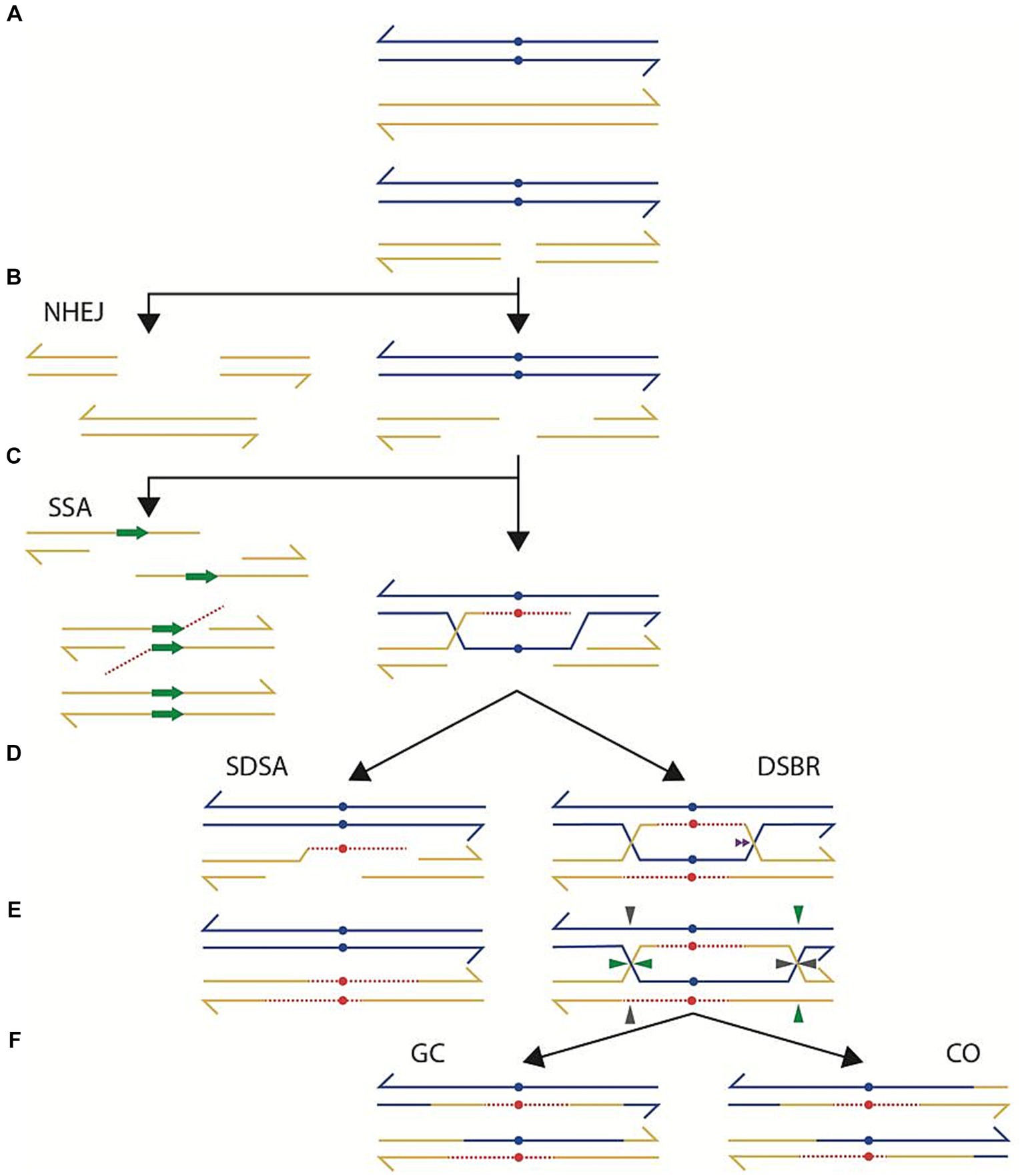
Figure 1. Repair of a double-strand break occurs through a variety of intracellular mechanisms. (A) depicts two DNA duplexes, carrying sequence differences (blue dots). One of the duplexes receives a double-strand break, which is processed by degradation (B, right part) to generate protruding 3’ends. One of the protruding 3’ends invades the uncut homolog (C, right part), synthesizing a complementary strand (interrupted red line) and displacing one of the strands from the uncut homolog. Annealing of the displaced strand with the cut homolog, according to the Double Strand Break Repair model (D, DSBR), allows complementary strand synthesis (red), generating a double Holiday junction that can migrate. Resolution of the double Holiday junction (E) by cutting in different orientations (horizontal-vertical or vertical-horizontal) generates a repaired product harboring a crossover (CO, F) with associated gene conversion. Alternatively, the intermediate in E can be cut in the same orientation (either horizontal-horizontal or vertical-vertical), generating a gene conversion product (GC, F) without an associated crossover. Other modes of repair of a DSB are also possible. According to the Synthesis-Dependent, Strand Annealing model (SDSA) the intermediate in C (right part), after DNA synthesis, may regress to its original location, effectively bridging the degraded sector (D, left part). DNA synthesis, using as a template the regressed strand, followed by ligation, generates a repaired product, harboring a gene conversion without an associated crossover. Other repair modes entail a more extensive degradation. In the case of Non-Homologous End Joining (NHEJ), the DSB in A is subjected to more extensive degradation (B, left), followed by ligation of the resulting ends. Alternatively, the intermediate in B (right part) is processed further by single-strand degradation until finding repeated sequences in a direct orientation (C, left part). Single-Strand Annealing (SSA) between the repeated regions, followed by gap repair and ligation generates a product with a deletion.
An alternative repair mechanism, prevalent in eukaryotes but present only in 20% of sequenced bacterial genomes (Sharda et al., 2020; Manoj and Kuhlman, 2023), is non-homologous end joining (NHEJ). Some bacteria such as Bacillus subtilis (De Vega, 2013), Pseudomonas putida (Paris et al., 2015), different Mycobacterium (Matthews and Simmons, 2014), Sinorhizobium meliloti (Kobayashi et al., 2008 Dupuy et al., 2017), and Streptomyces ambofaciens (Hoff et al., 2016) have a set of proteins that allows repair of DSB independently of RecA, through NHEJ. In these bacteria, NHEJ repair depends on a few functions: the recognition of the DSB, done by Ku protein, the remodeling of the 3′ terminus of the DSB, either by adding or deleting nucleotides, performed by the POL and PE domains of LigD, and the joining of broken ends, done by the LIG domain of LigD (Della et al., 2004). As depicted in Figure 1B, NHEJ is a simpler repair mechanism, than can generate deletions or nucleotide insertions.
Rhizobium etli is an α-proteobacterium able to fix atmospheric nitrogen in a symbiotic association with bean plants. In R. etli CFN42 (the type-strain), the genome comprises three kinds of circular molecules: the main chromosome, one secondary chromosome and five large, dispensable plasmids (González et al., 2006). One of these plasmids, p42d (371.2 kb), contains most of the genes required for nodulation and nitrogen fixation, and was designated as the symbiotic plasmid or pSym. R. etli has been shown to do homologous recombination efficiently. Homologous recombination between identical gene copies of the nitrogenase reductase gene (nifH) provokes a large deletion in the pSym (pSymΔ, 267.7 kb), being a frequent cause of loss of symbiotic abilities (Romero et al., 1991). Similar recombination events between other repeated sequences may also affect symbiotic abilities (Romero et al., 1995). The relative participation of homologous recombination proteins such as RecA (Martínez-Salazar et al., 1991), RecFOR and AddAB (Zuñiga-Castillo et al., 2004), MutS (Santoyo et al., 2005) and RuvB, RecG, and RadA (Martínez-Salazar and Romero, 2000; Castellanos and Romero, 2009; Martínez-Salazar et al., 2009) has already been evaluated in this bacterium, both for crossover formation and gene conversion. Other proteins such as RecQ, RecJ and RecN, among others, have been identified and while its function can be inferred from literature, their participation in DNA repair in R. etli has not been assessed yet.
R. etli also possesses all proteins required, in other bacteria, to repair DNA by NHEJ. Four copies of the ku gene have been identified, two adjacent to each other on the secondary chromosome (named p42e) and two on the main chromosome. Close to the ku gene copies there are copies of the ligD gene; only one of these (on the secondary chromosome) harbors the LIG, PE and POL domains. This suggests that R. etli can repair DSB by NHEJ, although no evidence for this type of repair has been described yet. A similar arrangement was described for the related bacterium Sinorhizobium meliloti, where four copies of the ku gene and five copies of the ligD gene were found (Kobayashi et al., 2008). Mutational analysis in S. meliloti revealed that the four copies of the ku gene are required for repair of DSBs induced by ionizing radiation treatment, The sku2 gene copy appears to be the more relevant gene for DSB repair in this bacterium, based on its expression pattern and the substantial reduction in survival of a mutant in this gene after treatment with ionizing radiation (Kobayashi et al., 2008). Interestingly, the NHEJ system in S. meliloti appears to contribute to resistance to hydrogen peroxide treatment and desiccation tolerance (Dupuy et al., 2017). Unfortunately, no attempts were made in these studies to characterize how DSBs were repaired. While NHEJ is well-regulated and plays a major role in DNA repair in eukaryotes, in bacteria homologous recombination is the most widespread mechanism for DNA repair. How and when NHEJ participates remains poorly understood. More importantly, the relative participation of NHEJ in the repair of DSB, along with other repair pathways, has been assessed only for a few bacteria, including Mycobacterium smegmatis (Gupta et al., 2011), Pseudomonas putida (Paris et al., 2015) and Sinorhizobium meliloti (Dupuy et al., 2019).
