- 1Department of Plant and Soil Sciences, University of Pretoria, Pretoria, South Africa
- 2Hans Merensky Chair in Avocado Research, Forestry and Agricultural Biotechnology Institute (FABI), University of Pretoria, Pretoria, South Africa
- 3Department of Biochemistry, Genetics and Microbiology, University of Pretoria, Pretoria, South Africa
- 4Theoretical Biology and Bioinformatics, Department of Biology, Science for Life, Utrecht University, Utrecht, Netherlands
- 5Westfalia iTeam, Westfalia Fruit, Tzaneen, South Africa
Introduction: The avocado rhizosphere supports diverse microbial communities essential for plant health and defence against pathogens. This study aimed to investigate the impact of Dematophora necatrix, the causal agent of white root rot (WRR), on the microbial composition and soil physicochemical properties of infected and non-infected avocado trees in two South African orchards.
Methods: ITS and 16S metabarcoding was used to compare the composition and diversity of the rhizosphere microbiome. Soil physicochemical properties were also assessed, and culturable bacterial and fungal isolates from the rhizosphere were screened for antagonistic activity against D. necatrix.
Results: We found that D. necatrix did not significantly alter overall microbial diversity but influenced relative abundance of specific taxa. In Orchard A, dominant bacterial genera included Sphingomonas, Rokubacteriales and Lysobacter, while Orchard B featured Sphingomonas and Acidothermus while beneficial microbes such as Streptomyces and Bacillus were enriched in WRR non-infected (WRR-N) soils. The fungal profiles revealed Trichoderma and Penicillium as potential biocontrol agents enriched in WRR-N soils. Furthermore, dual-culture assays demonstrated that Bacillus, Pseudomonas, Penicillium and Trichoderma isolates inhibited D. necatrix, highlighting their biocontrol potential. Key parameters, such as soil pH and iron (Fe), correlated strongly with microbial composition, suggesting they play an important role in pathogen resilience.
Discussion: These findings underscore the complexity of the avocado rhizosphere and its role in managing WRR, offering a foundation for developing integrated disease management strategies to enhance avocado productivity.
Introduction
Dematophora necatrix R. Hartig. (formerly Rosellinia necatrix Berl. ex Prill.) (Wittstein et al., 2020) causes white root rot (WRR) with more than 440 reported hosts (https://nt.ars-grin.gov/fungaldatabases/) (ten Hoopen and Krauss, 2006; Pliego et al., 2012; Arjona-López et al., 2024) in temperate and tropical regions globally (Petrini, 1993; Pliego et al., 2012). WRR affects several economically important fruit trees, including coffee (Castro et al., 2013), apples (Pasini et al., 2016), peaches (Dafny-Yelin et al., 2018), almonds (Palacio-Bielsa et al., 2017) and avocados (Pliego et al., 2012; van den Berg et al., 2018). WRR is a major limiting factor for avocado production in Spain (Pliego et al., 2009, 2012) and Israel (Dafny-Yelin et al., 2018). Owing to its global presence, the pathogen spread to the African continent, where it was first reported on apple trees in the Western Cape province of South Africa in the 1970s (van der Merwe and Matthee, 1974) and on avocado in the Limpopo province in 2016 (van den Berg et al., 2018). The pathogen has also been reported in Mozambique (CABI, 2024). WRR is characterized by above- and below-ground symptoms that can develop rapidly in young trees (~2–6 weeks) or gradually in older trees (~6–24 months) depending on environmental conditions. Symptoms include necrosis of the feeder roots, branch dieback, mummified fruits, yellowing and wilting of leaves, and development of white mycelial masses on roots and under the bark (Pliego et al., 2012; Granum et al., 2015). Symptoms progressively worsen leading to tree death, impacting soil health, and affecting the microbiome and physicochemical properties of the soil.
Research has shown the importance of the rhizosphere, a narrow zone of soil surrounding the roots, and endophytic microorganisms in maintaining plant health, which strongly correlates with changes in the microbial community composition in the soil (Qiao et al., 2024). Many root pathogens establish interactions with numerous microbes in the rhizosphere changing microbial diversity and richness (Chapelle et al., 2016). Pathogens like D. necatrix, compete with rhizosphere microbes to infect avocado trees, often producing antimicrobial effectors and toxins to suppress beneficial microbial communities which act as the first line of defense (Chavarro-Carrero et al., 2024). Previous studies in avocado, have also shown that the rhizosphere microbiome composition is significantly affected under pathogen pressure, such as Phytophthora cinnamomi infection (Solís-García et al., 2021; Reverchon et al., 2023). A study on citrus infected with huanglongbing disease, demonstrated that trees with slow disease progression had a higher proportion of beneficial microorganisms, while symptomatic trees were dominated by opportunistic pathogens (Ginnan et al., 2020). This shift led to an abundance of saprophytes in the advanced stages of infection. Thus, the invasion of pathogens in the rhizosphere of tree crops disrupts microbial equilibrium, resulting in dysbiosis.
The rhizosphere is further influenced by root exudates that directly affect microbial composition and diversity (Philippot et al., 2013). These exudates, including sugars, organic acids, secondary metabolites and amino acids, promote the proliferation of beneficial organisms, enhance nutrient availability, offer protection against pathogens, and sustain microbial communities essential for plant growth, health and productivity (Hinsinger et al., 2009; Hawes et al., 2012; Bulgarelli et al., 2013; Sasse et al., 2018). Previous studies have demonstrated the benefits of the rhizosphere microbiome, particularly in protecting avocado against root pathogens like P. cinnamomi (Solís-García et al., 2021; Reverchon et al., 2023), highlighting the importance of the microbial ecosystem in agriculture. In turn, plants devote a portion of their photosynthetically fixed carbon to nourish their associated microbial community (Santos and Olivares, 2021). Therefore, uncovering the relationship between microbial communities and plants is necessary to increase our understanding of disease development in the rhizosphere.
The composition of the rhizosphere microbial community also changes based on the time of planting, which can subsequently affect aerial tissue and the physicochemical properties of the soil (Chen et al., 2022). Such imbalances can contribute toward the aggressiveness of pathogens (Berendsen et al., 2012). The interaction between trees and the rhizosphere microbiome is unique among plants due to the long-lived perennial nature and extensive and deep root system of trees; the longevity of trees further shape the microbial community composition (Mercado-Blanco et al., 2018). Additionally, the composition and function of rhizosphere microbial communities are influenced by factors such as the plant species, cultivar, rootstock, and even the growth stage, and characteristics of the surrounding soil (Berendsen et al., 2012; Sasse et al., 2018; Castellano-Hinojosa et al., 2023). Therefore, the interactions between plants and their rhizosphere microbiomes are highly specific, underlining the need to study different host plants along with their associated microorganisms and environmental conditions.
Given the complex interactions between microbes, the host, and the variable nature of these communities this study aimed to investigate the effects of D. necatrix on the avocado rhizosphere. The study also confirmed the presence of P. cinnamomi, as this root pathogen is known to be present in all avocado growing regions and would also have an impact on the taxa relative abundance and diversity in the rhizosphere of avocado trees. We hypothesized that D. necatrix would change the composition and diversity of the rhizosphere, with beneficial bacteria and fungi being enriched in WRR non-infected trees. To test these hypotheses, we characterized the fungal and bacterial rhizosphere communities of WRR-infected and non-infected avocado trees through metabarcoding and soil physicochemical analyses. This study thereby provides a clearer understanding of the intricate relationship between avocado roots and their rhizosphere microbiome, as well as the effects thereon in the presence of D. necatrix.
Materials and methods
Study site selection and D. necatrix infection assessment
Two orchards in Tzaneen, Limpopo, South Africa (Supplementary Table 1), were previously assessed for the presence of D. necatrix (van den Berg et al., 2025). In short, trees were assessed and assigned a disease severity score, thereby classifying trees as either symptomatic or asymptomatic (Sherwood and Hagedorn, 1958; González-Sánchez et al., 2013). The presence of D. necatrix in the roots of assessed trees was determined using Taqman qPCR, species-specific primers R7 (5′-AACCATAGGCGAGATGAGAAAT-3′) and R10 (5′-CCCCTGTTGCTTAGTGTTGG-3′) (Schena et al., 2002) and the TR10-7 probe (5′-AGTCAGTGGCGGAGTCGGTC-3′) (Shishido et al., 2012). Asymptomatic trees that tested negative for the presence of D. necatrix were reclassified as uninfected. Therefore, trees in both orchards were assigned as either WRR-S (symptomatic, D. necatrix infected), WRR-AS (asymptomatic, D. necatrix infected) or WRR-N (asymptomatic, non-infected).
Rhizosphere soil, rootlets, and feeder roots sample collection
Rhizosphere soil rootlets and feeder root samples were collected from 30 avocado trees in each orchard (Orchards A and B) as described by Guevara-Avendaño et al. (2018). For each tree, approximately 5–20 g soil was collected around the tree, targeting areas where some feeder roots were present. Samples were homogenized to create a single rhizosphere soil sample for each tree. We collected 60 soil samples including feeder roots (10 trees per category per orchard; WRR-S, WRR-AS and WRR-N), samples were kept at 4°C prior to DNA extraction.
Confirmation of the presence of P. cinnamomi in orchard A and B
Rhizosphere soils, rootlets, and feeder root samples were collected for P. cinnamomi detection from both avocado orchards in similar trees (30 avocado trees per orchard) used for the detection of D. necatrix and metabarcoding analysis. Rootlets and feeder roots isolations were made by embedding root pieces in NARPH selective media (20% clarified V8 juice agar containing 50 mg/l nystatin, 200 mg/l ampicillin, 10 mg/l rifampicin, 20 mg/l pentachloronitrobenzene, 50 mg/l hymexazol, and 20 g/l agar) and incubated at 25°C for 3–4 days. Rhizosphere soil samples were prepared for detection of P. cinnamomi using the leaf disc baiting method (Sena et al., 2018). For each sample a 40 ml aliquot of soil was placed in a sterile 50-ml cup, flooded with sterile water, and baited with 5–10 avocado leaf disks (~5 mm in diameter) punched from surface-sterilized (wiped with 70% ethanol) leaves. Cups were incubated in a dark growth chamber at 25°C for 7 days. Following incubation, three leaf disks were placed on two plates containing NARPH selective media and incubated at 25°C for 3–4 days. For further analysis, NARPH cultures were transferred to ½ potato dextrose agar (PDA; 19.5 g/l PDA agar and 10 g/l agar) by excising a block of media where only one organism was growing or selectively transferring the P. cinnamomi mycelia (white fluffy mycelia) to fresh ½ PDA agar plates.
Cultures were incubated, in the dark, at 25°C for 2–3 days and used to excise single hyphal tips. Single hyphal tips were cultured on 2% malt extract agar (MEA; 20 g/l malt extract and 15 g/l agar). A mycelial mass was obtained, freeze-dried, and stored at −80°C. Genomic DNA was extracted from the freeze-dried mycelia using PrepMan Ultra Sample Preparation Reagent (Thermo Fisher Scientific, Waltham, USA) as described by Engelbrecht et al. (2017). The identity of isolates was confirmed by PCR using species-specific primers that amplify the Ypt1 gene (Ycin3F, 5′-GTCCTATTCGCCTGTTGGAA-3′; and Ycin4R, 5′-GGTTTTCTCTACATAACCATCCTATAA-3′) (Schena et al., 2008).
ITS and 16S rRNA metabarcoding
DNA extraction and PCR and sequencing
For metabarcoding, genomic DNA was extracted from the soil using the NucleoSpin® Soil DNA extraction kit (Macherey-Nagel, Düren, Germany), according to the manufacturer's instructions. DNA concentration and purity was assessed using a NanoDrop ND2000C spectrophotometer (Thermo Fisher Scientific, Waltham, USA), visualized on a 1.5% agarose gel, and stored at −20°C until further analysis. The presence of D. necatrix in the DNA extracted from the soil samples was confirmed as previously described.
For microbial community analysis, genomic DNA was sequenced by Inqaba Biotechnical Industries (Pty) Ltd. (Pretoria, South Africa) using PacBio Sequel IIe platform. Bacterial 16S rRNA were amplified using 27F_PB (5′-AGRGTTYGATYMTGGCTCAG-3′) and 1492R_PB (5′-RGYTACCTTGTTACGACTT-3′) primers. The fungal internal transcribed spacer (ITS) region was amplified using the ITS1F_PB (5′-CTTGGTCATTTAGAGGAAGTAA-3′) and ITS4_PB (5′-TCCTCCGCTTATTGATATGC-3′) primers. Barcoding and library preparation was conducted according to the manufacturer's procedures prior to sequencing (Pacific Biosciences®, Menlo Park, USA).
ITS and 16S rRNA metabarcoding read processing and generation of amplicon variants
Preprocessing and annotation of sequence reads
Longer reads resulted in too much variation to reliably identify amplicon sequence variants (ASVs); thus, the reads were trimmed using Cutadapt (Martin, 2011) to the same amplicon length as used in Illumina metabarcoding protocols. The primer sequences used for trimming the ITS region were ITS1 (5′-TCCGTAGGTGAACCTGCGG-3′) and ITS4 (5′-TCCTCCGCTTATTGATATGC-3′) (Solís-García et al., 2021). The 16S rRNA sequences were trimmed using F341 (5′-CCTACGGGNGGCWGCAG-3′) and R805 (5′-GACTACHVGGGTATCTAATCC-3′) primers (Herlemann et al., 2011; Qiu et al., 2020). Reads were filtered, trimmed, and denoised using DADA2 v.1.26.0 (Callahan et al., 2016), setting the parameters minLen = 400, maxLen = 600, maxN = 0, maxEE = 2, and minQ = 2. The reads were then merged, and chimeras (between 6,000 and 40,000 per sample) were removed using DADA2 to obtain ASVs. Taxonomic assignment was performed using the SILVA SSU r138.1 reference database for bacterial taxa (Pruesse et al., 2007; Quast et al., 2013), and the Unite database v10.5.2021 (Nilsson et al., 2019) for fungal taxa using the naïve Bayes classifier in QIIME2 (Bolyen et al., 2019). ASVs with very low read numbers (average relative abundance <1e − 5) were excluded from the dataset using phyloseq v. 3.19 (Mcmurdie and Holmes, 2013). The ASVs abundance tables and taxonomic classification output files from the DADA2 pipeline were used for downstream data exploration, statistical analysis, and visualization. ASVs filtering removed archaeal, eukaryotic, chloroplastic, and mitochondrial reads from the bacterial and fungal datasets.
Analysis of the rhizosphere microbiome using 16S rRNA and ITS metabarcoding
Fungal and bacterial community composition of healthy and WRR infected avocado trees
Following taxonomic classification, community composition was investigated at the genus level, clustering ASVs with a relative abundance > 1e − 5, using the phyloseq package (Mcmurdie and Holmes, 2013). Genera with a relative abundance >0.6% were selected to construct a bar graph displaying abundance patterns across the symptomatic (WRR-S), asymptomatic (WRR-AS), and non-infected (WRR-N) datasets, utilizing ggplot2 (Wickham, 2016).
Diversity analysis of bacterial and fungal communities of the avocado rhizosphere
Richness and evenness (alpha diversity) were determined using Shannon's diversity index (emphasizing species richness), Simpson's index (focussing on evenness), and Chao1 diversity index (for species richness). Significant differences in the alpha diversity across different populations were tested using the Kruskal-Wallis test; p < 0.05, followed by a Wilcoxon rank-sum test; p < 0.05, for pairwise comparisons. The Benjamini-Hochberg method was used to adjust the p-values for multiple comparisons.
Dissimilarities between communities were evaluated based on Bray-Curtis dissimilarity metrices, which consider both composition and abundance of ASVs. Data dispersion homogeneity within each group was confirmed using betadisper, followed by permutational multivariate analysis of variance; p > 0.05, using the Adonis function in the vegan package v.2.6-4 (Oksanen et al., 2022). Pairwise comparisons to identify significantly different populations were performed using the pairwise Adonis package, with p-values adjusted using the Benjamini-Hochberg method (Martínez Arbizu, 2020). In addition, distances were used to construct a non-metric multidimensional scaling ordination plot to visualize the clustering of individuals from all populations. All statistical analyses were performed with R v.4.0.3 (R Core Team, 2021) in RStudio (RStudio Team, 2021).
