- Department of Neurobiology and Anatomy, Drexel University College of Medicine, Philadelphia, PA, United States
Breathing constantly adapts to environmental, metabolic or behavioral changes by responding to different sensory information, including afferent feedback from muscles. Importantly, not just respiratory muscle feedback influences respiratory activity. Afferent sensory information from rhythmically moving limbs has also been shown to play an essential role in the breathing. The present review will discuss the neuronal mechanisms of respiratory modulation by activation of peripheral muscles that usually occurs during locomotion or exercise. An understanding of these mechanisms and finding the most effective approaches to regulate respiratory motor output by stimulation of limb muscles could be extremely beneficial for people with respiratory dysfunctions. Specific attention in the present review is given to the muscle stimulation to treat respiratory deficits following cervical spinal cord injury.
Limb Muscle Afferents and Respiration
Experimental studies of respiratory modulation via peripheral muscles can be divided into two overlapping groups: (i) investigation of functional interactions between locomotor and respiratory neural circuits and (ii) studying the effect of muscle afferent activation on cardio-respiratory system during exercise.
Functional Interaction Between Locomotion and Respiration
Many experimental studies have demonstrated that locomotion is functionally linked to respiration by coupling between central pattern generators (CPGs) for stepping and breathing (Boggs, 2002). This coupling has often been attributed to rhythmic activation of sensory inputs from the limbs during muscle contraction, affecting the activity of the respiratory CPG (Morin and Viala, 2002; Potts et al., 2005; Giraudin et al., 2008, 2012). It is important to note, however, that there are also central mechanisms of interaction between locomotor and respiratory systems. These include feedforward control of locomotion and respiration by cerebral cortex and subcortical structures (Krogh and Lindhard, 1913; Eldridge et al., 1981b, 1985; DiMarco et al., 1983; Waldrop et al., 1986), direct inputs to the respiratory CPG from the mesencephalic locomotor region (MLR) (Gariepy et al., 2012), interactions between spinal locomotor and brainstem respiratory CPGs (Persegol et al., 1988; Le Gal et al., 2014; Yazawa, 2014), or spinal locomotor and spinal respiratory circuits (Viala et al., 1979; Viala, 1986). The potential interactions between locomotor and respiratory systems are outlined in Figure 1. While the central mechanisms are significant, the focus of this review will be on studies of respiratory modulation by peripheral mechanisms (limb muscle afferents), with consideration to how these can be therapeutically targeted.
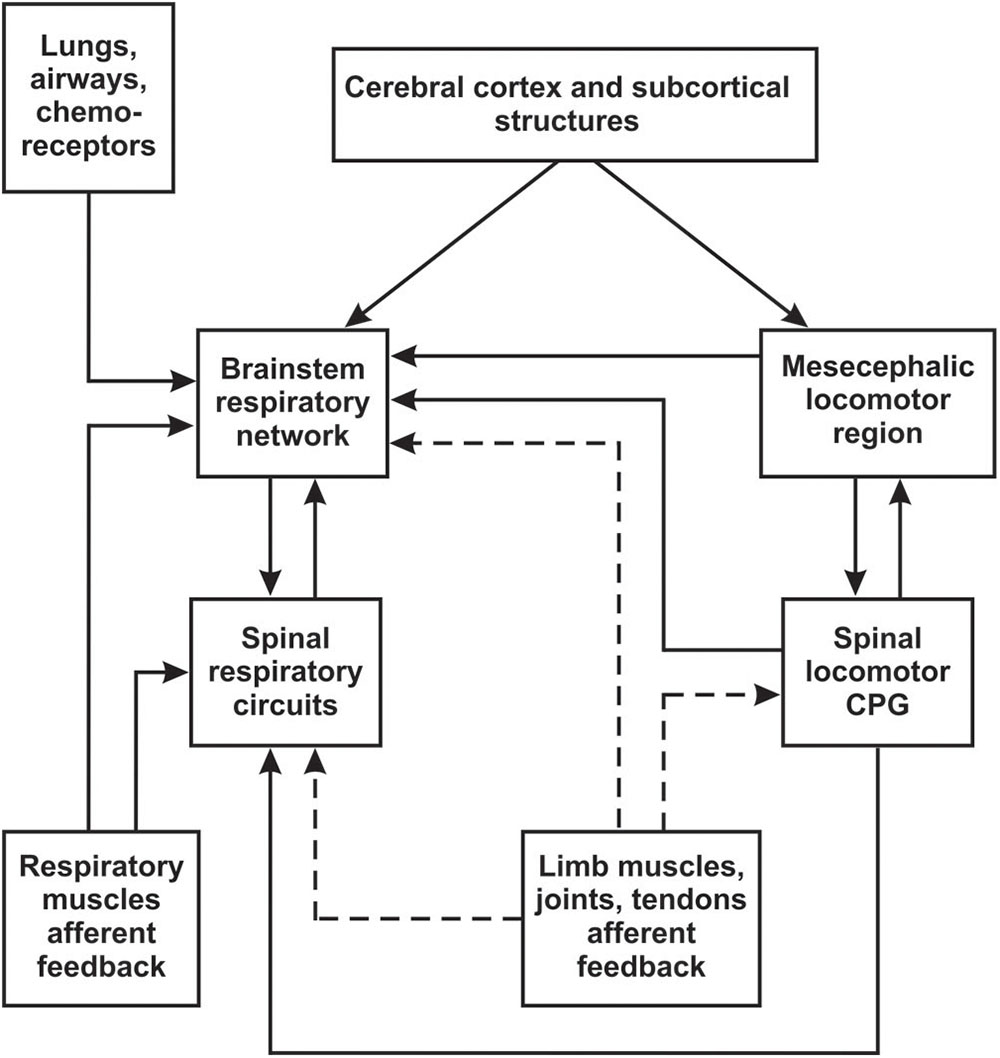
Figure 1. Schematic diagram of possible interactions between locomotor and respiratory systems. Brainstem respiratory network includes medulla and pons (see Section “Overview of Respiratory and Locomotor Networks”). Afferent inputs from limb muscles, joints and tendons are outlined by dashed line.
In the experimental setup to study afferent inputs from limb muscles to respiratory system, activation of muscle afferents can be achieved by nerve, muscle or dorsal roots electrical stimulation as well as by passive or active limb movements or stretch (Koizumi et al., 1961; Palisses et al., 1988; Persegol et al., 1988; Potts et al., 2005; Giraudin et al., 2008; Le Gal et al., 2016).
During experimental stimulations of limb muscle afferents, two major effects on respiration can be observed: entrainment of breathing and hyperpnoea. During entrained breathing the respiratory rhythm resets and follows the frequency of stimulus applied to muscle afferents (Iscoe and Polosa, 1976; Iscoe, 1981; Morin and Viala, 2002; Potts et al., 2005), whereas hyperpnoea is characterized by increased frequency of breathing and tidal volume (Koizumi et al., 1961; Tibes, 1977; Persegol et al., 1993). Entrainment of breathing is more related to locomotor-respiratory coupling, while hyperpnoea to exercise in general. Both supraspinal and spinal mechanisms could be involved in these respiratory responses.
Overview of Respiratory and Locomotor Networks
The supraspinal respiratory network is organized into several brainstem compartments that extend from the lower medulla to the pons (for review see Rybak and Smith, 2009; Smith et al., 2009). Respiratory rhythm originates within the ventral respiratory column (VRC) in the medulla. The VRC includes the Bötzinger Complex (BötC), pre-Bötzinger Complex (pre-BötC), rostral (rVRG) and caudal (cVRG) subregions of the ventral respiratory group (VRG). The pre-BötC contains mostly inspiratory neurons and is considered a primary source of rhythmic inspiratory activity. The BötC, containing mostly post-inspiratory and expiratory neurons, together with the adjacent pre-BötC represent a kernel of the respiratory CPG that is critically important for the generation of the basic respiratory activity. The pre-BötC and BötC receive multiple drives from other medullary and pontine regions, such as the parabrachial and Kolliker-Fuse nuclei (PBN/KF), comprising the pontine respiratory group (PRG), the retrotrapezoid nucleus/parafacial respiratory group (RTN/pFRG), raphe nucleus, nucleus tractus solitarius (NTS) in medulla and others. The NTS and adjacent reticular neurons form the dorsal respiratory group (DRG). The emerging view is that the VRC, DRG, and PRG collectively constitute the brainstem respiratory network. The activity of brainstem respiratory network is modulated by feedback from neurons in other regions of the brain, brainstem, spinal cord and periphery. The NTS processes peripheral chemo- and lung stretch receptors afferent feedback and provides increase in breathing (chemoreflex) or decrease in amplitude/duration of inspiration (Hering–Breuer reflex), respectively. RTN/pFRG is important for central chemoreception and formation of active expiratory pattern. The PBN/KF nuclei regulate the inspiratory-expiratory phase transition and mediate somatic afferent feedback from peripheral (limb) muscles to the VRC compartments of the respiratory CPG.
The primary spinal respiratory circuits comprise phrenic, intercostal, and abdominal interneurons and motoneurons, innervating the associated primary respiratory muscles (see Lane, 2011). These circuits receive inputs from the brainstem respiratory center and segmental peripheral afferent inputs (Sumi, 1963; Smith et al., 2009). In particular, the pre-BötC projects to the bulbospinal inspiratory neurons in the rVRG relaying the inspiratory drive to phrenic (PhMNs) and intercostal motoneurons innervating the diaphragm and external intercostal muscles. Bulbospinal expiratory neurons in the cVRG receive convergent inputs from the BötC and RTN/pFRG and shape expiratory drives to spinal thoracic and lumbar expiratory motoneurons. In addition, the spinal respiratory circuits receive the afferent feedback from respiratory muscles, and, as was shown experimentally, afferent inputs from limb muscles, either directly or via spinal locomotor circuits (Morin and Viala, 2002; Giraudin et al., 2012; Le Gal et al., 2014).
The spinal locomotor circuits represent a bilateral neural network located in the cervical and lumbar spinal segments, which are capable of generating the basic locomotor rhythm and shaping locomotor activity (Falgairolle et al., 2006; Kiehn, 2006; Guertin, 2012). This network includes the rhythm generating neurons, spinal interneurons and motoneurons, controlling activity of limb muscles. The spinal locomotor circuits receive sensory feedback from limb muscles afferents, inputs from the brainstem MLR and from higher brain structures. The activity of the left and right cervical and lumbar CPGs is coordinated by commissural and long propriospinal interneurons. In addition to these interneuronal populations, spinal projection neurons innervate supraspinal regions, relaying sensory afferent input from limb muscles.
