- 1School of Mechanical Engineering, Pusan National University, Busan, South Korea
- 2Department of Rehabilitation Medicine, Pusan National University School of Medicine, Pusan National University Yangsan Hospital, Yangsan-si, South Korea
Background: Brain disorders are gradually becoming the leading cause of death worldwide. However, the lack of knowledge of brain disease’s underlying mechanisms and ineffective neuropharmacological therapy have led to further exploration of optimal treatments and brain monitoring techniques.
Objective: This study aims to review the current state of brain disorders, which utilize transcranial electrical stimulation (tES) and daily usable noninvasive neuroimaging techniques. Furthermore, the second goal of this study is to highlight available gaps and provide a comprehensive guideline for further investigation.
Method: A systematic search was conducted of the PubMed and Web of Science databases from January 2000 to October 2020 using relevant keywords. Electroencephalography (EEG) and functional near-infrared spectroscopy were selected as noninvasive neuroimaging modalities. Nine brain disorders were investigated in this study, including Alzheimer’s disease, depression, autism spectrum disorder, attention-deficit hyperactivity disorder, epilepsy, Parkinson’s disease, stroke, schizophrenia, and traumatic brain injury.
Results: Sixty-seven studies (1,385 participants) were included for quantitative analysis. Most of the articles (82.6%) employed transcranial direct current stimulation as an intervention method with modulation parameters of 1 mA intensity (47.2%) for 16–20 min (69.0%) duration of stimulation in a single session (36.8%). The frontal cortex (46.4%) and the cerebral cortex (47.8%) were used as a neuroimaging modality, with the power spectrum (45.7%) commonly extracted as a quantitative EEG feature.
Conclusion: An appropriate stimulation protocol applying tES as a therapy could be an effective treatment for cognitive and neurological brain disorders. However, the optimal tES criteria have not been defined; they vary across persons and disease types. Therefore, future work needs to investigate a closed-loop tES with monitoring by neuroimaging techniques to achieve personalized therapy for brain disorders.
Introduction
Brain disorders represent a collection of syndromes characterized by abnormalities in memory, sensation, behavior, and even personality. Neurological disorders are the second leading cause of death worldwide (Feigin et al., 2019). The burden is recognized as a global public health challenge and will increase in the next few decades (Feigin et al., 2020). As reported by the World Health Organization, there are approximately 800,000 deaths from suicide each year (approximately one death every 40 s) due to mental health issues (World Health Organization, 2018). Through decades of research, health professionals have developed a set of systematic criteria for diagnosing brain disorders, together with pharmaceutical and psychological treatments; however, understanding of the neural substrates and mechanisms involved in these diseases is limited (Chou and Chouard, 2008). In addition, reliable neurological biomarkers for identifying brain disorders are insufficient (Chou and Chouard, 2008). Moreover, certain brain disorders remain substantially unaffected by neuropharmacological therapy (Ciullo et al., 2020), and the treatment options are far from optimal in terms of efficacy and specificity. Therefore, it is essential to find alternative therapies for brain disorders that are efficient in clinical practice.
Brain stimulation has been widely applied due to its ability to modulate brain plasticity in neuropsychiatric patients (Davis and Smith, 2019; Kim E. et al., 2020; Yoo et al., 2020). Typically, brain stimulation methods have been classified as invasive or noninvasive brain stimulation (NIBS). The invasive brain stimulation approach (i.e., pharmacological intervention, targeted microsimulation, optogenetics, etc.) has been commonly applied in animal models (Polanía et al., 2018). It can be used to demonstrate the relationships of its targets to brain function with high spatial precision (e.g., cell-type effect). NIBS provides a way to modulate brain function without opening the skull. Thus, many human studies have employed NIBS to explore the causal relationship between neurological function and behavior. Two mainstay NIBS approaches have emerged to treat brain disorders in the clinical context: transcranial magnetic stimulation (TMS) and transcranial electrical stimulation (tES). The principle underlying these two modalities is based on electromagnetism and harnesses weak electrical current stimulation. More specifically, TMS can depolarize neurons by generating a strong current, and tES can influence ion channels and gradients to modulate neuronal membrane potential (Fregni and Pascual-Leone, 2007). Comparing the spatial effectiveness of NIBS with other methods, TMS and tES, the best-known stimulation modalities, can generate relatively large magnetic and electrical fields in the brain. A third promising NIBS method, transcranial focused ultrasound stimulation (tFUS), provides a solution for the low degree of spatial localization in tES and TMS by projecting the acoustic intensity field beam into brain tissues (Pasquinelli et al., 2019). However, further investigation of neurophysiological foundations is required before applying tFUS as a safe therapy in daily routine (Polanía et al., 2018). Therefore, TMS and tES are the current primary noninvasive brain stimulation methods. Both modalities affect brain function by modulating the excitation or inhibition of interneuron circuits. Generally, tES can maintain a longer outlasting effect of neural excitability than can TMS (Schulz et al., 2013). In addition, tES offers the possibility of designing a reliable sham/placebo condition for double-blind controlled clinical trials since short-term tingling sensations gradually fade away after the onset of stimulation (Nitsche et al., 2008). Moreover, tES has the advantages of lower cost, portability, and ease of application. The temporal and spatial resolutions of various brain intervention methods are compared in Figure 1.
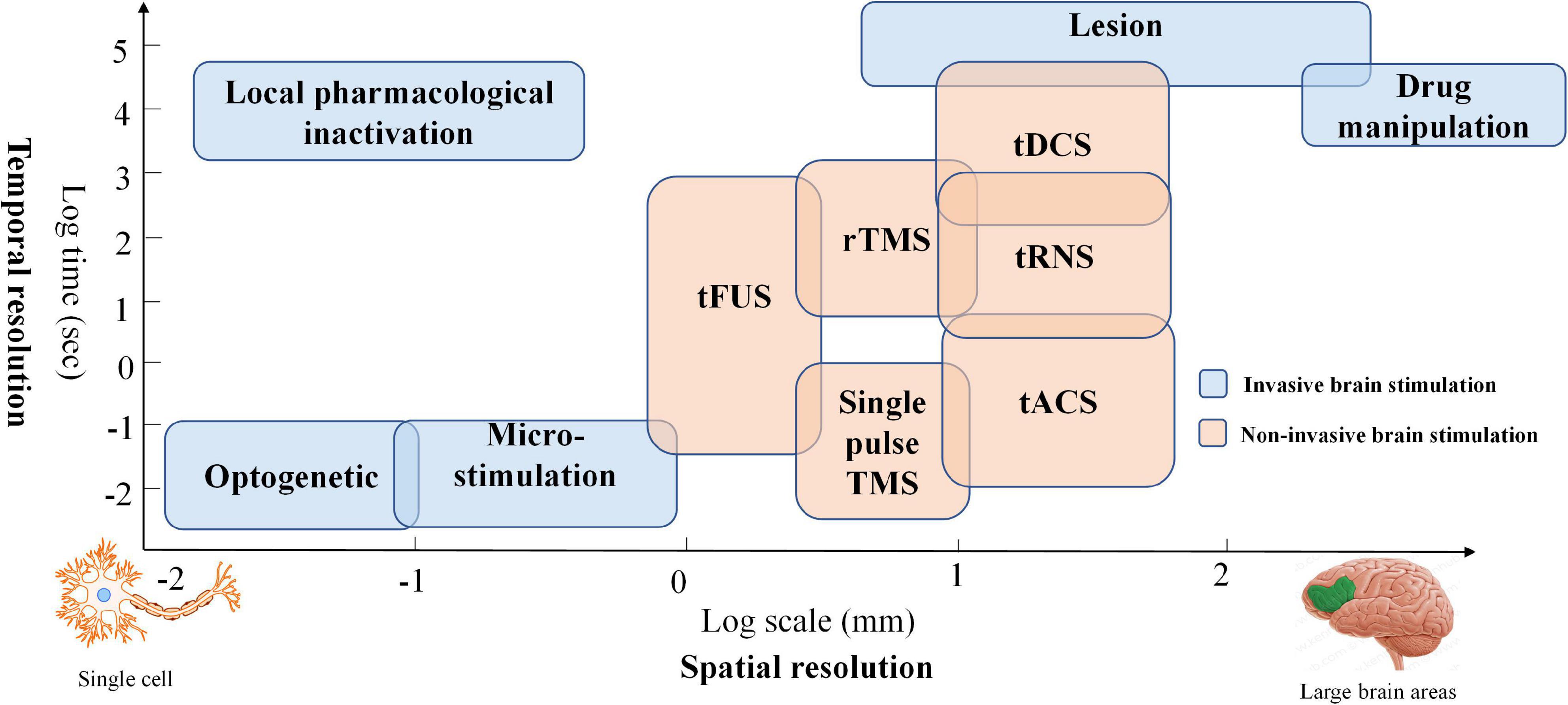
Figure 1. Temporal and spatial resolution of various brain intervention methods: Blue boxes represent invasive brain stimulation techniques, and orange boxes denote non-invasive stimulation methods.
Conventionally, there are three tES modalities: transcranial direct stimulation (tDCS), transcranial alternating current stimulation (tACS), and transcranial random noise stimulation (tRNS) (Brunyé, 2018; Bikson et al., 2019). The delivery method of the current is the main difference among the three modalities. tDCS typically transfers a homogeneous direct current ranging from 1 to 2 mA from the electrodes (i.e., two or more) on the scalp to modulate brain activation (Vosskuhl et al., 2018; Yaqub et al., 2018; Brunyé et al., 2019). In the tACS case, the current is delivered in an oscillating manner with a particular frequency and stimulation amplitude. tRNS differs from tDCS and tACS. The current amplitude for tRNS is randomly applied with a normal distribution around a specific mean strength. The principle of tACS and tRNS is the modulation of ongoing neural oscillations and the induction of the neuroplastic effect by using appropriate parameter values. tDCS is used to influence neuronal excitability by membrane polarization; for example, the anode causes depolarization, and the cathode results in hyperpolarization. The effect depends on various stimulation parameters, such as polarity, stimulation duration, intensity level, and the subject’s brain state. To date, there are no clear criteria or quantitative assessment techniques to provide guidelines for tES in terms of modulation duration and intensity or the locations where electrodes should be placed.
Neuroimaging refers to the use of magnetic and other techniques to understand the living brain system, which can reflect the properties of function, structure, or change in the brain in terms of temporal (i.e., functional imaging) and spatial localization (i.e., structural and functional imaging) (Li et al., 2018; Yin et al., 2019). Neuroimaging is commonly used to diagnose neuropsychiatric disorders and evaluate effects following therapy (i.e., brain stimulation) and includes techniques such as structural magnetic resonance imaging (MRI), functional magnetic resonance imaging (fMRI), single-photon emission computed tomography (SPECT), positron emission tomography (PET), functional near-infrared spectroscopy (fNIRS), and electroencephalography (EEG) (Naseer and Hong, 2015; Hong and Khan, 2017; Hong et al., 2020). Typically, MRI, fMRI, SPECT, and PET can offer an excellent spatial resolution for brain state examinations. However, these assessments can only be performed in restricted environments due to equipment size (i.e., bulkiness or lack of mobility) (Hong and Yaqub, 2019). Moreover, some techniques (e.g., PET and SPECT) require the insertion of radioactive tracers, limiting repeated measurements, especially for children and pregnant women (Irani et al., 2007). In addition, these systems, including MRI and fMRI, are costly, highly susceptible to motion artifacts, and have a low temporal resolution (compared with EEG and fNIRS).
As a noninvasive neuroimaging modality, EEG is one of the oldest techniques used to measure neural activation in the human brain for diagnosis or brain–computer interface (BCI) purposes (Naseer and Hong, 2013; Khan and Hong, 2017; Tanveer et al., 2019). Since they are portable and have the advantage of higher temporal resolution, EEG-based BCI applications have been widely designed for daily use (e.g., home automation control devices, EEG-based wheelchairs, brain disorder detection platforms) (Kim et al., 2019; Lee T. et al., 2020; Rashid et al., 2020; Yang et al., 2020b). EEG measurements are based on electrical potential differences between different electrodes on the scalp. A potential difference is caused by the propagation of the current flow induced by synchronized postsynaptic potentials in pyramidal neuron cell membranes. fNIRS is a promising noninvasive neuroimaging technique featuring the advantages of safety, low cost, mobility, excellent temporal resolution (compared with fMRI), moderate spatial resolution, and tolerance to motion artifacts (Nguyen et al., 2016; Ghafoor et al., 2019). The fNIRS principle is based on the absorption characteristics of oxygenated hemoglobin (HbO) and deoxygenated hemoglobin (HbR) in the spectrum ranging from 650 to 1,000 nm, for which brain tissues are more translucent than HbO or HbR (Aqil et al., 2012a,b). Changes in blood flow (i.e., increases or decreases) reflect a local brain region’s hemodynamic activity resulting from neuronal firing. More specifically, activation of brain cortical neurons results in greater blood flow (detected by the surplus of oxyhemoglobin in veins) than in brain regions with inactive neurons (Berger et al., 2019; Hu et al., 2019; Shin, 2020). Types of tES, the hemodynamic response caused by neural activity, EEG and fNIRS principles, and examples of extracted features are depicted in Figure 2. EEG and fNIRS are widely applied in clinical brain state monitoring (Yang et al., 2019b, 2020a). The development of therapeutic strategies for neuropsychiatric disorders is based on the properties described above: minimal invasiveness, safety, ease of use, and repeatability (Dubreuil-Vall et al., 2019; Ermolova et al., 2019).
