- 1UniLaSalle, AGHYLE, SFR NORVEGE FED, Mont-Saint-Aignan, France
- 2Biom InnoV, Saint-Malo, France
- 3Gaïago SAS, Saint-Malo, France
- 4Department of Biology, University of Fribourg, Fribourg, Switzerland
- 5Plant Production Systems, Agroscope, Nyon, Switzerland
Late blight, caused by Phytophthora infestans, is among the most destructive diseases affecting tomatoes and potatoes. The use of synthetic fungicides is becoming increasingly restricted due to the banning of several active ingredients for environmental and health reasons. Moreover, the rise of fungicide-resistant strains is compromising their effectiveness. Solutions for sustainable crop protection are thus urgently needed. Biocontrol products based on plant extracts appear to be a promising solution. This study aimed to evaluate in vitro inhibitory potential of a plant extract-based biocontrol product on the different stages of P. infestans lifecycle, including mycelial development and, formation and germination of infection structures (sporangia and zoospores). Non-target effects were also assessed using four fungi, three of which were isolated from the phyllosphere, and two ubiquitous bacteria. For this purpose, the formulated product (FV) and the plant extract at different concentrations (PE and CPE) were tested through bioassays. The results show that the mycelial growth of Phytophthora infestans was completely inhibited by the FV and less affected by the CPE. Infection structures were more sensitive to PE than mycelia, although FV was consistently the most effective inhibitor. Interestingly, at non-inhibitory doses, zoospore germination exhibited disturbances, such as an increase in abnormal germination phenotypes. Overall, PE showed significant inhibitory potential against the oomycete. FV exhibited a strong impact on mycelium, sporangia, and zoospores at very low concentrations (0.01–0.05%), suggesting an optimized inhibitory effect of PE. Non-target effects of FV on fungal and bacterial growth were observed only at concentrations substantially higher than those required to inhibit P. infestans in vitro. This study highlights the strong efficacy of the plant extract-based biocontrol product against the target oomycete, with minimal impact on non-target microorganisms. These findings support its potential as a promising anti-Phytophthora agent within integrated late blight management strategies.
1 Introduction
Phytophthora infestans (Mont.) de Bary, the oomycete responsible for late blight, is historically infamous for triggering the Irish potato famine of 1845. This pathogen continues to inflict significant economic losses on potato and tomato production worldwide, contributing annually to substantial yield reductions (Nowicki et al., 2012). The aggressiveness of P. infestans is partly attributed to its rapid asexual reproductive cycle, which relies on the production of two types of spores. At higher temperatures, airborne sporangia can directly germinate on the leaf surface, or they can, at lower temperatures, produce and release motile zoospores. Both processes lead to host tissue penetration, mycelial development, and sporangiophore production, enabling further infection cycles (Fry et al., 2015). Thus, due to these dual infection mechanisms in P. infestans, its effective control has become challenging.
The common method for controlling P. infestans in potato and tomato crops is synthetic fungicide application. However, repeated use of these chemicals is expensive and has led to the emergence of resistant strains (Fry, 2008). In recent years, fluazinam and mandipropamid resistant strains have emerged in Europe (Schepers et al., 2018; Abuley et al., 2023). Moreover, the non-target effects of fungicides pose a significant risk of altering the structure of microbial communities (Yang et al., 2011; Dela Cruz et al., 2022). To reduce their risks to environmental and human health, the European Commission has increasingly restricted the use of authorized active substances (Haverkort et al., 2008; Marchand, 2023). As a result, environmentally sustainable protection methods are urgently needed to provide effective alternatives for farmers. Among these, biocontrol strategies are increasingly recognized as viable solutions to reduce fungicide usage (Van Lenteren et al., 2018).
Biocontrol products, including microorganisms and natural substances, have shown significant promise for combating late blight and for being incorporated into pest management programs (Hashemi et al., 2022; Wang and Long, 2023). Potential candidates for biocontrol products include either the use of fungi and bacteria or their active ingredients or metabolites, mainly belonging to the genera Trichoderma (Yao et al., 2016; Alfiky et al., 2024), and, Bacillus and Pseudomonas, respectively (Hunziker et al., 2015; Caulier et al., 2018; De Vrieze et al., 2018, 2019; Wang et al., 2020a; Wang et al., 2020b). Plants represent an abundant source of biocontrol products due to their rich antimicrobial compounds, including terpenes, phenolic compounds, phytoalexins, alkaloids and flavonoids. These molecules can affect various microbial structures such as the cell wall, plasma membrane, mitochondria, and disturb pathways involved in DNA and protein synthesis (Cenobio-Galindo et al., 2024). Numerous studies have validated the efficacy of various plant extracts in vitro and in greenhouse experiments against late blight (Dorn et al., 2007; Forrer et al., 2017; La Torre et al., 2019; Najdabbasi et al., 2020). Their efficacy was evidenced by the direct effects of some plant extracts on mycelial growth, sporangia germination, and zoospore release. However, these studies rarely investigated the impact of plant extracts on zoospore germination, a key process in leaf infection (Fry, 2008). Like synthetic fungicides, biocontrol products may also exhibit non-target effects. For example, Teixeira et al. (2023) reported the inhibitory potential of garlic skin extract against phytopathogenic fungi and oomycetes. Additionally, Adamczyk et al. (2023) showed that spruce resin, containing terpenes, exhibits broad-spectrum inhibition affecting both oomycetes, among which Phytophthora species and various fungal species. Moreover, the formulation of biocontrol products is an essential process in optimizing the efficacy of active substances (Janisiewicz and Korsten, 2002; Hernandez-Tenorio et al., 2022). Incorporating co-formulants, such as surfactants, may enhance the effectiveness of plant- and algae-based extracts for controlling phytopathogens, both in vitro and in planta (Paris et al., 2016; Rashid et al., 2022).
The aim of the present study was to evaluate in vitro effects of a plant extract-based biocontrol product on P. infestans. Initially, both the plant extract at different concentrations (PE and CPE) and its formulated version (FV) were tested for their direct effects on mycelial growth, sporangial germination, zoospore release and germination. To understand the mode of action of this biocontrol product, experiments were conducted to assess membrane integrity within infection structures following treatment.
Finally, since this product is intended for foliar application, the potential non-target effects of both biocontrol versions were evaluated on microorganism’s representative of the plant-associated microbial communities and isolated from the tomato and maize phyllosphere in the laboratory. These included three fungal isolates from the phyllosphere and one phytopathogenic fungus, with the impact on the mycelial growth assessed. Fusarium sp. and Cladosporium sp. (Ascomycota) were selected due to their widespread presence in plant canopies and soil, as well as their diverse lifestyles, ranging from pathogenic to commensal and saprophytic species (Bensch et al., 2012; Nikitin et al., 2023). Mucor sp., (Mucoromycota) was included as a representative saprophytic fungus commonly found in soil and decaying plant material (Hoffmann et al., 2013; Pawłowska et al., 2019). The plant pathogen Sclerotinia sclerotiorum, responsible for white mold on various crops, was chosen for its agronomic importance and known interactions with biocontrol agents (Bolton et al., 2006). Additionally, two bacterial species, Bacillus subtilis and Pseudomonas fluorescens were included due to their widespread distribution in plant-associated microbiota and their roles in plant health and disease suppression (Haas and Défago, 2005; Earl et al., 2008). This selection ensures a relevant assessment of potential non-target effects on ecologically and functionally diverse microorganisms that are likely to interact with foliar biocontrol treatments.
2 Materials and methods
2.1 Phytophthora infestans and culture media
The Phytophthora infestans (oomycete) 36_A2 strain was obtained from inov3PT (Achicourt, France) and maintained in the dark at 15°C on agar plates supplemented with 10% V8 tomato juice and 0.1% (w/v) calcium carbonate. For the different tests, only plates with actively growing mycelium were used. Sporangia were collected by adding approximately 4 mL of sterile distilled water to the mycelial cultures. The mycelium was collected by scraping the plate surface with a sterile rake and transferring into a tube. To detach the sporangia from the mycelium, the suspension was shaken vigorously (but not vortexed) and then filtered through a 70 μm sieve to harvest only the sporangia. The sporangial concentration was determined using a Malassez cell and adjusted to 3 × 104 sporangia.mL−1. Zoospores were collected by inducing a cold shock. Briefly, 10 mL of sterile ice-cold distilled water was added to a P. infestans culture. To isolate the zoospores, 8 mL was pipetted from the surface of the suspension and transferred to a Falcon tube. The concentration was adjusted at 7 × 104 zoospore.mL−1 using a Malassez cell.
2.2 Fungi and bacterial strains and culture media
Sclerotinia sclerotiorum 1980 UF-70, kindly provided by Dr. Sylvain Raffaele (INRAE-CNRS, France), was cultured on Potato Dextrose Agar (PDA, Condalab) in the dark at 20°C. Three fungal strains from the laboratory strain library were also used in this study: Fusarium sp. and Mucor sp. (both isolated from the maize phyllosphere), and Cladosporium sp. (isolated from tomatoes phyllosphere). The Fusarium strain was grown on PDA, while Mucor and Cladosporium strains were cultivated on Sabouraud Dextrose Agar (SDA, Condalab). All fungal cultures were maintained at 20°C in the dark. Pseudomonas fluorescens CIP 104605 and Bacillus subtilis CIP 52.65 T, obtained from the Pasteur Institute (Paris, France) were cultured at 28°C in the dark on Luria-Bertani (LB) agar (1.5%) and Tryptic Soy Agar (TSA), respectively.
2.3 Biocontrol product
Two versions of the biocontrol product were tested: i. Plant extract at 2.50% (v/v) and at 10% (v/v), referred to as plant extract (PE) and concentrated plant extract (CPE), respectively; and ii. A formulated version (FV) consisting of a 2.50% plant extract mixed with co-formulants. The co-formulants included a preservative to maintain the integrity of the plant extract, a sticker to ensure adherence to plant surfaces, a surfactant to enhance application quality and penetration, and trace elements that provide additional nutritional benefits to the plant. This product was provided by Biom InnoV (Saint-Malo, France) and the specific nature of the plant extract and formulation composition are confidential (Patent n° WO 2024/023446 A1).
2.4 Effect on microorganism’s growth
The effect of biocontrol products on the growth of oomycetes, fungi, and bacteria was studied using appropriate agar culture media in Petri dishes. Sterilized culture medium, either supplemented with the biocontrol product or untreated (control), was poured into Petri dishes. To evaluate the effect of the biocontrol product on the mycelial growth of the oomycete and the fungi, a plug taken from the edge of an actively growing mycelium culture was placed at the center of the Petri dish containing the biocontrol product (FV, PE, or CPE at different doses) or a control. The tested concentrations ranged from 0.01 to 2.50% (v/v). Petri dishes were incubated in the dark at 20°C for P. infestans, Mucor sp., Fusarium sp., and Cladosporium sp., and at 24°C for S. sclerotiorum. Pictures were taken at two time points, depending on the growth speed of the mycelium in control plates. The first time point (early) was defined as when the mycelium covered half the Petri dish, and the second (late) was when the mycelium fully covered the entire Petri dish in the control condition. It is important to note that not all tested microorganisms have the same growth rate such as Cladosporium sp., (slow growth) and S. sclerotiorum (fast growth). The time points were as follows: 7 and 14 days for P. infestans, Fusarium sp., and Cladosporium sp.; 3 and 7 days for Mucor sp.; and 2 and 4 days for S. sclerotiorum.
The mycelial surface area was measured using ImageJ software and expressed as a percentage of mycelial growth, with the control condition sets at 100% for each time point using the following formula:
At least two independent experiments were conducted, each with a minimum of five technical replicates.
2.5 Effect on bacterial growth
To assess the effect of the biocontrol product on bacterial growth, cultures of P. fluorescens and B. subtilis were grown overnight on appropriate agar media. The bacterial cultures were then resuspended in sterile physiological water (pH 7.2–7.4) to achieve an optical density (OD) of 0.5 at 600 nm for B. subtilis and an OD of 0.8 at 580 nm for P. fluorescens (Picot et al., 2001; Massabò et al., 2018). Serial dilutions were performed, and 100 μL of each dilution was spread onto the surface of agar medium, either supplemented with the biocontrol product or untreated (control). Plates were incubated at 28°C in the dark. Colony counts were performed after 24 h for B. subtilis and after 48 h for P. fluorescens. Only plates with colony counts between 15 and 300 were considered for analysis. The colony-forming unit (CFU) concentration for each plate was calculated using the following formula:
The experiment was repeated independently three times, each including three technical replicates.
2.6 Effect on infection structures of Phytophthora infestans
The effects of the biocontrol product on sporangia and zoospores were studied using 96-well plates. The wells contained 50 μL of sporangia or zoospore suspension, to which 50 μL of diluted FV, PE or CPE were added, resulting in final concentrations ranging from 0.01 to 1.00% (v/v). Control wells contained 50 μL of sterile water and 50 μL of sporangia or zoospores suspension.
Sporangia and zoospore germination were assessed as described by De Vrieze et al. (2020), with modifications. For sporangia germination, the plates were incubated in the dark at 20°C for 24 h. Images per well were captured at 10x magnification using an inverted epifluorescence microscope (EVOS M5000, Invitrogen, Thermo Fisher Scientific). On average, 180 sporangia per well were counted and classified as germinated or non-germinated. For zoospore germination, plates were incubated in the dark at 18°C for 4 h. Images per well were captured at 10x magnification, and an average of 210 zoospores per well were counted and classified by phenotype. The experiment was repeated three times, with three technical replicates per experiment.