Limb Muscle Afferent Inputs to the Supraspinal Respiratory Network
Involvement of the supraspinal networks in locomotor-respiratory interaction suggests that sensory feedback from limb muscles can reset, entrain or facilitate the brainstem respiratory CPG (Morin and Viala, 2002; Potts et al., 2005; Giraudin et al., 2012). While supraspinal mechanisms of exercise hyperpnoea will be discussed in more details below, here we will focus on the entrainment of breathing. Repetitive stimulation of muscle afferents can produce 1:1 entrainment of the respiratory frequency (Kawahara et al., 1988; Palisses et al., 1988; Morin and Viala, 2002; Potts et al., 2005). Animal experiments have shown that the entrainment of respiratory rhythm is phase-dependent and restricted to stimulation during the expiratory phase (Kawahara et al., 1988; Potts et al., 2005). Studies in brainstem-spinal cord preparation of newborn rats in vitro have demonstrated that afferent inputs from the hindlimb muscles can be transmitted to spinal locomotor CPG and then to the brainstem (Le Gal et al., 2014) or directly to the brainstem respiratory network bypassing the lumbar locomotor CPG (Morin and Viala, 2002; Giraudin et al., 2012). This suggests that activation of the spinal locomotor CPG is not required for respiratory rhythm entrainment during rhythmic stimulation of limb afferents (Morin and Viala, 2002). Experiments with reverse blockade of the PBN (Potts et al., 2005) and brainstem transections (Giraudin et al., 2012) showed an inability to entrain breathing, implicating the pivotal role of PBN neurons in the locomotor-respiratory coupling during afferent stimulation of limb muscles when spinal locomotor CPG is not activated. Anatomical studies in rats confirmed the existence of afferent inputs to the PBN from the dorsal spinal cord and the spinal trigeminal nucleus (pars caudalis), mainly from lamina I (Cechetto et al., 1985; Bernard et al., 1995; Craig, 1995). Immunohistochemical and electrophysiological studies have shown that most PBN neurons receiving these inputs respond to nociceptive stimulation (Standaert et al., 1986; Bernard et al., 1994; Hermanson and Blomqvist, 1996). It was suggested that the PBN receives information from somatic nociceptive receptors and adjusts cardio-respiratory responses to their activation (Gauriau and Bernard, 2002). Thus, it is unclear if observed respiratory rhythm entrainment via the PBN is a specific result of stimulation of nociceptive afferents or it can be generally implied to activation of non-nociceptive muscle afferents. Experiments to selectively block different afferent subtypes are needed to confirm that nociception is not involved in respiratory entrainment. This raises an important question: what type of skeletal muscle afferents should be activated to trigger respiratory responses?
Muscle Afferent Fiber Types
Muscle afferents are represented by axons of sensory neurons that reside within the spinal dorsal root ganglion. These pseudo-unipolar neurons transfer sensory information from muscles to the spinal cord via spinal nerves. According to Lloyd’s classification (Lloyd, 1943), there are four major types of muscle afferent fibers [I (Ia and Ib), II, III, and IV], differing by their physiological characteristics as well as by the sensory inputs activating them. Specifically, type Ia fibers, located in the muscle spindles, respond to activation of rapidly adapting stretch receptors and signal the fast change in muscle length and velocity. Type Ib fibers respond to activation of Golgi tendon organ and signal the change in muscle force tension. The type I (Ia and Ib) fibers are thickly myelinated (12–20 μm), have a large diameter and fast conductive velocity (70–120 m/s in cats and dogs) (Lloyd, 1943; Whitwam, 1976; McCord and Kaufman, 2010). Type II fibers respond to activation of slow non-adapting stretch receptors and provide information about muscle length and limb position. They are also thickly myelinated (6–12 μm), but have slower conductive velocity than type I (30–70 m/s). Type III and IV free ending fibers provide sensory feedback related to different physiological conditions of a muscle: mechanical contractive and metabolic (byproducts of muscular work, e.g., potassium, prostoglandins, lactic and arachidonic acids, ATP et al.) conditions, including noxious sensation (McCord and Kaufman, 2010; Mense, 2010; Kaufman and Forster, 2011; Forster et al., 2012; Pedersen, 2013). Type III fibers are represented by thinly myelinated, small diameter (1–6 μm) and lower conductive velocity axons (2.5–30.0 m/s) and type IV – by unmyelinated, smallest diameter (less than 1 μm), lowest conductive velocity fibers (<2.5 m/s). Sensory responses to mechanical stimulation are provided mostly by type III fibers, whereas metabolic changes during muscular work are sensed by type IV fibers (Kaufman et al., 1983, 1984; Hayes and Kaufman, 2001). It has been demonstrated that the type III/IV non-nociceptive muscle fibers can be activated by both static and dynamic muscle contractions and respond to all types of movements including exercise (Kaufman et al., 1983, 1984).
Experimental studies suggest that entrainment of respiratory rhythm and exercise hyperpnoea could be triggered by different afferents. Studies in the newborn isolated brainstem-spinal cord preparation (Morin and Viala, 2002; Giraudin et al., 2008, 2012) have demonstrated that type I/II afferent fibers are responsible for respiratory rhythm entrainment. Here, we should keep in mind that results in newborn preparations in vitro might be different from adult motor circuits in vivo (Smith and Feldman, 1987). However, the major role of type I-II afferents in entrainment was supported by some in vivo investigations (Koizumi et al., 1961), but not all (Iscoe and Polosa, 1976; Kawahara et al., 1988). In addition, both experimental and clinical studies have revealed that stimulation of primary afferents (including type I-II) can entrain the spinal locomotor CPG (Kiehn et al., 1992; Guertin et al., 1995; Dietz et al., 2002; Lev-Tov et al., 2010) and, thus, may elicit ventilatory changes via direct interaction between the spinal locomotor and brainstem respiratory CPGs (Persegol et al., 1988; Le Gal et al., 2014; Yazawa, 2014), or spinal locomotor and spinal respiratory circuits (Viala et al., 1979; Viala, 1986) (see Figure 1). In contrast, however, most investigators believe that exercise hyperpnoea is triggered exclusively by stimulation of type III/IV fibers (Mateika and Duffin, 1995; Amann et al., 2010; Kaufman, 2010; Amann, 2012). Experiments with selective blocking and deactivating of the different afferent types have shown that activation of type III/IV non-nociceptive fibers significantly contribute to changes in ventilation during limb muscle activity (McCloskey and Mitchell, 1972b; Tibes, 1977; Amann et al., 2010).
Limb Muscle Afferent Inputs to the Spinal Respiratory Network
Though neural mechanisms responsible for generation of the respiratory rhythm and pattern have been extensively studied for many years, most of these studies were focused on respiratory circuits in the brainstem (Feldman et al., 2003; Smith et al., 2009, 2013). Less attention has been given to spinal neural circuits involved in modulation of the respiratory activity. Accordingly, their organization and functional contribution are poorly understood. Nevertheless, the importance of spinal interneurons for regulation of respiration in the intact and injured spinal cord has been demonstrated (Sumi, 1963; Palisses et al., 1989; Bellingham and Lipski, 1990; Lane et al., 2008, 2009; Lois et al., 2009; Sandhu et al., 2009; Marchenko et al., 2015; Zholudeva et al., 2017). Specifically, a subset of spinal interneurons (distributed mostly in V-VII and X layers of Rexed) that modulate the activity of phrenic motoneurons (PhMNs), controlling the contraction of the diaphragm – the main muscle of respiration, has been identified (Yates et al., 1999; Lane et al., 2008, 2009; Lois et al., 2009). Overall, respiratory spinal interneurons have been found to be involved in many functions including intra-/intersegmental and left-right coordination of respiratory motor outputs, integration of different motor activities, and promotion of respiratory plasticity following cervical spinal cord injury (Sumi, 1963; Palisses et al., 1989; Bellingham and Lipski, 1990; Lane et al., 2008, 2009; Sandhu et al., 2009; Darlot et al., 2012; Zholudeva et al., 2017). The diversity of spinal interneuron phenotypes and their known contributions to breathing are highlighted in Zholudeva et al. (2018).
However, the spinal mechanisms of interactions between locomotion and respiration are not well studied. It is believed that the spinal mechanisms can modulate ventilation via specific connections between afferent inputs from limb muscles and respiratory neural circuits at the spinal cord level (Koizumi et al., 1961; Eldridge et al., 1981a; Morin and Viala, 2002; Le Gal et al., 2016). The experimental results allow to suggest the existence of “shared” spinal interneurons that receive afferent feedback from limb muscles and are involved in both locomotor and respiratory spinal circuits (see Figure 2). Indeed, experiments in the isolated brainstem-spinal cord of newborn rats have shown excitatory connections between the lumbar locomotor CPG, which receives afferent feedback from muscles, and expiratory motor- and interneurons located at the lumbar and thoracic levels in the spinal cord (Le Gal et al., 2016). The activity of these neurons can be rhythmically modulated by the locomotor activity induced either pharmacologically or by afferent stimulation (Le Gal et al., 2016). These connections are exclusively intraspinal and, as authors suggest, may assist in the coordination of locomotion and respiration by facilitation of expiratory efforts.
