- 1Cooper Medical School of Rowan University, Camden, NJ, United States
- 2Department of Neuroradiology, Cooper University Health Care, Camden, NJ, United States
- 3Department of Neurology, Cooper University Health Care, Camden, NJ, United States
- 4Department of Neurosurgery, Cooper University Health Care, Camden, NJ, United States
- 5Department of Radiology, Cooper University Health Care, Camden, NJ, United States
Intracerebral hemorrhage (ICH) is characterized by hematoma development within the brain’s parenchyma, contributing significantly to the burden of stroke. While non-contrast head computed tomography (CT) remains the gold standard for initial diagnosis, this review underscores the pivotal role of magnetic resonance imaging (MRI) in ICH management. Beyond diagnosis, MRI offers invaluable insights into ICH etiology, prognosis, and treatment. Utilizing echo-planar gradient-echo or susceptibility-weighted sequences, MRI demonstrates exceptional sensitivity and specificity in identifying ICH, aiding in differentiation of primary and secondary causes. Moreover, MRI facilitates assessment of hemorrhage age, recognition of secondary lesions, and evaluation of perihematomal edema progression, thus guiding tailored therapeutic strategies. This comprehensive review discusses the multifaceted utility of MRI in ICH management, highlighting its indispensable role in enhancing diagnostic accuracy as well as aiding in prognostication. As MRI continues to evolve as a cornerstone of ICH assessment, future research should explore its nuanced applications in personalized care paradigms.
1 Introduction
Intracerebral hemorrhage (ICH) is characterized by hematoma development within the brain’s parenchyma. In the United States alone, it contributes to roughly 10–20% of the 795,000 annual stroke cases (Rajashekar and Liang, 2023; Unnithan et al., 2023). ICH can be broadly classified into primary and secondary forms, with primary ICH accounting for about 80% of cases, while secondary ICH makes up the remaining 20% (Macellari et al., 2014; Jain et al., 2021). Primary ICH stems from underlying small vessel diseases such as hypertension and cerebral amyloid angiopathy (CAA), whereas secondary ICH can result from various factors such as hemorrhagic conversion of acute ischemic stroke (AIS), vascular malformations, or other structural anomalies (Macellari et al., 2014; Raposo et al., 2023). This review delineates the role of magnetic resonance imaging (MRI) in ICH, including its use for diagnosis, treatment, and prognostication.
2 Need for imaging in ICH and urgency
ICH is associated with high rates of severe disability and mortality, including an approximately 40% mortality rate within 1 month and a combined severe disability and mortality rate of up to 75% within 1 year (van Asch et al., 2010; Jain et al., 2021). Given these data on the severity of the natural history of ICH, emergent evaluation is critical. Early identification enables initiation of ultra-early treatment to prevent hematoma expansion and mitigate neuroinflammation. The potential of reduced morbidity and mortality associated with ICH through timely recognition and intervention has led to an increasing emphasis on its prompt diagnosis (Li et al., 2024).
Initial imaging for ICH is predicated on non-contrast head computed tomography (NCCT), commonly performed in the emergency department. CT or MR angiography may be used to identify risk of hematoma expansion and vascular causes, while MRI is also effective in identifying other structural causes of ICH (Hemphill 3rd et al., 2015). Once an ICH is visualized using neuroimaging, early interventions via management of airway, hemostasis, seizures, hypertension, intracranial hypertension, hyperglycemia, fever, and surgical intervention are critical as they can contribute to decreased rates of morbidity and mortality (Morotti and Goldstein, 2016). Rapid imaging is crucial for swift diagnosis. While both CT and MRI are highly sensitive in diagnosing ICH, a NCCT is typically the first-line diagnostic tool due to its ability to differentiate between ischemic strokes and ICH, wider availability, shorter scanning time and patient factors such as clinical instability, presence of pacemaker or claustrophobia (Macellari et al., 2014; Morotti and Goldstein, 2016; Fandler-Höfler et al., 2023).
3 Role of MRI in ICH diagnosis
MRI demonstrates sensitivity, specificity, and overall accuracy, approaching nearly 100%, for diagnosing ICH in hyperacute and acute settings (Fiebach et al., 2004; Romanova et al., 2014). MRI, specifically echo-planar gradient-echo (GRE) or susceptibility-weighted imaging (SWI) sequences, are particularly useful in the hyperacute (less than 24 h of symptom onset) setting of ICH because as time progresses, the hemorrhage can obscure the underlying brain parenchyma, and this occurs to a greater degree after 24 h. MRI also has higher diagnostic accuracy for chronic ICH when compared to CT (Linfante et al., 1999; Kidwell et al., 2004; Macellari et al., 2014). However, despite this, MRI should not replace CT as the primary imaging method due to its greater expediency, which is crucial for promptly detecting ICH.