The effect of the biocontrol product on zoospore release was studied following the procedure described by Alfiky et al. (2024), with modifications. Plates containing the diluted FV and PE were pre-cooled before the addition of 50 μL of sporangia suspension to each well. To induce zoospore release, the plates were incubated at 4°C for 2 h and followed by incubation at room temperature for 45 min. Images were captured at 4x magnification using a Cytation 5 plate reader (Biotek, United States). Approximately 120 sporangia per well were counted, and the proportions of empty and full sporangia were calculated to measure zoospore release. The experiment was performed three times, with three technical replicates per experiment.
Sporangia and zoospore viability were determined as described by Joller et al. (2020), with modifications. After 4 h of incubation for sporangia or 30 min for zoospore at 20°C in the 96-well plates containing the biocontrol product or controls, propidium iodide was added to a final concentration of 20 μg.mL−1. Propidium iodide is a red fluorescent dye that binds to DNA only when membrane integrity is compromised. Images per well were captured using an inverted epifluorescence microscope (λex = 542/20 nm, λem = 593/40 nm, EVOS M5000, Invitrogen, Thermo Fisher Scientific). On average, 140 sporangia and 190 zoospores per well (stained or not) were counted. The experiment was repeated three times for sporangia (with three technical replicates) and four times for zoospores (with two technical replicates). The brightness and contrast of the fluorescent channel photos were adjusted to the same level in order to better visualize the stained sporangia and zoospores. Image analysis of all infection structures was performed using ImageJ software.
2.7 Data analysis
After checking data normality and homoscedasticity, a Kruskal-Wallis test was performed, followed by a Wilcoxon-Mann–Whitney multiple comparison test between treatments with a Bonferroni correction. Statistical significance was accepted when the p ≤ 0.05. All statistical analyses were conducted using R studio software (2024.04.2).
3 Results
3.1 Impact on Phytophthora infestans mycelial growth
To investigate the impact of plant extract versions (PE and CPE) and the formulated version (FV) as potential biocontrol agents against P. infestans, various doses of PE, CPE, and FV were added to the culture media. Mycelial growth was measured 7- and 14-days post-inoculation (dpi). PE exhibited no inhibitory effect at concentrations up to 2.50% (Supplementary Figures S1A–D). CPE, which is four times more concentrated than PE, showed no effect at concentrations ranging from 0.01 to 0.50% (Supplementary Figures S1E,F). However, at concentrations from 0.80 to 2.50% of CPE, a reduction in growth was observed (Figures 1A,B). At 7 dpi a dose-dependent effect on mycelial growth was observed, with a 30% decrease at lower concentrations and a maximum reduction of 67% at 2.50% (Figure 1A). At 14 dpi, mycelial growth was not significantly inhibited at 0.80 and 1.00%. A significant reduction was maintained only at concentrations above 1.50%, with a maximum decrease of 46% observed at 2.50% dose (Figure 1B). For FV, mycelial growth was reduced by 88% at 7 dpi at the three lowest concentrations (0.01 to 0.10%) (Figure 1C). At the highest dose (0.50%), the reduction was slightly less pronounced but still significant compared to the control, with an 80% reduction (Figure 1C). The same pattern of inhibition was observed at 14 dpi, with a clear difference between the highest and the other doses (Figure 1D). Mycelial growth decreased by 95% at 0.01% and 93% at 0.25%, while at 0.50%, the reduction was 79%. Interestingly, P. infestans grew slightly at the highest dose, however the mycelium appeared abnormally gray compared to the control (Figure 1D). In summary, high doses of CPE affected P. infestans mycelial growth while FV showed a stronger and more consistent effects at low doses.
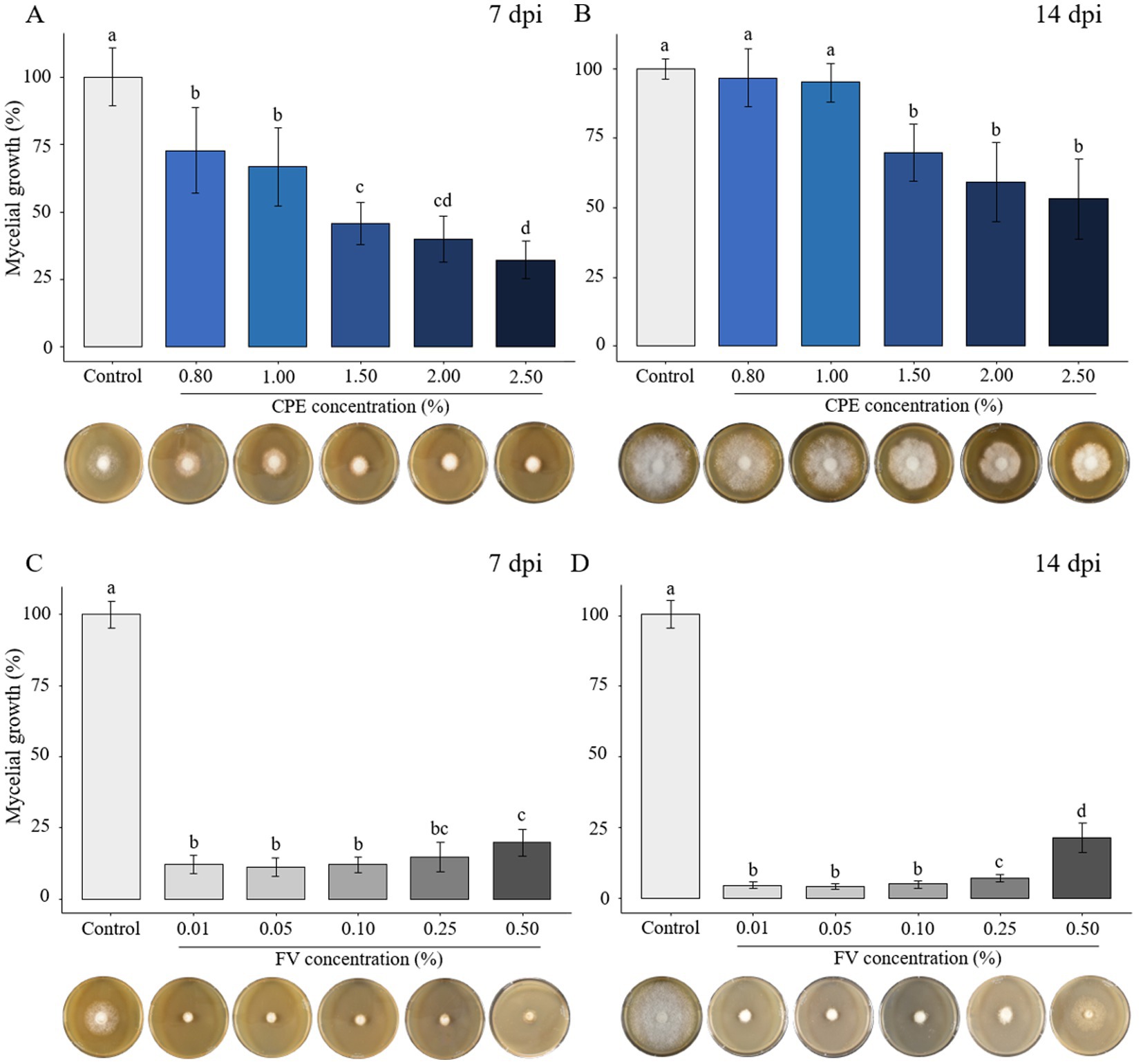
Figure 1. Effect of concentrated plant extract (CPE) and formulated version (FV) at different concentrations on Phytophthora infestans mycelial growth at early time (7 days post inoculation - dpi) and late time (14 dpi) (A,B and C,D, respectively). The percentage of growth was calculated by setting mycelial growth in control at 100%. The bars represent the average percentage growth with standard errors from 12 replicates (pooled from 3 independent experiments, each with four replicates). Representative pictures by time and dose are displayed below each graph. Letters indicate significant differences between treatments (Kruskal-Wallis test, and Wilcoxon rank-sum test for multiple comparisons, p ≤ 0.05, n = 12).
3.2 Phytophthora infestans infection structures impacted by plant extract and formulated version
3.2.1 Effect on sporangia germination
The sensitivity of sporangia germination was assessed after 24 h of incubation. Low doses of PE (0.01 and 0.05%) showed no impact on sporangia germination (Figures 2A,B). A significant effect was observed starting at 0.10%, though with high variability in response. At the highest concentrations (0.25 and 0.50%), sporangia germination was severely affected, with only 2% being able to germinate (Figures 2A,B). In contrast, FV strongly inhibited sporangia germination at all tested concentrations. At the lowest dose (0.01%), only 2% of sporangia germinated, compared to 40% in the control (Figure 2C). Complete inhibition was observed at 0.25 and 0.50% (Figures 2C,D). In conclusion, sporangia were sensitive to PE, particularly at high concentrations, where sporangia germination was significantly inhibited. Moreover, across all tested doses, FV completely inhibited the germination process.
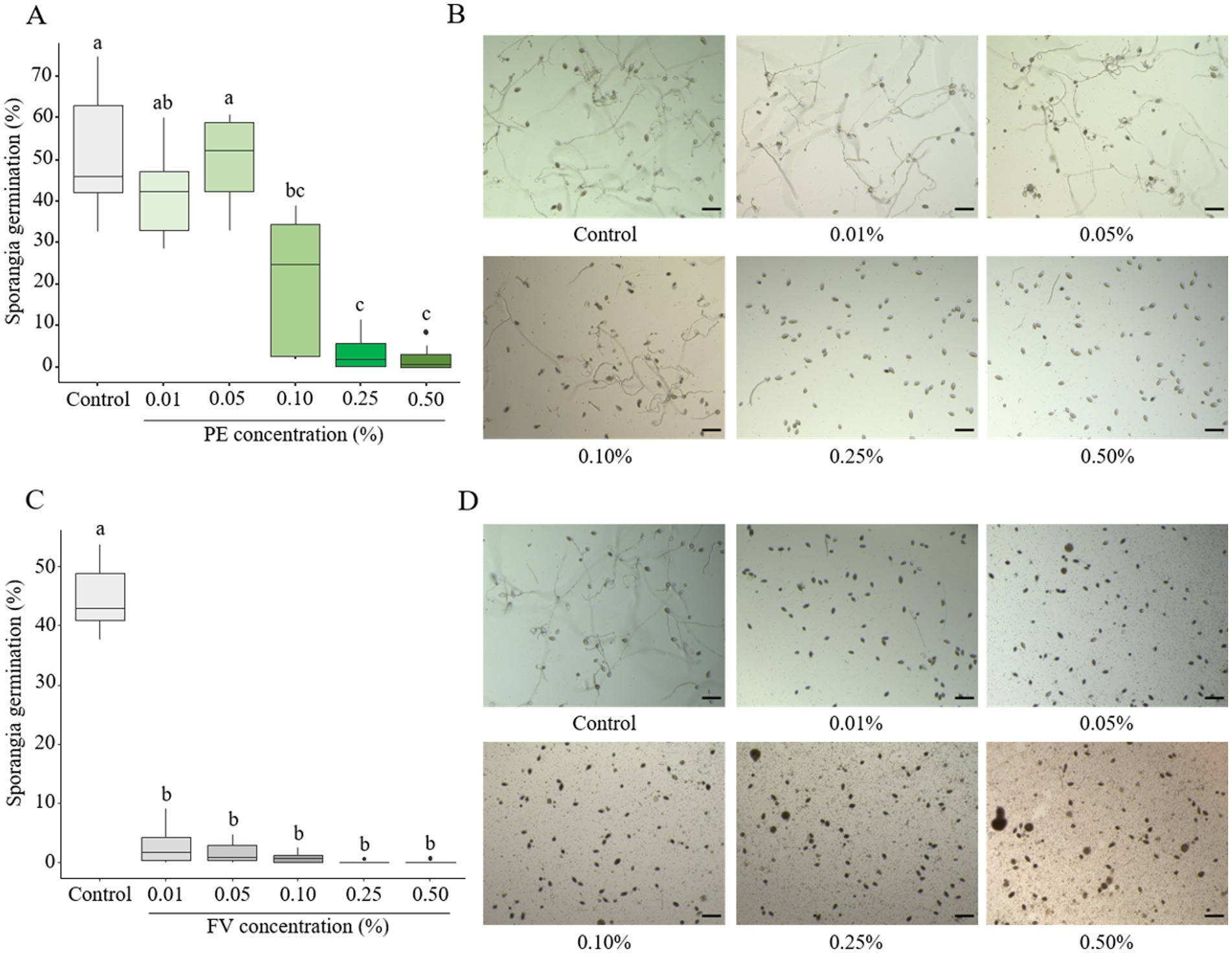
Figure 2. Impact of plant extract (PE) and formulated version (FV) at different concentrations on Phytophthora infestans sporangia germination after 24 h of incubation (A,B and C,D, respectively). Thick lines in boxplots indicate the median of 9 replicates (pooled from three independent experiments, each with three replicates). Letters indicate significant differences between treatments (Kruskal-Wallis test, and Wilcoxon rank-sum test for multiple comparisons, p ≤ 0.05, n = 9). Representative pictures of P. infestans sporangia in the presence of PE (B) or FV (D) at different doses. Pictures were captured using an inverted epifluorescence microscope (EVOS M5000, Invitrogen Thermo Fisher Scientific) at 10x magnification. Scale bars indicate 100 μm.