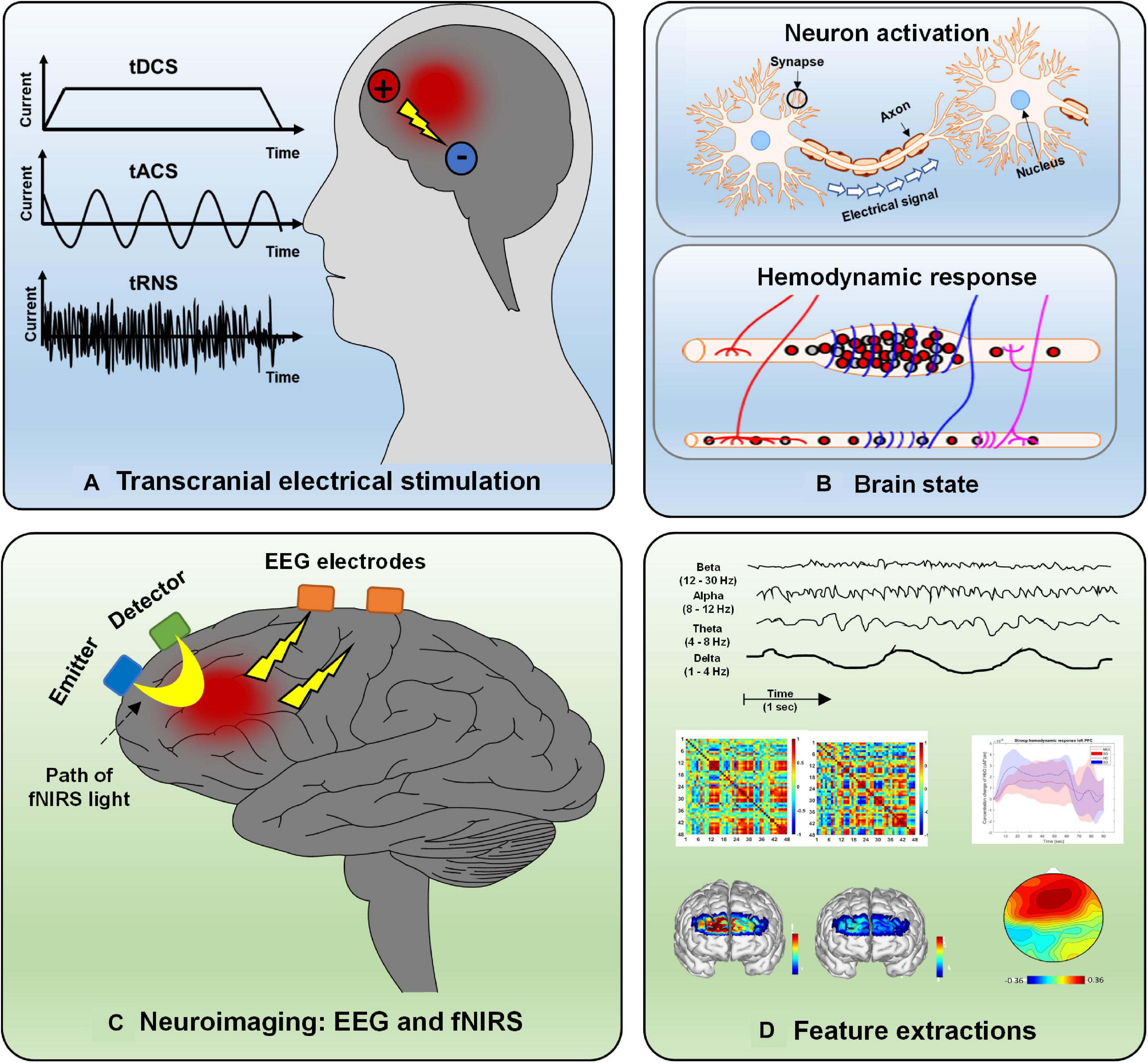
Figure 2. Overview of electrical stimulation, EEG, fNIRS, and feature extraction: (A) Three types of electrical stimulation (tDCS, tACS, and tRNS), (B) hemodynamic response caused by neural activity, (C) principle of EEG and fNIRS, and (D) various features extracted from neurological signals.
This study had two goals. The first aim was to review the current state of the application of tES, monitored by EEG and fNIRS, for treatment of nine common brain disorders: Alzheimer’s disease (AD) and mild cognitive impairment (MCI), depression, autism spectrum disorder (ASD), attention-deficit hyperactivity disorder (ADHD), epilepsy, schizophrenia, Parkinson’s disease (PD), stroke, and traumatic brain injury (TBI). Second, this study aimed to provide a general reference for future investigation of tES as a treatment for the nine diseases mentioned above: (i) how to conduct the stimulation (i.e., duration and intensity), (ii) where the electrodes should be placed, (iii) which types of EEG and fNIRS features can be used to evaluate the stimulation effect, and (iv) the behavioral and neurological effects following stimulation.
Methods
In this study, a two-stage literature search was performed to identify relevant investigations. First, an online search was conducted using the PubMed and Web of Science databases, which included peer-reviewed articles from January 2000 to October 2020 with the following keywords: (functional near-infrared spectroscopy OR fNIRS OR EEG OR electroencephalography) ++ (transcranial electrical stimulation OR transcranial direct current stimulation OR transcranial alternating current stimulation OR transcranial random noise stimulation) + Alzheimer’s disease OR AD OR depression OR autism spectrum disorder OR ASD OR attention-deficit hyperactivity disorder OR ADHD OR epilepsy OR schizophrenia OR Parkinson’s disease OR PD OR stroke OR traumatic brain injury OR TBI). Second, an additional literature search was conducted through the reference lists in selected studies or the related review paper. The aim of this two-stage literature search was to ensure that the studies included were as comprehensive as possible. As shown in Figure 3, the search identified 665 studies (i.e., 369 from PubMed and 296 from Web of Science). After removing 137 duplicates and 451 studies that did not relate to this review (i.e., review studies, animal model studies, meeting abstracts, investigations lacking stimulation or not involving brain disease, and those lacking EEG or fNIRS), 77 articles remained. Since several studies did not show the results (i.e., 10 trial studies), this systematized review consisted of 67 data sets (i.e., AD, 7; depression, 10; ASD, 2; ADHD, 4; epilepsy, 14; schizophrenia, 9; PD, 3; stroke, 14; and TBI, 4).
Alzheimer’s Diseases and Mild Cognitive Impairment
Approximately 60–70% of dementia is caused by AD. AD is a progressive brain disorder in which memory and cognitive function cause increasing impairment until death (Vuksanović et al., 2019; Feng et al., 2020). To date, there is no pharmacological cure available, except for treatment to manage symptoms. MCI is considered to be the middle stage between healthy controls and patients with dementia. It has been reported that approximately 32–38% of patients with MCI develop dementia within five or more years (Alzheimer’s Association, 2020). Brain stimulation is a promising therapeutic approach for improving memory and cognitive function in AD and preventing the progression from MCI to AD.
As shown in Table 1, six studies addressed AD (four studies) and MCI (three studies), and one was related to frontotemporal dementia. EEG neuroimaging techniques were conducted in all studies. The features extracted from EEG included the event-related potential, coherence, connectivity, and power spectra in different brain bands (e.g., alpha, beta, theta, gamma, etc.). The stimulation duration and intensity also differed for each study. One of the tACS studies (Naro et al., 2016) applied tACS to modulate gamma-band oscillations (GBOs) in AD, MCI, and HC groups. Anodal electrodes were positioned on the primary motor area (M1), premotor area (PMA), supplementary motor area (SMA), dorsolateral prefrontal cortex (DLPFC), and dorsomedial prefrontal cortex (DMPFC). The reference electrode was placed on the right mastoid. The entire stimulation procedure lasted 10 min with a 40–120-Hz stimulation frequency and a 1-mA current intensity. The tACS (GBO and neuropsychological test) effect differed among the three groups (AD, MCI, and HC). In the HC group, GBO increased. Partial improvement of GBO was observed in the MCI group, whereas there was no significant effect for individuals with AD. Interestingly, after 2 years of follow-up, the individuals with MCI who failed to show a significant effect had progressed to AD. These results indicate the difficulty of employing tACS to modulate the neuroplasticity of patients with AD using the stimulation parameters mentioned above. In another slow-oscillation tDCS (so-tDCS) study (Ladenbauer et al., 2017), the authors used slow oscillatory stimulation at 0.75 Hz to modulate the brain activity pattern and memory consolidation during the daytime nap period in patients with MCI, with the anodes and cathodes placed on the frontal area (F3 and F4) and mastoid location, respectively. The slow cortical oscillation (0.5–1 Hz), spindle power, and declarative memory significantly improved after stimulation compared to the sham group. Similarly, the authors of one of the frontotemporal dementia studies (Ferrucci et al., 2018) stated that the effect of anodal tDCS (current, 2 mA; duration, 20 min; anode, frontotemporal cortex; cathode, right deltoid muscle) might be correlated with low-frequency band oscillation during the attentional processes. Two of the AD studies were quite similar. Both studies used tDCS with a 1.5-mA current to conduct the stimulation within a day visit. The most significant differences were in the duration and location of the simulation. The first study (Marceglia et al., 2016) delivered 15 min of stimulation from the bilateral temporoparietal area (left side, P3-T5; right side, P6-T4) with the reference on the right deltoid muscle. The results revealed that high-frequency power in the temporoparietal area and coherence at the temporal–parietal–occipital junction were increased in the AD group. Stimulation was applied in the second study (Cespón et al., 2019) over the left DLPFC, and the return electrode was placed on the right shoulder, with a stimulation duration of 13 min. The working memory, P200 amplitude, and theta band brain activity in the frontal area increased after cathodal tDCS. These two studies indicate that brain stimulation may rely significantly on the interaction among tDCS polarity, stimulation location, and brain state. One of the MCI studies (Emonson et al., 2019) applied an extended stimulation period (i.e., 20 min) to deliver the stimulation current (1 mA) from the left DLPFC (F3) to the contralateral supraorbital area (Fp2). Event-related potential (ERP) differed before and after stimulation for younger and older adult groups. However, this phenomenon was not observed in the MCI group. This finding is consistent with that of prior AD studies (Cespón et al., 2019). To compare the effects of short- and long-term tDCS, Gangemi et al. (2020) suggested that if brain stimulation (i.e., tDCS) can be effective with steady-state neurocognitive function over the short term, then this effect could be prolonged for 8 months. In addition, this study (current, 2 mA; duration, 20 min; visit, 10 days or 8 months; anode, left frontotemporal cortex; cathode, right frontal lobe) (Gangemi et al., 2020) demonstrated that anodal tDCS provides a technique to slow the progression of AD by influencing neurological patterns in the brain.
Depressive Disorder
Depression presents with sorrow, guilt, fatigue, low self-worth, irregular sleep/appetite, loss of interest, and low concentration (Xu J. et al., 2019; Olejarczyk et al., 2020). Commonly, these symptoms persist in the long term and recur easily. It substantially influences the patient’s daily life. In the severe stage, depression may result in suicide. This disorder also occurs in children and adolescents (under 15 years old), but this age group’s prevalence is lower than in adults. Researchers have found altered electrical activity in specific brain regions in patients with depression with neural science development. The cortex represents excitability, and other regions exhibit lower activities. tES, as an electrical stimulation therapy modality, has shown a promising advantage for improving the symptoms of depression.
Ten studies of depression were included in this review article, summarized in Table 2. Nine papers used EEG as the neuroimaging method to evaluate the neurological effect, and only one fNIRS study assessed therapeutic performance from a hemodynamic perspective. All EEG studies employed the alpha band power spectrum to compare the difference between the pre- and poststimulation. Some studies have considered an alpha band from 8 to 12 Hz, while others regard the ranges between 8 and 13 Hz or 7.5 and 12 Hz as the alpha band. The ERP power spectra in the delta, theta, beta, and gamma bands were also investigated as extracted EEG-based features. In addition to the standard features extracted from the alpha band, all anode stimulation locations for tACS and tDCS included the left DLPFC (F3). A possible explanation is that the left DLPFC is associated with right prefrontal hyperfunction and dysfunction of brain plasticity in depression.
In the fNIRS study (Li et al., 2019), 26 patients with poststroke depression received tDCS for 20 min with a 2-mA current on the left DLPFC and a return electrode at the right DLPFC. Each subject underwent five stimulation sessions weekly for 4 weeks. The results showed that working memory task performance and HbO concentration were improved after the treatment. Alexander et al. (2019) examined the feasibility and efficacy of modulating alpha oscillations by applying tACS at 10 and 40 Hz in the DLPFC (left DLPFC, F3; right DLPFC, F4) (Alexander et al., 2019). For 5 consecutive days, subjects received 40 min of either 10 Hz tACS, 40 Hz tACS, or sham trial. Reduced power in the alpha band was observed after 2 weeks of the intervention applying the 10-Hz tACS protocol.
Similarly, one tDCS study (Nikolin et al., 2020) also used a 2-mA stimulation current for 40 min. The current was delivered from the left DLPFC to the right shoulder, with patients receiving the therapy three times per week for 6 weeks. Although behavioral performance (e.g., mood and working memory) improved, neurophysiological measurements (e.g., power spectrum and ERP) did not significantly change following the intervention. One comparative study (Nishida et al., 2019) conducted tDCS at an intensity of 1 mA for 20 min from the left DLPFC and DMPFC. The return electrode at the left shoulder indicated that the effect of tDCS on anxiety reduction depends on the site of stimulation. The effect of tDCS stimulation at the left DLPFC on patients with depression was correlated with theta band activity acquired from the rostral anterior cingulate cortex. The effect of DMPFC stimulation on anxiety reduction was related to alpha-band activity in the left inferior parietal lobule. Three investigations were performed applying current from the left DLPFC to the right supraorbital area with a current intensity of 2 mA for 20 min. These studies differed in duration of stimulation periods: 1 day, 5 consecutive days, and 5 consecutive days each week for 3 weeks. In another study (Powell et al., 2014), a significant reduction in the N200 amplitude and theta band activity was observed over the memory task’s frontal cortex substrate with one stimulation period. After five tDCS sessions, the depression score and the power spectrum (delta, theta, and low alpha band) were improved compared to the sham group, but this improvement was not sustained for 4 weeks (Liu et al., 2016). Long-term tDCS with 15 treatment periods has been found to affect mood and cognition in 50 and 60%, respectively, of individuals with depression (Al-Kaysi et al., 2017). Similarly, a lower-intensity (1 and 1.5 mA) tDCS protocol also resulted in reduced ERP (Shahsavar et al., 2018) and power spectra (e.g., delta, theta, and alpha frequency bands) (Palm et al., 2009; Khayyer et al., 2018) following stimulation. In particular, combined treatment with positive psychotherapy and tDCS can significantly affect depression. Effects are more pronounced following 3 months of follow-up than with the use of tDCS alone (Khayyer et al., 2018).
Autism Spectrum Disorder
ASD refers to a developmental disorder characterized by (i) impaired communication, social behavior, language expression, and (ii) repetition of limited interests and activities. Typically, it begins in childhood and persists in adolescence and adulthood. Most individuals with ASD cannot live independently and require life-long care (Górriz et al., 2019). Several psychosocial interventions (behavioral treatment and skill training) have been applied to reduce communication difficulties and improve individuals’ quality of life with ASD. However, the effect of psychosocial treatment depends on the individual’s state. In addition to behavioral treatment, brain stimulation, as an alternative strategy, may improve symptoms from a neurological perspective by modulating deficient neural patterns.
