- 1School of Bioengineering, Zunyi Medical University, Zhuhai, China
- 2Center of Ocean Expedition, School of Atmospheric Science, Sun Yat-sen University, Zhuhai, China
- 3Teaching and Experimental Center, Guangdong Pharmaceutical University, Guangzhou, China
Marine fungal natural products (MFNPs) are a vital source of pharmaceuticals, primarily synthesized by relevant biosynthetic gene clusters (BGCs). However, many of these BGCs remain silent under standard laboratory culture conditions, delaying the development of novel drugs from MFNPs to some extent. This review highlights recent efforts in genome mining and biosynthetic pathways of bioactive natural products from marine fungi, focusing on methods such as bioinformatics analysis, gene knockout, and heterologous expression to identify relevant BGCs and elucidate the biosynthetic pathways and enzyme functions of MFNPs. The research efforts presented in this review provide essential insights for future gene-guided mining and biosynthetic pathway analysis in MFNPs.
1 Introduction
The ocean, often regarded as the cradle of life, hosts a rich diversity of species within unique ecological niches, fostering distinctive marine organisms that have generated a plethora of structurally novel and biologically active metabolites essential for new drug development. By the end of 2022, over 37,542 new marine natural products (MNPs) have been documented, predominantly comprising polyketides, terpenoids, alkaloids, and non-ribosomal peptides (Carroll et al., 2022; Carroll et al., 2019, Blunt et al., 2018, Carroll et al., 2020, Carroll et al., 2021, Carroll et al., 2024, Carroll et al., 2023). Up to 15 MNP-derived pharmaceuticals have been approved for market, including cytarabine (Cytosar-U), vidarabine (Vira-A), and eribulin mesylate (Halaven) from sponges, ziconotide (Prialt) from the venom of the pacific fish-hunting marine mollusk Conus magus, omega-3-acid ethyl esters (Lovaza and Vascepa) from fish body oils, trabectedin (Yondelis), plitidepsin (Aplidin), and lurbinectedin (Zepzelca) from sea squirts, and brentuximab vedotin (Adcetris), enfortumab vedotin (Padcev), polatuzumab vedotin (Polivy), belantamab mafodotin (Blenrep) from Dolabella auricularia and Symploca sp (Papon et al., 2022). Additionally, 33 MNP-derived pharmaceutical were undergoing clinical trials, with 5 in Phase III, 12 in Phase II, and 16 in Phase I stages (Patridge et al., 2016). These findings underscore the pivotal role of marine natural products in pharmaceutical development.
Marine microorganisms, thriving in unique oceanic environments, possess specialized metabolic and defensive mechanisms, thereby facilitating the production of structurally novel bioactive MNPs, making marine microorganisms as crucial sources for new MNPs. Approximately 11,362 new MNPs have been discovered from marine microorganisms, constituting 30% of all known marine natural products. Among these, 63.8% (7,233) originate from marine fungi, 28.9% (3,294) from bacteria, and 7.3% (835) from cyanobacteria (Figure 1; Blunt et al., 2018, Carroll et al., 2019, Banerjee et al., 2022, Voser et al., 2022, Carroll et al., 2022, Carroll et al., 2020, Carroll et al., 2023, Carroll et al., 2024). Thus, marine fungi emerge as the predominant source of marine microbial natural products.
MFNPs represent a significant source of marine microbial natural products. However, most remain underdeveloped, with only a small fraction documented (Costantini, 2020, Wei et al., 2021a). This is largely due to the unexplored BGCs responsible for MFNPs production, indicating that activating these BGCs holds substantial potential for advancing drug discovery. Therefore, understanding and activating these silent BGCs is essential for advancing novel drug development, as well as for exploring biosynthetic pathways and identifying associated enzymes to enhance MFNPs development and discover new pharmaceuticals. Currently, various advanced genome mining strategies, including heterologous expression in model fungi (Biggins et al., 2011, Yuan et al., 2022a), targeted inactivation of key genes (Wei et al., 2021a,Ning et al., 2024), one-strain-many-compounds (OSMAC) (Scherlach and Hertweck, 2006, Scherlach et al., 2010), chemical epigenetic modifications (Zheng et al., 2017, Fan et al., 2017), and overexpression of transcription factor (Zhang et al., 2018), are widely employed to activate silent BGCs. These efficient methodologies facilitate the targeted discovery of bioactive compounds, addressing the challenges of randomness and inefficiency traditionally associated with natural product exploration. This review consolidates progress in the genome mining and biosynthesis of polyketides, terpenes, alkaloids, and cyclic peptides from marine fungi, providing insights for the future BGC-guided discovery of MFNPs (Table 1).
2 Marine fungi-derived natural products
2.1 Polyketides
2.1.1 Flavoglaucin
Flavoglaucin (1), dihydroauroglaucin (2) and isodihydroauroglaucin (3), are derived from various marine fungi, including those derived from sea lilies Eurotium cristatum (Zhang P. P. et al., 2019), the sponge-derived fungus Eurotium repens (Smetanina et al., 2007), and the bonito-derived fungus Eurotium herbariorum (Miyake et al., 2009). Compounds 1, 2 and 3 have exhibited significant inhibitory properties on lipopolysaccharide (LPS)-activated NO production, with IC50 values of 0.46, 3.30, and 0.46 μM, respectively. Additionally, compound 1 has demonstrated cytotoxic effects on HepG2 (liver cancer) and HeLa (cervical cancer) human cancer cell lines, with IC50 values of 41.48 ± 3.52 and 33.60 ± 1.32 μM, respectively (Zhang P. P. et al., 2019).
The BGC fog, responsible for the production of 1 and its derivatives, was identified by Li group from Aspergillus ruber through bioinformatic analysis (Nies et al., 2020). It was discovered that fog shares over 40% homology with the BGCs of trichoxide and sordarial, both analogs of 1, suggesting that its potential to produce salicylaldehyde natural products. The co-expression of highly reducing polyketide synthase (HR-PKS) (fogA), SDR (fogBD), and Cupin (fogC) of from fog in Aspergillus nidulans LO8030 led to the isolation of 4. Subsequent introduction of the prenyltransferase FogH and cytochrome P450 FogE led to the formation of isoprenylated 5. Eventually, feeding experiments demonstrated that 5 undergoes catalysis by the oxidoreductase FogF to produce 1 and its derivatives (Figure 2A; Nies et al., 2020).
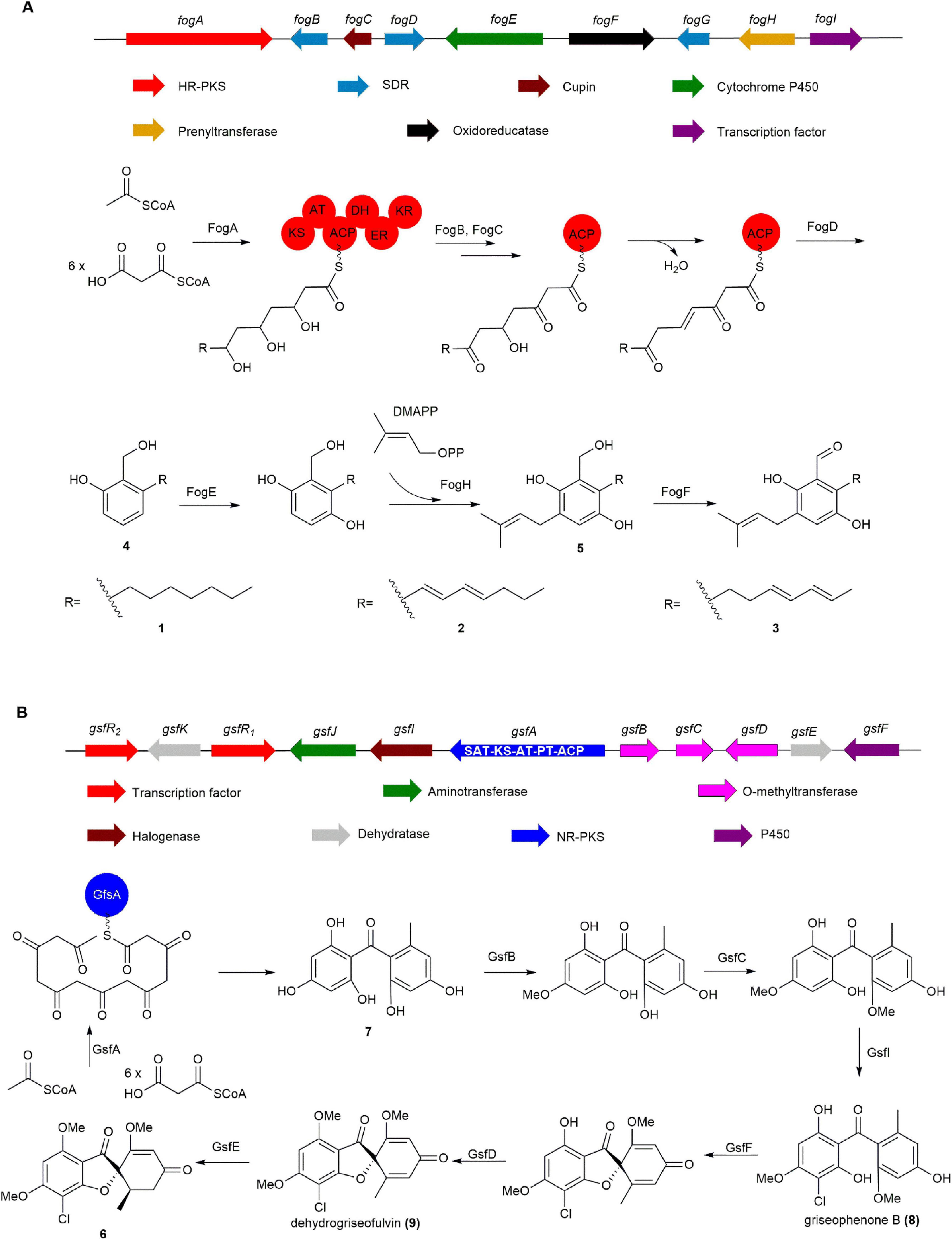
Figure 2. The BGCs and biosynthetic pathways of (A) flavoglaucin (1) and (B) griseofulvin (6). SAT, starter unit:ACP transacylase; KS, ketosynthase; AT, acyltransferase; ACP, acyl carrier protein; PT, product template.
2.1.2 Griseofulvin
Griseofulvin (6), an antifungal drug that disrupts fungal cell mitosis, is derived from Penicillium griseofulvum Dierckx, which was identified in the deep-sea region of the Indian Ocean in 1939 (Oxford et al., 1939, De Carli and Larizza, 1988). Compound 6 used to treat superficial infections, exhibits a fungistatic effect on various types of dermatophytes, including trichophyton, microsporum, achorion, and epidermophyton species (Vardanyan and Hruby, 2006). Furthermore, 6 possesses the ability to disrupt mitotic spindles and potentially inhibit centrosomal clustering, which are properties that hold promise for cancer treatment (Tsunematsu et al., 2020, Panda et al., 2005, Rebacz et al., 2007). Additionally, 6 has demonstrated significant apoptotic activity in diverse human and murine myeloma and lymphoma cell lines, as well as in human primary cells (Kim et al., 2011).
Tang group confirmed the BGC gsf of 6 through gene knockout experiments and successfully elucidated the biosynthesis of 6 by in vitro reconstitution of each enzyme in the gsf cluster. Gene deletions confirmed that non-reducing PKS (NR-PKS) gsfA is essential for the biosynthesis of 6, playing a pivotal role in catalyzing the formation of benzophenone 7. Diverging from conventional NR-PKS enzymes, GfsA does not incorporate a TE domain, thereby indicating that the release of 7 is likely mediated by its PT domain (Chooi et al., 2010, Cacho et al., 2013). Then 7 undergoes modification by two methoxyltransferases, GsfB and GsfC, and chlorination by the halogenating enzyme GsfI, resulting in the formation of griseophenone B (8). Subsequently, P450 enzyme GsfF and methoxyltransferase GsfD catalyze the formation of spirocyclic structures and subsequent methylation to yield dehydrogriseofulvin (9). Finally, GsfE reduces the C2–C3 double bond to a single bond, thereby producing the final product 6 (Figure 2B; Lane et al., 2002, Harris et al., 1976).
2.1.3 Sorbicillinoids
Sorbicillinoids are a family of hexaketide metabolites characterized by a distinctive sorbyl side chain residue, first isolated as impurities in penicillin in 1948 (Harned and Volp, 2011, Andrade et al., 1992). Sorbicillinoids natural products are widely present in various marine fungi, such as sponge derived fungi Trichoderma reesei4670 (Zhang P. et al., 2019), Trichoderma reesei (HN-2016-018) (Rehman et al., 2020), Stagonospora sp. SYSU-MS7888 (Chen et al., 2022b), and Penicillium sp. SCSIO06868 (Pang et al., 2022), and exhibit significant anti-inflammatory (Pang et al., 2022, Chen et al., 2022b,Zhang P. et al., 2019, Zhao et al., 2017), anticancer (Rehman et al., 2020), antibacterial (Warr et al., 1996), and anti-HIV activities (Zhao et al., 2017).
In 2014, the FAD-dependent monooxygenase gene sorC from Penicillium chrysogenum E01-10/3 was expressed in Escherichia coli by Cox group. SorC effectively catalyzed the oxidative dearomatization of sorbicillin (10) and dihydrosorbicillin (11), producing sorbicillinol (12) and dihydrosorbicillinol (13). Combining bioinformatic analysis with experimental data, the BGC responsible for sorbicillinoids was preliminarily confirmed (Fahad et al., 2014). Mach-Aigner group conducted further investigation into the biosynthetic pathway of 12 in T. reesei through gene knockout and in vitro enzyme catalysis. They discovered that knocking out the flavin-dependent monooxygenase gene sorD resulted in a significant increase in the amount of reduced branched double bonds in 12. This led to the inference that sorD primarily catalyzes the formation of branched double bonds at positions 2 and 3 in 12 (Derntl et al., 2017). However, subsequent research by the Cox group revealed that sorD also possesses dimerization activity. It can catalyze the Diels-Alder reaction of 12 to produce homodimerization product 13, as well as catalyze the Diels-Alder reaction between 12 and 14 to produce heterodimerization product 15. This marks the first report of sorD functioning as a dimerase that catalyzes intermolecular Diels-Alder reactions (Figure 3A; Kahlert et al., 2020). Trichodimerol (16) is a unique cage-like dimeric sorbicillinoid pigment commonly isolated from many marine fungi. In 2023, Gao group reported that a major facilitator superfamily transporter (StaE) from marine-derived fungus Stagonospora sp. SYSU-MS7888 is involved in the formation of 16 (Figure 3A; Ren et al., 2023).
2.1.4 Monodictyphenone
Monodictyphenone (17) is benzophenone derivatives with significant biological activities. Compound 17, isolated from the marine algicolous fungus Monodictys putredinis (Krick et al., 2007) and the ascidian-derived fungus Diaporthe sp. SYSU-MS4722 (Chen et al., 2022a), serves as common precursor for various complex natural products biosynthesis with anthraquinone and xanthone structures (Simpson, 2012, Neubauer et al., 2016, Sanchez et al., 2011, Schatzle et al., 2012, Matsuda et al., 2018, Griffiths et al., 2016, Wei and Matsuda, 2020, Wei et al., 2021c).
Compound 17 is a common precursor in the biosynthesis of anthraquinone and xanthone. The BGC of 17 was characterized by the Oakley group. They discovered that knocking out the cclA gene, responsible for histone H3K4 methylation, successfully led to the identification of 17 in A. nidulans. Further, knocking out the NR-PKS gene mdpG in the cclA-inactivated A. nidulans strain resulted in the complete disappearance of 17 in the mutant strain, thereby identifying the mdp BGC of 17 (Chiang et al., 2010). When the two transcription factor genes, mdpA and mdpE, in the mdp cluster were knocked out, the corresponding mutant strains showed a significant decrease in 17 production. This indicates that the transcription factors MdpA and MdpE play a positive regulatory role in the production of 17 in A. nidulans. Additionally, knocking out the β-lactamase gene mdpF resulted in the complete disappearance of 17, demonstrating that the β-lactamase MdpF is essential for the early biosynthesis of 17. Subsequently, the biosynthetic pathway of 17 was inferred through bioinformatics analysis (Figure 3B; Chiang et al., 2010).
2.1.5 Epicospirocins
Epicospirocins are natural products of the dibenzospirone class with various pharmacological activities, primarily derived from marine fungi. For instance, aspermicrones B (18) and C (19), isolated from the seaweed-derived endophytic fungus Aspergillus micronesiensis, show significant bioactivities. Compound 18 exhibited a selective cytotoxic effect toward the HepG2 cell line (IC50 = 9.9 μM), and both 18 and 19 displayed antimicrobial activity against Staphylococcus aureus (MIC = 123.2 μM for each compound) (Luyen et al., 2019).
In 2020, the Liu group used molecular network technology to uncover two pairs of dibenzospiroketal racemates, (±)-epicospirocin A (20a/20b) and (±)-1-epi-epicospirocin A (21a/21b), along with two (+)-enantiomers of aspermicrones, ent-aspermicrone B (18b) and ent-aspermicrone C (19b), and two hemiacetal epimeric mixtures, epicospirocin B/1-epi-epicospirocin B (22/23) and epicospirocin C/1-epi-epicospirocin C (24/25) from the fungus Epicoccum nigrum 09116. Through gene function annotation, gene knockout, and mass spectrometry analysis, they identified the BGC of epicospirocins and proposed its biosynthetic pathway. Knocking out the pks gene in the Δesp3 mutant strain resulted in the complete absence of epicospirocins and their analogs, indicating that Esp3 is crucial for the biosynthesis of the 5-methylorsellinic acid (26) skeleton. Subsequently, construction of a Δesp4 mutant strain led to the accumulation of a significant amount of 26, demonstrating that Esp4 recognizes 26 and reduces its carboxyl group to an aldehyde group in 26. Esp6 and Esp7 were found to be primarily responsible for the sequential hydroxylation of the benzene ring and methyl group, leading to the formation of 27 and 28. Ultimately, 27 and 28 are converted into epicospirocins through the actions of multiple post-modifying enzymes (Figure 4; Zhu et al., 2020).
