- Janssen Research and Development, LLC, San Diego, CA, United States
GPR139, a Gq-coupled receptor that is activated by the essential amino acids L-tryptophan and L-phenylalanine, is predominantly expressed in the brain and pituitary. The physiological function of GPR139 remains elusive despite the availability of pharmacological tool agonist compounds and knock-out mice. Whole tissue RNA sequencing data from human, mouse and rat tissues revealed that GPR139 and the dopamine D2 receptor (DRD2) exhibited some similarities in their distribution patterns in the brain and pituitary gland. To determine if there was true co-expression of these two receptors, we applied double in situ hybridization in mouse tissues using the RNAscope® technique. GPR139 and DRD2 mRNA co-expressed in a majority of same cells within part of the dopaminergic mesolimbic pathways (ventral tegmental area and olfactory tubercle), the nigrostriatal pathway (compact part of substantia nigra and caudate putamen), and also the tuberoinfundibular pathway (arcuate hypothalamic nucleus and anterior lobe of pituitary). Both receptors mRNA also co-express in the same cells of the brain regions involved in responses to negative stimulus and stress, such as lateral habenula, lateral septum, interpeduncular nucleus, and medial raphe nuclei. GPR139 mRNA expression was detected in the dentate gyrus and the pyramidal cell layer of the hippocampus as well as the paraventricular hypothalamic nucleus. The functional interaction between GPR139 and DRD2 was studied in vitro using a calcium mobilization assay in cells co-transfected with both receptors from several species (human, rat, and mouse). The dopamine DRD2 agonist did not stimulate calcium response in cells expressing DRD2 alone consistent with the Gi signaling transduction pathway of this receptor. In cells co-transfected with DRD2 and GPR139 the DRD2 agonist was able to stimulate calcium response and its effect was blocked by either a DRD2 or a GPR139 antagonist supporting an in vitro interaction between GPR139 and DRD2. Taken together, these data showed that GPR139 and DRD2 are in position to functionally interact in native tissue.
Introduction
GPR139 (aka GPRg1 or GPCR12) was originally cloned and characterized as a novel Gq-coupled orphan receptor (Liu et al., 2015). Several groups have reported surrogate ligands for GPR139, including TC-O 9311 [3,5-dimethoxybenzoic acid 2-[(1-naphthalenylamino)carbonyl]hydrazide] and JNJ-63533054 as potent and selective agonists (Hu et al., 2009; Shi et al., 2011; Dvorak et al., 2015; Wang et al., 2015). Based on known surrogate agonists, Isberg et al. (2014) disclosed a pharmacophore model and proposed that L-tryptophan (L-Trp) and L-phenylalanine (L-Phe) were the putative endogenous ligands for GPR139. Simultaneous to this, we provided additional and independent biological and pharmacological evidence to support that L-Trp and L-Phe activated GPR139 and supported their likelihood as endogenous ligands (Liu et al., 2015). Despite the availability of several selective agonists, the physiological function of GPR139 remains elusive. Its potential role in addiction (Kononoff et al., 2018), Parkinson’s disease (Bayer Andersen et al., 2016), locomotor activity (Liu et al., 2015), and schizophrenia (Atienza et al., 2018) has been suggested. L-Trp and L-Phe are the precursors for serotonin and dopamine biosynthesis, respectively, which are neurotransmitters implicated in mood regulation (Toker et al., 2010; Anjema et al., 2011; ten Hoedt et al., 2011; Jenkins et al., 2016). The amino acid sequence of GPR139 is highly conserved across species (Liu et al., 2015). Expression of GPR139 is almost exclusive to the central nervous system (CNS), except for expression observed in the pituitary (Matsuo et al., 2005; Susens et al., 2006; Liu et al., 2015). GPR139 mRNA is highly expressed in the lateral septum and medial habenula, and GPR139 immunoreactivity is also detected there (Liu et al., 2015). Relatively high expression of GPR139 mRNA is also found in the lateral caudate, zona incerta, and medial mammillary nucleus (Matsuo et al., 2005). As we compared the RNA sequence data between GPR139 and other known GPCRs in human, mouse, and rat tissues, we noticed that GPR139 and dopamine D2 receptor (DRD2) exhibited similar distribution patterns in the brain as well as in the pituitary gland across several species. Therefore, we investigated the possible co-expression of GPR139 and dopamine DRD2 mRNA in the same cells of brain as well as in pituitary by using RNAscope® double in situ hybridization in mouse tissue sections. We further explored a potential in vitro interaction between GPR139 and DRD2 by performing co-transfection experiments.
Materials and Methods
Compounds
Quinpirole (Quinpirole hydrochloride) was purchased from Sigma-Aldrich (St. Louis, MO, United States). L-741,626 [3-[4-(4-Chlorophenyl)-4-hydroxypiperidin-l-yl]methyl-1H-indole] was purchased from Tocris Bioscience (Bristol, United Kingdom). JNJ-3792165 [1H-pyrazole-3-carboxamide, 1-[(2,6-dichlorophenyl)methyl]-5-methyl-N-(3-methylphenyl)]and JNJ-63533054 [(S)-3-bromo-5-chloro-N-[2-oxo-2-[(1-phenylethyl)amino]ethyl] benzamide] were synthesized at Janssen Research and Development, LLC (San Diego, CA, United States) as previously described (Dvorak et al., 2015).
RNA Sequencing
Human RNA sequencing raw data of adult non-tumor tissue samples were obtained from the genotype-tissue expression (GTEx) project (GTExConsortium, 2015) version 6 release. Additional human tissue samples from individual donors were acquired from BioChian (Newark, CA, United States). Individual mouse samples (C57BL6, 10–12 weeks old, 5 mice for each tissue) were from Zyagen (San Diego, CA, United States). Rat pooled tissue samples (Sprague-Dawley, 10 weeks old, ≥4 rats for each tissue) were from Harlan Laboratories (Indianapolis, IN, United States). All samples were sequenced by BGI Americas (Cambridge, MA, United States) with the Illumina HiSeq (San Diego, CA, United States) platform using the same protocols applied in GTEx project. All RNA sequencing data were processed using Omicsoft tools (Cary, NC, United States). RNA sequencing reads were mapped onto human GRCh38, mouse GRCm38 and rat Rnor_5.0 genome assemblies. Ensemble gene models were applied to represent genes in each genome. Transcripts per kilobase million (TPM) was calculated to determine gene expression in each sample and to normalize across samples (Mortazavi et al., 2008). Samples with failing raw data and mapping quality control or having low consistency with other samples of the same tissue type, were removed from the final data calculations.
Tissue Preparation
Mouse coronal brain sections were obtained from Abbomax (San Jose, CA, United States). Mouse sagittal pituitary bulb sections were obtained from Zyagen (Cat. No. MP-502, San Diego, CA, United States). Both tissues were collected from total 3 adult C57BL/6 mice with gender randomly selected. Tissues were freshly harvested, fixed in 10% neutral buffered formalin solution (NBF), processed for paraffin embedding, and sectioned onto SuperFrost Plus slides (Fisher Scientific, Waltham, MA, United States) at 5 μm thickness. To reduce autofluorescence, the sectioned tissue samples were irradiated with UV light (30 Watt, 253 to 400 nm discrete emission, Philips, Amsterdam, Netherlands) at room temperature for 2 h before the in situ hybridization procedure.
RNAscope® in situ Hybridization
RNA in situ hybridization experiments were performed using RNAscope®, an RNA in situ hybridization technique described previously (Wang et al., 2012). The RNAscope® Fluorescent Multiplex Reagent Kit (Cat. No. 320850, Advanced Cell Diagnostics, Newark, CA, United States) was used according to the manufacturer’s instructions. Paired double-Z oligonucleotide probes were designed against target RNA using custom software to specifically detect the genes of interest. The following probes were used in this study: Mm-Gpr139 (Cat. 318051) and Mm-Drd2-C2 (Cat. 406501-C2). Probes were assigned to the following channel and fluorophore configuration: Mm-Gpr139-C1 (Cy3)/Mm-Drd2-C2 (Cy5). Formaldehyde fixed-paraffin embedded tissue section samples were sourced by ACD, and each sample was evaluated for RNA integrity with the positive control probes Mm-Ppib-C1 (Cat. 313911)/Mm-Polr2a-C2 (Cat. 312471-C2) and a 2-plex dapB negative control probe (Cat. 320751). Using the positive and negative control probes, optimization was performed to establish the following pretreatment conditions: 15 min target retrieval at 95–100°C and 30 min Protease Plus at 40°C. Staining was performed at ACD Newark by the Pharma Assay Services group. The nuclei of cells were counterstained with DAPI (4’,6-diamidino-2-phenylindole) nuclear stain before the slides were cover slipped. For brain slides, fluorescent images were acquired using a Zeiss LSM 700 confocal microscope. Confocal images were then processed and analyzed using Zen Lite 2.3 software (Blue Edition, Carl Zeiss Inc., Oberkocken, Germany). The pituitary slides were scanned and digitalized by using a Pannoramic SCAN II scanner (3DHISTECH Ltd, Budapest, Hungary) equipped with 40x objective lens, sCMOS color camera with 6.5um pixel size (pco.edge 4.2, PCO-Tech, Romulus, MI, United States) and a solid-state light source (Spectra X light engine, Lumencor, Beaverton, OR, United States). Resulting images were processed and analyzed with CaseViewer 2.1 software (3DHISTECH Ltd, Budapest, Hungary) and Zen Lite 2.3 software (Blue Edition, Carl Zeiss Inc., Oberkocken, Germany). GPR139 and DRD2 mRNA signals are illustrated with red and white color in the images, respectively.