The presence of abnormal gamma oscillations has been considered a biomarker and a target for therapeutic engagement; thus, tACS with a frequency-specific paradigm in the gamma band became the most suitable stimulation modality (Kayarian et al., 2020). Gamma band stimulation may also play a role in mediating the motor learning mechanism. Application of tACS at 70 Hz increased motor learning capacity compared to sham treatment and tACS at 10 and 20 Hz (Sugata et al., 2018). For exploring multiple therapeutic strategies for patients with ASD, tDCS was used to modulate brain function in patients from different perspectives (abnormal synaptic maturation and connectivity). Two studies employed a 1-mA current intensity for 20 min to stimulate ASD models at the DLPFC region and evaluated EEG output effects. One study (Amatachaya et al., 2015) used one tDCS treatment period. The poststimulation results showed significant improvement in the autism treatment evaluation checklist in the social and behavioral domains compared to prestimulation scores. Besides, peak values of the alpha band also increased after stimulation. Similarly, Kang et al. (2018) conducted tDCS treatments every 2 days for 10 sessions. The maximum entropy ratio (an index of EEG signal complexity) was extracted to examine the tDCS effect and increase significantly following treatment. tDCS may be capable of rehabilitating children with ASD, as might tACS with frequency-specific characteristics. The related ASD studies are listed in Table 3.
Attention-Deficit Hyperactivity Disorder
ADHD is also a developmental disorder characterized by two types of symptoms: (i) inattentiveness and (ii) hyperactivity/impulsiveness. Most cases are diagnosed at the ages of 6–12 years. Symptoms become particularly noticeable when circumstances change. Moreover, ADHD is commonly comorbid with other psychiatric disorders (e.g., depression and anxiety disorder), causing a substantial burden for patients and their families. Thus far, the medication-based intervention can achieve short-term effects, and the long-term effects of treatment for ADHD remain uncertain (Posner et al., 2020). It is essential to develop novel alternative strategies for treating ADHD.
There are four ADHD-related articles listed in Table 4, and all the studies used EEG for neuroimaging. Event-related potentials (P200 and P300) were employed in two of the papers and were used to evaluate the effects of tDCS (Breitling et al., 2020) and tACS (Dallmer-Zerbe et al., 2020), respectively. The remaining studies extracted functional brain connectivity (Cosmo et al., 2015b), power spectra (Dallmer-Zerbe et al., 2020), and statistical analysis (Cosmo et al., 2015a) as features for examining the neurological changes following tDCS. In all investigations, 20 min of tES therapy at low current intensity (1 mA) was applied. In the tACS study (Dallmer-Zerbe et al., 2020), the authors applied a stimulation with a mean frequency of 3 Hz, delivered from multiple electrodes (anodes: C3, C4, CP3, CP4, P3, and P4) and returned by cathodes at T7, T8, TP7, TP8, P7, and P8 (the distribution of electrodes following the international 10–20 EEG system). The neurological results following tACS demonstrated that the P300 amplitude significantly increased, accompanied by a decrease in omission errors compared to pre-tACS. Both tDCS studies share the same experimental paradigm (current intensity, duration, and stimulation location), but the results were reversed. The first study (Cosmo et al., 2015b) indicated that resting-state brain connectivity increased in individuals after DLPFC stimulation. The authors of the second study (Cosmo et al., 2015a) found no evidence supporting the capability of tDCS to improve inhibitory control by stimulating the left DLPFC in patients with ADHD performing the go/no–go task. A recent investigation (Breitling et al., 2020) compared the effectiveness of conventional (with one anodal electrode) and high-definition tDCS (with four anodal electrodes) for improving working memory performance, with the anode located near the right inferior frontal gyrus and the cathode placed over the contralateral supraorbital region. The results for working memory behavior were not generally influenced by conventional and high-definition tDCS (HD-tDCS). However, elevated P300 and N200 were observed after conventional and HD-tDCS since the current intensity differed between conventional tDCS (1 mA) and HD-tDCS (0.5 mA). The conclusion, which may be difficult to accept, is that HD-tDCS is equally suitable as conventional tDCS for improving the working memory performance of patients with ADHD. Therefore, comprehensive investigations are required to assess the effectiveness of tES for treating ADHD in the future.
Epilepsy
Epilepsy is a chronic brain disease characterized by brief involuntary movement in part or the entire body with recurrent unprovoked seizures. Sometimes, seizures result in loss of consciousness and control of bladder function. Patients with epilepsy have three times the risk of premature death as the general population. Fortunately, approximately 70% of epilepsy cases can be controlled using proper antiseizure medication. It is suggested that in the remaining patients (approximately 30%) with drug-resistant epilepsy, seizure control should be achievable through surgery and neurostimulation therapies (Devinsky et al., 2018).
Abnormal EEG patterns are among the most consistent predictors of seizure recurrence (Scheffer et al., 2009). As shown in Table 5, EEG was employed in all 14 epilepsy studies as the neuroimaging method to assess the tES effect. The evaluated features included the power spectrum, connectivity, mean peak amplitude, mean number of spikers, and seizure frequency. Each study applied a different stimulation strategy to explore the optimal stimulation protocol. In the tACS study (San-Juan et al., 2016), patients received a sinusoidal fluctuating current (frequency, 3 Hz; intensity, 1 mA) from Fp1 to Fp2. The stimulated location was determined by visual inspection of EEG signals for the most active epileptiform cortex. Sessions of 60-min duration were conducted daily for 4 consecutive days. At the 2-month follow-up, patients were asked to report whether they had experienced one or more seizures in the previous 15 days. A lower oscillation (0.75 Hz) tACS was used to investigate the enhancement of memory consolidation during slow-wave sleep in patients with temporal lobe epilepsy (TLE) (Del Felice et al., 2015). The anode was placed over the frontotemporal lobe, and the cathode was placed on the ipsilateral mastoid. Visuospatial memory performance, slow spindles (10–12 Hz), and fast spindles (12–14 Hz) were extracted as EEG features to assess the effect of stimulation. Both behavioral and neurological performance improved following stimulation. The results suggest that memory rehabilitation may be achieved by slow oscillatory stimulation in patients with TLE.
tDCS remains the primary intervention for epilepsy rehabilitation. Three of the studies applied HD-tDCS as the stimulation protocol with different stimulation parameters and locations. No adverse reactions were reported in any studies, but the reported results differed slightly. One of the studies (Meiron et al., 2019) stated that reduced interictal epileptic discharge and seizure-related delta activity changed. The stimulation intensity was set at 1 mA for 20 min in 20 interventions. Similarly, the interictal sharp wave amplitude after HD-tDCS (0.1–1 mA, 20 min, 10 sessions) was lower than the preintervention level. However, the seizure frequency was not significantly decreased (Meiron et al., 2018). On the other hand, Karvigh et al. (2017) reported that the mean seizure frequency decreased immediately after HD-tDCS (2 mA, 20 min, and 10 sessions). However, this reduction did not persist after 1 month of follow-up. Interestingly, the attention and working memory performance were improved in all patients even after 1 month. These discrepancies in results may have resulted from differences in stimulation protocol parameters (intensity, duration, and location). In five studies, cathodes were placed on the epileptogenic focus (i.e., focal disease-related area) to achieve adequate stimulation (Fregni et al., 2006; Auvichayapat et al., 2013; San-Juan et al., 2017; Lin et al., 2018; Tecchio et al, 2018). Although the stimulation parameters varied, a reduction in seizure frequency or a significant decrease in epileptic discharge was observed. Partial epileptic participants showed reduced seizure frequency when the anode–cathode pair was located between the temporoparietal and contralateral supraorbital regions (Tekturk et al., 2016; Yang et al., 2019a). In one of the studies (Faria et al., 2012), tDCS and EEG recordings were combined to investigate the feasibility of the simultaneous use of two modalities for continuous monitoring of epilepsy. The simultaneous recording signals were used to analyze the reduced interictal epileptiform EEG discharges. The feasibility of this technique may provide an approach for monitoring epileptic activity in real time during the intervention. However, the input current from the stimulation might also interfere with the recording of endogenous EEG signals. Therefore, future studies should consider the advantages and disadvantages of using these two tES modalities simultaneously.
Schizophrenia
Schizophrenia is a type of psychosis that presents distortions in cognition, thinking, perception, feeling, emotions, and language. Patients experience hallucinations and delusions. For instance, patients may see/hear nonexistent voices/things and develop supernatural beliefs. Quality of life for schizophrenia patients is highly influenced by various risk factors, such as diabetes, cardiovascular disease, and suicide (Patel et al., 2014). The underlying mechanism remains poorly understood, and the gap between research and practical applications is considerable. Further investigation is required to arrive at a better diagnosis and effective therapeutic strategies.
Nine schizophrenia studies were included in this review (Table 6). All the studies employed EEG as the neuroimaging modality. The extracted EEG features consisted of the power spectrum (e.g., delta, theta, and gamma frequency bands), event-related potentials (e.g., P300, P170, etc.), and functional connectivity. The prefrontal cortex plays a crucial role in working memory, cognition, planning, decision making, emotional regulation, and social interaction (Ferguson and Gao, 2018). In over half of the examined studies (five of nine), electrodes were placed on the prefrontal cortex. Only one study (Rassovsky et al., 2018) reported no significant cognitive or neurological (i.e., event-related potentials) effects in patients with schizophrenia. The remaining four studies (Hoy et al., 2015; Dunn et al., 2016; Ahn et al., 2019; Boudewyn et al., 2020) demonstrated improvement following the intervention, either in the behavioral (working memory performance, steady-state auditory response, and proactive cognitive control) or the neurological domains (functional connectivity, gamma oscillation, alpha oscillation, and P300). A comparative study (tDCS, tACS, and sham) (Ahn et al., 2019) reported that 10-Hz tACS stimulation achieved better performance than tDCS in enhancing alpha oscillation modulation of functional connectivity in the alpha band. A similar study (Singh et al., 2019) found that theta oscillations were significantly elevated following theta frequency stimulation, but this phenomenon was not seen for delta frequency with tACS. An improved theta oscillation was also found following medial frontal tDCS in another study (Reinhart et al., 2015), in which tDCS modulated low-frequency oscillation in the absence of synchrony in the patient’s brain. Some research teams have shifted the anodes’ locations from the frontal cortex to the temporal or occipital lobes to probe the effects on the abnormal symptoms of schizophrenia (visual processing abnormalities, working memory deficits, and auditory hallucinations). Working memory performance and auditory deviance detection were increased by anodal frontal and temporal tDCS (Impey et al., 2017). The plasticity effect following occipital tDCS was not found during anodal and cathodal stimulation (Jahshan et al., 2020). Although significant positive effects were not observed in the investigations, a comparison of the results reveals the importance of parameter selection and emphasizes comprehensive research requirements for future studies.
Parkinson’s Disease
PD is a neurodegenerative disorder that predominantly impacts the dopamine-producing neurons of the substantia nigra in the brain and further affects movement control. PD patients may experience outward symptoms such as tremors, bradykinesia, limb rigidity, and gait/balance problems. Treatment strategies are dependent on symptoms and may include medication therapy, surgical therapy, and lifestyle modification. Recently, deep brain stimulation (DBS) was approved by the Food and Drug Administration (FDA) to rehabilitate PD patients. However, the procedure for setting the DBS device requires a surgical step. Therefore, researchers have moved to the NIBS area, which seeks noninvasive therapy to avoid surgical pain.
Three PD studies (see Table 7) were included after screening by related keywords. Two of three studies applied EEG to describe the cortical activity, and one employed fNIRS as the neuroimaging modality. The extracted EEG/fNIRS-based features and applied stimulation protocols are varied. One of the studies (Schoellmann et al., 2019) used tDCS to deliver a current of 1 mA from the left sensorimotor cortex for 20 min and the return electrode to the right frontal lobe. After the intervention, the motor cortex’s cortical activity and synchronization were altered, but it did not change the cortical oscillatory of PD patients. However, the reduced beta rhythm oscillation was obtained by the theta-tACS and tRNS, in which research was performed in a personalized stimulation setting (Del Felice et al., 2019). One fNIRS research (Beretta et al., 2020) examined the tDCS effect with different intensities (1 mA, 2 mA, and sham). Both tDCS intensities showed a reduced time response to recover the balance post perturbation. The 2-mA stimulation displayed a better performance than that of 1 mA.
Stroke
Stroke, known as cerebrovascular disease, is the second leading cause of death and the third leading cause of disability worldwide. The risk of death depends on the category of stroke (transient ischemic attack, blockage of an artery, carotid stenosis, and rupture of a cerebral blood vessel). Since brain cells die due to insufficient blood support, survivors typically experience paralysis, vision or speech loss, and confusion. Several behavioral rehabilitation approaches, such as physical therapy and speech training, are provided to recover impaired brain regions for these patients. NIBS is an emerging method for facilitating neural plasticity in the damaged brain from a neurological perspective, and its potential and capability have received much attention.
Most of the studies (12 of 14) used EEG to monitor the effects of stimulation, as shown in Table 8. Additionally, two studies investigated damaged neurovascular coupling in stroke survivors using a combination of EEG and fNIRS modalities. Both joint EEG-fNIRS studies (Dutta et al., 2015; Jindal et al., 2015) concluded that anodal tDCS could modulate impaired neurovascular coupling. In particular, the log-transformed EEG mean power within 0.5–11.25 Hz correlated with the hemodynamic response of HbO (initial dip). Moreover, functional connectivity (Nicolo et al., 2018), event-related potentials (D’Agata et al., 2016), event-related desynchronization (Kasashima et al., 2012; Ang et al., 2015; Kasashima-Shindo et al., 2015; Naros and Gharabaghi, 2017), and power spectra (theta, alpha, beta, and gamma EEG bands) (Hordacre et al., 2018; Bao et al., 2019; Mane et al., 2019) were also possible biomarkers for checking recovery after tDCS. In the EEG studies, three studies explored the feasibility of tDCS for treating apraxia (swallowing apraxia and aphasia) in stroke. Lower current intensities (1 and 1.2 mA) were used over long-term (10 and 15 sessions, respectively) interventions. Positive behavioral and neurological results were obtained after tDCS for all three studies (Dominguez et al., 2014; Wu et al., 2015; Yuan et al., 2017). Anodal tDCS may offer a novel therapeutic means for the rehabilitation of aphasia and swallowing apraxia. Although all 14 stroke-related investigations applied different stimulation strategies, encouraging postintervention effects were achieved from either the neurological or cognitive perspective. These findings differ from the experimental results for other diseases (e.g., AD or schizophrenia), in which the various stimulation sites and parameters may lead to contrary results. Future studies should explore the underlying altered mechanism by monitoring prognostic neurological or hemodynamic biomarkers. Moreover, attention should be devoted to investigating HbO and neurovascular coupling concentration changes before and after the intervention, especially for small vessel diseases (such as stroke).
