- 1Department of Physiology, Faculty of Medical Sciences in Katowice, Medical University of Silesia, Katowice, Poland
- 2GlaucoTech Co., Katowice, Poland
- 3Department of Ophthalmology, Faculty of Medical Sciences in Katowice, Medical University of Silesia, Katowice, Poland
- 4Department of Ophthalmology, Professor K. Gibinski University Clinical Center, Medical University of Silesia, Katowice, Poland
Glaucoma is a complex and progressive disease that primarily affects the optic nerve axons, leading to irreversible vision loss. Although the exact molecular mechanisms underlying glaucoma pathogenesis are not fully understood, it is believed that except increased intraocular pressure, a combination of genetic and environmental factors play a role in the development of the disease. Animal models have been widely used in the study of glaucoma, allowing researchers to better understand the underlying mechanisms of the disease and test potential treatments. Several molecular pathways have been implicated in the pathogenesis of glaucoma, including oxidative stress, inflammation, and excitotoxic-induced neurodegeneration. This review summarizes the most important knowledge about molecular mechanisms involved in the glaucoma development. Although much research has been done to better understand the molecular mechanisms underlying this disease, there is still much to be learned to develop effective treatments and prevent vision loss in those affected by glaucoma.
Introduction
Glaucoma, a silent thief of vision, affects millions of people worldwide (Tham et al., 2014). It is a complex eye condition characterized by progressive damage to the optic nerve axons, which are responsible for transmitting visual information from the retina to the brain (Osborne, 2008; Weinreb et al., 2014). The onset of glaucoma can be gradual and often has no symptoms until it has caused significant vision impairment, making early detection and treatment crucial for preventing blindness (Wagner et al., 2022). There are various types of glaucoma, including primary open-angle glaucoma, angle-closure glaucoma, and secondary glaucoma, each with unique causes and risk factors (Davis et al., 2016; XXX, 2017; Nakazawa and Fukuchi, 2020). Risk factors for glaucoma include age, family history, elevated intraocular pressure (IOP), high refractive errors, this cornea, and certain medical conditions, such as diabetes and high blood pressure (Chauhan et al., 2010; Peters et al., 2013). Diagnosis of glaucoma involves a comprehensive eye examination, including measuring IOP, performing visual field tests, and evaluating the optic nerve for signs of damage. Treatment for glaucoma may include prescription eye drops, laser therapy, or surgery, depending on the type and severity of the condition. It is imperative for individuals to understand the causes, symptoms, and risk factors of glaucoma to take steps to protect their eye health and preserve their vision. Regular eye exams, especially for those at high risk for developing glaucoma, are critical for early detection and effective treatment of this condition.
Many glaucoma patients do not exhibit elevated IOP (Mallick et al., 2016). Consequently, conventional treatments primarily focused on reducing IOP may not effectively manage all forms of glaucoma. This highlights the heterogeneous nature of the disease, with multiple underlying mechanisms contributing to its development and progression. For individuals with normal tension glaucoma (NTG) or other forms where IOP remains within the normal range, alternative therapeutic approaches beyond IOP reduction are necessary to address the diverse etiology of the condition. Such approaches may include targeting neuroprotective pathways, addressing vascular dysfunction, mitigating neuroinflammation, or enhancing cellular resilience against oxidative stress. By recognizing the variability in IOP levels among glaucoma patients and acknowledging the limitations of IOP-centric treatments, there is an opportunity to develop more tailored and effective management strategies.
One of the key signaling pathways involved in glaucoma is the apoptotic pathway (Levkovitch-Verbin, 2015). Apoptosis, or programmed cell death, has been shown to play a role in the death of retinal ganglion cells (RGCs), the nerve cells responsible for transmitting visual information to the brain, in glaucoma. Studies have shown that elevated IOP and oxidative stress, two key factors in the development of glaucoma, can activate apoptotic signaling pathways, leading to the death of RGCs (Mochizuki et al., 2012; d’Azy et al., 2016). Another signaling pathway involved in glaucoma is the oxidative stress pathway (Levkovitch-Verbin et al., 2000; Ferreira et al., 2004; Ozdemir et al., 2009; Ghanem et al., 2010; Erdurmuş et al., 2011; Majsterek et al., 2011; Bagnis et al., 2012; Himori et al., 2013; Goyal et al., 2014; Lin and Kuang, 2014; d’Azy et al., 2016; Naguib et al., 2021; Sanz-Morello et al., 2021). Oxidative stress, a state of imbalance between pro-oxidant and antioxidant molecules in the eye, can induce the activation of signaling pathways that lead to cellular damage and death. In glaucoma, oxidative stress has been shown to induce the death of RGCs, and antioxidant therapy has been shown to be protective in some animal models of the disease (Ozdemir et al., 2009; Himori et al., 2013; Lin and Kuang, 2014; Chen L. et al., 2015; Hines-Beard et al., 2016; Wenmin et al., 2016). Inflammation is another signaling pathway that is involved in the development of glaucoma (Wei et al., 2019; Wong and Benowitz, 2022). Inflammation in the eye can activate signaling pathways that lead to the death of RGCs and the progression of the disease. Studies have shown that elevated IOP and oxidative stress can activate pro-inflammatory signaling pathways in glaucoma, leading to the release of cytokines and other signaling molecules that contribute to cellular damage and death (Yang et al., 2011; Wilson et al., 2015).
The molecular mechanisms underlying the development and progression of glaucoma are not fully understood, but recent research has shed light on some of the key processes involved. In this review, we will delve deeper into the molecular mechanisms of glaucoma. We will also examine the role of oxidative stress, inflammation, and neurodegeneration in the pathogenesis of the disease, and how they contribute to damage to the RGCs. Furthermore, we will review recent advances in molecular biology and genetics that have shed light on the genetic risk factors and molecular pathways that underlie the development of glaucoma. Understanding the molecular mechanisms of glaucoma is crucial for the development of effective treatments and preventative strategies, and ongoing research is likely to lead to new insights and therapeutic approaches for this debilitating disease (Sulak et al., 2024).
Animal models of glaucoma
Like many other neurological conditions, comprehending the pathophysiology of glaucoma presents challenges in both laboratory settings (in vitro) and living organisms (in vivo). As a result, investigators depend on diverse animal models to examine the disease, with many of these models accurately mimicking crucial facets of glaucoma. Rodents are presently the predominant choice among animals for conducting glaucoma research (Kimura et al., 2020; Pang and Clark, 2020). These animals are appealing for several reasons, including their suitability for experimental manipulation (including genetic manipulation), short lifespan, cost-effectiveness in terms of maintenance, and a relatively similar eye structure and physiology to humans. There are many ways to induce glaucoma in animal models. There are primary models in which glaucoma has been induced by genetic manipulation (Harada et al., 2019), both IOP-dependent and IOP-independent, and secondary models, involving the manual induction of RGCs death, both by increased IOP or non-IOP related mechanisms (Figure 1).
The DBA/2 J mouse strain stands out as the most commonly used and thoroughly studied primary genetic model. In this strain, mutations in the Tyrp1 and Gpnmb genes, which encode tyrosinase-related protein 1 and transmembrane glycoprotein Nmb, play a crucial role (Anderson et al., 2002). These mutations are thought to initiate the demise of iris cells by inducing toxicity related to melanogenesis. This leads to blocked outflow of aqueous fluid, resulting in increased IOP and canonical glaucoma symptoms (Libby et al., 2005).
The IOP independent primary genetic glaucoma model reassembles normal tension glaucoma (NTG) and involves genetic mutation within Tbk1 and Glast genes (Harada et al., 2007, 2010; Fingert et al., 2017). Mutations in the Tbk1 gene have been linked to NTG, a form of glaucoma that manifests with low or average IOP. Multiplications of the Tbk1 gene have been identified in approximately 1% of NTG cases, affecting individuals from various ethnic backgrounds. The precise mechanism through which mutations in Tbk1 gene contribute to NTG remains unclear. Nevertheless, this gene encodes proteins that play roles in the NF-κB signaling pathway and autophagy. Fingert et al. (2017) generated a transgenic mouse strain (referred to as Tg-TBK1) by integrating a single wild-type copy of the human TBK1 gene, along with its native promoter, into the mouse genome. Their findings demonstrated that these mice experience progressive retinal ganglion cell (RGC) loss despite maintaining normal IOP values. Within the mammalian retina, the glutamate/aspartate transporter (GLAST) plays a crucial role as a primary glutamate transporter. Depletion of GLAST is associated with optic nerve degeneration, resembling the characteristics of NTG, which can be explained by impaired oxidative balance due to low content of glutathione and subsequent excitotoxic insult (Harada et al., 2007, 2010).
Secondary glaucoma models with manual induction of increased IOP can be based among others on laser photocoagulation of the aqueous outflow tract, different microbeads obstruction of trabecular meshwork, as well as injection of hypertonic saline into the episcleral veins. These techniques result in a moderate increase in IOP, resulting in progressive RGCs loss and optic nerve degeneration (Morrison et al., 1997; Grozdanic et al., 2003; Moreno et al., 2005; Smedowski et al., 2014; Kimura et al., 2020; Pang and Clark, 2020). These techniques have several advantages and disadvantages, for example, the laser technique requires expensive, specialized equipment, while modulation of laser intensity and duration offers the possibility of great control over the induction of IOP. Saline injection requires less equipment, but it is a surgical technique that requires a great deal of precision and training, due to the small size of rodent ocular vessels. Microbeads injection is relatively simple and reproducible method; however, it may obscure the visual axis of the eye, making functional measurements impossible.
The secondary, inducible IOP-independent glaucoma models represent intraocular injection of excitotoxic agents into the vitreous body, which induce excessive activation of ionotropic glutamate receptors, resulting in an increase in intracellular calcium levels and ultimately to cell damage and death (Pang and Clark, 2020). Cells such as horizontal cells, bipolar cells, ganglion cells, and amacrine cells possess three types of ligand-gated ion channels: N-methyl-D-aspartate (NMDA) receptors, α-amino-3-hydroxy-5-methyl-4-isoxazolopropionic acid (AMPA) receptors, and kainate receptors. The targeted neurodegeneration of cells in the inner layers of the retina, including RGCs, is induced by the intraocular administration of ligands for these receptors (Sun et al., 2001; Casson and Franzco, 2006; Christensen et al., 2019).
Currently, most studies utilizing animal glaucoma models rely on quantification of RGCs to track the progression of neurodegeneration. There are many methods to visualize RGCs in wild-type and genetically engineered retinas, such as immunofluorescence staining with RGCs-specific antibodies (Brn3a, RBPMS, Tuj1, Thy1.1, Islet1; Nadal-Nicolás et al., 2009; Smedowski et al., 2014, 2016, 2018a; Pietrucha-Dutczak et al., 2017; Pacwa et al., 2023) or the use of retrograde tracers, such as FluoroGold (Smedowski et al., 2018b; Hu et al., 2021). Most of the animal models currently used show a rapid rate of degeneration of RGCs, which is atypical for most of human chronic glaucoma cases, but, up to now, these models are the most reliable tools for studying the mechanisms of RGCs degeneration and finding new drug targets.
Molecular mechanisms of glaucoma development
TGF-β signaling
Transforming growth factor beta (TGF-β) is a protein belonging to a larger family called the transforming growth factor beta superfamily, which includes bone morphogenetic proteins (BMPs), inhibins, activins, differentiation growth factors and other signaling molecules (Shi and Massagué, 2003; Massagué, 2012). TGF-β is a cytokine involved in signaling cascades related to cell differentiation, cell division, chemotaxis, and fibrosis. TGF-β has three isoforms: TGF-β1, TGF-β2, TGF-β3, of which TGF-β2 is most associated with eye function (De Caestecker, 2004). The cellular effect of TGF-β signaling depends on the type of protein isoform and its tissue localization. One of the effects of TGF-β is increased extracellular matrix production and remodeling.
The canonical TGF-β signaling pathway begins with its binding to the type II receptor, which leads to phosphorylation and activation of the type I receptor. This process activates intracellular SMADs proteins (Smad2, Smad3, Smad4), which form an oligomeric complex with co-SMAD (Feng and Derynck, 2005; Massagué et al., 2005). The Smad complex moves to the cell nucleus, where it activates gene transcription, leading to the production of extracellular matrix. In the healthy eye, TGF-β2 is involved in corneal healing and scar formation (De Caestecker, 2004). Studies in mice have shown that overexpression of TGF-β2 induces an IOP elevation in wild-type mice, while this effect was not observed in Smad3 knockout mice – thus inferring the importance of Smad proteins in the molecular mechanisms of glaucoma development (McDowell et al., 2013; Figure 2).