3.2.2 Effect on zoospore release
The impact of PE and FV on zoospore release was evaluated at the same concentrations as in the previous experiments. Empty sporangia (indicated by a black arrow in Figures 3B,D) were identified by the absence of cytoplasmic content and a clearly visible opening at one end. In contrast, full sporangia (indicated by a red arrow, Figures 3B,D) were characterized by retained cytoplasm and a closed sporangium. PE had no impact on zoospore release up to a concentration of 0.05% (Figure 3A). However, at 0.10%, only 9.5% of sporangia were empty. This reduction dropped further to 5% at the highest concentration (0.50%). In contrast, FV exhibited a stronger inhibitory effect. At the lowest dose (0.01%), only 7% of sporangia were empty compared to 68% in the control (Figure 3C). At the highest dose (0.50%), zoospore release was nearly completely inhibited, with only 2% of empty sporangia. In summary, FV affected zoospore release more than PE, with a threshold of 0.01% for FV compared to 0.10% for PE. However, the inhibitory effects of the two versions were comparable at concentrations of 0.25% and higher.
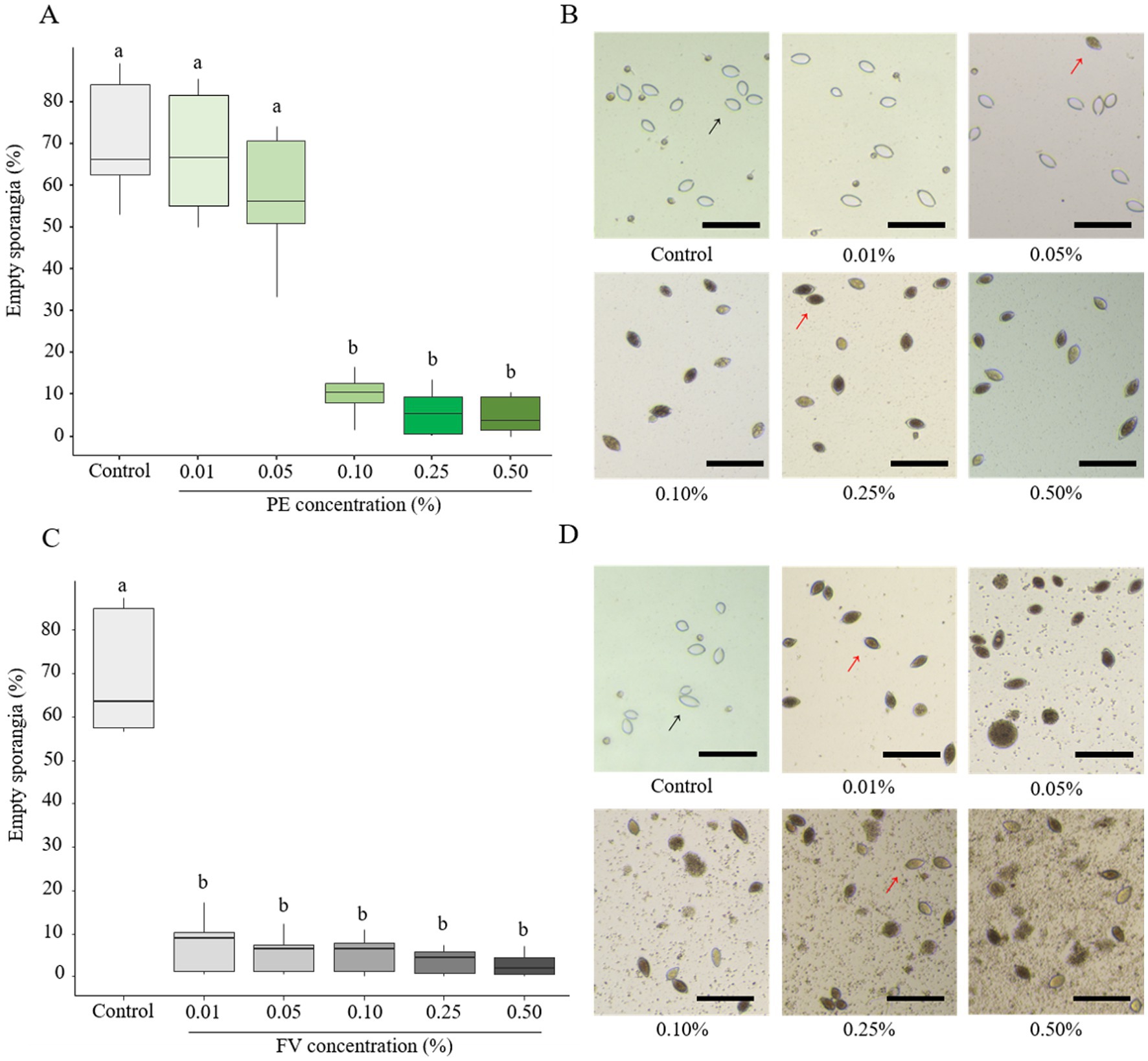
Figure 3. Influence of plant extract (PE) and formulated version (FV) at different concentrations on zoospore release (A,B and C,D, respectively). Thick lines in boxplots indicate the median of 9 replicates (pooled from three independent experiments, each with three replicates). Letters indicate significant differences between treatments (Kruskal-Wallis test, and Wilcoxon rank-sum test for multiple comparisons, p ≤ 0.05, n = 9). Representative pictures of sporangia in the presence of PE (B) or FV (D) at different doses. Pictures were captured using a Cytation 5 plate reader (Biotek, United States) at 4x magnification. Black arrows represent empty sporangia and red arrows full sporangia. Scale bars indicate 100 μm.
3.2.3 Effect on zoospore germination
The impact of FV and PE on zoospore germination was assessed, revealing significant alterations in both germination rates and phenotypes. Under control conditions, zoospores displayed diverse germination phenotypes, predominantly germination without appressorium formation and germination with short germ tubes with appressorium formation (Figures 4A–E). In contact with PE, total germination rates remained unaffected for the two lowest concentrations (0.01 and 0.05%; Figure 4A). However, at 0.05%, a higher proportion of zoospores with multiple germ tubes and appressorium-like formations were observed (Figures 4A,B). At 0.10%, PE reduced the germination rate by almost half compared to the control. At this concentration, zoospores exhibited the highest proportions of zoospores with short germ tubes without appressorium formation and germ tubes with appressorium-like formation. Moreover, the proportion of zoospores exhibiting short germ tubes with appressorium formation decreased sharply. At higher doses (0.25 and 0.50%), germination was almost completely inhibited, and only short germ tubes without appressoria were observed. In contrast, FV strongly affected the repartition of zoospore germination phenotypes at 0.01%. At this dose, despite the overall germination rate remaining similar to the control, zoospores developed the highest proportions of zoospores with multiple germ tubes and appressorium-like formation (Figures 4C,D). At 0.01%, the predominant germination phenotype observed in the control, characterized by a short germ tube with appressorium formation, was almost absent. At 0.05%, FV significantly reduced zoospore germination, with near-total inhibition observed at 0.50% (Figure 4C). The dominant phenotype at higher FV doses consisted of zoospores with very short germ tubes lacking appressorium formation, similar to the effects observed at the highest doses of PE. Overall, FV had a stronger effect than PE, affecting both germination rates and phenotypes at lower concentrations, while exhibiting similar inhibition outcomes at higher doses.
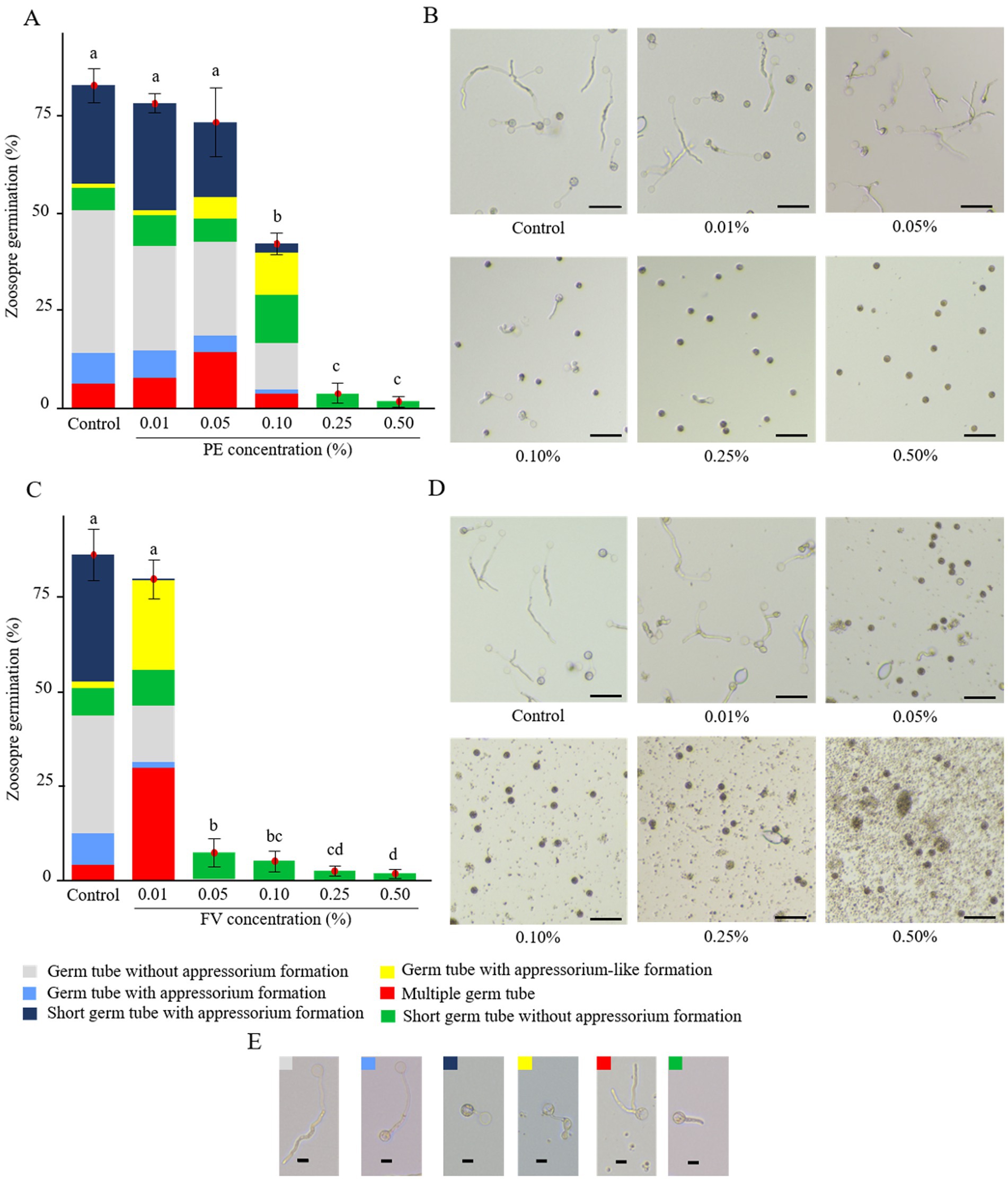
Figure 4. Impact of plant extract (PE) and formulated version (FV) at different concentrations on zoospores germination after 4 h of incubation (A,B and C,D, respectively). (A,C) The bars represent the average percentage of zoospore germination with standard errors of 9 replicates (pooled from three independent experiments, each with three replicates). Letters indicate significant differences between treatments (Kruskal-Wallis test, and Wilcoxon rank-sum test for multiple comparisons, p ≤ 0.05, n = 9). The colored bars represent the average frequency of the different germination phenotypes observed. (B,D) Representative pictures of zoospores in the presence of PE (B) or FV (D) at different doses and zoospores germination phenotypes (E). Pictures were captured using an inverted epifluorescence microscope (EVOS M5000, Invitrogen Thermo Fisher Scientific) at 10x magnification. Scale bars indicate 50 μm (B,D) and 10 μm (E).
3.2.4 Effect on sporangia membrane integrity
To characterize the direct effect of PE, CPE and FV on membrane integrity of P. infestans, sporangia were assessed using propidium iodide (PI) staining. PE at concentrations up to 0.25% did not induce observable membrane disruptions in sporangia (Figure 5A; Supplementary Figure S2). However, at 0.50%, PE increased the proportion of PI-labeled sporangia to 6.5%, rising to 11% at 1.00%. A similar trend for CPE at doses ranging from 0.01 to 0.25% was observed (Figure 5B; Supplementary Figure S2), though its impact on sporangial membrane integrity was more pronounced. At 0.50%, around 15% of sporangia were PI-labeled, increasing to 27% at 1.00%. Due to previous results indicating significant effects starting at 0.01% of FV, only the three lowest concentrations of FV were tested. A dose-dependent effect of FV on the proportion of stained sporangia was evident (Figure 5C; Supplementary Figure S2). At 0.01%, no significant impact on sporangia membrane integrity was observed, but at 0.05 and 0.10%, the proportion of stained sporangia increased to over 56 and 80% respectively, suggesting substantial membrane disruption at these concentrations. In conclusion, both PE and CPE exhibited a dose-dependent effect on sporangial membrane integrity at high concentrations (> 0.50%). However, FV had a much stronger impact, significantly compromising membrane integrity at lower doses (< 0.10%).
