- Institute of Physiology, Department of Physiology and Medical Physics, Medical University Innsbruck, Innsbruck, Austria
Increasing evidence links the gut microbiome and the nervous system in health and disease. This narrative review discusses current views on the interaction between the gut microbiota, the intestinal epithelium, and the brain, and provides an overview of the communication routes and signals of the bidirectional interactions between gut microbiota and the brain, including circulatory, immunological, neuroanatomical, and neuroendocrine pathways. Similarities and differences in healthy gut microbiota in humans and mice exist that are relevant for the translational gap between non-human model systems and patients. There is an increasing spectrum of metabolites and neurotransmitters that are released and/or modulated by the gut microbiota in both homeostatic and pathological conditions. Dysbiotic disruptions occur as consequences of critical illnesses such as cancer, cardiovascular and chronic kidney disease but also neurological, mental, and pain disorders, as well as ischemic and traumatic brain injury. Changes in the gut microbiota (dysbiosis) and a concomitant imbalance in the release of mediators may be cause or consequence of diseases of the central nervous system and are increasingly emerging as critical links to the disruption of healthy physiological function, alterations in nutrition intake, exposure to hypoxic conditions and others, observed in brain disorders. Despite the generally accepted importance of the gut microbiome, the bidirectional communication routes between brain and gut are not fully understood. Elucidating these routes and signaling pathways in more detail offers novel mechanistic insight into the pathophysiology and multifaceted aspects of brain disorders.
1 Introduction
Research in recent decades has explored the relationship between the gut and the brain, including inflammatory processes in the intestine, acute and chronic stress, cognitive deficits, and mental disorders (Rhee et al., 2009; Cryan and O’Mahony, 2011; Cryan et al., 2019). Although there is ample literature on bidirectional communication between the gut microbiome, and the brain some findings are ambiguous, sometimes even contradictory. Balancing microbiota in the gut may be a practical and appropriate method for improving mental diseases (Li R. et al., 2022). Some so-called “psychobiotic” probiotic supplementations containing Bifidobacterium bifidum, Bifidobacterium longus, Lactobacillus reuteri, Lactobacillus acidophilus, Lactobacillus plantarum, Lactobacillus casei and Lactobacillus rhamnosus for example attenuate anxiety behavior in neuropathic mice (Zhang et al., 2023). Therefore, the complex bidirectional interactions between microbiota and their host are gaining increasing interest in biomedicine.
2 Microbiota in the healthy intestine of mice and humans
The earliest evidence of microbial structures in seawater dates back to 3,700–3,800 million years ago (Nutman et al., 2016). The land surface, particularly in South Africa, was colonized around 3,220 million years ago (Homann et al., 2018). Since then, microbiota have developed symbiotic entities with other species whose complex rules and bidirectional interactions are beneficial for both the microbiota and the host. The host’s outer and inner surfaces such as skin or gastrointestinal epithelia are densely inhabited by microorganisms, and the human microbiome in the gut comprises an impressive number and diversity of microorganisms with numerous co-evolutionary associations (Kim et al., 2018). Due to the co-evolution of host and microbiota, symbiotic relationships have evolved, in which the bidirectional interactions between the host and their microflora influence health and disease, for example by impacting host energy, lipid and carbohydrate homeostasis as well as the physiology of organs like kidney, liver, heart or brain (see Sekirov et al., 2010; Cani and Knauf, 2016; Adolph et al., 2018; Stavropoulou et al., 2020; Zheng and Wang, 2021; Shahab and Shahab, 2022; Glorieux et al., 2023; Hsu and Schnabl, 2023; Nesci et al., 2023). Bacterial colonization of the gastrointestinal (GI) tract plays a dominant role in processes of human post-natal development and maturation of the immune, endocrine, and central nervous systems (CNS) (Afzal et al., 2020; Banfi et al., 2021; Hill et al., 2021; Ahmed et al., 2022; Yousefi et al., 2022; Sasso et al., 2023; Van Pee et al., 2023). Important interactions in particular between the microbiota in the gut and the host’s body and even brain as well as disturbed body-to-brain loops have been associated with mental disorders (Bravo et al., 2011; Cryan and Dinan, 2012; Jiang et al., 2017; Heintz-Buschart and Wilmes, 2018; Kim and Shin, 2018; Bassett et al., 2019; Cryan et al., 2019; Mörkl et al., 2020; Megur et al., 2021; Oroojzadeh et al., 2022).
The content of human and mouse gut microbiome show 90 and 89% similarity in phyla and genera (Krych et al., 2013). At first glance, these may seem to indicate a high similarity between the gut microflora of humans and rodents, however key differences, especially in the composition and number of microbes, exist particularly in humans. The ratio of the main phyla Firmicutes/Bacteroidetes is higher in humans compared to mice (Guinane et al., 2013; Krych et al., 2013; Alkadhi et al., 2014; Nagpal et al., 2018). Expectedly, the gut microbiota in humans seems to be closer to non-human primates than to mice (Table 1), and the differences in the qualitative composition of the microbiome may be related to the differences in nervous system function limiting the suitability and reproduction of humanized gnotobiotic mouse models, especially if the respective bаcteria have host-specific physiological effects (Park and Im, 2020).
Although viruses are the most ubiquitous living species on the planet, their involvement is often overlooked as a component of the gut microbiome, which, as part of the “gut virome” (Liang and Bushman, 2021), is dominated by the bacteriophages Caudovirales and Microviridae. Individuals with elevated levels of Caudovirales and Siphoviridae in the gut perform better in executive functioning and verbal memory. In contrast, increased levels of Microviridae correlate with deteriorating executive functioning. Transplantation of microbiota from human donors with a high content of specific Caudovirales (>90% from the Siphoviridae family) improves the recognition of new objects in mice and upregulates genes affecting memory development in the prefrontal cortex suggesting that the gut virome is moving into focus as an important player in the gut-brain axis (Mayneris-Perxachs et al., 2022).
3 Interactions of gut microbiota with epithelia and gut function
The gut epithelium serves important functions, such as nutrient absorption, water and salt homeostasis, surveillance of luminal content as well as protection of the body by building a physicochemical barrier and collaborating with the immune system (Kim and Ho, 2010; Sommer and Backhed, 2013; Konig et al., 2016; Allaire et al., 2018a; Soderholm and Pedicord, 2019). The epithelium of the gut is made up of a monolayer of intestinal epithelial cells (IEC), absorptive enterocytes (EC, small intestine) or colonocytes (CC, colon), as well as enteroendocrine (EEC) and enterochromaffin cells (ECC), goblet cells (GC), paneth cells (PC), microfold cells (M cells; MC) or tuft cells (TC). IEC respond to luminal content, contribute to nutrient, water, and ion absorption, or sense and transfer information about luminal content including microbiota to the lamina propria for further processing, e.g., by cells of the innate and adaptive immune system or the nervous system (Nagler-Anderson, 2001; Rhee et al., 2009; Peterson and Artis, 2014; Konig et al., 2016; Allaire et al., 2018a; Kayama et al., 2020; Gershon and Margolis, 2021; Iftekhar and Sigal, 2021). An excerpt of the functions of IEC is listed in Table 2.
The lamina propria of the gut is innervated by primary afferent neurons (PANs), which are either of extrinsic (dorsal root ganglia and vagal) or intrinsic (enteric) origin (Gershon and Margolis, 2021). PANs can be activated by mechanical and chemical signals, and thus transfer information on the status and content of the gut to the nervous (enteric and central), immune, and hormone system, allowing the integration of signals to promote gut and whole-body homeostasis (Rhee et al., 2009; Abdullah et al., 2020; Gershon and Margolis, 2021; Sharkey and Mawe, 2023). Gut innervating nociceptor neurons for example support the mucosal barrier by influencing intestinal microbiota composition (Zhang et al., 2022) and by activating mucus secretion of goblet cells (Yang et al., 2022).
Microbial density and diversity increases along the longitudinal as well as the transverse axis of the gut, e.g., from the duodenum to the colon and the apical side of the epithelium to the lumen (Sekirov et al., 2010; Sommer and Backhed, 2013; Donaldson et al., 2016; Tropini et al., 2017). In the mouse colon for example, the outer but not the inner of the two mucus layers that cover the luminal side of the epithelium is colonized by bacteria (Johansson et al., 2008; Hansson, 2020). The gut epithelial barrier combines physical (e.g., tight intestinal epithelium, glycocalyx of intestinal epithelial cells, secreted mucus layers), chemical (for example secreted antimicrobials like C-type lectins, cathelicidins, defensins, lactoferrin, lysozyme, Lypd8, or secretory immunoglobulin A), immune and microbial barriers. Collectively, these barriers separate and protect the host from microbiota including pathogens, facilitate the entrapment and subsequent removal of pathogens by intestinal motility (peristalsis), contribute to the regulation of the microbiota population, and help maintain mucosal and immune cell homeostasis (Mavris and Sansonetti, 2004; Peterson and Artis, 2014; Hansson, 2020; Kayama et al., 2020; Shu et al., 2023). However, when the intestinal barrier is compromised, pathogens may breach the barrier leading to infectious and inflammatory diseases, as observed for example in inflammatory bowel disease (IBD) or pancreatitis (Shu et al., 2023). Pathogens employ various approaches to overcome the intestinal barrier, for example through pili and fimbriae to attach to epithelial cells, virulence factors like lipopolysaccharides, toxins, and enzymes, or the use of a secretion system to modify epithelial cells (Mavris and Sansonetti, 2004; Shu et al., 2023). Manipulation of the intestinal barrier by pathogens affects epithelial permeability for example via alterations of epithelial cell tight junctions, epithelial repair, or IEC renewal, differentiation, and apoptosis (Mavris and Sansonetti, 2004; Shu et al., 2023). The pathological condition of a compromised integrity of the intestinal barrier is called “leaky gut syndrome,” and is provoked by for example physical, environmental, or psychological stressors. The “leaky gut syndrome” is accompanied by a systemic pro-inflammatory response, bacterial translocation, and disturbed immune homeostasis (Kinashi and Hase, 2021; Álvarez-Herms et al., 2023). In more severe instances, this could potentially result in IBD or other clinically significant consequences (Camilleri, 2019).
Microbial sensing by gut epithelia is achieved by pattern recognition receptors (PRRs) expressed by epithelial and innate immune cells. PRRs such as toll-like (TLRs), NOD-like (NLRs), RIG-1-like (RLRs), or C-type lectin receptors (CLRs) recognize pathogen-associated or (more generally) microbe-associated molecular patterns (PAMPs, MAMPs) like flaggelin, peptidoglycans, lipopeptides, lipopolysaccharides (LPS), and others (Eckmann, 2004; Mavris and Sansonetti, 2004; Wells et al., 2011; Bishu, 2016; Coleman and Haller, 2017; Kayama et al., 2020; Coquant et al., 2021). Furthermore, bacterial quorum sensing molecules (QSM) that are secreted and utilized by bacteria to signal and collect information about the properties of their environment, as well as to influence gene expression and group behavior in a bacterial population, can directly or indirectly impact gut physiology (Coquant et al., 2021; Uhlig and Hyland, 2022; Oliveira et al., 2023). By affecting epithelial permeability, IEC viability, migration, and mucus production, as well as innate and adaptive immune cells QSM can for example influence barrier function and immune responses (Coquant et al., 2021; Uhlig and Hyland, 2022).
In addition to pattern recognition receptors described above, microbial sensing by the gut may also involve transient receptor potential (TRP) channels, taste receptors, and aryl hydrocarbon receptors (Najjar et al., 2020; Uhlig and Hyland, 2022). Bacterial signals sensed by IEC are relayed to the lamina propria via the release by epithelial cells of for example nucleotides, neurotransmitters, proteases, chemokines, and cytokines. Epithelial cell mediators may then directly or indirectly affect the innate and adaptive immune system as well as signaling by primary afferent neurons (Wells et al., 2011; Coleman and Haller, 2017; Lomax et al., 2019; Najjar et al., 2020).
4 Bidirectional interaction between gut and brain
Bidirectional brain-gut communication has a significant role in the regulation and modulation of functions of the GI tract, such as secretion, motility and permeability of the intestinal barrier, blood flow intensity, the immune activity of mucus membranes, as well as visceral sensations, in addition to pain. Additionally, the importance of enteric microbiota for brain functions is recently emerging (Rhee et al., 2009; Carabotti et al., 2015; Raskov et al., 2016; Martin et al., 2018; Cryan et al., 2019).
The bidirectional crosstalk involves microbiota colonizing the host surface (gastro-intestinal mucus membranes), organs such as exo- and endocrine glands, immune cells, afferent and efferent neurons, as well as different areas of the brain (Cryan and Dinan, 2012; Montiel-Castro et al., 2013). The brain controls gut function and the intraluminal milieu via the overall control over the autonomic and enteric nervous system modulating motility, secretion, and permeability in the GI tract, and this involves communication to cells in the lamina propria, smooth muscle cells, ECCs, neurons and immune cells in the intestinal wall (Collins et al., 2012; Kim and Shin, 2018; Jain et al., 2023).
5 Routes of communication between gut and brain
Bidirectional loops between the brain and the gut may involve neural, hormonal and immunological routes or a combination thereof (Bravo et al., 2011; Diaz Heijtz et al., 2011; Cryan and Dinan, 2012; Clarke et al., 2013; O’Mahony et al., 2014; Desbonnet et al., 2015; Chen et al., 2017; Jameson and Hsiao, 2018; Bassett et al., 2019; Cryan et al., 2019; Rincel et al., 2019; Mörkl et al., 2020; Rajendiran et al., 2021; Wen et al., 2021; Wang Y. et al., 2021; Connell et al., 2022; Figure 1).
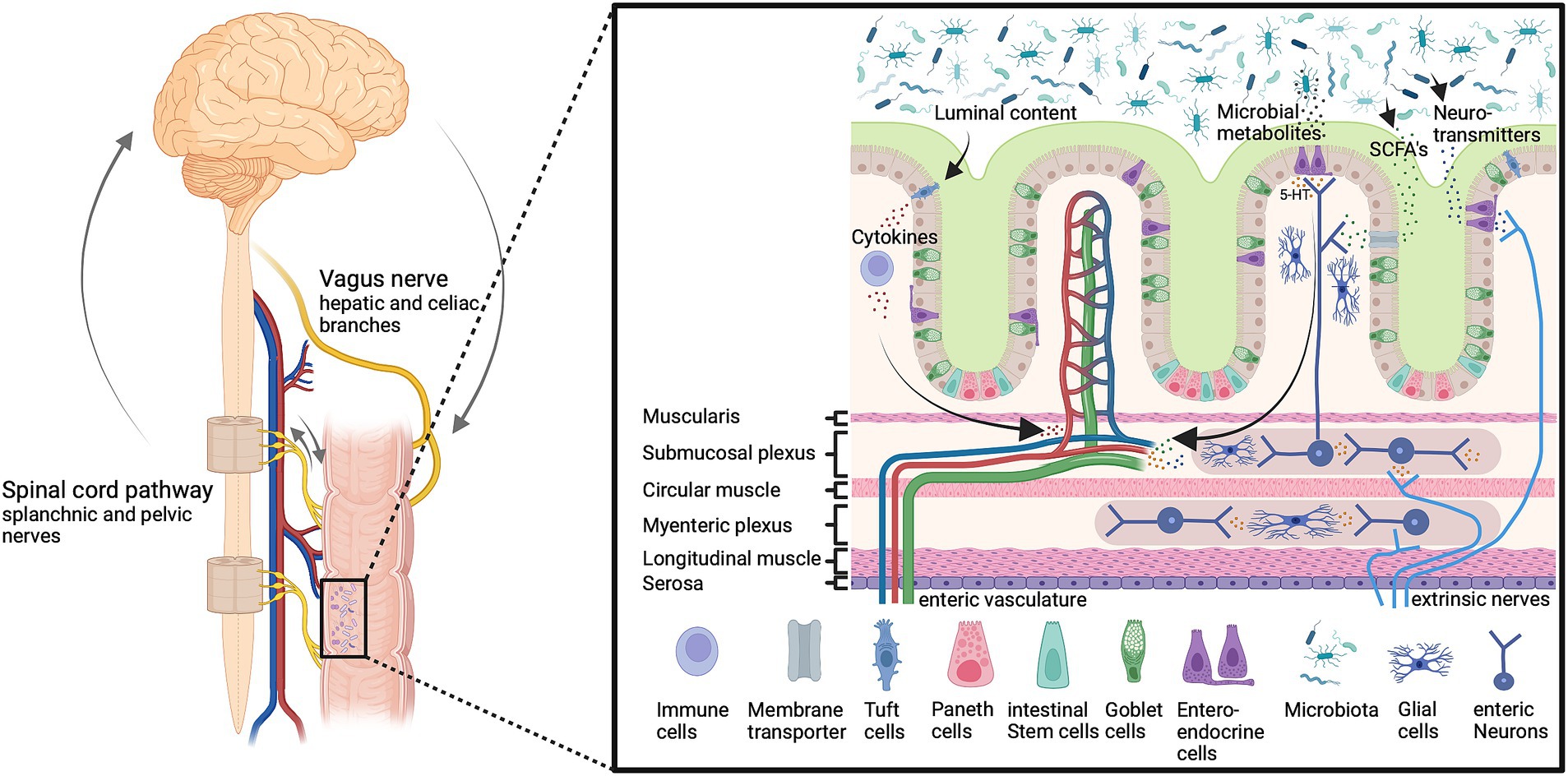
Figure 1. The microbiota-gut-brain-axis. Gut microbiota affect gut permeability and brain function through multiple humoral and neuronal signals and routes, including vagal and spinal afferents as well as circulating metabolites and immune cells. These pathways are important to maintain host homeostasis and gut function. Imbalances can lead to altered neuron function and information processing in the enteric, peripheral, and central nervous system and inflammation, which affects the host health status. Bioactive signals emerge from gut microbiota which releases a multitude of bioactive substances including neurotransmitters such as serotonin (5-HT), and short-chain fatty acids (SCFAs). Created with BioRender.com.
