- 1Institute for Biology, Freie Universität Berlin, Berlin, Germany
- 2Neuroscience Research Center, Charité - Universitätsmedizin Berlin, Berlin, Germany
- 3Bernstein Center for Computational Neuroscience Berlin, Berlin, Germany
- 4Institute for Theoretical Biology, Humboldt-Universität zu Berlin, Berlin, Germany
- 5Einstein Center for Neurosciences Berlin, Berlin, Germany
Among the different autism spectrum disorders, Fragile X syndrome (FXS) is the most common inherited cause of intellectual disability. Sensory and especially auditory hypersensitivity is a key symptom in patients, which is well mimicked in the Fmr1 -/- mouse model. However, the physiological mechanisms underlying FXS’s acoustic hypersensitivity in particular remain poorly understood. Here, we categorized spike response patterns to pure tones of different frequencies and intensities from neurons in the inferior colliculus (IC), a central integrator in the ascending auditory pathway. Based on this categorization we analyzed differences in response patterns between IC neurons of wild-type (WT) and Fmr1 -/- mice. Our results report broadening of frequency tuning, an increased firing in response to monaural as well as binaural stimuli, an altered balance of excitation-inhibition, and reduced response latencies, all expected features of acoustic hypersensitivity. Furthermore, we noticed that all neuronal response types in Fmr1 -/- mice displayed enhanced offset-rebound activity outside their excitatory frequency response area. These results provide evidence that the loss of Fmr1 not only increases spike responses in IC neurons similar to auditory brainstem neurons, but also changes response patterns such as offset spiking. One can speculate this to be an underlying aspect of the receptive language problems associated with Fragile X syndrome.
Introduction
Fragile X syndrome (FXS) is a major cause for intellectual disability (Ciaccio et al., 2017), affecting approximately 1 in 4000 males and 1 in 6000 females (Turner et al., 1996). It originates from an over expansion of the CGG triplet repeats on the Fragile X messenger ribonucleoprotein 1 (Fmr1) gene located on the X chromosome. This CGG over expansion leads to a loss of Fragile X messenger RibonucleoProtein (FMRP) resulting in a dysfunctional neuronal development. Patients with FXS display multiple abnormalities, including phenotypic features (e.g., protruding ears and macroorchidism), hypersensitivity to different sensory modalities, language retardation and social withdrawal (Finestack et al., 2009; Rais et al., 2018). A consensus has emerged that the animal model of Fragile X, the Fmr1 -/- mouse, recapitulates most neurologic symptoms of FXS patients, with hypersensitivity to sounds and the development of audiogenic seizures as one of the key sensory dysfunctions (Dolan et al., 2013). Enhanced population activity in combination with reduced habituation of auditory cortical neurons has been associated with the auditory hypersensitivity observed in patients with FXS and the FXS mouse model (Razak et al., 2021).
Audiogenic seizures in rodents originate in part from overactivity in the inferior colliculus (IC) (Ribak and Morin, 1995; Ribak, 2017), which is a prominent midbrain nucleus and a central hub for sound processing. Lower auditory brainstem nuclei, including the cochlear nucleus (CN), the lateral superior olive (LSO), and the nuclei of the lateral lemniscus (LL) almost exclusively project to the central nucleus of the IC, which itself projects upward through the thalamus - the Medial Geniculate Nucleus – finally reaching to the Auditory Cortex (AC). Functionally, the IC mediates ascending streams of information, but also receives direct descending streams of cognitively driven activity from corticofugal projections originating from layer V and VI of the AC and many non-auditory, cortical areas (Olthof et al., 2019; Yudintsev et al., 2021). These latter corticofugal projections likely support the plasticity observed in the IC when facing novelty in auditory stimuli (Ayala and Malmierca, 2013; Ayala et al., 2016). Such functions underpin the central role of the IC in many aspects of auditory-sensory processing, which may be directly affected in Fragile X related auditory dysfunctions. Indeed, a clear link has been proposed between audiogenic seizures during development and glutamatergic neurons in the IC by selectively deleting FMRP in VGluT2 neurons of the IC from a possible reduced feedback inhibition (Gonzalez et al., 2019).
Previous studies in the LSO (Garcia-Pino et al., 2017), the IC (Nguyen et al., 2020), and the auditory cortex (Rotschafer and Razak, 2013) relate the known acoustic hypersensitivity in Fmr1 -/- mice with higher neuronal firing rates. These effects have been associated with increased excitation and an elevated number of excitatory synapses in the LSO (Garcia-Pino et al., 2017; McCullagh et al., 2020b), little change in synaptic transmission but altered distribution of voltage-gated K+-channels in the medial nucleus of the trapezoid body (MNTB) (Strumbos et al., 2010; Wang et al., 2015; El-Hassar et al., 2019). An effect of Fmr1 deletion on inhibitory synapses is less clear (McCullagh et al., 2020b). Deletion of FMR1 also affects neuronal size and synaptic and dendritic morphology in auditory brainstem neurons in rodents and humans (Ruby et al., 2015; Wang et al., 2015). These physiological and anatomical changes emerge after hearing onset and are summarized in McCullagh et al. (2020b).
The present study explores to what extent the deletion of Fmr1 affects neuronal response patterns of IC neurons to monaural and binaural pure tone stimulation in adult mice. We used short pure tone stimulation across the entire hearing range which improves comparability to previous studies in the lower auditory brainstem (Wang et al., 2015; Garcia-Pino et al., 2017; McCullagh et al., 2020a). Since response patterns systematically differed across the population of IC neurons we classified response patterns into four different response types and compared Fragile X related changes separately for each type.
Materials and methods
Animal welfare
All experiments were performed with the approval of the German animal welfare legislation (Landesamt für Gesundheit und Soziales, LaGeSo, Berlin G0230/16). Wild-type (WT) and Fmr1 -/- mice colony founders were obtained from Jackson Laboratories (FVB.129P2-Pde6b_Tyrc-chFmr1tm1Cgr/J, stock #004624; RRID:IMSR_JAX:004624). Mouse lines were sustained in our animal facility, housed in a 12 h/12 h light/dark cycle, and had ad libitum access to food and drinking water. No conditioning or manipulations were performed prior to the recording day.
Experimental design and statistical analysis
All efforts were made to minimize both the suffering and the number of used animals in this study. Overall, 37 mice (21 WT and 16 Fmr1 -/-; littermates from both sexes) aged 46 to 107 days were used during this project following a drop out from bad recordings, bad surgery, and bad neuronal responses we analyzed 26 animals, 6WT (P48 to 91) and 2 Fmr1 -/- females (P48/55) together with 8 WT (P46 to 102) and 10 Fmr1 -/- males (P46 to 107). Experimenters were not blinded to the group identity of each subject, but this was not required for performing identical measurements and identical electrophysiology data analysis. We performed these studies in comparable numbers of subjects and experiments across groups. All recordings were made in mice aged between 6 weeks and 3 months, when auditory responses are mature (Ehret and Romand, 1992) and the FVB background strain does not show signs of old-age hearing loss (Ho et al., 2014). We therefore consider all neurons in our sample to belong to the age group “young-mature.” The unequal sex distribution with considerably less females originates from the breeding scheme proposed by the supplier. Since we did not observe sex differences in response patterns in auditory brainstem neurons in a previous study (Garcia-Pino et al., 2017), we did not correct for this discrepancy during the experiments what should probably be now taken into account given the recent publications showing otherwise (McCullagh et al., 2020a; Chawla and McCullagh, 2022). Statistical analyses were conducted with Matlab (RRID:SCR_001622). One-way or two-way ANOVA was applied when comparing age and genotypes while post hoc Bonferroni or Dunnett’s pairwise comparisons were performed only where two-way ANOVA gave statistically significant differences. The unpaired two-tailed Student’s t-test for normally distributed data was applied to probe differences between two means while Mann–Whitney test was used for pairwise comparison of non-parametrically distributed data. Throughout this manuscript the p-values significant outcomes are indicated either by /*/*/*** corresponding to the obtained p-values p = 0.05/0.01/0.001, respectively.
Surgery
Anesthesia was first induced by intraperitoneal injection of xylazine (Rompun 2%, 5 mg/kg) and ketamine (Ketavet 10%, 100 mg/kg). Additional doses were given subsequently during the course of the experiment based on vibrissa motility and heart-beat frequency. Rectal probe helped to maintain the animal body temperature between 36°C and 37°C (TC01, Multi-Channel-System). The frontal skull was first exposed and cleaned. A locally custom-made head post was attached to the frontal part of the skull using dental cement (Charisma, Heraeus-Kulzer). The animal was then placed into a computer-controlled stereotaxic frame (Neurostar) in a sound-attenuating chamber (Desone) where the head-to-stereotactic-arm alignment was thoroughly adjusted every day. A craniotomy was performed caudally to lambda (0 to −2 mm Rostro-Caudal and −2 mm to 0 Medio-Lateral) exposing the cerebellum and the underlying IC. Great care was used during the craniotomy to follow the posterior border of the lateral suture in order to preserve the underlying blood vessel. Durotomy was performed, tissue wetted with PBS, and heartbeat was constantly monitored.
Acoustic stimulation
Sound stimuli were designed using Brainware (TDT, Jan Schnupp). Sound stimuli were produced at the 195.3 kHz sampling rate of the RZ6 TDT amplifier and monitored by Real-Time Processor Visual Design Studio (RPvdsEx; Tucker Davis Technology TDT). Signals were delivered either monaurally or binaurally to the ears of the experimental animals by MF1 magnetic speakers (TDT, closed field configuration) and appropriate plastic tubing inserted into the animal’s pinnae. We calibrated our acoustic signals using a 1/4 inch measurement microphone (40 BF, G.R.A.S.), custom written Matlab software, and SigCalRP calibration software (TDT). Single neuron spiking activity was pre-amplified and band-pass filtered (300–3000 Hz) using a Model 3000 amplifier (A-M Systems). Neuronal spikes were digitized at 24.4 kHz by the RZ6 Processor (TDT). Real-time spiking activity was gathered according to stimuli and displayed using Brainware. White noise (100 ms duration, 5 ms rise/fall time, contralateral, 30 dB SPL) was used to detect neuronal firing during the search phase. Upon encounter spiking activity of a single neuron, frequency response areas (FRA) and firing patterns to pure tones were characterized by presenting a range of different sound frequencies and intensities (100 ms duration, 5 ms rise/fall time, 0–100 dB SPL, 1−40 kHz, for both contra- and ipsi-lateral ear to the recorded IC); the different conditions were randomized in frequency and intensity within each protocol. Binaural response properties of neurons were tested by presenting pure tones at each neuron’s characteristic frequency (CF), while the contralateral ear being stimulated with the corresponding range of interaural intensity differences (±30 dB). Stimulus Specific Adaptation (SSA, Supplementary Figure 3) was performed by first choosing 2 frequencies around the CF with 4 kHz difference (Duque and Malmierca, 2015). Then both frequencies were exposed contralaterally in an alternating oddball paradigm, i.e., the first frequency was exposed 10% of the time while the other 90% and then the protocol was repeated exposing the first frequency more frequently while the second 10% of the time.
Data acquisition
Recording electrodes were pulled from borosilicate glass capillaries (10–15 MOhm, BioMedical Instruments) with a PC-10 puller (Narishige) and then filled with 2 M NaCl solution, in which 2% HRP (Type II, Sigma-Aldrich) was dissolved. First, the electrode was stereotactically aligned above the IC coordinates (Figure 1), and then progressively lowered down in the tissue while presenting white noise to the animal. A careful approach to the recorded neuron guaranteed measurements of spikes from single neurons. In most parts of the IC, a crisp background response of Multi-Unit-Activity (MUA) to the noise stimulus could be monitored; recordings were considered to be single unit activity, when action potential amplitude was at least three-fold the signal-to-noise ratio (snr) of the local MUA. At the end of each recording session the neighboring neurons were labeled with an injection of HRP by three 90 V DC current injections over 3 min (ISO-STIM 01D, NPI electronic).