Enrichment of specific bacterial and fungal genera
We investigated the enrichment of fungal and bacterial genera among WRR-S, WRR-AS, and WRR-N categories. We employed DESeq2 v 1.30.1 (Love et al., 2014) in RStudio (RStudio Team, 2021), to identify genera that were significantly enriched. Results were filtered using an adjusted p-value (padj) of ≤ 0.05 and a log2 fold change of ±1. Genera were further filtered with Benjamini-Hochberg-corrected p-values < 0.001 in RStudio (RStudio Team, 2021).
Unique bacterial and fungal genera between WRR infected and non-infected avocado trees
We investigated unique genera within WRR-S, WRR-AS, and WRR-N categories. We identified bacterial and fungal genera with a relative abundance of 0.001. For analyses and visualization of intersections, UpSet plot graphs were created in RStudio (RStudio Team, 2021) using the UpSetR package v. 1.4.0 (Conway et al., 2017).
Rhizosphere soil physicochemical properties of two avocado orchards
Soil samples were collected from Orchards A and B, specifically targeting the rhizosphere soil around the tree trunk. Ten replicated samples were collected for each of the groups (WRR-S, WRR-AS and WRR-N) in two orchards. Rhizosphere soil physicochemical properties were analyzed at the Department of Plant and Soil Sciences Laboratory, University of Pretoria, South Africa. Details of the analyses used were outlined previously (van den Berg et al., 2025). Data were subjected to one way ANOVA and Tukey's test to determine significant differences at p ≤ 0.05.
The relationship between rhizosphere soil physicochemical properties and microbial community composition
The physicochemical properties and microbial communities of the rhizosphere soil were analyzed using principal component analysis (PCA) with R v.4.0.3 (R Core Team, 2021) in RStudio (RStudio Team, 2021). Variables representing physicochemical properties were included as meta-variables to explore their interactions with microbial communities. To perfom the PCA analyses, we selected the top (0.6%) fungal and bacterial genera from each category of trees in both orchards.
Assessing the in vitro antagonistic effect of fungi and bacteria isolated from the avocado rhizosphere
Isolation of culturable fungi and bacteria from rhizosphere soil
Rhizosphere soil samples obtained from WRR-N trees in Orchard B were used to isolate culturable fungi and bacteria. Bacteria and fungi were initially isolated using a serial dilution. Fungi were isolated by diluting soil samples up to 10−3, plating on ½ PDA with antibiotics (Ampicillin (200 μl/ml), Rifampicin (10 μl/ml) and Streptomycin (50 μl/ml)) and incubating at 25°C in the dark until growth appeared. Pure fungal cultures were obtained by transferring hyphal tips from the edges of colonies to fresh PDA. Fungal isolates were categorized into morphotypes based on macroscopic and microscopic features, with conidial shape, color, and size characterized using 25 conidia per isolate. To isolate bacteria, soil samples were diluted up to 10−5 and plated on Luria-Bertani (LB) medium (10 g/l tryptone, 5 g/l yeast extract, and 5 g/l NaCl). The plates were incubated in the dark at 25°C for 1–2 days. Pure cultures were obtained by selecting single colonies and streaking them on fresh LB agar plates.
Screening and determination of antagonistic effect of culturable bacteria and fungi
Initially, approximately 60 fungal and 100 bacterial isolates were screened in vitro for their ability to inhibit D. necatrix growth using the isolate ARP-2017-Rn2, a South African isolate previously shown to be highly virulent on avocado (van den Berg et al., 2018). Those isolates that showed some levels of inhibition (20 fungal and 22 bacterial isolates) were selected for further testing using dual-culture assays. The D. necatrix isolate was grown on ½ PDA at 25°C in the dark for 7 days. For fungal dual-culture assays, a 5-mm agar plug from a 5-day-old fungal culture was placed opposite a 5-mm plug from a 7-day-old D. necatrix culture (20 mm from the plate periphery) on a 90-mm Petri dish containing ½ PDA. For bacterial isolates, a 5-mm agar plug from a 7-day-old D. necatrix culture was placed in the center of each plate, and single bacterial colonies from 5-day-old cultures were positioned at four equidistant locations, 20 mm from the periphery. Each fungal and bacterial isolate was tested on a separate plate, with three replicates each. Control plates contained only D. necatrix. All plates were incubated at 25°C in the dark for 8 days, and the radial growth of D. necatrix was measured. The percentage inhibition (PI) of mycelial growth was calculated using Equation 1, where C is the diameter (mm) of the radial growth of D. necatrix in the control, and T is the diameter (mm) of the radial growth of D. necatrix in the presence of antagonistic strains. All analyses were performed in R v.4.0.3 (R Core Team, 2021) using RStudio (RStudio Team, 2021).
Molecular identification of bacterial and fungal isolates
Fungi and bacteria were identified by sequencing the ITS and 16S rRNA regions, respectively. DNA was extracted from one fungal isolate per morphotype (n = 10) using the CTAB protocol (Brunner et al., 2001). For bacteria, a single colony was added to 50 μl CTAB without grinding. Sequencing was performed using specific primers: fungal ITS sequencing using ITS1 and ITS4 primers (Solís-García et al., 2021), and bacterial 16S rRNA amplification using F341 and R805 primers (Herlemann et al., 2011; Qiu et al., 2020). The EmeraldAmp® GT PCR Master Mix (Takara Bio Inc., Kusatsu, Japan) was used with the following cycling conditions: 98°C for 1 min, 35 cycles at 98°C for 10 s, 60°C for 30 s, 72°C for 1 min, followed by 72°C for 1 min. Amplicons were purified using the EXO-SAP protocol and sequenced on an ABI 3500xl genetic analyser (Thermo Fisher Scientific, Waltham, USA).
Sequences were trimmed and consensus sequences were constructed using the CLC Main Workbench 22.0.1 (QIAGEN, Hilden, Germany). These sequences were then identified through Basic Local Alignment Search Tool (BLAST) analysis. This step involved comparing the obtained sequences to known sequences in NCBI GenBank (Sayers et al., 2020) to determine the species of fungi and bacteria. Data from these analyses, were compiled and visualized using R v.4.0.3 (R Core Team, 2021) and RStudio (RStudio Team, 2021).
Results
Confirmation of the presence of D. necatrix and P. cinnamomi in two avocado orchards
The qPCR analysis confirmed the presence of D. necatrix in infected samples. Based on these data, rhizosphere soil and root samples from 30 trees in both Orchards A and B were grouped into three categories: WRR-S (symptomatic and D. necatrix infected), WRR-AS (asymptomatic and D. necatrix infected), or WRR-N (asymptomatic and non-infected) with each category comprising of 10 trees per orchard (Table 1, Supplementary Table 2). As expected, P. cinnamomi was present in all 60 trees across the two orchards.
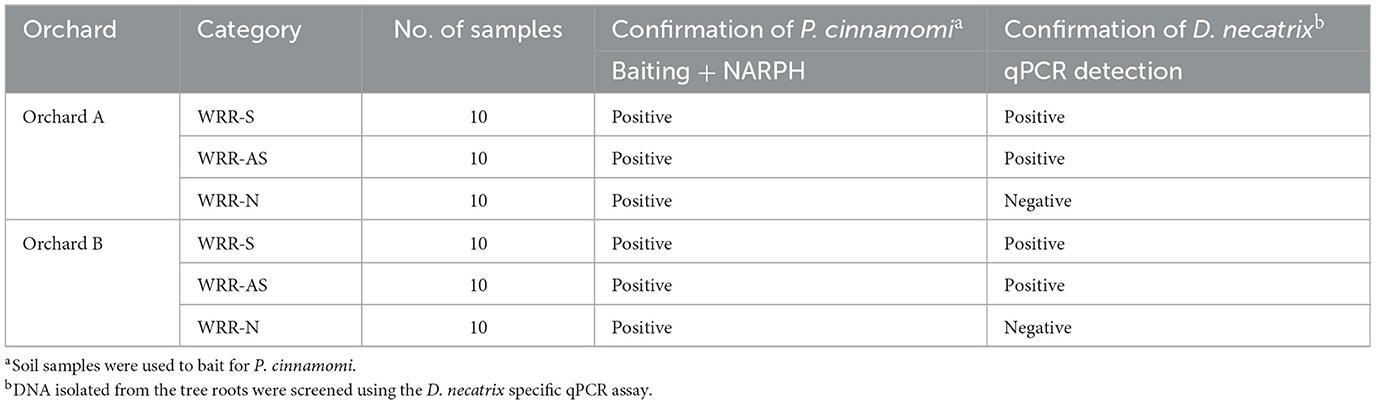
Table 1. Infection status of trees based on the detection of the root rot pathogens Phytophthora cinnamomi and Dematophora necatrix.
Diversity analysis of bacterial and fungal communities in rhizosphere soil from two avocado orchards
Following quality filtering, trimming and chimera removal, 8 310 bacterial and 1 919 fungal ASVs were obtained from Orchard A, and 9 656 bacterial and 1 771 fungal ASVs were obtained from Orchard B (Table 2). Sequences were submitted to the GenBank NCBI database under BioProject PRJNA1149920 (https://www.ncbi.nlm.nih.gov/bioproject/).
Alpha diversity indices (Chao1, Shannon, and Simpson; Figure 1) for bacterial and fungal communities in soil samples from Orchards A and B showed no significant differences across WRR-N, WRR-AS, and WRR-S categories, with one exception: in Orchard B, the bacterial Simpson index differed significantly between WRR-N and WRR-S (Figure 1B). The WRR-N trees demonstrated lower bacterial diversity than what was observed for WRR-S trees. Beta diversity, assessed using Bray-Curtis distances, also revealed no significant differences or clustering between bacterial and fungal communities across categories in either orchard (Supplementary Figure 1). Overall, the presence of D. necatrix and P. cinnamomi did not significantly impact microbial community diversity in the avocado rhizosphere.
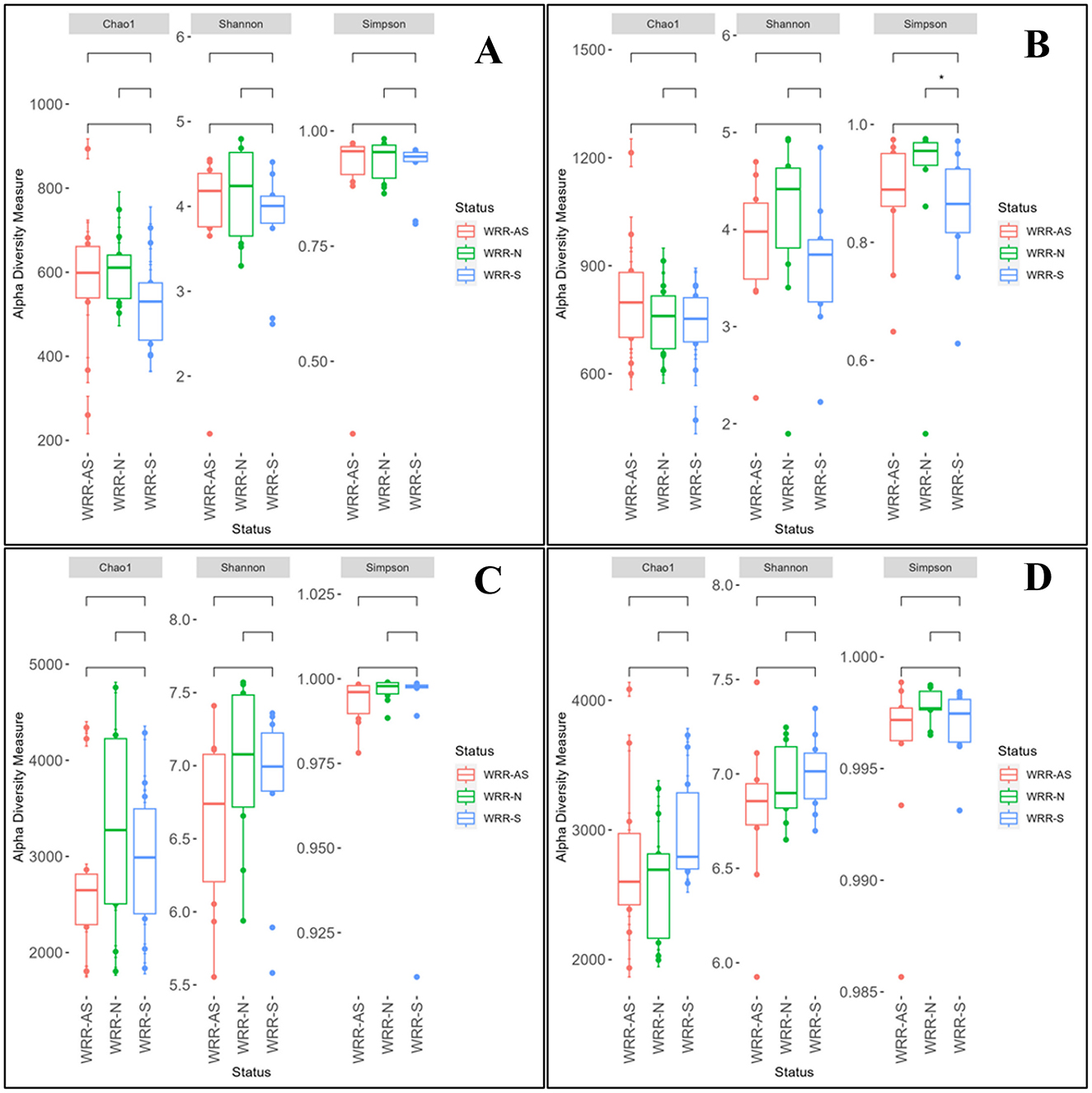
Figure 1. Alpha diversity of the microbiome of bacterial and fungal communities in the avocado rhizosphere. Samples were collected from soil around white root rot asymptomatic (WRR-AS), white root rot non-infected (WRR-N), and white root rot symptomatic (WRR-S) trees. The graphs represent alpha diversity indices for Chao1 diversity, Shannon diversity, and Simpson diversity of bacteria in Orchard A (A) and Orchard B (B); and fungi in Orchard A (C) and Orchard B (D). Horizontal bars within boxes represent the median. The top and bottom of boxes represent 75th and 25th quartiles, respectively. The upper and lower whiskers represent the range of non-outlier data values. The outliers were plotted as individual points. The star indicated significant differences among respective groups based on two-sided tests by Wilcoxon rank-sum test followed by Tukey multiple comparison test (Benjamini–Hochberg method adjusted p < 0.05).
Bacterial community composition of two avocado orchards
The community composition of bacteria in both orchards at phylum level was dominated by Actinobacteria, Acidobacteria, Chloroflexi, Gemmatimonadota, Proteobacteria, and Plactomycetota (Supplementary Figure 2). The bacterial communities exhibited similar dominant genera (>0.6%) across the WRR-S, WRR-AS, and WRR-N categories in both Orchard A and B (Figure 2). However, the number of ASVs across bacterial taxonomic classifications (phyla, class, order, family, and genus) varied between the two orchards (Supplementary Table 3).
