- 1Molecular Enzyme Technology and Biochemistry, Environmental Microbiology and Biotechnology, Centre for Water and Environmental Research (CWE), University of Duisburg-Essen, Essen, Germany
- 2Henry Wellcome Building for Biocatalysis, Biosciences, Faculty of Health and Life Sciences, University of Exeter, Exeter, United Kingdom
- 3Istituto di Scienze e Tecnologie Chimiche “G. Natta” (SCITEC), Consiglio Nazionale delle Ricerche, Milan, Italy
- 4Winogradsky Institute of Microbiology, Federal Research Center of Biotechnology, Russian Academy of Sciences, Moscow, Russia
- 5Living Systems Institute, Faculty of Health and Life Sciences, University of Exeter, Exeter, United Kingdom
- 6Corporate Innovation, Evonik Industries AG, Essen, Germany
- 7Department of Biochemistry, University of Stellenbosch, Matieland, South Africa
- 8Department of Molecular Cell Biology, Vrije Universiteit, Amsterdam, Netherlands
Extremolytes – unique compatible solutes produced by extremophiles - protect biological structures like membranes, proteins, and DNA under extreme conditions, including extremes of temperature and osmotic stress. These compounds hold significant potential for applications in pharmaceuticals, healthcare, cosmetics, and life sciences. However, despite their considerable potential, only a limited number of extremolytes – most notably ectoine and hydroxyectoine – have achieved commercial relevance, primarily due to the absence of efficient production strategies for the majority of other extremolytes. Cyclic 2,3-diphosphoglycerate (cDPG), a unique metabolite found in certain hyperthermophilic methanogenic Archaea, plays a key role in thermoprotection and is synthesized from 2-phosphoglycerate (2PG) through a two-step enzymatic process involving 2-phosphoglycerate kinase (2PGK) and cyclic-2,3-diphosphoglycerate synthetase (cDPGS). In this study, we present the development of an efficient in vitro enzymatic approach for the production of cDPG directly from 2,3-diphosphoglycerate (2,3DPG), leveraging the activity of the cDPGS from Methanothermus fervidus (MfcDPGS). We optimized the heterologous production of MfcDPGS in Escherichia coli by refining codon usage and expression conditions. The purification process was significantly streamlined through an optimized heat precipitation step, coupled with effective stabilization of MfcDPGS for both usage and storage by incorporating KCl, Mg2+, reducing agents and omission of an affinity tag. The recombinant MfcDPGS showed a Vmax of 38.2 U mg−1, with KM values of 1.52 mM for 2,3DPG and 0.55 mM for ATP. The enzyme efficiently catalyzed the complete conversion of 2,3DPG to cDPG. Remarkably, even at a scale of 100 mM, it achieved full conversion of 37.6 mg of 2,3DPG to cDPG within 180 min, using just 0.5 U of recombinant MfcDPGS at 55°C. These results highlight that MfcDPGS can be easily produced, rapidly purified, and sufficiently stabilized while delivering excellent conversion efficiency for cDPG synthesis as value added product. Additionally, a kinetic model for MfcDPGS activity was developed, providing a crucial tool to simulate and scale up cDPG production for industrial applications. This streamlined process offers significant advantages for the scalable synthesis of cDPG, paving the way for further biochemical and industrial applications of this extremolyte.
1 Introduction
Compatible solutes are ubiquitous and distributed across all three domains of life, accumulating within cells to high concentrations (up to 2 M) in response to diverse environmental stressors such as heat, cold, osmotic stress, and desiccation. These solutes integrate seamlessly with cellular metabolism, enabling organisms to thrive under harsh conditions by stabilizing and protecting nucleic acids, proteins and cellular structures (da Costa et al., 1998; Lamosa et al., 1998). Cells acquire compatible solutes either from their environment or synthesize them de-novo or semi-de-novo by use of precursor molecules. While their diversity is vast, compatible solutes generally fall into a few different chemical categories, including (i) amino acids (e.g., α-glutamate, β-glutamate) and amino acid derivatives (e.g., glycine-betaine, ectoine, hydroxyectoine) and (ii) sugars (e.g., trehalose, mannosylglycerate), polyols (e.g., glycerol) and their derivatives (Empadinhas and da Costa, 2006). Their remarkable protective properties for enzymes, DNA, membranes and entire cells have led to their incorporation into various commercial applications across industries such as food, health and consumer care, and cosmetics (Becker and Wittmann, 2020). Extremolytes - compatible solutes synthesized by extremophiles such as halophiles or (hyper) thermophiles - have garnered significant industrial interest. Beyond the ubiquitous, widely utilized compatible solute trehalose, the most commercially prominent extremolytes are ectoine and hydroxyectoine. (Lentzen and Schwarz, 2006a; Lentzen and Schwarz, 2006b; Lippert and Galinski, 1992; Kunte et al., 2014; Becker and Wittmann, 2020). Additionally, phosphorylated compounds have been identified as extremolytes in hyperthermophiles, including di-myo-1,1′-inositol-phosphate (DIP) (Scholz et al., 1992; Martins and Santos, 1995), α-diglycerol phosphate (DGP) (Borges et al., 2006), and cyclic 2,3-diphosphoglycerate (cDPG) (Seely and Fahrney, 1983; Hensel and König, 1988; Lehmacher et al., 1990). These compounds have been shown to protect cellular components and structures from damage in these hypthermophilic organisms under stress conditions (Lentzen and Schwarz, 2006b). The extremolyte cDPG has been uniquely reported in the hyperthermophilic Methanoarchaea, such as Methanothermus fervidus, Methanopyrus kandleri and Methanothermobacter thermoautotrophicus, at concentrations ranging from 0.3–1.1 M (Hensel and König, 1988; Ciulla et al., 1994; Matussek et al., 1998; Shima et al., 1998). It contributes to protein thermoprotection and has been shown to enhance the thermostability of various model enzymes (Hensel and Jakob, 1993; Shima et al., 1998; Borges et al., 2002). Additionally, cDPG protects plasmid DNA from oxidative damage by hydroxyl radicals and acts as a superoxide scavenger (Lentzen and Schwarz, 2006b). However, despite its promising properties, particularly cDPG and more generally also the phosphorylated extremolytes remain largely untapped for biotechnological and industrial applications, primarily due to the lack of suitable production hosts and cost-effective synthesis strategies.
The enzymatic synthesis of cDPG involves two distinct catalytic steps. First, 2-phosphoglycerate kinase (2PGK) catalyzes the conversion of 2-phosphoglycerate (2PG) into the phosphate diester 2,3-diphosphoglycerate (2,3DPG). In the subsequent step, cyclic-2,3-diphosphoglycerate synthetase (cDPGS) forms an intramolecular phosphoanhydride bond, yielding cDPG (Figure 1A). Despite the well-documented protective properties of cDPG and its industrial potential, an efficient production process remains elusive. This challenge stems from several factors, including the low cell yields and demanding cultivation requirements of natural producer strains, such as the obligate anaerobic growth conditions, high temperature and salt requirements of these methanogens. In addition, the lack of genetic tools for these organisms make a whole-cell in vivo approach for cDPG production in natural hosts impractical.
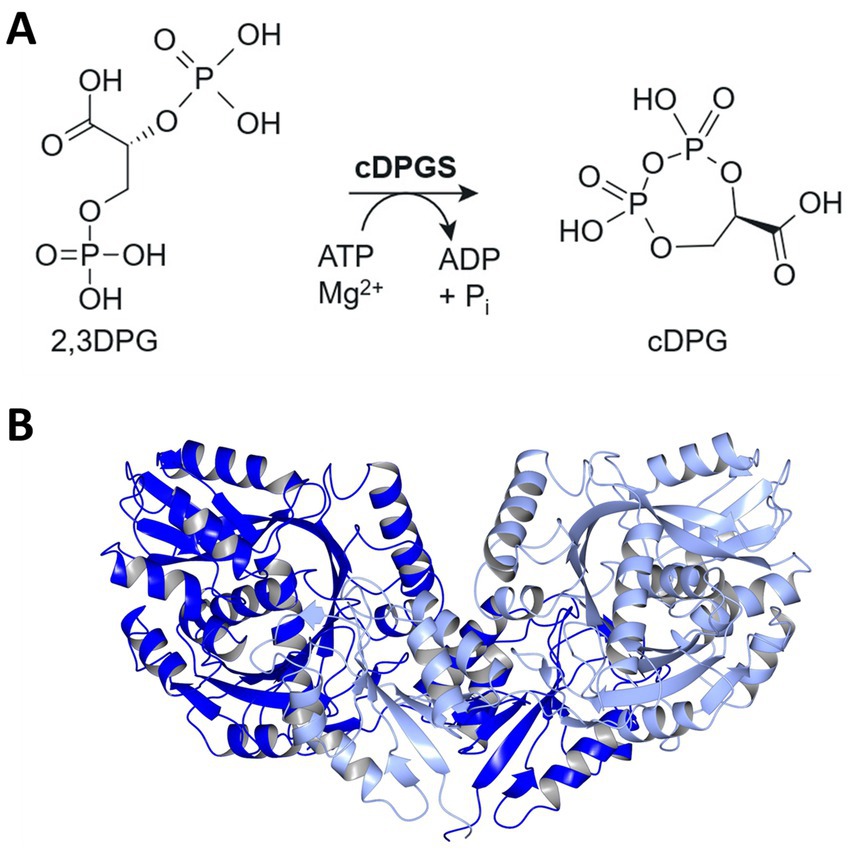
Figure 1. Pathway for cDPG synthesis and structure of MfcDPGS dimer. (A) Schematic representation of the enzymatic synthesis of cyclic 2,3-diphosphoglycerate (cDPG) by the formation of an intramolecular phosphoanhydride bond in 2,3-diphosphoglycerate (2,3DPG). (B) Enzyme structure of the Methanothermus fervidus cDPGS dimer PDB 8ORU viewed perpendicular to its molecular dyad. The two subunits are shown in blue and light blue [visualized using CCP4mg (McNicholas et al., 2011)].