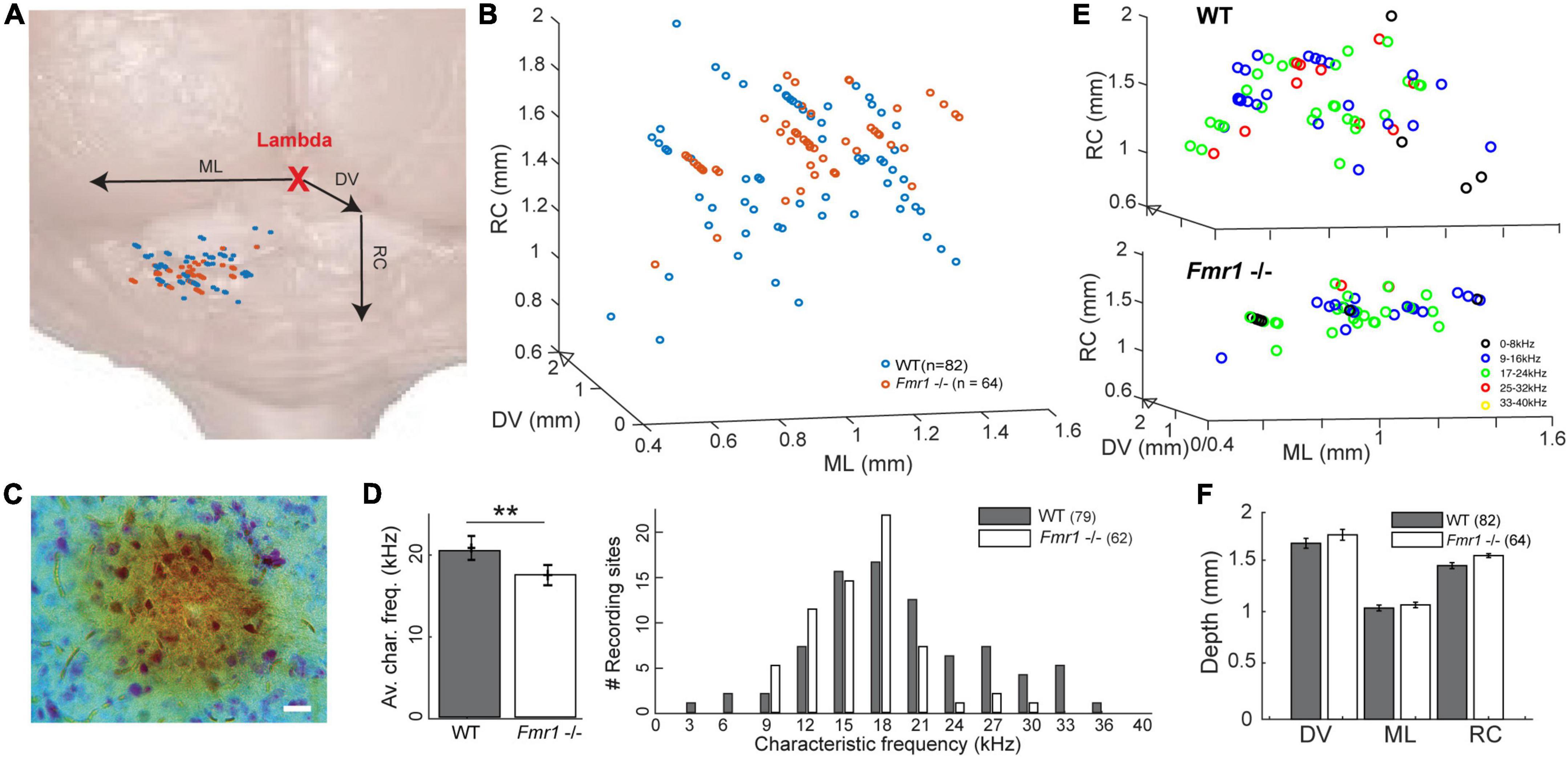
Figure 1. Location of the recorded IC neurons, with their characteristic frequencies. (A) Schematic reconstruction of stereotactic recording locations on top of a schematic brain picture, (orange) Fmr1 -/- neurons, (blue) WT. (B) 3D-reconstruction of the different stereotactic locations. (C) Picture of an HRP staining (20 μm). (D) (right) Histogram of all CFs. (Left) Averaged CF in the WT vs. Fmr1 -/- mice. (E) 3D stereotactic locations represented with color-coded CF (dark low, light color higher frequencies) in (up) all WT neurons, (down) all Fmr1 -/- neurons. (F) Averaged positions of all cells’ stereotactic locations in WT and Fmr1 -/- neurons.
Stereotactic reconstructions
At the end of each experiment, animals were sacrificed with a lethal dose of ketamine/xylazine. The brain was removed and immersed in 4% PFA overnight. Brains were then thoroughly washed at room temperature with 0.1 M PBS, pH 7.4, and 70 μm coronal brain sections were cut on a vibratome (VT1200, Leica). HRP staining was done by incubating the sections in a solution containing Diaminobenzidine reaction (DAB kit, Sigma-Aldrich) and a Nissl counterstain. The pipette tracks, together with our stereotactic reconstructions (Supplementary Figure 1 and Table 1), suggest that all neurons were recorded in the IC. All successful HRP staining confirmed that the recorded neurons were in the IC.

Table 1. Mean values (±SEM) of the stereotactic coordinates of the four classes of neurons (4 lines for Transient, Sustained, Inhibited, and Offset) in the two genotypes (WT left, Fmr1 -/- right) as shown in Supplementary Figure 1.
Data analysis
Only single-unit recordings with a good signal to noise ratio (≥3) and stable firing over the entire time course of the experiment were kept for further analysis. Overall, out of the 26 used animals we analyzed 100 WT neurons and 94 Fmr1 -/- neurons, having at least 2 neurons per animal and never more than 11. As detailed for each analysis, neurons were chosen based on the measurability of the desired parameter(s). To guarantee within one animal measurements to be from different neurons we only recorded from neurons that were at least 300 μm apart, either along a single vertical insertion or between two neighboring vertical insertions.
For each neuron, a single compound PSTH was defined as the combination of the spiking activity averaged from all the different stimulus conditions screened during the Frequency Response Area (FRA: 0−100 dB, 1−40 kHz). This observation led us to establish a user-based distinction of four types of neurons: Transient neurons, Sustained neurons, Offset neurons and Inhibited neurons. This distinction was made using a simple two-steps decision tree looking at the FRA of each neuron and the compound PSTH: 1- Does the neuron fire mostly after the tone termination (in the offset period in its PSTH)? If so, this describes an Offset Neurons cf. Figure 2D. In case of a negative answer then: 2- Does the FRA exhibit a negative tuning? This would be an “Inhibited neuron” cf. Figure 2C. Often, inhibited neurons had high spontaneous firing rates and their firing is reduced during tone exposure. If neither of these two conditions are matched -what was the case in about 80% of the neurons of our dataset- then the neuron belonged to one of the two more classical cell-types, the “Onset” or “Sustained” neurons, as broadly reported in the IC (Ehret et al., 2003; Gittelman et al., 2012). This user-based decision tree is the result of a very strong inhomogeneity of the physiology in our dataset and observed similarly in both genotypes.
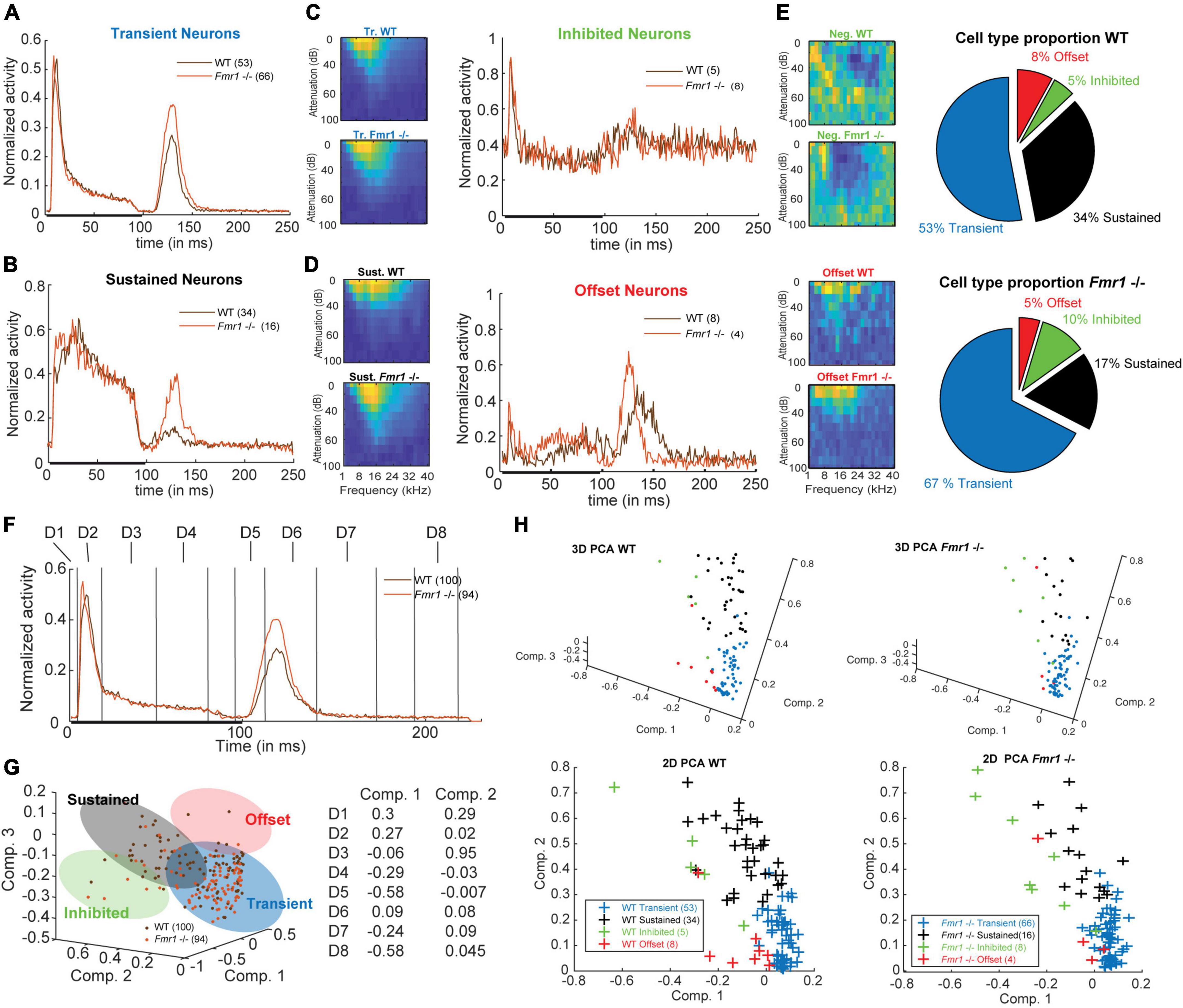
Figure 2. Four neuronal response patterns in both genotypes. (A) (left) Averaged compound PSTH of all transient neurons, (brown) WT and (orange) Fmr1 -/-; (right above) Averaged FRA of all WT transient neurons and (right below) FRA of all Fmr1 -/- transient neurons. (B) (left) Averaged PSTH of all sustained neurons WT, and Fmr1 -/-; (right above) FRA of all WT sustained neurons, and (right below) their Fmr1 -/- counterparts. (C) (left) PSTH of all inhibited neurons, WT and Fmr1 -/-; (right above) FRA of all WT inhibited neurons, and (right below) their Fmr1 -/- counterparts; note the negative tuning that these neurons’ FRAs exhibit. (D) (left) PSTH of all offset neurons, WT and Fmr1 -/-; (right above) FRA of all WT all offset neurons, and (right below) their Fmr1 -/- counterparts. (E) Proportion of each cell types in (above) WT and (below) Fmr1 -/- neurons. (F) All-cell average of all compound PSTHs in the WT and Fmr1 -/- mice. Different values of the transient firing are taken in each time-window for each cell (D1-D8), used for the following principal component analysis (PCA). (G) (left) 3D-representation of all recorded neurons along the first three components. Note the four shaded areas corresponding to the four cell types detailed above. (Right) Projections of the two first principal components on the eight time-windows. (H) (above left) 3D-representation of all WT neurons; (below left) same neurons represented along the two first components; (above right) 3D-representation of all the Fmr1 -/- neurons; (below right) 2-D projection of all the Fmr1 -/- neurons on the two first components.
