- 1College of Life Science, Baicheng Normal University, Baicheng, China
- 2Xiamen Jinfen Agricultural Research Institute, Xiamen, China
- 3Terra Research and Teaching Centre, Microbial Processes and Interactions (MiPI), Gembloux Agro-Bio Tech, University of Liège, Gembloux, Belgium
- 4Key Laboratory of Development and Application of Rural Renewable Energy, Biogas Institute of Ministry of Agriculture and Rural Affairs, Chengdu, China
Obesity is a growing global health challenge influenced by genetic and environmental factors. With economic development, obesity rates continue to rise globally. Recent research highlights the critical role of gut microbiota in obesity pathogenesis, particularly through microbial metabolites affecting metabolism and energy homeostasis. Recent studies indicate that gut microbiota significantly influences obesity development through metabolic interactions, including the regulation of glucose and lipid metabolism. Gut microbiota is involved in the metabolism of various nutrients such as glucose and lipids, and the change of gut microbiota leads to insulin resistance, adipose tissue accumulation and metabolic disorders, thus mediating the occurrence and development of metabolic diseases. For example, beneficial bacteria such as Bifidobacterium and Lactobacillus, which are in high abundance in animal intestinal tract, can prevent obesity by enhancing the intestinal barrier, improving insulin sensitivity and improving metabolic disorders. Many of these effects are mediated by metabolites produced by intestinal microbiota, such as short-chain fatty acids, which play an important role in preventing and treating obesity. In this research review, we used the PubMed database and Google Scholar and web of science to search for relevant literature within recent 20 years on the topic of microbes and microbial metabolites in relation to obesity. Key words used were obesity, metabolic diseases, gut microbiota, metabolic disorders, gut microbial metabolites to search for references. The purpose of this review article was to describe the current knowledge on the impact of gut microbiota and their metabolites on obesity, in order to provide a more comprehensive understanding of the relationship between gut microbiota and obesity for future clinical intervention and prevention of obesity.
1 Introduction
There are approximately 100 trillion bacteria in the human intestine, which make up the intestinal microbiota. The composition of the intestinal microbes is affected by pH value, oxygen and nutrients (Suau et al., 1999). The intestinal microbiota plays a key role in many physiological and pathological processes in humans (Becattini et al., 2016). Disturbances in the intestinal flora are closely related to the occurrence of metabolic diseases (Sittipo et al., 2018). There is increasing evidence that the intestinal microbiota regulates the body responses by improving the intestinal micro-environment and plays a key role in physiological homeostasis (Vallianou et al., 2021).
The composition of the intestinal microbe is mainly affected by environment and genetic factors (Rothschild et al., 2018). When genetic factors or environment change especially diet change, the intestinal microbial diversity will also change, which is called intestinal microbial imbalance (Vallianou et al., 2021). Conversely, intestinal microbial imbalance may also lead to metabolic disorders in the host, further causing the occurrence of metabolic diseases such as obesity, nonalcoholic fatty liver disease (NAFLD) and diabetes (Biedermann and Rogler, 2015; Hoozemans et al., 2021). A study has shown that animals of the same species with different body weights have significant differences in the composition of their intestinal microbiota (Saad et al., 2016). Intestinal microbial fermentation degrades carbohydrates in the intestine and produces short-chain fatty acids (SCFAs), such as acetate, propionate and butyrate (Cummings et al., 1987). The intestinal microbiota can affect lipid metabolism through the metabolites of short-chain fatty acids. Studies have shown that SCFAs are associated with the prevention and treatment of obesity-related insulin resistance (De Vadder et al., 2014; Mollica et al., 2017). The reduction of SCFAs may play an important role in reducing insulin sensitivity and the occurrence of obesity. In addition, SCFAs exert beneficial effects on body weight, inflammation, insulin sensitivity and glucose homeostasis (Canfora et al., 2017; Bouter et al., 2018). Apart from short-chain fatty acids, lipopolysaccharide, peptidoglycan, and trimethylamine are also important gut microbial metabolites, which play important role in intestinal homeostasis.
In this article, we mainly talk about three parts, the microbes preventing obesity, the microbes promoting obesity and the microbial metabolites with their effects. This review aims to discuss the effects of beneficial intestinal microbes such as Lactobacillus, Bifidobacterium and harmful bacteria such as Enterobacter clocae, as well as microbial metabolites on obesity in order to provide scientific reference for the prevention and treatment of obesity and metabolic diseases.
2 Gut microbiota, microbial metabolites and obesity
Currently, with the increasing incidence of obesity, it has become a major health problem from all over the world, affecting the occurrence of many chronic diseases, such as NAFLD, hyperlipidemia and diabetes (Scherrer et al., 2018; Cuthbertson et al., 2021). A study has shown that the intestinal flora plays a direct role in the development of obesity by regulating host genes involved in energy storage and consumption, thereby changing energy and fat storage (Tsukumo et al., 2015). Under normal physiological conditions, lipogenesis and lipolysis maintain a dynamic balance, excessive high-fat diet is the main cause of this imbalance (Wang et al., 2021), which may lead to lipid metabolism disorders. Activated Protein Kinase (AMPK) is an intracellular energy sensor involved in lipid metabolism. A high-fat diet can inactivate AMPK by regulating target genes, leading to excessive accumulation of fat in the body. In recent years, more and more studies have found that probiotics can improve lipid metabolism disorders induced by high-fat diet through the AMPK signaling pathway. GPR43 is an important member of G protein-coupled receptors (GPRs) involved in lipid metabolism in adipocytes. In an experiment on mice fed a high fat diet (HFD), GPR43 levels increased significantly after drug treatment. Its mechanism may be to regulate AMPK activity through the Ca2+/CAMKK pathway. BAA6 activates peroxisome proliferator-activated receptor α (PPARα) by targeting the acetic acid produced by the GPR43/AMPK signaling pathway, thereby promoting fatty acid oxidation (Ray et al., 2018). At the same time, a study has found that probiotics can significantly inhibit the activity of acetyl-CoA carboxylase (ACC) by activating the AMPK signaling pathway, thereby directly inhibiting lipid synthesis (Rahman et al., 2021). In addition, a high-fat diet can lead to excessive secretion of leptin by adipocytes. High levels of leptin can directly inhibit the activation of AMPK signaling, resulting in lipid metabolism disorders (Lindholm et al., 2013). A study has shown that probiotics can improve the development of obesity and metabolic diseases by inhibiting the secretion of leptin (Jeung et al., 2018). Probiotics can not only break down fats, but also promote digestion and metabolic functions by regulating intestinal microorganisms.
2.1 Microbes preventing obesity
2.1.1 Lactobacillus
In recent years, Lactobacillus has been a research hotspot in the Firmicutes, it can be used to treat obesity and improve intestinal microbial diversity (Song et al., 2021). In a study on preventing obesity by regulating the gut microbiota and protecting intestinal health, the proportion of Firmicutes in mice increased from 65 to 78%, while the proportion of Bacteroidetes decreased from 26 to 11% during the occurrence of obesity (Han et al., 2021). The ratio of Firmicutes to Bacteroidetes (F/B) in the mouse intestine is also an important factor affecting obesity (Ley et al., 2006). When the rate of F/B increases, the expression of polysaccharide digestive enzymes in the intestine increases, thereby improving the efficiency of obtaining energy from food and making obesity more likely to occur (Turnbaugh et al., 2006). A study has shown that the F/B ratio was increased in diabetic obese mice compared with normal mice. Among probiotics, Lactobacillus and Bifidobacterium are relevant to the treatment of metabolic syndrome. It exhibits significant anti-obesity effects in rodents and humans, for example regulating body weight, improving blood glucose and lipid metabolism, reducing insulin resistance (Gibson et al., 2017). Lactobacillus rhamnosus CGMCC1.3724 combined with a low-calorie diet resulted in significant weight loss in obese women compared with obese men (Sanchez et al., 2017). A study has shown that Lactobacillus reuteri intervention can significantly inhibit the weight gain of mice, while mice supplemented with Lactobacillus reuteri L6798 have a significant increase in body weight (Fåk and Bäckhed, 2012). Other study has found that (Kang et al., 2022) Lactobacillus sachusetts can reduce the levels of total cholesterol (TC), triglyceride (TG) and lipopolysaccharide (LPS) in the blood, while inhibiting the activation of nuclear factor kappa-B (NF-κB) and the expression of tumor necrosis factor α (TNF-α) induced by a high-fat diet. In obese mice, Lactobacillus sarcopeniae reduces obesity by regulating intestinal microiota, inducing AMPK activation, and increasing the expression of peroxisome proliferator-activated receptor-γ coactivator-1α (PGC-1α). The study by Kang et al. (2022) showed that Lactobacillus acidophilus down-regulated the expression of proinflammatory cytokines such as TNF-α, interleukin-1β (IL-1β) and interferon-γ (IFN-γ) in mice fed a high-fat diet, and up-regulated the expression of key transcription factors involved in lipid metabolism such as PPARα, PPAR-γ and C/EBP-α. By inhibiting the expression of NF-κB, thereby improving insulin sensitivity and restoring the ratio of Firmicutes to Bacteroidetes in the intestine, Lactobacillus has now become a candidate probiotic for improving obesity and related diseases (such as hyperlipidemia and non-alcoholic fatty liver disease) (Wang et al., 2023; Lau et al., 2024).
