- 1Department of Microbiology, Faculty of Advanced Science and Technology, Tehran Medical Science, Islamic Azad University, Tehran, Iran
- 2Department of Tissue Engineering and Applied Cell Sciences, School of Medicine, Qom University of Medical Sciences, Qom, Iran
- 3Cellular and Molecular Research Center, Qom University of Medical Sciences, Qom, Iran
- 4Department of Medical Microbiology, Faculty of Medicine, Bushehr University of Medical Sciences, Bushehr, Iran
- 5Fellowship in Clinical Laboratory Sciences, Mashhad University of Medical Sciences, Mashhad, Iran
- 6Nervous System Stem Cells Research Center, Semnan University of Medical Sciences, Semnan, Iran
- 7Department of Medical Microbiology, Faculty of Medicine, Shahed University, Tehran, Iran
- 8Tehran University of Medical Sciences, Tehran, Iran
The most prevalent and harmful injuries are burns, which are still a major global health problem. Burn injuries can cause issues because they boost the inflammatory and metabolic response, which can cause organ malfunction and systemic failure. On the other hand, a burn wound infection creates an environment that is conducive to the growth of bacteria and might put the patient at risk for sepsis. In addition, scarring is unavoidable, and this results in patients having functional and cosmetic issues. Wound healing is an amazing phenomenon with a complex mechanism that deals with different types of cells and biomolecules. Cell therapy using stem cells is one of the most challenging treatment methods that accelerates the healing of burn wounds. Since 2000, the use of mesenchymal stem cells (MSCs) in regenerative medicine and wound healing has increased. They can be extracted from various tissues, such as bone marrow, fat, the umbilical cord, and the amniotic membrane. According to studies, stem cell therapy for burn wounds increases angiogenesis, has anti-inflammatory properties, slows the progression of fibrosis, and has an excellent ability to differentiate and regenerate damaged tissue. Figuring out the main preclinical and clinical problems that stop people from using MSCs and then suggesting the right ways to improve therapy could help show the benefits of MSCs and move stem cell-based therapy forward. This review’s objective was to assess mesenchymal stem cell therapy’s contribution to the promotion of burn wound healing.
1 Introduction
One of the worst and most excruciating injuries anybody may experience is a burn injury (Kumari and Nanda, 2022). Burns are frequently understood to be skin sores caused by exposure to high temperature or heat, electricity, chemicals, or radiation (Jahromi et al., 2018). Thermal injuries account for 5–20% of all injuries and 4% of all fatalities (Schulman et al., 2022). Over 265,000 people worldwide die from burns each year (Wang et al., 2023). Burn injuries and the likelihood of dying from those injuries are both affected by age, occupation, and socio-economic status. Older buildings, lax safety standards, a lack of smoke alarms, and faulty electricity all lead to a higher risk of burn death and injury in low-development countries (Opriessnig et al., 2023).
The most common cause of mortality following a burn injury is wound infection (Wang et al., 2018). The body’s primary line of protection against dangerous foreign microbes is the skin. Burning destroys the skin’s integrity, allowing bacteria to enter and cause illness (Sun et al., 2022). In addition, burn infections hinder wound healing (Xiong Y. et al., 2023). Patients with severe burns are more prone to infection because their cutaneous barrier has been compromised and their systemic immune responses have been changed. The most prevalent cause of death in individuals with severe burns is septicemia, which occurs when bacteria infiltrate the deeper layers of damaged tissue and travel into the bloodstream (Sarker et al., 2022). Common pathogenic bacteria found in infected burn patients include Staphylococcus aureus, Pseudomonas aeruginosa, Acinetobacter baumannii, Klebsiella pneumoniae, and other coliform bacteria. Antimicrobial resistance is a major obstacle to treating many bacterial infections. Many studies have identified the most prevalent multidrug-resistant (MDR) bacteria in burn units (El Hamzaoui et al., 2020).
Despite general advancements in the treatment of individuals with acute burn injuries, morbidities related to more severe burn injuries continue to be widespread. Too frequently, burn victims experience severe tissue loss, scarring, and contractions that impair physical function and have long-term psychological and emotional effects (Schulman et al., 2022). In addition, large areas of deep burn wounds will disrupt the internal milieu and induce both local and systemic organ dysfunction if they are not treated quickly and effectively (Zhang et al., 2023). Over the past 10 years, significant progress has been made in the treatment and study of burn injuries. Examples of these developments include the creation of novel skin substitutes, the use of novel antimicrobial wound dressings and improved systemic drug delivery for the treatment of wound infection, the testing of novel pharmacological interventions, the identification of new targets for the control of wound pain, and sophisticated surgical techniques such as laser therapy, fat grafting, skin grafting, and coverage options such as design of a hydrogel system (Wang et al., 2018; Li et al., 2024).
Since stem cells have a higher capacity for regeneration and support the healing and regeneration processes in multiple ways, they offer numerous advantages over typical treatments based on growth factors or cytokine biologicals. Specifically, mesenchymal stem cells (MSCs) have demonstrated conclusive therapeutic benefits on a variety of tissue damage (Hu et al., 2022; Afkhami et al., 2023). MSCs are able to differentiate into many cell types and also have a robust ability for cell proliferation. They have the ability to differentiate into different types of tissues, such as bone, cartilage, adipose tissue, tendons, and muscles. The use of MSCs to speed the healing process after skin injuries, such as burns, has increased dramatically in recent years (Wang et al., 2021; Andalib et al., 2023).
One of the earliest studies on the use of MSCs to treat burn wounds in rats was reported in 2003 by Shumakov et al.; they utilized embryonic lung fibroblasts and bone marrow-derived MSCs in this research. According to their findings, MSCs have the ability to speed up the healing process of wounds and even rebuild damaged skin tissue (Gardien et al., 2014). Fu et al. (2004) treated minipigs with deep partial-thickness burns with MSCs and basic fibroblast growth factor (bFGF) (Rodgers and Jadhav, 2018). In 2005, MSCs were initially used to heal burns in people in Russia. Promising results were observed in five female patients treated with allogeneic MSCs (Mahmoudian-Sani et al., 2018). It is important for researchers to carefully consider the advantages and disadvantages of each MSC source. Patients with severe burns, for instance, may not be able to extract bone marrow. In contrast, a less invasive method can obtain MSCs in large quantities from adipose tissue (Ozturk and Karagoz, 2015).
The aim of this review is to investigate the treatment of burn wounds using stem cells derived from bone marrow, fat, umbilical cord, amniotic membrane, amniotic fluid, placental tissue, hair follicles, and dental pulp.
2 Skin structure and classification of burns
Regarding the application of MSCs in burn wounds, it is essential to comprehend normal skin structure and the pathological mechanisms of the skin following a burn (Xiong W. et al., 2023). The skin is the largest organ in the human body. In adults, it makes up approximately 15% of body weight and has an area of 1.5 to 2 m2. Skin is a vital organ that performs a variety of biological tasks, including excretion, heat management, vitamin D synthesis initiation, protection from toxins and infections, and hydration. Therefore, severe skin injuries may be dangerous (Tottoli et al., 2020; Huynh et al., 2022).
The skin is divided from top to bottom into three layers: the epidermis, dermis, and hypodermis (Jahromi et al., 2018; Andalib et al., 2023). There are five layers that make up the epidermis, arranged from outermost to lowest: the stratum corneum (SC), stratum lucidum, stratum granulosum (granular layer), stratum spinosum (spinous layer), and stratum basale (basal layer). From the basal layer, keratinocytes eventually separate and move outward. The viable epidermis is made up of the final four layers. Keratinocytes mature into corneocytes when they reach complete maturity. The dermis layer, which is made up of connective tissue and contains the skin’s hair follicles, sebaceous glands, and sweat glands, is located underneath the epidermis layer (Rahma and Lane, 2022).
The epidermis controls body temperature, the dermis preserves structural integrity and aids in feeling, and the hypodermis serves as mechanical protection and regulates body temperature (Irfan et al., 2022). Skin injuries are classified depending on the depth of their penetration into the skin, the amount of tissue destruction, and the percentage of total body surface area (TBSA) of the damaged wound (Xiong W. et al., 2023; Irfan et al., 2022) (Figure 1).
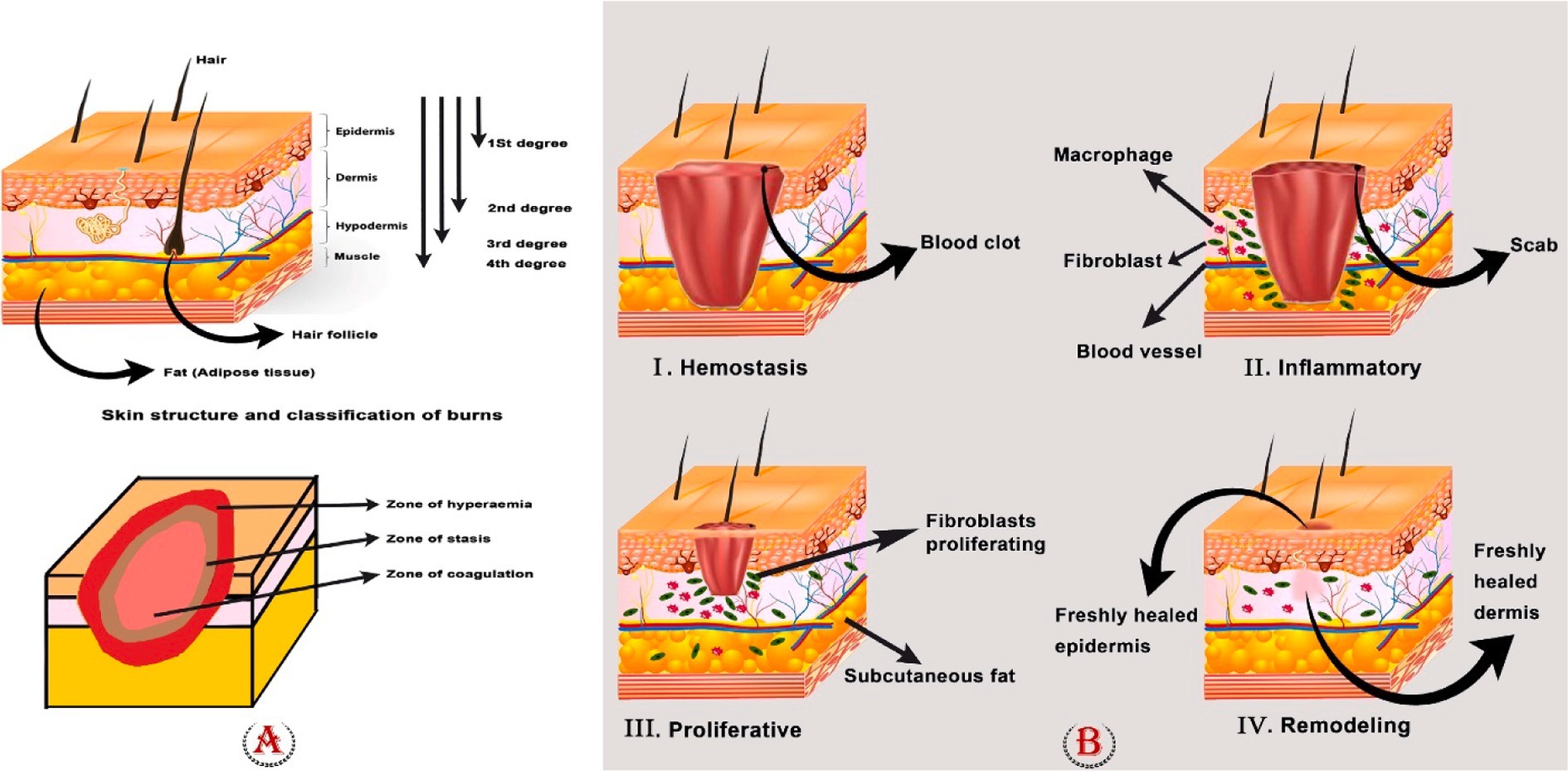
Figure 1. (A) Skin structure, classification of burns, and zones of the burn area. To determine the appropriate care for a patient, it is crucial to identify the depth of the burn. First-degree burns (those that involve only the outer layer of skin) are completely harmless, although they can be excruciatingly painful. They heal without leaving scars and typically do not require surgery. If a burn reaches the dermis, it is considered a second-degree or partial-thickness burn, and it will likely cause painful blisters. When third-degree (full thickness) or fourth-degree burns show, surgical intervention is required. (B) Four phases of wound healing: hemostasis, inflammation, proliferation, and remodeling phase.
First-degree burns, deep or superficial second-degree burns, third-degree burns, and fourth-degree burns are the different categories of burn injuries. Burns of the first degree only affect the epidermis, which is the skin’s outermost and superficial layer. In this instance, the epidermis turns red, and the pain lasts only a short while. Surface burns of the second degree cause destruction to the whole epidermis and a portion of the nipple layer. The skin turns red, swells, and hurts; it needs wound care to heal and typically recovers more quickly than deep second-degree wounds. The papillary dermis is injured in second-degree severe burns, although the dermis and skin appendages are still there. Edema is visible, and the skin turns red and white. Surgery is required for this kind of burn, and there are scars. In third-degree burns, the epidermis, dermis, underlying skin tissues, and even muscles and bones are destroyed. The wound surface is covered with necrotic tissue. Third-degree burns require surgery and will leave a large scar. Burns of the fourth degree injure the underlying muscle or bone more deeply. Due to the elimination of nerve endings, third- and fourth-degree burns are not painful. They require surgery and meticulous burn care to avoid infection. Loss of the affected area is often common in fourth-degree burns (Kumari and Nanda, 2022; Xiong W. et al., 2023; Luck et al., 2021).
Furthermore, burns can be categorized as major or minor. A minor burn is one that covers less than 10% of the TBSA. The definition of a major burn is not always clear. Guidelines for categorizing serious burn injuries are as follows: more than 20% TBSA in adults, more than 30% in children, and more than 10% in elderly patients (Jeschke et al., 2020).
3 Zones of the burn area
The tissue damage model proposed by Jackson categorizes tissue injury into three distinct zones, namely, the zone of coagulation, the zone of stasis, and the zone of hyperemia. Necrosis, loss of plasma membrane integrity, and denaturation of constituent proteins can all be seen in the coagulation zone. When it is possible, the tissue in the zone of coagulation is surgically removed because necrotic tissue is prone to infection and slows healing. Necrosis can be avoided by carefully controlling the stasis zone to treat ischemia. Yet abrupt tissue reperfusion may cause the release of inflammatory cytokines, which could lead to reperfusion damage. Vasodilatation caused by local inflammatory mediators and viable cells creates a zone of hyperemia. Unless there is a serious infection or hypoperfusion, the tissues in this zone eventually heal fully (Johnson et al., 2022; Abazari et al., 2022) (Figure 1).
4 Healing of burn wounds
Burn wound healing is a complex and vital mechanism that involves a number of molecular and cellular processes (Kumari and Nanda, 2022). Slow and incomplete healing can cause serious harm, including the loss of hair, glands, skin, and, in severe situations, tissue death (Sasmal and Ganguly, 2023). Four interrelated and overlapping phases make up this process: hemostasis, inflammation, proliferation, and remodeling (Abazari et al., 2022). Different growth factors, cytokines, chemokines, and various cells all play a role in coordinating the overlapping phases of wound healing (Su et al., 2019).
In brief, hemostasis is triggered to stop blood loss as soon as damage is sustained. The inflammatory response begins to eradicate the invading pathogens and prepare the tissue for healing. During the proliferative phase, neovascularization, fibroblast migration, and re-epithelialization occur in a suitable way. Ultimately, in the remodeling stage, a scar takes the place of the granulation tissue (Ma et al., 2023; Fakouri et al., 2024) (Figure 1).