In this work, we are interested in understanding how DSB are repaired on non-essential DNA such as R. etli megaplasmids. We evaluate the relative contribution of homologous recombination and no-homologous end joining for repair and characterize the type of events produced by non-homologous end joining.
Results
Inducing double-strand breaks on a megaplasmid
To gain insight into the relative role of homologous recombination and NHEJ in R. etli, we devised a genetic system, based on the symbiotic plasmid (371 kb) of this bacterium. To facilitate the analysis, a deleted version of the pSym was employed (pSymΔ, 268 kb) and cut in vivo with an I-SceI nuclease, which recognizes a unique 18 bp site, to generate double-strand breaks on R. etli pSymΔ.
To generate a R. etli strain with an I-SceI site on pSymΔ, we used plasmid pJGus28-ISceI (Yáñez-Cuna et al., 2016) as a template for amplifying a 260 bp DNA fragment containing the I-SceI site surrounded by 120 bp of the nifH gene. This PCR product was then cloned into an integrative plasmid (see Materials and Methods), giving rise to pOY-ISceI. Introduction of this plasmid into R. etli harboring pSymΔ generates a cointegrate molecule between pSymΔ and pOY-ISceI (Figure 2). There are two possible outcomes for this cointegration. In the first, non-reciprocal transfer of DNA (gene conversion) results in a cointegrate, with both truncated nifH copies now containing the I-SceI site (Figure 2B), giving rise to strain CFNX55D2. In the second, a reciprocal transfer of DNA upon recombination (simple crossover), generates a cointegrate with two truncated versions of the nifH gene, one of which harbors the I-SceI site (Figure 2C) giving rise to strain CFNX55D1. To induce a double-stranded break, biparental matings were done between either CFNX55D1 or CFNX55D2 with an E. coli carrying plasmid pJN105mega, which expresses the I-SceI gene under the control of the araBAD promoter. Isolates recovered after inducing a DSB can be readily classified into homologous recombination, NHEJ and other classes by determinations of plasmid size and amplification with specific primers (Materials and Methods and Supplementary Table S1).
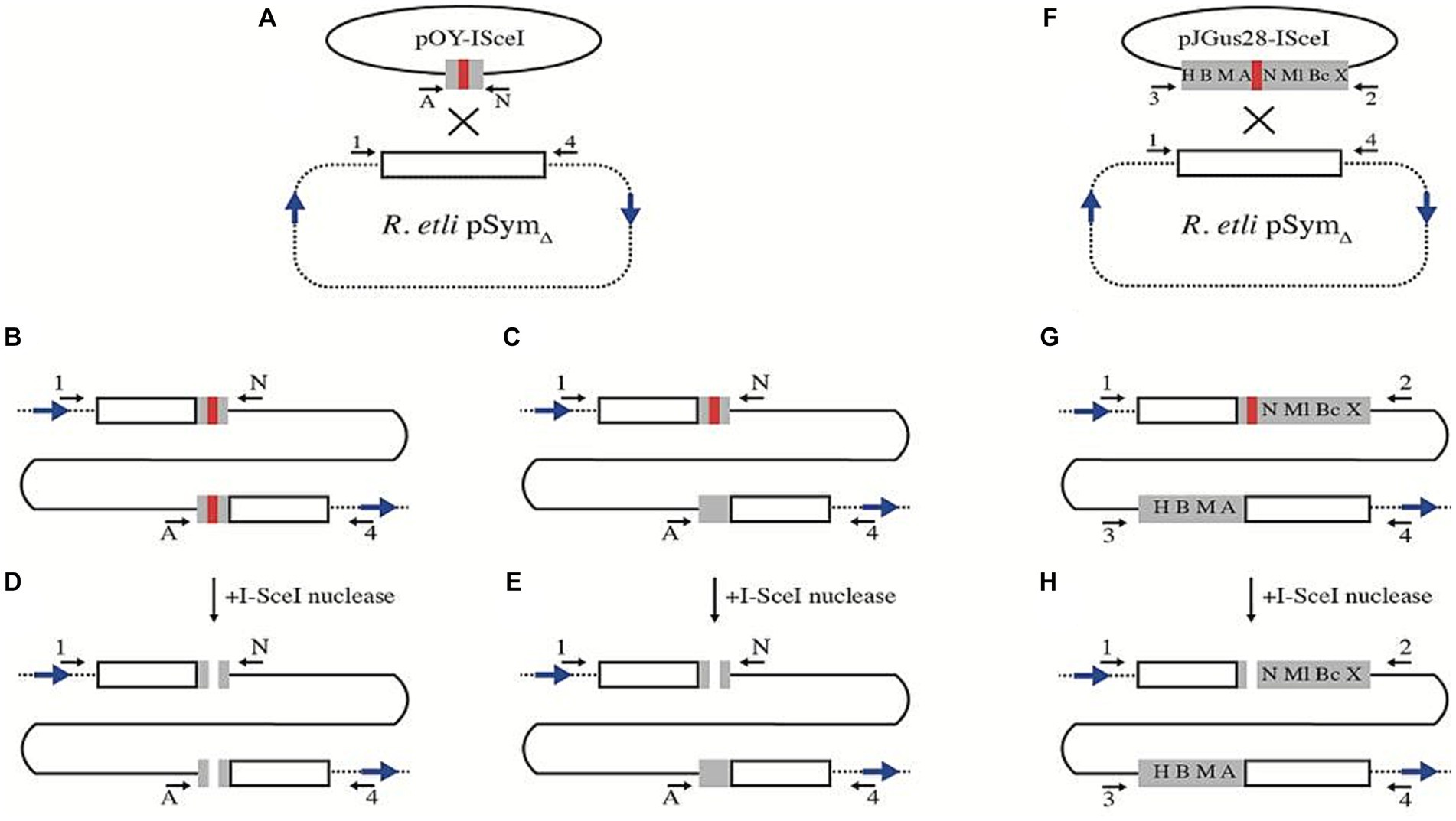
Figure 2. Strategy for the introduction of I-SceI sites on pSymΔ. (A) Plasmid pOY-ISceI, harboring a 260 bp segment from the nifH gene, interrupted by an I-SceI site (red bar) was integrated by homologous recombination into pSymΔ. When recombination is accompanied by gene conversion, both duplicated segments of nifH contain I-SceI sites (strain CFNX55D2, B). Recombination without an associated gene conversion generates a duplication of a segment of nifH, with the I-SceI site in one of the duplicates (strain CFNX55D1, C). Introduction of the I-SceI nuclease provokes the generation of DSBs either on both (D) or in a single nifH repeat (E). For introduction of a larger nifH repeat, plasmid pJGus28-ISceI was used (F). This plasmid carries a 960 bp sector from nifH, containing a series of single-base changes generating restriction sites (H, HindIII; B, BamHI; M, MaeIII; A, ApaLI; N, NarI; Ml, MluI; Bc, BclI; X, XbaI), as well as the I-SceI site (red bar). Recombination without gene conversion on pSymΔ leads to the formation of 960 bp nifH repeats, with the I-SceI site in one of the repeats (G). This repeat can be cut upon introduction of the I-SceI nuclease (H). In all panels, blue arrows mark the relative position of F7 repeats, while black arrows (either lettered or numbered) mark the position of PCR primers used for characterization (see Materials and Methods and Supplementary Table S1).
Repair of DNA breaks with no close homologous regions
Since strain CFNX55D2 contains a small, 260 bp repeated region comprising part of the nifH gene and the I-SceI site, expression of the I-SceI nuclease generates two double-strand breaks on pSymΔ, with no possibility for the homologous region to be used for repair (Figure 2B). In this case, there are three possible outcomes: (i) an unrepaired pSymΔ could get lost, given that there is no essential DNA on it or, (ii) a DSB could be repaired by NHEJ, leaving a pSymΔ roughly of the same size, or (iii) pSymΔ could be repaired by recombination between distant repeated sequences present on the plasmid. There is ample precedent for recombination events between repeated sequences, other than nifH, generating deletions on the pSym (Romero et al., 1995). The most prevalent deletion we obtained, type IV deletion, was generated by recombination between repeated sequences flanking nifH, producing a plasmid of 217 kb (Romero et al., 1995). However, the specific sequences involved in the deletion were not characterized at that time. To solve this, a bioinformatic search for nearly identical repeated sequences longer than 100 bp on the pSym was done (Materials and Methods and Supplementary Table S2). The list of repeated sequences was parsed, retaining only those that are in a direct orientation, flanking the nifH gene remaining in pSymΔ. Recombination between direct repeats of the F7 family (871 bp long, 98% identical) appear to be responsible for generation of type IV deletions.
Introduction by conjugation of plasmid pJN105mega (expressing the I-SceI nuclease) into R. etli strain CFNX55 (harboring pSymΔ without I-SceI sites) occurs readily, at a frequency of 7.43 ×10−4 (Table 1, line 1). In contrast, when pJN105mega was introduced into R. etli CFNX55D2 (harboring pSymΔ with two I-SceI sites), a one-thousand-fold reduction in conjugation frequency was seen (Table 1, line 2), suggesting a large effect of a DSB on transconjugant survival. To characterize the mode of repair of the DSB produced, ten independent biparental matings between R. etli CFNX55D2 and E. coli S17-I containing pJN105mega plasmid were set up, selecting not more than 5 transconjugants from each mating, to minimize the occurrence of siblings. Detailed characterization of the fifty transconjugants is presented in Supplementary Table S3, while an overview of the results is shown in Table 1.