MRI is a useful tool in determining the acuity of ICH, especially in instances where the ICH is composed of hemorrhagic components of different ages, or when the patient has had multiple ICHs. The findings on MRI are dependent on the age of the hemorrhage. As a hematoma ages, hemoglobin undergoes various transformations, transitioning through oxyhemoglobin, deoxyhemoglobin, methemoglobin, and ultimately leading to the breakdown of red blood cells (RBCs) into ferritin and hemosiderin (Bradley, 1993). As the hemoglobin breaks down from oxyhemoglobin to the rest of the RBC products, it transitions from diamagnetic material, having no unpaired electrons, to paramagnetic material, having unpaired electrons. The intensity seen on MRI depends on whether unpaired electrons are present, how many unpaired electrons there are, and the location of the RBC products. The phases of ICH (see Table 1) can be classified as hyperacute (< 24 h of the hemorrhage, intracellular oxyhemoglobin, Figure 1A, Images A1,A2), acute (1–3 days, intracellular deoxyhemoglobin, Figure 1A, Images B1,B2), early subacute (3–7 days, intracellular methemoglobin, Figure 1A, Images C1,C2), late subacute (7–28 days, extracellular methemoglobin, Figure 1A, Images D1,D2), or chronic (>1-month, extracellular ferritin and hemosiderin, Figure 1A, Images E1,E2) (Bradley, 1993; Weerink et al., 2023).
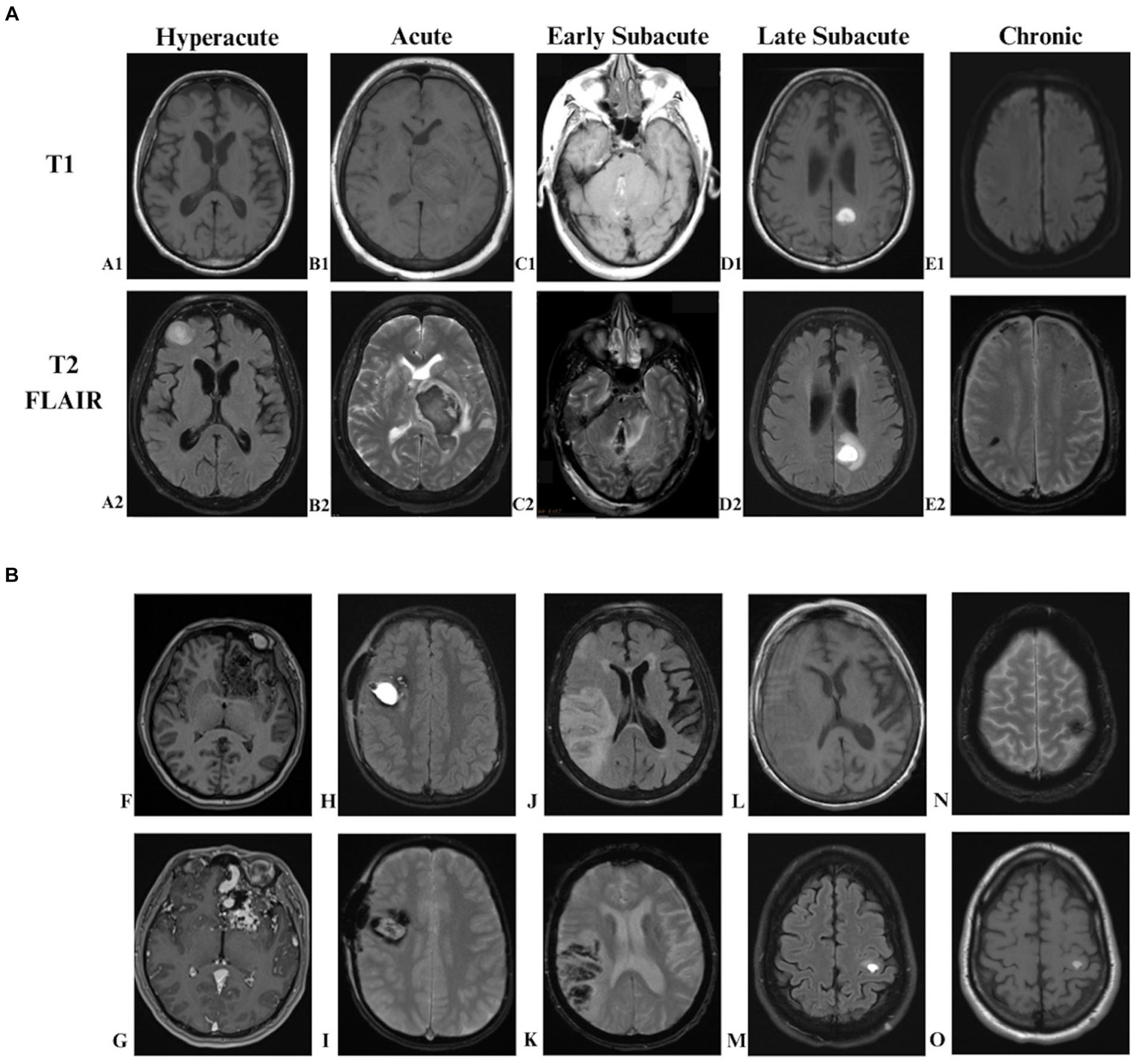
Figure 1. ICH stages of blood over time on MRI and common Etiologies of ICH on MRI. (A) Stages of blood in ICH: (A1) ICH hyperacute blood on T1, (A2) ICH hyperacute blood on T2 FLAIR, (B1) ICH acute blood on T1, (B2) ICH acute blood on T2 FLAIR, (C1) ICH early subacute blood on T1, (C2) ICH early subacute blood on T2 FLAIR, (D1) ICH late subacute blood on T1, (D2) ICH late subacute blood on T2, (E1) ICH chronic blood on T1. (E2) ICH chronic blood on GRE. (B) Common ICH etiologies on MRI: (F) AVM on T1 Pre-Contrast, (G) AVM on T1 Post-Contrast, (H) Cavernous hemangioma on T2 FLAIR, (I) Cavernous hemangioma on GRE, (J) Hemorrhagic conversion of ischemic stroke on T2 FLAIR, (K) Hemorrhagic