In a previous study, we successfully employed an in vivo metabolic engineering approach to produce cDPG in the thermophilic bacterium Thermus thermophilus. By introducing the Mf2PGK and MfcDPGS from M. fervidus, into T. thermophilus HB27, we achieved in vivo production of 650 μM cDPG within 16 h (De Rose et al., 2021). More recently, we solved the crystal structure of the MfcDPGS (PDB 8ORK, Figure 1B), including its complex with 2,3DPG and ADP (PDB 8ORU), offering novel mechanistic insights (De Rose et al., 2023). Notably, the MfcDPGS features a unique N-terminal domain (127 amino acids) with a novel fold, which is essential for stabilizing the active dimeric structure and facilitating ligand interactions (De Rose et al., 2023). In this study as an alternative approach to in vivo production in T. thermophilus, we explored an in vitro enzymatic approach for large-scale synthesis of cDPG. Therefore, to improve the efficiency of heterologous MfcDPGS expression in Escherichia coli, we applied codon optimization, omitted the affinity tag, refined expression conditions, and streamlined the purification process by incorporating a simple heat precipitation step. Additionally, we optimized the stabilization conditions of the recombinant protein for both immediate use and long-term storage. Finally, we demonstrated complete substrate conversion to the product, precisely described by our mathematical model, enabling straightforward upscaling and downstream processing of cDPG for future applications.
2 Materials and methods
2.1 Cloning, heterologous expression and purification of the recombinant proteins
2.1.1 Cloning
Initially, the cyclic 2,3-diphosphoglycerate synthetase (cdpgs, 1,383 bp) from M. fervidus (Mfer_0077, CAA70986) was amplified via PCR from chromosomal DNA of M. fervidus (for primers see Supplementary Table 1). The PCR product was purified using the Wizard® SV Gel and PCR Clean-Up kit (Promega, Fitchburg, USA) according to the instructions of the manufacturer. Restricted DNA fragments were cloned into the expression vector pET24a (KanR) (Merck, Darmstadt, Germany) with a C-terminal His-tag and were transformed into E. coli DH5α (Life Technologies, Carlsbad, USA). Later, the cdpgs gene was codon optimized (Eurofins, Ebersberg, Germany) for expression in E. coli and subcloned in pET15b (AmpR) (Merck, Darmstadt, Germany) either with a N-terminal His-tag or without an affinity tag (for primers see Supplementary Table 1). All sequences and plasmids were verified by sequencing (LGC genomics, Berlin, Germany).
2.1.2 Heterologous expression
Competent cells of the E. coli expression strains – BL21 (DE3) and BL21 (DE3) CodonPlus-pRIL (CamR, Merck, Darmstadt, Germany), and Rosetta (DE3) (CamR, Stratagene, San Diego, USA) – were transformed with the constructed plasmids and grown in 200 mL – 400 mL lysogeny broth (LB, Carl Roth GmbH + Co. KG, Karlsruhe, Germany) containing the appropriate antibiotics at different expression temperatures (37°C, 30°C, and 16°C). The expression was induced by the addition of 1 mM isopropyl-β-d-thiogalactopyranoside (IPTG) at an OD600 between 0.6–0.8. Cultures were incubated in a Unitron shaker (Infors, Basel, Switzerland) over night at 180 rpm and cells were harvested by centrifugation (6,000 x g, 20 min, 4°C). For affinity chromatography of His-tagged MfcDPGS, 1 g of cells (wet weight) were resuspended in 5 mL of 50 mM potassium phosphate buffer pH 8.0 containing 2.5 mM MgCl2, 5 mM DTT, and 300 mM KCl. For MfcDPGS purification without affinity-tag, 1 g of cells were resuspended in 50 mM 2-(N-morpholino) ethanesulfonic acid (MES/KOH) supplemented with 10 mM DTT, 10 mM MgCl2, and 400 mM KCl, at pH 6.5. For cell lysis, cells were passed three times through a French pressure cell at 20,000 psi. Cell debris was removed by centrifugation (25,000 x g, 45 min, 4°C).
2.1.3 Purification
The crude extract containing the recombinant thermophilic MfcDPGS was subjected to a heat precipitation step at 75°C for 30 min in an Eppendorf Thermomixer at 700 rpm (Eppendorf, Hamburg, Germany) followed by centrifugation (25,000xg, 45 min, 4°C) to remove denatured mesophilic host proteins. The His-tagged MfcDPGS was purified using affinity chromatography via Protino® Ni- tris-carboxymethyl ethylene diamine (TED) columns (0.25 g columns for 9.6 mg (1.37 mg/mL) and 0.5 g columns for 20.8 mg (4.15 mg/mL) total protein in the soluble fraction after heat precipitation) (Macherey-Nagel GmbH & Co. KG, Düren, Germany). The manufacturer’s instructions were followed with the exception of buffer composition during equilibration and washing. Instead of the supplied 50 mM sodium phosphate (NaH2PO4) (pH 8.0), 300 mM NaCl, 50 mM potassium phosphate (K2HPO4/KH2PO4) (pH 8), 2.5 mM MgCl2, 5 mM DTT, 300 mM KCl was used. This adjustment was made due to the methanogenic origin of the MfcDPGS, as the intracellular K+ concentration of M. fervidus ranges from 500 to 900 mM under optimal growth conditions, much higher than that of mesophilic methanogens or other thermophilic archaea (Hensel and König, 1988). For elution, the potassium phosphate buffer was supplemented with 250 mM imidazole. Elution fractions containing MfcDPGS activity were pooled and dialyzed using Spectra/Por® standard RC dialysis membrane (Repligen, Waltham, USA) with a molecular weight cutoff of 30 kDa. For the recombinant MfcDPGS without an affinity tag, the protein purification was further modified. The crude extract (13.1 mg/mL) was diluted with 50 mM MES/KOH (pH 6.5), 10 mM DTT, 10 mM MgCl2, and 400 mM KCl prior to the heat precipitation step. Dilutions of 1:2 (~ 6.6 mg/mL), 1:4 (~ 3.3 mg/mL), 1:6 (~ 2.2 mg/mL) were tested and incubated at 75°C for 30 min in an Eppendorf Thermomixer at 700 rpm (Eppendorf, Hamburg, Germany), followed by centrifugation (25,000xg, 45 min, 4°C) to remove denatured mesophilic host proteins. For further experiments a dilution at a 1:6 ratio was used.
Additional purification was achieved by size exclusion chromatography (SEC). Therefore, the soluble fraction after heat precipitation was concentrated using a Vivaspin® concentrator (30 kDa cutoff, Sartorius, Göttingen, Germany), filtered (0.45 μm polyvinylidene fluoride membrane, Carl Roth, Germany), and 5 mg of protein were loaded onto a Superose Increase 10/300 column (Cytiva Life Sciences, Freiburg, Germany). The column (24 mL volume, flow rate 0.5 mL/min) was equilibrated in 50 mM MES/KOH (pH 6.5), 400 mM KCl. Protein expression, purification and subunit molecular mass of MfcDPGS were analyzed by denaturing SDS-polyacrylamide gel electrophoresis. All protein concentrations were determined using a modified Bradford method (Zor and Selinger, 1996) with bovine serum albumin as a standard (Bio-Rad, Feldkirchen, Germany).
2.2 Methanothermus fervidus cDPGS activity
2.2.1 Continuous PK-LDH assay
The enzymatic activity of MfcDPGS was determined at 55°C by coupling the ADP formation from ATP to the oxidation of NADH, using pyruvate kinase (PK) and L-lactate dehydrogenase (LDH) from rabbit muscle (Merck, Darmstadt, Germany) as auxiliary enzymes. The assay mixtures (total volume 0.5 mL) contained 50 mM MES/KOH (pH 6.5) with 10 mM MgCl2, 400 mM KCl, 2.5 mM ATP, 0.5 mM NADH, 2 mM phosphoenolpyruvate (PEP), 8 U PK, 4 U LDH and 4.8 μg purified MfcDPGS (0.69 mg/mL). After 1 min preincubation at 55°C the reactions were started by the addition of 2,3DPG and the oxidation of NADH was followed in a Specord UV/VIS spectrophotometer (Analytic Jena AG, Jena, Germany) at 340 nm (extinction coefficient 6.22 mM−1 cm−1). To determine the kinetic constants for 2,3DPG and ATP, their concentrations were varied between 0 mM and 10 mM, while maintaining a constant Mg2+/ATP molar ratio of 0.5. Experimental data were fitted, and kinetic constants were determined using the NonlinearModelFit function in Wolfram Mathematica with a two substrate irreversible Michaelis Menten equation. The substrate 2,3DPG was used either as penta-sodium salt or as penta-cyclohexylammonium-salt (Merck, Darmstadt, Germany). It was ensured that the auxiliary enzymes were not rate limiting. One unit (1 U) of enzyme activity is defined as 1 μmol substrate of product (ADP) formed per minute. All measurements were performed in triplicates.