4.1 Hemostasis
Usually, burn wounds do not bleed right away after being injured. Still, hemostasis and vasoconstriction are the first steps in the healing process for burn wounds, just like they are for other traumas (Johnson et al., 2022). Constriction of the damaged vessels initiates hemostasis quickly after injury, preventing excessive blood loss. This process, also known as the pro-inflammatory stage, starts as soon as the damage occurs. After tissue damage, prostaglandin 2-α and thromboxane A2 are released into the injured area, leading to an extreme vasoconstrictor reaction that lasts for 5 to 10 min. The small vessels in the wound are then constricted to achieve hemostasis. Vasodilation, or the enlargement of blood vessels, comes next and reaches its peak 20 min after it. As a result, tissue hypoxia and acidosis develop, dampening the vasoconstrictive effect and heightening vascular permeability for inflammatory cells. At this point, platelets are crucial because they initiate the clotting process and release numerous signaling molecules such as platelet-derived growth factor (PDGF), epidermal growth factor (EGF), fibronectin, fibrinogen, histamine, serotonin, and the Von Willebrand factor (Abazari et al., 2022; Lasocka et al., 2019).
4.2 Inflammation
The inflammatory phase attracts more cells to the wound site, including neutrophils, mast cells, monocytes, and T lymphocytes. When a wound is this far along in its healing process, it shows changes in a number of different substances, including transforming growth factor-β (TGF-β), tumor necrosis factor alpha (TNF-α), EGF, PDGF, VEGF, FGF, IL-1, IL-6, IL-8, and IL-12. These mediators influence angiogenesis, collagen synthesis, epithelialization, and the regulation of the inflammatory process (Lasocka et al., 2019).
Macrophages stimulate cell growth and migration by secreting FGF, PDGF, VEGF, and TGF-α/TGF-β. In addition, macrophages eliminate pathogens and debris from the injury site (Bai et al., 2022). During the early phases of healing, M1 macrophages play a role in phagocytic activity, phagocytosing neutrophils, and eliminating any remaining bacteria or debris in a wound. The transition from M1 to M2 initiates fibroblast proliferation and angiogenic activity by producing anti-inflammatory mediators and extracellular matrix (ECM) (Miricescu et al., 2021). Other than leukocytes, regulatory T cells (Tregs) can control tissue inflammation by reducing the production of interferon-γ (IFN-γ) and augmenting the number of macrophages that promote inflammatory responses (Sorg et al., 2017). Once macrophages begin secreting EGF and PDGF, they create granular tissue, allowing tissue regeneration to progress into the proliferative phase (Abazari et al., 2022).
4.3 Proliferation
This stage involves the repair of blood vessels and the formation of granulation tissue at the wound site through a series of continuous processes, including angiogenesis, fibroblast migration, and epithelialization. It begins 4 days after the injury and lasts for 2 weeks (Abazari et al., 2022; Ayavoo et al., 2021; Fakouri et al., 2024). Angiogenesis occurs under particular conditions, such as low oxygen tension and low pH, in the wound’s area. Fibroblasts, macrophages, and epidermal cells produce bFGF, VEGF, and TGF-β, which promote it. Fibroblasts are the most common cells during the proliferative phase because they are the ones that create the new matrix needed to restore the structure and form of injured tissue. TGF-β and PDGF cause fibroblast proliferations, which are released from a hemostatic clot and migrate to the damaged arteries (Ayavoo et al., 2021). At this time, activated fibroblasts that have differentiated into myofibroblasts begin contracting the wound’s edges (Guillamat-Prats, 2021).
Fibrin, fibronectin, and collagen are all products of fibroblast cells attaching to a matrix. Tissue granulation begins at the wound site when collagen and fibronectin are secreted. Finally, epithelial cells build a full layer on the wound by increasing their mitotic activity, and they are attached to the cell matrix. The final step is the creation of the basement membrane by the aggregation of mature epithelial cells (Ayavoo et al., 2021). When the wound is fully covered and an intact epithelial barrier is reestablished, the process of epithelial migration and proliferation ends. M2 macrophages control the proliferation and migration of keratinocytes, fibroblasts, and endothelial cells, which leads to tissue regeneration and an abundance of ECM. High amounts of immature collagen type III are secreted into the matrix by fibroblasts (An et al., 2021).
4.4 Re-modeling
In the final stage of wound healing, termed as remodeling or maturation, cellular and vascular components gradually decrease. This phase normally begins after the finalization of granulation tissue growth and lasts for a very long time, anywhere from 21 days to a year following the injury (Qin et al., 2023). Wound re-epithelization by keratinocytes and ECM deposition by fibroblasts and endothelial cells mark the beginning of the remodeling phase of wound healing (El Ayadi et al., 2020). The last stage of wound healing ends when scar formation occurs (Sorg et al., 2017), which is largely dependent on how the collagen fibers are arranged. Small, parallel bundles of collagen are characteristic of normal scars, while hypertrophic scars are characterized by thinner, more numerous, and more cross-linked collagen fibers (El Ayadi et al., 2020). In the following, collagen I begins to gradually replace collagen III (Kirby et al., 2015). Collagen I is deposited more slowly than collagen III but has greater tensile strength and thus replaces collagen III in the ECM (El Ayadi et al., 2020). Matrix metalloproteinases (MMPs) and tissue inhibitors of metalloproteinases (TIMPs) are the main factors that regulate this process (Kirby et al., 2015).
5 Burn wound infections
Burn wound infection (BWI) has always been a great challenge of burn care (Guo et al., 2022). After extensive burn damage, multiple infectious problems may arise because the skin barrier has been damaged and the host’s cellular and humoral immune responses have been dampened both locally and systemically (Vinaik et al., 2019). The microorganisms that colonize burn wounds come from the endogenous flora of the patient. On the other hand, polluted hospital surfaces, water, pollutants, air, and healthcare personnel’s hands could also transmit them to the patient (Al-Maliki et al., 2022).
Microorganisms are present in nearly every wound; however, not all wounds become infected (Ding et al., 2022). Scar tissue is made up of avascular necrotic tissue and forms on the surface of deep partial-thickness and full-thickness burns. The scar provides a location rich in favorable proteins for microbial colonization and growth. Scar tissue not only limits host immune cell movement but also blocks the delivery of systemic antimicrobials to the site of injury. Also, the release of toxic compounds from the scar disrupts the majority of the host’s immunological responses. In addition to the nature and severity of thermal injury, the kinds and number of microorganisms present at the burn site influence the wound infection (Kirby et al., 2015).
According to research, wound infections account for 42 to 65% of burn-related fatalities (Maitz et al., 2023). Infection is caused by a high concentration of bacteria (>105 CFU) in and around the wound (Msheik et al., 2023). Burn wound infection usually occurs in the acute phase after injury. Patients of different ages experience significant changes in the incidence of infection. In general, compared to other age groups, elderly people (over 55 years old) and young children (under 4 years old) are more likely to get wound infections and mortality (Zhang et al., 2021). A person’s immune system, the depth of the wound, and the surrounding environment all play a role in what kinds of microorganisms infect the skin and the wound. Generally, wound infections caused by bacteria, fungi, or viruses on the skin can occur (Table 1) (Solanki and Nagori, 2013).
One major challenge is the prevalence of organisms that are resistant to drugs. Methicillin-resistant S. aureus (MRSA) is the most common resistant bacterium in burns, typically treated with the antiquated antibiotic vancomycin (Branski et al., 2009; Diederen et al., 2015). The resistance of organisms to antibiotics has led to the repurposing of old antibiotics. Recently, researchers have reinvigorated the antibiotic colistin, which caused side effects such as neurotoxicity and nephrotoxicity, to combat MDR. Researchers have demonstrated favorable therapeutic properties by conjugating colistin to dextrin in nano-antibiotic therapeutic polymers (Azzopardi et al., 2013a,b).
6 Mesenchymal stem cells
Alexander Fridenstein first defined MSCs in the 1960s. MSCs are adult multipotent stromal progenitor cells that are heterogeneous, non-hematopoietic, and capable of self-renewal as well as differentiation into many lineages and cell types (Al-Anazi et al., 2020; Mahjoor et al., 2023a). There is some evidence that stem cell origin, proliferation procedures, and the culture microenvironment all have a role in MSCs’ ability to differentiate (Yu et al., 2023). MSCs have been widely used in the fields of tissue engineering and regenerative medicine due to their low immunogenicity, high capacity for self-renewal, multidirectional differentiation, and comparatively simple, non-invasive access.
MSCs are almost always found in all tissues and have certain similarities in their phenotype, structure, and functions. Therefore, several sources have been proposed for their isolation (Najar et al., 2022). Bone marrow, adipose tissue, and the umbilical cord have been the main sources of MSCs for therapeutic use (Álvarez-Viejo, 2020). MSCs should have the following properties, as per the guidelines laid out by the International Society of Cell Therapy (ISCT) Committee: I. proficiency in adhering to and growing on plastic in a controlled laboratory setting; II. the capacity to undergo in vitro cell differentiation into osteoblasts, adipocytes, and chondroblasts; III. the presence of MSC-specific markers (Dabrowska et al., 2021).
MSCs lack the expression of markers such as CD45, CD35, CD19, CD11b, CD34, CD14, CD79α, and human leukocyte antigen-DR (HLA-DR) while expressing CD105, CD73, CD71, CD44, CD271, and CD90. Because MSCs lack MHCII and have low MHC1, they are immunologically inactive. Tissue regeneration is made possible by MSCs because of their immunomodulatory properties and their ability to transdifferentiate into different cell types. MSCs release prostaglandins, chemokines, and cytokines that impact the function of immune cells. In addition, they increase the production of regulatory T cells and subtypes of anti-inflammatory macrophages (Abdul Kareem et al., 2021; Zawrzykraj et al., 2023; Bhujel et al., 2023; de Witte et al., 2016). After MSCs are grafted into the host, they have been shown to be very immunogenic (Yu et al., 2023).
Various clinical investigations have demonstrated the usefulness of both autologous and allogeneic MSCs as sources for tissue formation. Specifically, researchers have assessed the safety of administering autologous MSCs and their capacity to reduce immunological risk. Research by Falanga et al. has shown that using autologous bone marrow mesenchymal stem cells (BM-MSCs) to treat wounds is an effective and safe option (Margiana et al., 2022).
6.1 BM-MSCs
BM-MSCs are a class of heterogeneous cells made up of multipotent stem cells that Frieden first recognized (Gao et al., 2022). Just 0.002% of all stromal cells are BM-MSCs (Mazini et al., 2019). They have the capacity to differentiate into a variety of cell types, including osteoblasts, chondrocytes, myocytes, adipocytes, epithelial cells, neuron cells, fibroblasts, myofibroblasts, keratinocytes, and endothelial cells (Revilla et al., 2023). The first type of cells to be employed in burn wound therapy is BM-MSCs. It has been demonstrated that BM-MSCs have the capacity to promote angiogenesis, scarless healing, and enhanced collagen formation, all of which are crucial factors in effective wound healing.
BM-MSCs have several benefits, but low yield and restricted availability of donors are among their disadvantages (Abdul Kareem et al., 2021). Despite the traditional harvesting of stem cells from the bone marrow, which is an invasive method with low efficiency, the ability of cell fusion enables them to regenerate tissue and immunity (Francis et al., 2019). BM-MSCs have the ability to modulate the immune system. They directly inhibit the proliferation of monocytes, DCs, and inflammatory T cells. In addition, they produce mediators that reduce inflammation, including IL-1Ra, PGE2, IDO, and IL-10 (Gherghel et al., 2023) (Table 2).
In a research by Xue et al., BM-MSC was injected into 30 mice that had burn injuries. In with the control group, the recovery period shortened from 25 to 20 days (Purwanthi, n.d.). Singer et al. conducted a similar investigation using a rat model and found that injecting rat BM-MSCs intravenously can postpone the course of burn damage in a rat comb-burn model measured from the necrotic region (Singer et al., 2013). Formigli et al. (2015) used a mouse model to study what happened when BM-MSCs were transplanted onto bioengineered scaffolds that had platelet-rich plasma (PRP) in them. The test results showed evidence of improved skin regeneration quality, less collagen deposition, more neoangiogenesis, and repaired sebaceous glands and hair follicles (Formigli et al., 2015).
Aryan et al. (2019) conducted a study with the aim of determining whether hBM-MSC can enhance wound healing in deep second-degree burns in male rats. They randomly divided 32 adult male rats per time point into four groups: (1) control group, (2) sham group (DMEM), (3) common treatment group (CT), and (4) conditioned media group (CM). They reported that, relative to the control and DMEM groups, the CM and CT groups demonstrated a significant enhancement in wound closure on the 15th and 28th days after the burn injury. In addition, hBM-MSC facilitated increased cell proliferation, and it promoted both collagen synthesis and angiogenesis at the injury site (Aryan et al., 2019).
6.2 Adipose-derived MSCs
The first study to describe AD-MSCs was by Zuk in 2001, which piqued the interest of researchers (Czerwiec et al., 2023). AD-MSCs exist between adipocytes and the vascular endothelium. Adipose tissue can be used to easily separate 100% of local MSCs, and retrieval does not need cell culture. These stem cells, which can repair all layers of skin, can also be extracted from discarded burn skin (Abdul Kareem et al., 2021). Fat stem cell research on mice has demonstrated that by boosting tissue renewal and cell proliferation, it also boosts the development of new blood vessels and the control of proteins (Lukomskyj et al., 2022). Furthermore, the advantages of AD-MSCs are their high availability, minimum invasiveness, and no restrictions (Nourian Dehkordi et al., 2019). Zhu et al. (2012) demonstrated that AD-MSCs grew and doubled quicker than BM-MSCs (Abu-El-Rub et al., 2024). Compared to BM-MSCs, adipose tissue extraction has fewer risks and negative effects. They can also develop into multiple cell lineages and produce nutrients and immune-modulating substances (Goncharova et al., 2019).
The best option in regenerative medicine is AD-MSCs since they are readily available, contain macrophages, fibroblasts, and endothelial stem cells, and have incredible pluripotent potential (Abdul Kareem et al., 2021). AD-MSCs reduce the inflammatory response by upregulating chemokines and cytokines through Th cells and IL-10 (Liu et al., 2018). Zhang et al. (2018) found that AD-MSCs’ paracrine activity boosts wound healing (Jo et al., 2021). Researchers have conducted clinical studies with AD-MSCs using both autologous and allogeneic transplants. The allograft method’s low immunological rejection risk is another benefit of AD-MSCs, but there are a few drawbacks to take into account before using AD-MSCs. This specific cell population has limited ability to self-renew (Czerwiec et al., 2023).
AD-MSCs are widely used in plastic surgery, and depending on the type of adipose tissue that is harvested, they have different functions. White adipose tissue, which is extracted from visceral and subcutaneous surfaces, has the ability to store triglycerides, and brown adipose tissue is extracted from the neck, mediastinum, supraclavicular, and par scapular regions. They are involved in the production of body heat, the ability to modulate the immune system, neoangiogenesis, and endogenous repair; they cause wound regeneration; and by releasing hormones such as leptin, they can be effective in wound healing (Francis et al., 2019). Paganelli et al. (2019) used MSCs sourced from adipose tissue to construct a dermal replacement for wound healing. This substitute exhibited excellent mechanical qualities and remarkable biocompatibility (Jo et al., 2021) (Table 2).