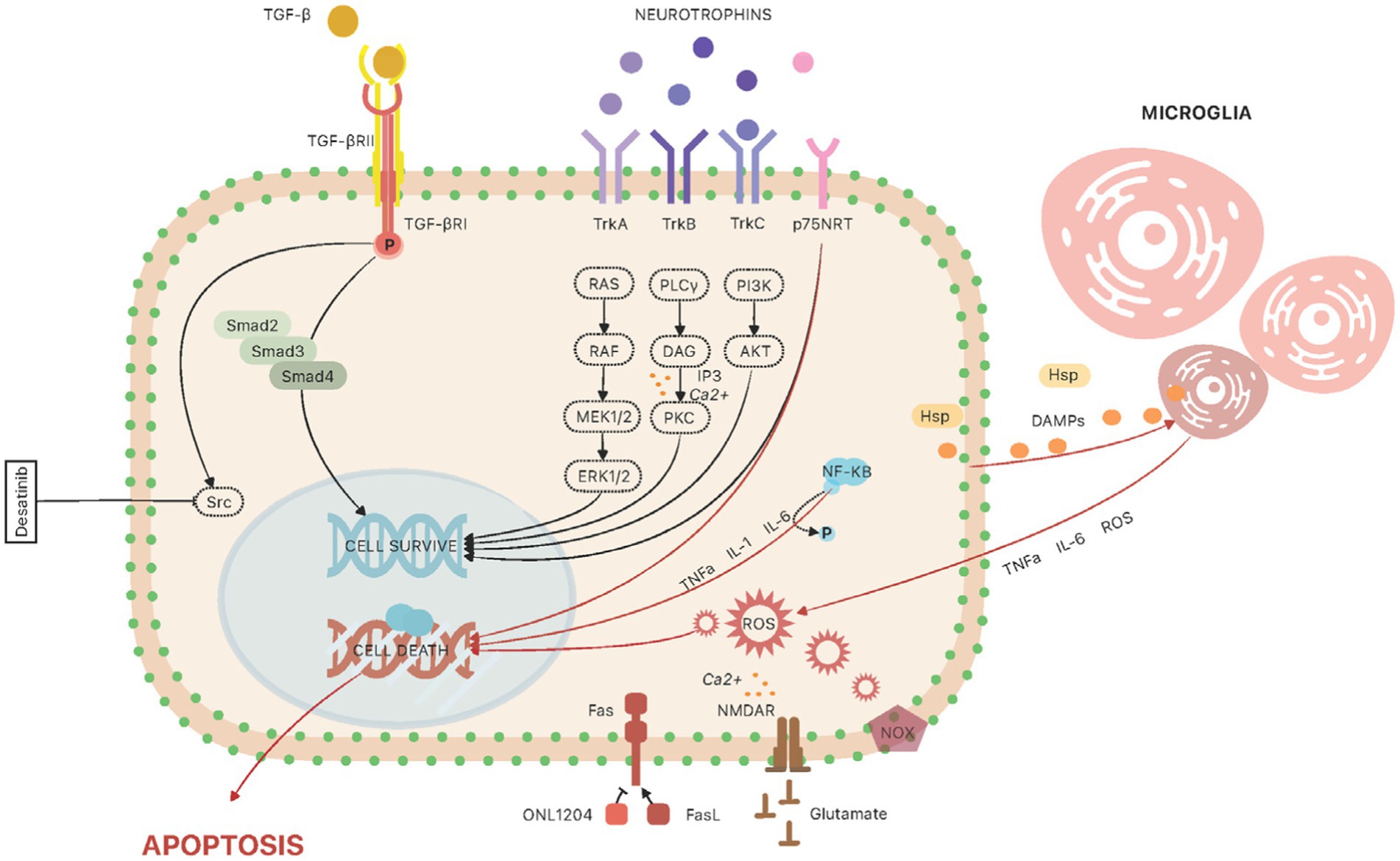
Figure 2. The molecular pathways involved in animal glaucoma models at the level of retinal ganglion cell. TGF-β signaling: The canonical TGF-β signaling pathway begins with its binding to the type II receptor (TGF-βRII), which leads to phosphorylation and activation of the type I receptor (TGF-βRI). This process activates intracellular SMADs proteins (Smad2, Smad3, Smad4). The Smad complex moves to the cell nucleus, where it activates gene transcription, leading to the production of extracellular matrix. In trabecular meshwork cells, studies have shown that another important regulator, involved in TGF-β2-induced IOP increase, is Src kinase; the use of a potent Src inhibitor, Desatinib, resulted in alleviation of TGF-β2-induced IOP increase in rat eyes. Neurotrophins: The neurotrophic pathways provide support and protection to RGCs, and the loss of this support can contribute to the death of RGCs in glaucoma. The action of neurotrophins is mainly manifested through activation of two types of transmembrane glycoproteins, tropomyosin receptor kinases (TrkA, TrkB, and TrkC) and the low affinity neurotrophin receptor p75NTR. Trk activation triggers intricate signaling cascades, activating the Ras/ERK (extracellular signal-regulated kinase) pathway and stimulating mitogen-activated protein kinases (MAPs). Simultaneously, the PI-3 kinase/Akt pathway engages, and phospholipase C (PLC)-γ1 is activated (Mallone et al., 2020). The activation of p75NTR arises from the binding of immature precursor forms of neurotrophins (proNT), eliciting a dual effect on cell fate—either apoptosis or survival. This outcome is contingent upon the simultaneous expression of Trk receptors. Consequently, maintaining cellular homeostasis hinges on achieving equilibrium in the availability of proNTs and their mature counterparts, alongside the presence of both Trk and p75NTR receptors. Neuroinflammation: Microglia and astroglia are the cells involved in inflammatory mechanisms in the retina. Their function is to regulate ion concentration, metabolic support of neurons and neuroprotective activity. Microglia cells, once mature, can be activated by damage-associated molecular pathways (DAMPs), which are released by neuronal cells. In addition, increased IOP stimulates RGCs to produce and secrete heat shock proteins (HSPs). These factors stimulate microglial cells to secrete cytokines and chemokines, such as tumor necrosis factor-alpha (TNF-α), interleukin-6 (IL-6) and complement factors, which promote the morphological transformation of microglia into M1 (proinflammatory) and M2 (anti-inflammatory) macrophages. Inflammatory pathways are involved in the regulation of various genes that are upregulated in the retina. One of the regulatory factors is nuclear factor-kappa B (NF-κB)—it leads to upregulation of IL-1 cytokine family, IL-6 and TNF-α. TNF-α and another proapoptotic protein, FasL, have been implicated in the pathogenesis of glaucoma. Fas/FasL signaling: Fas ligand (FasL) is a protein that belongs to the TNF family. When bound to the Fas receptor, it causes induction of apoptotic and pro-inflammatory pathways. This pathway has been identified as involved in axonal degeneration, death of RGCs and induction of neuroinflammation in a chronic and induced mouse model of glaucoma. Another study used the small peptide molecule ONL1204, an antagonist of the Fas receptor, which was administered to mice by injection into the vitreous body. NADPH oxidase: Several recent studies have pointed to a significant role for NADPH oxidase (NOX) in oxidative stress during glaucoma. NOX has been identified as a major source of oxidative stress in many retinal eye diseases, such as age-related macular degeneration and ischemic retinopathy. Reactive oxygen species produced by NOX affect the regulation of various cellular processes such as proliferation, differentiation, and migration. Excitotoxicity: Glutamate plays an important function in regulating neurophysiological processes. However, increased stimulation of relevant receptors with glutamate leads to neuronal damage and death.
Studies have shown that another important regulator, involved in TGF-β2-induced IOP increase, is Src kinase. The application of a potent Src inhibitor, Dasatinib, led to the mitigation of TGF-β2-induced IOP elevation in rat eyes (Tsukamoto et al., 2019). Moreover, investigations conducted on human trabecular meshwork cells demonstrated that TGF-β2 activates Src signaling, promoting enhanced cell adhesion, cytoskeletal remodeling, and the accumulation of extracellular matrix (ECM). Furthermore, the use of a Src inhibitor attenuated TGF-β2-induced changes. Src activity plays an important role in the TGF-β-induced elevation of intraocular pressure (IOP). Identifying Src signaling as a potential therapeutic target in glaucoma suggests promising avenues for intervention.
Neurotrophins
Neurotrophins (NTs) are central regulators, exerting a critical influence on diverse signaling pathways essential for both neuronal survival and differentiation. Additionally, they play a significant role in promoting synaptic plasticity and facilitating axon regeneration (Rudzinski et al., 2004; Skaper, 2008; Mallone et al., 2020). The neurotrophic pathways are also involved in the development of glaucoma (Rudzinski et al., 2004; Johnson et al., 2011; Weissmiller and Wu, 2012). The neurotrophic pathways provide support and protection to RGCs, and the loss of this support can contribute to the death of RGCs in glaucoma (Guo et al., 2014; Nuschke et al., 2015; Oddone et al., 2017; Dias et al., 2022; Figure 2). Recent literature highlights that heightened intraocular pressure (IOP) and oxidative stress are implicated in the reduction of available neurotrophic factors, ultimately contributing to the demise of retinal ganglion cells (RGCs) in glaucoma. The neurotrophin family comprises pivotal members such as nerve growth factor (NGF), brain-derived neurotrophic factor (BDNF), neurotrophin 3 (NT3), and neurotrophin 4/5 (NT4/5). Neurotrophin activities primarily hinge on the activation of two distinct transmembrane glycoproteins: tropomyosin receptor kinases (TrkA, TrkB, and TrkC) and the low-affinity neurotrophin receptor p75NTR. Trk activation triggers intricate signaling cascades, activating the Ras/ERK (extracellular signal-regulated kinase) pathway and stimulating mitogen-activated protein kinases (MAPs). Simultaneously, the PI-3 kinase/Akt pathway engages, and phospholipase C (PLC)-γ1 is activated. The activation of p75NTR arises from the binding of immature precursor forms of neurotrophins (proNT), eliciting a dual effect on cell fate-either apoptosis or survival. This outcome is contingent upon the simultaneous expression of Trk receptors. Consequently, maintaining cellular homeostasis hinges on achieving equilibrium in the availability of proNTs and their mature counterparts, alongside the presence of both Trk and p75NTR receptors (Levi-Montalcini et al., 1996; Frade and Barde, 1998; Skaper, 2008; Al-Qudah and Al-Dwairi, 2016; Platholi and Lee, 2018).
Nerve growth factor (NGF), the inaugural and extensively studied member of the neurotrophins (NTs) family, plays a pivotal role in neuronal differentiation and survival. Numerous studies highlight the influence of NGF on these processes, particularly within the optic nerve and visual pathway. Expression of NGF is observed in key ocular structures such as the retinal pigment epithelium, Müller cells, and retinal ganglion cells. Correspondingly, NGF receptors are expressed in these structures as well as in photoreceptors (Chakrabarti et al., 1990). Experimental investigations underscore the significance of NGF in promoting the survival and development of RGCs through its role as a local paracrine and autocrine mediator (Colafrancesco et al., 2011; Allen et al., 2013; Garcia et al., 2014; Roberti et al., 2014; Wang et al., 2014; Aloe et al., 2015; Chen Q. et al., 2015; Mallone et al., 2020; Li et al., 2022; Lin et al., 2022). NGF is delivered retrogradely along the axons of RGCs (Dias et al., 2022). Sposato et al. (2009) treated rats having elevated IOP with topical application of NGF. The results showed that increased IOP alters the basal content of NGF and NGF receptors in visual centers in brain and that NGF topical application normalized these deficits. These findings suggest that degeneration in retinal areas may contribute to a reduction in NGF levels. Following above, the clinical trial of topical application of NGF in glaucoma has been initiated (Beykin et al., 2022).