5.1 Vagal afferent and efferent neurons
Visceral afferents innervating the GI tract either travel with the vagus nerve to the brain stem or with peripheral nerves to the spinal cord (Cryan et al., 2019). The vagus nerve contains 80% afferent and 20% efferent nerve fibers that bidirectionally connect the nucleus tractus solitarii (NTS), and the GI tract in a bottom-up and top-down fashion (Agostoni et al., 1957; Harrington et al., 2018; Cryan et al., 2019; Prescott and Liberles, 2022). Glutamatergic vagal afferents project into the NTS working as coordinators and sending feedback from the gut to the brain to the periphery (Altschuler et al., 1993; Cryan et al., 2019; Muller et al., 2020). Their sensory nerve terminals in the muscular layers and mucosa in the vicinity of EECs detect stretch, tension, as well as chemical signals (neurotransmitter, hormones, and metabolites), and transport sensory information from the intestine to the NTS (Berthoud et al., 2004; Furness et al., 2013; Appleton, 2018; Kaelberer et al., 2018; Ye et al., 2021). The efferent fibers connect the vagal dorsal motor nucleus with post-ganglionic and enteric neurons in the GI wall (Bonaz et al., 2021). Enteric neurons regulate peristalsis as well as secretion in the GI tract and the efferent vagal nerve fibers regulate immune cells and the release of inflammatory cytokines in the gut by the release of the neurotransmitter acetylcholine (Pavlov and Tracey, 2005; Chalazonitis and Rao, 2018). The immune cells interact with visceral sensory neurons and resident macrophages are the key immune cells responsible for β-endorphin secretion (Hughes et al., 2014; Veening and Barendregt, 2015). The bidirectional signaling via vagal connections is not only important for gut function but also plays an important role in mood regulation and host behavior (Klarer et al., 2014; Breit et al., 2018; Cryan et al., 2019; Bonaz et al., 2021). Severing the vagus nerve can have a detrimental impact on neurogenesis, impairing proliferation and causing a decline in the quantity of immature neurons in the hippocampus (O’Leary et al., 2018; Kelly et al., 2021).
5.2 Spinal visceral pathways
Due to a rostrocaudal shift in innervation ratio, the predominant afferent innervation of the proximal GI tract is provided through the vagus nerve, while the distal GI tract is mostly innervated by afferent spinal projections (Harrington et al., 2018). The lumbar splanchnic nerve innervates the proximal colon; the distal colon and rectum receive dual innervation both from the lumbar splanchnic and the sacral pelvic nerve, with cell bodies located in the thoracolumbar (TL, T10-L1) and lumbosacral dorsal root ganglia (DRG) (LS, L6-S2) (Ozaki and Gebhart, 2001; Brierley et al., 2018; Harrington et al., 2018). RNA sequencing revealed seven neuronal subtypes, with five of these subtypes almost exclusively abundant in TL, and the other two subtypes in LS spinal levels (Hockley et al., 2019).
DRG neurons projecting to the gut terminate there as intraganglionic laminar endings (IGLEs), intramuscular arrays (IMAs), or mucosal endings (Ozaki and Gebhart, 2001; Harrington et al., 2018; Prescott and Liberles, 2022). Mucosal terminals are important for defecation and stool passage control (Brierley et al., 2018). Approximately 80–100% of the proximal mesenteric but only 10–15% of distal colonic ganglia are innervated by IGLEs, which function as tension receptors. IMAs are located within the circular and longitudinal muscle layer and function as both tension and length receptors (Berthoud et al., 2004; Harrington et al., 2018). Serosa and mesentery are innervated by spinal afferents, the colorectal mesenteric by splanchnic, and the serosa by pelvic and splanchnic nerve fibers (Brierley et al., 2004; Beyak et al., 2006). Sensory nerve terminals are predominantly located in the mucosa, muscle, and serosa extending through the lamina propria into crypts and villi (Berthoud et al., 1995; Beyak et al., 2006; Powley et al., 2011). Peptidergic neurons expressing calcitonin gene-related peptide (CGRP+) are predominantly found in the circular muscle, myenteric ganglia, and submucosa, whereas nonpeptidergic nerve endings (CGRP-) mainly innervate the mucosal crypts, myenteric ganglia, and submucosa suggesting different functions (Brookes et al., 2013; Spencer et al., 2022). Multiple serotonin (5-HT) receptor subtypes are located on spinal afferent nerve terminals in the different layers of the intestine and contribute to the communication with ECCs (Dodds et al., 2022), including 5-HT1-4 and 5-HT7, which are distributed across intrinsic and extrinsic afferent nerve fibers, smooth muscle cells, enterocytes, and immune cells. Among the most studied receptors, the 5-HT3 and 5-HT4 receptors play a pivotal role in gut motility, pain sensation, transit time, and sensitivity. 5-HT3 receptors are mainly expressed by nerve fibers in the submucosa extending into the mucosal layer in close proximity to the ECCs acting as sensors for signals emerging from the ECCs (Mawe and Hoffman, 2013; Kim and Khan, 2014; O’Mahony et al., 2015). ECCs secrete approximately 90% of the body’s 5-HT and via neuronal loops affect gut motility, secretion, and sensitivity by sensing not only nutrients and commensal bacteria but also pathogens, infections, and inflammatory processes (Bellono et al., 2017).
Spinal afferent neurons also serve efferent functions affecting vascular permeability, blood flow, motor reactions, or mucus secretion by releasing neuropeptides such as CGRP or Substance P upon activation (Holzer, 2006). The extrinsic efferent neural pathways regulating the intestine involve the autonomic nervous system, especially sympathetic spinal adrenergic efferents (Andrews and Ashley Blackshaw, 2010). The axons of the efferent fibers run in parallel to those of sensory afferents, with their cell bodies located in the lateral horn of the thoracolumbar spinal cord and traveling through the ventral roots to the abdominal prevertebral sympathetic ganglia (celiac, superior mesenteric, and inferior mesenteric ganglia), projecting to muscles, glands, and target organs (Wood, 2004; Gaman and Kuo, 2008; Kyloh et al., 2022). Due to the highly complex innervation, it has been difficult to experimentally dissect the precise contributions of spinal and vagal pathways within a living animal (Berthoud and Neuhuber, 2000; Sengupta, 2009; Kyloh et al., 2022). Only recently and with the development of novel tracing techniques and optogenetics, thoracolumbar and lumbosacral afferents to the gut are emerging as important for gut innervation, function, and visceral pain (Hibberd et al., 2018; Spencer and Hu, 2020; Kyloh et al., 2022). Nevertheless, comprehensive research is still needed to elucidate the complex interaction routes. Current investigations focus on the neuroimmune axis as well as on the release of neuropeptides among other immune mediators (Holzer and Farzi, 2014; Udit et al., 2022). Direct signaling of neurons can activate immune cells and thereby modulate inflammatory reactions (Udit et al., 2022). Transient receptor potential vanilloid 1 (TRPV1) expressing nociceptor neurons act on the enteric nervous system, as well as intestinal epithelial cells, thereby modulating gut permeability, microbiota abundance, and eliciting the release of signaling molecules by microbiota (Holzer and Farzi, 2014; Udit et al., 2022).
5.3 Humoral pathways
Chemical mediators such as chemokines, neuropeptides, neurotransmitters, endocrine messengers, cytokines, exotoxins, short-chain fatty acids (SCFAs), and other metabolites travel with the blood or lymphatic system (Cryan et al., 2019; Uhlig et al., 2020). The composition of microbiota is directly linked to the generation of SCFAs and each bacterial class generates specific SCFAs. SCFA metabolites not only exert local effects but also affect the host’s glucose homeostasis, satiety, immune system, and brain signaling (Crawford et al., 2022). Dysbiosis can be caused by an increase in sympathetic activity in the gut as a result of acute and chronic stress, which disrupts the intestinal barrier by activating mast cells through corticotropin-releasing hormone, which in turn allows antibodies, microbial metabolites, toxins, and lipopolysaccharides in the gut to enter the systemic circulation (Wallon et al., 2008; Kim and Shin, 2018; Toral et al., 2019; Shu et al., 2023). An important barrier for the humoral communication between the gut and the brain is the blood–brain barrier (BBB), and the significance of gut microbiota for BBB development has recently been shown in germ-free mice, who develop an increased BBB permeability which is restored by the recolonization of microbiota (Cryan et al., 2019). The hypothalamic–pituitary–adrenal (HPA) axis is related to psychological and physical stress and regulates multiple physiological systems including the gut permeability, causing bacterial translocation, and release of messenger substances, which affects the intestinal barrier and BBB permeability (Vagnerová et al., 2019; Geng et al., 2020). GF mice show decreased tyrosine (the rate-limiting substrate of noradrenaline and dopamine synthesis) and increased catecholamine levels which imply that gut microbiota modulate dopamine and noradrenaline turnover in the brain (Matsumoto et al., 2013; Nishino et al., 2013). Interestingly, SCFAs can pass the BBB under healthy conditions whereas neurotransmitters such as gamma-aminobutyric acid (GABA) and 5-HT do not pass in a healthy state, however, this may change during inflammation, allowing them to enter the CNS (Takanaga et al., 2001; Margolis et al., 2021; Modesto Lowe et al., 2023).
6 Metabolites and neurotransmitters secreted by gut microbiota
One of the main functions of intestinal bacteria is to help in food digestion and production of micronutrients that the human organism cannot synthesize on its own (Park and Im, 2020). Host species-specific characteristics of the gut microbiota in some of the most common animal models may reflect differences in host factors, such as diet, genetic background, sex, and age (Nagpal et al., 2018). For example, fecal levels of lactate are higher in mice, while acetate and propionate levels are highest in human feces (Nagpal et al., 2018). These differences may contribute to the translational gap between mice and men specifically regarding modeling brain functions and neuropsychiatric disorders.
6.1 Neurotransmitters
Bacteria produce a multitude of neurotransmitters and biologically active substances. Secondary bile acids and other metabolites are formed from primary bile acids produced by the liver in the intestine as a result of metabolism by the intestinal microbiota (Jiao et al., 2018; Funabashi et al., 2020). Bile acids can control neurotransmitter receptor functions, e.g., muscarinic acetylcholine and GABA receptors, and protect from neurodegeneration (Kiriyama and Nochi, 2019). Several strains of gut bacteria synthesize and release substances acting as or like neurotransmitters such as GABA, 5-HT, tryptamine, acetylcholine, L-dopa, norepinephrine, or histamine (Table 3; Kim et al., 2018). Of these, glutamate acts as a main excitatory neurotransmitter in the central nervous system (Henter et al., 2021), and excitatory amino acids associated with Lactobacillus are discussed as key factors in anxiety-like behavior (Oroojzadeh et al., 2022). The interactions along the gut-brain axis are bidirectional (Zhang et al., 2023) and for example, endogenous cannabinoids not only target neurons and immune cells but also play a major role in metabolism, energy homeostasis, and as regulators of the crosstalk between gut microbiota and host metabolism (Oroojzadeh et al., 2022; Zhang et al., 2023). However, the mutual crosstalk and feedback mechanisms between the CNS and the gut microbiota are still incompletely understood.
6.2 Non-coding RNAs
Only recently, non-coding RNA species, and in particular microRNA (miRNA), are emerging as hub regulators of entire gene sets that are essential for many cellular functions including pluripotency and developmental processes (Lagos-Quintana et al., 2001; Landgraf et al., 2007; Zeidler et al., 2021). In fecal samples from mice and humans, miRNAs are detectable in large quantities, with HoxP-positive cells being the main source (Liu et al., 2016). Gene regulation mediated through fecal miRNA enables the host to exert control over the gut microbiota (Liu et al., 2016). Several miRNAs (miR-515-5p and miR-1226-5p) are found in bacteria (Escherichia coli and Fusobacterium nucleatum), and they selectively affect bacterial gene transcripts and regulate bacterial growth (Liu et al., 2016). In mice with IEC-specific conditional depletion of miRNAs, there is an imbalance of the gut microbiota with symptoms of colitis, and transplantation of healthy fecal miRNAs restores fecal microbes and improves the course of colitis, indicating a major role of miRNAs for the bidirectional communication between host and microbiota in the gut (Liu et al., 2016).
7 Disturbed gut-brain communication and disease
The composition of the mammalian gut microbiome is critically important for the development of neural circuits that are involved in emotional processing, motor control, learning, and memory. Enteric microbiota can communicate with the host through several mechanisms, affecting epithelial cells, ECCs, and neurons (Carabotti et al., 2015; Cryan et al., 2019) and contribute to shape the intestinal permeability, motility, and mucus production (Yan and Kentner, 2017; Ait-Belgnaoui et al., 2018; Li R. et al., 2022). An altered quantitative and qualitative composition of the gut microbiome can induce the production of metabolites with cytotoxic effects, promote neuroinflammation, and disrupt immune cell function. This results in the inhibition of synaptic transmission and gamma oscillations in the hippocampus, a brain region that plays a crucial role in innate and cognitive behavior (Çalışkan et al., 2022; Connell et al., 2022). Studies in GF mice document the importance of bacterial colonization of the gut after birth for the development of brain functions and expression of miRNAs and messenger RNAs in the hippocampus that cannot be reversed by colonization of gut microbiota in adolescent mice (Chen et al., 2017). In the CNS, neurotransmission is profoundly disturbed in the absence of a normal gut microbiome (Clarke et al., 2013). GF mice exhibit increased exploratory and risk-taking behaviors as well as hyperlocomotion, and these behaviors are determined by early but not late bacterial colonization (Diaz Heijtz et al., 2011; Neufeld et al., 2011). As a consequence, the brain’s chemistry differs from that of “normal” mice with region-specific changes in the expression of 5-HT and brain derived neurotrophic factor (BDNF) (Wrase et al., 2006; Sampson and Mazmanian, 2015; Yano et al., 2015; Bauer et al., 2016; Sharon et al., 2016). Additionally, heightened levels of proteins that regulate the maturation and functionality of neural synapses, such as the synaptic vesicle glycoprotein synaptophysin and postsynaptic density protein 95 (PSD95), are present in the striatum of GF mice which show alterations in spatial working memory and reference memory, indicating impairment of the development of the hippocampus (Diaz Heijtz et al., 2011; Gareau et al., 2011; Glinert et al., 2022). The regional specificity suggests that the pathways underlying described diversities are specifically important for various brain regions, or that the timing of bacterial influences may vary across different areas of the brain (Collins et al., 2012). Microbiota seem to be crucial for the formation of stress response related brain circuits, while emotional and physiological stress affects the gut (Kim and Shin, 2018). Stress causes dysbiosis, which consecutively leads to altered synthesis of biologically active substances, including neurotransmitters (Bassett et al., 2019).
