- 1Department of Climate Change and Environment, Climate Change and Environmental Research Center, Pathumthani, Thailand
- 2Department of Social and Environmental Medicine, Faculty of Tropical Medicine, Mahidol University, Bangkok, Thailand
- 3Department of Chemical Engineering, Faculty of Engineering, Srinakharinwirot University, Nakhon Nayok, Thailand
- 4Department of Civil and Environmental Engineering, Faculty of Engineering, Srinakharinwirot University, Nakhon Nayok, Thailand
- 5Center of Excellence in Rail System Technology and Civil Engineering Material Innovation for Sustainable Infrastructure, Strategic Wisdom and Research Institute, Srinakharinwirot University, Bangkok, Thailand
Microplastic (MP) contamination in aquatic environments is a critical concern due to its potential effects on aquatic ecosystems. MP contamination is often unsatisfactorily eliminated using conventional wastewater treatment systems. Membrane bioreactor (MBR) is a modern solution for wastewater treatment offering significant advantages over traditional activated sludge systems, such as a smaller footprint and the ability to produce high-quality effluent. In this study, a pilot-scale MBR was conducted to evaluate MP removal from real greywater. The overall treatment performance for MP removal reached up to 90%, with the MP concentration in the permeate effluent being 0.02 MP L−1. The major MP size distribution was 101–300 μm, with polypropylene as the predominant MP type. Remarkably, polyester fibers were highly predominant in the suspended sludge. Furthermore, Alphaproteobacteria, Bacteroidetes, and Actinobacteria were the predominant communities in the MBR sludge, which preferably formed a biofilm associated with MP accumulation. This study underscores the potential of MBR technology for efficient MP removal in household buildings, contributing to the mitigation of MP discharge into the environment. Implementing MBR systems is a crucial step toward safeguarding aquatic ecosystems and preserving environmental integrity with respect to the corresponding increase in MP pollution.
1 Introduction
Managing waste, especially plastic waste, presents a significant challenge for many countries. Developing effective waste management strategies is essential, particularly for highly durable plastics that degrade very slowly in the environment. Over time, the extensive production and use of plastics have led to widespread pollution. Microplastics (MPs), plastic particles < 5 mm (Hongprasith et al., 2020), originate from various sources, both primary and secondary MPs. Sources of MPs include the direct manufacture of tiny plastic pellets and microbeads, frequently employed as constituents in cosmetics, synthetic textiles, and personal care products such as exfoliating scrubs, toothpaste, and lotions. Additionally, MPs can result from the breakdown of larger plastic items. These plastics are smaller fragments that persist in the environment, causing pollution (Hidalgo-Ruz et al., 2012). Thus, MPs are now pervasive in the environment, accumulating in food chains and posing toxicity risks (Teuten et al., 2009; Setälä et al., 2014). Their high surface area allows them to adsorb various environmental pollutants, including heavy metals and persistent organic pollutants. This ability to accumulate contaminants raises concerns about bioaccumulation and possible risks for ecosystems and human health (Rodrigues et al., 2019).
Research on the prevalence of MP pollution in aquatic environments has drawn considerable global attention. Numerous studies have reported the widespread presence of MPs in natural water sources worldwide (Kunz et al., 2023; Mennekes and Nowack, 2023). Understanding the pathways by which MPs move through these environments is crucial. Once discharged into water bodies, MPs can accumulate within wastewater treatment systems (Talvitie et al., 2017; Lares et al., 2018; Kittipongvises et al., 2022). Wastewater treatment systems are significant sources of secondary MP contamination (Habib et al., 2020; Liu et al., 2021).
Greywater, defined as domestic water excluding toilet waste, typically accounts for 50–80% of the total household water usage (Santasmasas et al., 2013) and is a major contributor to MP contamination. MPs from personal care products, laundry effluents, and cleaning agents enter the wastewater stream, eventually contaminating wastewater treatment systems (Esfandiari and Mowla, 2021; Jessieleena et al., 2023). Among these, synthetic fibers released during laundering are a predominant type of MPs found in greywater, primarily comprising polyester (PES), polyamide (PA), polypropylene (PP), and acrylic, with PES and PA accounting for 14–50% of the total MPs (Pedrotti et al., 2021).
Although some studies have examined the MP removal efficiency in greywater using methods coagulation combined with dissolved air flotation, achieving up to 90% removal (Esfandiari and Mowla, 2021), these were conducted at a laboratory scale with synthetic greywater and specific MP polymers such as polyethylene (PE) and microfiber. This may not fully reflect real greywater contamination and treatment conditions. Furthermore, limited studies have been conducted on MP removal in membrane bioreactors (MBRs) for MP removal in real greywater treatment. Existing research mainly compares the performance of MBRs to that of conventional activated sludge (CAS) processes in municipal wastewater treatment plants. For instance, Lares et al. (2018) reported that MBRs performed well in MP removal, with MBR permeate containing 0.4 MP L−1, compared to 1.0 MP L−1 in the final effluent of the CAS process, attributed to the microfiltration process. Similarly, Bayo et al. (2020) found that MBRs achieved better MP removal than rapid sand filtration in urban wastewater treatment. Recent studies further support these findings, demonstrating that MBR systems combined with ultrafiltration (UF) and reverse osmosis (RO) can achieve MP removal efficiencies of up to 98% (Cai et al., 2022). Additionally, Talvitie et al. (2017) reported that MBRs reduced MP concentrations from 6.9 ± 1.0 MP L−1 in the influent to as low as 0.005 ± 0.004 MP L−1 in the treated effluent, highlighting their superior efficiency over conventional treatment methods. These findings underscore the potential of MBR technology in reducing MP discharge into the environment while maintaining high-quality effluent. Additionally, MBRs require a smaller physical footprint compared to conventional treatment processes, making them suitable for urban and space-limited applications. Due to the membrane filtration step, MBR-treated effluent has significantly lower turbidity and suspended solids compared to conventional systems, ensuring higher water quality for potential reuse. Furthermore, MBRs perform efficiently under low organic-loading conditions, making them advantageous for decentralized and household greywater treatment. However, MBR performance specifically for treating MPs in real greywater remains unexplored. Therefore, identifying the types and loads of MPs in real greywater treated by MBR is essential for understanding their fate and stabilizing the performance of MBR treatment.
This study employed a pilot-scale MBR to treat real greywater from a dormitory. The research aimed to evaluate the performance of the MBR in removing MP while quantifying and characterizing MP contaminants in the permeate effluent and the sludge. This study also examined the predominant bacterial communities in the MBR sludge that could be associated with MP accumulation. Identifying the dominant MP types provides valuable insights into improving the efficiency of the MBR in MP removal from real greywater and classifying sources of contamination, supporting the development of targeted waste management strategies.
2 Materials and methods
2.1 MBR operation
Figure 1 illustrates the schematic diagram of the MBR system. This pilot plant comprised several key components: a membrane chamber, an aerobic tank with a capacity of 5 m3, a permeate tank, and multiple pumps. Submerged hollow fiber microfiltration membranes with a nominal pore size of 0.1 μm (provided by Sumitomo, Japan) covered a total surface area of 36 m2 within the membrane chamber. Aeration was employed to clean the membrane surface. An electrical controller linked to a level sensor connected to the feed pump maintained a consistent water level in the aerobic tank. The MBR operated to process a maximum of 10 m3 of wastewater daily, with a continuous hydraulic retention time of 12 h and no sludge withdrawal for 5 years. Excess sludge from the CAS process served as seed sludge and was introduced into the aeration tank. The operational cycle comprised a filtration time of 9 min and a relaxation time of 1 min. Throughout the experimental period, the average permeate flux was consistently regulated at 11.5 L m−2 h−1.