Molecular Cloning of GPR139 and Dopamine D2 Receptor
GPR139 from human, mouse and rat were cloned from respective brain cDNAs as previously described (Liu et al., 2015). DRD2 from human, mouse and rat were cloned from respective brain cDNAs. Specific primers (Forward primer: 5’- atg tca GAA TTC GCC ACC atg gat cca ctg aat ctg tcc tgg tat gat-3’; Reverse primer: 5’- tga cat GCG GCC GCt cag cag tga agg atc ttc agg aag gcc ttg cgg aa-3’) designed based on published human DRD2 cDNA coding region (Genbank Accession NO: M30625.1) were used for PCR amplification of the human brain cDNA (Clontech) using an Expanded High Fidelity PCR System (Roche). The resulting PCR product was cloned into pcDNA3.1 between EcoR1 and Not1 sites (Promega, Madison, WI, United States). The insert region was sequenced by Eton Biosciences (San Diego, CA, United States) to verify the identities. Similarly, mouse and rat DRD2 cDNA were PCR amplified using primers (mouse, Forward primer: 5’- atg tca GAA TTC GCC ACC atg gat cca ctg aac ctg tcc tgg tac-3’, Reverse primer: 5’- atg tca GCG GCC GCt cag cag tgc agg atc ttc atg aag gcc tt-3’; rat Forward primer: 5’- atg tca GAA TTC GCC ACC atg gat cca ctg aac ctg tcc tgg tac gat-3’, Reverse primer: 5’- atg tct GCG GCC GCt cag cag tgc aag atc ttc atg aag gcc tt-3’) designed specifically based on the mouse and rat DRD2 cDNA coding regions (Genbank accession Nos: mouse DRD2, NM_010077.3; rat DRD2, NM_012547.1). The mouse and rat brain cDNAs (Clontech) were used as the templates for cDNA amplifications.
Calcium Mobilization Assay
HEK293 cells transiently transfected with either Gqi5, DRD2 (human, mouse, and rat), GPR139 (human, mouse, and rat) together or alone, using Fugene HD reagent (Promega, Madison, WI, United States). For 10 cm dish, total of 10 μg of DNA were used for each transfection. For co-expression of GPR139 and DRD2, 5 μg each of the DNAs were used. For co-transfection of DRD2 and Gqi5, 7.5 μg of DRD2, and 2.5 μg of Gqi5 were used. The transfected cells were grown in DMEM F-12K culture media (Corning, Corning, NY, United States) containing 10% fetal bovine serum (FBS), 1× penicillin/streptomycin, 1× sodium pyruvate, 20 mM HEPES. Twenty-four hours after the transfection, cells were detached with 0.25% trypsin/2.25 mM EDTA and resuspended in plating media DMEM high glucose (Hyclone) containing 10% FBS, 1× penicillin/streptomycin, 1× sodium pyruvate, 20 mM HEPES. Cells were seeded at 50 μl/well (50,000 cells/well) in poly-D-lysine-coated, black-walled, clear-bottom 96-well tissue culture plates and incubated overnight at 37°C, 5% CO2. Forty-eight hours after the transfection, cell culture medium was aspirated and cells were loaded with 1× BD calcium loading dye (Becton Dickinson, Franklin Lakes, NJ, United States) solution at 100 μl/well and incubated at 37°C, 5% CO2 for 45 min. Compounds L-741,626, JNJ-3792165, and JNJ-63533054 dilutions were prepared in HBSS from 10 mM dimethyl sulfoxide (DMSO) stocks, while Quinpirole dilutions were prepared from 0.1 mM HCl stocks. Cells were treated with 10 μM of DRD2 antagonist L-741,626 or GPR139 antagonist JNJ-2165 for 10 min. Log dilution of 10 μM of DRD2 agonist Quinpirole and GPR139 agonist JNJ-3054 (20 μl) were done on the Fluorometric Imaging Plate Reader Tetra (FLIPR, Molecular Devices, Sunnyvale, CA, United States) and changes in fluorescence that reflect calcium mobilization were monitored at 1-s intervals for 90 s, followed by 3-s intervals for 60 s (excitation wavelength = 470–495 nm, emission wavelength = 515–575 nm). Data were exported as the difference between maximum and minimum fluorescence observed for each well. Data were expressed as relative fluorescence units (RFU). Results were calculated using non-linear regression to determine agonist EC50 values (expressed as mean ± S.E.M., Graphpad Prism 7 software, San Diego, CA, United States).
Results
GPR139 and DRD2 Share Similarities in Gene Expression Distribution Patterns
The RNA sequencing data from human tissues revealed that GPR139 and DRD2 exhibited some similarities in their distribution patterns in the CNS, such as olfactory, basal ganglia (including caudate nucleus, putamen, and nucleus accumbens), thalamus, hypothalamus, substantia nigra, medulla, and pons as well as pituitary gland (Figures 1A,B). Data from mouse and rat tissues are consistent with that of human, showing similar distribution patterns in CNS regions and in pituitary gland (Supplementary Figures S1, S2). It is noteworthy that DRD2 expression levels are much higher than GPR139 across all tissues and in all species. Besides, GPR139 expression was more abundant in rat pituitary compared to human and mouse pituitary tissues.
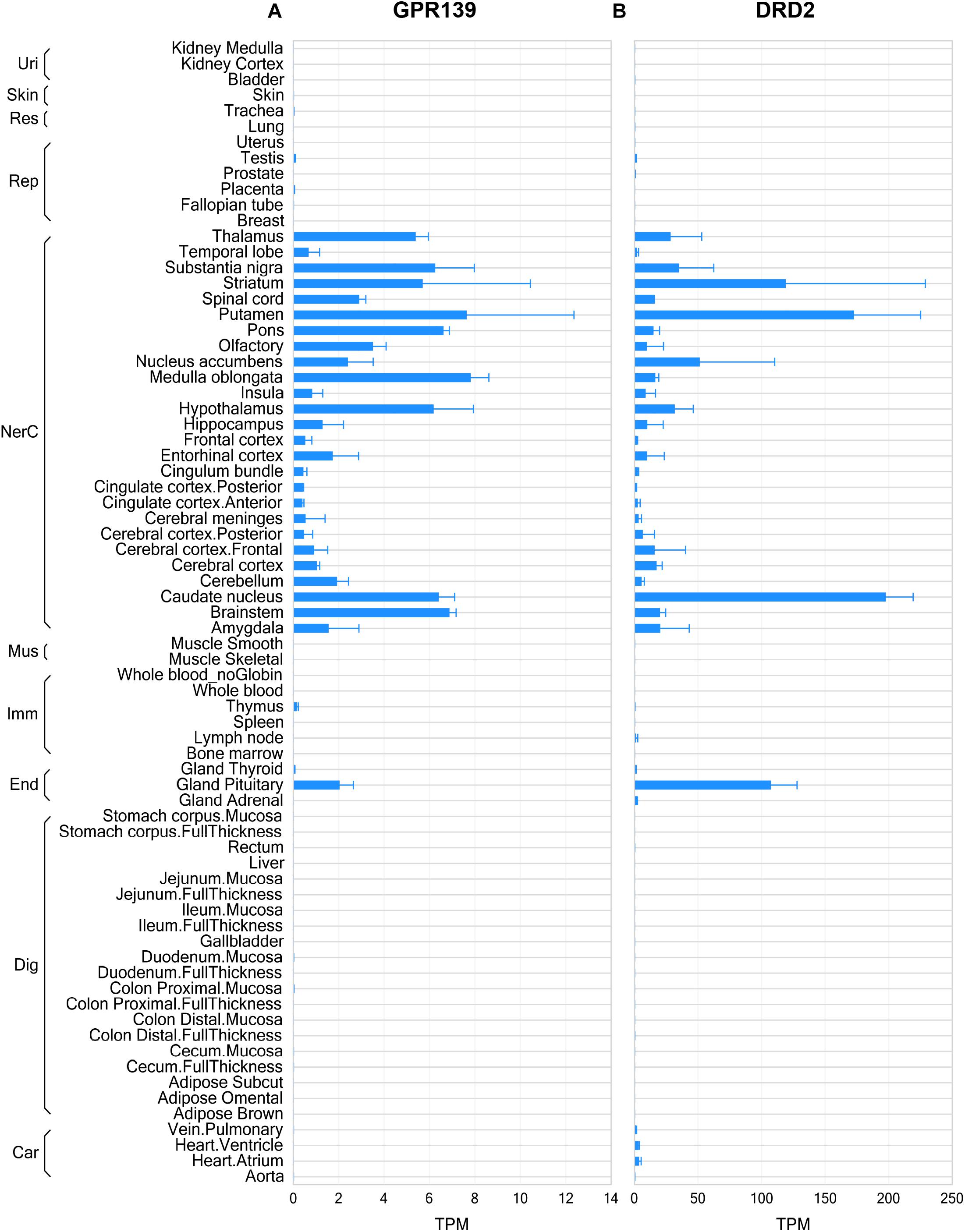
Figure 1. Gene expression of GPR139 and dopamine D2 receptor (DRD2) in human tissues by RNA sequencing. RNA sequencing data showing GPR139 (A) and DRD2 (B) mRNA expression in human tissues. Expression level is presented as transcripts per kilobase million (TPM). Tissues are grouped by organ systems: Uri, urinary; Res, respiratory; Rep, reproductive; NerC, central nervous; Mus. Muscular; Imm, lymphatic; End, endocrine; Dig, digestive; Car, cardiovascular.