2.2.2 Effect of salt and pH
The effect of salt on MfcDPGS enzyme activity was analyzed in the presence of KCl using the continuous PK-LDH assay at 55°C, with varying salt concentrations ranging from 0 to 400 mM KCl. The pH optimum was determined over a range from pH 5.5 to 8.0, using the following buffers: 50 mM MES/KOH (pH 5.5, 6.5), 50 mM potassium phosphate buffer pH 6.8 and 50 mM HEPES/KOH pH 7.0 and 8.0, all buffers were supplemented with 400 mM KCl and 10 mM MgCl2.
2.2.3 Optimal Mg2+/ATP ratio
To determine the optimal molar ratio of Mg2+/ATP for the synthesis of cDPG, the formation of ADP from ATP was coupled to the oxidation of NADH via the continuous PK-LDH assay at 55°C using 50 mM MES/KOH (pH 6.5), 400 mM KCl, 10 mM DTT, 10 mM 2,3DPG and 4.8 μg of the purified MfcDPGS (0.69 mg/mL). The assays were performed in the presence of different molar ratios of Mg2+/ATP (a concentration range of 1–50 mM was investigated, corresponding to a molar ratio of 0.02–1.0). It was ensured that the auxiliary enzymes as well as 2,3DPG were not rate limiting. All experiments were performed in triplicate.
2.2.4 Long-term stability
The stability of purified MfcDPGS was analyzed over a period of several month. To initiate the analysis, the enzyme stocks stored at −70°C were carefully thawed on ice and mixed thoroughly by pipetting. Subsequently, the residual enzymatic activity was measured using the continuous PK-LDH assay at 55°C.
2.2.5 Discontinuous assays for analysis of 2,3DPG conversion to cDPG
To determine the conversion of 1–100 mM 2,3DPG to cDPG over time, discontinuous enzyme assays were performed at 55°C. The assays were conducted in a reaction buffer containing 50 mM MES/KOH, 400 mM KCl, and 10 mM DTT, pH 6.5. The molar ratio of 2,3DPG to ATP was maintained at 1:2, with 2,3DPG concentrations ranging from 1 to 100 mM and ATP concentrations from 2 to 200 mM. Additionally, the Mg2+/ATP ratio was adjusted between 0.2 and 0.5 to optimize enzyme activity. 1 mL of the reaction mixtures were incubated at 850 rpm and 55°C in an Eppendorf ThermoMixer® (Eppendorf, Hamburg, Germany). Assays were performed in the presence of 0.5 U of the purified MfcDPGS. The protein was used from two different enzyme purifications. The concentration was 0.49 mg/mL used in 1–10 mM conversions, and 0.55 mg/mL used in 25–100 mM conversions. At regular time intervals (0 min – 1,250 min), 100 μL samples were withdrawn into pre-cooled reaction tubes and immediately stored at −70°C to halt the reaction. For the negative control, the same concentrations of 2,3DPG and ATP were used, but MfcDPGS was omitted. ADP formation from ATP at each time point was quantified using the PK-LDH assay as an indicator reaction. The indicator reaction was performed at 25°C in a total volume of 200 μL in 96-well plates (Sarstedt, Nümbrecht, Germany) using a Tecan Microplate Reader at 340 nm (Tecan group Ltd., Lifesciences, Männedorf, Switzerland). To quantify the NADH formed, a standard curve ranging from 0–0.7 mM NADH was used. To monitor the conversion of 2,3DPG to cDPG, aliquots from the enzyme assay were analyzed by 31P-NMR spectroscopy (Bruker Avance Neo 400 MHz, Bruker, Rheinstetten, Germany). Spectra were acquired in a H2O/D2O (8:2, v/v) mixture. Therefore, thawed samples from the respective time points were mixed with 20% (v/v) D2O (Merck, Darmstadt, Germany) and the assay buffer 50 mM MES/KOH, 400 mM KCl, pH 6.5. D2O was used in all experiments to provide a stable lock signal for the spectrometer (1H-NMR for NMR lock). The deuterium signal is used to stabilize the magnetic field, minimizing drift during long acquisitions and improving spectral quality. Spectra were processed, normalized and integrated using the TopSpin© 3.6.2 software (Bruker BioSpin, Rheinstetten, Germany). As a positive control, for product validation 5 mM cDPG was dissolved in 50 mM MES/KOH supplemented with 400 mM KCl, 10 mM MgCl2, pH 6.5 and 20% (v/v) D2O.
3 Results
3.1 Expression and purification of the Methanothermus fervidus cDPGS
Initially, the gene encoding MfcDPGS (cdpgs, Mfer_0077, CAA70986) was cloned into the pET24a vector with a C- terminal His-tag for recombinant expression in E. coli (Supplementary Figure S1). However, despite using various expression strains (Supplementary Table 1), and lowering the expression temperature to 16°C, the expression of the tagged recombinant MfcDPGS resulted only in low amounts of soluble protein. While small quantities were enriched in heat precipitation fractions at 75°C (1.37 mg/mL), the protein did not bind to the Ni-TED affinity column (Supplementary Figure S1). Hence, the cdpgs gene was further analyzed using the GenScript Rare Codon Analysis Tool (https://www.genscript.com/tools/rare-codon-analysis). The cdpgs sequence has a GC-content of 32.8%, and the Codon Adaption Index (CAI), which serves as a quantitative measure to predict gene expression levels in E. coli, yielded a value of only 0.54 (Supplementary Figures S2, S3). Additionally, the Codon Frequency Distribution (CFD) analysis, which compares the distribution of codons in cdpgs with the typical codon usage in E coli, revealed 14 codons with frequencies below 31%, which are likely to impair expression efficiency in E. coli (Supplementary Figure S4). To address this, the cdpgs gene was synthesized as a codon optimized construct (Eurofins, Ebersberg, Germany, CAI = 0.75, GC-content 47.2%) for improved expression in E. coli (Supplementary Table S2). For recombinant expression, the codon-optimized cdpgs was subcloned in pET15b (with N-terminal His-tag) and expressed in E. coli BL21(DE3)-CodonPlus-pRIL. This approach yielded higher amounts of soluble protein (4.15 mg/mL and 0.053 mg/mL after Ni-TED affinity chromatography) (Supplementary Figure S5). However, the obtained His-tagged MfcDPGS was relatively unstable in solution and precipitated during dialysis to remove imidazole. To address this, we expressed the codon optimized gene without the affinity tag and reduced the expression temperature to 16°C. After overnight expression, the majority of recombinant protein remained in the insoluble/membrane fraction (as was also observed for the tagged recombinant MfcDPGS in the unoptimized procedures described above), but a substantially higher amount of MfcDPGS could be detected in the soluble fraction (Supplementary Figure S6). The soluble, recombinant enzyme was purified to apparent homogeneity using a heat precipitation step (30 min at 75°C). We tested different protein concentrations (6.5–2.2 mg/mL), and 2.2 mg/mL yielded the best results for purity (Supplementary Figure S7). Size exclusion chromatography (SEC) was then used for further purification. From a 400 mL expression culture (1.7 g cell wet weight), 3.5 mg of recombinant MfcDPGS was obtained after size exclusion chromatography. The molecular mass of the recombinant MfcDPGS was determined under denaturing conditions by SDS-PAGE (Figure 2) and revealed a double band with a major band at 52 kDa which closely matches the calculated molecular mass of 50.7 kDa. The double band observed in SDS-PAGE analysis (Figure 2) has also been reported previously for the MfcDPGS (Matussek et al., 1998) and was attributed to conformational differences or chemical modifications, possibly further influenced by the instability of the protein. Analyses of the native molecular mass via SEC (Supplementary Figure S8) revealed that the MfcDPGS adopts both, a homodimeric (119 kDa) as well as a homotetrameric structure (218 kDa). However, the significantly higher protein yield (3.5 mg) and markedly higher specific activity (21.7 U/mg) of the homodimer compared to the tetramer (9.3 U/mg) indicates – in line with previous findings (Matussek et al., 1998; de Rose et al., 2023) – that the homodimer represents the functionally relevant conformation.