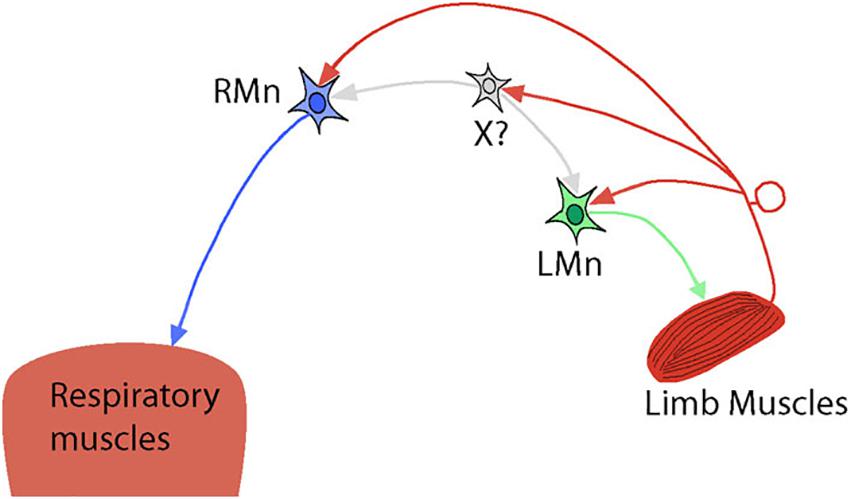
Figure 2. Simplified hypothetical diagram representing intraspinal connections between locomotor and respiratory spinal circuits. RMn – respiratory motoneuron. LMn – limb motoneuron. X? – shared interneuron that drives/modulates both RMn and LMn and receives afferent feedback from limb muscles. Possible direct inputs to RMn from limb muscle afferents are also shown.
Another study performed on the same brainstem-spinal cord preparation with intracellular recordings of PhMNs has demonstrated that ascending afferent fibers from hindlimb muscles give collaterals to these motoneurons (Morin and Viala, 2002). Activation of hindlimb afferents results in a monosynaptic short-latency activation of PhMNs, followed by their disynaptic inhibition via GABA-ergic spinal interneurons and polysynaptic excitation of a supraspinal origin (through the spinal-bulbar-spinal loop). To explain the disynaptic inhibitory effect on PhMN activity, the authors propose that inputs from limb afferents are necessary to “prepare the PhMN population to receive excitatory polysynaptic commands via the medullary respiratory network” (see Figure 7 in Morin and Viala, 2002). The role of the monosynaptic activation of PhMNs by afferent stimulation was not discussed in this study and could be addressed in future research by pharmacological disinhibition of PhMNs in a spinal preparation and rhythmic stimulation of limb muscles to see if this can elicit phrenic nerve activity and diaphragm contractions. However, one experimental work in decorticate and spinal rabbits was done in this direction and has shown that following activation of spinal cord with DOPA, periodic hindlimb movements were able to elicit large bursts in phrenic nerves, supporting the idea about direct excitatory inputs from hindlimb muscles afferents to PhMNs (Palisses et al., 1988) and their inhibition in intact spinal cord.
In vivo experiments in spinal cats (without supraspinal control) have demonstrated inhibitory effect of hindlimb afferent stimulation on phrenic (Eldridge et al., 1981a) and intercostal motoneuron activity (Koizumi et al., 1961). In the study by Eldridge et al. (1981a), the authors used intraspinal intercostal-to-phrenic reflex to evoke phrenic bursts in C1 transected cats by electrical stimulation of intercostal nerves or rhythmic manual compression/tapping of the lower thorax described by Decima et al. (1969). Continuous physical stimulation of the calf muscle or electrical stimulation of the tibial nerve for 60 sec produced inhibition of the evoked phrenic activity. The authors did not evaluate the type of afferents activated by this stimulation but suggested that small myelinated and unmyelinated fibers were involved. Interestingly, the extent of inhibition declined over the course of stimulation. Moreover, a brief increase in phrenic activity (rebound) was observed after stimulation. The decline in inhibition and rebound were attributed to accommodation and increase in neuronal excitability post-inhibition, which thought to be the common membrane properties of spinal motoneurons (Coombs et al., 1959; Sears, 1964). It should be noted that all studies considered above were focused on hindlimb afferent stimulation. Interactions between forelimb afferents and PhMNs have not been demonstrated yet.
Overall, these experiments confirm the presence of intraspinal connections between locomotor and respiratory motor systems. Interactions between supraspinal and spinal mechanisms of respiratory modulation by muscle afferent stimulation are not clear, however, and more experimental work is required.
Cardio-Respiratory Control During Exercise
Mechanisms of cardio-respiratory responses during exercise have been extensively studied for over a hundred years (see review by Mitchell, 2013). Exercise increases the oxygen demands, production of carbon dioxide and different metabolites in working muscles. Elevated ventilation, heart rate, and blood pressure are required to match metabolic changes in the organism and are correlated with the intensity of exercise (D’Angelo and Torelli, 1971; Casey et al., 1987; Iellamo et al., 1999). Many studies refer to these changes as the “exercise pressure reflex” (Mitchell et al., 1983; Kaufman and Hayes, 2002). Several mechanisms have been suggested to explain the cardio-respiratory responses during exercise: (i) feedforward central commands (Eldridge et al., 1981b, 1985; Waldrop et al., 1986); (ii) peripheral neural regulation through type III and IV muscle afferents (McCloskey and Mitchell, 1972a) and (iii) central integration of inputs from the peripheral and/or central chemoreceptors (see review Gariepy et al., 2010). Experiments in human subjects have confirmed the significant modulatory role of type III/IV muscle afferents in cardio-respiratory responses during exercise by using the anesthetic (lidocaine, fentanyl) injections into the lumbar epidural space to reduce sensation from those afferents and observing the attenuation of cardio-respiratory responses during exercise or electrical stimulation of hindlimb (Strange et al., 1993; Kjaer et al., 1994; Amann et al., 2010; Dempsey et al., 2014). However, the debate on the role of type III/IV muscle afferents in cardio-respiratory responses during exercise continues (Poon and Song, 2015).
Three phases of ventilatory response to mild-to-moderate exercise have been distinguished in a healthy subject: (1) a rapid increase in total ventilation at the exercise onset; (2) a transient phase with a further continuous increase of ventilation; and (3) the final steady phase characterized by plateaued ventilation (Whipp et al., 1982; Turner, 1991; Bruce, 2017). The rapid increase in ventilation in phase 1 cannot be explained by changes in metabolic level. It was proposed that at the exercise onset, neuronal inputs from motor cortex (central feedforward mechanism) and/or stimulation of muscle/joint afferents lead to a fast increase of the respiratory CPG output resulting in hyperpnoea. As exercise continues, the humoral mechanism was suggested to perform further tuning of respiration (Dejours, 1964; Griffiths et al., 1986; Whipp, 1994). At exercise offset, the removal of central and afferent signals leads to abrupt fall in hyperventilation, followed by slow decay in ventilation until humoral changes are diminished. Control of respiratory parameters by the type III/IV afferents is likely to be involved in all phases of ventilatory response to exercise (Kaufman et al., 1983, 1984; Amann, 2012; Duffin, 2014).
Anatomical and electrophysiological studies have shown that high threshold (III/IV type) muscle afferents have direct and non-direct projections (via spinal laminae I-V neurons) to the lower brainstem including the NTS (Kalia et al., 1981; Potts, 2001, 2006; Kawabe et al., 2007), reticular nuclei (Ciriello and Calaresu, 1977; Menetrey et al., 1983; Iwamoto et al., 1984) and rostroventrolateral medulla (RVLM) (Kawabe et al., 2007). It has been proposed that GABA-ergic neurons of the caudal NTS activated by somatic afferents may play a major role in inhibition of the baroreflex, which provides negative control on blood pressure and heart rate, resulting in increase in blood pressure and heart rate during exercise (Potts, 2001, 2006). We hypothesize that similar mechanism may inhibit inputs from lung stretch receptors, decreasing the strength of the Hering-Breuer reflex (by inhibiting of GABA-ergic pump cells projecting from the NTS to the respiratory CPG) (see Figure 3). On the one hand, direct activation of RVLM presympathetic neurons by muscle afferents during exercise can elicit an increase in blood pressure and heart rate. Suppression of the baro- and Hering-Breuer reflexes during exercise may lead to hypertension and increased tidal volume. On the other hand, it was demonstrated that activation of vagal afferents might inhibit the exercise effect in conscious rats (DiCarlo et al., 1994). Sensory feedback from baroreceptors and lung stretch receptors are mutually inhibitory with somatic afferents at the NTS, so the outcome of sensory stimuli will depend on the balance of all afferent inputs. Moreover, the recent work of Silva et al. (2018) has shown that simultaneous stimulation of muscle and peripheral chemosensory afferents during passive knee movement and hypoxia resulted in hyperadditive hyperventilation in healthy volunteers. These data may reflect the importance of integration between neurogenic (muscle afferents) and metabolic (peripheral chemoreceptors) inputs in the NTS and/or other structures of ponto-medullary respiratory network to provide an appropriate respiratory response to physical exercise.
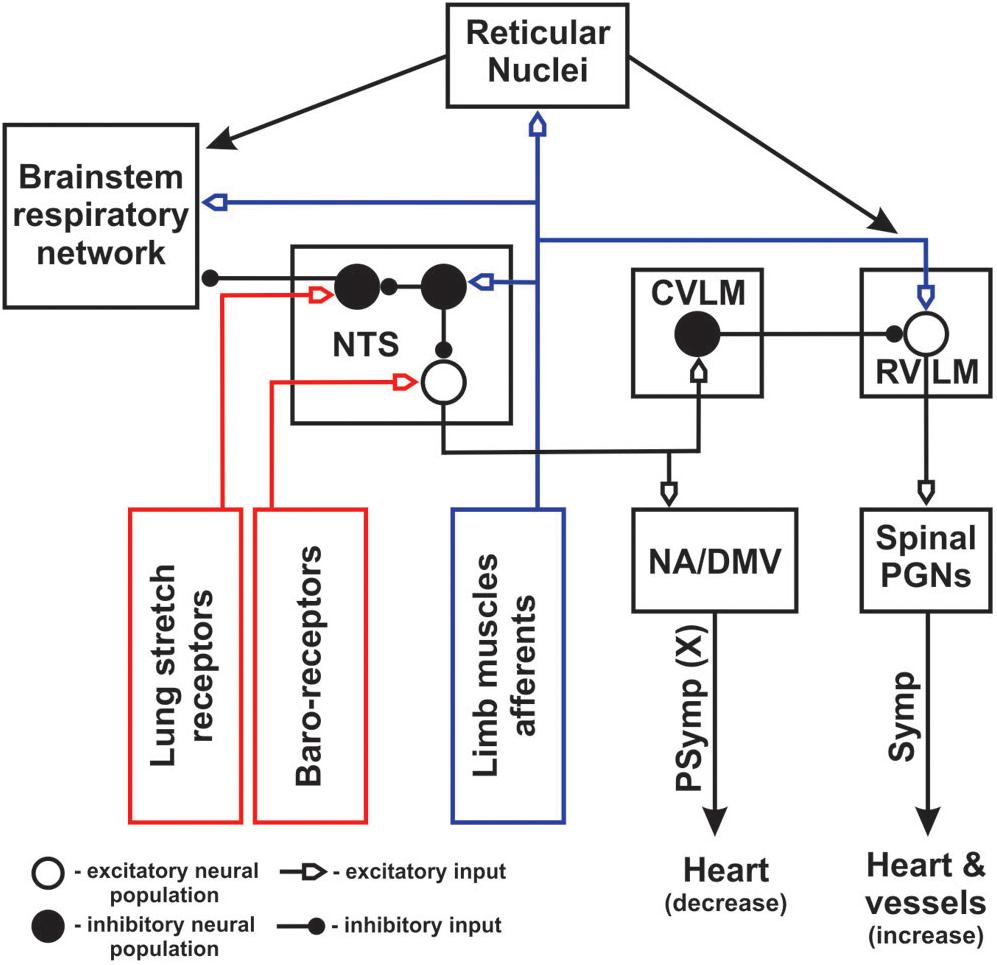
Figure 3. Schematic diagram of cardio-respiratory interactions during exercise. Blue color represents afferent feedback from limb muscles. Red color shows afferent feedback from baro- and lung stretch receptors. NA – nucleus ambiguous; DMV – dorsal motor nucleus of vagus; PGNs – preganglionic neurons; Symp – sympathetic outputs; PSymp – parasympathetic output; X – vagus nerve. Open and filled big circles represent excitatory and inhibitory neuron populations, respectively. Filled small circles show inhibitory inputs, open small arrows indicate excitatory inputs. NTS was moved out from the block presenting brainstem respiratory network to show its inputs and outputs in greater details. Peripheral/central chemoreception was not included for simplicity.