2.1.6 Chrysoxanthones
Using an epigenetic strategy, three heterodimeric tetrahydroxanthone–chromanone lactones, chrysoxanthones A–C (29–31), were discovered from the sponge-associated Penicillium chrysogenum HLS111 by treating it with the histone deacetylase inhibitor valproate sodium. Compounds 29–31 exhibited antibacterial activities against Bacillus subtilis, with minimum inhibitory concentration (MIC) values of 5–10 μg/mL (Zhen et al., 2018).
Following whole-genome sequencing of the fungus P. chrysogenum HLS111 and comparison with the known biosynthetic pathway of the tetrahydroxanthone dimer secalonic acid (Neubauer et al., 2016), a plausible biosynthetic pathway for chrysoxanthones was proposed. An iterative NR-PKS with KS-AT-PT-ACP architecture is responsible for synthesizing the octaketide (32). Atrochrysone carboxylic acid (33) is then released from the NR-PKS by a metallo-β-lactamase-type thioesterase (MβL-TE). This intermediate undergoes endogenous decarboxylation, dehydration, and oxidation to form anthraquinone (34). The final 29–31 are produced through successive dehydratase and oxygenase reactions (Figure 5A; Zhen et al., 2018).
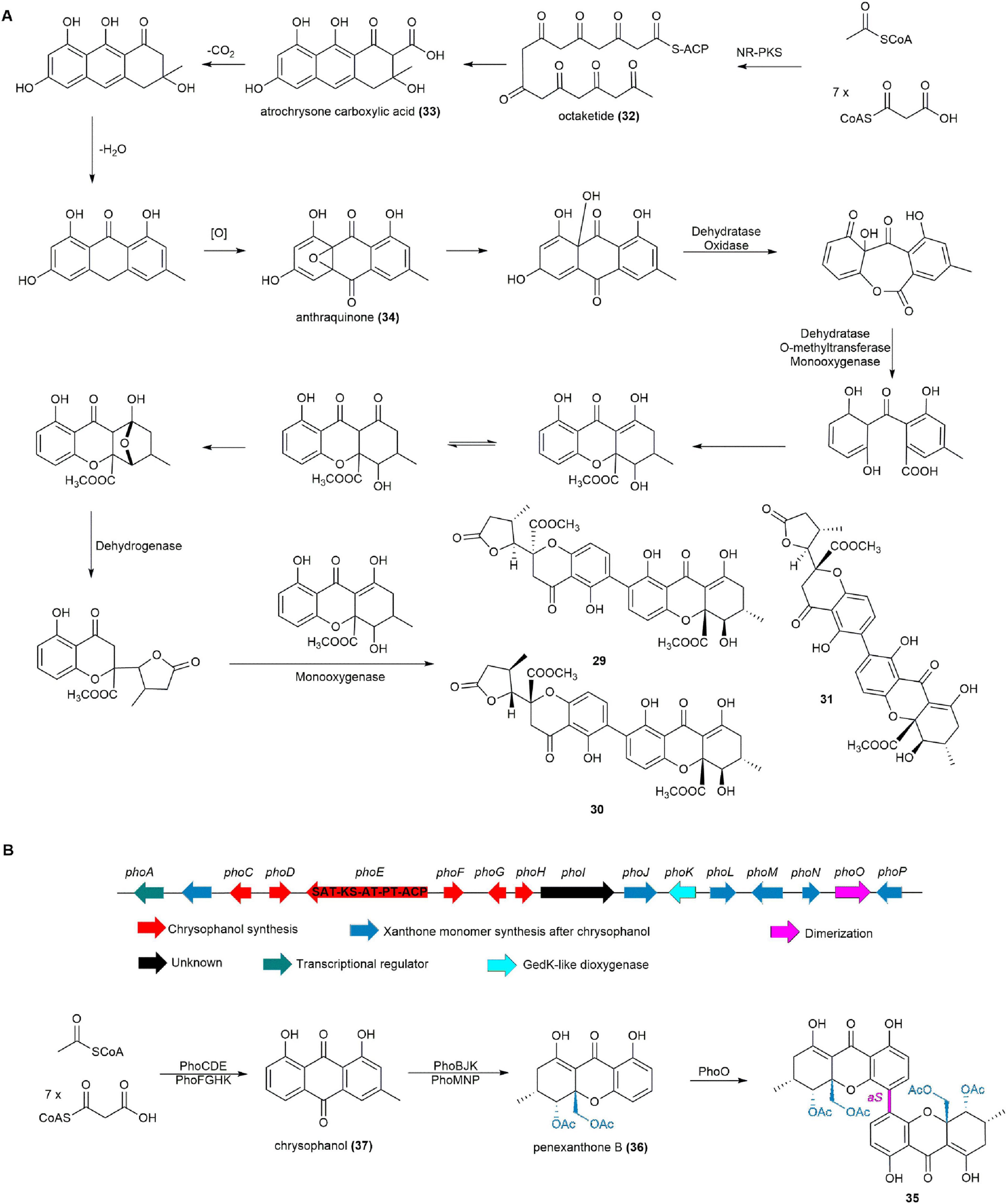
Figure 5. (A) The plausible biosynthetic pathway of chrysoxanthones. (B) The BGC and biosynthetic pathway of phomoxanthone A (35).
2.1.7 Phomoxanthone A
Phomoxanthone A (35), a homodimer of penexanthone B (36) formed through an unusual 4,4′-linkage, was isolated from various filamentous, including the ascidian-derived fungus Diaporthe sp. SYSU-MS4722 (Yuan et al., 2022b,Chen et al., 2022a). Compound 35 demonstrated superior cytotoxicity compared to cisplatin in both sensitive and resistant ovarian and bladder cancer cells. It induced mitochondrial depolarization, caspase activation, and apoptosis, specifically targeting the inner mitochondrial membrane without damaging plasma membranes. 35 also activated immune cells, potentially enhancing chemotherapy efficacy by overcoming resistance (Wang C. et al., 2019, Ronsberg et al., 2013, Frank et al., 2015). Additionally, 35 demonstrated antimicrobial activity against Bacillus megaterium and strong antifungal activity against the rice blast pathogen, Pyricularia oryzae (Elsässer et al., 2005).
The BGC, named pho, for 35 was definitively identified by completely deleting the phoE gene, a pks gene within the pho cluster potentially responsible for skeleton construction of 35, in Diaporthe sp. SYSU-MS4722 using an advanced CRISPR/Cas9 system, resulting in the cessation of 35 production and confirming the pivotal role of the pho cluster in 35 biosynthesis. Heterologous expression of 14 biosynthetic genes in A. oryzae NSAR1 revealed that PhoCDEFGHK catalyzes the initial steps of 35 biosynthesis to give chrysophanol (37). Subsequently, PhoBJKLMNP process 37 to 36. Feeding experiments indicated that PhoO, a cytochrome P450 enzyme, mediates the regioselective oxidative para-para coupling of 36 to yield 35 (Figure 5B; Yuan et al., 2022b).
2.1.8 Amphichopyrones A and B
Amphichopyrones A (38) and B (39), α-pyrone derivatives isolated from A. oryzae NSAR1 constructs containing amp BGC from the ascidian-derived fungus Amphichorda felina SYSU-MS7908, have shown significant anti-inflammatory activity by inhibiting nitric oxide production in RAW264.7 cells, with IC50 values of 18.09 ± 4.83 μM and 7.18 ± 0.93 μM, respectively (Yuan et al., 2022a).
The amp cluster consists of 10 biosynthetic genes and shares similarities with the sol cluster, which is responsible for the biosynthesis of α-pyrone solanapyrone D (Kasahara et al., 2010). Introducing only the ampB gene into A. oryzae NSAR1 resulted in the production of 38. When AmpC, a putative O-methyltransferase, was introduced into the AO-ampB construct, both 39 and udagawanone A (40) were produced. Adding the remaining eight genes, ampADEFGHIJ, to the AO-ampBC construct did not change the outcome, as 39 and 40 were still produced. These findings indicate that PKS AmyB is responsible for producing 38, while AmpC catalyzes the methylation of 38 at the C-4 hydroxyl to form 39. The subsequent hydroxylation of 39 to 40 is likely catalyzed by endogenous enzymes from the A. oryzae NSAR1 host (Figure 6A; Yuan et al., 2022a).
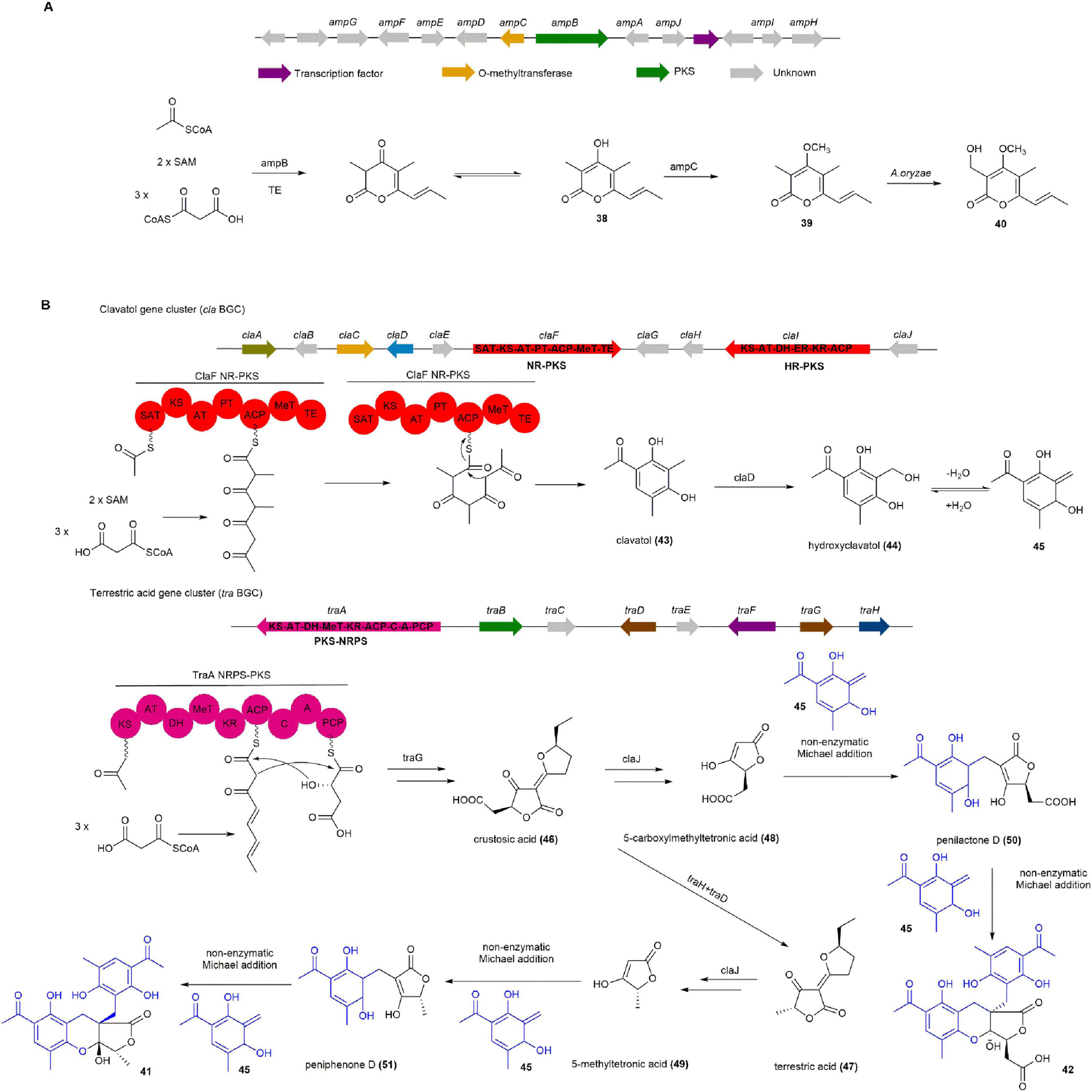
Figure 6. The BGCs and biosynthetic pathways of (A) amphichopyrone B (39) and (B) penilactone A (41) and B (42). SAT, starter unit:ACP transacylase; KS, ketosynthase; AT, acyltransferase; ACP, acyl carrier protein; PT, product template; DH, dehydratase; MeT, methyltransferase; ER, enoyl reductase; KR, ketoreductase; TE, thioesterase; A, adenylation; C, condensation; PCP, peptidyl carrier protein.
2.1.9 Penilactones A and B
Penilactones A (41) and B (42), the highly oxygenated fungal polyketides about non-enzymatic Michael addition mediated the coupling process of polyketide–polyketide hybrids, were firstly isolated from an Antarctic deep-sea derived fungus Penicillium crustosum PRB-2 (Wu et al., 2012). Compound 41 showed NF-κB inhibitory activity with 40% inhibition rate at a concentration of 10 mM using transient transfection and reporter gene expression assays (Peng et al., 2012, Wu et al., 2012).
The biosynthetic pathway for 41 and 42 is proposed to originate from the hybridization of an o-quinone methide (45) unit and a γ-butyrolactone moiety through a 1,4-Michael addition, completing their carbon skeleton construction. Two separate BGCs, termed cla and tra, are responsible for this process, identified through gene deletion and heterologous expression in A. nidulans. After the deletion of claF or traA, the mutant strains completely abolished the production of 41 and 42, suggesting that the associated BGC cla and tra are responsible for their biosynthesis. To determine the function of ClaF, claF was cloned into the expression vector pYH-wA-pyrG and expressed in A. nidulans. Clavatol (43) was successfully detected by LC-MS from the transformed A. nidulans. Furthermore, deletion of claD abolished the production of 41 and 42, while 43 was clearly accumulated, indicating that the core NR-PKS ClaF in the cla BGC synthesizes 43, which is subsequently oxidized by the non-heme FeII/2-oxoglutarate-dependent oxygenase ClaD to form hydroxyclavatol (44). Subsequent gene knockout experiments on other genes within the cla and tra were carried out, leading to a comprehensive elucidation of the biosynthetic pathway for 41 and 42. The subsequent biosynthetic pathway is as follows: 44 spontaneously dehydrates into the crucial intermediate 45. In the tra BGC, the PKS-NRPS TraA and the trans-acting enoyl reductase (ER) TraG together form crustosic acid (46). The non-heme FeII/2-oxoglutarate-dependent oxygenase TraH then oxidatively decarboxylates 46 into dehydroterrestric acid, with its terminal double-bond reduced by the flavin-dependent oxidoreductase TraD to produce terrestric acid (47). Feeding experiments in a ΔtraA mutant confirmed that 46 and 47 are intermediates that can be transformed into 5-carboxymethyl tetronic acid (48) and 5-methyltetronic acid (49), respectively. Notably, the enzyme(s) catalyzing the Michael addition were not identified. However, incubation of 48 with 44 at 25°C in water led to the formation of penilactone D (50) as the major product and 42 as the minor product. Similarly, incubation of 49 with 44 produced peniphenone D (51) as the major product and 41 as the minor product. Further incubation of 50 and 51 with 44 resulted in the formation of 41 and 42. These findings indicate that the Michael addition in the biosynthesis of 41 and 42 occurs non-enzymatically and can happen spontaneously (Figure 6B; Dai et al., 2022, Fan et al., 2019, Fan et al., 2020).
2.1.10 Alternapyrones G and H
Alternapyrones G (52) and H (53), α-pyrones with a 6-alkenyl chain, were isolated from a marine-derived strain of the fungus Arthrinium arundinis, and 52 not only suppressed M1 polarization in LPS-stimulated BV2 microglia but also stimulated dendrite regeneration and neuronal survival after Aβ treatment, suggesting its potential as a scaffold for Alzheimer’s disease drug discovery (Hu et al., 2024).
The BGC (alt′) for 52 and 53 was identified from A. arundinis ZSDS-F3 and validated by heterologous expression in A. nidulans. The alt′ BGC includes five open reading frames encoding a HR-PKS (alt5′), a flavin-linked oxidoreductase (alt4′), and three cytochrome P450 monooxygenases (alt3′, alt2′, and alt1′). The expression of HR-PKS alt5′ in A. nidulans led to the production of alternapyrone (55). Co-expression of alt5′ with alt1′ and alt4′ did not result in the formation of any new products, while co-expression of alt5′ with alt2′ and alt3′ led to the production of a set of products, including 53, 54, alternapyrone B (56), alternapyrone D (57), and alternapyrone E (58). Finally, the introduction of alt5′ along with the four alt genes (alt1′–4′) did not lead to the production of any new metabolites. Based on these results, the biosynthetic pathway of 52 and 53 are as follows: The HR-PKS Alt5′ synthesizes the polyketide chain from one acetyl-CoA, nine malonyl-CoA, and eight SAM molecules, followed by spontaneous lactonization to form 55. The cytochrome P450 monooxygenase Alt2′ successive converts the methyl group at position 26 to a OH and carboxyl group, producing 54 and 56. The cytochrome P450 monooxygenase Alt3′ then catalyzes successive hydroxylation, epoxidation, and oxidation steps to produce 52, 53, 57, and 58 from 56 (Figure 7A; Hu et al., 2024).