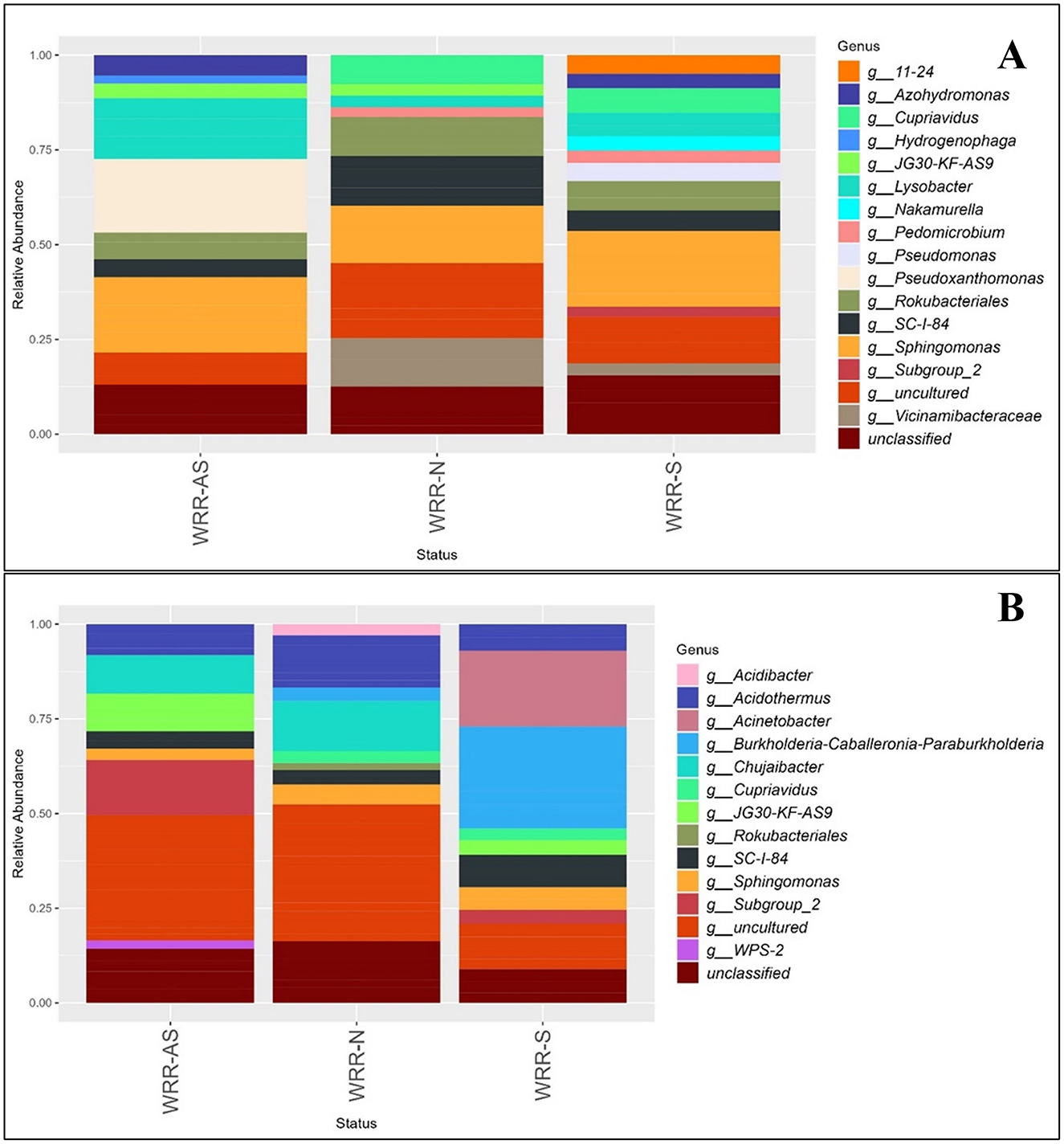
Figure 2. Relative abundance bar chart of the most abundant genera of the bacterial community in the rhizosphere of samples collected in (A) Orchard A and (B) Orchard B. Samples were collected from soil around white root rot asymptomatic (WRR-AS), white root rot non-infected (WRR-N), and white root rot symptomatic (WRR-S) trees. The most dominant genera above 0.6% were identified using 16S metabarcoding data. Unclassified indicates the proportion of ASVs which could not be described to genus level.
In Orchard A, Sphingomonas, Rokubacteriales, SC-I-84 and Lysobacter were abundant across all three tree categories (Figure 2A). Pseudoxanthomonas was the most prominent genus in the WRR-AS samples, but did not feature amongst the most dominant genera in either WRR-N or WRR-S. WRR-S samples featured Pseudomonas, Nakamurella, Subgroup_2 and 11-24, which were not amongst the most dominant genera in either WRR-AS or WRR-N samples. Pedomicrobium was one of the most dominant genera in WRR-N and WRR-S but did not feature in WRR-AS samples.
In Orchard B, Sphingomonas, SC-I-84, and Acidothermus was amongst the most dominant genera across all categories (Figure 2B). WRR-AS samples were dominated by genera Subgroup_2 and Chujaibacter, as well as WPS-2 which was not dominant in WRR-N or WRR-S. In contrast, the WRR-S sample profiles featured Acinetobacter and Burkholderia-Caballeronia-Paraburkholderia (BCP) as the most prominent genera. BCP was previously classified under Burkholderia, but has now been reclassified into separate genera.
In both orchards, uncultured and unclassified genera were present across all categories. WRR-N samples from both orchards shared similar dominant genera, while WRR-S and WRR-AS samples displayed more distinct bacterial community compositions.
Fungal community composition of two avocado orchards
The community composition of fungi at the phylum level in both orchards was dominated by Basidiomycota, Ascomycota, and Rozellomycota (Supplementary Figure 3). Fungal communities showed no significant shifts in dominant genera (>0.6%) across the WRR-S, WRR-AS and WRR-N categories (Figure 3). However, the number of ASVs varied across fungal taxonomic groups between the orchards (Supplementary Table 3).
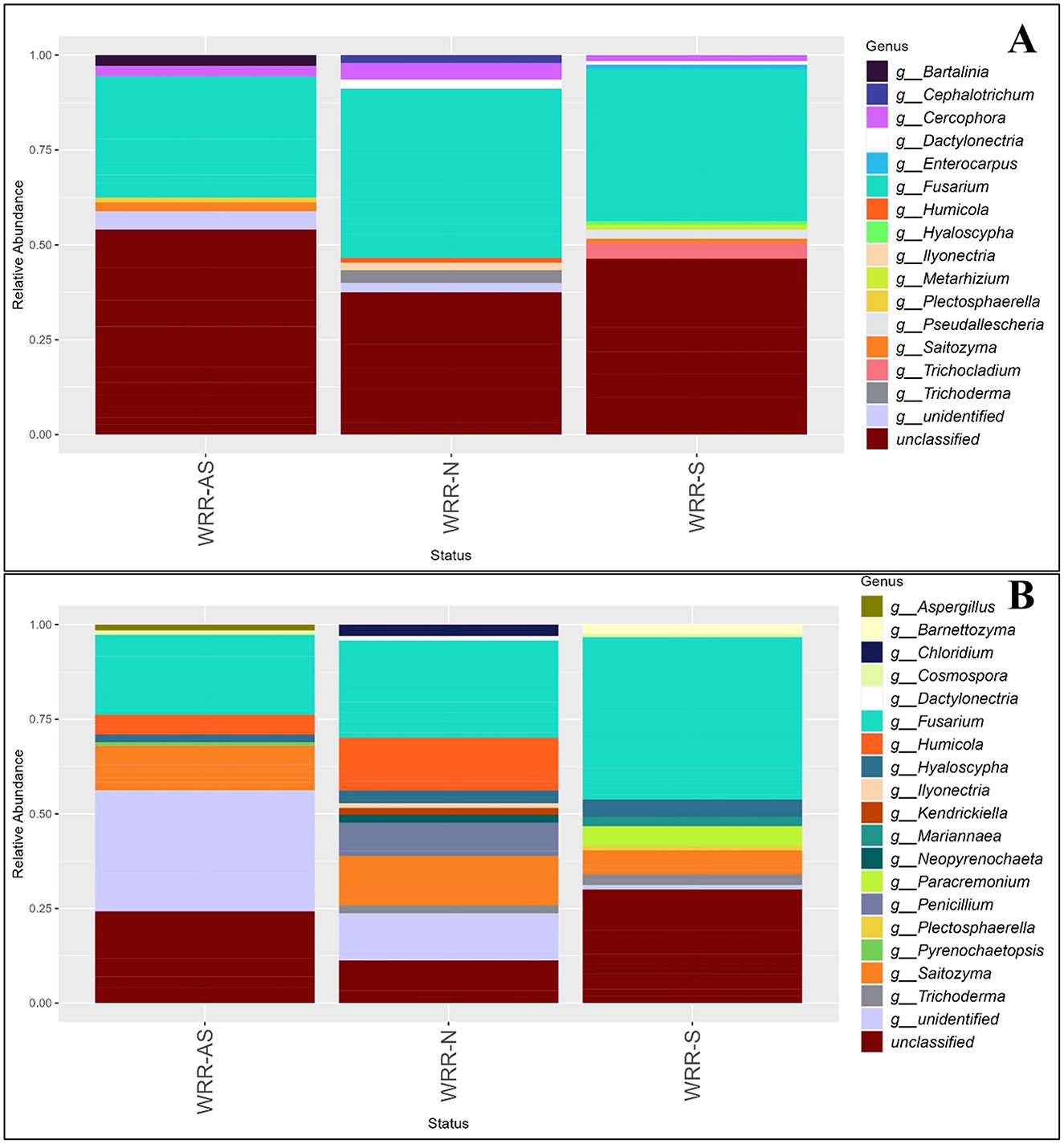
Figure 3. Relative abundance bar chart of the genera of the fungal community in the rhizosphere of samples collected in (A) Orchard A and (B) Orchard B. Samples were collected from soil around white root rot asymptomatic (WRR-AS), white root rot non-infected (WRR-N), and white root rot symptomatic (WRR-S) trees. The most dominant genera above 0.6% were identified using ITS metabarcoding data. Unclassified indicates the proportion of ASVs which could not be described to genus level.
In Orchard A, Fusarium and Cercophora were found amongst the dominant genera across all categories, with Fusarium being the most dominant (Figure 3A). Trichoderma, Ilyonectria, Humicola and Cephalotrichum featured among the dominant genera in WRR-N, but not in the WRR-AS and WRR-S samples. Unique dominant genera which featured in the WRR-S samples included Trichocladium, Metarhizium, Enterocarpus and Pseudallescheria; while WRR-AS featured Bartalinia. Dactylonectria was dominant genera in both WRR-N and WRR-S samples.
Again Fusarium was the dominant genus in all three tree categories from Orchard B, with Saitozyma and Hyaloscypha also featuring across all categories (Figure 3B). Notably, Penicillium and Chloridium were among the dominant genera in the WRR-N category, but were not dominant in the WRR infected categories. WRR-S samples featured Barnettozyma, Mariannaea, Cosmospora, Plectosphaerella and Paracremonium; but Humicola did not feature among the dominant genera as it did in WRR-AS and WRR-N. The WRR-N samples showed the greatest fungal diversity among the dominant genera, with Penicillium, Neopyrenochaeta, Kendrickiella, Illyonectria and Dactylonectria being dominant in WRR-N only.
Enrichment of specific bacterial and fungal genera in two avocado orchards
Bacterial genera in both orchards exhibited distinct enrichment patterns when comparing communities between the WRR-S, WRR-AS and WRR-N trees (Figure 4). In Orchard A, a comparison between WRR-S and WRR-N revealed 11 significant genera (Figure 4A). WRR-S was notably enriched in Pseudomonas, in high relative abundance, as well as Flavobacterium and Saccharococcus both present in lower relative abundance. In contrast, WRR-N samples showed enrichment for Streptomyces, Bacillus and Chujaibacter. When comparing WRR-S and WRR-AS, nine significant genera were observed (Figure 4B). WRR-S samples were enriched with Acidovorax, Pseudomonas, Comamonas, and Flavobacterium; while WRR-AS samples were enriched with Pseudoxanthomonas, Bacillus and Streptomyces. A comparison of WRR-AS and WRR-N revealed 24 significant genera (Figure 4C). WRR-AS samples were enriched for Pseudoxanthomas, Acidovorax, Azohydromonas, Hydrogenophaga and Stenotrophomonas; while WRR-N was enriched with Streptomyces which also showed a high relative abundance compared to Saccharococcus, PB19 and MND1. Thus, in Orchard A, WRR-N trees were found to be enriched for Streptomyces and Bacillium species when compared to WRR-infected trees.
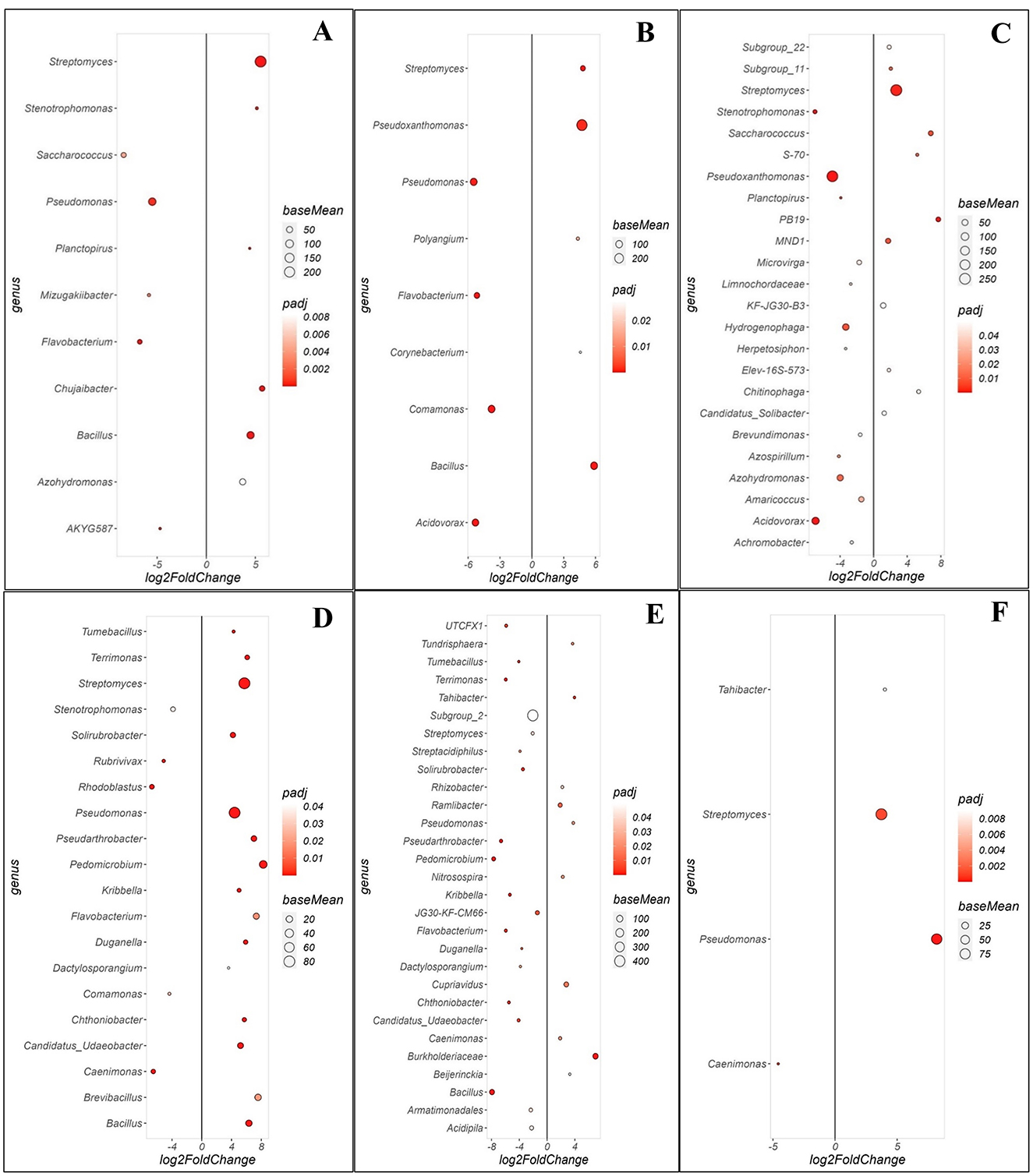
Figure 4. Comparison of specific bacterial genera that were either enriched or depleted between different groups of soil samples collected in the rhizosphere in Orchards A and B. Comparison of rhizosphere soil samples between (A) WRR-S vs. WRR-N, (B) WRR-S vs. WRR-AS, (C) WRR-AS vs. WRR-N from Orchard A and (D) WRR-S vs. WRR-N, (E) WRR-S vs. WRR-AS, (F) WRR-AS vs. WRR-N from Orchard B. The group mentioned first was used as a reference when determining statistical differences; in the figure it will always have a positive log2fold change and thus be enriched in the reference group. The fold-change is shown on the X-axis and the genera are listed on the Y-axis. Each colored dot represents a genus population that is significantly more or less abundant (p ≤ 0.05). The size of the circle shows the average abundance, and the color intensity of the circle shows padj value (significance level). A larger circle indicates higher relative abundance in each population.
In Orchard B, the comparison between WRR-S and WRR-N identified 20 significant genera (Figure 4D). WRR-S samples showed enrichment in Rhodoblastus, Comamonas, and Rubrivivax all in low abundance, while WRR-N samples were enriched in Streptomyces, Pseudomonas, Pedomicrobium and Bacillus. The comparison between WRR-S and WRR-AS revealed 29 significant genera (Figure 4E). In contrast to what was observed in Orchard A, WRR-S samples in Orchard B were enriched for Bacillus when compared to WRR-AS. WRR-AS was enriched in Burkholderaceae, Cupriavidus, and Ramlibacter although all in low relative abundance. When WRR-AS was compared to WRR-N, four significant genera were observed, with Streptomyces and Pseudomonas enriched in WRR-N (Figure 4F). As seen in Orchard A, WRR-N trees were found to be enriched for Streptomyces as compared to WRR-infected trees. In contrast to Orchard A, WRR-N in Orchard B was enriched for Pseudomonas when compared to both WRR-AS and WRR-S.