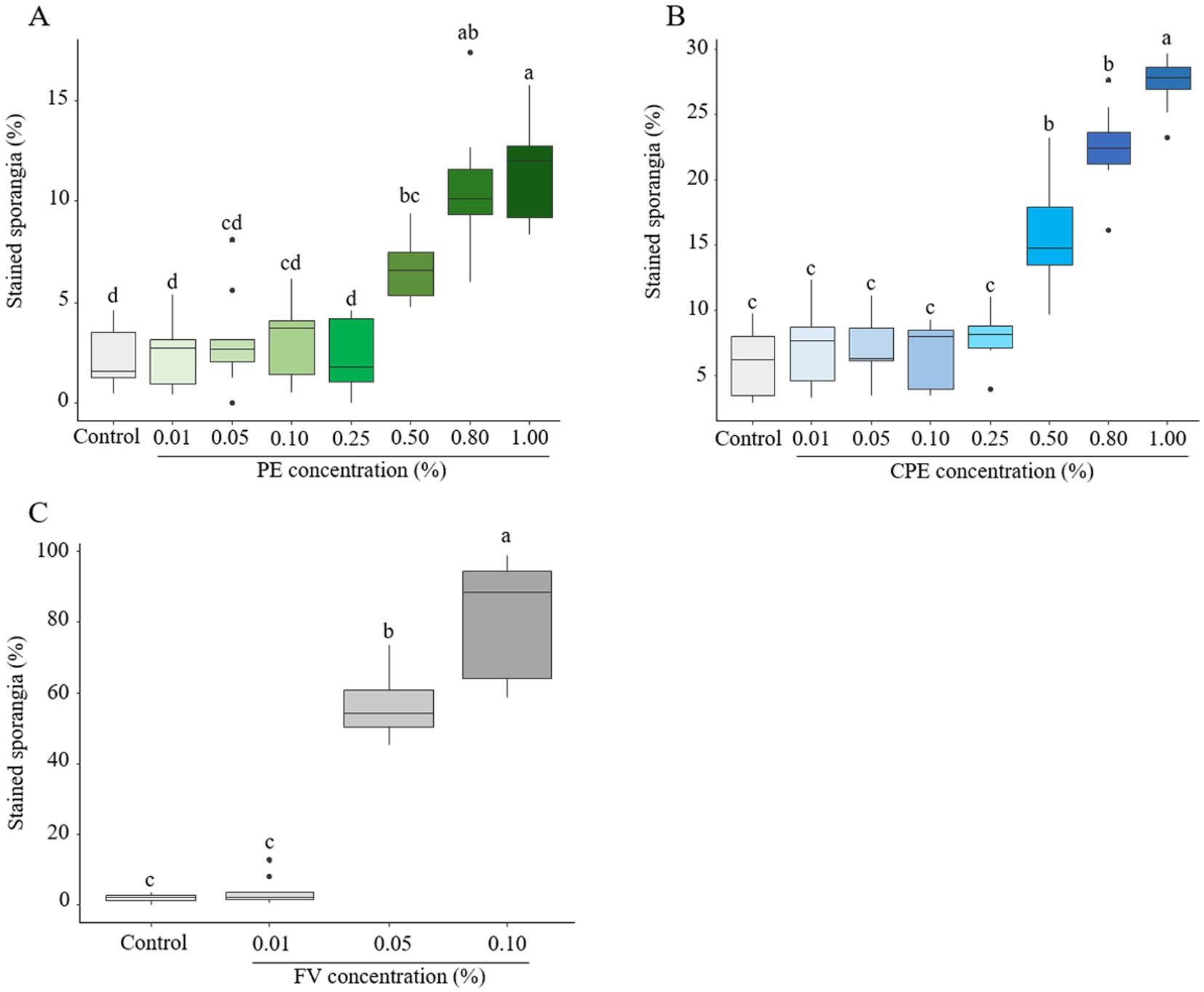
Figure 5. Effect of plant extract (PE), concentrated plant extract (CPE) and formulated version (FV) at different concentrations on the membrane integrity of sporangia after 4 h of incubation, followed by staining with propidium iodide at 20 μg/mL (A,B and C respectively). Thick lines in boxplots indicate the median of 9 replicates (pooled from three independent experiments, each with three replicates). Letters indicate significant differences between treatments (Kruskal-Wallis test, and Wilcoxon rank-sum test for multiple comparisons, p ≤ 0.05, n = 9).
3.2.5 Effect on zoospore membrane integrity
In contrast to sporangia, zoospores exhibited marked sensitivity to PE, even at low concentrations (Figure 6A). A clear dose-dependent effect was observed, with intermediate effects appearing at 0.10%, where more than 50% of zoospores were stained. At PE doses above 0.25%, nearly all zoospores exhibited PI staining, indicating severe membrane disruption (Figures 6A,C). Given these findings, further testing with CPE was considered unnecessary. On the other hand, FV induced a slight but significant impact on zoospore membrane integrity at concentrations as low as 0.01% (Figure 6B). At 0.05%, the effect became pronounced, with 74% of zoospores stained. This effect reached a plateau at 0.10%, where nearly all zoospores displayed compromised membrane integrity (Figures 6B,D). In summary, both PE and FV strongly affected zoospore membrane integrity. Zoospores demonstrated a higher sensitivity to FV compared to PE, especially at the lowest tested concentrations.
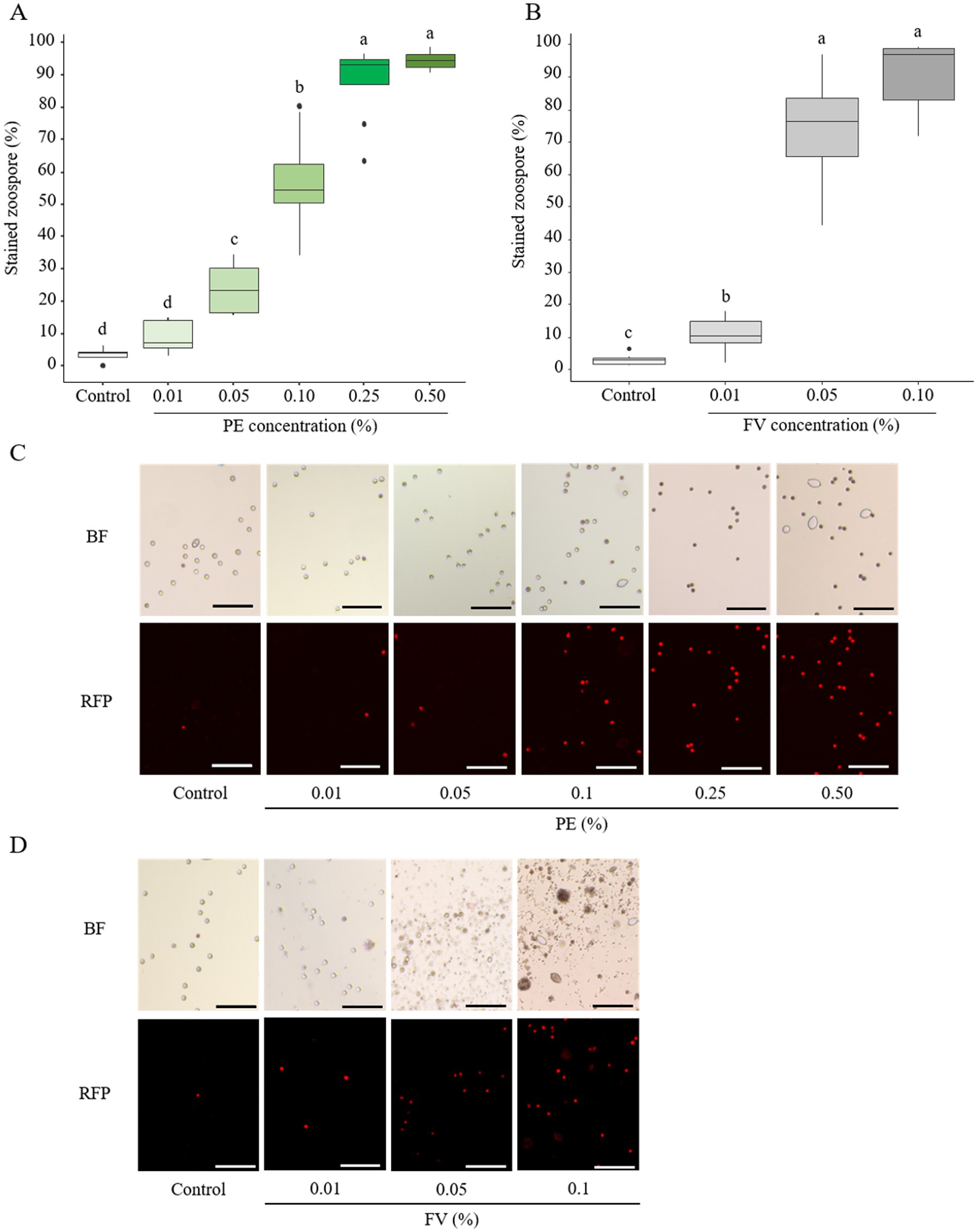
Figure 6. Effect of plant extract (PE) and formulated version (FV) at different concentrations on the membrane integrity of zoospores after 20–30 min of incubation, then stained with propidium iodide at 20 μg/mL (A,C and B,D respectively). Thick lines in boxplots indicate the median of 8 replicates (pooled from 4 independent experiments, each with 2 replicates). Letters indicate significant differences between treatments (Kruskal-Wallis test, and Wilcoxon rank-sum test for multiple comparisons, p ≤ 0.05, n = 8). Representative pictures of zoospores in the presence of PE (C) or FV (D) at different doses. Pictures were captured in bright field (BF) and red fluorescent protein (RFP) channel at 10x magnification using an inverted epifluorescence microscope (EVOS M5000, Invitrogen Thermo Fisher Scientific). Scale bars indicate 100 μm.
3.3 The formulated version and the plant extract affected fungal mycelial growth differently
To evaluate potential non-target effects of FV and PE, the mycelial growth of several fungal species was examined. Three fungi isolated from the phyllosphere: Mucor sp., Cladosporium sp., and Fusarium sp. were selected to assess possible impacts on microorganisms associated with leaf surfaces. Additionally, Sclerotinia sclerotiorum, a fungal pathogen that shares the same host plant as P. infestans, was included to provide a comparative analysis with the oomycete. To better assess its potential impact on non-target microorganisms, FV was also tested at an additional higher dose (2.00%). The activity profile of PE on mycelial growth of the tested fungi was distinct. Throughout both observation periods, no significant impact of PE was observed at concentrations up to 0.50% for S. sclerotiorum and Fusarium sp. (Supplementary Table S1). Cladosporium sp. showed a slight growth reduction at 0.50%, but only during the first observation period (Supplementary Table S1). Subsequently, a higher concentration range of PE (0.80 to 2.5%) was tested on these three fungi. After short-term exposure, Mucor sp. exhibited sensitivity to PE at 0.25 and 0.50%, with growth reductions of 29 and 53%, respectively, compared to the control (Table 1). A dose-dependent effect was observed in Cladosporium sp., Fusarium sp., and S. sclerotiorum, with initial sensitivity detected at 0.80%, resulting in growth reductions of 28, 18, and 24%, respectively (Table 1). A higher concentration was tested on Mucor sp. for comparison with the other fungi. At the high dose of 2.00%, PE caused the most pronounced growth inhibition in S. sclerotiorum (80%), while Fusarium sp. was the least affected, with a reduction of 42% (Table 1). After long-term exposure, the initial inhibition observed at 0.80% was completely overcome by Fusarium sp. and S. sclerotiorum, whereas Cladosporium sp. remained partially affected, with a sustained 20% growth reduction compared to the control. Mucor sp. did not fully recover from the initial inhibition at 0.25%, but its growth was reduced by only 14%. At 2.00%, none of the fungi were able to fully reverse the initial inhibition, although Fusarium sp. and S. sclerotiorum were able to grow further (Table 1). At 2.50% PE, S. sclerotiorum struggled more to overcome inhibition compared to 2.00%, in contrast to Fusarium sp., which demonstrated lesser sensitivity at these high doses (Table 1).
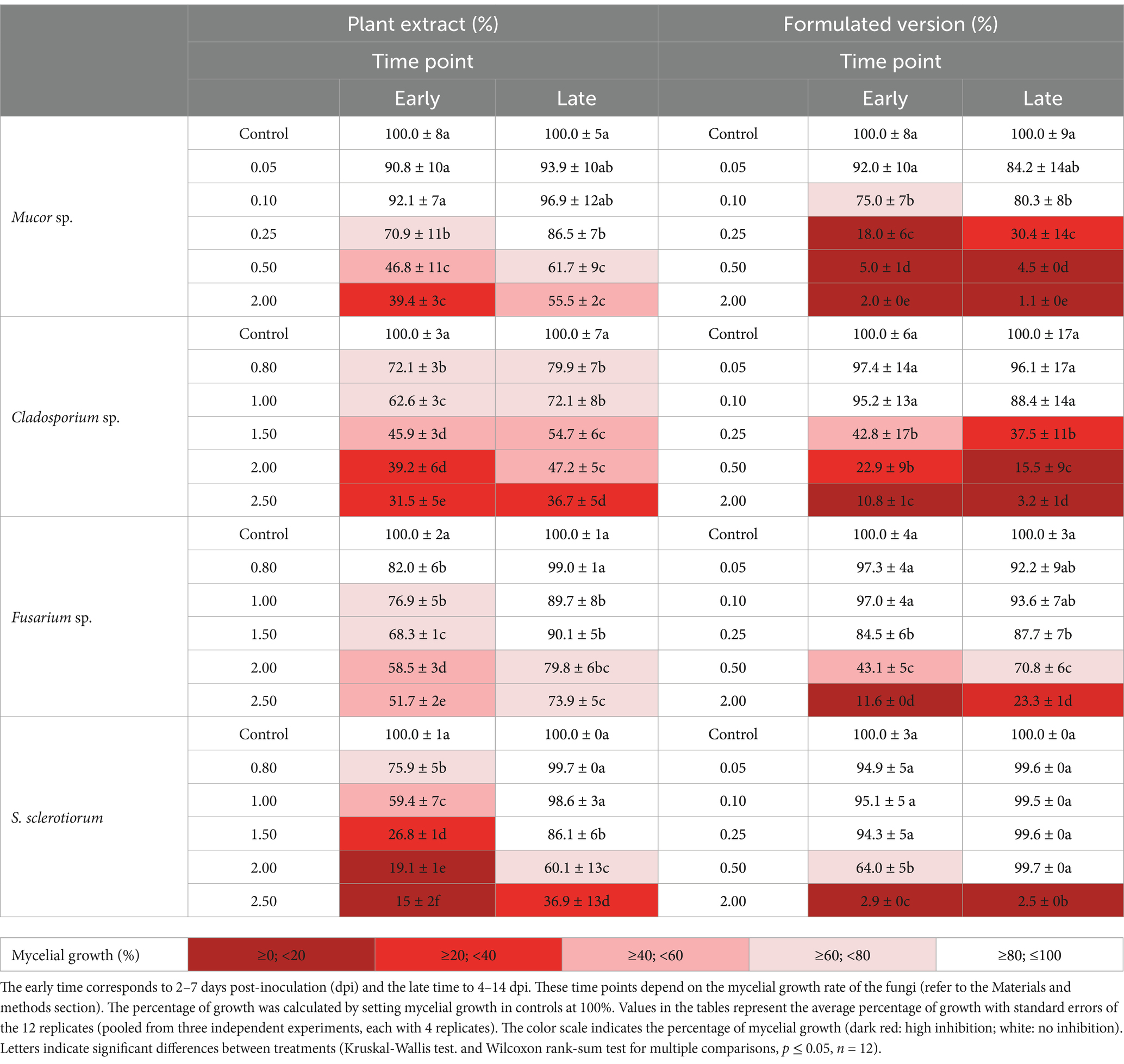
Table 1. Effect of plant extract and formulated version at different concentrations on the mycelial growth of Mucor sp., Cladosporium sp., Fusarium sp. and S. sclerotiorum at early time and late time.