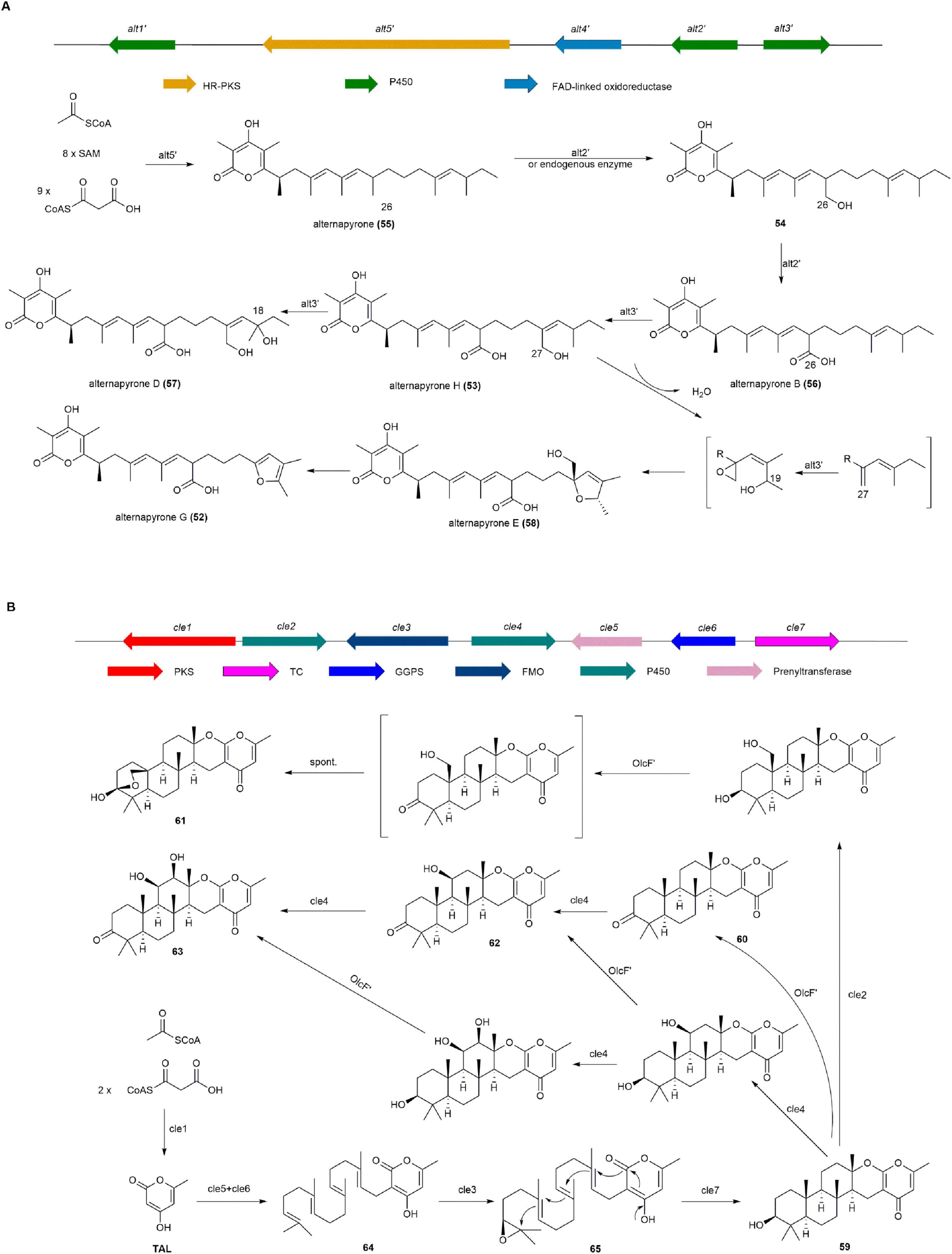
Figure 7. The BGCs and biosynthetic pathways of (A) alternapyrone G (52) and (B) chevalone E (59) and derivatives.
2.2 Terpenes
2.2.1 Chevalone E
Chevalone E (59), a class of meroterpenoids from the sponge fungus Aspergillus milianensis KUFA 0013, shows synergism with oxacillin against methicillin-resistant Staphylococcus aureus (MRSA) (Prompanya et al., 2014). It and its derivatives were discovered through heterologous expression of a cryptic gene cluster cle from Aspergillus versicolor 0312 in A. oryzae (Wang W.-G. et al., 2019). Additionally, chevalone analog, obtained via biocatalytic and chemical derivatization, such as chevalone F (60), N (61), O (62), and P (63), exhibit synergistic inhibition of MDA-MB-231 breast cancer cell viability when combined with doxorubicin (Xiao et al., 2022).
NR-PKS Cle1 was first expressed heterologously in A. oryzae, but no related products were detected. Co-expression of Cle1, Cle5, and Cle6 resulted in the production of 64, indicating Cle1 generates TAL, while Cle5 and Cle6 are responsible for isopentenylation of side chains. Co-expression of Cle1, Cle5, Cle6, and FMO Cle3 produced the side chain epoxidation product 65. Finally, 65 was converted to 59 by the cyclizing enzyme Cle7. Additionally, a series of 59 derivatives were obtained by expressing two P450 enzymes (Cle2 and Cle4) and a dehydrogenase OlcF′ from A. felis 0260 (Figure 7B; Wang W.-G. et al., 2019, Xiao et al., 2022).
2.2.2 Ophiobolins
Ophiobolins are sesterterpenoids characterized by a 5-8-5 tricyclic skeleton, predominantly isolated from marine Aspergillus species, and exhibit notable cytotoxic properties (Zhang et al., 2012, Tian et al., 2017, Yan et al., 2022, Chai et al., 2016). Ophiobolin A (66) demonstrates efficacy against CLL and P388 cell lines, while ophiobolin O (67) inhibits MCF-7 proliferation and reverses MCF-7/ADR resistance to adriamycin (Bladt et al., 2013, Shen et al., 1999, Yang et al., 2012, Sun et al., 2013). 67 holds potential as a novel therapeutic agent and antagonist for multi-drug-resistant tumors, underscoring its significant clinical relevance for cancer chemotherapy (Sun et al., 2013, Yang et al., 2012). Additionally, 6-epi ophiobolin G (68) functions as an estrogen receptor down-regulator, offering potential for breast cancer treatment (Zhao et al., 2019). Ophiobolin G (69), ophiobolin H (70), ophiobolin K (71), 6-epi-ophiobolin K (72), 67, and 6-epi-ophiobolin O (73) exhibit cytotoxicity against P388 cells, with IC50 values of 4.7, 9.3, 24.6, 105.7, 13.3 and 24.9 μM, respectively (Zhang et al., 2012). Notably, 66, ophiobolin B (74), ophiobolin C (75), and 71 induce apoptosis in leukemia cells at nanomolar concentrations (Bladt et al., 2013).
Five BGCs associated with ophiobolin (76) were identified through whole genome sequencing, gene inactivation, gene replacement, and in vitro enzyme catalysis experiments using endophytic fungus Aspergillus ustus 094102 derived from marine mangroves (Chai et al., 2016). They definitively established that these BGCs are responsible for producing natural products such as drimane (77), veridiene (78), 76, and ergosterol (79) with carbon skeletons of C15, C20, C25, and C30, respectively. Among these clusters, Au8003 is pivotal in elongating chains from DMAPP (80) and IPP (81) to GFPP (82), and subsequently cyclizing 82 to yield 76. The biosynthesis of 76 also involves complementary pathways, where Au6298, Au13192, and Au11565 catalyze the elongation of 80 and 81 to produce final products FPP (83), GGPP (84), and 82, respectively. 83 could be used for 77 synthesis by drimane synthetase or for HexPP (85) synthesis by Au3446, which may then be used to synthesize 79. Compound 84 produced by Au13192 serves as a crucial precursor not only for 76 but also for the production of 78 (Figure 8; Chai et al., 2016).
In 2022, the biosynthetic pathway of 71 was elucidated through transcriptome analysis, gene knockout, heterologous expression, and precursor feeding experiments on A. ustus 094102 by the Hong group. The terpene synthase OblAAu elongates and cyclizes 80 and 81 to form ophiobolin F (86), which is oxidized by the cytochrome P450 monooxygenase OblBAu to 75. The flavin-dependent oxidase OblCAu catalyzes the conversion of 86 and 75 to 16,17-dehydro-ophiobolin F (87) and 71, respectively. The transporter OblDAu moves 71 and 75 between the cell wall and membrane, reducing their toxicity and preventing inhibition of host cells, thereby playing a detoxifying role (Figure 9A; Yan et al., 2022).
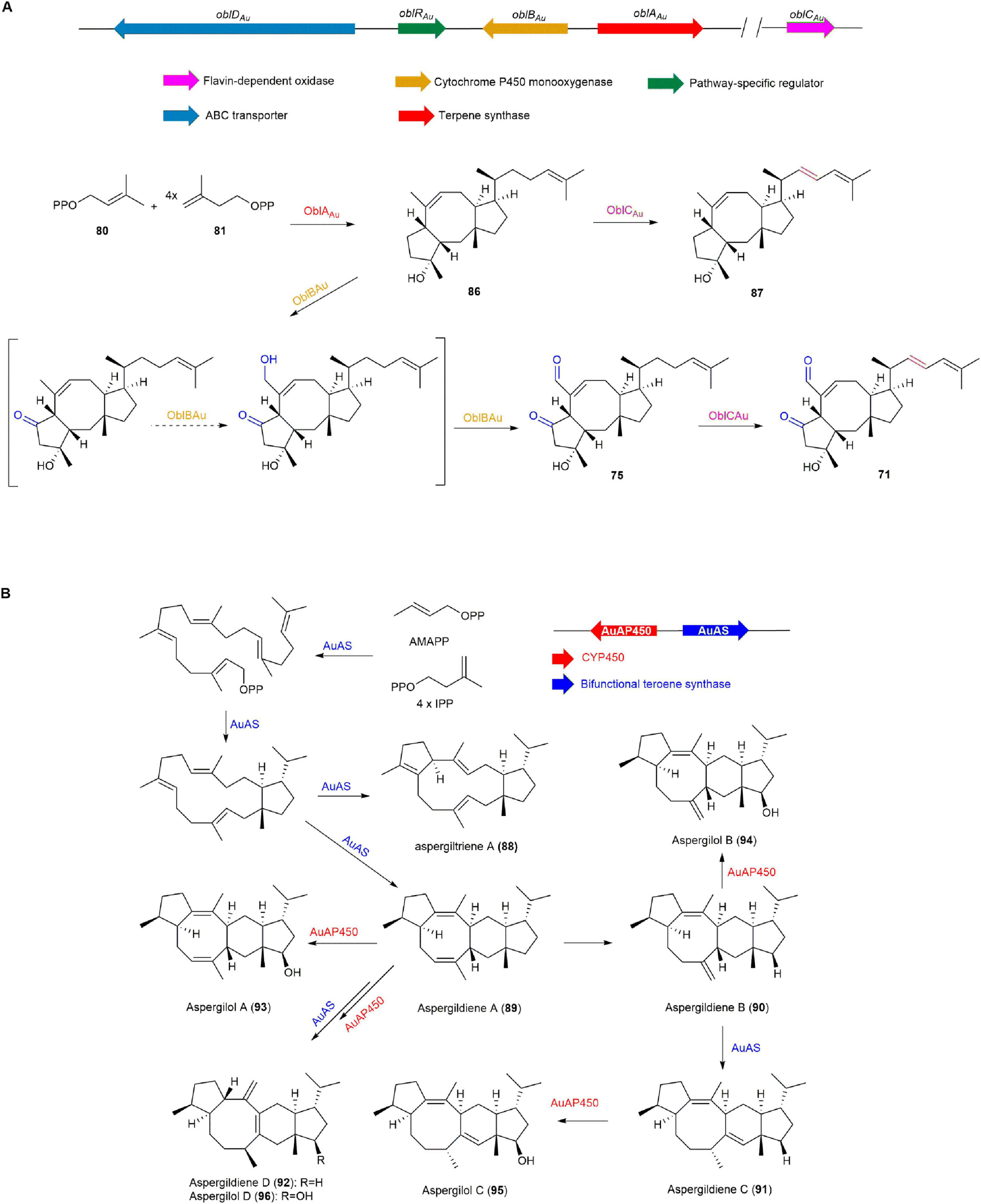
Figure 9. The BGCs and biosynthetic pathways of (A) ophiobolin K (71) and (B) aspergiltriene A (88) and derivatives.
2.2.3 Aspergildienes and aspergilols
In 2021, aspergildienes and aspergilols were discovered through genome mining of the marine-derived mangrove endophytic fungus Aspergillus ustus 094102 by the Hong group. Heterologous expression of AuAS, a bifunctional terpene synthase, in A. oryzae NSAR1, led to the discovery of five novel sesterterpenes, including a 5/12/5 tricyclic intermediate aspergiltriene A (88) and four 5/6/8/5 tetracyclic compounds aspergildiene A-D (89, 90, 91, 92) with rare stereochemistry. Coexpression with the upstream cytochrome P450 monooxygenase (AuAP450) led to the discovery of four new corresponding sesterterpene alcohols aspergilol A-D (93, 94, 95, 96). Among these, 93 was found to exhibit cytotoxicity against MCF-7, MDA-MB-231, and HepG2 cancer cells (IC50 21.20-48.76 μM), while 94 demonstrated cytotoxic effects specifically on MCF-7 cells (IC50 27.41 μM) (Figure 9B; Guo et al., 2021).
2.2.4 Spiromaterpenes
Spiromaterpenes, guaiane-type sesquiterpenes, emerged from the activation of a terpene-related BGC following the epigenetic manipulation of a deep-sea sediment-derived Spiromastix sp. fungus using suberoylanilide hydroxamic acid (SAHA). Spiromeroterpenes D-F (97, 98, 99) effectively inhibited NO production in LPS-induced BV2 microglial cells, with preliminary structure-activity relationship indicating that the 2(R),11-diol unit enhances their efficacy (Figure 10A). Notably, 98 prevented the LPS-induced translocation of NF-κB from the cytosol to the nucleus, and significantly reduced pro-inflammatory cytokines IL-1β, IL-6, and TNF-α, as well as iNOS and COX-2 at both the protein and mRNA levels in BV2 cells. These results highlight 98′s potential as a promising agent for further development in combating neuroinflammation (Guo et al., 2021).
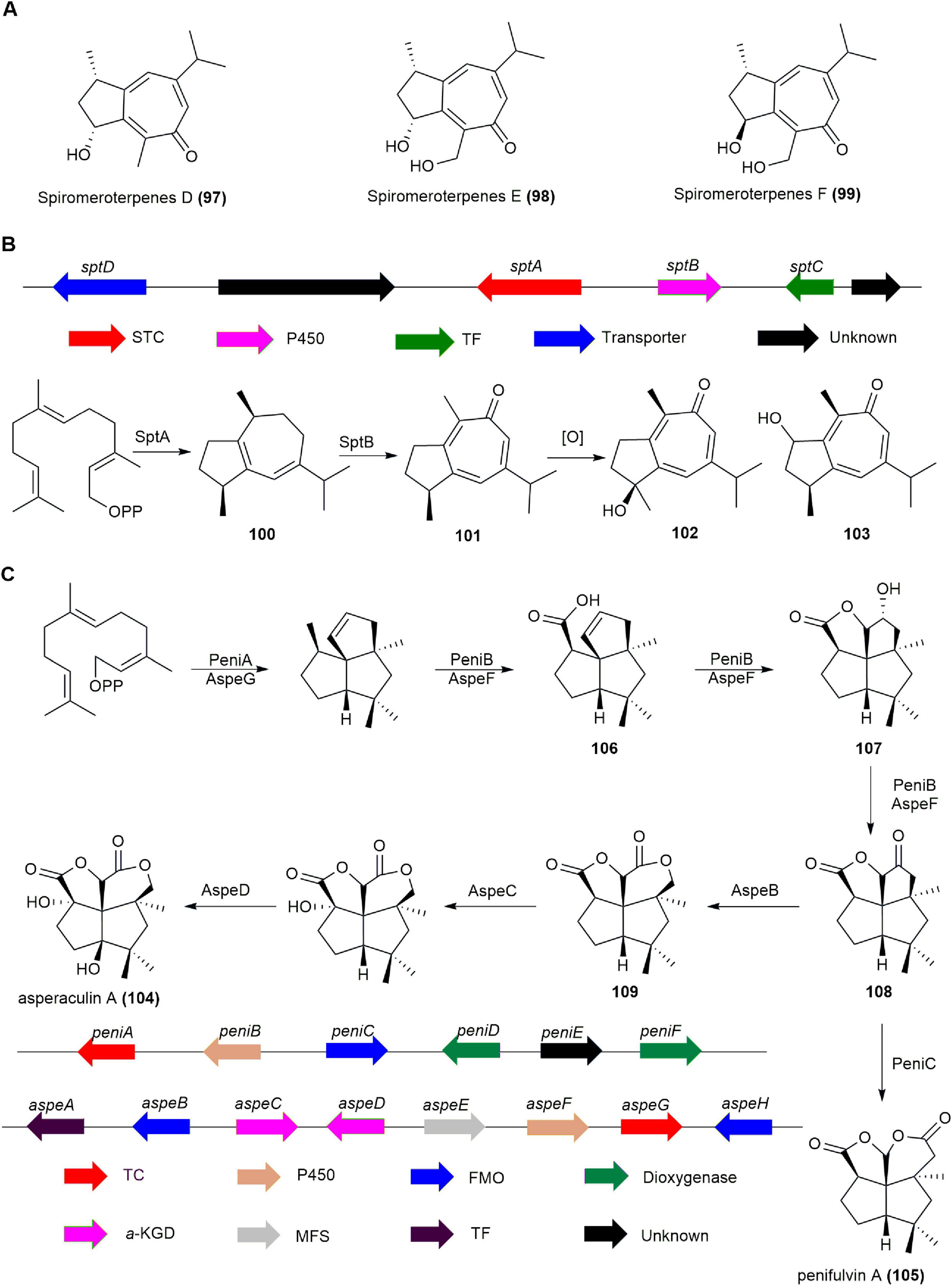
Figure 10. (A) The structures of spiromeroterpenes D-F (97, 98, 99). (B) The BGC and biosynthetic pathway of spiromaterpenes. (C) The BGCs and biosynthetic pathway of penifulvin A (105).