For instance, only 2 h of social separation alters the quantitative and qualitative composition of the gut microbiota in mice and leads to a decline in the Lactobacillus population (Galley et al., 2014). A brief disruption of the intestinal microbiota composition by administration of the antibiotic vancomycin has a significant effect on physiological or behavioral parameters in later life (Cryan and Dinan, 2012). Mechanistically, all mental disorders in Table 4 are associated with a leaky gut, neuroinflammation, and hyper-activated microglial cells, for which gut-residing bacteria and their metabolites are important contributors. Respectively, patients show a shift towards pro-inflammatory colonic microbiota, harboring more Gram-negative bacteria containing lipopolysaccharides (LPS) which can cause inflammatory reactions. It is also known that bacteria with pro-inflammatory properties, such as Alistipes, Eggerthella, and Flavonifractor, are found in greater numbers, whereas the number of bacteria with anti-inflammatory properties, in particular Bifidobacterium spp., Coprococcus, Eucbacterium, Eubacterium rectale, Faecalibacterium, Faecalibacterium prasunitzii, Lactobacillus spp., Prevotella, Roseburia, is decreased compared to healthy people. Various metabolites, mainly SCFAs, as well as bacterial metabolites, including neurotransmitters (acetylcholine, dopamine, norepinephrine, GABA, glutamate, 5-HT), are involved in the pathogenesis (Eicher and Mohajeri, 2022). Increasing evidence suggests that the gut microbiota may contribute to the pathogenesis of Alzheimer’s disease (AD) as a source of amyloid proteins.
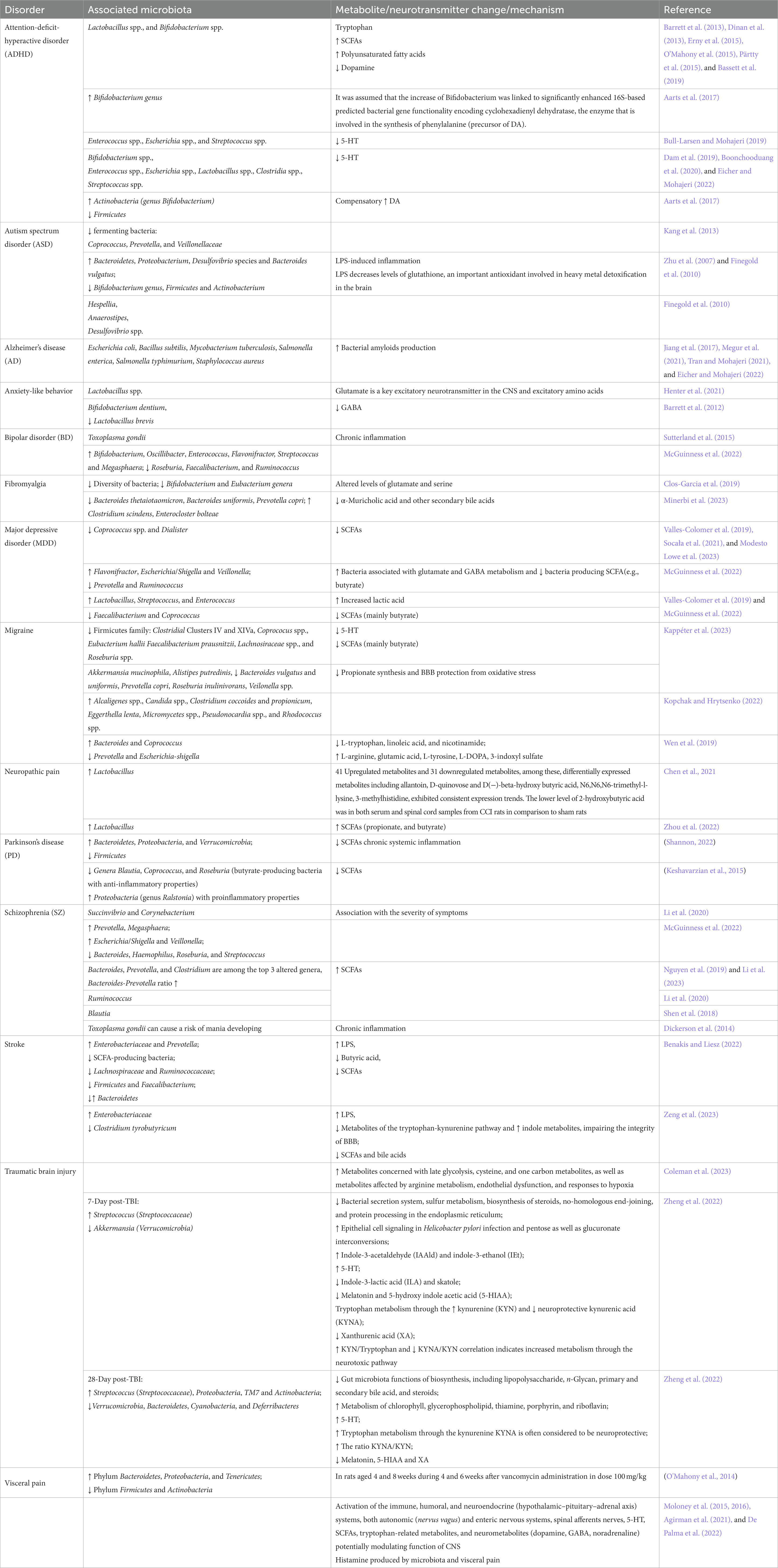
Table 4. Association of mental/neurological disorders with microbiota, metabolites, or neurotransmitter changes.
Despite the growing body of evidence linking dysbiosis with mental disorders such as schizophrenia (SZ), our understanding of the functional consequences of the gut microbiota and their influence on metabolite quantities in the blood and tissue of patients remains limited (Li et al., 2020, 2023). Metabolites such as butyric acid which is detectable in human breath gas are emerging for diagnosing SZ and major depressive disorders (Henning et al., 2023).
7.1 Gut microbiome and depression
Depression is a heterogeneous mood disorder with a complex yet not sufficiently understood neurobiology that has strong links to a dysfunction of the microbiome-gut-brain axis (Gheorghe et al., 2022). Clinical studies have found differences in the composition of the gut microbiota in patients with depression compared to individuals without mental disorders (Valles-Colomer et al., 2019; Socała et al., 2021; Green et al., 2023; Modesto Lowe et al., 2023). Common to all studies is an increase in the number of lactic acid-producing bacteria such as Lactobacillus, Streptococcus, and Enterococcus, and a decrease in the number of bacteria producing SCFAs (mainly butyrate) such as Faecalibacterium and Coprococcus (Table 4) (Valles-Colomer et al., 2019; McGuinness et al., 2022). There is evidence of a correlation between certain gut bacteria and depression symptoms (Simpson et al., 2021; McGuinness et al., 2022). However, their involvement in the pathophysiology of the mental disorder is not well understood (Green et al., 2023). Meta-analyses suggest that probiotics as an adjunctive treatment may reduce depressive symptoms, (Chao et al., 2020; El Dib et al., 2021; Green et al., 2023). However, probiotic preparations to date are limited to a single bacterial strain or, at best, a small number of strains (Green et al., 2023). In contrast, fecal microbiota transplantation (FMT), which encompasses the complete human gut microbiome containing thousands of potentially symbiotic strains (Strandwitz et al., 2019), may be better suitable since it alters the composition of the gut microbiota more effectively (Green et al., 2023).
One of the links in the pathogenesis of major depressive disorder (MDD) in men may be low testosterone levels, which is associated with disturbed gut microbiota, and as a result, with impaired functioning of the gut-brain axis (Dwyer et al., 2020; Gheorghe et al., 2022). Mycobacterium neoaurum produces the enzyme 3-beta-hydroxysteroid dehydrogenase (3β-HSD) and this may represent a new link between gut dysbiosis and depression in particular in men (Gheorghe et al., 2022; Li D. et al., 2022).
Thus, the mechanisms of MDD development involve the interaction of many components of biological origin, including the microbiota and gut-brain axis. Therefore, therapeutic and prophylactic strategies aiming at correcting the intestinal microbiome, such as prebiotics/probiotics, and FMT, are considered promising treatment options for MDD (Donoso et al., 2023).
8 Gut microbiome and pain disorders
Disruption of microbiota colonization caused by antibiotics in early life is not only associated with mental disorders but also visceral hypersensitivity and altered spinal cord signaling in adults (O’Mahony et al., 2014). Temporary changes in the gut microbiome composition during the critical period in newborn rats have long-term effects on nociceptive pathways, and the maturating pain system is influenced by the microbiome (Cryan and Dinan, 2012). Although the mechanisms as well as their up- and down-stream signaling pathways are largely unknown, there is a growing focus on the role of intestinal microbiota and their metabolites in neuropathic pain disorders (Li R. et al., 2022). The microbiota content in the gut is altered in rodent injury models of neuropathic pain (Chen et al., 2021). Specifically, the abundance of Lactobacillus phyla is significantly increased in the gut and accompanied by changes in serum and spinal cord metabolites (Table 4). Recent studies support a link between intestinal microorganisms and neuropathic pain in patients (Guo et al., 2019; Ding et al., 2021; Li R. et al., 2022; Zhang et al., 2023) suggesting, that alterations of specific microbiota strains are causally involved in metabolic disturbances associated with neuropathic pain. Microbiota-derived LPS, SCFAs, peptidoglycans, trimethylamine, or secondary bile acids affect neurons and non-neuronal cells along the pain pathway like immune cells, microglia, or astrocytes, resulting in elevated plasma levels of pro-inflammatory cytokines (IL-1β, IL-6, and TNF-α), chemokines (CCL2 and CXCL1), anti-inflammatory IL-4 as well as neuropeptides and opioids (Cryan et al., 2019; Park and Kim, 2021; Liu et al., 2022). Metabolites or even short RNAs directly act on receptors and ion channels (GABA receptors, TLRs, TRP channels, acid-sensitive ion channels) expressed by nociceptive primary afferents, and induce nociceptor activation and sensitization (Ji et al., 2014, 2016; Lutz et al., 2014; Chow and Gulbransen, 2017; Chiu, 2018). Evoked intestinal permeability increases the levels of pro-inflammatory factors circulating in the blood plasma, and the intensity of pain (Piche et al., 2009; Ernberg et al., 2018). Several of the above-mentioned inflammatory factors penetrate through the BBB and may even act on circuits in the spinal dorsal horn or brain areas processing painful stimuli (Varatharaj and Galea, 2017; Zhu et al., 2018; Çalışkan et al., 2022). As a consequence bacteria can alter emotional, motivational, and cognitive functions giving rise to mental comorbidities of pain such as depression or sleep disturbance (Takanaga et al., 2001; Kawashima et al., 2007; Bravo et al., 2011; Ozogul, 2011; Barrett et al., 2012; Kuley et al., 2012; Holzer and Farzi, 2014; Janik et al., 2016; Kim and Shin, 2018; Li R. et al., 2022). On the other hand, antinociceptive effects of certain microbiota are emerging such as Lactobacillus reuteri targeting the nociceptive transducer ion channel TRPV1, and visceral antinociceptive effects are emerging for probiotic B. infantis 35,624 (Mckernan et al., 2010; Perez-Burgos et al., 2015).
A second pain disorder with strong links to the gut microbiome is migraine, and migraine pathophysiology involves the 5-HT pathway or SCFAs (Arzani et al., 2020; Lanza et al., 2021; Crawford et al., 2022; Kappéter et al., 2023). SCFAs reduce hyperalgesia and decrease the release of TNFα and IL1-β in the gut in a rodent migraine model (Crawford et al., 2022). Therefore, the loss of 5-HT and SCFAs producing bacteria in the gut, such as the Firmicutes family (Faecalibacterium prausnitzii, Coprococus spp., Roseburia spp., Lachnosiraceae spp., Clostridial Clusters IV and XIVa, and Eubacterium hallii) is considered a highly important factor in migraine pathogenesis (Kappéter et al., 2023). Another important link is the intestinal propionate synthesis and BBB protection from oxidative stress due to the decrease of Akkermansia mucinophila, Alistipes putredinis, Bacteroides vulgatus and uniformis, Prevotella copri, Roseburia inulinivorans, and Veilonella spp. (Table 4; Kappéter et al., 2023), with probiotic dietary supplements or FMT effectively decreaseing the frequency and intensity of migraine attacks (Crawford et al., 2022; Kappéter et al., 2023). These discoveries paved the way for the development of personalized migraine therapies based on the microbiome (Crawford et al., 2022).
Microbiota dysbiosis also contributes to the pathogenesis of visceral pain in irritable bowel disease which affects between 5 and 10% of the general population worldwide and involves multiple processes including immune, humoral and neuroendocrine (HPA axis) factors, autonomic (nervus vagus) and enteric nervous systems, spinal afferents nerves, 5-HT, SCFAs, tryptophan-related metabolites, gut hormones, and neurometabolites (dopamine, GABA, noradrenaline) (Moloney et al., 2015, 2016; Agirman et al., 2021). After vancomycin administration Bacteroidetes, Proteobacteria, and Tenericutes increase, and the phylum Firmicutes and Actinobacteria, decreases in animals (Table 4). Temporary changes in the composition of the GI microbiota during a critical developmental period have long-term effects on nociceptive pathways, and the gut microbiome in particular in male newborns but not adult rats (O’Mahony et al., 2014). Histamine is emerging as a relevant mediator of visceral hyperalgesia (De Palma et al., 2022), and multiple studies investigating the highly complex processes are well summarised in several recent review articles (Alizadeh et al., 2022; Ustianowska et al., 2022; Mayer et al., 2023; Pujo et al., 2023; Sarnoff et al., 2023; Shaikh et al., 2023; Shin and Kashyap, 2023).
9 Sex-specific differences
In general, men are more prone than women to develop brain and nervous system disorders with impaired synthesis of neuroactive substances, and the male gender is a significant risk factor for delirium following surgery (Wang H. et al., 2021; Wittmann et al., 2022; Van Pee et al., 2023). This may be related to disturbed gut microbiota potentially due to unhealthy lifestyles which are more common in the male gender (Rincel et al., 2019; Wang H. et al., 2021; Gamage et al., 2023). Increasing evidence points to differences in the gut microbiota composition, neuronal processing in the CNS, and the HPA axis between men and women that may be related to respective differences in cognitive strategies and brain function in health and disease (Sisk-Hackworth et al., 2023). Male GF mice, in comparison to conventionally colonized control animals, exhibit a significantly higher hippocampal concentration of 5-HT and its main metabolite 5-hydroxyindoleacetic acid, in contrast to immunological and neuroendocrine manifestations that occur in both genders (Clarke et al., 2013; Rieder et al., 2017). The amount of tryptophan, a precursor of 5-HT, is increased in the blood of male GF rodents, indicating a humoral pathway through which microbiota can affect serotonergic neurotransmission in the CNS (Clarke et al., 2013). Interestingly, the post-weaning colonization of GF mice with gut microbiota could not rescue the neurochemical alterations caused by the absence of microorganisms in early life, although peripheral tryptophan availability was restored and changes in anxiety-like behavior were normalized. These findings indicate, that neurotransmission in the brain can be significantly impaired by the absence of normal gut microbiota and that this altered neurochemical profile persists despite the restoration of a normal microbiome later in life (Clarke et al., 2013).