6.3 Umbilical-derived MSCs
One of the finest sources of MSCs is the umbilical cord. They include both hematopoietic and non-hematopoietic cells, as well as endothelial progenitor cells. Different stem cell types may be extracted from the numerous layers of the umbilical cord, including Wharton’s jelly, veins, arteries, the lining of the cord, and the sub meningeal and perivascular areas (Abdul Kareem et al., 2021; Phan et al., 2023). Due to their adaptability, simplicity in isolation and culture, rapid proliferation, easier differentiation, and immunosuppressive qualities, umbilical cord-derived MSCs have enormous potential as a therapeutic tool in tissue engineering and regenerative medicine (Jo et al., 2021; Phan et al., 2023).
According to the studies, it was shown that the umbilical cord stem cell has a high ability to heal burn wounds by increasing skin appendages and creating fibers. However, this source has more limited access compared to fat tissue and bone marrow (Lukomskyj et al., 2022). Umbilical cord cells have abilities such as detecting inflammatory tissues, differentiating to prevent inflammation, and performing anti-inflammatory activities. By releasing cytokines, they cause wound tissue repair and regeneration. In laboratory conditions, they have the ability to transfer to the body and differentiate into efficient cells (Atluri et al., 2020). Studies in rats indicated that UC-MSCs hastened wound healing by blocking inflammatory cell infiltration, decreasing IL-6, 1, and TNF-a, and boosting IL-10 and TSG-6 (Nourian Dehkordi et al., 2019). According to a study by Liu et al. (2016), UC-MSCs lessened acute inflammation in rats that had suffered severe burns. They demonstrated the TNF-stimulated gene/protein 6 (TSG-6) anti-inflammatory mechanism (Xiong W. et al., 2023).
An abundant supply of MSCs can be found in the umbilical cord’s Wharton’s jelly-like matrix (Nazempour et al., 2020). MSCs from Wharton’s jelly (WJ-MSCs) promote wound healing by reducing inflammation (Mansour et al., 2023). There are several advantages to using WJ-MSCs, including its accessibility to a broad pool of donors, ease of acquisition, lack of danger to the donor, lack of ethical constraints, low immunogenic potential, and high differentiation capability (Owczarczyk-Saczonek et al., 2018) (Table 2). They show significant immunomodulatory activity similar to BM-MSCs (Nazempour et al., 2020). These cells have the potential to transform into cells that gland-like cells, which speeds up the healing process of the skin (Hashemi et al., 2020). In addition, the donor is completely safe because contact with infectious pathogens is so infrequent (Owczarczyk-Saczonek et al., 2018).
6.4 Placenta-derived MSCs
Over a century has passed since placental tissue (PL) was first used to treat wounds (Azzopardi et al., 2013b). PL is an excellent source of growth factors and stem cells, which are vital to healing and creating new tissues (Teoh et al., 2023). There are several benefits associated with the placenta: the ability to obtain the maximum number of cells; the possibility of harvesting them using non-invasive techniques; a lower immune response than BM-MSCs due to their embryonic origin; and favorable immunomodulatory effects in vitro (Abd-Allah et al., 2015; Maxson et al., 2012) (Table 2).
In 2006, Chang et al. showed that PL-MSCs had a much stronger immunosuppressive impact than BM-MSCs (Malek and Bersinger, 2011). PL-MSCs differentiate into adipocytes, osteocytes, chondrocytes, endothelial cells, and neuronal cells (Maxson et al., 2012; Makhoul et al., 2013). Compared to BM-MSCs, PL-MSCs exhibit greater proliferation ability. However, Li et al. showed that BM-MSCs migrate faster than PL-MSCs, which suggests that BM-MSCs are better able to get through the endothelial blood vessel barrier (Makhoul et al., 2013). PL-MSCs’ ability to stimulate neovascularization, wound diminution, and enhanced blood flow speeds up the healing process (Shojaei et al., 2019).
Research has shown that PL-MSCs exhibit high quantities of the cell adhesion molecules programmed death ligands 1 and 2 (PD-L1 and PD-L2), which may block T-cell proliferation by stopping the cell cycle (Shojaei et al., 2019).
6.5 Amniotic membrane-derived MSCs
The amniotic membrane (AM) has been the subject of extensive studies as a cell source for regenerative therapies. This membrane is responsible for the fetus’s physical defense, pH control, and the release of anti-inflammatory substances (Miatmoko et al., 2023). The AM lacks vascular tissue and contains a three-layer structure. It consists of an epithelial cell monolayer, an acellular intermediate layer, and an outer layer that contains MSCs (Roubelakis et al., 2012). Generally, the AM includes amniotic membrane-derived MSCs (AMSCs), amniotic epithelial cells (AECs), and fibroblasts (Hu et al., 2023; Nejad et al., 2021). The AM-MSCs have the ability to differentiate into cells of the ectodermal, mesodermal, and endodermal layers (Kim et al., 2014).
The epithelium seems to be a source of biologically significant stem cells. Extracellular matrix proteins such as collagen and fibronectin make up the basement membrane. Both the stroma and the spongy layer contain vital chemicals and agents for regeneration (Pfister et al., 2023). AECs secrete several substances that suppress T and B lymphocyte proliferation as well as the chemotactic abilities of neutrophils and macrophages in inflammatory circumstances such as wounds. Another interesting finding is that AM binds to T cells and other leukocytes, preventing them from taking part in the inflammatory process (Aghayan et al., 2022).
AM-MSCs are the sources of keratinocyte growth factors (KGF) and EGF (Miatmoko et al., 2023). Since 1910, the usage of human amniotic membrane (hAM) grafts in the therapy of burn wound healing has increased significantly (Rahman et al., 2019). The AM influences wound healing through the absorption and transplantation of endogenous progenitor cells, which promotes neovascularization and wound repair. AM decreases the local inflammatory response as a result of the release of chemokines and cytokines that regulate the immune response at the site of the wound. Consequently, AM can have a favorable impact on wound healing (Pfister et al., 2023).
Studies using mouse models have demonstrated that AM usage decreases local inflammation, promotes cell renewal, and also promotes collagen formation. In addition, the use of AM was linked to a reduction in the number of bacteria in infected mouse burn wounds. In a sheep model, Fraser et al. demonstrated that AM therapy at burn sites considerably reduced the quantity of scar tissue (Pfister et al., 2023). Some advantages of using amniotic membrane stem cells in the healing process include alleviating pain, reducing inflammation, regulating fluid loss, decreasing bacterial colonization, preventing scars, the minimum ethical considerations, and low immunogenicity. All of these advantages make amniotic membrane stem cells appealing to people and give them the chance to be used in cell therapy and regenerative medicine (Hu et al., 2023; Pfister et al., 2023) (Table 2).
6.6 Amniotic fluid-derived MSCs
Amniotic fluid (AF) is a feeding and protecting liquid that helps the embryo develop normally (Harrell et al., 2019). Amniotic fluid (AF) contains high concentrations of α-defensin, lysozyme, calprotectin, and cathelicidin, as well as TGFα, TGFβ1, insulin-like growth factor 1 (IGF1), and erythropoietin (EPO) (Nyman et al., 2022). AF-MSCs were first identified as a source for wound healing in 2013 (Rahman et al., 2019). AF-MSCs are obtained from AF samples during the second trimester (16–28 weeks) through amniocentesis (Harrell et al., 2019; Anudeep et al., 2022). Many research studies have shown that volume, donor variability, and gestational stage are the three most important factors that affect the number of cells obtained from amniocentesis (Teoh et al., 2023) (Table 2).
The risks of AF harvesting during the second or third trimester include harm to the mother or fetus, infection, premature labor, and even miscarriage. Nonetheless, AF obtained following cesarean section births appears to be a non-invasive, abundant, and high-yield cell source for cell treatment (Mankuzhy et al., 2021). AF-MSCs are adult, fibroblast-like, self-renewable, multipotent stem cells with broad distinction and minimal immunogenicity (Harrell et al., 2019). AF-MSCs express a variety of antigens, such as HLA-ABC, CD73, CD44, CD105, CD166, CD117, CD29, CD49e, CD58, and CD90, but these cells do not express hematopoietic markers such as CD34, CD14, CD45, CD133, CD31, and HLA-DR (Mankuzhy et al., 2021).
6.7 Hair follicle-derived MSCs
The hair follicle (HF) is a dynamic little organ that supports several vital bodily biological processes. HFs are a readily available source of stem cells that may self-renew, differentiate, control hair growth, and help maintain skin homeostasis. Research has demonstrated that hair follicle stem cells (HFSC) are both multipotent and extremely proliferative in laboratory settings (Mistriotis and Andreadis, 2013). HFSCs exhibit immunological rejection, making them the ideal donors for cell-based therapies. According to Li et al.’s study, HFSCs are superior to other cell types at repairing wounds (Abdul Kareem et al., 2021).
Studies on the usage of HF-MSCs in wound healing are still limited (Ou et al., 2020). There are a number of benefits to using HF-MSCs, including an abundance of sources, ease of access, robust cell proliferation, broad differentiation potentials, low immunogenicity, lack of age limits, no ethical issues, and non-carcinogenic (Liu et al., 2024; Li S. et al., 2022; Zaki et al., 2020). They have the potential to develop into HFs, sweat glands, sebaceous glands, keratinocytes, and inter-follicular epidermis. So, it has the potential to improve the healing process of wounds (Zaki et al., 2020) (Table 2).
According to reports by Kevin et al., HF-MSCs can aid in the healing of chronic wounds (Liu et al., 2024). When HF-MSC is used to treat wounds in vivo, it accelerates the transition from fibroblasts to myofibroblasts, which in turn reduces the duration of the proliferation stage (Yang H. et al., 2023).
6.8 Dental pulp-derived MSCs
Because dental pulp has a high content of cells and requires relatively few invasive methods for cell separation, it has recently been regarded as a potential source of MSCs (Abbas et al., 2018). Improvements have been shown after transplantation of DP-MSCs (derived from the teeth of adult patients), which are attributed to the production of paracrine substances by these cells (Gomes et al., 2010). Through paracrine impact, the DP-MSCS may drive endogenous stem cells to regenerate damaged tissues by secreting growth factors, cytokines, chemokines, angiogenic factors, and exosomes (Ichi et al., 2023). Studies have shown that DP-MSCs have more angiogenic potential in comparison with ASCs and BMSCs. In addition, DP-MSCs have a high level of CD73 expression (Abbas et al., 2018) (Table 2).
6.9 Burn-derived MSCs
Research has shown that the use of human MSCs extracted from burned skin accelerates the healing process in rat and pig burn models (Surowiecka et al., 2022). Amini-Nik et al. demonstrated for the first time the presence of viable mesenchymal skin stem cells in full-thickness burned skin. Furthermore, based on their reports, these cells were easily removed, expanded in vitro, and then added to wound covering in a simple and inexpensive way. The majority of patients are willing to donate these discarded tissues. Cell isolation from burned skin is a non-invasive procedure that poses no danger to the patient. Because they are the patient’s own skin stem cells, the risk of immunological response and rejection is minimal (Amini-Nik et al., 2018) (Table 2).
In another study, MSCs were extracted from the eschar by Van der Veen et al. They believed that MSCs from the burn eschar had moved from another source of MSCs, such as subcutaneous fat, into the wound region. In addition, it has been found that burn patients’ blood has higher concentrations of circulating MSCs. The function of these cells and when they are introduced to the wound site remain uncertain. These cells have the myofibroblast phenotype, which produces ECM and contracts wounds; therefore, they may aid healing (Van Der Veen et al., 2012).
7 Function of MSCs in burn wound healing and skin regeneration
MSCs can help with wound healing by changing into other cell types such as fibroblasts, epithelial cells, and keratinocytes. MSCs have the potential to modulate the local reparative responses in injured areas by attracting host cells, including fibroblasts, keratinocytes, macrophages, and progenitor cells. Then, the paracrine effects that lead to an increase in angiogenesis, neovascularization, de-epithelization, collagen production, and, in the end, the release of several growth factors and cytokines promote wound healing (Mahmoudian-Sani et al., 2018; Maranda et al., 2017; Mirshekar et al., 2023).
Paracrine factors increase homeostatic and anti-apoptotic genes and decrease nucleic acid, protein metabolism, and apoptotic genes (Tamama and Kerpedjieva, 2012). Following severe burn injury, the hypermetabolic response is triggered by the systemic inflammatory response, which initiates protein catabolism and breakdown. This leads to the uncontrollable release of pro-inflammatory mediators, which exacerbates organ dysfunction and protein loss (El-Sayed et al., 2024).
Van Badiavas’s laboratory has shown in vitro that MSCs may also promote wound healing by the production of exosomes that include transcription factors, mRNA, and miRNA that are essential for wound healing (Maranda et al., 2017). Aryan et al. (2019) used stereological techniques to examine mice with profound second-degree burns that had received human bone marrow mesenchymal stem cell-conditioned media (hBM-MSC-CM). According to this study, hBM-MSC-CM promotes basal cell and fibroblast growth, stimulates collagen and blood vessel production, and has anti-inflammatory properties that aid in the healing of skin lesions. In addition, the stereological data showed that the hBM-MSC-CM group had epithelialization with thick dermis, fibrous, and granular tissue, while the control group had less collagen production and more inflammatory cells (Aryan et al., 2019).
7.1 Effects of MSCs in homeostasis phase regulation
Significant amounts of phosphatidylserine and tissue factor (TF) are present on the surface of MSCs and MSC-derived extracellular vesicles (EVs), which induce coagulation. In fact, clot formation can be exacerbated because of the expression of these two elements that trigger a thrombotic reaction. Some research has demonstrated that Annexin V is on the surface of MSCs. It indicates that phosphatidylserine is present, which is what makes clots form (Guillamat-Prats, 2021).
7.2 Effects of MSCs on the inflammatory response
MSCs begin to have immunosuppressive effects once they arrive at injury sites (Hu et al., 2018). MSCs immediately reduce the inflammatory response. In addition, MSCs regulate it by lowering the quantity of neutrophils, macrophages, and activated T cells (Maxson et al., 2012; Mahjoor et al., 2023b). Pro-inflammatory cytokines, including IL-1, TNF-α, and IFN-γ, are reduced in MSC-treated wounds, while anti-inflammatory cytokines such as IL-10 and IL-4 are increased. The immunosuppressive phenotype of MSCs is activated with exposure to pro-inflammatory cytokines such as IFN-γ, TNF-α, IL-1α, and IL-1β, leading to the expression of chemokines and inducible nitric oxide synthase (iNOS), all of which inhibit T-cell responsiveness to inflammation (Maxson et al., 2012; Hu et al., 2018).
The presence of TNF-α and IFN-γ may increase the production of cytokines such as cyclooxygenase 2 (COX2), hepatocyte growth factor (HGF), and prostaglandin E2 (PGE2) for inhibiting T-cell proliferation. Furthermore, they aid in the synthesis of chemokines such as CCR5, CCR10, and CXCL9, which prevent the growth of immunological effector cells (Huang et al., 2022). T cells emit less IFN-γ and more IL-4 to respond to MSC activity. Thus, the number of regulatory T cells rises. In addition, MSCs control the growth, development, and activity of B cells and natural killer cells (NK cells), leading to reduced IFN-γ secretion by NK cells (Maxson et al., 2012; Hu et al., 2018). MSCs can cause a switch from the pro-inflammatory M1 state to the anti-inflammatory M2 state of macrophage polarization (Ulivi et al., 2014).
7.3 Improving the proliferative stage using MSCs
Fibroblasts and myofibroblasts are crucial during the third phase of wound healing. Proliferation and restoration of epithelial cells, as well as the synthesis of collagen and ECM proteins, occur during this phase (Andalib et al., 2023). A vast array of growth factors, including PDGF, VEGF, FGF, and tumor necrosis factor-induced Dutch gene-6 (TSG-6), are also produced by MSCs and can promote the reparative abilities of fibroblasts, endothelial cells, and tissue precursor cells. MSCs, by acting on PDGF-BB, promote the migration, secretion, and proliferation of fibroblasts (Liu et al., 2022).