In addition, polysynaptic ascending spinal pathways activated by somatic muscle afferents include the spino-reticular tracts. Spino-reticular neurons project to reticular structures in the vicinity of the ventrolateral medulla (Iwamoto et al., 1984, 1989; Iwamoto and Kaufman, 1987; Bauer et al., 1990) and can modulate the respiratory CPG (Jones et al., 2016), bulbospinal inspiratory/expiratory (Dobbins and Feldman, 1994) and presympathetic RVLM neurons (Ciriello and Calaresu, 1977; Iwamoto et al., 1989; Bauer et al., 1990; Babic and Ciriello, 2004; Guyenet and Stornetta, 2004) regulating cardiovascular function. Activation of these projections during exercise can directly change the frequency/amplitude of the breathing and increase blood pressure/heart rate. The conceptual schematic of the central interactions between somatic afferents and cardio-respiratory networks during exercise is shown in Figure 3.
Effects of Different Muscles Stimulation
Stimulation of different muscles (hindlimb vs. forelimb or flexors vs. extensors) may have different effects on respiration during locomotion or exercise. Moreover, prior studies indicate that even activation of the same muscle can have inconsistent effects between animal species (Boggs, 2002). This is likely due to differences in the way these muscles are used in different species, for instance, the role of fore- and hindlimb muscles in bipedal and quadrupedal animals. In bipeds, hindlimbs carry 100% of static body weight whereas, in quadrupeds, forelimbs usually support about 55–60% of body weight during standing. Some quadrupedal animals can use their forelimbs to carry and manipulate objects, some do not. Worth noting, however, is that in most animals used in electrophysiological experiments (mice, rats, cats, rabbits, dogs), the hindlimb muscles have larger muscle mass than forelimbs. This fact makes hindlimb muscles more popular for experimental and clinical research (Koizumi et al., 1961; Iscoe and Polosa, 1976; Tibes, 1977; Viala, 1986; Viala et al., 1987; Palisses et al., 1988; Persegol et al., 1993). However, some investigations have used forelimb muscle stimulation as well (Potts et al., 2005). Nevertheless, electrical stimulation of dorsal roots at either cervical or lumbar levels in the isolated newborn rat brainstem-spinal cord preparation revealed a similar effect on respiration (Giraudin et al., 2008). The published results on flexor vs. extensor muscle afferent stimulation are contradictory. Specifically, some studies show a greater increase in respiratory output with hindlimb flexor stimulation (Persegol et al., 1993; Giraudin et al., 2012), while others report the opposite (Viala et al., 1987; Palisses et al., 1988), or even equal effects regardless of muscle stimulated (Koizumi et al., 1961). These contradictory results can be explained by using various experimental models and paradigms of muscle stimulation. Further studies are needed for clarification of the effect of different muscle stimulation on modulation of cardio-respiratory output.
Human studies suggest that the magnitude of cardiovascular and respiratory response during exercise might depend on the mass of a muscle involved (Iellamo et al., 1999). Therefore, in general, the effect on the ventilatory parameters is expected to be higher during lower limb vs. upper limb exercise. This could be explained by activation of a higher number of afferent fibers, more metabolites production and increased blood flow capacity in muscles with larger mass. However, many clinical studies have shown the same effect of upper vs. lower limb activation during exercises of the same intensity (Kang et al., 1997; Faria and Faria, 1998). One possible explanation of this inconsistency could be spatial distribution and activation of different muscle fiber types that were associated not only with different physiological but also metabolic properties (slow-twitch (type 1) fibers – resistant to fatigue vs. fast twitch (type 2) fibers that fatigue much faster, but produce more force). It was shown that the type 2 fibers (glycolytic) are more metabolically active than type 1 (oxidative) (Saltin and Gollnick, 1983; Casey et al., 1996). Therefore, stimulation of muscles with a higher proportion of type 2 fibers will likely produce a bigger increase in ventilation and cardiovascular response than stimulation of muscles with a higher proportion of type 1 fibers (see for review Fisher and White, 2004). In general, lower limb muscles in human have a higher proportion of slow-twitch fibers (type 1) compared to upper limbs (Johnson et al., 1973; Edgerton et al., 1975; Dahmane et al., 2005), perhaps, because of their role in bipedal locomotion. Thus, the effect on respiratory responses during upper limb vs. lower limbs exercising may be comparable given the opposing differences in the number of type 1–2 fibers and muscle mass, which could compensate each other.
Another consideration is that, even within lower limb muscles, there is a specific pattern of distribution of different type fibers. Deep extensor lower limb muscles predominantly contain type 1 fibers when more peripheral muscles have a higher proportion of type 2 fibers. This is correlated with the recruitment of muscles during locomotion: deep muscles are activated during postural standing and walking, while peripheral muscles are activated through running and jumping (Armstrong and Laughlin, 1985). Therefore, certain type of exercises can affect different type of muscles in the same limb and elicit different cardio-respiratory responses. In addition, the distribution of type 1 and 2 fibers in and between muscles also depends on type of training and genetics (Simoneau and Bouchard, 1995; Andersen and Aagaard, 2010; Wilson et al., 2012).
Overall, the published experimental results suggest that targeting the muscles with a larger mass and containing a higher proportion of type 2 fibers can be more effective to elicit cardio-respiratory responses. Worth noting again, however, is that type 2 fibers are more susceptible to fatigue (Saltin and Gollnick, 1983).
Perspectives of Limb Muscles Stimulation as a Therapy to Treat Respiratory Dysfunction Following Spinal Cord Injury
Therapies based on repetitive limb movements and stimulation are becoming more recognized in treating respiratory dysfunctions in people with different medical conditions. For example, upper limb exercising was incorporated in pulmonary rehabilitation for patients with chronic obstructive pulmonary disease (COPD) and demonstrated a reduction in dyspnea episodes (Gigliotti et al., 2005; Ries et al., 2007; Costa et al., 2011; Rekha et al., 2016). In individuals with multiple sclerosis, exercise programs for upper extremities have been shown to significantly improve respiratory function (Mutluay et al., 2007). Strength exercises for upper limbs were reported to be beneficial for respiration in children with cerebral palsy (Shin and Kim, 2017) and stroke patients (Kim and Jang, 2016). Although these two studies were focused on increasing of muscular strength of upper limbs/trunk and normalizing vertebral alignment, the afferent activation of working muscles could also play a significant role in the improvement of respiratory function. As could be predicted from the discussion so far, lower extremity activation can also promote respiratory recovery. Daily electrical stimulation of leg muscles in critically ill patients admitted to the intensive care unit reduced the time for assisted ventilation and patients were weaned earlier that non-stimulated group (Abu-Khaber et al., 2013; Leite et al., 2018). Daily vibratory stimulation over hand and foot proprioceptors has been demonstrated to reduce the number of apneic and hypoxic episodes in premature infants (<34 weeks), supporting again the idea that limb muscle stimulation can facilitate and improve breathing (Kesavan et al., 2016).
Clinical studies also suggest that stimulation of limb muscles may be employed for respiratory rehabilitation in people with spinal cord injuries (SCI). Repetitive activation of the impaired respiratory system by peripheral muscle stimulation might retrain spared neural networks (activity-based therapy) and improve their function post-SCI (Hormigo et al., 2017). This activation can be achieved via supraspinal (in the case of a non-complete SCI) as well as via spinal mechanisms discussed above. Support for this hypothesis can be found in a number of studies that have demonstrated a beneficial effect of lower and upper limb afferent activation on respiratory function in SCI patients. Rhythmic stimulation of lower extremities by assisted treadmill locomotion was able to elicit metabolic responses and increase ventilatory parameters in individuals with chronic complete and non-complete cervical SCI (Nash et al., 2004; Carvalho et al., 2005). Regular treadmill training with body weight support (Terson et al., 2013; Tiftik et al., 2015) or accompanied by functional electrical stimulation (FES) (de Carvalho et al., 2006) significantly increased respiratory motor function in patients with cervical and thoracic SCI. The exercises involving upper extremities (Silva et al., 1998; Le Foll-de et al., 2005) have also been shown to improve ventilatory function in individuals with thoracic SCI.
