- 1Institute of Electrical Engineering, Yanshan University, Qinhuangdao, China
- 2Department of Magnetic Resonance Imaging, Qinhuangdao Municipal No. 1 Hospital, Qinhuangdao, China
Transcranial direct-current stimulation (DCS) offers a method for noninvasive neuromodulation usable in basic and clinical human neuroscience. Laser-speckle contrast imaging (LSCI), a powerful, low-cost method for obtaining images of dynamic systems, can detect regional blood-flow distributions with high spatial and temporal resolutions. Here, we used LSCI for measuring DCS-induced cerebral blood flow in real-time. Results showed that the change-rate of cerebral blood flow could reach approximately 10.1 ± 5.1% by DCS, indicating that DCS can increase cerebral blood flow and alter cortical hemodynamic responses. Thus, DCS shows potential for the clinical treatment and rehabilitation of ischemic strokes.
Introduction
Transcranial direct-current stimulation (DCS), which utilizes weak currents to regulate the activity of neurons in the cerebral cortex, is a noninvasive method for performing transcranial stimulation. In DCS, electrodes are placed on the scalp, and a weak direct current is applied to the cerebral cortex, changing its excitability (Ferrucci and Priori, 2014). The effects of DCS have the characteristics of polarization. Anodal stimulation can enhance cortical excitability, and cathodal stimulation can reduce cortical excitability. Stimulation effects are determined by measuring the duration and current intensity of the stimuli. If the stimulus duration is long enough, changes in cortical excitability can last up to 1 h. The effect can be controlled by adjusting the current intensity and duration of the stimuli (Antal et al., 2003; Siebner et al., 2004; Jeffery et al., 2007; Ragert et al., 2008; Hunter et al., 2009; Tanaka et al., 2009). The duration of continuous stimulation may play an important role in prolonging the duration of DCS (Kwon et al., 2008). Clinical applications of DCS have been proven useful in treating many conditions, such as fibromyalgia (Marlow et al., 2013), spinal-cord injuries (Soler et al., 2010), schizophrenia (Agarwal et al., 2013), depression (Nitsche et al., 2009), Parkinson's disease (Benninger et al., 2010), epilepsy (San-juan et al., 2015), and strokes (Baker et al., 2010). Previous studies also demonstrated that DCS could alter blood flow in the brain (Paquette et al., 2011; Zheng et al., 2011). Researchers using either laser Doppler flowmetry (LDF) (Wachter et al., 2011), functional near-infrared spectroscopy (Giovannella et al., 2018) or functional magnetic resonance imaging (fMRI) (Baudewig et al., 2001) for measuring cerebral blood flow observed that blood flow changed across a given region. Thus, the techniques could not indicate the structure of the blood vessels or determine the changes of blood-flow velocity in the blood vessels. This problem can be solved by using laser-speckle contrast imaging (LSCI) with DCS to study hemodynamics.
Laser-speckle contrast imaging (LSCI) technology, proposed by Briers and Webster (1996) and Briers et al. (1999), can obtain regional blood-flow distributions without scanning. In addition, this method uses a reflection model to measure blood flow and does not require the blood vessels to be transparent. In addition to its capability to image rapid blood flow (Zakharov et al., 2009; Dunn, 2012), LSCI also offers many advantages over other traditional methods, such as high spatial and temporal resolutions, imaging without contrast agents and real-time imaging. LSCI has seen wide use in nerve blood-flow imaging and is especially suited to the study of neural activity and hemodynamics (Ponticorvo and Dunn, 2010; Li et al., 2011, 2014; Zhang et al., 2012; Chao and Li, 2016).
In our study, we built up an LSCI system for real-time monitoring of rat cerebral hemodynamics induced by DCS. The laser-speckle contrast images obtained before and after DCS were recorded, and the distribution of blood flow was reconstructed by employing temporal laser-speckle contrast imaging methods. The rate of change of cerebral blood flow (ΔCBF) was determined to perform a quantitative evaluation of changes in cerebral blood flow.
