- 1School of Geography and Environment, Liaocheng University, Liaocheng, China
- 2Department of Agricultural and Environmental Engineering, College of Technology, University of Bamenda, Bambili, Cameroon
- 3Shandong Academy of Innovation and Development, Jinan, China
Introduction: Biochar is recognized as a promising soil amendment for maintaining soil fertility and improving soil conditions. Alkaline farmland is widely distributed globally. Soil microbial taxa, including rare, intermediate, and abundant bacteria, fungi, protists, and phoD-harboring microbes, play essential roles in carbon, nitrogen, and phosphorus cycling. However, the impacts of biochar on the community composition of these taxa in alkaline farmland are not well understood. Gaining insights into how the soil microbiome responds to biochar application and its association with crop biomass is crucial for sustainable agriculture. In particular, the responses of rare microbial communities, such as rare protists and phoD-harboring microbial taxa, to biochar and their relationship with crop biomass remain largely unexplored.
Methods: In this study, topsoil (0–10 cm) samples were collected from a three-year field experiment in a wheat (Triticum aestivum cv. Jimai 22)-maize (Zea mays cv. Jiyuan 169) rotational cropping system. The experiment included treatments with and without biochar application (CK). Gene abundance of bacterial 16S rRNA and phoD, a gene encoding an alkaline phosphatase involved in phosphorus cycling, was quantified using quantitative polymerase chain reaction (qPCR). The compositions and diversities of bacterial, fungal, protistan, and phoD-harboring microbial communities were analyzed by Illumina MiSeq sequencing.
Results: Biochar application significantly reduced soil total phosphorus (TP) and ammonium nitrogen (NH4+-N) contents. It increased soil N:P ratios by 19.63%, 2.80%, 23.36%, and 27.10% in B0.5, B1.0, B1.5, and B2.0 treatments, respectively. Soil dissolved organic carbon (DOC) positively correlated with bacterial 16S rRNA gene abundance, while total nitrogen (TN) linked to the ratio of phoD to bacterial 16S rRNA gene abundance and rare protistan taxa. In terms of crop yield, the B1.5 treatment (3.42 t ha−1) increased wheat yield by 35% compared to the CK treatment. Mantel test and random forest analyses indicated that rare phoD-harboring, protistan, and fungal communities significantly contributed to wheat growth.
Discussion: This study offers valuable insights into the effects of biochar on soil microbiomes, especially the responses of abundant, intermediate, and rare taxa. The changes in soil nutrient contents and the correlations between soil properties and microbial communities suggest that biochar can modify the soil environment and microbial structure. The significant contribution of rare microbial communities to wheat growth emphasizes their importance in maintaining agricultural ecosystem health and ensuring sustainable ecosystem services. These findings can guide the rational application of biochar in alkaline farmland to promote sustainable agriculture.
1 Introduction
Soil microorganisms play a crucial role in soil element biogeochemical processes, such as carbon (C) degradation, nitrogen (N) mineralization, and phosphorus (P) solubilization (Jansson and Hofmockel, 2018; Arenberg and Arai, 2021). Currently, the rare biosphere is attracting the attention of researchers. Previous studies indicated that the entire microbial communities, such as bacteria and fungi, mediate soil ecosystem functions (Liu et al., 2021; Rachel et al., 2018). Nevertheless, in recent studies, rare taxa of those communities have proven to be essential in maintaining soil ecosystem functions and microbial diversity (Wang et al., 2023; Wang et al., 2024; Ma et al., 2024). Biochar, as a soil amendment, influences N-related processes such as ammonium absorption and nitrification rate, as well as processes related to phosphorus transformation, mainly through its interactions with microorganisms (primarily bacteria and fungi) (Clough and Condron, 2010; Lehmann et al., 2011; Zhang et al., 2018; Zhao et al., 2020). Despite their significance in nutrient cycling, particularly P solubilization and soil fertility maintenance, the roles of protists and phoD-harboring microbes in the biochar-soil-plant continuum remain poorly understood (Xiong et al., 2020).
Biochar is a porous and highly aromatic substance produced anoxically through the pyrolysis of biomass (e.g., crop waste and lignocellulosic biomass) (Dai et al., 2021). Biochar contains abundant functional groups, including carbonyl (C=O) and carboxyl (–COOH) groups, giving it exceptional adsorption capabilities for both organic and inorganic ions (Zama et al., 2018). Despite these properties of biochar, the effects of biochar on specific microbial groups, such as protists and phoD-harboring microbes, have been rarely reported (Yin et al., 2023; Rasit, 2022). Studies have found that biochar significantly influences soil microbial community composition, especially in bacteria and fungi (Shamim Gul et al., 2015; Luo et al., 2017a; Xu et al., 2020; Sarauer and Coleman, 2023). Beyond bacteria and fungi, protists are vital in mediating carbon, nitrogen and phosphorus cycling in agroecosystems (Li et al., 2021; Zhang et al., 2022). Research has primarily focused on how biochar affects soil bacteria, fungi, and specific functional microorganisms involved during nitrogen and carbon transformations (Ameloot et al., 2014; Rutigliano et al., 2014), neglecting its effect on protists in biochar-incorporated soils. Although protists are central in soil nutrient cycling, fertility maintenance, and agricultural ecosystem stability (Asiloglu et al., 2021; Ceja-Navarro et al., 2021; Nguyen et al., 2021; Sun et al., 2021) and are also important bioindicators of soil quality (Xiong et al., 2018), they are overlooked components of agricultural ecosystems with biochar. Consequently, understanding how biochar influences soil protists is of paramount importance.
P, an indispensable nutrient for microbes and plants, determines agricultural productivity and function (Bai et al., 2013; Shi et al., 2015). Only inorganic phosphates are accessible to microbes and plants (Garland et al., 2018). However, inorganic phosphate in the soil combines with iron and aluminum oxides and calcium-containing minerals, forming insoluble inorganic phosphate that accounts for 82% of the total P (TP) in soil and is unavailable to microbes and plants (Parent et al., 2014). In alkaline soils, alkaline phosphomonoesterases (ALPs) are crucial in the release of inorganic phosphate for plant and microbe uptake (Liang et al., 2020). Generally, three homologous genes (phoD, phoA, and phoX; produced by microorganisms) encode phosphatases (Fraser et al., 2015). Owing to its widespread distribution in bacteria and across soil types, the phoD gene is considered the most significant alkaline phosphatase-encoding gene (Ragot et al., 2015). Wei et al. (2019) demonstrated that rare taxa of phoD-harboring microorganisms were important for mediating P mineralization, while Li et al. (2023) found that they contributed greatly to C, N, and P cycling. Currently, the effects of biochar on these communities remain poorly understood. Therefore, understanding how biochar application affects the abundance and community composition of rare, intermediate, and abundant bacterial, fungal, protistan, and phoD-harboring microbe taxa is important for ensuring sustainable agricultural ecosystems.
This field-based study aimed to determine how varying biochar doses affect soil physicochemical properties; rare, intermediate, and abundant bacterial, fungal, protistan, and phoD-harboring microbial taxa; and wheat yield. The correlations between soil physicochemical properties, microbial communities, and wheat biomass after biochar application were also assessed. Thus, a comprehensive understanding of the soil microbiome response to biochar incorporation in agricultural ecosystems was obtained. We hypothesized that biochar affects wheat biomass primarily by influencing rare taxa of microorganisms. Understanding how biochar application affects the abundance and community composition of rare, intermediate, and abundant bacterial, fungal, protistan, and phoD-harboring microbe taxa and their relationships with wheat yield is important in sustainable agricultural ecosystems.
2 Materials and methods
2.1 Characteristics of experimental site, soil, and biochar
The field experiment is situated in western Shandong Province, specifically the wheat (Triticum aestivum cv. Jimai 22) and corn (Zea mays cv. Jiyuan 169) rotation area at the Soil Experiment Base of Liaocheng University, Shandong Province, China (Figure 1). This area is fluvo-aquic (WRB, 2015) with a soil bulk density, soil field capacity, pH, soil organic matter content, total nitrogen (TN), and soil labile P of 1.50 g cm−3, 26.80% (v/v), 8.78, 10.93 g kg−1, 0.71 g kg−1, and 18.49 mg kg−1, respectively. The climate is temperate monsoon climate with continental influences, and the annual average precipitation is 540 mm, concentrated in summer (June to August). The average annual temperature is approximately 13.5°C, while the frost-free period lasts 208 days. The field experiment began in June 2018, and the soil samples were collected in June 2021 throughout this period, wheat and corn were rotated. Wheat was planted from October of each year to May of the following year, while corn was planted from June to September of each year. Field management methods, such as fertilization and pest control, were the same as those of local farmers.
Fresh and uncontaminated corn straw was collected from corn fields after grain harvest. To reduce the moisture content, the straw was first spread out for nature air-drying in a well-ventilated and sunny area. After the moisture content reached approximately 10–15%, the dried straw was cut into 2–5 cm pieces using a sharp-bladed cutter or a forage chopper to ensure uniform heating during pyrolysis. For the pyrolysis of the pretreated corn straw, a muffle furnace was used in the experiment. The cut and dried corn straw pieces were placed in a heat-resistant crucible and loaded into the muffle furnace. The pyrolysis was carried out in an oxygen-limited environment. The heating rate was set at 10°C/min, and the target pyrolysis temperature was 450°C. Once the target temperature was reached, it was maintained for 2 h to allow for complete thermal decomposition of the corn straw’s cellulose, hemicellulose, and lignin into volatile gases, bio-oil, and solid biochar. After pyrolysis, the crucible with the biochar was removed form the muffle furnace and allowed to cool to room temperature in a desiccator. This rapid cooling process was crucial to preserve the biochar’s structure and properties. Subsequently, the cooled biochar was grounded into a fine powder. The grounded biochar was then sieved through a 2 mm mesh sieve to obtain a uniform particle size. The sieved biochar was stored in air-tight containers to prevent re-adsorption of moisture and contaminants prior to its incorporation into the soil. Additionally, the basic properties of the biochar were determined, including pH value of 7.44, TN content of 15.45 g kg−1, TC content of 761.20 g kg−1, H/C of 0.57%, electrical conductivity of 2,470 μS cm−1, available phosphorus content of 1,151.85 mg kg−1 and TP content of 4.20 g kg−1.