Another of NTs is brain-derived neurotrophic factor (BDNF), which, due to its potential neuroprotective effects, is the subject of much research in developing therapies for diseases such as Parkinson’s disease, amyotrophic lateral sclerosis, Alzheimer’s disease, and glaucoma (Arancibia et al., 2008; Greenberg et al., 2009; Allen et al., 2013; Domenici et al., 2014; Kimura et al., 2016). Synthesized in the brain, BDNF undergoes retrograde transport to RGCs (Dias et al., 2022). Impaired axonal transport from the brain to RGCs is considered a critical factor contributing to RGC death and optic nerve degeneration in glaucoma. BDNF is also locally produced in the ganglion cell layer (GCL), the inner nuclear layer, and in Müller cells. Notably, retinal BDNF levels were observed to increase in a pressure-induced animal model of glaucoma (Guo et al., 2010). Increased retinal BDNF level was shown to be neuroprotective in optic nerve injury models, including glaucoma (Ritch and Sharma, 2000; Arancibia et al., 2008; Domenici et al., 2014; Sanna et al., 2017; Wójcik-Gryciuk et al., 2020). BDNF plays a crucial role in maintaining the integrity of the inner retina under normal conditions, and its deficiency has been linked to retinal and optic nerve damage during progressive glaucoma (Gupta et al., 2014). The functional activity of BDNF is manifested through its interaction with the TrkB receptor, leading to the activation of intracellular signaling pathways associated with cell viability, including the phosphatidylinositol-3 kinase (PI-3 K)/Akt and extracellular signal-regulated kinases 1/2 (Erk1/2) pathways. Both of these signaling pathways have been implicated in experimental glaucoma (Cheng et al., 2002; Carlotta et al., 2016; Liang et al., 2019). There are several studies using different models of glaucoma that indicate that local administration of BDNF to the eye results in reduced loss of RGCs. Moreover, there is speculation that combining BDNF administration to the eyeball with administration to visual centers in the brain could enhance the survival of RGCs in animals with induced optic nerve damage (Weber et al., 2010; Weber and Harman, 2013).
Neuroinflammation
Several studies suggest that glaucoma development has analogies with neurodegenerative diseases connected with inflammatory responses (Yang et al., 2011; Olmos and Lladó, 2014; Wilson et al., 2015; Parsadaniantz et al., 2020; Rolle et al., 2021; Wong and Benowitz, 2022). In this chapter, we will discuss the possible molecular mechanisms of neuroinflammation development (Figure 2).
Within the retina, microglia and astroglia emerge as pivotal players in the orchestration of inflammatory processes (García-Bermúdez et al., 2021; Quan et al., 2022). Their function is to regulate ion concentration, metabolic support of neurons and neuroprotective activity. Microglia cells, once mature, can be activated by damage-associated molecular pathways (DAMPs), which are released by neuronal cells (Gülke et al., 2018). In addition, increased IOP stimulates RGCs to produce and secrete heat shock proteins (HSPs; Piri et al., 2016). These factors stimulate microglial cells to secrete cytokines and chemokines, such as tumor necrosis factor-alpha (TNF-α), interleukin-6 (IL-6) and complement factors, which promote the morphological transformation of microglia into M1 (proinflammatory) and M2 (anti-inflammatory) macrophages (Li and Barres, 2018). Microglial cells communicate with astroglia by secreting signaling molecules that can lead to the differentiation of astroglia cells into two phenotypes: harmful A1 and neuroprotective A2 (Li et al., 2020). Both of these cell types, microglia and astroglia, are significantly involved in the formation and regulation of neuroinflammation.
Inflammatory pathways are involved in the regulation of various genes that are upregulated in the retina. One of the regulatory factors is nuclear factor-kappa B (NF-κB) – it leads to upregulation of IL-1 cytokine family, IL-6 and TNF-α. TNF-α and another proapoptotic protein, FasL, have been implicated in the pathogenesis of glaucoma (Gregory et al., 2011). Recent research has focused on identifying new therapeutic targets that can reduce or completely abolish the deleterious effects of neuroinflammation.
Astroglial NF-κB has been identified as one of the possible therapeutic targets for modulating the inflammatory response (Yang et al., 2020). The experimental use of mice with induced glaucoma and with or without deletion of astroglial IκKβ, which is the main activating kinase involved in IκB degradation through the canonical pathway of NF-κB activation, showed reduced production of inflammatory cytokines in brain ganglia lacking IκKβ, including TNF-α, which can induce apoptosis of RGCs and axonal degeneration in glaucoma.
Another target of research is Fas/FasL signaling pathway. Fas ligand (FasL) is a protein that belongs to the TNF family. When bound to the Fas receptor, it causes induction of apoptotic and pro-inflammatory pathways (Ju et al., 1995; Gregory et al., 2011). This pathway has been identified as involved in axonal degeneration, death of RGCs and induction of neuroinflammation in a chronic and induced mouse model of glaucoma (Krishnan et al., 2016, 2019) Another study used the small peptide molecule ONL1204, an antagonist of the Fas receptor, which was administered to mice by injection into the vitreous body (Krishnan et al., 2019). The results showed reduced death ratio of RGCs in high-tension glaucoma model. Reduced expression of genes involved in the pathogenesis of glaucoma was also observed, including chemokines and cytokines (GFAP, Caspase-8, TNFα, IL-1β, IL-6, IL-18, MIP-1α, MIP-1β, MIP-2, MCPI, and IP10), components of the complement cascade (C3, C1Q), Toll-like receptor pathway (TLR4).
Recent studies in animal models suggest that monocyte-like cells enter the optic nerve head in an ocular hypertensive mouse model of glaucoma (DBA/2 J) and produce damaging molecules that lead to neurodegeneration (Howell et al., 2012; Wilson et al., 2015; Williams et al., 2017). However, the mechanisms of this action are not fully understood. To study these interactions, monocyte infiltration inhibitors were used: DS-SILY, a peptidoglycan that provides a barrier to platelet adhesion to the vessel endothelium and to monocytes, and genetic targeting of Itgam (CD11b, an immune cell receptor that blocks extravasation of immune cells; Williams et al., 2019). The results identified new monocyte-related inflammatory pathways and blocking these pathways reduced monocyte infiltration and provided neuroprotection in DBA/2 J mice.
The anti-inflammatory effects of Ibudilast, a cAMP phosphodiesterase inhibitor, were also investigated in a rat model of ocular hypertension (Vargas et al., 2016). Intraocular administration of Ibudilast reduced macroglia and microglia reactivity in the retina and optic nerve, thereby decreasing the amount of pro-inflammatory cytokines secreted. The study also showed that elevated IOP increased A-type PDE4 activity in Müller cells, and Ibudilast administration resulted in an increase in cellular cAMP. The combined injection of Ibudilast and Rp-cAMP (a non-hydrolysable analog of cAMP that inhibits protein kinase A) resulted in complete blockade of Ibudilast-induced neuroprotection. These results indicate that Ibudilast may be a well-tolerated and safe modulator of neuroinflammation in glaucoma, and that PDE4 represents a potential target for research into inhibiting chronic gliosis and neuroinflammation in glaucoma (Rolle et al., 2021).
Another pathway involved in neuroinflammation represents Toll-like receptors (TLR) signaling, particularly TLR2 and TLR4 present in microglia. Studies have shown that these pathways are involved in the transmission of signals induced by heat shock proteins and are associated with oxidative stress (Luo et al., 2010). It has also been observed that Tenascin C, which is one of the ligands for TLR4, undergoes increased expression both in human glaucoma patients and in animal models (Johnson et al., 2007; Wallace et al., 2015). TLRs are therefore an interesting target for research on inhibition and reduction of neuroinflammation. There are studies intensively using human umbilical cord mesenchymal stem cells (hUC-MSCs) to show their immunomodulatory properties (Cislo-Pakuluk and Marycz, 2017). These cells show the ability to regulate the function of microglia and astrocytes (Jose et al., 2014; Wang et al., 2018). They have also been used in studies to inhibit the activity of TLRs, with a positive effect in reduced neuroinflammation in a rat model of glaucoma (Lund et al., 2009; Zwart et al., 2009; Ji et al., 2018).
The NLRP3 inflammasome, a complex of multiple proteins, plays a crucial role in initiating inflammatory responses. Comprising NLRP3, ASC (apoptosis-associated speck-like protein containing a caspase recruitment domain), and pro-caspase-1, this inflammasome is activated by various triggers. Upon activation, pro-caspase-1 undergoes cleavage into its active form, initiating the maturation and release of pro-inflammatory cytokines, including interleukin-1β (IL-1β) and interleukin-18 (IL-18; Chi et al., 2014).
Recent research has compellingly demonstrated the activation of the NLRP3 pathway in different types of glaucoma. Elevated levels of NLRP3 inflammasome components have been identified in the trabecular meshwork, retina, and optic nerve head of eyes affected by glaucoma (Ward et al., 2019). This upregulation of NLRP3 is closely associated with neuroinflammation and the death of retinal ganglion cells (RGCs), contributing to the progressive nature of glaucomatous optic neuropathy. A noteworthy connection has been suggested between the activation of the NLRP3 inflammasome and increased intraocular pressure (IOP). Mechanical stress, a common factor in glaucoma, may serve as a trigger for NLRP3 inflammasome activation, resulting in the release of pro-inflammatory cytokines. This cascade of events is implicated in the perpetuation of neuroinflammation and the subsequent damage to the optic nerve. The potential therapeutic implications of targeting the NLRP3 pathway in glaucoma are promising. Inhibiting NLRP3 activation, whether through pharmacological agents or gene therapies, has demonstrated neuroprotective effects in experimental models (Coll et al., 2015; Gong et al., 2019; Zhang et al., 2019; Coyle et al., 2021). However, further research is necessary to thoroughly explore the safety and efficacy of these interventions, paving the way for potential translation into clinical applications.
Chronic oxidative stress
Oxidative stress is an imbalance between free radicals and antioxidant scavengers that can result in tissue damage (Pickering et al., 2013). Reactive oxygen species (ROS) are free radicals that are the main source of oxidative stress formation. These include superoxide anion (O2−), lipid radical, hydroxyl radical and nitric oxide (NO). Uncontrolled production of ROS leads to oxidation of biological molecules such as proteins, lipids and DNA contained in the cell, which triggers activation of mechanisms leading to cell death by apoptosis or necrosis (Garza-Lombó et al., 2020). There are several mechanisms leading to ROS production, and the most important include uncoupled NO synthase, mitochondrial dysfunction, xanthine oxidase and NADPH oxidase (Dalle-Donne et al., 2006). Mechanisms related to oxidative stress are considered an important factor in the development of neurodegenerative diseases, including glaucoma (Levkovitch-Verbin et al., 2000; Himori et al., 2013; Lin and Kuang, 2014; Naguib et al., 2021; Sanz-Morello et al., 2021).
Autophagy is a lysosomal degradation of the cellular constituents. Cellular homeostasis is maintained by removing damaged organelles, such as mitochondria, in the autophagic process of mitophagy (Terman et al., 2006). However, intralysosomal accumulation of waste material increases with age. Oxidation of this material leads to the formation of a non-degradable substance called lipofuscin (Grune et al., 2005). Lipofuscin has been shown to accumulate in glaucomatous human lamina cribrosa cells. This accumulation may in turn alter cellular authophagic activity. In addition, autophagy markers have been found in lamina cribrosa cells (McElnea et al., 2011). Furthermore, studies using DBA/2 J mice showed that in myelinated optic nerve axons the number of mitochondria was increased, and they had significantly smaller surface area. These results indicate that mitochondrial pathology may be the cause of the energy deficit in glaucomatous optic nerve. An increased number of autophagosomes was observed, which, together with increased number of mitochondria and decreased Lamp1 (lysosomal associated membrane protein 1) gene, suggests that deteriorating mitochondria do not recycle efficiently enough in the mitophagy process (Coughlin et al., 2015). These findings suggest that mitochondrial dysfunction and the resulting oxidative stress may be an important factor in the pathogenesis of glaucoma.
In addition to the well-known correlation between mitochondrial dysfunction and oxidative stress, several recent studies have pointed to a significant role for NADPH oxidase (NOX) in oxidative stress during glaucoma. NOX has been identified as a major source of oxidative stress in many retinal eye diseases, such as age-related macular degeneration and ischemic retinopathy (Ruan et al., 2020). Reactive oxygen species produced by NOX affect the regulation of various cellular processes such as proliferation, differentiation, and migration (Chan et al., 2009). There are seven NOX isoforms, three of which are the most studied in the eye pathology: NOX1, NOX2 and NOX4. NOX1 and NOX2 produce superoxide, while NOX4 mainly produce hydrogen superoxide (Griendling et al., 2000). The enzymatic activity of NOX can be induced by various stimuli: hypoxia (Dvoriantchikova et al., 2012), proinflammatory cytokines, i.e., TNF-α (Geng et al., 2020) or heat shock proteins (Sakai et al., 2003). All of these stimuli are associated with glaucoma pathology. There are many studies using animal glaucoma models that show the relationship between NOX activity and pathological features (Yokota et al., 2011; Dvoriantchikova et al., 2012). Recently, many studies have focused on using pharmacological inhibition to block the contribution of NADPH oxidase in an animal model of retinal pathological conditions, such as ischemic retinopathy and retinal inflammation. However, there are very limited studies on the pharmacological inhibition of NOX in animal glaucoma models. In the ischemia reperfusion (I/R) injury model, the role of NOX2 in neuronal cell death was also examined (Yokota et al., 2011). The results show that I/R induces the retinal cell death, indicated by numerous TUNEL-positive cells in the inner retina and significant decrease in neuronal cell density. This effect was abolished in mice with Nox2 gene deletion, indicating a role for NOX2 NADPH oxidase in I/R-induces injury of the inner retina. Furthermore, Apocynin, a non-selective inhibitor of NADPH oxidase, was used in the study. Apocynin improved retinal morphology in NOX2−/− with I/R injury, indicating the need for studies using other selective NOX inhibitors in glaucoma models.