The biosynthetic pathway of spiromeroterpenes in Spiromastix sp. was elucidated by heterologous expression, biochemical characterization, and incubation experiments. Co-expression of the sesquiterpene cyclase SptA, a homologous protein of the known fungal guaiane-type sesquiterpene cyclase FfSTC5 (Burkhardt et al., 2016), and cytochrome P450 SptB in A. nidulans LO8030 successfully produced spiromeroterpene A (101) and its derivatives 102 and 103. Subsequently, SptA was expressed and purified in Escherichia coli, then incubated with FPP and Mg2+, yielding compound 100. By introducing the P450 enzyme SptB separately into A. nidulans LO8030 and using 100 as a substrate, the target product 101 and its derivatives 102 and 103 were also obtained. These findings suggest that SptA catalyzes the production of guaia-1(5),6-diene, while cytochrome P450 SptB is responsible for the formation of the tropone ring (Figure 10B; Liu et al., 2022).
2.2.5 Asperaculin A
Asperaculin A (104), a sesquiterpenoid with a unique [5,5,5,6] dioxafenestrane ring system, was isolated from the marine fungus Aspergillus aculeatus CRI323-04. It closely resembles penifulvin A (105) from the terrestrial fungus Penicillium griseofulvum NRRL35584 but is distinguished by a transposed γ-lactone ring and an additional hydroxyl group at C9 (Ingavat et al., 2011, Das and Chakraborty, 2016).
The BGC known as aspe in Aspergillus aculeatus CRI323-04, which is homologous to the BGC peni for 105 with a similar dioxa [5.5.5.6] fenestrane core, was confirmed to be responsible for 104 biosynthesis through heterologous expression in A. nidulans. Heterologous reconstruction of aspe and peni clusters in A. nidulans showed that the sesquiterpene synthases (PeniA and AspeG) and cytochrome P450 enzymes (PeniB and AspeF) perform identical functions, producing intermediates 106, 107, and 108. Co-expression of aspeGFB in A. nidulans resulted in the generation of oxidation product 109, while constructs harboring aspeGF + peniC produced 105. This indicates that PeniC and AspeB selectively undergo Baeyer–Villiger oxidation at different positions of the same substrate 108 to generate distinct esterification products, compounds 105 and 109. The final product, compound 104, is formed through the action of two dioxygenases, AspeCD (Figure 10C; Wei et al., 2021b,Zeng et al., 2019, George et al., 2021).
2.2.6 Talaronoids
Talaronoids, fusicoccane diterpenoids with a unique tricyclic 5/8/6 ring system, were discovered from the marine-derived fungus Aspergillus flavipes CNL-338 (Zhang et al., 2022). Talaronoids A–D (110, 111, 112, 113) showed butyrylcholinesterase (BChE) inhibitory activity with IC50 values of 14.71 ± 1.07, 26.47 ± 0.35, 31.51 ± 0.28, and 11.37 ± 0.85 μM, respectively (Zhang et al., 2020).
After sequencing the whole genome of A. flavipes CNL-338, the BGC known as tnd, responsible for talaronoid production, was confirmed through heterologous expression. The tndC gene, encoding a protein homologous to the known diterpene synthase PaFS (Toyomasu et al., 2007) and the sesterterpene synthase AcOS (Chiba et al., 2013), was expressed in Saccharomyces cerevisiae, leading to the detection of talarodiene (114). Stable isotope tracer experiments further demonstrated the conversion of geranylgeranyl diphosphate to 114, suggesting that TndC is a novel bifunctional diterpene synthase. Finally, a cytochrome P450 enzyme (TndB), an aldehyde reductase (TndE), and an alcohol dehydrogenase (TndF) were proposed to collectively catalyze the conversion of 114 into compounds 110, 111, 112, 113 (Figure 11A; Zhang et al., 2022).
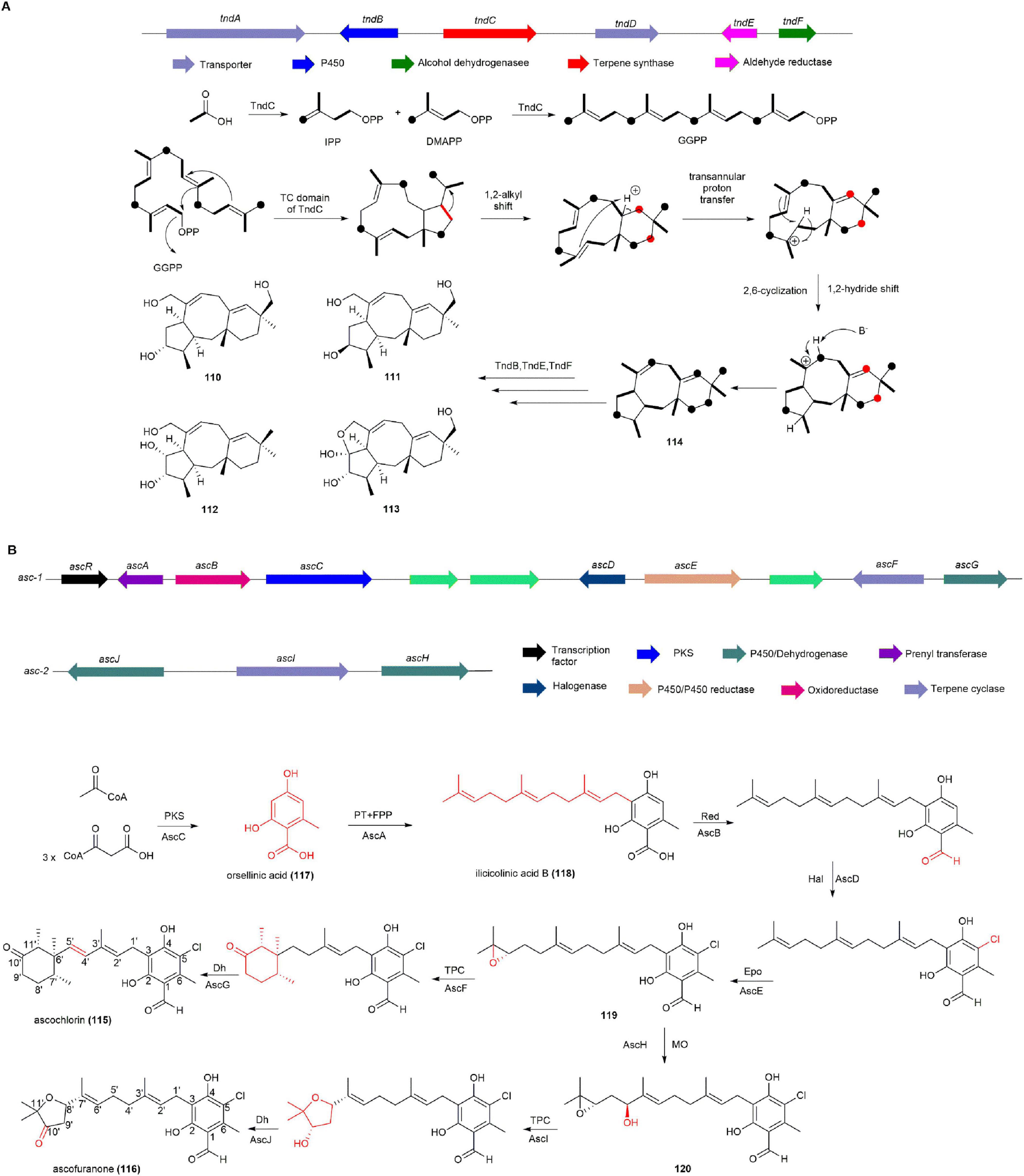
Figure 11. The BGCs and biosynthetic pathways of (A) talaronoids and (B) ascochlorin (115) and ascofuranone (116).
2.3 Meroterpenoids
2.3.1 Ascochlorin and ascofuranone
Ascochlorin (115) is a meroterpenoid with 5-chloroorcylaldehyde substituted at C-3 by a cyclized sesquiterpene side chain, extractable from marine-derived fungus Acremonium Sclerotigenum (Luo et al., 2021) and Stilbella fimetaria (Subko et al., 2021). 115 and its derivatives exhibit a wide range of physiological activities, including antibacterial, antitumor, antiviral, hypolipidemic, antihypertensive, and anti-inflammatory effects, as well as improving type I and II diabetes by reducing serum cholesterol and triglyceride levels (Kawaguchi et al., 2013, Luo et al., 2021, Subko et al., 2021, Lee et al., 2016, Magae et al., 1982, Seephonkai et al., 2004, Tamura et al., 1968, Takatsuki et al., 1969, Magae et al., 1988, Sasaki et al., 1973). Additionally, ascofuranone (116) shows promise as a candidate for treating African trypanosomiasis (Yabu et al., 2003, Shiba et al., 2013).
The BGCs of 115 and 116 have been identified through transcriptome analysis, gene knockout, and heterologous expression in the fungus Acremonium egyptiacum. The production of 115 and 116 in A. egyptiacum varied depending on the culture medium, with 0.96 mg of 116 produced in F1 medium and 399 mg of 116 in AF medium. After isolating poly(A)-selected RNAs from mycelia grown in both F1 and AF media and conducting transcriptome analysis, it was found that the expression of genes (ascABCDEFGR) in the asc-1 cluster and genes (ascHIJ) in the asc-2 cluster were more strongly induced in AF medium than in F1 medium. This suggests that asc-1 and asc-2 clusters are responsible for the biosynthesis of 115 and 116. Further, heterologous expression in A. oryzae and targeted gene knockouts in asc-1 and asc-2 were performed to fully elucidate the biosynthetic pathways of 115 and 116. Asc-1 comprises eight genes, including NR-PKS AscC responsible for producing the precursor orsellinic acid (117). AscA, an isopentenyl transferase, catalyzes the formation of ilicicolinic acid B (118) from farnesyl pyrophosphate (FPP) and 117, which undergoes subsequent reduction by AscB, chlorination by AscD, and epoxidation by AscE to form compound 119, which is then converted to 116 by the terpenoid cyclase AscF and the oxidase AscG. In addition, 119, recognized by the P450 enzyme AscH from asc-2, undergoes hydroxylation at its isopentenyl group, leading to the formation of 120. Subsequently, 120 undergoes cyclization catalyzed by the terpenoid cyclase AscI, followed by oxidation by AscJ, resulting in the production of 116 (Figure 11B; Araki et al., 2019).
2.3.2 Chrodrimanins, verruculides, and talaromyides
Chrodrimanins, verruculides, and talaromyides, polycyclic meroterpenoids with a seco-drimane unit and an isocoumarin core, have been isolated from marine-derived fungi Talaromyces sp. CX11 (Cao et al., 2019), Talaromyces purpureogenus (Cao et al., 2020), Penicillium sp. SCS-KFD09 associated with the marine worm Sipunculus nudus (Kong et al., 2017), and ascidian-derived Penicillium verruculosum TPU1311 (Yamazaki et al., 2015). Talaromyolide D (121) exhibits potent antiviral activity against pseudorabies virus (PRV) with a CC50 of 3.35 μM (Cao et al., 2019), while talaromyolide I (122) and K (123) show dose-dependent inhibition of PRV, with 123 demonstrating the most significant effects at 50 mg/mL (Cao et al., 2020). Chrodrimanin O (124), R (125), S (126), verruculide A (127), and chrodrimanin A (128), B (129), and H (130) exhibit protein tyrosine phosphatase 1B (PTP1B) inhibitory activity, with IC50 values ranging from 71.6 to 8.4 μM, suggesting potential for development as drugs targeting type 2 diabetes or obesity (Kong et al., 2017, Yamazaki et al., 2015).
The BGC responsible for 129, designated as the cdm cluster, underwent characterization through whole genome sequencing, heterologous reconstitution in A. oryzae, and in vitro enzyme reactions. Initially, the PKS CdmE, serving as the 6-hydroxymellein synthase, was expressed in A. oryzae, resulting in the production of 131. Co-expression of cdmE with the prenyltransferase gene cdmH yielded the hydrophobic metabolite verruculide C (132). Subsequent incorporation of the FMO gene cdmI yielded verruculide B (133), while introduction of the terpene cyclase gene cdmG generated the pentacyclic molecule 3-hydroxypentacecilide A (134). Integration of cdmF into A. oryzae producing compound 134 led to the production of chrodrimanin C (135), confirming CdmF as a 3-hydroxy dehydrogenase. Additionally, the Fe(II)/α-ketoglutarate (αKG)-dependent dioxygenase CdmA exhibited dehydrogenation activity between C-1 and C-2 in 135 and 130, resulting in the formation of 127 and chrodrimanin E (136). Furthermore, CdmD, another Fe(II)/αKG-dependent dioxygenase, catalyzed β-hydroxylation at C-7′ to produce chrodrimanin T (137) and 128. The cytochrome P450 monooxygenase CdmJ accepted compounds 127, 134, 135 and 137 as substrates, acting as a C-7 β-hydroxylase to produce chrodrimanin F (138), 130, 136 and 128, respectively. Finally, the acetyltransferase CdmC converted compound 128 into the final product 129 (Figure 12A; Bai et al., 2018).
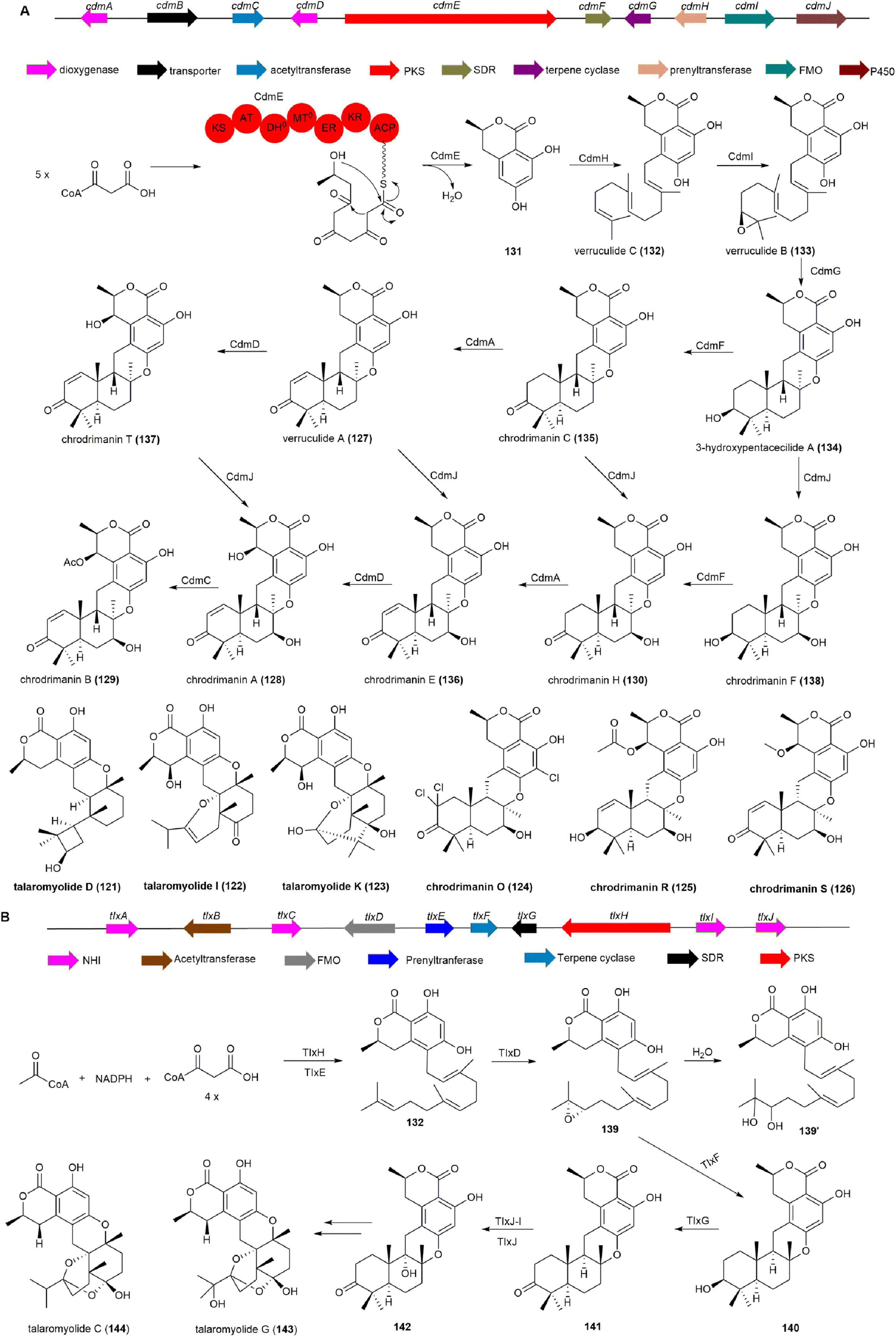
Figure 12. (A) The BGC, biosynthetic pathway and structures of chrodrimanins, verruculides and talaromyides. (B) The BGC and biosynthetic pathway of talaromyolide G (143) and C (144).
The BGC responsible for the production of talaromyolides, a unique group of 6/6/6/6/6/6 hexacyclic meroterpenoids, was identified in the marine fungus T. purpureogenus and designated as the tlx cluster. As expected, compound 131, a precursor molecule of talaromyolides, was produced in A. oryzae harboring the PKS TlxH, which shares 66% homology with CdmE (Bai et al., 2018). Coexpression of tlxH and tlxE led to the production of 132. Subsequent introduction of tlxD in A. oryzae harboring tlxHE resulted in the production of dihydroxyfarnesyl-9 (139′) presumably derived from 139 by epoxide opening through attack of water. The transformant expressing tlxHEDF yielded 140, Further introduction of tlxG in the A. oryzae harboring tlxHEDF resulted in the generation of 141. Ultimately, the heterodimer of non-heme iron (NHI) enzyme TlxJ catalyzed the hydroxylation of 141 at C-9α to produce 142, and co-incubation with TlxI efficiently yielded the target products talaromyolide G (143) and C (144) (Figure 12B; Li et al., 2021).