Fungal community comparisons revealed distinct enrichment patterns across the WRR-S, WRR-AS and WRR-N categories (Figure 5). When comparing WRR-S and WRR-N in Orchard A, 27 significant fungal genera were identified (Figure 5A). The WRR-S tree communities were enriched for Papiliotrema and Metarhizium in high relative abundance as well as Alternaria and Cladosporium in lower relative abundance. Nineteen genera were enriched in WRR-N including Trichoderma, Penicillium and Ilyonectria in high relative abundance while Aspergillus, Lasiodiplodia, Verticillium and Mortierella occured in lower relative abundance. The WRR-S vs. WRR-AS comparison identified 14 significant genera (Figure 5B). WRR-S showed enrichment in Papiliotrema and Furcasterigmium while WRR-AS samples were enriched in Trichoderma, Botryotrichum Lasiodiplodia, Myrmecridium, and Penicillium. The WRR-AS vs. WRR-N comparison revealed 21 enriched genera (Figure 5C), with WRR-N enriched in Verticillium, Trichoderma, Mortierella, Lasiodiplodia, Ilyonectria Aspergillus.
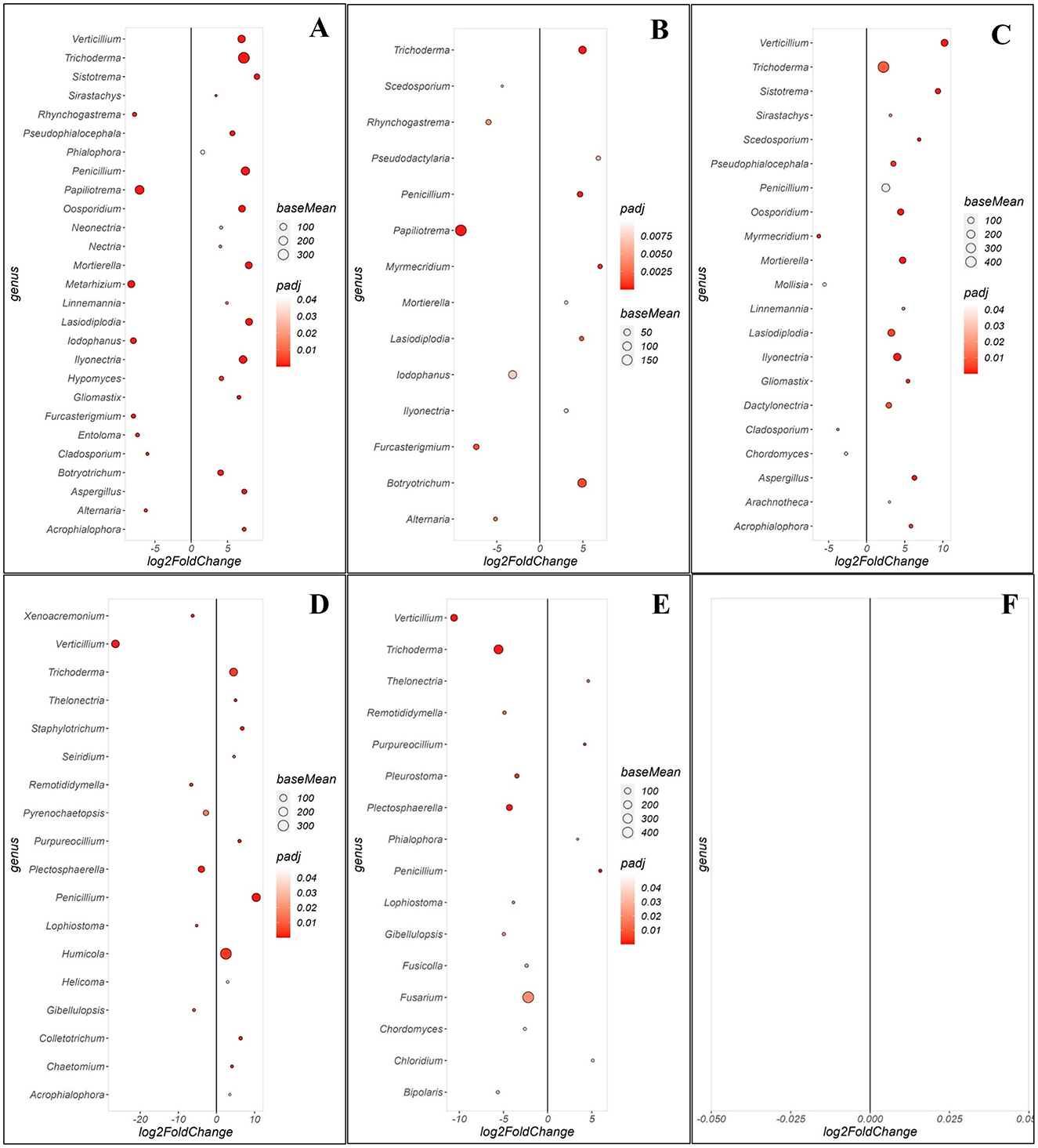
Figure 5. Comparison of specific fungal genera that were either enriched or depleted between different groups of soil samples collected in the rhizosphere at Orchards A and B. Comparison of rhizosphere soil samples between (A) WRR-S vs. WRR-N, (B) WRR-S vs. WRR-AS, (C) WRR-AS vs. WRR-N from Orchard A and (D) WRR-S vs. WRR-N, (E) WRR-S vs. WRR-AS, (F) WRR-AS vs. WRR-N from Orchard B. The group mentioned first was used as a reference when determining statistical significant differences; nj in the figure it will always have a positive log2fold change and thus be enriched in the reference group. The fold-change is shown on the X-axis and the genera are listed on the Y-axis. Each colored dot represents a genus population that is significantly more or less abundant (p ≤ 0.05). The size of the circle shows the average abundance, and the color intensity of the circle shows padj value (significance level). A larger circle indicates higher relative abundance in each population.
In Orchard B, the WRR-S vs. WRR-N comparison identified 18 significant fungal genera (Figure 5D). WRR-S was enriched with Verticillium, Plectosphaerella and Pyrenochaetopsis. In turn, WRR-N was enriched for Humicola, Trichoderma and Penicillium and these genera were all present in high relative abundance. The WRR-S vs. WRR-AS comparison identified 16 significant fungal genera (Figure 5E). WRR-S was enriched in Fusarium, Verticillium, and surprisingly Trichoderma which showed a high relative abundance in the WRR-S samples. WRR-AS was enriched in Penicillium, albeit in low relative abundance. However, the comparison between WRR-AS vs. WRR-N did not identify any significant fungal genera (Figure 5F). These results highlight the distinct fungal community shifts between soil categories in both orchards, but with some specific genera showing the same enrichment patterns across both orchards, such as Trichoderma and Penicillium in WRR-N as compared to WRR-S.
Unique bacterial and fungal genera identified in the microbiomes of WRR-symptomatic, WRR-asymptomatic, and WRR-non infected avocado trees
We employed an UpSet plot to assess the number of unique genera in both the fungal and bacterial communities of the two orchards (Supplementary Figure 4), revealing notable differences between Orchard A and B. In Orchard A, there were 12 unique bacterial genera in WRR-S, 20 in WRR-AS and 25 in WRR-N (Supplementary Figure 4A, Supplementary Table 4). In Orchard B, there were 26 unique bacterial genera in WRR-S, 18 in WRR-AS and 21 in WRR-N (Supplementary Figure 4B, Supplementary Table 5). For unique fungal genera, Orchard A had 14 in WRR-S, 13 in WRR-AS and 22 in WRR-N (Supplementary Figure 4C, Supplementary Table 6). In Orchard B, 25 fungal genera were unique in WRR-S, 16 in WRR-AS and 21 in WRR-N (Supplementary Figure 4D, Supplementary Table 7).
Additionally, specific genera were unique, enriched and highly abundant within particular tree categories. Chitinophaga (Figure 4C, Supplementary Table 4); as well as Gliomastix and Linnemannia (Figure 5C, Supplementary Table 5) were found to be enriched, abundant and unique within the WRR-N samples from Orchard A. In Orchard B, Acrophialophora (Figure 5D, Supplementary Table 7) was enriched and unique to WRR-N. Similarly for genera within the WRR-S samples, UTCFX1 (Figure 4E, Supplementary Table 5) and Lophiostoma, Xenoacremonium, Gibellulopsis and Verticillium (Figure 5D, Supplementary Table 7) were found to be enriched, abundant and unique in Orchard B. These results highlight significant variability in microbial community composition between orchards and categories, with key genera exhibiting potential ecological or functional relevance.
Rhizosphere soil physicochemical properties of two avocado orchards
The physicochemical properties of rhizosphere soil from Orchards A and B were analyzed to evaluate the impact of D. necatrix on soil health (Table 3, Supplementary Tables 8, 9). Potassium (K) levels was significantly lower in Orchard A and significantly higher in Orchard B in WRR-S samples compared to WRR-AS or WRR-N. Low Phosphorus (P) levels were observed in the soil samples, with the lowest concentrations observed in WRR-S samples. In Orchard A, nitrogen (N) and magnesium (Mg) were significantly higher in healthy (WRR-N) samples than infected samples, while sodium (Na) and zinc (Zn) levels declined with increasing disease severity. Conversely, in Orchard B, pH, Na, and Zn were significantly higher in healthy samples (WRR-N) compared to WRR-S samples. The variability in soil properties between the two orchards highlights the potential influence of orchard-specific management practices, which appear to play a more critical role in shaping soil physicochemical characteristics than the direct effects of D. necatrix.
The relationship between rhizosphere soil physicochemical properties and microbial community composition
To investigate the association between soil physicohemical properties and microbial communities, dominant fungal and bacterial genera (>0.6%) were selected for principal component analysis (PCA). The analysis revealed distinct patterns in microbial associations and soil properties across Orchards A and B (Figure 6), with variations contributing to the observed clustering. In Orchard A, the analysis showed that dimension 1 and 2 explained 23 and 20.2% of the total variance (Figure 6A). WRR-AS samples (in black) clustered negatively along Dim1, with correlations with Acinetobacter (An) and Cupriavidus (Cp), as well as Al. WRR-S samples (in green) clustered negatively on Dim1 but varied across Dim2, correlating positively with N-NO3, N, K, EC, and the Burkholderia-Caballeronia-Paraburkholderia complex (Bu). WRR-N samples (in blue) clustered positively on Dim1, and associated with pH, Mg, Na, Zn, N-NO2, Mn, available P, Cu, Fe, and bacterial genera like Sphingomonas (Sp) and Chujaibacter (Ch).
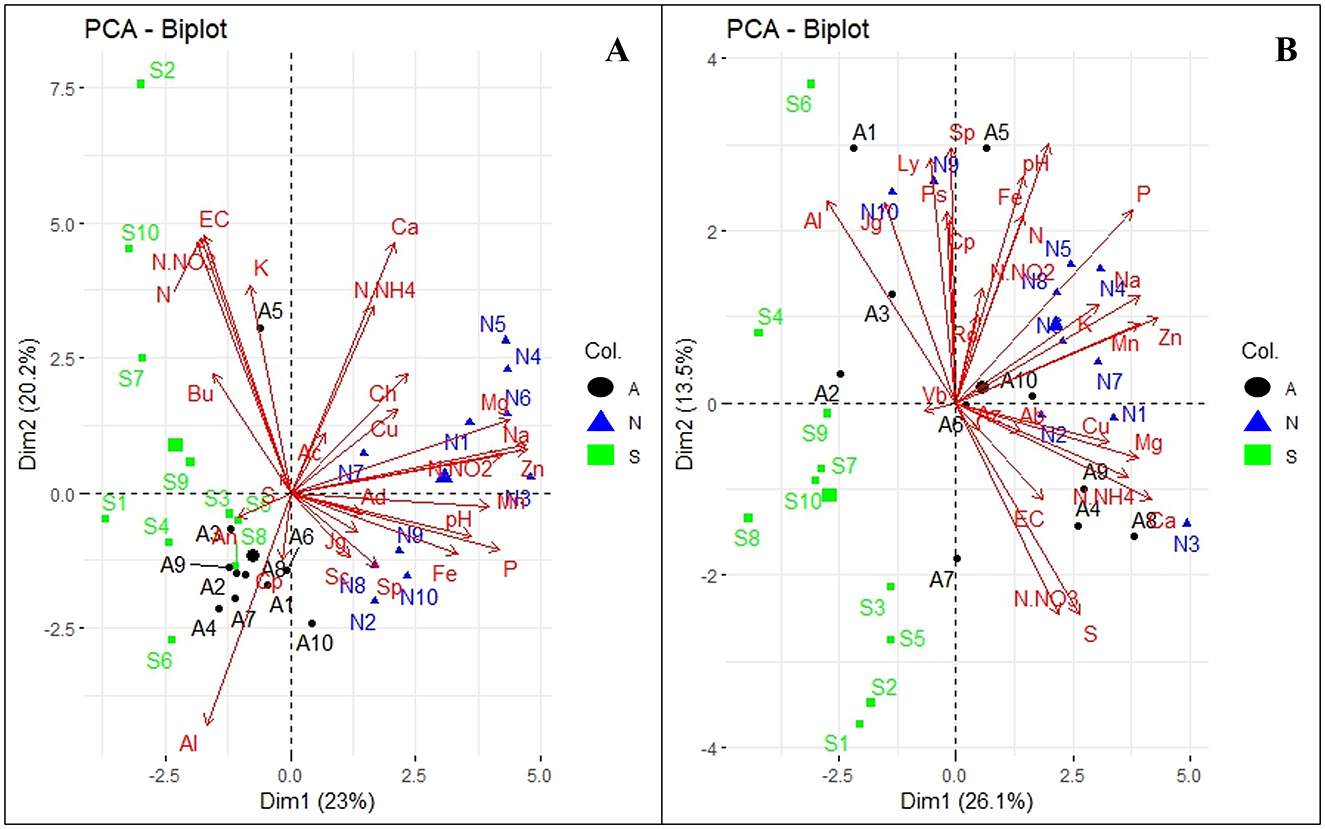
Figure 6. Principal Component Analysis (PCA) of abundant bacterial genera and soil physicochemical properties. (A) Orchard A and (B) Orchard B. The tree categories: WRR-S (S in green), WRR-AS (A in black) and WRR-N (N in blue). The top 0.6% bacterial genera: Acidibacter (Ac), Acidothermus (Ad), Acinetobacter (An), Burkholderia-Caballeronia-Paraburkholderia (Bu), Chujaibacter (Ch), Cupriavidus (Cp), JG30-KF-AS9 (Jg), SC-I-84 (Sc), Sphingomonas (Sp), 11-24 (Ab), Azohydromonas (Az), Lysobacter (Ly), Pseudomonas (Ps), Rokubacteriales (Ro) and Vicinamibacteraceae (Vb). Soil physicochemical properties: exchangeable cations (EC), potential of hydrogen (pH), Nitrogen dioxide (N-NO2), Nitrate Nitrogen (N-NO3), Ammonium nitrate (N-NH4), Nitrogen (N), Potassium (K), Phosphorus (P), Magnesium (Mg), Calcium (Ca), Sodium (Na), Sulphur (S), Aluminium (Al), Copper (Cu), Manganese (Mn), Iron (Fe), Zinc (Zn). The arrow lengths in the plot represent the association strength between the soil physicochemical properties and the bacterial genera (the longer the arrows, the stronger the association). The perpendicular distance between microbes and soil physicochemical properties axes reflects their correlation (the smaller the distance, the stronger the association).
In Orchard B, the PCA of thee bacterial genera and soil propeerties showed that the two dimensions combined contributed 39.6% of the variability observed in the dataset (Figure 6B). WRR-S samples (in green) clustered primarily on the negative side of Dim1 and Dim2, indicating a negative association with soil properties and bacterial genera. In contrast, WRR-N (in blue) and WRR-AS samples (in black) clustered positively along Dim1, demonstrating strong associations with soil factors such as pH, Fe, N, available P, exchangeable K, Mg, Ca, Na, Zn, Mn, Cu, S, N-NH4, N-NO3, and EC. These samples also correlated with bacterial genera such as Azohydromonas (Az), and members of the Rokubacteriales (Ro) order.