10 Conclusion and future perspectives
Over the past decade, scientists and clinicians have been actively searching for basic mechanistic insight into the pathogenesis of highly prevalent mental disorders, including schizophrenia, depression, migraine, and neuropathic pain. Qualitative and quantitative changes in the gut microbiota and changes in the amount of neurotransmitters and metabolites of microbial origin have been identified, and support a relevant role of the gut-brain axis in the development of these conditions. Although the beneficial effects of probiotics, certain metabolites, and FMT on migraine and other neurological disorders favor this concept, there are major gaps in understanding their etiology and pathogenesis, and it is not yet clear which of the microbiome related neurotransmitters, metabolites, and pathways are causally involved. Nonetheless, the gut microbiome has an important influence on brain functions and mental health including pain disorders, and there is increasing interest in microbiota with probiotic properties, as novel and safe treatment options.
New technologies such as the use of reporter mouse lines and optogenetic tools have become available recently to specifically dissect the precise communication routes between the gut and the brain including the roles of particular cell types and neuron populations in the gut and the brain (Crock et al., 2012; Makadia et al., 2018; Spencer et al., 2018). Based on the emerging importance of the gut microbiome for the function of the entire organism and the bidirectional communications paths between the gut microbiome and the nervous system affecting mental health, novel opportunities for clinical applications of gut microbiome related therapies are expected to emerge for highly prevalent medical conditions including irritable bowel disease, migraine, and mental disorders.
Author contributions
LR: Writing – review & editing. JF: Writing – review & editing. MK: Writing – review & editing. NR: Writing – review & editing.
Funding
The author(s) declare financial support was received for the research, authorship, and/or publication of this article. This work was funded by the Austrian Science Fund - FWF - Project number No. P34403 to MK.
Conflict of interest
The authors declare that the research was conducted in the absence of any commercial or financial relationships that could be construed as a potential conflict of interest.
The author(s) declared that they were an editorial board member of Frontiers, at the time of submission. This had no impact on the peer review process and the final decision.
Publisher’s note
All claims expressed in this article are solely those of the authors and do not necessarily represent those of their affiliated organizations, or those of the publisher, the editors and the reviewers. Any product that may be evaluated in this article, or claim that may be made by its manufacturer, is not guaranteed or endorsed by the publisher.
References
Aarts, E., Ederveen, T. H. A., Naaijen, J., Zwiers, M. P., Boekhorst, J., Timmerman, H. M., et al. (2017). Gut microbiome in ADHD and its relation to neural reward anticipation. PLoS One 12:e0183509. doi: 10.1371/journal.pone.0183509
Abdullah, N., Defaye, M., and Altier, C. (2020). Neural control of gut homeostasis. Am. J. Physiol. Gastrointest. Liver Physiol. 319, G718–G732. doi: 10.1152/ajpgi.00293.2020
Adolph, T. E., Grander, C., Moschen, A. R., and Tilg, H. (2018). Liver-microbiome Axis in health and disease. Trends Immunol. 39, 712–723. doi: 10.1016/j.it.2018.05.002
Afzal, M., Mazhar, S. F., Sana, S., Naeem, M., Rasool, M. H., Saqalein, M., et al. (2020). Neurological and cognitive significance of probiotics: a holy grail deciding individual personality. Future Microbiol. 15, 1059–1074. doi: 10.2217/fmb-2019-0143
Agirman, G., Yu, K. B., and Hsiao, E. Y. (2021). Signaling inflammation across the gut-brain axis. Science 374, 1087–1092. doi: 10.1126/science.abi6087
Agostoni, E., Chinnock, J. E., De Daly, M. B., and Murray, J. G. (1957). Functional and histological studies of the vagus nerve and its branches to the heart, lungs and abdominal viscera in the cat. J. Physiol. 135, 182–205. doi: 10.1113/jphysiol.1957.sp005703
Ahmed, H., Leyrolle, Q., Koistinen, V., Karkkainen, O., Laye, S., Delzenne, N., et al. (2022). Microbiota-derived metabolites as drivers of gut-brain communication. Gut Microbes 14:2102878. doi: 10.1080/19490976.2022.2102878
Ait-Belgnaoui, A., Payard, I., Rolland, C., Harkat, C., Braniste, V., Theodorou, V., et al. (2018). Bifidobacterium longum and Lactobacillus helveticus synergistically suppress stress-related visceral hypersensitivity through hypothalamic-pituitary-adrenal Axis modulation. J Neurogastroenterol Motil 24, 138–146. doi: 10.5056/jnm16167
Alizadeh, N., Naderi, G., Kahrizi, M. S., Haghgouei, T., Mobed, A., and Shah-Abadi, M. E. (2022). Microbiota-pain association; recent discoveries and research Progress. Curr. Microbiol. 80:29. doi: 10.1007/s00284-022-03124-9
Alkadhi, S., Kunde, D., Cheluvappa, R., Randall-Demllo, S., and Eri, R. (2014). The murine appendiceal microbiome is altered in spontaneous colitis and its pathological progression. Gut Pathog 6:25. doi: 10.1186/1757-4749-6-25
Allaire, J. M., Crowley, S. M., Law, H. T., Chang, S. Y., Ko, H. J., and Vallance, B. A. (2018a). The intestinal epithelium: central coordinator of mucosal immunity. Trends Immunol. 39, 677–696. doi: 10.1016/j.it.2018.04.002
Allaire, J. M., Morampudi, V., Crowley, S. M., Stahl, M., Yu, H., Bhullar, K., et al. (2018b). Frontline defenders: goblet cell mediators dictate host-microbe interactions in the intestinal tract during health and disease. Am. J. Physiol. Gastrointest. Liver Physiol. 314, G360–G377. doi: 10.1152/ajpgi.00181.2017
Altschuler, S. M., Escardo, J., Lynn, R. B., and Miselis, R. R. (1993). The central organization of the vagus nerve innervating the colon of the rat. Gastroenterology 104, 502–509. doi: 10.1016/0016-5085(93)90419-D
Álvarez-Herms, J., González, A., Corbi, F., Odriozola, I., and Odriozola, A. (2023). Possible relationship between the gut leaky syndrome and musculoskeletal injuries: the important role of gut microbiota as indirect modulator. AIMS Public Health 10, 710–738. doi: 10.3934/publichealth.2023049
Andrews, J. M., and Ashley Blackshaw, L. (2010). “Chapter 97 - small intestinal motor and sensory function and dysfunction” in Sleisenger and Fordtran’s Gastrointestinal and Liver Disease. eds. M. Feldman, L. S. Friedman, and L. J. Brandt. 9th ed (Philadelphia: W.B. Saunders), 1643–1658.e2.
Appleton, J. (2018). The gut-brain Axis: influence of microbiota on mood and mental health. Integr Med (Encinitas) 17, 28–32.
Arora, T., Vanslette, A. M., Hjorth, S. A., and Backhed, F. (2021). Microbial regulation of enteroendocrine cells. Med 2, 553–570. doi: 10.1016/j.medj.2021.03.018
Arzani, M., Jahromi, S. R., Ghorbani, Z., Vahabizad, F., Martelletti, P., Ghaemi, A., et al. (2020). Gut-brain axis and migraine headache: a comprehensive review. J. Headache Pain 21:15. doi: 10.1186/s10194-020-1078-9
Banfi, D., Moro, E., Bosi, A., Bistoletti, M., Cerantola, S., Crema, F., et al. (2021). Impact of microbial metabolites on microbiota-gut-brain Axis in inflammatory bowel disease. Int. J. Mol. Sci. 22:1623. doi: 10.3390/ijms22041623
Barreto, E. B. L., Rattes, I. C., da Costa, A. V., and Gama, P. (2022). Paneth cells and their multiple functions. Cell Biol. Int. 46, 701–710. doi: 10.1002/cbin.11764
Barrett, E., Kerr, C., Murphy, K., O’Sullivan, O., Ryan, C. A., Dempsey, E. M., et al. (2013). The individual-specific and diverse nature of the preterm infant microbiota. Arch Dis Child Fetal Neonatal Ed 98, F334–F340. doi: 10.1136/archdischild-2012-303035
Barrett, E., Ross, R. P., O’Toole, P. W., Fitzgerald, G. F., and Stanton, C. (2012). Gamma-aminobutyric acid production by culturable bacteria from the human intestine. J. Appl. Microbiol. 113, 411–417. doi: 10.1111/j.1365-2672.2012.05344.x
Bas, J., Jay, P., and Gerbe, F. (2023). Intestinal tuft cells: sentinels, what else? Semin. Cell Dev. Biol. 150-151, 35–42. doi: 10.1016/j.semcdb.2023.02.012
Bassett, S. A., Young, W., Fraser, K., Dalziel, J. E., Webster, J., Ryan, L., et al. (2019). Metabolome and microbiome profiling of a stress-sensitive rat model of gut-brain axis dysfunction. Sci. Rep. 9:14026. doi: 10.1038/s41598-019-50593-3
Bauer, K. C., Huus, K. E., and Finlay, B. B. (2016). Microbes and the mind: emerging hallmarks of the gut microbiota–brain axis. Cell. Microbiol. 18, 632–644. doi: 10.1111/cmi.12585
Bayrer, J. R., Castro, J., Venkataraman, A., Touhara, K. K., Rossen, N. D., Morrie, R. D., et al. (2023). Gut enterochromaffin cells drive visceral pain and anxiety. Nature 616, 137–142. doi: 10.1038/s41586-023-05829-8
Bellono, N. W., Bayrer, J. R., Leitch, D. B., Castro, J., Zhang, C., O’Donnell, T. A., et al. (2017). Enterochromaffin cells are gut Chemosensors that couple to sensory neural pathways. Cells 170, 185–198.e16. doi: 10.1016/j.cell.2017.05.034
Benakis, C., and Liesz, A. (2022). The gut-brain axis in ischemic stroke: its relevance in pathology and as a therapeutic target. Neurol. Res. Pract. 4:57. doi: 10.1186/s42466-022-00222-8
Berthoud, H. R., Blackshaw, L. A., Brookes, S. J., and Grundy, D. (2004). Neuroanatomy of extrinsic afferents supplying the gastrointestinal tract. Neurogastroenterol. Motil. 16, 28–33. doi: 10.1111/j.1743-3150.2004.00471.x
Berthoud, H. R., Kressel, M., Raybould, H. E., and Neuhuber, W. L. (1995). Vagal sensors in the rat duodenal mucosa: distribution and structure as revealed by in vivo DiI-tracing. Anat Embryol (Berl) 191, 203–212. doi: 10.1007/BF00187819
Berthoud, H.-R., and Neuhuber, W. L. (2000). Functional and chemical anatomy of the afferent vagal system. Auton. Neurosci. 85, 1–17. doi: 10.1016/S1566-0702(00)00215-0
Beyak, M. J., Bulmer, D. C. E., Jiang, W., Keating, C., Rong, W., and Grundy, D. (2006). “Chapter 25 - extrinsic sensory afferent nerves innervating the gastrointestinal tract” in Physiology of the Gastrointestinal Tract. ed. L. R. Johnson. 4th ed (Burlington: Academic Press), 685–725.
Bishu, S. (2016). Sensing of nutrients and microbes in the gut. Curr. Opin. Gastroenterol. 32, 86–95. doi: 10.1097/MOG.0000000000000246
Bonaz, B., Sinniger, V., and Pellissier, S. (2021). Therapeutic potential of Vagus nerve stimulation for inflammatory bowel diseases. Front. Neurosci. 15:650971. doi: 10.3389/fnins.2021.650971
Boonchooduang, N., Louthrenoo, O., Chattipakorn, N., and Chattipakorn, S. C. (2020). Possible links between gut–microbiota and attention-deficit/hyperactivity disorders in children and adolescents. Eur. J. Nutr. 59, 3391–3403. doi: 10.1007/s00394-020-02383-1
Bravo, J. A., Forsythe, P., Chew, M. V., Escaravage, E., Savignac, H. M., Dinan, T. G., et al. (2011). Ingestion of Lactobacillus strain regulates emotional behavior and central GABA receptor expression in a mouse via the vagus nerve. Proc. Natl. Acad. Sci. U. S. A. 108, 16050–16055. doi: 10.1073/pnas.1102999108
Breit, S., Kupferberg, A., Rogler, G., and Hasler, G. (2018). Vagus nerve as modulator of the brain–gut Axis in psychiatric and inflammatory disorders. Front. Psych. 9:44. doi: 10.3389/fpsyt.2018.00044
Brierley, S. M., Hibberd, T. J., and Spencer, N. J. (2018). Spinal afferent innervation of the Colon and Rectum. Front. Cell. Neurosci. 12:467. doi: 10.3389/fncel.2018.00467
Brierley, S. M., Jones, R. C. 3rd, Gebhart, G. F., and Blackshaw, L. A. (2004). Splanchnic and pelvic mechanosensory afferents signal different qualities of colonic stimuli in mice. Gastroenterology 127, 166–178. doi: 10.1053/j.gastro.2004.04.008
Brookes, S. J., Spencer, N. J., Costa, M., and Zagorodnyuk, V. P. (2013). Extrinsic primary afferent signalling in the gut. Nat. Rev. Gastroenterol. Hepatol. 10, 286–296. doi: 10.1038/nrgastro.2013.29
Bull-Larsen, S., and Mohajeri, M. H. (2019). The potential influence of the bacterial microbiome on the development and progression of ADHD. Nutrients 11:2805. doi: 10.3390/nu11112805
Çalışkan, G., French, T., Enrile Lacalle, S., del Angel, M., Steffen, J., Heimesaat, M. M., et al. (2022). Antibiotic-induced gut dysbiosis leads to activation of microglia and impairment of cholinergic gamma oscillations in the hippocampus. Brain Behav. Immun. 99, 203–217. doi: 10.1016/j.bbi.2021.10.007
Camilleri, M. (2019). Leaky gut: mechanisms, measurement and clinical implications in humans. Gut 68, 1516–1526. doi: 10.1136/gutjnl-2019-318427
Cani, P. D., and Knauf, C. (2016). How gut microbes talk to organs: the role of endocrine and nervous routes. Mol Metab 5, 743–752. doi: 10.1016/j.molmet.2016.05.011
Carabotti, M., Scirocco, A., Maselli, M. A., and Severi, C. (2015). The gut-brain axis: interactions between enteric microbiota, central and enteric nervous systems. Ann. Gastroenterol. 28, 203–209.