2.4 Non-ribosomal peptides
2.4.1 Gliotoxin
Gliotoxin (145), featuring a diketopiperazine core with a disulfide bridge, is isolated from various fungal species, including marine fungus Neosartorya pseudofischeri found in the inner tissue of the starfish Acanthaster planci (Liang et al., 2014, Scharf et al., 2016). Compound 145 exhibits a diverse range of biological activities, such as antimicrobial, antifungal, antiviral, and immunomodulating properties (Scharf et al., 2016, Waring and Beaver, 1996). 145 and dithiol gliotoxin (146) show significant inhibitory activity against Gram-positive Staphylococcus aureus (ATCC29213) and methicillin-resistant Staphylococcus aureus (R3708), as well as Gram-negative Escherichia coli (ATCC25922), with MIC values ranging from 1.52 to 97.56 μM, and notably exhibit potent inhibition against Staphylococcus aureus R3708 with MIC values of 1.53 and 1.52 μM, respectively (Liang et al., 2014). Structure-activity relationship analysis suggests that the disulfide bridge or its reduced form is essential for antibacterial activity, which is influenced by modifications on the six-membered ring with two conjugated double bonds, where a hydroxyl group at C-6 enhances activity compared to an acetyl group. The α-methylene ketone group is also crucial for antibacterial activity (Liang et al., 2014). Furthermore, 145 and 146 also demonstrate excellent cytotoxic activity against the human embryonic kidney (HEK) 293 cell line and human colon cancer cell lines, HCT-116 and RKO, with IC50 values of 0.41 and 1.58 μM, respectively (Liang et al., 2014, Watts et al., 2010, Waring et al., 1995, Waring and Beaver, 1996).
The BGC of 145, known as gli and consisting of 13 genes, was identified by the Howlett group through whole genome sequencing and bioinformatics analysis of Aspergillus fumigatus (Gardiner and Howlett, 2005). Within this cluster, GliZ, a transcription factor, upregulates gliotoxin biosynthesis (Bok et al., 2006). Furthermore, the NRPS GliP catalyzes the production of the precursor 147 (Balibar and Walsh, 2006), which is subsequently hydroxylated by GliC to form 148 (Chang et al., 2013). Additionally, GliG, a glutathione S-transferase, catalyzes the formation of 149 from 148 and two molecules of glutathione, providing the sulfur source for 145 (Davis et al., 2011, Scharf et al., 2011). Then, glutamic acid transferase GliK removes glutamyl to generate 150, which is further modified by GliI and methyltransferase GliN to produce 146 (Gallagher et al., 2012, Scharf et al., 2013, Scharf et al., 2012). Finally, the oxidoreductase GliT catalyzes the formation of disulfide bridges, yielding the final product 145 (Figure 13A; Scharf et al., 2014).
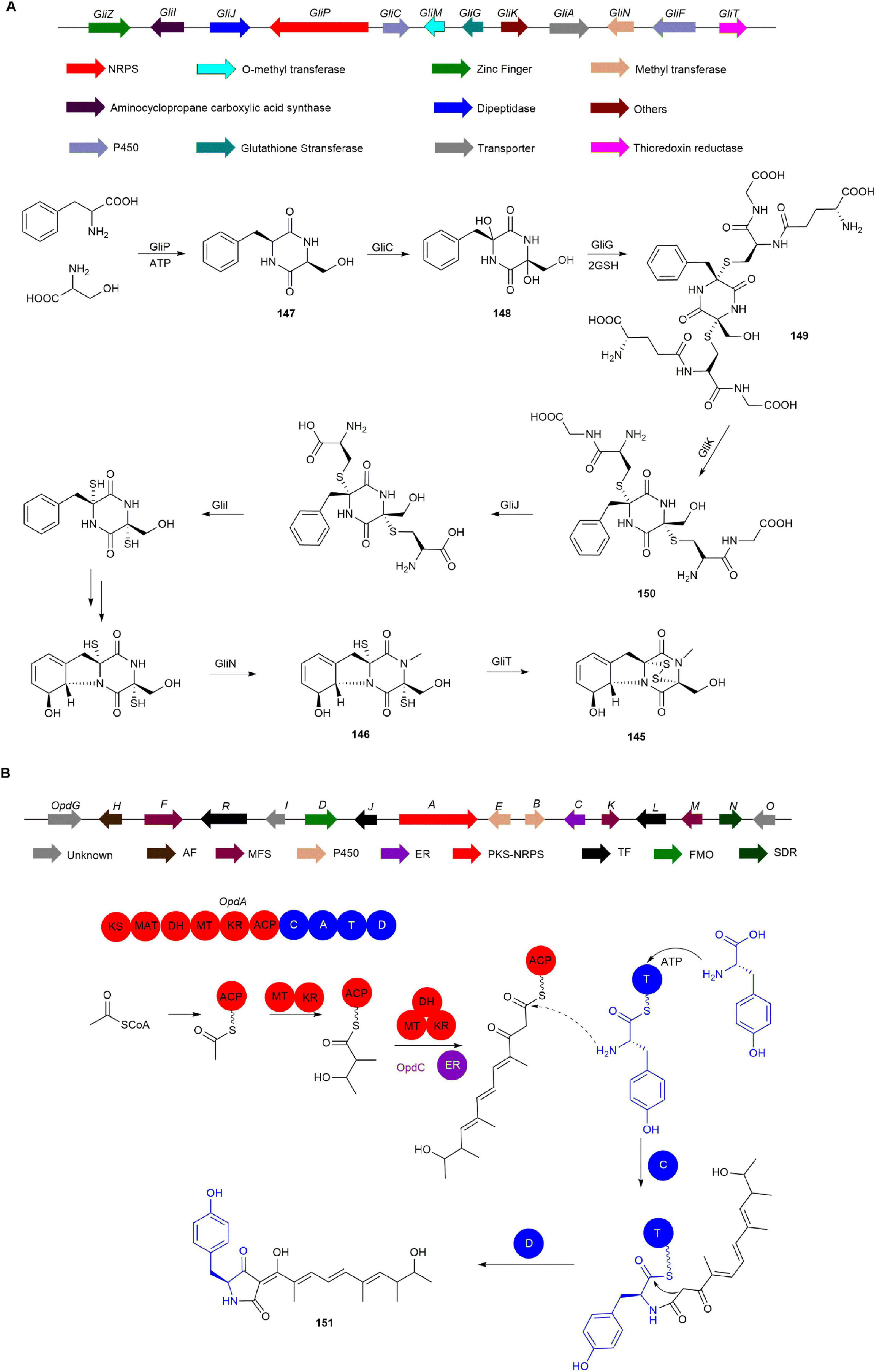
Figure 13. The BGCs and biosynthetic pathways of (A) gliotoxin (145) and (B) oxopyrrolidine A (151).
2.4.2 Oxopyrrolidines
Tetramic acid derivatives, featuring a pyrrolidine-2,4-dione moiety, are crucial in medicinal chemistry and biochemistry due to their antibiotic (Segeth et al., 2003), antifungal (Sata et al., 1999), and cytotoxic activities (Holzapfel, 1968). Oxopyrrolidines, a type of tetramic acid derivative, have been isolated from the marine-derived fungus Penicillium oxalicum MEFC104 (Li et al., 2022).
Bioinformatic analysis of the aspyridone gene cluster, which has a structure similar to oxopyrrolidine A (151), identified the candidate opd gene cluster responsible for 151 production in P. oxalicum MEFC104 (Bergmann et al., 2007). The opd cluster was further confirmed by inactivating the PKS-NRPS gene opdA, resulting in a mutant that lost the ability to produce 151. Further analysis of the 16 genes within the opd BGC identified OpdJ, OpdL, and OpdR as transcription factors. 151 was absent in the ΔopdJ mutant, while mutants without opdL or opdR showed no significant changes, indicating that OpdJ is the cluster-specific transcription factor regulating the opd cluster. Deletion of the MFS transporter genes opdF, opdK, and opdM did not affect 151 biosynthesis, suggesting these transporters are not involved in 151 production. Of the remaining eight genes (opdBCDEGNOI), only the ΔopdC mutant completely lost the ability to produce 151, with no accumulation of intermediates. This indicates that OpdC acts as a trans-acting ER essential for the reduction step in the polyketide assembly process. Thus, the biosynthesis of 151 primarily relies on the actions of OpdA and OpdC (Figure 13B; Li et al., 2022).
2.4.3 Psychrophilins
Psychrophilins, featuring a rare amide linkage between the carboxylic acid in anthranilic acid (ATA) and the nitrogen from an indole moiety, were isolated from the marine-derived fungus Aspergillus versicolor ZLN-60 and marine algae-derived fungi of the genus Aspergillus (Ebada et al., 2014, Peng et al., 2014). Psychrophilin G (152) exhibits potent lipid-lowering effects in HepG2 hepatocarcinoma cells (IC50 = 10 μg/mL) (Peng et al., 2014). Psychrophilin E (153) shows strong anti-proliferative activity against the HCT116 (colon) cell line (IC50 = 28.5 μg/mL) with high selectivity and demonstrates more potent cytotoxic activity than cisplatin, a clinically used chemotherapeutic agent (IC50 = 33.4 μg/mL) (Ebada et al., 2014, Ngen et al., 2016).
Sequencing the genome of the psychrophilin B (154) producing fungus Penicillium rivulum revealed two candidate BGCs encoded in scaffold 46 and scaffold 182 as likely involved in 154 biosynthesis (Dalsgaard et al., 2004, Zhao et al., 2016). Gene knockout of scaffold 46 using homologous recombination resulted in the complete abolishment of 154 production, confirming scaffold 46 as the responsible gene cluster, named psy. The psy cluster contains two independent NRPS coding genes psyA and psyB. Single-gene knockouts of psyA and psyB resulted in the complete loss of 154 production, with no related intermediates detected. However, in the P450 deletion strain ΔpsyC, psychrophilin I (155) was detected. Feeding 155 to the ΔpsyA and ΔpsyB strains led to the detection of the target 154, suggesting 155 as the penultimate intermediate in 154 biosynthesis. Based on genetic inactivation and chemical complementation studies, the proposed biosynthetic pathway for 154 is as follows: the dimodular NRPS PsyA incorporates L-Trp and L-Val to yield the L-Trp–L-Val dipeptidyl thioester (156). The monomodular NRPS PsyB activates Ant, which is then condensed with 156 by the terminal C domain in PsyA to yield the tripeptidyl thioester (157). The CT domain of PsyB utilizes the indole nitrogen in a nucleophilic attack of the thioester to release 155, which is then catalyzed by P450 PsyC to form 154 (Figure 14A; Zhao et al., 2016).
2.4.4 Asperalins
Asperalins, viridicatin-type quinolone alkaloids, are significant natural products with various biological activities, including insecticidal (Uchida et al., 2006), antibacterial (Hu et al., 2023), antifungal (Mousa et al., 2015), antitumor (Larsen et al., 2002, He et al., 2005), and antiviral properties (Chen et al., 2014). Recently, Gao group isolated novel asperalins from the seagrass-derived fungus Aspergillus alabamensis SYSU-6778, which exhibit moderate to potent inhibitory effects against fish pathogenic bacteria, such as Edwardsiella ictaluri, Streptococcus iniae, and Streptococcus parauberis (Hu et al., 2023).
The asperalins BGC, named as apl, from A. alabamensis SYSU-6778 was confirmed via heterologous expression in A. oryzae NSAR1, incorporating aspects of viridicatin-type quinolone alkaloid biosynthesis (Kishimoto et al., 2018, Zou et al., 2017, Zou et al., 2015). Heterologous expression of AplLCK in A. oryzae NSAR1resulted in the detection of 160, 161, 162 and viridicatin (163), indicating that the pathway of asperalins initiates with the dual-module NRPS aplL. AplL catalyzes the condensation of o-aminobenzoic acid (158) and L-phenylalanine (159) to form 160, which is then converted by the dioxygenase aplC into 161 and subsequently epoxidized to form 162. A zinc-dependent protein aplK facilitates the ring contraction of 162, producing 163 through the elimination of methyl isocyanate. Feeding 163 into AO-AplB constructs results in aflaquinolone G (164), generated by hydroxylation via the FAD-dependent monooxygenase aplB. The NRPS aplJ transforms 164 into asperalin G (165), which is subsequently processed by the P450 enzyme aplF into asperalin H (166). Compound 166 undergoes O-prenylation by aplE to produce asperalin A (167), while chlorase aplN converts both 167 into asperalin D (168) and 166 into asperalin F (169) (Figure 14B; Zeng et al., 2024).
2.5 Alkaloids
2.5.1 Isoindolinones
Isoindolinones, isolated from the marine fungus Stachybotrys longispora FG216, are known for their potent plasminogen-activating properties (Shinohara et al., 1996, Hu et al., 2001, Hasegawa et al., 2010, Koide et al., 2012, Yin et al., 2017). Isoindolinones exhibit strong fibrinolytic effects and have shown promising results in treating thrombotic strokes in primates, enhancing thrombolysis and minimizing hemorrhagic activity (Hasegawa et al., 2010, Hu et al., 2012). Consequently, isoindolinones hold significant potential for the development of cardiovascular drugs (Sawada et al., 2014, Yan et al., 2015).
Ilicicolin B (170), synthesized by NR-PKS StbA, UbiA-like prenyltransferase StbC, and NRPS-like enzyme StbB in Stachybotrys bisbyi PYH05-7, is the precursor of all isoindolinone derivatives (Nishimura et al., 2012, Li et al., 2016). Based on these core genes (stbABC), the BGC of isoindolinones was identified in S. longispora FG216 through genome mining (Yin et al., 2017). The biosynthetic pathway of isoindolinones, as deduced from bioinformatics analysis, starts with the synthesis of orsellinic acid (117) by NR-PKS IdlA, followed by the transfer of farnesyl pyrophosphate (FPP) by PT IdlC to form ilicicolin acid (118), which is then converted by NRPS IdlB into 170. Finally, through epoxidation, cyclization, and oxidation steps, the phthalic aldehyde precursor (171) is formed, which combines with ammonium ions or amino compounds to produce various isoindolinones (Figure 15; Yin et al., 2017).
3 Conclusion
MFNPs represent a significant source of pharmaceuticals, exhibiting remarkable bioactivity and therapeutic potential. With the rapid advancement of genomic sequencing technologies, genome mining has emerged as a crucial strategy for discovering new MFNPs. The BGCs was primarily identified by comparative transcriptome analysis (ophiobolins, ascochlorin and ascofuranone) and bioinformatic analysis of the sequenced genome of producing strains. Heterologous expression in Saccharomyces cerevisiae, Aspergillus nidulans, and Aspergillus oryzae, along with gene knockout techniques in producing strains, are essential for unlocking these dormant biosynthetic pathways. The majority of these MFNPs discussed in this review are derived from the genera Penicillium (griseofulvin, sorbicillinoids, monodictyphenone, chrysoxanthones, penilactones A, penilactones B, chrodrimanins, verruculides, talaromyides, penifulvin A and oxopyrrolidines) and Aspergillus (epicospirocins, chevalone E, ophiobolins, aspergildienes, aspergilols, asperaculin A, talaronoids, psychrophilins and asperalins). The pharmacological activities of these MFNPs are prominently featured in anti-inflammatory activities (flavoglaucin, dihydroauroglaucin, isodihydroauroglaucin, sorbicillinoids, amphichopyrone A, amphichopyrone B, penilactones A, ascochlorin, spiromeroterpenes D-F), cytotoxic activities (flavoglaucin, aspermicrones B, phomoxanthone A, oxopyrrolidines, psychrophilin G, psychrophilin E, ophiobolins and aspergilols), and antimicrobial activities (griseofulvin, monodictyphenone, aspermicrone B, aspermicrone C, chrysoxanthones A-C, phomoxanthone A, chevalone E, ascochlorin, gliotoxin, oxopyrrolidines). The research efforts outlined in this review offer valuable perspectives for future gene-guided mining and analysis of biosynthetic pathways in MFNPs.
4 Discussion and outlook
MFNPs represent a rich source of structurally diverse bioactive compounds with significant therapeutic potential. Notable examples, such as ziconotide (Prialt), trabectedin (Yondelis), and lurbinectedin (Zepzelca), are marine-derived drugs that continue to offer substantial benefits to human health. However, the discovery of novel MFNPs has been hindered by challenges in current discovery technologies, cultivation methods, and screening models, which often lack integration with genomic approaches (Atanasov et al., 2021). Consequently, MFNPs remain underexplored relative to their synthetic counterparts, limiting their full potential in drug development. Recent advances in genome mining, including gene editing, gene synthesis, and heterologous expression systems, have revolutionized the discovery of marine fungal natural products (MFNPs) by enabling the identification of previously cryptic BGCs (Costantini, 2020, Wei et al., 2021a). The increasing availability of sequenced marine fungal genomes has uncovered a wealth of untapped BGCs, and when coupled with advanced bioinformatics tools, these resources significantly enhance the efficiency of bioactive MFNPs identification. Moreover, the elucidation of biosynthetic pathways lays the groundwork for metabolic engineering strategies that can optimize the production of these compounds, addressing the low natural yields often encountered in MFNP discovery.
Despite these advancements, several challenges persist in genome mining: (1) Some BGCs remain silent, even with multiple activation strategies. (2) Current bioinformatics tools like AntiSMASH and 2nFinder, while invaluable, still fail to predict all critical genes or enzymes with novel functions. (3) Gene manipulation in wild-type strains is hindered by difficulties in protoplast preparation, limiting genetic modification options. Overcoming these challenges requires the development of more robust bioinformatic tools, improved BGC activation methods, and advanced genetic techniques tailored to filamentous fungi. The rapid development and integration of technologies such as gene editing, directed evolution, artificial intelligence (AI), AlphaFold, de novo protein design, and synthetic biology provide unprecedented opportunities, significantly accelerating research and application in MFNPs (Atanasov et al., 2021). Bioinformatics and AI have further enabled the rational design, analysis, and modification of key biosynthetic genes for MFNPs production. The activation of silent BGCs, optimization of production conditions, and application of metabolic engineering to enhance MFNPs yields will be critical in advancing MFNPs discovery. Interdisciplinary approaches that bridge genomics, chemistry, and pharmacology will be essential for translating these findings into clinical applications. By overcoming the remaining challenges in genome mining, the full potential of marine fungi as a source of novel bioactive molecules can be realized, paving the way for the next generation of marine-derived therapeutics.