conversion of ischemic stroke on GRE, (L) Hemorrhagic conversion of ischemic stroke on T1, (M) Hemorrhagic metastasis on T2 FLAIR, (N) Hemorrhagic metastasis on GRE, (O) Hemorrhagic metastasis on T1. ICH = Intracerebral hemorrhage, FLAIR = fluid-attenuated inversion recovery, GRE = gradient-echo, AVM = arteriovenous malformation.
4 Role of MRI in identifying an ICH etiology
MRI is useful in identifying primary causes of ICH, such as small-vessel disease (SVD) or cerebral amyloid angiopathy (CAA). In particular, findings of lobar macrohemorrhage, exclusively cortical microbleeds (CMBs), cortical superficial siderosis (cSS), and a multispot pattern of white matter hyperintensities have been associated with CAA, whereas CMBs in subcortical locations and basal ganglia white matter hyperintensities may indicate SVD as the ICH etiology (Charidimou et al., 2016, 2022). Making the distinction between CAA and SVD is essential, as it has implications on risk of ICH recurrence, progression, and decision-making regarding the safety of antithrombotic treatments (Charidimou et al., 2019).
MRI plays a critical role in identifying secondary causes of ICH as well. These include structural vascular lesions such as arteriovenous malformations (AVMs), cavernomas, or dural arteriovenous fistulae. Other readily apparent secondary causes of ICH diagnosed predominantly via MRI include hemorrhagic conversion of AIS, cerebral neoplasms, along with cerebral venous thrombosis (CVT), arterial dissection and non-atheromatous vasculopathies such as moyamoya disease, vasculitis, reversible cerebral vasoconstriction syndrome (RCVS) and infective endocarditis (IE) (Wijman et al., 2010; Macellari et al., 2014; Morotti and Goldstein, 2016; Fandler-Höfler et al., 2023; Raposo et al., 2023). In addition, MRI can even be used to aid diagnosing IE in individuals with silent emboli, without neurologic symptoms (Habib et al., 2015). Given its utility in identifying an etiology for ICH, MRI is recommended in all patients without a clear macrovascular cause of ICH identified on a CTA (Greenberg et al., 2022).