2.2 Experimental design
A completely randomized block experiment was performed to obtain five different amounts of biochar. Biochar was applied in accordance with the actual quantity of maize straw produced in a plot. The application rate of biochar was as follows: (1) CK: no biochar addition, (2) B0.5 (1.14 t ha−1): 0.5 times the amount of biochar equivalent to the carbonized maize straw produced per plot. (3) B1.0 (2.28 t ha−1): 1.0 time the amount of biochar equivalent to the carbonized maize straw produced per plot. (4) B1.5 (3.42 t ha−1): 1.5 times the amount of biochar equivalent to the carbonized maize straw produced from per plot. (5) B2.0 (4.56 t ha−1): 2.0 times the amount of biochar equivalent to the carbonized maize straw produced per plot. There were three replicates per treatment, resulting in 15 plots in total, with each plot being 8 m2.
Biochar was applied in the first year of the experiment and was not added thereafter. Biochar produced from maize straw was evenly spread on the soil surface before sowing winter wheat and then turned into tilled soil. The same amount of fertilizer was applied to each plot, including N (urea, 46% nitrogen, 225 kg ha−1), phosphate (P2O5, 150 kg ha−1), and potassium fertilizer (potassium chloride, 51% K2O, 150 kg ha−1). 50% of the total fertilizer was applied as base fertilizer before summer maize sowing, and the remaining 50% was applied during the jointing stage. The management measures were identical to the local agricultural management measures.
2.3 Soil sample collection and analyses
To compare the changes in soil physicochemical properties and microbial indicators of biochar amendment treatments with those of a control treatment, soil samples were collected in June 2021, 3 years after the experiment began. Stem, leaf, tassel, and grain samples, collected in the same year after the wheat had ripened, were oven-dried (70°C) until reaching a constant weight, to determine their dry weights. Fifteen soil core samples were collected at a 0–10 cm depth, completely mixed, and sieved through a 2 mm mesh to remove roots and rocks. The mixed samples were separated into two. The two subsamples were maintained at 4°C and −20°C to assess soil physicochemical properties and for DNA extraction and subsequent analysis, respectively.
The soil pH was determined using a soil and water suspension at a 1:2.5 ratio (w/w). Soil dissolved organic C was analyzed using a TOC analyzer (Vario TOC, Elementar, Germany). Soil TP was measured using the ammonium molybdate method after digestion with perchloric and sulfuric acids. Soil available P was determined using a UV-V spectrophotometer equipped with phosphomolybdate blue. Soil NH4+-N and NO3−-N concentrations were analyzed using UV spectrophotometry. Soil TC and TN were measured using an elemental analyzer (Vario MICRO cube, Elementar, Germany).
ALP activity was determined as described by Tabatabai (1994). One gram of fresh soil was incubated for 1 h at 37°C in a pH 11.0 buffer containing p-nitrophenol phosphate, after being agitated with 0.5 mL toluene for 15 min. The process was stopped using 0.5 M NaOH. UV spectrophotometry was performed at 420 nm to examine p-nitrophenol production. The μg p-nitrophenol g−1 soil h−1 were used to express ALP activity.
2.4 Soil DNA extraction and quantification of bacterial 16S rRNA and phoD genes
A Fast DNA SPIN Kit for soils (MP Biomedicals, United States) was used to extract genomic DNA from frozen soil samples (0.5 g) according to the manufacturer’s instructions. A NanoDrop ND-2000 spectrophotometer (NanoDrop Co., United States) was used to assess the DNA extracts’ quality and quantity. The abundance of bacterial 16S rRNA and phoD genes in the soil samples was measured using quantitative real-time polymerase chain reaction (qPCR) and an iQTM5 Thermocycler (Bio-Rad). The phoD gene was quantified using a SYBR Green assay. TaqMan qPCR was used for detecting bacterial 16S rRNA genes. The final step produced a melting curve used to guarantee the amplification specificity of the reaction. The cloned plasmids were diluted 10 times in serial order to create a standard curve. All assay efficiencies exceeded 90%. The conditions and programs for qPCR are shown in Supplementary Table S1.
2.5 Illumina sequencing and data processing
Bacteria, fungi, protists, and phoD-containing microorganisms were evaluated by Illumina MiSeq sequencing. Three commonly used primer sets were used to study microbial communities, targeting bacterial 16S rRNA and fungal and protistan 18S rRNA genes (Rousk et al., 2010; Stoeck et al., 2010; Fadeev et al., 2021). Details of the primers are shown in Supplementary Table S2. The phoD gene was amplified using primers phoD-F733 and phoD-R1083, following Chen et al. (2019). PCR reactions were conducted in 25 μL mixtures (12.5 μL PremixTaqTM, 0.5 μL of each primer, 2 μL of temperate DNA, and 9.5 μL of sterilized ddH2O). The PCR conditions for each primer set are listed in Supplementary Table S2. Sequencing experiments were performed at Shanghai Personalbio Technology Co., Ltd. (Shanghai, China). After sequencing, the raw data were uploaded to the Sequence Read Archive database of the National Center for Biotechnology Information (NCBI), and the BioProject IDs were PRJNA970957 and PRJNA1111768.
Protocols by Fadrosh et al. (2014) were used to process raw sequencing read. Barcodes from sequences were extracted during pre-processing, and a TagCleaner was used to clean primer sequences. Quantitative Insights Into Microbial Ecology was used to analyze the filtered reads. Low-quality reads, namely chimeras and erroneous sequences, were removed using quality filtering during ChimeraSlayer and demultiplexing, respectively. Operational taxonomic units (OTUs) were classified with 97% similarity. To eliminate the effect of uneven sequencing depth among samples, sequences in OTU tables were re-sampled to 51,295, 70,452, 58,299, and 40,373 for bacteria, fungi, protists, and phoD-harboring microbes, respectively. The OTU taxonomy was identified against the 16S/Silva/v132 database for bacterial sequences, 18S/Silva/v132 for micro-eukaryotic sequences, and NCBI for phoD-harboring microbial sequences. Based on research by Jiao and Lu (2020), we defined rare, intermediate, and abundant taxa according to their relative abundances (Jiao and Lu, 2020). Rare taxa with relative abundances of <0.01% the total sequences were analyzed. Conversely, the relative abundance of intermediate taxa fell within 0.01–0.1% (Jiao and Lu, 2020). The most abundant taxa were OTUs with relative abundances exceeding 0.1% of the total sequences (Jiao and Lu, 2020). A total of 769,425 high-quality bacterial reads were obtained and assigned to 24,999 OTUs at a 97% similarity level. In total, 93.37% of OTUs were classified as rare taxa, accounting for 30.43% of the average relative abundance across the entire sample (Supplementary Table S3). In contrast, a small percentage of OTUs (0.43%) were classified as abundant taxa; however, they accounted for 21.82% of the overall sample’s average relative abundance (Supplementary Table S3). The average relative abundance of all the sample’s intermediate taxa, accounting for 6.20% of the total, was 47.75% (Supplementary Table S3). For fungi, 1,056,780 high-quality sequences were obtained, assigned to 3,032 OTUs at a 97% similarity level. Although a large percentage of the OTUs (89.64%) were rare taxa, they accounted for only 3.63% of the average relative abundance across all samples (Supplementary Table S3). Meanwhile, only 2.41% of OTUs were classified as abundant taxa, although accounting for 89.67% of the whole sample’s average relative abundance (Supplementary Table S3). Intermediate taxa comprised 7.95%, accounting for 6.70% of the total sample’s average relative abundance. A total of 874,485 high-quality protist sequences were obtained and assigned to 7,572 OTUs at a 97% similarity level. A total of 12.42% of the average relative abundance of the entire sample was comprised of rare taxa, which accounted for 87.67% of the OTUs (Supplementary Table S3). Only 1.60% of the OTUs were classified as abundant taxa, despite comprising 65.74% of the total sample’s average relative abundance (Supplementary Table S3). The average relative abundance of all the sample’s intermediate taxa, accounting for 10.74% of the total, was 21.84% (Supplementary Table S3). A total of 605,595 high-quality sequences of phoD-harboring microbial communities were obtained, assigned to 9,614 OTUs at a 97% similarity level. The majority of the OTUs (88.17%) classified as rare taxa accounted for 15.42% of the average relative abundance across all samples (Supplementary Table S3). Only 1.65% of OTUs were classified as abundant taxa; however, they accounted for 56.25% of the average relative abundances across all samples (Supplementary Table S3). The percentage of intermediate taxa was 10.17%, accounting for 28.33% of the average relative abundance in all the samples (Supplementary Table S3).
2.6 Statistical analysis
Unless otherwise stated, all statistical analyses were performed in the R environment,1 and most statistics were constructed using “ggplot2,” “vegan,” “ggpubr,” “ape,” “agricolae,” and “randomForest.” We considered p < 0.05 to be statistically significant, unless otherwise stated. To evaluate the normality and homogeneity of variance of each edaphic component, we employed the Shapiro–Wilk and Levene tests, respectively. One-way analysis of variance (ANOVA) was used to assess the differences in the physicochemical properties, the abundance of microbial gene copies and the composition of related microbial communities between the biochar treatments.
Non-metric multidimensional scaling analysis (NMDS) based on the Bray–Curtis distance matrices of communities was performed to analyze the abundant, intermediate, and rare bacterial, fungal, protistan, and phoD-containing microbe communities’ structures in R using the “vegan” and “ggplot2” packages. And the dissimilarity test of Adonis was utilized to demonstrate the significance of community compositional changes. Mantel’s test was used to evaluate correlations between soil properties factors, wheat yield factors and soil bacterial, fungal, protistan and phoD-harboring communities, using the “vegan” package. A machine-learning model (random forest) was used to investigate the robustness of associations between both wheat yield and soil physicochemical properties and microbial taxa represented by NMDS1 using the R package “randomForest.”
3 Results
3.1 Soil physicochemical properties
Biochar incorporation significantly affected soil physicochemical properties (Table 1). Soil NH4+-N and NO3−-N were significantly lower in B2.0 and in both B1.0 and B1.5 than in CK, respectively. Biochar incorporation significantly decreased soil TP in B0.5 and B1.5, whereas differences between biochar treatments and CK for AP were nonsignificant. Soil TN in B1.0, B1.5, and B2.0 exceeded that in CK. Biochar incorporation increased soil N:P by 19.63, 2.80, 23.36, and 27.10% in B0.5, B1.0, B1.5, and B2.0, respectively. No significant differences in pH, DOC, TC, C:N, and ALP activity were observed between the biochar treatments and CK.