7.4 Modifying the remodeling phase with MSCs
In the latter phases of wound healing, MSCs effectively manage matrix remodeling and scar reduction (Magne et al., 2018). MSCs play a role by secreting MMPs to induce matrix deposition and TIMPs to prevent ECM deposition. It is thought that MSCs can reduce hypertrophic scarring by secreting HGF, FGF, adrenomedullin, and TGF-3 (Riedl et al., 2021). Stoff et al. (2009) found that after transplanting human MSCs into rabbit wounds, the wounds’ tensile strength rose and scar formation was greatly reduced. These results show that future MSC therapy may result in less scarring (Li et al., 2015).
8 The potential anti-infection effect and drug delivery system of MSCs in burn wound
Researchers are increasingly studying the potential anti-infection effect of MSCs in burn wounds, which can treat immune and inflammatory diseases caused by infection due to their paracrine function (Miao et al., 2021). Direct and indirect processes contribute to MSC antimicrobial activity. Direct methods involve the production of antimicrobial factors such as LL-37, and indirect methods involve the release of immunomodulatory substances that stimulate immune cell phagocytosis and the death of pathogens (Maxson et al., 2012).
Interestingly, MSCs are employed not only in regenerative medicine but also as carriers for drug delivery. MSCs offer advantages such as low immunogenicity, homing ability, and tumor tropism, making them ideal for targeted drug delivery systems (Matsuzaka and Yashiro, 2024). Despite the promising future of MSCs-DDS in targeting drug delivery, several inherent limitations of MSCs, such as poor drug loading capacity, limited homing efficiency, and potentials risk of living cells administration have so far restricted their practical applications for disease treatment (Senthilkumar et al., 2020).
Several novel technologies are being developed in parallel to improve the efficiency or safety of this system. Among technologies, nanotechnology and genome engineering are most employed to improve the drug loading capacity and homing efficiency of MSCs. Biomimetic technology has recently been proposed as a revolutionary approach to further improve the cell-based DDS, offering the superior benefits of high drug loading efficiency with similar or even better homing capability compared to cellular carriers, and also avoiding the potential risks of using living cells. Thus far, numerous technologies have been applied to overcome the aforementioned challenges of MSCs-DDS, and some of them have successfully improved the performance of MSCs in drug delivery (Su et al., 2021).
9 The immunomodulatory properties of MSCs
One of the main characteristics of MSCs that makes them a desirable instrument for cell therapy is their immunomodulatory capabilities (Sarsenova et al., 2022). In 2002, Bartholomew et al. conducted the first study to demonstrate that MSCs reduce lymphocyte proliferation in vitro and increase skin graft survival in vivo (Radmanesh et al., 2020). MSCs have the ability to sense an injury and activate both innate and adaptive immunity, even if the response is weak. In addition, if the immune cells in the wounded area are overactive, they can be suppressed. This function is also referred to as the “sensor and switcher of the immune system,” which is controlled by many mechanisms (Sarsenova et al., 2022) (Figure 2).
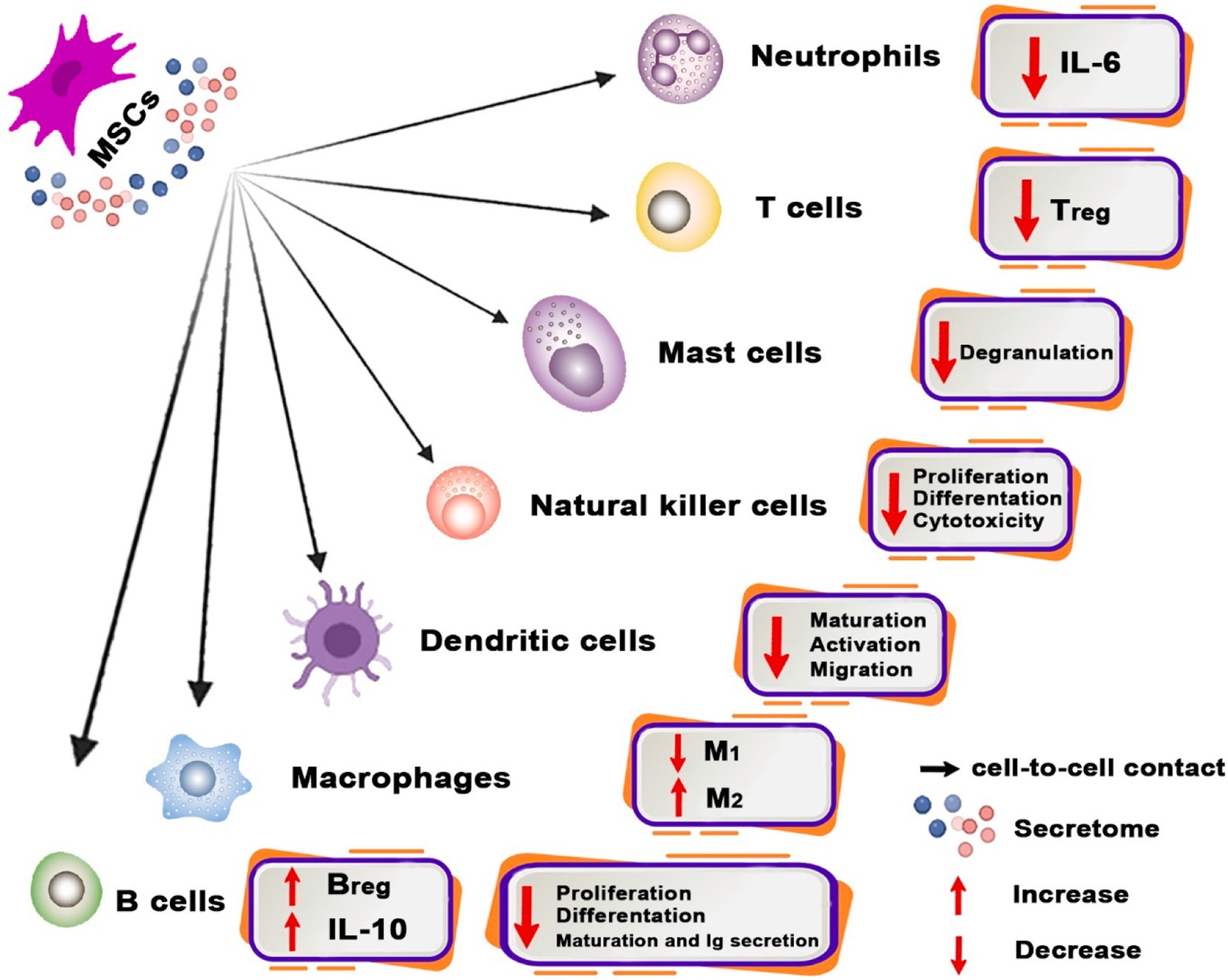
Figure 2. Immunomodulatory properties of MSC and related mechanisms. Immunomodulatory properties of MSCs possess the capability to modulate the functions of diverse immune system cells. MSCs facilitate the transition of macrophages from the pro-inflammatory M1 phenotype to the pro-healing M2 phenotype and regulate the development of dendritic cells (DCs) into a tolerogenic phenotype. Moreover, MSCs inhibit mast cell granulation and diminish IL-6 generation by neutrophils, as well as the proliferation, differentiation, and cytotoxicity of NK cells. Moreover, MSCs alter the phenotypic of B cells toward Breg and reduce Treg numbers.
MSCs generally perform as immunomodulators by preventing pro-inflammatory or effector immune cells from proliferating and maturing and also by directing certain immune cells into tolerogenic and anti-inflammatory phenotypes (Sinha et al., 2023). MSCs demonstrate their ability to modulate the immune system by inducing functional alterations in many immune cell types, including macrophages, dendritic cells (DCs), neutrophils, mast cells, natural killer (NK) cells, T cells, and B cells (Díaz-Prado et al., 2011; Subhan et al., 2021).
9.1 Association of MSCs with innate immune cells
9.1.1 Macrophages
Macrophages are a vital component of the innate immune response and play a major role in the control of the inflammatory response (Maranda et al., 2017). Macrophages may be categorized into pro-inflammatory M1 and anti-inflammatory M2 macrophages based on their phenotypic and functional characteristics (Mirshekar et al., 2023). In contrast to M1 macrophages, which secrete pro-inflammatory cytokines such as IL-6, IFN-γ, TNF-α, and iNOS, M2 macrophages suppress inflammatory responses and hasten the healing of wounds by secreting a plethora of anti-inflammatory cytokines, including IL-4, IL-10, and arginase-1 (Arg-1) (Maranda et al., 2017).
Multiple studies have shown that MSCs have the ability to diminish the production of pro-inflammatory cytokines in macrophages, hence inducing a shift in macrophage polarization toward the anti-inflammatory M2 phenotype both in vivo and in vitro (Tamama and Kerpedjieva, 2012). Many molecules, such as PGE2, indolamin-2,3-dioxygenase (IDO), IL-6, HGF, IL-1 receptor antagonist (IL-1RA), TSG-6, and TGF-β, contribute to effective M2 macrophage polarization promotion (Mirshekar et al., 2023). Toll-like receptors (TLRs) allow MSCs to sense various danger signals. MSCs react to excessive pro-inflammatory signals through TNF-α, IFN-γ, and IL-1β receptors. Thus, MSCs release cytokines that either stimulate or inhibit immune responses to preserve the immunological balance. TLR2, TLR3, TLR4, TLR7, and TLR9 are all expressed by MSCs. Depending on the tissue from which these TLRs originate, their expression levels differ considerably. MSCs’ pro-inflammatory or anti-inflammatory phenotype is TLR-type dependent. As an example, TLR4 activation results in a pro-inflammatory phenotype, whereas TLR3 activation results in an anti-inflammatory phenotype (Jiang and Xu, 2020).
9.1.2 DCs
DCs are the most crucial antigen-presenting cells (APCs) (Yang et al., 2021). There are typically two phases for DCs: the immature phase and the mature phase. Phenotypic and functional changes distinguish these phases. When immature DCs that process antigens develop into mature DCs that present antigens, the adaptive immune system is activated. Mature DCs can release cytokines that boost the immune system and cause inflammation. Whereas immature DCs stop adaptive immune cells and help maintain immunological tolerance. Thus, DCs immunomodulation can be accomplished by controlling their migration and maturation (Alahdal et al., 2020; Liu X. et al., 2023). MSCs not only hinder monocyte differentiation into DCs, but they can also impede DC maturation, attraction, and migration (Alvites et al., 2022). It is known that MSCs impede this differentiation process by secreting PGE2 (Seo and Jung, 2016). MSCs limit DCs’ ability to process and present antigens by stopping mitogen-activated protein kinases in vivo. In addition, in a model of mice, MSCs increased Tregs and activated functional tolerogenic DCs (Peng et al., 2023).
9.1.3 Neutrophils
MSCs have the potential to extend the activity and survival of neutrophils and enhance the synthesis of factors including TGF-β, IFN-α, and granulocyte colony-stimulating factor. Their activation and invasion are mostly influenced by the production of IL-6 and other variables, including CXCL1, CXLC2, CXCL5, and CXCL8 (Alvites et al., 2022). To prevent neutrophil infiltration into the wound, MSCs secrete IL-10. In addition, to prevent neutrophil rolling and transendothelial migration, MSCs release TSG-6, which binds to protein ligands (Seo and Jung, 2016).
9.1.4 Mast cells
Research has shown that mast cells (MC) have close ties to scar formation and wound healing. At the start of an infection, activated MCs release certain particles, such as histamine, 5-hydroxytryptamine, heparin, trypsin, chymotrypsin, and others. They also attract other inflammatory cells, such as monocytes, macrophages, and neutrophils. Another crucial function of MCs is to identify and present foreign antigens to T and B lymphocytes. Thus, MCs play a role in adaptive immunity (Zhao et al., 2023).
9.1.5 NK cells
NKs target HLA-negative cells and have the ability to lyse MSCs because their actions are dependent on signals sent by receptors linked to HLA molecules (Alvites et al., 2022). Notably, MSCs perform through IDO, PGE2, and TGF-β1 to hinder NK cell proliferation and function (Seo and Jung, 2016; Weiss and Dahlke, 2019).
9.2 8Association of MSCs with adaptive immune cells
9.2.1 T cells
MSCs have the ability to promote the differentiation of T cells from a pro-inflammatory state to an anti-inflammatory one, primarily via the inhibition of lymphocyte proliferation and the generation of pro-inflammatory cytokines (Yang et al., 2021). MSCs impede the proliferation of activated helper T (Th) cells, which results in a reduction in the secretion of IFN-γ and IL-17 by Th1 and Th17 cells. In addition, increased IL-4 secretion by Th cells demonstrates that Th cells change from a pro-inflammatory to an anti-inflammatory phenotype (Wang et al., 2021). In CD4+ T cells co-cultured with MSCs, the Notch1/forkhead box P3 (FOXP3) pathway was shown to be activated, which increased the number of CD4+ CD25 (high) FOXP3+ cells and mediated the induction of Tregs. While suppressing TGF-β and IL-10 at the same time inhibits Treg induction in co-cultured cells, it indicates that the two factors have a significant impact on the immune-tolerance system (Yang G. et al., 2023).
9.2.2 B cells
There are three subtypes of B cells: B1, B2, and regulatory B cells (Bregs). The majority of B1 cells develop in the fetal liver and include B1a and B1b subsets. B2 cells can be further divided into follicular B (FOB) and marginal zone B (MZB) cells, which originate from bone marrow (Wang et al., 2020). FOB cells develop into plasma cells, which then secrete antimicrobial antibodies with high affinity. B1 and MZB cells have the ability to synthesize natural antibodies either in a T-cell-dependent or non-T-cell-dependent way. Bregs is responsible for suppressing the immune system through the secretion of cytokines such as IL-10, IL-35, and TGF-β or the expression of molecules that act as negative stimuli, such as FasL and PD-L1 (Liu P. et al., 2023). The ability of Bregs to suppress Th1 and Th17 responses and induce FOXP3+ has been proven in earlier research (Liu et al., 2020). MSCs can control B-cell proliferation and differentiation and prevent B-cell apoptosis. The first evidence that they can directly interact with B cells to stop their proliferation and apoptosis was found by Anna Corcione et al. in 2006 (Wang et al., 2020).
10 Experimental studies and clinical practice
The effectiveness and safety of MSCs in treating burn wounds are being investigated in clinical studies. In 2005, Rasulov et al. performed the first human study on a middle-aged female patient. She had 40% TBSA burns, 30% of which were full-thickness burns. Deep tissue was injected with BM-MSCs that resembled allogenic fibroblasts. They observed a marked improvement in hemostasis and wound bed epithelization without serious side effects (Schulman et al., 2022; Ahmadi et al., 2019). The use of autologous BM-MSCs for the treatment of severe radiation burns has been reported by Lataillade et al. (2007). This group saw the expected clinical progression and no recurrence of inflammatory radiation in 2010 after performing five local MSC transplants in conjunction with skin autograft (Bey et al., 2010).
Similarly, Mancilla et al. (2015) used a fibrin spray containing MSCs derived from bone marrow to treat a young man with 60% total burns (Ahmadi et al., 2019; Schulman et al., 2018). In addition, Portas et al. (2016) validated the use of cadaveric BM-MSCs in treating the chronic radiation-induced skin lesion (Golchin et al., 2019).