GPR139 and DRD2 Co-express in the Same Cells of the Brain
We then investigated whether GPR139 and DRD2 co-express in the same cells of mouse brain. Double fluorescent in situ hybridization revealed cellular co-expression of these two receptors’ mRNA throughout the brain. In the telencephalon, moderate level of colocalization of GPR139 and DRD2 mRNA were observed in several regions, including olfactory tubercle (Tu, Figures 2A,B), ventral part of lateral septal nucleus (LSV, Figures 2C,D), and lateral region of caudate putamen (CPu, Figures 2E,F). In the diencephalon, GPR139 and DRD2 mRNA co-express in the same cells of ventral part of zona incerta (ZIV, Figures 3A,B), and posterior hypothalamus (PH, Figures 3C,D). While low level of colocalization were observed in lateral habenular nucleus (LHb, Figures 4A,B) and both dorsal and lateral part of arcuate hypothalamic nucleus (ArcD and ArcL, Figures 4C,D). In the midbrain, moderate level of co-expression of GPR139 and DRD2 mRNA in the same cells were found in periaqueductal gray (PAG, Figures 5A,B), ventral tegmental area (VTA, Figures 5C,D), and compact part of substantia nigra (SNC, Figures 5E,F). Additionally, low levels of co-expression in the same cells were also found in the reticular part of substantia nigra (SNR, Figures 6A,B), interpeduncular fossa (IPF, Figures 6C,D) and median raphe nucleus (MnR, Figures 6E,F).
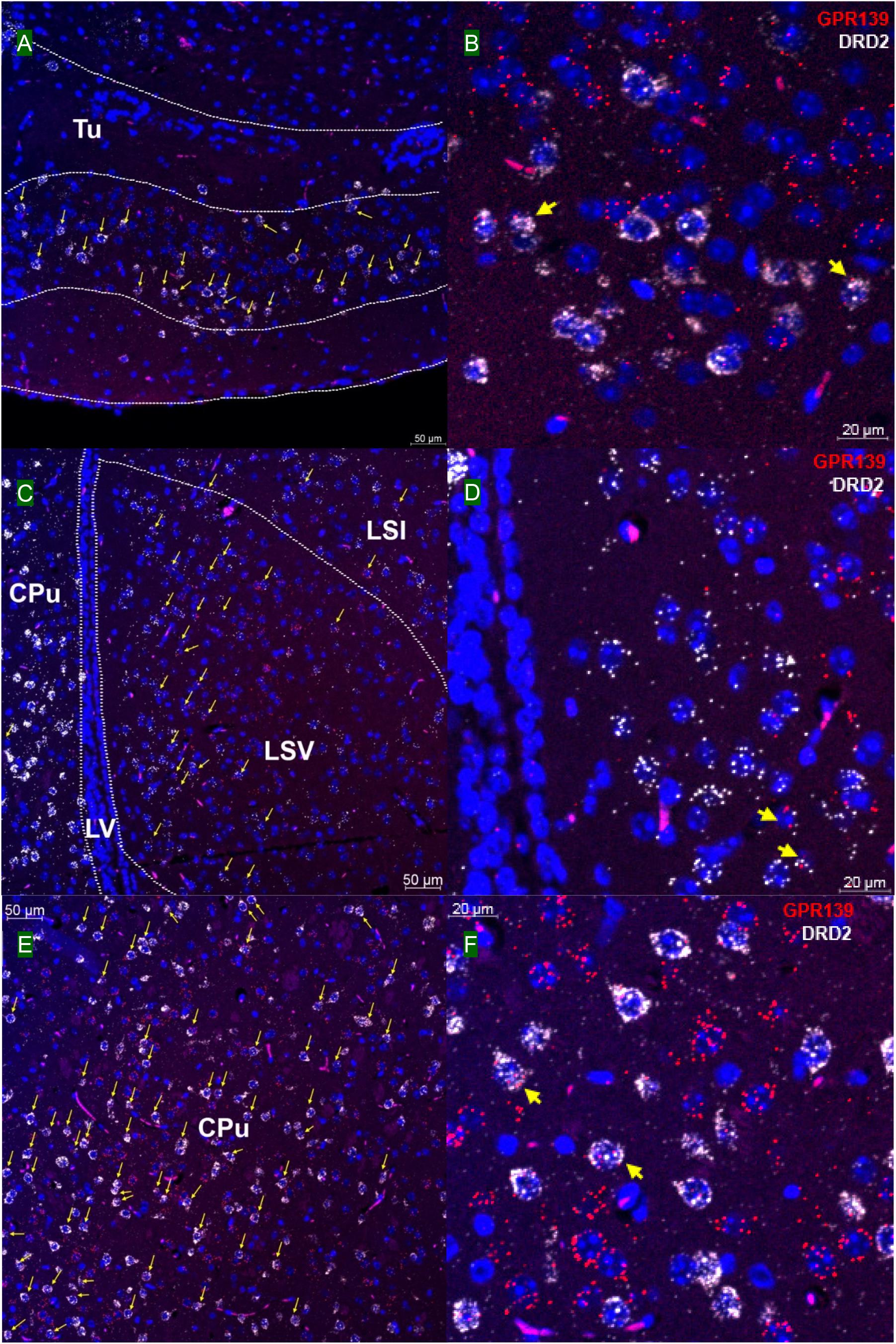
Figure 2. Double in situ hybridization of GPR139 (red) and DRD2 (white) mRNA in mouse telencephalon. Yellow arrows indicate colocalization of GPR139 and DRD2 mRNA in the same cells, including olfactory tubercle (Tu, A), ventral part of lateral septal nucleus (LSV, C), and lateral region of caudate putamen (CPu, E). Magnified images of Tu (B), LSV(D), and CPu (F) are shown with yellow arrows indicating example of colocalization signals. The nuclei of cells were stained with DAPI (blue). LSI, lateral septal nucleus, intermediate part; LV, lateral ventricle.
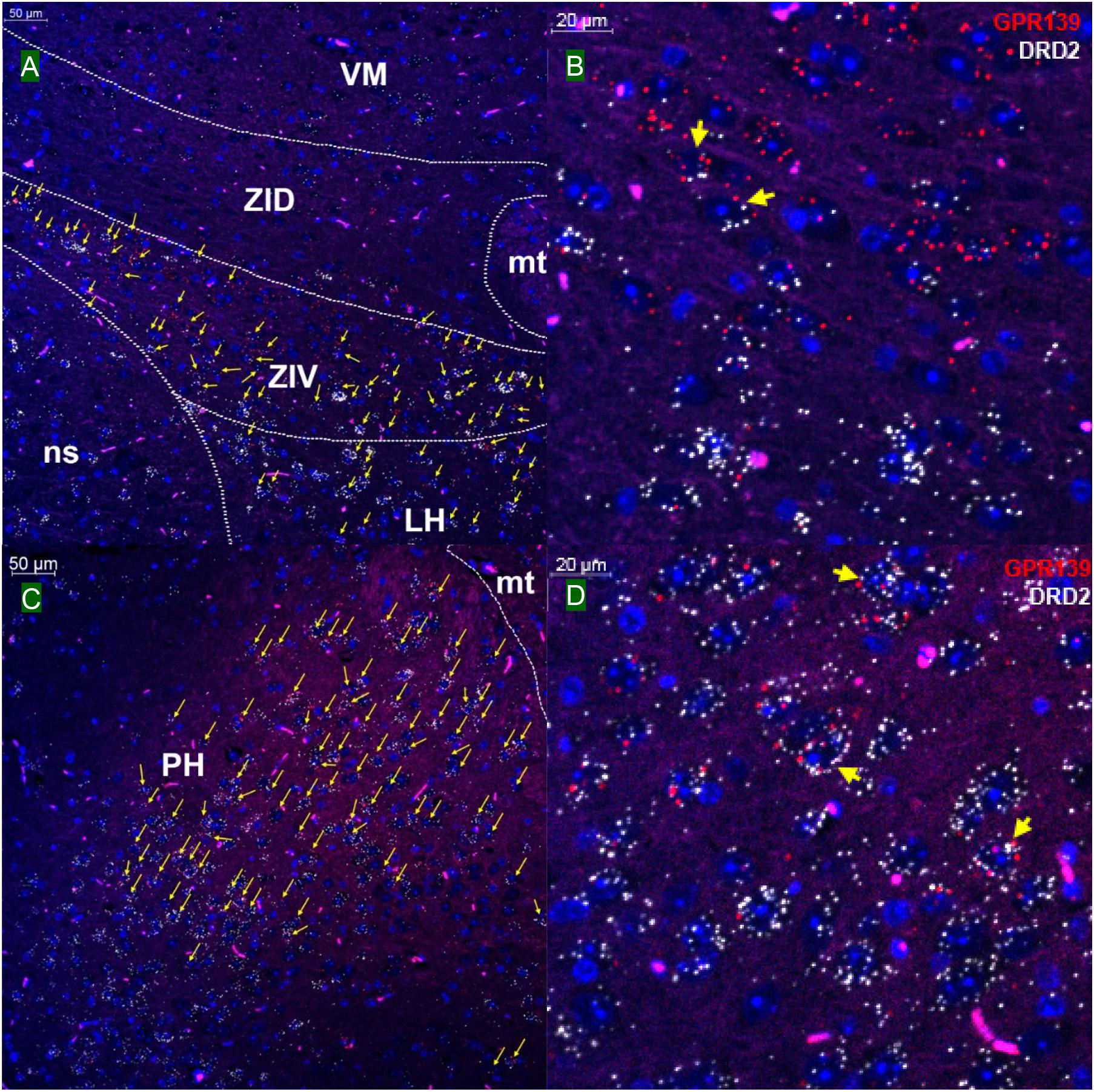
Figure 3. Double in situ hybridization of GPR139 (red) and DRD2 (white) mRNA in mouse diencephalon. Yellow arrows indicate colocalization of GPR139 and DRD2 mRNA in the same cells, including ventral part of zona incerta (ZIV, A) and posterior hypothalamus (PH, C). Magnified images of ZIV (B), PH(D) are shown with yellow arrows indicating example of colocalization signals. The nuclei of cells were stained with DAPI (blue). VM, ventromedial thalamic area; ZID, zona incerta, dorsal part; mt, mammillothalamic tract; ns, nigrostriatal bundle; LH, lateral hypothalamic area.