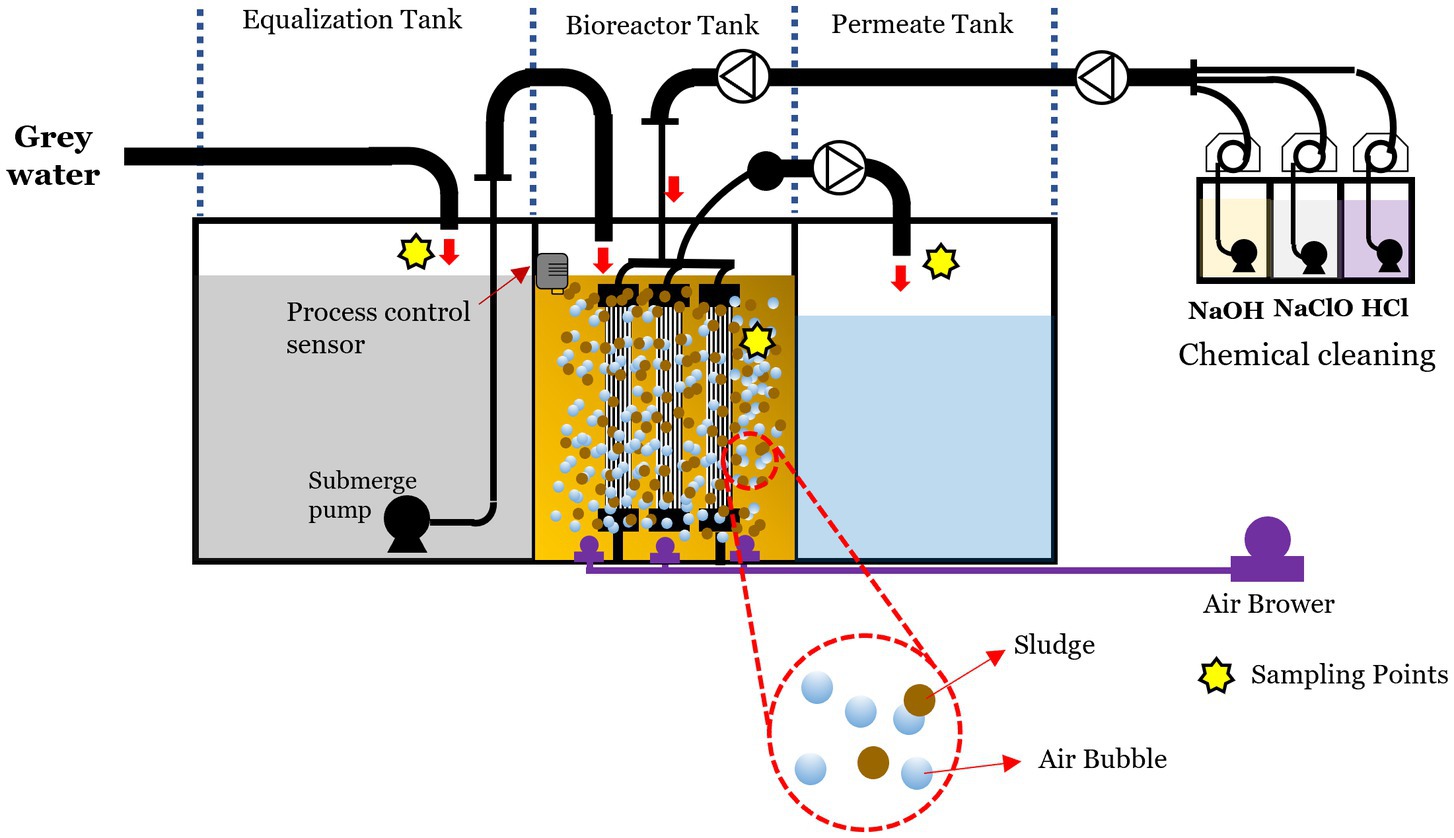
Figure 1. Schematic diagram of MBR pilot plant showing the different compartments, flow directions, and main instruments and equipment.
The system was installed to treat greywater from a dormitory located in a university residential zone in Nakhon Nayok Province, Thailand. The eight-story building consists of 64 rooms, including 48 single rooms and 16 family rooms. Its sanitary system separates blackwater from toilets to a septic tank, while greywater from showers, hand-wash basins, kitchens, and laundry is directed to the treatment system. Sampling was conducted during February–March 2023, during which the greywater influent was continuously fed into the MBR system.
2.2 Sampling and collection of water and sludge samples
All samples were collected at two time points and were referred to as I and II. The second sampling was conducted one month after the first. After passing through a 1 cm screen chamber, influent samples with a volume of 1,000 L were collected. These samples were transferred to a series of four stainless steel test sieves with mesh sizes of 5 mm, 1 mm, 300 μm, and 100 μm using a submerged pump (Razeghi et al., 2021). Particles retained by the 5 mm sieve were discarded, whereas those retained by the 1 mm, 300 μm, and 100 μm sieves were gently rinsed with distilled water and individually collected into glass bottles. Thorough rinsing with distilled water was conducted to ensure the proper transfer of all particles. Similarly, the permeate from the MBR pilot plant was collected using the same method. Activated sludge samples (1 L) were gathered using a 10 L stainless steel bucket and transferred into glass bottles. All samples were transported to the laboratory and stored at 4°C in the dark until further processing.
2.3 Examination and identification of MPs
The water and sludge samples were treated based on the method proposed by Masura et al. (2015), with minor adjustments. The sieved samples were placed in a 60°C drying oven overnight until almost dry. The pretreated samples underwent digestion via wet peroxide oxidation to remove organic matter contamination. This oxidation process involved the addition of 20 mL of a 0.05 M aqueous Fe (II) solution and 20 mL of 30% hydrogen peroxide (H2O2) to the pretreated samples. The mixture was allowed to settle for 5 min at room temperature before agitation on a hotplate with a magnetic stirrer at 60°C, covered with a watch glass. Depending on the organic matter content in the sample, an additional 20 mL of H2O2 might have been added to complete the oxidative reaction. Density separation was conducted using a saturated sodium chloride solution. After the samples were left to settle overnight, the settled particles were discarded. The supernatant was then filtered through an Anodisc filter of 0.2 μm. The filtered samples were kept in glass Petri dishes before further analysis.
To identify the samples, optical microscopy and Fourier transform infrared microscopy (ALPHA II, Bruker Optik GmbH, Germany) were utilized. The analysis was conducted in the transmittance mode, with 128 scans taken to generate spectra ranging from 1,200 to 4,000 cm−1 at a spectral resolution of 4 cm−1. These spectra were compared to the libraries provided by Bruker for identification.
2.4 Quality assurance and quality control
To prevent MP contamination, cotton clothing and gloves were worn during the experiments, and only nonplastic equipment was used. All glassware and stainless-steel equipment were washed with detergent and rinsed with ultrapure (Wesch et al., 2017). For blank controls, ultrapure water was filtered using the same sieve sets for sample collection.
2.5 Water quality analysis
Dissolved oxygen (DO), pH, and temperature were measured using appropriate probes (YSI 60, United States). The chemical oxygen demand (COD) was analyzed using Hach methods (HACH, United States). Total nitrogen (TN) was analyzed by the total organic carbon analyzer (TOC-L, Shimadzu, Japan). Turbidity was determined using a turbidity meter (Thermo Fisher, United States). Total suspended solids (TSS) were analyzed using the standard method (APHA, 2017). The mixed liquor suspended solids (MLSS) concentration was determined using Japanese standard methods (JSWA, 1997).
2.6 DNA extraction, sequencing, and bioinformatic processing analysis
DNA extraction and sequencing were conducted by the Zymobiomics service (Zymo Research, Irvine, CA, United States). DNA extraction was performed using the ZymoBIOMICs-96 MagBead DNA Kit. Zymo Research custom-designed two primer sets, V1-V2 and V3-V4, to ensure optimal coverage while maintaining high sensitivity. The PCR reactions were conducted in real-time PCR machines to control cycles and minimize PCR chimera formation. The final library was sequenced on an Illumina® MiSeq™ platform with a v3 reagent kit (600 cycles), with the addition of a 10% PhiX spike-in. Unique amplicon sequences were identified from the raw reads through analysis using the Dada2 pipeline (Callahan et al., 2018).
Chimeric sequences were also removed with the Dada2 pipeline. Taxonomic classification was conducted using Uclust from Qiime version 1.9.1 (Caporaso et al., 2010), using the Zymo Research Database. The significant abundance among different groups was identified by LEfSe (Segata et al., 2011). The relative bacterial abundance was characterized at the phylum level, and a phylum with <2% an abundance was considered a minor phylum. Class and order were also categorized together, and percentage levels of the identified genus are shown.