Chalazonitis, A., and Rao, M. (2018). Enteric nervous system manifestations of neurodegenerative disease. Brain Res. 1693, 207–213. doi: 10.1016/j.brainres.2018.01.011
Chao, L., Liu, C., Sutthawongwadee, S., Li, Y., Lv, W., Chen, W., et al. (2020). Effects of probiotics on depressive or anxiety variables in healthy participants under stress conditions or with a depressive or anxiety diagnosis: a meta-analysis of randomized controlled trials. Front. Neurol. 11:421. doi: 10.3389/fneur.2020.00421
Chen, P., Wang, C., Ren, Y.-N., Ye, Z.-J., Jiang, C., and Wu, Z.-B. (2021). Alterations in the gut microbiota and metabolite profiles in the context of neuropathic pain. Mol. Brain 14:50. doi: 10.1186/s13041-021-00765-y
Chen, J. J., Zeng, B. H., Li, W. W., Zhou, C. J., Fan, S. H., Cheng, K., et al. (2017). Effects of gut microbiota on the microRNA and mRNA expression in the hippocampus of mice. Behav. Brain Res. 322, 34–41. doi: 10.1016/j.bbr.2017.01.021
Chiu, I. M. (2018). Infection, pain, and itch. Neurosci. Bull. 34, 109–119. doi: 10.1007/s12264-017-0098-1
Chow, A. K., and Gulbransen, B. D. (2017). Potential roles of enteric glia in bridging neuroimmune communication in the gut. Am. J. Physiol. Gastrointest. Liver Physiol. 312, G145–G152. doi: 10.1152/ajpgi.00384.2016
Clarke, G., Grenham, S., Scully, P., Fitzgerald, P., Moloney, R. D., Shanahan, F., et al. (2013). The microbiome-gut-brain axis during early life regulates the hippocampal serotonergic system in a sex-dependent manner. Mol. Psychiatry 18, 666–673. doi: 10.1038/mp.2012.77
Clos-Garcia, M., Andrés-Marin, N., Fernández-Eulate, G., Abecia, L., Lavín, J. L., van Liempd, S., et al. (2019). Gut microbiome and serum metabolome analyses identify molecular biomarkers and altered glutamate metabolism in fibromyalgia. EBioMedicine 46, 499–511. doi: 10.1016/j.ebiom.2019.07.031
Coleman, J. R., D’Alessandro, A., LaCroix, I., Dzieciatkowska, M., Lutz, P., Mitra, S., et al. (2023). A metabolomic and proteomic analysis of pathologic hypercoagulability in traumatic brain injury patients after dura violation. J Trauma Acute Care Surg 95, 925–934. doi: 10.1097/TA.0000000000004019
Coleman, O. I., and Haller, D. (2017). Bacterial signaling at the intestinal epithelial interface in inflammation and cancer. Front. Immunol. 8:1927. doi: 10.3389/fimmu.2017.01927
Collins, J., Auchtung, J. M., Schaefer, L., Eaton, K. A., and Britton, R. A. (2015). Humanized microbiota mice as a model of recurrent Clostridium difficile disease. Microbiome 3:35. doi: 10.1186/s40168-015-0097-2
Collins, S. M., Surette, M., and Bercik, P. (2012). The interplay between the intestinal microbiota and the brain. Nat. Rev. Microbiol. 10, 735–742. doi: 10.1038/nrmicro2876
Connell, E., Le Gall, G., Pontifex, M. G., Sami, S., Cryan, J. F., Clarke, G., et al. (2022). Microbial-derived metabolites as a risk factor of age-related cognitive decline and dementia. Mol. Neurodegener. 17:43. doi: 10.1186/s13024-022-00548-6
Coquant, G., Aguanno, D., Pham, S., Grellier, N., Thenet, S., Carriere, V., et al. (2021). Gossip in the gut: quorum sensing, a new player in the host-microbiota interactions. World J. Gastroenterol. 27, 7247–7270. doi: 10.3748/wjg.v27.i42.7247
Crawford, J., Liu, S., and Tao, F. (2022). Gut microbiota and migraine. Neurobiol Pain 11:100090. doi: 10.1016/j.ynpai.2022.100090
Crock, L. W., Kolber, B. J., Morgan, C. D., Sadler, K. E., Vogt, S. K., Bruchas, M. R., et al. (2012). Central amygdala metabotropic glutamate receptor 5 in the modulation of visceral pain. J. Neurosci. 32, 14217–14226. doi: 10.1523/JNEUROSCI.1473-12.2012
Cryan, J. F., and Dinan, T. G. (2012). Mind-altering microorganisms: the impact of the gut microbiota on brain and behaviour. Nat. Rev. Neurosci. 13, 701–712. doi: 10.1038/nrn3346
Cryan, J. F., and O’Mahony, S. M. (2011). The microbiome-gut-brain axis: from bowel to behavior. Neurogastroenterol. Motil. 23, 187–192. doi: 10.1111/j.1365-2982.2010.01664.x
Cryan, J. F., O’Riordan, K. J., Cowan, C. S. M., Sandhu, K. V., Bastiaanssen, T. F. S., Boehme, M., et al. (2019). The microbiota-gut-brain Axis. Physiol. Rev. 99, 1877–2013. doi: 10.1152/physrev.00018.2018
Cui, C., Wang, F., Zheng, Y., Wei, H., and Peng, J. (2023). From birth to death: the hardworking life of Paneth cell in the small intestine. Front. Immunol. 14:1122258. doi: 10.3389/fimmu.2023.1122258
Dam, S. A., Mostert, J. C., Szopinska-Tokov, J. W., Bloemendaal, M., Amato, M., and Arias-Vasquez, A. (2019). The role of the gut-brain axis in attention-deficit/hyperactivity disorder. Gastroenterol. Clin. 48, 407–431. doi: 10.1016/j.gtc.2019.05.001
De Palma, G., Shimbori, C., Reed, D. E., Yu, Y., Rabbia, V., Lu, J., et al. (2022). Histamine production by the gut microbiota induces visceral hyperalgesia through histamine 4 receptor signaling in mice. Sci. Transl. Med. 14:eabj1895. doi: 10.1126/scitranslmed.abj1895
Desbonnet, L., Clarke, G., Traplin, A., O’Sullivan, O., Crispie, F., Moloney, R. D., et al. (2015). Gut microbiota depletion from early adolescence in mice: implications for brain and behaviour. Brain Behav. Immun. 48, 165–173. doi: 10.1016/j.bbi.2015.04.004
Diaz Heijtz, R., Wang, S., Anuar, F., Qian, Y., Bjorkholm, B., Samuelsson, A., et al. (2011). Normal gut microbiota modulates brain development and behavior. Proc. Natl. Acad. Sci. U. S. A. 108, 3047–3052. doi: 10.1073/pnas.1010529108
Dickerson, F., Stallings, C., Origoni, A., Vaughan, C., Katsafanas, E., Khushalani, S., et al. (2014). Antibodies to toxoplasma gondii in individuals with mania. Bipolar Disord. 16, 129–136. doi: 10.1111/bdi.12123
Dinan, T. G., Stanton, C., and Cryan, J. F. (2013). Psychobiotics: a novel class of psychotropic. Biol. Psychiatry 74, 720–726. doi: 10.1016/j.biopsych.2013.05.001
Ding, W., You, Z., Chen, Q., Yang, L., Doheny, J., Zhou, X., et al. (2021). Gut Microbiota Influences Neuropathic Pain Through Modulating Proinflammatory and Anti-inflammatory T Cells. Anesth. Analg. 132, 1146–1155. doi: 10.1213/ANE.0000000000005155
Dodds, K. N., Travis, L., Kyloh, M. A., Jones, L. A., Keating, D. J., and Spencer, N. J. (2022). The gut-brain axis: spatial relationship between spinal afferent nerves and 5-HT-containing enterochromaffin cells in mucosa of mouse colon. Am. J. Physiol. Gastrointest. Liver Physiol. 322, G523–g533. doi: 10.1152/ajpgi.00019.2022
Donaldson, G. P., Lee, S. M., and Mazmanian, S. K. (2016). Gut biogeography of the bacterial microbiota. Nat. Rev. Microbiol. 14, 20–32. doi: 10.1038/nrmicro3552
Donoso, F., Cryan, J. F., Olavarría-Ramírez, L., Nolan, Y. M., and Clarke, G. (2023). Inflammation, lifestyle factors, and the microbiome-gut-brain Axis: relevance to depression and antidepressant action. Clin. Pharmacol. Ther. 113, 246–259. doi: 10.1002/cpt.2581
Dwyer, J. B., Aftab, A., Radhakrishnan, R., Widge, A., Rodriguez, C. I., Carpenter, L. L., et al. (2020). Hormonal treatments for major depressive disorder: state of the art. Am. J. Psychiatr. 177, 686–705. doi: 10.1176/appi.ajp.2020.19080848
Eckmann, L. (2004). Innate immunity and mucosal bacterial interactions in the intestine. Curr. Opin. Gastroenterol. 20, 82–88. doi: 10.1097/00001574-200403000-00006
Eicher, T. P., and Mohajeri, M. H. (2022). Overlapping mechanisms of action of brain-active Bacteria and bacterial metabolites in the pathogenesis of common brain diseases. Nutrients 14:2661. doi: 10.3390/nu14132661
El Dib, R., Periyasamy, A. G., de Barros, J. L., França, C. G., Senefonte, F. L., Vesentini, G., et al. (2021). Probiotics for the treatment of depression and anxiety: A systematic review and meta-analysis of randomized controlled trials. Clinical Nutrition ESPEN 45, 75–90. doi: 10.1016/j.clnesp.2021.07.027
Ernberg, M., Christidis, N., Ghafouri, B., Bileviciute-Ljungar, I., Lofgren, M., Bjersing, J., et al. (2018). Plasma cytokine levels in fibromyalgia and their response to 15 weeks of progressive resistance exercise or relaxation therapy. Mediat. Inflamm. 2018:3985154. doi: 10.1155/2018/3985154
Erny, D., Hrabě de Angelis, A. L., Jaitin, D., Wieghofer, P., Staszewski, O., David, E., et al. (2015). Host microbiota constantly control maturation and function of microglia in the CNS. Nat. Neurosci. 18, 965–977. doi: 10.1038/nn.4030
Fekete, E., and Buret, A. G. (2023). The role of mucin O-glycans in microbiota dysbiosis, intestinal homeostasis, and host-pathogen interactions. Am. J. Physiol. Gastrointest. Liver Physiol. 324, G452–G465. doi: 10.1152/ajpgi.00261.2022
Finegold, S. M., Dowd, S. E., Gontcharova, V., Liu, C., Henley, K. E., Wolcott, R. D., et al. (2010). Pyrosequencing study of fecal microflora of autistic and control children. Anaerobe 16, 444–453. doi: 10.1016/j.anaerobe.2010.06.008
Funabashi, M., Grove, T. L., Wang, M., Varma, Y., McFadden, M. E., Brown, L. C., et al. (2020). A metabolic pathway for bile acid dehydroxylation by the gut microbiome. Nature 582, 566–570. doi: 10.1038/s41586-020-2396-4
Furness, J. B., Rivera, L. R., Cho, H. J., Bravo, D. M., and Callaghan, B. (2013). The gut as a sensory organ. Nat. Rev. Gastroenterol. Hepatol. 10, 729–740. doi: 10.1038/nrgastro.2013.180
Galley, J. D., Nelson, M. C., Yu, Z., Dowd, S. E., Walter, J., Kumar, P. S., et al. (2014). Exposure to a social stressor disrupts the community structure of the colonic mucosa-associated microbiota. BMC Microbiol. 14:189. doi: 10.1186/1471-2180-14-189
Gamage, H., Robinson, K. J., Luu, L., Paulsen, I. T., and Laird, A. S. (2023). Machado Joseph disease severity is linked with gut microbiota alterations in transgenic mice. Neurobiol. Dis. 179:106051. doi: 10.1016/j.nbd.2023.106051
Gaman, A., and Kuo, B. (2008). Neuromodulatory processes of the brain–gut Axis. Neuromodulation 11, 249–259. doi: 10.1111/j.1525-1403.2008.00172.x
Gareau, M. G., Wine, E., Rodrigues, D. M., Cho, J. H., Whary, M. T., Philpott, D. J., et al. (2011). Bacterial infection causes stress-induced memory dysfunction in mice. Gut 60, 307–317. doi: 10.1136/gut.2009.202515
Geng, S., Yang, L., Cheng, F., Zhang, Z., Li, J., Liu, W., et al. (2020). Gut microbiota are associated with psychological stress-induced defections in intestinal and blood–brain barriers. Front. Microbiol. 10:3067. doi: 10.3389/fmicb.2019.03067
Gershon, M. D., and Margolis, K. G. (2021). The gut, its microbiome, and the brain: connections and communications. J. Clin. Invest. 131:e143768. doi: 10.1172/JCI143768
Gheorghe, C. E., Cryan, J. F., and Clarke, G. (2022). Debugging the gut-brain axis in depression. Cell Host Microbe 30, 281–283. doi: 10.1016/j.chom.2022.02.007
Glinert, A., Turjeman, S., Elliott, E., and Koren, O. (2022). Microbes, metabolites and (synaptic) malleability, oh my! The effect of the microbiome on synaptic plasticity. Biol. Rev. Camb. Philos. Soc. 97, 582–599. doi: 10.1111/brv.12812
Glorieux, G., Nigam, S. K., Vanholder, R., and Verbeke, F. (2023). Role of the microbiome in gut-heart-kidney Cross talk. Circ. Res. 132, 1064–1083. doi: 10.1161/CIRCRESAHA.123.321763
Green, J. E., McGuinness, A. J., Berk, M., Castle, D., Athan, E., Hair, C., et al. (2023). Safety and feasibility of faecal microbiota transplant for major depressive disorder: study protocol for a pilot randomised controlled trial. Pilot Feasibility Stud. 9:5. doi: 10.1186/s40814-023-01235-z
Guinane, C. M., Tadrous, A., Fouhy, F., Ryan, C. A., Dempsey, E. M., Murphy, B., et al. (2013). Microbial composition of human appendices from patients following appendectomy. mBio 4:e00366-12. doi: 10.1128/mBio.00366-12
Guo, R., Chen, L. H., Xing, C., and Liu, T. (2019). Pain regulation by gut microbiota: molecular mechanisms and therapeutic potential. Br. J. Anaesth. 123, 637–654. doi: 10.1016/j.bja.2019.07.026
Hansson, G. C. (2020). Mucins and the microbiome. Annu. Rev. Biochem. 89, 769–793. doi: 10.1146/annurev-biochem-011520-105053
Harrington, A. M., Castro, J., Erickson, A., Grundy, L., and Brierley, S. M. (2018). “Chapter 17 - extrinsic sensory afferent nerves innervating the gastrointestinal tract in health and disease” in Physiology of the Gastrointestinal Tract. ed. H. M. Said. 6th ed (London, United Kingdom: Academic Press), 387–418.