3.2 The abundance of phoD and bacterial 16S rRNA gene and the ratio of phoD to bacterial 16S rRNA gene
The quantitative PCR results showed that phoD gene copy numbers were high (2.20 × 108–3.56 × 108 per g of dry soil) in all soil samples (Figure 2A). Biochar incorporation did not significantly alter phoD abundance compared with that in CK. The abundance of bacterial 16S rRNA gene copies ranged from 7.31 × 108 to 1.49 × 109 per g of dry soil (Figure 2B). Bacterial abundance did not differ significantly among treatments. However, biochar incorporation significantly increased the ratio of phoD-harboring microbes to bacteria (Figure 2C). Compared with CK, higher ratios of phoD-harboring microbes to bacterial 16S rRNA genes existed in B0.5, B1.0, and B2.0. Typically, the ratios increased to 31.46, 40.49, and 47.68% for B0.5, B1.0, and B2.0, respectively (Figure 2C). The ratio of phoD to bacterial 16S rRNA genes in B1.5 was 32.56%, which was higher than that in CK, although the difference was nonsignificant (Figure 2C).
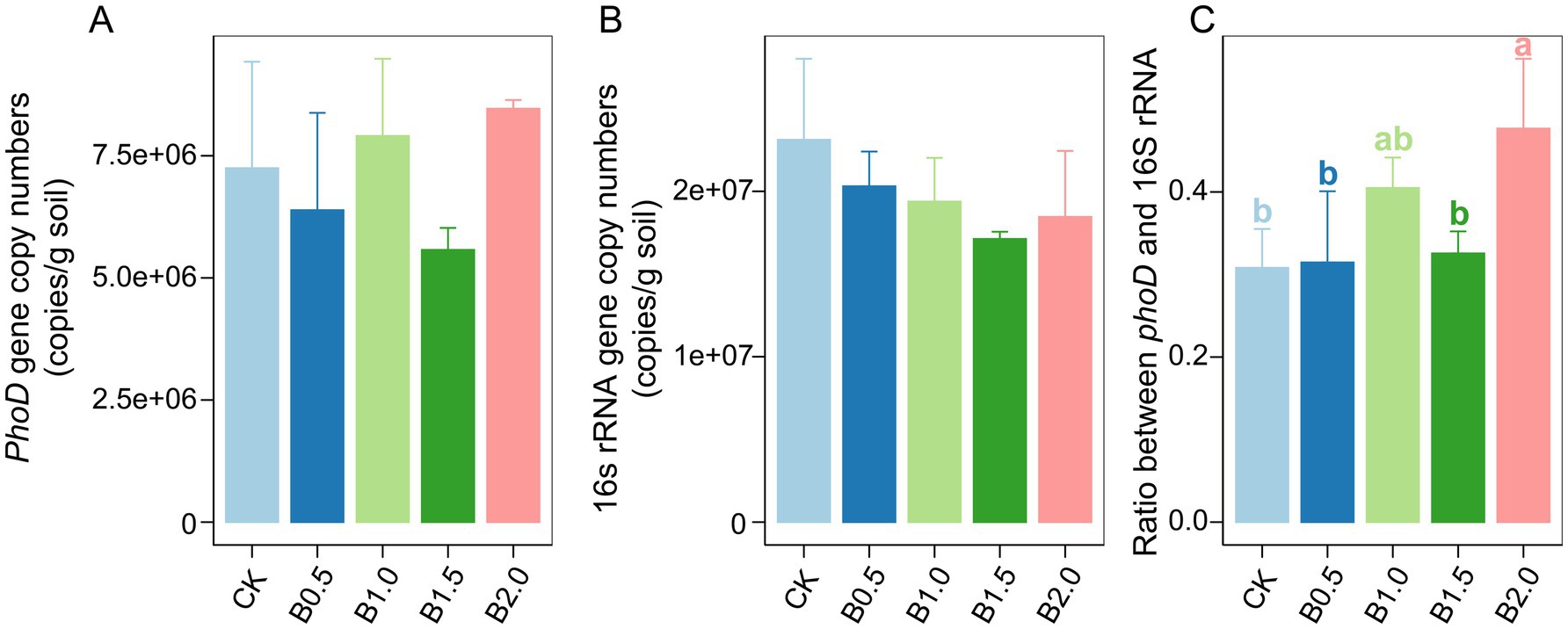
Figure 2. phoD (A) and bacterial 16S rRNA gene (B) and ratio of phoD to bacterial 16S rRNA gene (C) copy numbers in different treatments. CK: without biochar incorporation, B0.5: plot amended with 1.14 t ha−1 of biochar, B1.0: plot amended with 2.28 t ha−1 of biochar, B1.5: plot amended with 3.42 t ha−1 of biochar, B2.0: plot amended with 4.56 t ha−1 of biochar.
Mantel’s test was used to investigate associations between soil physicochemical properties and three key metrics: phoD gene copies, bacterial 16S rRNA gene copies, and the ratio of phoD gene to bacterial 16S rRNA gene copies across the five different treatments. The soil 16S rRNA gene copy number and soil DOC correlated significantly. Furthermore, a positive correlation existed between the ratio of phoD gene copy number to bacterial 16S rRNA gene copy number and soil TN across treatments (Supplementary Figure S1).
3.3 The structures of bacterial, fungal, protistan, and phoD-harboring microbial abundant, intermediate, and rare taxa
NMDS-based OTU matrices were used to visualize the differentiation in the abundant, intermediate, and rare bacterial, fungal, protistan, and phoD-containing microbe taxa in the five treatments (Figure 3). For bacteria, NMDS clustering of the intermediate and rare taxa showed a large degree of overlap among treatments, implying that the community structures had not changed significantly (Figures 3A,B). Regarding the abundance of bacterial taxa, subsample points of B1.0 were notably distant from those of B0.5, B1.5, and B2.0 (Figure 3C). Species composition analysis shows that Actinobacteria and Proteobacteria were the dominant species in the in the abundant and intermediate bacterial communities (Supplementary Figure S3). And Actinobacteria, Bacteroidetes and Proteobacteria were the dominant species in the in the rare bacterial community (Supplementary Figure S3).
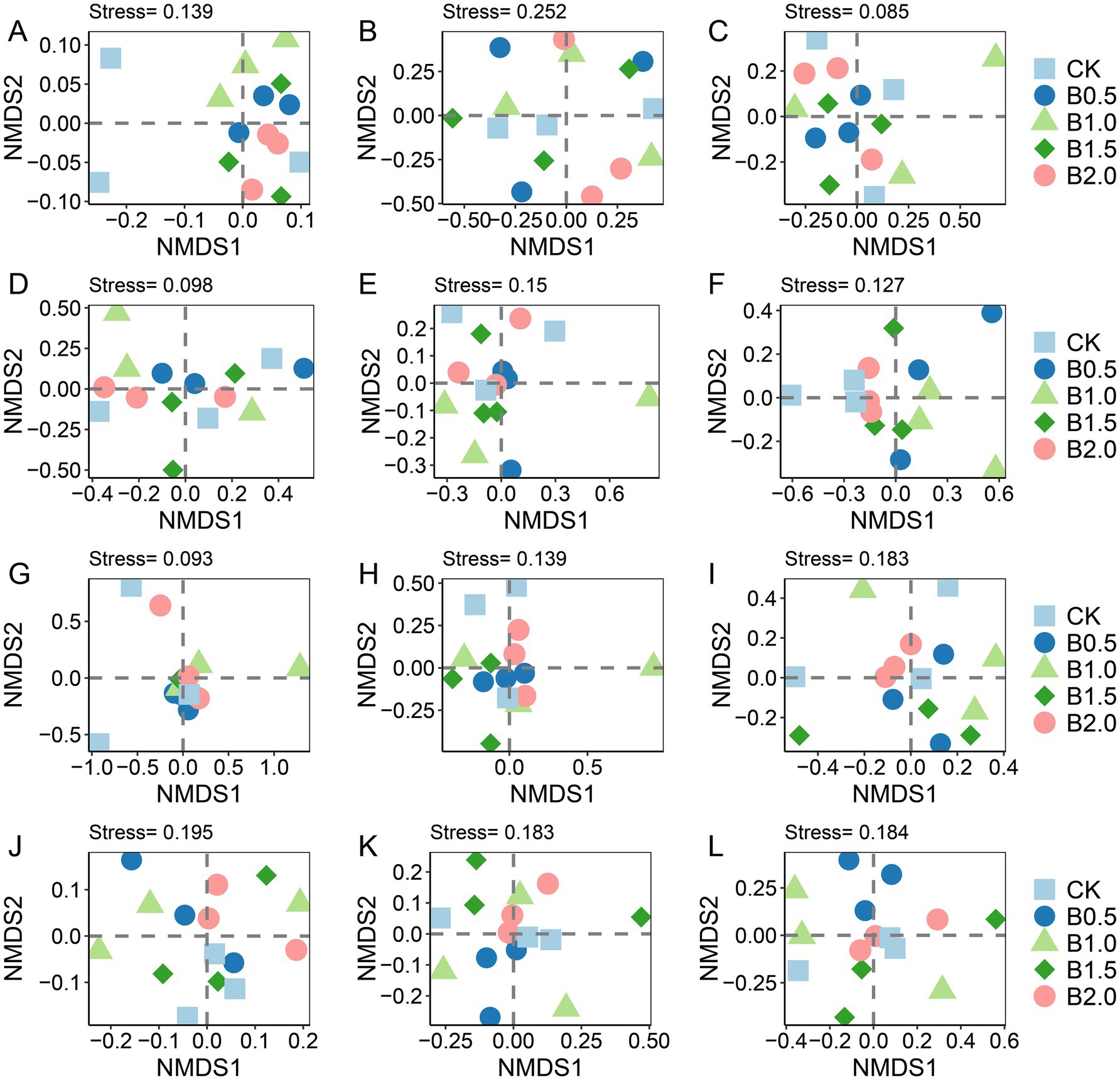
Figure 3. Non-metric multidimensional scaling (NMDS) analysis based on the Bray–Curtis distance matrix for all OTUs was performed on the abundant, intermediate, and rare taxa of bacteria (A–C), fungi (D–F), protist (G–I) and phoD-harboring microbial communities (J–L) in the samples. CK: without biochar incorporation, B1: plot amended with 1.14 t ha−1 of biochar, B2: plot amended with 2.28 t ha−1 of biochar, B3: plot amended with 3.42 t ha−1 of biochar, B4: plot amended with 4.56 t ha−1 of biochar.