MRI is the most sensitive and specific method for identifying cerebral cavernous malformations, which often exhibit a distinct “popcorn” appearance on T2-weighted imaging, with central hyperintensity indicating recent bleeding and a surrounding hypointense halo indicative of hemosiderin from prior bleeding events (Figure 1B, Images H,I). In cases of CVT, contrast-enhanced MR venography provides detailed visualization of thrombosed segments within the venous sinus, showing strong correlation with conventional digital subtraction angiography (DSA) findings and distinguishing anatomical variations, such as hypoplastic sinuses, from CVT. CVT, which is defined as a thrombus in a venous sinus, superficial intracranial vein, or deep intracranial vein (Oliveira et al., 2022) can also be visualized on MRI. This is due to the fact that patent dural sinuses typically appear as a flow void – a signal loss that occurs within moving fluids. Meanwhile CVTs can be recognized best on T2 or fluid-attenuated inversion recovery (FLAIR) sequences as an absence of a flow void (Chiewvit et al., 2011; Oliveira et al., 2022). MRI is also useful in identifying AVMs, where clusters of hypointense vascular channels and enlarged draining veins (pre-contrast, Figure 1B, Image F) enhance following contrast administration (post-contrast, Figure 1B, Image G).
Diffusion-weighted imaging (DWI) sequences of MRI play a crucial role in the evaluation of ICH, as they can help to differentiate between AIS with hemorrhagic conversion and primary ICH. Central hyperintensity on DWI can be seen in both AIS as well as hyperacute hematomas, and these etiologies can appear very similar on T2 weighted imaging. However, using FLAIR sequences can allow for the visualization of the peripheral hypointense rim that is present in hyperacute hematoma and not in AIS (Kang et al., 2001). AIS with hemorrhagic conversion may also have a mixed appearance on DWI sequence, and have microbleeds on GRE or SWI, as opposed to a more homogenous appearance of ICHs (Bradley, 1993).
5 Role of MRI in ICH treatment
ICH results in blood–brain barrier (BBB) disruption and parenchymal cellular swelling, contributing to perihematomal edema (PHE). This can cause brain tissue compression, intracranial hypertension, herniation and death. The fluid-attenuated inversion recovery (FLAIR) sequence of the MRI can be used to quantify the volume of PHE, which appears hyperintense (Urday et al., 2015; Ironside et al., 2019). CT can be used for this purpose, however both manual and automated quantification are limited by interference of leukoariosis and cerebrospinal fluid, which are of similar Hounsfield unit densities (Urday et al., 2015). While PHE evolution may correlate with functional outcomes, minimally invasive surgery (MIS) for hematoma evacuation has been shown to be associated with decreased clot burden, PHE, and more recently improved functional outcomes (Mould et al., 2019; Hieber et al., 2023; Kellner and Mocco, 2023; Pradilla et al., 2024). The degree of clot evacuation by MIS has correlated with a decrease in pericavity edema (PCE) and PCE remained static following MIS ICH evacuation in one study (Horowitz et al., 2022).
Patients with ICH may also be at risk of AIS and other cardiovascular events. When antithrombotic or anticoagulant medications are considered for secondary stroke prevention, risk of hemorrhage needs to be carefully weighed against the risk of ischemic and vaso-occlusive diseases (Greenberg et al., 2022). MRI can identify ICH causes that have a heightened risk of ICH recurrence such as CAA, or IE hence can provide useful information when making these treatment decisions. In patients with IE, MRI can also aid in lesion characterization such as identification of abscesses, mycotic aneurysms, lobar hematomas and territorial strokes prior to surgical evaluation for valve surgery (Chakraborty et al., 2019).
If the initial MRI does not reveal an underlying etiology of the ICH, a follow-up MRI could be useful to alter future management. Limited literature suggests that the yield of repeat MRI to detect a secondary lesion varies between 0 to 16% of patients with spontaneous ICH, with secondary lesions typically found to be tumors or cavernomas (Mouchtouris et al., 2021; Wilson et al., 2023). Studies evaluating outpatient follow-up MRI in this setting have included studies performed any time from 1 day to 2 years post-ICH, and the optimal timing of this repeat imaging remains unclear (particularly given the complex evolution of blood products discussed above) (Mouchtouris et al., 2021; Wilson et al., 2023). Future research is required to determine optimal timing for follow-up MRI scans, as well as to identify the specific patient cohorts who would benefit most from such assessments. This may involve considering factors such as the location of ICH and other pertinent imaging characteristics. Such efforts may enhance diagnostic accuracy while minimizing unnecessary testing and associated costs.
6 Role of MRI in ICH risk stratification and prognostication
In the acute phase, an MRI can provide more detailed information on the severity of ICH-related injury such as hematoma volume, the degree of surrounding edema and midline shift, assisting in neuroprognostication. Involvement of critical parts of the ascending reticular activating system, caudate nucleus, thalami or diffuse damage impairing network connectivity identified by MRI, can aid in prediction of recovery from disorders of consciousness caused by ICH (Rohaut et al., 2019). Task-based and resting-state functional MRI can reveal cognitive motor dissociation in patients that appear to be unresponsive on bedside examination and predict re-emergence of consciousness (Edlow et al., 2021).