Traumatic Brain Injury
TBI is a disorder characterized by disrupted brain function that is generally caused by an external bump, violent blow, or jolt to the head. TBI may cause a wide range of symptoms due to various brain injury regions, including physical impairment, psychological changes, and sensory or cognitive alterations. Like the rehabilitation method for stroke, the most popular rehabilitation therapies for TBI include physical training, cognitive therapy, and psychological counseling. As reported in animal studies, tES can improve motor deficits by changing the neuroplasticity of TBI. NIBS may provide the potential to promote cognitive or motor recovery in TBI patients.
As shown in Table 9, there are four relevant types of research exploring the feasibility of tDCS for TBI treatment, in which EEG was used to examine neurological alterations. Although the studies employed various stimulation strategies, the outcomes, either in terms of behavioral measurement or neuroimaging, showed positive effects. For example, the positive effects included increased excitability of the sensorimotor brain area (Ulam et al., 2015), improvement of consciousness as measured by the revised JFK coma recovery scale (Straudi et al., 2019; Zhang et al., 2020), improved auditory memory function (O’Neil-Pirozzi et al., 2017), and reduced apathy level (Zhang et al., 2020). Most of these studies employed longer-term stimulation (i.e., 3, 10, and 40 sessions) with a higher current intensity (2 mA) and delivery of the current at the prefrontal cortex. Although some of the studies used different stimulation protocols, the stimulation effect was still achieved. The underlying mechanism needs to be investigated through a comprehensive evaluation to shine a light for future work. The extracted features included the approximate entropy, power spectra (delta, theta, alpha, and beta bands), and ERP (P300), selected as the characteristics for decoding brain signals to assess interventions’ effects. Among those EEG features, delta band declines were significantly associated with neuropsychological test performance following tDCS. As the evidence indicates, the EEG pattern may be correlated with the severity of brain injury. Generally, the power in the slow frequency bands (delta and theta) increased, and the high-frequency band’s power was reduced. Therefore, a possible explanation is that reduction in delta band power is a biomarker for recovery from brain injury in TBI and can be considered a reversed neurological symptom. Therefore, reversed features can be biomarkers for the initial diagnosis to monitor the effects of stimulation.
Discussion
In this study, we investigated the current state of tES utilizing noninvasive neuroimaging techniques (EEG and fNIRS) to monitor altered neurological activity in brain disorders, including AD, MCI, depression, ASD, ADHD, epilepsy, schizophrenia, PD, stroke, and TBI. Moreover, this study presents general guidelines for selecting stimulation parameters for tES and examining the extracted EEG/fNIRS-based features, based on the results of 67 studies with a dataset of 1,385 patients. Epilepsy (20.9%), stroke (20.9%), and depression (14.93%) were most commonly considered. Most of the studies utilized EEG as the neuroimaging technique (Figure 4). This study is the first work to investigate the integration of noninvasive neuroimaging and neuromodulation methods in nine brain disorders to the best of our knowledge.
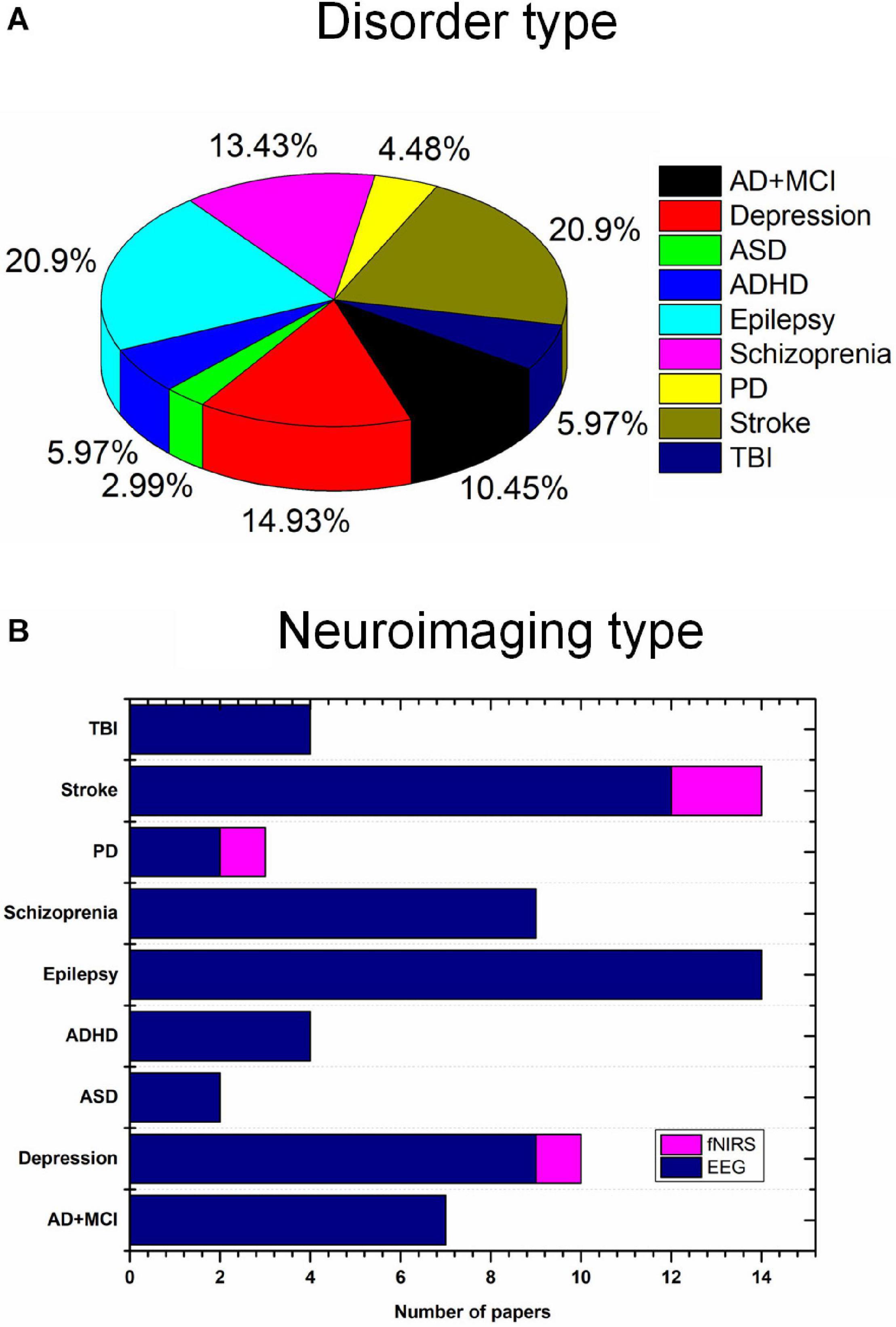
Figure 4. Brain disorders and imaging techniques: (A) Nine brain disorders, (B) number of EEG/fNIRS papers per brain disorder.
Stimulation Modality of tES
tDCS is still a representative NIBS, and approximately 82.61% of the investigated studies employed tDCS as the stimulation modality. tACS and the other modalities accounted for 11.59 and 5.8%, respectively, as shown in Figure 5A. The other modalities included slow oscillation between tDCS and tRNS. There is no significant evidence to support the superiority of the treatment effect for each stimulation modality. However, some comparative studies have concluded that tACS or tRNS are the most effective NIBS methods for rehabilitating stroke (Inukai et al., 2016) or schizophrenia (Ahn et al., 2019). These claims still require validation by large sample sizes and multiple examination perspectives. Generally, tDCS cannot modulate the specific frequencies of oscillation but can induce excitability/inhibition, altering certain regions’ cortical activity. Decreasing r-aminobutyric acid (GABA) and increasing glutamate/glutamine concentrations have been reported as the physiological mechanisms of tDCS (Reed and Cohen Kadosh, 2018). Declines in GABA have, in turn, been associated with the alteration of resting-state connectivity. It is consistent with studies that have demonstrated changes in functional connectivity following tDCS intervention. Unlike tDCS, tACS can entrain a large number of neuronal firing events with an exogenous frequency. Many studies have stated that tACS can selectively entrain brain oscillations. The entrainment of tACS is most effective when the endogenous oscillation is similar to the frequency of stimulation (Fröhlich and McCormick, 2010). This observation may explain the reported dependence of tACS on brain states. Similarly, altered GABA levels have been observed after tACS intervention (Nowak et al., 2017). Therefore, functional connectivity would be expected to change following stimulation. A future study could consider functional connectivity as an altered biomarker for monitoring the tES effect. The mechanism underlying tRNS, a novel tES modality for stimulating the human brain, is still not clearly understood. One research group (Inukai et al., 2016) concluded that tRNS is more effective than tDCS or tACS for inducing neural excitability. Since stimulation frequency in tRNS can vary, with normal distribution between 0.1 and 640 Hz, some researchers (Campana et al., 2016) have claimed that the opposite effect of cortical excitability would appear after low- and high-frequency stimulation. Two hypotheses have been proposed to explain physiological and pharmacological results. One states that tRNS induces random cortical activity and boosts the brain’s sensitivity to further external input (Van Doren et al., 2014). Alternatively, the other hypothesis holds that repeated random stimulation prevents homeostasis in the brain and potentiates event-related neural activity (Fertonani et al., 2011). Much work remains to identify the mechanism underlying tRNS and obtain a reliable stimulation protocol.
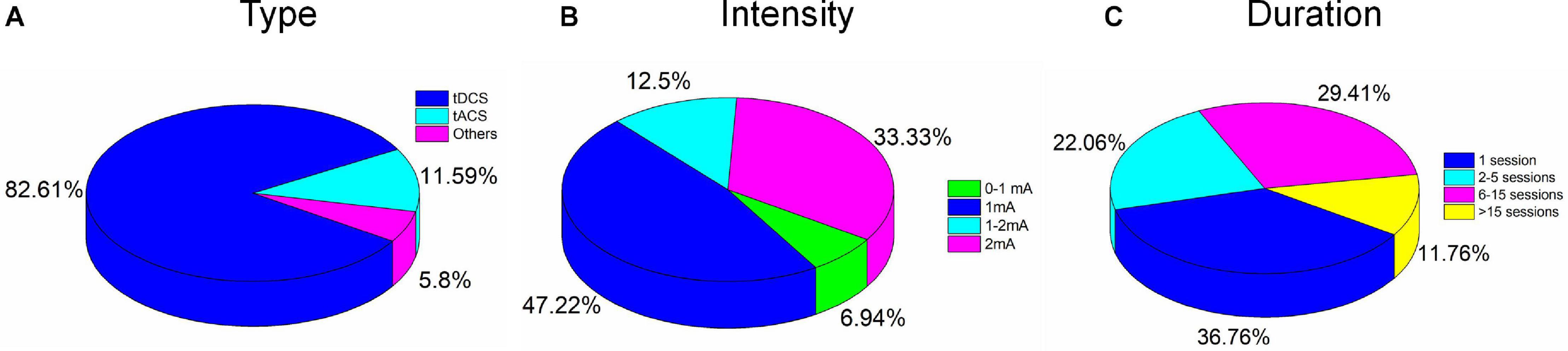
Figure 5. Percentages of electrical stimulation parameters: (A) Type, (B) intensity, and (C) duration.
Stimulation Intensity and Duration of tES
None of the 67 studies reported adverse effects. Conventionally, to avoid skin burns or irritation due to the increased temperature of the electrodes, care must be taken in the choice of stimulation parameters such as duration (<40 min) and intensity (<4 mA) (Bikson et al., 2016). Typically, for tACS and tRNS, patients experience a minimal perception of current relative to tDCS. Overall, differences in duration, intensity, location of stimulation, and combinations of these parameters and tasks performed can result in various postintervention outcomes. Stimulation intensities of 1 mA (47%) and 2 mA (33.3%) were commonly used to deliver the current in the reviewed studies, as shown in Figure 5B. For pediatric participants, the application of reduced current intensities (approximately 1 mA) was suggested. However, a review article with a dataset of 2,800 sessions across approximately 500 pediatric subjects reported that trials with 2 mA did not show severe adverse effects (Bikson et al., 2016). It is consistent with results for studies of children (e.g., ASD, ADHD) included in our review (Tables 3, 4). There was no significant correlation between the stimulation effect and intervention duration. Most of the studies employed a 16–20-min (68.66%) or 21–30-min (8.96%) stimulation for each session, as shown in Figure 6. Although some of the studies applied the intervention in small time windows (<5 min), behavioral or neurological alterations could be observed. Few studies have explored and discussed the appropriate stimulation duration, either long term or short term. Most of the studies conducted tES in a single session (36.76%) or two to five sessions (22.06%), as illustrated in Figure 5C. One of the stroke studies (Dominguez et al., 2014) stated that more prolonged stimuli might lead to more remarkable recovery, which was not consistent with results comparing patients with AD between short- and long-term neurostimulation. In this comparative research, long-term intervention (8 months) slowed AD progression similar to the effect of the short-term intervention (10 days). It is essential to investigate whether it is necessary to apply a long-term neuromodulation session to treat each disorder. Perhaps, a closed-loop stimulation system could overcome this issue, in which the current (e.g., intensity, duration, etc.) is applied based on the real-time brain state.