Author contributions
CH: Formal analysis, Software, Writing – original draft. AS: Formal analysis, Writing – original draft. YH: Investigation, Writing – original draft. LY: Writing – original draft. LC: Writing – original draft. WD: Writing – original draft. QW: Writing – original draft. SY: Data curation, Formal analysis, Funding acquisition, Investigation, Methodology, Project administration, Resources, Software, Supervision, Validation, Visualization, Writing – original draft, Writing – review and editing.
Funding
The authors declare that financial support was received for the research, authorship, and/or publication of this article. This work was financially supported by the Youth Science and Technology Talent Development Project of the Guizhou Provincial Department of Education (No. Qian Jiao Ji [2024] 135), the Zhuhai Campus Technology Innovation Team of Zunyi Medical University (No. ZHTD2024-3), and the Guizhou provincial Science and Technology Projects (No. Qian Ke He Jichu-[2024] Qingnian 308).
Conflict of interest
The authors declare that the research was conducted in the absence of any commercial or financial relationships that could be construed as a potential conflict of interest.
Generative AI statement
The authors declare that no Generative AI was used in the creation of this manuscript.
Publisher’s note
All claims expressed in this article are solely those of the authors and do not necessarily represent those of their affiliated organizations, or those of the publisher, the editors and the reviewers. Any product that may be evaluated in this article, or claim that may be made by its manufacturer, is not guaranteed or endorsed by the publisher.
References
Andrade, R., Ayer, W. A., and Mebe, P. P. (1992). The metabolites of Trichoderma longibrachiatum. Part 1. Isolation of the metabolites and the structure of trichodimerol. Can. J. Chem. 70, 2526–2535. doi: 10.1139/v92-320
Araki, Y., Awakawa, T., Matsuzaki, M., Cho, R., Matsuda, Y., Hoshino, S., et al. (2019). Complete biosynthetic pathways of ascofuranone and ascochlorin in Acremonium egyptiacum. Proc. Natl. Acad. Sci. U.S.A. 116, 8269–8274. doi: 10.1073/pnas.1819254116
Atanasov, A. G., Zotchev, S. B., and Dirsch, V. M., The International Natural Product Sciences, and Supuran, C. T. (2021). Natural products in drug discovery: advances and opportunities. Nat. Rev. Drug Discov. 20, 200–216. doi: 10.1038/s41573-020-00114-z
Bai, T. X., Quan, Z. Y., Zhai, R., Awakawa, T., Matsuda, Y., and Abe, I. (2018). Elucidation and heterologous reconstitution of chrodrimanin B biosynthesis. Org. Lett. 20, 7504–7508. doi: 10.1021/acs.orglett.8b03268
Balibar, C. J., and Walsh, C. T. (2006). GliP, a multimodular nonribosomal peptide synthetase in Aspergillus fumigatus, makes the diketopiperazine scaffold of gliotoxin. Biochemistry 45, 15029–15038. doi: 10.1021/bi061845b
Banerjee, P., Mandhare, A., and Bagalkote, V. (2022). Marine natural products as source of new drugs: an updated patent review (July 2018-July 2021). Expert Opin. Ther. Pat. 32, 317–363. doi: 10.1080/13543776.2022.2012150
Bergmann, S., Schumann, J., Scherlach, K., Lange, C., Brakhage, A. A., and Hertweck, C. (2007). Genomics-driven discovery of PKS-NRPS hybrid metabolites from Aspergillus nidulans. Nat. Chem. Biol. 3, 213–217. doi: 10.1038/nchembio869
Biggins, J. B., Liu, X., Feng, Z., and Brady, S. F. (2011). Metabolites from the induced expression of cryptic single operons found in the genome of Burkholderia pseudomallei. J. Am. Chem. Soc. 133, 1638–1641. doi: 10.1021/ja1087369
Bladt, T. T., Durr, C., Knudsen, P. B., Kildgaard, S., Frisvad, J. C., Gotfredsen, C. H., et al. (2013). Bio-activity and dereplication-based discovery of ophiobolins and other fungal secondary metabolites targeting leukemia cells. Molecules 18, 14629–14650. doi: 10.3390/molecules181214629
Blunt, J. W., Carroll, A. R., Copp, B. R., Davis, R. A., Keyzers, R. A., and Prinsep, M. R. (2018). Marine natural products. Nat. Prod. Rep. 35, 8–53. doi: 10.1039/C7NP00052A
Bok, J. W., Chung, D., Balajee, S. A., Marr, K. A., Andes, D., Nielsen, K. F., et al. (2006). GliZ, a transcriptional regulator of gliotoxin biosynthesis, contributes to Aspergillus fumigatus virulence. Infect. Immun. 74, 6761–6768. doi: 10.1128/IAI.00780-06
Burkhardt, I., Siemon, T., Henrot, M., Studt, L., Rosler, S., Tudzynski, B., et al. (2016). Mechanistic characterisation of two sesquiterpene cyclases from the plant pathogenic fungus Fusarium fujikuroi. Angew Chem. Int. Ed. Engl. 55, 8748–8751. doi: 10.1002/anie.201603782
Cacho, R. A., Chooi, Y. H., Zhou, H., and Tang, Y. (2013). Complexity generation in fungal polyketide biosynthesis: a spirocycle-forming P450 in the concise pathway to the antifungal drug griseofulvin. ACS Chem. Biol. 8, 2322–2330. doi: 10.1021/cb400541z
Cao, X., Shi, Y. T., Wu, X. D., Wang, K. W., Huang, S. H., Sun, H. X., et al. (2019). Talaromyolides A-D and talaromytin: polycyclic meroterpenoids from the fungus Talaromyces sp. CX11. Org. Lett. 21, 6539–6542. doi: 10.1021/acs.orglett.9b02466
Cao, X., Shi, Y., Wu, S., Wu, X., Wang, K., Sun, H., et al. (2020). Polycyclic meroterpenoids, talaromyolides E - K for antiviral activity against pseudorabies virus from the endophytic fungus Talaromyces purpureogenus. Tetrahedron 76:131349. doi: 10.1016/j.tet.2020.131349
Carroll, A. R., Copp, B. R., Davis, R. A., Keyzers, R. A., and Prinsep, M. R. (2019). Marine natural products. Nat. Prod. Rep. 36, 122–173. doi: 10.1039/C8NP00092A
Carroll, A. R., Copp, B. R., Davis, R. A., Keyzers, R. A., and Prinsep, M. R. (2020). Marine natural products. Nat. Prod. Rep. 37, 175–223. doi: 10.1039/c9np00069k
Carroll, A. R., Copp, B. R., Davis, R. A., Keyzers, R. A., and Prinsep, M. R. (2021). Marine natural products. Nat. Prod. Rep. 38, 362–413. doi: 10.1039/d0np00089b
Carroll, A. R., Copp, B. R., Davis, R. A., Keyzers, R. A., and Prinsep, M. R. (2022). Marine natural products. Nat. Prod. Rep. 39, 1122–1171. doi: 10.1039/d1np00076d
Carroll, A. R., Copp, B. R., Davis, R. A., Keyzers, R. A., and Prinsep, M. R. (2023). Marine natural products. Nat. Prod. Rep. 40, 275–325. doi: 10.1039/d2np00083k
Carroll, A. R., Copp, B. R., Grkovic, T., Keyzers, R. A., and Prinsep, M. R. (2024). Marine natural products. Nat. Prod. Rep. 41, 162–207. doi: 10.1039/d3np00061c
Chai, H. Z., Yin, R., Liu, Y. F., Meng, H. Y., Zhou, X. Q., Zhou, G. L., et al. (2016). Sesterterpene ophiobolin biosynthesis involving multiple gene clusters in Aspergillus ustus. Sci. Rep. 6:27181. doi: 10.1038/srep27181
Chang, S. L., Chiang, Y. M., Yeh, H. H., Wu, T. K., and Wang, C. C. (2013). Reconstitution of the early steps of gliotoxin biosynthesis in Aspergillus nidulans reveals the role of the monooxygenase GliC. Bioorg. Med. Chem. Lett. 23, 2155–2157. doi: 10.1016/j.bmcl.2013.01.099
Chen, M., Shao, C. L., Meng, H., She, Z. G., and Wang, C. Y. (2014). Anti-respiratory syncytial virus prenylated dihydroquinolone derivatives from the gorgonian-derived fungus Aspergillus sp. XS-20090B15. J. Nat. Prod. 77, 2720–2724. doi: 10.1021/np500650t
Chen, S., Guo, H., Jiang, M., Wu, Q., Li, J., Shen, H., et al. (2022a). Mono- and dimeric xanthones with anti-glioma and anti-inflammatory activities from the ascidian-derived fungus diaporthe sp. SYSU-MS4722. Mar. Drugs 20:51. doi: 10.3390/md20010051
Chen, S., Guo, H., Wu, Z., Wu, Q., Jiang, M., Li, H., et al. (2022b). Targeted discovery of sorbicillinoid pigments with anti-inflammatory activity from the sponge-derived fungus Stagonospora sp. SYSU-MS7888 using the PMG strategy. J. Agric. Food Chem. 70, 15116–15125. doi: 10.1021/acs.jafc.2c05940
Chiang, Y.-M., Szewczyk, E., Davidson, A. D., Entwistle, R., Keller, N. P., Wang, C. C. C., et al. (2010). Characterization of the Aspergillus nidulans monodictyphenone gene cluster. Appl. Environ. Microbiol. 76, 2067–2074. doi: 10.1128/aem.02187-09
Chiba, R., Minami, A., Gomi, K., and Oikawa, H. (2013). Identification of ophiobolin F synthase by a genome mining approach: a sesterterpene synthase from Aspergillus clavatus. Org. Lett. 15, 594–597. doi: 10.1021/ol303408a
Chooi, Y. H., Cacho, R., and Tang, Y. (2010). Identification of the viridicatumtoxin and griseofulvin gene clusters from Penicillium aethiopicum. Chem. Biol. 17, 483–494. doi: 10.1016/j.chembiol.2010.03.015
Costantini, M. (2020). Genome mining and synthetic biology in marine natural product discovery. Mar. Drugs 18:615. doi: 10.3390/md18120615
Dai, G., Shen, Q., Zhang, Y., and Bian, X. (2022). Biosynthesis of fungal natural products involving two separate pathway crosstalk. J. Fungi 8:320. doi: 10.3390/jof8030320
Dalsgaard, P. W., Blunt, J. W., Munro, M. H., Larsen, T. O., and Christophersen, C. (2004). Psychrophilin B and C: cyclic nitropeptides from the psychrotolerant fungus Penicillium rivulum. J. Nat. Prod. 67, 1950–1952. doi: 10.1021/np0497954
Das, D., and Chakraborty, T. K. (2016). An overview of the recent synthetic studies toward penifulvins and other fenestranes. Tetrahedron Lett. 57, 3665–3677. doi: 10.1016/j.tetlet.2016.07.011
Davis, C., Carberry, S., Schrettl, M., Singh, I., Stephens, J. C., Barry, S. M., et al. (2011). The role of glutathione S-transferase GliG in gliotoxin biosynthesis in Aspergillus fumigatus. Chem. Biol. 18, 542–552. doi: 10.1016/j.chembiol.2010.12.022
De Carli, L., and Larizza, L. (1988). Griseofulvin. Mutat. Res. 195, 91–126. doi: 10.1016/0165-1110(88)90020-6
Derntl, C., Guzmán-Chávez, F., Mello-de-Sousa, T. M., Busse, H.-J., Driessen, A. J. M., Mach, R. L., et al. (2017). In Vivo study of the sorbicillinoid gene cluster in Trichoderma reesei. Front. Microbiol. 8:2037. doi: 10.3389/fmicb.2017.02037
Ebada, S. S., Fischer, T., Hamacher, A., Du, F. Y., Roth, Y. O., Kassack, M. U., et al. (2014). Psychrophilin E, a new cyclotripeptide, from co-fermentation of two marine alga-derived fungi of the genus Aspergillus. Nat. Prod. Res. 28, 776–781. doi: 10.1080/14786419.2014.880911
Elsässer, B., Krohn, K., Flörke, U., Root, N., Aust, H. J., Draeger, S., et al. (2005). X-ray structure determination, absolute configuration and biological activity of phomoxanthone A. Eur. J. Organ. Chem. 2005, 4563–4570. doi: 10.1002/ejoc.200500265
Fahad, A. A., Abood, A., Fisch, K. M., Osipow, A., Davison, J., Avramovic, M., et al. (2014). Oxidative dearomatisation: the key step of sorbicillinoid biosynthesis. Chem. Sci. 5, 523–527. doi: 10.1039/c3sc52911h
Fan, A., Mi, W., Liu, Z., Zeng, G., Zhang, P., Hu, Y., et al. (2017). Deletion of a histone acetyltransferase leads to the pleiotropic activation of natural products in Metarhizium robertsii. Org. Lett. 19, 1686–1689. doi: 10.1021/acs.orglett.7b00476
Fan, J., Liao, G., Kindinger, F., Ludwig-Radtke, L., Yin, W. B., and Li, S. M. (2019). Peniphenone and penilactone formation in Penicillium crustosum via 1,4-Michael additions of ortho-quinone methide from hydroxyclavatol to gamma-butyrolactones from crustosic acid. J. Am. Chem. Soc. 141, 4225–4229. doi: 10.1021/jacs.9b00110
Fan, J., Liao, G., Ludwig-Radtke, L., Yin, W. B., and Li, S. M. (2020). Formation of terrestric acid in Penicillium crustosum requires redox-assisted decarboxylation and stereoisomerization. Org. Lett. 22, 88–92. doi: 10.1021/acs.orglett.9b04002
Frank, M., Niemann, H., Bohler, P., Stork, B., Wesselborg, S., Lin, W., et al. (2015). Phomoxanthone A–from mangrove forests to anticancer therapy. Curr. Med. Chem. 22, 3523–3532. doi: 10.2174/0929867322666150716115300
Gallagher, L., Owens, R. A., Dolan, S. K., O’Keeffe, G., Schrettl, M., Kavanagh, K., et al. (2012). The Aspergillus fumigatus protein GliK protects against oxidative stress and is essential for gliotoxin biosynthesis. Eukaryot Cell 11, 1226–1238. doi: 10.1128/EC.00113-12
Gardiner, D. M., and Howlett, B. J. (2005). Bioinformatic and expression analysis of the putative gliotoxin biosynthetic gene cluster of Aspergillus fumigatus. FEMS Microbiol. Lett. 248, 241–248. doi: 10.1016/j.femsle.2005.05.046
George, I. R., Lopez-Tena, M., Sundin, A. P., and Strand, D. (2021). A unifying bioinspired synthesis of (-)-asperaculin A and (-)-penifulvin D. Org. Lett. 23, 3536–3540. doi: 10.1021/acs.orglett.1c00955
Griffiths, S., Mesarich, C. H., Saccomanno, B., Vaisberg, A., De Wit, P. J., Cox, R., et al. (2016). Elucidation of cladofulvin biosynthesis reveals a cytochrome P450 monooxygenase required for anthraquinone dimerization. Proc. Natl. Acad. Sci. U.S.A. 113, 6851–6856. doi: 10.1073/pnas.1603528113
Guo, J. H., Cai, Y. S., Cheng, F. C., Yang, C. J., Zhang, W. Q., Yu, W. L., et al. (2021). Genome mining reveals a multiproduct sesterterpenoid biosynthetic gene cluster in Aspergillus ustus. Org. Lett. 23, 1525–1529. doi: 10.1021/acs.orglett.0c03996
Guo, X., Meng, Q. Y., Niu, S. W., Liu, J., Guo, X. C., Sun, Z. L., et al. (2021). Epigenetic manipulation to trigger production of guaiane-type sesquiterpenes from a marine-derived Spiromastix sp. fungus with antineuroinflammatory effects. J. Nat. Prod. 84, 1993–2003. doi: 10.1021/acs.jnatprod.1c00293
Harned, A. M., and Volp, K. A. (2011). The sorbicillinoid family of natural products: isolation, biosynthesis, and synthetic studies. Nat. Prod. Rep. 28, 1790–1810. doi: 10.1039/c1np00039j
Harris, C. M., Roberson, J. S., and Harris, T. M. (1976). Biosynthesis of griseofulvin. J. Am. Chem. Soc. 98, 5380–5386. doi: 10.1021/ja00433a053
Hasegawa, K., Koide, H., Hu, W. M., Nishimura, N., Narasaki, R., Kitano, Y., et al. (2010). Structure-activity relationships of 11 new congeners of the SMTP plasminogen modulator. J. Antibiot (Tokyo) 63, 589–593. doi: 10.1038/ja.2010.101
He, J., Lion, U., Sattler, I., Gollmick, F. A., Grabley, S., Cai, J. M., et al. (2005). Diastereomeric quinolinone alkaloids from the marine-derived fungus Penicillium janczewskii. J. Nat. Prod. 68, 1397–1399. doi: 10.1021/np058018g
Holzapfel, C. W. (1968). The isolation and structure of cyclopiazonic acid, a toxic metabolite of Penicillium cyclopium Westling. Tetrahedron 24, 2101–2119. doi: 10.1016/0040-4020(68)88113-x
Hu, W. M., Narasaki, R., Nishimura, N., and Hasumi, K. (2012). SMTP (Stachybotrys microspora triprenyl phenol) enhances clot clearance in a pulmonary embolism model in rats. Thromb. J. 10:2. doi: 10.1186/1477-9560-10-2
Hu, W., Narasaki, R., Ohyama, S., and Hasumi, K. (2001). Selective production of staplabin and SMTPs in cultures of Stachybotrys microspora fed with precursor amines. J. Antibiot (Tokyo) 54, 962–966. doi: 10.7164/antibiotics.54.962
Hu, Y. W., Zhao, X. Y., Song, Y., Jiang, J. H., Long, T., Cong, M. J., et al. (2024). Anti-inflammatory and neuroprotective alpha-pyrones from a marine-derived strain of the fungus Arthrinium arundinis and their heterologous expression. J. Nat. Prod. 87, 1975–1982. doi: 10.1021/acs.jnatprod.4c00393
Hu, Z. B., Zhu, Y. J., Chen, J. J., Chen, J., Li, C. Y., Gao, Z. Z., et al. (2023). Discovery of novel bactericides from Aspergillus alabamensis and their antibacterial activity against fish pathogens. J. Agric. Food Chem. 71, 4298–4305. doi: 10.1021/acs.jafc.2c09141
Ingavat, N., Mahidol, C., Ruchirawat, S., and Kittakoop, P. (2011). Asperaculin A, a sesquiterpenoid from a marine-derived fungus, Aspergillus aculeatus. J. Nat. Prod. 74, 1650–1652. doi: 10.1021/np200221w
Kahlert, L., Bassiony, E. F., Cox, R. J., and Skellam, E. J. (2020). Diels-Alder reactions during the biosynthesis of sorbicillinoids. Angew Chem. Int. Ed. Engl. 59, 5816–5822. doi: 10.1002/anie.201915486
Kasahara, K., Miyamoto, T., Fujimoto, T., Oguri, H., Tokiwano, T., Oikawa, H., et al. (2010). Solanapyrone synthase, a possible Diels-Alderase and iterative type I polyketide synthase encoded in a biosynthetic gene cluster from Alternaria solani. Chembiochem 11, 1245–1252. doi: 10.1002/cbic.201000173
Kawaguchi, M., Fukuda, T., Uchida, R., Nonaka, K., Masuma, R., and Tomoda, H. (2013). A new ascochlorin derivative from Cylindrocarpon sp. FKI-4602. J. Antibiot (Tokyo) 66, 23–29. doi: 10.1038/ja.2012.75