However, cervical level SCIs are the most devastating and often impair the majority of motor functions below the injury, including paralysis/paresis of both, lower and upper limbs. Treadmill rehabilitation in tetraplegic individual represents a huge logistical and economic burden. Therefore, using FES of upper extremities in people with cervical SCI could be a more suitable alternative. This type of rehabilitation is non-invasive (cutaneous electrode placement), requires less personnel and can be applied in a home environment. Arm-cranking exercise assisted by FES (Hunt et al., 2003; Coupaud et al., 2008) is one of the examples of upper extremities stimulation to enhance respiration in individuals with cervical SCI. Even so, the above studies were not designed to improve respiratory function, a significant increase in oxygen uptake by the end of the training sessions was determined in some participants. Also, it should be noted specifically that combined strategies of FES and exercise (bicycle, arm-cranking, arm-cycling, or their hybrids) show greater potentials for recovery in SCI individuals by producing more effect on cardio-respiratory function (Hettinga and Andrews, 2008; Hasnan et al., 2013). While the clinical studies conducted so far have unfolded exciting results, there is still a great deal that needs to be investigated both clinically and pre-clinically. Reverse translation of these documented studies to animal models of SCI will likely reveal how to optimize these promising strategies for improving ventilation in injured people.
Conclusion
Overall, despite a significant number of experimental and clinical studies, the detailed mechanisms of respiratory control by stimulation of peripheral muscles in the intact and, especially, injured spinal cord are still unclear and require more thorough investigations. An understanding of these mechanisms will help to develop improved stimulation paradigms and devices for limb FES in combination with different exercises, targeting the most effective choice of muscles to enhance respiratory plasticity and recovery in individuals with cervical SCI and other respiratory dysfunctions. As neural engineering advances the field of neural interfacing, we can expect to see even greater improvements in these described results, with better strategies for improving breathing.
Author Contributions
NS, VM, and TB made a substantial contribution to the drafting, figure making and discussion of this work and approved it for publication.
Funding
This work was supported by Conquer Paralysis Now (TB), the Edward Jekkal Muscular Dystrophy Association Fellowship (TB) and the Spinal Cord Research Center at Drexel University, College of Medicine (NIH, P01 NS 055976).
Conflict of Interest Statement
The authors declare that the research was conducted in the absence of any commercial or financial relationships that could be construed as a potential conflict of interest.
Acknowledgments
The authors would like to thank Dr. Michael A. Lane for helpful discussions and comments in preparation of the manuscript.
References
Abu-Khaber, H. A., Abouelela, A. M. Z., and Abdelkarim, E. M. (2013). Effect of electrical muscle stimulation on prevention of ICU acquired muscle weakness and facilitating weaning from mechanical ventilation. Alexandria J. Med. 49, 309–315. doi: 10.1016/j.ajme.2013.03.011
Amann, M. (2012). Significance of Group III and IV muscle afferents for the endurance exercising human. Clin. Exp. Pharmacol. Physiol. 39, 831–835. doi: 10.1111/j.1440-1681.2012.05681.x
Amann, M., Blain, G. M., Proctor, L. T., Sebranek, J. J., Pegelow, D. F., and Dempsey, J. A. (2010). Group III and IV muscle afferents contribute to ventilatory and cardiovascular response to rhythmic exercise in humans. J. Appl. Physiol. (1985) 109, 966–976. doi: 10.1152/japplphysiol.00462.2010
Andersen, J. L., and Aagaard, P. (2010). Effects of strength training on muscle fiber types and size; consequences for athletes training for high-intensity sport. Scand. J. Med. Sci. Sports 20(Suppl. 2), 32–38. doi: 10.1111/j.1600-0838.2010.01196.x
Armstrong, R. B., and Laughlin, M. H. (1985). Metabolic indicators of fibre recruitment in mammalian muscles during locomotion. J. Exp. Biol. 115, 201–213.
Babic, T., and Ciriello, J. (2004). Medullary and spinal cord projections from cardiovascular responsive sites in the rostral ventromedial medulla. J. Comp. Neurol. 469, 391–412. doi: 10.1002/cne.11024
Bauer, R. M., Iwamoto, G. A., and Waldrop, T. G. (1990). Discharge patterns of ventrolateral medullary neurons during muscular contraction. Am. J. Physiol. 259(3 Pt 2), R606–R611. doi: 10.1152/ajpregu.1990.259.3.R606
Bellingham, M. C., and Lipski, J. (1990). Respiratory interneurons in the C5 segment of the spinal cord of the cat. Brain Res. 533, 141–146. doi: 10.1016/0006-8993(90)91807-S
Bernard, J. F., Dallel, R., Raboisson, P., Villanueva, L., and Le Bars, D. (1995). Organization of the efferent projections from the spinal cervical enlargement to the parabrachial area and periaqueductal gray: a PHA-L study in the rat. J. Comp. Neurol. 353, 480–505. doi: 10.1002/cne.903530403
Bernard, J. F., Huang, G. F., and Besson, J. M. (1994). The parabrachial area: electrophysiological evidence for an involvement in visceral nociceptive processes. J. Neurophysiol. 71, 1646–1660. doi: 10.1152/jn.1994.71.5.1646
Boggs, D. F. (2002). Interactions between locomotion and ventilation in tetrapods. Compar. Biochem. Physiol. A Mol. Integr. Physiol. 133, 269–288. doi: 10.1016/S1095-6433(02)00160-5
Bruce, R. M. (2017). The control of ventilation during exercise: a lesson in critical thinking. Adv. Physiol. Educ. 41, 539–547. doi: 10.1152/advan.00086.2017
Carvalho, D. C., de Cassia Zanchetta, M., Sereni, J. M., and Cliquet, A. (2005). Metabolic and cardiorespiratory responses of tetraplegic subjects during treadmill walking using neuromuscular electrical stimulation and partial body weight support. Spinal Cord. 43, 400–405. doi: 10.1038/sj.sc.3101730
Casey, A., Constantin-Teodosiu, D., Howell, S., Hultman, E., and Greenhaff, P. L. (1996). Metabolic response of type I and II muscle fibers during repeated bouts of maximal exercise in humans. Am. J. Physiol. 271(1 Pt 1), E38–E43. doi: 10.1152/ajpendo.1996.271.1.E38
Casey, K., Duffin, J., Kelsey, C. J., and McAvoy, G. V. (1987). The effect of treadmill speed on ventilation at the start of exercise in man. J. Physiol. 391, 13–24. doi: 10.1113/jphysiol.1987.sp016722
Cechetto, D. F., Standaert, D. G., and Saper, C. B. (1985). Spinal and trigeminal dorsal horn projections to the parabrachial nucleus in the rat. J. Compar. Neurol. 240, 153–160. doi: 10.1002/cne.902400205
Ciriello, J., and Calaresu, F. R. (1977). Lateral reticular nucleus: a site of somatic and cardiovascular integration in the cat. Am. J. Physiol. 233, R100–R109. doi: 10.1152/ajpregu.1977.233.3.R100
Coombs, J. S., Curtis, D. R., and Eccles, J. C. (1959). The electrical constants of the motoneurone membrane. J. Physiol. 145, 505–528. doi: 10.1113/jphysiol.1959.sp006158
Costa, D., Cancelliero, K. M., Ike, D., Laranjeira, T. L., Pantoni, C. B., and Borghi-Silva, A. (2011). Strategy for respiratory exercise pattern associated with upper limb movements in COPD patients. Clinics (São Paulo) 66, 299–305. doi: 10.1590/S1807-59322011000200020
Coupaud, S., Gollee, H., Hunt, K. J., Fraser, M. H., Allan, D. B., and McLean, A. N. (2008). Arm-cranking exercise assisted by functional electrical stimulation in C6 tetraplegia: a pilot study. Technol. Health Care 16, 415–427.
Craig, A. D. (1995). Distribution of brainstem projections from spinal lamina I neurons in the cat and the monkey. J. Compar. Neurol. 361, 225–248. doi: 10.1002/cne.903610204
Dahmane, R., Djordjevic, S., Simunic, B., and Valencic, V. (2005). Spatial fiber type distribution in normal human muscle histochemical and tensiomyographical evaluation. J. Biomech. 38, 2451–2459. doi: 10.1016/j.jbiomech.2004.10.020
D’Angelo, E., and Torelli, G. (1971). Neural stimuli increasing respiration during different types of exercise. J. Appl. Physiol. 30, 116–121. doi: 10.1152/jappl.1971.30.1.116
Darlot, F., Cayetanot, F., Gauthier, P., Matarazzo, V., and Kastner, A. (2012). Extensive respiratory plasticity after cervical spinal cord injury in rats: axonal sprouting and rerouting of ventrolateral bulbospinal pathways. Exp. Neurol. 236, 88–102. doi: 10.1016/j.expneurol.2012.04.004
de Carvalho, D. C., Martins, C. L., Cardoso, S. D., and Cliquet, A. (2006). Improvement of metabolic and cardiorespiratory responses through treadmill gait training with neuromuscular electrical stimulation in quadriplegic subjects. Artif. Organs 30, 56–63. doi: 10.1111/j.1525-1594.2006.00180.x
Decima, E. E., von Euler, C., and Thoden, U. (1969). Intercostal-to-phrenic reflexes in the spinal cat. Acta Physiol. Scand. 75, 568–579. doi: 10.1111/j.1748-1716.1969.tb04412.x
Dejours, P. (1964). Control of Respiration on Muscular Exercise. Handbook of Physiology, Respiration. Washington DC: American Physiological Society.