For fungal genera in Orchard A, Dim1 and Dim2 explained 10.8 and 21.9% of the total variance, respectively (Figure 7A). WRR-S samples (in green) were associated with genera such as Ilyonectria (Iy), Bartalinia (Ba), Cephalotrichum (Ct), and Cercophora (Ce). In contrast, WRR-N (in blue) and WRR-AS samples (in black) clustered positively along Dim2, correlating with fungal genera including Fusarium (Fu), Enterocarpus (En), Trichocladium (Tc), Dactylonectria (Da), Trichoderma (Tr), Pseudallescheria (Pu), Metarhizium (Me) and Humicola (Hu), as well as soil properties like pH, P, N, Fe, Na, Mn, Zn, and K.
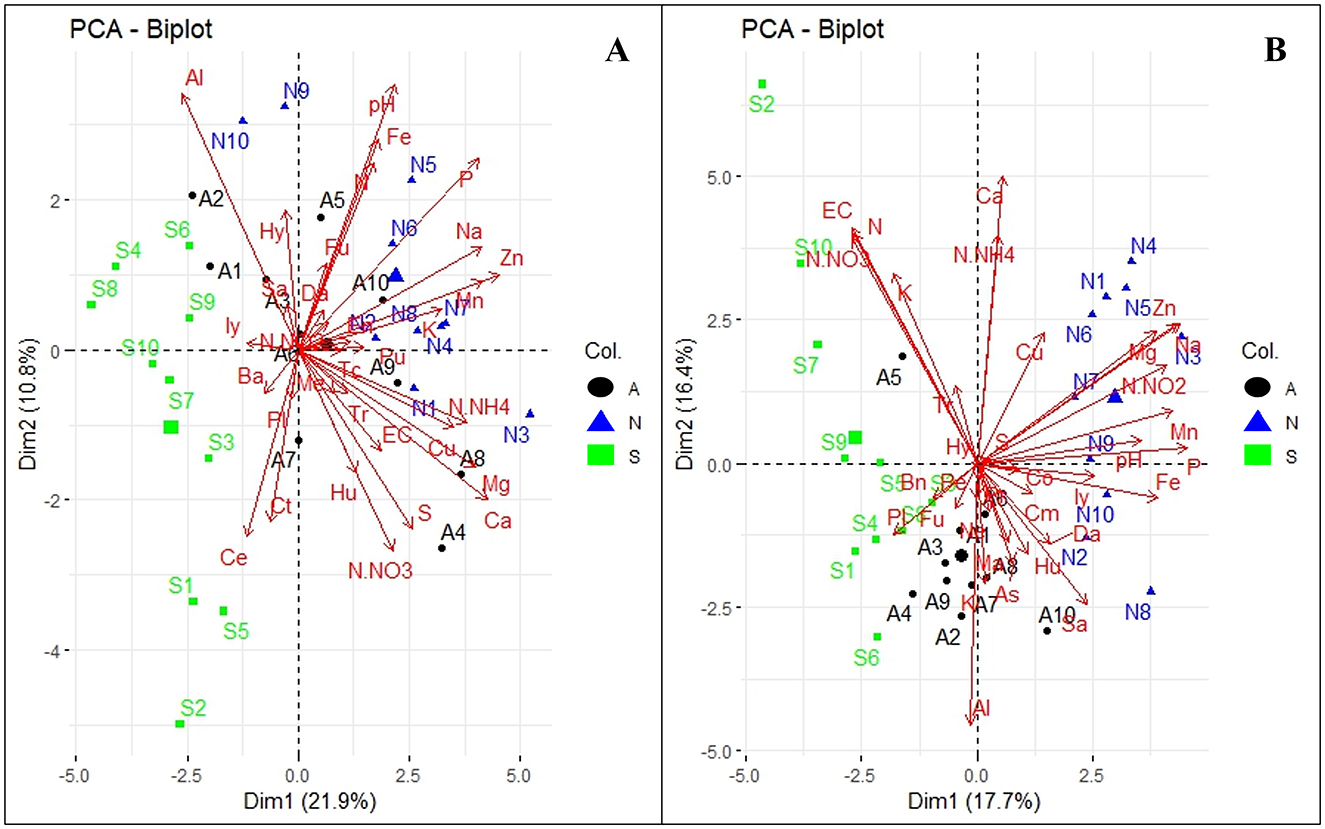
Figure 7. Principal Component Analysis (PCA) of abundant fungal genera and soil physicochemical properties. (A) Orchard A and (B) Orchard B. The tree categories: WRR-S (S in green), WRR-AS (A in black) and WRR-N (N in blue). The top 0.6% fungal genera: Bartalinia (Ba) Cephalotrichum, (Ct), Cercophora (Ce), Dactylonectria, (Da), Enterocarpus (En), Fusarium (Fu), Humicola (Hu), Hyaloscypha (Hy), Ilyonectria (Iy), Metarhizium (Me), Plectosphaerella (PI), Pseudallescheria (Pu), Saitozyma (Sa), Trichocladium (Tc), Trichoderma (Tr), Aspergillus (As), Barnettozyma (Bn), Chloridium (Cm), Cosmospora (Co), Kendrickiella (KI), Mariannaea (Ma), Neopyrenochaeta (Ne), Paracremonium (Pr), and Penicillium (Pe). Soil physicochemical properties: exchangeable cations (EC), potential of hydrogen (pH), Nitrogen dioxide (N-NO2), Nitrate Nitrogen (N-NO3), Ammonium nitrate (N-NH4), Nitrogen (N), Potassium (K), Phosphorus (P), Magnesium (Mg), Calcium (Ca), Sodium (Na), Sulphur (S), Aluminium (Al), Copper (Cu), Manganese (Mn), Iron (Fe), Zinc (Zn). The arrow lengths in the plot represent the association strength between the soil physicochemical properties and the fungal genera (the longer the arrows, the stronger the association). The perpendicular distance between microbes and soil physicochemical properties axes reflects their association (the smaller the distance, the stronger the association).
Orchard B exhbited similar fungal profiles, with dimensions accounting for 16.4% and 17.7% of the total variance, respectively (Figure 7B). WRR-S samples (in green) clustered negatively along Dim1 and were associated with N-NO3, N, K, and EC, and fungal genera such as Plectosphaerella (PI) and Fusarium (Fu). WRR-AS samples (in black) occupied a central position and were linked to Penicillium (Pe), Fusarium (Fu), and Kendrickiella (KI), as well as Al. WRR-N samples (in blue) were associated with Ilyonectria (Iy) as well as pH, Fe, P, Mn, N-NO2, Na and Zn.
Overall, the PCA results demonstrated distinct microbial and soil properties across disease categories and orchards. While there were similarities in the fungal and bacterial profiles of the two orchards, differences in clustering patterns were observed. These findings might underscore the complexity of soil-microbial interactions and their relationship to environmental conditions and orchard management.
In vitro antagonistic effect of fungi and bacteria against D. necatrix
A total of 20 fungal and 22 bacterial isolates were isolated from healthy soil and assessed for their ability to inhibit D. necatrix growth (Supplementary Tables 10, 11, respectively). Among the fungal isolates, eight demonstrated significant inhibitory activity, reducing the mycelial growth of D. necatrix by more than 50%. These isolates included Trichoderma longibrachiatum (AS6735), Fusarium solani (AS6733, AS1533, and AS1532), Mortierella alpina (AS1034), Podila minutissima (AS6739), Linnemannia elongate (AS191F) and Penicillium citreonigrum (AS1038) (Figure 8). The remaining 12 fungal isolates showed less than 50% inhibition, indicating limited effectiveness against D. necatrix (Figure 9).
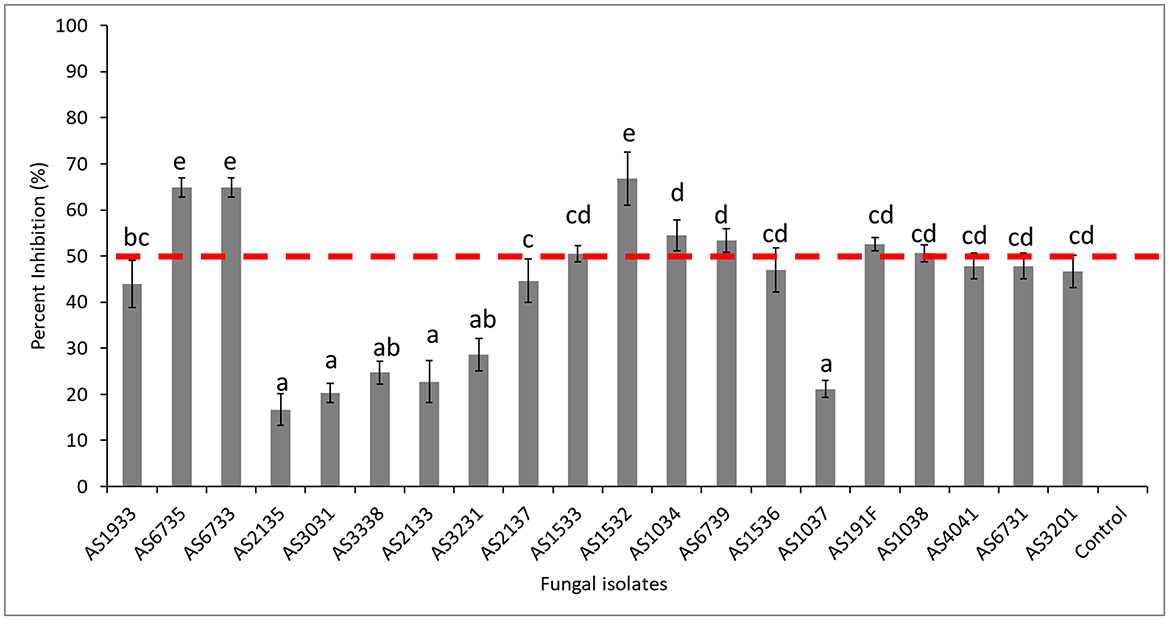
Figure 8. Percent inhibition (%) of selected fungal isolates showing antagonistic activity against Dematophora necatrix. AS1034 (Mortierella alpina), AS1037 (Penicillium chrysogenum), AS1038 (Penicillium citreonigrum), AS1532 (Fusarium solani), AS1533 (Fusarium solani), AS1536 (Aspergillus flavus), AS191F (Linnemannia elongate), AS1933 (Cladosporium halotolerans), AS2133 (Dactylonectria novozelandica), AS2135 (Neopyrenochaeta acicola), AS2137 (Penicillium expansum), AS3031 (Subramaniula cuniculorum), AS3201 (Geomyces asperulatus), AS3231 (Purpureocillium lilacinum), AS3338 (Gloeotinia temulenta), AS4041 (Penicillium buchwaldii), AS6731 (Talaromyces variabilis), AS6733 (Fusarium solani), AS6735 (Trichoderma longibrachiatum), AS6739 (Podila minutissima), and Control (Dematophora necatrix only). The mean inhibition rates indicated by the same letter are not significantly different according to Tukey's test (p ≤ 0.05).
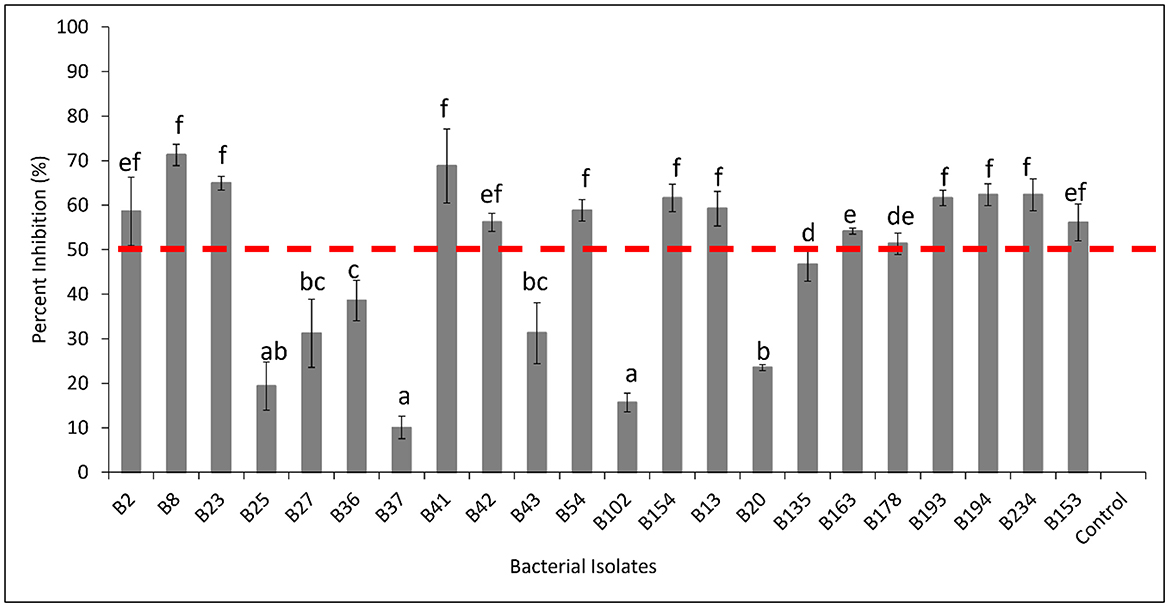
Figure 9. Percent inhibition (%) of selected bacterial isolates showing antagonistic activity against Dematophora necatrix. B102 (Serratia odorifera), B13 (Stremptomyces sp.), B135 (Pseudomonas baetica), B153 (Pseudomonas koreensis), B154 (Pseudomonas koreensis), B163 (Pseudomonas koreensis), B178 (Pseudomonas fluorenscens), B193 (Psedomonas koreensis), B194 (Pseudomonas fluorescens), B2 (Bacillus subtilis), B20 (Serratia odorifera), B23 (Bacillus subtilis), B234 (Pseudomonas sp.), B25 (Microbactrium testaceum), B27 (Microbacterium sp.), B36 (Microbactrium testaceum), B37 (Microbactrium testaceum), B41 (Bacillus tequilensis), B42 (Bacillus subtilis), B43 (Microbacterium trichothecenolyticum), B54 (Pseudomonas sp.), B8 (Bacillus subtilis) and Control (Dematophora necatrix only). The mean inhibition rates indicated by the same letter are not significantly different according to Tukey's test (p ≤ 0.05).
Among the bacterial isolates, 14 exhbited strong inhibitory effects, reducing D. necatrix growth with more than 50%. These isolates included Bacillus subtilis (B2, B8, B23 and B42), Bacillus tequilensis (B41), Pseudomonas sp. (B54 and B234), Pseudomonas koreensis (B154, B163, B193, B153), Pseudomonas fluorenscens (B178 and B194), and Streptomyces sp. (B13) (Figure 9). These results highlight the potential of several fungal and bacterial isolates as biocontrol agents against D. necatrix, with particular promise shown by members of the Trichoderma, Fusarium, Pseudomonas, and Bacillus genera. Further studies are needed to explore their mechanisms of action and potential application in soil disease management.
Discussion
This study investigated the bacterial and fungal communities in the rhizosphere of WRR- asymptomatic (WRR-AS), symptomatic (WRR-S), and non-infected (WRR-N) avocado trees, along with soil health parameters, to assess the impact of D. necatrix on microbial composition and diversity. The presence of D. necatrix in both orchards confirmed earlier reports by van den Berg et al. (2018) for South African avocado orchards. Consistent with global findings, P. cinnamomi was also detected in all samples of this study (Duvenhage et al., 1991; Pérez-Jiménez, 2008; Engelbrecht and van den Berg, 2013; Ramírez-Gil et al., 2017). Interestingly, D. necatrix did not significantly alter the overall diversity of the avocado rhizosphere microbiome.
The genera Fusarium and Sphingomonas were the only abundant genera across WRR-S, WRR-AS, and WRR-N soil samples in both orchards. WRR-N samples showed stronger associations with key soil physicochemical properties, and fungal and bacterial microbial composition, indicating healthy soil in areas where D. necatrix was absent. Beneficial microorganisms such as Trichoderma, Streptomyces and Bacillus were more enriched in WRR-N samples in both orchards, while Pseudomonas was only prevalent in healthy soil in Orchard B (Iacomino et al., 2022; Singh et al., 2022; Prigigallo et al., 2023). Notably, isolates of Bacillus, Pseudomonas, Trichoderma and Penicillium recovered from healthy soil in this study demonstrated significant inhibition of D. necatrix growth in dual-culture assays, highlighting their potential use as biocontrol agents. A previous study demonstrated the inoculation of the Morchella importuna mycosphere with Pseudomonas chlororaphis led to reduction of Paecilomyces penicillatus, indicating a restoration of a healthy soil microbiome and enhanced biocontrol properties (Yu et al., 2025). These findings provide valuable insights into the interaction between D. necatrix and the avocado rhizosphere, offering potential avenues for effective disease management.