Kim, Y., Alpmann, P., Blaum-Feder, S., Kramer, S., Endo, T., Lu, D. S., et al. (2011). In vivo efficacy of griseofulvin against multiple myeloma. Leuk. Res. 35, 1070–1073. doi: 10.1016/j.leukres.2010.10.008
Kishimoto, S., Hara, K., Hashimoto, H., Hirayama, Y., Champagne, P. A., Houk, K. N., et al. (2018). Enzymatic one-step ring contraction for quinolone biosynthesis. Nat. Commun. 9:2826. doi: 10.1038/s41467-018-05221-5
Koide, H., Hasegawa, K., Nishimura, N., Narasaki, R., and Hasumi, K. (2012). A new series of the SMTP plasminogen modulators with a phenylamine-based side chain. J. Antibiot (Tokyo) 65, 361–367. doi: 10.1038/ja.2012.29
Kong, F. D., Zhang, R. S., Ma, Q. Y., Xie, Q. Y., Wang, P., Chen, P. W., et al. (2017). Chrodrimanins O-S from the fungus Penicillium sp. SCS-KFD09 isolated from a marine worm, Sipunculusnudus. Fitoterapia 122, 1–6. doi: 10.1016/j.fitote.2017.08.002
Krick, A., Kehraus, S., Gerhäuser, C., Klimo, K., Nieger, M., Maier, A., et al. (2007). Potential cancer chemopreventive in vitro activities of monomeric xanthone derivatives from the marine algicolous fungus Monodictys putredinis. J. Nat. Prod. 70, 353–360. doi: 10.1021/np060505o
Lane, M. P., Nakashima, T. T., and Vederas, J. C. (2002). Biosynthetic source of oxygens in griseofulvin. Spin-echo resolution of oxygen-18 isotope shifts in carbon-13 NMR spectroscopy. J. Am. Chem. Soc. 104, 913–915. doi: 10.1021/ja00367a071
Larsen, T. O., Gareis, M., and Frisvad, J. C. (2002). Cell cytotoxicity and mycotoxin and secondary metabolite production by common penicillia on cheese agar. J. Agric. Food Chem. 50, 6148–6152. doi: 10.1021/jf020453i
Lee, S. H., Kwak, C. H., Lee, S. K., Ha, S. H., Park, J., Chung, T. W., et al. (2016). Anti-inflammatory effect of ascochlorin in LPS-stimulated RAW 264.7 macrophage cells is accompanied with the down-regulation of iNOS, COX-2 and proinflammatory cytokines through NF−κB, ERK1/2, and p38 signaling pathway. J. Cell. Biochem. 117, 978–987. doi: 10.1002/jcb.25383
Li, C., Matsuda, Y., Gao, H., Hu, D., Yao, X. S., and Abe, I. (2016). Biosynthesis of LL-Z1272beta: discovery of a new member of NRPS-like enzymes for aryl-aldehyde formation. Chembiochem 17, 904–907. doi: 10.1002/cbic.201600087
Li, H. C., Zhang, W., Zhang, X., Tang, S., Men, P., Xiong, M. Y., et al. (2022). Identification of PKS-NRPS hybrid metabolites in marine-derived Penicillium oxalicum. Mar. Drugs 20:523. doi: 10.3390/md20080523
Li, X. Y., Awakawa, T., Mori, T., Ling, M. Q., Hu, D., Wu, B., et al. (2021). Heterodimeric non-heme Iron enzymes in fungal meroterpenoid biosynthesis. J. Am. Chem. Soc. 143, 21425–21432. doi: 10.1021/jacs.1c11548
Liang, W. L., Le, X., Li, H. J., Yang, X. L., Chen, J. X., Xu, J., et al. (2014). Exploring the chemodiversity and biological activities of the secondary metabolites from the marine fungus Neosartorya pseudofischeri. Mar. Drugs 12, 5657–5676. doi: 10.3390/md12115657
Liu, J., Guo, X., Guo, X. C., Zhong, B. Y., Wang, T., Liu, D., et al. (2022). Concise biosynthesis of tropone-containing spiromaterpenes by a sesquiterpene cyclase and a multifunctional P450 from a deep-sea-derived Spiromastix sp. Fungus. J. Nat. Prod. 85, 2723–2730. doi: 10.1021/acs.jnatprod.2c00614
Luo, X. W., Cai, G. D., Guo, Y. F., Gao, C. H., Huang, W. F., Zhang, Z. H., et al. (2021). Exploring marine-derived ascochlorins as novel human dihydroorotate dehydrogenase Inhibitors for treatment of triple-negative breast cancer. J. Med. Chem. 64, 13918–13932. doi: 10.1021/acs.jmedchem.1c01402
Luyen, N. D., Huong, L. M., Thi Hong Ha, T., Cuong, L. H., Thi Hai Yen, D., Nhiem, N. X., et al. (2019). Aspermicrones A-C, novel dibenzospiroketals from the seaweed-derived endophytic fungus Aspergillus micronesiensis. J. Antibiot (Tokyo) 72, 843–847. doi: 10.1038/s41429-019-0214-8
Magae, J., Hayasaki, J., Matsuda, Y., Hotta, M., Hosokawa, T., Suzuki, S., et al. (1988). Antitumor and antimetastatic activity of an antibiotic, ascofuranone, and activation of phagocytes. J. Antibiot (Tokyo) 41, 959–965. doi: 10.7164/antibiotics.41.959
Magae, J., Hosokawa, T., Ando, K., Nagai, K., and Tamura, G. (1982). Antitumor protective property of an isoprenoid antibiotic, ascofuranone. J. Antibiot (Tokyo) 35, 1547–1552. doi: 10.7164/antibiotics.35.1547
Matsuda, Y., Gotfredsen, C. H., and Larsen, T. O. (2018). Genetic characterization of neosartorin biosynthesis provides insight into heterodimeric natural product generation. Organ. Lett. 20, 7197–7200. doi: 10.1021/acs.orglett.8b03123
Miyake, Y., Ito, C., Itoigawa, M., and Osawa, T. (2009). Antioxidants produced by Eurotium herbariorum of filamentous fungi used for the manufacture of karebushi, dried bonito (Katsuobushi). Biosci. Biotechnol. Biochem. 73, 1323–1327. doi: 10.1271/bbb.80887
Mousa, W. K., Schwan, A., Davidson, J., Strange, P., Liu, H., Zhou, T., et al. (2015). An endophytic fungus isolated from finger millet (Eleusine coracana) produces anti-fungal natural products. Front. Microbiol. 6:1157. doi: 10.3389/fmicb.2015.01157
Neubauer, L., Dopstadt, J., Humpf, H.-U., and Tudzynski, P. (2016). Identification and characterization of the ergochrome gene cluster in the plant pathogenic fungus Claviceps purpurea. Fungal Biol. Biotechnol. 3:2. doi: 10.1186/s40694-016-0020-z
Ngen, S. T., Kaur, H., Hume, P. A., Furkert, D. P., and Brimble, M. A. (2016). Synthesis of psychrophilin E. J. Org. Chem. 81, 7635–7643. doi: 10.1021/acs.joc.6b01369
Nies, J., Ran, H., Wohlgemuth, V., Yin, W. B., and Li, S. M. (2020). Biosynthesis of the prenylated salicylaldehyde flavoglaucin requires temporary reduction to salicyl alcohol for decoration before reoxidation to the final product. Org. Lett. 22, 2256–2260. doi: 10.1021/acs.orglett.0c00440
Ning, Y., Gu, Q., Zheng, T., Xu, Y., Li, S., Zhu, Y., et al. (2024). Genome mining leads to diverse sesquiterpenes with anti-inflammatory activity from an arctic-derived fungus. J. Nat. Prod. 87, 1426–1440. doi: 10.1021/acs.jnatprod.4c00237
Nishimura, Y., Suzuki, E., Hasegawa, K., Nishimura, N., Kitano, Y., and Hasumi, K. (2012). Pre-SMTP, a key precursor for the biosynthesis of the SMTP plasminogen modulators. J. Antibiot (Tokyo) 65, 483–485. doi: 10.1038/ja.2012.47
Oxford, A. E., Raistrick, H., and Simonart, P. (1939). Studies in the biochemistry of micro-organisms: griseofulvin, C(17)H(17)O(6)Cl, a metabolic product of Penicillium griseofulvum Dierckx. Biochem. J. 33, 240–248. doi: 10.1042/bj0330240
Panda, D., Rathinasamy, K., Santra, M. K., and Wilson, L. (2005). Kinetic suppression of microtubule dynamic instability by griseofulvin: implications for its possible use in the treatment of cancer. Proc. Natl. Acad. Sci. U.S.A. 102, 9878–9883. doi: 10.1073/pnas.0501821102
Pang, X. Y., Wang, P., Liao, S. R., Zhou, X. F., Lin, X. P., Yang, B., et al. (2022). Three unusual hybrid sorbicillinoids with anti-inflammatory activities from the deep-sea derived fungus Penicillium sp. SCSIO06868. Phytochemistry 202:113311. doi: 10.1016/j.phytochem.2022.113311
Papon, N., Copp, B. R., and Courdavault, V. (2022). Marine drugs: biology, pipelines, current and future prospects for production. Biotechnol. Adv. 54:107871. doi: 10.1016/j.biotechadv.2021.107871
Patridge, E., Gareiss, P., Kinch, M. S., and Hoyer, D. (2016). An analysis of FDA-approved drugs: natural products and their derivatives. Drug Discov. Today 21, 204–207. doi: 10.1016/j.drudis.2015.01.009
Peng, J. X., Gao, H. Q., Zhang, X. M., Wang, S., Wu, C. M., Gu, Q. Q., et al. (2014). Psychrophilins E-H and versicotide C, cyclic peptides from the marine-derived fungus Aspergillus versicolor ZLN-60. J. Nat. Prod. 77, 2218–2223. doi: 10.1021/np500469b
Peng, J. X., Jiao, J. Y., Li, J., Wang, W., Gu, Q. Q., Zhu, T. J., et al. (2012). Pyronepolyene C-glucosides with NF-kappaB inhibitory and anti-influenza A viral (H1N1) activities from the sponge-associated fungus Epicoccum sp. JJY40. Bioorg. Med. Chem. Lett. 22, 3188–3190. doi: 10.1016/j.bmcl.2012.03.044
Prompanya, C., Dethoup, T., Bessa, L. J., Pinto, M. M., Gales, L., Costa, P. M., et al. (2014). New isocoumarin derivatives and meroterpenoids from the marine sponge-associated fungus Aspergillus similanensis sp. nov. KUFA 0013. Mar. Drugs 12, 5160–5173. doi: 10.3390/md12105160
Rebacz, B., Larsen, T. O., Clausen, M. H., Ronnest, M. H., Loffler, H., Ho, A. D., et al. (2007). Identification of griseofulvin as an inhibitor of centrosomal clustering in a phenotype-based screen. Cancer Res. 67, 6342–6350. doi: 10.1158/0008-5472.CAN-07-0663
Rehman, S. U., Yang, L. J., Zhang, Y. H., Wu, J. S., Shi, T., Haider, W., et al. (2020). Sorbicillinoid derivatives from sponge-derived fungus Trichoderma reesei (HN-2016-018). Front. Microbiol. 11:1334. doi: 10.3389/fmicb.2020.01334
Ren, S. Y., Zeng, Y. J., Wang, Q., Lin, Q. F., Yin, X. J., Chen, S. H., et al. (2023). Major facilitator superfamily transporter participates in the formation of dimeric sorbicillinoids pigments. J. Agric. Food Chem. 71, 12216–12224. doi: 10.1021/acs.jafc.3c03004
Ronsberg, D., Debbab, A., Mandi, A., Vasylyeva, V., Bohler, P., Stork, B., et al. (2013). Pro-apoptotic and immunostimulatory tetrahydroxanthone dimers from the endophytic fungus Phomopsis longicolla. J. Org. Chem. 78, 12409–12425. doi: 10.1021/jo402066b
Sanchez, J. F., Entwistle, R., Hung, J. H., Yaegashi, J., Jain, S., Chiang, Y. M., et al. (2011). Genome-based deletion analysis reveals the prenyl xanthone biosynthesis pathway in Aspergillus nidulans. J. Am. Chem. Soc. 133, 4010–4017. doi: 10.1021/ja1096682
Sasaki, H., Hosokawa, T., Sawada, M., and Ando, K. (1973). Isolation and structure of ascofuranone and ascofranol, antibiotics with hypolipidemic activity. J. Antibiot (Tokyo) 26, 676–680. doi: 10.7164/antibiotics.26.676
Sata, N. U., Wada, S.-I., Matsunaga, S., Watabe, S., van Soest, R. W. M., and Fusetani, N. (1999). Rubrosides A-H, new bioactive tetramic acid glycosides from the marine sponge Siliquariaspongia japonica. J. Organ. Chem. 64, 2331–2339. doi: 10.1021/jo981995v
Sawada, H., Nishimura, N., Suzuki, E., Zhuang, J., Hasegawa, K., Takamatsu, H., et al. (2014). SMTP-7, a novel small-molecule thrombolytic for ischemic stroke: a study in rodents and primates. J. Cereb. Blood Flow Metab. 34, 235–241. doi: 10.1038/jcbfm.2013.191
Scharf, D. H., Brakhage, A. A., and Mukherjee, P. K. (2016). Gliotoxin–bane or boon? Environ. Microbiol. 18, 1096–1109. doi: 10.1111/1462-2920.13080
Scharf, D. H., Chankhamjon, P., Scherlach, K., Heinekamp, T., Roth, M., Brakhage, A. A., et al. (2012). Epidithiol formation by an unprecedented twin carbon–sulfur lyase in the gliotoxin pathway. Angew. Chem. 124, 10211–10215. doi: 10.1002/ange.201205041
Scharf, D. H., Chankhamjon, P., Scherlach, K., Heinekamp, T., Willing, K., Brakhage, A. A., et al. (2013). Epidithiodiketopiperazine biosynthesis: a four-enzyme cascade converts glutathione conjugates into transannular disulfide bridges. Angew. Chem. Int. Ed. Engl. 52, 11092–11095. doi: 10.1002/anie.201305059
Scharf, D. H., Habel, A., Heinekamp, T., Brakhage, A. A., and Hertweck, C. (2014). Opposed effects of enzymatic gliotoxin N- and S-methylations. J. Am. Chem. Soc. 136, 11674–11679. doi: 10.1021/ja5033106
Scharf, D. H., Remme, N., Habel, A., Chankhamjon, P., Scherlach, K., Heinekamp, T., et al. (2011). A dedicated glutathione S-transferase mediates carbon-sulfur bond formation in gliotoxin biosynthesis. J. Am. Chem. Soc. 133, 12322–12325. doi: 10.1021/ja201311d
Schatzle, M. A., Husain, S. M., Ferlaino, S., and Muller, M. (2012). Tautomers of anthrahydroquinones: enzymatic reduction and implications for chrysophanol, monodictyphenone, and related xanthone biosyntheses. J. Am. Chem. Soc. 134, 14742–14745. doi: 10.1021/ja307151x
Scherlach, K., and Hertweck, C. (2006). Discovery of aspoquinolones A-D, prenylated quinoline-2-one alkaloids from Aspergillus nidulans, motivated by genome mining. Org. Biomol. Chem. 4, 3517–3520. doi: 10.1039/b607011f
Scherlach, K., Schuemann, J., Dahse, H. M., and Hertweck, C. (2010). Aspernidine A and B, prenylated isoindolinone alkaloids from the model fungus Aspergillus nidulans. J. Antibiot (Tokyo) 63, 375–377. doi: 10.1038/ja.2010.46
Seephonkai, P., Isaka, M., Kittakoop, P., Boonudomlap, U., and Thebtaranonth, Y. (2004). A novel ascochlorin glycoside from the insect pathogenic fungus Verticillium hemipterigenum BCC 2370. J. Antibiot (Tokyo) 57, 10–16. doi: 10.7164/antibiotics.57.10
Segeth, M. P., Bonnefoy, A., Bronstrup, M., Knauf, M., Schummer, D., Toti, L., et al. (2003). Coniosetin, a novel tetramic acid antibiotic from Coniochaeta ellipsoidea DSM 13856. J. Antibiot (Tokyo) 56, 114–122. doi: 10.7164/antibiotics.56.114
Shen, X. Y., Krasnoff, S. B., Lu, S. W., Dunbar, C. D., O’Neal, J., Turgeon, B. G., et al. (1999). Characterization of 6-epi-3-anhydroophiobolin B from Cochliobolus heterostrophus. J. Nat. Prod. 62, 895–897. doi: 10.1021/np980462e
Shiba, T., Kido, Y., Sakamoto, K., Inaoka, D. K., Tsuge, C., Tatsumi, R., et al. (2013). Structure of the trypanosome cyanide-insensitive alternative oxidase. Proc. Natl. Acad. Sci. U.S.A. 110, 4580–4585. doi: 10.1073/pnas.1218386110
Shinohara, C., Hasumi, K., Hatsumi, W., and Endo, A. (1996). Staplabin, a novel fungal triprenyl phenol which stimulates the binding of plasminogen to fibrin and U937 cells. J. Antibiot (Tokyo) 49, 961–966. doi: 10.7164/antibiotics.49.961
Simpson, T. J. (2012). Genetic and biosynthetic studies of the fungal prenylated xanthone shamixanthone and related metabolites in Aspergillus spp. Revisited. Chembiochem 13, 1680–1688. doi: 10.1002/cbic.201200014
Smetanina, O. F., Kalinovskii, A. I., Khudyakova, Y. V., Slinkina, N. N., Pivkin, M. V., and Kuznetsova, T. A. (2007). Metabolites from the marine fungus Eurotium repens. Chem. Nat. Compounds 43, 395–398. doi: 10.1007/s10600-007-0147-5
Subko, K., Kildgaard, S., Vicente, F., Reyes, F., Genilloud, O., and Larsen, T. O. (2021). Bioactive ascochlorin analogues from the marine-derived fungus Stilbella fimetaria. Mar. Drugs 19:46. doi: 10.3390/md19020046
Sun, W. X., Lv, C. T., Zhu, T. H., Yang, X., Wei, S. J., Sun, J. Y., et al. (2013). Ophiobolin-O reverses adriamycin resistance via cell cycle arrest and apoptosis sensitization in adriamycin-resistant human breast carcinoma (MCF-7/ADR) cells. Mar. Drugs 11, 4570–4584. doi: 10.3390/md11114570
Takatsuki, A., Tamura, G., and Arima, K. (1969). Antiviral and antitumor antibiotics. XIV. effects of ascochlorin and other respiration inhibitors on multiplication of newcastle disease virus in cultured cells. Appl. Microbiol. 17, 825–829. doi: 10.1128/am.17.6.825-829.1969
Tamura, G., Suzuki, S., Takatsuki, A., Ando, K., and Arima, K. (1968). Ascochlorin, a new antibiotic, found by the paper-disc agar-diffusion method. Isolation, I., biological and chemical properties of ascochlorin (Studies on antiviral and antitumor antibiotics. I). J. Antibiot (Tokyo) 21, 539–544. doi: 10.7164/antibiotics.21.539
Tian, W., Deng, Z. X., and Hong, K. (2017). The biological activities of sesterterpenoid-type ophiobolins. Mar. Drugs 15:229. doi: 10.3390/md15070229
Toyomasu, T., Tsukahara, M., Kaneko, A., Niida, R., Mitsuhashi, W., Dairi, T., et al. (2007). Fusicoccins are biosynthesized by an unusual chimera diterpene synthase in fungi. Proc. Natl. Acad. Sci. U.S.A. 104, 3084–3088. doi: 10.1073/pnas.0608426104
Tsunematsu, Y., Hirayama, Y., Masuya, T., and Watanabe, K. (2020). Oxidative modification enzymes in polyketide biosynthetic pathways. Compr. Na. Prod. III 1, 479–505. doi: 10.1016/B978-0-12-409547-2.14637-2
Uchida, R., Imasato, R., Yamaguchi, Y., Masuma, R., Shiomi, K., Tomoda, H., et al. (2006). Yaequinolones, new insecticidal antibiotics produced by Penicillium sp. FKI-2140. Taxonomy, I., fermentation, isolation and biological activity. J. Antibiot (Tokyo) 59, 646–651. doi: 10.1038/ja.2006.86
Vardanyan, R. S., and Hruby, V. J. (2006). “35- Antifungal drugs” in Synthesis of essential drugs, ed. R. S. Vardanyan and V. J. Hruby (Amsterdam: Elsevier Science), 535–547.