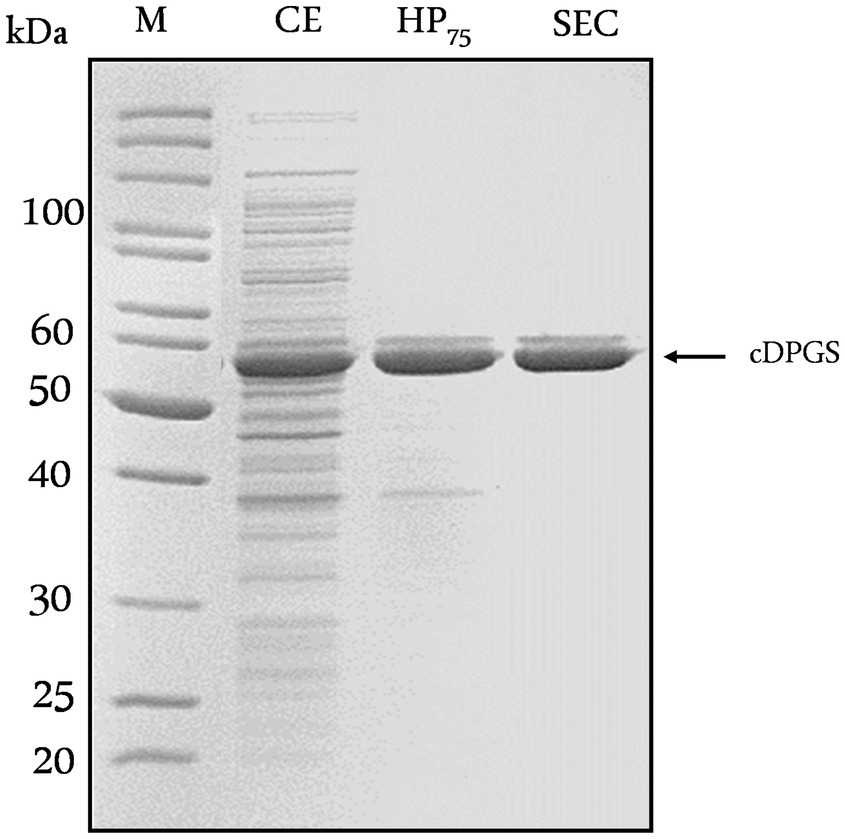
Figure 2. Purification of recombinant MfcDPGS expressed in Escherichia coli. The codon-optimized cdpgs gene was cloned into the pET15b vector without an affinity tag, and expression was optimized in Escherichia coli BL21(DE3)-Codon-Plus. The recombinant protein was then purified by heat precipitation and size exclusion chromatography (SEC). Protein samples (5–10 μg) from each purification steps were analyzed by SDS-PAGE (12.5%) and the gel was stained with Coomassie Brilliant Blue. CE: Crude extract, HP75: Soluble fraction after heat precipitation (75°C, 30 min), SEC: Fraction after size exclusion chromatography. M: Protein marker, unstained protein ladder (Thermo Fisher Scientific, Carlsbad, USA).
3.2 Characterization of the Methanothermus fervidus cDPGS
MfcDPGS catalyzes the ATP-depended formation of an intramolecular phosphoanhydride bond in 2,3DPG, resulting in cyclic 2,3-diphosphoglycerate (cDPG, Figure 1A). The enzyme activity of the purified MfcDPGS (without a tag, after SEC) was determined at 55°C by following the ADP-formation from ATP using the continuous PK-LDH assay.
Preliminary tests (Mg2+/ATP ratio of 1.0) revealed that the activity of MfcDPGS is highly salt-dependent, with a 5.5-fold activation observed in the presence of 400 mM KCl (Figure 3A). The optimal pH for MfcDPGS activity was found to be at pH 6.5 (Figure 3B). The optimal buffer conditions for enzyme characterization were identified as 50 mM MES/KOH, pH 6.5, supplemented with 400 mM KCl, 10 mM MgCl2 and 10 mM DTT. Notably, the inclusion of KCl, MgCl2 and DTT as reducing agent was essential throughout all purification steps. The rate dependence of the MfcDPGS followed classical Michaelis–Menten kinetics, with a Vmax value of 38.2 ± 1.7 U mg−1 and KM values of 1.52 ± 0.4 mM and 0.55 ± 0.08 mM for 2,3DPG and ATP, respectively (Figure 4). For long-term storage at −70°C, the addition of 25% (v/v) glycerol to the same buffer was necessary to preserve enzyme activity. After one week of storage at −70°C, the enzyme retained 100% of its activity. After 1.5 months, nearly full activity (95%) was maintained, and after 3.5 month 83% residual activity was observed (Figure 5).
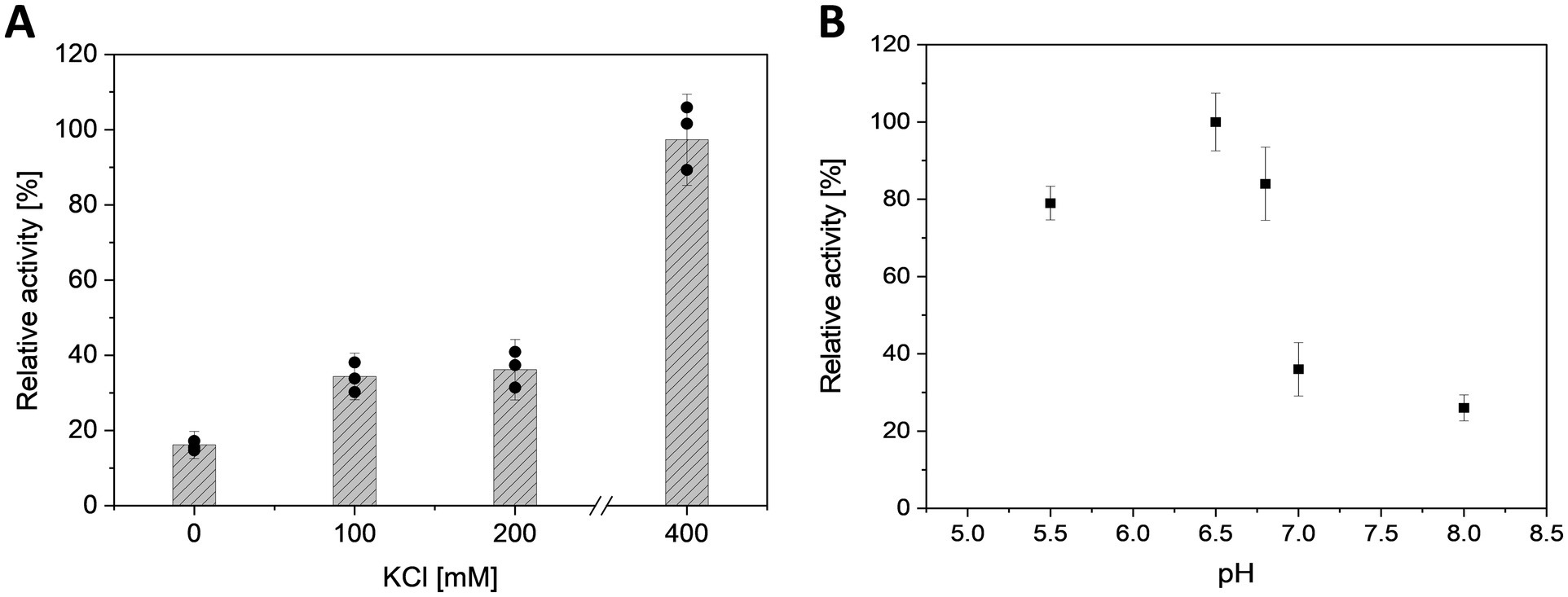
Figure 3. Effect of salt concentration and pH on the activity of MfcDPGS. (A) Effect of KCl concentration (0, 100, 200, 400 mM) and (B) effect of pH (pH 5.5 to 8.0) on the activity of partially purified MfcDPGS (16.8 μg) after heat precipitation (75°C, 30 min). The enzyme activity was determined using the continuous PK-LDH assay at 55°C, which couples the formation of ADP from ATP to the oxidation of NADH monitored by the absorbance decrease at 340 nm. The pH optimum was determined using buffer systems as described in the Material and Method section, with each buffer supplemented with 400 mM KCl and 10 mM MgCl2. The relative activity is expressed as a percentage, where 100% corresponds to a specific activity of 1.8 U mg−1. All assays were performed in technical triplicates, and the error bars indicate the standard deviation of the mean.
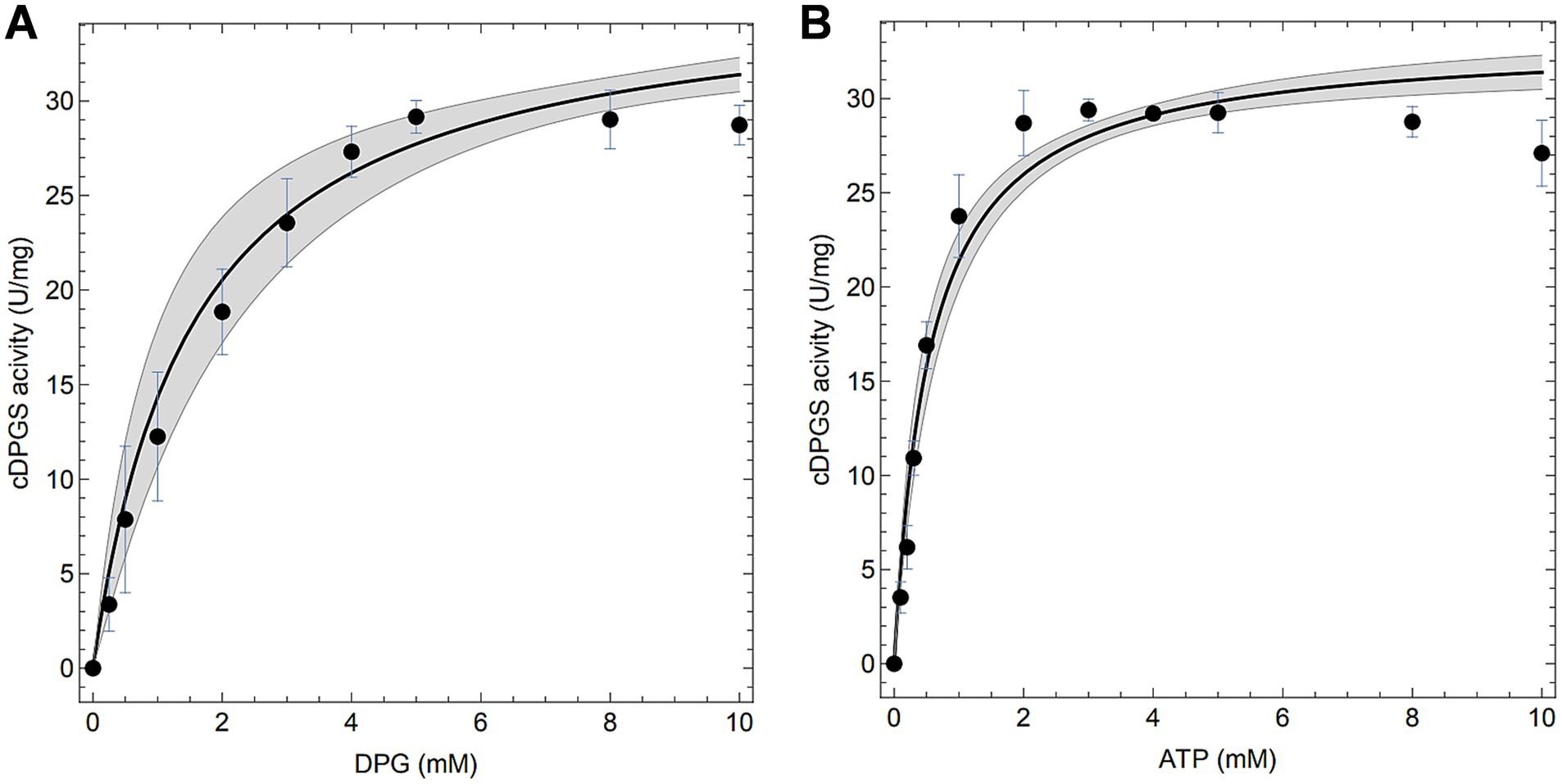
Figure 4. Enzymatic properties of the recombinant MfcDPGS. Enzymatic activity (A,B) was measured at 55°C (340 nm) with 4.8 μg of purified enzyme using the continuous PK-LDH assay. (A) The specific activity of purified MfcDPGS was determined with varying concentration of 2,3DPG (0–10 mM) and a constant 10 mM ATP. (B) The specific activity was determined with varying concentrations of ATP (0–10 mM) and a constant 10 mM 2,3DPG. All assays were performed in technical triplicates, and the error bars represent the standard deviation of the mean. Computational fits to the complete dataset are shown as solid black lines and the mean prediction confidence intervals are represented as shaded areas.