After short-term exposure to FV, Mucor sp. was again the most sensitive, with its growth partially reduced at 0.10%, unlike the other fungi, where this concentration was ineffective (Table 1). At 0.25%, the growth of Cladosporium sp. decreased by 57% compared to the control, similar to Fusarium sp., but to a lesser extent (Table 1). S. sclerotiorum showed sensitivity starting at 0.50% FV, with a 36% reduction in growth compared to the control (Table 1). At 0.50%, Mucor sp. and Cladosporium sp. showed the most pronounced growth inhibition, with reductions of 95 and 77%, respectively, compared to the control, while Fusarium sp. displayed a more moderate growth reduction of 57% (Table 1). Overall, at 2.00%, the four tested fungi exhibited strong growth reductions (88 to 98%; Table 1). After long-term exposure, the initial growth reduction of Cladosporium sp. and Fusarium sp. started at 0.25%, although the impact on Fusarium sp. was less severe, consistent with earlier observations (Table 1). A similar pattern of sustained inhibition was observed for Mucor sp., where growth remained suppressed starting from 0.10%. In contrast, S. sclerotiorum completely overcame the initial reduction at 0.50% (Table 1). Notably, at 2.00% FV, Fusarium sp. exhibited slight growth progression, accompanied by changes in its appearance and color. Meanwhile, at this concentration, S. sclerotiorum, Mucor sp., and Cladosporium sp. continued to show strong growth reductions (> 97%; Table 1; Supplementary Figure S3). Overall, FV had stronger antifungal activity than PE, with varying sensitivities observed among the fungal species at high concentrations.
3.4 The formulated version and the plant extract exhibited distinct effects on bacterial growth
To further explore the non-target effects, the sensitivity of two ubiquitous bacteria, Bacillus subtilis (Gram-positive) and Pseudomonas fluorescens (Gram-negative), to PE and FV was evaluated at concentrations of 0.10, 0.50, and 2.00%. After the incubation period, neither bacterial strain showed sensitivity to PE (Table 2). In contrast, a dose-dependent effect was observed with FV. B. subtilis showed a 1-log reduction in CFU compared to the control at 0.10%. Notably, P. fluorescens showed sensitivity to FV only at 0.50%. At 2.00% FV, no colonies were detected across all dilutions, indicating complete growth inhibition of both bacterial strains. In summary, while PE had no observable effect on either bacterial species, FV demonstrated antibacterial activity at higher doses, with B. subtilis displaying greater sensitivity than P. fluorescens.
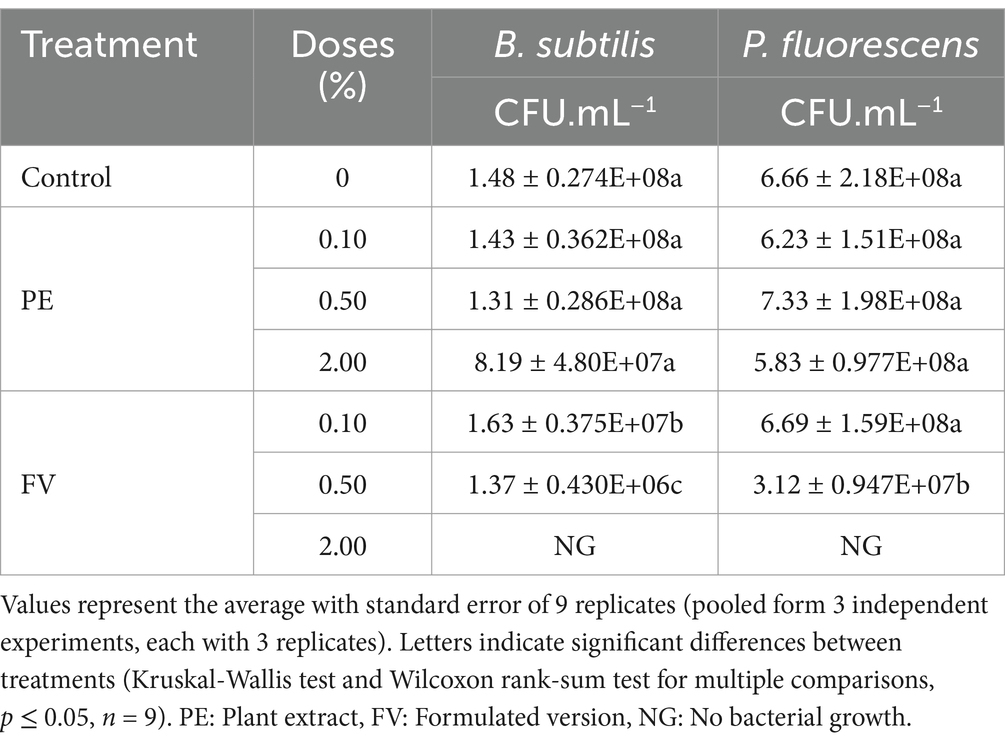
Table 2. Effect of PE and FV on bacterial growth of Bacillus subtilis and Pseudomonas fluorescens 24 h and 48 h of incubation, respectively.
4 Discussion
In the context of reducing fungicide usage, exploring sustainable strategies for managing late blight is essential (Popp et al., 2013). Biocontrol agents are emerging as promising alternatives for protecting crops against Phytophthora infestans (Hashemi et al., 2022). Beyond understanding their modes of action, assessing their effects on a broad range of organisms is equally important to ensure efficacy and safety. This study applied an in vitro approach to evaluate the biocontrol product, focusing on its formulated version (FV), plant extract (PE) and concentrated plant extract (CPE), to investigate their effects on three critical lifecycle structures of this oomycete, and their impact on non-target fungi and bacteria. This dual approach enables us to understand the mechanisms of action while assessing the ecological compatibility of the biocontrol agents, providing insights into their potential role in integrated pest management systems.
4.1 The biocontrol product affected mycelial growth of Phytophthora infestans
This study revealed strong inhibition of P. infestans mycelial growth at low concentrations of FV, while plant extract CPE demonstrated efficacy at higher concentrations (0.80 to 2.50%). The results obtained with CPE suggest that increasing the dose of the plant extract improves its efficacy in a dose-dependent manner. Moreover, these findings highlight the role of the formulation in enhancing the anti-oomycete activity of the plant extract. Among co-formulants, surfactants perform multiple functions, such as enhancing spray quality, improving adhesion, and facilitating the penetration of the active compound into the leaf tissue (Jibrin et al., 2021). Enhanced solubilization of the plant extract in its formulated version may provide a plausible explanation for the observed increase in efficacy. Recent studies have demonstrated that the incorporation of surfactants enhances the efficacy of plant and algal extracts against a range of pathogenic microorganisms. Indeed, Rashid et al. (2022) reported in in vitro assays, that formulation was more effective than the crude extract of Rhus coriaria in inhibiting the growth of several fungal and bacterial pathogens of tomato, suggesting that surfactants optimize the biocontrol product’s activity. During in planta experiments on grapevines Paris et al. (2016) demonstrated a significant enhancement in protection against Plasmopara viticola when a surfactant was added to an algal extract. While P. infestans exhibited sensitivity to high concentrations of CPE, it showed the ability to overcome the initial inhibitory effects at 0.80 to 1% CPE at 14 dpi. This observation may suggest a potential adaptive response to the plant extract, or alternatively, the decline in inhibitory activity against the oomycete could be attributed to a reduction of the product’s efficacy over time. Similar responses have been observed with prolonged exposure to S-methyl methanethiosulfonate (MMTS) and caraway (Carum carvi) essential oil (Quintanilla et al., 2002; Joller et al., 2020). Interestingly, the highest concentration of FV (0.50%) was less effective than lower doses, a difference that became more apparent 14 dpi. This outcome may resemble the paradoxical effect observed in fungi exposed to echinocandins targeting β-1,3-glucan synthase, where strains resume growth at higher antifungal concentrations despite being inhibited at lower doses (Bizerra et al., 2011; Juvvadi et al., 2015). This effect is believed to involve an increase in cell wall chitin, followed by the reactivation of β-1,3-glucan synthase (Wagener and Loiko, 2018). In this study, although the highest dose reduced efficacy, mycelial growth remained minimal with noticeable morphological differences compared to the control. Degradation of biocontrol products may also play a role. For example, Beauveria bassiana can tolerate, bioaccumulate, and solubilize copper through mechanisms such as cell wall binding, cellular absorption, chelation, and precipitation via secreted molecules (Cervantes and Gutierrez-Corona, 1994; Martins et al., 2012). To eliminate the possibility of potential adaptation, successive transplanting experiments conducted in the presence of this biocontrol product could serve as a valuable approach (Yang et al., 2008; Ajouz et al., 2010; Childers et al., 2015), even though the adaptation to a plant extract-based biocontrol product with a multiple mode of action is not well recognized in the literature (Bardin et al., 2015). The reduced efficacy observed at the highest FV doses suggests that further investigations are needed to assess whether prolonged exposure could lead to tolerance or resistance mechanisms in P. infestans. Future investigations should focus on mycelial viability, potential shifts in sensitivity over successive generations, and product quantification in both the mycelium and the culture medium. However, the complexity of the formulation may present challenges.
4.2 Impact of FV and PE on Phytophthora infestans infection structures
The two infection structures of P. infestans, sporangia and zoospores, contribute to its high infectivity and make it particularly challenging to control. Airborne sporangia can either germinate directly to penetrate the leaf cuticle or release multiple zoospores that germinate and infect the leaves (Fry, 2008; Skelsey et al., 2009). These diverse infection mechanisms highlight the necessity of targeting both structures to ensure effective oomycete control. This study demonstrated that both FV and PE have a strong impact on these infection structures. Consistent with the results from mycelial growth experiments, FV significantly affected sporangia at the lowest concentration tested. Interestingly, while PE had no impact on mycelial growth, it disturbed sporangia germination at a concentration of 0.10%. An important difference between the two tests is the nature of contact between the sporangia and the mycelium with PE. Sporangia suspended in water are in direct contact with PE, while the mycelium grows on a nutrient medium containing PE. In the latter case, the contact might be reduced, where the medium might represent an obstacle for direct contact between the mycelium and PE. The differences or similarities in sensitivity between mycelial growth and sporangia observed in our study align with findings from several other studies. For instance, La Torre et al. (2019) demonstrated similar sensitivity between these two structures when exposed to beet (Beta vulgaris subsp. Vulgaris) molasses vinasse biocontrol products at a concentration of 0.60%. Dorn et al. (2007) demonstrated variable efficacy of certain plant extract treatments on mycelial growth and sporangia. For example, biocontrol product Inulex, based of extract of Inula viscosa at 1.00%, strongly inhibited mycelial growth but weakly affected sporangia germination, while Rheum rhabarbarum extract at 5.00% affected sporangial germination thought had no impact on mycelial growth. In this study, both sporangial germination and zoospore release were reduced at 0.01% FV and 0.10% PE, suggesting similar sensitivities of these structures to the treatments. These results had a comparable impact on these processes like other biocontrol agents, such as fengycin B, while the volatile compound 1-undecene, produced by Pseudomonas strains, inhibited sporangial germination at lower concentrations than those required to affect zoospore release (Hunziker et al., 2015; Wang et al., 2020a). These findings suggest that the inhibition of zoospore release and sporangial germination is dependent on the specific active substance used.