None of the isolates characterized presented the loss of pSymΔ. In most of the cases (46 out of 50), pSymΔ was reduced in size from 268 kb to 217 kb, coinciding with the plasmid size expected for a type IV deletion. Such a deletion would remove a large segment, including the pOY-ISceI cointegrate and the two I-SceI sites, thus avoiding further events of DSB. To verify that homologous recombination between distant F7 repeats generated this deletion, amplification with appropriate primers was done, evaluating the presence of each individual repeat (F7a and F7b) and the predicted joinpoint (F7a/b, see Materials and Methods). In these 46 cases, no amplification was observed for the individual repeats, but a strong amplification was detected for the predicted joinpoint. Absence of the pOY-ISceI cointegrate and corresponding I-SceI sites was also verified by specific amplification reactions. These results validate that repair of the DSB was achieved by homologous recombination between distant F7 repeats. Of the remaining transconjugants, three of them appear to use a common mechanism for survival. All three have a plasmid matching in size pSymΔ; amplifications with appropriate primers revealed that the pOY-ISceI plasmid is still cointegrated and that both I-SceI sites are still present, ruling out NHEJ. To verify the integrity of the I-SceI gene, amplifications were carried out with primers complementary to that gene. In all three transconjugants, this reaction produced a larger PCR product than expected for the nuclease gene, indicating that an insertion had inactivated the function of the nuclease (see below). PCRs from the last transconjugant indicated that a repair process different from the ones described before had happened. PCRs targeting the nifH gene and the I-SceI sites did not gave a product, which meant that the digestion had happened yet either the pOY-ISceI plasmid was still cointegrated or that whole region was deleted. The latter being demonstrated by the fact the transconjugant could not grow in kanamycin media. PCRs targeting the F7b repeat generated a product, but the one targeting the F7a repeat, did not. We did not investigate the origin of this derivative any further.
Repair of DNA breaks with an adjacent homologous region
As explained before, cointegration of pOY-ISceI into pSymΔ may also generate a strain in which only one truncated nifH gene contains an I-SceI recognition site (strain CFNX55D1, Figure 2C). Upon expression of the I-SceI nuclease in this strain, a DSB should occur on the truncated version of the nifH harboring the I-SceI site (Figure 2E). Since the other truncated version of the nifH gene remains uncut, it is available for recombinational repair of the DSB. The homologous region between the truncated copies of nifH is 244 bp long before digestion with I-SceI, and about 119 bp on each side after digestion. Although small, the adjacent homologous region should suffice for recombination, allowing the DNA break repair (Figure 3). Recombination between adjacent nifH repeats would occur by simple crossover (leading to excision of the pOY-ISceI cointegrate, Figure 3E) or by gene conversion (maintaining the pOY-ISceI cointegrate but eliminating the I-SceI site by gene conversion using the uncut homolog as a donor, Figure 3F). In this strain, recombination between distant F7 repeats is also possible (Figure 3D), as well as SceI inactivation (Figure 3G) and non-homologous end joining (NHEJ, Figure 3H).
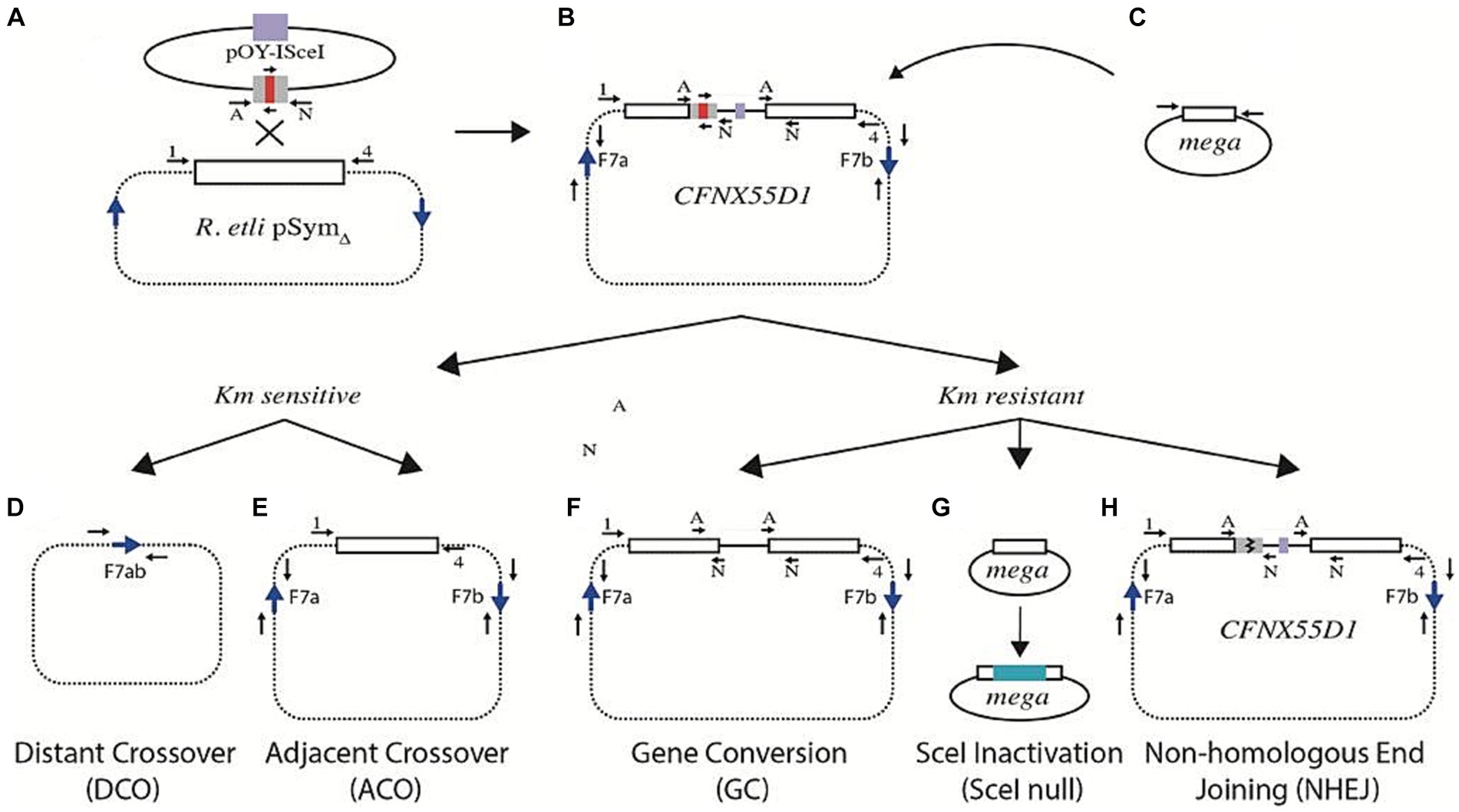
Figure 3. Possible events responsible for repair of a DSB in strain CFNX55D1. (A) Plasmid pOY-ISceI [carrying a 260 bp segment from the nifH gene interrupted by an I-SceI site (red bar)] was integrated by homologous recombination into pSymΔ, leading to introduction of an I-SceI site (B). Upon introduction of a small plasmid carrying the gene for the I-SceI meganuclease (C), a DSB is generated. Events leading to the repair of the DSB include homologous recombination between repeated sequences, either remote (distant crossover, D) or contiguous (adjacent crossover, E). Both types of events generate derivatives sensitive to kanamycin. Kanamycin resistant derivatives may arise by gene conversion (F), inactivation of the I-SceI gene (G), or non-homologous end joining (H). For all panels, blue arrows mark the relative position of F7 repeats, while small black arrows (either lettered or numbered) mark the position of PCR primers used for characterization (see Materials and Methods and Supplementary Table S1).
Introduction of a plasmid expressing the I-SceI nuclease (pJN105mega) into R. etli CFNX55D1 (harboring pSymΔ with one I-SceI site) occurred at a low frequency (Table 1, line 3). For characterization of the repair mode after inducing a DSB, twenty independent biparental matings between CFNX55D1 and E. coli containing pJN105mega plasmid were done, from which 100 randomly selected transconjugants were analyzed (Table 1, detailed information is given in Supplementary Table S4). Seventy-two transconjugants were sensitive to kanamycin, indicating that repair was achieved through homologous recombination, either with distant (Figure 3D) or adjacent (Figure 3E) repeats. Sixty-two of these presented all the hallmarks of repair by homologous recombination between distant F7 repeats (pSymΔ with a size of 217 kb, lack of amplification of F7a and F7b repeats, amplification of a F7a/b joinpoint, absence of the pOY-ISceI cointegrate and I-SceI site, and sensitivity to kanamycin, Figure 3D). Nine of the kanamycin-sensitive transconjugants were classified as being generated by a crossover between adjacent nifH repeats, based on a pSymΔ normal size (268 kb) and absence of both the pOY-ISceI cointegrate and I-SceI site (Figure 3E). One of the transconjugants sensitive to kanamycin had a smaller pSymΔ than the others and was not investigated further.