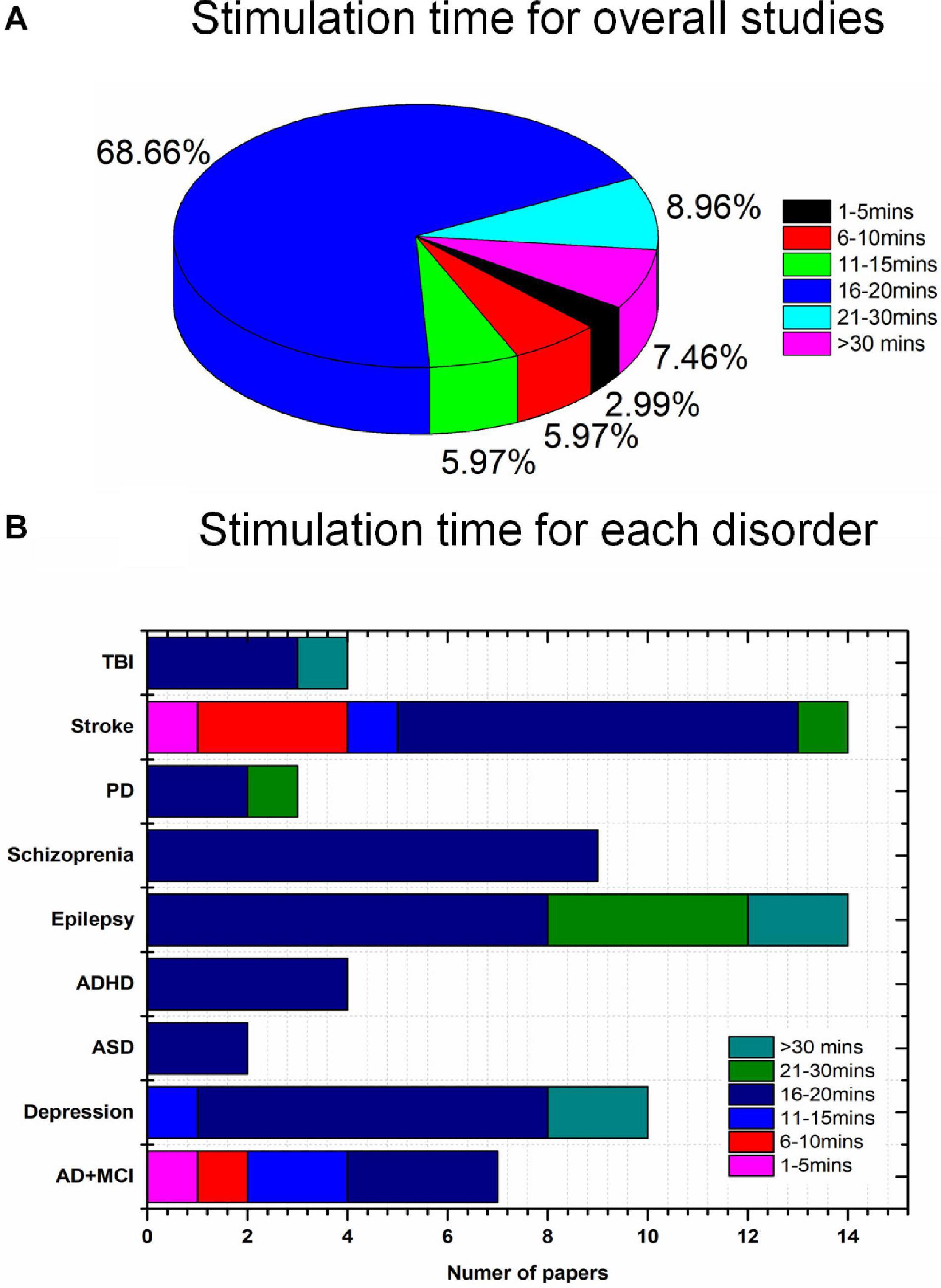
Figure 6. Disease-wise stimulation time distribution (total 67 studies): (A) overall, (B) disease-wise.
Selection of Location for tES Stimulation
The placement of the anode and cathode is a crucial factor affecting the stimulation effect. Various intervention results have been achieved owing to the different stimulation locations. The frontal cortex (46.38%) has been widely used for the placement of the anode; in particular, over 80% of studies (Figure 7) of mental disorders (depression, ASD, ADHD, and schizophrenia) delivered current to the frontal brain region. This choice for anode location was mostly due to the specific cortical function and monitored deficits/changes in certain brain areas. For instance, the frontal cortex is used as the region of interest for mental disorders because of its importance in cognition, planning, emotional expression, decision making, and social behavior. Similarly, approximately 85% of stroke studies employed the motor cortex for stimulation due to the symptoms (i.e., trouble walking and loss of balance) in stroke patients. In studies of epilepsy, anodes are positioned in the epileptogenic focus area. Cathodes are usually placed on the supraorbital, mastoid, left/right shoulder, or brain scalp. To date, there is insufficient evidence to support the selection of cathodes to induce particular stimulation effects. Conceptually, the differences in the placement of the return electrode could result in various current trajectories. It would be interesting to investigate the proper placement of the anode and cathode for each brain disorder.
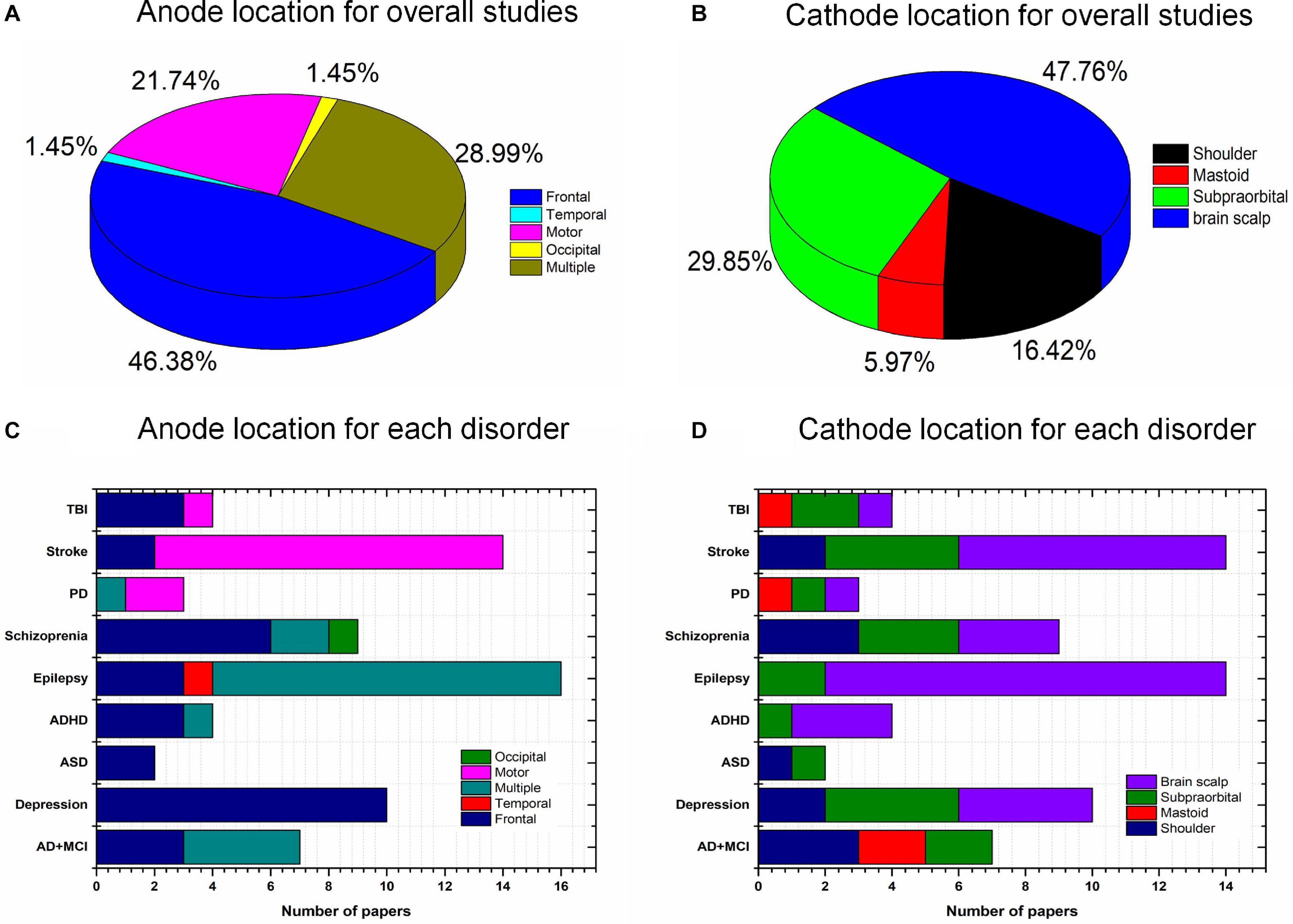
Figure 7. Anode and cathode distributions: (A) Anode (overall), (B) cathode (overall), (C) anode locations for individual diseases, and (D) cathode locations for individual disorders.
Neuroimaging in tES
Neuroimaging techniques enable monitoring brain states concerning localized neural activity and the cortical brain network, either offline or online. Neuroimaging is widely used as a monitoring/quantitative tool during or after neuromodulation to elucidate the mechanisms underlying altered symptoms (behavioral and cognitive domains) of brain disorders. It is employed to establish which stimulation protocols respond favorably in terms of symptoms and how the brain is affected from the functional and structural perspectives. Besides, the combination of neuromodulation and neuroimaging techniques enables a causal evaluation of the dynamic interactions among different brain regions (Shafi et al., 2012). As shown in Figure 4B, EEG was used in most tES studies as the neuroimaging method. A few studies utilized fNIRS to measure hemodynamic activity after the intervention. The extracted EEG/fNIRS-based features, such as power spectra, cortical oscillations, network complexity, and functional connectivity, could provide evidence for neuro-modulatory effects. The power spectrum can describe an EEG signal’s power distribution by frequency and time (Kim S. Y. et al., 2020). The commonly applied algorithm is a short-time Fourier transform and wavelet transform. Brain network complexity and connectivity reflect the information transmission status in a brain, determined by entropy analysis, Pearson correlation coefficients, spectral coherence, phase-locking values, and the phase lag index (Song et al., 2019; Fan et al., 2020). Nevertheless, EEG is limited in simultaneous use with the brain stimulation device because recorded signals affect the electric and magnetic fields. However, specific signal-processing algorithms can remove interference components, such as principal component analysis, independent component analysis, and adaptive filter algorithms (Deng et al., 2019; Hong and Pham, 2019; Yoo, 2019; Park et al., 2020). Thus, a technique free of limitation, such as a noninvasive neuroimaging modality (i.e., fNIRS), as a promising method, should be considered the first choice. In addition, hybrid neuroimaging modalities have been suggested to evaluate the neuromodulation effect to seek reliable results (Kwon et al., 2020; Lee S. et al., 2020). For instance, hybrid neuroimaging modalities could improve detection accuracy and provide more compelling support from different perspectives (e.g., electrophysiological, electromagnetic, and hemodynamic) to examine cerebral reactions (Duan et al., 2019; Zhang et al., 2019; Tortora et al., 2020). Meanwhile, the neurovascular coupling can be further investigated as a biomarker for specific blood vascular diseases (e.g., stroke).
Various EEG- and fNIRS-based features were selected to quantify brain state alterations, including the power spectrum, ERP, brain connectivity, and complexity index. The power spectrum (45.68%) was widely used as the monitoring index to examine modulations’ effects (Figure 8). Most of the studies did not explain feature selection or the mechanisms underlying altered features. Interestingly, one of the tACS studies (Del Felice et al., 2019) analyzed resting-state EEG signals in patients with PD to determine the neural oscillation deficit and brain location affected in each individual. The tACS delivered a sinusoidal fluctuating current with no frequency band to the detected location of the deficit. This investigation may offer a hint that the quantitative features for examining the effects of tES should be extracted based on diagnostic biomarkers in future studies. In other words, the diagnostic biomarker indicated abnormal features of patients relative to healthy controls. Similarly, therapy rehabilitation, in turn, should minimize this difference (diagnostic biomarkers).
Future Directions
As discussed above, brain states vary among individuals, and standard stimulation criteria are lacking. Therefore, a closed-loop brain stimulation strategy was proposed, providing a customized spatial, temporal, and parameter-specific stimulation protocol for participants based on integrating neuroimaging and neuromodulation techniques. In a strict sense, the closed-loop tES system interactively controls specific variables (current intensity, oscillation frequency, and stimulation duration) using an algorithm to adjust or minimize the error (Anna Paula et al., 2020). Error is generated between the feedback signals and the reference signals (healthy control signals and predefined thresholds) (Lv et al., 2021). Independent variable(s) can be established as any stimulation parameter(s) that need to be optimized (Xu H. et al., 2019). The overall diagram of the closed-loop stimulation is shown in Figure 9. Neurological information (power spectrum and hemodynamic response) and behavioral performance (working memory score and the response time for attention) could quantify the brain state as feedback signals to adjust the system. Future studies should develop a closed-loop tES system by combining different neuroimaging modalities to increase the therapy’s precision and effectiveness. A meta-analysis of a specific disorder/neuroimaging modality/stimulation parameter is also recommended and could reference future studies in quantitative aspects.
Conclusion
This study investigated 67 studies with a total sample size of 1,385 patients to review the current state of brain disorder therapy applying tES intervention and monitored by noninvasive neuroimaging methods (EEG and fNIRS). Nine brain disorders were reviewed in this article, including AD, depression, ASD, ADHD, epilepsy, schizophrenia, PD, stroke, and TBI. This study presents a conclusive overview of the current development of tES as a neuromodulation modality for the disorders mentioned above. In addition, the summarized stimulation protocols from the 67 studies provide a reference for selecting stimulation parameters for future investigations. Moreover, a closed-loop stimulation strategy was suggested to be a customized tES therapy for patients with brain disease to achieve optimal efficacy and specificity.
Author Contributions
DY conducted the literature review and wrote the first draft of the manuscript. Y-IS participated in revising the manuscript. K-SH conceived the idea, corrected the manuscript, and finalized the work. All authors have approved the final manuscript.
Conflict of Interest
The authors declare that the research was conducted in the absence of any commercial or financial relationships that could be construed as a potential conflict of interest.
Acknowledgments
This work was supported by the National Research Foundation (NRF) of Korea under the auspices of the Ministry of Science and ICT, Korea (Grant Nos. NRF-2017M3A9E9032772 and NRF-2020R1A2B5B03096000).