The bacterial and fungal diversity of the avocado rhizosphere is not altered by D. necatrix
Contrary to our hypothesis, D. necatrix did not significantly alter the microbiome in the two avocado orchards studied, this is consistent with previous findings for P. cinnamomi, which similarly did also not affect species richness or diversity in the avocado rhizosphere (Solís-García et al., 2021). The lack of D. necatrix on diversity metrics could be due to various factors, such as the buffering effect of P. cinnamomi, and the plant defense response. The gap in knowledge may be addressed using in depth root exudates analysis which can clarify the interactions between pathogens and their effect on the rhizosphere microbiome. A similar lack of impact on the diversity of the rhizosphere fungal communities was reported for kiwifruit vine decline syndrome-affected kiwifruit plants when compared to healthy plants (Guaschino et al., 2023).
Abundance of bacterial genera of two avocado orchards
In Orchard A, the shared most abundant bacterial genera across WRR-S, WRR-AS, and WRR-N included Sphingomonas, SC-I-84, Rokubacteriales, and Lysobacter. The presence of Sphingomonas, which has previously been shown to be associated with plant stress (Asaf et al., 2020), suggests stress due to the presence of P. cinnamomi and D. necatrix. Additionally, Lysobacter, a known biological control agent (BCA) against Fusarium oxysporum, Colletotrichum gloeosporiodes, and Rhizoctonia spp., was similarly identified in the rhizosphere of avocado trees exhibiting Fusarium dieback (Bejarano-Bolívar et al., 2021). Interestingly, Pseudomonas was abundant only in WRR-S samples in Orchard A. This may reflect plant responses to pathogen stress through the release of root exudates as plants under stress may recruit beneficial microbes to enhance defenses (Rolfe et al., 2019; Qu et al., 2020). In orchard B however, Pseudomonas was associated with WRR-N samples, which may be related to local soil properties, host age or genotype, rather than to the presence/absence of D. necatrix. The presence of P. cinnamomi in avocado orchards was previously shown to increase the abundance of Pseudomonades and Burkholderiales in the rhizosphere, while reducing the abundance of Actinobacteria, Bacillus, and Rhizobiales (Solís-García et al., 2021).
In Orchard B, Sphingomonas, SC-I-84, and Acidothermus were the most abundant shared genera across all samples. Acidothermus, known for increasing soil nutrient content by breaking down cellulose (Lin et al., 2022) was less abundant in WRR-S and WRR-AS compared to healthy soil (WRR-N), possibly reflecting reduced biomass utilization in diseased trees (Ren et al., 2021). The recruitment of Sphingomonas, observed in high abundance in this study, may have been triggered by root exudates in response to P. cinnamomi (present in all samples) and D. necatrix, as similar mechanisms have been reported where root exudates from cucumber infected with Fusarium oxysporum f.sp. cucumerinum attract beneficial microbes to counter pathogen invasion (Wen et al., 2023). These findings highlight the potential role of rhizosphere bacteria in mitigating plant stress and maintaining soil health.
The enrichment of specific bacterial genera between WRR-S, WRR-AS and WRR-N categories of avocado trees
Enrichment refers to the increased prevalence or activity of specific microorganisms driven by environmental conditions or pathogen presence. Pseudomonas, known for its ability to evade or suppress plant defenses and colonize the rhizosphere (Liu et al., 2018; Mendes et al., 2011; Yu et al., 2019), was abundant in WRR-S samples consistent with findings by Solís-García et al. (2021). The authors reported the presence of Pseudomonadales, Burkholderiales, Beta- and Gamma-Proteobacteria in the rhizosphere of root rot symptomatic avocado trees. These genera are fast-growing colonizers supported by carbon rich root exudates released under stress and by rotting roots (Eilers et al., 2010; Trivedi et al., 2012).
In Orchard B, WRR-N samples were enriched with Streptomyces (Actinobacteria), Pseudomonas, and Bacillus (Firmicutes). Previous studies linked Acidobacteria, Actinobacteria and Firmicutes with asymptomatic avocado trees infected with P. cinnamomi (Solís-García et al., 2021), supporting our results. The presence of Streptomyces in WRR-N may offer disease-suppressive potential, as a reduction in Streptomyces sp. has been linked to the lack of disease suppression in strawberry plants (Kim et al., 2019). Bacillus sp., typically associated with healthy samples in this study, are known to suppress Phytophtora root rot (PRR) in avocado (Guevara-Avendaño et al., 2018). These findings underscore the potential of beneficial microbes like Streptomyces and Bacillus in managing WRR and other opportunistic pathogens in avocado rhizospheres.
Abundance and enrichment of fungal genera in two avocado orchards
Ascomycetes and Basidiomycetes were the dominant fungi in both avocado orchards. In Orchard A, Fusarium and Cercophora were the most prevalent genera across all categories. The presence of the pathogens Ilyonectria and Dactylonectria in WRR-N samples was not unexpected as these samples were positive for P. cinnamomi, and these genere were previously reported in the pathobiome of avocado trees infected with P. cinnamomi (Solís-García et al., 2021).
Orchard B exhibited dominance of Fusarium, Saitozyma, and Hyaloscypha across all three categories. Similarly to Orchard A, WRR-N was characterized by Trichoderma, Penicillium and the two Nectriaceae pathogens. WRR-S featured Trichoderma and Paracremonium. These findings align with studies on the impact of Phytophthora on rhizosphere microbiomes (Solís-García et al., 2021; Reverchon et al., 2023). Penicillium and Trichoderma were detected in WRR-N trees, in agreement with Handique et al. (2024), who found the microbes more abundant in uninfected Khasi Mandarin as compared to Phytophthora infected-Khasi Mandarin citrus trees.
Previous research has indicated that P. cinnamomi can alter the microbial structure and composition of the avocado rhizospheres and is often associated with other pathogens that contribute to root rot (Yang et al., 2001; Shu et al., 2019; Solís-García et al., 2021). This may explain the co-existence of P. cinnamomi, D. necatrix and opportunistic genera such as Fusarium in our study; Fusarium spp. were prevalent in both orchards, particularly in WRR-S samples. Our findings align with studies on Phytophthora-infected soil in the rhizosphere of citrus, where Fusarium thrives in pathogen-stressed soils (Handique et al., 2024). Infected citrus plants also exhibited high abundance of Aspergillus, Saitozyma and Colletotrichum, while non-infected plants were dominated by Trichoderma, Penicillium, Mortierella and Saitozyma (Handique et al., 2024). While the Fusarium genus contains several notable pathogenic species; such as Fusarium solani, F. oxysporum, F. equisetti and F. chlamydosporum (Burgess et al., 1994; Chimbekujwo, 2000; Crous et al., 2021); most isolates that are abundant in the soil, frequently associate with plant roots as saprophytes (Nelson et al., 1994). Some Fusarium spp. may even act as endophytes of avocado and have even been reported to have anti-oomycete activity and plant growth promoting properties (Nieves-Campos et al., 2024).
The presence of Ilyonectria (previously classified under Cylindrocarpon) in both avocado orchards is concerning, as it is linked to root rot, stem lesions, damping off, branch and crown cankers, fruit disease, reduced yield, and increased susceptibility to other pathogens (Chaverri et al., 2011). Cylindrocarpon destructans, Cylindrocladium parvum and less often Cylindrocladium scoparium were isolated in South African avocado orchards in the 1970s (Darvas, 1978), while Cylindrocarpon sp. was isolated in Spain in the 1980s from severly affected avocado trees (López-Herrera and Melero-Vara, 1992). These studies have suggested that these fungi exacerbate vulnerability to D. necatrix and P. cinnamomi. Similarly, Calonectria, Cylindrocladiella and Neonectria have been reported from diseased avocado roots in Australia (Dann et al., 2012).
The co-occurence of D. necatrix and P. cinnamomi, Ilyonectria and opportunistic pathogens like Fusarium, suggests a disrupted microbial community structure that impacts avocado tree health. The South African avocado industry is ill-equipped to manage such fungal threats, especially as climate change increases pathogen pressures. Similar challenges have already been observed with Ilyonectria in avocado orchards in Spain and Australia (López-Herrera and Melero-Vara, 1992; Dann et al., 2012). Without effective intervention, pathogens like Fusarium and Ilyonectria could become increasingly dominant, posing a severe threat to avocado cultivation.
As expected, Trichoderma was abundant and enriched in WRR-N soil in the two orchards, suggesting its potential as an indicator of tree health. Known for its biocontrol properties, Trichoderma has been shown to inhibit D. necatrix effectively in laboratory and greenhouse studies (Freeman et al., 1986; Ruano-Rosa and López-Herrera, 2009; Ruano-Rosa et al., 2010). Its presence highlights the importance of beneficial fungi in maintaining soil health and suppressing diseases.
Unique bacterial and fungal genera between WRR-S, WRR-AS and WRR-N categories of avocado trees
The presence of unique genera offers opportunities to discover beneficial microbes for pathogen management and to preserve microbial diversity. The current study identified distinct fungal and bacterial genera associated with WRR-S, WRR-AS, and WRR-N categories, with some genera uniquely enriched in specific groups. In Orchard A, Chitinophaga was enriched and unique to WRR-N. This genus, belonging to the phylum Bacteroidetes, is involved in plant biomass degradation (Funnicelli et al., 2021). Previous studies showed that Chitinophaga flava isolated from the rhizosphere soil of tomato plants, demonstrated high levels of antifungal activity against gray mold disease caused by Botrytis cinerea, exhibiting potential biocontrol activity (Kim et al., 2023). Also unique to WRR-N in Orchard A were Gliomastix and Linnemannia, with species like Linnemannia elongate playing a role in chitin-based plant growth promotion (De Tender et al., 2024). In Orchard B, Bartalinia was highly abundant and unique to WRR-AS. Very little is known about this wood-decomposing genus in South Africa (Marincowitz et al., 2010).
No unique and abundant fungi were identified in orchard A. In Orchard B, Colletotrichum, Lophiostoma, Xenoacremonium, Gibellulopsis, Bipolaris and Verticillium were enriched and unique to WRR-S samples. Many of these genera are known plant pathogens causing significant losses (Barbara and Clewes, 2003; De Silva et al., 2017; Al-Sadi, 2021; Amani et al., 2023). Colletotrichum species are primarily considered foliar and fruit pathogens, causing anthracnose diseases in a wide range of crops such as red rot of sugarcane, crown rot of strawberry, and banana (Lenné, 2002). However, some species, such as Colletotrichum graminicola in maize, have been reported as soilborne pathogens, persisting in soil as saprophytes, surviving on plant debris and infecting plants through root-associated interactions (Sukno et al., 2008). Verticillium dahlia, for example, secretes effectors that manipulate bacterial microbiomes promoting infection (Snelders et al., 2022). The presence of Verticillium in WRR-S avocado plants may contribute to increased susceptibility and disease progression. A similar phenomenon has been reported with D. necatrix where microbial interactions exacerbated disease severity in cotton (Chavarro-Carrero et al., 2024). The findings suggest that WRR infected trees attract opportunistic pathogens, reducing resilience against infections, as previously observed in avocado orchards affected by PRR (Solís-García et al., 2021). WRR significantly alters the rhizosphere microbiome, enriching unique genera in both infected and non-infected trees, which may influence disease dynamics and plant health.
The association of microbial communities with soil physicochemical analysis
The study demonstrated a strong association between fungal and bacterial microbial community structure and rhizosphere soil properties in WRR-N samples compared to WRR-S and WRR-AS samples. For example, soil pH and Fe were strongly related with several bacteria and fungi, emphasizing the key role pH plays in shaping microbial diversity and driving processes like nitrification and denitrification (Hayatsu et al., 2021). This distinction may be attributed to enhanced microbial activity, which facilitates nutrient cycling and organic matter decomposition, ultimately improving soil fertility and plant growth (Condron et al., 2010). The observed association highlight the intricate relationship between soil physicochemical properties and microbial ecology, reinforcing the importance of maintaining healthy soils for sustainable productivity. Finally, WRR-N soils clustered on the opposite side of the PCA to WRR-AS and WRR-S soils, together with several soil properties; this may indicate patterns likely to support a decreased incidence of WRR.
Screening and determination of antagonistic effects of culturable bacteria and fungi
This study identified bacterial and fungal microorganisms from healthy soil and demonstated antagonistic activity against D. necatrix. The characterization of antagonistic activity and activities associated with plant growth has previously enabled the identification of BCAs against D. necatrix (Pliego et al., 2007; Ruano-Rosa et al., 2010). Dual-culture assays showed that bacterial genera such as Pseudomonas, Bacillus and Streptomyces effectively inhibited mycelial growth of D. necatrix, consistent with their role in disease suppression and plant growth promotion. For example, Pseudomonas chlororaphis PCL1606 exhibits antifungal activity against D. necatrix by competing for space and root exudate nutrients (Calderón et al., 2014). Additionally in support of our findings, B. subtilis strains isolated from healthy avocado rhizospheres have demonstrated antifungal activity against D. necatrix in both in vitro and in vivo studies, directly attacking soil pathogens and stimulating plant defenses (Cazorla et al., 2007). Similarly, rhizosphere isolates of Bacillus have been shown to reduce mycelial growth of Fusarium euwallaceae, a causal agent of Fusarium dieback in avocado (Guevara-Avendaño et al., 2019). These findings suggest the potential of bacteria with antifungal properties to mitigate D. necatrix in avocado orchards.
Fungal genera such as Trichoderma, Penicillium, and Mortierella also inhibited D. necatrix in dual-culture assays. Trichoderma is well known for promoting plant growth and suppressing soil-borne diseases, with previous studies demonstrating its efficacy against D. necatrix in vitro and in greenhouse trials (Freeman et al., 1986; Ruano-Rosa et al., 2010). Additionally, Fusarium and Aspergillus species also showed antagonistic activity. This suggests that there is intense competition within the avocado rhizosphere, where D. necatrix must contend with other fungal pathogens to establish infection. Future studies should prioritize field trials with promising fungal and bacterial isolates to develop effective biocontrol strategies for avocado orchards.
Conclusion
This study provides valuable insights into the microbial dynamics of avocado rhizospheres infected with D. necatrix and highlights potential biocontrol organisms. While D. necatrix did not significantly alter overall microbial diversity, its presence, alongside P. cinnamomi, influenced the enrichment of specific bacterial and fungal genera, shaping the rhizosphere composition. Beneficial genera such as Trichoderma, Bacillus, Streptomyces, and Pseudomonas were associated with WRR-N soils, reflecting a potential to develop a more resilient rhizosphere microbiome and enhanced disease suppression potential compared to WRR-S and WRR-AS soils. Future research should focus on pathogenicity trials in the greenhouse and field trials to validate the efficacy of identified microbial biocontrol agents under orchard conditions and further explore the mechanisms underlying microbial interactions. Additionally, metagenomic, metatranscriptomic, and, metabolomic approaches could provide comprehensive insights into the functional roles of rhizosphere microbiota, enabling tailored interventions to enhance avocado tree health and resilience.
Data availability statement
The datasets presented in this study can be found in online repositories. The names of the repository/repositories and accession number(s) can be found in the article/Supplementary material.
Author contributions
PM: Conceptualization, Data curation, Formal analysis, Investigation, Methodology, Project administration, Resources, Software, Validation, Visualization, Writing – original draft, Writing – review & editing. VS: Conceptualization, Methodology, Visualization, Writing – review & editing, Funding acquisition, Project administration, Resources, Supervision. AF: Conceptualization, Methodology, Visualization, Writing – review & editing, Data curation, Software. AV: Investigation, Methodology, Visualization, Writing – review & editing. JN: Investigation, Methodology, Visualization, Writing – review & editing. ZR: Resources, Writing – review & editing. NB: Conceptualization, Formal analysis, Funding acquisition, Project administration, Resources, Supervision, Visualization, Writing – review & editing.
Funding
The author(s) declare that financial support was received for the research and/or publication of this article. This study was funded by the Hans Merensky Legacy Foundation and the South African Avocado Growers Association.
Acknowledgments
We are grateful to Westfalia Fruit Estate for allowing us access to their orchards. We wish to thank Dr. Robert Backer for his valuable inputs on the data analysis.