Regarding the relative abundances of rare, intermediate, and abundant bacterial taxa, the relative abundances of intermediate and abundant bacterial taxa were higher and lower in biochar-amended treatments, respectively, than in CK (Table 2). B1.5 showed the highest relative abundance of rare bacterial taxa (Table 2). The links between soil physicochemical properties and bacterial abundant, intermediate, and rare species was investigated using Mantel’s test. No significant correlation existed between the soil physicochemical properties and the abundance of the different bacterial taxa (Supplementary Figure S2A), suggesting that their distribution and abundance may be influenced by factors other than soil physicochemical properties in the studied environment.
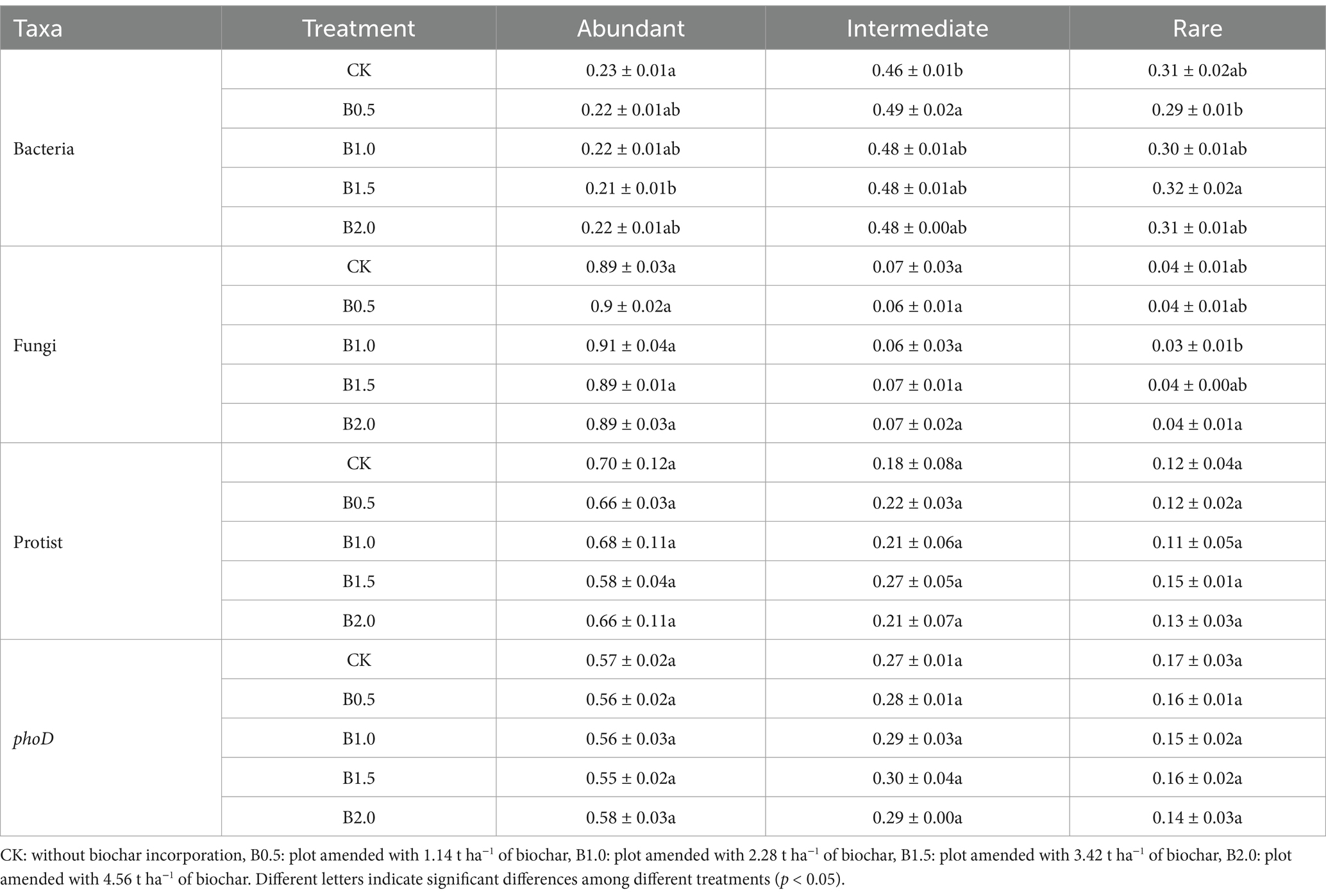
Table 2. Relative abundance of the abundant, intermediate and rare taxa of bacterial, fungal, protistan, and phoD-harboring microorganisms among different treatments.
For fungal communities, no significant difference in the abundant and intermediate fungal community taxa existed between treatments (Figures 3D,E). The distribution of sample points for rare fungal taxa was relatively dispersed across different treatments, suggesting that biochar addition had a pronounced effect on the fungal community structure, particularly for rare taxa (Figure 3F). Species composition analysis shows that Ascomycota and Basidiomycota were the dominant species in the in the abundant, intermediate and rare fungal communities (Supplementary Figure S4). Interestingly, we found that the abundance of Protosteliales increased significantly in fungal intermediate taxa. Moreover, the relative abundances of two subcommunities (abundant and intermediate taxa) in the biochar-amended treatments were not significantly different from that of CK, whereas that of rare fungal taxa was significantly lower in B1.0 than in CK (Table 2). Crucial elements influencing the assembly of three fungal subcommunities (abundant, intermediate, and rare) were determined. Mantel’s test was used to assess relationships between soil characteristics and the three fungal subcommunities among the five treatments. No significant correlation existed between the soil physicochemical properties and rare fungal taxa abundance (Figure 4A).
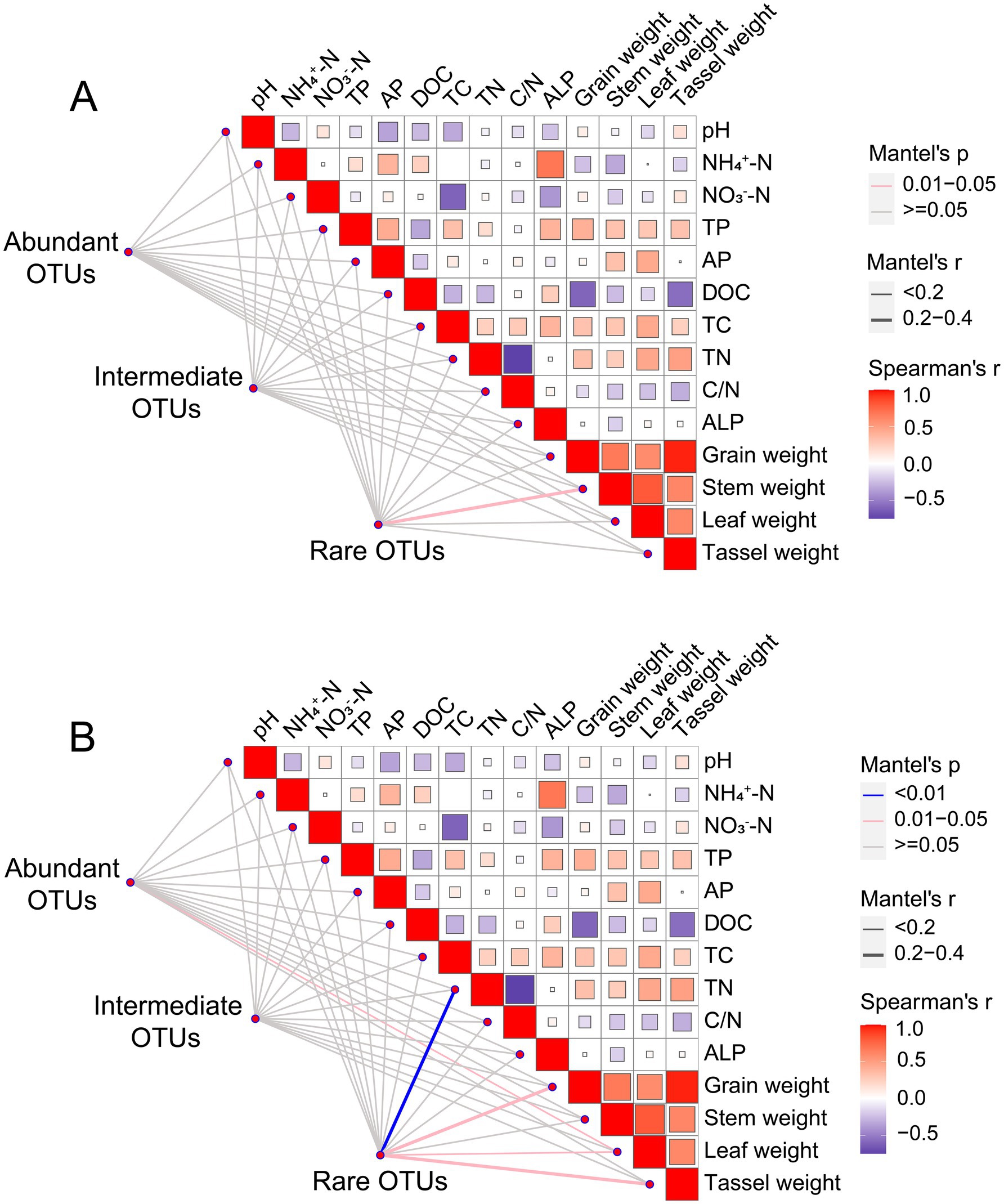
Figure 4. Pairwise comparisons of environmental factors are shown in the upper right corner, with a color gradient denoting Spearman’s correlation coefficient. Abundant (relative abundance >0.1%), intermediate (0.01% <relative abundance <0.1%) and rare OTUs (relative abundance <0.01%) of fungi (A) and protist (B) are related to each soil attribute and wheat yield by Mantel test. Edge color denotes the statistical significance and edge width corresponds to the Mantel’s r statistic for the corresponding distance correlations. TP, total phosphorus; AP, available phosphorus; TN, total nitrogen; TC, total carbon; NO3−-N, dissolved nitrate nitrogen; NH4+-N, dissolved ammonium nitrogen; DOC, dissolved organic carbon; ALP, potential alkaline phosphatase activity.