2.1.2 Akkermansia muciniphila
Studies have shown that the diversity of intestinal microbiota in obese mice is significantly lower than that in normal mice (Li et al., 2022). In addition to the Firmicutes and Bacteroidetes, Akkermansia muciniphila has also received extensive attention and research, it has been reported to have closely links with human obesity (Lee et al., 2019). In recent years, more and more studies have shown that the relative abundance of Akkermansia muciniphila has a significant negative correlation with obesity-related indicators such as body mass index (BMI) and waistline (Dao et al., 2016; De La Cuesta-Zuluaga et al., 2017; Journey et al., 2020). Higher levels of Akkermansia muciniphila correlate with leanness by enhancing metabolic rate and restoring intestinal barrier function (Plovier et al., 2017). In a recent study of overweight women with PCOS, the abundance of Akkermansia muciniphila in the intestines of women who ate a healthy diet and had a lower BMI (Łagowska et al., 2022). One study showed that mice treated with anthocyanins not only had healthier intestines, but also contained a higher proportion of Akkermansia muciniphila (Li et al., 2022) which was able to reduce inflammation and obesity by reducing lipid deposition and inhibiting the proportion of macrophages (Xie et al., 2021). In addition, Marvasti et al. (2020) first reported that the relative abundance of Akkermansia muciniphila was significantly reduced in obese people in Iran. Therefore, Akkermansia muciniphila is beneficial to intervene and treat human obesity as well as metabolic diseases.
2.1.3 Bifidobacterium
Currently, a large number of studies have shown that Bifidobacteria play a beneficial role in improving obesity-related symptoms (Gibson and Roberfroid, 1995; Kalliomäki et al., 2008). The increased abundance of Bifidobacterium in the intestine can increase the content of SCFAs, reduce the content of LPS and improve the intestinal barrier function. Compared with normal person, the abundance of Bifidobacterium in the intestine of obese people is significantly reduced (Singh et al., 2017). Oral Bifidobacterium to mice can reduce their weight gain (Schroeder et al., 2018; Zou et al., 2018) and prevent metabolic disorders induced by a high-fat diet (Mischke et al., 2018). Supplementation with Bifidobacteria can reduce the levels of TC, TG and glucose, at the same time effectively reduced the body weight and fat deposition. This effect was mainly due to the metabolites of Bifidobacteria regulating low-density lipoprotein cholesterol (LDL-C), thereby improving the abnormal fat metabolism in obese mice (Chen M. et al., 2021). In another study, supplementation with Bifidobacterium improved antioxidant capacity in overweight women, possibly due to the interaction of short-chain fatty acids with G protein-coupled receptors, affecting adipocyte sensitivity to insulin, thereby regulating energy metabolism (Gomes et al., 2017). The use of Bifidobacterium improved dyslipidemia caused by obesity, possibly by inhibiting the expression of intestinal adipokines during the fasting period, thereby further affecting the production of short-chain fatty acids (Gomes et al., 2017). In summary, Bifidobacterium has important research value in the prevention and treatment of obesity.
2.2 Microbes promoting obesity 2.2.1 Enterobacter clocae
In addition to the above-mentioned beneficial bacteria that can prevent and alleviate obesity, there are also some intestinal flora that are considered to be potential bacteria that cause obesity. For example, Enterobacter clocae is a Gram-negative bacterium isolated from the intestinal microbiota of obese people. When oral gavage it into germ-free mice, resulting in obesity and insulin resistance (Fei and Zhao, 2013). Another study on 123 obese people who used dietary intervention to control intestinal microbiota showed that in the process of weight loss, the relative abundance of Enterobacteriaceae and Desulfovibrionaceae in the intestine decreased. At the same time, the relative abundance of Escherichia and Shigella was positively correlated with systolic blood pressure, Klebsiella was positively correlated with fasting blood glucose (FBG), Citrobacter was positively correlated with body weight, BMI and IL-1β (Xiao et al., 2014). The study found that acute lung infection induced by intranasal drip of Escherichia coli can aggravate lipid metabolism disorders in obese mice. Meanwhile, serum free fatty acids (FFA), TG and very low density lipoprotein (VLDL) were significantly increased after infection, while TC, LDL-C and high density lipoprotein cholesterol (HDL-C) levels showed a downward trend after infection, among which HDL-C decreased the most (Adeghate, 2004). As a lipoprotein, HDL-C not only promotes the transport of cholesterol, but is also very important in the process of anti-infection. Its reduction may aggravate the disorder of blood lipid metabolism in obese mice. In addition, intestinal microbial diversity imbalance is positively correlated with endotoxin content. When the abundance of Escherichia coli increases and the dominant bacteria decreases significantly, the intestinal mucosal structure is destroyed and the level of enteric endotoxin gradually increases. Serum endotoxin can enter the liver through the portal vein, inducing hepatocytes to produce inflammatory cytokines such as tumor necrosis factor and interleukin-1, thereby promoting the development of non-alcoholic fatty liver disease (Cui et al., 2019). Therefore, reducing the relative abundance of Escherichia coli is also an effective way to intervene and treat obesity and metabolic diseases. The relationships between gut microbiota and obesity-related phenotypes summarized in this article are shown in Table 1.
2.3 Microbial metabolites and their effects
2.3.1 Short-chain fatty acids (SCFAs)
Short-chain fatty acids (SCFAs), are metabolites of intestinal microbiota, particularly acetate, propionate, and butyrate, regulate metabolic homeostasis by modulating gut hormone secretion, energy expenditure, and insulin sensitivity. Their role in obesity prevention and treatment is an emerging area of interest in metabolic research. More than 95% of all SCFAs and are usually present in the intestine at a concentration ratio of 3:1:1 (Sun et al., 2017; Faits et al., 2020). They are mainly produced by the breakdown of carbohydrates such as resistant starch (Feng et al., 2018) and can be converted into each other in the body. Among them, acetic acid and propionic acid are usually transported to the liver to participate in energy metabolism. Acetate can be used for the synthesis of cholesterol, fatty acids and glutamine, propionate is an important substrate for gluconeogenesis and can inhibit the synthesis of fat (Kimura et al., 2019; Blaak et al., 2020). SCFAs play an important role in maintaining the homeostasis of the internal environment. They can maintain the homeostasis of the microbiota and promote digestion and absorption. They can also affect body weight by regulating fat synthesis, increasing insulin sensitivity, improving glucose tolerance and thus affecting lipid metabolism (Blaak et al., 2020). The experimental results of Den Besten et al. (2015) showed that SCFAs activate carnitine palmitoyl transferase 1 (CPT1) to participate in the supply of fatty acid substrates, among which the down-regulation of PPARγ activates the UCP2-AMPK-ACC metabolic pathway, converting fat into fatty acid oxidation. Therefore, short-chain fatty acids play a key role in the pathophysiology of obesity. In addition, SCFAs can reduce intestinal barrier dysfunction and metabolic abnormalities induced by a high-fat diet. A study has reported (Matheus et al., 2017) that butyrate alleviates high-fat diet-induced metabolic disorders in mice by inhibiting insulin resistance, which is related to the secretory function of pancreatic B cells and the intestinal barrier mediated by tight junction proteins.
SCFAs can regulate fat production, improve insulin resistance and reduce free fatty acids in overweight people (Zhu et al., 2020), the mechanism is related to the increased secretion of gastrointestinal hormones. SCFAs mainly regulate energy metabolism by modulating the GPRs pathway, activating the MAPK signal transduction pathway of intestinal epithelial cells, and promoting the secretion of hormones such as 5-hydroxytryptamine (5-HT) and gastroinhibitory peptide (GIP) by L cells in the intestine, thereby increasing satiety, reducing gastric emptying and reducing body weight (Aktar et al., 2020). Butyric acid in SCFAs can be converted to acetyl-CoA in cells and oxidized in the circulation to produce ATP, which can activate the expression of regulatory gluconeogenic genes; Propionate binds to neuronal GPRs, resulting in a decrease in cAMP levels, thereby regulating glucose and lipid metabolism in the body (Morrison and Preston, 2016); Acetate may be converted to acetyl-CoA and participate in the tricarboxylic acid cycle in various peripheral tissues. It may also affect metabolism through increased oxidative capacity via 5′AMP-activated AMPK phosphorylation effects (Yamashita et al., 2009; Maruta et al., 2016). As one of the important signaling molecules regulating intestinal mucosal immunity, SCFAs can regulate PPARγ/GPR-mediated adipogenesis by inhibiting histone deacetylase activity, increasing the activity of triglyceride-degrading lipase and sensitive lipase (Blaak et al., 2020). SCFAs can regulate extracellular specific G protein-coupled receptors, mainly intervening in the free fatty acid receptors GPR41, GPR43 and hydroxycarboxylic acid receptor 2 (HCAR2) present in intestinal endocrine cells, enteric neurons and intestinal epithelial cells. GPR43 is highly expressed in white adipose tissue, neutrophils and pancreatic B cells (Lu et al., 2016). Intestinal microbial diversity imbalance often leads to an imbalance in SCFAs levels, thereby affecting metabolism and causing obesity.