Conflict of interest
The authors declare that the research was conducted in the absence of any commercial or financial relationships that could be construed as a potential conflict of interest.
The author(s) declared that they were an editorial board member of Frontiers, at the time of submission. This had no impact on the peer review process and the final decision.
Generative AI statement
The author(s) declare that no Gen AI was used in the creation of this manuscript.
Publisher's note
All claims expressed in this article are solely those of the authors and do not necessarily represent those of their affiliated organizations, or those of the publisher, the editors and the reviewers. Any product that may be evaluated in this article, or claim that may be made by its manufacturer, is not guaranteed or endorsed by the publisher.
Supplementary material
The Supplementary Material for this article can be found online at: https://www.frontiersin.org/articles/10.3389/fmicb.2025.1583797/full#supplementary-material
References
Al-Sadi, A. M. (2021). Bipolaris sorokiniana-induced black point, common root rot, and spot blotch diseases of wheat: a review. Front. Cell Infect. Microbiol. 11:584899. doi: 10.3389/fcimb.2021.584899
Amani, M., Farokhinejad, R., and Mehrabii-Koushki, M. (2023). Xenoacremonium palmarum sp. nov., a novel species associated with Phoenix dactylifera in Iran. Phytotaxa 632, 165–174. doi: 10.11646/phytotaxa.632.2.6
Arjona-López, J. M., Monferrer-Salinas, J. A., Cantero-Sánchez, J. L., Romero-Rodríguez, E., López-Herrera, C. J., and Arenas-Arenas, F. J. (2024). Evaluation of susceptibility of commercial citrus rootstocks to white root rot incited by Rosellinia necatrix. J. Plant Pathol. 106, 633–642. doi: 10.1007/s42161-024-01599-y
Asaf, S., Numan, M., Khan, A. L., and Al-Harrasi, A. (2020). Sphingomonas: from diversity and genomics to functional role in environmental remediation and plant growth. Crit. Rev. Biotechnol. 40, 138–152. doi: 10.1080/07388551.2019.1709793
Barbara, D. J., and Clewes, E. (2003). Plant pathogenic Verticillium species: how many of them are there? Mol. Plant Pathol. 4, 297–305. doi: 10.1046/j.1364-3703.2003.00172.x
Bejarano-Bolívar, A. A., Lamelas, A., Aguirre von Wobeser, E., Sánchez-Rangel, D., Méndez-Bravo, A., Eskalen, A., et al. (2021). Shifts in the structure of rhizosphere bacterial communities of avocado after Fusarium dieback. Rhizosphere 18:100333. doi: 10.1016/j.rhisph.2021.100333
Berendsen, R. L., Pieterse, C. M., and Bakker, P. A. (2012). The rhizosphere microbiome and plant health. Trends in Plant Sci. 17, 478–486. doi: 10.1016/j.tplants.2012.04.001
Bolyen, E., Rideout, J. R., Dillon, M. R., Bokulich, N. A., Abnet, C. C., Al-Ghalith, G. A., et al. (2019). Reproducible, interactive, scalable and extensible microbiome data science using QIIME 2. Nat. Biotechnol. 37, 852–857. doi: 10.1038/s41587-019-0209-9
Brunner, I., Brodbeck, S., Büchler, U., and Sperisen, C. (2001). Molecular identification of fine roots of trees from the Alps: reliable and fast DNA extraction and PCR-RFLP analyses of plastid DNA. Mol. Ecol. 10, 2079–2087. doi: 10.1046/j.1365-294X.2001.01325.x
Bulgarelli, D., Schlaeppi, K., Spaepen, S., Van Themaat, E. V. L., and Schulze-Lefert, P. (2013). Structure and functions of the bacterial microbiota of plants. Annu. Rev. Plant Biol. 64, 807–838. doi: 10.1146/annurev-arplant-050312-120106
Burgess, L. W., Summerell, B. A., Bullock, S., Gott, K. P., and Backhouse, D. (1994). Laboratory Manual for Fusarium Research, 3rd Edn. University of Sydney Australia.
CABI (2024). Dematophora necatrix (dematophora root rot). CABI Compendium [Online]. Available online at: https://www.cabidigitallibrary.org/doi/10.1079/cabicompendium.47860#tab-citations (accessed July 12, 2024).
Calderón, C. E., de Vicente, A., and Cazorla, F. M. (2014). Role of 2-hexyl, 5-propyl resorcinol production by Pseudomonas chlororaphis PCL1606 in the multitrophic interactions in the avocado rhizosphere during the biocontrol process. FEMS Microbiol. Ecol. 89, 20–31. doi: 10.1111/1574-6941.12319
Callahan, B. J., Mcmurdie, P. J., Rosen, M. J., Han, A. W., Johnson, A. J. A., Holmes, S. P., et al. (2016). DADA2: high-resolution sample inference from Illumina amplicon data. Nat. Methods 13, 581–583. doi: 10.1038/nmeth.3869
Castellano-Hinojosa, A., Albrecht, U., and Strauss, S. L. (2023). Interactions between rootstocks and compost influence the active rhizosphere bacterial communities in citrus. Microbiome 11:79. doi: 10.1186/s40168-023-01524-y
Castro, B. L., Carreño, A. J., Galeano, N. F., Roux, J., Wingfield, M. J., Gaitán, Á. L., et al. (2013). Identification and genetic diversity of Rosellinia spp. associated with root rot of coffee in Colombia. Australas. Plant Pathol. 42, 515–523. doi: 10.1007/s13313-013-0205-3
Cazorla, F., Romero, D., Pérez-García, A., Lugtenberg, B., Vicente, A. D., and Bloemberg, G. (2007). Isolation and characterization of antagonistic Bacillus subtilis strains from the avocado rhizoplane displaying biocontrol activity. J. Appl. Microbiol. 103, 1950–1959. doi: 10.1111/j.1365-2672.2007.03433.x
Chapelle, E., Mendes, R., Bakker, P. A. H., and Raaijmakers, J. M. (2016). Fungal invasion of the rhizosphere microbiome. ISME J. 10, 265–268. doi: 10.1038/ismej.2015.82
Chavarro-Carrero, E. A., Snelders, N. C., Torres, D. E., Kraege, A., López-moral, A., Petti, G. C., et al. (2024). The soil-borne white root rot pathogen Rosellinia necatrix expresses antimicrobial proteins during host colonization. PLoS Pathog. 20:e1011866. doi: 10.1371/journal.ppat.1011866
Chaverri, P., Salgado, C., Hirooka, Y., Rossman, A., and Samuels, G. (2011). Delimitation of Neonectria and Cylindrocarpon (Nectriaceae, Hypocreales, Ascomycota) and related genera with Cylindrocarpon-like anamorphs. Stud. Mycol. 68, 57–78. doi: 10.3114/sim.2011.68.03
Chen, J., Deng, Z., Jiang, Z., Sun, J., Meng, F., Zuo, X., et al. (2022). Variations of rhizosphere and bulk soil microbial community in successive planting of Chinese fir (Cunninghamia lanceolata). Front. Plant Sci. 13:954777. doi: 10.3389/fpls.2022.954777
Chimbekujwo, I. B. (2000). Frequency and pathogenicity of Fusarium wilts (Fusarium solani and Fusarium equiseti) of cotton (Gossypium hirsutum) in Adamawa, Nigeria. Rev. Biol. Trop. 48, 1–5.
Condron, L., Stark, C., O'Callaghan, M., Clinton, P., and Huang, Z. (2010). “The role of microbial communities in the formation and decomposition of soil organic matter,” in Soil Microbiology and Sustainable Crop Production, eds. G. Dixon and E. Tilston (Dordrecht: Springer), 81–118. doi: 10.1007/978-90-481-9479-7_4
Conway, J. R., Lex, A., and Gehlenborg, N. (2017). UpSetR: an R package for the visualization of intersecting sets and their properties. Bioinformatics 33, 2938–2940. doi: 10.1093/bioinformatics/btx364
Crous, P. W., Lombard, L., Sandoval-denis, M., Seifert, K. A., Schroers, H. J., Chaverri, P., et al. (2021). Fusarium: more than a node or a foot-shaped basal cell. Stud. Mycol. 98:100116. doi: 10.1016/j.simyco.2021.100116
Dafny-Yelin, M., Mairesse, O., Moy, J., Dor, S., and Malkinson, D. (2018). Genetic diversity and infection sources of Rosellinia necatrix in northern Israel. Phytopathol. Mediterr. 57, 37–47. doi: 10.14601/Phytopathol_Mediterr-22478
Dann, E., Cooke, A., Forsberg, L., Pegg, K., Tan, Y. P., Shivas, R., et al. (2012). Pathogenicity studies in avocado with three nectriaceous fungi, Calonectria ilicicola, Gliocladiopsis sp, Ilyonectria liriodendri. Plant Pathol. 61, 896–902. doi: 10.1111/j.1365-3059.2011.02579.x
Darvas, J. (1978). Common root pathogens from avocados. S. Afr. Avocado Grow. Assoc. Res. Rep. 2, 3–4.
De Silva, D. D., Crous, P. W., Ades, P. K., Hyde, K. D., and Taylor, P. W. (2017). Life styles of Colletotrichum species and implications for plant biosecurity. Fungal Biol. Rev. 31, 155–168. doi: 10.1016/j.fbr.2017.05.001
De Tender, C., Vandecasteele, M., Ommeslag, S., De Zutter, N., Vandenbussche, E., Haegeman, A., et al. (2024). Linnemannia elongata: a key species in chitin-based plant growth promotion. Phytobiomes J. 8, 366–377. doi: 10.1094/PBIOMES-05-23-0031-R
Duvenhage, J., Kotzé, J., and Maas, E. M. (1991). Suppressive soils and biological control of Phytophthora root rot. S. Afr. Avocado Grow. Assoc. Yearb. 14, 06–11.
Eilers, K. G., Lauber, C. L., Knight, R., and Fierer, N. (2010). Shifts in bacterial community structure associated with inputs of low molecular weight carbon compounds to soil. Soil Biol. Biochem. 42, 896–903. doi: 10.1016/j.soilbio.2010.02.003
Engelbrecht, J., Duong, T. A., and van den Berg, N. (2017). New microsatellite markers for population studies of Phytophthora cinnamomi, an important global pathogen. Sci. Rep. 7:17631. doi: 10.1038/s41598-017-17799-9
Engelbrecht, J., and van den Berg, N. (2013). Expression of defence-related genes against Phytophthora cinnamomi in five avocado rootstocks. S. Afr. J. Sci. 109, 1–8. doi: 10.1590/sajs.2013/20120058
Freeman, S., Sztejnberg, A., and Chet, I. (1986). Evaluation of trichoderma as a biocontrol agent for Rosellinia necatrix. Plant Soil 94, 163–170. doi: 10.1007/BF02374340
Funnicelli, M. I. G., Pinheiro, D. G., Gomes-pepe, E. S., De Carvalho, L. A. L., Campanharo, J. C., Fernandes, C. C., et al. (2021). Metagenome-assembled genome of a Chitinophaga sp, its potential in plant biomass degradation, as well of affiliated Pandoraea and Labrys species. World J. Microbiol. Biotechnol. 37:162. doi: 10.1007/s11274-021-03128-w
Ginnan, N. A., Dang, T., Bodaghi, S., Ruegger, P. M., Mccollum, G., England, G., et al. (2020). Disease-induced microbial shifts in citrus indicate microbiome-derived responses to huanglongbing across the disease severity spectrum. Phytobiomes J. 4, 375–387. doi: 10.1094/PBIOMES-04-20-0027-R
González-Sánchez, M. Á., De Vicente, A., Pérez-García, A., Pérez-Jiménez, R., Romero, D., and Cazorla, F. M. (2013). Evaluation of the effectiveness of biocontrol bacteria against avocado white root rot occurring under commercial greenhouse plant production conditions. Biol. Control. 67, 94–100. doi: 10.1016/j.biocontrol.2013.08.009
Granum, E., Pérez-Bueno, M. L., Calderón, C. E., Ramos, C., de Vicente, A., Cazorla, F. M., et al. (2015). Metabolic responses of avocado plants to stress induced by Rosellinia necatrix analysed by fluorescence and thermal imaging. Europ. J. Plant Pathol. 142, 625–632. doi: 10.1007/s10658-015-0640-9
Guaschino, M., Garello, M., Zhimo, Y. V., Droby, S., and Spadaro, D. (2023). Soil, rhizosphere, and root microbiome in kiwifruit vine decline, emerging multifactorial disease. Front. Microbiol. 15:1330865. doi: 10.3389/fmicb.2024.1330865
Guevara-Avendaño, E., Bejarano-Bolívar, A. A., Kiel-Martínez, A-. L., Ramírez-Vázquez, M., Méndez-Bravo, A., Von Wobeser, E. A., et al. (2019). Avocado rhizobacteria emit volatile organic compounds with antifungal activity against Fusarium solani, Fusarium sp. associated with Kuroshio shot hole borer, and Colletotrichum gloeosporioides. Microbiol. Res. 219, 74–83. doi: 10.1016/j.micres.2018.11.009
Guevara-Avendaño, E., Carrillo, J. D., Ndinga-Muniania, C., Moreno, K., Méndez-Bravo, A., Guerrero-Analco, J. A., et al. (2018). Antifungal activity of avocado rhizobacteria against Fusarium euwallaceae and Graphium spp., associated with Euwallacea spp. nr. fornicatus, and Phytophthora cinnamomi. Antonie van Leeuwenhoek 111, 563–572. doi: 10.1007/s10482-017-0977-5
Handique, M., Bora, P., Ziogas, V., Srivastava, A. K., Jagannadham, P. T. K., Das, A. K., et al. (2024). Phytophthora infection reorients the composition of rhizospheric microbial assembly in Khasi mandarin (Citrus reticulata Blanco). Agronomy 14:661. doi: 10.3390/agronomy14040661
Hawes, M. C., Curlango-Rivera, G., Xiong, Z., and Kessler, J. O. (2012). Roles of root border cells in plant defense and regulation of rhizosphere microbial populations by extracellular DNA 'trapping'. Plant Soil 355, 1–16. doi: 10.1007/s11104-012-1218-3
Hayatsu, M., Katsuyama, C., and Tago, K. (2021). Overview of recent researches on nitrifying microorganisms in soil. Soil Sci. Plant Nutr. 67, 619–632. doi: 10.1080/00380768.2021.1981119
Herlemann, D. P., Labrenz, M., Jürgens, K., Bertilsson, S., Waniek, J. J., Andersson, A. F., et al. (2011). Transitions in bacterial communities along the 2000 km salinity gradient of the Baltic sea. ISME J. 5, 1571–1579. doi: 10.1038/ismej.2011.41
Hinsinger, P., Bengough, A. G., Vetterlein, D., and Young, I. M. (2009). Rhizosphere: biophysics, biogeochemistry and ecological relevance. Plant Soil 321, 117–152. doi: 10.1007/s11104-008-9885-9
Iacomino, G., Staropoli, A., Prigigallo, M. I., Bubici, G., Scagliola, M., Salerno, P., et al. (2022). Beneficial microbes application on tomato significantly improves accumulation of metabolites with nutraceutical value. Chem. Proc. 10:75. doi: 10.3390/IOCAG2022-12238
Kim, D. R., Cho, G., Jeon, C. W., Weller, D. M., Thomashow, L. S., Paulitz, T. C., et al. (2019). A mutualistic interaction between Streptomyces bacteria, strawberry plants and pollinating bees. Nat. Commun. 10:4802. doi: 10.1038/s41467-019-12785-3
Kim, D. Y., Han, J. W., Lee, J. W., Kim, B., Kim, Y. S., Kim, H. T., et al. (2023). Biocontrol potential of Chitinophaga flava HK235 producing antifungal-related peptide chitinocin. Front. Microbiol. 14:1170673. doi: 10.3389/fmicb.2023.1170673
Lenné, J. M. (2002). “Some major plant diseases,” in Plant Pathologist's Pocketbook, 3rd Edn, eds. J. M. Waller, J. M. Lenné, and S. J. Waller (CABI: Wallingford), 4–18.