For phoD-harboring microbes, Adonis results revealed significant differences between the community structures of intermediate (p = 0.092) and rare (p = 0.08) phoD-harboring microbial taxa among five treatments. NMDS clustering of intermediate and rare phoD-harboring microbial taxon communities showed that the sample points of B2.0 did not overlap those of CK and B0.5, implying that the community structures of intermediate and rare phoD-harboring microbial taxa changed significantly in B2.0 (Figures 3H,I). Species composition analysis shows that Proteobacteria and Actinobacteria were the dominant species in the in the abundant, intermediate and rare phoD-harboring communities (Supplementary Figure S5). And we found that the rare phoD-harboring communities were more diverse. Furthermore, we examined the relative abundances of abundant, rare, and intermediate phoD-harboring microbial taxa in the five treatments. Although no discernible differences in the relative abundances of abundant, intermediate, or rare phoD-harboring microbial taxa occurred between the biochar-amended and CK, the relative abundance of intermediate phoD-harboring microbial taxa was higher (Table 2). Mantel’s test was also utilized among the five treatments to investigate the relationship between soil characteristics and phoD-harboring microbes, specifically focusing on abundant, rare, and intermediate taxa. AP correlated significantly with phoD-harboring intermediate taxa (Supplementary Figure S2B).
NMDS clustering of abundant and intermediate protistan taxon communities showed a large degree of overlap among treatments, implying that the community structure had not changed significantly (Figures 3J,K). The consistently significant distance between subsample points in the rare taxa of the protistan community between CK and B1.5 indicated that biochar incorporation significantly affected the community structure. This suggested that biochar addition caused distinct changes in the rare protist taxa, potentially altering their diversity, abundance, or distribution (Figure 3L). We found alterations in the dominant species of the abundant, intermediate and rare protistan taxon communities (Supplementary Figure S6). Arthropoda was the dominant species in the abundant protistan taxon community (Supplementary Figure S6). Nematoda was the dominant species in the intermediate protistan taxon community (Supplementary Figure S6). And Ascomycota was the dominant species in the rare protistan taxon community (Supplementary Figure S6). The relative abundance of abundant, intermediate, and rare protistan taxa did not differ significantly between biochar-added treatments and CK (Table 2). However, the relative abundance of rare protistan taxa was generally higher in B1.5 and B2.0 than in CK, indicating that biochar application, specifically in B1.5 and B2.0 levels, may have promoted the growth or persistence of rare protistan taxa in the soil. Mantel’s test was also used to investigate associations between soil characteristics and abundant, rare, and intermediate protist species for all treatments. Abundant and intermediate protistan taxa did not correlate with soil attributes, whereas rare protist taxa correlated significantly with soil TN (Figure 4B).
3.4 Wheat yield
In the third year after biochar application, we measured the grain, stem, tassel, and leaf weights of wheat. Biochar application did not significantly affect wheat growth (Figure 5). However, different biochar treatments slightly increased the grain, stem, tassel, and leaf weights of wheat. The highest increase occurred in B1.5. Although the statistical analysis is not significant, the treatment of B1.5 (3.42 t ha−1) has increased the wheat yield by 35% compared to the control without the addition of biochar. We then conducted Manel’s test and random forest regression analyses between soil physicochemical properties, biological factors, and wheat yield. No significant associations occurred between abundance of phoD gene copies, the abundance of bacterial 16S RNA gene copies, and their ratios with wheat growth parameters such as grain, stem, leaf, and tassel weights (Supplementary Figure S1). Notably, Mantel’s analysis revealed a significant effect of rare fungal taxon communities on stem weight, whereas rare protistan taxon communities significantly affected grain, tassel, and leaf weights (Figure 4). Random forest regression analyses revealed that rare phoD-harboring microbial taxon communities significant influenced grain weight, stem weight, tassel weight and leaf weight of wheat (Figure 6). Furthermore, DOC and TC correlated significantly with grain and leaf weights, respectively (Figure 6).
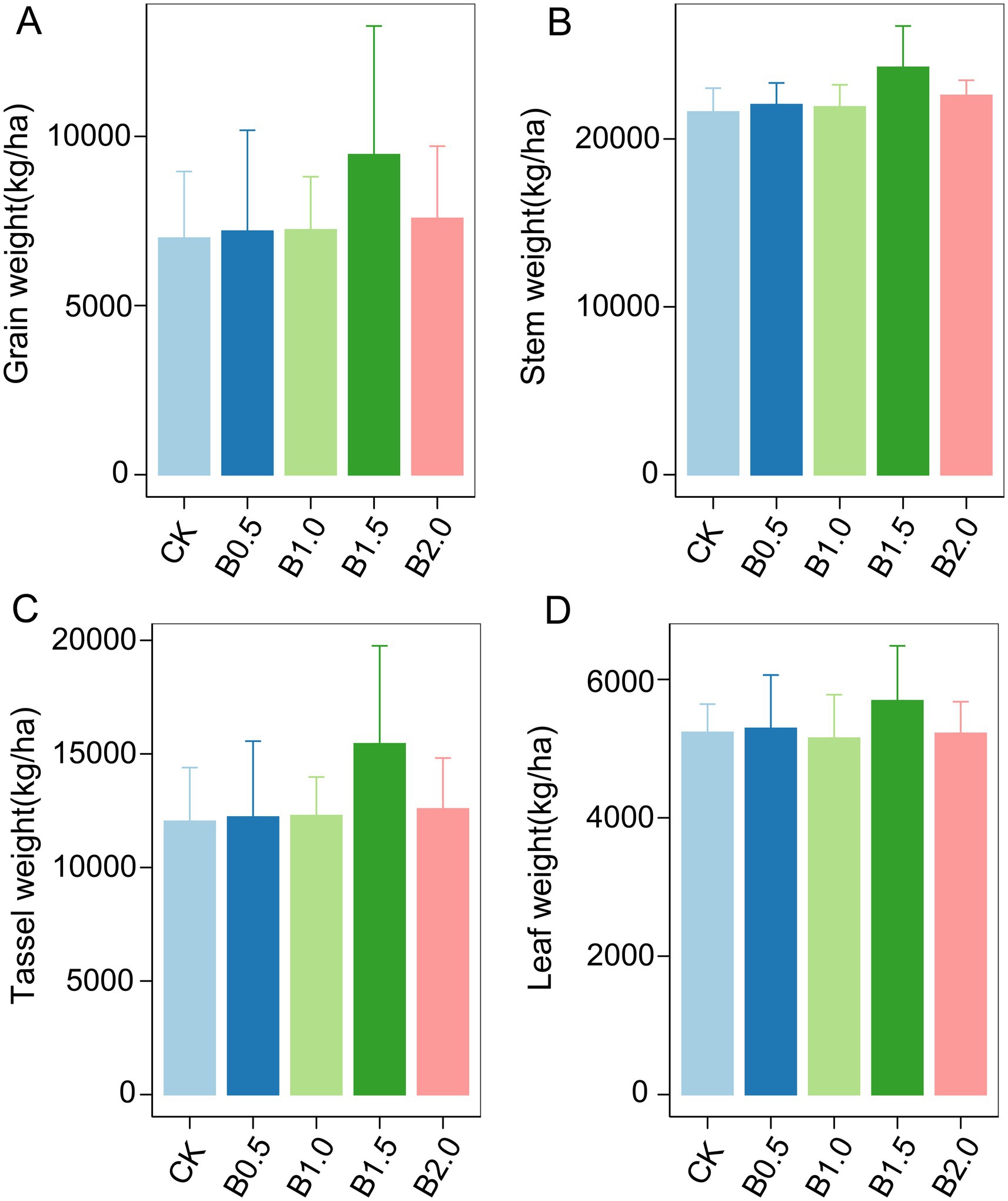
Figure 5. Above-ground biomass during the wheat growth across different treatments are presented. (A) is grain weight; (B) is stem weight; (C) is tassel weight; (D) is leaf weight. Error bars indicate that the standard deviation of triplicate analyses. No lowercase letters indicate no significant differences among different treatments (p > 0.05). CK: without biochar incorporation, B0.5: Plot amended with 1.14 t ha−1 of biochar, B1.0: Plot amended with 2.28 t ha−1 of biochar, B1.5: Plot amended with 3.42 t ha−1 of biochar, B2.0: Plot amended with 4.56 t ha−1 of biochar.