Abo-Elkheir et al. compared the wound healing effects of BM-MSCs, UC-MSCs, and early excision and transplant in patients with full-thickness burns in a study. They demonstrated that using BM-MSC and UC-MSC therapies significantly improved the rate of healing in a patient with a thermal full-thickness burn as compared with conventional methods (Abo-Elkheir et al., 2017).
Brennan et al. (2017) showed that, in contrast to BM-MSCs, AD-MSCs exhibit greater angiogenesis and inferior osteogenic capabilities in vivo.
11 Novel technologies in MSC application methods
The major treatments for full-thickness skin defects are autologous skin grafting and flap grafting. Autologous skin grafting needs a large amount of skin and may be limited by significant skin defects or pathological skin disorders (Zhao et al., 2024). MSCs, when used in conjunction with other tissue engineering methods, can improve their effectiveness in repairing skin tissue. These methods include the following:
1. Scaffolds combined with MSCs produce remarkable results. Stem cells can get nutrients and exchange gases in a controlled environment provided by tissue scaffolds. Scaffolding biomaterials must be tailored to the specific needs of the target tissue (Katiyar et al., 2022; Wang et al., 2021).
For example, chitosan and collagen together can create a scaffold that can even cure wounds completely, overcoming the drawbacks of conventional collagen scaffolds (Wu et al., 2024). Daniela et al. (2014) described mesenchymal stem cells (MSCs) seeded onto nanofibers as a potential novel alternative to split-thickness autologous skin grafting. This research demonstrated that the scaffolds increased cicatrization and extended MSC function (Steffens et al., 2014). Gholipour-Kanani (2012, 2014) and Shokrgozar (2012) introduced porous scaffolds populated with xenogeneic human and allogeneic mesenchymal stem cells, respectively. They assessed a quicker wound healing rate and histologically improved re-epithelialization compared to the scaffold-only group. Nonetheless, these investigations did not use any statistical analysis (Rangatchew et al., 2021). Clover et al. (2015) demonstrated that BM-MSC-seeded scaffolds markedly enhanced the wound healing rate by day 14 post-transplantation.
1. Compared to monolayer culture, MSCs are cultured in three-dimensional (3D) bioprinting scaffolds, which increase the synthesis of anti-inflammatory compounds (Riedl et al., 2021). Furthermore, research has demonstrated that 3D living dressings can control the immune response and stimulate neovascularization, two features essential to successful treatment (Turner et al., 2022). Materials such as polycaprolactone (mPCL) and polyethylene terephthalate (PET) can be used to 3D bioprinting scaffolds that efficiently transfer stem cells to the intended healing location (Brennan et al., 2017).
2. Hydrogels are water-absorbing polymers that maintain a moist environment, which is crucial for burn wound healing. Their properties include the following: (1) Moisture retention prevents desiccation and promotes cell migration. (2) Thermal regulation provides a cooling effect and protects against temperature fluctuations. (3) Biocompatibility reduces irritation and supports cellular interactions (Olteanu et al., 2024). (4) Hydrogels also have the potential to serve as the primary raw material for delivery carriers due to their skin-like rheological characteristics (Chai et al., 2017). Hydrogel dressings serve as carriers for mesenchymal stem cells (MSCs), facilitating their localized and sustained release. Research has demonstrated that this combination speeds up the healing process and decreases the amount of time it takes for epithelialization to occur. The anti-inflammatory properties of MSCs combined with the moist environment of hydrogels result in improved cosmetic outcomes (Khayambashi et al., 2021; Li Q. et al., 2022). Ozpur et al. (2016) used fibrin hydrogel containing keratinocytes and AD-MSCs to create in vitro skin tissue in one study. The results showed that the dermal substitute with AD-MSCs improved blood vessel growth and covered the wound again (Ozpur et al., 2016).
3. Modifying MSCs genetically can enhance their immunomodulatory activities and stimulate skin regeneration. It is possible to alter specific pathways, overexpress or suppress gene expression, or both in MSCs through genetic modification. Researchers have demonstrated that modifying MSCs using CRISPR/Cas-based non-viral gene editing enhances the transplanted MSCs’ survivability by elevating HIF1a gene levels (Riedl et al., 2021). Overcoming restrictions in editing efficiency and cytotoxicity, the CRISPR-Cas9 method enables accurate genome editing in MSCs. The development of genetically engineered MSCs with enhanced therapeutic capabilities has been made possible by ribonucleoprotein (RNP) delivery techniques, which have demonstrated low cell death and high indel frequencies (Han et al., 2024). MSCs can be engineered to overexpress growth factors, including PDGF-B, to improve their capacity to aid in wound healing (Kosaric et al., 2017).
12 The challenges of using MSCs on burn wounds
The treatment of MScs is not without challenges:
1. The issue pertains to both mass production and accessibility. Cell therapy methods typically use MSCs for tissue regeneration, and each treatment typically requires hundreds of millions of MSCs. As a result, larger bioreactors and longer in vitro cell culture growth are required (Miatmoko et al., 2023).
2. The immune system of the receiver may be impacted by the use of stem cells. The immunogenicity of the cells may change when given in non-physiological places (Miatmoko et al., 2023).
3. MSCs from diverse donors vary in proliferation, immunomodulation, and secretion. Thus, using MSCs from several unrelated donors makes it impossible to standardize and compare clinical trial data. A homogeneous cell supply with steady phenotypic and functional properties would be beneficial for regular MSC-based cellular treatment with consistent effects (Teshima, 2024).
4. Another danger is the transmission of bacterial, viral, fungal, or prion infections from the donor to the receiver, which can be threatening and even fatal (Miatmoko et al., 2023).
5. A number of issues limit the usefulness of in vivo models, including the high expense, difficulties in controlling and standardizing experimental conditions, and ethical issues surrounding the use of animals. Moreover, disparities between animal and human physiology may influence the applicability of findings to clinical settings. It is essential to weigh the benefits and drawbacks of in vivo models to obtain significant and pertinent insights into the mechanisms of burn wound healing (Das et al., 2024). Ultimately, researchers must explore alternative methodologies, such as in vitro models or computational simulations, that may provide more controlled environments and reduce ethical concerns. By integrating these approaches, the scientific community can enhance the reliability and relevance of their findings, leading to improved strategies for managing burn injuries in humans.
6. There are various ethical issues with the use of MSCs, particularly those obtained from allogeneic sources such amniotic fluid and umbilical cord:
• The procurement of MSCs from the umbilical cord and amniotic fluid often transpires during parturition. It is critical to make sure that parents are aware of the hazards and benefits of cell research before they give their consent (Corsano et al., 2015).
• As stem cell therapies become more commercialized, growing financial incentives for biological material donation raise concerns about potential exploitation of donors. This prompts questions about the commercialization of human tissues and the validity of consent. Therapies produced from these MSCs may not be accessible to everyone. Advanced treatments may be more beneficial to wealthier people or communities, which could exacerbate already-existing health disparities (Corsano et al., 2015; Mitrossili et al., 2015).
Due to the serious moral and legal concerns raised by MSCs derived from allogeneic origins, a worldwide regulatory framework is urgently required. We must promote equal access to these precious resources while also ensuring respect for individual dignity and autonomy (Mitrossili et al., 2015).
13 Conclusion
MSCs possess considerable potential in improving the repair of burn injuries owing to their regenerative capabilities. Large-scale clinical trials often lack control groups, which complicates conclusive determinations regarding efficacy and safety. The heterogeneity in MSC qualities due to donor features and isolation methods can result in variable therapeutic effects. In the future, researchers should work on standardizing how to treat MSCs and looking into combination therapies that use MSCs along with other ways to repair cells, such as biomaterials or gene therapy. By addressing these issues, researchers can enhance the reliability of MSC therapies and potentially improve patient outcomes. This approach may lead to more effective treatment options for burn injuries and other regenerative applications.
Author contributions
SA-S: Conceptualization, Data curation, Investigation, Software, Writing – original draft. MY: Data curation, Investigation, Software, Writing – original draft. MG: Conceptualization, Data curation, Formal analysis, Investigation, Methodology, Writing – review & editing. MK: Data curation, Software, Writing – review & editing. HA: Methodology, Project administration, Supervision, Validation, Writing – original draft, Writing – review & editing. SG: Project administration, Supervision, Writing – review & editing.
Funding
The author(s) declare that no financial support was received for the research, authorship, and/or publication of this article.
Conflict of interest
The authors declare that the research was conducted in the absence of any commercial or financial relationships that could be construed as a potential conflict of interest.
Publisher’s note
All claims expressed in this article are solely those of the authors and do not necessarily represent those of their affiliated organizations, or those of the publisher, the editors and the reviewers. Any product that may be evaluated in this article, or claim that may be made by its manufacturer, is not guaranteed or endorsed by the publisher.
References
Abazari, M., Ghaffari, A., Rashidzadeh, H., Badeleh, S. M., and Maleki, Y. (2022). A systematic review on classification, identification, and healing process of burn wound healing. Int J Low Extrem Wounds 21, 18–30. doi: 10.1177/1534734620924857
Abbas, O. L., Özatik, O., Gönen, Z. B., Öğüt, S., Özatik, F. Y., Salkın, H., et al. (2018). Comparative analysis of mesenchymal stem cells from bone marrow, adipose tissue, and dental pulp as sources of cell therapy for zone of stasis burns. J. Investig. Surg. 32, 477–490. doi: 10.1080/08941939.2018.1433254
Abd-Allah, S. H., El-Shal, A. S., Shalaby, S. M., Abd-Elbary, E., Mazen, N. F., and Abdel Kader, R. R. (2015). The role of placenta-derived mesenchymal stem cells in healing of induced full-thickness skin wound in a mouse model. IUBMB Life 67, 701–709. doi: 10.1002/iub.1427
Abdul Kareem, N., Aijaz, A., and Jeschke, M. G. (2021). Stem cell therapy for burns: story so far. Biologics 15, 379–397. doi: 10.2147/BTT.S259124
Abo-Elkheir, W., Hamza, F., Elmofty, A. M., Emam, A., Abdl-Moktader, M., Elsherefy, S., et al. (2017). Role of cord blood and bone marrow mesenchymal stem cells in recent deep burn: a case-control prospective study. Am. J. Stem Cells 6:23.
Abu-El-Rub, E., Khaswaneh, R. R., Almahasneh, F. A., Almazari, R., and Alzu’bi, A. (2024). Adipose tissue and bone marrow-derived mesenchymal stem cells are not really the same: investigating the differences in their immunomodulatory, migratory, and adhesive profile. Biochem. Genet. 5, 1–15. doi: 10.1007/s10528-024-10724-6
Afkhami, H., Mahmoudvand, G., Fakouri, A., Shadab, A., Mahjoor, M., and Komeili Movahhed, T. (2023). New insights in application of mesenchymal stem cells therapy in tumor microenvironment: pros and cons. Front. Cell Dev. Biol. 11:1255697. doi: 10.3389/fcell.2023.1255697
Aghayan, H. R., Hosseini, M. S., Gholami, M., Mohamadi-Jahani, F., Tayanloo-Beik, A., Alavi-Moghadam, S., et al. (2022). Mesenchymal stem cells’ seeded amniotic membrane as a tissue-engineered dressing for wound healing. Drug Deliv. Transl. Res. 12, 538–549. doi: 10.1007/s13346-021-00952-3
Ahmadi, A. R., Chicco, M., Huang, J., Qi, L., Burdick, J., Williams, G. M., et al. (2019). Stem cells in burn wound healing: A systematic review of the literature. Burns 45, 1014–1023. doi: 10.1016/j.burns.2018.10.017
Alahdal, M., Zhang, H., Huang, R., Sun, W., Deng, Z., Duan, L., et al. (2020). Potential efficacy of dendritic cell immunomodulation in the treatment of osteoarthritis. Rheumatology 60, 507–517. doi: 10.1093/rheumatology/keaa745
Al-Anazi, K. A., Al-Anazi, W. K., and Al-Jasser, A. M. (2020). The rising role of mesenchymal stem cells in the treatment of various infectious complications. Update on mesenchymal and induced pluripotent. Stem Cells, 4–6. doi: 10.5772/intechopen.91475
Al-Maliki, H. K., Al-Luaibi, Y., and Chiad, A. (2022) Molecular screening the emergence of vancomycin and gentamycin resistant Enterococcus species in burn'patients in Basrah government, Iraq. Int. J. Health Sci. 6, 500–516. doi: 10.53730/ijhs.v6n7.11067
Álvarez-Viejo, M. (2020). Mesenchymal stem cells from different sources and their derived exosomes: a pre-clinical perspective. World J. Stem Cells 12, 100–109. doi: 10.4252/wjsc.v12.i2.100
Alvites, R., Branquinho, M., Sousa, A. C., Lopes, B., Sousa, P., and Maurício, A. C. (2022). Mesenchymal stem/stromal cells and their paracrine activity—immunomodulation mechanisms and how to influence the therapeutic potential. Pharmaceutics 14:381. doi: 10.3390/pharmaceutics14020381
Amini-Nik, S., Dolp, R., Eylert, G., Datu, A.-K., Parousis, A., Blakeley, C., et al. (2018). Stem cells derived from burned skin-the future of burn care. EBioMedicine 37, 509–520. doi: 10.1016/j.ebiom.2018.10.014
An, Y., Lin, S., Tan, X., Zhu, S., Nie, F., Zhen, Y., et al. (2021). Exosomes from adipose-derived stem cells and application to skin wound healing. Cell Prolif. 54:e12993. doi: 10.1111/cpr.12993
Andalib, E., Kashfi, M., Mahmoudvand, G., Rezaei, E., Mahjoor, M., Torki, A., et al. (2023). Application of hypoxia-mesenchymal stem cells in treatment of anaerobic bacterial wound infection: wound healing and infection recovery. Front. Virol. 14:1251956. doi: 10.3389/fmicb.2023.1251956
Anudeep, T. C., Jeyaraman, M., Muthu, S., Rajendran, R. L., Gangadaran, P., Mishra, P. C., et al. (2022). Advancing regenerative cellular therapies in non-scarring alopecia. Pharmaceutics 14:612. doi: 10.3390/pharmaceutics14030612
Aryan, A., Bayat, M., Bonakdar, S., Taheri, S., Haghparast, N., Bagheri, M., et al. (2019). Human bone marrow mesenchymal stem cell conditioned medium promotes wound healing in deep second-degree burns in male rats. Cells Tissues Organs 206, 317–329. doi: 10.1159/000501651
Atluri, S., Manchikanti, L., and Hirsch, J. A. (2020). Expanded umbilical cord mesenchymal stem cells (UC-MSCs) as a therapeutic strategy in managing critically ill COVID-19 patients: the case for compassionate use. Pain Physician 23, E71–E83. doi: 10.36076/ppj.2020/23/E71
Ayavoo, T., Murugesan, K., and Gnanasekaran, A. (2021). Roles and mechanisms of stem cell in wound healing. Stem Cell Investig. 8:8. doi: 10.21037/sci-2020-027
Azzopardi, E. A., Boyce, D. E., Thomas, D. W., and Dickson, W. A. (2013a). Colistin in burn intensive care: back to the future? Burns 39, 7–15. doi: 10.1016/j.burns.2012.07.015
Azzopardi, E. A., Ferguson, E. L., and Thomas, D. W. (2013b). The enhanced permeability retention effect: a new paradigm for drug targeting in infection. J. Antimicrob. Chemother. 68, 257–274. doi: 10.1093/jac/dks379
Bai, Q., Zheng, C., Chen, W., Sun, N., Gao, Q., Liu, J., et al. (2022). Current challenges and future applications of antibacterial nanomaterials and chitosan hydrogel in burn wound healing. Mater. Adv. 3, 6707–6727. doi: 10.1039/D2MA00695B
Berebichez-Fridman, R., and Montero-Olvera, P. R. (2018). Sources and clinical applications of mesenchymal stem cells: state-of-the-art review. Sultan Qaboos Univ. Med. J. 18:e264. doi: 10.18295/squmj.2018.18.03.002
Bernabé-García, Á., Liarte, S., Rodríguez-Valiente, M., and Nicolás, F. J. (2021). Chronic wound healing by amniotic membrane: TGF-β and EGF signaling modulation in re-epithelialization. Front. Bioeng. Biotechnol. 9:689328. doi: 10.3389/fbioe.2021.689328
Bey, E., Prat, M., Duhamel, P., Benderitter, M., Brachet, M., Trompier, F., et al. (2010). Emerging therapy for improving wound repair of severe radiation burns using local bone marrow-derived stem cell administrations. Wound Repair Regen. 18, 50–58. doi: 10.1111/j.1524-475X.2009.00562.x
Bhujel, B., Oh, S.-H., Kim, C.-M., Yoon, Y.-J., Kim, Y.-J., Chung, H.-S., et al. (2023). Mesenchymal stem cells and exosomes: A novel therapeutic approach for corneal diseases. Int. J. Mol. Sci. 24:10917. doi: 10.3390/ijms241310917
Branski, L. K., Al-Mousawi, A., Rivero, H., Jeschke, M. G., Sanford, A. P., and Herndon, D. N. (2009). Emerging infections in burns. Surg. Infect. 10, 389–397. doi: 10.1089/sur.2009.024
Brennan, M. A., Renaud, A., Guilloton, F., Mebarki, M., Trichet, V., Sensebé, L., et al. (2017). Inferior in vivo osteogenesis and superior angiogeneis of human adipose-derived stem cells compared with bone marrow-derived stem cells cultured in xeno-free conditions. Stem Cells Transl. Med. 6, 2160–2172. doi: 10.1002/sctm.17-0133
Chai, Q., Jiao, Y., and Yu, X. (2017). Hydrogels for biomedical applications: their characteristics and the mechanisms behind them. Gels 3:6. doi: 10.3390/gels3010006
Cheng, J. Z., Farrokhi, A., Ghahary, A., and Jalili, R. B. (2018). Therapeutic use of stem cells in treatment of burn injuries. J. Burn Care Res. 39, 175–182. doi: 10.1097/BCR.0000000000000571
Clover, A. J., Kumar, A. H., Isakson, M., Whelan, D., Stocca, A., Gleeson, B. M., et al. (2015). Allogeneic mesenchymal stem cells, but not culture modified monocytes, improve burn wound healing. Burns 41, 548–557. doi: 10.1016/j.burns.2014.08.009
Corsano, B., Sacchini, D., Šuleková, M., Minacori, R., Refolo, P., and Spagnolo, A. G. (2015). Allogeneic versus autologous: ethical issues in umbilical cord blood use. Jahr Eur. J. Bioeth. 6, 67–86.