To address the validity of such a distinction we performed a few controls: a Principle Component Analysis (PCA) to illustrate the diversity of the compound PSTHs, a comparison of stereotactic depth (Table 1) and a more detailed PSTH comparison for FRA sub-sections. The PCA was based on the average spiking rate in eight different time windows (Dimension 1: 0−5 ms, Dim. 2: 5−20 ms, Dim. 3: 20−50 ms, Dim. 4: 50−90 ms, Dim. 5: 95−110 ms, Dim. 6: 110−140 ms, Dim. 7: 140−170 ms, and Dim. 8: 190−230 ms).
Quantification of frequency response area
The CF was defined as the frequency at which each neuron responded (firing 3x-larger than spontaneous activity) to the lowest intensity (threshold). Sharpness of tuning was analyzed as previously published (Garcia-Pino et al., 2017) and reported in Q10, 30, and 50 values (Figure 3); Q10 values were calculated as the response bandwidth at 10 dB above each neuron’s threshold divided by the CF; Q30 and Q50 were calculated accordingly at 30 dB and 50 dB above each neuron’s threshold.
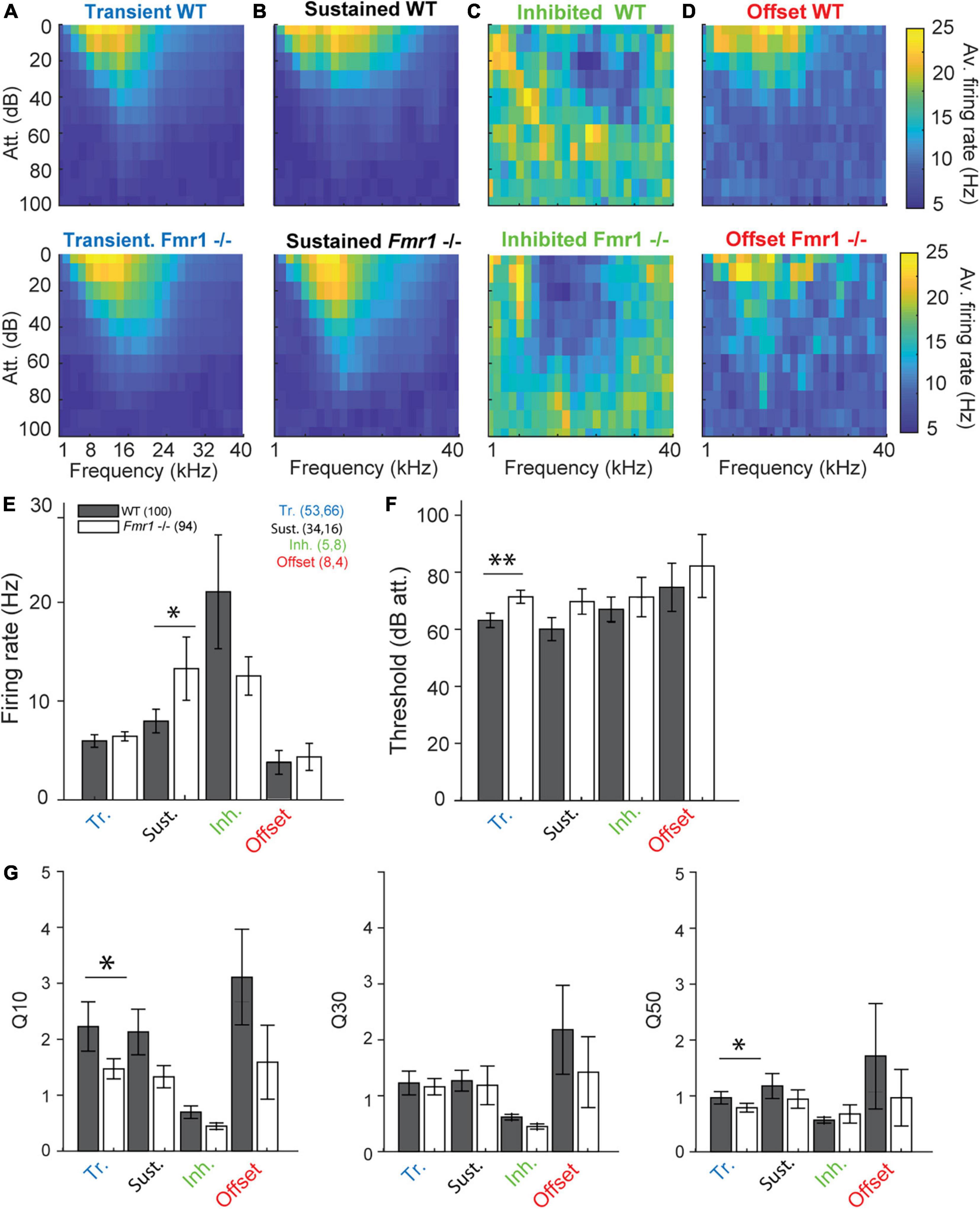
Figure 3. Fmr1 -/- neurons are over-activated and oversensitive. (A) (above) Averaged of all WT transient neurons FRA, and (below) all their Fmr1 -/- counterparts. (B) (above) Averaged of all WT sustained neurons FRA, and (below) their Fmr1 -/- counterparts. (C) (above) Averaged of all WT inhibited neurons FRA, and (below) their Fmr1 -/- counterparts. (D) (above) Averaged of all WT offset neurons FRA, and (below) their Fmr1 -/- counterparts. (E) Averaged firing rate of each of the 4 classes. (F) Average threshold of the 4 classes together. (G) Averaged Q-values measured in each neuron, resp. (Left) Q10, (middle) Q30, (right) Q50.
Further data analysis
First spike latency was measured at the CF (Figure 4) as the time point following tone exposure when the average spiking of each neuron reached three times its own spontaneous firing baseline, measured in the 5 ms before tone exposure. Inhibited and offset neurons were not reported as their spiking patterns do not exhibit such measurable increases: Offset neurons barely have tone modulations at the beginning of the tone, inhibited neurons have a decreasing firing concomitant to the activation of the other neurons, suggesting a possible inhibitory modulatory role. The time of maximum firing was measured at the peak of the compound PSTH. Rebound activity was characterized by averaging the firing rate over a time window following tone exposure (100 to 150 ms). The Interaural-Level-Difference (ILD) curve was obtained by gathering the different ipsilateral and contralateral stimulus intensities according to their respective ILD values. Once a neuron’s CF and threshold was identified, a set of different intensities were presented (i.e., threshold/−10/−20/−30 dB) with contralateral intensities ranging between −30 and +30 dB ILD for each ipsilateral intensity. The spikes elicited by each neuron were summed up and normalized to the different ipsilateral intensities within a fixed ILD window (−30 and +30 dB) to produce the estimated spike rate per cells for each different ILD. Once averaged the obtained ILD curve was then characterized by the level of the half-maximum and by the overall slope steepness measured from the maximum to the minimum of the obtained normalized ILD curve. Finally, the adaptive behavior of neurons to novel stimuli was measured by performing mismatch of two pure tones (Supplementary Figure 3A), choosing two frequencies (f1 and f2) ± 4 kHz around the CF at 30 dB above the threshold in a 10−90% alternation paradigm (Ulanovsky et al., 2003; Malmierca et al., 2009). To exclude plasticity mechanisms based on different frequency tuning properties (Ayala and Malmierca, 2013) only neurons with similar firing rates to f1 and f2 were included. As inclusion criteria we used firing ratios (f1/f2) from 0.5 to 2.
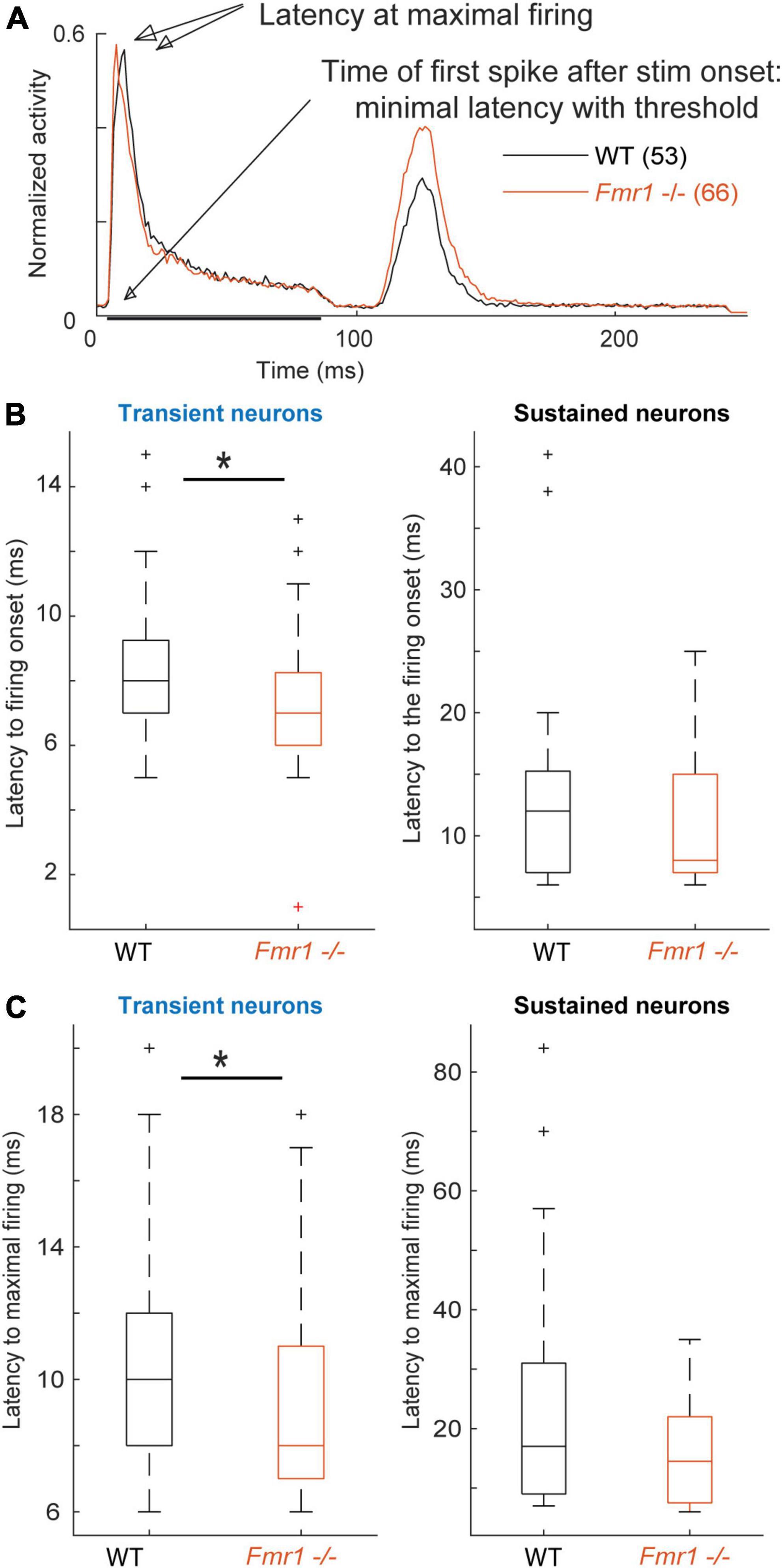
Figure 4. Fmr1 -/- neurons exhibit an earlier and stronger activation. (A) Averaged and normalized PSTH of all cells over all conditions during the FRA for all transient WT and Fmr1 -/- neurons. (B) Boxplot representation of the averaged latency in transient (53 WT, 66 Fmr1 -/-) and sustained neurons (34 WT, 16 Fmr1 -/-). (C) Boxplot representation of the timing of maximal spiking in transient and sustained neurons from WT and Fmr1 -/- mice.