3 Results and discussion
3.1 Operating conditions of the MBR for treating greywater
The MBR operated at a capacity of 10.2 ± 1.1 m3 d−1. Table 1 details the water quality characteristics of the greywater and MBR effluent and the overall treatment performance. Throughout the operation, the pH of the influent and MBR effluent remained neutral at ambient temperature. DO levels in the influent were <0.1 mg L−1, whereas the DO levels in the effluent increased to 4.6 mg L−1 due to aeration in the MBR tank. The influent exhibited average COD and TN levels of 93.5 mg L−1 and 8.8 mg L−1, respectively. Conversely, the MBR effluent showed average COD and TN levels of 24.2 mg L−1 and 4.0 mg L−1, respectively, with average treatment efficiencies of 74 and 55%, respectively. Greywater composition primarily originates from bath, shower, and laundry sources, resulting in low COD and TN concentrations. When comparing the treatment performance of greywater between low and high loading capacity, it was found that the removal efficiency of the MBR process could be a stable treatment (Ittisupornrat and Theepharaksapan, 2023). Due to the low COD concentration in the influent (ranging from 57 to 130 mg L−1), the average MLSS concentration increased from 0.8 to 1.4 g L−1 after one month of operation, reaching an average of 1.1 g L−1. In addition, various anti-bacterial agents and some surfactants from shampoos and body soaps may contribute to inhibit bacterial growth (Liu et al., 2005; Lamine et al., 2012). The average TSS level in the influent was 9.4 mg L−1, corresponding to the turbidity value of 16.1 NTU. Meanwhile, TSS and turbidity were completely removed through the treatment, with values higher than 99% due to microfiltration by membrane (Ittisupornrat et al., 2019).
3.2 Removal efficiency and characteristics of MPs in the MBR
Figure 2 presents the analysis of MP contamination and highlights the predominant polymer types in the influent and effluent samples across two sampling events. The list of influents includes PP, polypropylene glycol (PPG), styrene–ethylene–butylene–styrene (SEBS), polyethylene glycol (PEG), PE, acrylonitrile butadiene styrene (ABS), polystyrene (PS), copolymer styrene–butadiene (SBC), PES, and others, whereas the list of effluents includes PP, SEBS, PE, ABS, PES, polyvinyl chloride (PVC), and others. In the influent, PP was the most abundant polymer with concentrations ranging from 47 to 72 MP m−3, accounting for an average of 37% of MPs, followed by PPG (7–18 MP m−3) and SEBS (8–12 MP m−3). In the effluent, PP remained the dominant polymer with concentrations ranging from 3 to 4 MP m−3, representing 23% of MPs followed by PE (2 MP m−3) and ABS (1–2 MP m−3). This is in agreement with Wei et al. (2020), who reported that PP was the most prevalent polymer type in rural domestic wastewater. In addition to this polymer, PE, PS, and PES are commonly used in personal care products and cosmetics, and cleaning products. These primary and secondary MPs are derived from our everyday activities and are generated through abrasion of various packaging materials and synthetic clothing (Mishra et al., 2022; Jessieleena et al., 2023; Song and Li, 2023).
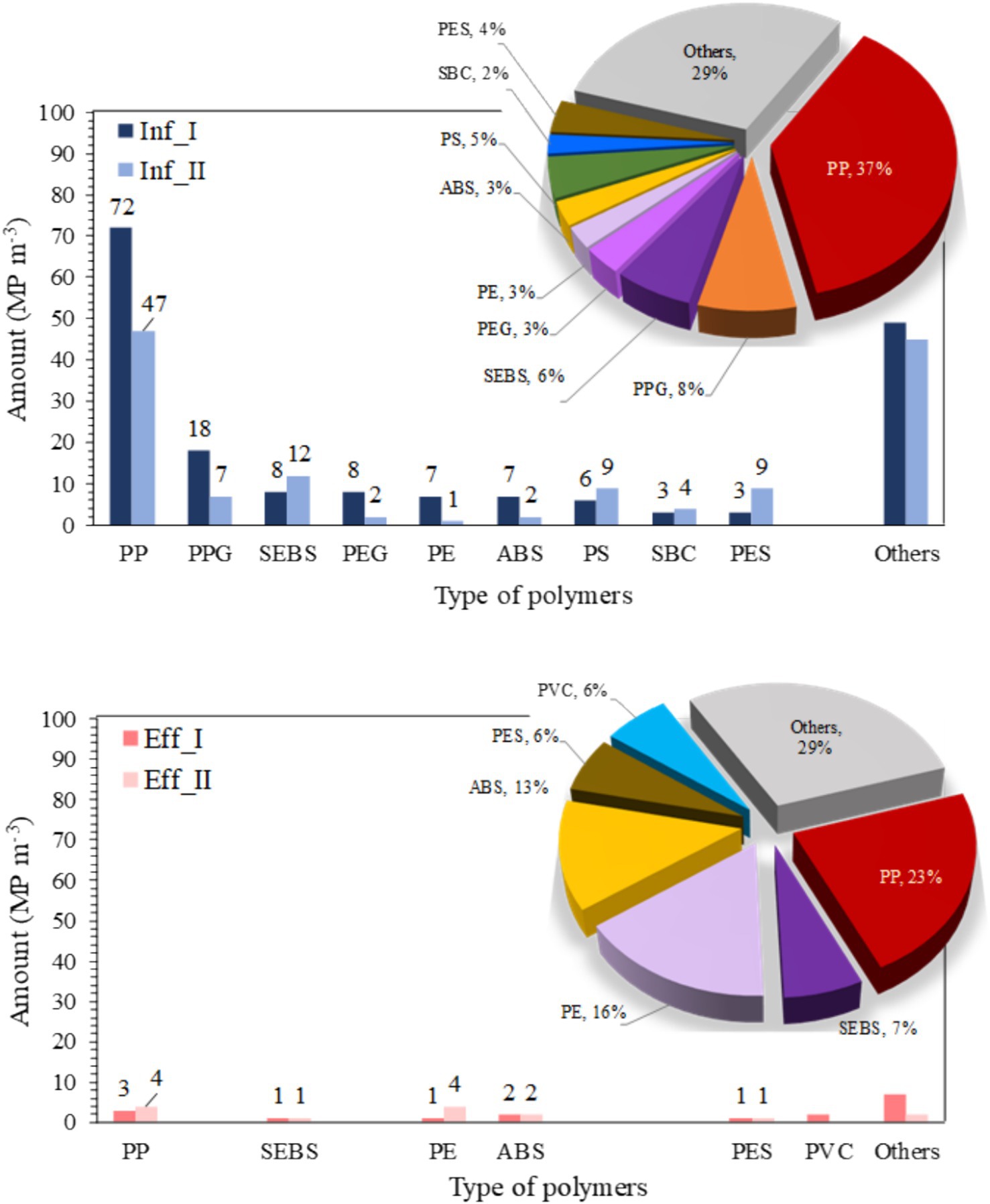
Figure 2. The predominant polymer types in microplastic contamination were analyzed in the influent (top) and effluent (bottom) samples, with corresponding percentages shown in the pie charts. I and II represent the first and second water sampling events, respectively. PP, polypropylene; PPG, polypropylene glycol; SEBS, styrene–ethylene–butylene-styrene; PEG, polyethylene glycol; PE, polyethylene; ABS, acrylonitrile butadiene styrene; PS, polystyrene; SBC, copolymer styrene–butadiene; PES, polyester; PVC, polyvinylchloride.
SEBS and ABS are technical thermoplastics widely used in typical applications, such as toys, automotive applications, general purpose molded goods, window and door seals, and electrical and electronic spare parts (Peydro et al., 2013). The proportion of different polymers varied only slightly between I and II when MPs from both samples were considered. PPG and PEG were the important plasticizers in the detergents, which were detected in influent samples but not in effluent samples (Piorkowska et al., 2006). This could be because these polymers are easily degraded by microorganisms (Kadac-Czapska et al., 2023).
Figure 3 presents the MP size distribution and occurrence percentage in the influent and MBR effluent samples. Visual examples provided in Figure 3 (right) illustrate various MPs in the influent and effluent, including fragments and fibers. The MP size distribution is an important parameter associated with their removal efficiencies. In this study, the predominant size of MPs was 101–300 μm (only in length measurement) in both influent and MBR effluent, accounting for 62 and 76%, respectively, on average, followed by an MP size of 301–500 μm accounting for 20 and 16%, respectively. Meanwhile, MP sizes of 501–1,000 and > 1,000 μm were rarely measured (<10%), in accordance with (Egea-Corbacho et al., 2023), who described small-sized particles (100–355 μm) as the major size in influent and effluent samples. Similarly, Liu et al. (2019) found 65–87% of MPs < 1 mm in the influent originating from municipal wastewater but 81–91% in the effluent of the wastewater treatment process, implying that the origin MP particles are decomposed to secondary MPs by physical, chemical or biological processes during the operation (Song and Li, 2023). Furthermore, this experiment measured MPs only in the length mode (not thickness), and the observed MP size mostly seemed bigger than the nominal pore size of the membrane (0.1 μm). Based on the phenomena of membrane microfiltration, the pore size of the membrane should not allow such large particles to pass through, but it was observed in an effluent, perhaps due to leakage from long-term operation (Egea-Corbacho et al., 2023). Conversely, the high suction pressure used in membrane filtration may force small-sized MPs to pass through membrane pores. This observation aligns with previous studies reporting the presence of MPs in MBR effluents, indicating the potential risk of MP leakage from such systems (Talvitie et al., 2017; Bayo et al., 2020; Cai et al., 2022) effluent that there is a risk of MP release from MBR systems. However, the concentration of MPs released from the MBR was lower than that from the conventional wastewater treatment plant (Lares et al., 2018).