References
Ahn, S., Mellin, J. M., Alagapan, S., Alexander, M. L., Gilmore, J. H., Jarskog, L. F., et al. (2019). Targeting reduced neural oscillations in patients with schizophrenia by transcranial alternating current stimulation. Neuroimage 186, 126–136. doi: 10.1016/j.neuroimage.2018.10.056
Alexander, M. L., Alagapan, S., Lugo, C. E., Mellin, J. M., Lustenberger, C., Rubinow, D. R., et al. (2019). Double-blind, randomized pilot clinical trial targeting alpha oscillations with transcranial alternating current stimulation (tACS) for the treatment of major depressive disorder (MDD). Transl. Psychiatry 9:106. doi: 10.1038/s41398-019-0439-0
Al-Kaysi, A. M., Al-Ani, A., Loo, C. K., Powell, T. Y., Martin, D. M., Breakspear, M., et al. (2017). Predicting tDCS treatment outcomes of patients with major depressive disorder using automated EEG classification. J. Affect. Disord. 208, 597–603. doi: 10.1016/j.jad.2016.10.021
Alzheimer’s Association. (2020). 2020 Alzheimer’s disease facts and figures. Alzheimer’s Dement. 16, 391–460. doi: 10.1002/alz.12068
Amatachaya, A., Ensen, M. P., Patjanasoontorn, N., Auvichayapat, N., Suphakunpinyo, C., Janjarasjitt, S., et al. (2015). The short-term effects of transcranial direct current stimulation on electroencephalography in children with autism: a randomized crossover controlled trial. Behav. Neurol. 2015:928631.
Ang, K. K., Guan, C., Phua, K. S., Wang, C., Zhao, L., Teo, W. P., et al. (2015). Facilitating effects of transcranial direct current stimulation on motor imagery brain-computer interface with robotic feedback for stroke rehabilitation. Arch. Phys. Med. Rehabil. 96, S79–S87. doi: 10.1016/j.apmr.2014.08.008
Anna Paula, A. P. V., Acioli, G., and Barros, P. R. (2020). Evaluation and redesign of the inverted decoupler: open and closed-loop approaches. Int. J. Control. Autom. Syst. 18, 1435–1444. doi: 10.1007/s12555-019-0371-3
Aqil, M., Hong, K.-S., Jeong, M. Y., and Ge, S. S. (2012a). Cortical brain imaging by adaptive filtering of NIRS signals. Neurosci. Lett. 514, 35–41. doi: 10.1016/j.neulet.2012.02.048
Aqil, M., Hong, K.-S., Jeong, M. Y., and Ge, S. S. (2012b). Detection of event-related hemodynamic response to neuroactivation by dynamic modeling of brain activity. Neuroimage 63, 553–568. doi: 10.1016/j.neuroimage.2012.07.006
Auvichayapat, N., Rotenberg, A., Gersner, R., Ngodklang, S., Tiamkao, S., Tassaneeyakul, W., et al. (2013). Transcranial direct current stimulation for treatment of refractory childhood focal epilepsy. Brain Stimul. 6, 696–700. doi: 10.1016/j.brs.2013.01.009
Bao, S. C., Wong, W. W., Leung, T. W. H., and Tong, K. Y. (2019). Cortico-muscular coherence modulated by high-definition transcranial direct current stimulation in people with chronic stroke. IEEE Trans. Neural Syst. Rehabil. Eng. 27, 304–313. doi: 10.1109/TNSRE.2018.2890001
Beretta, V. S., Vitório, R., Nóbrega-Sousa, P., Conceição, N. R., Orcioli-Silva, D., Pereira, M. P., et al. (2020). Effect of different intensities of transcranial direct current stimulation on postural response to external perturbation in patients with parkinson’s disease. Neurorehabil. Neural Repair 34, 1009–1019. doi: 10.1177/1545968320962513
Berger, A., Horst, F., Müller, S., Steinberg, F., and Doppelmayr, M. (2019). Current state and future prospects of EEG and fNIRS in robot-assisted gait rehabilitation: a brief review. Front. Hum. Neurosci. 13:172. doi: 10.3389/fnhum.2019.00172
Bikson, M., Esmaeilpour, Z., Adair, D., Kronberg, G., Tyler, W. J., Antal, A., et al. (2019). Transcranial electrical stimulation nomenclature. Brain Stimul. 12, 1349–1366. doi: 10.1016/j.brs.2019.07.010
Bikson, M., Grossman, P., Thomas, C., Zannou, A. L., Jiang, J., Adnan, T., et al. (2016). Safety of transcranial direct current stimulation: evidence based update 2016. Brain Stimul. 9, 641–661. doi: 10.1016/j.brs.2016.06.004
Boudewyn, M. A., Scangos, K., Ranganath, C., and Carter, C. S. (2020). Using prefrontal transcranial direct current stimulation (tDCS) to enhance proactive cognitive control in schizophrenia. Neuropsychopharmacology 45, 1877–1883. doi: 10.1038/s41386-020-0750-8
Breitling, C., Zaehle, T., Dannhauer, M., Tegelbeckers, J., Flechtner, H. H., and Krauel, K. (2020). Comparison between conventional and HD-tDCS of the right inferior frontal gyrus in children and adolescents with ADHD. Clin. Neurophysiol. 131, 1146–1154. doi: 10.1016/j.clinph.2019.12.412
Brunyé, T. T. (2018). Modulating spatial processes and navigation via transcranial electrical stimulation: a mini review. Front. Hum. Neurosci. 11:649. doi: 10.3389/fnhum.2017.00649
Brunyé, T. T., Hussey, E. K., Fontes, E. B., and Ward, N. (2019). Modulating applied task performance via transcranial electrical stimulation. Front. Hum. Neurosci. 13:140. doi: 10.3389/fnhum.2019.00140
Campana, G., Camilleri, R., Moret, B., Ghin, F., and Pavan, A. (2016). Opposite effects of high-and low-frequency transcranial random noise stimulation probed with visual motion adaptation. Sci. Rep. 6:38919. doi: 10.1038/srep38919
Cespón, J., Rodella, C., Miniussi, C., and Pellicciari, M. C. (2019). Behavioural and electrophysiological modulations induced by transcranial direct current stimulation in healthy elderly and Alzheimer’s disease patients: a pilot study. Clin. Neurophysiol. 130, 2038–2052. doi: 10.1016/j.clinph.2019.08.016
Chou, I. H., and Chouard, T. (2008). Neuropsychiatric disease. Nature 455, 889–899. doi: 10.1038/455889a
Ciullo, V., Spalletta, G., Caltagirone, C., Banaj, N., Vecchio, D., Piras, F., et al. (2020). Transcranial direct current stimulation and cognition in neuropsychiatric disorders: systematic review of the evidence and future directions. Neuroscientist [Epub ahead of print] doi: 10.1177/1073858420936167
Cosmo, C., Baptista, A. F., De Araújo, A. N., Do Rosário, R. S., Miranda, J. G. V., Montoya, P., et al. (2015a). A randomized, double-blind, sham-controlled trial of transcranial direct current stimulation in attention-deficit/hyperactivity disorder. PLoS One 10:e0135371. doi: 10.1371/journal.pone.0135371
Cosmo, C., Ferreira, C., Miranda, J. G. V., do Rosário, R. S., do, Baptista, A. F., et al. (2015b). Spreading effect of tDCS in individuals with attention-deficit/hyperactivity disorder as shown by functional cortical networks: a randomized, double-blind, sham-controlled trial. Front. Psychiatry 6:111. doi: 10.3389/fpsyt.2015.00111
D’Agata, F., Peila, E., Cicerale, A., Caglio, M. M., Caroppo, P., Vighetti, S., et al. (2016). Cognitive and neurophysiological effects of non-invasive brain stimulation in stroke patients after motor rehabilitation. Front. Behav. Neurosci. 10:135. doi: 10.3389/fnbeh.2016.00135
Dallmer-Zerbe, I., Popp, F., Lam, A. P., Philipsen, A., and Herrmann, C. S. (2020). Transcranial alternating current stimulation (tacs) as a tool to modulate p300 amplitude in attention deficit hyperactivity disorder (ADHD): preliminary findings. Brain Topogr. 33, 191–207. doi: 10.1007/s10548-020-00752-x
Davis, S. E., and Smith, G. A. (2019). Transcranial direct current stimulation use in warfighting: benefits, risks, and future prospects. Front. Hum. Neurosci. 13:114. doi: 10.3389/fnhum.2019.00114
Del Felice, A., Castiglia, L., Formaggio, E., Cattelan, M., Scarpa, B., Manganotti, P., et al. (2019). Personalized transcranial alternating current stimulation (tACS) and physical therapy to treat motor and cognitive symptoms in Parkinson’s disease: a randomized cross-over trial. NeuroImage Clin. 22:101768. doi: 10.1016/j.nicl.2019.101768
Del Felice, A., Magalini, A., and Masiero, S. (2015). Slow-oscillatory transcranial direct current stimulation modulates memory in temporal lobe epilepsy by altering sleep spindle generators: a possible rehabilitation tool. Brain Stimul. 8, 567–573. doi: 10.1016/j.brs.2015.01.410
Deng, F., Yang, H. L., and Wang, L. J. (2019). Adaptive unscented Kalman filter based estimation and filtering for dynamic positioning with model uncertainties. Int. J. Control. Autom. Syst. 17, 667–678. doi: 10.1007/s12555-018-9503-4
Devinsky, O., Vezzani, A., O’Brien, T. J., Jette, N., Scheffer, I. E., De Curtis, M., et al. (2018). Epilepsy. Nat. Rev. Dis. Prim. 4:18024. doi: 10.1038/nrdp.2018.24
Dominguez, A., Socas, R., Marrero, H., Leon, N., LLabres, J., and Enriquez, E. (2014). Transcranial direct current stimulation improves word production in conduction aphasia: electroencephalographic and behavioral evidences. Int. J. Clin. Heal. Psychol. 14, 240–245. doi: 10.1016/j.ijchp.2014.02.001
Duan, X., Xie, S., Xie, X., Meng, Y., and Xu, Z. (2019). Quadcopter flight control using a non-invasive multi-modal brain computer interface. Front. Neurorobot. 13:23. doi: 10.3389/fnbot.2019.00023
Dubreuil-Vall, L., Chau, P., Ruffini, G., Widge, A. S., and Camprodon, J. A. (2019). tDCS to the left DLPFC modulates cognitive and physiological correlates of executive function in a state-dependent manner. Brain Stimul. 12, 1456–1463. doi: 10.1016/j.brs.2019.06.006
Dunn, W., Rassovsky, Y., Wynn, J. K., Wu, A. D., Iacoboni, M., Hellemann, G., et al. (2016). Modulation of neurophysiological auditory processing measures by bilateral transcranial direct current stimulation in schizophrenia. Schizophr. Res. 174, 189–191. doi: 10.1016/j.schres.2016.04.021
Dutta, A., Jacob, A., Chowdhury, S. R., Das, A., and Nitsche, M. A. (2015). EEG-NIRS based assessment of neurovascular coupling during anodal transcranial direct current stimulation - a stroke case series. J. Med. Syst. 39:205. doi: 10.1007/s10916-015-0205-7
Emonson, M. R. L., Fitzgerald, P. B., Rogasch, N. C., and Hoy, K. E. (2019). Neurobiological effects of transcranial direct current stimulation in younger adults, older adults and mild cognitive impairment. Neuropsychologia 125, 51–61. doi: 10.1016/j.neuropsychologia.2019.01.003
Ermolova, M., Belyaeva, V., Novikov, N., Gutkin, B., Feurra, M., and Fedele, T. (2019). Changes in neuronal oscillations account for working memory dynamics: EEG-tACS study. Brain Stimul. 12:e168. doi: 10.1016/j.brs.2019.06.007
Fan, Y., Chen, J., Song, C., and Wang, Y. (2020). Event-triggered coordination control for multi-agent systems with connectivity preservation. Int. J. Control. Autom. Syst. 18, 966–979. doi: 10.1007/s12555-018-0700-y
Faria, P., Fregni, F., Sebastião, F., Dias, A. I., and Leal, A. (2012). Feasibility of focal transcranial DC polarization with simultaneous EEG recording: preliminary assessment in healthy subjects and human epilepsy. Epilepsy Behav. 25, 417–425. doi: 10.1016/j.yebeh.2012.06.027
Feigin, V. L., Nichols, E., Alam, T., Bannick, M. S., Beghi, E., Blake, N., et al. (2019). Global, regional, and national burden of neurological disorders, 1990–2016: a systematic analysis for the Global Burden of Disease Study 2016. Lancet Neurol. 18, 459–480. doi: 10.1016/S1474-4422(18)30499-X
Feigin, V. L., Vos, T., Nichols, E., Owolabi, M. O., Carroll, W. M., Dichgans, M., et al. (2020). The global burden of neurological disorders: translating evidence into policy. Lancet Neurol. 19, 255–265. doi: 10.1016/S1474-4422(19)30411-9
Feng, W., Halm-Lutterodt, N., Van, Tang, H., Mecum, A., Mesregah, M. K., et al. (2020). Automated RI-based deep learning model for detection of Alzheimer’s disease process. Int. J. Neural Syst. 30:2050032. doi: 10.1142/S012906572050032X
Ferguson, B. R., and Gao, W. J. (2018). Pv interneurons: critical regulators of E/I balance for prefrontal cortex-dependent behavior and psychiatric disorders. Front. Neural Circuits 12:37. doi: 10.3389/fncir.2018.00037
Ferrucci, R., Mrakic-Sposta, S., Gardini, S., Ruggiero, F., Vergari, M., Mameli, F., et al. (2018). Behavioral and neurophysiological effects of transcranial direct current stimulation (tDCS) in fronto-temporal dementia. Front. Behav. Neurosci. 12:235. doi: 10.3389/fnbeh.2018.00235
Fertonani, A., Pirulli, C., and Miniussi, C. (2011). Random noise stimulation improves neuroplasticity in perceptual learning. J. Neurosci. 31, 15416–15423. doi: 10.1523/JNEUROSCI.2002-11.2011
Fregni, F., and Pascual-Leone, A. (2007). Technology insight: non-invasive brain stimulation in neurology - perspectives on the therapeutic potential of rTMS and tDCS. Nat. Clin. Pract. Neurol. 3, 383–393. doi: 10.1038/ncpneuro0530
Fregni, F., Thome-Souza, S., Nitsche, M. A., Freedman, S. D., Valente, K. D., and Pascual-Leone, A. (2006). A controlled clinical trial of cathodal DC polarization in patients with refractory epilepsy. Epilepsia 47, 335–342. doi: 10.1111/j.1528-1167.2006.00426.x
Fröhlich, F., and McCormick, D. A. (2010). Endogenous electric fields may guide neocortical network activity. Neuron 67, 129–143. doi: 10.1016/j.neuron.2010.06.005
Gangemi, A., Colombo, B., and Fabio, R. A. (2020). Effects of short- and long-term neurostimulation (tDCS) on Alzheimer’s disease patients: two randomized studies. Aging Clin. Exp. Res. 33, 383–390. doi: 10.1007/s40520-020-01546-8
Ghafoor, U., Lee, J. H., Hong, K.-S., Park, S. S., Kim, J., and Yoo, H. R. (2019). Effects of acupuncture therapy on MCI patients using functional near-infrared spectroscopy. Front. Aging Neurosci. 11:237. doi: 10.3389/fnagi.2019.00237