2.3.2 Lipopolysaccharide (LPS)
LPS, which is an important molecule in the cell wall of Gram-negative bacteria, is the main metabolite secreted after the lysis of intestinal microbes. There is approximately 1 g LPS in human intestine (Erridge et al., 2007). In healthy individuals, the intestinal epithelium is an effective barrier against LPS, but in disease states, impaired intestinal epithelial function may cause the translocation of LPS. However, low concentrations of LPS can be detected in the blood of healthy animals and humans, indicating that small amounts of LPS continuously cross the intestinal epithelial barrier to the blood (Laugerette et al., 2011). Studies have shown that a high-fat diet for 4 weeks increased the plasma LPS concentration of mice by two to three times and increased the proportion of LPS containing microbiota in the intestine. Long-term infusion of LPS can induce weight gain in mice, so obesity is related to the increase of LPS in plasma (Cani et al., 2007).
LPS contains lipid A, which can pass the intestinal mucosa through tight junctions. It has no toxic effects, but as a nonspecific immunogen, it interacts with the host after entering the micro-circulation. The secreted bio-active molecules can cause fever and inflammatory response in the body. This inflammatory response interferes with the metabolism of glucose and lipids, promotes the production and storage of adipose tissue, leads to metabolic diseases such as insulin resistance (Yaribeygi et al., 2019). In turn, LPS forms a complex with lipopolysaccharide binding protein, acts on Toll-like receptor 4 (TLR4) on the surface of intestinal immune cells, activates the Myd88/NF-κB signaling pathway, thus affects changes in the structure of the intestinal flora (Larraufie et al., 2018). TLR4 is the main mediator of inflammation and immune response, it is widely distributed on the membrane surface of various immune cells, especially monocytes and macrophages. After LPS binds to TLR4 on macrophages and adipose tissue, it activates the gene expression of pro-inflammatory proteins, such as NF-κB and activator protein 1 (AP-1), through the activation of the MyD88 signaling pathway. It activates a series of inflammatory and immune responses, which in turn affects the normal lipid metabolism process and leads to the occurrence of lipid metabolism-related diseases (Lancaster et al., 2018).
The intestinal mucosa plays an important role in nutrient absorption, maintaining intestinal barrier function, and preventing bacterial translocation (Yuan et al., 2018). Studies have shown that a high-fat diet can change the composition of intestinal microorganisms, damage the intestinal mucosa, alter intestinal permeability, and mediate the occurrence of obesity and insulin resistance (Tomas et al., 2016). Obesity is considered a chronic inflammatory response, LPS can stimulate fat deposition and exacerbate inflammation by activating receptors in adipocytes, such as TLR4. During obesity, intestinal permeability increases, which is manifested by increased levels of inflammatory factors in plasma as well as LPS. LPS is an inducer of inflammation, which activates the NF-κB signaling pathway, leading to chronic inflammation and further increasing fat accumulation.
2.3.3 Bile acid (BA)
BA is an important component of bile. It is synthesized by cholesterol oxidation in the liver via cytochrome P450, it is mainly involved in the absorption of bile and fat-soluble vitamins in the intestine (Chiang, 2002). The primary bile acids produced by the direct decomposition of cholesterol mainly combine with glycine in the human liver, increasing the solubility of bile to play a detoxification role. Primary bile acids in the intestine are synthesized into secondary bile acids under the action of the microbes and metabolized in the intestine. The composition of the bile acid profile is closely related to the diversity of intestinal flora. Dysbiosis of intestinal flora can lead to an imbalance in the ratio of primary bile acids to secondary bile acids, causing disorders in signal metabolic pathways, thereby promoting the occurrence of metabolic diseases such as obesity (Sayin et al., 2013). Rats with non-alcoholic fatty liver model fed a high-fat diet mainly showed increased in secondary bile acids (Jiao et al., 2018), and a significant imbalance in the ratio of primary and secondary bile acids. Arab et al. (2017) found that secondary bile acids are associated with the occurrence of non-alcoholic fatty liver disease. The mechanism may be that the synthesis of secondary bile acids leads to increasing permeability of the intestinal barrier, further inducing endotoxemia, resulting in insulin resistance, finally promoting the occurrence of metabolic diseases such as obesity.
A study has shown that BA also plays a role in regulating metabolism as hormones or signaling molecules (Houten et al., 2006). Bile salt hydrolase (BSH) in the intestine can dissociate taurine-and glycine-bound bile acids to form free bile acids. Bile acids converted by intestinal microorganisms can activate bile acid receptors in the host liver, intestine, and peripheral tissues, such as farnesoid X receptor (FXR) and G protein-coupled bile acid receptor 5 (TGR5), thereby activating different signaling pathways to regulate enterohepatic circulation and also regulate triglyceride, cholesterol, energy, and glucose homeostasis. The FXR-mediated signaling pathway is crucial for bile acid synthesis and metabolism. Primary bile acids can increase insulin sensitivity in mice, inhibit lipogenesis, and further promote fatty acid oxidation by activating FXR signaling, while secondary bile acids inhibit FXR signaling and thus affect energy metabolism (Kakiyama et al., 2013). Among them, TGR5 is widely expressed throughout the body, especially in brown adipose tissue. BA can promote lipolysis through TGR5 and play an important role in regulating energy metabolism. The occurrence of obesity is related to the browning of fat. A study found (Perino et al., 2014) that macrophage-specific TGR5 deficiency exacerbated insulin resistance in obese mice, while drug activation of TGR5 significantly reduced LPS induced macrophage chemokine expression. BA activates TGR5 to promote intracellular mitochondrial respiration to increase thermogenesis in mouse and human brown adipocytes (Broeders et al., 2015). Furthermore, TGR5 activation in white adipocytes participates in the browning process by increasing fatty acid β-oxidation and improving mitochondrial function (Velazquez-Villegas et al., 2018). In addition to acting on G protein-coupled receptors in the intestine, bile acid receptors are also present in adipose tissue. Studies have shown that bile acids can promote beigeization of adipose tissue, increase heat production, and improve energy metabolism (Broeders et al., 2015). Bile acids alter the composition of the intestinal microbiota and affect thermogenesis in white adipose tissue to improve glucose homeostasis and increase energy expenditure (Voreades et al., 2014).
In addition, bile acids can regulate appetite through the brain-gut axis. Studies have shown that bile acids bind to FXR receptors in the intestine to produce fibroblast growth factor 19/15 (FGF19/15), which reaches the hypothalamus through the brain-gut axis to regulate glucose metabolism, FGF19 in the hypothalamus has the effect of suppressing appetite and regulating energy metabolism (Kübeck et al., 2016; Zhi et al., 2019). Bile acids can also pass through the blood–brain barrier and bind to TGR5 and FXR to alleviate high-fat diet-induced obesity and improve energy metabolism (Santos-Marcos et al., 2019).
2.3.4 Peptidoglycan (PGN)
PGN is a unique component of the cell wall in Gram-positive bacteria. Like TLR4, TLR2 can also induce innate immune responses by recognizing pathogen associated molecular patterns (PAMPs) and some endogenous toxic substances. The occurrence of obesity is often accompanied by inflammatory response, which is closely related to the activation of innate immunity. PGNs of different bacteria activate multiple signals through TLR2, such as NF-κB and JNK. When PGN is recognized by the innate immune system as PAPM, it activates a series of intracellular signaling pathways such as NF-κB and induces the synthesis of antimicrobial peptides (Pazos and Peters, 2019). Activation of the NF-κB signaling pathway leads to chronic inflammation and fat accumulation, thus promoting obesity.