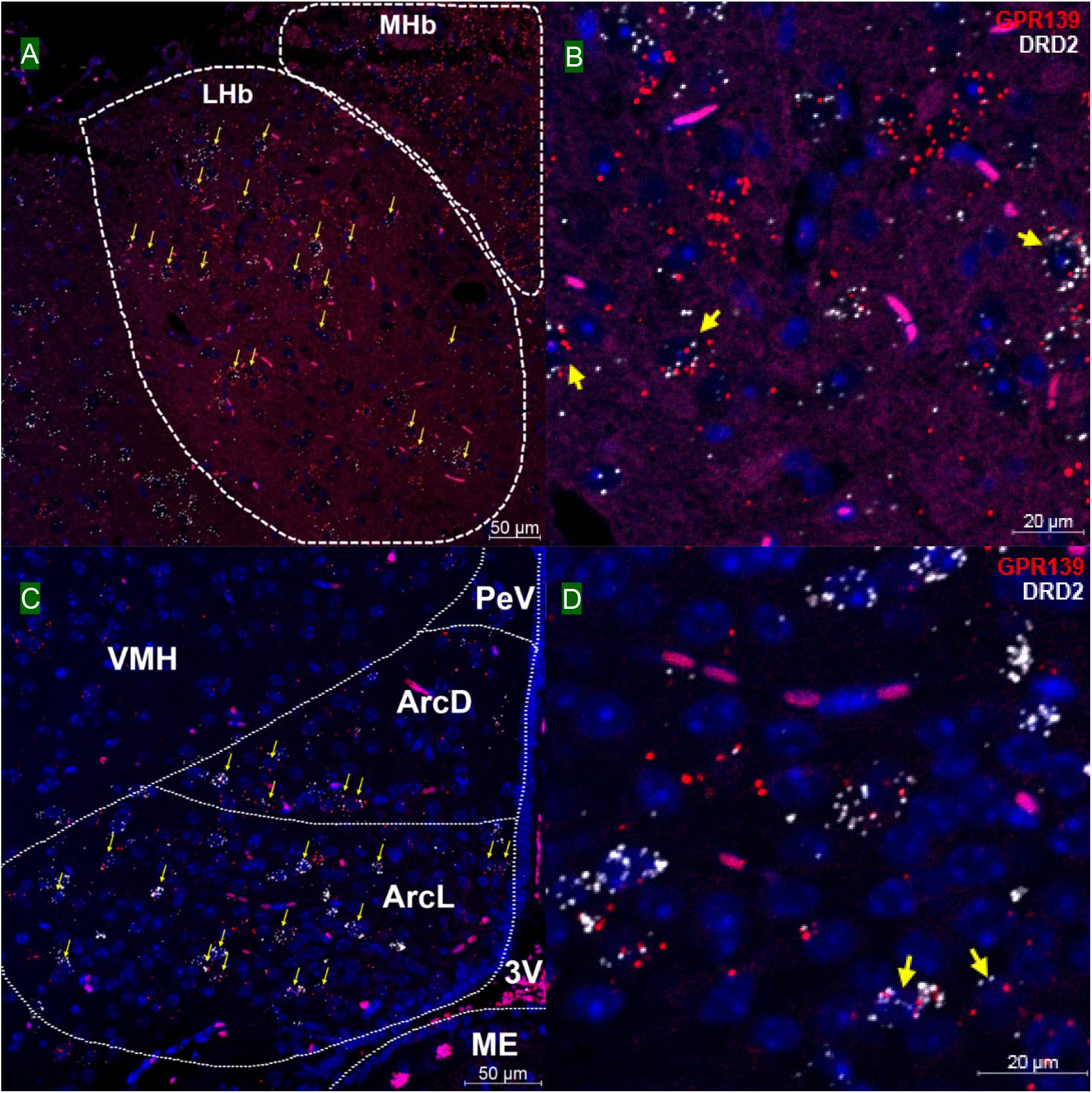
Figure 4. Double in situ hybridization of GPR139 (red) and DRD2 (white) mRNA in mouse diencephalon (continued). Yellow arrows indicate colocalization of GPR139 and DRD2 mRNA in the same cells, including lateral habenular nucleus (LHb, A) and both dorsal and lateral part of arcuate hypothalamic nucleus (ArcD and ArcL, C). Magnified images of LHb (B), ArcL(D) are shown with yellow arrows indicating example of colocalization signals. The nuclei of cells were stained with DAPI (blue). MHb, medial habenular nucleus; VMH, ventromedial hypothalamic nucleus; PeV, periventricular hypothalamic nucleus; 3V, 3rd ventricle; ME, median eminence.
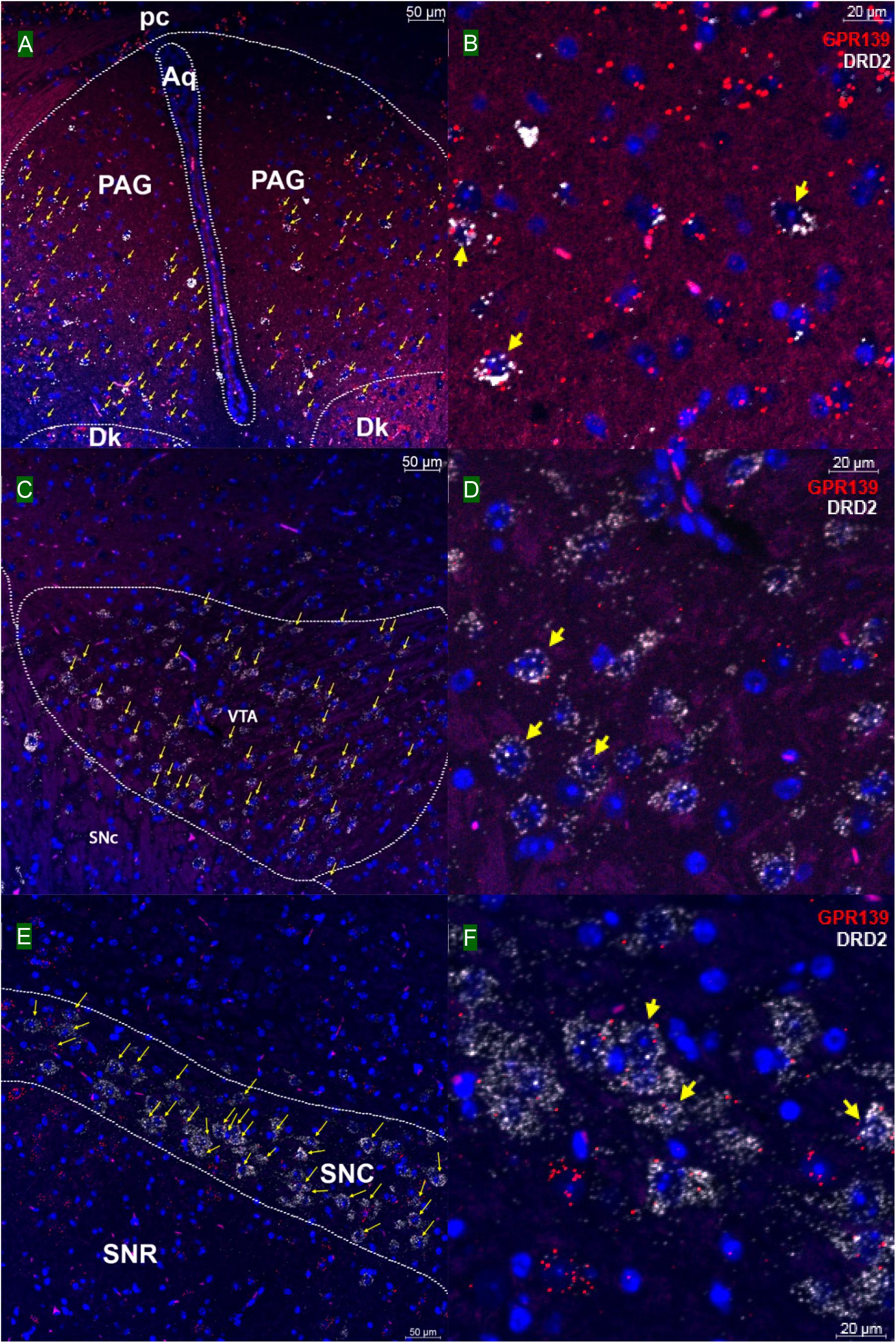
Figure 5. Double in situ hybridization of GPR139 (red) and DRD2 (white) mRNA in mouse mid brain. Yellow arrows indicate colocalization of GPR139 and DRD2 mRNA in the same cells, including periaqueductal gray (PAG, A), ventral tegmental area (VTA, C), and compact part of substantia nigra (SNC, E). Magnified images of PAG (B), VTA(D), and SNC (F) are shown with yellow arrows indicating example of colocalization signals. The nuclei of cells were stained with DAPI (blue). pc, posterior commissure; Aq, aqueduct; Dk, nucleus of Darkschewitsch; SNR, substantia nigra, reticular part.