Materials and Methods
Experimental Setup for LSCI and DCS
The schematic of the experimental setup is shown in Figure 1A. A diode laser (MW-SGX-635; 635 nm, 20 mW; Leishi Co., Ltd., Changchun City, China) coupled to a 600-μm-diameter silica optical fiber was used as the light source. The light from the optical fiber illuminated the brain tissue. The light scattered by the brain tissues and blood vessels passed through a trinocular stereo microscope (XTL-165 Series, Phenix Optical Holding Stock Co., Ltd., Shangrao City, China) and was recorded by a charge-coupled device (CCD) camera (pco.pixelfly usb, 14 bit, PCO AG, Kelheim, Germany) that was mounted to the trinocular stereo microscope. The laser-speckle images were acquired at 21 fps with an exposure time of 20 ms. Figure 1D presents a sequential diagram of the experimental procedure. In the experiment, the CCD camera recorded images for 160 s, including periods of 20 s before initiating an application of DCS to establish a baseline, 10 s of DCS, and 130 s after the DCS.
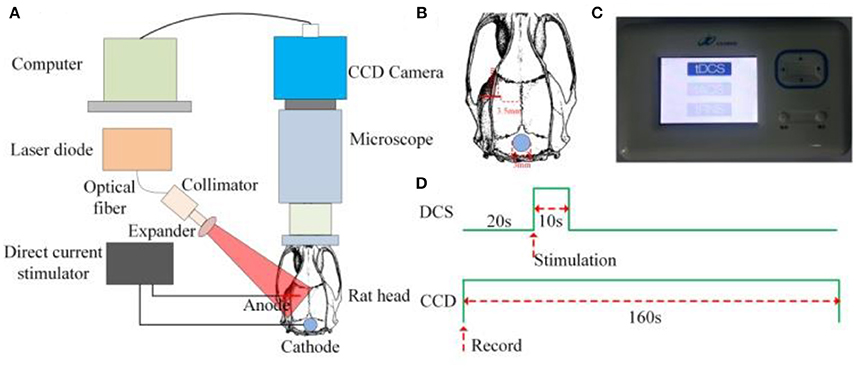
Figure 1. Experimental setup for LSCI and DCS (A) Schematic of the experimental setup. (B) AP and ML coordinates of position, 3.5 and 2.5 mm, respectively, of the anode-stimulating electrode. (C) Photograph of the direct-current stimulator. (D) Sequential diagram of the experiment.
Animal Surgery and Anesthesia
A total of 11 Sprague-Dawley rats (3-month-old males, body masses of approximately 270 g) were selected for experiment. The study was approved by the ethics committee of Qinhuangdao First Hospital. Surgical anesthesia was induced with sodium pentobarbital (3%, 5 mg/100 g, i.p.). The anesthetized rats were fixed to a stereotaxic apparatus (ST-5ND-C, Stoelting Co., Wood Dale, U.S.A.) with ear bars and a clamping device and body temperature was maintained at 37°C using a heating pad (NF, Nuanfeng Co., Guangdong, China). The fur covering each rat's skull was shaved, and the skin was cleaned with a 0.9% sodium chloride physiological solution. Last, the iodine was used to disinfect for the skin. The scalp was cut along the midline of the skull, and the subcutaneous tissue and periosteum were removed. A section of the skull was removed to expose the brain tissue in an area of approximately 0.5 × 0.5 cm.
Temporal Laser-Speckle Contrast Imaging
After completion of the surgical procedures, a customized anode-stimulating electrode with a diameter of 150 μm was mounted on the surface of the cerebral cortex. The anteroposterior (AP) and mediolateral (ML) coordinates of the position of the anode-stimulating electrode were 3.5 and 2.5 mm, respectively, as shown in Figure 1B. A customized circular cathode-stimulating electrode with a diameter of 3 mm was fixed on the skull after lambda. A direct-current stimulator (Jielian Technology Co., Ltd., Yiwu City, China), shown in Figure 1C, supplied direct current of 15 μA.