Results
Higher frequencies are under-represented in the inferior colliculus of the Fragile X mouse model
A recent study provided evidence that sound representation is altered in IC neurons of developing Fmr1 -/- mice (P21-P39, Nguyen et al., 2020), however, it is unclear whether these differences are maintained in fully mature mice. To do so we presented 100 ms long tones of different frequencies (1−40 kHz, 25 steps) and different sound intensities (0−100 dB att., 10 dB steps) to the contralateral ear. From this FRA the CF of each neuron, the frequency where a neuron responds to the lowest intensity, was extracted (Figures 3A−D). The CF was correlated with the location of each neuron within the IC as confirmed by HRP staining (Figure 1A, see section “Materials and methods”) and subsequent reconstruction according to the stereotactic coordinates. Neurons were located in the entire inferior colliculus, including the dorsal and external cortices of the IC (Figure 1F). The superimposed average location estimated from the stereotactic coordinates of the recorded neurons did not differ between genotypes in either of the three axes of the micromanipulator arm in our reference frame (Figures 1B−F). Nevertheless, neurons of WT mice responded best to significantly higher frequencies compared to neurons in Fmr1 -/- mice (Figure 1D, left, 20.43 kHz ± 0.77 vs. 17.48 kHz ± 0.56, unpaired t-test, P = 0.0074). In fact, CF higher than 24 kHz were almost absent in Fmr1 -/- mouse IC (Figure 1D, right) despite being recorded in similar if not deeper depth (Supplementary Figure 1 and Table 1). Whether a loss of FMRP impacts tonotopic representation in the IC, as previously shown in the MNTB and indirectly in the IC (Strumbos et al., 2010; McCullagh et al., 2017; Chawla and McCullagh, 2022), needs to be confirmed using different methods.
Four different response patterns of inferior colliculus neurons in both genotypes
Previous studies reported broader tuning, higher thresholds, increased spiking activity and latency changes in the ascending auditory pathway of adult Fmr1 -/- mice in the MNTB and LSO (Strumbos et al., 2010; Brown and Kaczmarek, 2011; Garcia-Pino et al., 2017). Analyzing these parameters across our dataset of all recorded IC neurons did not yield any statistical differences between WT and Fmr1 -/- mice. Using molecular, anatomical and physiological markers the auditory midbrain is composed of different neuron types, however a comprehensive neuron type classification combining various methodologies has not been achieved in this brain region (Wallace et al., 2021). Moreover, extracellular spike shape analysis, which is widely used in other brain regions for neuron type classification, has not been proven successful in the IC (Ito, 2020; Wallace et al., 2021). We therefore classified IC neurons, as previously done (Tan et al., 2007; Palmer et al., 2013; Akimov et al., 2017), according to their spike response patterns to pure tone stimulation with different frequencies using a simplified decision tree (see section “Materials and methods”). As response patterns change depending on the frequency and intensity of the sound, we used a compound PSTH defined as the combination of all the spiking patterns gathered from all different conditions. The averages of these compound PSTHs for each category of the different neuron types are shown in Figures 2A−C. Transient neurons, which are present in both genotypes, are characterized by transient responses to the onset of the tone (Figure 2A). In many cases, they show an offset-rebound activity following the termination of tone exposure (Figure 2A, left). Both genotypes exhibit classical frequency tuning (Figure 2A, right). Those neurons represent the majority of the recorded neurons (67% in the Fmr1 -/-, 53% in the WT, Figure 2E, out of resp. 94 Fmr1 -/- neurons and 100 WT neurons) and likely cover the previously reported “ON,” “Long-Latency,” and “ON-OFF” neurons from other studies (Duque et al., 2012; Palmer et al., 2013; Akimov et al., 2017). They may also overlap with the “Sensitive-symmetrical,” the “Sensitive-asymmetrical” and the “Selective” neurons from other authors (Lee et al., 2019). Second in proportion, the sustained neurons exhibit a more regular firing during the entire duration of sound exposure (Figure 2B, left). They also exhibit a more classical excitatory frequency tuning (Figure 2B, right). The sustained neurons are more abundant in the WT compared to Fmr1 -/- mice (34% vs. 17%, Figure 2E). This neuronal type likely covers the “ON-Sustained” and “Sustained” neuron type from other studies (Duque et al., 2012; Palmer et al., 2013; Akimov et al., 2017). Third, inhibited neurons exhibit the very peculiar “negative tuning” characteristic of their FRA (Figure 2C, right); they have a negative firing modulation during tone exposure (Figure 2C, left). This neuron type exhibits a high spontaneous firing compared to the other types; it represents a minority of 10% of Fmr1 -/- and 5% of WT neurons. This neuron type matches very well the already reported “inhibitory frequency response area” with un-classical FRA; they were initially reported to be exclusively present in awake mice (Duque and Malmierca, 2015) as well as the inhibited neurons in the IC’s cortex (Wong and Borst, 2019). Fourth, offset neurons exhibit the largest response after the termination of the sound exposure (Figure 2D, left); they exhibit a more usual FRA (Figure 2D, right). These neurons represent 8 and 5% of the whole dataset in resp. WT and Fmr1 -/- neurons. All further analysis of response characteristics were performed using these groups.
To address the validity of such a distinction based on a simple decision tree, we controlled for few aspects supporting this categorization: first, we projected the PSTH of each cell, showing their discrepancy of firing pattern using Principal Component Analysis (PCA) in both genotypes (Figures 2F−H). Our PCA was based on average spiking rate in eight different time windows (Figure 2F). The values decomposition of the first principal component having the highest weight (Figure 2G) are based on the transient onset (Dim. 2), the sustained firing (Dim. 3−4) and the offset responses (Dim. 5, Dim. 7−8). An unexpected outcome of this PCA is the similar clustering of the four neuronal cell types observed in both WT and Fmr1 -/- mice (Figures 2G,H). Second, we controlled that the stereotactic locations of the recorded neurons were not accounting for neuron type in either depth or location (Supplementary Figures 1A,B and Table 1). These data suggest that the different cell types were pooled from a homogenous region and were not the result of differences in the physiology of IC cortex vs. IC central nucleus neurons. Third, to prevent any bias resulting from oversampling of PSTHs for multiple tone frequencies or intensities, we split the conditions recorded during the FRA into different features (all intensity at one frequency, all frequency for one intensity, etc.) and observed consistent differences in spiking patterns according to the distinguished cell-types in both genotypes (Supplementary Figure 2). Interestingly, we consistently observed offset responses in all cell types when gathering spiking outside their excitatory tuning area (Supplementary Figures 2D,F).
Fmr1 -/- neurons have lower thresholds, broader tuning and shorter response latencies compared to wild-type neurons
We reexamined thresholds, firing rates, frequency tuning and response latencies of different IC neuron types in WT and Fmr1 -/- mice. The class-averages of all FRAs within each neuron type exhibited similar tuning between the different genotypes (Figures 3A−D). Nevertheless, when processing each neuron type individually, we revealed a clear bias of lower thresholds in all neuron types of Fmr1 -/- mice (Figures 3E−G). This bias reached significance levels only in transient neurons (Figure 3 F, 71.66 dB att. ± 2.3 in the Fmr1 -/-, 63.39 dB att. ± 2.54 in the WT, unpaired t-test P = 0.0173). All other neuron types exhibited lower thresholds in Fmr1 -/- mice but failed to pass significance probably due to the limited sampling. Sustained Fmr1 -/- neurons showed significantly higher firing rates than their WT counterparts (Figure 3 E, 13.27 Hz ± 2.6 in Fmr1 -/-, 7.97 Hz ± 1.19 in WT neurons, P = 0.0255). The firing rates in other Fmr1 -/- neuron types were also higher (Figure 3E), except for the Fmr1 -/- inhibited neurons. Taken together, these results indicate a generally increased firing in the Fmr1 -/- mice, which matches the broadly reported hyper-active, hyper-sensitive phenotype of neuronal sound representation in FXS mice. However, no difference in spontaneous activity was observed in the different classes of neurons between genotypes (transient: 0.018 Hz ± 0.003 in Fmr1 -/-, 0.014 Hz ± 0.002 in WT neurons, P = 0.104; sustained: 0.056 Hz ± 0.017 in Fmr1 -/-, 0.067 Hz ± 0.014 in WT neurons, P = 0.47; inhibited: 0.31 Hz ± 0.07 in Fmr1 -/-, 0.31 Hz ± 0.04 in WT neurons, P = 1; offset: 0.062 Hz ± 0.06 in Fmr1 -/-, 0.061 Hz ± 0.08 in WT neurons, P = 0.78). We next analyzed frequency tuning width of each neuron using the Q10, Q30, and Q50 values as a measurement for tuning widths, with lower Q-values indicating broader tuning (see section “Materials and methods”). Surprisingly, only transient neurons showed significantly broader tuning for Q10 values (Figure 3G, in the transient neurons 1.45 ± 0.18, in the Fmr1 -/-, 2.23 ± 0.44 in the WT, P = 0.0495) and Q50 values (0.74 ± 0.07, in the Fmr1 -/-, 0.80 ± 0.10 in the WT, P = 0.0437). In all other neuron types tuning width was similar for both genotypes.
Analyzing first spike latency and the latency of maximal firing revealed shorter latencies for transient and sustained neurons of Fmr1 -/- mice (Figure 4). Transient and sustained Fmr1 -/- neurons exhibit a significant shorter first spike latency in the IC (Figure 4B, 7.60 ms ± 0.27 in the Fmr1 -/- vs. 8.53 ms ± 0.35 in the WT for transient cell, unpaired t-test P = 0.0489; 11.36 ms ± 1.85 in the Fmr1 -/- vs. 13.81 ms ± 1.64 in the WT for sustained cell, unpaired t-test P = 0.0709). They also exhibit significantly earlier maximal firing (Figure 4C, 9.42 ms ± 0.36 in the Fmr1 -/- vs. 10.36 ms ± 0.44 in the WT for transient cell, unpaired t-test P = 0.0426; 15.7 ms ± 2.22 in the Fmr1 -/- vs. 23.05 ms ± 0.39 in the WT for sustained cell, unpaired t-test P = 0.3310).
Taken together, these results suggest that Fmr1 deletion has similar, but not larger effects on spike number and tuning width in the IC when compared to auditory brainstem neurons (Garcia-Pino et al., 2017).
Enhanced offset-rebound activity in sustained and transient neurons of Fmr1 -/- mice
Unexpectedly, post-stimulus or offset-rebound activity differed between Fmr1 -/- mice and WT neurons (Figure 5). In both genotypes and in both transient and sustained neurons offset-rebound activity was present (Figures 5A,B). However, more neurons exhibited offset-rebound activity in Fmr1 -/- compared to WT (Figures 5A,B). Quantification of offset-rebound firing yielded significant higher values for Fmr1 -/- compared to WT mice (Figure 5C, left: 0.53 Hz ± 0.03 in Fmr1 -/- 0.37 Hz ± 0.04 in the WT transient neurons, unpaired t-test P = 0.0014, 0.46 Hz ± 0.07 in Fmr1 -/- 0.26 Hz ± 0.04 in the WT sustained neurons, unpaired t-test P = 0.0254, and the corresponding histogram Figure 5C, right). It is also noticeable that in the Fmr1 -/- mice, almost all transient neurons exhibited rebound activity (60/66), while in the WT only about two third of the neurons showed an offset response (39/54). Furthermore, the tuning exhibited by the rebound activity was always “negative” (Figure 5D) and most likely related to “inhibitory frequency response areas” as characterized by other authors (Duque and Malmierca, 2015).