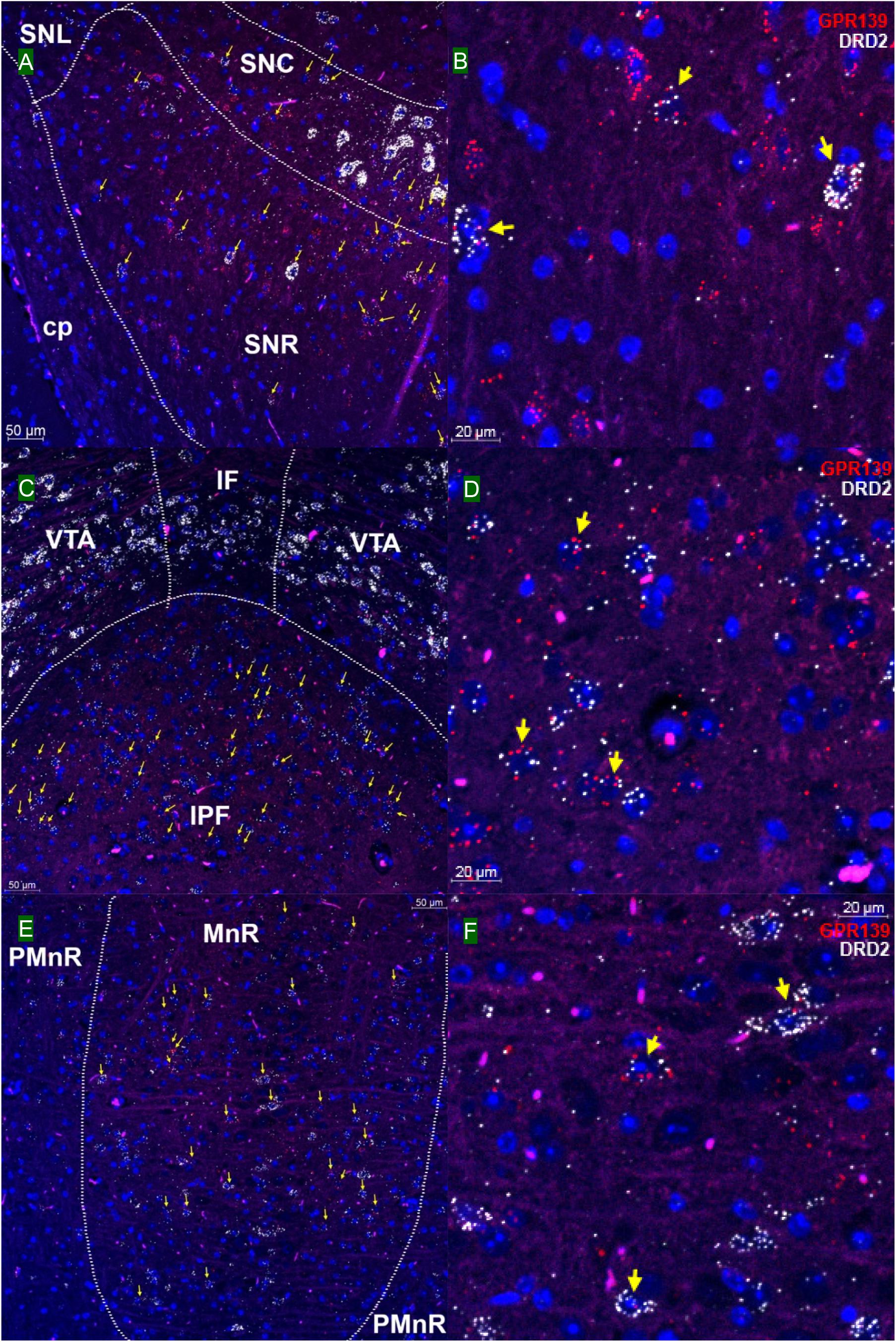
Figure 6. Double in situ hybridization of GPR139 (red) and DRD2 (white) mRNA in mouse mid brain (continued). Yellow arrows indicate colocalization of GPR139 and DRD2 mRNA in the same cells, including reticular part of substantia nigra (SNR, A), interpeduncular fossa (IPF, C) and median raphe nucleus (MnR, E). Magnified images of SNR (B), IPF(D), and MnR(F) are shown with yellow arrows indicating example of colocalization signals. The nuclei of cells were stained with DAPI (blue). SNC, substantia nigra, compact part; SNL, substantia nigra, lateral part; cp, cerebral, peduncle, basal part; VTA, ventral tegmental area; IF, interfascicular nucleus; PMnR, paramedian raphe nucleus.
Our in situ hybridization results confirmed the previous report that high expression of GPR139 mRNA was observed in medial habenula (MHb, Figure 4A) and lateral septum (LSV, Figures 2C,D), where GPR139 immunoreactivity are also detected (Liu et al., 2015). High expression of GPR139 mRNA has also been confirmed in caudate putamen (Figures 2E,F), zona incerta (ZIV, Figures 3A,B), and median part of medial mammalian nucleus (data not shown). Moderate expression was observed in the lateral habenula (LHb, Figures 4A,B), parafascicular thalamic nucleus (data not shown), arcuate hypothalamus (Figures 4C,D), the nucleus of Darkschewitsch and the medial vestibular nucleus (data not shown) (Matsuo et al., 2005). We also found that GPR139 mRNA was moderately expressed in the granular layer of the dentate gyrus in hippocampus (GrDG, Supplementary Figure S3A) and paraventricular hypothalamic nucleus (PVN, Supplementary Figure S3C). High level of GPR139 mRNA expression was also detected in pyramidal cell layer of the hippocampus (Py, Supplementary Figure S3B). No co-expression of GPR139 and DRD2 were detected among these regions. The distribution pattern of DRD2 mRNA reported in the present study are also consistent with literatures (Mansour et al., 1990; Gehlert et al., 1992; Missale et al., 1998; Beaulieu and Gainetdinov, 2011). As negative controls, negative signals of GPR139 and DRD2 mRNA in representative brain regions are shown in Supplementary Figure S6.
GPR139 and DRD2 Co-express in the Same Cells of Pituitary
Double in situ hybridization was also applied on mouse pituitary sections. GPR139 mRNA was observed exclusively in the anterior lobe of pituitary with moderate co-expression with DRD2 mRNA in the same cells throughout the entire anterior lobe of pituitary (Figures 7A,B; Mansour et al., 1990).
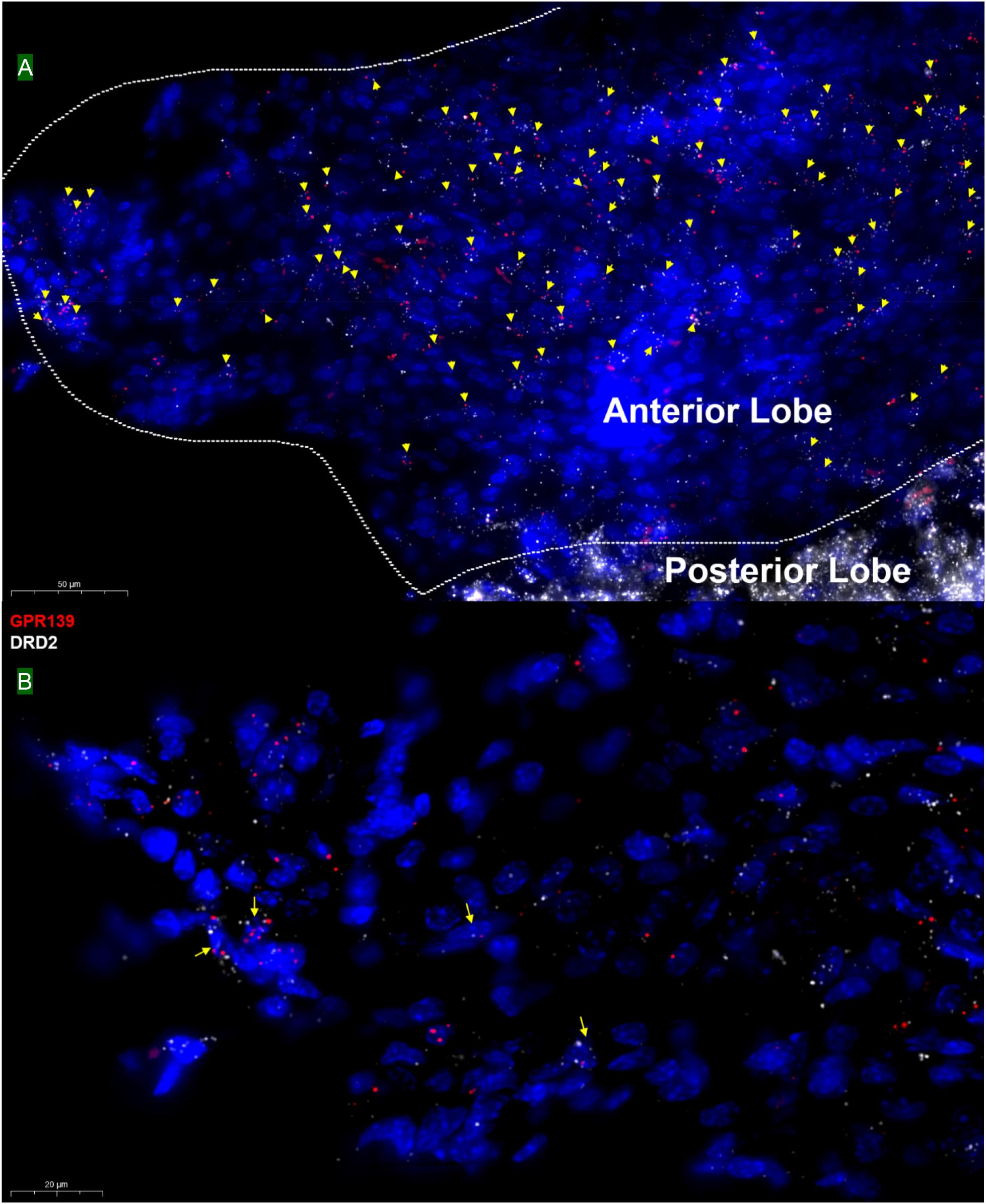
Figure 7. Double in situ hybridization of GPR139 (red) and DRD2 (white) mRNA in mouse pituitary. Yellow arrows indicate colocalization of GPR139 and DRD2 mRNA in the same cells of the anterior lobe (A). Magnified image (B) is shown with yellow arrow indicating example of colocalization signals. The nuclei of cells were stained with DAPI (blue).