Numerous studies have demonstrated the effects of plant extract-based biocontrol products on mycelium and sporangia, but their impact on zoospores has been less explored (La Torre et al., 2019; Najdabbasi et al., 2020). Zoospores, like sporangia, are crucial for successful infection (Fry, 2008). Our results showed that while PE remained effective from 0.10%, FV inhibited zoospore germination at 0.05%, a concentration higher than that needed to affect sporangial germination. Interestingly, the germination rate of sporangia under control conditions averaged 45%, while that of zoospores was 86%. These observations are in line with previous studies, which also reported a significantly higher natural germination rate for zoospores compared to sporangia under in vitro conditions (Bruisson et al., 2019; De Vrieze et al., 2020). This higher germination capacity of zoospores may explain their differential susceptibility to FV. A recent study by Wu et al. (2021) has demonstrated the inhibitory effects of a Myxococcus xanthus extract on the overall germination of P. infestans zoospores. Similarly, Matheron and Porchas (2000) reported the impact of various fungicides on the zoospore’s germination from multiple Phytophthora species. Beyond germination rates, the phenotypes of germinating zoospores can provide critical insights (De Vrieze et al., 2020). In our study, non-inhibitory concentrations of FV and PE induced phenotypic changes with a higher occurrence of phenotypes, such as zoospores with multiple germ tubes and appressorium-like formation. Previous studies on cellulose synthase (CesA) and transglutaminase (TGase) genes, both involved in maintaining P. infestans cell wall integrity, also reported similar germination phenotypes, referred to as “appressorium and germ tube aberrant” (Grenville-Briggs et al., 2008; Brus-Szkalej et al., 2021). Consistent with our results, a higher proportion of these phenotypes was observed in zoospores exposed to a TGase inhibitor and in TGase or CesA silenced lines. Additionally, zoospore lines with silenced TGase or CesA genes showed reduced or no pathogenicity when inoculated on leaves compared to control lines. De Vrieze et al. (2020) also reported shifts in zoospore phenotypes when exposed to biocontrol strains of Pseudomonas, although the specific germination phenotypes described here were not reported. Consistent with our findings, they observed a reduction in the proportion of zoospores named regular appressorium formation when exposed to a strain of Pseudomonas, corresponding to the category we defined as short germ tube with appressorium formation. When exposed to inhibitory concentrations of FV and PE, the few zoospores that germinated exhibited short germ tubes and no appressoria formation. Similarly, Bruisson et al. (2019) observed abnormal germination in zoospores and reported a significant proportion of zoospores with retarded germination when incubated with some phyllosphere bacteria. These findings suggest that the biocontrol product may impair cell wall integrity, though further research is required to confirm this hypothesis.
To understand the impact of biocontrol products (FV and PE) on the infection structures, membrane integrity was monitored using propidium iodide staining (Zhu et al., 2023). Both FV and PE were found to compromise the membrane integrity of sporangia and zoospores, with zoospores exhibiting a greater sensitivity, particularly following PE treatment in comparison to sporangia. Similar findings were reported by Joller et al. (2020), though to a lesser extent. The lack of a cell wall before zoospore encystment could facilitate easier diffusion of the biocontrol product, enabling it to more effectively reach and disrupt the plasma membrane. The observed membrane integrity disruption in both infection structures at lower doses for FV supports the hypothesis that the formulation enhances the plant extract’s activity on membrane integrity. The precise mechanism underlying membrane integrity disruption has not yet been fully elucidated, although several hypotheses can be proposed based on previous studies. To test this, it would be useful to assess mycelium membrane permeability using propidium iodide (PI). Additionally, studies on the effects of amphiphilic molecules, whether of microbial or plant origin, have provided insights into these mechanisms. For instance, Botcazon et al. (2022) used Alexa Fluor 488-Annexin V staining, on Sclerotinia sclerotiorum mycelium, in addition to PI, which specifically binds to phosphatidylserine (PS) exposed on the outer membrane, an early marker of cell death. Recently, Wang et al. (2020b) also supported their observations on P. infestans mycelium by measuring electrolyte leakage into the culture medium, which can be quantified through conductivity measurements. Furthermore, additional analyses such as quantifying reactive oxygen species (ROS), measuring lipid peroxidation, and using transmission electron microscopy (TEM), would help confirm the impact of these molecules on membrane integrity (Ito et al., 2007; Wang et al., 2020b; Adamczyk et al., 2023).
4.3 Differential effects of FV and PE on non-target fungal and bacterial species
In this study, the non-target effects of FV and PE were assessed on three phyllosphere fungi (Mucor sp., Cladosporium sp., and Fusarium sp.) and the plant pathogen S. sclerotiorum. The results revealed that while all tested fungi exhibited sensitivity to both versions, this sensitivity occurred mainly at higher concentrations, with FV consistently demonstrating greater impact than PE. Among the tested fungi, Mucor sp. demonstrated the highest sensitivity to both versions, followed by Cladosporium sp., while Fusarium sp. and S. sclerotiorum were the least affected. This variation in sensitivity may reflect biochemical differences across fungal phyla (Ghormade et al., 2017; Wang et al., 2021). Our previous results on the infection structures of P. infestans suggest that the fungal cell wall and plasma membrane are potential primary targets of FV and PE. This may explain the heightened susceptibility of Mucor sp., belonging to the Mucoromycetes phylum, which has a unique cell wall composition (Dzurendova et al., 2022; Sokołowska et al., 2023). Unlike the cell walls of Ascomycetes and Basidiomycetes phylum, which predominantly contain chitin and glucans (Bowman and Free, 2006), Mucoromycetes cell walls are mainly composed of chitin and chitosan with minimal amounts of glucans (Bartnicki-Garcia and Reyes, 1964; Ghormade et al., 2017). Furthermore, the monosaccharide profile of Mucoromycetes cell walls, which includes fucose and glucuronic acid, contrasts with the glucose-, mannose-, and galactose-rich walls of ascomycetes and Basidiomycetes (Yugueros et al., 2024). Further investigations could clarify Mucor sp. susceptibility to FV and PE. Enzymatic assays (e.g., chitinases, chitosanases, and glucanases) could reveal whether the biocontrol product mimics these enzymes. Fluorescence or electron microscopy with specific stains (Calcofluor White for chitin, Aniline Blue for β-glucans) could identify structural alterations, while propidium iodide (PI) staining could assess membrane integrity. At the molecular level, gene expression analysis of cell wall biosynthesis and remodeling enzymes could help determine whether Mucor sp. sensitivity stems from intrinsic cell wall composition or differential stress adaptation mechanisms.
Differences in plasma membrane composition may also influence the response of fungi to the biocontrol product. For instance, Avis and Bélanger (2001) previously showed that fungal susceptibility to membranotropic compounds was associated with the total membrane lipid content. Among these lipids, ergosterol is a major sterol in fungal plasma membranes which is crucial for maintaining membrane integrity (Rattray et al., 1975; Avis, 2007). However, ergosterol content may vary across fungal species and isolates (Avis and Bélanger, 2001; Botcazon et al., 2022). This variation has been shown to influence fungal sensitivity to membranotropic compounds, as observed in S. sclerotiorum isolates (Wise et al., 2014; Botcazon et al., 2022). In our study S. sclerotiorum and Fusarium sp. were the fungi least sensitive to FV and PE, often overcoming initial inhibition at certain doses. Reduced sensitivity of Fusarium sp. compared to other fungi has been similarly observed in a study involving exposure to terpenes (Adamczyk et al., 2023). This resilience may be linked to their respective lifestyles: S. sclerotiorum is a necrotroph, while Fusarium spp., mostly phytopathogenic exhibits a hemibiotrophic lifestyle (Bolton et al., 2006; Pradhan et al., 2020; Nikitin et al., 2023). Fungi with these lifestyles are known to detoxify antifungal compounds via enzymatic degradation and efflux mechanisms (Westrick et al., 2021). For example, Fusarium oxysporum f. sp. lycopersici degrades the membrane-disrupting saponin α-tomatine into less-toxic tomatidine (Osbourn, 1996; Sandrock and VanEtten, 1997). Additionally, efflux transport proteins, such as ABC and MFS transporters, are also implicated in detoxification. In contrast, bacterial species showed differential sensitivity to FV. The Gram-positive bacterium B. subtilis was more susceptible than the Gram-negative P. fluorescens, likely due to structural differences in their cell envelopes (Beveridge, 1999). The presence of an outer lipid membrane in Gram-negative bacteria, which shields the peptidoglycan layer, likely reduces FV accessibility compared to Gram-positive bacteria, which lack this barrier (Nikaido and Nakae, 1980). While plant extracts have shown antibacterial potential (Nourbakhsh et al., 2022), some studies reported their ineffectiveness on bacterial growth (Erdogrul, 2002). Similarly, our results showed that PE had no effect on bacterial growth. The effect of FV at higher concentrations, particularly at 0.10%, on the fungus Mucor sp. and the bacterium Bacillus subtilis raises questions about their respective roles in ecosystems. The Mucor genus, which is predominantly saprophytic, plays a key role in organic matter decomposition and biogeochemical cycles (Hoffmann et al., 2013). Bacillus subtilis, in addition to being a plant growth-promoting rhizobacterium (PGPR), also exhibits biocontrol activity (Blake et al., 2021). Given the observed effects on these microorganisms, it is possible that other, more sensitive species could also be impacted. The inhibition observed in our study results from direct interaction between these microorganisms and the biocontrol product, but its broader impact on microbial dynamics within the ecosystem requires further investigation. Therefore, it is essential to conduct additional experiments to assess the effect of FV on in planta microbial communities, particularly through metabarcoding analysis. The phyllosphere hosts essential microbial communities, including many beneficial microorganism and natural biocontrol agents (Legein et al., 2020; Sohrabi et al., 2023). Since biocontrol products are often applied repeatedly during the crop cycle, evaluating the long-term effects of FV on the foliar microbiome is crucial. Some studies have highlighted the resilience of vineyard phyllosphere microbial communities to fungicides (Perazzolli et al., 2014). However, recent research suggests that repeated fungicide use can significantly alter the composition of soil and phyllosphere microbial communities. It is therefore essential to determine whether prolonged application of FV could induce similar changes, potentially affecting the stability and functionality of the foliar microbiome.
Comparing the impact of the biocontrol products on the mycelial growth of oomycetes, fungi, and bacteria growth, P. infestans demonstrated the highest sensitivity to FV compared to fungi showed the highest sensitivity to PE. However, the results obtained with the concentrated plant extract (CPE) on P. infestans mycelial growth presented a dose-dependent response on plant extract. Based on these findings the high efficacy of FV at low concentrations suggests the plant extract activity optimization by the formulation. Moreover, this differential sensitivity of P. infestans to FV and PE in comparison to the other microorganisms tested could be explained by its auxotrophic characteristic for sterols. In addition, the unique composition of P. infestans cell wall, which contains cellulose and lacks chitin may have also a role in this variation (Bartnicki-Garcia, 1968; Wang et al., 2021). The hypothesis considering the cell wall and plasma membrane as potential targets of biocontrol products is supported by the observed impact on infection structures. However, it is possible that the biocontrol products also interact with other cellular components. Numerous studies have reported non-target effects of plant extracts and plant-derived compounds, meanwhile our findings suggest that the biocontrol product has minimal non-target effects, particularly at low doses (Adamczyk et al., 2023; Teixeira et al., 2023). The plant extract showed efficacy against P. infestans infection structures at a dose (0.10%) where bacterial and fungal growth was not compromised. The formulated version provided better efficacy against P. infestans, but a moderate impact was observed from 0.10% on bacterial growth of B. subtilis and Mucor sp. This study shows that the choice of dose is crucial to control P. infestans infection while avoiding a non-target effect on microorganisms in the phyllosphere. To achieve a better balance between efficacy and safety, work on formulation might be also a determining factor to limit at the maximum the impact on non-target microorganisms.
In conclusion, this study underscores in vitro efficacy of the low-dose biocontrol product against P. infestans, including its infection structures and mycelial growth, while demonstrating minimal impact on non-target microorganisms. The addition of co-formulants appears to be a promising approach for enhancing the activity of existing plant extracts, highlighting the need for further investigation to better understand the underlying mechanisms. Future research should aim to elucidate the mode of action of the plant extract and its formulated version, assess their effects on microbial diversity in plant and soil ecosystems, and explore their potential to induce plant resistance against late blight.
Data availability statement
The raw data supporting the conclusions of this article will be made available by the authors, without undue reservation.
Author contributions
VP: Conceptualization, Formal analysis, Investigation, Methodology, Visualization, Writing – original draft. AA: Conceptualization, Methodology, Supervision, Writing – review & editing. MV: Methodology, Resources, Writing – review & editing. MB: Methodology, Writing – review & editing. ME: Investigation, Writing – review & editing. AB-M: Conceptualization, Funding acquisition, Project administration, Resources, Supervision, Writing – review & editing. IT-G: Funding acquisition, Project administration, Writing – review & editing. KL: Conceptualization, Funding acquisition, Methodology, Project administration, Supervision, Writing – review & editing. AG: Conceptualization, Funding acquisition, Methodology, Project administration, Supervision, Writing – review & editing.
Funding
The author(s) declare that financial support was received for the research and/or publication of this article. Valentin Penaud’s Ph.D. was supported through funding provided by the French National Research and Technology Association (ANRT) under the CIFRE program (CIFRE No. 2021/1362). This funding is allocated to the company, which in turn covers Valentin Penaud’s salary. The BIOMES Chair for Research and Innovation is supported by Gaïago SAS, a company that takes advantage of the French research tax credit program, designed to promote research and development initiatives within businesses in France.
Acknowledgments
The authors would like to thank the team of the AGHYLE research unit and also the AGROBIOTECH platform for the help and support. We would like to thank Laure Weisskopf for her hospitality and generous sharing of her expertise during our time in her research laboratory. We would also like to thank Sylvain Raffaele for providing the Sclerotinia sclerotiorum strain and for his valuable guidance. I would also like to thank Mébarek Temagoult from AGHYLE for his contribution in the fluorescence microscopy analysis and Elisa Barray for her help.