Na Li et al. presented a method for calculating blood flow with temporal LSCI (Li et al., 2009). The velocity information in the blur can be extracted and mapped to contrast using statistical arguments. Thus, the laser-speckle contrast CM can be defined by
where 〈IM〉 and are the average and the mean-square values of the time-varying speckle intensity over M observations, respectively, and is the square of the standard deviation of the time-varying speckle intensity. The contrast CM can be calculated over time using a time stack of images. In this case, a pixel window is moved across a time stack of M images to obtain the statistics leading to a temporally contrasted image.
The velocity v of the scatting particles and the speckle contrast CM are related by the integration time, where
Here, T is the integration time and w is the radius of the illuminating beam.
In our trials, we obtained one contrast image from 63 original laser-speckle images. Because the laser-speckle images were acquired at 21 fps, the time resolution of the laser-speckle contrast imaging was 3 s. We determined the ΔCBF to evaluate quantitatively the change of cerebral blood flow, where
Here, (1/CM) Exp is the reciprocal of the laser-speckle contrast with FUS, and (1/CM)Bas is the reciprocal of the laser-speckle contrast before DCS.
Results
Figure 2 shows the laser-speckle contrast images of one rat before and after DCS. Compared with the results at −10 s, shown in Figure 2a, the blood-flow velocity significantly accelerated at 30 s, seen in Figure 2b. The blood-flow velocity gradually decreased during the period from 30 to 90 s, as shown in Figures 2c,d. From 90 to 120 s, as shown in Figures 2d,e, the blood-flow velocity gradually grew stable and approximated the results obtained before stimulation at −10 s. In order to make the difference obvious, we choose and zoom in an interested region marked by white dashed frame in Figure 2b. And then we obtain the enlarged image shown in Figures 2g–k at −10, 30, 60, 90, and 120 s, respectively. We also can clearly see that the blood-flow velocity significantly accelerated at 30 s, and gradually decreased during the period from 30 to 90 s. These results show that DCS can affect the blood-flow velocity. The change of blood-flow velocity traversed three stages: enhancement, weakening, and recovery of stability.
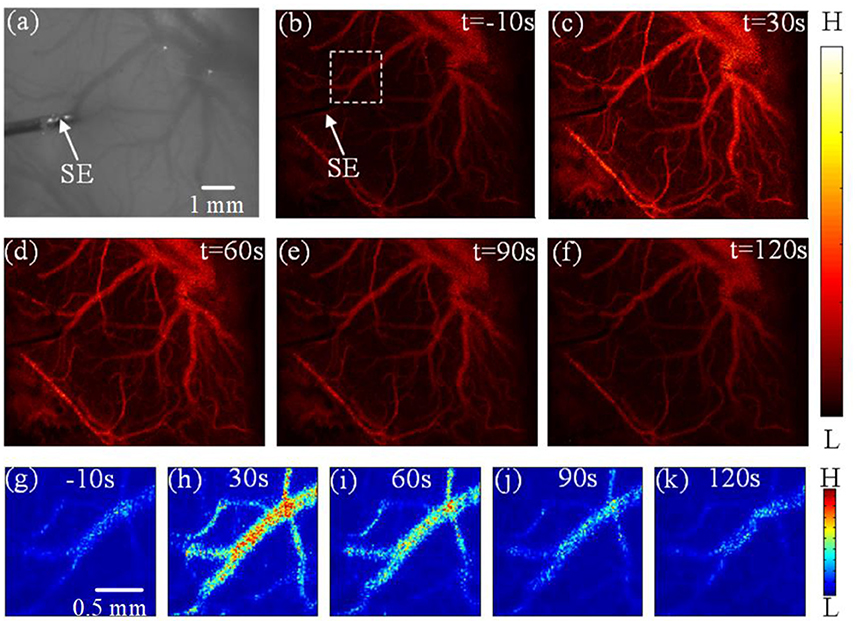
Figure 2. Experimental results depicting the effects of DCS (a) Photograph of the imaging region. (b–f) Laser-speckle contrast images of blood flow in the rat cerebral cortex at times of −10 s, 30 s, 60 s, 90 s, and 120 s, respectively. (g–k) the enlarged image of interested region marked by white dashed frame in (b) at −10, 30, 60, 90, and 120 s, respectively. Here, SE notes the stimulating electrode.