DRD2 Activates Calcium Mobilization Through GPR139 in vitro
Based on the relatively high level of cellular colocalization between GPR139 and DRD2 mRNAs, we studied their in vitro functional interaction in HEK293 cells transiently transfected with human GPR139 and DRD2. Consistent with the Gi transduction pathway of DRD2, the DRD2 agonist quinpirole was not able to elicit a calcium response in HEK293 cells transfected with DRD2 alone (blue line, Figure 8A). Gqi5 is a chimeric G-protein of Gq protein with its last 5 amino acid residues at the very C-terminus replaced by the last C-terminal 5 residues of Gi protein (Conklin et al., 1993). When co-expressed with a GPCRs normally signal through Gi signaling pathway, Gqi5 is able to convert the Gi signal of GPCRs into Gq signal pathway, namely cause calcium mobilization. When co-transfected with Gqi5, quinpirole was able to stimulate a calcium response in DRD2 expressing cells in the low nM range (EC50 = 1.72 ± 1.11 nM, blue line, Figure 8B). This effect on calcium mobilization was blocked by the DRD2 antagonist L-741,626 (black line, Figure 8B). GPR139 is coupled to Gq coupled receptor and therefore, the GPR139 agonist, JNJ-63533054, was able to elicit calcium mobilization in HEK293 cells transfected with GPR139 alone, as previously reported (EC50 = 4.46 ± 1.26 nM, purple line, Figure 8C). This effect was antagonized by the GPR139 antagonist JNJ-3792165 (green line, Figure 8C). When DRD2 and GPR139 were co-transfected, the DRD2 agonist quinpirole stimulated calcium mobilization in the absence of Gqi5 (EC50 = 2.04 ± 1.32 nM, blue line, Figure 8D). This response was antagonized by either the DRD2 antagonist L-741,626 (black line, Figure 8D) or the GPR139 antagonist JNJ-3792165 (yellow line, Figure 8D). No calcium response was observed by either agonist in un-transfected HEK 293 cells or cells only expressing Gqi5 (Figures 8E,F). Similar interaction between GPR139 and DRD2 were observed using rat or mouse orthologue from each receptor (Supplementary Figures S4, S5). These results demonstrated an in vitro functional interaction between dopamine DRD2 and GPR139 in HEK293 cells.
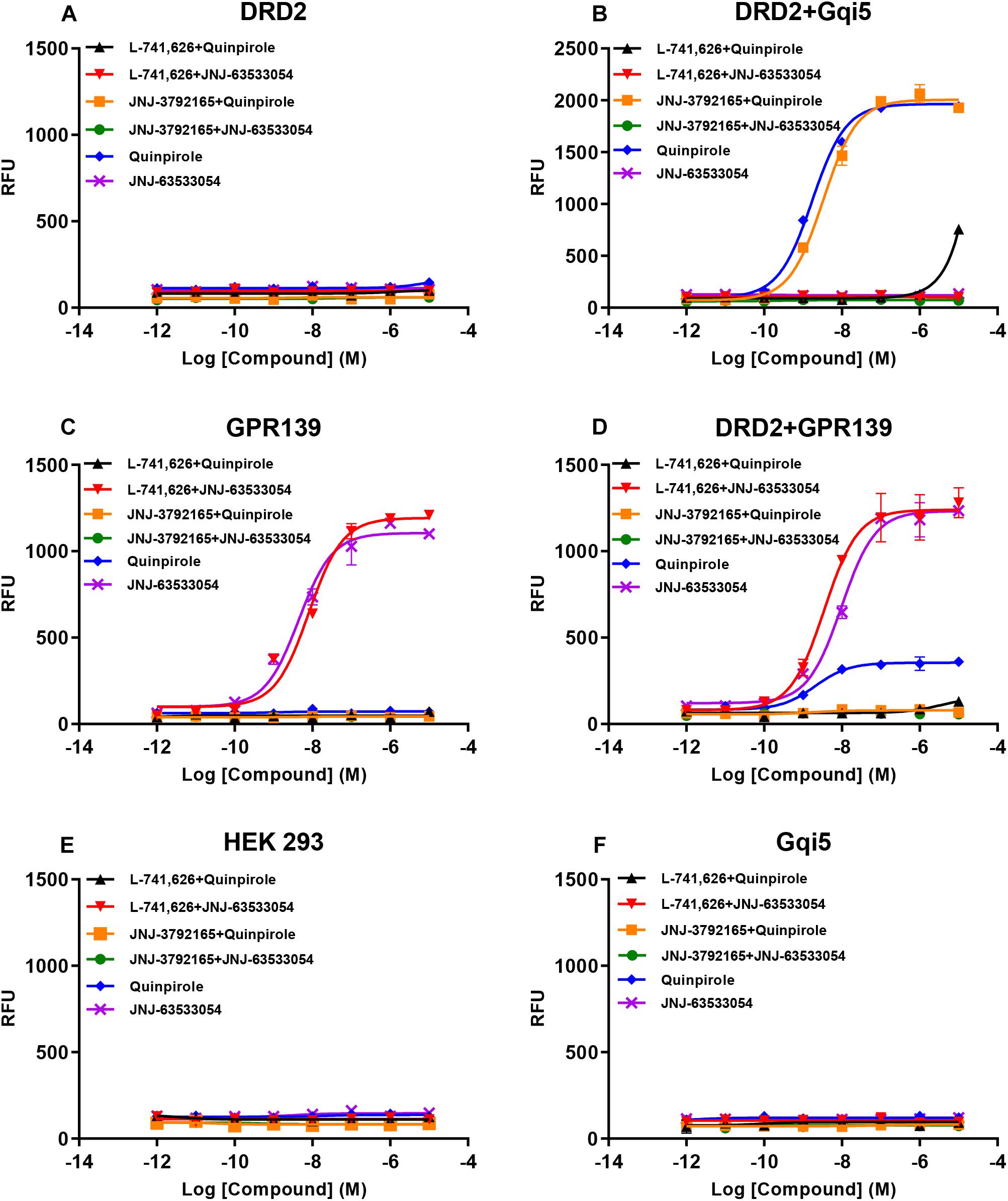
Figure 8. Concentration responses of the DRD2 agonist quinpirole and GPR139 agonist JNJ-63533054 calcium mobilization with or without 10 μM of the DRD2 antagonist L-741,626 or GPR139 antagonist JNJ-3792165 in HEK 293 cells transfected with only human DRD2 (A), human DRD2 and Gqi5 (B), only human GPR139 (C), human DRD2 and GPR139 (D), no receptors (E), or only Gqi5 (F). Only GPR139 (C) but not DRD2 (A) transfected alone elicited Ca2+ mobilization. DRD2 was able to elicit Ca2+ mobilization when co-transfected with either Gqi5 (B) or GPR139 (D). Neither control HEK293 cells (E) nor HEK293 cells transfected only with Gqi5 (F) elicited Ca2+ mobilization. Error bars represent standard error of the mean of duplicate measurements for each point (n = 2).
Discussion
Using the highly sensitive and selective RNAscope® technique, we demonstrated co-expression in the same cells of GPR139 and DRD2 mRNA in the ventral tegmental area (VTA, Figures 5C,D), olfactory tubercle (Tu, Figures 2A,B), substantia nigra pars compacta (SNC, Figures 5E,F), caudate and putamen (CPu, Figures 2E,F), arcuate hypothalamic nucleus (ArcH, Figures 4C,D) as well as the anterior lobe of pituitary gland (Figures 7A,B). All these brain regions are part of the dopaminergic afferent and efferent signaling network. Interestingly, in light of the DRD2/GPR139 mRNAs co-expression found in the mesolimbic pathway (projects from VTA to nucleus accumbens and Tu) (Ikemoto, 2010), a recent preclinical study using the selective GPR139 agonist JNJ-63533054 showed that GPR139 receptor activation reverses key addiction-like behaviors in dependent animals (Kononoff et al., 2018). Another GPR139 agonist 4-oxo-3,4-dihydro-1,2,3-benzotriazine has been reported to improve social withdrawal in a preclinical model of the negative symptom of schizophrenia (Atienza et al., 2018), involving the mesocortical pathway (initiate from VTA but instead project to the prefrontal cortex) (Ekhtiari and Paulus, 2016). A recent study reported that the GPR139 agonist TC-O 9311 (structurally identical to compound 1a used in this study) protected primary mesencephalic dopamine neurons against 1-methyl-4-phenylpyridinium (MPP+)-mediated degeneration, indicating a potential role of GPR139 in neuroprotection and Parkinson’s disease (Bayer Andersen et al., 2016). Meanwhile, it has also been reported that the activation of DRD2 by its agonist quinpirole protected dopamine neurons from MPP+ induced cell death (Wiemerslage et al., 2013). The co-expression of GPR139 and DRD2 mRNA in the same cells of nigrostriatal pathway (projects from SNC to CPu) and their in vitro signaling interaction may indicate the possibility of their functional interaction in terms of neuroprotection. And lastly the co-expression of GPR139 and DRD2 mRNA in the same cells of ArcH and the anterior lobe of pituitary gland match the tuberoinfundibular pathway (projects from ArcH to the median eminence which controls the secretion of prolactin from the anterior lobe of pituitary gland). While the physiological role of GPR139 in prolactin regulation has not been reported, dopamine is known as prolactin inhibitor and reduces prolactin release by binding to DRD2 expressed on the cell membrane of the lactotroph in the pituitary (Ben-Jonathan and Hnasko, 2001). Taken together, the co-expression of GPR139 and DRD2 mRNA in the same cells within dopaminergic signaling pathways may indicate a potential functional interaction between these two receptors under physiological conditions, which merits further in vivo investigation.