Excitotoxicity
Glutamate plays an important function in regulating neurophysiological processes. However, increased stimulation of relevant receptors with glutamate leads to neuronal damage and death. A similar mechanism takes place in the eye. Glutamate is secreted by ganglion cells, bipolar cells, and photoreceptors in response to light stimuli. Its homeostasis is ensured by the activity of microglia and macroglia cells. When glutamate homeostasis is disrupted, its uncontrolled increased concentration leads to excitotoxicity (Harada et al., 2007). Glutamate binds to the N-methyl-D-aspartic acid receptor (NMDAR), affecting the disruption of K+/Na+ ion homeostasis—there is an increased influx of Na+ ions into cells, resulting in the release of calcium ions, activation of the ERK 1/2 signaling pathway, formation of reactive oxygen species (ROS), oxidative stress and mitochondrial dysfunction (Arundine and Tymianski, 2003). All these factors lead to apoptotic death of retinal cells. Intravitreal injection of glutamate in rats resulted in loss of RGCs, which is considered a major cause of vision loss in glaucoma (Lam et al., 1999).
There are attempts to interfere with the mechanisms of excitotoxicity in ischemic models. The first idea was the use NMDA receptor antagonists in a rat model of retinal ischemia, but its complete blockade affects other physiological processes of nerve cells (Gómez-Vicente et al., 2015). Another approach was the use of brimonidine, a selective α2-adrenergic agonist that shows the ability to lower IOP and prevent the excitotoxicity in RGCs. Its proven mechanism of action is based on lowering glutamate secretion, regulating Ca2+ ion homeostasis, reducing NMDA receptor expression, or decreasing production of aqueous humor by the ciliary body (Lee et al., 2012; Villanueva et al., 2020). In vivo studies showed that intrathecal injection of brimonidine prevented the death of RCGs in a rabbit model with NMDA-induced excitotoxicity and in rat model of high-tension glaucoma (Dong et al., 2008; Ozdemir et al., 2009).
Other studies use glutamate transporters found in retinal cells as targets. One of these, called GLAST, found mainly in Müller cells, removes most glutamate molecules from the extracellular space (Vorwerk et al., 1999). Studies using GLAST knock-out mice have shown that about 50% of glutamate is captured by GLAST (Shi et al., 1997). Other studies indicate that increased IOP in animal models results in decreased GLAST expression (Schuettauf et al., 2007) and knocking out Glast gene leads to spontaneous degeneration of RGCs in normal tension conditions (Harada et al., 2010). Increasing glutamate transport is therefore an interesting target for studies on reducing pressure-induced neurodegeneration. There are studies in which the estrogen modulator, Tamoxifen, was used and showed neuroprotective properties due to up-regulation of GLAST, which reduced excitotoxicity (Lee et al., 2009). In a rat model of glaucoma, eye drops containing 17β-Estradiol were also used, which had the effect of reducing neurodegeneration (Prokai-Tatrai et al., 2013). Estradiol and its analogs are therefore interesting targets capable of increasing GLAST expression, which could be exploited in glaucoma patients.
ABCA1
Recent research has linked ATP binding cassette transporter A1 (ABCA1) to POAG. ABCA1 is a member of a superfamily of ABC transmembrane transporters responsible for removing cholesterol by transferring it to lipid-free apolipoprotein A-I and apolipoprotein E (Danik et al., 1999). Its transcription is regulated by liver X receptor (LXR), a member of the nuclear receptors superfamily (Yang et al., 2011). ABCA1 is prominently expressed in various ocular tissues including the trabecular meshwork, Schlemm’s canal, endothelial cells, optic nerve, and retina, particularly in retinal ganglion cells (Cai et al., 2015). Recent studies suggest ABCA1’s potential role in regulating intraocular pressure (IOP), although the precise mechanism remains unclear. Hu et al. propose a connection between ABCA1-mediated IOP regulation and the Cav1/eNOS/NO signaling pathway, which is implicated in glaucoma pathogenesis in POAG patients. ABCA1 interacts with caveolin-1 (Cav1), a key player in cellular processes like vesicular transport, cholesterol regulation, and mechanosignal transmission (Kuo et al., 2011; Gu et al., 2017). Cav1 regulates vasodilation by modulating endothelial nitric oxide synthase (eNOS), known as a pressure-dependent regulator of IOP through the conventional outflow tract pathway (Daniel Stamer et al., 2011). ABCA1 expression levels were examined in samples of trabecular meshwork from POAG patients and human donor eyes. ABCA1 expression levels were analyzed in samples of trabecular meshwork from POAG patients and human donor eyes, showing higher expression in POAG patients compared to controls. Studies on cells and animal models suggest that ABCA1 can modulate Cav1 expression, alleviating Cav1’s inhibitory effect on eNOS, thus increasing eNOS-derived production and reducing IOP (Hu et al., 2020). Based on these findings, enhancing the ABCA1 signaling pathway is proposed as a potential therapeutic approach for glaucoma and ocular hypertension treatment.
Rho-kinase
The Rho kinase (ROCK) activation pathway is a critical signaling pathway involved in various cellular processes such as cell migration, proliferation, adhesion, and smooth muscle contraction (Thumkeo et al., 2013). Rho-associated protein kinases (ROCK) are a serine/threonine protein kinases, that are downstream effectors of small GTPase Rho. The activation process involves the binding of Rho-GTP to the kinase domain of ROCK, resulting in a conformational change that enhances ROCK activity. Activated ROCK phosphorylates various downstream substrates, including myosin light chain (MLC), myosin phosphatase substrate 1 (MYPT1), kinase C-potentiated phosphatase inhibitor 17 (CP1-17), LIM kinase (LIMK), calmodulin (CaM), ezrin, radixin, and moesin. Through these interactions, the Rho-ROCK signaling pathway orchestrates the dynamics of actin cytoskeleton, contraction mediated by actomyosin, adherence between cells, cellular morphology, and the reorganization of the extracellular matrix (ECM; Amano et al., 2010). Researchers have indicated that ROCK inhibitors have the capability to influence trabecular meshwork, enhancing the drainage of aqueous humor from the eye and subsequently decreasing IOP (Honjo and Tanihara, 2018). Currently, there are several ROCK inhibitors in use as a novel class of drugs that can act directly on trabecular meshwork. Three of them (ripasudil, netarsudil and fasudil) are commercially used in the treatment of glaucoma in some countries. Two of the ROCK inhibitors have not been approved yet (SNJ-1656—phase II clinical trial, Y-27632—pre-clinical phase; Pagano et al., 2023). With the rise in the use of ROCK inhibitors by ophthalmologists, it becomes important to enhance our comprehension of the potential advantages and safety profiles associated with these pharmaceuticals, whether employed in approved or off-label capacities.
Purinergic signaling pathway
Purinergic P2 receptors binds extracellular nucleotides. The superfamily contains two types of receptors. First, G protein-coupled P2Y binds ATP, ADP, UTP, UDP, UDP-sugars and interacts with G proteins inducting intracellular signaling pathways, and ionotropic P2X, which binds ATP and has two or three transmembrane domains and form an ion channel gating Na+, K+, and Ca2+ (Shinozaki et al., 2023). Normally, the level of extracellular ATP is extremely low. ATP levels can rise due to uncontrolled release from necrotic cells or controlled release via exocytosis or channel-mediated mechanisms. Ocular tissues like the lens, retina, corneal endothelial cells, ciliary body, and retinal astrocytes are known to release ATP and nucleotides. There are indications that a sudden increase in intraocular pressure (IOP) prompts ATP release from the retina. Within the dynamics of aqueous humor, an increase in ATP or UTP prompts the activation of P2Y2 receptors, resulting in an escalation of aqueous humor production. On the other hand, when there’s less ADP/UDP, it stops the receptors P2Y1/6 from slowing down or speeding up fluid production or drainage (Shevozaki et al., 2005; Taguchi et al., 2016; Hamada et al., 2021). These changes make the IOP go up, which could harm the RGCs. Disrupted purinergic signaling may damage RGCs through autonomous and non-autonomous pathways. P2X7 receptors, found in RGCs, microglia, astrocytes, and Müller cells, play an important role. RGCs experience excitotoxicity when these receptors are activated. In glial cells, activation leads to neurotoxic responses, including microglia releasing pro-inflammatory cytokines and ROS that harm RGCs. Astrocytes release glutamate upon activation, overstimulating glutamate receptors in RGCs, while Müller cells reduce glutamate uptake, further damaging RGCs. Additionally, P2X7 receptor activation in Müller cells triggers inflammation.
Shevozaki et al. (2005) showed that P2Y6 lowers IOP – ablation of the P2Y6 gene in mice (P2Y6 KO) results in hypertensive glaucoma–like optic neuropathy—exhibited sustained elevation of IOP, age-dependent damage to the optic nerve, thinning of ganglion cell inner layers, and a reduction of RGC numbers. Topically applied uridine diphosphate, an endogenous selective agonist for the P2Y6 receptor, decreases IOP. In that case P2 receptor ligands may be a promising candidate for glaucoma treatment.
Conclusion
Animal glaucoma models are used to study various molecular pathways associated with the development and progression of glaucoma, which is a complex eye disease characterized by progressive vision loss, involves the dysregulation of numerous molecular pathways. These pathways encompass various mechanisms critical to the pathogenesis of the condition. Among them are processes associated with intraocular pressure (IOP) regulation, neuroinflammation, oxidative stress, and neurodegeneration, which contribute to the damage of retinal ganglion cells and their axons. Excitotoxicity, vascular dysregulation, and gliosis further exacerbate neuronal injury, while deficits in axonal transport and protein misfolding/aggregation disrupt cellular function and integrity. Additionally, abnormalities in autophagy and apoptosis pathways contribute to tissue degeneration. However, the exploration of neuroprotective mechanisms and the influence of genetic factors offer hope for understanding and potentially mitigating the progression of glaucoma. By studying these molecular pathways in animal glaucoma models, researchers aim to gain insights into the disease’s mechanisms and identify potential therapeutic targets for the treatment and prevention of glaucoma. By understanding the molecular mechanisms of glaucoma, we can develop new strategies to prevent or slow the progression of this debilitating disease and preserve vision.
Author contributions
KB: Conceptualization, Software, Writing – original draft, Writing – review & editing. AP: Funding acquisition, Software, Writing – original draft, Writing – review & editing. AS: Conceptualization, Funding acquisition, Resources, Supervision, Writing – original draft, Writing – review & editing.
Funding
The author(s) declare that financial support was received for the research, authorship, and/or publication of this article. The study was funded by Medical University of Silesia grant no. PCN-2-073/N/2/0 and the Polish National Science Center Preludium BIS, grant no. 2021/43/O/NZ5/00282.
Conflict of interest
AS and AP were employees of GlaucoTech Co.
The remaining author declares that the research was conducted in the absence of any commercial or financial relationships that could be construed as a potential conflict of interest.
Publisher’s note
All claims expressed in this article are solely those of the authors and do not necessarily represent those of their affiliated organizations, or those of the publisher, the editors and the reviewers. Any product that may be evaluated in this article, or claim that may be made by its manufacturer, is not guaranteed or endorsed by the publisher.