For the remaining 28 kanamycin-resistant transconjugants, all of them showed a normally sized pSymΔ. To determine if the I-SceI site was still present, amplifications were carried out with primers that anneal upstream and in the I-SceI site (primers 1 U/ ISceI chK Lw), respectively. Six transconjugants still carried the I-SceI site; amplification of the I-SceI gene in these revealed a larger amplification product, indicating that the I-SceI gene was inactivated by insertion (Figure 3G). To distinguish if the remaining 22 transconjugants were repaired by gene conversion (Figure 3F) or by NHEJ (Figure 3H), a PCR using primers ApaLIU/NarL was set to amplify an area around the expected I-SceI site. Two PCR products were expected because both truncated versions of the nifH gene can be amplified with such primers. If repair was achieved by gene conversion, both products should be of the same size (249 bp); in contrast, repair through NHEJ should reveal two different products, one of 260 bp (corresponding to the one containing an incomplete I-SceI site) and another of 249 bp (from the one lacking the I-SceI site). Results showed that six transconjugants were repaired by gene conversion (Figure 3F), while 16 corresponded to NHEJ events (Figure 3H).
To summarize, in this configuration, homologous recombination accounted for 77% of the repair events observed (62% by recombination between distant repeats, while 15% were by recombination between adjacent repeats, including simple crossover and gene conversion). NHEJ events were detected in 16% of the cases. Other, less frequent events were inactivation of the I-SceI nuclease gene (6%) and one unidentified event.
Extending the size of an adjacent homologous region in the vicinity of the DSB
Although recombination between distant homologous sequences has been the most prevalent form of DNA repair after induction of DSB, recombination between adjacent homologous sequences in the vicinity of the DSB accounted for 15% of all recombination events. Since the size of the adjacent homologous region available for recombination was small (260 bp), we wanted to test whether a larger homologous region could increase the amount of recombination in the vicinity of the DSB. To increase the size of the homologous region, we employed the integrative plasmid pJGus28-ISceI. This plasmid contains a full copy of the nifH gene, with single-base modifications every 100 bp, generating eight different restriction sites. It also has the I-SceI recognition site at the same position as plasmid pOY-ISceI. Cointegration of pJGus28-ISceI into pSymΔ generate a strain with adjacent repeats that are nearly one-kb long, but with the I-SceI recognition site located in only one of the repeats (strain CFNX55O1, Figures 2F–H). As seen before, introduction of a plasmid expressing the I-SceI nuclease (pJN105mega) into R. etli CFNX55O1 occurred at a low frequency (Table 1, line 4). For characterization of the repair mode after inducing a DSB, twenty independent biparental matings between CFNX55O1 and E. coli containing pJN105mega plasmid were done, from which 100 randomly selected transconjugants were analyzed under the same criteria as before (Table 1, detailed information is given in Supplementary Table S5). Sixty of the transconjugants repaired the DSB by distant crossover between the F7 repeats. Fourteen transconjugants displayed recombination with the extended nifH gene copies, of which 11 had an adjacent crossover event, while the remaining three had a gene conversion event. Three transconjugants did not experienced a DSB, due to inactivation of the I-SceI nuclease by an inserted sequence (see below). The remaining 23 transconjugants were repaired by NHEJ. To characterize the extent of NHEJ, sequence of the region surrounding the I-SceI site was obtained for each transconjugant. As shown in Figure 4A, digestion of the I-SceI site generates 4 nt cohesive ends. Sequence analysis of transconjugants showed deletions removing from 1 to 4 nts on the sequence corresponding to the cohesive ends after I-SceI digestion; in one case removal of three nts of the I-SceI site was accompanied by deletion of an extra 2 nt next to the cohesive ends (Figures 4B–F).
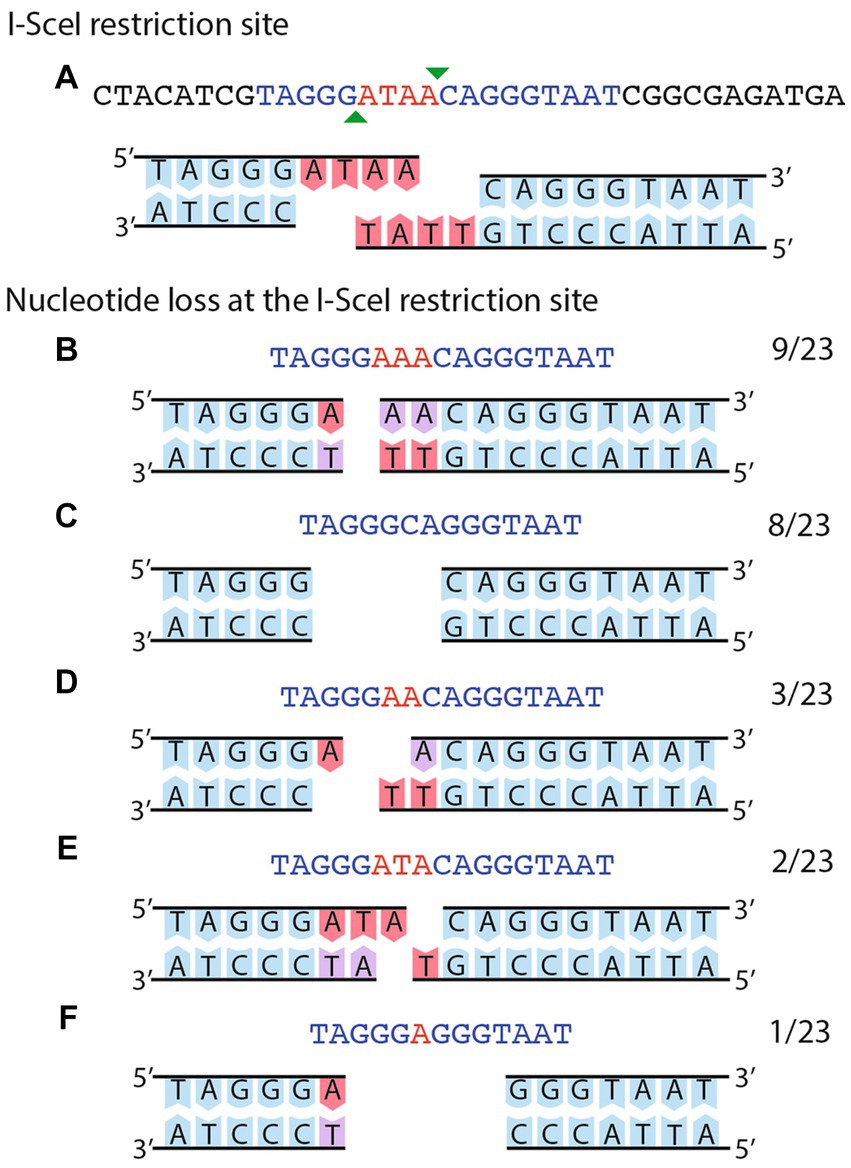
Figure 4. Sequence analysis of NHEJ events obtained in strain CFNX55O1. (A) The cut I-SceI site is shown as a double strand sequence. The extent of the I-SceI site is shown in blue, while the protruding ends are shown in red. The bottom part shows the sequence of the top strand. (B–F) shows different instances of deletions generated by NHEJ, with the substrate in (A) modified to show which nucleotides were deleted. Numbers at the right of each panel show the fraction of events belonging to each class.
Apparent lethality of a DSB on a recA mutant
Since NHEJ is independent of recA function, we reasoned that induction of a DSB on a recA mutant background would allow us to detect NHEJ events, without interference by homologous recombination. To explore that, the pSymΔ of strain CFNX55D2 was transferred by conjugation into R. etli CFNX101 (a recA mutant), thus creating strain CFNX101D2. The introduction of a plasmid expressing the I-SceI nuclease into a recA mutant background (strain CFNX101) occurred at a ten-fold lower frequency, compared to its introduction in a wild-type background (Table 1, compare rows 1 and 5). A similar effect was observed upon the transfer of pJN105 (a similar plasmid without the nuclease) into strain CFNX101D2. This reduction in conjugation frequency may be explained by the reduced viability of a recA mutant. In contrast, when pJN105mega was introduced into R. etli CFNX101D2 (recA mutant, harboring pSymΔ with two I-SceI sites), a ten-thousand-fold reduction in conjugation frequency was seen (Table 1, row 7), being the lowest conjugation frequency observed in this work.
To characterize the events that allowed survival after a DSB, ten independent biparental matings between strains CFNX101D2 and E. coli S17/pJN105mega were carried out, and 46 randomly selected transconjugants were analyzed as before (Table 1). Although we were anticipating the absence of products arising by homologous recombination, to our surprise, no products generated by NHEJ were detected. All the transconjugants showed a normal-sized pSymΔ, preserving the pOY-ISceI cointegrate and both I-SceI sites. Analyzing the status of the I-SceI gene by amplification with specific primers, revealed that most of the transconjugants harbor structural alterations in this gene. Thirty-eight of the transconjugants carried insertions (estimated size, 0.7 kb) in the I-SceI gene, while two others had larger insertions, ranging in size from1.4 to 2.1 kb. Two of the transconjugants had deletions in this gene (estimated from 0.1 to 0.15 kb), while four transconjugants carried a normal-sized I-SceI gene. Sequence analysis was done for one of the isolates harboring a deletion, one with an apparently normal I-SceI gene, the two transconjugants carrying the largest insertions and one of the transconjugants bearing a 0.7 kb insertion. A 150-bp deletion was detected, eliminating nt 129 to 279 from the I-SceI gene. For the transconjugant with an apparently normal I-SceI gene, multiple sequence changes were detected (deletion of A73; G > C 429; C > G 654; C > G 660). The largest insertions were caused by incorporation of IS21 (2.1 kb, at nt 249) or IS421 (1.3 kb, at nt 250) into the I-SceI gene; the medium-sized alteration was caused by insertion of IS1A (0.76 kb, at nt 537). Since these insertion sequences originate from the E. coli genome (and are absent from R. etli) these mutants should have arisen in E. coli. Alterations like these were observed previously during this work, but at low frequencies. What is surprising is the predominance of inactivation of the I-SceI gene when a DSB is attempted in a recA background. Possible reasons for this are explored in discussion.