Dempsey, J. A., Blain, G. M., and Amann, M. (2014). Are type III-IV muscle afferents required for a normal steady-state exercise hyperpnoea in humans? J. Physiol. 592, 463–474. doi: 10.1113/jphysiol.2013.261925
DiCarlo, S. E., Collins, H. L., and Chen, C. Y. (1994). Vagal afferents reflexly inhibit exercise in conscious rats. Med. Sci. Sports Exerc. 26, 459–462. doi: 10.1249/00005768-199404000-00010
Dietz, V., Muller, R., and Colombo, G. (2002). Locomotor activity in spinal man: significance of afferent input from joint and load receptors. Brain 125(Pt 12), 2626–2634. doi: 10.1093/brain/awf273
DiMarco, A. F., Romaniuk, J. R., Von Euler, C., and Yamamoto, Y. (1983). Immediate changes in ventilation and respiratory pattern associated with onset and cessation of locomotion in the cat. J. Physiol. 343, 1–16. doi: 10.1113/jphysiol.1983.sp014878
Dobbins, E. G., and Feldman, J. L. (1994). Brainstem network controlling descending drive to phrenic motoneurons in rat. J. Compar. Neurol. 347, 64–86. doi: 10.1002/cne.903470106
Duffin, J. (2014). The fast exercise drive to breathe. J. Physiol. 592, 445–451. doi: 10.1113/jphysiol.2013.258897
Edgerton, V. R., Smith, J. L., and Simpson, D. R. (1975). Muscle fibre type populations of human leg muscles. Histochem. J. 7, 259–266. doi: 10.1007/BF01003594
Eldridge, F. L., Gill-Kumar, P., Millhorn, D. E., and Waldrop, T. G. (1981a). Spinal inhibition of phrenic motoneurones by stimulation of afferents from peripheral muscles. J. Physiol. 311, 67–79. doi: 10.1113/jphysiol.1981.sp013573
Eldridge, F. L., Millhorn, D. E., and Waldrop, T. G. (1981b). Exercise hyperpnea and locomotion: parallel activation from the hypothalamus. Science 211, 844–846. doi: 10.1126/science.7466362
Eldridge, F. L., Millhorn, D. E., Kiley, J. P., and Waldrop, T. G. (1985). Stimulation by central command of locomotion, respiration and circulation during exercise. Respir. Physiol. 59, 313–337. doi: 10.1016/0034-5687(85)90136-7
Falgairolle, M., de Seze, M., Juvin, L., Morin, D., and Cazalets, J. R. (2006). Coordinated network functioning in the spinal cord: an evolutionary perspective. J. Physiol. Paris 100, 304–316. doi: 10.1016/j.jphysparis.2007.05.003
Faria, E. W., and Faria, I. E. (1998). Cardiorespiratory responses to exercises of equal relative intensity distributed between the upper and lower body. J. Sports Sci. 16, 309–315. doi: 10.1080/02640419808559359
Feldman, J. L., Mitchell, G. S., and Nattie, E. E. (2003). Breathing: rhythmicity, plasticity, chemosensitivity. Annu. Rev. Neurosci. 26, 239–266. doi: 10.1146/annurev.neuro.26.041002.131103
Fisher, J. P., and White, M. J. (2004). Muscle afferent contributions to the cardiovascular response to isometric exercise. Exp. Physiol. 89, 639–646. doi: 10.1113/expphysiol.2004.028639
Forster, H. V., Haouzi, P., and Dempsey, J. A. (2012). Control of breathing during exercise. Compar. Physiol. 2, 743–777. doi: 10.1002/cphy.c100045
Gariepy, J. F., Missaghi, K., Chevallier, S., Chartre, S., Robert, M., Auclair, F., et al. (2012). Specific neural substrate linking respiration to locomotion. Proc. Natl. Acad. Sci. U.S.A. 109, E84–E92. doi: 10.1073/pnas.1113002109
Gariepy, J. F., Missaghi, K., and Dubuc, R. (2010). The interactions between locomotion and respiration. Prog. Brain Res. 187, 173–188. doi: 10.1016/B978-0-444-53613-6.00012-5
Gauriau, C., and Bernard, J. F. (2002). Pain pathways and parabrachial circuits in the rat. Exp. Physiol. 87, 251–258. doi: 10.1113/eph8702357
Gigliotti, F., Coli, C., Bianchi, R., Grazzini, M., Stendardi, L., Castellani, C., et al. (2005). Arm exercise and hyperinflation in patients with COPD: effect of arm training. Chest 128, 1225–1232. doi: 10.1378/chest.128.3.1225
Giraudin, A., Cabirol-Pol, M. J., Simmers, J., and Morin, D. (2008). Intercostal and abdominal respiratory motoneurons in the neonatal rat spinal cord: spatiotemporal organization and responses to limb afferent stimulation. J. Neurophysiol. 99, 2626–2640. doi: 10.1152/jn.01298.2007
Giraudin, A., Le Bon-Jego, M., Cabirol, M. J., Simmers, J., and Morin, D. (2012). Spinal and pontine relay pathways mediating respiratory rhythm entrainment by limb proprioceptive inputs in the neonatal rat. J. Neurosci. 32, 11841–11853. doi: 10.1523/JNEUROSCI.0360-12.2012
Griffiths, T. L., Henson, L. C., and Whipp, B. J. (1986). Influence of inspired oxygen concentration on the dynamics of the exercise hyperpnoea in man. J. Physiol. 380, 387–403. doi: 10.1113/jphysiol.1986.sp016292
Guertin, P., Angel, M. J., Perreault, M. C., and McCrea, D. A. (1995). Ankle extensor group I afferents excite extensors throughout the hindlimb during fictive locomotion in the cat. J. Physiol. 487, 197–209. doi: 10.1113/jphysiol.1995.sp020871
Guertin, P. A. (2012). Central pattern generator for locomotion: anatomical, physiological, and pathophysiological considerations. Front. Neurol. 3:183. doi: 10.3389/fneur.2012.00183
Guyenet, P. G., and Stornetta, R. L. (2004). “The presympathetic cells of the rostral ventrolateral medulla (RVLM): anatomy, physiology and role in the control of circulation,” in Neural Mechanisms of Cardiovascular Regulation, eds N. J. Dun, B. H. Machado, and P. M. Pilowsky (Boston, MA: Springer), 187–218.
Hasnan, N., Ektas, N., Tanhoffer, A. I., Tanhoffer, R., Fornusek, C., Middleton, J. W., et al. (2013). Exercise responses during functional electrical stimulation cycling in individuals with spinal cord injury. Med. Sci. Sports Exerc. 45, 1131–1138. doi: 10.1249/MSS.0b013e3182805d5a
Hayes, S. G., and Kaufman, M. P. (2001). Gadolinium attenuates exercise pressor reflex in cats. Am. J. Physiol. Heart Circ. Physiol. 280, H2153–H2161. doi: 10.1152/ajpheart.2001.280.5.H2153
Hermanson, O., and Blomqvist, A. (1996). Subnuclear localization of FOS-like immunoreactivity in the rat parabrachial nucleus after nociceptive stimulation. J. Comp. Neurol. 368, 45–56.
Hettinga, D. M., and Andrews, B. J. (2008). Oxygen consumption during functional electrical stimulation-assisted exercise in persons with spinal cord injury: implications for fitness and health. Sports Med. 38, 825–838. doi: 10.2165/00007256-200838100-00003
Hormigo, K. M., Zholudeva, L. V., Spruance, V. M., Marchenko, V., Cote, M. P., Vinit, S., et al. (2017). Enhancing neural activity to drive respiratory plasticity following cervical spinal cord injury. Exp. Neurol. 287(Pt 2), 276–287. doi: 10.1016/j.expneurol.2016.08.018
Hunt, K. J., McLean, A. N., Coupaud, S., and Gollee, H. (2003). Upper-limb exercise in tetraplegia using functional electrical stimulation. ACNR 3, 24–25.
Iellamo, F., Massaro, M., Raimondi, G., Peruzzi, G., and Legramante, J. M. (1999). Role of muscular factors in cardiorespiratory responses to static exercise: contribution of reflex mechanisms. J. Appl. Physiol. (1985) 86, 174–180. doi: 10.1152/jappl.1999.86.1.174
Iscoe, S. (1981). Respiratory and stepping frequencies in conscious exercising cats. J. Appl. Physiol. Respir. Environ. Exerc. Physiol. 51, 835–839. doi: 10.1152/jappl.1981.51.4.835
Iscoe, S., and Polosa, C. (1976). Synchronization of respiratory frequency by somatic afferent stimulation. J. Appl. Physiol. 40, 138–148. doi: 10.1152/jappl.1976.40.2.138
Iwamoto, G. A., and Kaufman, M. P. (1987). Caudal ventrolateral medullary cells responsive to muscular contraction. J. Appl. Physiol. (1985) 62, 149–157. doi: 10.1152/jappl.1987.62.1.149
Iwamoto, G. A., Parnavelas, J. G., Kaufman, M. P., Botterman, B. R., and Mitchell, J. H. (1984). Activation of caudal brainstem cell groups during the exercise pressor reflex in the cat as elucidated by 2-[14C]deoxyglucose. Brain Res. 304, 178–182. doi: 10.1016/0006-8993(84)90878-3
Iwamoto, G. A., Waldrop, T. G., Bauer, R. M., and Mitchell, J. H. (1989). Pressor responses to muscular contraction in the cat: contributions by caudal and rostral ventrolateral medulla. Prog. Brain Res. 81, 253–263. doi: 10.1016/S0079-6123(08)62015-4
Johnson, M. A., Polgar, J., Weightman, D., and Appleton, D. (1973). Data on the distribution of fibre types in thirty-six human muscles: an autopsy study. J. Neurol. Sci. 18, 111–129. doi: 10.1016/0022-510X(73)90023-3
Jones S. E., Stanic, D., and Dutschmann, M. (2016). Dorsal and ventral aspects of the most caudal medullary reticular formation have differential roles in modulation and formation of the respiratory motor pattern in rat. Brain Struct. Funct. 221, 4353–4368. doi: 10.1007/s00429-015-1165-x
Kalia, M., Mei, S. S., and Kao, F. F. (1981). Central projections from ergoreceptors (C fibers) in muscle involved in cardiopulmonary responses to static exercise. Circ. Res. 48(6 Pt 2), I48–I62.
Kang, J., Robertson, R. J., Goss, F. L., Dasilva, S. G., Suminski, R. R., Utter, A. C., et al. (1997). Metabolic efficiency during arm and leg exercise at the same relative intensities. Med. Sci. Sports Exerc. 29, 377–382. doi: 10.1097/00005768-199703000-00013
Kaufman, M. P. (2010). Control of breathing during dynamic exercise by thin fiber muscle afferents. J. Appl. Physiol. (1985) 109, 947–948. doi: 10.1152/japplphysiol.00892.2010
Kaufman, M. P., and Forster, H. V. (2011). “Reflexes controlling circulatory, ventilatory and airway responses to exercise,” in Handbook of Physiology, Exercise: Regulation and Integration of Multiple Systems, eds L. B. Rowell and J. T. Shepherd (Rockville, MD: American Physiological Society), 381–447.