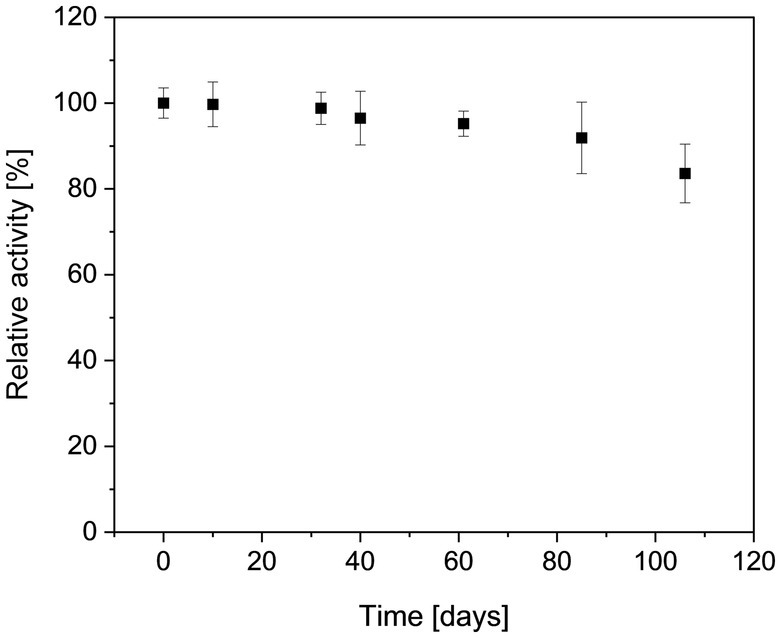
Figure 5. Long term stability of the recombinant MfcDPGS. The enzyme was stored in aliquots at −70°C in a buffer containing MES/KOH (pH 6.5), 25% (v/v) glycerol, 400 mM KCl, 10 mM MgCl2 and 10 mM DTT. Aliquots were thawed gently on ice and mixed thoroughly by pipetting before activity measurement. The residual activity of the thawed enzyme samples was determined at the indicated time points using the continuous PK-LDH assay. 100% of relative activity corresponds to a specific activity of 18.7 U mg−1. All measurements were performed in technical triplicates, the error bars represent the standard deviation of the mean. Results are based on two biological replicates, each from a separate expression and purification run.
3.3 cDPG production
To determine the in vitro production of cDPG from 2,3DPG, we conducted discontinuous enzyme assays over a range of 2,3DPG concentrations (1:2 molar ratio of 2,3DPG: ATP) and analyzed the conversion efficiencies both enzymatically (ADP determination), and via 31P-NMR spectroscopy (2,3DPG consumption and cDPG production). In initial experiments optimal Mg2+/ATP ratios and product inhibition was investigated. To determine the optimal Mg2+/ATP ratio, a range of 0.02–1.0 (1–50 mM) was investigated, with the optimal ratio found to be between 0.2–0.5 (Supplementary Figure S9). Additionally, potential product inhibition by cDPG (0.5–20 mM) was assessed at a 1:2 molar ratio (5 mM 2,3DPG/10 mM ATP) and a 0.5 Mg2+/ATP ratio (5 mM Mg2+, 10 mM ATP) at 55°C. However, no significant inhibitory effects were observed (Supplementary Figure S10). Based on these findings, optimized in vitro conversions were performed using the following conditions: 50 mM MES/KOH (pH 6.5), 400 mM KCl, 10 mM DTT, a 1:2 molar ratio of 2,3 DPG/ATP, a Mg2+/ATP ratio of 0.2–0.5, and 0.5 U of the purified recombinant MfcDPGS. To monitor the conversion of 2,3DPG to cDPG, 31P-NMR analyses were conducted under these conditions. A 5 mM cDPG standard was used for validation to facilitate accurate signal interpretation under these conditions (Supplementary Figure S11).
Finally, the conversion of 1 mM, 4 mM, 10 mM, 25 mM, 50 mM and 100 mM 2,3DPG to cDPG was monitored (1 mL reactions, 0.5 U MfcDPGS) over time (0–180 min, for 100 mM 2,3 DPG, also after 20 h) using both analytical approaches. Remarkably, complete conversion of 2,3DPG to cDPG was observed for all concentrations tested (Figure 6, and Supplementary Figures S12A–F). 31P-NMR experiments showed full conversion of 1 mM, 4 mM, and 10 mM 2,3DPG after 25–40 min (Figure 6 A, and Supplementary Figures S13 A–C). For higher concentrations of 25 mM, 50 mM, and 100 mM 2,3 DPG, complete conversion was achieved after 180 min (Figure 6B and Supplementary Figures S13E,F). The enzymatic assay for ADP formation generally follows the same trend, although some deviations were observed due to experimental factors such as sample dilution.
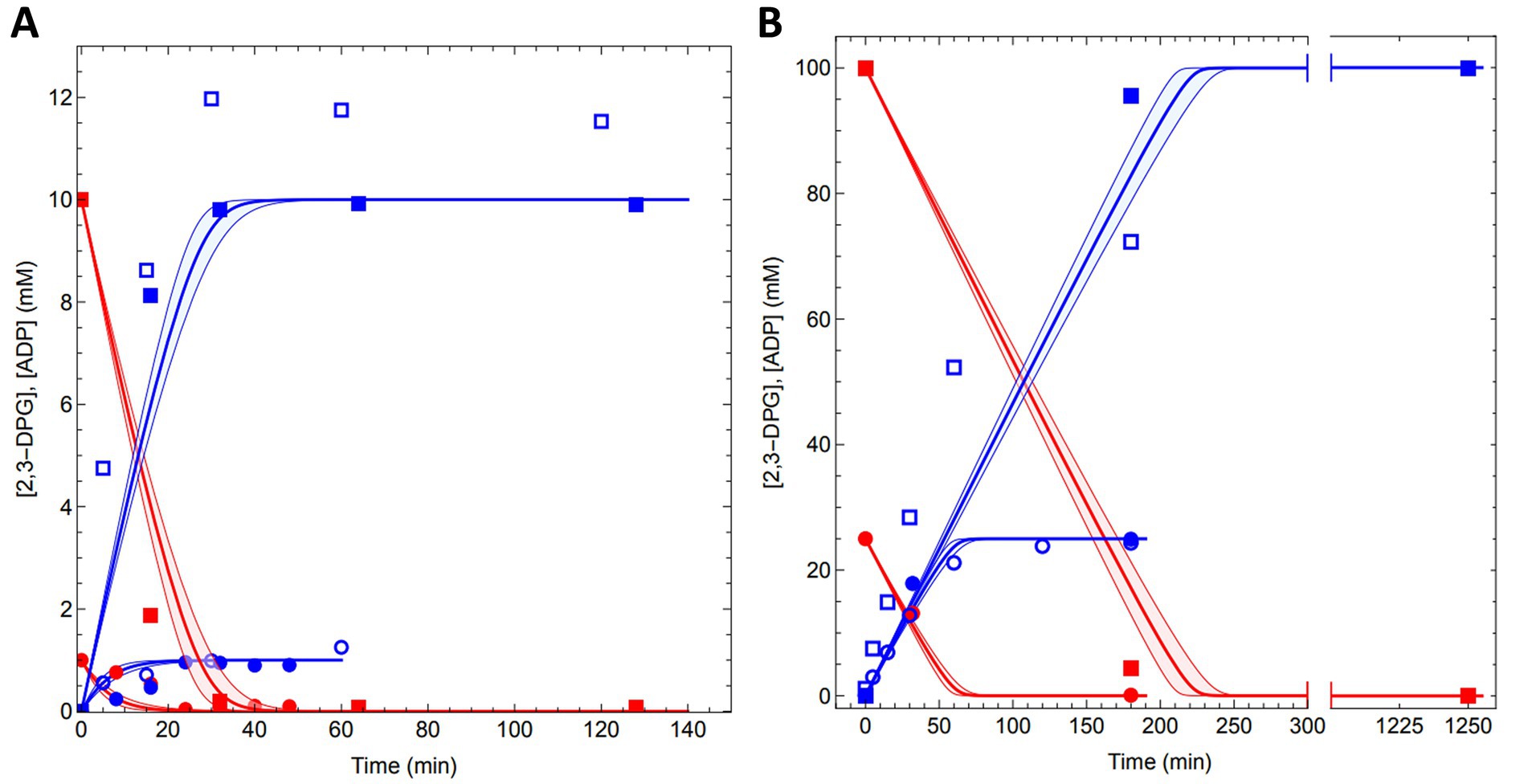
Figure 6. Conversion of 2,3DPG to cDPG by recombinant MfcDPGS. The time-dependent conversion results for (A) 1 mM (circles) and 10 mM (squares) conversion of 2,3DPG and (B) 25 mM (circles) and 100 mM (squares) 2,3 DPG to cDPG are shown. Assays were performed at 55°C in 50 mM MES/KOH (pH 6.5), 400 mM KCl and a Mg2+/ATP ratio of 0.2 using 0.5 U of purified MfcDPGS. Samples were taken at regular time intervals, and resulting ADP from ATP was quantified using the PK-LDH assay and are shown with blue open symbols. Each data point represents the mean value of three independent technical replicates. The redfilled symbols represent the 2,3DPG concentrations, while the blue filled symbols represent the cDPG concentrations, both quantified via 31P-NMR spectroscopy. Model (Equations 1, 2) predictions for the experiments are shown with a red line for 2,3DPG consumption and a blue line for cDPG production.