References
Allen, S. J., Watson, J. J., Shoemark, D. K., Barua, N. U., and Patel, N. K. (2013). GDNF, NGF and BDNF as therapeutic options for neurodegeneration. Pharmacol. Ther. 138, 155–175. doi: 10.1016/j.pharmthera.2013.01.004
Aloe, L., Rocco, M., Balzamino, B., and Micera, A. (2015). Nerve growth factor: a focus on neuroscience and therapy. Curr. Neuropharmacol. 13, 294–303. doi: 10.2174/1570159x13666150403231920
Al-Qudah, M. A., and Al-Dwairi, A. (2016). Mechanisms and regulation of Neurotrophin synthesis and secretion. Neurosciences 21:306. doi: 10.17712/NSJ.2016.4.20160080
Amano, M., Nakayama, M., and Kaibuchi, K. (2010). Rho-kinase/ROCK: a key regulator of the cytoskeleton and cell polarity. Cytoskeleton 67, 545–554. doi: 10.1002/cm.20472
Anderson, M. G., Smith, R. S., Hawes, N. L., Zabaleta, A., Chang, B., Wiggs, J. L., et al. (2002). Mutations in genes encoding Melanosomal proteins cause pigmentary Glaucoma in DBA/2J mice. Nat. Genet. 30, 81–85. doi: 10.1038/ng794
Arancibia, S., Silhol, M., Moulière, F., Meffre, J., Höllinger, I., Maurice, T., et al. (2008). Protective effect of BDNF against Beta-amyloid induced neurotoxicity in vitro and in vivo in rats. Neurobiol. Dis. 31, 316–326. doi: 10.1016/j.nbd.2008.05.012
Arundine, M., and Tymianski, M. (2003). Molecular mechanisms of calcium-dependent neurodegeneration in excitotoxicity. Cell Calcium 34, 325–337. doi: 10.1016/S0143-4160(03)00141-6
Bagnis, A., Izzotti, A., Centofanti, M., and Saccà, S. C. (2012). Aqueous humor oxidative stress proteomic levels in primary open angle Glaucoma. Exp. Eye Res. 103, 55–62. doi: 10.1016/j.exer.2012.07.011
Beykin, G., Stell, L., Halim, M. S., Nuñez, M., Popova, L., Nguyen, B. T., et al. (2022). Phase 1b randomized controlled study of short course topical recombinant human nerve growth factor (RhNGF) for Neuroenhancement in Glaucoma: safety, tolerability, and efficacy measure outcomes. Am. J. Ophthalmol. 234, 223–234. doi: 10.1016/J.AJO.2021.11.002
Cai, J., Perkumas, K. M., Qin, X., Hauser, M. A., Daniel Stamer, W., and Liu, Y. (2015). Expression profiling of human Schlemm’s canal endothelial cells from eyes with and without Glaucoma. Invest. Ophthalmol. Vis. Sci. 56:6747. doi: 10.1167/IOVS.15-17720
Carlotta, F., Elisa, C., Sara, O., Marco, S., and Luciano, D. (2016). Changes in BDNF and MAPK signaling pathways in experimental Glaucoma. J Clin Exp Opthalmol 7:30. doi: 10.4172/2155-9570.1000530
Casson, R. J., and Franzco, D. (2006). Perspective possible role of excitotoxicity in the pathogenesis of Glaucoma. Clin. Exp. Ophthalmol. 34, 54–63. doi: 10.1111/j.1442-9071.2006.1146.x
Chakrabarti, S., Sima, A. A. F., Lee, J., Brachet, P., and Dicou, E. (1990). Nerve growth factor (NGF), ProNGF and NGF receptor-like immunoreactivity in BB rat retina. Brain Res. 523, 11–15. doi: 10.1016/0006-8993(90)91630-Y
Chan, E. C., Jiang, F., Peshavariya, H. M., and Dusting, G. J. (2009). Regulation of cell proliferation by NADPH oxidase-mediated signaling: potential roles in tissue repair, regenerative medicine and tissue engineering. Pharmacol. Ther. 122, 97–108. doi: 10.1016/j.pharmthera.2009.02.005
Chauhan, B. C., Mikelberg, F. S., Artes, P. H., Balazsi, A. G., LeBlanc, R. P., Lesk, M. R., et al. (2010). Canadian Glaucoma study: 3. Impact of risk factors and intraocular pressure reduction on the rates of visual field change. Arch. Ophthalmol. 128, 1249–1255. doi: 10.1001/ARCHOPHTHALMOL.2010.196
Chen, L., Qi, Y., and Yang, X. (2015). Neuroprotective effects of Crocin against oxidative stress induced by ischemia/reperfusion injury in rat retina. Ophthalmic Res. 54, 157–168. doi: 10.1159/000439026
Chen, Q., Wang, H., Liao, S., Gao, Y., Liao, R., Little, P. J., et al. (2015). Nerve growth factor protects retinal ganglion cells against injury induced by retinal ischemia-reperfusion in rats. Growth Factors 33, 149–159. doi: 10.3109/08977194.2015.1010642
Cheng, L., Sapieha, P., Kittlerova, P., Hauswirth, W. W., and Di Polo, A. (2002). TrkB gene transfer protects retinal ganglion cells from Axotomy-induced death in vivo. J Neurosci 22, 3977–3986. doi: 10.1523/JNEUROSCI.22-10-03977.2002
Chi, W., Li, F., Chen, H., Wang, Y., Zhu, Y., Yang, X., et al. (2014). Caspase-8 promotes NLRP1/NLRP3 Inflammasome activation and IL-1β production in acute Glaucoma. Proc. Natl. Acad. Sci. U. S. A. 111, 11181–11186. doi: 10.1073/pnas.1402819111
Christensen, I., Lu, B., Yang, N., Huang, K., Wang, P., and Tian, N. (2019). The susceptibility of retinal ganglion cells to glutamatergic excitotoxicity is type-specific. Front. Neurosci. 13:219. doi: 10.3389/fnins.2019.00219
Cislo-Pakuluk, A., and Marycz, K. (2017). A promising tool in retina regeneration: current perspectives and challenges when using mesenchymal progenitor stem cells in veterinary and human ophthalmological applications. Stem Cell Rev. Rep. 13, 598–602. doi: 10.1007/s12015-017-9750-4
Colafrancesco, V., Parisi, V., Sposato, V., Rossi, S., Russo, M. A., Coassin, M., et al. (2011). Ocular application of nerve growth factor protects degenerating retinal ganglion cells in a rat model of Glaucoma. J. Glaucoma 20, 100–108. doi: 10.1097/IJG.0b013e3181d787e5
Coll, R. C., Robertson, A. A. B., Chae, J. J., Higgins, S. C., Muñoz, R., Inserra, M. C., et al. (2015). Potential therapeutic for inflammatory diseases. Nat. Med. 21, 248–255. doi: 10.1038/nm.3806.A
Coughlin, L., Morrison, R. S., Horner, P. J., and Inman, D. M. (2015). Mitochondrial morphology differences and Mitophagy deficit in murine glaucomatous optic nerve. Invest. Ophthalmol. Vis. Sci. 56, 1437–1446. doi: 10.1167/iovs.14-16126
Coyle, S., Khan, M. N., Chemaly, M., Callaghan, B., Doyle, C., Willoughby, C. E., et al. (2021). Targeting the Nlrp3 Inflammasome in Glaucoma. Biomol. Ther. 11, 1–14. doi: 10.3390/biom11081239
d’Azy, B., Cédric, B. P., Chiambaretta, F., and Dutheil, F. (2016). Oxidative and anti-oxidative stress markers in chronic Glaucoma: a systematic review and Meta-analysis. PLoS One 11:e0166915. doi: 10.1371/journal.pone.0166915
Dalle-Donne, I., Rossi, R., Colombo, R., Giustarini, D., and Milzani, A. (2006). Biomarkers of oxidative damage in human disease. Clin. Chem. 52, 601–623. doi: 10.1373/clinchem.2005.061408
Daniel Stamer, W., Lei, Y., Boussommier-Calleja, A., Overby, D. R., and Ross Ethier, C. (2011). ENOS, a pressure-dependent regulator of intraocular pressure. Invest. Ophthalmol. Vis. Sci. 52:9438. doi: 10.1167/IOVS.11-7839
Danik, M., Champagne, D., Petit-Turcotte, C., Beffert, U., and Poirier, J. (1999). Brain lipoprotein metabolism and its relation to neurodegenerative disease. Crit. Rev. Neurobiol. 13, 357–407. doi: 10.1615/CRITREVNEUROBIOL.V13.I4.20
Davis, B. M., Crawley, L., Pahlitzsch, M., Javaid, F., and Cordeiro, M. F. (2016). Glaucoma: the retina and beyond. Acta Neuropathol. 132, 807–826. doi: 10.1007/s00401-016-1609-2
De Caestecker, M. (2004). The transforming growth factor-β superfamily of receptors. Cytokine Growth Factor Rev. 15, 1–11. doi: 10.1016/j.cytogfr.2003.10.004
Dias, M. S., Luo, X., Ribas, V. T., Petrs-Silva, H., and Koch, J. C. (2022). The role of axonal transport in Glaucoma. Int. J. Mol. Sci. 23:935. doi: 10.3390/IJMS23073935
Domenici, L., Origlia, N., Falsini, B., Cerri, E., Barloscio, D., Fabiani, C., et al. (2014). Rescue of Retinal Function by BDNF in a mouse model of Glaucoma. PLoS One 9:e115579. doi: 10.1371/journal.pone.0115579
Dong, C. J., Guo, Y., Agey, P., Wheeler, L., and Hare, W. A. (2008). Α2 adrenergic modulation of NMDA receptor function as a major mechanism of RGC protection in experimental Glaucoma and retinal excitotoxicity. Invest. Ophthalmol. Vis. Sci. 49, 4515–4522. doi: 10.1167/iovs.08-2078
Dvoriantchikova, G., Grant, J., Andrea, R. C., Santos, E. H., and Ivanov, D. (2012). Neuronal NAD(P)H oxidases contribute to ROS production and mediate RGC death after ischemia. Invest. Ophthalmol. Vis. Sci. 53, 2823–2830. doi: 10.1167/iovs.12-9526
Erdurmuş, M., Yağcı, R., Atış, Ö., Karadağ, R., Akbaş, A., and Hepşen, İ. F. (2011). Antioxidant status and oxidative stress in primary open angle Glaucoma and Pseudoexfoliative Glaucoma. Curr. Eye Res. 36, 713–718. doi: 10.3109/02713683.2011.584370
Feng, X. H., and Derynck, R. (2005). Specificity and versatility in TGF-β signaling through Smads. Annu. Rev. Cell Dev. Biol. 21, 659–693. doi: 10.1146/annurev.cellbio.21.022404.142018
Ferreira, S. M., FabiÁn Lerner, S., and Brunzini, R. (2004). Oxidative stress markers in aqueous humor of Glaucoma patients. Am. J. Ophthalmol. 137, 62–69. doi: 10.1016/S0002-9394(03)00788-8,Pablo a. Evelson, and Susana F. Llesuy
Fingert, J. H., Miller, K., Hedberg-Buenz, A., Roos, B. R., Lewis, C. J., Mullins, R. F., et al. (2017). Transgenic TBK1 mice have features of Normal tension Glaucoma. Hum. Mol. Genet. 26, 124–132. doi: 10.1093/HMG/DDW372
Frade, J. M., and Barde, Y. A. (1998). Nerve growth factor: two receptors, multiple functions. Bioessays 20, 137–145. doi: 10.1002/(SICI)1521-1878(199802)20:2<137::AID-BIES6>3.0.CO;2-Q
Garcia, T. B., Pannicke, T., Vogler, S., Berk, B. A., Grosche, A., Wiedemann, P., et al. (2014). Nerve growth factor inhibits osmotic swelling of rat retinal glial (M??Ller) and bipolar cells by inducing glial cytokine release. J. Neurochem. 131, 303–313. doi: 10.1111/jnc.12822
García-Bermúdez, M. Y., Freude, K. K., Mouhammad, Z. A., van Wijngaarden, P., Martin, K. K., and Kolko, M. (2021). Glial cells in Glaucoma: friends, foes, and potential therapeutic targets. Front. Neurol. 12:624983. doi: 10.3389/fneur.2021.624983
Garza-Lombó, C., Pappa, A., Panayiotidis, M. I., and Franco, R. (2020). Redox homeostasis, oxidative stress and Mitophagy. Mitochondrion 51, 105–117. doi: 10.1016/j.mito.2020.01.002
Geng, L., Fan, L. M., Liu, F., Smith, C., and Li, J. M. (2020). Nox2 dependent redox-regulation of microglial response to amyloid-β stimulation and microgliosis in aging. Sci. Rep. 10, 1–11. doi: 10.1038/s41598-020-58422-8
Ghanem, A. A., Arafa, L. F., and El-Baz, A. (2010). Oxidative stress markers in patients with primary open-angle Glaucoma. Curr. Eye Res. 35, 295–301. doi: 10.3109/02713680903548970