Conflict of interest
AB-M is the CEO of the company Biom InnoV. The only financial interest to note is that the product studied is currently under research and development process by the company funding the project. The UniLaSalle research unit AGHYLE retains the right to terminate the collaboration contract immediately if the company’s expectations and or demands conflict with ethical standards, research integrity, or scientific principles. Beyond these disclosures, the authors declare no other relevant financial or non-financial interests.
The remaining authors declare that the research was conducted in the absence of any commercial or financial relationships that could be construed as a potential conflict of interest.
Generative AI statement
The authors declare that no Gen AI was used in the creation of this manuscript.
Publisher’s note
All claims expressed in this article are solely those of the authors and do not necessarily represent those of their affiliated organizations, or those of the publisher, the editors and the reviewers. Any product that may be evaluated in this article, or claim that may be made by its manufacturer, is not guaranteed or endorsed by the publisher.
Supplementary material
The Supplementary material for this article can be found online at: https://www.frontiersin.org/articles/10.3389/fmicb.2025.1569281/full#supplementary-material
References
Abuley, I. K., Lynott, J. S., Hansen, J. G., Cooke, D. E. L., and Lees, A. K. (2023). The EU43 genotype of Phytophthora infestans displays resistance to mandipropamid. Plant Pathol. 72, 1305–1313. doi: 10.1111/ppa.13737
Adamczyk, S., Latvala, S., Poimala, A., Adamczyk, B., Hytönen, T., and Pennanen, T. (2023). Diterpenes and triterpenes show potential as biocides against pathogenic fungi and oomycetes: a screening study. Biotechnol. Lett. 45, 1555–1563. doi: 10.1007/s10529-023-03438-z
Ajouz, S., Nicot, P. C., and Bardin, M. (2010). Adaptation to pyrrolnitrin in Botrytis cinerea and cost of resistance. Plant Pathol. 59, 556–566. doi: 10.1111/j.1365-3059.2009.02230.x
Alfiky, A., Abou-Mansour, E., De Vrieze, M., L’Haridon, F., and Weisskopf, L. (2024). Newly isolated Trichoderma spp. show multifaceted biocontrol strategies to inhibit potato late blight causal agent Phytophthora infestans both in vitro and in planta. Phytobiomes J. 8, 70–84. doi: 10.1094/PBIOMES-01-23-0002-R
Avis, T. J. (2007). Antifungal compounds that target fungal membranes: applications in plant disease control. Can. J. Plant Pathol. 29, 323–329. doi: 10.1080/07060660709507478
Avis, T. J., and Bélanger, R. R. (2001). Specificity and mode of action of the antifungal fatty acid cis-9-Heptadecenoic acid produced by Pseudozyma flocculosa. Appl. Environ. Microbiol. 67, 956–960. doi: 10.1128/AEM.67.2.956-960.2001
Bardin, M., Ajouz, S., Comby, M., Lopez-Ferber, M., Graillot, B., Siegwart, M., et al. (2015). Is the efficacy of biological control against plant diseases likely to be more durable than that of chemical pesticides? Front. Plant Sci. 6:566. doi: 10.3389/fpls.2015.00566
Bartnicki-Garcia, S. (1968). Cell Wall chemistry, morphogenesis, and taxonomy of Fungi. Ann. Rev. Microbiol. 22, 87–108. doi: 10.1146/annurev.mi.22.100168.000511
Bartnicki-Garcia, S., and Reyes, E. (1964). Chemistry of spore wall differentiation in Mucor rouxii. Arch. Biochem. Biophys. 108, 125–133. doi: 10.1016/0003-9861(64)90363-7
Bensch, K., Braun, U., Groenewald, J. Z., and Crous, P. W. (2012). The genus Cladosporium. Stud. Mycol. 72, 1–401. doi: 10.3114/sim0003
Beveridge, T. J. (1999). Structures of gram-negative cell walls and their derived membrane vesicles. J. Bacteriol. 181, 4725–4733. doi: 10.1128/jb.181.16.4725-4733.1999
Bizerra, F. C., Melo, A. S. A., Katchburian, E., Freymüller, E., Straus, A. H., Takahashi, H. K., et al. (2011). Changes in cell wall synthesis and ultrastructure during paradoxical growth effect of caspofungin on four different Candida species. Antimicrob Agents Chemother 55, 302–310. doi: 10.1128/AAC.00633-10
Blake, C., Christensen, M. N., and Kovács, Á. T. (2021). Molecular aspects of plant growth promotion and protection by Bacillus subtilis. Mol. Plant Microbe Interact. 34, 15–25. doi: 10.1094/MPMI-08-20-0225-CR
Bolton, M. D., Thomma, B. P. H. J., and Nelson, B. D. (2006). Sclerotinia sclerotiorum (lib.) de Bary: biology and molecular traits of a cosmopolitan pathogen. Mol. Plant Pathol. 7, 1–16. doi: 10.1111/j.1364-3703.2005.00316.x
Botcazon, C., Bergia, T., Lecouturier, D., Dupuis, C., Rochex, A., Acket, S., et al. (2022). Rhamnolipids and fengycins, very promising amphiphilic antifungal compounds from bacteria secretomes, act on Sclerotiniaceae fungi through different mechanisms. Front. Microbiol. 13:633. doi: 10.3389/fmicb.2022.977633
Bowman, S. M., and Free, S. J. (2006). The structure and synthesis of the fungal cell wall. BioEssays 28, 799–808. doi: 10.1002/bies.20441
Bruisson, S., Zufferey, M., L’Haridon, F., Trutmann, E., Anand, A., Dutartre, A., et al. (2019). Endophytes and epiphytes from the grapevine leaf microbiome as potential biocontrol agents against Phytopathogens. Front. Microbiol. 10:726. doi: 10.3389/fmicb.2019.02726
Brus-Szkalej, M., Andersen, C. B., Vetukuri, R. R., and Grenville-Briggs, L. J. (2021). A family of cell wall transglutaminases is essential for appressorium development and pathogenicity in Phytophthora infestans. BioRxiv 2021:665. doi: 10.1101/2021.11.23.469665
Caulier, S., Gillis, A., Colau, G., Licciardi, F., Liépin, M., Desoignies, N., et al. (2018). Versatile antagonistic activities of soil-borne Bacillus spp. and Pseudomonas spp. against Phytophthora infestans and other potato pathogens. Front. Microbiol. 9:143. doi: 10.3389/fmicb.2018.00143
Cenobio-Galindo, A. D. J., Hernández-Fuentes, A. D., González-Lemus, U., Zaldívar-Ortega, A. K., González-Montiel, L., Madariaga-Navarrete, A., et al. (2024). Biofungicides based on plant extracts: on the road to organic farming. Int. J. Mol. Sci. 25:6879. doi: 10.3390/ijms25136879
Cervantes, C., and Gutierrez-Corona, F. (1994). Copper resistance mechanisms in bacteria and fungi. FEMS Microbiol. Rev. 14, 121–137. doi: 10.1111/j.1574-6976.1994.tb00083.x
Childers, R., Danies, G., Myers, K., Fei, Z., Small, I. M., and Fry, W. E. (2015). Acquired resistance to Mefenoxam in sensitive isolates of Phytophthora infestans. Phytopathology 105, 342–349. doi: 10.1094/PHYTO-05-14-0148-R
De Vrieze, M., Germanier, F., Vuille, N., and Weisskopf, L. (2018). Combining different potato-associated Pseudomonas strains for improved biocontrol of Phytophthora infestans. Front. Microbiol. 9:573. doi: 10.3389/fmicb.2018.02573
De Vrieze, M., Gloor, R., Massana Codina, J., Torriani, S., Gindro, K., L’Haridon, F., et al. (2019). Biocontrol activity of three Pseudomonas in a newly assembled collection of Phytophthora infestans isolates. Phytopathology 109, 1555–1565. doi: 10.1094/PHYTO-12-18-0487-R
De Vrieze, M., Varadarajan, A. R., Schneeberger, K., Bailly, A., Rohr, R. P., Ahrens, C. H., et al. (2020). Linking comparative genomics of nine potato-associated Pseudomonas isolates with their differing biocontrol potential against late blight. Front. Microbiol. 11:857. doi: 10.3389/fmicb.2020.00857
Dela Cruz, J. A., Camenzind, T., and Rillig, M. C. (2022). Sub-lethal fungicide concentrations both reduce and stimulate the growth rate of non-target soil fungi from a natural grassland. Front. Environ. Sci. 10:465. doi: 10.3389/fenvs.2022.1020465
Dorn, B., Musa, T., Krebs, H., Fried, P. M., and Forrer, H. R. (2007). Control of late blight in organic potato production: evaluation of copper-free preparations under field, growth chamber and laboratory conditions. Eur. J. Plant Pathol. 119, 217–240. doi: 10.1007/s10658-007-9166-0
Dzurendova, S., Losada, C. B., Dupuy-Galet, B. X., Fjær, K., and Shapaval, V. (2022). Mucoromycota fungi as powerful cell factories for modern biorefinery. Appl. Microbiol. Biotechnol. 106, 101–115. doi: 10.1007/s00253-021-11720-1
Earl, A. M., Losick, R., and Kolter, R. (2008). Ecology and genomics of Bacillus subtilis. Trends Microbiol. 16, 269–275. doi: 10.1016/j.tim.2008.03.004
Erdogrul, Ö. T. (2002). Antibacterial activities of some plant extracts used in folk medicine. Pharm. Biol. 40, 269–273. doi: 10.1076/phbi.40.4.269.8474
Forrer, H.-R., Vogelgsang, S., and Musa, T. (2017). Botanicals and phosphonate show potential to replace copper for control of potato late blight. J. Fungi 3:65. doi: 10.3390/jof3040065
Fry, W. (2008). Phytophthora infestans: the plant (and R gene) destroyer. Mol. Plant Pathol. 9, 385–402. doi: 10.1111/j.1364-3703.2007.00465.x
Fry, W. E., Birch, P. R. J., Judelson, H. S., Grünwald, N. J., Danies, G., Everts, K. L., et al. (2015). Five reasons to consider Phytophthora infestans a reemerging pathogen. Phytopathology 105, 966–981. doi: 10.1094/PHYTO-01-15-0005-FI
Ghormade, V., Pathan, E. K., and Deshpande, M. V. (2017). Can fungi compete with marine sources for chitosan production? Int. J. Biol. Macromol. 104, 1415–1421. doi: 10.1016/j.ijbiomac.2017.01.112
Grenville-Briggs, L. J., Anderson, V. L., Fugelstad, J., Avrova, A. O., Bouzenzana, J., Williams, A., et al. (2008). Cellulose synthesis in Phytophthora infestans is required for Normal Appressorium formation and successful infection of potato. Plant Cell 20, 720–738. doi: 10.1105/tpc.107.052043
Haas, D., and Défago, G. (2005). Biological control of soil-borne pathogens by fluorescent pseudomonads. Nat. Rev. Microbiol. 3, 307–319. doi: 10.1038/nrmicro1129
Hashemi, M., Tabet, D., Sandroni, M., Benavent-Celma, C., Seematti, J., Andersen, C. B., et al. (2022). The hunt for sustainable biocontrol of oomycete plant pathogens, a case study of Phytophthora infestans. Fungal Biol. Rev. 40, 53–69. doi: 10.1016/j.fbr.2021.11.003
Haverkort, A. J., Boonekamp, P. M., Hutten, R., Jacobsen, E., Lotz, L. A. P., Kessel, G. J. T., et al. (2008). Societal costs of late blight in potato and prospects of durable resistance through Cisgenic modification. Potato Res. 51, 47–57. doi: 10.1007/s11540-008-9089-y
Hernandez-Tenorio, F., Miranda, A. M., Rodríguez, C. A., Giraldo-Estrada, C., and Sáez, A. A. (2022). Potential strategies in the biopesticide formulations: a bibliometric analysis. Agronomy 12:2665. doi: 10.3390/agronomy12112665
Hoffmann, K., Pawłowska, J., Walther, G., Wrzosek, M., de Hoog, G. S., Benny, G. L., et al. (2013). The family structure of the Mucorales: a synoptic revision based on comprehensive multigene-genealogies. Persoonia 30, 57–76. doi: 10.3767/003158513X666259
Hunziker, L., Bönisch, D., Groenhagen, U., Bailly, A., Schulz, S., and Weisskopf, L. (2015). Pseudomonas strains naturally associated with potato plants produce volatiles with high potential for inhibition of Phytophthora infestans. Appl. Environ. Microbiol. 81, 821–830. doi: 10.1128/AEM.02999-14
Ito, S., Ihara, T., Tamura, H., Tanaka, S., Ikeda, T., Kajihara, H., et al. (2007). α-Tomatine, the major saponin in tomato, induces programmed cell death mediated by reactive oxygen species in the fungal pathogen Fusarium oxysporum. FEBS Lett. 581, 3217–3222. doi: 10.1016/j.febslet.2007.06.010
Janisiewicz, W. J., and Korsten, L. (2002). Biological control of postharvest diseases of fruits. Annu. Rev. Phytopathol. 40, 411–441. doi: 10.1146/annurev.phyto.40.120401.130158
Jibrin, M. O., Liu, Q., Jones, J. B., and Zhang, S. (2021). Surfactants in plant disease management: a brief review and case studies. Plant Pathol. 70, 495–510. doi: 10.1111/ppa.13318
Joller, C., De Vrieze, M., Moradi, A., Fournier, C., Chinchilla, D., L’Haridon, F., et al. (2020). S-methyl Methanethiosulfonate: promising late blight inhibitor or broad range toxin? Pathogens 9:496. doi: 10.3390/pathogens9060496
Juvvadi, P. R., Muñoz, A., Lamoth, F., Soderblom, E. J., Moseley, M. A., Read, N. D., et al. (2015). Calcium-mediated induction of paradoxical growth following Caspofungin treatment is associated with Calcineurin activation and phosphorylation in Aspergillus fumigatus. Antimicrob. Agents Chemother. 59, 4946–4955. doi: 10.1128/aac.00263-15
La Torre, A., Righi, L., Iovino, V., and Battaglia, V. (2019). Control of late blight in organic farming with low copper dosages or natural products as alternatives to copper. Eur. J. Plant Pathol. 155, 769–778. doi: 10.1007/s10658-019-01804-0
Legein, M., Smets, W., Vandenheuvel, D., Eilers, T., Muyshondt, B., Prinsen, E., et al. (2020). Modes of action of microbial biocontrol in the Phyllosphere. Front. Microbiol. 11:619. doi: 10.3389/fmicb.2020.01619
Marchand, P. A. (2023). Evolution of plant protection active substances in Europe: the disappearance of chemicals in favour of biocontrol agents. Environ. Sci. Pollut. Res. 30, 1–17. doi: 10.1007/s11356-022-24057-7
Martins, F., Soares, M. E., Oliveira, I., Pereira, J. A., de Lourdes Bastos, M., and Baptista, P. (2012). Tolerance and bioaccumulation of copper by the Entomopathogen Beauveria bassiana (Bals.-Criv.) Vuill. Exposed to various copper-based fungicides. Bull. Environ. Contam. Toxicol. 89, 53–60. doi: 10.1007/s00128-012-0628-5
Massabò, D., Danelli, S. G., Brotto, P., Comite, A., Costa, C., Di Cesare, A., et al. (2018). ChAMBRe: a new atmospheric simulation chamber for aerosol modelling and bio-aerosol research. Atmos. Meas. Tech. 11, 5885–5900. doi: 10.5194/amt-11-5885-2018
Matheron, M. E., and Porchas, M. (2000). Impact of Azoxystrobin, Dimethomorph, fluazinam, Fosetyl-Al, and Metalaxyl on growth, sporulation, and zoospore cyst germination of three Phytophthora spp. Plant Dis. 84, 454–458. doi: 10.1094/PDIS.2000.84.4.454
Najdabbasi, N., Mirmajlessi, S. M., Dewitte, K., Landschoot, S., Mänd, M., Audenaert, K., et al. (2020). Biocidal activity of plant-derived compounds against Phytophthora infestans: an alternative approach to late blight management. Crop Prot. 138:105315. doi: 10.1016/j.cropro.2020.105315
Nikaido, H., and Nakae, T. (1980). “The outer membrane of gram-negative Bacteria” in Advances in microbial physiology. eds. A. H. Rose and J. G. Morris (Cambridge, MA: Academic Press), 163–250.