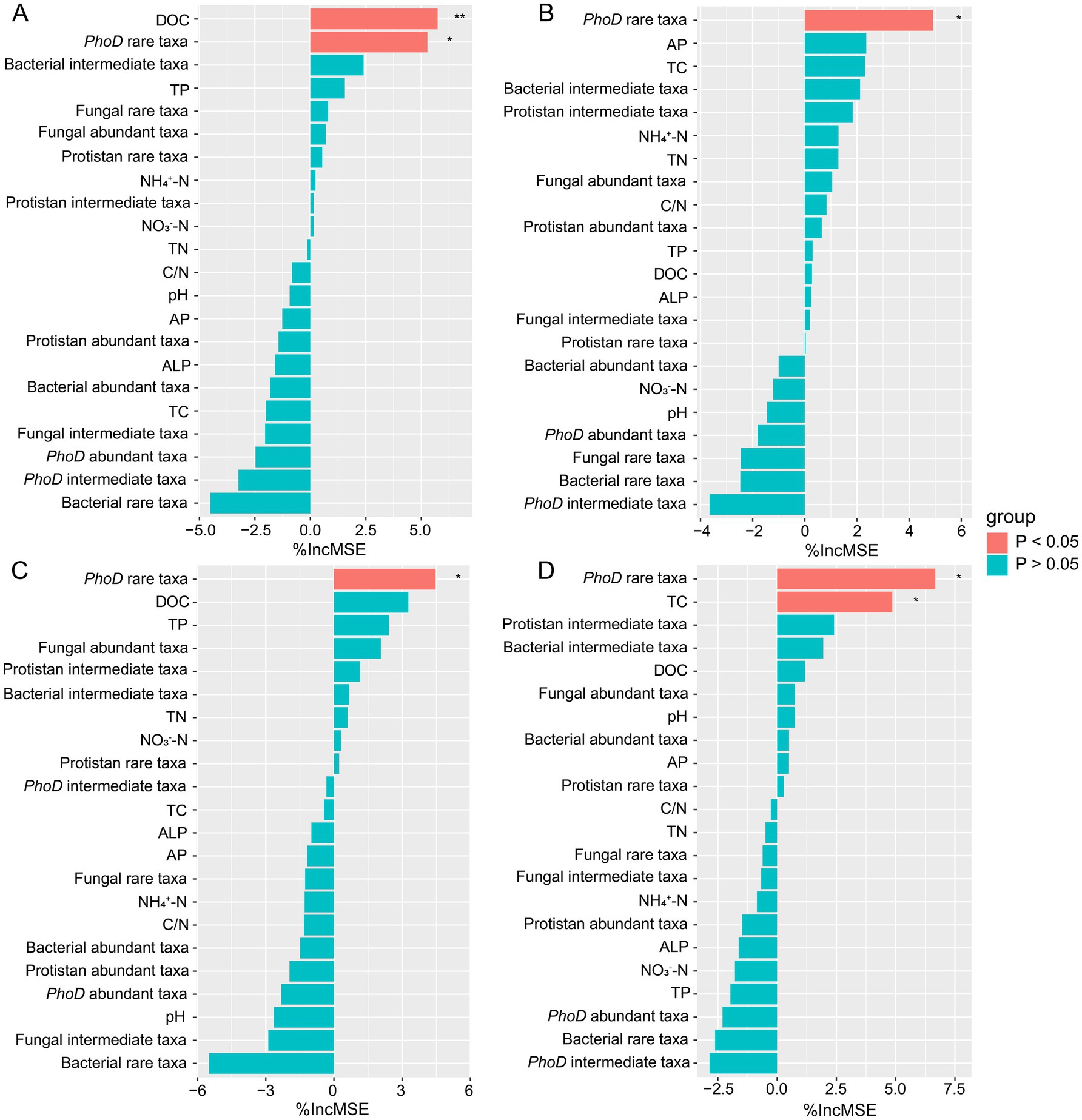
Figure 6. Random forest analysis against abiotic and biotic factors were analyzed for wheat grain weight (A), stem weight (B), tassel weight (C) and leaf weight (D). **p < 0.01 and *p < 0.05.
4 Discussion
4.1 Effect of biochar on the soil physicochemical properties
Biochar incorporation significantly influenced soil physicochemical properties, although the effects varied depending on the specific property and treatment. Among the five treatments, no significant differences were detected in AP (Table 1). This finding aligns with previous research indicating that the biochar application does not always significantly affect soil AP (An et al., 2022; Yuan et al., 2022). In our study, soil AP content decreased in biochar-added treatments, except for B1.0 (Table 1). The exception in B1.0 might be attributed to the specific interaction between biochar properties and soil conditions at this application rate, which warrants further investigation. For TP, biochar amendment led to a decrease in soil TP in B0.5, B1.5, and B2.0. This reduction could be attributed to biochar’s strong adsorption capacity, as demonstrated by Bornø et al. (2018), which may immobilize phosphorus and reduce its availability in the soil.
Biochar incorporation did not significantly alter soil pH, which contrasts with some previous studies (Tian et al., 2021; Yuan et al., 2022). This discrepancy may be related to the inherently alkaline nature of the original soil (pH 8.78) and the relatively low biochar application rate in this study used. The buffering capacity of the soil likely mitigated any potential pH changes induced by biochar. Although there were no significant differences in soil NH4+-N content among the five treatments, NH4+-N levels were lower in biochar-amended treatments compared to the control (CK). This observation aligns with the findings of Lehmann et al. (2011), who reported that biochar’s porous structure and negatively charged functional groups can enhance NH4+-N adsorption, potentially reducing its availability in the soil solution. This mechanism may explain the lower NH4+-N content in biochar-amended treatments. Additionally, biochar incorporation altered microbial communities, which might immobilize nitrogen, reducing the available NH4+-N for crops in the short term.
4.2 Effects of biochar on bacterial 16S rRNA and phoD genes copies and the ratio of phoD to bacterial 16S rRNA gene
In this study, a significant and positive correlation was observed between soil DOC and bacterial 16S rRNA gene copy number (Supplementary Figure S1). This suggests that labile carbon, represented by DOC, directly influences soil bacterial abundance. This finding is consistent with previous studies indicating that biochar can provide a carbon source, particularly labile C, for microorganisms, thereby impacting bacterial abundance (Cross and Sohi, 2011; Rutigliano et al., 2014; Huang et al., 2021). For instance, Dai et al. (2018) reported that labile carbon from biochar affects microbial biomass in farmland soil. Additionally, Luo et al. (2017b) utilized isotopic tracing methods to demonstrate that the labile carbon fraction of biochar strongly correlates with microbial respiration, which in turn influences bacterial abundance. These findings support our results and highlight the importance of labile carbon in regulating microbial dynamics.
Furthermore, soil TN correlated positively with the ratio of phoD gene abundance to bacterial 16S rRNA gene abundance (Supplementary Figure S1). This relationship may be explained by the fact that N amendments can increase P fertilizer availability, and N incorporation prompts microorganisms to invest in phosphatase production (Arenberg and Arai, 2019). A meta-analysis by Margalef et al. (2017) also revealed that TN is a key factor influencing regional variations in phosphatase activity. Additionally, ammonium has been shown to significantly promote alkaline phosphatase activity generated by phoD-containing microorganisms (Arenberg and Arai, 2019). Dai et al. (2018) reported that biochar influences soil P cycling by affecting the activity and communities of P-solubilizing microbes. Microbial biomass size, activity, and reproduction are determined based on soil nutrient availability (Fu et al., 2021; Xue et al., 2023). Moreover, Zhang et al. (2023) reported that phoD abundance correlates positively with TN, a notable environmental parameter affecting phoD-harboring bacteria. These findings suggest that biochar-induced changes in soil N availability may indirectly influence P cycling by modulating the activity and abundance of phoD-harboring microbes.
4.3 Effects of biochar on abundant, intermediate, and rare bacterial, fungal, protistan, and phoD-harboring microbe taxa after biochar incorporation
The Adonis results indicated that biochar incorporation had no significant impact on the composition of abundant, intermediate, and rare bacterial taxa or abundant and intermediate fungal taxa. This finding contrasts with some previous studies, which have reported varying effects of biochar on soil microbial communities depending on application rates, biochar type, and soil conditions (Lehmann et al., 2011; Gul and Whalen, 2016; Dai et al., 2018; Dai et al., 2021; Huang et al., 2021). In our study, the relatively low biochar application rates (0–4.56 t ha−1) may explain the lack of significant effects on these microbial groups (Zhang et al., 2021). Notably, rare fungal taxon exhibited significant differences among treatments, suggesting that they are more responsive to biochar addition, which often occupy narrow ecological niches and are more susceptible to environmental changes.
For phoD-harboring microbes, Adonis analysis revealed that biochar addition significantly altered the structure of intermediate and rare taxa. The finding aligns with Wei et al. (2019), who implicated rare taxa in mediating soil phosphorus mineralization. Mantel’s analysis further revealed a significant correlation between AP and intermediate phoD-harboring taxa (Supplementary Figure S2B). This suggests that intermediate phoD-harboring taxa may play a key role in P cycling under biochar-amended conditions. Additionally, rare protistan taxa were significantly affected by biochar addition, with their community structure showing distinct changes in responses to treatment (Figure 3L). Rare protists, despite their low abundance, are increasingly recognized as important components of soil ecosystems (Jousset et al., 2017). They play a crucial role in maintaining the ecological balance and resilience of soil ecosystems and contribute to nutrient cycling (Jousset et al., 2017). Phagotrophic protists among them are key factors in controlling bacterial and fungal communities in soil (Xiong et al., 2018). Through predation, they release nutrients immobilized in microbial biomass, which could accelerate nutrient turnover and increases plant nutrient uptake (Xiong et al., 2018). Protists also contribute significantly to nutrient cycling, being involved in organic matter degradation and carbon fixation processes, with their activities facilitating nutrient transformation in the soil (Xiong et al., 2020). The significant correlation between rare protistan taxa and soil TN (Figure 4B) further highlights their potential role in N dynamics.
4.4 Effect of biochar on the wheat yield
Biochar application did not significantly increase wheat yield compared to in the control (CK) (Figure 5). This study is consistent with some previous studies, which have reported limited or no yield response to biochar application (Ye et al., 2019; Xu et al., 2015; Bai et al., 2022). The lack of a significant yield increase may be attributed to the low nutrient content of the maize straw biochar used in this study, which may have limited its ability to enhance soil fertility and plant growth. Additionally, the relatively low application rates of biochar (up to 4.56 t ha−1) may have been insufficient to induce significant changes in soil properties or crop performance.
Although the statistical analysis is not significant, the treatment of B1.5 (3.42 t ha−1) has increased the wheat yield by 35% compared to the control without the addition of biochar. Random forest regression analysis indicated that DOC and TC significantly affected crop growth (Figure 6). However, DOC and TC concentrations did not differ significantly among treatments, suggesting that other factors, such as microbial community composition, may have played a more important role in influencing yield. Notably, rare fungal and protistan taxa were significantly correlated with wheat growth parameters, including stem weight, grain weight, tassel weight, and leaf weight (Figure 4). These findings highlight the potential importance of rare microbial taxa in regulating crop performance and suggest that biochar-induced changes in microbial communities may indirectly influence yield.
5 Conclusion
Our findings indicate that biochar addition to fluvo-aquic soil altered soil physicochemical properties, particularly TP and NH4+-N. The application of biochar at 3.42 t ha−1 slightly increased wheat yield, although the effect was not statistically significant. Rare phoD-harboring taxa and DOC were identified as key factors influencing wheat grain yield. Additionally, rare protistan taxa significantly correlated with wheat growth, highlighting their potential role in nutrient cycling and crop performance. Future studies should further investigate the functional roles of rare phoD-harboring and protistan taxa to fully understand their interactions with soil P cycling and crop growth in biochar-amended soil. Moreover, longer-term field trials are needed to validate these findings and explore the potential benefits of higher biochar application rates.
Data availability statement
The datasets presented in this study can be found in online repositories. The names of the repository/repositories and accession number(s) can be found in the article/Supplementary material.