Gómez-Vicente, V., Lax, P., Fernández-Sánchez, L., Rondón, N., Esquiva, G., Germain, F., et al. (2015). Neuroprotective effect of Tauroursodeoxycholic acid on N-methyl-Daspartate-induced retinal ganglion cell degeneration. PLoS One 10, 1–14. doi: 10.1371/journal.pone.0137826
Gong, Y., Cao, X., Gong, L., and Li, W. (2019). Sulforaphane alleviates retinal ganglion cell death and inflammation by suppressing Nlrp3 Inflammasome activation in a rat model of retinal ischemia/reperfusion injury. Int. J. Immunopathol. Pharmacol. 33:1777. doi: 10.1177/2058738419861777
Goyal, A., Srivastava, A., Sihota, R., and Kaur, J. (2014). Evaluation of oxidative stress markers in aqueous humor of primary open angle Glaucoma and primary angle closure Glaucoma patients. Curr. Eye Res. 39, 823–829. doi: 10.3109/02713683.2011.556299
Greenberg, M. E., Xu, B., Lu, B., and Hempstead, B. L. (2009). New insights in the biology of BDNF synthesis and release: implications in CNS function. J. Neurosci. 29, 12764–12767. doi: 10.1523/JNEUROSCI.3566-09.2009
Gregory, M. S., Hackett, C. G., Abernathy, E. F., Lee, K. S., Saff, R. R., Hohlbaum, A. M., et al. (2011). Opposing roles for membrane bound and soluble Fas ligand in Glaucoma-associated retinal ganglion cell death. PLoS One 6:17659. doi: 10.1371/JOURNAL.PONE.0017659
Griendling, K. K., Sorescu, D., and Ushio-Fukai, M. (2000). NAD(P)H oxidase: role in cardiovascular biology and disease. Circ. Res. 86, 494–501. doi: 10.1161/01.RES.86.5.494
Grozdanic, S. D., Betts, D. M., Sakaguchi, D. S., Allbaugh, R. A., Kwon, Y. H., and Kardon, R. H. (2003). Laser-induced mouse model of chronic ocular hypertension. Invest. Ophthalmol. Vis. Sci. 44, 4337–4346. doi: 10.1167/iovs.03-0015
Grune, T., Merker, K., Jung, T., Sitte, N., and Davies, K. J. A. (2005). Protein oxidation and degradation during Postmitotic senescence. Free Radic. Biol. Med. 39, 1208–1215. doi: 10.1016/j.freeradbiomed.2005.06.009
Gu, X., Reagan, A. M., McClellan, M. E., and Elliott, M. H. (2017). Caveolins and Caveolae in ocular physiology and pathophysiology. Prog. Retin. Eye Res. 56:84. doi: 10.1016/J.PRETEYERES.2016.09.005
Gülke, E., Gelderblom, M., and Magnus, T. (2018). Danger signals in stroke and their role on microglia activation after ischemia. Ther. Adv. Neurol. Disord. 11, 1–14. doi: 10.1177/1756286418774254
Guo, Y., Johnson, E. C., Cepurna, W. O., Dyck, J. A., Doser, T., and Morrison, J. C. (2010). Early gene expression changes in the retinal ganglion cell layer of a rat Glaucoma model. Invest. Ophthalmol. Vis. Sci. 52, 1460–1473. doi: 10.1167/iovs.10-5930
Guo, X. J., Tian, X. S., Zhi Ruan, Y., Chen, T., Lei, W., Gong, Q., et al. (2014). Dysregulation of neurotrophic and inflammatory systems accompanied by decreased CREB signaling in ischemic rat retina. Exp. Eye Res. 125, 156–163. doi: 10.1016/j.exer.2014.06.003
Gupta, V., You, Y., Li, J., Gupta, V., Golzan, M., Klistorner, A., et al. (2014). BDNF impairment is associated with age-related changes in the inner retina and exacerbates experimental Glaucoma. Biochim. Biophys. Acta 1842, 1567–1578. doi: 10.1016/j.bbadis.2014.05.026
Hamada, K., Shinozaki, Y., Namekata, K., Matsumoto, M., Ohno, N., Segawa, T., et al. (2021). Loss of P2Y1 receptors triggers Glaucoma-like pathology in mice. Br. J. Pharmacol. 178, 4552–4571. doi: 10.1111/BPH.15637
Harada, T., Harada, C., Nakamura, K., Hun, M. A., Quah, A. O., Namekata, K., et al. (2007). The potential role of glutamate transporters in the pathogenesis of Normal tension Glaucoma. J. Clin. Invest. 117, 1763–1770. doi: 10.1172/JCI30178
Harada, C., Kimura, A., Guo, X., Namekata, K., and Harada, T. (2019). Recent advances in genetically modified animal models of Glaucoma and their roles in drug repositioning. Br. J. Ophthalmol. 103, 161–166. doi: 10.1136/BJOPHTHALMOL-2018-312724
Harada, C., Namekata, K., Guo, X., Yoshida, H., Mitamura, Y., Matsumoto, Y., et al. (2010). ASK1 deficiency attenuates neural cell death in GLAST-deficient mice, a model of Normal tension Glaucoma. Cell Death Differ. 17, 1751–1759. doi: 10.1038/CDD.2010.62
Himori, N., Yamamoto, K., Maruyama, K., Ryu, M., Taguchi, K., Yamamoto, M., et al. (2013). Critical role of Nrf2 in oxidative stress-induced retinal ganglion cell death. J. Neurochem. 127, 669–680. doi: 10.1111/jnc.12325
Hines-Beard, J., Bond, W. S., Backstrom, J. R., and Rex, T. S. (2016). Virus-mediated EpoR76E gene therapy preserves vision in a Glaucoma model by modulating Neuroinflammation and decreasing oxidative stress. J. Neuroinflammation 13:39. doi: 10.1186/s12974-016-0499-5
Honjo, M., and Tanihara, H. (2018). Impact of the clinical use of ROCK inhibitor on the pathogenesis and treatment of Glaucoma. Jpn. J. Ophthalmol. 62, 109–126. doi: 10.1007/s10384-018-0566-9
Howell, G. R., Soto, I., Zhu, X., Ryan, M., Macalinao, D. G., Sousa, G. L., et al. (2012). Radiation treatment inhibits monocyte entry into the optic nerve head and prevents neuronal damage in a mouse model of Glaucoma. J. Clin. Invest. 122, 1246–1261. doi: 10.1172/JCI61135
Hu, H., Liu, Y., Li, K., Fang, M., Zou, Y., Wang, J., et al. (2021). Retrograde Fluorogold labeling of retinal ganglion cells in neonatal mice. Annals Transl Med 9:878. doi: 10.21037/atm-21-2022
Hu, C., Niu, L., Li, L., Song, M., Zhang, Y., Lei, Y., et al. (2020). ABCA1 regulates IOP by modulating Cav1/ENOS/NO signaling pathway. Invest. Ophthalmol. Vis. Sci. 61:33. doi: 10.1167/IOVS.61.5.33
Ji, S., Lin, S., Chen, J., Huang, X., Wei, C. C., Li, Z., et al. (2018). Neuroprotection of transplanting human umbilical cord mesenchymal stem cells in a microbead induced ocular hypertension rat model. Curr. Eye Res. 43, 810–820. doi: 10.1080/02713683.2018.1440604
Johnson, T. V., Bull, N. D., and Martin, K. R. (2011). Neurotrophic factor delivery as a protective treatment for Glaucoma. Exp. Eye Res. 93, 196–203. doi: 10.1016/J.EXER.2010.05.016
Johnson, E. C., Jia, L., Cepurna, W. O., Doser, T. A., and Morrison, J. C. (2007). Global changes in optic nerve head gene expression after exposure to elevated intraocular pressure in a rat Glaucoma model. Invest. Ophthalmol. Vis. Sci. 48, 3161–3177. doi: 10.1167/iovs.06-1282
Jose, S., Tan, S. W., Ooi, Y. Y., Ramasamy, R., and Vidyadaran, S. (2014). Mesenchymal stem cells exert anti-proliferative effect on lipopolysaccharide-stimulated BV2 microglia by reducing tumour necrosis factor-α levels. J. Neuroinflammation 11, 1–13. doi: 10.1186/s12974-014-0149-8
Ju, S. T., Panka, D. J., Cui, H., Ettinger, R., Ei-Khatib, M., Sherrf, D. H., et al. (1995). Fas(CD95)/FasL interactions required for programmed cell death after T-cell activation. Nature 373, 444–448. doi: 10.1038/373444a0
Kimura, A., Namekata, K., Guo, X., Harada, C., and Harada, T. (2016). Neuroprotection, growth factors and BDNF-TRKB Signalling in retinal degeneration. Int. J. Mol. Sci. 17:1584. doi: 10.3390/ijms17091584
Kimura, A., Noro, T., and Harada, T. (2020). Role of animal models in Glaucoma research. Neural Regen. Res. 15, 1257–1258. doi: 10.4103/1673-5374.272578
Krishnan, A., Fei, F., Jones, A., Busto, P., Marshak-Rothstein, A., Ksander, B. R., et al. (2016). Overexpression of soluble Fas ligand following adeno-associated virus gene therapy prevents retinal ganglion cell death in chronic and acute murine models of Glaucoma. J. Immunol. 197, 4626–4638. doi: 10.4049/JIMMUNOL.1601488
Krishnan, A., Kocab, A. J., Zacks, D. N., Marshak-Rothstein, A., and Gregory-Ksander, M. (2019). A small peptide antagonist of the Fas receptor inhibits Neuroinflammation and prevents axon degeneration and retinal ganglion cell death in an inducible mouse model of Glaucoma. J. Neuroinflammation 16:3. doi: 10.1186/S12974-019-1576-3
Kuo, C. Y., Lin, Y. C., Yang, J. J., and Yang, V. C. (2011). Interaction abolishment between mutant Caveolin-1(Δ62-100) and ABCA1 reduces HDL-mediated cellular cholesterol efflux. Biochem. Biophys. Res. Commun. 414, 337–343. doi: 10.1016/J.BBRC.2011.09.070
Lam, T. T., Abler, A. S., Kwong, J. M. K., and Tso, M. O. M. (1999). In rat retina. Invest. Ophthalmol. Vis. Sci. 40, 2391–2397.
Lee, E. S. Y., Sidoryk, M., Jiang, H., Yin, Z., and Aschner, M. (2009). Estrogen and tamoxifen reverse manganese-induced glutamate transporter impairment in astrocytes. J. Neurochem. 110, 530–544. doi: 10.1111/j.1471-4159.2009.06105.x
Lee, D., Kim, K. Y., Noh, Y. H., Chai, S., Lindsey, J. D., Ellisman, M. H., et al. (2012). Brimonidine blocks glutamate excitotoxicity-induced oxidative stress and preserves mitochondrial transcription factor a in ischemic retinal injury. PLoS One 7:7098. doi: 10.1371/journal.pone.0047098
Levi-Montalcini, R., Skaper, S. D., Dal Toso, R., Petrelli, L., and Leon, A. (1996). Nerve growth factor: from Neurotrophin to Neurokine. Trends Neurosci. 19, 514–520. doi: 10.1016/S0166-2236(96)10058-8
Levkovitch-Verbin, H. (2015). Retinal ganglion cell apoptotic pathway in Glaucoma: initiating and downstream mechanisms. Prog. Brain Res. 220, 37–57. doi: 10.1016/bs.pbr.2015.05.005
Levkovitch-Verbin, H., Harris-Cerruti, C., Groner, Y., Wheeler, L. A., Schwartz, M., and Yoles, E. (2000). RGC death in mice after optic nerve crush injury: oxidative stress and neuroprotection. Invest. Ophthalmol. Vis. Sci. 41, 4169–4174.
Li, Q., and Barres, B. A. (2018). Microglia and macrophages in brain homeostasis and disease. Nat. Rev. Immunol. 18, 225–242. doi: 10.1038/nri.2017.125
Li, T., Liu, T., Chen, X., Li, L., Feng, M., Zhang, Y., et al. (2020). Microglia induce the transformation of A1/A2 reactive astrocytes via the CXCR7/PI3K/Akt pathway in chronic post-surgical pain. J. Neuroinflammation 17, 1–15. doi: 10.1186/s12974-020-01891-5
Li, B., Ning, B., Yang, F., and Guo, C. (2022). Nerve growth factor promotes retinal neurovascular unit repair: a review. Curr. Eye Res. 47, 1095–1105. doi: 10.1080/02713683.2022.2055084
Liang, Y., Yu, Y., Yu, H., and Ma, L. (2019). Effect of BDNF-TrKB pathway on apoptosis of retinal ganglion cells in glaucomatous animal model, 3561–3568.