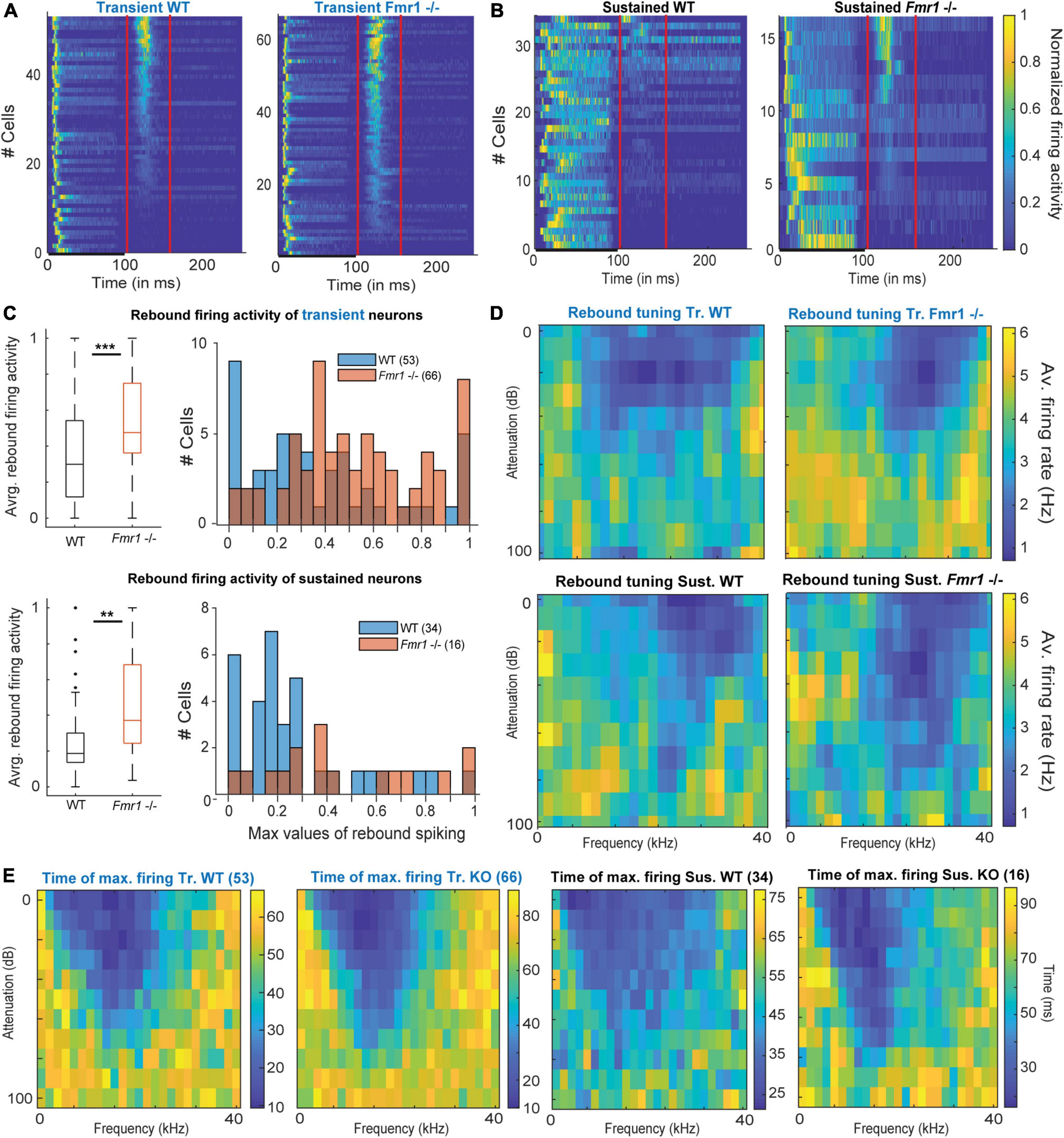
Figure 5. Offset-rebound activity in Fmr1 -/- neurons. (A) Normalized PSTH for (left) all WT transient neurons and (right) their Fmr1 -/- counterparts; note that the red lines indicate the limits used to quantify the tuning of the rebound-offset activity. (B) Normalized PSTH (left) all WT sustained neurons and (right) all their Fmr1 -/- counterparts. (C) (above left) Averaged and normalized rebound-offset activity in all WT and Fmr1 -/- transient neurons; (above right) Corresponding histograms, note the semi-Gaussian organization of the Fmr1 -/- offset-rebound; (below left) Averaged and normalized rebound-offset activity in all WT and Fmr1 -/- sustained neurons; (below right) Corresponding histogram with a bin size of 0.05. (D) Averaged normalized FRA from spikes selected from offset-rebound activity that illustrates the tuning exhibited by the rebound-offset activity in respectively: (above left) all WT transient neurons, (above right) all Fmr1 -/- transient neurons, (below left) all WT sustained neurons, (below right) all Fmr1 -/- sustained neurons. (E) Similarly for each single individual frequency and attenuation, the time of maximal firing is averaged within the populations and illustrated in the corresponding color code to report the later peak in the PSTH outside of the FRA for (left) Transient WT, (middle left) transient Fmr1 -/-, (middle right) sustained WT and (right) Fmr1 -/- neurons.
Our results indicate that most IC neurons (transient and sustained) exhibited a classical FRA firing to pure tone exposure, which is characterized by spiking activity during the stimulus presentation. In many transient neurons, this is then followed by an offset-rebound firing whose tuning is outside of the excitatory tuning area (Figure 5E). Similar response features were also observed in sustained neurons (Figure 5E), arguing for a cell-type independent mechanism. An increased offset-rebound activity could potentially arise from a change in each neuron’s intrinsic ion channel composition and/or an enhanced lateral inhibition (Kopp-Scheinpflug et al., 2018). This indicates that not only excitation but also inhibition is homeostatically upregulated in Fmr1 -/- mice.
Binaural balance of excitation and inhibition (EI-balance) is shifted in Fmr1 -/- mice
Using binaural stimulation our previous study in the LSO revealed enhanced ipsilateral, excitatory and no change in contralateral, inhibitory input strength in Fmr1 -/- mice thereby shifting the responses to binaural stimulation toward the ipsilateral ear (Garcia-Pino et al., 2017). We were interested in whether these binaural response properties are preserved at the level of the IC or whether additional homeostatic mechanisms compensate or enhance the properties observed in Fmr1 -/- LSO neurons. Accordingly, we presented a set of different sound intensities at the ipsi- and contralateral ear. Spike responses with sounds of different intensities but same ILDs were summed up and normalized to the maximal responses. Figure 6A displays the normalized response levels to these ILDs for each cell. Only neurons with a sustained and transient response were included in the analysis (83 out of 100 WT neurons and 84 out of 94 Fmr1 -/- neurons), but neurons were not selected for binaural sensitivity. Interestingly, the summed binaural responses of all WT neurons revealed a strong bias with excitation predominating for contralateral stimulation and inhibition for increasing ipsilateral stimulation.
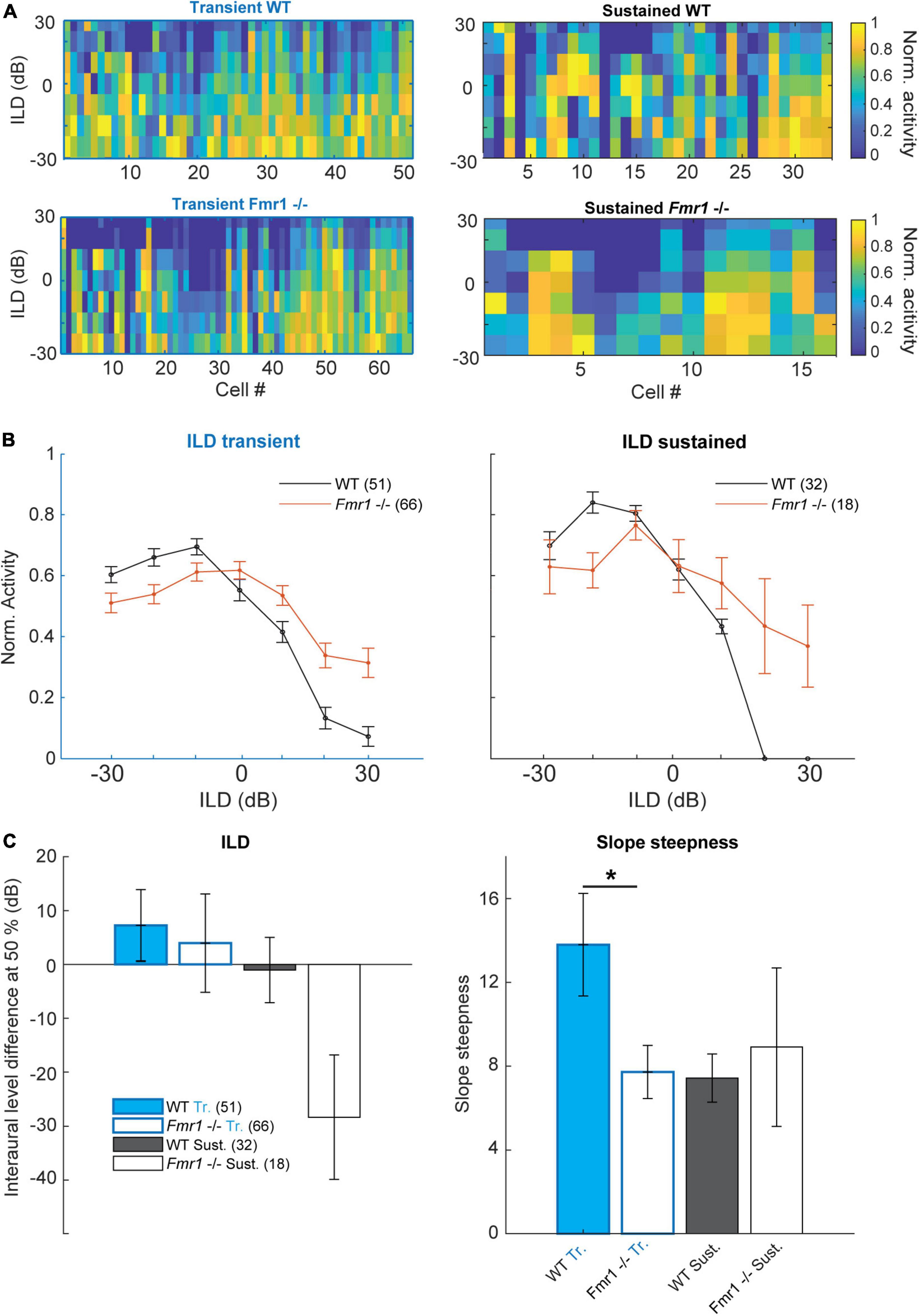
Figure 6. Binaural integration of ILDs is impaired in the Fmr1 -/- mice. (A) Normalized spike rate per neuron for the different ILD conditions for: (above left) all WT transient neurons; (below left) all Fmr1 -/- transient neurons; (above right) all WT sustained neurons and (below right) all Fmr1 -/- sustained neurons. (B) (left) Averaged normalized ILD for all transient neurons WT and Fmr1 -/-; (right) ILD for all sustained neurons WT and Fmr1 -/-. (C) Quantification of resp. (Left) averaged ILD as the half height of the corresponding fitted sigmoid; (right) for the slope steepness of resp. WT and Fmr1 -/- ILDs.
This was not the case in Fmr1 -/- neurons, where ILD functions were much flatter and ipsilateral stimulation suppressed contralateral evoked spiking much less (Figures 6A−C). This was observed in both sustained and transient firing neurons. In transient neurons we observed a small shift of the ILD function in Fmr1 -/- mice in combination with a stronger firing for ILDs larger than 20 dB. In both transient and sustained neurons, a clear difference of ILD turning point was observed (Figure 6C) and a significant difference in the slope was seen in the transient neurons (Fmr1 -/- 7.72 ± 1.27 vs. WT 13.79 ± 2.45, unpaired t-test P = 0.0457). In the sustained neurons we observed a similar difference also indicating a shift in the balance of excitation and inhibition. This suggests that the imbalance of binaural excitation and inhibition, as observed in the LSO, is mostly preserved at the level of the IC. Whether this also holds for stimuli with more complex sound features remains to be shown.