We calculated the ΔCBF for three regions of interest (ROI) to evaluate quantitatively the effects of DCS on cerebral blood flow. Figures 3a–d depict the results of ΔCBF at 30, 60, 90, and 120 s, respectively. We calculated the ΔCBF, obtaining the average values of all pixels in ROI1, ROI2, ROI3, and ROI4; the corresponding results appear in Figures 3e–h, respectively. Figure 3e presents the ΔCBF of ROI1 at 30, 60, 90, and 120 s as 11.7 ± 5.2%, 2.9 ± 2.5%, 1.3 ± 1.1%, and 0.8 ± 0.68%, respectively, where each result is of the form of mean ± s.t.d. Figure 3f shows the ΔCBF of ROI2 at 30, 60, 90, and 120 s as 14.1 ± 6.3%, 4.4 ± 3.8%, 1. 6± 1.5%, and 0.7 ± 0.59%, respectively. Then, Figure 3g depicts the ΔCBF of ROI3 at 30, 60, 90, and 120 s as 11.7 ± 5.6%, 2.3 ±2.1%, 1.0 ± 0.8%, and 0.7 ± 0.65%, respectively. Finally, Figure 3h presents the ΔCBF of ROI4 at 30, 60, 90, and 120 s as 10.6 ± 6.3%, 2.2 ± 1.9%, 0.9 ± 0.6%, and 0.6 ± 0.53%, respectively. Thus, these results show that DCS significantly increased the blood flow in the rat cerebral cortex.
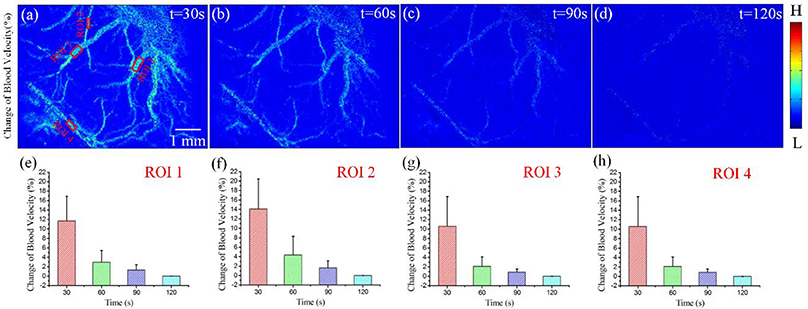
Figure 3. ΔCBF for varying periods of DCS. (a–d) ΔCBF with DCS at times 30, 60, 90, and 120 s, respectively. (e–h) ΔCBF at different times for ROI1, ROI2, ROI3, and ROI4, respectively.
Finally, we calculated the average values of all pixels in the ROIs of each rat, and then obtained the average values across 11 rats. The statistical analysis results appear in Figure 4. The laser-speckle contrast at −10 s served as the baseline. Then, we calculated the ΔCBF at 30, 60, 90, and 120 s and determined that the corresponding values were 10.1 ± 5.1%, 3.3 ± 2.1%, 1.5 ± 1.1%, and 1.1 ± 0.89%, respectively. Here, the values have the form of mean ± s.t.d. and were determined by conducting a paired t-test with a baseline at every imaging time point, where *P < 0.05.