Author contributions
J-QQ: Data curation, Formal analysis, Writing – original draft. H-YY: Conceptualization, Funding acquisition, Methodology, Supervision, Writing – original draft, Writing – review & editing. S-CS: Conceptualization, Investigation, Methodology, Writing – review & editing. EZ: Writing – review & editing. B-XT: Writing – review & editing. JL: Writing – review & editing. B-HZ: Writing – review & editing.
Funding
The author(s) declare that financial support was received for the research and/or publication of this article. This work was funded by the National Natural Science Foundation of China (42007219), Natural Science Foundation of Shandong Province, China (ZR2020MD004), Students’ Innovation and Entrepreneurship Training Program of Liaocheng University (S202210447046), and Doctoral Scientific Research Foundation of Liaocheng University (318052034 and 318012114).
Conflict of interest
The authors declare that the research was conducted in the absence of any commercial or financial relationships that could be construed as a potential conflict of interest.
Generative AI statement
The authors declare that no Gen AI was used in the creation of this manuscript.
Publisher’s note
All claims expressed in this article are solely those of the authors and do not necessarily represent those of their affiliated organizations, or those of the publisher, the editors and the reviewers. Any product that may be evaluated in this article, or claim that may be made by its manufacturer, is not guaranteed or endorsed by the publisher.
Supplementary material
The Supplementary material for this article can be found online at: https://www.frontiersin.org/articles/10.3389/fmicb.2025.1563712/full#supplementary-material
Footnotes
References
Ameloot, N., Sleutel, S., Case, S. D. C., Alberti, G., Mcnamara, N. P., Zavalloni, C., et al. (2014). C mineralization and microbial activity in four biochar field experiments several years after incorporation. Soil Biol. Biochem. 78, 195–203. doi: 10.1016/j.soilbio.2014.08.004
An, N., Zhang, L., Liu, Y., Shen, S., Li, N., Wu, Z., et al. (2022). Biochar application with reduced chemical fertilizers improves soil pore structure and rice productivity. Chemosphere 298:134304. doi: 10.1016/j.chemosphere.2022.134304
Arenberg, M. R., and Arai, Y. (2019). Chapter three-uncertainties in soil physicochemical factors controlling phosphorus mineralization and immobilization processes. Adv. Agron. 154, 153–200. doi: 10.1016/bs.agron.2018.11.005
Arenberg, M. R., and Arai, Y. (2021). Nitrogen species specific phosphorus mineralization in temperate floodplain soils. Sci. Rep. 11:17430. doi: 10.1038/s41598-021-96885-5
Asiloglu, R., Kenya, K., Samuel, S. O., Sevilir, B., Murase, J., Suzuki, K., et al. (2021). Top-down effects of protists are greater than bottom-up effects of fertilisers on the formation of bacterial communities in a paddy field soil. Soil Biol. Biochem. 156:108186. doi: 10.1016/j.soilbio.2021.108186
Bai, Z., Li, H., Yang, X., Zhou, B., Shi, X., Wang, B., et al. (2013). The critical soil P levels for crop yield, soil fertility and environmental safety in different soil types. Plant Soil 372, 27–37. doi: 10.1007/s11104-013-1696-y
Bai, S. H., Omidvar, N., Gallart, M., Kamper, W., Tahmasbian, I., Farrar, M. B., et al. (2022). Combined effects of biochar and fertilizer applications on yield: a review and meta-analysis. Sci. Total Environ. 808:152073. doi: 10.1016/j.scitotenv.2021.152073
Bornø, M. L., Müller-Stöver, D. S., and Liu, F. (2018). Contrasting effects of biochar on phosphorus dynamics and bioavailability in different soil types. Sci. Total Environ. 627, 963–974. doi: 10.1016/j.scitotenv.2018.01.283
Ceja-Navarro, J. A., Wang, Y., Ning, D., Arellano, A., Ramanculova, L., Yuan, M. M., et al. (2021). Protist diversity and community complexity in the rhizosphere of switchgrass are dynamic as plants develop. Microbiome 9:96. doi: 10.1186/s40168-021-01042-9
Chen, X., Jiang, N., Condron, L. M., Dunfield, K. E., Chen, Z., Wang, J., et al. (2019). Soil alkaline phosphatase activity and bacterial phoD gene abundance and diversity under long-term nitrogen and manure inputs. Geoderma 349, 36–44. doi: 10.1016/j.geoderma.2019.04.039
Clough, T. J., and Condron, L. M. (2010). Biochar and the nitrogen cycle: introduction. J. Environ. Qual. 39, 1218–1223. doi: 10.2134/jeq2010.0204
Cross, A., and Sohi, S. P. (2011). The priming potential of biochar products in relation to labile carbon contents and soil organic matter status. Soil Biol. Biochem. 43, 2127–2134. doi: 10.1016/j.soilbio.2011.06.016
Dai, Z., Enders, A., Rodrigues, J. L. M., Hanley, K. L., Brookes, P. C., Xu, J., et al. (2018). Soil fungal taxonomic and functional community composition as affected by biochar properties. Soil Biol. Biochem. 126, 159–167. doi: 10.1016/j.soilbio.2018.09.001
Dai, Z., Xiong, X., Zhu, H., Xu, H., Leng, P., Li, J., et al. (2021). Association of biochar properties with changes in soil bacterial, fungal and fauna communities and nutrient cycling processes. Biochar 3, 239–254. doi: 10.1007/s42773-021-00099-x
Fadeev, E., Cardozo-Mino, M. G., Rapp, J. Z., Bienhold, C., Salter, I., Salman-Carvalho, V., et al. (2021). Comparison of two 16S rRNA primers (V3–V4 and V4–V5) for studies of Arctic microbial communities. Front. Microbiol. 12:637526. doi: 10.3389/fmicb.2021.637526
Fadrosh, D. W., Ma, B., Gajer, P., Sengamalay, N., Ott, S., Brotman, R. M., et al. (2014). An improved dual-indexing approach for multiplexed 16S rRNA gene sequencing on the Illumina MiSeq platform. Microbiome 2:6. doi: 10.1186/2049-2618-2-6
Fraser, T. D., Lynch, D. H., Bent, E., Entz, M. H., and Dunfield, K. E. (2015). Soil bacterial phoD gene abundance and expression in response to applied phosphorus and long-term management. Soil Biol. Biochem. 88, 137–147. doi: 10.1016/j.soilbio.2015.04.014
Fu, Y., Kumar, A., Chen, L., Jiang, Y., Ling, N., Wang, R., et al. (2021). Rhizosphere microbiome modulated effects of biochar on ryegrass 15N uptake and rhizodeposited 13C allocation in soil. Plant Soil 463, 359–377. doi: 10.1007/s11104-021-04845-9
Garland, G., Bünemann, E. K., Oberson, A., Frossard, E., Snapp, S., Chikowo, R., et al. (2018). Phosphorus cycling within soil aggregate fractions of a highly weathered tropical soil: a conceptual model. Soil Biol. Biochem. 116, 91–98. doi: 10.1016/j.soilbio.2017.10.007
Gul, S., and Whalen, J. K. (2016). Biochemical cycling of nitrogen and phosphorus in biochar-amended soils. Soil Biol. Biochem. 103, 1–15. doi: 10.1016/j.soilbio.2016.08.001
Huang, Y., Dai, Z., Lin, J., Li, D., Ye, H., Dahlgren, R. A., et al. (2021). Labile carbon facilitated phosphorus solubilization as regulated by bacterial and fungal communities in Zea mays. Soil Biol. Biochem. 163:108465. doi: 10.1016/j.soilbio.2021.108465
Jansson, J. K., and Hofmockel, K. S. (2018). The soil microbiome-from metagenomics to metaphenomics. Curr. Opin. Microbiol. 43, 162–168. doi: 10.1016/j.mib.2018.01.013
Jiao, S., and Lu, Y. (2020). Abundant fungi adapt to broader environmental gradients than rare fungi in agricultural fields. Glob. Change Biol. 26, 4506–4520. doi: 10.1111/gcb.15130
Jousset, A., Bienhold, C., Chatzinotas, A., Gallien, L., Gobet, A., Kurm, V., et al. (2017). Where less may be more: how the rare biosphere pulls ecosystems strings. ISME J. 11, 853–862. doi: 10.1038/ismej.2016.174
Lehmann, J., Rillig, M. C., Thies, J., Masiello, C. A., Hockaday, W. C., and Crowley, D. (2011). Biochar effects on soil biota—a review. Soil Boil. Biochem. 43, 1812–1836. doi: 10.1016/j.soilbio.2011.04.022
Li, Y., Gao, W., Wang, C., and Gao, M. (2023). Distinct distribution patterns and functional potentials of rare and abundant microorganisms between plastisphere and soils. Sci. Total Environ. 873:162413. doi: 10.1016/j.scitotenv.2023.162413
Li, F., Sun, A., Jiao, X., Bi, L., Zheng, Y., He, J.-Z., et al. (2021). Specific protistan consumers and parasites are responsive to inorganic fertilization in rhizosphere and bulk soils. J. Soils Sediments 21, 3801–3812. doi: 10.1007/s11368-021-03052-4
Liang, Y., Li, M., Pan, F., Ma, J., Yang, Z., Ling, T., et al. (2020). Alkaline phosphomonoesterase-harboring microorganisms mediate soil phosphorus transformation with stand age in Chinese Pinus massoniana plantations. Front. Microbiol. 11:571209. doi: 10.3389/fmicb.2020.571209
Liu, X., Liu, Y., Zhang, L., Yin, R., and Wu, G. (2021). Bacterial contributions of bio-crusts and litter crusts to nutrient cycling in the Mu Us Sandy Land. Catena 199:105090. doi: 10.1016/j.catena.2020.105090
Luo, Y., Lin, Q., Durenkamp, M., Dungait, A. J., and Brookes, P. C. (2017a). Soil priming effects following substrates addition to biochar-treated soils after 431 days of pre-incubation. Biol. Fertil. Soils 53, 315–326. doi: 10.1007/s00374-017-1180-6