Stimulus-specific adaptation in the inferior colliculus does not differ between wild-type and Fmr1 -/- mice
People with Fragile X syndrome show decreased processing abilities of novel stimuli and less habituation when presented with an auditory odd-ball paradigm (Ethridge et al., 2020). Also, neurons in the IC are known to exhibit an enhanced representation to pure tones when they are presented in an oddball fashion. This is usually measured by choosing two tones around a neuron’s CF (Duque and Malmierca, 2015; Supplementary Figure 3A). In addition, the upper or the lower frequency is presented irregularly (10% randomized) (Supplementary Figure 3B). In many neurons this induces a reduction in firing rate in the response to the odd (10% presentation) stimulus when compared to the frequent (90% presentation) stimulus (Supplementary Figure 3C). This adaptation is called stimulus-specific adaption (SSA) and was reported previously for many neurons in the IC (Ayala and Malmierca, 2013). In our data set, the specific adaptation indices (SI), a measurement for adaptation for each frequency (f1 and f2), displayed a similar distribution for both WT and Fmr1-/- mice and ranged for most neurons between 0 and 1 (Supplementary Figure 3D). In both genotypes only few neurons exhibited adaptation above 0.5. Similarly, the common stimulus-specific adaptations index (CSI), measuring responses to both frequencies, showed on average a similar profile for both genotypes and for both transient and sustained neurons (Supplementary Figure 3E). It is known that SSA is more pronounced in neurons in the lateral and external cortex of the IC, where neurons also exhibit much broader tuning (Ayala and Malmierca, 2013; Ayala et al., 2016). To find out whether in our data the degree of adaptation correlated with the broadness of tuning, we plotted the CSI against the Q30 value for each transient and sustained firing neurons and both genotypes (Supplementary Figure 3F). However, only a small trend of broader tuning correlating with larger SSA was observed, which was again not different between WT and Fmr1-/- animals. Similar was true, when we correlated CSI with the specific location of recorded neurons as drawn from our stereotactic measurements. However, assigning the recording site to a specific part of the IC solely based on stereotactic measurements is rather difficult. Also, delineation of different subparts of the IC, such as central nucleus, dorsal and lateral cortex and the rostral shell are not well understood and may differ between species (Choy Buentello et al., 2015; Lesicko et al., 2016). We conclude from out data that at the level of the IC Fmr1-/- mice do not exhibit impaired processing of novel stimuli presented as an oddball paradigm. Perceptional deficits of novel stimuli in humans with FXS, may thus arise from circuit changes in higher cortical areas.
Discussion
We studied classical features of IC neuron responses presented with simple sounds (pure tones) to obtain further insights into the pathophysiological basis of distorted auditory processing in FXS. One major finding that has not been previously described was that the post-inhibitory rebound response, mainly evoked by stimulating outside of the excitatory receptive field, was substantially enhanced in neurons from Fmr1 -/- mice. All other response features such as total spike number, tuning width and binaural integration were similar to the ones observed in lower brainstem nuclei. Due to the diversity of IC neuron types, we observed statistical differences between WT and Fmr1 -/- mice, only once IC neurons had been categorized according to their response patterns to pure tones of different frequencies and intensities.
Categorization of inferior colliculus response types is similar in wild-type and Fmr1 -/- mice
Comparing neuronal responses to pure tones between WT and Fmr1 -/- mice in all neurons did not yield substantial differences. Consequently, we grouped the neurons based on their firing patterns to short pure tones of different frequencies and intensities, and on whether neurons were excited or inhibited by sound stimulation. Similar classes of response patterns in the IC have been suggested in various species including bats and mice (Xie et al., 2007; Palmer et al., 2013; Akimov et al., 2017). The transient and sustained neurons compose most of our dataset, which matches most of the published literature, whereas offset neurons and inhibited neurons remain a minority in our recordings and in the published literature (Willott et al., 1988; Kasai et al., 2012; Wong and Borst, 2019). One reason for the low number of inhibited neurons reported so far could be that measurement of inhibited neurons is tricky. As these neurons exhibit a very high spontaneous firing rate, they can be miss-identified as dying neurons.
In previous studies classification of firing types in IC neurons was mostly based on the firing pattern to a single frequency and intensity, mostly at the CF (Nasimi and Rees, 2010; Gourévitch et al., 2020) or, as in other studies, by the shape of the excitatory and/or inhibitory frequency response areas (Palmer et al., 2013). With our approach, we included information on active inputs across the entire FRA into the classification scheme, by using the summed spiking activity to all presented frequencies and intensities similarly to a previous study (Egorova et al., 2020). This includes not only information on the excitatory but also on inhibitory receptive fields. This inhibition has been shown to induce rebound activity after sound termination in many cases (Kopp-Scheinpflug et al., 2018). We then used a simple user-based decision tree to categorize spiking patterns, which was followed by PCA confirmation. For larger datasets, such a PCA representation could be used to cluster the neuronal response types to replace the user-based decision tree. However, in our case it would lack reproducibility due to the relatively small sample size. Very recently, several new methods have been developed to categorize neuron types based on spike patterns. This includes dimensionality reduction algorithms such as UMAP (Lee et al., 2021) or t-SNE (Dimitriadis et al., 2018). However, these algorithms require larger datasets, as acquired with for example high-density silicon probe recordings.
Our data also indicate that Fmr1 deletion decreases cell type diversity as transient neurons represent 53% of all neurons in the WT and 67% in the Fmr1 -/-. Furthermore, the representation of response features of each neuron in both WT and Fmr1 -/- using PCA illustrates that Fmr1 -/- neurons exhibit less clear boundaries between the different neuron types compared to WT neurons, where transient neurons exhibit a more compact cloud of points. Up to now correlating spiking response patterns or spike shape of extracellularly recorded IC neurons with other molecular, cellular or morphological features has not been successful (Ito, 2020; Wallace et al., 2021). More studies on this matter are needed to obtain a better understanding of IC network functions.
Genotype specific changes in threshold, firing rate, frequency tuning and binaural response properties in inferior colliculus neurons are similar to auditory brainstem neurons
In contrast to many previous studies we found a trend for a decrease in response thresholds that reached significance for transient firing neurons. All studies that reported increased thresholds used auditory brainstem responses (ABRs) which measure population activity including synaptic field potentials for threshold determination (Rotschafer et al., 2015; McCullagh et al., 2020b; Chawla and McCullagh, 2022). These thresholds always take into account the total number of neurons being activated and may therefore differ, when compared to thresholds obtained by recordings of single neuron action potentials. Previous studies on response thresholds using single cell recordings did not yield threshold differences between genotypes or even showed a trend for lower thresholds in FMR1 -/- mice similar to our data (Garcia-Pino et al., 2017; Nguyen et al., 2020).
We observed a significant increase in firing rate in Fmr1 -/- mice only in sustained neurons. As the proportion of transient to sustained firing neurons also increased in Fmr1-/-, the interpretation of this result is difficult. It is possible that firing patterns have changed upon gene deletion resulting in different categorization of neurons. Nevertheless, these data indicate that a transition of onset or transient firing neurons in WT to sustained firing neurons in Fmr1 -/- mice, as previously shown in the LSO (Garcia-Pino et al., 2017), is not occurring in the IC. Similar increases in IC firing rate have been reported in previous studies and were either small (Mott and Wei, 2014) or selective to neurons with CF below 20 kHz (Nguyen et al., 2020). Taken together, the data suggest that firing rates in Fmr1 -/- mice are not substantially increasing between the auditory brainstem and IC, possibly due to homeostatic compensatory mechanisms being activated. Recordings from awake behaving mice may shed further light on the correlation of neuronal activity patterns with the clear acoustic hypersensitivity observed in FXS (Sinclair et al., 2017).
Similar to previous studies we measured broader frequency tuning in Fmr1 -/- neurons (Rotschafer and Razak, 2013; Mott and Wei, 2014; Nguyen et al., 2020), but tuning width, as quantified by Q-values, was only significant in transient firing neurons. Tuning bandwidth in Fmr1 -/- LSO neurons is also significantly wider, which we suggested to be a reflection of reduced pruning of excitatory inputs during development (Garcia-Pino et al., 2017). Since frequency response tuning in the IC is not a mere projection from the auditory brainstem but reshaped by the interaction of excitatory and inhibitory inputs (Alkhatib et al., 2006; Lee et al., 2019), the broadening of tuning in Fmr1 -/- neurons may also occur in the IC directly.
Our data also suggest that ILD tuning in the IC is severely affected by Fmr1 deletion with ipsilateral evoked inhibition being weakened. This is different to the changes in ILD representation in the LSO of Fmr1 -/- mice where ipsilateral excitation was exaggerated whereas contralateral inhibition was largely unaffected (Garcia-Pino et al., 2017). One possible explanation may be that in adult animals, inhibition to LSO neurons is transmitted by glycine, whereas in the IC GABA receptors predominate. Indeed, GABAergic, parvalbumin expressing neurons or reduced GABA transmission was observed in cortical circuits of Fmr1 -/- mice (Selby et al., 2007; Song et al., 2022). Many binaural features in the IC are either refined or even created de novo in the IC (Park et al., 2004; Pollak, 2012), and GABAergic inhibitory inputs either from the dorsal nucleus of the lateral lemniscus or from the opposite IC may be impaired by Fmr1 deletion.
In general, response features of IC neurons in Fmr1 -/- are overall not much different to neurons in the auditory brainstem indicating homeostatic compensatory mechanisms to be effective and thus preventing the development of exaggerated spike responses in the IC. These compensatory mechanisms seem to be activated later during development, since a previous study suggests that response increases in the IC are largest in juvenile Fmr1 -/- animals (P14-P21) and decreases again once animals have reached adulthood (P34) (Nguyen et al., 2020).
Enhanced activity to the offset of a sound in Fmr1 -/- mice
An interesting feature we found was an increased offset response in Fmr1 -/- mice in both transient and sustained firing neurons. Generally, largest offset responses were elicited in both genotypes, when neurons were stimulated above or below their excitatory frequency response area, where inhibitory inputs predominate. Indeed, offset spiking, defined as spiking activity in response to the end of a sound stimulus, has been reported in the IC (Tan et al., 2007; Kasai et al., 2012) and in other nuclei of the auditory brainstem, especially in neurons of the superior paraolivary nucleus (Felix et al., 2011; Kopp-Scheinpflug et al., 2011). In the IC, offset activity may be evoked by excitatory synaptic inputs occurring after sound termination or by inhibitory rebound activity (Kasai et al., 2012). The temporal pattern of the excitatory and inhibitory responses to the beginning and the end of a sound stimulus also determines the selectivity of neurons to the duration of a sound (Ono and Oliver, 2014; Valdizón-Rodríguez and Faure, 2017; Valdizón-Rodríguez et al., 2019). What are the possible underlying cellular mechanisms associated with this enhanced offset activity in Fmr1 -/- neurons? Rebound spiking from inhibition depends on the interplay of inhibitory inputs in combination with the activation of Hyperpolarization-activated and Cyclic Nucleotide gated (HCN) channel and in many cases low-threshold calcium-channels (Kopp-Scheinpflug et al., 2011, 2018, Naumov et al., 2019). Enhancement in any of these ionic currents may lead to the increased offset activity in Fmr1 -/- mice. However, previous studies on inhibitory synaptic transmission indicate a reduced inhibitory transmission and not an enhanced inhibitory transmission (Cellot and Cherubini, 2014), whereas HCN channel activity can be modulated in both directions by Fmr1 deletion (Brager et al., 2012; Brandalise et al., 2020). Functionally, this enhanced representation of sound termination in IC neurons of Fmr1-/- may contribute to the observed deficits in the processing of speech and communication sounds in humans with FXS and in Fragile X animal models (Engineer et al., 2014; McCullagh et al., 2020b).
Conclusion
Despite almost three decades of research on the Fragile X mouse model, we still fail to precisely understand where and how the genetic malformation impairs the brain and its auditory system in particular. Recent evidence suggests that the IC plays a predominant role in FXS as the deletion of Fmr1 -/- expression specifically in VGlut2 neurons both the cortex and the IC is sufficient to induce audiogenic seizure, while IC Ntsr1 specific Fmr1 deletion is necessary but not sufficient to induce audiogenic seizure in the Cortex (Gonzalez et al., 2019).
Regarding this fact, it is surprising to see spike rates in IC neurons of Fmr1 -/- mice being only moderately elevated, considering how strong the acoustic behavior of FXS patients and mice is affected. One likely explanation is that anesthesia has different effects in the genotypes and therefore reduces the robust genotype effects observed in awake behaving animals. The larger and faster excitation seems to be present at every stage of the ascending auditory system, however, upregulation of inhibition, probably by homeostatic regulation, may come into place at the level of the IC and prevent IC neurons from overexcitation. These compensatory mechanisms may directly influence spike patterns, as we observed with the enhanced offset activity to the end of a sound stimulus. To what extent this impairs the analysis of complex sound patterns needs to be addressed in future studies.
Data availability statement
The raw data supporting the conclusions of this article will be made available by the authors, without undue reservation.
Ethics statement
This animal study was reviewed and approved by the Landesamt für Gesundheit und Soziales, Berlin, Germany.
Author contributions
JS and UK contributed to the conception and design of the study and wrote the manuscript. JS performed the experiments and analyzed the data. All authors contributed to the article and approved the submitted version.