Kaufman, M. P., and Hayes, S. G. (2002). The exercise pressor reflex. Clin. Auton Res. 12, 429–439. doi: 10.1007/s10286-002-0059-1
Kaufman, M. P., Longhurst, J. C., Rybicki, K. J., Wallach, J. H., and Mitchell, J. H. (1983). Effects of static muscular contraction on impulse activity of groups III and IV afferents in cats. J. Appl. Physiol. Respir. Environ. Exerc. Physiol. 55(1 Pt 1), 105–112. doi: 10.1152/jappl.1983.55.1.105
Kaufman, M. P., Waldrop, T. G., Rybicki, K. J., Ordway, G. A., and Mitchell, J. H. (1984). Effects of static and rhythmic twitch contractions on the discharge of group III and IV muscle afferents. Cardiovasc. Res. 18, 663–668. doi: 10.1093/cvr/18.11.663
Kawabe, T., Kawabe, K., and Sapru, H. N. (2007). Cardiovascular responses to somatosensory stimulation and their modulation by baroreflex mechanisms. Clin. Exp. Hypertens. 29, 403–418. doi: 10.1080/10641960701578402
Kawahara, K., Kumagai, S., Nakazono, Y., and Miyamoto, Y. (1988). Analysis of entrainment of respiratory rhythm by somatic afferent stimulation in cats using phase response curves. Biol. Cybernet. 58, 235–242. doi: 10.1007/BF00364129
Kesavan, K., Frank, P., Cordero, D. M., Benharash, P., and Harper, R. M. (2016). Neuromodulation of limb proprioceptive afferents decreases apnea of prematurity and accompanying intermittent hypoxia and bradycardia. PLoS One 11:e0157349. doi: 10.1371/journal.pone.0157349
Kiehn, O. (2006). Locomotor circuits in the mammalian spinal cord. Annu. Rev. Neurosci. 29, 279–306. doi: 10.1146/annurev.neuro.29.051605.112910
Kiehn, O., Iizuka, M., and Kudo, N. (1992). Resetting from low threshold afferents of N-methyl-D-aspartate-induced locomotor rhythm in the isolated spinal cord-hindlimb preparation from newborn rats. Neurosci. Lett. 148, 43–46. doi: 10.1016/0304-3940(92)90800-M
Kim, D. H., and Jang, S. H. (2016). Effects of an upper-limb exercise program for improving muscular strength and range of movement on respiratory function of stroke patients. J. Phys. Ther. Sci. 28, 2785–2788. doi: 10.1589/jpts.28.2785
Kjaer, M., Perko, G., Secher, N. H., Boushel, R., Beyer, N., Pollack, S., et al. (1994). Cardiovascular and ventilatory responses to electrically induced cycling with complete epidural anaesthesia in humans. Acta Physiol. Scand. 151, 199–207. doi: 10.1111/j.1748-1716.1994.tb09738.x
Koizumi, K., Ushiyama, J., and Brooks, C. M. (1961). Muscle afferents and activity of respiratory neurons. Am. J. Physiol. 200, 679–684. doi: 10.1152/ajplegacy.1961.200.4.679
Krogh, A., and Lindhard, J. (1913). The regulation of respiration and circulation during the initial stages of muscular work. J. Physiol. 47, 112–136. doi: 10.1113/jphysiol.1913.sp001616
Lane, M. A. (2011). Spinal respiratory motoneurons and interneurons. Respir. Physiol. Neurobiol. 179, 3–13. doi: 10.1016/j.resp.2011.07.004
Lane, M. A., Lee, K. Z., Fuller, D. D., and Reier, P. J. (2009). Spinal circuitry and respiratory recovery following spinal cord injury. Respir. Physiol. Neurobiol. 169, 123–132. doi: 10.1016/j.resp.2009.08.007
Lane, M. A., White, T. E., Coutts, M. A., Jones, A. L., Sandhu, M. S., Bloom, D. C., et al. (2008). Cervical prephrenic interneurons in the normal and lesioned spinal cord of the adult rat. J. Compar. Neurol. 511, 692–709. doi: 10.1002/cne.21864
Le Foll-de, Moro, D., Tordi, N., Lonsdorfer, E., and Lonsdorfer, J. (2005). Ventilation efficiency and pulmonary function after a wheelchair interval-training program in subjects with recent spinal cord injury. Arch. Phys. Med. Rehabil. 86, 1582–1586. doi: 10.1016/j.apmr.2005.03.018
Le Gal, J. P., Juvin, L., Cardoit, L., and Morin, D. (2016). Bimodal respiratory-locomotor neurons in the neonatal rat spinal cord. J. Neurosci. 36, 926–937. doi: 10.1523/JNEUROSCI.1825-15.2016
Le Gal, J. P., Juvin, L., Cardoit, L., Thoby-Brisson, M., and Morin, D. (2014). Remote control of respiratory neural network by spinal locomotor generators. PLoS One 9:e89670. doi: 10.1371/journal.pone.0089670
Leite, M. A., Osaku, E. F., Albert, J., Costa, C., Garcia, A. M., Czapiesvski, F. D. N., et al. (2018). Effects of neuromuscular electrical stimulation of the quadriceps and diaphragm in critically Ill patients: a pilot study. Crit. Care Res. Pract. 2018:4298583. doi: 10.1155/2018/4298583
Lev-Tov, A., Etlin, A., and Blivis, D. (2010). Sensory-induced activation of pattern generators in the absence of supraspinal control. Ann. N. Y. Acad. Sci. 1198, 54–62. doi: 10.1111/j.1749-6632.2009.05424.x
Lloyd, D. P. (1943). Neuron patterns controlling transmission of ipsilateral hind limb reflexes in cat. J. Neurophysiol. 6, 293–315. doi: 10.1152/jn.1943.6.4.293
Lois, J. H., Rice, C. D., and Yates, B. J. (2009). Neural circuits controlling diaphragm function in the cat revealed by transneuronal tracing. J. Appl. Physiol. (1985) 106, 138–152. doi: 10.1152/japplphysiol.91125.2008
Marchenko, V., Ghali, M. G., and Rogers, R. F. (2015). The role of spinal GABAergic circuits in the control of phrenic nerve motor output. Am. J. Physiol. Regul. Integr. Compar. Physiol. 308, R916–R926. doi: 10.1152/ajpregu.00244.2014
Mateika, J. H., and Duffin, J. (1995). A review of the control of breathing during exercise. Eur. J. Appl. Physiol. Occupat. Physiol. 71, 1–27. doi: 10.1007/BF00511228
McCloskey, D. I., and Mitchell, J. H. (1972a). Reflex cardiovascular and respiratory responses originating in exercising muscle. J. Physiol. 224, 173–186. doi: 10.1113/jphysiol.1972.sp009887
McCloskey, D. I., and Mitchell, J. H. (1972b). The use of differential nerve blocking techniques to show that the cardiovascular and respiratory reflexes originating in exercising muscle are not mediated by large myelinated afferents. J. Physiol. 222, 50P–51P.
McCord, J. L., and Kaufman, M. P. (2010). “Reflex autonomic responses evoked by group III and IV muscle afferents,” in Translational Pain Research: From Mouse to Man, eds. L. Kruger & A.R. Light. (Boca Raton, FL: Taylor & Francis Group).
Menetrey, D., Roudier, F., and Besson, J. M. (1983). Spinal neurons reaching the lateral reticular nucleus as studied in the rat by retrograde transport of horseradish peroxidase. J. Compar. Neurol. 220, 439–452. doi: 10.1002/cne.902200406
Mense, S. (2010). “Functional anatomy of muscle: muscle, nociceptors and afferent fibers,” in Muscle Pain: Understanding the Mechanisms, eds S. Mense and R. D. Gerwin (Berlin: Springer), 17–48.
Mitchell, J. H. (2013). Neural circulatory control during exercise: early insights. Exp. Physiol. 98, 867–878. doi: 10.1113/expphysiol.2012.071001
Mitchell, J. H., Kaufman, M. P., and Iwamoto, G. A. (1983). The exercise pressor reflex: its cardiovascular effects, afferent mechanisms, and central pathways. Annu. Rev. Physiol. 45, 229–242. doi: 10.1146/annurev.ph.45.030183.001305
Morin, D., and Viala, D. (2002). Coordinations of locomotor and respiratory rhythms in vitro are critically dependent on hindlimb sensory inputs. J. Neurosci. 22, 4756–4765. doi: 10.1523/JNEUROSCI.22-11-04756.2002
Mutluay, F. K., Demir, R., Ozyilmaz, S., Caglar, A. T., Altintas, A., and Gurses, H. N. (2007). Breathing-enhanced upper extremity exercises for patients with multiple sclerosis. Clin. Rehabil. 21, 595–602. doi: 10.1177/0269215507075492
Nash, M. S., Jacobs, P. L., Johnson, B. M., and Field-Fote, E. (2004). Metabolic and cardiac responses to robotic-assisted locomotion in motor-complete tetraplegia: a case report. J. Spinal Cord Med. 27, 78–82. doi: 10.1080/10790268.2004.11753734
Palisses, R., Persegol, L., and Viala, D. (1989). Evidence for respiratory interneurones in the C3-C5 cervical spinal cord in the decorticate rabbit. Exp Brain Res. 78, 624–632. doi: 10.1007/BF00230250
Palisses, R., Persegol, L., Viala, D., and Viala, G. (1988). Reflex modulation of phrenic activity through hindlimb passive motion in decorticate and spinal rabbit preparation. Neuroscience 24, 719–728. doi: 10.1016/0306-4522(88)90364-8
Pedersen, B. K. (2013). Muscle as a secretory organ. Compar. Physiol. 3, 1337–1362. doi: 10.1002/cphy.c120033
Persegol, L., Jordan, M., Viala, D., and Fernandez, C. (1988). Evidence for central entrainment of the medullary respiratory pattern by the locomotor pattern in the rabbit. Exp. Brain Res. 71, 153–162. doi: 10.1007/BF00247530
Persegol, L., Palisses, R., and Viala, D. (1993). Characterization of hindlimb muscle afferents involved in ventilatory effects observed in decerebrate and spinal preparations. Exp. Brain Res. 92, 495–501. doi: 10.1007/BF00229038
Poon, C. S., and Song, G. (2015). Type III-IV muscle afferents are not required for steady-state exercise hyperpnea in healthy subjects and patients with COPD or heart failure. Respir. Physiol. Neurobiol. 216, 78–85. doi: 10.1016/j.resp.2015.04.007
Potts, J. T. (2001). Exercise and sensory integration. Role of the nucleus tractus solitarius. Ann. N. Y. Acad. Sci. 940, 221–236. doi: 10.1111/j.1749-6632.2001.tb03679.x
Potts, J. T. (2006). Inhibitory neurotransmission in the nucleus tractus solitarii: implications for baroreflex resetting during exercise. Exp. Physiol. 91, 59–72. doi: 10.1113/expphysiol.2005.032227
Potts, J. T., Rybak, I. A., and Paton, J. F. (2005). Respiratory rhythm entrainment by somatic afferent stimulation. J. Neurosci. 25, 1965–1978. doi: 10.1523/JNEUROSCI.3881-04.2005
Rekha, K., Vijayalakshmi, A., Doss, D. S. S., and Anandh, V. (2016). Effects of home based upper extremity exercise in chronic obstructive pulmonary disease. Int. J. Pharm. Clin. Res. 8, 1351–1355.