Libby, R. T., Anderson, M. G., Pang, I.-h., Robinson, Z. H., Savinova, O. V., Cosma, I. M., et al. (2005). Inherited Glaucoma in DBA 0 2J mice: Pertinent disease features for studying the neurodegeneration. Eur. Rev. Med. Pharmacol. Sci. 23, 637–648. doi: 10.26355/eurrev_201905_17777
Lin, W.-j., and Kuang, H.-y. (2014). Oxidative stress induces autophagy in response to multiple noxious stimuli in retinal ganglion cells. Autophagy 10, 1692–1701. doi: 10.4161/auto.36076
Lin, B., Zhang, X., and Xuegu, X. (2022). Nerve growth factor protects retinal ganglion cells related to inhibiting endoplasmic reticulum stress by inhibiting IRE1-JNK-CHOP signaling pathway. Ocul. Immunol. Inflamm. 30, 1341–1346. doi: 10.1080/09273948.2021.1872651
Lund, R. D., Wang, S., Bin, L., Girman, S., Holmes, T., Sauvé, Y., et al. (2009). Cells isolated from umbilical cord tissue rescue photoreceptors and visual functions in a rodent model of retinal disease. Stem Cells 25, 602–611. doi: 10.1634/stemcells.2006-0308
Luo, C., Yang, X., Kain, A. D., Powell, D. W., Kuehn, M. H., and Tezel, G. (2010). Glaucomatous tissue stress and the regulation of immune response through glial toll-like receptor signaling. Invest. Ophthalmol. Vis. Sci. 51, 5697–5707. doi: 10.1167/iovs.10-5407
Majsterek, I., Malinowska, K., Stanczyk, M., Kowalski, M., Blaszczyk, J., Kurowska, A. K., et al. (2011). Evaluation of oxidative stress markers in pathogenesis of primary open-angle Glaucoma. Exp. Mol. Pathol. 90, 231–237. doi: 10.1016/j.yexmp.2011.01.001
Mallick, J., Devi, L., Malik, P. K., and Mallick, J. (2016). Update on Normal tension Glaucoma. J. Ophthalmic Vis. Res. 11:204. doi: 10.4103/2008-322X.183914
Mallone, F., Sacchetti, M., Bruscolini, A., Scuderi, L., Marenco, M., and Lambiase, A. (2020). Neurotrophic factors in glaucoma and innovative delivery systems. Applied Sci. 10:9015. doi: 10.3390/app10249015
Massagué, J. (2012). TGFβ Signalling in context. Nat. Rev. Mol. Cell Biol. 13, 616–630. doi: 10.1038/nrm3434
Massagué, J., Seoane, J., and Wotton, D. (2005). Smad transcription factors. Genes Dev. 19, 2783–2810. doi: 10.1101/gad.1350705
McDowell, C. M., Tebow, H. E., Wordinger, R. J., and Clark, A. F. (2013). Smad3 is necessary for transforming growth factor-Beta2 induced ocular hypertension in mice. Exp. Eye Res. 116, 419–423. doi: 10.1016/j.exer.2013.10.017
McElnea, E. M., Quill, B., Docherty, N. G., Irnaten, M., Siah, W. F., Clark, A. F., et al. (2011). Oxidative stress, mitochondrial dysfunction and calcium overload in human Lamina Cribrosa cells from Glaucoma donors. Mol. Vis. 17, 1182–1191.
Mochizuki, H., Murphy, C. J., Brandt, J. D., Kiuchi, Y., and Russell, P. (2012). Altered stability of MRNAs associated with Glaucoma progression in human trabecular meshwork cells following oxidative stress. Invest. Ophthalmol. Vis. Sci. 53, 1734–1741. doi: 10.1167/iovs.12-7938
Moreno, M. C., Aldana, H. J., Marcos, J. O., Croxatto, P. H., Sande, J. C., Jaliffa, C. O., et al. (2005). A new experimental model of Glaucoma in rats through Intracameral injections of hyaluronic acid. Exp. Eye Res. 81, 71–80. doi: 10.1016/j.exer.2005.01.008
Morrison, J. C., Moore, C. G., Deppmeier, L. M. H., Gold, B. G., Meshul, C. K., and Johnson, E. C. (1997). A rat model of chronic pressure-induced optic nerve damage. Exp. Eye Res. 64, 85–96. doi: 10.1006/exer.1996.0184
Nadal-Nicolás, F. M., Jiménez-López, M., Sobrado-Calvo, P., Nieto-López, L., Cánovas-Martinez, I., Salinas-Navarro, M., et al. (2009). Brn3a as a marker of retinal ganglion cells: qualitative and quantitative time course studies in Naïve and optic nerve-injured retinas. Invest. Ophthalmol. Vis. Sci. 50, 3860–3868. doi: 10.1167/iovs.08-3267
Naguib, S., Backstrom, J. R., Gil, M., Calkins, D. J., and Rex, T. S. (2021). Retinal oxidative stress activates the NRF2/ARE pathway: an early endogenous protective response to ocular hypertension. Redox Biol. 42:1883. doi: 10.1016/j.redox.2021.101883
Nakazawa, T., and Fukuchi, T. (2020). What is glaucomatous optic neuropathy? Jpn. J. Ophthalmol. 64, 243–249. doi: 10.1007/S10384-020-00736-1
Nuschke, A. C., Farrell, S. R., Levesque, J. M., and Chauhan, B. C. (2015). Assessment of retinal ganglion cell damage in glaucomatous optic neuropathy: axon transport, injury and Soma loss. Exp. Eye Res. 141, 111–124. doi: 10.1016/j.exer.2015.06.006
Oddone, F., Roberti, G., Micera, A., Busanello, A., Bonini, S., Quaranta, L., et al. (2017). Exploring serum levels of brain derived neurotrophic factor and nerve growth factor across Glaucoma stages. PLoS One 12:e0168565. doi: 10.1371/journal.pone.0168565
Olmos, G., and Lladó, J. (2014). Tumor necrosis factor alpha: a link between Neuroinflammation and excitotoxicity. Mediators Inflamm. 2014:861231. doi: 10.1155/2014/861231
Osborne, N. N. (2008). Pathogenesis of ganglion ‘cell death’ in Glaucoma and neuroprotection: focus on ganglion cell axonal mitochondria. Prog. Brain Res. 173, 339–352. doi: 10.1016/S0079-6123(08)01124-2
Ozdemir, G., Tolun, F. I., Gul, M., and Imrek, S. (2009). Retinal oxidative stress induced by intraocular hypertension in rats may be ameliorated by Brimonidine treatment and N-acetyl cysteine supplementation. J. Glaucoma 18, 662–665. doi: 10.1097/IJG.0b013e31819c46b1
Pacwa, A., Machowicz, J., Akhtar, S., Rodak, P., Liu, X., Pietrucha-Dutczak, M., et al. (2023). Deficiency of the RNA-binding protein ELAVL1/HuR leads to the failure of endogenous and exogenous neuroprotection of retinal ganglion cells. Front. Cell. Neurosci. 17:57. doi: 10.3389/FNCEL.2023.1131356
Pagano, L., Lee, J. W., Posarelli, M., Giannaccare, G., Kaye, S., and Borgia, A. (2023). ROCK inhibitors in corneal diseases and Glaucoma—a comprehensive review of these emerging drugs. J. Clin. Med. 12:6736. doi: 10.3390/jcm12216736
Pang, I. H., and Clark, A. F. (2020). Inducible rodent models of Glaucoma. Prog. Retin. Eye Res. 75:799. doi: 10.1016/J.PRETEYERES.2019.100799
Parsadaniantz, M., Stéphane, A. R.-l., Goazigo, A. S., Habas, C., and Baudouin, C. (2020). Glaucoma: a degenerative optic neuropathy related to Neuroinflammation? Cells 9:535. doi: 10.3390/cells9030535
Peters, D., Bengtsson, B., and Heijl, A. (2013). Lifetime risk of blindness in open-angle Glaucoma. Am. J. Ophthalmol. 156, 724–730. doi: 10.1016/J.AJO.2013.05.027
Pickering, A. M., Vojtovich, L., Tower, J., and Davies, K. J. A. (2013). Oxidative stress adaptation with acute, chronic, and repeated stress. Free Radic. Biol. Med. 55, 109–118. doi: 10.1016/j.freeradbiomed.2012.11.001
Pietrucha-Dutczak, M., Smedowski, A., Liu, X., Matuszek, I., Varjosalo, M., and Lewin-Kowalik, J. (2017). Candidate proteins from Predegenerated nerve exert time-specific protection of retinal ganglion cells in Glaucoma. Sci. Rep. 7:14540. doi: 10.1038/s41598-017-14860-5
Piri, N., Kwong, J. M. K., Lei, G., and Caprioli, J. (2016). Heat shock proteins in the retina: focus on HSP70 and alpha Crystallins in ganglion cell survival. Prog. Retin. Eye Res. 52, 22–46. doi: 10.1016/j.preteyeres.2016.03.001
Platholi, J., and Lee, F. S. (2018). “Chapter 5 - Neurotrophic factors,” in Handbook of developmental neurotoxicology (Second Edition). Eds. W. Slikker, M. G. Paule, and C. Wang (Academic Press), 55–64.
Prokai-Tatrai, K., Xin, H., Nguyen, V., Szarka, S., Blazics, B., Prokai, L., et al. (2013). 17Β-estradiol eye drops protect the retinal ganglion cell layer and preserve visual function in an in vivo model of Glaucoma. Mol. Pharm. 10, 3253–3261. doi: 10.1021/mp400313u
Quan, L., Uyeda, A., and Muramatsu, R. (2022). Central nervous system regeneration: the roles of glial cells in the potential molecular mechanism underlying Remyelination. Inflamm Regener 42, 1–12. doi: 10.1186/S41232-022-00193-Y
Ritch, R., and Sharma, S. C. (2000). The combined effect of brain-derived neurotrophic factor and a free radical scavenger in experimental Glaucoma. Invest. Ophthalmol. Vis. Sci. 41, 2967–2971.