Funding
This study was provided by the Freie Universität Berlin (UK), the SFB665 (UK), and the DFG Emmy-Noether grant KR 4062/4-1 (JK). We acknowledge support by the Open Access Publication Initiative of Freie Universität Berlin.
Conflict of interest
The authors declare that the research was conducted in the absence of any commercial or financial relationships that could be construed as a potential conflict of interest.
Publisher’s note
All claims expressed in this article are solely those of the authors and do not necessarily represent those of their affiliated organizations, or those of the publisher, the editors and the reviewers. Any product that may be evaluated in this article, or claim that may be made by its manufacturer, is not guaranteed or endorsed by the publisher.
Supplementary material
The Supplementary Material for this article can be found online at: https://www.frontiersin.org/articles/10.3389/fnins.2022.987939/full#supplementary-material
Supplementary Figure 1 | Detailed stereotactic recording locations in both genotypes. (A) Quantification of recording stereotactic depths in respectively: (left) transient neurons, (middle left) sustained neurons, (middle right) inhibited neurons, (right) offset neurons. (B) Quantifications of the respective Rostro-Caudal to Medio-Lateral stereotactic recording locations in resp.: (left) transient neurons, (middle left) sustained neurons, (middle right) inhibited neurons, (right) offset neurons.
Supplementary Figure 2 | Localized estimates of the different firing patterns exhibited in defined sub-sections of the FRA. (A) Averaged compound PSTH of all conditions in the FRA for respectively: (above) all transient WT and all Fmr1 -/- neurons, (middle above) all inhibited WT and all Fmr1 -/- neurons, (middle below) all offset WT and all Fmr1 -/- neurons, (below) all sustained WT and all Fmr1 -/- neurons. (B) Averaged PSTH at the CF for all intensity above threshold for respectively: (above) transient WT and Fmr1 -/-, (middle above) inhibited WT and Fmr1 -/-, (middle below) offset WT and Fmr1 -/-, (below) sustained WT and Fmr1 -/-. (C) Averaged PSTH of 0 dB attenuation for all frequencies for respectively: (above) transient WT and Fmr1 -/-, (middle above) inhibited WT and Fmr1 -/-, (middle below) offset WT and Fmr1 -/-, (below) sustained WT and Fmr1 -/-. (D) Averaged PSTH of 1 kHz pure tone stimulations at all intensities for respectively: (above) transient WT and Fmr1 -/-, (middle above) inhibited WT and Fmr1 -/-, (middle below) offset WT and Fmr1 -/-, (below) sustained WT and Fmr1 -/-. (E) Schematic overview of a FRA with red highlights the different sub-section chosen for the illustrated PSTH. (F) Averaged PSTH of 40 kHz pure tone stimulation for all intensities for respectively: (above) transient WT and Fmr1 -/-, (middle above) inhibited WT and Fmr1 -/-, (middle below) offset WT and Fmr1 -/-, (below) sustained WT and Fmr1 -/-.
Supplementary Figure 3 | Neurons in the IC exhibit poor stimulus-specific adaptaion. (A) Schematic FRA and the location around the CF chosen to identify f1 and f2. (B) Representation of (blue and red) the two frequencies of stimulation with the repeats used to bot oddball protocols. (C) PSTH to the different conditions in an adaptive neuron, (left) response to a (red) f1 odd, (blue) f1 frequent stimulation; (right) responses to an (red) f1 odd, (dashed-blue) f2 frequent stimulations. (D) Quantifications of the SSA to a standard 90/10% oddball paradigm represented according to each neuron’s adaptive responses to both the lower frequency (f1-x-axis) and the higher frequency (f2-y-axis). (E) Averaged adaptation strength to both frequencies quantified by the common SSA index (CSI). F Individual CSI plotted against their respective Q30 in both WT and Fmr1 -/- Transient neurons (left) and Sustained neurons (right). (F) Illustrations of the tuning width versus the CSI values for (left) transient neurons versus (right) Fmr1 -/- neurons.
References
Akimov, A. G., Egorova, M. A., and Ehret, G. (2017). Spectral summation and facilitation in on- and off-responses for optimized representation of communication calls in mouse inferior colliculus. Eur. J. Neurosci. 45, 440–459. doi: 10.1111/ejn.13488
Alkhatib, A., Biebel, U. W., and Smolders, J. W. T. (2006). Inhibitory and excitatory response areas of neurons in the central nucleus of the inferior colliculus in unanesthetized chinchillas. Exp. Brain Res. 174, 124–143. doi: 10.1007/s00221-006-0424-8
Ayala, Y. A., and Malmierca, M. S. (2013). Stimulus-specific adaptation and deviance detection in the inferior colliculus. Front. Neural Circuits 6:89. doi: 10.3389/fncir.2012.00089
Ayala, Y. A., Pérez-González, D., and Malmierca, M. S. (2016). Stimulus-specific adaptation in the inferior colliculus: The role of excitatory, inhibitory and modulatory inputs. Biol. Psychol. 116, 10–22. doi: 10.1016/j.biopsycho.2015.06.016
Brager, D. H., Akhavan, A. R., and Johnston, D. (2012). Impaired Dendritic Expression and Plasticity of h-Channels in the fmr1−/y Mouse Model of Fragile X Syndrome. Cell Rep. 1, 225–233. doi: 10.1016/j.celrep.2012.02.002
Brandalise, F., Kalmbach, B. E., Mehta, P., Thornton, O., Johnston, D., Zemelman, B. V., et al. (2020). Fragile X Mental Retardation Protein Bidirectionally Controls Dendritic Ih in a Cell Type-Specific Manner between Mouse Hippocampus and Prefrontal Cortex. J. Neurosci. 40, 5327–5340. doi: 10.1523/JNEUROSCI.1670-19.2020
Brown, M. R., and Kaczmarek, L. K. (2011). Potassium channel modulation and auditory processing. Hear Res. 279, 32–42. doi: 10.1016/j.heares.2011.03.004
Cellot, G., and Cherubini, E. (2014). GABAergic signaling as therapeutic target for autism spectrum disorders. Child Neurodev. Psychiatry 2:70. doi: 10.3389/fped.2014.00070
Chawla, A., and McCullagh, E. A. (2022). Auditory Brain Stem Responses in the C57BL/6J Fragile X Syndrome-Knockout Mouse Model. Front. Integr. Neurosci. 15:803483. doi: 10.3389/fnint.2021.803483
Choy Buentello, D., Bishop, D. C., and Oliver, D. L. (2015). Differential distribution of GABA and glycine terminals in the inferior colliculus of rat and mouse. J. Comp. Neurol. 523, 2683–2697. doi: 10.1002/cne.23810
Ciaccio, C., Fontana, L., Milani, D., Tabano, S., Miozzo, M., and Esposito, S. (2017). Fragile X syndrome: A review of clinical and molecular diagnoses. Ital. J. Pediatr. 43:39. doi: 10.1186/s13052-017-0355-y
Dimitriadis, G., Neto, J. P., and Kampff, A. R. (2018). t-SNE Visualization of Large-Scale Neural Recordings. Neural Comput. 30, 1750–1774. doi: 10.1162/neco_a_01097
Dolan, B. M., Duron, S. G., Campbell, D. A., Vollrath, B., Shankaranarayana, Rao BS, Ko, H. Y., et al. (2013). Rescue of fragile X syndrome phenotypes in Fmr1 KO mice by the small-molecule PAK inhibitor FRAX486. Proc. Natl. Acad. Sci. U.S.A. 110, 5671–5676. doi: 10.1073/pnas.1219383110
Duque, D., and Malmierca, M. S. (2015). Stimulus-specific adaptation in the inferior colliculus of the mouse: Anesthesia and spontaneous activity effects. Brain Struct. Funct. 220, 3385–3398. doi: 10.1007/s00429-014-0862-1
Duque, D., Pérez-González, D., Ayala, Y. A., Palmer, A. R., and Malmierca, M. S. (2012). Topographic Distribution, Frequency, and Intensity Dependence of Stimulus-Specific Adaptation in the Inferior Colliculus of the Rat. J. Neurosci. 32, 17762–17774. doi: 10.1523/JNEUROSCI.3190-12.2012
Egorova, M. A., Akimov, A. G., Khorunzhii, G. D., and Ehret, G. (2020). Frequency response areas of neurons in the mouse inferior colliculus. III. Time-domain responses: Constancy, dynamics, and precision in relation to spectral resolution, and perception in the time domain. PLoS One 15:e0240853. doi: 10.1371/journal.pone.0240853
Ehret, G., and Romand, R. (1992). Development of tone response thresholds, latencies and tuning in the mouse inferior colliculus. Dev. Brain Res. 67, 317–326. doi: 10.1016/0165-3806(92)90233-M
Ehret, G., Egorova, M., Hage, S. R., and Müller, B. A. (2003). Spatial map of frequency tuning-curve shapes in the mouse inferior colliculus. NeuroReport 14, 1365–1369. doi: 10.1097/00001756-200307180-00017
El-Hassar, L., Song, L., Tan, W. J. T., Large, C. H., Alvaro, G., Santos-Sacchi, J., et al. (2019). Modulators of Kv3 Potassium Channels Rescue the Auditory Function of Fragile X Mice. J. Neurosci. 39, 4797–4813. doi: 10.1523/JNEUROSCI.0839-18.2019
Engineer, C. T., Centanni, T. M., Im, K. W., Rahebi, K. C., Buell, E. P., and Kilgard, M. P. (2014). Degraded speech sound processing in a rat model of fragile X syndrome. Brain Res. 1564, 72–84. doi: 10.1016/j.brainres.2014.03.049
Ethridge, L., Thaliath, A., Kraff, J., Nijhawan, K., and Berry-Kravis, E. (2020). Development of Neural Response to Novel Sounds in Fragile X Syndrome: Potential Biomarkers. Am. J. Intellect. Dev. Disabil. 125, 449–464. doi: 10.1352/1944-7558-125.6.449
Felix, R. A., Fridberger, A., Leijon, S., Berrebi, A. S., and Magnusson, A. K. (2011). Sound Rhythms Are Encoded by Postinhibitory Rebound Spiking in the Superior Paraolivary Nucleus. J. Neurosci. 31, 12566–12578. doi: 10.1523/JNEUROSCI.2450-11.2011
Finestack, L. H., Richmond, E. K., and Abbeduto, L. (2009). Language Development in Individuals With Fragile X Syndrome. Top Lang. Disord. 29, 133–148. doi: 10.1097/TLD.0b013e3181a72016
Garcia-Pino, E., Gessele, N., and Koch, U. (2017). Enhanced Excitatory Connectivity and Disturbed Sound Processing in the Auditory Brainstem of Fragile X Mice. J. Neurosci. 37, 7403–7419. doi: 10.1523/JNEUROSCI.2310-16.2017
Gittelman, J., Wang, L., Colburn, S., and Pollak, G. (2012). Inhibition shapes response selectivity in the inferior colliculus by gain modulation. Front. Neural Circuits 6:67. doi: 10.3389/fncir.2012.00067
Gonzalez, D., Tomasek, M., Hays, S., Sridhar, V., Ammanuel, S., Chang, C., et al. (2019). Audiogenic Seizures in the Fmr1 Knock-Out Mouse Are Induced by Fmr1 Deletion in Subcortical, VGlut2-Expressing Excitatory Neurons and Require Deletion in the Inferior Colliculus. J. Neurosci. 39, 9852–9863. doi: 10.1523/JNEUROSCI.0886-19.2019
Gourévitch, B., Mahrt, E. J., Bakay, W., Elde, C., and Portfors, C. V. (2020). GABAA receptors contribute more to rate than temporal coding in the IC of awake mice. J. Neurophysiol. 123, 134–148. doi: 10.1152/jn.00377.2019
Ho, M. K., Li, X., Wang, J., Ohmen, J. D., and Friedman, R. A. (2014). FVB/NJ mice demonstrate a youthful sensitivity to noise-induced hearing loss and provide a useful genetic model for the study of neural hearing loss. Audiol. Neurotol. Extra 4, 1–11. doi: 10.1159/000357770