NHEJ was readily observed after induction of a DSB in a small, stable plasmid
Given the inability to induce a DSB on pSymΔ in a recA mutant background, we sought alternative ways to explore this issue. For this, we employed plasmid pTR101, a small plasmid containing the origin of replication and the toxin-antitoxin parDE operon from plasmid RK2, which has been shown to confer stability in Sinorhizobium meliloti (Weinstein et al., 1992). This plasmid lacks extensive repeated sequences. Plasmid pTR101 was modified by introduction of a I-SceI site on it (see Materials and Methods). Both plasmids (pTR101 and pTR101-SceI) were introduced separately into a recA mutant background (strain CFNX101). Introduction of pJN105mega by conjugation into strain CFNX101/pTR101 occurred readily (at a frequency of 1 × 10−5). In contrast, a strong reduction in transfer frequency of pJN105mega was detected when strain CFNX101/pTR101-SceI was used as a recipient (at a frequency of 1 × 10−7).
Sequence analyses of the region surrounding the I-SceI site were obtained for eleven transconjugants of strain CFNX101/pTR101-SceI, harboring pJN105mega (Figure 5). All these showed changes consistent with the operation of NHEJ. Nine of the transconjugants harbored deletions ranging in size from one to six nucleotides, affecting the 5’half of the I-SceI site, while one has a 19 bp deletion that eliminates the I-SceI site. The most extensive deletion removes 136 bp, towards the 5’half of the I-SceI site and adjacent sequences. No microhomologies were detected at the end of these deletions.
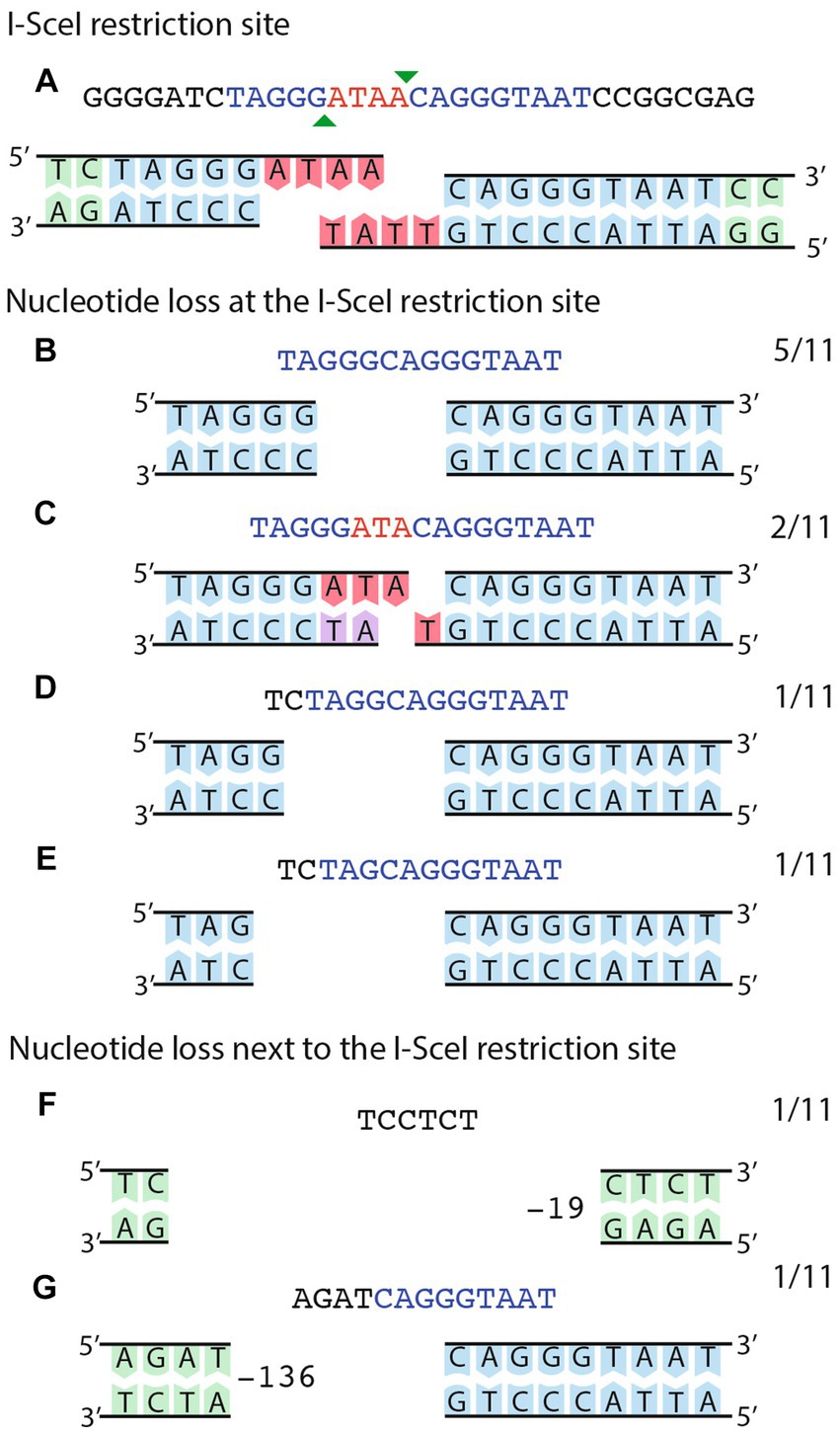
Figure 5. Sequence analysis of NHEJ events obtained on a small, stable plasmid. (A) The cut I-SceI site is shown as a double strand sequence. The extent of the I-SceI site is shown in blue, while the protruding ends are shown in red. The bottom part shows the sequence of the top strand. (B–G) deletion events generated by NHEJ, either on the I-SceI site (B–E) or affecting the I-SceI site and adjacent sequences (F–G). For F–G, the number of deleted nucleotides is indicated. Numbers at the right of each panel show the fraction of events belonging to each class.
Discussion
The main motivations for this work were to explore the relative contributions of homologous recombination and NHEJ for repairing a double-strand break in R. etli and, if NHEJ was detected, to characterize the different events. For that, we employed a system in which either single (strains CFNX55D1 and CFNX55O1) or double (strain CFNX55D2) recognition sites for the I-SceI endonuclease were introduced into pSym derivatives; generation of a DSB was achieved by introduction of a small, self-replicating plasmid harboring the I-SceI gene. Given the configuration and number of I-SceI sites, in strain CFNX55D2 a DSB should be repaired through homologous recombination between distant repeats or by NHEJ, but not by recombination between adjacent repeats. Repair of a DSB in strains CFNX55D1 and CFNX55O1 should be achieved not only by homologous recombination (either between distant or adjacent repeats), but through NHEJ as well (Figure 3). Since the R. etli symbiotic plasmid lacks essential genes, we were expecting that the induction of a DSB would not be deleterious for viability.
As expected, repair of DSBs on strain CFNX55D2 was achieved mainly by recombination between distant repeats (92%), with a small contribution of other events (8%), including Sce-I inactivation. We think that NHEJ was not detected in this case due to a small sample size. The relative contribution of homologous recombination and NHEJ is clearly seen in the results obtained with strains CFNX55D1 and CFNX55O1 (Table 1). In these strains, repair by homologous recombination may occur through distant or adjacent repeats, so we combined the abundance of these events. For strain CFNX55D1, 87% of repair events were achieved through homologous recombination, 16% by NHEJ, and 7% by other events, including I-Sce-I inactivation. For strain CFNX55O1 (which contained an adjacent repeat larger than the one in strain CFNX55D1, albeit interrupted by single-base changes), homologous recombination is also the main repair event (74%), compared to NHEJ (23%) or other events (3%). These results support the view that repair of DSBs in R. etli is achieved mainly by homologous recombination, being 4 to 5 times more frequent that NHEJ. When repaired by homologous recombination, in both strains recombination between distant repeats was roughly four times more frequent (CFNX55D1, 62%; CFNX55O1, 60%) than recombination between adjacent repeats (CFNX55D1, 15%; CFNX55O1, 14%). We think this difference is due to the size and identity of distant vs. adjacent repeats. Distant repeats are 871 bp long, while adjacent repeats are either 247 bp long (CFNX55D1) or 900 bp long (CFNX55O1). Although in the last case the size of adjacent repeats resembles the size of distant repeats, the adjacent repeats carry single base changes every 100 bp, while distant repeats are identical. We have shown previously that sequence differences limit the use of these repeats for homologous recombination, through the operation of the MutS system (Santoyo et al., 2005).
The prevalence of homologous recombination over NHEJ in the repair of DSB reported here is consistent with data in other systems. In Mycobacterium smegmatis, using a chromosomal system where DSBs were generated with I-SceI, DSBs were repaired by homologous recombination in 40% of the cases, by NHEJ (12%) or by a SSA pathway (48%). It was suggested that the SSA pathway may be particularly relevant in repetitive areas of the mycobacterial chromosome (Gupta et al., 2011). For Sinorhizobium meliloti, a larger role of homologous recombination over NHEJ in repair of DSBs has also been suggested, based on similar survival rates to ionizing radiation in the wild type and NHEJ mutant strains, while a mutant in recA showed a strong reduction in survival (Kobayashi et al., 2008; Dupuy et al., 2017). Interestingly, this difference was observed in growing bacteria, while in stationary phase both homologous recombination and NHEJ contribute to survival from ionizing radiation (Dupuy et al., 2017). A significant contribution of NHEJ was also observed in stationary phase cells of S. meliloti, both for repair of DNA ends provided by transformation of linear plasmids or upon delivery of a DSB on the chromosome by in vivo digestion with I-SceI (Dupuy et al., 2019).