Furthermore, we simulated our experimental data with a computational model based on the kinetic parameters of MfcDPGS. For the simulations we integrated the enzyme kinetic rate equation (Equation 1) as ordinary differential equations (Equation 2), using the experimentally determined kinetic parameters.
The model predicted the conversion rates for all experiments, aligning closely with our measured data (solid blue and red lines, Figures 6A,B and Supplementary Figures S12A–F). The model also provided predictions for the time required to achieve complete conversion at various 2,3DPG concentrations: 1 mM (~20 min), 4 mM (~20 min), 10 mM (~30 min), 25 mM (~70 min), 50 mM (~120 min), and 100 mM (~200 min). Importantly, the model can be extended to predict conversion rates and outcomes for significantly larger production scales. The kinetic data and model simulations are available as Excel files and Mathematica notebooks on the FAIRDOMHub (https://fairdomhub.org/investigations/719).
In conclusion, using the optimized protocol, the MfcDPGS (0.5 U/mL) catalyzed the complete conversion of up to 100 mM 2,3DPG to cDPG in a total volume of 1 mL within approximately 180 min at 55°C, resulting in 37.6 mg of synthesized cDPG.
Finally, to further streamline the enzymatic production of cDPG, we investigated whether the MfcDPGS obtained after heat precipitation could be used directly for the conversion, thereby omitting the additional time-and cost-intensive purification step of SEC. The conversion of 10 mM 2,3DPG with 0.65 U/mL of heat-precipitated MfcDPGS was monitored using 31P-NMR Complete substrate-to-product conversion was achieved within 16 min (Supplementary Figure S14). This simplified approach significantly reduces both protein production costs and process complexity, making it highly suitable for future applications.
4 Discussion
cDPG is a valuable extremolyte for diverse industrial and therapeutic applications. Despite its promising properties and potential applications, an efficient metabolic engineering strategy for cDPG production has not yet been established. A promising alternative to metabolic engineering is cell-free in vitro bioproduction using purified enzymes and cofactors in combination with enzyme kinetic modeling (Shen et al., 2020). This approach can circumvent challenges such as cellular regulation, competing metabolic pathways, kinetic bottlenecks, and toxicity issues (Fessner, 2015; Claassens et al., 2019). However, efficient in vitro production depends on optimized enzyme expression and purification protocols, as well as strategies to ensure enzyme stability for prolonged use and storage.
To address these challenges, we optimized the recombinant production of the M. fervidus cDPGS and established a streamlined, one-step cell-free in-vitro production system for converting 2,3DPG to cDPG. Although the expression of the MfcDPGS has been previously reported, earlier attempts at heterologous production yielded low expression levels and protein yields, with only 0.09 mg g−1 (wet weight) cells (Matussek et al., 1998). This limitation was also evident in our initial experiments.
Heterologous expression of methanogenic genes in non-methanogens has historically been challenging, often due to differences in gene structure and codon usage (Lange and Ahring, 2001). Methanogens frequently use rare codons, such as AUA, AGA, and AGG, which are uncommon in E. coli, leading to poor expression and low protein yields (Reeve, 1993; Rosano and Ceccarelli, 2009). Additionally, the prevalence of adenines (A) and thymines (T) at the third codon position in methanogenic genes further complicates expression in other host systems (Lange and Ahring, 2001). Improved expression of archaeal methanogenic proteins in E. coli has been achieved in several instances through co-expression of rare tRNAs, such as argU, ileY, ileX, and leuW (Kim et al., 1998; Rosano and Ceccarelli, 2009). To overcome these challenges, we opted to codon-optimize the MfcDPGS-encoding gene for expression in E. coli and lowered the expression temperature, a widely used strategy for improving protein solubility and yield (Vera et al., 2007).
In addition to the expression challenges, the recombinant MfcDPGS exhibited significant instability, consistent with previous studies on MfcDPGS (Matussek et al., 1998; van Alebeek et al., 1994) and other methanogen-derived proteins (Breitung et al., 1992; Hensel and Jakob, 1993; Shima et al., 1998; Liu et al., 2012; Reed et al., 2013). Furthermore, MfcDPGS requires high concentrations of potassium ions (up 1 M) and magnesium ions (10 mM) for full activity (Lehmacher et al., 1990; Lehmacher and Hensel, 1994; van Alebeek et al., 1994). Potassium ions and reducing agents like DTT also enhance enzyme stability and minimize oxygen susceptibility, as observed for other methanogens such as M. kandleri and M. thermoautotrophicus (Breitung et al., 1992; Liu et al., 2012; Reed et al., 2013).
The primary challenge in this study was to optimize conditions for the expression, purification and stabilization of MfcDPGS. We demonstrated that the enzyme’s activity was significantly enhanced by the addition of 400 mM KCl, 10 mM DTT, and 10 mM Mg2+. Furthermore, supplementing the storage buffer with 25% (v/v) glycerol enabled long-term storage at −70°C for several months, with minimal loss of activity.
Interestingly, the N-terminal His-tag introduced for purification appeared to exacerbate recombinant MfcDPGS instability. This aligns with reports showing that affinity tags can sometimes interfere with protein function, folding, activity, and stability (Freydank et al., 2008; Young et al., 2012; Booth et al., 2018). A different construct was used for optimal expression of the MfcDPGS to obtain the optimal solubility and oligomeric homogeneity required for crystallization studies (De Rose et al., 2023).
The optimized procedure for MfcDPGS production involved expressing the recombinant enzyme without any tags and performing all purification steps in the presence of 400 mM KCl, 10 mM MgCl2 and 10 mM DTT. The enzyme was efficiently purified using an optimized heat precipitation step. Although the thermal stability of the MfcDPGS has not been determined in previous studies presumably due to the known general instability of the enzyme (see above) (Lehmacher et al., 1990; Matussek et al., 1998), the satisfactory yields upon heat treatment are well in line with the thermophilic origin of the enzyme (optimal growth temperature of M. fervidus is 83°C) and its reported temperature optimum of 75–80°C (Lehmacher et al., 1990). For further refinement, SEC could be added if required. This streamlined approach yielded a more than twenty-fold increase in protein recovery after SEC (2 mg g−1 cell wet weight) compared to previous reports, which achieved only 0.09 mg g−1 cells wet weight after affinity chromatography on reactive green 19 agarose (Matussek et al., 1998). A significant advantage of this method was maintaining a consistently high KCl concentration throughout the purification process. In contrast, the earlier method required low salt conditions during the affinity chromatography step, likely leading to substantial protein losses.
The recombinant MfcDPGS produced by this new method exhibited markedly improved catalytic properties, with a significantly higher Vmax of 38.2 U mg−1 at 55°C - more than double the previously reported value of 17 U mg−1 at 83°C (not considering the difference in assay temperature) - and closely approaching the activity described for the native enzyme (32 U mg−1, 83°C) (Matussek et al., 1998). Additionally, the KM values for 2,3DPG (1.52 mM) and ATP (0.55 mM) were significantly lower than those reported by Matussek and coworkers (KM 2,3 DPG = 5.9 mM, KM ATP = 3.0 mM) (Supplementary Table S3), likely reflecting differences in purification protocols and assay conditions between both studies.