Heintz-Buschart, A., and Wilmes, P. (2018). Human gut microbiome: function matters. Trends Microbiol. 26, 563–574. doi: 10.1016/j.tim.2017.11.002
Hemarajata, P., Gao, C., Pflughoeft, K. J., Thomas, C. M., Saulnier, D. M., Spinler, J. K., et al. (2013). Lactobacillus reuteri-specific immunoregulatory gene rsiR modulates histamine production and immunomodulation by Lactobacillus reuteri. J. Bacteriol. 195, 5567–5576. doi: 10.1128/JB.00261-13
Henning, D., Lüno, M., Jiang, C., Meyer-Lotz, G., Hoeschen, C., and Frodl, T. (2023). Gut–brain axis volatile organic compounds derived from breath distinguish between schizophrenia and major depressive disorder. J. Psychiatry Neurosci. 48, E117–E125. doi: 10.1503/jpn.220139
Henter, I. D., Park, L. T., and Zarate, C. A. (2021). Novel glutamatergic modulators for the treatment of mood disorders: current status. CNS Drugs 35, 527–543. doi: 10.1007/s40263-021-00816-x
Hibberd, T. J., Feng, J., Luo, J., Yang, P., Samineni, V. K., Gereau, R. W., et al. (2018). Optogenetic induction of colonic motility in mice. Gastroenterology 155, 514–528.e6. doi: 10.1053/j.gastro.2018.05.029
Hill, L., Popov, J., Figueiredo, M., Caputi, V., Hartung, E., Moshkovich, M., et al. (2021). Protocol for a systematic review on the role of the gut microbiome in paediatric neurological disorders. Acta Neuropsychiatr 33, 211–216. doi: 10.1017/neu.2021.8
Hockley, J. R. F., Taylor, T. S., Callejo, G., Wilbrey, A. L., Gutteridge, A., Bach, K., et al. (2019). Single-cell RNAseq reveals seven classes of colonic sensory neuron. Gut 68, 633–644. doi: 10.1136/gutjnl-2017-315631
Holzer, P. (2006). Efferent-like roles of afferent neurons in the gut: blood flow regulation and tissue protection. Auton. Neurosci. 125, 70–75. doi: 10.1016/j.autneu.2006.01.004
Holzer, P., and Farzi, A. (2014). Neuropeptides and the microbiota-gut-brain axis. Adv. Exp. Med. Biol. 817, 195–219. doi: 10.1007/978-1-4939-0897-4_9
Homann, M., Sansjofre, P., Van Zuilen, M., Heubeck, C., Gong, J., Killingsworth, B., et al. (2018). Microbial life and biogeochemical cycling on land 3,220 million years ago. Nat. Geosci. 11, 665–671. doi: 10.1038/s41561-018-0190-9
Hosseinkhani, F., Heinken, A., Thiele, I., Lindenburg, P. W., Harms, A. C., and Hankemeier, T. (2021). The contribution of gut bacterial metabolites in the human immune signaling pathway of non-communicable diseases. Gut Microbes 13, 1–22. doi: 10.1080/19490976.2021.1882927
Hsu, C. L., and Schnabl, B. (2023). The gut-liver axis and gut microbiota in health and liver disease. Nat. Rev. Microbiol. 21, 719–733. doi: 10.1038/s41579-023-00904-3
Hughes, P. A., Moretta, M., Lim, A., Grasby, D. J., Bird, D., Brierley, S. M., et al. (2014). Immune derived opioidergic inhibition of viscerosensory afferents is decreased in irritable bowel syndrome patients. Brain Behav. Immun. 42, 191–203. doi: 10.1016/j.bbi.2014.07.001
Iftekhar, A., and Sigal, M. (2021). Defence and adaptation mechanisms of the intestinal epithelium upon infection. Int. J. Med. Microbiol. 311:151486. doi: 10.1016/j.ijmm.2021.151486
Jain, A., Gyori, B. M., Hakim, S., Bunga, S., Taub, D. G., Ruiz-Cantero, M. C., et al. (2023). Nociceptor neuroimmune interactomes reveal cell type- and injury-specific inflammatory pain pathways. bioRxiv. doi: 10.1101/2023.02.01.526526
Jameson, K. G., and Hsiao, E. Y. (2018). Linking the gut microbiota to a brain neurotransmitter. Trends Neurosci. 41, 413–414. doi: 10.1016/j.tins.2018.04.001
Janik, R., Thomason, L. A. M., Stanisz, A. M., Forsythe, P., Bienenstock, J., and Stanisz, G. J. (2016). Magnetic resonance spectroscopy reveals oral Lactobacillus promotion of increases in brain GABA, N-acetyl aspartate and glutamate. Neuroimage 125, 988–995. doi: 10.1016/j.neuroimage.2015.11.018
Ji, R. R., Chamessian, A., and Zhang, Y. Q. (2016). Pain regulation by non-neuronal cells and inflammation. Science 354, 572–577. doi: 10.1126/science.aaf8924
Ji, R. R., Xu, Z. Z., and Gao, Y. J. (2014). Emerging targets in neuroinflammation-driven chronic pain. Nat. Rev. Drug Discov. 13, 533–548. doi: 10.1038/nrd4334
Jiang, C., Li, G., Huang, P., Liu, Z., and Zhao, B. (2017). The gut microbiota and Alzheimer’s disease. J. Alzheimers Dis. 58, 1–15. doi: 10.3233/JAD-161141
Jiao, N., Baker, S. S., Chapa-Rodriguez, A., Liu, W., Nugent, C. A., Tsompana, M., et al. (2018). Suppressed hepatic bile acid signalling despite elevated production of primary and secondary bile acids in NAFLD. Gut 67, 1881–1891. doi: 10.1136/gutjnl-2017-314307
Johansson, M. E., Phillipson, M., Petersson, J., Velcich, A., Holm, L., and Hansson, G. C. (2008). The inner of the two Muc2 mucin-dependent mucus layers in colon is devoid of bacteria. Proc. Natl. Acad. Sci. U. S. A. 105, 15064–15069. doi: 10.1073/pnas.0803124105
Kaelberer, M. M., Buchanan, K. L., Klein, M. E., Barth, B. B., Montoya, M. M., Shen, X., et al. (2018). A gut-brain neural circuit for nutrient sensory transduction. Science 361:eaat5236. doi: 10.1126/science.aat5236
Kanaya, T., Williams, I. R., and Ohno, H. (2020). Intestinal M cells: tireless samplers of enteric microbiota. Traffic 21, 34–44. doi: 10.1111/tra.12707
Kang, D. W., Park, J. G., Ilhan, Z. E., Wallstrom, G., Labaer, J., Adams, J. B., et al. (2013). Reduced incidence of Prevotella and other fermenters in intestinal microflora of autistic children. PLoS One 8:e68322. doi: 10.1371/journal.pone.0068322
Kappéter, Á., Sipos, D., Varga, A., Vigvári, S., Halda-Kiss, B., and Péterfi, Z. (2023). Migraine as a disease associated with Dysbiosis and possible therapy with fecal microbiota transplantation. Microorganisms 11:2083. doi: 10.3390/microorganisms11082083
Kawashima, K., Misawa, H., Moriwaki, Y., Fujii, Y. X., Fujii, T., Horiuchi, Y., et al. (2007). Ubiquitous expression of acetylcholine and its biological functions in life forms without nervous systems. Life Sci. 80, 2206–2209. doi: 10.1016/j.lfs.2007.01.059
Kayama, H., Okumura, R., and Takeda, K. (2020). Interaction between the microbiota, epithelia, and immune cells in the intestine. Annu. Rev. Immunol. 38, 23–48. doi: 10.1146/annurev-immunol-070119-115104
Kelly, J. R., Minuto, C., Cryan, J. F., Clarke, G., and Dinan, T. G. (2021). The role of the gut microbiome in the development of schizophrenia. Schizophr. Res. 234, 4–23. doi: 10.1016/j.schres.2020.02.010
Keshavarzian, A., Green, S. J., Engen, P. A., Voigt, R. M., Naqib, A., Forsyth, C. B., et al. (2015). Colonic bacterial composition in Parkinson’s disease. Mov. Disord. 30, 1351–1360. doi: 10.1002/mds.26307
Kim, Y. S., and Ho, S. B. (2010). Intestinal goblet cells and mucins in health and disease: recent insights and progress. Curr. Gastroenterol. Rep. 12, 319–330. doi: 10.1007/s11894-010-0131-2
Kim, J. J., and Khan, W. I. (2014). 5-HT7 receptor signaling: improved therapeutic strategy in gut disorders. Front. Behav. Neurosci. 8:396. doi: 10.3389/fnbeh.2014.00396
Kim, Y. K., and Shin, C. (2018). The microbiota-gut-brain Axis in neuropsychiatric disorders: pathophysiological mechanisms and novel treatments. Curr. Neuropharmacol. 16, 559–573. doi: 10.2174/1570159X15666170915141036
Kim, N., Yun, M., Oh, Y. J., and Choi, H. J. (2018). Mind-altering with the gut: modulation of the gut-brain axis with probiotics. J. Microbiol. 56, 172–182. doi: 10.1007/s12275-018-8032-4
Kimura, S. (2018). Molecular insights into the mechanisms of M-cell differentiation and transcytosis in the mucosa-associated lymphoid tissues. Anat. Sci. Int. 93, 23–34. doi: 10.1007/s12565-017-0418-6
Kinashi, Y., and Hase, K. (2021). Partners in Leaky gut Syndrome: intestinal Dysbiosis and autoimmunity. Front. Immunol. 12:673708. doi: 10.3389/fimmu.2021.673708
Kiriyama, Y., and Nochi, H. (2019). The biosynthesis, signaling, and neurological functions of bile acids. Biomol. Ther. 9:232. doi: 10.3390/biom9060232
Klarer, M., Arnold, M., Günther, L., Winter, C., Langhans, W., and Meyer, U. (2014). Gut vagal afferents differentially modulate innate anxiety and learned fear. J. Neurosci. 34, 7067–7076. doi: 10.1523/JNEUROSCI.0252-14.2014
Knoop, K. A., and Newberry, R. D. (2018). Goblet cells: multifaceted players in immunity at mucosal surfaces. Mucosal Immunol. 11, 1551–1557. doi: 10.1038/s41385-018-0039-y
Kobayashi, N., Takahashi, D., Takano, S., Kimura, S., and Hase, K. (2019). The roles of Peyer’s patches and microfold cells in the gut immune system: relevance to autoimmune diseases. Front. Immunol. 10:2345. doi: 10.3389/fimmu.2019.02345
Konig, J., Wells, J., Cani, P. D., Garcia-Rodenas, C. L., MacDonald, T., Mercenier, A., et al. (2016). Human intestinal barrier function in health and disease. Clin. Transl. Gastroenterol. 7:e196. doi: 10.1038/ctg.2016.54
Koopman, N., Katsavelis, D., Hove, A. S. T., Brul, S., Jonge, W. J., and Seppen, J. (2021). The multifaceted role of serotonin in intestinal homeostasis. Int. J. Mol. Sci. 22:9487. doi: 10.3390/ijms22179487
Kopchak, O., and Hrytsenko, O. (2022). Feature of gut microbiota in patients with migraine and healthy individuals. Georgian Med. News 327, 13–17.
Krych, L., Hansen, C. H., Hansen, A. K., van den Berg, F. W., and Nielsen, D. S. (2013). Quantitatively different, yet qualitatively alike: a meta-analysis of the mouse core gut microbiome with a view towards the human gut microbiome. PLoS One 8:e62578. doi: 10.1371/journal.pone.0062578
Kuley, E., Balıkcı, E., Özoğul, I., Gökdogan, S., and Ozoğul, F. (2012). Stimulation of cadaverine production by foodborne pathogens in the presence of Lactobacillus, Lactococcus, and Streptococcus spp. J. Food Sci. 77, M650–M658. doi: 10.1111/j.1750-3841.2012.02825.x
Kunisawa, J., and Kiyono, H. (2013). Immune regulation and monitoring at the epithelial surface of the intestine. Drug Discov. Today 18, 87–92. doi: 10.1016/j.drudis.2012.08.001
Kyloh, M. A., Hibberd, T. J., Castro, J., Harrington, A. M., Travis, L., Dodds, K. N., et al. (2022). Disengaging spinal afferent nerve communication with the brain in live mice. Commun. Biol. 5:915. doi: 10.1038/s42003-022-03876-x
Lagos-Quintana, M., Rauhut, R., Lendeckel, W., and Tuschl, T. (2001). Identification of novel genes coding for small expressed RNAs. Science 294, 853–858. doi: 10.1126/science.1064921
Landete, J. M., De Las Rivas, B., Marcobal, A., and Munoz, R. (2008). Updated molecular knowledge about histamine biosynthesis by bacteria. Crit. Rev. Food Sci. Nutr. 48, 697–714. doi: 10.1080/10408390701639041
Landgraf, P., Rusu, M., Sheridan, R., Sewer, A., Iovino, N., Aravin, A., et al. (2007). A mammalian microRNA expression atlas based on small RNA library sequencing. Cells 129, 1401–1414. doi: 10.1016/j.cell.2007.04.040
Lanza, M., Filippone, A., Ardizzone, A., Casili, G., Paterniti, I., Esposito, E., et al. (2021). SCFA treatment alleviates pathological signs of migraine and related intestinal alterations in a mouse model of NTG-induced migraine. Cells 10:2756. doi: 10.3390/cells10102756
Li, D., Liu, R., Wang, M., Peng, R., Fu, S., Fu, A., et al. (2022). 3β-Hydroxysteroid dehydrogenase expressed by gut microbes degrades testosterone and is linked to depression in males. Cell Host Microbe 30, 329–339.e5. e5. doi: 10.1016/j.chom.2022.01.001
Li, Z., Qing, Y., Cui, G., Li, M., Liu, T., Zeng, Y., et al. (2023). Shotgun metagenomics reveals abnormal short-chain fatty acid-producing bacteria and glucose and lipid metabolism of the gut microbiota in patients with schizophrenia. Schizophr. Res. 255, 59–66. doi: 10.1016/j.schres.2023.03.005
Li, R., Wang, F., Dang, S., Yao, M., Zhang, W., and Wang, J. (2022). Integrated 16S rRNA gene sequencing and metabolomics analysis to investigate the important role of Osthole on gut microbiota and serum metabolites in neuropathic pain mice. Front. Physiol. 13:813626. doi: 10.3389/fphys.2022.813626
Li, S., Zhuo, M., Huang, X., Huang, Y., Zhou, J., Xiong, D., et al. (2020). Altered gut microbiota associated with symptom severity in schizophrenia. PeerJ 8:e9574. doi: 10.7717/peerj.9574
Liang, G., and Bushman, F. D. (2021). The human virome: assembly, composition and host interactions. Nat. Rev. Microbiol. 19, 514–527. doi: 10.1038/s41579-021-00536-5
Liu, S. R., da Cunha, A. P., Rezende, R. M., Cialic, R., Wei, Z. Y., Bry, L., et al. (2016). The host shapes the gut microbiota via fecal MicroRNA. Cell Host Microbe 19, 32–43. doi: 10.1016/j.chom.2015.12.005
Liu, J., Tan, Y., Cheng, H., Zhang, D., Feng, W., and Peng, C. (2022). Functions of gut microbiota metabolites, current status and future perspectives. Aging Dis. 13, 1106–1126. doi: 10.14336/AD.2022.0104
Liu, Y., Yu, Z., Zhu, L., Ma, S., Luo, Y., Liang, H., et al. (2023). Orchestration of MUC2 - the key regulatory target of gut barrier and homeostasis: A review. Int. J. Biol. Macromol. 236:123862. doi: 10.1016/j.ijbiomac.2023.123862
Lomax, A. E., Pradhananga, S., Sessenwein, J. L., and O’Malley, D. (2019). Bacterial modulation of visceral sensation: mediators and mechanisms. Am. J. Physiol. Gastrointest. Liver Physiol. 317, G363–G372. doi: 10.1152/ajpgi.00052.2019
Lueschow, S. R., and McElroy, S. J. (2020). The Paneth cell: the curator and defender of the immature small intestine. Front. Immunol. 11:587. doi: 10.3389/fimmu.2020.00587
Lutz, B. M., Bekker, A., and Tao, Y. X. (2014). Noncoding RNAs: new players in chronic pain. Anesthesiology 121, 409–417. doi: 10.1097/ALN.0000000000000265
Mabbott, N. A., Donaldson, D. S., Ohno, H., Williams, I. R., and Mahajan, A. (2013). Microfold (M) cells: important immunosurveillance posts in the intestinal epithelium. Mucosal Immunol. 6, 666–677. doi: 10.1038/mi.2013.30
Makadia, P. A., Najjar, S. A., Saloman, J. L., Adelman, P., Feng, B., Margiotta, J. F., et al. (2018). Optogenetic activation of Colon epithelium of the mouse produces high-frequency bursting in extrinsic Colon afferents and engages visceromotor responses. J. Neurosci. 38, 5788–5798. doi: 10.1523/JNEUROSCI.0837-18.2018
Margolis, K. G., Cryan, J. F., and Mayer, E. A. (2021). The microbiota-gut-brain axis: from motility to mood. Gastroenterology 160, 1486–1501. doi: 10.1053/j.gastro.2020.10.066