Nucleotide-binding Oligomerization Domain containing 2 (NOD2) is a product of peptidoglycan that is located in epithelial and immune cells. This cytosol is critical for inflammatory immune responses and can affect mucosal bacterial colonization. NOD2 is a pattern recognition receptor that regulates the host innate immune response and reduces inflammation, inhibiting steatosis and obesity (Gurses et al., 2020). Increased expression of NOD2 has been reported in a range of human metabolic diseases, including obesity, diabetes, nonalcoholic fatty liver disease, and metabolic syndrome (Cavallari et al., 2020). Studies have shown that mice lacking NOD2 appeared hyperglycemia, adipose tissue and liver inflammation under high-fat diet conditions, demonstrating that the NOD2-regulated microbiota can alleviate diet-induced obesity and metabolic dysfunction in mice (Rodriguez-Nunez et al., 2017). PGN also plays an important role in insulin resistance and metabolic inflammation. Jin et al. (2020) found that PGN significantly increased the triglyceride content and the expression of lipogenesis-related genes in primary hepatocytes. In addition, peptidoglycan can inhibit the browning of adipose tissue (Chen H. et al., 2021). Studies have shown that PGN can inhibit white fat browning by activating TLR2-NF-κB signaling through adipocytes. At the same time, intestinal flora can produce peptidoglycan, which can stimulate inflammatory cells to trigger an immune response. In particular, when the permeability of the intestinal barrier changes, peptidoglycan is easily bound to the vascular endothelium and promotes the progression of atherosclerosis (Chistiakov et al., 2015). Therefore, intestinal microbiota regulates the occurrence of obesity by secreting peptidoglycan.
2.3.5 Trimethylamine (TMA)
Choline is an important component of cell membranes. Choline is metabolized by microbial enzymes to produce TMA, which is further metabolized in the liver by flavin containing monooxygenase 3 (FMO3) to produce trimethylamine N-oxide (TMAO). Some scholars have demonstrated through experiments that there is a significant correlation between plasma TMAO levels and body mass index, which may be the result of affecting the intestinal microbes (Chen et al., 2024). Study had found that TMAO was involved in the energy metabolism of obese mice, meanwhile FMO3 was found in white adipose tissue, which was positively correlated with body mass index (Schugar et al., 2017). Targeted inhibition of the TMAO pathway by the gut microbiota protects mice from diet-induced obesity or leptin deficiency-related metabolic disorders (Schugar et al., 2022). Obesity is an important risk factor for atherosclerosis and coronary heart disease. Atherosclerosis, as the main pathophysiological basis of coronary heart disease, involves lipid metabolism and inflammatory response. Metabolomics technology has revealed that TMAO has pro-atherosclerotic properties and can be used as a potential biomarker for predicting the occurrence of cardiovascular diseases (Kong et al., 2024). Wang et al. (2011) first discovered in 2011 that TMAO plays a key role in the development of atherosclerosis; Ma and Li (2018) confirmed that TMAO can cause endothelial dysfunction. TMAO produced by intestinal flora enters the blood after being absorbed by intestinal epithelial cells, affecting the host’s metabolic capacity. These metabolites have been shown to affect obesity, insulin resistance, atherosclerosis and coronary heart disease (Schiattarella et al., 2019; Tang et al., 2019; Xu et al., 2020). Koeth et al. (2013) found that feeding mice with TMAO could lead to down-regulation of bile acid synthase expression, thereby inhibiting bile acid synthesis and causing fat deposition. At the same time, trimethylamine can also affect the expression of cholesterol 7α-hydroxylase and bile acid transporter. When the intestinal flora is unbalanced, resulting in excessive accumulation of TMA, a large amount of TMAO may be produced under the action of FMO3 in the liver, which can further cause metabolic disorders and obesity (Lin et al., 2012).
However, some studies have suggested that TMAO has no significant promoting effect on the occurrence of obesity. When studying the relationship between TMAO and aortic sclerosis, Collins et al. (2016) found that TMAO levels were inversely proportional to the degree of lesions in the aortic root and thoracic aorta. High levels of TMAO were significantly correlated with a reduction in the area of aortic lesions. At the same time, plasma lipid and lipoprotein levels were not correlated with TMAO. Due to the mouse model used in this experiment was ApoE (−/−) transgenic mice, which may be the reason affecting the experimental results. Gao et al. (2020) found that serum TMAO levels were positively correlated with age in the general population, while serum TMAO levels were not significantly correlated with obesity. In human studies, the association between serum TMAO and obesity has been inconsistent, which may be due to the different dietary patterns and genetic factors of the participants. Therefore, factors that may affect the experimental results need to be excluded in the study to ensure the reliability of the experimental results and to confirm the effect of TMAO on obesity. The relationships between gut microbial metabolites and obesity-related phenotypes summarized in this article are shown in Table 2.
3 Conclusion and perspectives
Currently, the interaction between intestinal flora and body metabolism has become a hot topic. Intestinal microbiota is closely related to various metabolic diseases such as obesity. It fully participates in the digestion and absorption of nutrients and the metabolism of energy in the body. Changes in microbial diversity and metabolites affect the occurrence and development of obesity. As shown in Figure 1, beneficial bacteria such as Bifidobacterium and Lactobacillus can prevent the occurrence of obesity by strengthening the intestinal barrier, improving insulin sensitivity and metabolic disorders; Pathogenic bacteria such as Escherichia coli, Ruminococcaceae, and Desulfovibrionaceae are closely related to the formation of obesity; Metabolites produced by intestinal microbes, such as short-chain fatty acids, lipopolysaccharides and bile acids, play an important role in the prevention and treatment of obesity. With the further development of research, the association mechanism among intestinal flora, its metabolites and metabolic diseases such as obesity has been revealed, however, the intestinal microorganisms are a large and complex system. The interaction between the intestinal flora and the host, as well as the connection between the intestinal flora and various tissues and organs, still need to be explored, for example: studies in recent years have shown that adipose tissue is involved in immune responses. From an immunological perspective, what kind of dialogue takes place between intestinal flora and adipose tissue? On the other hand, with the continuous development of technology in recent years, in addition to metagenomics and 16S rDNA sequencing, the combined application of multi-omics such as single-cell transcriptomics, spatial transcriptomics and quantitative metabolomics will also help to explore the relationship between intestinal flora and obesity. This review highlights the pivotal role of gut microbiota and its metabolites in obesity pathophysiology. Future research should focus on translating microbiome-based findings into targeted clinical interventions, such as personalized probiotic therapies and dietary modifications, to combat obesity more effectively. In conclusion, intestinal microbes and its metabolites can be used as probiotics in clinical intervention in order to provide more solutions for the prevention and treatment of obesity in the future.
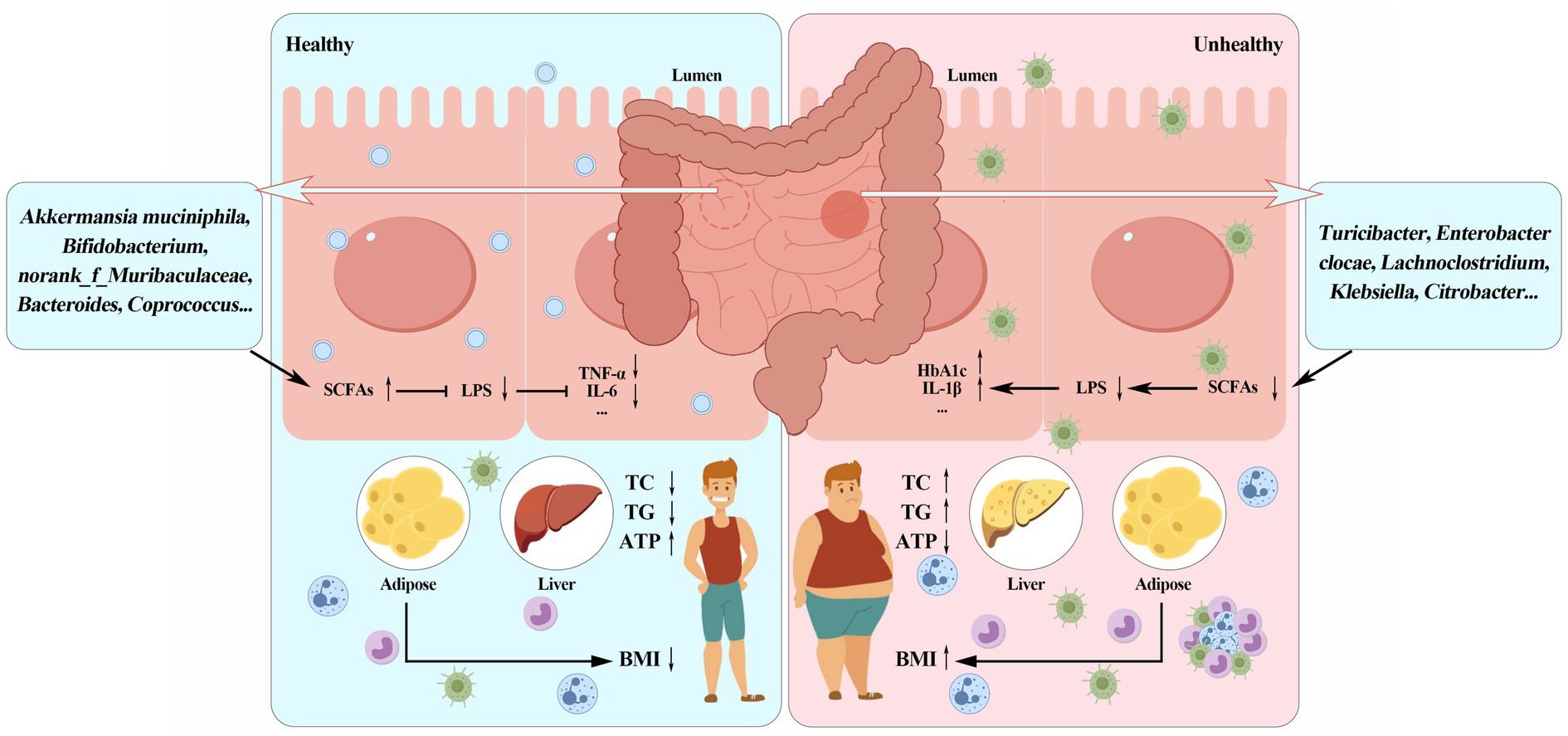
Figure 1. Schematic diagram of the relationship among gut microbiota, microbial metabolites and obesity.