MRI can also aid in individual ICH risk stratification. In patients with ICH, features suggestive of CAA on MRI are associated with the highest ICH recurrence (Greenberg et al., 2022). MRI findings suggestive of cerebral small vessel disease (SVD) and CMBs strongly correlate with poorer functional and cognitive outcomes, an increased risk of ICH recurrence and increased long-term mortality (Greenberg et al., 2022). Notably, in patients with lobar ICH, the presence of cSS, a marker of hemorrhagic risk in CAA, has been associated with higher odds of hematoma expansion and an independent biomarker of poor prognosis (Boulouis et al., 2016; Sporns et al., 2021). The presence of cSS is also associated with a higher recurrence risk in patients with lobar ICH, worse cognitive trajectories, and a higher incidence of post-ICH dementia. In patients with AIS, CMBs detected on GRE or SWI are associated with higher rates of hemorrhagic transformation (Dar et al., 2018). In addition to identification of macrovascular causes of ICH, MRI can identify small intraventricular hemorrhages that may not be detected on CT, allowing a superior estimation of ICH recurrence (Romanova et al., 2014).
PHE, which represents the inflammatory and cytotoxic responses of the tissue surrounding the ICH and can be a quantifiable marker of secondary brain injury (SBI). Variations in pathophysiological mechanisms could affect temporal patterns of PHE formation. While it has been suggested that peak PHE volume typically occurs between 2 to 3 weeks after ICH, observations suggest that PHE can continue to progress for up to 21 days following the onset of ICH (Urday et al., 2015; Ironside et al., 2019; Chen et al., 2021). PHE volume on admission in small ICHs, and PHE increase at 72 h, have been correlated with worse functional outcomes in small studies, with variable methods of PHE quantification (Sansing et al., 2011; Appelboom et al., 2013; Urday et al., 2015).
DWI sequences can also detect small remote DWI hyperintensities in patients with ICH (Xu et al., 2017). Studies have shown a correlation between aggressive blood pressure reduction, systolic blood pressure variability, and the occurrence of DWI MRI lesions, suggesting that acute disruptions in blood pressure autoregulation may contribute to their development (Kidwell et al., 2017). These lesions have been associated with conditions such as SVD and CAA, and their pathogenesis may involve additional factors related to microangiopathy and characteristics specific to ICH (Wu et al., 2015; Murthy et al., 2020; Xu et al., 2022) Despite being often subclinical and unidentifiable on CT, the presence of punctate ischemic DWI lesions increases the risk of subsequent ischemic stroke by 2.5 times, and may be associated with worse long-term outcomes (Murthy et al., 2021). Identifying these DWI lesions through MRI could provide valuable insight for stratifying patients based on potential outcomes, and could even guide acute blood pressure targets, highlighting the utility of MRI in assessing patient prognosis.
Finally, previous literature has utilized MRI in cases of ICH and IVH to stratify patients with the van Swieten scale (vSS) to grade severity of leukoaraiosis via the Fazekas Score (FS) with severe leukoaraiosis defined as FS > 3 or deep FS 2 to 3. Patients with this definition of severe leukoaraiosis were found to have persistently poor outcomes 1 year after their hemorrhagic event. This exemplifies another utilization of MRI for the long term prognostication of ICH patients (Shah et al., 2022).
7 Conclusion
MRI is a pivotal tool in the evaluation of ICH. It has a very high sensitivity, specificity, and accuracy in diagnosing ICH, particularly in the hyperacute stage. Beyond its hyperacute utility, MRI can also be utilized to determine ICH age and differentiation of underlying causes, thereby influencing disease-specific treatment strategies, and offering prognostic value. As MRI continues to serve as a cornerstone of ICH assessment and becomes standard of care globally, future studies are needed to assess its use in individualized clinical scenarios, such as the diagnostic value of performing serial studies and the utility of MRI in guiding specific medical and procedural interventions.
Author contributions
MP: Conceptualization, Data curation, Methodology, Writing – original draft, Writing – review & editing. KK: Writing – original draft, Writing – review & editing. JT: Supervision, Visualization, Writing – review & editing. DT: Investigation, Supervision, Writing – review & editing. CR: Conceptualization, Data curation, Writing – review & editing. SR: Conceptualization, Supervision, Writing – original draft, Writing – review & editing.
Funding
The author(s) declare that no financial support was received for the research, authorship, and/or publication of this article.