Martin, C. R., Osadchiy, V., Kalani, A., and Mayer, E. A. (2018). The brain-gut-microbiome Axis. Cell. Mol. Gastroenterol. Hepatol. 6, 133–148. doi: 10.1016/j.jcmgh.2018.04.003
Matsumoto, M., Kibe, R., Ooga, T., Aiba, Y., Sawaki, E., Koga, Y., et al. (2013). Cerebral low-molecular metabolites influenced by intestinal microbiota: a pilot study. Front. Syst. Neurosci. 7:9. doi: 10.3389/fnsys.2013.00009
Mavris, M., and Sansonetti, P. (2004). Microbial-gut interactions in health and disease. Epithelial cell responses. Best Pract. Res. Clin. Gastroenterol. 18, 373–386. doi: 10.1016/j.bpg.2003.10.007
Mawe, G. M., and Hoffman, J. M. (2013). Serotonin signalling in the gut—functions, dysfunctions and therapeutic targets. Nat. Rev. Gastroenterol. Hepatol. 10, 473–486. doi: 10.1038/nrgastro.2013.105
Mayer, E. A., Ryu, H. J., and Bhatt, R. R. (2023). The neurobiology of irritable bowel syndrome. Mol. Psychiatry 28, 1451–1465. doi: 10.1038/s41380-023-01972-w
Mayneris-Perxachs, J., Castells-Nobau, A., Arnoriaga-Rodríguez, M., Garre-Olmo, J., Puig, J., Ramos, R., et al. (2022). Caudovirales bacteriophages are associated with improved executive function and memory in flies, mice, and humans. Cell Host Microbe 30, 340–356.e8. doi: 10.1016/j.chom.2022.01.013
McCauley, H. A., and Guasch, G. (2015). Three cheers for the goblet cell: maintaining homeostasis in mucosal epithelia. Trends Mol. Med. 21, 492–503. doi: 10.1016/j.molmed.2015.06.003
McGuinness, A. J., Davis, J. A., Dawson, S. L., Loughman, A., Collier, F., O’Hely, M., et al. (2022). A systematic review of gut microbiota composition in observational studies of major depressive disorder, bipolar disorder and schizophrenia. Mol. Psychiatry 27, 1920–1935. doi: 10.1038/s41380-022-01456-3
Mckernan, D. P., Fitzgerald, P., Dinan, T. G., and Cryan, J. F. (2010). The probiotic Bifidobacterium infantis 35624 displays visceral antinociceptive effects in the rat. Neurogastroenterol. Motil. 22, 1029–e268. doi: 10.1111/j.1365-2982.2010.01520.x
Megur, A., Baltriukienė, D., Bukelskienė, V., and Burokas, A. (2021). The microbiota–gut–brain Axis and Alzheimer’s disease: Neuroinflammation is to blame? Nutrients 13:37. doi: 10.3390/nu13010037
Meyer, R. K., and Duca, F. A. (2023). Endocrine regulation of metabolic homeostasis via the intestine and gut microbiome. J. Endocrinol. 258:e230019. doi: 10.1530/JOE-23-0019
Minerbi, A., Gonzalez, E., Brereton, N., Fitzcharles, M. A., Chevalier, S., and Shir, Y. (2023). Altered serum bile acid profile in fibromyalgia is associated with specific gut microbiome changes and symptom severity. Pain 164, e66–e76. doi: 10.1097/j.pain.0000000000002694
Modesto Lowe, V., Chaplin, M., and Sgambato, D. (2023). Major depressive disorder and the gut microbiome: what is the link? Gen Psychiatr 36:e100973. doi: 10.1136/gpsych-2022-100973
Moloney, R. D., Johnson, A. C., O’Mahony, S. M., Dinan, T. G., Greenwood-Van Meerveld, B., and Cryan, J. F. (2016). Stress and the microbiota-gut-brain Axis in visceral pain: relevance to irritable bowel syndrome. CNS Neurosci. Ther. 22, 102–117. doi: 10.1111/cns.12490
Moloney, R. D., O’Mahony, S. M., Dinan, T. G., and Cryan, J. F. (2015). Stress-induced visceral pain: toward animal models of irritable-bowel syndrome and associated comorbidities. Front. Psychiatry 6:15. doi: 10.3389/fpsyt.2015.00015
Montiel-Castro, A. J., González-Cervantes, R. M., Bravo-Ruiseco, G., and Pacheco-López, G. (2013). The microbiota-gut-brain axis: neurobehavioral correlates, health and sociality. Front. Integr. Neurosci. 7:70. doi: 10.3389/fnint.2013.00070
Mörkl, S., Butler, M. I., Holl, A., Cryan, J. F., and Dinan, T. G. (2020). Probiotics and the microbiota-gut-brain Axis: focus on psychiatry. Curr. Nutr. Rep. 9, 171–182. doi: 10.1007/s13668-020-00313-5
Muller, P. A., Schneeberger, M., Matheis, F., Wang, P., Kerner, Z., Ilanges, A., et al. (2020). Microbiota modulate sympathetic neurons via a gut–brain circuit. Nature 583, 441–446. doi: 10.1038/s41586-020-2474-7
Nagler-Anderson, C. (2001). Man the barrier! Strategic defences in the intestinal mucosa. Nat. Rev. Immunol. 1, 59–67. doi: 10.1038/35095573
Nagpal, R., Wang, S., Solberg Woods, L. C., Seshie, O., Chung, S. T., Shively, C. A., et al. (2018). Comparative microbiome signatures and short-chain fatty acids in mouse, rat, non-human primate, and human feces. Front Microbiol 9:2897. doi: 10.3389/fmicb.2018.02897
Najjar, S. A., Davis, B. M., and Albers, K. M. (2020). Epithelial-neuronal communication in the Colon: implications for visceral pain. Trends Neurosci. 43, 170–181. doi: 10.1016/j.tins.2019.12.007
Nesci, A., Carnuccio, C., Ruggieri, V., D’Alessandro, A., Di Giorgio, A., Santoro, L., et al. (2023). Gut microbiota and cardiovascular disease: evidence on the metabolic and inflammatory background of a complex relationship. Int. J. Mol. Sci. 24:9087. doi: 10.3390/ijms24109087
Neufeld, K. M., Kang, N., Bienenstock, J., and Foster, J. A. (2011). Reduced anxiety-like behavior and central neurochemical change in germ-free mice. Neurogastroenterol. Motil. 23, 255–264, e119. doi: 10.1111/j.1365-2982.2010.01620.x
Nguyen, T. T., Kosciolek, T., Maldonado, Y., Daly, R. E., Martin, A. S., McDonald, D., et al. (2019). Differences in gut microbiome composition between persons with chronic schizophrenia and healthy comparison subjects. Schizophr. Res. 204, 23–29. doi: 10.1016/j.schres.2018.09.014
Nishino, R., Mikami, K., Takahashi, H., Tomonaga, S., Furuse, M., Hiramoto, T., et al. (2013). Commensal microbiota modulate murine behaviors in a strictly contamination-free environment confirmed by culture-based methods. Neurogastroenterol. Motil. 25, 521–e371. doi: 10.1111/nmo.12110
Nutman, A. P., Bennett, V. C., Friend, C. R., Van Kranendonk, M. J., and Chivas, A. R. (2016). Rapid emergence of life shown by discovery of 3,700-million-year-old microbial structures. Nature 537, 535–538. doi: 10.1038/nature19355
O’Mahony, S. M., Clarke, G., Borre, Y., Dinan, T. G., and Cryan, J. (2015). Serotonin, tryptophan metabolism and the brain-gut-microbiome axis. Behav. Brain Res. 277, 32–48. doi: 10.1016/j.bbr.2014.07.027
O’Leary, O. F., Ogbonnaya, E. S., Felice, D., Levone, B. R. L. C. C., Fitzgerald, P., Bravo, J. A., et al. (2018). The vagus nerve modulates BDNF expression and neurogenesis in the hippocampus. Eur. Neuropsychopharmacol. 28, 307–316. doi: 10.1016/j.euroneuro.2017.12.004
Oliveira, R. A., Cabral, V., Torcato, I., and Xavier, K. B. (2023). Deciphering the quorum-sensing lexicon of the gut microbiota. Cell Host Microbe 31, 500–512. doi: 10.1016/j.chom.2023.03.015
O’Mahony, S. M., Felice, V. D., Nally, K., Savignac, H. M., Claesson, M. J., Scully, P., et al. (2014). Disturbance of the gut microbiota in early-life selectively affects visceral pain in adulthood without impacting cognitive or anxiety-related behaviors in male rats. Neuroscience 277, 885–901. doi: 10.1016/j.neuroscience.2014.07.054
Oroojzadeh, P., Bostanabad, S. Y., and Lotfi, H. (2022). Psychobiotics: the influence of gut microbiota on the gut-brain Axis in neurological disorders. J. Mol. Neurosci. 72, 1952–1964. doi: 10.1007/s12031-022-02053-3
Osinski, C., Moret, D., Clement, K., Serradas, P., and Ribeiro, A. (2022). Enteroendocrine system and gut barrier in metabolic disorders. Int. J. Mol. Sci. 23:3732. doi: 10.3390/ijms23073732
Ozaki, N., and Gebhart, G. F. (2001). Characterization of mechanosensitive splanchnic nerve afferent fibers innervating the rat stomach. Am. J. Physiol. Gastrointest. Liver Physiol. 281, G1449–G1459. doi: 10.1152/ajpgi.2001.281.6.G1449
Ozogul, F. (2011). Effects of specific lactic acid bacteria species on biogenic amine production by foodborne pathogen. Int. J. Food Sci. Technol. 46, 478–484. doi: 10.1111/j.1365-2621.2010.02511.x
Park, J. C., and Im, S.-H. (2020). Of men in mice: the development and application of a humanized gnotobiotic mouse model for microbiome therapeutics. Exp. Mol. Med. 52, 1383–1396. doi: 10.1038/s12276-020-0473-2
Park, J., and Kim, C. H. (2021). Regulation of common neurological disorders by gut microbial metabolites. Exp. Mol. Med. 53, 1821–1833. doi: 10.1038/s12276-021-00703-x
Pärtty, A., Kalliomäki, M., Wacklin, P., Salminen, S., and Isolauri, E. (2015). A possible link between early probiotic intervention and the risk of neuropsychiatric disorders later in childhood: a randomized trial. Pediatr. Res. 77, 823–828. doi: 10.1038/pr.2015.51
Pavlov, V. A., and Tracey, K. J. (2005). The cholinergic anti-inflammatory pathway. Brain Behav. Immun. 19, 493–499. doi: 10.1016/j.bbi.2005.03.015
Pelaseyed, T., Bergstrom, J. H., Gustafsson, J. K., Ermund, A., Birchenough, G. M., Schutte, A., et al. (2014). The mucus and mucins of the goblet cells and enterocytes provide the first defense line of the gastrointestinal tract and interact with the immune system. Immunol. Rev. 260, 8–20. doi: 10.1111/imr.12182
Pelaseyed, T., and Hansson, G. C. (2020). Membrane mucins of the intestine at a glance. J. Cell Sci. 133:jcs240929. doi: 10.1242/jcs.240929
Perez-Burgos, A., Wang, L., McVey Neufeld, K.-A., Mao, Y.-K., Ahmadzai, M., Janssen, L. J., et al. (2015). The TRPV1 channel in rodents is a major target for antinociceptive effect of the probiotic Lactobacillus reuteri DSM 17938. J. Physiol. 593, 3943–3957. doi: 10.1113/JP270229
Peterson, L. W., and Artis, D. (2014). Intestinal epithelial cells: regulators of barrier function and immune homeostasis. Nat. Rev. Immunol. 14, 141–153. doi: 10.1038/nri3608
Petrie, G. N., Nastase, A. S., Aukema, R. J., and Hill, M. N. (2021). Endocannabinoids, cannabinoids and the regulation of anxiety. Neuropharmacology 195:108626. doi: 10.1016/j.neuropharm.2021.108626
Piche, T., Barbara, G., Aubert, P., Bruley Des Varannes, S., Dainese, R., Nano, J. L., et al. (2009). Impaired intestinal barrier integrity in the colon of patients with irritable bowel syndrome: involvement of soluble mediators. Gut 58, 196–201. doi: 10.1136/gut.2007.140806
Powley, T. L., Spaulding, R. A., and Haglof, S. A. (2011). Vagal afferent innervation of the proximal gastrointestinal tract mucosa: chemoreceptor and mechanoreceptor architecture. J. Comp. Neurol. 519, 644–660. doi: 10.1002/cne.22541
Prescott, S. L., and Liberles, S. D. (2022). Internal senses of the vagus nerve. Neuron 110, 579–599. doi: 10.1016/j.neuron.2021.12.020
Pujo, J., De Palma, G., Lu, J., Galipeau, H. J., Surette, M. G., Collins, S. M., et al. (2023). Gut microbiota modulates visceral sensitivity through calcitonin gene-related peptide (CGRP) production. Gut Microbes 15:2188874. doi: 10.1080/19490976.2023.2188874
Rajendiran, E., Ramadass, B., and Ramprasath, V. (2021). Understanding connections and roles of gut microbiome in cardiovascular diseases. Can. J. Microbiol. 67, 101–111. doi: 10.1139/cjm-2020-0043
Raskov, H., Burcharth, J., Pommergaard, H. C., and Rosenberg, J. (2016). Irritable bowel syndrome, the microbiota and the gut-brain axis. Gut Microbes 7, 365–383. doi: 10.1080/19490976.2016.1218585
Rhee, S. H., Pothoulakis, C., and Mayer, E. A. (2009). Principles and clinical implications of the brain-gut-enteric microbiota axis. Nat. Rev. Gastroenterol. Hepatol. 6, 306–314. doi: 10.1038/nrgastro.2009.35
Rieder, R., Wisniewski, P. J., Alderman, B. L., and Campbell, S. C. (2017). Microbes and mental health: A review. Brain Behav. Immun. 66, 9–17. doi: 10.1016/j.bbi.2017.01.016
Rincel, M., Aubert, P., Chevalier, J., Grohard, P. A., Basso, L., Monchaux de Oliveira, C., et al. (2019). Multi-hit early life adversity affects gut microbiota, brain and behavior in a sex-dependent manner. Brain Behav. Immun. 80, 179–192. doi: 10.1016/j.bbi.2019.03.006
Sampson, T. R., and Mazmanian, S. K. (2015). Control of brain development, function, and behavior by the microbiome. Cell Host Microbe 17, 565–576. doi: 10.1016/j.chom.2015.04.011
Sarnoff, R. P., Bhatt, R. R., Osadchiy, V., Dong, T., Labus, J. S., Kilpatrick, L. A., et al. (2023). A multi-omic brain gut microbiome signature differs between IBS subjects with different bowel habits. Neuropharmacology 225:109381. doi: 10.1016/j.neuropharm.2022.109381
Sasso, J. M., Ammar, R. M., Tenchov, R., Lemmel, S., Kelber, O., Grieswelle, M., et al. (2023). Gut microbiome-brain Alliance: A landscape view into mental and gastrointestinal health and disorders. ACS Chem. Neurosci. 14, 1717–1763. doi: 10.1021/acschemneuro.3c00127
Schneider, C., O’Leary, C. E., and Locksley, R. M. (2019). Regulation of immune responses by tuft cells. Nat. Rev. Immunol. 19, 584–593. doi: 10.1038/s41577-019-0176-x
Sekirov, I., Russell, S. L., Antunes, L. C., and Finlay, B. B. (2010). Gut microbiota in health and disease. Physiol. Rev. 90, 859–904. doi: 10.1152/physrev.00045.2009
Sengupta, J. N. (2009). Visceral pain: the neurophysiological mechanism. Handb. Exp. Pharmacol. 194, 31–74. doi: 10.1007/978-3-540-79090-7_2
Seo, K., Seo, J., Yeun, J., Choi, H., Kim, Y. I., and Chang, S. Y. (2021). The role of mucosal barriers in human gut health. Arch. Pharm. Res. 44, 325–341. doi: 10.1007/s12272-021-01327-5
Shahab, M., and Shahab, N. (2022). Coevolution of the human host and gut microbiome: metagenomics of microbiota. Cureus 14:e26310. doi: 10.7759/cureus.26310
Shaikh, S. D., Sun, N., Canakis, A., Park, W. Y., and Weber, H. C. (2023). Irritable bowel syndrome and the gut microbiome: a comprehensive review. J. Clin. Med. 12:2558. doi: 10.3390/jcm12072558
Shannon, K. M. (2022). Gut-derived sterile inflammation and Parkinson’s disease. Front. Neurol. 13:831090. doi: 10.3389/fneur.2022.831090
Sharkey, K. A., and Mawe, G. M. (2023). The enteric nervous system. Physiol. Rev. 103, 1487–1564. doi: 10.1152/physrev.00018.2022
Sharon, G., Sampson, T. R., Geschwind, D. H., and Mazmanian, S. K. (2016). The central nervous system and the gut microbiome. Cells 167, 915–932. doi: 10.1016/j.cell.2016.10.027
Shen, Y., Xu, J., Li, Z., Huang, Y., Yuan, Y., Wang, J., et al. (2018). Analysis of gut microbiota diversity and auxiliary diagnosis as a biomarker in patients with schizophrenia: a cross-sectional study. Schizophr. Res. 197, 470–477. doi: 10.1016/j.schres.2018.01.002