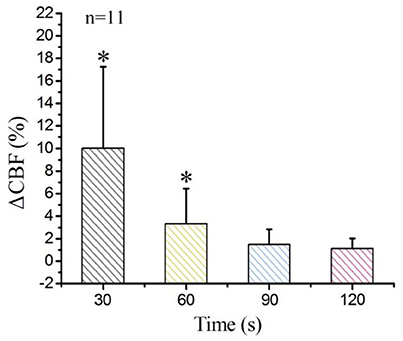
Figure 4. Statistical analysis of ΔCBF at different times for the 11 examined rats. *P < 0.05, paired t-test.
Discussion
First, we qualitatively analyzed the laser-speckle contrast image obtained from one rat. The results showed that DCS enhanced cerebral blood flow. At first, the blood-flow velocity increased with DCS, and then, as time passed, it decreased until remaining steady at 90 s. Next, we used the ΔCBF to analyse quantitatively the changes of blood flow for four ROIs. The results showed that the blood flows of three regions all increased; the values of ΔCBF of ROI1, ROI2, ROI3, and ROI4 were 11.7 ± 5.2%, 14.1 ± 6.3%, 11.7 ± 5.6%, and 10.6 ± 6.3%, respectively, at the time of 30 s. These results indicate that DCS significantly increases the cerebral blood flow. We conclude that the direct-current-induced nerve excitement and increased oxygen consumption led to the increases of blood-flow velocity. Finally, we analyzed the ΔCBF for the 11 rats and the statistical analysis of the results also showed that the ΔCBF increased with DCS.
Nan Li et al. studied hemodynamic responses produced by pulsed electric stimulation with a 1 ms pulse train at 5 Hz for a duration of 10 s using a stimulator (Li et al., 2009). They found that the pulsed electric stimulation can enhance the cerebral blood flow of rat. In our paper, we evaluate the blood flow with DCS. Compared to pulsed electric stimulation, DCS is a noninvasive neuromodulation technology. The different results were reduced by different simulation model and parameters.
A previous study utilized LDF to evaluate cerebral hemodynamic responses induced by DCS (Wachter et al., 2011). However, in our study, we used LSCI method to measure the blood flow induced by DCS. First, in imaging technology, LDF that is a method for evaluating cerebral hemodynamic responses can only provide information about the cerebral blood flow in the local region of the brain (approximately 1 mm3), and the spatial resolution is not high enough (Shepherd and Berg, 1990). Compared to LDF, LSCI can only not obtain regional blood-flow distributions without scanning, but also offers many advantages such as high spatial and temporal resolutions, imaging without contrast agents and real-time imaging. LSCI can measure the spread of cerebral blood flow change in horizontal direction due to two-dimensional imaging. However, since the LSCI can not supply depth information of the imaging region, we do not obtain the spread of cerebral blood flow change in depth direction. A major contribution on understanding of mechanism of DCS is that we measured the spread of cerebral blood flow change in horizontal direction and monitored the cerebral blood flow change of each blood vessel as shown in Figure 3a. Second, in imaging results, the previous study showed that anodal DCS can increase cerebral blood flow for periods up to 30 min. In our study, the duration of ΔCBF induced by DCS was not more than 90 s, significant less than the duration employed in the previous study. In that study, the current intensity and duration of the stimulus were 100 μA and 15 min, respectively. In our study, in contrast, the current intensity and duration of the stimulus were 15 μA and 10 s, respectively. We conclude that low stimulus-current intensity and short stimulus duration reduce the duration of ΔCBF. In further research, we will stimulate the brain cortices of rats with different current intensities and stimulus durations to analyze the relationship between duration of ΔCBF and stimulus current intensity.
In this study, we merely placed the anode electrode against the surface of the brain for direct electrical stimulation and did not monitor the blood flow under DCS. Results from this work will form the foundation for further studies in which we will use LSCI to monitor changes of blood flow with DCS.