Co-expression of GPR139 and DRD2 mRNA in the same cells has also been demonstrated in lateral habenula (LHb, Figures 4A,B), lateral septum (LSV, Figures 2C,D), interpeduncular fossa (IPF, Figures 6C,D), and median raphe nucleus (MnR, Figures 6E,F). Specifically, the functions of lateral habenula has been attributed to several common mechanisms (Hikosaka, 2010). It is proposed that the LHb responds to the negative stimulus (e.g., failure and punishment) that derives from basal ganglia and transmit the signaling to dopaminergic neurons located in SNC and VTA, which ultimately leads to motor suppression (aversive outcome). On the other hand, painful or stressful stimulus activates LHb through limbic system (e.g., septum and medial frontal cortex), and LHb further inhibits both dopaminergic neurons in SNC and VTA, as well as serotonergic neurons in raphe nuclei (dorsal and medial, DnR, and MnR) through the projection from interpeduncular nucleus (IPN). These results may also suggest future research directions to their potential interaction in terms of habenula based neurotransmitter regulation and physiological functions.
Apart from the previously reported regions, our current study also revealed several other brain areas with GPR139 mRNA expression, such as dentate gyrus in hippocampus (GrDG, Supplementary Figure S3A), pyramidal cell layer of the hippocampus (Py, Supplementary Figure S3B) and paraventricular hypothalamic nucleus (PVN, Supplementary Figure S3C), which has not been reported previously. We also demonstrated the site of GPR139 mRNA expression in the pituitary (Figures 7A,B). The expression of GPR139 mRNA in the PVN and anterior lobe of pituitary may suggest a possible role for GPR139 in the hypothalamic-pituitary-adrenal (HPA) axis (Malenka et al., 2009), however this will remain to be determined.
Nonetheless, our previous immunohistochemistry results only showed positive GPR139 protein expression in medial habenula and lateral septum (Liu et al., 2015), which may indicate the variation of sensitivity between the methods detecting mRNA and protein expressions. In the current study, the RNAscope® technique utilizes two independent probes, which hybridize to their own target sequence in tandem for signal amplification. The situation that two independent probes hybridize to a non-specific target right next to each other is highly unlikely, which ensures selective amplification of target-specific signals and elimination of background noise. Moreover, GPR139 is activated by the essential amino acids L-Trp and L-Phe with EC50 values in the 30- to 300-μM range, which is consistent with the physiologic concentrations of both amino acids (Liu et al., 2015). Thus, it is probable that GPR139 is constitutively activated under native in vivo conditions. The constant activation of GPR139 may result in constant desensitization and receptor internalization, which may further disturb antibody binding and protein detection in regions with weak to moderate mRNA expression levels. Additional studies are required to prove the protein expression of GPR139 in those regions.
Once we observed that GPR139 and DRD2 appear to be co-expressed in the same cells in various brain regions, we investigated a possible in vitro interaction between these two receptors. We observed an intriguing signaling interaction where the presence of GPR139 was required to observe DRD2 agonist-elicited calcium mobilization when co-transfected with GPR139 in HEK 293 cells. This effect was blocked by either a DRD2 antagonist or a GPR139 antagonist (Figure 8D), indicating that the DRD2 agonist-stimulated Ca2+ signaling involves the activation of both DRD2 and GPR139. Just recently our group reported another in vitro signaling interaction between GPR139 and melanocortin receptors (MC3R, MC4R, and MC5R) (Nepomuceno et al., 2018). One of the possible mechanisms is the formation of heteroreceptor complexes between these receptors resulting in calcium signaling cross talk (Werry et al., 2003). The allosteric communication and functional cross talk between GPCR homo- and heterodimerization have been extensively reported (Borroto-Escuela et al., 2014, 2017). Specifically, DRD2 forms heteroreceptor complexes with other GPCRs or ion channel receptors, such as A2AR (adenosine 2A receptor)-DRD2 (Trifilieff et al., 2011), A2AR-DRD2-mGluR5 (metabotropic glutamate receptor 5) (Cabello et al., 2009), A2AR-DRD2-Sigma1R (Pinton et al., 2015), DRD2-NMDAR (N-methyl-D-aspartate receptor) (Liu et al., 2006), DRD2-5-HT2AR (serotonin 2A receptor) (Albizu et al., 2011), and DRD2-OXTR (oxytocin receptor) (de la Mora et al., 2016). These DRD2 heteroreceptor complexes are mainly found in the striatum (Borroto-Escuela et al., 2017). Additional studies using biochemical approach, such as fluorescence resonance energy transfer technique, or co-immunoprecipitation using specific antibodies for GPR139 and DRD2 would further support the interaction between these two receptors.
In conclusion, we found that GPR139 and the dopamine DRD2 mRNA co-express in the same cells of certain brain regions and that they functionally interact in an in vitro system. It is worthwhile to mention that there was one phase 1 clinical trial (NCT02959892) studied the effect of a GPR139 agonist (TAK-041) on amphetamine induced dopamine release with no result posted. It remains to be determined if the same intriguing in vitro signaling interaction will be observed in vivo.
Author Contributions
LW, PB, TL, and CL participated in research design. LW, GL, CK, and XY conducted the experiments. LW, GL, and XY performed the data analysis. LW, GL, XY, AH, PB, TL, and CL wrote or contributed to the writing of the manuscript.
Conflict of Interest Statement
All authors are paid employees at Janssen Research and Development, LLC.
Supplementary Material
The Supplementary Material for this article can be found online at: https://www.frontiersin.org/articles/10.3389/fnins.2019.00281/full#supplementary-material
References
Albizu, L., Holloway, T., González-Maeso, J., and Sealfon, S. C. (2011). Functional crosstalk and heteromerization of serotonin 5-HT2A and dopamine D2 receptors. Neuropharmacology 61, 770–777. doi: 10.1016/j.neuropharm.2011.05.023
Anjema, K., van Rijn, M., Verkerk, P. H., Burgerhof, J. G., Heiner-Fokkema, M. R., and van Spronsen, F. J. (2011). PKU: high plasma phenylalanine concentrations are associated with increased prevalence of mood swings. Mol. Genet. Metab. 104, 231–234. doi: 10.1016/j.ymgme.2011.05.017
Atienza, J., Reichard, H., Mulligan, V., Cilia, J., Monenschein, H., Collia, D., et al. (2018). S39. gpr139 an ophan gpcr affecting negative domains of schizophrenia. Schizophr. Bull. 44(Suppl. 1), S339–S339. doi: 10.1093/schbul/sby018.826
Bayer Andersen, K., Leander Johansen, J., Hentzer, M., Smith, G. P., and Dietz, G. P. (2016). Protection of primary dopaminergic midbrain neurons by GPR139 agonists supports different mechanisms of MPP(+) and rotenone toxicity. Front. Cell Neurosci. 10:164. doi: 10.3389/fncel.2016.00164
Beaulieu, J.-M., and Gainetdinov, R. R. (2011). The physiology, signaling, and pharmacology of dopamine receptors. Pharmacol. Rev. 63, 182–217. doi: 10.1124/pr.110.002642
Ben-Jonathan, N., and Hnasko, R. (2001). Dopamine as a prolactin (PRL) inhibitor. Endocrine Rev. 22, 724–763. doi: 10.1210/edrv.22.6.0451
Borroto-Escuela, D. O., Brito, I., Romero-Fernandez, W., Di Palma, M., Oflijan, J., Skieterska, K., et al. (2014). The G protein-coupled receptor heterodimer network (GPCR-HetNet) and its hub components. Int. J. Mol. Sci. 15,8570–8590. doi: 10.3390/ijms15058570
Borroto-Escuela, D. O., Carlsson, J., Ambrogini, P., Narváez, M., Wydra, K., Tarakanov, A. O., et al. (2017). Understanding the role of GPCR heteroreceptor complexes in modulating the brain networks in health and disease. Front. Cell. Neurosci. 11:37. doi: 10.3389/fncel.2017.00037
Cabello, N., Gandía, J., Bertarelli, D. C., Watanabe, M., Lluís, C., Franco, R., et al. (2009). Metabotropic glutamate type 5, dopamine D2 and adenosine A2a receptors form higher-order oligomers in living cells. J. Neurochem. 109, 1497–1507. doi: 10.1111/j.1471-4159.2009.06078.x