Ito, T. (2020). Different coding strategy of sound information between GABAergic and glutamatergic neurons in the auditory midbrain. J. Physiol. 598, 1039–1072. doi: 10.1113/JP279296
Kasai, M., Ono, M., and Ohmori, H. (2012). Distinct neural firing mechanisms to tonal stimuli offset in the inferior colliculus of mice in vivo. Neurosci. Res. 73, 224–237. doi: 10.1016/j.neures.2012.04.009
Kopp-Scheinpflug, C., Sinclair, J. L., and Linden, J. F. (2018). When Sound Stops: Offset Responses in the Auditory System. Trends Neurosci. 41, 712–728. doi: 10.1016/j.tins.2018.08.009
Kopp-Scheinpflug, C., Tozer, A. J. B., Robinson, S. W., Tempel, B. L., Hennig, M. H., and Forsythe, I. D. (2011). The Sound of Silence: Ionic Mechanisms Encoding Sound Termination. Neuron 71, 911–925. doi: 10.1016/j.neuron.2011.06.028
Lee, E. K., Balasubramanian, H., Tsolias, A., Anakwe, S. U., Medalla, M., Shenoy, K. V., et al. (2021). Non-linear dimensionality reduction on extracellular waveforms reveals cell type diversity in premotor cortex Salinas E, Frank MJ, eds. eLife 10:e67490. doi: 10.7554/eLife.67490
Lee, J., Lin, J., Rabang, C., and Wu, G. K. (2019). Differential Inhibitory Configurations Segregate Frequency Selectivity in the Mouse Inferior Colliculus. J. Neurosci. 39, 6905–6921. doi: 10.1523/JNEUROSCI.0659-19.2019
Lesicko, A. M. H., Hristova, T. S., Maigler, K. C., and Llano, D. A. (2016). Connectional Modularity of Top-Down and Bottom-Up Multimodal Inputs to the Lateral Cortex of the Mouse Inferior Colliculus. J. Neurosci. 36, 11037–11050. doi: 10.1523/JNEUROSCI.4134-15.2016
Malmierca, M. S., Cristaudo, S., Pérez-González, D., and Covey, E. (2009). Stimulus-Specific Adaptation in the Inferior Colliculus of the Anesthetized Rat. J. Neurosci. 29, 5483–5493. doi: 10.1523/JNEUROSCI.4153-08.2009
McCullagh, E. A., Rotschafer, S. E., Auerbach, B. D., Klug, A., Kaczmarek, L. K., Cramer, K. S., et al. (2020b). Mechanisms underlying auditory processing deficits in Fragile X syndrome. FASEB J. 34, 3501–3518. doi: 10.1096/fj.201902435R
McCullagh, E. A., Poleg, S., Greene, N. T., Huntsman, M. M., Tollin, D. J., and Klug, A. (2020a). Characterization of Auditory and Binaural Spatial Hearing in a Fragile X Syndrome Mouse Model. eNeuro 7:ENEURO.0300–19.2019. doi: 10.1523/ENEURO.0300-19.2019
McCullagh, E. A., Salcedo, E., Huntsman, M. M., and Klug, A. (2017). Tonotopic alterations in inhibitory input to the medial nucleus of the trapezoid body in a mouse model of Fragile X syndrome. J. Comp. Neurol. 25, 3543–3562. doi: 10.1002/cne.24290
Mott, B., and Wei, S. (2014). Firing Property of Inferior Colliculus Neurons Affected by FMR1 Gene Mutation. J. Otol. 9, 86–90. doi: 10.1016/S1672-2930(14)50020-7
Nasimi, A., and Rees, A. (2010). Regularly firing neurons in the inferior colliculus have a weak interaural intensity difference sensitivity. J. Comp. Physiol. A 196, 889–897. doi: 10.1007/s00359-010-0532-6
Naumov, V., Heyd, J., de Arnal, F., and Koch, U. (2019). Analysis of excitatory and inhibitory neuron types in the inferior colliculus based on Ih properties. J. Neurophysiol. 121, 2126–2139. doi: 10.1152/jn.00594.2018
Nguyen, A. O., Binder, D. K., Ethell, I. M., and Razak, K. A. (2020). Abnormal development of auditory responses in the inferior colliculus of a mouse model of Fragile X Syndrome. J. Neurophysiol. 123, 2101–2121. doi: 10.1152/jn.00706.2019
Olthof, B. M. J., Rees, A., and Gartside, S. E. (2019). Multiple Nonauditory Cortical Regions Innervate the Auditory Midbrain. J. Neurosci. 39, 8916–8928. doi: 10.1523/JNEUROSCI.1436-19.2019
Ono, M., and Oliver, D. L. (2014). Asymmetric temporal interactions of sound-evoked excitatory and inhibitory inputs in the mouse auditory midbrain. J. Physiol. 592, 3647–3669. doi: 10.1113/jphysiol.2014.275446
Palmer, A. R., Shackleton, T. M., Sumner, C. J., Zobay, O., and Rees, A. (2013). Classification of frequency response areas in the inferior colliculus reveals continua not discrete classes. J. Physiol. 591, 4003–4025. doi: 10.1113/jphysiol.2013.255943
Park, T. J., Klug, A., Holinstat, M., and Grothe, B. (2004). Interaural Level Difference Processing in the Lateral Superior Olive and the Inferior Colliculus. J. Neurophysiol. 92, 289–301. doi: 10.1152/jn.00961.2003
Pollak, G. D. (2012). Circuits for processing dynamic interaural intensity disparities in the inferior colliculus. Hear Res. 288, 47–57. doi: 10.1016/j.heares.2012.01.011
Rais, M., Binder, D. K., Razak, K. A., and Ethell, I. M. (2018). Sensory Processing Phenotypes in Fragile X Syndrome. ASN Neuro 10:175909141 8801092. doi: 10.1177/1759091418801092
Razak, K. A., Binder, D. K., and Ethell, I. M. (2021). Neural Correlates of Auditory Hypersensitivity in Fragile X Syndrome. Front. Psychiatry 12:720752. doi: 10.3389/fpsyt.2021.720752
Ribak, C. E. (2017). An Abnormal GABAergic System in the Inferior Colliculus Provides a Basis for Audiogenic Seizures in Genetically Epilepsy-Prone Rats. Epilepsy Behav. 71, 160–164. doi: 10.1016/j.yebeh.2015.02.024
Ribak, C. E., and Morin, C. L. (1995). The role of the inferior colliculus in a genetic model of audiogenic seizures. Anat. Embryol. 1, 279–295. doi: 10.1007/BF00534681
Rotschafer, S. E., Marshak, S., and Cramer, K. S. (2015). Deletion of Fmr1 Alters Function and Synaptic Inputs in the Auditory Brainstem. PLoS One 10:e0117266. doi: 10.1371/journal.pone.0117266
Rotschafer, S., and Razak, K. (2013). Altered auditory processing in a mouse model of fragile X syndrome. Brain Res. 1506, 12–24. doi: 10.1016/j.brainres.2013.02.038
Ruby, K., Falvey, K., and Kulesza, R. J. (2015). Abnormal neuronal morphology and neurochemistry in the auditory brainstem of Fmr1 knockout rats. Neuroscience 10, 285–298. doi: 10.1016/j.neuroscience.2015.06.061
Selby, L., Zhang, C., and Sun, Q.-Q. (2007). Major defects in neocortical GABAergic inhibitory circuits in mice lacking the fragile X mental retardation protein. Neurosci. Lett. 412, 227–232. doi: 10.1016/j.neulet.2006.11.062
Sinclair, D., Oranje, B., Razak, K. A., Siegel, S. J., and Schmid, S. (2017). Sensory processing in autism spectrum disorders and Fragile X syndrome-From the clinic to animal models. Neurosci. Biobehav. Rev. 76, 235–253. doi: 10.1016/j.neubiorev.2016.05.029
Song, Y. J., Xing, B., Barbour, A. J., Zhou, C., and Jensen, F. E. (2022). Dysregulation of GABAA Receptor-Mediated Neurotransmission during the Auditory Cortex Critical Period in the Fragile X Syndrome Mouse Model. Cereb. Cortex 32, 197–215.
Strumbos, J. G., Brown, M. R., Kronengold, J., Polley, D. B., and Kaczmarek, L. K. (2010). Fragile X Mental Retardation Protein Is Required for Rapid Experience-Dependent Regulation of the Potassium Channel Kv3.1b. J. Neurosci. 30, 10263–10271. doi: 10.1523/JNEUROSCI.1125-10.2010
Tan, M. L., Theeuwes, H. P., Feenstra, L., and Borst, J. G. G. (2007). Membrane properties and firing patterns of inferior colliculus neurons: An in vivo patch-clamp study in rodents. J. Neurophysiol. 98, 443–453. doi: 10.1152/jn.01273.2006
Turner, G., Webb, T., Wake, S., and Robinson, H. (1996). Prevalence of fragile X syndrome. Am. J. Med. Genet. 64, 196–197. doi: 10.1002/(SICI)1096-8628(19960712)64:1<196::AID-AJMG35>3.0.CO;2-G
Ulanovsky, N., Las, L., and Nelken, I. (2003). Processing of low-probability sounds by cortical neurons. Nat. Neurosci. 6, 391–398. doi: 10.1038/nn1032
Valdizón-Rodríguez, R., and Faure, P. A. (2017). Frequency tuning of synaptic inhibition underlying duration-tuned neurons in the mammalian inferior colliculus. J. Neurophysiol. 117, 1636–1656. doi: 10.1152/jn.00807.2016
Valdizón-Rodríguez, R., Kovaleva, D., and Faure, P. A. (2019). Effect of sound pressure level on contralateral inhibition underlying duration-tuned neurons in the mammalian inferior colliculus. J. Neurophysiol. 122, 184–202. doi: 10.1152/jn.00653.2018
Wallace, M. N., Shackleton, T. M., Thompson, Z., and Palmer, A. R. (2021). Juxtacellular Labeling of Stellate, Disk and Basket Neurons in the Central Nucleus of the Guinea Pig Inferior Colliculus. Front. Neural Circuits 15:721015. doi: 10.3389/fncir.2021.721015
Wang, T., de Kok, L., Willemsen, R., Elgersma, Y., and Borst, J. G. G. (2015). In vivo synaptic transmission and morphology in mouse models of Tuberous sclerosis, Fragile X syndrome, Neurofibromatosis type 1, and Costello syndrome. Front. Cell. Neurosci. 9:234. doi: 10.3389/fncel.2015.00234
Willott, J. F., Parham, K., and Hunter, K. P. (1988). Response properties of inferior colliculus neurons in middle-aged C57BL/6J mice with presbycusis. Hear Res. 37, 15–27. doi: 10.1016/0378-5955(88)90074-3
Wong, A. B., and Borst, J. G. G. (2019). Tonotopic and non-auditory organization of the mouse dorsal inferior colliculus revealed by two-photon imaging King AJ, Bathellier B, Bathellier B, Dahmen JC, Llano D, eds. eLife 8:e49091. doi: 10.7554/eLife.49091
Xie, R., Gittelman, J. X., and Pollak, G. D. (2007). Rethinking tuning: In vivo whole-cell recordings of the inferior colliculus in awake bats. J. Neurosci. 27, 9469–9481. doi: 10.1523/JNEUROSCI.2865-07.2007
Keywords: inferior colliculus, Fragile X syndrome, auditory processing, auditory hypersensitivity, spike pattern, autism spectrum disorder
Citation: Sibille J, Kremkow J and Koch U (2022) Absence of the Fragile X messenger ribonucleoprotein alters response patterns to sounds in the auditory midbrain. Front. Neurosci. 16:987939. doi: 10.3389/fnins.2022.987939
Received: 06 July 2022; Accepted: 26 August 2022;
Published: 16 September 2022.
Edited by:
Manuel S. Malmierca, University of Salamanca, SpainReviewed by:
Alice Lisa Burghard, UCONN Health, United StatesElizabeth Anne McCullagh, Oklahoma State University, United States
Charles H. Large, Autifony Therapeutics Ltd., United Kingdom
Copyright © 2022 Sibille, Kremkow and Koch. This is an open-access article distributed under the terms of the Creative Commons Attribution License (CC BY). The use, distribution or reproduction in other forums is permitted, provided the original author(s) and the copyright owner(s) are credited and that the original publication in this journal is cited, in accordance with accepted academic practice. No use, distribution or reproduction is permitted which does not comply with these terms.
*Correspondence: Jérémie Sibille, jeremie.sibille@charite.de, jeremie.sibille@college-de-france.fr; Ursula Koch, ursula.Koch@fu-berlin.de