Repair of I-SceI induced DSBs by NHEJ obtained in this work, either on pSymΔ (strain CFNX55O1) or on a small plasmid (strain CFNX101/pTR101-SceI) were characterized by DNA sequencing. In most of the cases (32 out of 34) deletions, ranging in size from 1–6 bp were observed, centered on the protruding single-strand ends generated by I-SceI digestion. Two cases of larger deletions (19 and 136 bases) were also detected. For repair of a chromosomal DSB in M. smegmatis by NHEJ, small deletions (2 to 5 bp) centered on the I-SceI protruding ends were also seen in 7 out of 15 cases; interestingly, the rest of the events carry more extensive deletions, as large as 1,399 bp (Gupta et al., 2011). A similar distribution of deletion sizes was observed for repair events obtained from a recA strain. In S. meliloti, most of the NHEJ repair events after inflicting a DSB in the chromosome (37 out of 40) were small deletions of 1–4 bp, centered on the protruding ends of the I-SceI site; one of these deletions also exhibited a one-bp insertion. Three cases of large deletions (from 214 to 343 bp) were reported as well (Dupuy et al., 2019). For all three organisms, the main NHEJ repair events are small deletions surrounding the region of the DSB, with varying proportions of larger deletions depending on the organism. The different proportions of large deletions may be due to variability in exonuclease activities between organisms upon delivering a DSB.
An interesting, yet unexpected aspect of our work was the inability to observe derivatives that have lost pSymΔ after delivering a DSB. We were expecting to see this kind of derivatives since sequence analysis reveal the absence of any essential genes on this plasmid (González et al., 2003). Elimination of the pSym of R. etli was observed before (Brom et al., 1992, 2000), albeit at a very low frequency (10−7). Notably, every time a DSB was inflicted on the pSymΔ a strong reduction in viability (more than a thousand-fold, see Table 1) was observed. A situation like this is commonly observed whenever a DSB is inflicted on a chromosome (Gupta et al., 2011; Dupuy et al., 2019; Uribe et al., 2021), but it is unusual when the DSB occurs on a nonessential plasmid. The inability to eliminate pSymΔ, or even to instigate a DSB on it in a recA background, can be explained by invoking that, once that homologous recombination (the predominant mode for repair of a DSB) is eliminated, many pSymΔ molecules are left unrepaired. This situation might lead to the elimination of pSymΔ, but this could be prevented by the operation of a putative toxin-antitoxin module on pSymΔ, killing the cells that have an unrepaired plasmid. Lower frequency alternatives, such as NHEJ, are unlikely to predominate under such circumstances. Under this idea, there should be a strong selection favoring the acquisition of mutations in the I-SceI gene. On the pSym of R. etli CFN42 there are at least four potential toxin-antitoxin modules. None of these have been characterized experimentally. Experiments are underway to evaluate the operation of these modules in preventing pSym loss. Interestingly, when a DSB was inflicted on the E. coli chromosome using a CRISPR-Cas9 system, a similar frequency of events inactivating Cas9 was observed, whenever the strain is unable to achieve repair by homologous recombination (Uribe et al., 2021).
In conclusion, we have shown that homologous recombination is the main mechanism in R. etli for the repair of a DSB. NHEJ is also operational, although it occurs at a four-fold lower frequency. Focusing our work on a nonessential plasmid also revealed an unexpected stability of this plasmid.
Materials and methods
Bacterial strains and media
All Rhizobium etli strains were grown at 30°C in PY medium (Noel et al., 1984) supplemented with 700 μM CaCl2. Escherichia coli strains were grown in LB medium at 37°C. Antibiotics were used at the following concentrations (in μg ml−1): nalidixic acid, 20; kanamycin, 30; gentamicin 30, spectinomycin 100 and tetracycline 5.
Plasmid and strain construction
Construction of pOY-ISceI
A 247 bp nifH gene fragment that includes an I-SceI restriction site was obtained by PCR amplification from pJGus28-ISceI plasmid (Yáñez-Cuna et al., 2016) using primer combination ApaLIU/NarL under the following amplification protocol: 30 cycles of denaturation (94°C, 30 s), annealing (58°C, 30s), and extension (72°C 30s) using Taq DNA polymerase (Thermo Scientific). This PCR product was digested with HindIII and XbaI restriction enzymes and subsequently ligated into a similarly digested pK18mobsacB (Ref) using T4 DNA ligase, giving rise to pOY-ISceI. The ligation mixture was transformed into E. coli DH5α [λ− Φ80dlacZΔM15 Δ(lacZYA-argF) U169 recA1 endA1 hsdR17(rK− mK−) supE44 thi-1 gyrA relA1] (Bethesda Research Laboratories, 1986) and transformants were selected in LB media containing kanamycin. Clones were verified by PCR using primers ApaLIU/NarL.
Construction of a pTR101 plasmid with I-SceI site
A self-replicating, stable plasmid in Rhizobium, containing the I-SceI site, was constructed using the pTR plasmids (Weinstein et al., 1992). Plasmid pTR101 contains a RK2 stability region, allowing stable maintenance in Rhizobium, even without positive selection. To introduce the I-SceI site into this plasmid, 10 μM of primers SceI site Up and SceI site Lw were annealed on annealing buffer (100 mM Tris pH 7.5, 500 mM NaCl, and 10 mM EDTA) by heating the sample at 95°C for 5 min and then cooled slowly at room temperature. The annealed primers were phosphorylated with T4 Polynucleotide Kinase. Plasmid pTR101 was digested with BamHI and treated with Alkaline Phosphatase. Phosphorylated annealed primers were then cloned into pTR101 using T4 DNA ligase, and ligation mixtures were transformed into E. coli DH5α, giving rise to pTR101-ISceI. To test that the resulting plasmid had an I-SceI site, a PCR reaction was set up using primer combination SceI site Lw and bla using a protocol of denaturation (94°C, 30s), annealing (55°C, 30s), extension (72°C, 1 m) using Taq DNA polymerase. Plasmids pTR101 and pTR101-ISceI were each transformed into an E. coli S-17 and then conjugated into R. etli CFNX101 on solid PY medium plates (see below). Transconjugants were selected on nalidixic acid and tetracycline.
Construction of CFNX55D1 and CFNX55D2 strains
pOY-ISceI was transformed into an E. coli S17 (F− pro-82 thi-1 endA1 hsdR17 supE44 recA13; chromosomally integrated RP-4-2 [Tc::Mu Km::Tn7]) (Simon et al., 1983) and then transferred by conjugation to R. etli CFNX55 (Romero et al., 1991). Transconjugants were selected on solid PY medium with nalidixic acid and kanamycin. Since pOY-ISceI does not replicate on R. etli, this selects for cointegration with R. etli pSymΔ plasmid, interrupting the nifH gene. To verify this, a PCR reaction was done using Taq polymerase and primer combination 1 U/4 L, which flanks D plasmid nifH gene, under a regimen of 30 cycles of denaturation (94°C, 30s), annealing (48°C, 30s) and extension (72°C, 1m15s). A single crossover event between plasmids generates a cointegrate with only one I-SceI site (CFNX55D1); whereas a gene conversion event, a cointegrate with two I-SceI sites (CFNX55D2). To determine the presence of I-SceI sites at each side of the cointegrate, two PCRs reactions were done with primer combinations 1 U/ISceI chK Lw for one side of the cointegrate and ISceI chK Up/4 L for the other side, using a 30-cycle amplification protocol with Taq polymerase, comprising denaturation (94°C 30s), annealing (44°C, 30s), extension (72°C, 30s). CFNX55D1 and CFNX55D2 strains were also screened for their plasmid profile by the in-gel lysis method of Eckhardt (1978) as modified by Hynes and McGregor (1990).
To generate a strain with one I-SceI site but with a larger homology fragment for recombination, a biparental mating between R. etli CFNX55 and E. coli S-17 harboring the pJGus28-ISceI plasmid was set up. pJGus28-ISceI plasmid contains a modified version of R. etli nifH gene that carries eight single-base pair changes (generating novel restriction sites), and an I-SceI recognition site in the middle of the gene. Transconjugants were selected on solid PY medium with nalidixic acid and kanamycin. Since pJGus28-ISceI does not replicate on R. etli, the selection is applied for the cointegration of this plasmid into R. etli pSymΔ plasmid. Single crossover recombination between this plasmid and the nifH copy on CFNX55 pSymΔ plasmid generates a cointegrate with two full copies of the nifH gene. To determine that transconjugants had two copies of the nifH gene, a PCR reaction was set up using 1 U/2 L and 3 U/4 L primer combinations with the following PCR amplification protocol: denaturation (94°C, 45 s), annealing (47°C, 45 s), extension (72°C 1 m 15 s) and Taq polymerase. Primers 3 U and 2 L flank nifH gene on the pJGus28 plasmid. Transconjugants were also screened for the presence of only one I-SceI site, as described previously, and its plasmid profile, giving rise to CFNX55O1.