The most significant outcome of this study was the demonstration that the recombinant MfcDPGS catalyzed the complete conversion of 2,3 DPG to cDPG. In a 1 mL reaction volume, 100 μmol of substrate was fully converted within 180 min using 0.5 U of the purified enzyme, as confirmed by experimental data and computational modeling. The enzyme’s preference for the synthetic direction of the reaction is supported by thermodynamic considerations. The formation of the phosphoanhydride bond in 2,3DPG, driven by ATP hydrolysis, was shown to be thermodynamically favorable. While ATP hydrolysis to ADP and Pi has a ΔG0’ of −26 kJ mol−1, the hydrolysis of the anhydride bond in trianionic pyrophosphate and cDPG (Lentzen and Schwarz, 2006b) has a calculated ΔG0’ of approximately −16 kJ mol−1 under standard conditions, pH 7.0, ionic strength 0.1 M (eQuilibrator) (Flamholz et al., 2012). This suggests that the overall reaction is exergonic by approximately −10 kJ mol−1, providing a thermodynamic basis for the observed complete conversion. Given that the intracellular cDPG product concentration in the previously reported in vivo approach using T. thermophilus was quantified to be 650 μM (De Rose et al., 2021) and that the intracellular space corresponds to roughly (less than) 1% of the total culture volume (Volkmer and Heinemann, 2011), the pure volumetric productivity of the in vitro approach can be roughly estimated to be in the range of 10,000-fold higher (concentration range of 10 μM per liter culture in vivo compared to 100 mM per liter in the conversion assay in vitro).
The recently solved crystal structure of MfcDPGS provides valuable insights into its substrate specificity and catalytic mechanism, further supporting the exergonic nature of the cyclisation reaction (De Rose et al., 2023). The structure reveals that MfcDPGS remains in an “open” conformation until ATP binds correctly, triggering domain closure and creating a hydrophobic environment essential for the cyclisation reaction. Upon binding of ATP and 2,3 DPG, conformational changes position key residues in proximity, facilitating the catalytic process. The domain closure aligns the ATP γ-phosphate for a nucleophilic substitution (SN2) reaction, transferring the phosphate group to the 2-phophate of 2,3DPG. A second SN2 reaction then occurs, where the 3-phosphate of the intermediate attacks the 2-pyrophosphate, leading to the release of inorganic phosphate. This release of phosphate renders the reaction highly exergonic under standard conditions, providing the energy required for the formation of the phosphoanhydride bond in cDPG (De Rose et al., 2023). These mechanistic insights underscore the enzyme’s efficiency and the thermodynamic feasibility of the reaction.
5 Conclusion
In conclusion, we have successfully developed an efficient protocol for the production and purification of MfcDPGS to be used for cDPG production including strategies to stabilize the enzyme. Furthermore, we demonstrated the enzyme’s capability to achieve complete substrate conversion, enabling a streamlined and straightforward one-step biocatalytic synthesis of cDPG. This simplified approach enhances production efficiency and reduces downstream processing complexity. The established one-step in vitro synthesis is scalable and adaptable, with the potential to be extended into an enzyme cascade for the synthesis of cDPG from more cost-effective substrates, such as glycerate. These advancements pave the way for the cost-effective production of cDPG as a high-value product for diverse biotechnological applications.
Data availability statement
The datasets presented in this study can be found in online repositories. The names of the repository/repositories and accession number(s) can be found in the article/supplementary material.
Author contributions
CS: Writing – review & editing, Writing – original draft, Methodology, Investigation, Formal analysis, Validation. BM: Writing – review & editing. SD: Writing – review & editing. EF: Writing – review & editing. IK: Writing – review & editing. MI: Writing – review & editing. NH: Writing – review & editing. DM: Writing – review & editing. JL: Writing – review & editing. FM: Writing – review & editing, Supervision. JS: Writing – review & editing. CB: Writing – review & editing, Writing – original draft, Software, Methodology. BS: Writing – review & editing, Writing – original draft, Conceptualization, Methodology, Project administration, Funding acquisition, Supervision, Data curation.
Funding
The author(s) declare that financial support was received for the research and/or publication of this article. CS acknowledges funding by an Evonik Industries AG Scholarship. CS, BM, CB and BS acknowledge funding by the German Federal Ministry of Education and Research (BMBF) grant HotSolute, 031B0612A within the ERA CoBioTech funding initiative. HotSolute has received funding from the European Union’s Horizon 2020 research and innovations programme under grant agreement No [722361]. JL, SAR, MNI, NJH acknowledge BBSRC BB/R02166X/1 grant for funding. JLS acknowledges funding from the DSI/NRF in South Africa (grant NRF-SARCHI-82813). DM acknowledges funding from the Italian Ministry of Education and Research (MIUR), Fondo per le agevolazioni alla ricerca “First 2016” (Decreto n. 110/2019). We thank Michaela Boraja for technical assistance. In addition, we would like to extend our gratitude to Bitop AG (Dortmund, Germany) for generously providing cDPG. We acknowledge support by the Open Access Publication Fund of the University of Duisburg-Essen.
Conflict of interest
The authors declare that the research was conducted in the absence of any commercial or financial relationships that could be construed as a potential conflict of interest.
The author(s) declared that they were an editorial board member of Frontiers, at the time of submission. This had no impact on the peer review process and the final decision.
Generative AI statement
The author(s) declare that no Gen AI was used in the creation of this manuscript.
Publisher’s note
All claims expressed in this article are solely those of the authors and do not necessarily represent those of their affiliated organizations, or those of the publisher, the editors and the reviewers. Any product that may be evaluated in this article, or claim that may be made by its manufacturer, is not guaranteed or endorsed by the publisher.
Supplementary material
The Supplementary material for this article can be found online at: https://www.frontiersin.org/articles/10.3389/fmicb.2025.1601972/full#supplementary-material
Abbreviations
(cDPGS), cyclic-2,3-diphosphoglycerate synthetase; (2,3DPG), 2,3-diphosphoglycerate; (cDPG), cyclic 2,3-diphosphoglycerate; (2PG), 2-phosphoglycerate; (2PGK), 2-phosphoglycerate kinase.
References
Becker, J., and Wittmann, C. (2020). Microbial production of extremolytes — high-value active ingredients for nutrition, health care, and well-being. Curr. Opin. Biotechnol. 65, 118–128. doi: 10.1016/j.copbio.2020.02.010
Booth, W., Schlachter, C., Pote, S., Ussin, N., Mank, N., Klapper, V., et al. (2018). Impact of an N-terminal Polyhistidine tag on protein thermal stability. ACS Omega 3, 760–768. doi: 10.1021/acsomega.7b01598
Borges, N., Gonçalves, L., Rodrigues, M., Siopa, F., Ventura, R., Maycock, C., et al. (2006). Biosynthetic pathways of inositol and glycerol phosphodiesters used by the hyperthermophile Archaeoglobus fulgidus in stress adaptation. J. Bacteriol. 188, 8128–8135. doi: 10.1128/JB.01129-06
Borges, N., Ramos, A., Raven, N. D., Sharp, R. J., and Santos, H. (2002). Comparative study of the thermostabilizing properties of mannosylglycerate and other compatible solutes on model enzymes. Extremophiles 6, 209–216. doi: 10.1007/s007920100236
Breitung, J., Börner, G., Scholz, S., Linder, D., Stetter, K. O., and Thauer, R. K. (1992). Salt dependence, kinetic properties and catalytic mechanism of N-formylmethanofuran:tetrahydromethanopterin formyltransferase from the extreme thermophile Methanopyrus kandleri. Eur. J. Biochem. 210, 971–981. doi: 10.1111/j.1432-1033.1992.tb17502.x
Ciulla, R., Clougherty, C., Belay, N., Krishnan, S., Zhou, C., Byrd, D., et al. (1994). Halotolerance of Methanobacterium thermoautotrophicum delta H and Marburg. J. Bacteriol. 176, 3177–3187. doi: 10.1128/jb.176.11.3177-3187.1994
Claassens, N. J., Burgener, S., Vögeli, B., Erb, T. J., and Bar-Even, A. (2019). A critical comparison of cellular and cell-free bioproduction systems. Curr. Opin. Biotechnol. 60, 221–229. doi: 10.1016/j.copbio.2019.05.003
da Costa, M., Santos, H., and Galinski, E. (1998). An overview of the role and diversity of compatible solutes in Bacteria and Archaea. Adv. Biochem. Eng. Biotechnol. 61, 117–153. doi: 10.1007/BFb0102291