Czerwiec, K., Zawrzykraj, M., Deptuła, M., Skoniecka, A., Tymińska, A., Zieliński, J., et al. (2023). Adipose-derived mesenchymal stromal cells in basic research and clinical applications. Int. J. Mol. Sci. 24:3888. doi: 10.3390/ijms24043888
Dabrowska, S., Andrzejewska, A., Janowski, M., and Lukomska, B. (2021). Immunomodulatory and regenerative effects of mesenchymal stem cells and extracellular vesicles: therapeutic outlook for inflammatory and degenerative diseases. Front. Immunol. 11:591065. doi: 10.3389/fimmu.2020.591065
Das, P., Pal, D., Roy, S., Chaudhuri, S., Kesh, S. S., Basak, P., et al. (2024). Unveiling advanced strategies for therapeutic stem cell interventions in severe burn injuries: a comprehensive review. Int. J. Surg. 110, 6382–6401. doi: 10.1097/JS9.0000000000001812
de Witte, S. F. H., Franquesa, M., Baan, C. C., and Hoogduijn, M. J. (2016). Toward development of iMesenchymal stem cells for immunomodulatory therapy. Front. Immunol. 6:648. doi: 10.3389/fimmu.2015.00648
Díaz-Prado, S., Muiños-López, E., Hermida-Gómez, T., Cicione, C., Rendal-Vázquez, M. E., Fuentes-Boquete, I., et al. (2011). Human amniotic membrane as an alternative source of stem cells for regenerative medicine. Differentiation 81, 162–171. doi: 10.1016/j.diff.2011.01.005
Diederen, B. M., Wardle, C. L., Krijnen, P., Tuinebreijer, W. E., and Breederveld, R. S. (2015). Epidemiology of clinically relevant bacterial pathogens in a burn center in the Netherlands between 2005 and 2011. J. Burn Care Res. 36, 446–453. doi: 10.1097/BCR.0000000000000144
Ding, X., Tang, Q., Xu, Z., Xu, Y., Zhang, H., Zheng, D., et al. (2022). Challenges and innovations in treating chronic and acute wound infections: from basic science to clinical practice. Burns Trauma 10:tkac014. doi: 10.1093/burnst/tkac014
Dini, T., Nugraha, Y., Revina, R., and Karina, K. (2022). Safety and efficacy of mesenchymal stem cells in burn therapy: systematic review. Mol. Cell. Biomed. Sci. 6, 104–116. doi: 10.21705/mcbs.v6i3.252
El Ayadi, A., Jay, J. W., and Prasai, A. (2020). Current approaches targeting the wound healing phases to attenuate fibrosis and scarring. Int. J. Mol. Sci. 21:1105. doi: 10.3390/ijms21031105
El Hamzaoui, N., Barguigua, A., Larouz, S., and Maouloua, M. (2020). Epidemiology of burn wound bacterial infections at a Meknes hospital, Morocco. New Microbes New Infect. 38:100764. doi: 10.1016/j.nmni.2020.100764
El-Sayed, M. E., Atwa, A., Sofy, A. R., Helmy, Y. A., Amer, K., Seadawy, M. G., et al. (2024). Mesenchymal stem cell transplantation in burn wound healing: uncovering the mechanisms of local regeneration and tissue repair. Histochem. Cell Biol. 161, 165–181. doi: 10.1007/s00418-023-02244-y
Fairbairn, N., Randolph, M., and Redmond, R. (2014). The clinical applications of human amnion in plastic surgery. J. Plast. Reconstr. Aesthet. Surg. 67, 662–675. doi: 10.1016/j.bjps.2014.01.031
Fakouri, A., Razavi, Z.-S., Mohammed, A. T., Hussein, A. H. A., Afkhami, H., Hooshiar, M. H. J. B., et al. (2024). Applications of mesenchymal stem cell-exosome components in wound infection healing: new insights. Burns Trauma 12:tkae021. doi: 10.1093/burnst/tkae021
Formigli, L., Paternostro, F., Tani, A., Mirabella, C., Quattrini Li, A., Nosi, D., et al. (2015). MSCs seeded on bioengineered scaffolds improve skin wound healing in rats. Wound Repair Regen. 23, 115–123. doi: 10.1111/wrr.12251
Francis, E., Kearney, L., and Clover, J. (2019). The effects of stem cells on burn wounds: a review. Int. J. Burns Trauma 9, 1–12.
Gao, Q., Wang, L., Wang, S., Huang, B., Jing, Y., and Su, J. (2022). Bone marrow mesenchymal stromal cells: identification, classification, and differentiation. Front. Cell Dev. Biol. 9:787118. doi: 10.3389/fcell.2021.787118
Gardien, K. L., Middelkoop, E., and Ulrich, M. M. (2014). Progress towards cell-based burn wound treatments. Regen. Med. 9, 201–218. doi: 10.2217/rme.13.97
Gherghel, R., Macovei, L. A., Burlui, M.-A., Cardoneanu, A., Rezus, I.-I., Mihai, I. R., et al. (2023). Osteoarthritis—the role of mesenchymal stem cells in cartilage regeneration. Appl. Sci. 13:10617. doi: 10.3390/app131910617
Golchin, A., Farahany, T. Z., Khojasteh, A., Soleimanifar, F., and Ardeshirylajimi, A. (2019). The clinical trials of mesenchymal stem cell therapy in skin diseases: an update and concise review. Curr. Stem Cell Res. Ther. 14, 22–33. doi: 10.2174/1574888X13666180913123424
Gomes, J. Á. P., Monteiro, B. G., Melo, G. B., Smith, R. L., da Silva, M. C. P., Lizier, N. F., et al. (2010). Corneal reconstruction with tissue-engineered cell sheets composed of human immature dental pulp stem cells. Invest. Ophthalmol. Vis. Sci. 51, 1408–1414. doi: 10.1167/iovs.09-4029
Goncharova, Y., Sudoma, I., Pylyp, L., and Kremenska, Y. (2019). Application of autologous adipose-derived stem cells for thin endometrium treatment in patients with failed ART programs. J. Stem Cell Ther. Transplant. 3, 001–008. doi: 10.29328/journal.jsctt.1001013
Guillamat-Prats, R. (2021). The role of MSC in wound healing, scarring and regeneration. Cells 10:1729. doi: 10.3390/cells10071729
Guo, L., Xu, H., and Yue, Z. (2022). Antibiotic resistance pattern of Pseudomonas aeruginosa wound isolates among Chinese burn patients: A systematic review and meta analysis. Asian Pac J Trop Med 15, 17–25. doi: 10.4103/1995-7645.335703
Han, A. R., Shin, H. R., Kweon, J., Lee, S. B., Lee, S. E., Kim, E.-Y., et al. (2024). Highly efficient genome editing via CRISPR-Cas9 ribonucleoprotein (RNP) delivery in mesenchymal stem cells. BMB Rep. 57, 60–65. doi: 10.5483/BMBRep.2023-0113
Harrell, C. R., Gazdic, M., Fellabaum, C., Jovicic, N., Djonov, V., Arsenijevic, N., et al. (2019). Therapeutic potential of amniotic fluid derived mesenchymal stem cells based on their differentiation capacity and immunomodulatory properties. Curr. Stem Cell Res. Ther. 14, 327–336. doi: 10.2174/1574888X14666190222201749
Hashemi, S. S., Pourfath, M. R., Derakhshanfar, A., Behzad-Behbahani, A., and Moayedi, J. (2020). The role of labeled cell therapy with and without scaffold in early excision burn wounds in a rat animal model. Iran. J. Basic Med. Sci. 23, 673–679. doi: 10.22038/ijbms.2020.34324.8156
Hassanshahi, A., Hassanshahi, M., Khabbazi, S., Hosseini-Khah, Z., Peymanfar, Y., Ghalamkari, S., et al. (2019). Adipose-derived stem cells for wound healing. J. Cell. Physiol. 234, 7903–7914. doi: 10.1002/jcp.27922
Hu, M. S., Borrelli, M. R., Lorenz, H. P., Longaker, M. T., and Wan, D. C. (2018). Mesenchymal stromal cells and cutaneous wound healing: a comprehensive review of the background, role, and therapeutic potential. Stem Cells Int. 2018:6901983. doi: 10.1155/2018/6901983
Hu, Z., Luo, Y., Ni, R., Hu, Y., Yang, F., Du, T., et al. (2023). Biological importance of human amniotic membrane in tissue engineering and regenerative medicine. Mater. Today Bio 22:100790. doi: 10.1016/j.mtbio.2023.100790
Hu, J.-C., Zheng, C.-X., Sui, B.-D., Liu, W.-J., and Jin, Y. (2022). Mesenchymal stem cell-derived exosomes: A novel and potential remedy for cutaneous wound healing and regeneration. World J. Stem Cells 14, 318–329. doi: 10.4252/wjsc.v14.i5.318
Huang, Y., Wu, Q., and Tam, P. K. H. (2022). Immunomodulatory mechanisms of mesenchymal stem cells and their potential clinical applications. Int. J. Mol. Sci. 23:10023. doi: 10.3390/ijms231710023
Huynh, P. D., Tran, Q. X., Nguyen, V. Q., and Vu, N. B. (2022). Mesenchymal stem cell therapy for wound healing: An update to 2022. Biomed. Res. Ther. 9, 5437–5449. doi: 10.15419/bmrat.v9i12.782
Hwang, S., Sung, D. K., Kim, Y. E., Yang, M., Ahn, S. Y., Sung, S. I., et al. (2023). Mesenchymal stromal cells primed by toll-like receptors 3 and 4 enhanced anti-inflammatory effects against LPS-induced macrophages via extracellular vesicles. Int. J. Mol. Sci. 24:16264. doi: 10.3390/ijms242216264
Ichi, E., Triwahyuni, I. E., and Sari, D. S. (2023). Accelerating wound healing of traumatic ulcer with topical application of dental pulp mesenchymal stem cell Secretome and Robusta green coffee bean extract combination in vivo. Pesquisa Brasileira em Odontopediatria e Clínica Integrada 23:e210116. doi: 10.1590/pboci.2023.018
Irfan, F., Jameel, F., Khan, I., Aslam, R., Faizi, S., and Salim, A. (2022). Role of quercetin and rutin in enhancing the therapeutic potential of mesenchymal stem cells for cold induced burn wound. Regen. Ther. 21, 225–238. doi: 10.1016/j.reth.2022.07.011
Jahromi, M. A. M., Zangabad, P. S., Basri, S. M. M., Zangabad, K. S., Ghamarypour, A., Aref, A. R., et al. (2018). Nanomedicine and advanced technologies for burns: preventing infection and facilitating wound healing. Adv. Drug Deliv. Rev. 123, 33–64. doi: 10.1016/j.addr.2017.08.001
Jeschke, M. G., van Baar, M. E., Choudhry, M. A., Chung, K. K., Gibran, N. S., and Logsetty, S. (2020). Burn injury. Nat. Rev. Dis. Prim. 6:11. doi: 10.1038/s41572-020-0145-5
Jiang, W., and Xu, J. (2020). Immune modulation by mesenchymal stem cells. Cell Prolif. 53:e12712. doi: 10.1111/cpr.12712
Jo, H., Brito, S., Kwak, B. M., Park, S., Lee, M.-G., and Bin, B.-H. (2021). Applications of mesenchymal stem cells in skin regeneration and rejuvenation. Int. J. Mol. Sci. 22:2410. doi: 10.3390/ijms22052410
Johnson, B., Stevenson, A., Barrett, L., Fear, M., Wood, F., and Linden, M. (2022). Platelets after burn injury–hemostasis and beyond. Platelets 33, 655–665. doi: 10.1080/09537104.2021.1981849
Katiyar, S., Singh, D., Kumari, S., Srivastava, P., and Mishra, A. (2022). Novel strategies for designing regenerative skin products for accelerated wound healing. 3 Biotech 12:316. doi: 10.1007/s13205-022-03331-y
Khayambashi, P., Iyer, J., Pillai, S., Upadhyay, A., Zhang, Y., and Tran, S. D. (2021). Hydrogel encapsulation of mesenchymal stem cells and their derived exosomes for tissue engineering. Int. J. Mol. Sci. 22:684. doi: 10.3390/ijms22020684