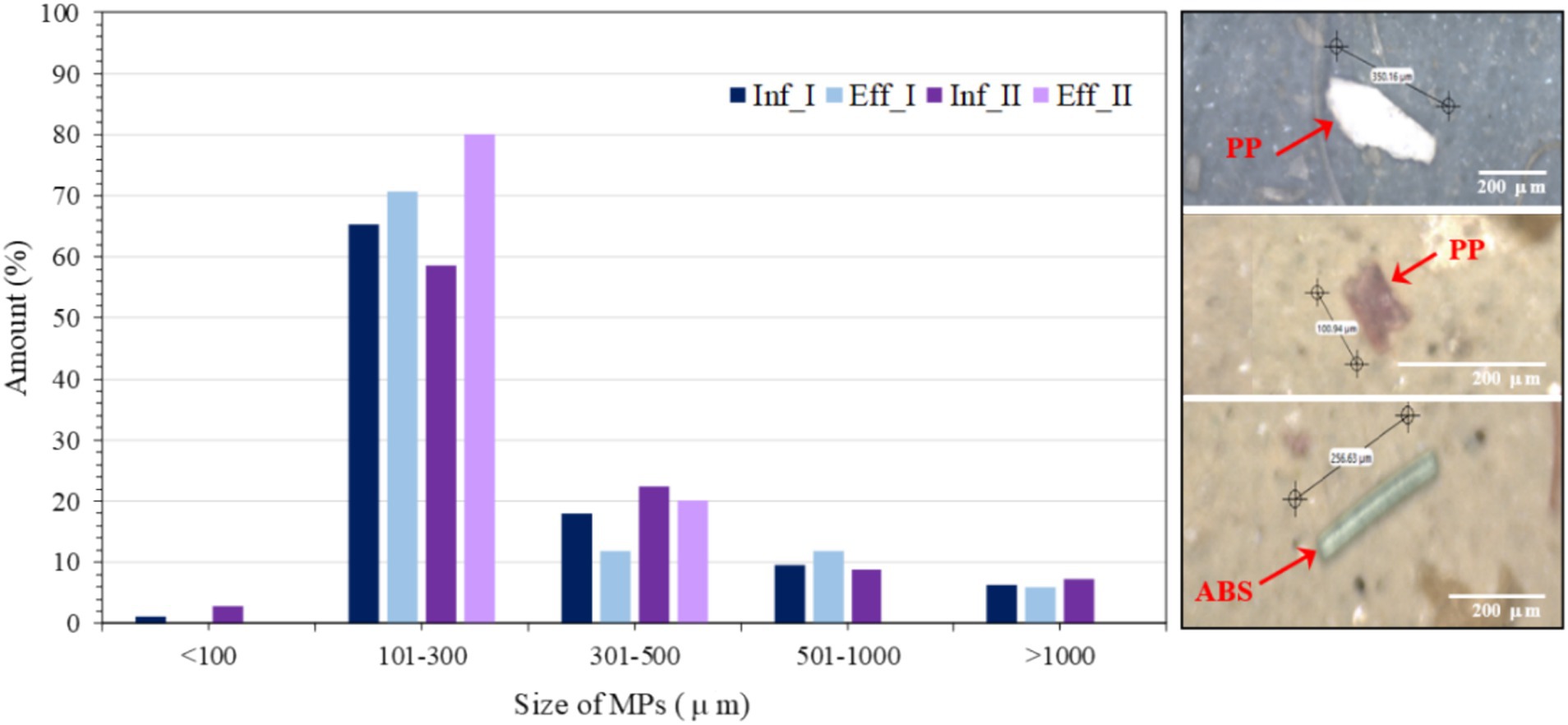
Figure 3. Size distribution and occurrence percentage of microplastics in the influent and MBR effluent. The right panel shows examples of microplastics. I and II represent the first and second water sampling events, respectively.
The average MPs found in the influent and effluent were 159.5 and 15.5 MP m−3, respectively, resulting in a 90.2% removal efficiency of MPs (Table 2). Results were in accordance with previous studies conducted in MBR, indicating that MP concentrations in the MBR permeate (0.4 MP L−1) were measured (Lares et al., 2018; Iyare et al., 2020), implying the system’s ability to efficiently reject large MP particles by membrane microfiltration (Li et al., 2020). The MBR process is strongly recommended as the best cost-saving technology for reducing MPs in the aquatic environment (Vuori and Ollikainen, 2022). During this operation, no membrane fouling was observed. However, membrane fouling is one of the main drawbacks of membrane separation. Several studies noted that different MP particle diameters influence the biofouling of MBRs in different ways (Wang et al., 2022; Acarer, 2023).
3.3 Characteristics of MPs in the sludge
MPs in the MBR sludge were analyzed, revealing that PES was the major microfiber, with an average concentration of 28 MP g−1 MLSS, accounting for 20% of the total MPs (Figure 4; Table 3). PA and PP followed, with average concentrations of 14 and 13 MP g−1 MLSS, respectively. The accumulation characteristics of MPs in the sludge are presented in Figure 5. These results are in accordance with those of Lares et al. (2018), who found secondary MPs originating from microfibers (24.1 ± 6.1 MP g−1 dry weight) to be the dominant type of MPs in the MBR sludge. The observed PES may originate from washing of clothes (Hazlehurst et al., 2023). Among PP, PA, PU, PE, polyethylene terephthalate (PET), and PVC have also been widely used for producing clothes and textiles, as well as all common plastic materials used in daily life (Song and Li, 2023). Moreover, polyvinyl alcohol (PVA) was detected (7%), which is commercially used in laundry and dish detergent pods (Rolsky and Kelkar, 2021). Furthermore, some studies revealed that ≥90% of MPs removed in wastewater treatment plants are entrapped in the sludge (Reddy and Nair, 2022), and effective sludge management to mitigate their dispersion into the environment should be considered.
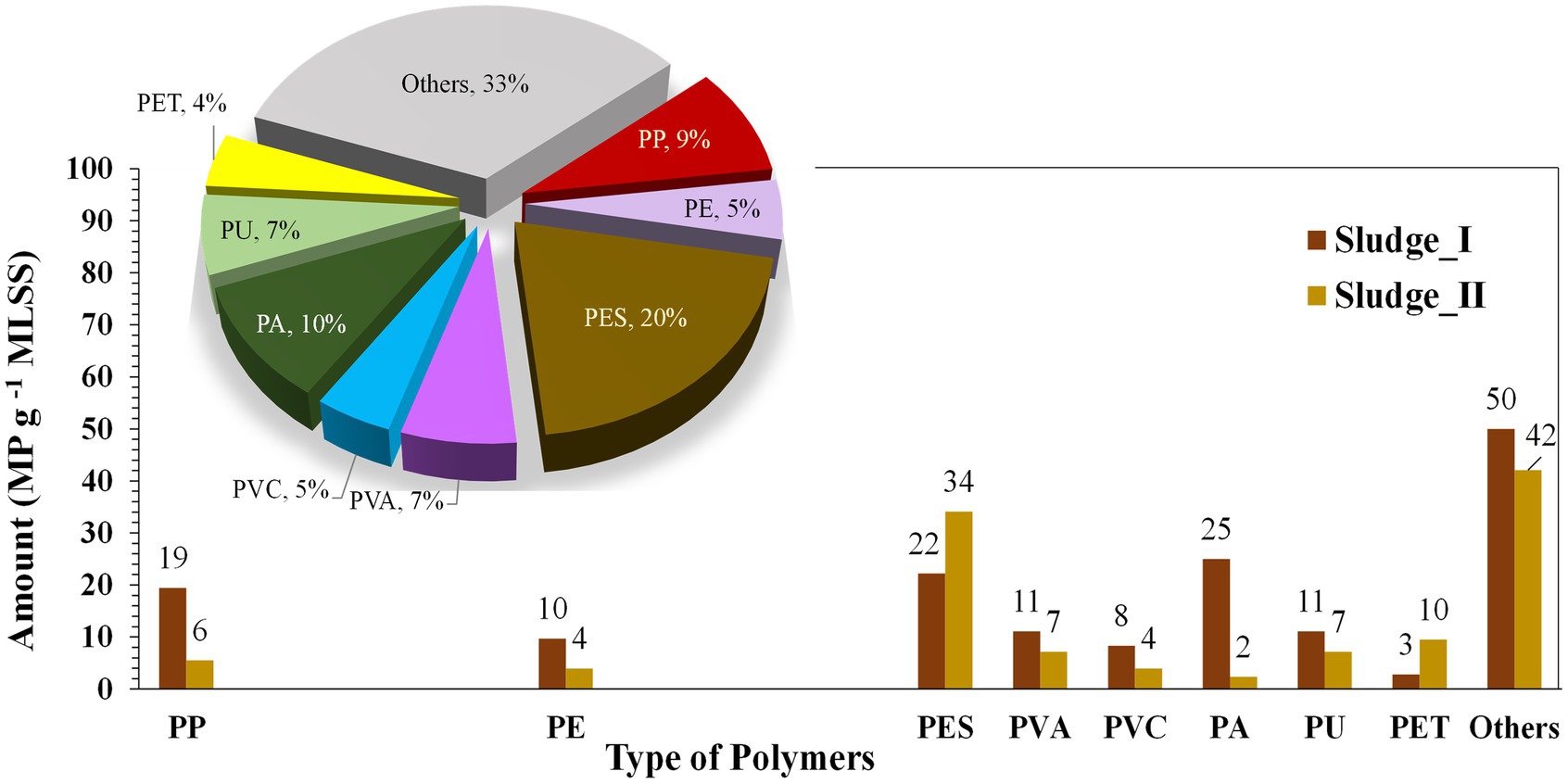
Figure 4. The predominant polymer types in microplastic contamination were analyzed in the sludge samples, with corresponding percentages shown in the pie charts. I and II represent the first and second sludge sampling events, respectively. Abbreviations: PP: polypropylene; PE: polyethylene; PES: polyester; PVA: polyvinyl alcohol; PVC: polyvinylchloride; PA: polyamide; PU: Polyurethane; PET: polyethylene terephthalate.