Conklin, B. R., Farfel, Z., Lustig, K. D., Julius, D., and Bourne, H. R. (1993). Substitution of three amino acids switches receptor specificity of Gqα to that of Giα. Nature 363:274. doi: 10.1038/363274a0
de la Mora, M. P., Pérez-Carrera, D., Crespo-Ramírez, M., Tarakanov, A., Fuxe, K., and Borroto-Escuela, D. O. ( (2016). Signaling in dopamine D2 receptor-oxytocin receptor heterocomplexes and its relevance for the anxiolytic effects of dopamine and oxytocin interactions in the amygdala of the rat. Biochim. et Biophys. Acta 1862, 2075–2085. doi: 10.1016/j.bbadis.2016.07.004
Dvorak, C. A., Coate, H., Nepomuceno, D., Wennerholm, M., Kuei, C., Lord, B., et al. (2015). Identification and SAR of glycine benzamides as potent agonists for the GPR139 receptor. ACS Med. Chem. Lett. 6, 1015–1018. doi: 10.1021/acsmedchemlett.5b00247
Ekhtiari, H., and Paulus, M. (2016). Preface: neuroscience for addiction medicine: from prevention to rehabilitation. Prog. Brain Res. 223, xxv–xxvi. doi: 10.1016/S0079-6123(16)00011-X
Gehlert, D. R., Gackenheimer, S. L., Seeman, P., and Schaus, J. (1992). Autoradiographic localization of [3H] quinpirole binding to dopamine D2 and D3 receptors in rat brain. Eur. J. Pharmacol. 211, 189–194. doi: 10.1016/0014-2999(92)90528-C
Hikosaka, O. (2010). The habenula: from stress evasion to value-based decision-making. Nat. Rev. Neurosci. 11:503. doi: 10.1038/nrn2866
Hu, L. A., Tang, P. M., Eslahi, N. K., Zhou, T., Barbosa, J., and Liu, Q. (2009). Identification of surrogate agonists and antagonists for orphan G-protein-coupled receptor GPR139. J. Biomol. Screen 14, 789–797. doi: 10.1177/1087057109335744
Ikemoto, S. (2010). Brain reward circuitry beyond the mesolimbic dopamine system: a neurobiological theory. Neurosci. Biobehav. Rev. 35, 129–150. doi: 10.1016/j.neubiorev.2010.02.001
Isberg, V., Andersen, K. B., Bisig, C., Dietz, G. P., Brauner-Osborne, H., and Gloriam, D. E. (2014). Computer-aided discovery of aromatic l-alpha-amino acids as agonists of the orphan G protein-coupled receptor GPR139. J. Chem. Inf. Model 54, 1553–1557. doi: 10.1021/ci500197a
Jenkins, T. A., Nguyen, J. C., Polglaze, K. E., and Bertrand, P. P. (2016). Influence of tryptophan and serotonin on mood and cognition with a possible role of the gut-brain axis. Nutrients 8:E56. doi: 10.3390/nu8010056
Kononoff, J., Kallupi, M., Kimbrough, A., Conlisk, D., de Guglielmo, G., and George, O. (2018). Systemic and intra-habenular activation of the orphan g protein-coupled receptor GPR139 decreases compulsive-like alcohol drinking and hyperalgesia in alcohol-dependent rats. eNeuro 5, ENEURO.0153–ENEURO.1118. doi: 10.1523/ENEURO.0153-18.2018
Liu, C., Bonaventure, P., Lee, G., Nepomuceno, D., Kuei, C., Wu, J., et al. (2015). GPR139, an orphan receptor highly enriched in the habenula and septum, is activated by the essential amino acids l-tryptophan and l-phenylalanine. Mol. Pharmacol. 88, 911–925. doi: 10.1124/mol.115.100412
Liu, X.-Y., Chu, X.-P., Mao, L.-M., Wang, M., Lan, H.-X., Li, M.-H., et al. (2006). Modulation of D2R-NR2B interactions in response to cocaine. Neuron 52, 897–909. doi: 10.1016/j.neuron.2006.10.011
Malenka, R., Nestler, E., and Hyman, S. (2009). “Chapter 10: Neural andNeuroendocrine Control of the Internal Milieu,” in Molecular Neuropharmacology: A Foundation for Clinical Neuroscience, eds A. Sydor, and R. Y. Brown (New York, NY: McGraw-Hill Medica).
Mansour, A., Meador-Woodruff, J., Bunzow, J., Civelli, O., Akil, H., and Watson, S. (1990). Localization of dopamine D2 receptor mRNA and D1 and D2 receptor binding in the rat brain and pituitary: an in situ hybridization-receptor autoradiographic analysis. J. Neurosci. 10, 2587–2600. doi: 10.1523/JNEUROSCI.10-08-02587.1990
Matsuo, A., Matsumoto, S., Nagano, M., Masumoto, K. H., Takasaki, J., Matsumoto, M., et al. (2005). Molecular cloning and characterization of a novel Gq-coupled orphan receptor GPRg1 exclusively expressed in the central nervous system. Biochem. Biophys. Res. Commun. 331, 363–369. doi: 10.1016/j.bbrc.2005.03.174
Missale, C., Nash, S. R., Robinson, S. W., Jaber, M., and Caron, M. G. (1998). Dopamine receptors: from structure to function. Physiol. Rev. 78, 189–225. doi: 10.1152/physrev.1998.78.1.189
Mortazavi, A., Williams, B. A., McCue, K., Schaeffer, L., and Wold, B. (2008). Mapping and quantifying mammalian transcriptomes by RNA-Seq. Nat. Methods 5, 621–628. doi: 10.1038/nmeth.1226
Nepomuceno, D., Kuei, C., Dvorak, C., Lovenberg, T., Liu, C., and Bonaventure, P. (2018). Re-evaluation of adrenocorticotropic hormone and melanocyte stimulating hormone activation of GPR139 in vitro. Front. Pharmacol. 9:157. doi: 10.3389/fphar.2018.00157
Pinton, L., Borroto-Escuela, D. O., Narváez, M., Oflijan, J., Agnati, L. F., and Fuxe, K. (2015). Evidence for the existence of dopamine D2R and Sigma 1 allosteric receptor-receptor interaction in the rat brain: role in brain plasticity and cocaine action. SpringerPlus 4:37. doi: 10.1186/2193-1801-4-S1-P37
Shi, F., Shen, J. K., Chen, D., Fog, K., Thirstrup, K., Hentzer, M., et al. (2011). Discovery and SAR of a series of agonists at orphan g protein-coupled receptor 139. ACS Med. Chem. Lett. 2, 303–306. doi: 10.1021/ml100293q
Susens, U., Hermans-Borgmeyer, I., Urny, J., and Schaller, H. C. (2006). Characterisation and differential expression of two very closely related G-protein-coupled receptors, GPR139 and GPR142, in mouse tissue and during mouse development. Neuropharmacology 50, 512–520. doi: 10.1016/j.neuropharm.2005.11.003
ten Hoedt, A. E., de Sonneville, L. M., Francois, B., ter Horst, N. M., Janssen, M. C., Rubio-Gozalbo, M. E., et al. (2011). High phenylalanine levels directly affect mood and sustained attention in adults with phenylketonuria: a randomised, double-blind, placebo-controlled, crossover trial. J. Inherit. Metab. Dis. 34, 165–171. doi: 10.1007/s10545-010-9253-9259
Toker, L., Amar, S., Bersudsky, Y., Benjamin, J., and Klein, E. (2010). The biology of tryptophan depletion and mood disorders. Isr. J. Psychiatry Relat. Sci. 47, 46–55.
Trifilieff, P., Rives, M.-L., Urizar, E., Piskorowski, R. A., Vishwasrao, H. D., Castrillon, J., et al. (2011). Detection of antigen interactions ex vivo by proximity ligation assay: endogenous dopamine D2-adenosine A2A receptor complexes in the striatum. Biotechniques 51, 111–118. doi: 10.2144/000113719
Wang, F., Flanagan, J., Su, N., Wang, L. C., Bui, S., Nielson, A., et al. (2012). RNAscope: a novel in situ RNA analysis platform for formalin-fixed, paraffin-embedded tissues. J. Mol. Diagn. 14, 22–29. doi: 10.1016/j.jmoldx.2011.08.002
Wang, J., Zhu, L. Y., Liu, Q., Hentzer, M., Smith, G. P., and Wang, M. W. (2015). High-throughput screening of antagonists for the orphan G-protein coupled receptor GPR139. Acta Pharmacol. Sin. 36, 874–878. doi: 10.1038/aps.2015.12
Werry, T. D., Wilkinson, G. F., and Willars, G. B. (2003). Mechanisms of cross-talk between G-protein-coupled receptors resulting in enhanced release of intracellular Ca2+. Biochem. J. 374, 281–296. doi: 10.1042/bj20030312
Keywords: GPR139, dopamine D2 receptor, co-expression, in situ hybridization, in vitro interaction, calcium mobilization
Citation: Wang L, Lee G, Kuei C, Yao X, Harrington A, Bonaventure P, Lovenberg TW and Liu C (2019) GPR139 and Dopamine D2 Receptor Co-express in the Same Cells of the Brain and May Functionally Interact. Front. Neurosci. 13:281. doi: 10.3389/fnins.2019.00281
Received: 30 November 2018; Accepted: 11 March 2019;
Published: 26 March 2019.
Edited by:
George Panagis, University of Crete, GreeceReviewed by:
George Liapakis, University of Crete, GreeceGunnar P.H. Dietz, University of Göttingen, Germany
Copyright © 2019 Wang, Lee, Kuei, Yao, Harrington, Bonaventure, Lovenberg and Liu. This is an open-access article distributed under the terms of the Creative Commons Attribution License (CC BY). The use, distribution or reproduction in other forums is permitted, provided the original author(s) and the copyright owner(s) are credited and that the original publication in this journal is cited, in accordance with accepted academic practice. No use, distribution or reproduction is permitted which does not comply with these terms.
*Correspondence: Changlu Liu, Q0xpdTlAaXRzLmpuai5jb20=
†These authors have contributed equally to this work