Conflict of interest
The authors declare that the research was conducted in the absence of any commercial or financial relationships that could be construed as a potential conflict of interest.
Publisher’s note
All claims expressed in this article are solely those of the authors and do not necessarily represent those of their affiliated organizations, or those of the publisher, the editors and the reviewers. Any product that may be evaluated in this article, or claim that may be made by its manufacturer, is not guaranteed or endorsed by the publisher.
References
Appelboom, G., Bruce, S. S., Hickman, Z. L., Zacharia, B. E., Carpenter, A. M., Vaughan, K. A., et al. (2013). Volume-dependent effect of perihaematomal oedema on outcome for spontaneous intracerebral haemorrhages. J. Neurol. Neurosurg. Psychiatry 84, 488–493. doi: 10.1136/jnnp-2012-303160
Boulouis, G., van Etten, E. S., Charidimou, A., Auriel, E., Morotti, A., Pasi, M., et al. (2016). Association of key Magnetic Resonance Imaging Markers of cerebral small vessel disease with hematoma volume and expansion in patients with lobar and deep intracerebral hemorrhage. JAMA Neurol. 73, 1440–1447. doi: 10.1001/jamaneurol.2016.2619
Bradley, W. G. Jr. (1993). MR appearance of hemorrhage in the brain. Radiology 189, 15–26. doi: 10.1148/radiology.189.1.8372185
Chakraborty, T., Scharf, E., DeSimone, D., el Rafei, A., Brinjikji, W., Baddour, L. M., et al. (2019). Variable significance of brain MRI findings in infective endocarditis and its effect on surgical decisions. Mayo Clin. Proc. 94, 1024–1032. doi: 10.1016/j.mayocp.2018.09.015
Charidimou, A., Boulouis, G., Frosch, M. P., Baron, J. C., Pasi, M., Albucher, J. F., et al. (2022). The Boston criteria version 2.0 for cerebral amyloid angiopathy: a multicentre, retrospective, MRI-neuropathology diagnostic accuracy study. Lancet Neurol. 21, 714–725. doi: 10.1016/S1474-4422(22)00208-3
Charidimou, A., Boulouis, G., Haley, K., Auriel, E., van Etten, E. S., Fotiadis, P., et al. (2016). White matter hyperintensity patterns in cerebral amyloid angiopathy and hypertensive arteriopathy. Neurology 86, 505–511. doi: 10.1212/WNL.0000000000002362
Charidimou, A., Boulouis, G., Xiong, L., Pasi, M., Roongpiboonsopit, D., Ayres, A., et al. (2019). Cortical Superficial Siderosis Evolution. Stroke 50, 954–962. doi: 10.1161/STROKEAHA.118.023368
Chen, Y., Chen, S., Chang, J., Wei, J., Feng, M., and Wang, R. (2021). Perihematomal edema after intracerebral hemorrhage: an update on pathogenesis, risk factors, and therapeutic advances. Front. Immunol. 12:740632. doi: 10.3389/fimmu.2021.740632
Chiewvit, P., Piyapittayanan, S., and Poungvarin, N. (2011). Cerebral venous thrombosis: diagnosis dilemma. Neurol. Int. 3:e13:13. doi: 10.4081/ni.2011.e13
Dar, N. Z., Ain, Q. U., Nazir, R., and Ahmad, A. (2018). Cerebral microbleeds in an acute ischemic stroke as a predictor of hemorrhagic transformation. Cureus 10:e3308. doi: 10.7759/cureus.3308
Edlow, B. L., Claassen, J., Schiff, N. D., and Greer, D. M. (2021). Recovery from disorders of consciousness: mechanisms, prognosis and emerging therapies. Nat. Rev. Neurol. 17, 135–156. doi: 10.1038/s41582-020-00428-x
Fandler-Höfler, S., Obergottsberger, L., Ambler, G., Eppinger, S., Wünsch, G., Kneihsl, M., et al. (2023). Association of the Presence and Pattern of MRI markers of cerebral small vessel disease with recurrent intracerebral hemorrhage. Neurology 101, e794–e804. doi: 10.1212/WNL.0000000000207510
Fiebach, J. B., Schellinger, P. D., Gass, A., Kucinski, T., Siebler, M., Villringer, A., et al. (2004). Stroke magnetic resonance imaging is accurate in Hyperacute intracerebral hemorrhage. Stroke 35, 502–506. doi: 10.1161/01.STR.0000114203.75678.88