Direct-current stimulation (DCS) can affect the resting potential of the neuronal membrane and neural activity (Agarwal et al., 2013; Zhang and Song, 2018). Anodal DCS has excitatory effects, and cathodal DCS has inhibitory effects on the underlying cortex. As we know, neurons and astrocytes directly regulate the local blood flow within the capillaries, resulting in local neurovascular coupling (Liao et al., 2013; Kalchenko et al., 2014). Therefore, DCS can causes the change of hemodynamics by neurovascular coupling.
Thus, anodal DCS can enhance cerebral blood flow and alter cortical hemodynamic responses. This finding indicates that DCS shows potential for use as a powerful and noninvasive method for creating therapeutic interventions with ischemic strokes.
Ethics Statement
All procedures were carried out in accordance with the Animal Ethics and Administrative Council of Yanshan University and Hebei Province, People's Republic of China. Animal owners agreed that the rats were used in the experiment. There is not any additional considerations.
Author Contributions
SH, TZ, and LL designed and coordinated the study, SH, TZ, JD, and LL carried out experiment and data process, and drafted the manuscript. All authors gave final approval for publication.
Conflict of Interest Statement
The authors declare that the research was conducted in the absence of any commercial or financial relationships that could be construed as a potential conflict of interest.
Acknowledgments
This research was supported by Technology Supporting Program of Hebei Province (17277718D).
References
Agarwal, S. M., Shivakumar, V., Bose, A., Subramaniam, A., Nawai, H., Chhabra, H., et al. (2013). Transcranial direct current stimulation in schizophrenia. Clin. Psychopharmacol. Neurosci. 11, 118–125. doi: 10.9758/cpn.2013.11.3.118
Antal, A., Kincses, T. Z., Nitsche, M. A., and Paulus, W. (2003). Manipulation of phosphene thresholds by transcranial direct current stimulation in man. Exp. Brain. Res. 150, 375–378. doi: 10.1007/s00221-003-1459-8
Baker, J. M., Rorden, C., and Fridriksson, J. (2010). Using transcranial direct-current stimulation to treat stroke patients with aphasia. Stroke 41, 1229–1236. doi: 10.1161/STROKEAHA.109.576785
Baudewig, J., Nitsche, M. A., Paulus, W., and Frahm, J. (2001). Regional modulation of BOLD MRI responses to human sensorimotor activation by transcranialdirect current stimulation. Magn. Reson. Med. 45, 196–201. doi: 10.1002/1522-2594(200102)45:2<196::AID-MRM1026>3.0.CO;2-1
Benninger, D. H., Lomarev, M., Lopez, G., Wassermann, E. M., Li, X., Considine, E., et al. (2010). Transcranial direct currentstimulation for the treatment of Parkinson's disease. J. Neurol. Neurosurg. Psychiatr. 81, 1105–1111. doi: 10.1136/jnnp.2009.202556
Briers, J. D., Richards, G., and He, X. W. (1999). Capillary blood flow monitoring using laser speckle contrast analysis (LASCA). J. Biomed. Opt. 4, 164–175. doi: 10.1117/1.429903
Briers, J. D., and Webster, S. (1996). Laser speckle contrast analysis (LASCA): a nonscanning, full-field technique for monitoring capillary blood flow. J. Biomed. Opt. 1, 174–179. doi: 10.1117/12.231359
Chao, P. Y., and Li, P. C. (2016). Three-dimensional shear wave imaging based on full-field laser speckle contrast imaging with one dimensional mechanical scanning. Opt. Express 24, 18860–18870. doi: 10.1364/OE.24.018860
Dunn, A. K. (2012). Laser speckle contrast imaging of cerebral blood flow. Ann. Biomed. Eng. 40, 367–377. doi: 10.1007/s10439-011-0469-0
Ferrucci, R., and Priori, A. (2014). Transcranial cerebellar direct current stimulation (tcDCS): motor control, cognition, learning and emotions. NeuroImage 85, 918–923. doi: 10.1016/j.neuroimage.2013.04.122