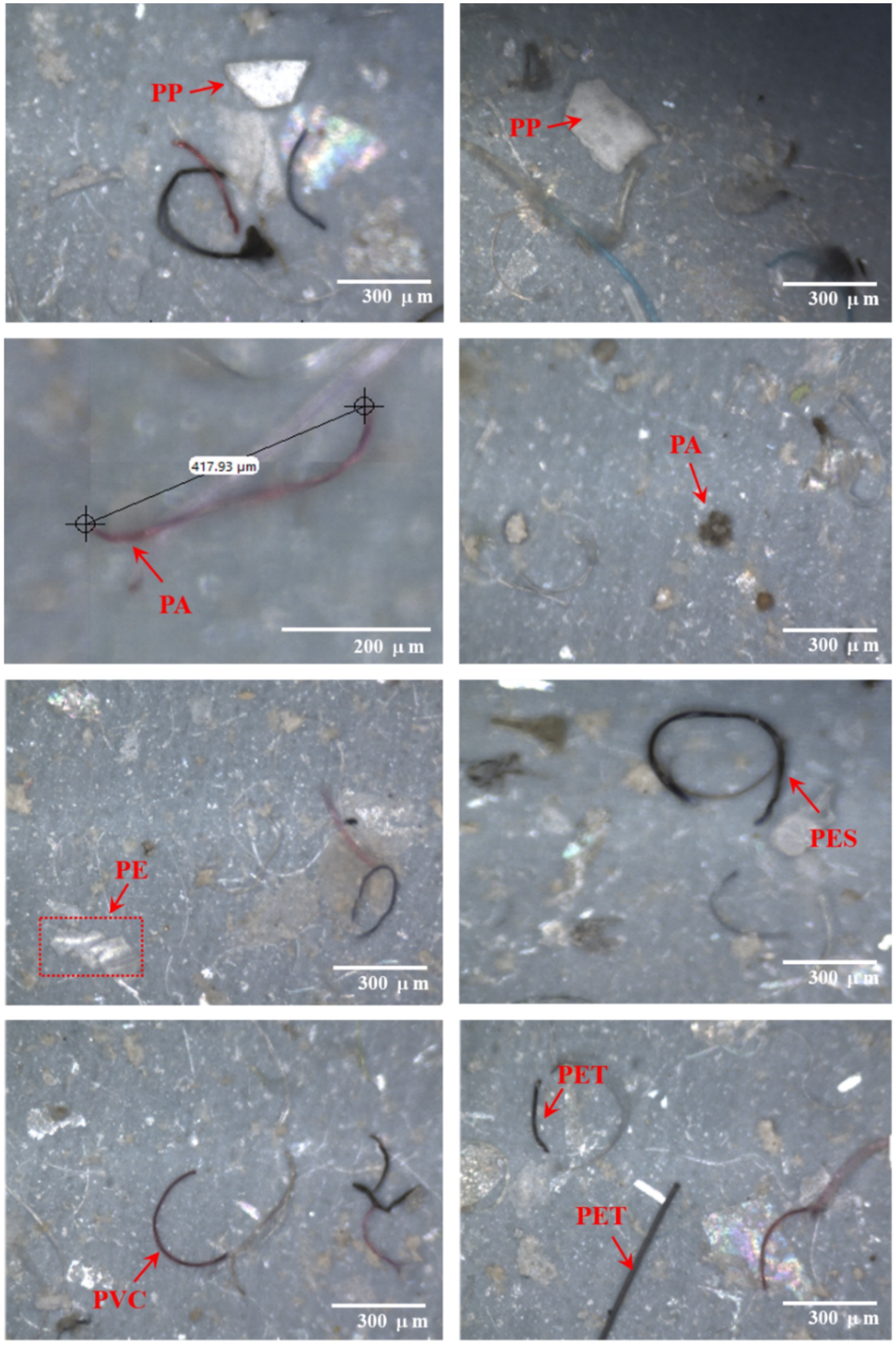
Figure 5. Occurrence of polymer types of microplastics in MBR sludge. PP, polypropylene; PA, polyamide; PE, polyethylene; PES, polyester; PVC, polyvinylchloride; PET, polyethylene terephthalate.
3.4 Bacterial community in the MBR sludge
The bacterial community composition showed similarities between the first and second sampling events, with slight variations in relative abundance. The structure of the dominant phyla is shown in Supplementary Figure S1. The bacterial population was predominantly composed of Proteobacteria (40.5–50.7%), followed by Actinobacteria (10.1–11.5%) and Bacteroidetes (9.6–9.8%). Proteobacteria emerged as the most dominant phylum during MBR operation (Ittisupornrat et al., 2021) as they play a primary role in the biodegradation of various organic compounds within the MBR (Sanguanpak et al., 2019; Ittisupornrat and Theepharaksapan, 2023). Regarding MP concerns, although this study did not confirm the detection of the bacterial community on the MP surface, this study aligned with previous findings, indicating that Proteobacteria and Bacteroidetes are typically the predominant phyla detected in plastisphere environments (Gong et al., 2019; Yang et al., 2020; Aguila-Torres et al., 2022; Nguyen et al., 2022). In particular, Qiu et al. (2024) suggested that the main bacterial communities of Proteobacteria, Actinobacteria, and Bacteroidetes were involved in the metabolism of dissolved organic matter released by MP. Furthermore, previous research investigated their community biofilm on the surface membrane made from polyvinylidene fluoride polymers in treated greywater (Ittisupornrat and Theepharaksapan, 2023).
Although Wang et al. (2022) reported that the dominant PP could improve Clostridia abundance and reduce the Proteobacteria in MBR treating municipal wastewater, this study not obviously observed dominated Clostridia and declining Proteobacteria. In addition to the different origins of wastewater sources, the opposite results may be attributed to other intricate causes that might be further investigated. Some Acidobacteria (5.0–5.1%), Chloroflexi (4.4–5.6%), Chlorobi (0.6–2.0%), and Nitrospirae (1.6–2.1%) were slightly elevated. Only Planctomycetes was obviously decreased from 7.3 to 4.2%. The decreasing trend of this phylum was observed when MBR was simultaneously operated to treat greywater during a long-term period. However, the influence of MPs on this phylum remains unclear, presenting an opportunity for further research to understand its role and impact in MBR systems.