Shin, A., and Kashyap, P. C. (2023). Multi-omics for biomarker approaches in the diagnostic evaluation and management of abdominal pain and irritable bowel syndrome: what lies ahead. Gut Microbes 15:2195792. doi: 10.1080/19490976.2023.2195792
Shu, L. Z., Ding, Y. D., Xue, Q. M., Cai, W., and Deng, H. (2023). Direct and indirect effects of pathogenic bacteria on the integrity of intestinal barrier. Ther. Adv. Gastroenterol. 16:17562848231176427. doi: 10.1177/17562848231176427
Simpson, C. A., Diaz-Arteche, C., Eliby, D., Schwartz, O. S., Simmons, J. G., and Cowan, C. S. (2021). The gut microbiota in anxiety and depression–A systematic review. Clin. Psychol. Rev. 83:101943. doi: 10.1016/j.cpr.2020.101943
Sisk-Hackworth, L., Kelley, S. T., and Thackray, V. G. (2023). Sex, puberty, and the gut microbiome. Reproduction 165, R61–R74. doi: 10.1530/REP-22-0303
Socała, K., Doboszewska, U., Szopa, A., Serefko, A., Włodarczyk, M., Zielińska, A., et al. (2021). The role of microbiota-gut-brain axis in neuropsychiatric and neurological disorders. Pharmacol. Res. 172:105840. doi: 10.1016/j.phrs.2021.105840
Soderholm, A. T., and Pedicord, V. A. (2019). Intestinal epithelial cells: at the interface of the microbiota and mucosal immunity. Immunology 158, 267–280. doi: 10.1111/imm.13117
Sommer, F., and Backhed, F. (2013). The gut microbiota — masters of host development and physiology. Nat. Rev. Microbiol. 11, 227–238. doi: 10.1038/nrmicro2974
Spencer, N. J., Hibberd, T. J., Lagerström, M., Otsuka, Y., and Kelley, N. (2018). Visceral pain - novel approaches for optogenetic control of spinal afferents. Brain Res. 1693, 159–164. doi: 10.1016/j.brainres.2018.02.002
Spencer, N. J., Hibberd, T., Xie, Z., and Hu, H. (2022). How should we define a nociceptor in the gut-brain axis? Front. Neurosci. 16:1096405. doi: 10.3389/fnins.2022.1096405
Spencer, N. J., and Hu, H. (2020). Enteric nervous system: sensory transduction, neural circuits and gastrointestinal motility. Nat. Rev. Gastroenterol. Hepatol. 17, 338–351. doi: 10.1038/s41575-020-0271-2
Stavropoulou, E., Kantartzi, K., Tsigalou, C., Konstantinidis, T., Romanidou, G., Voidarou, C., et al. (2020). Focus on the gut-kidney Axis in health and disease. Front Med (Lausanne) 7:620102. doi: 10.3389/fmed.2020.620102
Strandwitz, P., Kim, K. H., Terekhova, D., Liu, J. K., Sharma, A., Levering, J., et al. (2019). GABA-modulating bacteria of the human gut microbiota. Nat. Microbiol. 4, 396–403. doi: 10.1038/s41564-018-0307-3
Strine, M. S., and Wilen, C. B. (2022). Tuft cells are key mediators of interkingdom interactions at mucosal barrier surfaces. PLoS Pathog. 18:e1010318. doi: 10.1371/journal.ppat.1010318
Sutterland, A. L., Fond, G., Kuin, A., Koeter, M. W. J., Lutter, R., van Gool, T., et al. (2015). Beyond the association. Toxoplasma gondii in schizophrenia, bipolar disorder, and addiction: systematic review and meta-analysis. Acta Psychiatr. Scand. 132, 161–179. doi: 10.1111/acps.12423
Takanaga, T., Ohtsuki, S., Hosoya, K. I., and Terasaki, T. (2001). GAT2/BGT-1 as a system responsible for the transport of gamma-aminobutyric acid at the mouse blood-brain barrier. J. Cereb. Blood Flow Metab. 21, 1232–1239. doi: 10.1097/00004647-200110000-00012
Thomas, C. M., Hong, T., van Pijkeren, J. P., Hemarajata, P., Trinh, D. V., Hu, W. D., et al. (2012). Histamine derived from probiotic Lactobacillus reuteri suppresses TNF via modulation of PKA and ERK signaling. PLoS One 7:e31951. doi: 10.1371/journal.pone.0031951
Toral, M., Robles-Vera, I., de la Visitación, N., Romero, M., Yang, T., Sánchez, M., et al. (2019). Critical role of the interaction gut microbiota - sympathetic nervous system in the regulation of blood pressure. Front. Physiol. 10:231. doi: 10.3389/fphys.2019.00231
Tran, S. M.-S., and Mohajeri, M. H. (2021). The role of gut bacterial metabolites in brain development, aging and disease. Nutrients 13:732. doi: 10.3390/nu13030732
Tropini, C., Earle, K. A., Huang, K. C., and Sonnenburg, J. L. (2017). The gut microbiome: connecting spatial organization to function. Cell Host Microbe 21, 433–442. doi: 10.1016/j.chom.2017.03.010
Turnbaugh, P. J., Ridaura, V. K., Faith, J. J., Rey, F. E., Knight, R., and Gordon, J. I. (2009). The effect of diet on the human gut microbiome: a metagenomic analysis in humanized gnotobiotic mice. Sci. Transl. Med. 1:6ra14. doi: 10.1126/scitranslmed.3000322
Udit, S., Blake, K., and Chiu, I. M. (2022). Somatosensory and autonomic neuronal regulation of the immune response. Nat. Rev. Neurosci. 23, 157–171. doi: 10.1038/s41583-021-00555-4
Uhlig, F., Grundy, L., Garcia-Caraballo, S., Brierley, S. M., Foster, S. J., and Grundy, D. (2020). Identification of a quorum sensing-dependent communication pathway mediating Bacteria-gut-brain Cross talk. iScience 23:101695. doi: 10.1016/j.isci.2020.101695
Uhlig, F., and Hyland, N. P. (2022). Making sense of quorum sensing at the intestinal mucosal interface. Cells 11:1734. doi: 10.3390/cells11111734
Ustianowska, K., Ustianowski, Ł., Machaj, F., Gorący, A., Rosik, J., Szostak, B., et al. (2022). The role of the human microbiome in the pathogenesis of pain. Int. J. Mol. Sci. 23:13267. doi: 10.3390/ijms232113267
Vagnerová, K., Vodička, M., Hermanová, P., Ergang, P., Šrůtková, D., Klusoňová, P., et al. (2019). Interactions between gut microbiota and acute restraint stress in peripheral structures of the hypothalamic–pituitary–adrenal Axis and the intestine of male mice. Front. Immunol. 10:2655. doi: 10.3389/fimmu.2019.02655
Valles-Colomer, M., Falony, G., Darzi, Y., Tigchelaar, E. F., Wang, J., Tito, R. Y., et al. (2019). The neuroactive potential of the human gut microbiota in quality of life and depression. Nat. Microbiol. 4, 623–632. doi: 10.1038/s41564-018-0337-x
Van Pee, T., Hogervorst, J., Dockx, Y., Witters, K., Thijs, S., Wang, C., et al. (2023). Accumulation of black carbon particles in placenta, cord blood, and childhood urine in association with the intestinal microbiome diversity and composition in four- to six-year-old children in the ENVIRONAGE birth cohort. Environ. Health Perspect. 131:17010. doi: 10.1289/EHP11257
Varatharaj, A., and Galea, I. (2017). The blood-brain barrier in systemic inflammation. Brain Behav. Immun. 60, 1–12. doi: 10.1016/j.bbi.2016.03.010
Veening, J. G., and Barendregt, H. P. (2015). The effects of beta-endorphin: state change modification. Fluids Barriers CNS 12:3. doi: 10.1186/2045-8118-12-3
Wallaeys, C., Garcia-Gonzalez, N., and Libert, C. (2023). Paneth cells as the cornerstones of intestinal and organismal health: a primer. EMBO Mol. Med. 15:e16427. doi: 10.15252/emmm.202216427
Wallon, C., Yang, P.-C., Keita, Å. V., Ericson, A.-C., McKay, D. M., Sherman, P. M., et al. (2008). Corticotropin-releasing hormone (CRH) regulates macromolecular permeability via mast cells in normal human colonic biopsies in vitro. Gut 57, 50–58. doi: 10.1136/gut.2006.117549
Wang, H., Guo, X., Zhu, X., Li, Y., Jia, Y., Zhang, Z., et al. (2021). Gender differences and postoperative delirium in adult patients undergoing cardiac valve surgery. Front Cardiovasc Med 8:751421. doi: 10.3389/fcvm.2021.751421
Wang, Y., Tong, Q., Ma, S. R., Zhao, Z. X., Pan, L. B., Cong, L., et al. (2021). Oral berberine improves brain dopa/dopamine levels to ameliorate Parkinson’s disease by regulating gut microbiota. Signal Transduct. Target. Ther. 6:77. doi: 10.1038/s41392-020-00456-5
Wei, L., Singh, R., and Ghoshal, U. C. (2022). Enterochromaffin cells-gut microbiota crosstalk: underpinning the symptoms, pathogenesis, and pharmacotherapy in disorders of gut-brain interaction. J Neurogastroenterol Motil 28, 357–375. doi: 10.5056/jnm22008
Wells, J. M., Rossi, O., Meijerink, M., and van Baarlen, P. (2011). Epithelial crosstalk at the microbiota-mucosal interface. Proc. Natl. Acad. Sci. U. S. A. 108, 4607–4614. doi: 10.1073/pnas.1000092107
Wen, Z., He, M., Peng, C., Rao, Y., Li, J., Li, Z., et al. (2019). Metabolomics and 16S rRNA gene sequencing analyses of changes in the intestinal Flora and Biomarkers induced by Gastrodia-Uncaria treatment in a rat model of chronic migraine. Front. Pharmacol. 10:1425. doi: 10.3389/fphar.2019.01425
Wen, C., Wei, S., Zong, X., Wang, Y., and Jin, M. (2021). Microbiota-gut-brain axis and nutritional strategy under heat stress. Anim Nutr 7, 1329–1336. doi: 10.1016/j.aninu.2021.09.008
Wittmann, M., Kirfel, A., Jossen, D., Mayr, A., and Menzenbach, J. (2022). The impact of perioperative and predisposing risk factors on the development of postoperative delirium and a possible gender difference. Geriatrics 7:65. doi: 10.3390/geriatrics7030065
Wood, J. D. (2004). “Sympathetic innervation” in Encyclopedia of Gastroenterology. ed. L. R. Johnson (New York: Elsevier), 484–485.
Wrase, J., Reimold, M., Puls, I., Kienast, T., and Heinz, A. (2006). Serotonergic dysfunction: brain imaging and behavioral correlates. Cogn. Affect. Behav. Neurosci. 6, 53–61. doi: 10.3758/CABN.6.1.53
Xu, X., Chen, R., Zhan, G., Wang, D., Tan, X., and Xu, H. (2021). Enterochromaffin cells: sentinels to gut microbiota in hyperalgesia? Front. Cell. Infect. Microbiol. 11:760076. doi: 10.3389/fcimb.2021.760076
Yan, S., and Kentner, A. C. (2017). Mechanical allodynia corresponds to Oprm1 downregulation within the descending pain network of male and female rats exposed to neonatal immune challenge. Brain Behav. Immun. 63, 148–159. doi: 10.1016/j.bbi.2016.10.007
Yang, D., Jacobson, A., Meerschaert, K. A., Sifakis, J. J., Wu, M., Chen, X., et al. (2022). Nociceptor neurons direct goblet cells via a CGRP-RAMP1 axis to drive mucus production and gut barrier protection. Cells 185, 4190–4205.e25. doi: 10.1016/j.cell.2022.09.024
Yano, J. M., Yu, K., Donaldson, G. P., Shastri, G. G., Ann, P., Ma, L., et al. (2015). Indigenous bacteria from the gut microbiota regulate host serotonin biosynthesis. Cells 161, 264–276. doi: 10.1016/j.cell.2015.02.047
Ye, L., Bae, M., Cassilly, C. D., Jabba, S. V., Thorpe, D. W., Martin, A. M., et al. (2021). Enteroendocrine cells sense bacterial tryptophan catabolites to activate enteric and vagal neuronal pathways. Cell Host Microbe 29, 179–196.e9. doi: 10.1016/j.chom.2020.11.011
Yousefi, B., Kokhaei, P., Mehranfar, F., Bahar, A., Abdolshahi, A., Emadi, A., et al. (2022). The role of the host microbiome in autism and neurodegenerative disorders and effect of epigenetic procedures in the brain functions. Neurosci. Biobehav. Rev. 132, 998–1009. doi: 10.1016/j.neubiorev.2021.10.046
Yu, L., and Li, Y. (2022). Involvement of intestinal Enteroendocrine cells in neurological and psychiatric disorders. Biomedicine 10:2577. doi: 10.3390/biomedicines10102577
Yu, L. C., Wang, J. T., Wei, S. C., and Ni, Y. H. (2012). Host-microbial interactions and regulation of intestinal epithelial barrier function: from physiology to pathology. World J Gastrointest Pathophysiol 3, 27–43. doi: 10.4291/wjgp.v3.i1.27
Zeidler, M., Kummer, K. K., Schopf, C. L., Kalpachidou, T., Kern, G., Cader, M. Z., et al. (2021). NOCICEPTRA: gene and microRNA signatures and their trajectories characterizing human iPSC-derived nociceptor maturation. Adv Sci (Weinh) 8:e2102354. doi: 10.1002/advs.202102354
Zeng, M., Peng, M., Liang, J., and Sun, H. (2023). The role of gut microbiota in blood–brain barrier disruption after stroke. Mol. Neurobiol. doi: 10.1007/s12035-023-03512-7 [Epub ahead of print].
Zhang, W., Lyu, M., Bessman, N. J., Xie, Z., Arifuzzaman, M., Yano, H., et al. (2022). Gut-innervating nociceptors regulate the intestinal microbiota to promote tissue protection. Cells 185, 4170–4189.e20. doi: 10.1016/j.cell.2022.09.008
Zhang, J. L., Xian, H., Zhao, R., Luo, C., Xie, R. G., Tian, T., et al. (2023). Brachial plexus avulsion induced changes in gut microbiota promotes pain related anxiety-like behavior in mice. Front. Neurol. 14:1084494. doi: 10.3389/fneur.2023.1084494
Zheng, Z., and Wang, B. (2021). The gut-liver Axis in health and disease: the role of gut microbiota-derived signals in liver injury and regeneration. Front. Immunol. 12:775526. doi: 10.3389/fimmu.2021.775526
Zheng, Z., Wang, S., Wu, C., Cao, Y., Gu, Q., Zhu, Y., et al. (2022). Gut microbiota Dysbiosis after traumatic brain injury contributes to persistent microglial activation associated with upregulated Lyz2 and shifted tryptophan metabolic phenotype. Nutrients 14:3467. doi: 10.3390/nu14173467
Zhou, B., Jin, G., Pang, X., Mo, Q., Bao, J., Liu, T., et al. (2022). Lactobacillus rhamnosus GG colonization in early life regulates gut-brain axis and relieves anxiety-like behavior in adulthood. Pharmacol. Res. 177:106090. doi: 10.1016/j.phrs.2022.106090
Zhu, Y., Carvey, P. M., and Ling, Z. (2007). Altered glutathione homeostasis in animals prenatally exposed to lipopolysaccharide. Neurochem. Int. 50, 671–680. doi: 10.1016/j.neuint.2006.12.013
Zhu, H., Liu, W., and Fang, H. (2018). Inflammation caused by peripheral immune cells across into injured mouse blood brain barrier can worsen postoperative cognitive dysfunction induced by isoflurane. BMC Cell Biol. 19:23. doi: 10.1186/s12860-018-0172-1
Glossary
Keywords: gut-brain axis, neuropathic pain, migraine mental disorder, schizophrenia, major depressive disorder
Citation: Riehl L, Fürst J, Kress M and Rykalo N (2024) The importance of the gut microbiome and its signals for a healthy nervous system and the multifaceted mechanisms of neuropsychiatric disorders. Front. Neurosci. 17:1302957. doi: 10.3389/fnins.2023.1302957
Edited by:
Takefumi Kikusui, Azabu University, JapanReviewed by:
Shiro Tochitani, Suzuka University of Medical Science, JapanAimee Parker, Quadram Institute, United Kingdom
Copyright © 2024 Riehl, Fürst, Kress and Rykalo. This is an open-access article distributed under the terms of the Creative Commons Attribution License (CC BY). The use, distribution or reproduction in other forums is permitted, provided the original author(s) and the copyright owner(s) are credited and that the original publication in this journal is cited, in accordance with accepted academic practice. No use, distribution or reproduction is permitted which does not comply with these terms.
*Correspondence: Nadiia Rykalo, bmFkaWlhLnJ5a2Fsb0BpLW1lZC5hYy5hdA==