Greenberg, S. M., Ziai, W. C., Cordonnier, C., Dowlatshahi, D., Francis, B., Goldstein, J. N., et al. (2022). 2022 guideline for the Management of Patients with Spontaneous Intracerebral Hemorrhage: a guideline from the American Heart Association/American Stroke Association. Stroke 53, e282–e361. doi: 10.1161/STR.0000000000000407
Habib, G., Lancellotti, P., Antunes, M. J., Bongiorni, M. G., Casalta, J. P., del Zotti, F., et al. (2015). 2015 ESC guidelines for the management of infective endocarditis: the task force for the Management of Infective Endocarditis of the European Society of Cardiology (ESC)endorsed by: European Association for Cardio-Thoracic Surgery (EACTS), the European Association of Nuclear Medicine (EANM). Eur. Heart J. 36, 3075–3128. doi: 10.1093/eurheartj/ehv319
Hemphill, J. C. 3rd, Greenberg, S. M., Anderson, C. S., Becker, K., Bendok, B. R., Cushman, M., et al. (2015). Guidelines for the Management of Spontaneous Intracerebral Hemorrhage: a guideline for healthcare professionals from the American Heart Association/American Stroke Association. Stroke 46, 2032–2060. doi: 10.1161/STR.0000000000000069
Hieber, M., Lambeck, J., Halaby, A., Roelz, R., Demerath, T., Niesen, W. D., et al. (2023). Minimally-invasive bedside catheter haematoma aspiration followed by local thrombolysis in spontaneous supratentorial intracerebral haemorrhage: a retrospective single-center study. Front. Neurol. 14:1188717. doi: 10.3389/fneur.2023.1188717
Horowitz, M. E., Ali, M., Chartrain, A. G., Allen, O. S., Scaggiante, J., Glassberg, B., et al. (2022). Definition and time course of pericavity edema after minimally invasive endoscopic intracerebral hemorrhage evacuation. J Neurointerv Surg. 14, 149–154. doi: 10.1136/neurintsurg-2020-017077
Ironside, N., Chen, C. J., Ding, D., Mayer, S. A., and Connolly, E. S. Jr. (2019). Perihematomal edema after spontaneous intracerebral hemorrhage. Stroke 50, 1626–1633. doi: 10.1161/STROKEAHA.119.024965
Jain, A., Malhotra, A., and Payabvash, S. (2021). Imaging of spontaneous intracerebral hemorrhage. Neuroimaging Clin. N. Am. 31, 193–203. doi: 10.1016/j.nic.2021.02.003
Kang, B. K., Na, D. G., Ryoo, J. W., Byun, H. S., Roh, H. G., and Pyeun, Y. S. (2001). Diffusion-weighted MR imaging of intracerebral hemorrhage. Korean J Radiol. 2, 183–191. doi: 10.3348/kjr.2001.2.4.183
Kellner, C. P., and Mocco, J. (2023). Long-awaited success on the intracerebral hemorrhage front. J Neurointerv Surg. 15, 937–938. doi: 10.1136/jnis-2023-020532
Kidwell, C. S., Chalela, J. A., Saver, J. L., Starkman, S., Hill, M. D., Demchuk, A. M., et al. (2004). Comparison of MRI and CT for detection of acute intracerebral hemorrhage. JAMA 292, 1823–1830. doi: 10.1001/jama.292.15.1823
Kidwell, C. S., Rosand, J., Norato, G., Dixon, S., Worrall, B. B., James, M. L., et al. (2017). Ischemic lesions, blood pressure dysregulation, and poor outcomes in intracerebral hemorrhage. Neurology 88, 782–788. doi: 10.1212/WNL.0000000000003630
Li, Q., Yakhkind, A., Alexandrov, A. W., Alexandrov, A. V., Anderson, C. S., Dowlatshahi, D., et al. (2024). Code ICH: a call to action. Stroke 55, 494–505. doi: 10.1161/STROKEAHA.123.043033
Linfante, I., Llinas, R. H., Caplan, L. R., and Warach, S. (1999). MRI features of intracerebral hemorrhage within 2 hours from symptom onset. Stroke 30, 2263–2267. doi: 10.1161/01.str.30.11.2263
Macellari, F., Paciaroni, M., Agnelli, G., and Caso, V. (2014). Neuroimaging in intracerebral hemorrhage. Stroke 45, 903–908. doi: 10.1161/STROKEAHA.113.003701