Figure 6 shows the distribution of the bacterial population at the order level in each class. Alphaproteobacteria (18.2–20.4%) and Betaproteobacteria (14.6–24.6%) were the most dominant, similar to Martínez-Campos et al. (2021), who reported that Alphaproteobacteria (24.2%) and Betaproteobacteria (21.4%) were dominantly observed. Within the Alphaproteobacteria class, Caulobacterales, Rhizobiales, Rhodobacteriales, Rhodospirillales, and Shingomonadales orders were observed, with Rhizobiales (5.8–6.8%) being the most predominant. Only two strains could be identified at the genus level: Hyphomicrobium sp. (1.2–1.4%) and Alysiophaera sp. (1.3–2.0%). These orders were observed to be predominant in the biofilm community (Ittisupornrat and Theepharaksapan, 2023). Many studies have pointed out that MPs may encourage bacterial adhesion and subsequently biofilm development and support biofilm community niches because MPs may act as a repository for bacteria (Yang et al., 2019; Martínez-Campos et al., 2021; Sooriyakumar et al., 2022; Ladeia Ramos et al., 2024). Similarly, Jiang et al. (2018) also mentioned that these orders represented important bacterial associations within bacterial communities of the plastisphere. Furthermore, many studies showed that MP biofilms may selectively harbor bacterial pathogens and antibiotic-resistant bacteria (Gong et al., 2019; Parsaeimehr et al., 2023). However, no pathogenic bacteria were found in the bacterial community population of this study, possibly because the greywater constituents contained various detergents and antibacterial agents that could inhibit bacterial pathogen generation. Within the Betaproteobacteria class, two orders of Burkholderiales (6.1–7.0%) and Hydrogenophilales (6.5–16.2%) were observed. Hydrogenophilales remarkably increased from 6.5 to 16.2%. Only one genus was identified as Thiobacillus sp. (3.1–3.9%), whereas the dominant genus (increased from 3.1 to 12.3%) could not be identified as belonging to this order. Regarding the Acidobacteria order, only Blastocatella sp. (2.3–2.7%) was identified. In addition, the abundance of other orders seemed relatively constant in I and II. Unfortunately, most bacteria could not be identified at the genus level. Nitrospira sp. (1.6–2.1%) was the main contributor to the nitrogen transformation that was observed in the Nitrospirales order resulting in high nitrogen removal performance, suggesting that the presence of MPs might not pose a threat to the abundance and functionality of nitrite-oxidizing bacteria (Cui et al., 2021; Wang et al., 2021). Similarly, Huang et al. revealed that MPs drive nitrification by enhancing the abundance of functional microorganisms in aquaculture pond waters, including ammonia- and nitrite-oxidizing bacteria (Huang et al., 2022).
Based on the acquired knowledge from this study, it suggests a possible link between microbial communities and MPs; the precise interactions and mechanisms remain to be fully elucidated. Further research is necessary to determine how these bacterial populations contribute to MP aggregation, degradation, or transport within the MBR system. To avoid any negative impact on MP contamination into the environment, Parsaeimehr et al. (2023) recommended the use of a closed and controlled wastewater treatment system. Therefore, MBR should be offered for prominent wastewater treatment processes to remove and retain MPs in the system (Vuori and Ollikainen, 2022).
3.5 Significant implications and future perspectives
Studies on the removal of MP using membrane technologies are ongoing. Lack of implemented standards or relevant policies for the detection, removal, or discharge of MPs is driving the research on applications of the membrane process focusing on MP removal.
This study highlights the significant potential of MBR technology in addressing the global challenge of MP pollution. The system achieved an average MP removal efficiency of 90%, reducing the effluent concentrations to ≤0.02 MP L−1, while achieving significant COD removal at 74%. These results underscore the ability of MBR to integrate microfiltration with biological activity, enabling simultaneous removal of MPs and decreasing COD. This dual functionality represents a novel advancement over conventional wastewater treatment methods, particularly for real greywater, where complex contaminants and low organic loads pose unique challenges. Furthermore, the role of dominant bacterial communities, such as Alphaproteobacteria and Actinobacteria, in biofilm formation enhances the efficiency of MP removal and offers insights into bacteria can aid in optimizing the MBR performance.
In addition to its technological benefits, MBR systems offer practical solutions for urban and residential wastewater treatment, where space and sustainability are critical. The compact design and high pollutant removal efficiency make MBR systems a promising alternative to conventional methods. Policymakers can integrate MBR technology into existing wastewater infrastructure to meet stricter environmental regulations, reduce MP discharge, and mitigate risks to aquatic ecosystems. Although MBR systems have higher initial costs than conventional methods, their long-term benefits are reduced environmental risks, lower maintenance needs for downstream ecosystems, and potential savings in regulatory compliance. A detailed cost–benefit analysis could further help in improving their economic feasibility, emphasizing their value as a sustainable investment in water management that should be more focused for further study. Furthermore, this study provides a foundation for future research into bacterial interactions with MPs, biofilm dynamics, and operational challenges such as membrane fouling and potential MP leakage. By focusing on these aspects, MBR systems can be further optimized to deliver enhanced performance and sustainability. Overall, these findings highlight the use of MBR technology for greywater treatment as a transformative solution for advancing wastewater treatment while protecting aquatic ecosystems from emerging pollutants.
4 Conclusion
Results of this study highlight the significant potential of MBR technology in mitigating MP pollution, achieving a removal efficiency of 90% and reducing the effluent MP concentrations to ≤0.02 MP L−1. These findings demonstrate that MBR systems can effectively address the growing environmental threat posed by MPs, offering a sustainable solution for greywater treatment in residential and urban applications. Furthermore, they underscore the role of bacterial communities, such as Alphaproteobacteria and Actinobacteria, in biofilm formation and enhanced MP removal. By integrating MBR technology into wastewater treatment frameworks, policymakers can reduce MP discharge into aquatic ecosystems and meet stricter environmental regulations. Future research should explore microbial interactions with MPs and address operational challenges to optimize MBR performance and sustainability. This study lays the foundation for advancing wastewater treatment technologies to protect ecosystems and improve environmental resilience.
Data availability statement
The original contributions presented in the study are publicly available. The sequence datasets of bacterial community for this study can be found in the NCBI Sequence Read Archive (SRA) under BioProject PRJNA1089773 (https://www.ncbi.nlm.nih.gov/bioproject/PRJNA1089773).
Author contributions
SI: Conceptualization, Funding acquisition, Writing – review & editing, Project administration, Writing – original draft. CN: Data curation, Investigation, Methodology, Visualization, Writing – review & editing. AP: Conceptualization, Data curation, Investigation, Writing – review & editing. PS: Conceptualization, Data curation, Investigation, Writing – review & editing. ST: Conceptualization, Data curation, Investigation, Formal Analysis, Visualization, Writing – original draft, Writing – review & editing.
Funding
The author(s) declare that financial support was received for the research and/or publication of this article. This research was supported by the Thailand Science Research and Innovation (Grant numbers. 161421/2022, 180101/2023).
Acknowledgments
The authors would also like to sincerely thank the Faculty of Engineering, Srinakharinwirot University, Thailand for their help and facilitation.
Conflict of interest
The authors declare that the research was conducted in the absence of any commercial or financial relationships that could be construed as a potential conflict of interest.
Generative AI statement
The author(s) declare that Generative AI was used in the creation of this manuscript. ChatGPT by OpenAI, GPT-4 (April 2024) was used to assist with language editing and phrasing.
Publisher’s note
All claims expressed in this article are solely those of the authors and do not necessarily represent those of their affiliated organizations, or those of the publisher, the editors and the reviewers. Any product that may be evaluated in this article, or claim that may be made by its manufacturer, is not guaranteed or endorsed by the publisher.
Supplementary material
The Supplementary material for this article can be found online at: https://www.frontiersin.org/articles/10.3389/fmicb.2025.1519230/full#supplementary-material
Supplementary Figure S1 | Bacterial community compositions at the phylum level of the sludge samples in MBR. Abundance of phyla <2% was termed as Minor phyla. I and II represent the first and second sludge sampling events, respectively.
References
Acarer, S. (2023). A review of microplastic removal from water and wastewater by membrane technologies. Water Sci. Technol. 88, 199–219. doi: 10.2166/wst.2023.186
Aguila-Torres, P., González, M., Maldonado, J. E., Miranda, R., Zhang, L., González-Stegmaier, R., et al. (2022). Associations between bacterial communities and microplastics from surface seawater of the northern Patagonian area of Chile. Environ. Pollut. 306:119313. doi: 10.1016/j.envpol.2022.119313
APHA (2017). Standard methods for the examination of water and wastewater. 23rd Edn. Washington, D.C.: American Public Health Association, American Water Works Association, and Water Environment Federation.