De Rose, S., Finnigan, W., Harmer, N., Littlechild, J., Consortium, T., Bettina, S., et al. (2021). Production of the Extremolyte cyclic 2,3-Diphosphoglycerate using Thermus thermophilus as a whole-cell factory. Front. Catalysis 1:803416. doi: 10.3389/fctls.2021.803416
De Rose, S. A., Isupov, M. N., Worthy, H. L., Stracke, C., Harmer, N. J., Siebers, B., et al. (2023). Structural characterization of a novel cyclic 2,3-diphosphoglycerate synthetase involved in extremolyte production in the archaeon Methanothermus fervidus. Front. Microbiol. 14:1267570. doi: 10.3389/fmicb.2023.1267570
Empadinhas, N., and da Costa, M. S. (2006). Diversity and biosynthesis of compatible solutes in hyper/thermophiles. Int. Microbiol. 9, 199–206. doi: 10.2436/20.7010.01.65
Fessner, W. D. (2015). Systems biocatalysis: development and engineering of cell-free "artificial metabolisms" for preparative multi-enzymatic synthesis. New Biotechnol. 32, 658–664. doi: 10.1016/j.nbt.2014.11.007
Flamholz, A., Noor, E., Bar-Even, A., and Milo, R. (2012). eQuilibrator-the biochemical thermodynamics calculator. Nucleic Acids Res. 40 (Database issue): D770-775, D770–D775. doi: 10.1093/nar/gkr874
Freydank, A.-C., Brandt, W., and Dräger, B. (2008). Protein structure modeling indicates hexahistidine-tag interference with enzyme activity. Proteins 72, 173–183. doi: 10.1002/prot.21905
Hensel, R., and Jakob, I. (1993). Stability of Glyceraldehyde-3-phosphate dehydrogenases from Hyperthermophilic Archaea at high temperature. Syst. Appl. Microbiol. 16, 742–745. doi: 10.1016/S0723-2020(11)80348-6
Hensel, R., and König, H. (1988). Thermoadaptation of methanogenic bacteria by intracellular ion concentration. FEMS Microbiol. Lett. 49, 75–79. doi: 10.1111/j.1574-6968.1988.tb02685.x
Kim, R., Sandler, S. J., Goldman, S., Yokota, H., Clark, A. J., and Kim, S.-H. (1998). Overexpression of archaeal proteins in Escherichia coli. Biotechnol. Lett. 20, 207–210. doi: 10.1023/A:1005305330517
Kunte, H., Lentzen, G., and Galinski, E. (2014). "industrial production of the cell protectant Ectoine: protection mechanisms, processes, and products." current. Biotechnology 3, 10–25. doi: 10.2174/22115501113026660037
Lamosa, P., Martins, L., da Costa, M., and Santos, H. (1998). ‘Effects of temperature, salinity, and medium composition on compatible solute accumulation by thermococcus spp,’ in Applied and Environmental Microbiology 64. doi: 10.1128/AEM.64.10.3591-3598.1998
Lange, M., and Ahring, B. K. (2001). A comprehensive study into the molecular methodology and molecular biology of methanogenic Archaea. FEMS Microbiol. Rev. 25, 553–571. doi: 10.1111/j.1574-6976.2001.tb00591.x
Lehmacher, A., and Hensel, R. (1994). Cloning, sequencing and expression of the gene encoding 2-phosphoglycerate kinase from Methanothermus fervidus. Mol. Gen. Genet. 242, 163–168. doi: 10.1007/BF00391009
Lehmacher, A., Vogt, A. B., and Hensel, R. (1990). Biosynthesis of cyclic 2,3-diphosphoglycerate. Isolation and characterization of 2-phosphoglycerate kinase and cyclic 2,3-diphosphoglycerate synthetase from Methanothermus fervidus. FEBS Lett. 272, 94–98. doi: 10.1016/0014-5793(90)80456-S
Lentzen, G., and Schwarz, T. (2006a). Kompatible Solute: Mikrobielle Herstellung und Anwendung. Angwandte Mikrobiologie. Berlin, Heidelberg: Springer, 355–370.
Lentzen, G., and Schwarz, T. (2006b). Extremolytes: natural compounds from extremophiles for versatile applications. Appl. Microbiol. Biotechnol. 72, 623–634. doi: 10.1007/s00253-006-0553-9
Lippert, K., and Galinski, E. A. (1992). Enzyme stabilization be ectoine-type compatible solutes: protection against heating, freezing and drying. Appl. Microbiol. Biotechnol. 37, 61–65. doi: 10.1007/BF00174204
Liu, Y. F., Zhang, N., Liu, X., Wang, X., Wang, Z. X., Chen, Y., et al. (2012). Molecular mechanism underlying the interaction of typical Sac10b family proteins with DNA. PLoS One 7:e34986. doi: 10.1371/journal.pone.0034986
Martins, L. O., and Santos, H. (1995). Accumulation of Mannosylglycerate and Di-myo-inositol-phosphate by Pyrococcus furiosus in response to salinity and temperature. Appl. Environ. Microbiol. 61, 3299–3303. doi: 10.1128/aem.61.9.3299-3303.1995
Matussek, K., Moritz, P., Brunner, N., Eckerskorn, C., and Hensel, R. (1998). Cloning, sequencing, and expression of the gene encoding cyclic 2, 3-diphosphoglycerate synthetase, the key enzyme of cyclic 2, 3-diphosphoglycerate metabolism in Methanothermus fervidus. J. Bacteriol. 180, 5997–6004. doi: 10.1128/JB.180.22.5997-6004.1998
McNicholas, S., Potterton, E., Wilson, K. S., and Noble, M. E. (2011). Presenting your structures: the CCP4mg molecular-graphics software. Acta Crystallogr. D Biol. Crystallogr. 67, 386–394. doi: 10.1107/S0907444911007281
Reed, C. J., Lewis, H., Trejo, E., Winston, V., and Evilia, C. (2013). Protein adaptations in archaeal extremophiles. Archaea 2013:373275. doi: 10.1155/2013/373275
Reeve, J. N. (1993). Structure and Organization of Genes. Methanogenesis: Ecology, Physiology, Biochemistry & Genetics J. G. Ferry. Boston, MA: Springer US, 493–526.
Rosano, G., and Ceccarelli, E. (2009). Rare codon content affects the solubility of recombinant proteins in a codon bias-adjusted Escherichia coli strain. Microb. Cell Factories 8:41. doi: 10.1186/1475-2859-8-41
Scholz, S., Sonnenbichler, J., Schäfer, W., and Hensel, R. (1992). Di-myo-inositol-1,1′-phosphate: a new inositol phosphate isolated from Pyrococcus woesei. FEBS Lett. 306, 239–242. doi: 10.1016/0014-5793(92)81008-A
Seely, R. J., and Fahrney, D. E. (1983). A novel diphospho-P,P'-diester from Methanobacterium thermoautotrophicum. J. Biol. Chem. 258, 10835–10838. doi: 10.1016/S0021-9258(17)44350-X
Shen, L., Kohlhaas, M., Enoki, J., Meier, R., Schönenberger, B., Wohlgemuth, R., et al. (2020). A combined experimental and modelling approach for the Weimberg pathway optimisation. Nat. Commun. 11:1098. doi: 10.1038/s41467-020-14830-y
Shima, S., Hérault, D. A., Berkessel, A., and Thauer, R. K. (1998). Activation and thermostabilization effects of cyclic 2,3-diphosphoglycerate on enzymes from the hyperthermophilic Methanopyrus kandleri. Arch. Microbiol. 170, 469–472. doi: 10.1007/s002030050669
van Alebeek, G.-J. W. M., Tafazzul, G., Kreuwels, M. J. J., Keltjens, J. T., and Vogels, G. D. (1994). Cyclic 2,3-diphosphoglycerate metabolism in Methanobacterium thermoautotrophicum (strain ΔH): characterization of the synthetase reaction. Arch. Microbiol. 162, 193–198. doi: 10.1007/BF00314474
Vera, A., González-Montalbán, N., Arís, A., and Villaverde, A. (2007). The conformational quality of insoluble recombinant proteins is enhanced at low growth temperatures. Biotechnol. Bioeng. 96, 1101–1106. doi: 10.1002/bit.21218
Volkmer, B., and Heinemann, M. (2011). Condition-dependent cell volume and concentration of Escherichia coli to facilitate data conversion for systems biology modeling. PLoS One 6:e23126. doi: 10.1371/journal.pone.0023126
Young, C. L., Britton, Z. T., and Robinson, A. S. (2012). Recombinant protein expression and purification: a comprehensive review of affinity tags and microbial applications. Biotechnol. J. 7, 620–634. doi: 10.1002/biot.201100155
Keywords: hyperthermophiles, archaea, stress response, compatible solutes, extremolytes, thermoprotection, 2-phosphoglycerate kinase, cyclic-2,3-diphosphoglycerate synthetase
Citation: Stracke C, Meyer BH, De Rose SA, Ferrandi EE, Kublanov IV, Isupov MN, Harmer NJ, Monti D, Littlechild J, Müller F, Snoep JL, Bräsen C and Siebers B (2025) Establishment of an efficient one-step enzymatic synthesis of cyclic-2,3-diphosphoglycerate. Front. Microbiol. 16:1601972. doi: 10.3389/fmicb.2025.1601972
Edited by:
Rosa María Martínez-Espinosa, University of Alicante, SpainReviewed by:
Quan Luo, Chinese Academy of Sciences (CAS), ChinaJulia Esclapez, University of Alicante, Spain
Copyright © 2025 Stracke, Meyer, De Rose, Ferrandi, Kublanov, Isupov, Harmer, Monti, Littlechild, Müller, Snoep, Bräsen and Siebers. This is an open-access article distributed under the terms of the Creative Commons Attribution License (CC BY). The use, distribution or reproduction in other forums is permitted, provided the original author(s) and the copyright owner(s) are credited and that the original publication in this journal is cited, in accordance with accepted academic practice. No use, distribution or reproduction is permitted which does not comply with these terms.
*Correspondence: Christina Stracke, Y2hyaXN0aW5hLnN0cmFja2VAdW5pLWR1ZS5kZQ==; Christopher Bräsen, Y2hyaXN0b3BoZXIuYnJhZXNlbkB1bmktZHVlLmRl; Bettina Siebers, YmV0dGluYS5zaWViZXJzQHVuaS1kdWUuZGU=
†Present address: Ilya V. Kublanov, Institute of Environmental Sciences, Hebrew University of Jerusalem, Rehovot, Israel