Górriz, J. M., Ramírez, J., Segovia, F., Martínez, F. J., Lai, M. C., Lombardo, M. V., et al. (2019). A machine learning approach to reveal the neurophenotypes of autisms. Int. J. Neural Syst. 29:1850058. doi: 10.1142/S0129065718500582
Hong, K.-S., Ghafoor, U., and Khan, M. J. (2020). Brain–machine interfaces using functional near-infrared spectroscopy: a review. Artif. Life Robot. 25, 204–218. doi: 10.1007/s10015-020-00592-9
Hong, K.-S., and Khan, M. J. (2017). Hybrid brain-computer interface techniques for improved classification accuracy and increased number of commands: a review. Front. Neurorobot. 11:35. doi: 10.3389/fnbot.2017.00035
Hong, K.-S., and Pham, P. T. (2019). Control of axially moving systems: a review. Int. J. Control. Autom. Syst. 17, 2983–3008. doi: 10.1007/s12555-019-0592-5
Hong, K.-S., and Yaqub, M. A. (2019). Application of functional near-infrared spectroscopy in the healthcare industry: a review. J. Innov. Opt. Health Sci. 12:1930012. doi: 10.1142/S179354581930012X
Hordacre, B., Moezzi, B., and Ridding, M. C. (2018). Neuroplasticity and network connectivity of the motor cortex following stroke: a transcranial direct current stimulation study. Hum. Brain Mapp. 39, 3326–3339. doi: 10.1002/hbm.24079
Hoy, K. E., Bailey, N. W., Arnold, S. L., and Fitzgerald, P. B. (2015). The effect of transcranial direct current stimulation on gamma activity and working memory in schizophrenia. Psychiatry Res. 228, 191–196. doi: 10.1016/j.psychres.2015.04.032
Hu, X., Zhuang, C., Wang, F., Liu, Y. J., Im, C. H., and Zhang, D. (2019). FNIRS evidence for recognizably different positive emotions. Front. Hum. Neurosci. 13:120. doi: 10.3389/fnhum.2019.00120
Impey, D., Baddeley, A., Nelson, R., Labelle, A., and Knott, V. (2017). Effects of transcranial direct current stimulation on the auditory mismatch negativity response and working memory performance in schizophrenia: a pilot study. J. Neural Transm. 124, 1489–1501. doi: 10.1007/s00702-017-1783-y
Inukai, Y., Saito, K., Sasaki, R., Tsuiki, S., Miyaguchi, S., Kojima, S., et al. (2016). Comparison of three non-invasive transcranial electrical stimulation methods for increasing cortical excitability. Front. Hum. Neurosci. 10:668. doi: 10.3389/fnhum.2016.00668
Irani, F., Platek, S. M., Bunce, S., Ruocco, A. C., and Chute, D. (2007). Functional near infrared spectroscopy (fNIRS): an emerging neuroimaging technology with important applications for the study of brain disorders. Clin. Neuropsychol. 21, 9–37. doi: 10.1080/13854040600910018
Jahshan, C., Wynn, J. K., Roach, B. J., Mathalon, D. H., and Green, M. F. (2020). Effects of transcranial direct current stimulation on visual neuroplasticity in schizophrenia. Clin. EEG Neurosci. 51, 382–389. doi: 10.1177/1550059420925697
Jindal, U., Sood, M., Dutta, A., and Chowdhury, S. R. (2015). Development of point of care testing device for neurovascular coupling from simultaneous recording of EEG and NIRS during anodal transcranial direct current stimulation. IEEE J. Transl. Eng. Heal. Med. 3:2000112. doi: 10.1109/JTEHM.2015.2389230
Kang, J., Cai, E., Han, J., Tong, Z., Li, X., Sokhadze, E. M., et al. (2018). Transcranial direct current stimulation (tDCS) can modulate EEG complexity of children with autism spectrum disorder. Front. Neurosci. 12, AN:201. doi: 10.3389/fnins.2018.00201
Karvigh, S. A., Motamedi, M., Arzani, M., and Roshan, J. H. N. (2017). HD-tDCS in refractory lateral frontal lobe epilepsy patients. Seizure 47, 74–80. doi: 10.1016/j.seizure.2017.03.005
Kasashima, Y., Fujiwara, T., Matsushika, Y., Tsuji, T., Hase, K., Ushiyama, J., et al. (2012). Modulation of event-related desynchronization during motor imagery with transcranial direct current stimulation (tDCS) in patients with chronic hemiparetic stroke. Exp. Brain Res. 221, 263–268. doi: 10.1007/s00221-012-3166-9
Kasashima-Shindo, Y., Fujiwara, T., Ushiba, J., Matsushika, Y., Kamatani, D., Oto, M., et al. (2015). Brain-computer interface training combined with transcranial direct current stimulation in patients with chronic severe hemiparesis: proof of concept study. J. Rehabil. Med. 47, 318–324. doi: 10.2340/16501977-1925
Kayarian, F. B., Jannati, A., Rotenberg, A., and Santarnecchi, E. (2020). Targeting gamma−related pathophysiology in autism spectrum disorder using transcranial electrical stimulation: opportunities and challenges. Autism Res. 13, 1051–1071. doi: 10.1002/aur.2312
Khan, M. J., and Hong, K.-S. (2017). Hybrid EEG-FNIRS-based eight-command decoding for BCI: application to quadcopter control. Front. Neurorobot. 11:6. doi: 10.3389/fnbot.2017.00006
Khayyer, Z., Ngaosuvan, L., Sikström, S., and Ghaderi, A. H. (2018). Transcranial direct current stimulation based on quantitative electroencephalogram combining positive psychotherapy for major depression. J. Integr. Neurosci. 17, 141–155. doi: 10.3233/JIN-170045
Kim, E., Meinhold, W., Shinohara, M., and Ueda, J. (2020). Statistical inter-stimulus interval window estimation for transient neuromodulation via paired mechanical and brain stimulation. Front. Neurorobot. 14:1. doi: 10.3389/fnbot.2020.00001
Kim, M., Kim, M. K., Hwang, M., Kim, H. Y., Cho, J., and Kim, S. P. (2019). Online home appliance control using EEG-Based brain–computer interfaces. Electronics 8:1101. doi: 10.3390/electronics8101101
Kim, S. Y., Kang, C. H., and Park, C. G. (2020). Multiple frequency tracking and mitigation based on RSPWVD and adaptive multiple linear kalman notch filter. Int. J. Control. Autom. Syst. 18, 1139–1149. doi: 10.1002/navi.332
Kwon, J., Shin, J., and Im, C. H. (2020). Toward a compact hybrid brain-computer interface (BCI): performance evaluation of multi-class hybrid EEG-fNIRS BCIs with limited number of channels. PLoS One 15:e0230491. doi: 10.1371/journal.pone.0230491
Ladenbauer, J., Ladenbauer, J., Külzowä, N., De Boor, R., Avramova, E., Grittner, U., et al. (2017). Promoting sleep oscillations and their functional coupling by transcranial stimulation enhances memory consolidation in mild cognitive impairment. J. Neurosci. 37, 7111–7124. doi: 10.1523/JNEUROSCI.0260-17.2017
Lee, S., Lee, T., Yang, T., Yoon, C., and Kim, S. P. (2020). Detection of drivers’ anxiety invoked by driving situations using multimodal biosignals. Processes 8:155. doi: 10.3390/pr8020155
Lee, T., Kim, M., and Kim, S. (2020). Improvement of P300-based brain–computer interfaces for home appliances control by data balancing techniques. Sensors 20:5576. doi: 10.3390/s20195576
Li, C. T., Chen, M. H., Juan, C. H., Liu, R. S., Lin, W. C., Bai, Y. M., et al. (2018). Effects of prefrontal theta-burst stimulation on brain function in treatment-resistant depression: a randomized sham-controlled neuroimaging study. Brain Stimul. 11, 1054–1062. doi: 10.1016/j.brs.2018.04.014
Li, H., Zhu, N., Klomparens, E. A., Xu, S., Wang, M., Wang, Q., et al. (2019). Application of functional near-infrared spectroscopy to explore the neural mechanism of transcranial direct current stimulation for post-stroke depression. Neurol. Res. 41, 714–721. doi: 10.1080/01616412.2019.1612539
Lin, L. C., Ouyang, C., Sen, Chiang, C. T., Yang, R. C., Wu, R. C., et al. (2018). Cumulative effect of transcranial direct current stimulation in patients with partial refractory epilepsy and its association with phase lag index-A preliminary study. Epilepsy Behav. 84, 142–147. doi: 10.1016/j.yebeh.2018.04.017
Liu, A., Bryant, A., Jefferson, A., Friedman, D., Minhas, P., Barnard, S., et al. (2016). Exploring the efficacy of a 5-day course of transcranial direct current stimulation (TDCS) on depression and memory function in patients with well-controlled temporal lobe epilepsy. Epilepsy Behav. 55, 11–20. doi: 10.1016/j.yebeh.2015.10.032
Lv, X., Fang, Y., Mao, Z., Jiang, B., and Qi, R. (2021). Fault detection for a class of closed-loop hypersonic vehicle system via hypothesis test method. Int. J. Control. Autom. Syst. 19, 350–362. doi: 10.1007/s12555-019-0906-7
Mane, R., Chew, E., Phua, K. S., Ang, K. K., Robinson, N., Vinod, A. P., et al. (2019). Prognostic and monitory EEG-biomarkers for BCI upper-limb stroke rehabilitation. IEEE Trans. Neural Syst. Rehabil. Eng. 27, 1654–1664. doi: 10.1109/TNSRE.2019.2924742
Marceglia, S., Mrakic-Sposta, S., Rosa, M., Ferrucci, R., Mameli, F., Vergari, M., et al. (2016). Transcranial direct current stimulation modulates cortical neuronal activity in Alzheimer’s disease. Front. Neurosci. 10:134. doi: 10.3389/fnins.2016.00134
Meiron, O., Gale, R., Namestnic, J., Bennet-Back, O., David, J., Gebodh, N., et al. (2018). High-definition transcranial direct current stimulation in early onset epileptic encephalopathy: a case study. Brain Inj. 32, 135–143. doi: 10.1080/02699052.2017.1390254
Meiron, O., Gale, R., Namestnic, J., Bennet-Back, O., Gebodh, N., Esmaeilpour, Z., et al. (2019). Antiepileptic effects of a novel non-invasive neuromodulation treatment in a subject with early-onset epileptic encephalopathy: case report with 20 sessions of HDTDCS intervention. Front. Neurosci. 13:547. doi: 10.3389/fnins.2019.00547
Naro, A., Corallo, F., De Salvo, S., Marra, A., Di Lorenzo, G., Muscarà, N., et al. (2016). Promising role of neuromodulation in predicting the progression of mild cognitive impairment to dementia. J. Alzheimer’s Dis. 53, 1375–1388. doi: 10.3233/JAD-160305
Naros, G., and Gharabaghi, A. (2017). Physiological and behavioral effects of β-tACS on brain self-regulation in chronic stroke. Brain Stimul. 10, 251–259. doi: 10.1016/j.brs.2016.11.003
Naseer, N., and Hong, K.-S. (2013). Classification of functional near-infrared spectroscopy signals corresponding to the right- and left-wrist motor imagery for development of a brain-computer interface. Neurosci. Lett. 553, 84–89. doi: 10.1016/j.neulet.2013.08.021
Naseer, N., and Hong, K.-S. (2015). fNIRS-based brain-computer interfaces: a review. Front. Hum. Neurosci. 9:3. doi: 10.3389/fnhum.2015.00003
Nguyen, H.-D., Hong, K.-S., and Shin, Y.-I. (2016). Bundled-optode method in functional near-infrared spectroscopy. PLoS One 11:e0165146. doi: 10.1371/journal.pone.0165146
Nicolo, P., Magnin, C., Pedrazzini, E., Plomp, G., Mottaz, A., Schnider, A., et al. (2018). Comparison of Neuroplastic responses to cathodal transcranial direct current stimulation and continuous theta burst stimulation in subacute stroke. Arch. Phys. Med. Rehabil. 99, 862.e1–872.e1. doi: 10.1016/j.apmr.2017.10.026
Nikolin, S., Martin, D., Loo, C. K., Iacoviello, B. M., and Boonstra, T. W. (2020). Assessing neurophysiological changes associated with combined transcranial direct current stimulation and cognitive-emotional training for treatment-resistant depression. Eur. J. Neurosci. 51, 2119–2133. doi: 10.1111/ejn.14656
Nishida, K., Koshikawa, Y., Morishima, Y., Yoshimura, M., Katsura, K., Ueda, S., et al. (2019). Pre-stimulus brain activity is associated with state-anxiety changes during single-session transcranial direct current stimulation. Front. Hum. Neurosci. 13:266. doi: 10.3389/fnhum.2019.00266
Nitsche, M. A., Cohen, L. G., Wassermann, E. M., Priori, A., Lang, N., Antal, A., et al. (2008). Transcranial direct current stimulation: state of the art 2008. Brain Stimul. 1, 206–223. doi: 10.1016/j.brs.2008.06.004
Nowak, M., Hinson, E., Van Ede, F., Pogosyan, A., Guerra, A., Quinn, A., et al. (2017). Driving human motor cortical oscillations leads to behaviorally relevant changes in local GABAA inhibition: a tACS-TMS study. J. Neurosci. 37, 4481–4492. doi: 10.1523/JNEUROSCI.0098-17.2017
Olejarczyk, E., Zuchowicz, U., Wozniak-Kwasniewska, A., Kaminski, M., Szekely, D., and David, O. (2020). The impact of repetitive transcranial magnetic stimulation on functional connectivity in major depressive disorder and bipolar disorder evaluated by directed transfer function and indices based on graph theory. Int. J. Neural Syst. 30:2050015. doi: 10.1142/S012906572050015X
O’Neil-Pirozzi, T. M., Doruk, D., Thomson, J. M., and Fregni, F. (2017). Immediate memory and electrophysiologic effects of prefrontal cortex transcranial direct current stimulation on neurotypical individuals and individuals with chronic traumatic brain injury: a pilot study. Int. J. Neurosci. 127, 592–600. doi: 10.1080/00207454.2016.1216415
Palm, U., Keeser, D., Schiller, C., Fintescu, Z., Reisinger, E., Baghai, T. C., et al. (2009). Transcranial direct current stimulation in a patient with therapy-resistant major depression. World J. Biol. Psychiatry 10, 632–635. doi: 10.1080/15622970802480905
Park, B. J., Pham, P. T., and Hong, K. S. (2020). Model reference robust adaptive control of control element drive mechanism in a nuclear power plant. Int. J. Control. Autom. Syst. 18, 1651–1661. doi: 10.1007/s12555-019-0987-3
Pasquinelli, C., Hanson, L. G., Siebner, H. R., Lee, H. J., and Thielscher, A. (2019). Safety of transcranial focused ultrasound stimulation: a systematic review of the state of knowledge from both human and animal studies. Brain Stimul. 12, 1367–1380. doi: 10.1016/j.brs.2019.07.024
Patel, K. R., Cherian, J., Gohil, K., and Atkinson, D. (2014). Schizophrenia: overview and treatment options. P T 39, 638–645.