Voser, T. M., Campbell, M. D., and Carroll, A. R. (2022). How different are marine microbial natural products compared to their terrestrial counterparts? Nat. Prod. Rep. 39, 7–19. doi: 10.1039/d1np00051a
Wang, C., Engelke, L., Bickel, D., Hamacher, A., Frank, M., Proksch, P., et al. (2019). The tetrahydroxanthone-dimer phomoxanthone A is a strong inducer of apoptosis in cisplatin-resistant solid cancer cells. Bioorg. Med. Chem. 27:115044. doi: 10.1016/j.bmc.2019.115044
Wang, W.-G., Du, L.-Q., Sheng, S.-L., Li, A., Li, Y.-P., Cheng, G.-G., et al. (2019). Genome mining for fungal polyketide-diterpenoid hybrids: discovery of key terpene cyclases and multifunctional P450s for structural diversification. Org. Chem. Front. 6, 571–578. doi: 10.1039/c8qo01124a
Waring, P., and Beaver, J. (1996). Gliotoxin and related epipolythiodioxopiperazines. Gen. Pharmacol. 27, 1311–1316. doi: 10.1016/s0306-3623(96)00083-3
Waring, P., Sjaarda, A., and Lin, Q. H. (1995). Gliotoxin inactivates alcohol dehydrogenase by either covalent modification or free radical damage mediated by redox cycling. Biochem. Pharmacol. 49, 1195–1201. doi: 10.1016/0006-2952(95)00039-3
Warr, G. A., Veitch, J. A., Walsh, A. W., Hesler, G. A., Pirnik, D. M., Leet, J. E., et al. (1996). BMS-182123, a fungal metabolite that inhibits the production of TNF-.ALPHA. by macrophages and monocytes. J. Antibiot. 49, 234–240. doi: 10.7164/antibiotics.49.234
Watts, K. R., Ratnam, J., Ang, K. H., Tenney, K., Compton, J. E., McKerrow, J., et al. (2010). Assessing the trypanocidal potential of natural and semi-synthetic diketopiperazines from two deep water marine-derived fungi. Bioorg. Med. Chem. 18, 2566–2574. doi: 10.1016/j.bmc.2010.02.034
Wei, Q., Bai, J., Yan, D., Bao, X., Li, W., Liu, B., et al. (2021a). Genome mining combined metabolic shunting and OSMAC strategy of an endophytic fungus leads to the production of diverse natural products. Acta Pharm. Sin. B 11, 572–587. doi: 10.1016/j.apsb.2020.07.020
Wei, Q., Zeng, H. C., and Zou, Y. (2021b). Divergent biosynthesis of fungal fioxafenestrane sesquiterpenes by the cooperation of distinctive Baeyer–Villiger Monooxygenases and α-Ketoglutarate-Dependent dioxygenases. ACS Catal. 11, 948–957. doi: 10.1021/acscatal.0c05319
Wei, X. X., and Matsuda, Y. (2020). Unraveling the fungal strategy for tetrahydroxanthone biosynthesis and diversification. Org. Lett. 22, 1919–1923. doi: 10.1021/acs.orglett.0c00285
Wei, X. X., Chen, X., Chen, L., Yan, D. X., Wang, W. G., and Matsuda, Y. (2021c). Heterologous biosynthesis of tetrahydroxanthone dimers: determination of key factors for selective or divergent synthesis. J. Nat. Prod. 84, 1544–1549. doi: 10.1021/acs.jnatprod.1c00022
Wu, G., Ma, H., Zhu, T., Li, J., Gu, Q., and Li, D. (2012). Penilactones A and B, two novel polyketides from antarctic deep-sea derived fungus Penicillium crustosum PRB-2. Tetrahedron 68, 9745–9749. doi: 10.1016/j.tet.2012.09.038
Xiao, Z.-H., Dong, J.-Y., Li, A., Dai, J.-M., Li, Y.-P., Hu, Q.-F., et al. (2022). Biocatalytic and chemical derivatization of the fungal meroditerpenoid chevalone E. Org. Chem. Front. 9, 1837–1843. doi: 10.1039/d2qo00055e
Yabu, Y., Yoshida, A., Suzuki, T., Nihei, C., Kawai, K., Minagawa, N., et al. (2003). The efficacy of ascofuranone in a consecutive treatment on Trypanosoma brucei brucei in mice. Parasitol. Int. 52, 155–164. doi: 10.1016/s1383-5769(03)00012-6
Yamazaki, H., Nakayama, W., Takahashi, O., Kirikoshi, R., Izumikawa, Y., Iwasaki, K., et al. (2015). Verruculides A and B, two new protein tyrosine phosphatase 1B inhibitors from an Indonesian ascidian-derived Penicillium verruculosum. Bioorg. Med. Chem. Lett. 25, 3087–3090. doi: 10.1016/j.bmcl.2015.06.026
Yan, J. J., Pang, J. M., Liang, J. J., Yu, W. L., Liao, X. Q., Aobulikasimu, A., et al. (2022). The biosynthesis and transport of ophiobolins in Aspergillus ustus 094102. Int. J. Mol. Sci. 23:1903. doi: 10.3390/ijms23031903
Yan, T., Wu, W. H., Su, T. W., Chen, J. J., Zhu, Q. G., Zhang, C. Y., et al. (2015). Effects of a novel marine natural product: pyrano indolone alkaloid fibrinolytic compound on thrombolysis and hemorrhagic activities in vitro and in vivo. Arch. Pharm. Res. 38, 1530–1540. doi: 10.1007/s12272-014-0518-y
Yang, T. T., Lu, Z. Y., Meng, L., Wei, S. J., Hong, K., Zhu, W. M., et al. (2012). The novel agent ophiobolin O induces apoptosis and cell cycle arrest of MCF-7 cells through activation of MAPK signaling pathways. Bioorg. Med. Chem. Lett. 22, 579–585. doi: 10.1016/j.bmcl.2011.10.079
Yin, Y., Fu, Q., Wu, W. H., Cai, M. H., Zhou, X. S., and Zhang, Y. X. (2017). Producing novel fibrinolytic isoindolinone derivatives in marine fungus Stachybotrys longispora FG216 by the rational supply of amino compounds according to its biosynthesis pathway. Mar. Drugs 15:214. doi: 10.3390/md15070214
Yuan, S. W., Chen, L. T., Wu, Q. L., Jiang, M. H., Guo, H., Hu, Z. B., et al. (2022a). Genome mining of alpha-pyrone natural products from ascidian-derived fungus Amphichordafelina SYSU-MS7908. Mar. Drugs 20:294. doi: 10.3390/md20050294
Yuan, S. W., Chen, S. H., Guo, H., Chen, L. T., Shen, H. J., Liu, L., et al. (2022b). Elucidation of the complete biosynthetic pathway of phomoxanthone A and identification of a para-para selective phenol coupling dimerase. Org. Lett. 24, 3069–3074. doi: 10.1021/acs.orglett.2c01050
Zeng, H. C., Yin, G. P., Wei, Q., Li, D. H., Wang, Y., Hu, Y. C., et al. (2019). Unprecedented [5.5.5.6]dioxafenestrane ring construction in fungal insecticidal sesquiterpene biosynthesis. Angew. Chem. Int. Ed. Engl. 58, 6569–6573. doi: 10.1002/anie.201813722
Zeng, Y. J., Lu, T. T., Ren, S. Y., Hu, Z. B., Fang, J., Guan, Z. F., et al. (2024). Biosynthesis of ester-bond containing quinolone alkaloids with (3R,4S) stereoconfiguration. Org. Lett. 26, 6692–6697. doi: 10.1021/acs.orglett.4c02372
Zhang, D., Fukuzawa, S., Satake, M., Li, X., Kuranaga, T., Niitsu, A., et al. (2012). Ophiobolin O and 6-Epi-Ophiobolin O, two new cytotoxic sesterterpenes from the marine derived fungus Aspergillus sp. Nat. Prod. Commun. 7, 1411–1414. doi: 10.1177/1934578x1200701102
Zhang, M., Yan, S., Liang, Y., Zheng, M., Wu, Z., Zang, Y., et al. (2020). Talaronoids A–D: four fusicoccane diterpenoids with an unprecedented tricyclic 5/8/6 ring system from the fungus Talaromyces stipitatus. Organ. Chem. Front. 7, 3486–3492. doi: 10.1039/d0qo00960a
Zhang, P. P., Jia, C. X., Deng, Y. L., Chen, S. H., Chen, B., Yan, S. W., et al. (2019). Anti-inflammatory prenylbenzaldehyde derivatives isolated from Eurotium cristatum. Phytochemistry 158, 120–125. doi: 10.1016/j.phytochem.2018.11.017
Zhang, P., Deng, Y., Lin, X., Chen, B., Li, J., Liu, H., et al. (2019). Anti-inflammatory mono- and dimeric sorbicillinoids from the marine-derived fungus Trichoderma reesei 4670. J. Nat. Prod. 82, 947–957. doi: 10.1021/acs.jnatprod.8b01029
Zhang, P., Wu, G. W., Heard, S. C., Niu, C. S., Bell, S. A., Li, F. L., et al. (2022). Identification and characterization of a cryptic bifunctional type I diterpene synthase iInvolved in talaronoid biosynthesis from a marine-derived fungus. Org. Lett. 24, 7037–7041. doi: 10.1021/acs.orglett.2c02904
Zhang, T., Wan, J., Zhan, Z., Bai, J., Liu, B., and Hu, Y. (2018). Activation of an unconventional meroterpenoid gene cluster in Neosartorya glabra leads to the production of new berkeleyacetals. Acta Pharm. Sin. B. 8, 478–487. doi: 10.1016/j.apsb.2017.12.005
Zhao, J. L., Zhang, M., Liu, J. M., Tan, Z., Chen, R. D., Xie, K. B., et al. (2017). Bioactive steroids and sorbicillinoids isolated from the endophytic fungus Trichoderma sp. Xy24. J. Asian Nat. Prod. Res. 19, 1028–1035. doi: 10.1080/10286020.2017.1285908
Zhao, M., Lin, H.-C., and Tang, Y. (2016). Biosynthesis of the α-nitro-containing cyclic tripeptide psychrophilin. J. Antibiot. 69, 571–573. doi: 10.1038/ja.2016.33
Zhao, Y., Zhao, C., Lu, J., Wu, J., Li, C., Hu, Z., et al. (2019). Sesterterpene MHO7 suppresses breast cancer cells as a novel estrogen receptor degrader. Pharmacol. Res. 146:104294. doi: 10.1016/j.phrs.2019.104294
Zhen, X., Gong, T., Wen, Y. H., Yan, D. J., Chen, J. J., and Zhu, P. (2018). Chrysoxanthones A(-)C, three new xanthone(-)chromanone heterdimers from sponge-associated Penicillium chrysogenum HLS111 treated with histone deacetylase inhibitor. Mar. Drugs 16:357. doi: 10.3390/md16100357
Zheng, Y., Ma, K., Lyu, H., Huang, Y., Liu, H., Liu, L., et al. (2017). Genetic manipulation of the COP9 signalosome subunit PfCsnE leads to the discovery of pestaloficins in Pestalotiopsis fici. Org. Lett. 19, 4700–4703. doi: 10.1021/acs.orglett.7b02346
Zhu, G. L., Hou, C. J., Yuan, W. Z., Wang, Z. Z., Zhang, J. Y., Jiang, L., et al. (2020). Molecular networking assisted discovery and biosynthesis elucidation of the antimicrobial spiroketals epicospirocins. Chem. Commun (Camb) 56, 10171–10174. doi: 10.1039/d0cc03990j
Zou, Y., Garcia-Borras, M., Tang, M. C., Hirayama, Y., Li, D. H., Li, L., et al. (2017). Enzyme-catalyzed cationic epoxide rearrangements in quinolone alkaloid biosynthesis. Nat. Chem. Biol. 13, 325–332. doi: 10.1038/nchembio.2283
Keywords: marine fungi, marine natural products, biosynthetic gene clusters, genome mining, biosynthesis
Citation: Han C, Song A, He Y, Yang L, Chen L, Dai W, Wu Q and Yuan S (2024) Genome mining and biosynthetic pathways of marine-derived fungal bioactive natural products. Front. Microbiol. 15:1520446. doi: 10.3389/fmicb.2024.1520446
Received: 31 October 2024; Accepted: 02 December 2024;
Published: 12 December 2024.
Edited by:
Runying Zeng, State Oceanic Administration, ChinaReviewed by:
Wei-Guang Wang, Yunnan Minzu University, ChinaPriscilla Zwiercheczewski De Oliveira, University of Mons, Belgium
Copyright © 2024 Han, Song, He, Yang, Chen, Dai, Wu and Yuan. This is an open-access article distributed under the terms of the Creative Commons Attribution License (CC BY). The use, distribution or reproduction in other forums is permitted, provided the original author(s) and the copyright owner(s) are credited and that the original publication in this journal is cited, in accordance with accepted academic practice. No use, distribution or reproduction is permitted which does not comply with these terms.
*Correspondence: Siwen Yuan, eXVhbnNpd2VuQHptdXpoLmVkdS5jbg==; Qilin Wu, d3VxaWxpbkB6bXV6aC5lZHUuY24=