Ries, A. L., Bauldoff, G. S., Carlin, B. W., Casaburi, R., Emery, C. F., Mahler, D. A., et al. (2007). Pulmonary rehabilitation: joint ACCP/AACVPR evidence-based clinical practice guidelines. Chest 131(5 Suppl.), 4S–42S. doi: 10.1378/chest.06-2418
Rybak, I. A., and Smith, J. C. (2009). “Computational modeling of the respiratory network,” in Encyclopedia of Neuroscience, eds M. D. Binder, N. Hirokawa, U. Windhorst, and M. C. Hirsch (Berlin: Springer-Verlag), 824–832.
Saltin, B., and Gollnick, P. D. (1983). “Skeletal muscle adaptability: significance for metabolism and performance,” in Handbook of Physiology – Skeletal Muscle, eds L. D. Peachy, R. H. Adrian, and S. R. Geiger (Baltimore, MD: Williams & Wilkins), 555–631.
Sandhu, M. S., Dougherty, B. J., Lane, M. A., Bolser, D. C., Kirkwood, P. A., Reier, P. J., et al. (2009). Respiratory recovery following high cervical hemisection. Respir. Physiol. Neurobiol. 169, 94–101. doi: 10.1016/j.resp.2009.06.014
Sears, T. A. (1964). The slow potentials of thoracic respiratory motoneurones and their relation to breathing. J. Physiol. 175, 404–424. doi: 10.1113/jphysiol.1964.sp007524
Shin, S. O., and Kim, N. S. (2017). Upper extremity resistance exercise with elastic bands for respiratory function in children with cerebral palsy. J. Phys. Ther. Sci. 29, 2077–2080. doi: 10.1589/jpts.29.2077
Silva, A. C., Neder, J. A., Chiurciu, M. V., Pasqualin, D. C., da Silva, R. C., Fernandez, A. C., et al. (1998). Effect of aerobic training on ventilatory muscle endurance of spinal cord injured men. Spinal Cord 36, 240–245. doi: 10.1038/sj.sc.3100575
Silva, T. M., Aranda, L. C., Paula-Ribeiro, M., Oliveira, D. M., Medeiros, W. M., Vianna, L. C., et al. (2018). Hyperadditive ventilatory response arising from interaction between the carotid chemoreflex and the muscle mechanoreflex in healthy humans. J. Appl. Physiol. (1985) 125, 215–225. doi: 10.1152/japplphysiol.00009.2018
Simoneau, J. A., and Bouchard, C. (1995). Genetic determinism of fiber type proportion in human skeletal muscle. FASEB J. 9, 1091–1095. doi: 10.1096/fasebj.9.11.7649409
Smith, J. C., Abdala, A. P., Borgmann, A., Rybak, I. A., and Paton, J. F. (2013). Brainstem respiratory networks: building blocks and microcircuits. Trends Neurosci. 36, 152–162. doi: 10.1016/j.tins.2012.11.004
Smith, J. C., Abdala, A. P., Rybak, I. A., and Paton, J. F. (2009). Structural and functional architecture of respiratory networks in the mammalian brainstem. Philos. Trans. R. Soc. Lond. B Biol. Sci. 364, 2577–2587. doi: 10.1098/rstb.2009.0081
Smith, J. C., and Feldman, J. L. (1987). In vitro brainstem-spinal cord preparations for study of motor systems for mammalian respiration and locomotion. J. Neurosci. Methods 21, 321–333. doi: 10.1016/0165-0270(87)90126-9
Standaert, D. G., Watson, S. J., Houghten, R. A., and Saper, C. B. (1986). Opioid peptide immunoreactivity in spinal and trigeminal dorsal horn neurons projecting to the parabrachial nucleus in the rat. J. Neurosci. 6, 1220–1226. doi: 10.1523/JNEUROSCI.06-05-01220.1986
Strange, S., Secher, N. H., Pawelczyk, J. A., Karpakka, J., Christensen, N. J., Mitchell, J. H., et al. (1993). Neural control of cardiovascular responses and of ventilation during dynamic exercise in man. J. Physiol. 470, 693–704. doi: 10.1113/jphysiol.1993.sp019883
Sumi, T. (1963). Organization of spinal respiratory neurons. Ann. N. Y. Acad. Sci. 109, 561–570. doi: 10.1111/j.1749-6632.1963.tb13487.x
Terson, de Paleville, D., McKay, W., Aslan, S., Folz, R., Sayenko, D., et al. (2013). Locomotor step training with body weight support improves respiratory motor function in individuals with chronic spinal cord injury. Respir. Physiol. Neurobiol. 189, 491–497. doi: 10.1016/j.resp.2013.08.018
Tibes, U. (1977). Reflex inputs to the cardiovascular and respiratory centers from dynamically working canine muscles. Some evidence for involvement of group III or IV nerve fibers. Circ. Res. 41, 332–341. doi: 10.1161/01.RES.41.3.332
Tiftik, T., Gokkaya, N. K., Malas, F. U., Tunc, H., Yalcin, S., Ekiz, T., et al. (2015). Does locomotor training improve pulmonary function in patients with spinal cord injury? Spinal Cord 53, 467–470. doi: 10.1038/sc.2014.251
Turner, D. L. (1991). Cardiovascular and respiratory control mechanisms during exercise: an integrated view. J. Exp. Biol. 160, 309–340.
Viala, D. (1986). Evidence for direct reciprocal interactions between the central rhythm generators for spinal “respiratory” and locomotor activities in the rabbit. Exp. Brain Res. 63, 225–232. doi: 10.1007/BF00236841
Viala, D., Persegol, L., and Palisses, R. (1987). Relationship between phrenic and hindlimb extensor activities during fictive locomotion. Neurosci. Lett. 74, 49–52. doi: 10.1016/0304-3940(87)90049-8
Viala, D., Vidal, C., and Freton, E. (1979). Coordinated rhythmic bursting in respiratory and locomotor muscle nerves in the spinal rabbit. Neurosci. Lett. 11, 155–159. doi: 10.1016/0304-3940(79)90119-8
Waldrop, T. G., Mullins, D. C., and Millhorn, D. E. (1986). Control of respiration by the hypothalamus and by feedback from contracting muscles in cats. Respir. Physiol. 64, 317–328. doi: 10.1016/0034-5687(86)90125-8
Whipp, B. J. (1994). Peripheral chemoreceptor control of exercise hyperpnea in humans. Med. Sci. Sports Exerc. 26, 337–347. doi: 10.1249/00005768-199403000-00010
Whipp, B. J., Ward, S. A., Lamarra, N., Davis, J. A., and Wasserman, K. (1982). Parameters of ventilatory and gas exchange dynamics during exercise. J. Appl. Physiol. Respir. Environ. Exerc. Physiol. 52, 1506–1513. doi: 10.1152/jappl.1982.52.6.1506
Whitwam, J. G. (1976). Classification of peripheral nerve fibres. An historical perspective. Anaesthesia 31, 494–503. doi: 10.1111/j.1365-2044.1976.tb12354.x
Wilson, J. M., Loenneke, J. P., Jo, E., Wilson, G. J., Zourdos, M. C., and Kim, J. S. (2012). The effects of endurance, strength, and power training on muscle fiber type shifting. J. Strength Cond. Res. 26, 1724–1729. doi: 10.1519/JSC.0b013e318234eb6f
Yates, B. J., Smail, J. A., Stocker, S. D., and Card, J. P. (1999). Transneuronal tracing of neural pathways controlling activity of diaphragm motoneurons in the ferret. Neuroscience 90, 1501–1513. doi: 10.1016/S0306-4522(98)00554-5
Yazawa, I. (2014). Reciprocal functional interactions between the brainstem and the lower spinal cord. Front. Neurosci. 8:124. doi: 10.3389/fnins.2014.00124
Zholudeva, L. V., Karliner, J. S., Dougherty, K. J., and Lane, M. A. (2017). Anatomical recruitment of spinal V2a interneurons into phrenic motor circuitry after high cervical spinal cord injury. J. Neurotrauma 34, 3058–3065. doi: 10.1089/neu.2017.5045
Keywords: respiration, muscle stimulation, exercise, muscle afferents, spinal cord injury
Citation: Shevtsova NA, Marchenko V and Bezdudnaya T (2019) Modulation of Respiratory System by Limb Muscle Afferents in Intact and Injured Spinal Cord. Front. Neurosci. 13:289. doi: 10.3389/fnins.2019.00289
Received: 13 December 2018; Accepted: 11 March 2019;
Published: 26 March 2019.
Edited by:
Erwin Lemche, King’s College London, United KingdomReviewed by:
Daniel B. Zoccal, São Paulo State University, BrazilLinda F. Hayward, University of Florida, United States
Copyright © 2019 Shevtsova, Marchenko and Bezdudnaya. This is an open-access article distributed under the terms of the Creative Commons Attribution License (CC BY). The use, distribution or reproduction in other forums is permitted, provided the original author(s) and the copyright owner(s) are credited and that the original publication in this journal is cited, in accordance with accepted academic practice. No use, distribution or reproduction is permitted which does not comply with these terms.
*Correspondence: Tatiana Bezdudnaya, dGJlemR1ZG5AZ21haWwuY29t