Luo, Y., Zang, H., Yu, Z., Chen, Z., Gunina, A., Kuzyakov, Y., et al. (2017b). Priming effects in biochar enriched soils using a three-source-partitioning approach: 14C labelling and 13C natural abundance. Soil Biol. Biochem. 106, 28–35. doi: 10.1016/j.soilbio.2016.12.006
Ma, L., Niu, W., Li, G., Du, Y., Sun, J., Zhang, Q., et al. (2024). Crucial role of rare taxa in preserving bacterial community stability. Land Degrad. Dev. 35, 1397–1410. doi: 10.1002/ldr.4994
Margalef, O., Sardans, J., Fernandez-Martinez, M., Molowny-Horas, R., Janssens, I. A., Ciais, P., et al. (2017). Global patterns of phosphatase activity in natural soils. Sci. Rep. 7:1337. doi: 10.1038/s41598-017-01418-8
Nguyen, B.-A. T., Chen, Q.-L., Yan, Z.-Z., Li, C., He, J.-Z., and Hu, H.-W. (2021). Distinct factors drive the diversity and composition of protistan consumers and phototrophs in natural soil ecosystems. Soil Biol. Biochem. 160:108317. doi: 10.1016/j.soilbio.2021.108317
Parent, L. E., Parent, S.-É., and Ziadi, N. (2014). Biogeochemistry of soil inorganic and organic phosphorus: a compositional analysis with balances. J. Geochem. Explor. 141, 52–60. doi: 10.1016/j.gexplo.2014.01.030
Rachel, C. W., John, K. S., Brad, M. P., Michael, E. V., Joseph, K. B., and Jennifer, A. S. (2018). Soil fungi underlie a phylogenetic pattern in plant growth responses to nitrogen enrichment. J. Ecol. 106, 2161–2175. doi: 10.1111/1365-2745.12983
Ragot, S. A., Kertesz, M. A., and Bunemann, E. K. (2015). PhoD alkaline phosphatase gene diversity in soil. Appl. Environ. Microbiol. 81, 7281–7289. doi: 10.1128/AEM.01823-15
Rasit, A. (2022). Biochar-microbe interaction: more protist research is needed. Biochar 4:72. doi: 10.1007/s42773-022-00195-6
Rousk, J., Baath, E., Brookes, P. C., Lauber, C. L., Lozupone, C., Caporaso, J. G., et al. (2010). Soil bacterial and fungal communities across a pH gradient in an arable soil. ISME J. 4, 1340–1351. doi: 10.1038/ismej.2010.58
Rutigliano, F. A., Romano, M., Marzaioli, R., Baglivo, I., Baronti, S., Miglietta, F., et al. (2014). Effect of biochar addition on soil microbial community in a wheat crop. Eur. J. Soil Biol. 60, 9–15. doi: 10.1016/j.ejsobi.2013.10.007
Sarauer, J. L., and Coleman, M. D. (2023). Microbial communities of biochar amended forest soils in northwestern USA. Appl. Soil Ecol. 188:104875. doi: 10.1016/j.apsoil.2023.104875
Shamim Gul, J. K. W., Thomas, B. W., Sachdeva, V., and Deng, H. (2015). Physico-chemical properties and microbial responses in biochar-amended soils: mechanisms and future directions. Plant Soil 206, 46–59. doi: 10.1016/j.agee.2015.03.015
Shi, L.-L., Shen, M.-X., Lu, C.-Y., Wang, H.-H., Zhou, X.-W., Jin, M.-J., et al. (2015). Soil phosphorus dynamic, balance and critical P values in long-term fertilization experiment in Taihu Lake region, China. J. Integr. Agr. 14, 2446–2455. doi: 10.1016/s2095-3119(15)61183-2
Stoeck, T., Bass, D., Nebel, M., Christen, R., Jones, M. D., Breiner, H. W., et al. (2010). Multiple marker parallel tag environmental DNA sequencing reveals a highly complex eukaryotic community in marine anoxic water. Mol. Ecol. 19, 21–31. doi: 10.1111/j.1365-294X.2009.04480.x
Sun, A., Jiao, X. Y., Chen, Q., Trivedi, P., Li, Z., Li, F., et al. (2021). Fertilization alters protistan consumers and parasites in crop-associated microbiomes. Environ. Microbiol. 23, 2169–2183. doi: 10.1111/1462-2920.15385
Tabatabai, M. (1994). “Soil enzymes” in Methods of soil analysis. Part 2: Microbiological and biochemical properties. ed. J. M. Bigham (Madison, WI: Soil Science Society of America), 775–833.
Tian, J., Kuang, X., Tang, M., Chen, X., Huang, F., Cai, Y., et al. (2021). Biochar application under low phosphorus input promotes soil organic phosphorus mineralization by shifting bacterial phoD gene community composition. Sci. Total Environ. 779:146556. doi: 10.1016/j.scitotenv.2021.146556
Wang, C., Guo, L., and Shen, R.-F. (2023). Rare microbial communities drive ecosystem multifunctionality in acidic soils of southern China. Appl. Soil Ecol. 189:104895. doi: 10.1016/j.apsoil.2023.104895
Wang, H., Tian, D., Liu, H., Wang, Z., He, Y., Lu, J., et al. (2024). Rare rather than abundant taxa of soil bacteria and fungi regulate soil multifunctionality in Eucalyptus plantations. Catena 245:108303. doi: 10.1016/j.catena.2024.108303
Wei, X., Hu, Y., Razavi, B. S., Zhou, J., Shen, J., Nannipieri, P., et al. (2019). Rare taxa of alkaline phosphomonoesterase-harboring microorganisms mediate soil phosphorus mineralization. Soil Biol. Biochem. 131, 62–70. doi: 10.1016/j.soilbio.2018.12.025
Xiong, W., Jousset, A., Guo, S., Karlsson, I., Zhao, Q., Wu, H., et al. (2018). Soil protist communities form a dynamic hub in the soil microbiome. ISME J. 12, 634–638. doi: 10.1038/ismej.2017.171
Xiong, W., Song, Y., Yang, K., Gu, Y., Wei, Z., Kowalchuk, G. A., et al. (2020). Rhizosphere protists are key determinants of plant health. Microbiome 8:27. doi: 10.1186/s40168-020-00799-9
Xu, C.-Y., Bai, S. H., Hao, Y., Rachaputi, R. C. N., Xu, Z., and Wallace, H. M. (2015). Peanut shell biochar improves soil properties and peanut kernel quality on a red ferrosol. J. Soils Sediments 15, 2220–2231. doi: 10.1007/s11368-015-1242-z
Xu, W., Whitman, W. B., Gundale, M. J., Chien, C. C., and Chiu, C. Y. (2020). Functional response of the soil microbial community to biochar applications. Glob. Change Biol. Bioenergy 13, 269–281. doi: 10.1111/gcbb.12773
Xue, P., Minasny, B., Mcbratney, A., Jiang, Y., and Luo, Y. (2023). Land use effects on soil protists and their top-down regulation on bacteria and fungi in soil profiles. Appl. Soil Ecol. 185:104799. doi: 10.1016/j.apsoil.2022.104799
Ye, L., Camps-Arbestain, M., Shen, Q., Lehmann, J., Singh, B., Sabir, M., et al. (2019). Biochar effects on crop yields with and without fertilizer: a meta-analysis of field studies using separate controls. Soil Use Manag. 36, 2–18. doi: 10.1111/sum.12546
Yin, Y., Yang, C., Li, M., Yang, S., Tao, X., Zheng, Y., et al. (2023). Biochar reduces bioavailability of phosphorus during swine manure composting: role of phoD-harboring bacterial community. Sci. Total Environ. 858:159926. doi: 10.1016/j.scitotenv.2022.159926
Yuan, J., Wang, Y., Zhao, X., Chen, H., Chen, G., and Wang, S. (2022). Seven years of biochar amendment has a negligible effect on soil available P and a progressive effect on organic C in paddy soils. Biochar 4:1. doi: 10.1007/s42773-021-00127-w
Zama, E. F., Reid, B. J., Arp, H. P. H., Sun, G.-X., Yuan, H.-Y., and Zhu, Y.-G. (2018). Advances in research on the use of biochar in soil for remediation: a review. J. Soils Sediments 18, 2433–2450. doi: 10.1007/s11368-018-2000-9
Zhang, T., Chen, H. Y. H., and Ruan, H. (2018). Global negative effects of nitrogen deposition on soil microbes. ISME J. 12, 1817–1825. doi: 10.1038/s41396-018-0096-y
Zhang, H., Wang, S., Zhang, J., Tian, C., and Luo, S. (2021). Biochar application enhances microbial interactions in mega-aggregates of farmland black soil. Soil Till. Res. 213:105145. doi: 10.1016/j.still.2021.105145
Zhang, T., Wang, J., Zhou, S., Chen, Y., and Li, D. (2023). Spatio-temporal dynamic diversity of bacterial alkaline phosphatase phoD gene and its environmental drivers in sediments during algal blooms: a case study of shallow Lake Taihu. J. Environ. Manag. 336:117595. doi: 10.1016/j.jenvman.2023.117595
Zhang, S., Zhang, H., Liu, H., Wang, H., Xiu, W., Li, G., et al. (2022). Fertilization drives distinct biotic and abiotic factors in regulating functional groups of protists in a 5-year fertilization system. Front. Microbiol. 13:1036362. doi: 10.3389/fmicb.2022.1036362
Keywords: biochar, rare taxa, phoD-harboring microorganism, protist, fungi, wheat yield
Citation: Qi J-Q, Yuan H-Y, Sun S-C, Zama EF, Tao B-X, Liu J and Zhang B-H (2025) Biochar amendment alters rare microbial taxa and enhances wheat growth in alkaline farmland: insights into soil microbiome dynamics. Front. Microbiol. 16:1563712. doi: 10.3389/fmicb.2025.1563712
Edited by:
Ahmed Abdelhafez, The New Valley University, EgyptReviewed by:
Mohamed A. M. Atia, Agricultural Research Center (Egypt), EgyptEsko Salo, VTT Technical Research Centre of Finland Ltd., Finland
Copyright © 2025 Qi, Yuan, Sun, Zama, Tao, Liu and Zhang. This is an open-access article distributed under the terms of the Creative Commons Attribution License (CC BY). The use, distribution or reproduction in other forums is permitted, provided the original author(s) and the copyright owner(s) are credited and that the original publication in this journal is cited, in accordance with accepted academic practice. No use, distribution or reproduction is permitted which does not comply with these terms.
*Correspondence: Hai-Yan Yuan, MTg4MTA2NDExOTJAMTM5LmNvbQ==; Jin Liu, bGl1amluNzI5NEAxNjMuY29t