Polanía, R., Nitsche, M. A., and Ruff, C. C. (2018). Studying and modifying brain function with non-invasive brain stimulation. Nat. Neurosci. 21, 174–187. doi: 10.1038/s41593-017-0054-4
Posner, J., Polanczyk, G. V., and Sonuga-Barke, E. (2020). Attention-deficit hyperactivity disorder. Lancet 395, 450–462. doi: 10.1016/S0140-6736(19)33004-1
Powell, T. Y., Boonstra, T. W., Martin, D. M., Loo, C. K., and Breakspear, M. (2014). Modulation of cortical activity by transcranial direct current stimulation in patients with affective disorder. PLoS One 9:0098503. doi: 10.1371/journal.pone.0098503
Rashid, M., Sulaiman, N., Abdul Majeed, A., Musa, R. M., Ahmad, A. F., Bari, B. S., et al. (2020). Current status, challenges, and possible solutions of EEG-based brain-computer interface: a comprehensive review. Front. Neurorobot. 14:25. doi: 10.3389/fnbot.2020.00025
Rassovsky, Y., Dunn, W., Wynn, J. K., Wu, A. D., Iacoboni, M., Hellemann, G., et al. (2018). Single transcranial direct current stimulation in schizophrenia: randomized, cross-over study of neurocognition, social cognition. ERPs, and side effects. PLoS One 13:e0197023. doi: 10.1371/journal.pone.0197023
Reed, T., and Cohen Kadosh, R. (2018). Transcranial electrical stimulation (tES) mechanisms and its effects on cortical excitability and connectivity. J. Inherit. Metab. Dis. 41, 1123–1130. doi: 10.1007/s10545-018-0181-4
Reinhart, R. M. G., Zhu, J., Park, S., and Woodman, G. F. (2015). Synchronizing theta oscillations with direct-current stimulation strengthens adaptive control in the human brain. Proc. Natl. Acad. Sci. U.S.A. 112, 9448–9453. doi: 10.1073/pnas.1504196112
San-Juan, D., Espinoza López, D. A., Vázquez Gregorio, R., Trenado, C., Fernández-González Aragón, M., Morales-Quezada, L., et al. (2017). Transcranial direct current stimulation in mesial temporal lobe epilepsy and hippocampal sclerosis. Brain Stimul. 10, 28–35. doi: 10.1016/j.brs.2016.08.013
San-Juan, D., Sarmiento, C. I., Hernandez-Ruiz, A., Elizondo-Zepeda, E., Santos-Vázquez, G., Reyes-Acevedo, G., et al. (2016). Transcranial alternating current stimulation: a potential risk for genetic generalized epilepsy patients (study case). Front. Neurol. 7:213. doi: 10.3389/fneur.2016.00213
Scheffer, M., Bascompte, J., Brock, W. A., Brovkin, V., Carpenter, S. R., Dakos, V., et al. (2009). Early-warning signals for critical transitions. Nature 461, 53–59. doi: 10.1038/nature08227
Schoellmann, A., Scholten, M., Wasserka, B., Govindan, R. B., Krüger, R., Gharabaghi, A., et al. (2019). Anodal tDCS modulates cortical activity and synchronization in Parkinson’s disease depending on motor processing. NeuroImage Clin. 22:101689. doi: 10.1016/j.nicl.2019.101689
Schulz, R., Gerloff, C., and Hummel, F. C. (2013). Non-invasive brain stimulation in neurological diseases. Neuropharmacology 64, 579–587. doi: 10.1016/j.neuropharm.2012.05.016
Shafi, M. M., Westover, M. B., Fox, M. D., and Pascual-Leone, A. (2012). Exploration and modulation of brain network interactions with non-invasive brain stimulation in combination with neuroimaging. Eur. J. Neurosci. 35, 805–825. doi: 10.1111/j.1460-9568.2012.08035.x
Shahsavar, Y., Ghoshuni, M., and Talaei, A. (2018). Quantifying clinical improvements in patients with depression under the treatment of transcranial direct current stimulation using event related potentials. Australas. Phys. Eng. Sci. Med. 41, 973–983. doi: 10.1007/s13246-018-0696-x
Shin, J. (2020). Random subspace ensemble learning for functional near-infrared spectroscopy brain-computer interfaces. Front. Hum. Neurosci. 14:236. doi: 10.3389/fnhum.2020.00236
Smith, R. C., Boules, S., Mattiuz, S., Youssef, M., Tobe, R. H., Sershen, H., et al. (2015). Effects of transcranial direct current stimulation (tDCS) on cognition, symptoms, and smoking in schizophrenia: a randomized controlled study. Schizophr. Res. 168, 260–266. doi: 10.1016/j.schres.2015.06.011
Singh, A., Trapp, N. T., De Corte, B., Cao, S., Kingyon, J., Boes, A. D., et al. (2019). Cerebellar theta frequency transcranial pulsed stimulation increases frontal theta oscillations in patients with schizophrenia. Cerebellum 18, 489–499. doi: 10.1007/s12311-019-01013-9
Song, Y., Park, J., Lee, K. C., and Lee, S. (2019). Reducing the design complexity of automated vehicle electrical and electronic systems using a cyber-physical system concept. Int. J. Control. Autom. Syst. 17, 500–508. doi: 10.1007/s12555-018-0246-z
Straudi, S., Bonsangue, V., Mele, S., Craighero, L., Montis, A., Fregni, F., et al. (2019). Bilateral M1 anodal transcranial direct current stimulation in post traumatic chronic minimally conscious state: a pilot EEG-tDCS study. Brain Inj. 33, 490–495. doi: 10.1080/02699052.2019.1565894
Sugata, H., Yagi, K., Yazawa, S., Nagase, Y., Tsuruta, K., Ikeda, T., et al. (2018). Modulation of motor learning capacity by transcranial alternating current stimulation. Neuroscience 391, 131–139. doi: 10.1016/j.neuroscience.2018.09.013
Tanveer, M. A., Khan, M. J., Qureshi, M. J., Naseer, N., and Hong, K. S. (2019). Enhanced drowsiness detection using deep learning: an fNIRS Study. IEEE Access. 7, 137920–137929. doi: 10.1109/ACCESS.2019.2942838
Tecchio, F., Cottone, C., Porcaro, C., Cancelli, A., Lazzaro, V., and Di et al (2018). Brain functional connectivity changes after transcranial direct current stimulation in epileptic patients. Front. Neural Circuits 12:044. doi: 10.3389/fncir.2018.00044
Tekturk, P., Erdogan, E. T., Kurt, A., Vanli-yavuz, E. N., Ekizoglu, E., Kocagoncu, E., et al. (2016). The effect of transcranial direct current stimulation on seizure frequency of patients with mesial temporal lobe epilepsy with hippocampal sclerosis. Clin. Neurol. Neurosurg. 149, 27–32. doi: 10.1016/j.clineuro.2016.07.014
Tortora, S., Tonin, L., Chisari, C., Micera, S., Menegatti, E., and Artoni, F. (2020). Hybrid human-machine interface for gait decoding through Bayesian fusion of EEG and EMG classifiers. Front. Neurorobot. 14:582728. doi: 10.3389/fnbot.2020.582728
Ulam, F., Shelton, C., Richards, L., Davis, L., Hunter, B., Fregni, F., et al. (2015). Cumulative effects of transcranial direct current stimulation on EEG oscillations and attention/working memory during subacute neurorehabilitation of traumatic brain injury. Clin. Neurophysiol. 126, 486–496. doi: 10.1016/j.clinph.2014.05.015
Van Doren, J., Langguth, B., and Schecklmann, M. (2014). Electroencephalographic effects of transcranial random noise stimulation in the auditory cortex. Brain Stimul. 7, 807–812. doi: 10.1016/j.brs.2014.08.007
Vosskuhl, J., Strüber, D., and Herrmann, C. S. (2018). Non-invasive brain stimulation: a paradigm shift in understanding brain oscillations. Front. Hum. Neurosci. 12:211. doi: 10.3389/fnhum.2018.00211
Vuksanović, V., Staff, R. T., Ahearn, T., Murray, A. D., and Wischik, C. M. (2019). Cortical thickness and surface area networks in healthy aging, Alzheimer’s disease and behavioral variant fronto-temporal dementia. Int. J. Neural Syst. 29:1850055. doi: 10.1142/S0129065718500557
Wu, D., Wang, J., and Yuan, Y. (2015). Effects of transcranial direct current stimulation on naming and cortical excitability in stroke patients with aphasia. Neurosci. Lett. 589, 115–120. doi: 10.1016/j.neulet.2015.01.045
Xu, H., Ding, F., Alsaedi, A., and Hayat, T. (2019). Recursive identification algorithms for a class of linear closed-loop systems. Int. J. Control Autom. Syst. 17, 3194–3204. doi: 10.1007/s12555-018-0640-6
Xu, J., Wang, J., Bai, T., Zhang, X., Li, T., Hu, Q., et al. (2019). Electroconvulsive therapy induces cortical morphological alterations in major depressive disorder revealed with surface-based morphometry analysis. Int. J. Neural Syst. 29:1950005. doi: 10.1142/S0129065719500059
Yang, D., Du, Q., Huang, Z., Li, L., Zhang, Z., Zhang, L., et al. (2019a). Transcranial direct current stimulation for patients with pharmacoresistant epileptic spasms: a pilot study. Front. Neurol. 10:50. doi: 10.3389/fneur.2019.00050
Yang, D., Hong, K.-S., Yoo, S.-H., and Kim, C.-S. (2019b). Evaluation of neural degeneration biomarkers in the prefrontal cortex for early identification of patients with mild cognitive impairment: an fNIRS study. Front. Hum. Neurosci. 13:317. doi: 10.3389/FNHUM.2019.00317
Yang, D., Huang, R., Yoo, S. H., Shin, M. J., Yoon, J. A., and Shin, Y. II, et al (2020a). Detection of mild cognitive impairment using convolutional neural network: temporal-feature maps of functional near-infrared spectroscopy. Front. Aging Neurosci. 12:141. doi: 10.3389/fnagi.2020.00141
Yang, D., Nguyen, T.-H., and Chung, W. (2020b). A bipolar-channel hybrid brain-computer interface system for home automation control utilizing steady-state visually evoked potential and eye-blink signals. Sensors 20:5474. doi: 10.3390/s20195474
Yaqub, M. A., Woo, S., and Hong, K. (2018). Effects of HD-tDCS on resting-state functional connectivity in the prefrontal cortex?: an fNIRS study. Complexity 2018:1613402.
Yin, Z., Luo, Y., Jin, Y., Yu, Y., Zheng, S., Duan, J., et al. (2019). Is awake physiological confirmation necessary for DBS treatment of Parkinson’s disease today? A comparison of intraoperative imaging, physiology, and physiology imaging-guided DBS in the past decade. Brain Stimul. 12, 893–900. doi: 10.1016/j.brs.2019.03.006
Yoo, W. K., Vernet, M., Hoon Kim, J., Brem, A. K., Bashir, S., Ifert-Miller, F., et al. (2020). Interhemispheric and intrahemispheric connectivity from the left pars opercularis within the language network is modulated by transcranial stimulation in healthy subjects. Front. Hum. Neurosci. 14:63. doi: 10.3389/fnhum.2020.00063
Yoo, Y. J. (2019). Fault detection method using multi-mode principal component analysis based on gaussian mixture model for sewage source heat pump system. Int. J. Control Autom. Syst. 17, 2125–2134. doi: 10.1007/s12555-018-0758-6
Yuan, Y., Wang, J., Wu, D., Huang, X., and Song, W. (2017). Effect of transcranial direct current stimulation on swallowing apraxia and cortical excitability in stroke patients. Top. Stroke Rehabil. 24, 503–509. doi: 10.1080/10749357.2017.1322250
Zhang, J., Wang, B., Zhang, C., Xiao, Y., and Wang, M. Y. (2019). An EEG/EMG/EOG-based multimodal human-machine interface to real-time control of a soft robot hand. Front. Neurorobot. 13:7. doi: 10.3389/fnbot.2019.00007
Keywords: transcranial electrical stimulation, transcranial direct current stimulation, transcranial alternation stimulation, transcranial random noise stimulation, electroencephalography, functional near-infrared spectroscopy, brain disease
Citation: Yang D, Shin Y-I and Hong K-S (2021) Systemic Review on Transcranial Electrical Stimulation Parameters and EEG/fNIRS Features for Brain Diseases. Front. Neurosci. 15:629323. doi: 10.3389/fnins.2021.629323
Received: 14 November 2020; Accepted: 25 February 2021;
Published: 26 March 2021.
Edited by:
Oscar Arias-Carrion, Hospital General Dr. Manuel Gea Gonzalez, MexicoReviewed by:
Chia-Wei Sun, National Chiao Tung University, TaiwanEstate M. Sokhadze, University of South Carolina, United States
Copyright © 2021 Yang, Shin and Hong. This is an open-access article distributed under the terms of the Creative Commons Attribution License (CC BY). The use, distribution or reproduction in other forums is permitted, provided the original author(s) and the copyright owner(s) are credited and that the original publication in this journal is cited, in accordance with accepted academic practice. No use, distribution or reproduction is permitted which does not comply with these terms.
*Correspondence: Keum-Shik Hong, a3Nob25nQHB1c2FuLmFjLmty