Bayo, J., López-Castellanos, J., and Olmos, S. (2020). Membrane bioreactor and rapid sand filtration for the removal of microplastics in an urban wastewater treatment plant. Mar. Pollut. Bull. 156:111211. doi: 10.1016/j.marpolbul.2020.111211
Cai, Y., Wu, J., Lu, J., Wang, J., and Zhang, C. (2022). Fate of microplastics in a coastal wastewater treatment plant: microfibers could partially break through the integrated membrane system. Front. Environ. Sci. Eng. 16:96. doi: 10.1007/s11783-021-1517-0
Callahan, B., Paul, M. M., and Rosen, M. (2018). DADA2: high-resolution sample inference from Illumina amplicon data. Nat. Methods 13, 581–583. doi: 10.1038/nmeth.3869
Caporaso, J., Stombaugh, J., Costello, E. K., Lozupone, C. A., McDonald, D., Pirrung, M., et al. (2010). QIIME allows analysis of high-throughput community sequencing data. Nat. Methods 7, 335–336. doi: 10.1038/nmeth.f.303
Cui, Y., Gao, J., Zhang, D., Li, D., Dai, H., Wang, Z., et al. (2021). Responses of performance, antibiotic resistance genes and bacterial communities of partial nitrification system to polyamide microplastics. Bioresour. Technol. 341:125767. doi: 10.1016/j.biortech.2021.125767
Egea-Corbacho, A., Martín-García, A. P., Franco, A. A., Quiroga, J. M., Andreasen, R. R., Jørgensen, M. K., et al. (2023). Occurrence, identification and removal of microplastics in a wastewater treatment plant compared to an advanced MBR technology: full-scale pilot plant. J. Environ. Chem. Eng. 11:109644. doi: 10.1016/j.jece.2023.109644
Esfandiari, A., and Mowla, D. (2021). Investigation of microplastic removal from greywater by coagulation and dissolved air flotation. Process. Saf. Environ. Prot. 151, 341–354. doi: 10.1016/j.psep.2021.05.027
Gong, M., Yang, G., Zhuang, L., and Zeng, E. Y. (2019). Microbial biofilm formation and community structure on low-density polyethylene microparticles in lake water microcosms. Environ. Pollut. 252, 94–102. doi: 10.1016/j.envpol.2019.05.090
Habib, R. Z., Thiemann, T., and Al Kendi, R. (2020). Microplastics and wastewater treatment plants—a review. J. Water Resour. Prot. 12, 1–35. doi: 10.4236/jwarp.2020.121001
Hazlehurst, A., Tiffin, L., Sumner, M., and Taylor, M. (2023). Quantification of microfibre release from textiles during domestic laundering. Environ. Sci. Pollut. Res. 30, 43932–43949. doi: 10.1007/s11356-023-25246-8
Hidalgo-Ruz, V., Gutow, L., Thompson, R. C., and Thiel, M. (2012). Microplastics in the marine environment: a review of the methods used for identification and quantification. Environ. Sci. Technol. 46, 3060–3075. doi: 10.1021/es2031505
Hongprasith, N., Kittimethawong, C., Lertluksanaporn, R., Eamchotchawalit, T., Kittipongvises, S., and Lohwacharin, J. (2020). IR microspectroscopic identification of microplastics in municipal wastewater treatment plants. Environ. Sci. Pollut. Res. 27, 18557–18564. doi: 10.1007/s11356-020-08265-7
Huang, J. N., Wen, B., Miao, L., Liu, X., Li, Z. J., Ma, T. F., et al. (2022). Microplastics drive nitrification by enriching functional microorganisms in aquaculture pond waters. Chemosphere 309:136646. doi: 10.1016/j.chemosphere.2022.136646
Ittisupornrat, S., Phetrak, A., Theepharaksapan, S., Mhuantong, W., and Tobino, T. (2021). Effect of prolonged sludge retention times on the performance of membrane bioreactor and microbial community for leachate treatment under restricted aeration. Chemosphere 284:131153. doi: 10.1016/j.chemosphere.2021.131153
Ittisupornrat, S., Phihusut, D., Kitkaew, D., Sangkarak, S., and Phetrak, A. (2019). Performance of dissolved organic matter removal from membrane bioreactor effluent by magnetic powdered activated carbon. J. Environ. Manag. 248:109314. doi: 10.1016/j.jenvman.2019.109314
Ittisupornrat, S., and Theepharaksapan, S. (2023). Greywater treatment performance of a pilot-scale membrane bioreactor and characteristics of bacterial biofilm signatures in permeate effluent. J. Water Process Eng. 55:104098. doi: 10.1016/j.jwpe.2023.104098
Iyare, P. U., Ouki, S. K., and Bond, T. (2020). Microplastics removal in wastewater treatment plants: a critical review. Environ. Sci. Water Res. Technol. 6, 2664–2675. doi: 10.1039/d0ew00397b
Jessieleena, A., Rathinavelu, S., Velmaiel, K. E., John, A. A., and Nambi, I. M. (2023). Residential houses—a major point source of microplastic pollution: insights on the various sources, their transport, transformation, and toxicity behaviour. Environ. Sci. Pollut. Res. 30, 67919–67940. doi: 10.1007/s11356-023-26918-1
Jiang, P., Zhao, S., Zhu, L., and Li, D. (2018). Microplastic-associated bacterial assemblages in the intertidal zone of the Yangtze estuary. Sci. Total Environ. 624, 48–54. doi: 10.1016/j.scitotenv.2017.12.105
JSWA. (1997). Japanese standard methods of the examination of wastewater. Tokyo, Japan: Japanese Sewage Works Association.
Kadac-Czapska, K., Knez, E., Gierszewska, M., Olewnik-Kruszkowska, E., and Grembecka, M. (2023). Microplastics derived from food packaging waste—their origin and health risks. Materials 16:674. doi: 10.3390/ma16020674
Kittipongvises, S., Phetrak, A., Hongprasith, N., and Lohwacharin, J. (2022). Unravelling capability of municipal wastewater treatment plant in Thailand for microplastics: effects of seasonality on detection, fate and transport. J. Environ. Manag. 302:113990. doi: 10.1016/j.jenvman.2021.113990
Kunz, A., Schneider, F., Anthony, N., and Lin, H. T. (2023). Microplastics in rivers along an urban-rural gradient in an urban agglomeration: correlation with land use, potential sources and pathways. Environ. Pollut. 321:121096. doi: 10.1016/j.envpol.2023.121096
Ladeia Ramos, R., Rodrigues dos Santos, C., Pinheiro Drumond, G., de Souza, V., Santos, L., Amaral, C. S., et al. (2024). Critical review of microplastic in membrane treatment plant: removal efficiency, environmental risk assessment, membrane fouling, and MP release. Chem. Eng. J. 480:148052. doi: 10.1016/j.cej.2023.148052
Lamine, M., Samaali, D., and Ghrabi, A. (2012). Greywater treatment in a submerged membrane bioreactor with gravitational filtration. Desalin. Water Treat. 46, 182–187. doi: 10.1080/19443994.2012.677553
Lares, M., Ncibi, M. C., Sillanpää, M., and Sillanpää, M. (2018). Occurrence, identification and removal of microplastic particles and fibers in conventional activated sludge process and advanced MBR technology. Water Res. 133, 236–246. doi: 10.1016/j.watres.2018.01.049
Li, L., Liu, D., Song, K., and Zhou, Y. (2020). Performance evaluation of MBR in treating microplastics polyvinylchloride contaminated polluted surface water. Mar. Pollut. Bull. 150:110724. doi: 10.1016/j.marpolbul.2019.110724
Liu, R., Huang, X., Chen, L., Wen, X., and Qian, Y. (2005). Operational performance of a submerged membrane bioreactor for reclamation of bath wastewater. Process Biochem. 40, 125–130. doi: 10.1016/j.procbio.2003.11.038
Liu, X., Yuan, W., Di, M., Li, Z., and Wang, J. (2019). Transfer and fate of microplastics during the conventional activated sludge process in one wastewater treatment plant of China. Chem. Eng. J. 362, 176–182. doi: 10.1016/j.cej.2019.01.033
Liu, W., Zhang, J., Liu, H., Guo, X., Zhang, X., Yao, X., et al. (2021). A review of the removal of microplastics in global wastewater treatment plants: characteristics and mechanisms. Environ. Int. 146:106277. doi: 10.1016/j.envint.2020.106277
Martínez-Campos, S., González-Pleiter, M., Fernández-Piñas, F., Rosal, R., and Leganés, F. (2021). Early and differential bacterial colonization on microplastics deployed into the effluents of wastewater treatment plants. Sci. Total Environ. 757:143832. doi: 10.1016/j.scitotenv.2020.143832
Masura, J., Baker, J. E., and Foster, G. D.. (2015). Laboratory methods for the analysis of microplastics in the marine environment: recommendations for quantifying synthetic particles in waters and sediments. NOAA Marine Debris Division: Silver Spring, MD (2015).