Construction of CFNX101D2 strain
CFNX101 (Martínez-Salazar et al., 1991) is an R. etli CFN42 recA strain, to get proof that the recA gene is modified, a PCR reaction was set up using primer combination RecAUp/RecALw using the following amplification protocol: 30 cycles of denaturation (94°C, 30s), annealing (55°C, 30s), amplification (72°C, 2m30s) and Taq polymerase. To check for UV sensitivity, CFNX101 was irradiated with UV light at 40 J/m2. To transfer our modified pSymΔ plasmid (D2) into a recA mutant strain, a biparental mating was done between CFNX55D2 and CFNX101. Selection for transconjugants was done on solid PY medium with spectinomycin and kanamycin. Transconjugants were also screened for the presence of two I-SceI sites using a PCR reaction as described previously. CFNX101D2 strain was also screened for its plasmid profile as described before.
Molecular characterization of transconjugants
To generate double-stranded breaks at a specific site in the genome, the broad-host-range expression plasmid pJN105meganuclease (Yáñez-Cuna et al., 2016) carrying the gene for the I-SceI nuclease was used. Biparental matings were set up in solid PY medium between E. coli S-17 carrying the pJN105meganuclease plasmid and an R. etli strain (CFNX55D1, CFNX55D2, CFNX55O1, CFNX101D2). Since pJN105meganuclease plasmid replicates on R. etli, the selection causes the transfer of the plasmid into R. etli and thus, the expression of the I-SceI nuclease. To reduce the occurrence of siblings in the analysis, 10 independent matings were done for the CFNX55D2 and CFNX101D2 strains and 20, for the CFNX55D1, CFNX55O1, out of which 5 transconjugants were selected randomly for further analyses per mating. Transconjugants were screened for their plasmid profile, as described previously. To determine whether the pOY-ISceI cointegrate had been lost (see Results), transconjugants were grown on solid PY medium with nalidixic acid and kanamycin. DNA from each transconjugant was isolated and subjected to the following analysis: To determine if the repair of DNA involved a crossover event, therefore the pOY-ISceI cointegrate had been lost, we checked for the presence of the nifH gene on D plasmid using oligo combinations 1 U/4 L as described before. To determine if D plasmid had a type IV deletion (repair by F7 repeats), we used the following set of three PCR reactions: (1) To screen for the presence of F7 repeat at position 356 Kb we used 356 Fwd/F7 Rev. primer combination; (2) for the presence of F7 repeat at position 202 Kb, we used F7 Fwd/202 Rev. primer combination using the following amplification protocol: denaturation (94°C, 30s), annealing (64°C, 30s), extension (72°C, 1 m) and Taq polymerase; (3) to screen for a recombination event between F7 repeats, a PCR reaction was set up using F7 Fwd/F7 Rev. primer combination using an amplification protocol of denaturation (94°C, 15 s), annealing (68°C, 15 s) and extension (72°C, 1 m) and Taq polymerase. To ensure that a functional I-SceI nuclease had digested DNA at its recognition site, a PCR reaction using primer combination 1 U/ ISceI chK Lw was used, as described before. Since primer ISceI chK Lw anneals into the I-SceI site, point mutations or small deletions generated after DNA repair on the I-SceI site could prevent primer binding and thus, successful PCR. To differentiate between loss of the I-SceI site or changes that prevented the ISceI chK Lw primer to anneal, a PCR was made using ApaLIU/NarL primer combination and Taq polymerase as described before, for all transconjugants that had a negative PCR amplification with 1 U/ ISceI chK Lw primers. Moreover, CFNX55O1 pJN105meganuclease transconjugants whose I-SceI site was inferred to be present although with some changes that prevented the PCR from amplifying successfully were sent for sequencing. Primer combination Up 525/Lw 525 was used to screen for the nuclease using the following protocol: denaturation (94°C, 15 s), annealing (60°C, 15 s), extension (72°C, 45 s), and Taq polymerase.
Biparental matings were also set up in solid PY medium between E. coli S-17 carrying the pJN105meganuclease plasmid and R. etli CFNX101/pTR101 and CFNX101/pTR101-ISceI. Transconjugants were selected on nalidixic acid and gentamycin. To determine if the corresponding pTR plasmid was still present in the transconjugants, these were checked for resistance to tetracycline.
Estimation of conjugation frequencies
Conjugation frequencies were estimated for all conjugations between an E. coli S17-I with plasmids pJN105 or pJN105mega and any R. etli strain. Conjugation frequency was calculated as transconjugants between viable recipient cells. After biparental matings were grown for 12 h on solid PY media without antibiotics, cells were resuspended in MgSO4 10 mM and tween 0.01% solution and serially diluted 10-fold to 10–8. Dilutions were plated on nalidixic acid for viable recipient cells, and nalidixic acid and gentamicin, for transconjugants. Colonies grown on plates were counted to estimate viable recipient cells and transconjugants. Several conjugation experiments were done for each strain, we report the mean frequency with its standard deviation.
Bioinformatic tools
REPuter software (Kurtz et al., 2001) was used to search for repeated sequences on R. etli p42d. Parameters were set up for finding repeated sequences larger than 100 bp and 99% of identity.
Data availability statement
The original contributions presented in the study are included in the article/Supplementary material, further inquiries can be directed to the corresponding author.
Author contributions
FY-C: Formal analysis, Investigation, Writing – original draft. DA-G: Formal analysis, Investigation, Writing – review & editing. AD: Formal analysis, Investigation, Writing – review & editing. DR: Conceptualization, Formal analysis, Funding acquisition, Supervision, Writing – original draft.
Funding
The author(s) declare financial support was received for the research, authorship, and/or publication of this article. This work was conducted with financial support from the Centro de Ciencias Genómicas, Universidad Nacional Autónoma de México.
Acknowledgments
We gratefully acknowledge Susana Brom and Lourdes Girard for helpful comments during this work, Laura Cervantes for general technical support, Paul Gaytán and Eugenio López (Unidad de Síntesis de Oligonucleótidos, IBt-UNAM) for oligonucleotide synthesis and Jorge Yáñez (Unidad de Secuenciación, IBt-UNAM) for Sanger sequencing.
Conflict of interest
The authors declare that the research was conducted in the absence of any commercial or financial relationships that could be construed as a potential conflict of interest.
The author(s) declared that they were an editorial board member of Frontiers, at the time of submission. This had no impact on the peer review process and the final decision.
Publisher’s note
All claims expressed in this article are solely those of the authors and do not necessarily represent those of their affiliated organizations, or those of the publisher, the editors and the reviewers. Any product that may be evaluated in this article, or claim that may be made by its manufacturer, is not guaranteed or endorsed by the publisher.
Supplementary material
The Supplementary material for this article can be found online at: https://www.frontiersin.org/articles/10.3389/fmicb.2024.1333194/full#supplementary-material
References
Ayora, S., Carrasco, B., Cárdenas, P. P., César, C. E., Cañas, C., Yadav, T., et al. (2011). Double-strand break repair in bacteria: a view from Bacillus subtilis. FEMS Microbiol. Rev. 35, 1055–1081. doi: 10.1111/j.1574-6976.2011.00272.x
Baharoglu, Z., and Mazel, D. (2014). SOS, the formidable strategy of bacteria against aggressions. FEMS Microbiol. Rev. 38, 1126–1145. doi: 10.1111/1574-6976.12077
Bethesda Research Laboratories (1986). BRL pUC host: E. coli DH5 competent cells. Bethesda Res. Lab. Focus 8, 9–12.