Nikitin, D. A., Ivanova, E. A., Semenov, M. V., Zhelezova, A. D., Ksenofontova, N. A., Tkhakakhova, A. K., et al. (2023). Diversity, ecological characteristics and identification of some problematic phytopathogenic Fusarium in soil: a review. Diversity 15:49. doi: 10.3390/d15010049
Nourbakhsh, F., Lotfalizadeh, M., Badpeyma, M., Shakeri, A., and Soheili, V. (2022). From plants to antimicrobials: natural products against bacterial membranes. Phytother. Res. 36, 33–52. doi: 10.1002/ptr.7275
Nowicki, M., Foolad, M. R., Nowakowska, M., and Kozik, E. U. (2012). Potato and tomato late blight caused by Phytophthora infestans: an overview of pathology and resistance breeding. Plant Dis. 96, 4–17. doi: 10.1094/PDIS-05-11-0458
Osbourn, A. (1996). Saponins and plant defence–a soap story. Trends Plant Sci. 1, 4–9. doi: 10.1016/S1360-1385(96)80016-1
Paris, F., Krzyżaniak, Y., Gauvrit, C., Jamois, F., Domergue, F., Joubès, J., et al. (2016). An ethoxylated surfactant enhances the penetration of the sulfated laminarin through leaf cuticle and stomata, leading to increased induced resistance against grapevine downy mildew. Physiol. Plant. 156, 338–350. doi: 10.1111/ppl.12394
Pawłowska, J., Okrasińska, A., Kisło, K., Aleksandrzak-Piekarczyk, T., Szatraj, K., Dolatabadi, S., et al. (2019). Carbon assimilation profiles of mucoralean fungi show their metabolic versatility. Sci. Rep. 9:11864. doi: 10.1038/s41598-019-48296-w
Perazzolli, M., Antonielli, L., Storari, M., Puopolo, G., Pancher, M., Giovannini, O., et al. (2014). Resilience of the natural Phyllosphere microbiota of the grapevine to chemical and biological pesticides. Appl. Environ. Microbiol. 80, 3585–3596. doi: 10.1128/AEM.00415-14
Picot, L., Abdelmoula, S. M., Merieau, A., Leroux, P., Cazin, L., Orange, N., et al. (2001). Pseudomonas fluorescens as a potential pathogen: adherence to nerve cells. Microbes Infect. 3, 985–995. doi: 10.1016/S1286-4579(01)01462-9
Popp, J., Pető, K., and Nagy, J. (2013). Pesticide productivity and food security. A review. Agron. Sustain. Dev. 33, 243–255. doi: 10.1007/s13593-012-0105-x
Pradhan, M., Pandey, P., Baldwin, I. T., and Pandey, S. P. (2020). Argonaute4 modulates resistance to Fusarium brachygibbosum infection by regulating Jasmonic acid signaling. Plant Physiol. 184, 1128–1152. doi: 10.1104/pp.20.00171
Quintanilla, P., Rohloff, J., and Iversen, T.-H. (2002). Influence of essential oils on Phytophthora infestans. Potato Res. 45, 225–235. doi: 10.1007/BF02736117
Rashid, T. S., Awla, H. K., and Sijam, K. (2022). Formulation, characterization and antimicrobial activity of Rhus coriaria aqueous crude extract. Biocatal. Agric. Biotechnol. 45:102519. doi: 10.1016/j.bcab.2022.102519
Rattray, J. B., Schibeci, A., and Kidby, D. K. (1975). Lipids of yeasts. Bacteriol. Rev. 39, 197–231. doi: 10.1128/br.39.3.197-231.1975
Sandrock, R. W., and VanEtten, H. D. (1997). Fungal sensitivity to and enzymatic degradation of the phytoanticipin α-Tomatine. Phytopathology 88, 137–143. doi: 10.1094/PHYTO.1998.88.2.137
Schepers, H. T. A. M., Kessel, G. J. T., Lucca, F., Förch, M. G., van den Bosch, G. B. M., Topper, C. G., et al. (2018). Reduced efficacy of fluazinam against Phytophthora infestans in the Netherlands. Eur. J. Plant Pathol. 151, 947–960. doi: 10.1007/s10658-018-1430-y
Skelsey, P., Kessel, G. J. T., Holtslag, A. A. M., Moene, A. F., and van der Werf, W. (2009). Regional spore dispersal as a factor in disease risk warnings for potato late blight: a proof of concept. Agric. For. Meteorol. 149, 419–430. doi: 10.1016/j.agrformet.2008.09.005
Sohrabi, R., Paasch, B. C., Liber, J. A., and He, S. Y. (2023). Phyllosphere microbiome. Annu. Rev. Plant Biol. 74, 539–568. doi: 10.1146/annurev-arplant-102820-032704
Sokołowska, B., Orłowska, M., Okrasińska, A., Piłsyk, S., Pawłowska, J., and Muszewska, A. (2023). What can be lost? Genomic perspective on the lipid metabolism of Mucoromycota. IMA Fungus 14:22. doi: 10.1186/s43008-023-00127-4
Teixeira, A., Sánchez-Hernández, E., Noversa, J., Cunha, A., Cortez, I., Marques, G., et al. (2023). Antifungal activity of plant waste extracts against Phytopathogenic Fungi: Allium sativum peels extract as a promising product targeting the fungal plasma membrane and Cell Wall. Horticulturae 9:136. doi: 10.3390/horticulturae9020136
Van Lenteren, J. C., Bolckmans, K., Köhl, J., Ravensberg, W. J., and Urbaneja, A. (2018). Biological control using invertebrates and microorganisms: plenty of new opportunities. BioControl 63, 39–59. doi: 10.1007/s10526-017-9801-4
Wagener, J., and Loiko, V. (2018). Recent insights into the paradoxical effect of Echinocandins. J. Fungi 4:5. doi: 10.3390/jof4010005
Wang, W., Liu, X., and Govers, F. (2021). The mysterious route of sterols in oomycetes. PLoS Pathog. 17:e1009591. doi: 10.1371/journal.ppat.1009591
Wang, W., and Long, Y. (2023). A review of biocontrol agents in controlling late blight of potatoes and tomatoes caused by Phytophthora infestans and the underlying mechanisms. Pest Manag. Sci. 79, 4715–4725. doi: 10.1002/ps.7706
Wang, Y., Zhang, C., Liang, J., Wang, L., Gao, W., Jiang, J., et al. (2020a). Surfactin and fengycin B extracted from Bacillus pumilus W-7 provide protection against potato late blight via distinct and synergistic mechanisms. Appl. Microbiol. Biotechnol. 104, 7467–7481. doi: 10.1007/s00253-020-10773-y
Wang, Y., Zhang, C., Liang, J., Wu, L., Gao, W., and Jiang, J. (2020b). Iturin a extracted from Bacillus subtilis WL-2 affects Phytophthora infestans via cell structure disruption, oxidative stress, and energy supply dysfunction. Front. Microbiol. 11:536083. doi: 10.3389/fmicb.2020.536083
Westrick, N. M., Smith, D. L., and Kabbage, M. (2021). Disarming the host: detoxification of plant defense compounds during fungal Necrotrophy. Front. Plant Sci. 12:716. doi: 10.3389/fpls.2021.651716
Wise, C., Falardeau, J., Hagberg, I., and Avis, T. J. (2014). Cellular lipid composition affects sensitivity of plant pathogens to Fengycin, an antifungal compound produced by Bacillus subtilis strain CU12. Phytopathology 104, 1036–1041. doi: 10.1094/PHYTO-12-13-0336-R
Wu, Z. H., Ma, Q., Sun, Z. N., Cui, H. C., and Liu, H. R. (2021). Biocontrol mechanism of Myxococcus fulvus B25-I-3 against Phytophthora infestans and its control efficiency on potato late blight. Folia Microbiol. 66, 555–567. doi: 10.1007/s12223-021-00865-1
Yang, C., Hamel, C., Vujanovic, V., and Gan, Y. (2011). Fungicide: modes of action and possible impact on nontarget microorganisms. Int. Sch. Res. Notices 2011:130289, 1–8. doi: 10.5402/2011/130289
Yang, X. J., Yang, L. J., Zeng, F. S., Xiang, L. B., Wang, S. N., Yu, D. Z., et al. (2008). Distribution of baseline sensitivities to natural product Physcion among isolates of Sphaerotheca fuliginea and Pseudoperonospora cubensis. Plant Dis. 92, 1451–1455. doi: 10.1094/PDIS-92-10-1451
Yao, Y., Li, Y., Chen, Z., Zheng, B., Zhang, L., Niu, B., et al. (2016). Biological control of potato late blight using isolates of Trichoderma. Am. J. Potato Res. 93, 33–42. doi: 10.1007/s12230-015-9475-3
Yugueros, S. I., Peláez, J., Stajich, J. E., Fuertes-Rabanal, M., Sánchez-Vallet, A., Largo-Gosens, A., et al. (2024). Study of fungal cell wall evolution through its monosaccharide composition: an insight into fungal species interacting with plants. Cell Surf. 11:100127. doi: 10.1016/j.tcsw.2024.100127
Keywords: biocontrol, Phytophthora infestans, plant extract, sporangia, zoospores, fungi, bacteria
Citation: Penaud V, Alahmad A, De Vrieze M, Bouteiller M, Eude M, Bernardon-Mery A, Trinsoutrot-Gattin I, Laval K and Gauthier A (2025) In vitro biocontrol potential of plant extract-based formulation against infection structures of Phytophthora infestans along with lower non-target effects. Front. Microbiol. 16:1569281. doi: 10.3389/fmicb.2025.1569281
Edited by:
Md Motaher Hossain, Bangabandhu Sheikh Mujibur Rahman Agricultural University, BangladeshReviewed by:
Talwinder Kaur, Guru Nanak Dev University, IndiaNathalie Marquez, Instituto Nacional de Tecnología Agropecuaria (Argentina), Argentina
Zaiton Sapak, MARA University of Technology, Malaysia
Copyright © 2025 Penaud, Alahmad, De Vrieze, Bouteiller, Eude, Bernardon-Mery, Trinsoutrot-Gattin, Laval and Gauthier. This is an open-access article distributed under the terms of the Creative Commons Attribution License (CC BY). The use, distribution or reproduction in other forums is permitted, provided the original author(s) and the copyright owner(s) are credited and that the original publication in this journal is cited, in accordance with accepted academic practice. No use, distribution or reproduction is permitted which does not comply with these terms.
*Correspondence: Valentin Penaud, dmFsZW50aW4ucGVuYXVkQHVuaWxhc2FsbGUuZnI=; dmFsZW50aW4ucGVuYXVkMzFAZ21haWwuY29t