Author contributions
YG: Funding acquisition, Writing – original draft. WL: Data curation, Software, Writing – review & editing. XM: Writing – original draft. MX: Software, Supervision, Writing – original draft. YL: Software, Supervision, Writing – original draft. SB: Software, Supervision, Writing – original draft. HL: Funding acquisition, Project administration, Writing – review & editing.
Funding
The author(s) declare that financial support was received for the research and/or publication of this article. The research review was supported by the funding of Baicheng Normal University Doctoral Research Initiation Fund Project (90024169041) and Natural Science Foundation of Inner Mongolia (2022LHMS03005).
Conflict of interest
The authors declare that the research was conducted in the absence of any commercial or financial relationships that could be construed as a potential conflict of interest.
Generative AI statement
The authors declare that no Gen AI was used in the creation of this manuscript.
Publisher’s note
All claims expressed in this article are solely those of the authors and do not necessarily represent those of their affiliated organizations, or those of the publisher, the editors and the reviewers. Any product that may be evaluated in this article, or claim that may be made by its manufacturer, is not guaranteed or endorsed by the publisher.
References
Adeghate, E. (2004). An update on the biology and physiology of resistin. Cell. Mol. Life Sci. 61, 2485–2496. doi: 10.1007/s00018-004-4083-2
Aktar, R., Parkar, N., Stentz, R., Baumard, L., Parker, A., Goldson, A., et al. (2020). Human resident gut microbe Bacteroides thetaiotaomicron regulates colonic neuronal innervation and neurogenic function. Gut Microbes 11, 1745–1757. doi: 10.1080/19490976.2020.1766936
Arab, J. P., Karpen, S. J., Dawson, P. A., Arrese, M., and Trauner, M. (2017). Bile acids and nonalcoholic fatty liver disease: molecular insights and therapeutic perspectives. Hepatology 65, 350–362. doi: 10.1002/hep.28709
Becattini, S., Taur, Y., and Pamer, E. G. (2016). Antibiotic-induced changes in the intestinal microbiota and disease. Trends Mol. Med. 22, 458–478. doi: 10.1016/j.molmed.2016.04.003
Biedermann, L., and Rogler, G. (2015). The intestinal microbiota: its role in health and disease. Eur. J. Pediatr. 174, 151–167. doi: 10.1007/s00431-014-2476-2
Blaak, E., Canfora, E., Theis, S., Frost, G., Groen, A., Mithieux, G., et al. (2020). Short chain fatty acids in human gut and metabolic health. Benefic. Microbes 11, 411–455. doi: 10.3920/BM2020.0057
Bouter, K. E., Bakker, G., Levin, E., Hartstra, A., Kootte, R., Udayappan, S., et al. (2018). Differential metabolic effects of oral butyrate treatment in lean versus metabolic syndrome subjects. Clin. Transl. Gastroenterol. 9:e155. doi: 10.1038/s41424-018-0025-4
Broeders, E. P., Nascimento, E. B., Havekes, B., Brans, B., Roumans, K. H., Tailleux, A., et al. (2015). The bile acid chenodeoxycholic acid increases human brown adipose tissue activity. Cell Metab. 22, 418–426. doi: 10.1016/j.cmet.2015.07.002
Canfora, E. E., van der Beek, C. M., Jocken, J. W., Goossens, G. H., Holst, J. J., Olde Damink, S. W., et al. (2017). Colonic infusions of short-chain fatty acid mixtures promote energy metabolism in overweight/obese men: a randomized crossover trial. Sci. Rep. 7:2360. doi: 10.1038/s41598-017-02546-x
Cani, P. D., Amar, J., Iglesias, M. A., Poggi, M., Knauf, C., Bastelica, D., et al. (2007). Metabolic endotoxemia initiates obesity and insulin resistance. Diabetes 56, 1761–1772. doi: 10.2337/db06-1491
Cavallari, J. F., Pokrajac, N. T., Zlitni, S., Foley, K. P., Henriksbo, B. D., and Schertzer, J. D. (2020). NOD2 in hepatocytes engages a liver-gut axis to protect against steatosis, fibrosis, and gut dysbiosis during fatty liver disease in mice. Am. J. Physiol. Endocrinol. Metab. 319, E305–E314. doi: 10.1152/ajpendo.00181.2020
Chen, S., Chen, X. Y., Huang, Z. H., Fang, A. P., Li, S. Y., Huang, R. Z., et al. (2024). Correlation between serum trimethylamine-N-oxide and body fat distribution in middle-aged and older adults: a prospective cohort study. Nutr. J. 23:70. doi: 10.1186/s12937-024-00974-w
Chen, H., Sun, L., Feng, L., Mulholland, M., Zhang, W., and Yin, Y. (2021). Peptidoglycan inhibits beigeing of adipose tissue. Acta Pharm. Sin. B 12, 990–993. doi: 10.1016/j.apsb.2021.11.015
Chen, M., Xing, J., Pan, D., and Gao, P. (2021). Effect of Chinese herbal medicine mixture 919 syrup on regulation of the ghrelin pathway and intestinal microbiota in rats with non-alcoholic fatty liver disease. Front. Microbiol. 12:793854. doi: 10.3389/fmicb.2021.793854
Chiang, J. Y. (2002). Bile acid regulation of gene expression: roles of nuclear hormone receptors. Endocr. Rev. 23, 443–463. doi: 10.1210/er.2000-0035
Chistiakov, D. A., Bobryshev, Y. V., Kozarov, E., Sobenin, I. A., and Orekhov, A. N. (2015). Role of gut microbiota in the modulation of atherosclerosis-associated immune response. Front. Microbiol. 6:671. doi: 10.3389/fmicb.2015.00671
Collins, H. L., Drazul-Schrader, D., Sulpizio, A. C., Koster, P. D., Williamson, Y., Adelman, S. J., et al. (2016). L-carnitine intake and high trimethylamine N-oxide plasma levels correlate with low aortic lesions in ApoE−/− transgenic mice expressing CETP. Atherosclerosis 244, 29–37. doi: 10.1016/j.atherosclerosis.2015.10.108
Cui, Y., Wang, Q., Chang, R., Zhou, X., and Xu, C. (2019). Intestinal barrier function–non-alcoholic fatty liver disease interactions and possible role of gut microbiota. J. Agric. Food Chem. 67, 2754–2762. doi: 10.1021/acs.jafc.9b00080
Cummings, J. H., Pomare, E., Branch, W., Naylor, C., and MacFarlane, G. (1987). Short chain fatty acids in human large intestine, portal, hepatic and venous blood. Gut 28, 1221–1227. doi: 10.1136/gut.28.10.1221
Cuthbertson, D. J., Koskinen, J., Brown, E., Magnussen, C. G., Hutri-Kähönen, N., Sabin, M., et al. (2021). Fatty liver index predicts incident risk of prediabetes, type 2 diabetes and non-alcoholic fatty liver disease (NAFLD). Ann. Med. 53, 1257–1265. doi: 10.1080/07853890.2021.1956685
Dao, M. C., Everard, A., Aron-Wisnewsky, J., Sokolovska, N., Prifti, E., Verger, E. O., et al. (2016). Akkermansia muciniphila and improved metabolic health during a dietary intervention in obesity: relationship with gut microbiome richness and ecology. Gut 65, 426–436. doi: 10.1136/gutjnl-2014-308778