Giovannella, M., Ibañez, D., Gregori-Pla, C., Kacprzak, M., Mitjà, G., Ruffini, G., et al. (2018). Concurrent measurement of cerebral hemodynamics and electroencephalography during transcranial direct current stimulation. Neurophotonics 5:015001. doi: 10.1117/1.NPh.5.1.015001
Hunter, T., Sacco, P., Nitsche, M. A., and Turner, D. L. (2009). Modulation of internal model formation during force field-induced motor learning by anodal transcranial direct current stimulation of primary motor cortex. J. Physiol. 587, 2949–2961. doi: 10.1113/jphysiol.2009.169284
Jeffery, D. T., Norton, J. A., Roy, F. D., and Gorassini, M. A. (2007). Effects of transcranial direct current stimulation on the excitability of the leg motor cortex. Exp. Brain. Res. 182, 281–287. doi: 10.1007/s00221-007-1093-y
Kalchenko, V., Israeli, D., Kuznetsov, Y., and Harmelin, A. (2014). Transcranial optical vascular imaging (TOVI) of cortical hemodynamics in mouse brain. Sci. Rep. 25:5839. doi: 10.1038/srep05839
Kwon, Y. H., Ko, M. H., Ahn, S. H., Kim, Y. H., Song, J. C., Lee, C. H., et al. (2008). Primary motor cortex activation by transcranial direct current stimulation in the human brain. Neurosci. Lett. 435, 56–59. doi: 10.1016/j.neulet.2008.02.012
Li, H., Liu, Q., Lu, H. Y., Li, Y., Zhang, H. F., and Tong, S. B. (2014). Directly measuring absolute flow speed by frequency-domain laser speckle imaging. Opt. Express 22, 21079–21087. doi: 10.1364/OE.22.021079
Li, M. H., Miao, P., Zhu, Y. S., and Tong, S. B. (2011). Functional laser speckle imaging of cerebral blood flow under hypothermia. J. Biomed. Opt. 16:086011. doi: 10.1117/1.3610995
Li, N., Jia, X. F., Murari, K., Parlapalli, R., Rege, A., and Thakor, N. V. (2009). High spatiotemporal resolution imaging of the neurovascular response to electrical stimulation of rat peripheral trigeminal nerve as revealed by in vivo temporal laser speckle contrast. J. Neurosci. Methods 176, 230–236. doi: 10.1016/j.jneumeth.2008.07.013
Liao, L. D., Tsytsarev, V., Delgado-Martínez, I., Li, M. L., Erzurumlu, R., Vipin, A., et al. (2013). Neurovascular coupling: in vivo optical techniques for functional brain imaging. Biomed. Eng. 12:38. doi: 10.1186/1475-925X-12-38
Marlow, N. M., Bonilha, H. S., and Short, E. B. (2013). Efficacy of transcranial direct current stimulation and repetitive transcranial magnetic stimulation for treating fibromyalgia syndrome: a systematic review. Pain Pract. 13, 131–145. doi: 10.1111/j.1533-2500.2012.00562.x
Nitsche, M. A., Boggio, P. S., Fregni, F., and Pascual-Leone, A. (2009). Treatment of depression with transcranial direct current stimulation (tDCS): a review. Exp. Neurol. 219, 14–19. doi: 10.1016/j.expneurol.2009.03.038
Paquette, C., Sidel, M., Radinska, B. A., Soucy, J. P., and Thiel, A. (2011). Bilateral transcranial direct current stimulation modulates activation-induced regional blood flow changes during voluntary movement. J. Cereb. Blood Flow Metab. 31, 2086–2095. doi: 10.1038/jcbfm.2011.72
Ponticorvo, A., and Dunn, A. K. (2010). Simultaneous imaging of oxygen tension and blood flow in animals using a digital micromirror device. Opt. Express 18, 8160–8170. doi: 10.1364/OE.18.008160
Ragert, P., Vandermeeren, Y., Camus, M., and Cohen, L. G. (2008). Improvement of spatial tactile acuity by transcranial direct current stimulation. Clin. Neurophysiol. 119, 805–811. doi: 10.1016/j.clinph.2007.12.001
San-juan, D., Morales-Quezada, L., Orozco Garduño, A. J., Alonso-Vanegas, M., González-Aragón, M. F., Espinoza López, D. A., et al. (2015). Transcranial direct current stimulation in epilepsy. Brain Stimul. 8, 455–464. doi: 10.1016/j.brs.2015.01.001
Shepherd, A. P., and Berg, P. (1990). Laser-Doppler Blood Flowmetry. Amsterdam: Springer Netherlands.