Brom, S., García de los Santos, A., Stepkowsky, T., Flores, M., Dávila, G., Romero, D., et al. (1992). Different plasmids of Rhizobium leguminosarum bv. Phaseoli are required for optimal symbiotic performance. J. Bacteriol. 174, 5183–5189. doi: 10.1128/jb.174.16.5183-5189.1992
Brom, S., García-de los Santos, A., Cervantes, L., Palacios, R., and Romero, D. (2000). In Rhizobium etli symbiotic plasmid transfer, nodulation Competitivity and cellular growth require interaction among different replicons. Plasmid 44, 34–43. doi: 10.1006/plas.2000.1469
Bzymek, M., and Lovett, S. T. (2001). Evidence for two mechanisms of palindrome-stimulated deletion in Escherichia coli: single-Strand annealing and replication slipped mispairing. Genetics 158, 527–540. doi: 10.1093/genetics/158.2.527
Castellanos, M., and Romero, D. (2009). The extent of migration of the Holliday junction is a crucial factor for gene conversion in Rhizobium etli. J. Bacteriol. 191, 4987–4995. doi: 10.1128/jb.00111-09
De Vega, M. (2013). The minimal Bacillus subtilis nonhomologous end joining repair machinery. PLoS One 8:e64232. doi: 10.1371/journal.pone.0064232
Della, M., Pl, P., Tseng, H. M., Tonkin, L. M., Daley, J. M., Topper, L. M., et al. (2004). Mycobacterial Ku and ligase proteins constitute a two-component NHEJ repair machine. Science 306, 683–685. doi: 10.1126/science.109982
Dupuy, P., Gourion, B., Sauviac, L., and Bruand, C. (2017). DNA double-strand break repair is involved in desiccation resistance of Sinorhizobium meliloti, but is not essential for its symbiotic interaction with Medicago truncatula. Microbiology 163, 333–342. doi: 10.1099/mic.0.000400
Dupuy, P., Sauviac, L., and Bruand, C. (2019). Stress-inducible NHEJ in bacteria: function in DNA repair and acquisition of heterologous DNA. Nucleic Acids Res. 47, 1335–1349. doi: 10.1093/nar/gky1212
Eckhardt, T. (1978). A rapid method for the identification of plasmid desoxyribonucleic acid in bacteria. Plasmid 1, 584–588. doi: 10.1016/0147-619X(78)90016-1
Glickman, M. S. (2014). Double-Strand DNA break repair in mycobacteria. Microbiol. Spectr. 2:MGM2-0024-2013. doi: 10.1128/microbiolspec.mgm2-0024-2013
González, V., Bustos, P., Ramírez-Romero, M. A., Medrano-Soto, A., Salgado, H., Hernández-González, I., et al. (2003). The mosaic structure of the symbiotic plasmid of Rhizobium etli CFN42 and its relation to other symbiotic genome compartments. Genome Biol. 4, R36–R13. doi: 10.1186/gb-2003-4-6-r36
González, V., Santamaría, R. I., Bustos, P., Hernández-González, I., Medrano-Soto, A., Moreno-Hagelsieb, G., et al. (2006). The partitioned Rhizobium etli genome: genetic and metabolic redundancy in seven interacting replicons. Proc. Natl. Acad. Sci. USA 103, 3834–3839. doi: 10.1073/pnas.0508502103
Gupta, R., Barkan, D., Redelman-Sidi, G., Shuman, S., and Glickman, M. S. (2011). Mycobacteria exploit three genetically distinct DNA double-strand break repair pathways. Mol. Microbiol. 79, 316–330. doi: 10.1111/j.1365-2958.2010.07463.x
Hoff, G., Bertrand, C., Zhang, L., Piotrowski, E., Chipot, L., Bontemps, C., et al. (2016). Multiple and variable NHEJ-like genes are involved in resistance to DNA damage in Streptomyces ambofaciens. Front. Microbiol. 7:1901. doi: 10.3389/fmicb.2016.01901
Hynes, M. F., and McGregor, N. F. (1990). Two plasmids other than the nodulation plasmid are necessary for formation of nitrogen-fixing nodules by Rhizobium leguminosarum. Mol. Microbiol. 4, 567–574. doi: 10.1111/j.1365-2958.1990.tb00625.x
Ithurbide, S., Bentchikou, E., Coste, G., Bost, B., Servant, P., and Sommer, S. (2015). Single Strand annealing plays a major role in RecA-independent recombination between repeated sequences in the Radioresistant Deinococcus radiodurans bacterium. PLoS Genet. 11:e1005636. doi: 10.1371/journal.pgen.1005636
Kobayashi, H., Simmons, L. A., Yuan, D. S., Broughton, W. J., and Walker, G. C. (2008). Multiple Ku orthologues mediate DNA non-homologous end-joining in the free-living form and during chronic infection of Sinorhizobium meliloti. Mol. Microbiol. 67, 350–363. doi: 10.1111/j.1365-2958.2007.06036.x
Kowalczykowski, S. C. (2015). An overview of the molecular mechanisms of Recombinational DNA repair. Cold Spring Harb. Perspect. Biol. 7:a016410. doi: 10.1101/cshperspect.a016410
Kurtz, S., Choudhuri, J. V., Ohlebusch, E., Schleiermacher, C., Stoye, J., and Giegerich, R. (2001). REPuter: the manifold applications of repeat analysis on a genomic scale. Nucleic Acids Res. 29, 4633–4642. doi: 10.1093/nar/29.22.4633
Manoj, F., and Kuhlman, T. E. (2023). Phylogenetic distribution of prokaryotic non-homologous end joining DNA repair Systems in Bacteria and Archaea. bioRxiv 2023:560322. doi: 10.1101/2023.09.30.560322
Martínez-Salazar, J. M., and Romero, D. (2000). Role of the ruvB gene in homologous and homeologous recombination in Rhizobium etli. Gene 243, 125–131. doi: 10.1016/s0378-1119(99)00548-x
Martínez-Salazar, J. M., Romero, D., Girard, M. L., and Dávila, G. (1991). Molecular cloning and characterization of the recA gene of Rhizobium phaseoli and construction of recA mutants. J. Bacteriol. 173, 3035–3040. doi: 10.1128/jb.173.10.3035-3040.1991
Martínez-Salazar, J. M., Zuñiga-Castillo, J., and Romero, D. (2009). Differential roles of proteins involved in migration of Holliday junctions on recombination and tolerance to DNA damaging agents in Rhizobium etli. Gene 432, 26–32. doi: 10.1016/j.gene.2008.11.009
Matthews, L. A., and Simmons, L. A. (2014). Bacterial nonhomologous end joining requires teamwork. J. Bacteriol. 196, 3363–3365. doi: 10.1128/jb.02042-14
Michel, B., and Leach, D. (2012). Homologous recombination enzymes and pathways. EcoSal Plus 5:10.1128/ecosalplus.7.2.7. doi: 10.1128/ecosalplus.7.2.7
Noel, K. D., Sanchez, A., Fernandez, L., Leemans, J., and Cevallos, M. A. (1984). Rhizobium phaseoli symbiotic mutants with transposon Tn5 insertions. J. Bacteriol. 158, 148–155. doi: 10.1128/jb.158.1.148-155.1984
Paris, Ü., Mikkel, K., Tavita, K., Saumaa, S., Teras, R., and Kivisaar, M. (2015). NHEJ enzymes LigD and Ku participate in stationary-phase mutagenesis in Pseudomonas putida. DNA Repair (Amst) 31, 11–18. doi: 10.1016/j.dnarep.2015.04.005
Romero, D., Brom, S., Martínez-Salazar, J., Girard, M. L., Palacios, R., and Dávila, G. (1991). Amplification and deletion of a nod-nif region in the symbiotic plasmid of Rhizobium phaseoli. J. Bacteriol. 173, 2435–2441. doi: 10.1128/jb.173.8.2435-2441.1991
Romero, D., Martínez-Salazar, J., Girard, L., Brom, S., Dávilla, G., Palacios, R., et al. (1995). Discrete amplifiable regions (amplicons) in the symbiotic plasmid of Rhizobium etli CFN42. J. Bacteriol. 177, 973–980. doi: 10.1128/jb.177.4.973-980.1995
Santoyo, G., Martínez-Salazar, J. M., Rodríguez, C., and Romero, D. (2005). Gene conversion tracts associated with crossovers in Rhizobium etli. J. Bacteriol. 187, 4116–4126. doi: 10.1128/jb.187.12.4116-4126.2005
Sharda, M., Badrinarayanan, A., and Seshasayee, A. S. N. (2020). Evolutionary and comparative analysis of bacterial non-homologous end joining repair. Genome Biol. Evol. 12, 2450–2466. doi: 10.1093/gbe/evaa223
Simon, R., Priefer, U., and Pühler, A. (1983). A broad host range mobilization system for in vivo genetic engineering: transposon mutagenesis in gram-negative bacteria. Nat. Biotechnol. 1, 784–791. doi: 10.1038/nbt1183-784
Tomso, D. J., and Kreuzer, K. N. (2000). Double-Strand break repair in tandem repeats during bacteriophage T4 infection. Genetics 155, 1493–1504. doi: 10.1093/genetics/155.4.1493
Uribe, R. V., Rathmer, C., Jahn, L. J., Ellabaan, M. M. H., Li, S. S., and Sommer, M. O. A. (2021). Bacterial resistance to CRISPR-Cas antimicrobials. Sci. Rep. 11:17267. doi: 10.1038/s41598-021-96735-4
Verma, P., and Greenberg, R. A. (2016). Noncanonical views of homology-directed DNA repair. Genes Dev. 30, 1138–1154. doi: 10.1101/gad.280545.116
Weinstein, M., Roberts, R. C., and Helinski, D. R. (1992). A region of the broad-host-range plasmid RK2 causes stable in planta inheritance of plasmids in Rhizobium meliloti cells isolated from alfalfa root nodules. J. Bacteriol. 174, 7486–7489. doi: 10.1128/jb.174.22.7486-7489.1992
Yáñez-Cuna, F. O., Castellanos, M., and Romero, D. (2016). Biased gene conversion in Rhizobium etli is caused by preferential double-Strand breaks on one of the recombining homologs. J. Bacteriol. 198, 591–599. doi: 10.1128/jb.00768-15
Zuñiga-Castillo, J., Romero, D., and Martínez-Salazar, J. M. (2004). The recombination genes addAB are not restricted to gram-positive Bacteria: genetic analysis of the recombination initiation enzymes RecF and AddAB in Rhizobium etli. J. Bacteriol. 186, 7905–7913. doi: 10.1128/jb.186.23.7905-7913.2004
Keywords: double-strand break, DNA repair, non-homologous end joining, bacterial recombination, gene conversion
Citation: Yáñez-Cuna FO, Aguilar-Gómez D, Dávalos A and Romero D (2024) Prevalent role of homologous recombination in the repair of specific double-strand breaks in Rhizobium etli. Front. Microbiol. 15:1333194. doi: 10.3389/fmicb.2024.1333194
Edited by:
Alicja Wegrzyn, University of Gdansk, PolandReviewed by:
Zheng Lu, Hainan University, ChinaGustavo Santoyo, Universidad Michoacana de San Nicolás de Hidalgo, Mexico
Copyright © 2024 Yáñez-Cuna, Aguilar-Gómez, Dávalos and Romero. This is an open-access article distributed under the terms of the Creative Commons Attribution License (CC BY). The use, distribution or reproduction in other forums is permitted, provided the original author(s) and the copyright owner(s) are credited and that the original publication in this journal is cited, in accordance with accepted academic practice. No use, distribution or reproduction is permitted which does not comply with these terms.
*Correspondence: David Romero, ZHJvbWVyb0BjY2cudW5hbS5teA==
†Present address: Diana Aguilar-Gómez, Department of Ecology and Evolutionary Biology, University of California, Los Angeles, CA, United States