Kim, E. Y., Lee, K.-B., and Kim, M. K. (2014). The potential of mesenchymal stem cells derived from amniotic membrane and amniotic fluid for neuronal regenerative therapy. BMB Rep. 47, 135–140. doi: 10.5483/BMBRep.2014.47.3.289
Kirby, G. T., Mills, S. J., Cowin, A. J., and Smith, L. E. (2015). Stem cells for cutaneous wound healing. Biomed. Res. Int. 2015, 1–11. doi: 10.1155/2015/285869
Kosaric, N., Srifa, W., Gurtner, G. C., and Porteus, M. H. (2017). Human mesenchymal stromal cells engineered to overexpress PDGF-B using CRISPR/Cas9/rAAV6-based tools improve wound healing. Plast. Reconstr. Surg. 5:74. doi: 10.1097/01.GOX.0000516619.11665.84
Kumari, M., and Nanda, D. K. (2022). Potential of curcumin nanoemulsion as antimicrobial and wound healing agent in burn wound infection. Burns 49, 1003–1016. doi: 10.1016/j.burns.2022.10.008
Lasocka, I., Jastrzębska, E., Szulc-Dąbrowska, L., Skibniewski, M., Pasternak, I., Kalbacova, M. H., et al. (2019). The effects of graphene and mesenchymal stem cells in cutaneous wound healing and their putative action mechanism. Int. J. Nanomedicine 14, 2281–2299. doi: 10.2147/IJN.S190928
Lataillade, J., Doucet, C., Bey, E., Carsin, H., Huet, C., Clairand, I., et al. (2007). New approach to radiation burn treatment by dosimetry-guided surgery combined with autologous mesenchymal stem cell therapy. Regen. Med. 2, 785–794. doi: 10.2217/17460751.2.5.785
Li, S., Li, H., Zhangdi, H., Xu, R., Zhang, X., Liu, J., et al. (2022). Hair follicle-MSC-derived small extracellular vesicles as a novel remedy for acute pancreatitis. J. Control. Release 352, 1104–1115. doi: 10.1016/j.jconrel.2022.11.029
Li, X., Lin, H., Yu, Y., Lu, Y., He, B., Liu, M., et al. (2024). In situ rapid-formation sprayable hydrogels for challenging tissue injury management. Adv. Mater. 36:e2400310. doi: 10.1002/adma.202400310
Li, Q., Wang, D., Jiang, Z., Li, R., Xue, T., Lin, C., et al. (2022). Advances of hydrogel combined with stem cells in promoting chronic wound healing. Front. Chem. 10:1038839. doi: 10.3389/fchem.2022.1038839
Li, M., Zhao, Y., Hao, H., Han, W., and Fu, X. (2015). Mesenchymal stem cell–based therapy for nonhealing wounds: today and tomorrow. Wound Repair Regen. 23, 465–482. doi: 10.1111/wrr.12304
Liu, J., Liu, Q., and Chen, X. (2020). The immunomodulatory effects of mesenchymal stem cells on regulatory B cells. Front. Immunol. 11:1843. doi: 10.3389/fimmu.2020.01843
Liu, J., Ren, J., Su, L., Cheng, S., Zhou, J., Ye, X., et al. (2018). Human adipose tissue-derived stem cells inhibit the activity of keloid fibroblasts and fibrosis in a keloid model by paracrine signaling. Burns 44, 370–385. doi: 10.1016/j.burns.2017.08.017
Liu, C., Wang, C., Yang, F., Lu, Y., Du, P., Hu, K., et al. (2022). The conditioned medium from mesenchymal stromal cells pretreated with proinflammatory cytokines promote fibroblasts migration and activation. PLoS One 17:e0265049. doi: 10.1371/journal.pone.0265049
Liu, X., Wei, Q., Lu, L., Cui, S., Ma, K., Zhang, W., et al. (2023). Immunomodulatory potential of mesenchymal stem cell-derived extracellular vesicles: targeting immune cells. Front. Immunol. 14:1094685. doi: 10.3389/fimmu.2023.1094685
Liu, D., Xu, Q., Meng, X., Liu, X., and Liu, J. (2024). Status of research on the development and regeneration of hair follicles. Int. J. Med. Sci. 21, 80–94. doi: 10.7150/ijms.88508
Liu, P., Yao, L., Hu, X., Wang, Z., Xiong, Z., and Jiang, Y. (2023). Recent advances in the immunomodulation mechanism of mesenchymal stem cell therapy in liver diseases. J. Gastroenterol. Hepatol. 38, 1099–1106. doi: 10.1111/jgh.16247
Luan, Y., Kong, X., and Feng, Y. (2021). Mesenchymal stem cells therapy for acute liver failure: recent advances and future perspectives. Liver Res. 5, 53–61. doi: 10.1016/j.livres.2021.03.003
Luck, M. E., Herrnreiter, C. J., and Choudhry, M. A. (2021). Gut microbial changes and their contribution to post-burn pathology. Shock 56, 329–344. doi: 10.1097/SHK.0000000000001736
Lukomskyj, A. O., Rao, N., Yan, L., Pye, J. S., Li, H., Wang, B., et al. (2022). Stem cell-based tissue engineering for the treatment of burn wounds: A systematic review of preclinical studies. Stem Cell Rev. Rep. 18, 1926–1955. doi: 10.1007/s12015-022-10341-z
Ma, H., Siu, W.-S., and Leung, P.-C. (2023). The potential of MSC-based cell-free therapy in wound healing—A thorough literature review. Int. J. Mol. Sci. 24:9356. doi: 10.3390/ijms24119356
Magne, B., Lataillade, J.-J., and Trouillas, M. (2018). Mesenchymal stromal cell preconditioning: the next step toward a customized treatment for severe burn. Stem Cells Dev. 27, 1385–1405. doi: 10.1089/scd.2018.0094
Mahjoor, M., Afkhami, H., Najafi, M., Nasr, A., and Khorrami, S. J. (2023a). The role of microRNA-30c in targeting interleukin 6, as an inflammatory cytokine, in the mesenchymal stem cell: a therapeutic approach in colorectal cancer. J. Cancer Res. Clin. Oncol. 149, 3149–3160. doi: 10.1007/s00432-022-04123-w
Mahjoor, M., Fakouri, A., Farokhi, S., Nazari, H., Afkhami, H., and Heidari, F. (2023b). Regenerative potential of mesenchymal stromal cells in wound healing: unveiling the influence of normoxic and hypoxic environments. Front. Cell Dev. Biol. 11:11. doi: 10.3389/fcell.2023.1245872
Mahmoudian-Sani, M. R., Rafeei, F., Amini, R., and Saidijam, M. (2018). The effect of mesenchymal stem cells combined with platelet-rich plasma on skin wound healing. J. Cosmet. Dermatol. 17, 650–659. doi: 10.1111/jocd.12512
Maitz, J., Merlino, J., Rizzo, S., McKew, G., and Maitz, P. (2023). Burn wound infections microbiome and novel approaches using therapeutic microorganisms in burn wound infection control. Adv. Drug Deliv. Rev. 196:114769. doi: 10.1016/j.addr.2023.114769
Makhoul, G., Chiu, R. C., and Cecere, R. (2013). Placental mesenchymal stem cells: a unique source for cellular cardiomyoplasty. Ann. Thorac. Surg. 95, 1827–1833. doi: 10.1016/j.athoracsur.2012.11.053
Malek, A., and Bersinger, N. A. (2011). Human placental stem cells: biomedical potential and clinical relevance. J. Stem Cells 6, 75–92.
Mankuzhy, P. D., Ramesh, S. T., Thirupathi, Y., Mohandas, P. S., Chandra, V., and Sharma, T. G. (2021). The preclinical and clinical implications of fetal adnexa derived mesenchymal stromal cells in wound healing therapy. Wound Repair Regen. 29, 347–369. doi: 10.1111/wrr.12911
Mansour, R. N., Hasanzadeh, E., Abasi, M., Gholipourmalekabadi, M., Mellati, A., and Enderami, S. E. (2023). The effect of fetal bovine acellular dermal matrix seeded with Wharton’s jelly mesenchymal stem cells for healing full-thickness skin wounds. Genes 14:909. doi: 10.3390/genes14040909
Maranda, L., Rodriguez-Menocal, L., and Badiavas, E. V. (2017). Role of mesenchymal stem cells in dermal repair in burns and diabetic wounds. Curr. Stem Cell Res. Ther. 12, 61–70. doi: 10.2174/1574888X11666160714115926
Margiana, R., Markov, A., Zekiy, A. O., Hamza, M. U., Al-Dabbagh, K. A., Al-Zubaidi, S. H., et al. (2022). Clinical application of mesenchymal stem cell in regenerative medicine: a narrative review. Stem Cell Res Ther 13:366. doi: 10.1186/s13287-022-03054-0
Matsuzaka, Y., and Yashiro, R. J. P. (2024). Current strategies and therapeutic applications of mesenchymal stem cell-based drug delivery. Pharmaceuticals 17:707. doi: 10.3390/ph17060707
Maxson, S., Lopez, E. A., Yoo, D., Danilkovitch-Miagkova, A., and LeRoux, M. A. (2012). Concise review: role of mesenchymal stem cells in wound repair. Stem Cells Transl. Med. 1, 142–149. doi: 10.5966/sctm.2011-0018
Mazini, L., Rochette, L., Amine, M., and Malka, G. (2019). Regenerative capacity of adipose derived stem cells (ADSCs), comparison with mesenchymal stem cells (MSCs). Int. J. Mol. Sci. 20:2523. doi: 10.3390/ijms20102523
Miao, J., Ren, Z., Zhong, Z., Yan, L., Xia, X., Wang, J., et al. (2021). Mesenchymal stem cells: potential therapeutic prospect of paracrine pathways in neonatal infection. J. Interf. Cytokine Res. 41, 365–374. doi: 10.1089/jir.2021.0094
Miatmoko, A., Hariawan, B. S., Cahyani, D. M., Dewangga, S. S., Handoko, K. K., Purwati,, et al. (2023). Prospective use of amniotic mesenchymal stem cell metabolite products for tissue regeneration. J. Biol. Eng. 17:11. doi: 10.1186/s13036-023-00331-1
Min-hong, J. (2020). Human amniotic fluid-derived stem cell patch affects the healing process of burn wound: Graduate school. Seoul, South Korea: Yonsei University.
Miricescu, D., Badoiu, S. C., Stanescu-Spinu, I.-I., Totan, A. R., Stefani, C., and Greabu, M. (2021). Growth factors, reactive oxygen species, and metformin—promoters of the wound healing process in burns? Int. J. Mol. Sci. 22:9512. doi: 10.3390/ijms22179512
Mirshekar, M., Afkhami, H., Razavi, S., Jazi, F. M., Darban-Sarokhalil, D., Ohadi, E., et al. (2023). Potential antibacterial activity and healing effect of topical administration of bone marrow and adipose mesenchymal stem cells encapsulated in collagen-fibrin hydrogel scaffold on full-thickness burn wound infection caused by Pseudomonas aeruginosa. Burns 49, 1944–1957. doi: 10.1016/j.burns.2023.01.005
Mistriotis, P., and Andreadis, S. T. (2013). Hair follicle: a novel source of multipotent stem cells for tissue engineering and regenerative medicine. Tissue Eng. Part B Rev. 19, 265–278. doi: 10.1089/ten.teb.2012.0422
Mitrossili, M., Sarris, M., and Nikolados, Y. (2015). “Ethical and legal issues in cord blood stem cells and biobanking” in Cord Blood Stem Cells and Regenerative Medicine. eds. C. Stavropoulos-Giokas, D. Charron, and C. Navarrete (United States: Elsevier), 313–324.
Msheik, L., Jaber, J., Taher, B., Ghauch, J., and Hamdar, H. (2023). Infections in burn patients: literature review. Open J. Clin. Med. Images 3:1124.