Roberti, G., Mantelli, F., Macchi, I., Massaro-Giordano, M., and Centofanti, M. (2014). Nerve growth factor modulation of retinal ganglion cell physiology. J. Cell. Physiol. 229, 1130–1133. doi: 10.1002/jcp.24573
Rolle, T., Ponzetto, A., and Malinverni, L. (2021). The role of Neuroinflammation in Glaucoma: an update on molecular mechanisms and new therapeutic options. Front. Neurol. 11:2422. doi: 10.3389/FNEUR.2020.612422
Ruan, Y., Jiang, S., Musayeva, A., and Gericke, A. (2020). Oxidative stress and vascular dysfunction in the retina: therapeutic strategies. Antioxidants 9, 1–30. doi: 10.3390/antiox9080761
Rudzinski, M., Wong, T. P., and Uri Saragovi, H. (2004). Changes in retinal expression of Neurotrophins and Neurotrophin receptors induced by ocular hypertension. J. Neurobiol. 58, 341–354. doi: 10.1002/neu.10293
Sakai, M., Sakai, H., Nakamura, Y., Fukuchi, T., and Sawaguchi, S. (2003). Immunolocalization of heat shock proteins in the retina of Normal monkey eyes and monkey eyes with laser-induced Glaucoma. Jpn. J. Ophthalmol. 47, 42–52. doi: 10.1016/S0021-5155(02)00627-5
Sanna, M. D., Ghelardini, C., and Galeotti, N. (2017). HuD-mediated distinct BDNF regulatory pathways promote regeneration after nerve injury. Brain Res. 1659, 55–63. doi: 10.1016/j.brainres.2017.01.019
Sanz-Morello, B., Ahmadi, H., Vohra, R., Saruhanian, S., Freude, K. K., Hamann, S., et al. (2021). Oxidative stress in optic neuropathies. Antioxidants 10:1538. doi: 10.3390/ANTIOX10101538
Schuettauf, F., Thaler, S., Bolz, S., Fries, J., Kalbacher, H., Mankowska, A., et al. (2007). Alterations of amino acids and glutamate transport in the DBA/2J mouse retina; possible clues to degeneration. Graefes Arch. Clin. Exp. Ophthalmol. 245, 1157–1168. doi: 10.1007/s00417-006-0531-z
Shevozaki, Y., Koizumi, S., Ishida, S., Sawada, J. I., Ohno, Y., and Inoue, K. (2005). Cytoprotection against oxidative stress-induced damage of astrocytes by extracellular ATP via P2Y1 receptors. Glia 49, 288–300. doi: 10.1002/GLIA.20118
Shi, Y., and Massagué, J. (2003). Mechanisms of TGF-β signaling from cell membrane to the nucleus. Cell 113, 685–700. doi: 10.1016/s0092-8674(03)00432-x
Shi, J., Zhang, Y. Q., and Simpkins, J. W. (1997). Effects of 17β-estradiol on glucose transporter 1 expression and endothelial cell survival following focal ischemia in the rats. Exp. Brain Res. 117, 200–206. doi: 10.1007/s002210050216
Shinozaki, Y., Saito, K., Kashiwagi, K., and Koizumi, S. (2023). Ocular P2 receptors and Glaucoma. Neuropharmacology 222:109302. doi: 10.1016/j.neuropharm.2022.109302
Skaper, S. (2008). The biology of Neurotrophins, Signalling pathways, and functional peptide mimetics of Neurotrophins and their receptors. CNS Neurol Disord 7, 46–62. doi: 10.2174/187152708783885174
Smedowski, A., Liu, X., Pietrucha-Dutczak, M., Matuszek, I., Varjosalo, M., and Lewin-Kowalik, J. (2016). Predegenerated Schwann cells-a novel Prospect for cell therapy for Glaucoma: neuroprotection, Neuroregeneration and neuroplasticity. Sci. Rep. 6:23187. doi: 10.1038/srep23187
Smedowski, A., Liu, X., Podracka, L., Akhtar, S., Trzeciecka, A., Pietrucha-Dutczak, M., et al. (2018a). Increased intraocular pressure alters the cellular distribution of HuR protein in retinal ganglion cells – a possible sign of endogenous neuroprotection failure. BBA-Mol. Basis Dis. 1864, 296–306. doi: 10.1016/j.bbadis.2017.10.030
Smedowski, A., Pietrucha-Dutczak, M., Kaarniranta, K., and Lewin-Kowalik, J. (2014). A rat experimental model of Glaucoma incorporating rapid-onset elevation of intraocular pressure. Sci. Rep. 4:5910. doi: 10.1038/srep05910
Smedowski, A., Pietrucha-Dutczak, M., Maniar, R., Ajeleti, M., Matuszek, I., and Lewin-Kowalik, J. (2018b). FluoroGold-labeled Organotypic retinal explant culture for neurotoxicity screening studies. Oxid. Med. Cell. Longev. 2018:7473. doi: 10.1155/2018/2487473
Sposato, V., Parisi, V., Manni, L., Teresa, M., Di, V., Sornelli, F., et al. (2009). Glaucoma alters the expression of NGF and NGF receptors in visual cortex and geniculate nucleus of rats: Effect of Eye NGF Application. Vision Res. 49, 54–63. doi: 10.1016/j.visres.2008.09.024
Sulak, R., Liu, X., and Smedowski, A. (2024). The concept of gene therapy for Glaucoma: the dream that has not come true yet. Neural Regen. Res. 19:92. doi: 10.4103/1673-5374.375319
Sun, Q., Ooi, V. E. C., and Chan, S. O. (2001). N-methyl-D-aspartate-induced excitotoxicity in adult rat retina is antagonized by single systemic injection of MK-801. Exp. Brain Res. 138, 37–45. doi: 10.1007/s002210100688
Taguchi, M., Shinozaki, Y., Kashiwagi, K., Shigetomi, E., Robaye, B., and Koizumi, S. (2016). Müller cell-mediated neurite outgrowth of the retinal ganglion cells via P2Y6 receptor signals. J. Neurochem. 136, 741–751. doi: 10.1111/JNC.13427
Terman, A., Gustafsson, B., and Brunk, U. T. (2006). Mitochondrial damage and Intralysosomal degradation in cellular aging. Mol. Aspects Med. 27, 471–482. doi: 10.1016/j.mam.2006.08.006
Tham, Y. C., Li, X., Wong, T. Y., Quigley, H. A., Aung, T., and Cheng, C. Y. (2014). Global prevalence of Glaucoma and projections of Glaucoma burden through 2040: a systematic review and Meta-analysis. Ophthalmology 121, 2081–2090. doi: 10.1016/J.OPHTHA.2014.05.013
Thumkeo, D., Watanabe, S., and Narumiya, S. (2013). Physiological roles of rho and rho effectors in mammals. Eur. J. Cell Biol. 92, 303–315. doi: 10.1016/j.ejcb.2013.09.002
Tsukamoto, T., Kajiwara, K., Nada, S., and Okada, M. (2019). Src mediates TGF-β-induced intraocular pressure elevation in Glaucoma. J. Cell. Physiol. 234, 1730–1744. doi: 10.1002/jcp.27044
Vargas, C., Jorge, L., Belforte, N., and Di Polo, A. (2016). The glial cell modulator Ibudilast attenuates Neuroinflammation and enhances retinal ganglion cell viability in Glaucoma through protein kinase a signaling. Neurobiol. Dis. 93, 156–171. doi: 10.1016/j.nbd.2016.05.002
Villanueva, J. R., Esteban, J. M., Rodríguez, L. J., and Villanueva, S. (2020). Retinal cell protection in ocular excitotoxicity diseases. Possible alternatives offered by microparticulate drug delivery systems and future prospects. Pharmaceutics 12:94. doi: 10.3390/pharmaceutics12020094
Vorwerk, C. K., Naskar, R., and Dreyer, E. B. (1999). Excitotoxicity and Glaucoma. Klinische Monatsblatter Fur Augenheilkunde 214, 2–11. doi: 10.1055/s-2008-1034740
Wagner, I. V., Stewart, M. W., and Dorairaj, S. K. (2022). Updates on the diagnosis and Management of Glaucoma. Mayo Clin Proc Innov Qual Outcomes 6, 618–635. doi: 10.1016/J.MAYOCPIQO.2022.09.007
Wallace, D. M., Pokrovskaya, O., and O’Brien, C. J. (2015). The function of Matricellular proteins in the Lamina Cribrosa and trabecular meshwork in Glaucoma. J. Ocul. Pharmacol. Ther. 31, 386–395. doi: 10.1089/jop.2014.0163
Wang, L., Pei, S., Han, L., Guo, B., Li, Y., Duan, R., et al. (2018). Mesenchymal stem cell-derived exosomes reduce A1 astrocytes via downregulation of phosphorylated NFκB P65 subunit in spinal cord injury. Cell. Physiol. Biochem. 50, 1535–1559. doi: 10.1159/000494652
Wang, H., Wang, R., Thrimawithana, T., Little, P. J., Jiangping, X., Feng, Z. P., et al. (2014). The nerve growth factor signaling and its potential as therapeutic target for Glaucoma. Biomed. Res. Int. 2014:759473. doi: 10.1155/2014/759473
Ward, R., Li, W., Abdul, Y., Jackson, L. D., Dong, G., Jamil, S., et al. (2019). NLRP3 Inflammasome inhibition with MCC950 improves diabetes-mediated cognitive impairment and Vasoneuronal remodeling after ischemia. Pharmacol. Res. 142, 237–250. doi: 10.1016/j.phrs.2019.01.035
Weber, A. J., and Harman, C. D. (2013). BDNF treatment and extended recovery from optic nerve trauma in the cat. Invest. Ophthalmol. Vis. Sci. 54, 6594–6604. doi: 10.1167/iovs.13-12683
Weber, A. J., Viswana, S., and Harman, C. D. (2010). Combined application of BDNF to the eye and brain enhances ganglion cell survival and function in the cat after optic nerve injury. Invest. Ophthalmol. Vis. Sci. 51, 327–334. doi: 10.1167/iovs.09-3740
Wei, X., Cho, K. S., Thee, E. F., Jager, M. J., and Chen, D. F. (2019). Neuroinflammation and microglia in Glaucoma: time for a paradigm shift. J. Neurosci. Res. 97, 70–76. doi: 10.1002/JNR.24256
Weinreb, R. N., Aung, T., and Medeiros, F. A. (2014). The pathophysiology and treatment of Glaucoma: a review. JAMA 131:1901. doi: 10.1001/jama.2014.3192
Weissmiller, A. M., and Wu, C. (2012). Current advances in using neurotrophic factors to treat neurodegenerative disorders. Transl Neurodegener. 1:14. doi: 10.1186/2047-9158-1-14
Wenmin, J., Luosheng, T., Jun, Z., and Baihua, C. (2016). Adeno-associated virus mediated SOD gene therapy protects the retinal ganglion cells from chronic intraocular pressure elevation induced injury via attenuating oxidative stress and improving mitochondrial dysfunction in a rat model. Am. J. Transl. Res. 8, 799–810.
Williams, P. A., Braine, C. E., Foxworth, N. E., Cochran, K. E., and John, S. W. M. (2017). GlyCAM1 negatively regulates monocyte entry into the optic nerve head and contributes to radiation-based protection in Glaucoma. J. Neuroinflammation 14, 1–12. doi: 10.1186/s12974-017-0868-8
Williams, P. A., Braine, C. E., Kizhatil, K., Foxworth, N. E., Tolman, N. G., Harder, J. M., et al. (2019). Inhibition of monocyte-like cell extravasation protects from neurodegeneration in DBA/2J Glaucoma. Mol. Neurodegener. 14, 1–23. doi: 10.1186/s13024-018-0303-3
Wilson, G. N., Inman, D. M., Denger-Crish, C. M., Smith, M. A., and Crish, S. D. (2015). Early pro-inflammatory cytokine elevations in the DBA/2J mouse model of Glaucoma. J. Neuroinflammation 12:176. doi: 10.1186/s12974-015-0399-0
Wójcik-Gryciuk, A., Gajewska-Woźniak, O., Kordecka, K., Boguszewski, P. M., Waleszczyk, W., and Skup, M. (2020). Neuroprotection of retinal ganglion cells with AAV2-BDNF pretreatment restoring Normal TrkB receptor protein levels in Glaucoma. Int. J. Mol. Sci. 21, 1–15. doi: 10.3390/IJMS21176262
Wong, K. A., and Benowitz, L. I. (2022). Retinal ganglion cell survival and axon regeneration after optic nerve injury: role of inflammation and other factors. Int. J. Mol. Sci. 23:179. doi: 10.3390/IJMS231710179
XXX (2017). European Glaucoma society terminology and guidelines for Glaucoma, 4th edition—chapter 3: treatment principles and options supported by the EGS foundation: part 1: foreword; introduction; glossary; chapter 3 treatment principles and options. Br. J. Ophthalmol. 101, 130–195. doi: 10.1136/bjophthalmol-2016-EGSguideline.003
Yang, X., Luo, C., Cai, J., Powell, D. W., Dahai, Y., Kuehn, M. H., et al. (2011). Neurodegenerative and inflammatory pathway components linked to TNF-α/TNFR1 signaling in the glaucomatous human retina. Invest. Ophthalmol. Vis. Sci. 52, 8442–8454. doi: 10.1167/iovs.11-8152
Yang, X., Zeng, Q., Barlş, M., and Tezel, G. (2020). Transgenic inhibition of Astroglial NF-ΚB restrains the Neuroinflammatory and neurodegenerative outcomes of experimental mouse Glaucoma. J. Neuroinflammation 17, 1–18. doi: 10.1186/s12974-020-01930-1
Yokota, H., Narayanan, S. P., Zhang, W., Liu, H., Rojas, M., Zhimin, X., et al. (2011). Neuroprotection from retinal ischemia/reperfusion injury by NOX2 NADPH oxidase deletion. Invest. Ophthalmol. Vis. Sci. 52, 8123–8131. doi: 10.1167/iovs.11-8318
Zhang, Y., Yang, X., Sun, Q., Xue, S., Guan, H., and Ji, M. (2019). Activation of P2X7R-NLRP3 pathway in retinal microglia contribute to retinal ganglion cells death in chronic ocular hypertension (COH). Exp. Eye Res. 188:107771. doi: 10.1016/j.exer.2019.107771
Keywords: glaucoma, glaucoma models, apoptosis, retinal ganglion cell, neuroprotection
Citation: Bugara K, Pacwa A and Smedowski A (2024) Molecular pathways in experimental glaucoma models. Front. Neurosci. 18:1363170. doi: 10.3389/fnins.2024.1363170
Edited by:
Enping Huang, Southern Medical University, ChinaReviewed by:
Yukie Yamahashi, Fujita Health University, JapanBijay Parajuli, University of Yamanashi, Japan
Copyright © 2024 Bugara, Pacwa and Smedowski. This is an open-access article distributed under the terms of the Creative Commons Attribution License (CC BY). The use, distribution or reproduction in other forums is permitted, provided the original author(s) and the copyright owner(s) are credited and that the original publication in this journal is cited, in accordance with accepted academic practice. No use, distribution or reproduction is permitted which does not comply with these terms.
*Correspondence: Adrian Smedowski, asmedowski@sum.edu.pl