Lin, W., Liu, L., Liang, J., Tang, X., Shi, J., Zhang, L., et al. (2022). Changes of endophytic microbial community in Rhododendron simsii roots under heat stress and its correlation with leaf physiological indicators. Front. Microbiol. 13:1006686. doi: 10.3389/fmicb.2022.1006686
Liu, Z., Beskrovnaya, P., Melnyk, R. A., Hossain, S. S., Khorasani, S., O'Sullivan, L. R., et al. (2018). A genome-wide screen identifies genes in rhizosphere-associated Pseudomonas required to evade plant defenses. mBio 9, 00433–18. doi: 10.1128/mBio.00433-18
López-Herrera, C. J., and Melero-Vara, J. M. (1992). “Diseases of avocado caused by soil fungi in the southern Mediterranean coast of Spain,” in Proceedings of the Second World Avocado Congress California Avocado Society: The Shape of Things to Come (Riverside, CA: California Avocado Society), 119–121.
Love, M. I., Huber, W., and Anders, S. (2014). Moderated estimation of fold change and dispersion for RNA-seq data with DESeq2. Genome Biol. 15:550. doi: 10.1186/s13059-014-0550-8
Marincowitz, S., Gryzenhout, M., and Wingfield, M. J. (2010). New and rare coelomycetes with appendage-bearing conidia from Pondoland, South Africa. Mycotaxon 111, 309–322. doi: 10.5248/111.309
Martin, M. (2011). Cutadapt removes adapter sequences from high-throughput sequencing reads. EMBnet. J. 17, 10–12. doi: 10.14806/ej.17.1.200
Martínez Arbizu, P. (2020). pairwiseAdonis: Pairwise Multilevel Comparison Using Adonis. R package version 0.4. Available online at: https://github.com/pmartinezarbizu/pairwiseAdonis
Mcmurdie, P. J., and Holmes, S. (2013). phyloseq: an R package for reproducible interactive analysis and graphics of microbiome census data. PLoS ONE 8:e61217. doi: 10.1371/journal.pone.0061217
Mendes, R., Kruijt, M., de Bruijn, I., Dekkers, E., van der Voort, M., Schneider, J. H., et al. (2011). Deciphering the rhizosphere microbiome for disease-suppressive bacteria. Science 332, 1097–1100. doi: 10.1126/science.1203980
Mercado-Blanco, J., Abrantes, I., Barra Caracciolo, A., Bevivino, A., Ciancio, A., Grenni, P., et al. (2018). Belowground microbiota and the health of tree crops. Front. Microbiol. 9:1006. doi: 10.3389/fmicb.2018.01006
Nelson, P. E., Dignani, M. C., and Anaisse, E. J. (1994). Taxonomy, biology, and clinical aspects of Fusarium species. Clin. Microbiol. Rev. 7, 479–504.
Nieves-Campos, E. I., Méndez-Bravo, A., Pérez-Bautista, Y., Llanderal-Mendoza, J., Guevara-Avendaño, E., Solís-García, I. A., et al. (2024). Anti-oomycete activity and plant growth promoting properties of avocado fungal endophytes. Rhizosphere 31:100931. doi: 10.1016/j.rhisph.2024.100931
Nilsson, R. H., Larsson, K. H., Taylor, A. F. S., Bengtsson-Palme, J., Jeppesen, T. S., Schigel, D., et al. (2019). The UNITE database for molecular identification of fungi: handling dark taxa and parallel taxonomic classifications. Nucleic Acids Res. 47, D259–D264. doi: 10.1093/nar/gky1022
Oksanen, J., Simpson, G., Blanchet, F., Kindt, R., Legendre, P., Minchin, P., et al. (2022). vegan: Community Ecology Package. R package version 2, 6-4.
Palacio-Bielsa, A., Cambra, M., Martinez, C., Olmos, A., Pallás, V., López, M. M., et al. (2017). “Almond diseases,” in Almonds: Botany Production and Uses (Wallingford UK: CABI), 321–374. doi: 10.1079/9781780643540.0321
Pasini, L., Prodorutti, D., Pastorelli, S., and Pertot, I. (2016). Genetic diversity and biocontrol of Rosellinia necatrix infecting apple in northern Italy. Plant Dis. 100, 444–452. doi: 10.1094/PDIS-04-15-0480-RE
Pérez-Jiménez, R. M. (2008). Significant avocado diseases caused by fungi and oomycetes. Eur. J. Plant Sci. Biotechnol. 2, 1–24.
Philippot, L., Raaijmakers, J. M., Lemanceau, P., and van der Putten, W. H. (2013). Going back to the roots: the microbial ecology of the rhizosphere. Nat. Rev. Microbiol. 11, 789–799. doi: 10.1038/nrmicro3109
Pliego, C., Cazorla, F. M., González-Sánchez, M. Á., Pérez-Jiménez, R. M., de Vicente, A., and Ramos, C. (2007). Selection for biocontrol bacteria antagonistic toward Rosellinia necatrix by enrichment of competitive avocado root tip colonizers. Res. Microbiol. 158, 463–470. doi: 10.1016/j.resmic.2007.02.011
Pliego, C., Kanematsu, S., Ruano-Rosa, D., de Vicente, A., López-Herrera, C., Cazorla, F., et al. (2009). GFP sheds light on the infection process of avocado roots by Rosellinia necatrix. Fungal Genet. Biol. 46, 137–145. doi: 10.1016/j.fgb.2008.11.009
Pliego, C., López-Herrera, C., Ramos, C., and Cazorla, F. M. (2012). Developing tools to unravel the biological secrets of Rosellinia necatrix, an emergent threat to woody crops. Mol. Plant Pathol. 13, 226–239. doi: 10.1111/j.1364-3703.2011.00753.x
Prigigallo, M. I., Staropoli, A., Vinale, F., and Bubici, G. (2023). Interactions between plant-beneficial microorganisms in a consortium: Streptomyces microflavus and Trichoderma harzianum. Microb. Biotechnol. 16, 2292–2312. doi: 10.1111/1751-7915.14311
Pruesse, E., Quast, C., Knittel, K., Fuchs, B. M., Ludwig, W., Peplies, J., et al. (2007). SILVA: a comprehensive online resource for quality checked and aligned ribosomal RNA sequence data compatible with ARB. Nucleic Acids Res. 35, 7188–7196. doi: 10.1093/nar/gkm864
Qiao, R., Xu, M., Jiang, J., Song, Z., Wang, M., Yang, L., et al. (2024). Plant growth promotion and biocontrol properties of a synthetic community in the control of apple disease. BMC Plant Biol. 24:546. doi: 10.1186/s12870-024-05253-8
Qiu, Z., Wang, J., Delgado-Baquerizo, M., Trivedi, P., Egidi, E., Chen, Y. M., et al. (2020). Plant microbiomes: do different preservation approaches and primer sets alter our capacity to assess microbial diversity and community composition? Front. Plant Sci. 11:993. doi: 10.3389/fpls.2020.00993
Qu, Q., Zhang, Z., Peijnenburg, W., Liu, W., Lu, T., Hu, B., et al. (2020). Rhizosphere microbiome assembly and its impact on plant growth. J. Agric. Food Chem. 68, 5024–5038. doi: 10.1021/acs.jafc.0c00073
Quast, C., Pruesse, E., Yilmaz, P., Gerken, J., Schweer, T., Yarza, P., et al. (2013). The SILVA ribosomal RNA gene database project: improved data processing and web-based tools. Nucleic Acids Res. 41, D590–D596. doi: 10.1093/nar/gks1219
R Core Team (2021). R: A Language and Environment for Statistical Computing [Online]. Vienna: R Foundation for Statistical Computing.
Ramírez-Gil, J. G., Castañeda-Sánchez, D. A., and Morales-Osorio, J. G. (2017). Production of avocado trees infected with Phytophthora cinnamomi under different management regimes. Plant Pathol. 66, 623–632. doi: 10.1111/ppa.12620
Ren, C., Zhang, X., Zhang, S., Wang, J., Xu, M., Guo, Y., et al. (2021). Altered microbial CAZyme families indicated dead biomass decomposition following afforestation. Soil Biol. Biochem. 160:108362. doi: 10.1016/j.soilbio.2021.108362
Reverchon, F., García-Meléndez, M., Guevara-Avendaño, E., Mora-Chávez, O., Solís-García, I. A., Dáttilo, W., et al. (2023). Shifts in the rhizosphere microbiome and exudation profile of avocado (Persea americana Mill.) during infection by Phytophthora cinnamomi and in presence of a biocontrol bacterial strain. CABI Agric. Biosci. 4:23. doi: 10.1186/s43170-023-00167-1
Rolfe, S. A., Griffiths, J., and Ton, J. (2019). Crying out for help with root exudates: adaptive mechanisms by which stressed plants assemble health-promoting soil microbiomes. Curr. Opin. Microbiol. 49, 73–82. doi: 10.1016/j.mib.2019.10.003
Ruano-Rosa, D., Del Moral-Navarrete, L., and López-Herrera, C. (2010). Selection of Trichoderma spp. isolates antagonistic to Rosellinia necatrix. Span. J. Agric. Res. 8, 1084–1097. doi: 10.5424/sjar/2010084-1403
Ruano-Rosa, D., and López-Herrera, C. J. (2009). Evaluation of Trichoderma spp. as biocontrol agents against avocado white root rot. Biol. Control 51, 66–71. doi: 10.1016/j.biocontrol.2009.05.005
Santos, L. F., and Olivares, F. L. (2021). Plant microbiome structure and benefits for sustainable agriculture. Curr. Plant Biol. 26:100198. doi: 10.1016/j.cpb.2021.100198
Sasse, J., Martinoia, E., and Northen, T. (2018). Feed your friends: do plant exudates shape the root microbiome? Trends in Plant Sci. 23, 25–41. doi: 10.1016/j.tplants.2017.09.003
Sayers, E. W., Cavanaugh, M., Clark, K., Ostell, J., Pruitt, K. D., Karsch-Mizrachi, I., et al. (2020). GenBank. Nucleic Acids Res. 48, D84–D86. doi: 10.1093/nar/gkz956
Schena, L., Duncan, J., and Cooke, D. (2008). Development and application of a PCR-based ‘molecular tool box' for the identification of Phytophthora species damaging forests and natural ecosystems. Plant Pathol. 57, 64–75. doi: 10.1111/j.1365-3059.2007.01689.x
Schena, L., Nigro, F., and Ippolito, A. (2002). Identification and detection of Rosellinia necatrix by conventional and real-time scorpion-PCR. Eur. J. Plant Pathol. 108, 355–366. doi: 10.1023/A:1015697813352
Sena, K., Dreaden, T. J., Crocker, E., and Barton, C. (2018). Detection of Phytophthora cinnamomi in forest soils by PCR on DNA extracted from leaf disc baits. Plant Health Prog. 19, 193–200. doi: 10.1094/PHP-01-18-0004-RS
Sherwood, R., and Hagedorn, D. J. (1958). Determining Common Root Rot Potential of Pea Fields. Agricultural Experiment Station, University of Wisconsin–Madison.
Shishido, M., Kubota, I., and Nakamura, H. (2012). Development of real-time PCR assay using TaqMan probe for detection and quantification of Rosellinia necatrix in plant and soil. J. Gen. Plant Pathol. 78, 115–120. doi: 10.1007/s10327-012-0366-x
Shu, B., Liu, L., Wei, Y., Zhang, D., and Shi, S. (2019). Differential selection pressure exerted by root rot disease on the microbial communities in the rhizosphere of avocado (Persea americana Mill.). Ann. Appl. Biol. 175, 376–387. doi: 10.1111/aab.12547
Singh, P., Singh, R. K., Zhou, Y., Wang, J., Jiang, Y., Shen, N., et al. (2022). Unlocking the strength of plant growth promoting Pseudomonas in improving crop productivity in normal and challenging environments: a review. J. Plant Interact. 17, 220–238. doi: 10.1080/17429145.2022.2029963
Snelders, N. C., Rovenich, H., and Thomma, B. P. (2022). Microbiota manipulation through the secretion of effector proteins is fundamental to the wealth of lifestyles in the fungal kingdom. FEMS Microbiol. Rev. 46:fuac022. doi: 10.1093/femsre/fuac022
Solís-García, I. A., Ceballos-Luna, O., Cortazar-Murillo, E. M., Desgarennes, D., Garay-Serrano, E., Patiño-Conde, V., et al. (2021). Phytophthora root rot modifies the composition of the avocado rhizosphere microbiome and increases the abundance of opportunistic fungal pathogens. Front. Microbiol. 11:3484. doi: 10.3389/fmicb.2020.574110
Sukno, S. A., García, V. M., Shaw, B. D., and Thon, M. R. (2008). Root infection and systemic colonization of maize by Colletotrichum graminicola. Appl. Environ. Microbiol. 74, 823–832. doi: 10.1128/AEM.01165-07
ten Hoopen, G. M., and Krauss, U. (2006). Biology and control of Rosellinia bunodes, Rosellinia necatrix and Rosellinia pepo: a review. Crop Prot. 25, 89–107. doi: 10.1016/j.cropro.2005.03.009
Trivedi, P., He, Z., Van Nostrand, J. D., Albrigo, G., Zhou, J., Wang, N., et al. (2012). Huanglongbing alters the structure and functional diversity of microbial communities associated with citrus rhizosphere. ISME J. 6, 363–383. doi: 10.1038/ismej.2011.100
van den Berg, N., Hartley, J., Engelbrecht, J., Mufamadi, Z., Van Rooyen, Z., Mavuso, Z., et al. (2018). First report of white root rot caused by Rosellinia necatrix on Persea americana in South Africa. Plant Dis. 102:1850. doi: 10.1094/PDIS-10-17-1637-PDN
van den Berg, N., Magagula, P., Backer, R., and Swart, V. (2025). Towards developing an integrated approach for the treatment of white root rot in commercial avocado orchards. Phytoparasitica 53:32. doi: 10.1007/s12600-025-01250-1
van der Merwe, J., and Matthee, F. (1974). Rosellinia-root rot of apple and pear trees in South Africa. Phytophylactica 5, 55–58.
Wen, T., Ding, Z., Thomashow, L. S., Hale, L., Yang, S., Xie, P., et al. (2023). Deciphering the mechanism of fungal pathogen-induced disease-suppressive soil. New Phytol. 238, 2634–2650. doi: 10.1111/nph.18886
Wickham, H. (2016). ggplot2: Elegant Graphics for Data Analysis. New York: Springer-Verlag. doi: 10.1007/978-3-319-24277-4_9
Wittstein, K., Cordsmeier, A., Lambert, C., Wendt, L., Weber, J., Wurzler, N., et al. (2020). Identification of Rosellinia species as producers of cyclodepsipeptide PF1022 A and resurrection of the genus Dematophora as inferred from polythetic taxonomy. Stud. Mycol. 96, 1–16. doi: 10.1016/j.simyco.2020.01.001
Yang, C. H., Crowley, D., and Menge, J. (2001). 16S rDNA fingerprinting of rhizosphere bacterial communities associated with healthy and Phytophthora infected avocado roots. FEMS Microbiol. Ecol. 35, 129–136. doi: 10.1111/j.1574-6941.2001.tb00796.x
Yu, K., Liu, Y., Tichelaar, R., Savant, N., Lagendijk, E., Van Kuijk, S. J., et al. (2019). Rhizosphere-associated Pseudomonas suppress local root immune responses by gluconic acid-mediated lowering of environmental pH. Curr. Biol. 29, 3913–3920, e4. doi: 10.1016/j.cub.2019.09.015
Keywords: Dematophora necatrix, metabarcoding, microbiome, soil physicochemical properties, Persea americana
Citation: Magagula P, Swart V, Fourie A, Vermeulen A, Nelson JH, van Rooyen Z and van den Berg N (2025) Avocado rhizosphere community profiling: white root rot and its impact on microbial composition. Front. Microbiol. 16:1583797. doi: 10.3389/fmicb.2025.1583797
Received: 26 February 2025; Accepted: 25 April 2025;
Published: 23 May 2025.
Edited by:
Agnieszka Kuźniar, The John Paul II Catholic University of Lublin, PolandReviewed by:
Artur Banach, The John Paul II Catholic University of Lublin, PolandHao Tan, Sichuan Academy of Agricultural Sciences, China
Copyright © 2025 Magagula, Swart, Fourie, Vermeulen, Nelson, van Rooyen and van den Berg. This is an open-access article distributed under the terms of the Creative Commons Attribution License (CC BY). The use, distribution or reproduction in other forums is permitted, provided the original author(s) and the copyright owner(s) are credited and that the original publication in this journal is cited, in accordance with accepted academic practice. No use, distribution or reproduction is permitted which does not comply with these terms.
*Correspondence: Noëlani van den Berg, bm9lbGFuaS52ZGJlcmdAZmFiaS51cC5hYy56YQ==