Siebner, H. R., Lang, N., Rizzo, V., Nitsche, M. A., Paulus, W., Lemon, R. N., et al. (2004). Preconditioning of low-frequency repetitive transcranial magnetic stimulation with transcranial direct current stimulation: evidence for homeostatic plasticity in the human motor cortex. J. Neurosci. 24, 3379–3385. doi: 10.1523/JNEUROSCI.5316-03.2004
Soler, M., Kumru, H., Pelayo, R., Vidal, J., Tormos, J. M., Fregni, F., et al. (2010). Effectiveness of transcranial direct current stimulation and visual illusion on neuropathic pain in spinal cord injury. Brain 133, 2565–2577. doi: 10.1093/brain/awq184
Tanaka, S., Hanakawa, T., Honda, M., and Watanabe, K. (2009). Enhancement of pinch force in the lower leg by anodal transcranial direct current stimulation. Exp. Brain. Res. 196, 459–465. doi: 10.1007/s00221-009-1863-9
Wachter, D., Wrede, A., Schulz-Schaeffer, W., Taghizadeh-Waghefi, A., Nitsche, M. A., Kutschenko, A., et al. (2011). Transcranial direct current stimulation induces polarity-specific changes of cortical blood perfusion in the rat. Exp. Neurol. 227, 322–327. doi: 10.1016/j.expneurol.2010.12.005
Zakharov, P., Völker, A. C., Wyss, M. T., Haiss, F., Calcinaghi, N., Zunzunegui, C., et al. (2009). Dynamic laser speckle imaging of cerebral blood flow. Opt. Express 17, 13904–13917. doi: 10.1364/OE.17.013904
Zhang, H., Li, P., Feng, N., Qiu, J., Li, B., Luo, W., et al. (2012). Correcting the detrimental effects of nonuniform intensity distribution on fiber-transmitting laser speckle imaging of blood flow. Opt. Express 20, 508–517. doi: 10.1364/OE.20.000508
Zhang, Y., and Song, W. (2018). Transcranial direct current stimulation in disorders of consciousness: a review. Int. J. Neurosci. 128, 255–261. doi: 10.1080/00207454.2017.1381094
Keywords: direct-current stimulation, laser-speckle contrast imaging, cerebral blood flow, hemodynamic response, rat
Citation: Hu S, Zheng T, Dong Y, Du J and Liu L (2018) Effect of Anodal Direct-Current Stimulation on Cortical Hemodynamic Responses With Laser-Speckle Contrast Imaging. Front. Neurosci. 12:503. doi: 10.3389/fnins.2018.00503
Received: 24 March 2018; Accepted: 04 July 2018;
Published: 26 July 2018.
Edited by:
Takashi Hanakawa, National Center of Neurology and Psychiatry, JapanReviewed by:
Vassiliy Tsytsarev, University of Maryland, College Park, United StatesTomoko Tanaka, Tokyo Metropolitan Institute of Medical Science, Japan
Copyright © 2018 Hu, Zheng, Dong, Du and Liu. This is an open-access article distributed under the terms of the Creative Commons Attribution License (CC BY). The use, distribution or reproduction in other forums is permitted, provided the original author(s) and the copyright owner(s) are credited and that the original publication in this journal is cited, in accordance with accepted academic practice. No use, distribution or reproduction is permitted which does not comply with these terms.
*Correspondence: Lanxiang Liu, liulanxiang66@sina.com