Najar, M., Melki, R., Khalife, F., Lagneaux, L., Bouhtit, F., Moussa Agha, D., et al. (2022). Therapeutic mesenchymal stem/stromal cells: value, challenges and optimization. Front. Cell. Dev. Biol. 9:716853. doi: 10.3389/fcell.2021.716853
Nazempour, M., Mehrabani, D., Mehdinavaz-Aghdam, R., Hashemi, S. S., Derakhshanfar, A., Zare, S., et al. (2020). The effect of allogenic human Wharton's jelly stem cells seeded onto acellular dermal matrix in healing of rat burn wounds. J. Cosmet. Dermatol. 19, 995–1001. doi: 10.1111/jocd.13109
Nejad, A. R., Hamidieh, A. A., Amirkhani, M. A., and Sisakht, M. M. (2021). Update review on five top clinical applications of human amniotic membrane in regenerative medicine. Placenta 103, 104–119. doi: 10.1016/j.placenta.2020.10.026
Nourian Dehkordi, A., Mirahmadi Babaheydari, F., Chehelgerdi, M., and Raeisi Dehkordi, S. (2019). Skin tissue engineering: wound healing based on stem-cell-based therapeutic strategies. Stem Cell Res Ther 10, 1–20. doi: 10.1186/s13287-019-1212-2
Nyman, E., Lindholm, E., Rakar, J., Junker, J. P., and Kratz, G. (2022). Effects of amniotic fluid on human keratinocyte gene expression: implications for wound healing. Exp. Dermatol. 31, 764–774. doi: 10.1111/exd.14515
Olteanu, G., Neacșu, S. M., Joița, F. A., Musuc, A. M., Lupu, E. C., Ioniță-Mîndrican, C.-B., et al. (2024). Advancements in regenerative hydrogels in skin wound treatment: A comprehensive review. Int. J. Mol. Sci. 25:3849. doi: 10.3390/ijms25073849
Opriessnig, E., Luze, H., Smolle, C., Draschl, A., Zrim, R., Giretzlehner, M., et al. (2023). Epidemiology of burn injury and the ideal dressing in global burn care–regional differences explored. Burns 49, 1–14. doi: 10.1016/j.burns.2022.06.018
Ou, K.-L., Kuo, Y.-W., Wu, C.-Y., Huang, B.-H., Pai, F.-T., Chou, H.-H., et al. (2020). The potential of a hair follicle mesenchymal stem cell-conditioned medium for wound healing and hair follicle regeneration. Appl. Sci. 10:2646. doi: 10.3390/app10082646
Owczarczyk-Saczonek, A., Krajewska-Włodarczyk, M., Kruszewska, A., Banasiak, Ł., Placek, W., Maksymowicz, W., et al. (2018). Therapeutic potential of stem cells in follicle regeneration. Stem Cells Int. 2018, 1–16. doi: 10.1155/2018/1049641
Ozpur, M. A., Guneren, E., Canter, H. I., Karaaltin, M. V., Ovali, E., Yogun, F. N., et al. (2016). Generation of skin tissue using adipose tissue-derived stem cells. Plast. Reconstr. Surg. 137, 134–143. doi: 10.1097/PRS.0000000000001927
Ozturk, S., and Karagoz, H. (2015). Experimental stem cell therapies on burn wound: do source, dose, timing and method matter? Burns 41, 1133–1139. doi: 10.1016/j.burns.2015.01.005
Peng, Y. Q., Deng, X. H., Xu, Z. B., Wu, Z. C., and Fu, Q. L. (2023). Mesenchymal stromal cells and their small extracellular vesicles in allergic diseases: from immunomodulation to therapy. Eur. J. Immunol. 53:e2149510. doi: 10.1002/eji.202149510
Pfister, P., Wendel-Garcia, P. D., Meneau, I., Vasella, M., Watson, J. A., Bühler, P., et al. (2023). Human amniotic membranes as an allogenic biological dressing for the treatment of burn wounds: protocol for a randomized-controlled study. Contemp. Clin. Trials Commun. 36:101209. doi: 10.1016/j.conctc.2023.101209
Phan, M. H., Pham-Thi, N. N., Nguyen, H. H., and Dinh, V. H. Growth characteristics and efficacy of umbilical cord lining mesenchymal stem cells in burn wound treatment. (2023). doi: 10.20944/preprints202306.2119.v1
Qin, X., He, J., Wang, X., Wang, J., Yang, R., and Chen, X. (2023). The functions and clinical application potential of exosomes derived from mesenchymal stem cells on wound repair: a review of recent research advances. Front. Immunol. 14:1256687. doi: 10.3389/fimmu.2023.1256687
Radmanesh, F., Mahmoudi, M., Yazdanpanah, E., Keyvani, V., Kia, N., Nikpoor, A. R., et al. (2020). The immunomodulatory effects of mesenchymal stromal cell-based therapy in human and animal models of systemic lupus erythematosus. IUBMB Life 72, 2366–2381. doi: 10.1002/iub.2387
Rahma, A., and Lane, M. E. (2022). Skin barrier function in infants: update and outlook. Pharmaceutics 14:433. doi: 10.3390/pharmaceutics14020433
Rahman, M. S., Islam, R., Rana, M. M., Spitzhorn, L.-S., Rahman, M. S., Adjaye, J., et al. (2019). Characterization of burn wound healing gel prepared from human amniotic membrane and Aloe vera extract. BMC Complement. Altern. Med. 19, 1–15. doi: 10.1186/s12906-019-2525-5
Rangatchew, F., Vester-Glowinski, P., Rasmussen, B. S., Haastrup, E., Munthe-Fog, L., Talman, M.-L., et al. (2021). Mesenchymal stem cell therapy of acute thermal burns: a systematic review of the effect on inflammation and wound healing. Burns 47, 270–294. doi: 10.1016/j.burns.2020.04.012
Revilla, G., Saputra, D., and Nurhasanah, D. (2023). Effect of human bone marrow mesenchymal stem cells on burn healing in granulation tissue formation in diabetic rats. Jurnal Kesehatan Andalas 11, 177–183. doi: 10.25077/jka.v11i3.2089
Riedl, J., Popp, C., Eide, C., Ebens, C., and Tolar, J. (2021). Mesenchymal stromal cells in wound healing applications: role of the secretome, targeted delivery and impact on recessive dystrophic epidermolysis bullosa treatment. Cytotherapy 23, 961–973. doi: 10.1016/j.jcyt.2021.06.004
Rodgers, K., and Jadhav, S. S. (2018). The application of mesenchymal stem cells to treat thermal and radiation burns. Adv. Drug Deliv. Rev. 123, 75–81. doi: 10.1016/j.addr.2017.10.003
Roubelakis, M. G., Trohatou, O., and Anagnou, N. P. (2012). Amniotic fluid and amniotic membrane stem cells: marker discovery. Stem Cells Int. 2012, 1–9. doi: 10.1155/2012/107836
Sarker, R. R., Tsunoi, Y., Haruyama, Y., Sato, S., and Nishidate, I. (2022). Depth distributions of bacteria for the Pseudomonas aeruginosa-infected burn wounds treated by methylene blue-mediated photodynamic therapy in rats: effects of additives to photosensitizer. J. Biomed. Opt. 27:018001. doi: 10.1117/1.JBO.27.1.018001
Sarsenova, M., Kim, Y., Raziyeva, K., Kazybay, B., Ogay, V., and Saparov, A. (2022). Recent advances to enhance the immunomodulatory potential of mesenchymal stem cells. Front. Immunol. 13:1010399. doi: 10.3389/fimmu.2022.1010399
Sasmal, P. K., and Ganguly, S. (2023). Polymer in hemostasis and follow-up wound healing. J. Appl. Polym. Sci. 140:e53559. doi: 10.1002/app.53559
Schulman, C., Candanedo, A., Rodriguez-Menocal, L., Guzman, W., McBride, J., Pizano, L., et al. (2018). 354 clinical trial of allogeneic mesenchymal stem cells in second degree burns: prelim results. J. Burn Care Res. 39:S147. doi: 10.1093/jbcr/iry006.276
Schulman, C. I., Namias, N., Pizano, L., Rodriguez-Menocal, L., Aickara, D., Guzman, W., et al. (2022). The effect of mesenchymal stem cells improves the healing of burn wounds: a phase 1 dose-escalation clinical trial. Scars Burns Heal. 8:20595131211070783. doi: 10.1177/20595131211070783
Senthilkumar, S., Venugopal, C., Parveen, S., Shobha, K., Rai, K. S., Kutty, B. M., et al. (2020). Remarkable migration propensity of dental pulp stem cells towards neurodegenerative milieu: An in vitro analysis. Neurotoxicology 81, 89–100. doi: 10.1016/j.neuro.2020.08.006
Seo, B. F., and Jung, S.-N. (2016). The immunomodulatory effects of mesenchymal stem cells in prevention or treatment of excessive scars. Stem Cells Int. 2016:6937976. doi: 10.1155/2016/6937976
Shojaei, F., Rahmati, S., and Banitalebi Dehkordi, M. (2019). A review on different methods to increase the efficiency of mesenchymal stem cell-based wound therapy. Wound Repair Regen. 27, 661–671. doi: 10.1111/wrr.12749
Singer, D. D., Singer, A. J., Gordon, C., and Brink, P. (2013). The effects of rat mesenchymal stem cells on injury progression in a rat model. Acad. Emerg. Med. 20, 398–402. doi: 10.1111/acem.12116
Sinha, Y., Nair, G. J., and Sharma, A. Immunomodulatory effects of mesenchymal stem cells on B and T cells. (2023). doi: 10.30574/wjbphs.2023.15.3.0365
Solanki, R., and Nagori, B. (2013). A review on microorganisms causing wound infections on skin. Asian J. Pharm. Technol. 3, 119–122.
Sorg, H., Tilkorn, D. J., Hager, S., Hauser, J., and Mirastschijski, U. (2017). Skin wound healing: an update on the current knowledge and concepts. Eur. Surg. Res. 58, 81–94. doi: 10.1159/000454919
Steffens, D., Leonardi, D., da Luz Soster, P. R., Lersch, M., Rosa, A., Crestani, T., et al. (2014). Development of a new nanofiber scaffold for use with stem cells in a third degree burn animal model. Burns 40, 1650–1660. doi: 10.1016/j.burns.2014.03.008
Su, Y., Zhang, T., Huang, T., and Gao, J. (2021). Current advances and challenges of mesenchymal stem cells-based drug delivery system and their improvements. Int. J. Pharm. 600:120477. doi: 10.1016/j.ijpharm.2021.120477
Su, L., Zheng, J., Wang, Y., Zhang, W., and Hu, D. (2019). Emerging progress on the mechanism and technology in wound repair. Biomed. Pharmacother. 117:109191. doi: 10.1016/j.biopha.2019.109191
Subhan, B. S., Kwong, J., Kuhn, J. F., Monas, A., Sharma, S., and Rabbani, P. S. (2021). Amniotic fluid-derived multipotent stromal cells drive diabetic wound healing through modulation of macrophages. J. Transl. Med. 19, 1–11. doi: 10.1186/s12967-020-02674-5
Sun, A., Hu, D., He, X., Ji, X., Li, T., Wei, X., et al. (2022). Mussel-inspired hydrogel with injectable self-healing and antibacterial properties promotes wound healing in burn wound infection. NPG Asia Mater. 14:86. doi: 10.1038/s41427-022-00434-z
Surowiecka, A., Chrapusta, A., Klimeczek-Chrapusta, M., Korzeniowski, T., Drukała, J., and Strużyna, J. (2022). Mesenchymal stem cells in burn wound management. Int. J. Mol. Sci. 23:15339. doi: 10.3390/ijms232315339
Tamama, K., and Kerpedjieva, S. S. (2012). Acceleration of wound healing by multiple growth factors and cytokines secreted from multipotential stromal cells/mesenchymal stem cells. Adv. Wound Care 1, 177–182. doi: 10.1089/wound.2011.0296
Teoh, P. L., Akhir, H. M., Ajak, W. A., and Hiew, V. V. (2023). Human mesenchymal stromal cells derived from perinatal tissues: sources, characteristics and isolation methods. Malays. J. Med. Sci. 30, 55–68. doi: 10.21315/mjms2023.30.2.5
Teshima, T. (2024). Heterogeneity of mesenchymal stem cells as a limiting factor in their clinical application to inflammatory bowel disease in dogs and cats. Vet. J. 304:106090. doi: 10.1016/j.tvjl.2024.106090
Tottoli, E. M., Dorati, R., Genta, I., Chiesa, E., Pisani, S., and Conti, B. (2020). Skin wound healing process and new emerging technologies for skin wound care and regeneration. Pharmaceutics 12:735. doi: 10.3390/pharmaceutics12080735
Turner, P. R., McConnell, M., Young, S. L., and Cabral, J. D. (2022). 3D living dressing improves healing and modulates immune response in a thermal injury model. Tissue Eng. Part C Methods 28, 431–439. doi: 10.1089/ten.tec.2022.0088
Ulivi, V., Tasso, R., Cancedda, R., and Descalzi, F. (2014). Mesenchymal stem cell paracrine activity is modulated by platelet lysate: induction of an inflammatory response and secretion of factors maintaining macrophages in a proinflammatory phenotype. Stem Cells Dev. 23, 1858–1869. doi: 10.1089/scd.2013.0567
Van Der Veen, V. C., Vlig, M., Van Milligen, F. J., De Vries, S. I., Middelkoop, E., and Ulrich, M. M. W. (2012). Stem Cells in Burn Eschar. Cell Transplant. 21, 933–942. doi: 10.3727/096368911X600993
Vathulya, M., Kapoor, A., Chattopadhyay, D., and Rao, N. (2022). Role of systemic antibiotic prophylaxis and burn dressings in preventing invasive burn infections–A systematic review. J. Med. Evid. 3, 28–41. doi: 10.4103/JME.JME_9_20
Vinaik, R., Barayan, D., Shahrokhi, S., and Jeschke, M. G. (2019). Management and prevention of drug resistant infections in burn patients. Expert Rev. Anti-Infect. Ther. 17, 607–619. doi: 10.1080/14787210.2019.1648208
Wang, Y., Beekman, J., Hew, J., Jackson, S., Issler-Fisher, A. C., Parungao, R., et al. (2018). Burn injury: challenges and advances in burn wound healing, infection, pain and scarring. Adv. Drug Deliv. Rev. 123, 3–17. doi: 10.1016/j.addr.2017.09.018
Wang, Y., Liu, J., Burrows, P. D., and Wang, J.-Y. (2020). B cell development and maturation. Adv. Exp. Med. Biol. 1254, 1–22. doi: 10.1007/978-981-15-3532-1_1
Wang, Y., Wu, J., Chen, J., Lu, C., Liang, J., Shan, Y., et al. (2023). Mesenchymal stem cells paracrine proteins from three-dimensional dynamic culture system promoted wound healing in third-degree burn models. Bioeng. Transl. Med. 8:e10569. doi: 10.1002/btm2.10569
Wang, M., Xu, X., Lei, X., Tan, J., and Xie, H. (2021). Mesenchymal stem cell-based therapy for burn wound healing. Burns Trauma 9:tkab002. doi: 10.1093/burnst/tkab002
Weiss, A. R. R., and Dahlke, M. H. (2019). Immunomodulation by mesenchymal stem cells (MSCs): mechanisms of action of living, apoptotic, and dead MSCs. Front. Immunol. 10:1191. doi: 10.3389/fimmu.2019.01191
Wu, S., Sun, S., Fu, W., Yang, Z., Yao, H., and Zhang, Z. (2024). The role and prospects of mesenchymal stem cells in skin repair and regeneration. Biomedicines 12:743. doi: 10.3390/biomedicines12040743
Xiong, Y., Xu, Y., Zhou, F., Hu, Y., Zhao, J., Liu, Z., et al. (2023). Bio-functional hydrogel with antibacterial and anti-inflammatory dual properties to combat with burn wound infection. Bioeng. Transl. Med. 8:e10373. doi: 10.1002/btm2.10373
Xiong, W., Zhang, R., Zhou, H., Liu, Y., Liang, M., Li, K., et al. (2023). Application of nanomedicine and mesenchymal stem cells in burn injuries for the elderly patients. Smart Mater. Med. 4, 78–90. doi: 10.1016/j.smaim.2022.08.001
Yang, G., Fan, X., Liu, Y., Jie, P., Mazhar, M., Liu, Y., et al. (2023). Immunomodulatory mechanisms and therapeutic potential of mesenchymal stem cells. Stem Cell Rev. Rep. 19, 1214–1231. doi: 10.1007/s12015-023-10539-9
Yang, C., Sun, J., Tian, Y., Li, H., Zhang, L., Yang, J., et al. (2021). Immunomodulatory effect of MSCs and MSCs-derived extracellular vesicles in systemic lupus erythematosus. Front. Immunol. 12:714832. doi: 10.3389/fimmu.2021.714832
Yang, H., Zhang, Y., Du, Z., Wu, T., and Yang, C. (2023). Hair follicle mesenchymal stem cell exosomal lncRNA H19 inhibited NLRP3 pyroptosis to promote diabetic mouse skin wound healing. Aging (Albany NY) 15, 791–809. doi: 10.18632/aging.204513
Yu, X., Liu, P., Li, Z., and Zhang, Z. (2023). Function and mechanism of mesenchymal stem cells in the healing of diabetic foot wounds. Front. Endocrinol. 14:1099310. doi: 10.3389/fendo.2023.1099310
Zaki, A. K. A., Almundarij, T. I., and Abo-Aziza, F. A. (2020). Comparative characterization and osteogenic/adipogenic differentiation of mesenchymal stem cells derived from male rat hair follicles and bone marrow. Cell Regen. 9, 1–14. doi: 10.1186/s13619-020-00051-7
Zawrzykraj, M., Deptuła, M., Kondej, K., Tymińska, A., and Pikuła, M. (2023). The effect of chemotherapy and radiotherapy on stem cells and wound healing. Current perspectives and challenges for cell-based therapies. Biomed. Pharmacother. 168:115781. doi: 10.1016/j.biopha.2023.115781
Zhang, G., Li, J., Wang, D., Lou, H., Zhang, C., and Liu, W. (2023). The mechanisms related to fibroblasts in burn surface. Skin Res. Technol. 29:e13431. doi: 10.1111/srt.13431
Zhang, P., Zou, B., Liou, Y.-C., and Huang, C. (2021). The pathogenesis and diagnosis of sepsis post burn injury. Burns Trauma 9:9. doi: 10.1093/burnst/tkaa047
Zhao, R., Zhang, X., Geng, Y., and Cao, Y. (2024). Advances in adipose-derived mesenchymal stem cells for burn wound healing. Int. J. Biol. Life Sci. 6, 1–7. doi: 10.54097/r91kv866
Keywords: mesenchymal stem cells (MSCs), burn injury, infection, stem cell-based therapy, wound healing
Citation: Aliniay-Sharafshadehi S, Yousefi MH, Ghodratie M, Kashfi M, Afkhami H and Ghoreyshiamiri SM (2024) Exploring the therapeutic potential of different sources of mesenchymal stem cells: a novel approach to combat burn wound infections. Front. Microbiol. 15:1495011. doi: 10.3389/fmicb.2024.1495011
Edited by:
Sunil D. Saroj, Symbiosis International University, IndiaReviewed by:
Shahper Nazeer Khan, University of Manitoba, CanadaRajashri Banerji, National Institutes of Health (NIH), United States
Copyright © 2024 Aliniay-Sharafshadehi, Yousefi, Ghodratie, Kashfi, Afkhami and Ghoreyshiamiri. This is an open-access article distributed under the terms of the Creative Commons Attribution License (CC BY). The use, distribution or reproduction in other forums is permitted, provided the original author(s) and the copyright owner(s) are credited and that the original publication in this journal is cited, in accordance with accepted academic practice. No use, distribution or reproduction is permitted which does not comply with these terms.
*Correspondence: Hamed Afkhami, aGFtZWRhZmtoYW1pNzBAZ21haWwuY29t; Seyed Mehdi Ghoreyshiamiri, TWVoZHkzMjc0OUB5YWhvby5jb20=
†These authors share first authorship