Mennekes, D., and Nowack, B. (2023). Predicting microplastic masses in river networks with high spatial resolution at country level. Nat. Water 1, 523–533. doi: 10.1038/s44221-023-00090-9
Mishra, S., Swain, S., Sahoo, M., Mishra, S., and Das, A. P. (2022). Microbial colonization and degradation of microplastics in aquatic ecosystem: a review. Geomicrobiol J. 39, 259–269. doi: 10.1080/01490451.2021.1983670
Nguyen, H. T., Choi, W., Kim, E. J., and Cho, K. (2022). Microbial community niches on microplastics and prioritized environmental factors under various urban riverine conditions. Sci. Total Environ. 849:157781. doi: 10.1016/j.scitotenv.2022.157781
Parsaeimehr, A., Miller, C. M., and Ozbay, G. (2023). Microplastics and their interactions with microbiota. Heliyon 9:e15104. doi: 10.1016/j.heliyon.2023.e15104
Pedrotti, M. L., Petit, S., Eyheraguibel, B., Kerros, M. E., Elineau, A., Ghiglione, J. F., et al. (2021). Pollution by anthropogenic microfibers in north-West Mediterranean Sea and efficiency of microfiber removal by a wastewater treatment plant. Sci. Total Environ. 758:144195. doi: 10.1016/j.scitotenv.2020.144195
Peydro, M. A., Parres, F., Crespo, J. E., and Navarro, R. (2013). Recovery of recycled acrylonitrile-butadiene-styrene, through mixing with styrene-ethylene/butylene-styrene. J. Mater. Process. Technol. 213, 1268–1283. doi: 10.1016/j.jmatprotec.2013.02.012
Piorkowska, E., Kulinski, Z., Galeski, A., and Masirek, R. (2006). Plasticization of semicrystalline poly(l-lactide) with poly(propylene glycol). Polymer 47, 7178–7188. doi: 10.1016/j.polymer.2006.03.115
Qiu, X., Ma, S., Pan, J., Cui, Q., Zheng, W., Ding, L., et al. (2024). Microbial metabolism influences microplastic perturbation of dissolved organic matter in agricultural soils. ISME J. 18:wrad017. doi: 10.1093/ismejo/wrad017
Razeghi, N., Hamidian, A. H., Wu, C., Zhang, Y., and Yang, M. (2021). Microplastic sampling techniques in freshwaters and sediments: a review. Environ. Chem. Lett. 19, 4225–4252. doi: 10.1007/s10311-021-01227-6
Reddy, A. S., and Nair, A. T. (2022). The fate of microplastics in wastewater treatment plants: an overview of source and remediation technologies. Environ. Technol. Innov. 28:102815. doi: 10.1016/j.eti.2022.102815
Rodrigues, J. P., Duarte, A. C., Santos-Echeandía, J., and Rocha-Santos, T. (2019). Significance of interactions between microplastics and POPs in the marine environment: a critical overview. TrAC Trends Anal. Chem. 111, 252–260. doi: 10.1016/j.trac.2018.11.038
Rolsky, C., and Kelkar, V. (2021). Degradation of polyvinyl alcohol in us wastewater treatment plants and subsequent nationwide emission estimate. Int. J. Environ. Res. Public Health 18:6027. doi: 10.3390/ijerph18116027
Sanguanpak, S., Chiemchaisri, W., and Chiemchaisri, C. (2019). Membrane fouling and micro-pollutant removal of membrane bioreactor treating landfill leachate. Rev. Environ. Sci. Biotechnol. 18, 715–740. doi: 10.1007/s11157-019-09514-z
Santasmasas, C., Rovira, M., Clarens, F., and Valderrama, C. (2013). Grey water reclamation by decentralized MBR prototype. Resour. Conserv. Recycl. 72, 102–107. doi: 10.1016/j.resconrec.2013.01.004
Segata, N., Izard, J., Waldron, L., Gevers, D., Miropolsky, L., Garrett, W. S., et al. (2011). Metagenomic biomarker discovery and explanation. Genome Biol. 12:R60. doi: 10.1186/gb-2011-12-6-r60
Setälä, O., Fleming-Lehtinen, V., and Lehtiniemi, M. (2014). Ingestion and transfer of microplastics in the planktonic food web. Environ. Pollut. 185, 77–83. doi: 10.1016/j.envpol.2013.10.013
Song, K., and Li, L. (2023). Occurrence of microplastics in wastewater treatment plants. Microplast. Urban Water Manag., 91–117. doi: 10.1002/9781119759379.ch3
Sooriyakumar, P., Bolan, N., Kumar, M., Singh, L., Yu, Y., Li, Y., et al. (2022). Biofilm formation and its implications on the properties and fate of microplastics in aquatic environments: a review. J. Hazard. Mater. Adv. 6:100077. doi: 10.1016/j.hazadv.2022.100077
Talvitie, J., Mikola, A., Koistinen, A., and Setälä, O. (2017). Solutions to microplastic pollution – removal of microplastics from wastewater effluent with advanced wastewater treatment technologies. Water Res. 123, 401–407. doi: 10.1016/j.watres.2017.07.005
Teuten, E. L., Saquing, J. M., Knappe, D. R. U., Barlaz, M. A., Jonsson, S., Björn, A., et al. (2009). Transport and release of chemicals from plastics to the environment and to wildlife. Philos. Trans. R. Soc. B Biol. Sci. 364, 2027–2045. doi: 10.1098/rstb.2008.0284
Vuori, L., and Ollikainen, M. (2022). How to remove microplastics in wastewater? A cost-effectiveness analysis. Ecol. Econ. 192:107246. doi: 10.1016/j.ecolecon.2021.107246
Wang, Z., Gao, J., Dai, H., Zhao, Y., Li, D., Duan, W., et al. (2021). Microplastics affect the ammonia oxidation performance of aerobic granular sludge and enrich the intracellular and extracellular antibiotic resistance genes. J. Hazard. Mater. 409:124981. doi: 10.1016/j.jhazmat.2020.124981
Wang, Q. Y., Li, Y. L., Liu, Y. Y., Zhou, Z., Hu, W. J., Lin, L. F., et al. (2022). Effects of microplastics accumulation on performance of membrane bioreactor for wastewater treatment. Chemosphere 287:131968. doi: 10.1016/j.chemosphere.2021.131968
Wei, S., Luo, H., Zou, J., Chen, J., Pan, X., Rousseau, D. P. L., et al. (2020). Characteristics and removal of microplastics in rural domestic wastewater treatment facilities of China. Sci. Total Environ. 739:139935. doi: 10.1016/j.scitotenv.2020.139935
Wesch, C., Elert, A. M., Wörner, M., Braun, U., Klein, R., and Paulus, M. (2017). Assuring quality in microplastic monitoring: about the value of clean-air devices as essentials for verified data. Sci. Rep. 7:5424. doi: 10.1038/s41598-017-05838-4
Yang, Y., Liu, G., Song, W., Ye, C., Lin, H., Li, Z., et al. (2019). Plastics in the marine environment are reservoirs for antibiotic and metal resistance genes. Environ. Int. 123, 79–86. doi: 10.1016/j.envint.2018.11.061
Keywords: microplastic contamination, membrane bioreactor, greywater treatment, polyester fibers, bacterial community
Citation: Ittisupornrat S, Namyuang C, Phetrak A, Sriromreun P and Theepharaksapan S (2025) Microplastic contamination and removal efficiency in greywater treatment using a membrane bioreactor. Front. Microbiol. 16:1519230. doi: 10.3389/fmicb.2025.1519230
Edited by:
Tomohiro Tobino, The University of Tokyo, JapanCopyright © 2025 Ittisupornrat, Namyuang, Phetrak, Sriromreun and Theepharaksapan. This is an open-access article distributed under the terms of the Creative Commons Attribution License (CC BY). The use, distribution or reproduction in other forums is permitted, provided the original author(s) and the copyright owner(s) are credited and that the original publication in this journal is cited, in accordance with accepted academic practice. No use, distribution or reproduction is permitted which does not comply with these terms.
*Correspondence: Suthida Theepharaksapan, U3V0aGlkYXRAZy5zd3UuYWMudGg=