De La Cuesta-Zuluaga, J., Mueller, N. T., Corrales-Agudelo, V., Velásquez-Mejía, E. P., Carmona, J. A., Abad, J. M., et al. (2017). Metformin is associated with higher relative abundance of mucin-degrading Akkermansia muciniphila and several short-chain fatty acid–producing microbiota in the gut. Diabetes Care 40, 54–62. doi: 10.2337/dc16-1324
De Vadder, F., Kovatcheva-Datchary, P., Goncalves, D., Vinera, J., Zitoun, C., Duchampt, A., et al. (2014). Microbiota-generated metabolites promote metabolic benefits via gut-brain neural circuits. Cell 156, 84–96. doi: 10.1016/j.cell.2013.12.016
Den Besten, G., Bleeker, A., Gerding, A., van Eunen, K., Havinga, R., van Dijk, T. H., et al. (2015). Short-chain fatty acids protect against high-fat diet–induced obesity via a PPARγ-dependent switch from lipogenesis to fat oxidation. Diabetes 64, 2398–2408. doi: 10.2337/db14-1213
Erridge, C., Attina, T., Spickett, C. M., and Webb, D. J. (2007). A high-fat meal induces low-grade endotoxemia: evidence of a novel mechanism of postprandial inflammation. Am. J. Clin. Nutr. 86, 1286–1292. doi: 10.1093/ajcn/86.5.1286
Faits, T., Walker, M. E., Rodriguez-Morato, J., Meng, H., Gervis, J. E., Galluccio, J. M., et al. (2020). Exploring changes in the human gut microbiota and microbial-derived metabolites in response to diets enriched in simple, refined, or unrefined carbohydrate-containing foods: a post hoc analysis of a randomized clinical trial. Am. J. Clin. Nutr. 112, 1631–1641. doi: 10.1093/ajcn/nqaa254
Fåk, F., and Bäckhed, F. (2012). Lactobacillus reuteri prevents diet-induced obesity, but not atherosclerosis, in a strain dependent fashion in Apoe−/− mice. PLoS One 7:e46837. doi: 10.1371/journal.pone.0046837
Fei, N., and Zhao, L. (2013). An opportunistic pathogen isolated from the gut of an obese human causes obesity in germfree mice. ISME J. 7, 880–884. doi: 10.1038/ismej.2012.153
Feng, W., Ao, H., and Peng, C. (2018). Gut microbiota, short-chain fatty acids, and herbal medicines. Front. Pharmacol. 9:1354. doi: 10.3389/fphar.2018.01354
Gao, X., Sun, G., Randell, E., Tian, Y., and Zhou, H. (2020). Systematic investigation of the relationships of trimethylamine N-oxide and L-carnitine with obesity in both humans and rodents. Food Funct. 11, 7707–7716. doi: 10.1039/D0FO01743D
Gibson, G. R., Hutkins, R., Sanders, M. E., Prescott, S. L., Reimer, R. A., Salminen, S. J., et al. (2017). Expert consensus document: the International Scientific Association for Probiotics and Prebiotics (ISAPP) consensus statement on the definition and scope of prebiotics. Nat. Rev. Gastroenterol. Hepatol. 14, 491–502. doi: 10.1038/nrgastro.2017.75
Gibson, G. R., and Roberfroid, M. B. (1995). Dietary modulation of the human colonic microbiota: introducing the concept of prebiotics. J. Nutr. 125, 1401–1412. doi: 10.1093/jn/125.6.1401
Gomes, A. C., de Sousa, R. G. M., Botelho, P. B., Gomes, T. L. N., Prada, P. O., and Mota, J. F. (2017). The additional effects of a probiotic mix on abdominal adiposity and antioxidant status: a double-blind, randomized trial. Obesity 25, 30–38. doi: 10.1002/oby.21671
Gurses, S. A., Banskar, S., Stewart, C., Trimoski, B., Dziarski, R., and Gupta, D. (2020). Nod2 protects mice from inflammation and obesity-dependent liver cancer. Sci. Rep. 10:20519. doi: 10.1038/s41598-020-77463-7
Han, M., Zhang, M., Wang, X., Bai, X., Yue, T., and Gao, Z. (2021). Cloudy apple juice fermented by Lactobacillus prevents obesity via modulating gut microbiota and protecting intestinal tract health. Nutrients 13:971. doi: 10.3390/nu13030971
Hoozemans, J., de Brauw, M., Nieuwdorp, M., and Gerdes, V. (2021). Gut microbiome and metabolites in patients with NAFLD and after bariatric surgery: a comprehensive review. Meta 11:353. doi: 10.3390/metabo11060353
Houten, S. M., Watanabe, M., and Auwerx, J. (2006). Endocrine functions of bile acids. EMBO J. 25, 1419–1425. doi: 10.1038/sj.emboj.7601049
Jeung, W. H., Shim, J.-J., Woo, S.-W., Sim, J.-H., and Lee, J.-L. (2018). Lactobacillus curvatus HY7601 and Lactobacillus plantarum KY1032 cell extracts inhibit adipogenesis in 3T3-L1 and HepG2 cells. J. Med. Food 21, 876–886. doi: 10.1089/jmf.2017.4157
Jiao, N., Baker, S. S., Chapa-Rodriguez, A., Liu, W., Nugent, C. A., Tsompana, M., et al. (2018). Suppressed hepatic bile acid signalling despite elevated production of primary and secondary bile acids in NAFLD. Gut 67, 1881–1891. doi: 10.1136/gutjnl-2017-314307
Jin, M., Lai, Y., Zhao, P., Shen, Q., Su, W., Yin, Y., et al. (2020). Effects of peptidoglycan on the development of steatohepatitis. Biochimica et Biophysica Acta (BBA)-molecular and cell biology of. Lipids 1865:158595. doi: 10.1016/j.bbalip.2019.158595
Journey, E. K., Ortega-Santos, C. P., Bruening, M., and Whisner, C. M. (2020). Changes in weight status and the intestinal microbiota among college freshman, aged 18 years. J. Adolesc. Health 66, 166–171. doi: 10.1016/j.jadohealth.2019.06.005
Kakiyama, G., Pandak, W. M., Gillevet, P. M., Hylemon, P. B., Heuman, D. M., Daita, K., et al. (2013). Modulation of the fecal bile acid profile by gut microbiota in cirrhosis. J. Hepatol. 58, 949–955. doi: 10.1016/j.jhep.2013.01.003
Kalliomäki, M., Collado, M. C., Salminen, S., and Isolauri, E. (2008). Early differences in fecal microbiota composition in children may predict overweight. Am. J. Clin. Nutr. 87, 534–538. doi: 10.1093/ajcn/87.3.534
Kang, Y., Kang, X., Yang, H., Liu, H., Yang, X., Liu, Q., et al. (2022). Lactobacillus acidophilus ameliorates obesity in mice through modulation of gut microbiota dysbiosis and intestinal permeability. Pharmacol. Res. 175:106020. doi: 10.1016/j.phrs.2021.106020
Kimura, I., Ichimura, A., Ohue-Kitano, R., and Igarashi, M. (2019). Free fatty acid receptors in health and disease. Physiol. Rev. 100, 171–210. doi: 10.1152/physrev.00041.2018
Koeth, R. A., Wang, Z., Levison, B. S., Buffa, J. A., Org, E., Sheehy, B. T., et al. (2013). Intestinal microbiota metabolism of L-carnitine, a nutrient in red meat, promotes atherosclerosis. Nat. Med. 19, 576–585. doi: 10.1038/nm.3145
Kong, H., Cen, J., Yang, X., Xu, Z., Liang, J., Xiong, Q., et al. (2024). Research progress in the relationship between trimethylamine oxide and coronary heart disease. Altern. Ther. Health Med. 30, 220–227.
Kübeck, R., Bonet-Ripoll, C., Hoffmann, C., Walker, A., Müller, V. M., Schüppel, V. L., et al. (2016). Dietary fat and gut microbiota interactions determine diet-induced obesity in mice. Mol. Metab. 5, 1162–1174. doi: 10.1016/j.molmet.2016.10.001
Łagowska, K., Malinowska, A. M., Kapczuk, K., Mikołajczyk-Stecyna, J., Chmurzyńska, A., and Schmidt, M. (2022). β-Glucuronidase activity is associated with carbohydrate metabolism but not with androgen status in overweight and obese women with polycystic ovary syndrome. Nutrition 97:111606. doi: 10.1016/j.nut.2022.111606
Lancaster, G. I., Langley, K. G., Berglund, N. A., Kammoun, H. L., Reibe, S., Estevez, E., et al. (2018). Evidence that TLR4 is not a receptor for saturated fatty acids but mediates lipid-induced inflammation by reprogramming macrophage metabolism. Cell Metab. 27, 1096–1110-e5. doi: 10.1016/j.cmet.2018.03.014
Larraufie, P., Martin-Gallausiaux, C., Lapaque, N., Dore, J., Gribble, F., Reimann, F., et al. (2018). SCFAs strongly stimulate PYY production in human enteroendocrine cells. Sci. Rep. 8:74. doi: 10.1038/s41598-017-18259-0
Lau, H. C., Zhang, X., Ji, F., Lin, Y., Liang, W., Li, Q., et al. (2024). Lactobacillus acidophilus suppresses non-alcoholic fatty liver disease-associated hepatocellular carcinoma through producing valeric acid. EBioMedicine 100:104952. doi: 10.1016/j.ebiom.2023.104952
Laugerette, F., Vors, C., Géloën, A., Chauvin, M.-A., Soulage, C., Lambert-Porcheron, S., et al. (2011). Emulsified lipids increase endotoxemia: possible role in early postprandial low-grade inflammation. J. Nutr. Biochem. 22, 53–59. doi: 10.1016/j.jnutbio.2009.11.011
Layden, B. T., Yalamanchi, S. K., Wolever, T. M., Dunaif, A., and Lowe, W. L. Jr. (2012). Negative association of acetate with visceral adipose tissue and insulin levels. Diabetes Metab. Syndr Obesity 5, 49–55. doi: 10.2147/DMSO.S29244
Lee, P., Yacyshyn, B. R., and Yacyshyn, M. B. (2019). Gut microbiota and obesity: an opportunity to alter obesity through faecal microbiota transplant (FMT). Diabetes. Obes. Metab. 21, 479–490. doi: 10.1111/dom.13561
Ley, R. E., Turnbaugh, P. J., Klein, S., and Gordon, J. I. (2006). Human gut microbes associated with obesity. Nature 444, 1022–1023. doi: 10.1038/4441022a
Li, Q., Zhou, H., Ouyang, J., Guo, S., Zheng, J., and Li, G. (2022). Effects of dietary tryptophan supplementation on body temperature, hormone, and cytokine levels in broilers exposed to acute heat stress. Trop. Anim. Health Prod. 54:164. doi: 10.1007/s11250-022-03161-3
Liang, D., Zhang, L., Chen, H., Zhang, H., Hu, H., and Dai, X. (2021). Potato resistant starch inhibits diet-induced obesity by modifying the composition of intestinal microbiota and their metabolites in obese mice. Int. J. Biol. Macromol. 180, 458–469. doi: 10.1016/j.ijbiomac.2021.02.209
Lin, C.-S., Lin, F.-Y., Ho, L.-J., Tsai, C.-S., Cheng, S.-M., Wu, W.-L., et al. (2012). PKCδ signalling regulates SR-A and CD36 expression and foam cell formation. Cardiovasc. Res. 95, 346–355. doi: 10.1093/cvr/cvs189
Lindholm, C. R., Ertel, R. L., Bauwens, J. D., Schmuck, E. G., Mulligan, J. D., and Saupe, K. W. (2013). A high-fat diet decreases AMPK activity in multiple tissues in the absence of hyperglycemia or systemic inflammation in rats. J. Physiol. Biochem. 69, 165–175. doi: 10.1007/s13105-012-0199-2
Lu, Y., Fan, C., Li, P., Lu, Y., Chang, X., and Qi, K. (2016). Short chain fatty acids prevent high-fat-diet-induced obesity in mice by regulating G protein-coupled receptors and gut microbiota. Sci. Rep. 6:37589. doi: 10.1038/srep37589
Ma, J., and Li, H. (2018). The role of gut microbiota in atherosclerosis and hypertension. Front. Pharmacol. 9:1082. doi: 10.3389/fphar.2018.01082
Maruta, H., Yoshimura, Y., Araki, A., Kimoto, M., Takahashi, Y., and Yamashita, H. (2016). Activation of AMP-activated protein kinase and stimulation of energy metabolism by acetic acid in L6 myotube cells. PLoS One 11:e0158055. doi: 10.1371/journal.pone.0158055
Marvasti, F. E., Moshiri, A., Taghavi, M. S., Riazi, S., Taati, M., Sadati, S. F., et al. (2020). The first report of differences in gut microbiota composition between obese and normal weight Iranian subjects. Iran. Biomed. J. 24, 148–154. doi: 10.29252/ibj.24.3.148
Matheus, V., Monteiro, L., Oliveira, R., Maschio, D., and Collares-Buzato, C. (2017). Butyrate reduces high-fat diet-induced metabolic alterations, hepatic steatosis and pancreatic beta cell and intestinal barrier dysfunctions in prediabetic mice. Exp. Biol. Med. 242, 1214–1226. doi: 10.1177/1535370217708188
Mischke, M., Arora, T., Tims, S., Engels, E., Sommer, N., van Limpt, K., et al. (2018). Specific synbiotics in early life protect against diet-induced obesity in adult mice. Diabetes. Obes. Metab. 20, 1408–1418. doi: 10.1111/dom.13240
Mollica, M. P., Mattace Raso, G., Cavaliere, G., Trinchese, G., De Filippo, C., Aceto, S., et al. (2017). Butyrate regulates liver mitochondrial function, efficiency, and dynamics in insulin-resistant obese mice. Diabetes 66, 1405–1418. doi: 10.2337/db16-0924
Morrison, D. J., and Preston, T. (2016). Formation of short chain fatty acids by the gut microbiota and their impact on human metabolism. Gut Microbes 7, 189–200. doi: 10.1080/19490976.2015.1134082
Pazos, M., and Peters, K. (2019). Peptidoglycan. Subcell Biochem 92, 127–168. doi: 10.1007/978-3-030-18768-2_5
Perino, A., Pols, T. W. H., Nomura, M., Stein, S., Pellicciari, R., and Schoonjans, K. (2014). TGR5 reduces macrophage migration through mTOR-induced C/EBPβ differential translation. J. Clin. Invest. 124, 5424–5436. doi: 10.1172/JCI76289
Plovier, H., Everard, A., Druart, C., Depommier, C., Van Hul, M., Geurts, L., et al. (2017). A purified membrane protein from Akkermansia muciniphila or the pasteurized bacterium improves metabolism in obese and diabetic mice. Nat. Med. 23, 107–113. doi: 10.1038/nm.4236
Rahman, M. S., Kang, I., Lee, Y., Habib, M. A., Choi, B. J., Kang, J. S., et al. (2021). Bifidobacterium longum subsp. infantis YB0411 inhibits adipogenesis in 3T3-L1 pre-adipocytes and reduces high-fat-diet-induced obesity in mice. J. Agric. Food Chem. 69, 6032–6042. doi: 10.1021/acs.jafc.1c01440
Ray, M., Hor, P., Ojha, D., Soren, J., Singh, S., and Mondal, K. (2018). Bifidobacteria and its rice fermented products on diet induced obese mice: analysis of physical status, serum profile and gene expressions. Benefic. Microbes 9, 441–452. doi: 10.3920/BM2017.0056
Rodriguez-Nunez, I., Caluag, T., Kirby, K., Rudick, C. N., Dziarski, R., and Gupta, D. (2017). Nod2 and Nod2-regulated microbiota protect BALB/c mice from diet-induced obesity and metabolic dysfunction. Sci. Rep. 7:548. doi: 10.1038/s41598-017-00484-2
Rothschild, D., Weissbrod, O., Barkan, E., Kurilshikov, A., Korem, T., Zeevi, D., et al. (2018). Environment dominates over host genetics in shaping human gut microbiota. Nature 555, 210–215. doi: 10.1038/nature25973
Saad, M., Santos, A., and Prada, P. (2016). Linking gut microbiota and inflammation to obesity and insulin resistance. Physiology 31, 283–293. doi: 10.1152/physiol.00041.2015
Sanchez, M., Darimont, C., Panahi, S., Drapeau, V., Marette, A., Taylor, V. H., et al. (2017). Effects of a diet-based weight-reducing program with probiotic supplementation on satiety efficiency, eating behaviour traits, and psychosocial behaviours in obese individuals. Nutrients 9:284. doi: 10.3390/nu9030284
Santos-Marcos, J. A., Perez-Jimenez, F., and Camargo, A. (2019). The role of diet and intestinal microbiota in the development of metabolic syndrome. J. Nutr. Biochem. 70, 1–27. doi: 10.1016/j.jnutbio.2019.03.017
Sayin, S. I., Wahlström, A., Felin, J., Jäntti, S., Marschall, H.-U., Bamberg, K., et al. (2013). Gut microbiota regulates bile acid metabolism by reducing the levels of tauro-beta-muricholic acid, a naturally occurring FXR antagonist. Cell Metab. 17, 225–235. doi: 10.1016/j.cmet.2013.01.003
Scherrer, J. F., Salas, J., Lustman, P. J., Van Den Berk-Clark, C., Schnurr, P. P., Tuerk, P., et al. (2018). The role of obesity in the association between posttraumatic stress disorder and incident diabetes. JAMA Psychiatry 75, 1189–1198. doi: 10.1001/jamapsychiatry.2018.2028
Schiattarella, G. G., Sannino, A., Esposito, G., and Perrino, C. (2019). Diagnostics and therapeutic implications of gut microbiota alterations in cardiometabolic diseases. Trends Cardiovasc. Med. 29, 141–147. doi: 10.1016/j.tcm.2018.08.003
Schroeder, B. O., Birchenough, G. M., Ståhlman, M., Arike, L., Johansson, M. E., Hansson, G. C., et al. (2018). Bifidobacteria or fiber protects against diet-induced microbiota-mediated colonic mucus deterioration. Cell Host Microbe 23, 27–40.e7. doi: 10.1016/j.chom.2017.11.004
Schugar, R. C., Gliniak, C. M., Osborn, L. J., Massey, W., Sangwan, N., Horak, A., et al. (2022). Gut microbe-targeted choline trimethylamine lyase inhibition improves obesity via rewiring of host circadian rhythms. eLife 11:e63998. doi: 10.7554/eLife.63998
Schugar, R. C., Shih, D. M., Warrier, M., Helsley, R. N., Burrows, A., Ferguson, D., et al. (2017). The TMAO-producing enzyme flavin-containing monooxygenase 3 regulates obesity and the beiging of white adipose tissue. Cell Rep. 19, 2451–2461. doi: 10.1016/j.celrep.2017.05.077
Simon, M.-C., Strassburger, K., Nowotny, B., Kolb, H., Nowotny, P., Burkart, V., et al. (2015). Intake of Lactobacillus reuteri improves incretin and insulin secretion in glucose-tolerant humans: a proof of concept. Diabetes Care 38, 1827–1834. doi: 10.2337/dc14-2690
Singh, R. K., Chang, H.-W., Yan, D., Lee, K. M., Ucmak, D., Wong, K., et al. (2017). Influence of diet on the gut microbiome and implications for human health. J. Transl. Med. 15, 1–17. doi: 10.1186/s12967-017-1175-y
Sittipo, P., Lobionda, S., Lee, Y. K., and Maynard, C. L. (2018). Intestinal microbiota and the immune system in metabolic diseases. J. Microbiol. 56, 154–162. doi: 10.1007/s12275-018-7548-y
Song, W., Song, C., Li, L., Wang, T., Hu, J., Zhu, L., et al. (2021). Lactobacillus alleviated obesity induced by high-fat diet in mice. J. Food Sci. 86, 5439–5451. doi: 10.1111/1750-3841.15971
Suau, A., Bonnet, R., Sutren, M., Godon, J.-J., Gibson, G. R., Collins, M. D., et al. (1999). Direct analysis of genes encoding 16S rRNA from complex communities reveals many novel molecular species within the human gut. Appl. Environ. Microbiol. 65, 4799–4807. doi: 10.1128/AEM.65.11.4799-4807.1999
Sun, M., Wu, W., Liu, Z., and Cong, Y. (2017). Microbiota metabolite short chain fatty acids, GPCR, and inflammatory bowel diseases. J. Gastroenterol. 52, 1–8. doi: 10.1007/s00535-016-1242-9
Tang, W. W., Bäckhed, F., Landmesser, U., and Hazen, S. L. (2019). Intestinal microbiota in cardiovascular health and disease: JACC state-of-the-art review. J. Am. Coll. Cardiol. 73, 2089–2105. doi: 10.1016/j.jacc.2019.03.024
Tomas, J., Mulet, C., Saffarian, A., Cavin, J.-B., Ducroc, R., Regnault, B., et al. (2016). High-fat diet modifies the PPAR-γ pathway leading to disruption of microbial and physiological ecosystem in murine small intestine. Proc. Natl. Acad. Sci. 113, E5934–E5943. doi: 10.1073/pnas.1612559113
Tsukumo, D. M., Carvalho, B. M., Carvalho Filho, M. A., and Saad, M. J. (2015). Translational research into gut microbiota: new horizons on obesity treatment: updated 2014. Arch. Endocrinol. Metab. 59, 154–160. doi: 10.1590/2359-3997000000029
Turnbaugh, P. J., Ley, R. E., Mahowald, M. A., Magrini, V., Mardis, E. R., and Gordon, J. I. (2006). An obesity-associated gut microbiome with increased capacity for energy harvest. Nature 444, 1027–1031. doi: 10.1038/nature05414
Vallianou, N., Christodoulatos, G. S., Karampela, I., Tsilingiris, D., Magkos, F., Stratigou, T., et al. (2021). Understanding the role of the gut microbiome and microbial metabolites in non-alcoholic fatty liver disease: current evidence and perspectives. Biomol. Ther. 12:56. doi: 10.3390/biom12010056
Velazquez-Villegas, L. A., Perino, A., Lemos, V., Zietak, M., Nomura, M., Pols, T. W. H., et al. (2018). TGR5 signalling promotes mitochondrial fission and beige remodelling of white adipose tissue. Nat. Commun. 9:245. doi: 10.1038/s41467-017-02068-0
Voreades, N., Kozil, A., and Weir, T. L. (2014). Diet and the development of the human intestinal microbiome. Front. Microbiol. 5:494. doi: 10.3389/fmicb.2014.00494
Wang, Z., Klipfell, E., Bennett, B. J., Koeth, R., Levison, B. S., DuGar, B., et al. (2011). Gut flora metabolism of phosphatidylcholine promotes cardiovascular disease. Nature 472, 57–63. doi: 10.1038/nature09922
Wang, J., Liu, A., Li, A., Song, H., Luo, P., Zhan, M., et al. (2023). Lactobacillus fermentum CKCC1858 alleviates hyperlipidemia in golden hamsters on a high-fat diet via modulating gut microbiota. Food Funct. 14, 9580–9590. doi: 10.1039/d3fo02618c
Wang, Y., Tian, Y., Zhang, N., Li, X., Wang, X., Wang, W., et al. (2021). Pediococcus pentosaceus PP04 improves high-fat diet-induced liver injury by the modulation of gut inflammation and intestinal microbiota in C57BL/6N mice. Food Funct. 12, 6851–6862. doi: 10.1039/D1FO00857A
Xiao, S., Fei, N., Pang, X., Shen, J., Wang, L., Zhang, B., et al. (2014). A gut microbiota-targeted dietary intervention for amelioration of chronic inflammation underlying metabolic syndrome. FEMS Microbiol. Ecol. 87, 357–367. doi: 10.1111/1574-6941.12228
Xie, B., Yang, X., Yang, L., Wen, X., and Zhao, G. (2021). Adding polyethylene glycol to steer ration containing sorghum tannins increases crude protein digestibility and shifts nitrogen excretion from feces to urine. Anim. Nutr. 7, 779–786. doi: 10.1016/j.aninu.2021.03.002
Xu, H., Wang, X., Feng, W., Liu, Q., Zhou, S., Liu, Q., et al. (2020). The gut microbiota and its interactions with cardiovascular disease. Microb. Biotechnol. 13, 637–656. doi: 10.1111/1751-7915.13524
Yamashita, H., Maruta, H., Jozuka, M., Kimura, R., Iwabuchi, H., Yamato, M., et al. (2009). Effects of acetate on lipid metabolism in muscles and adipose tissues of type 2 diabetic Otsuka long-Evans Tokushima fatty (OLETF) rats. Biosci. Biotechnol. Biochem. 73, 570–576. doi: 10.1271/bbb.80634
Yaribeygi, H., Farrokhi, F. R., Butler, A. E., and Sahebkar, A. (2019). Insulin resistance: review of the underlying molecular mechanisms. J. Cell. Physiol. 234, 8152–8161. doi: 10.1002/jcp.27603
Yuan, K., Lin, N., and Chen, H. (2018). Relationship between intestinal flora and the pathogenesis of obesity. Med Recapitul. 24, 4166–4171.
Zhi, C., Huang, J., Wang, J., Cao, H., Bai, Y., Guo, J., et al. (2019). Connection between gut microbiome and the development of obesity. Eur. J. Clin. Microbiol. Infect. Dis. 38, 1987–1998. doi: 10.1007/s10096-019-03623-x
Zhu, X., Zhang, X., Gao, X., Yi, Y., Hou, Y., Meng, X., et al. (2020). Effects of inulin propionate ester on obesity-related metabolic syndrome and intestinal microbial homeostasis in diet-induced obese mice. ACS Omega 5, 12865–12876. doi: 10.1021/acsomega.0c00649
Keywords: obesity, metabolic diseases, gut microbiota, metabolic disorders, gut microbial metabolites
Citation: Gao Y, Liu W, Ma X, Xue M, Li Y, Bi S and Leng H (2025) The role of intestinal microbiota and its metabolites in the occurrence and intervention of obesity. Front. Microbiol. 16:1559178. doi: 10.3389/fmicb.2025.1559178
Edited by:
Jun Zhou, Shandong First Medical University, ChinaCopyright © 2025 Gao, Liu, Ma, Xue, Li, Bi and Leng. This is an open-access article distributed under the terms of the Creative Commons Attribution License (CC BY). The use, distribution or reproduction in other forums is permitted, provided the original author(s) and the copyright owner(s) are credited and that the original publication in this journal is cited, in accordance with accepted academic practice. No use, distribution or reproduction is permitted which does not comply with these terms.
*Correspondence: Wenhui Liu, MTA5NjU3NTM1QHFxLmNvbQ==; Huan Leng, bGVuZ2h1YW5AY2Fhcy5jbg==
†These authors have contributed equally to this work