Morotti, A., and Goldstein, J. N. (2016). Diagnosis and Management of Acute Intracerebral Hemorrhage. Emerg. Med. Clin. North Am. 34, 883–899. doi: 10.1016/j.emc.2016.06.010
Mouchtouris, N., Saiegh, F. A., Chalouhi, N., Sweid, A., Papai, E. J., Wong, D., et al. (2021). Low diagnostic yield in follow-up MR imaging in patients with spontaneous intracerebral hemorrhage with a negative initial MRI. Neuroradiology 63, 1009–1012. doi: 10.1007/s00234-020-02570-1
Mould, W. A., Muschelli, J., Avadhani, R., McBee, N., Lane, K., Thompson, R., et al. (2019). Abstract 15: reduction in Perihematomal edema leads to improved clinical outcomes: results from the MISTIE III trial. Stroke 50:A15. doi: 10.1161/str.50.suppl_1.15
Murthy, S. B., Cho, S. M., Gupta, A., Shoamanesh, A., Navi, B. B., Avadhani, R., et al. (2020). A pooled analysis of diffusion-weighted imaging lesions in patients with acute intracerebral hemorrhage. JAMA Neurol. 77, 1390–1397. doi: 10.1001/jamaneurol.2020.2349
Murthy, S. B., Zhang, C., Gupta, A., Cho, S. M., Rivera-Lara, L., Avadhani, R., et al. (2021). Diffusion-weighted imaging lesions after intracerebral hemorrhage and risk of stroke: a MISTIE III and ATACH-2 analysis. Stroke 52, 595–602. doi: 10.1161/STROKEAHA.120.031628
Oliveira, I. M., Duarte, J., Dalaqua, M., Jarry, V. M., Pereira, F. V., and Reis, F. (2022). Cerebral venous thrombosis: imaging patterns. Radiol Bras. 55, 54–61. doi: 10.1590/0100-3984.2021.0019
Pradilla, G., Ratcliff, J. J., Hall, A. J., Saville, B. R., Allen, J. W., Paulon, G., et al. (2024). Trial of early minimally invasive removal of intracerebral hemorrhage. N. Engl. J. Med. 390, 1277–1289. doi: 10.1056/NEJMoa2308440
Rajashekar, D., and Liang, J. W. (2023). Intracerebral hemorrhage. Treasure Island, FL, USA: StatPearls.
Raposo, N., Zanon Zotin, M. C., Seiffge, D. J., Li, Q., Goeldlin, M. B., Charidimou, A., et al. (2023). A causal classification system for intracerebral hemorrhage subtypes. Ann. Neurol. 93, 16–28. doi: 10.1002/ana.26519
Rohaut, B., Doyle, K. W., Reynolds, A. S., Igwe, K., Couch, C., Matory, A., et al. (2019). Deep structural brain lesions associated with consciousness impairment early after hemorrhagic stroke. Sci. Rep. 9:4174. doi: 10.1038/s41598-019-41042-2
Romanova, A. L., Nemeth, A. J., Berman, M. D., Guth, J. C., Liotta, E. M., Naidech, A. M., et al. (2014). Magnetic resonance imaging versus computed tomography for identification and quantification of intraventricular hemorrhage. J. Stroke Cerebrovasc. Dis. 23, 2036–2040. doi: 10.1016/j.jstrokecerebrovasdis.2014.03.005
Sansing, L. H., Messe, S. R., Cucchiara, B. L., Lyden, P. D., and Kasner, S. E. (2011). Anti-adrenergic medications and edema development after intracerebral hemorrhage. Neurocrit. Care. 14, 395–400. doi: 10.1007/s12028-010-9498-z
Shah, V. A., Thompson, R. E., Yenokyan, G., Acosta, J. N., Avadhani, R., Dlugash, R., et al. (2022). One-year outcome trajectories and factors associated with functional recovery among survivors of intracerebral and intraventricular hemorrhage with initial severe disability. JAMA Neurol. 79, 856–868. doi: 10.1001/jamaneurol.2022.1991
Sporns, P. B., Psychogios, M. N., Boulouis, G., Charidimou, A., Li, Q., Fainardi, E., et al. (2021). Neuroimaging of acute intracerebral hemorrhage. J. Clin. Med. 10:1086. doi: 10.3390/jcm10051086
Unnithan, A. K. A., Joe, M. D., and Mehta, P. (2023). Hemorrhagic stroke. Treasure Island (FL): StatPearls.
Urday, S., Beslow, L. A., Goldstein, D. W., Vashkevich, A., Ayres, A. M., Battey, T. W. K., et al. (2015). Measurement of perihematomal edema in intracerebral hemorrhage. Stroke 46, 1116–1119. doi: 10.1161/STROKEAHA.114.007565
van Asch, C. J., Luitse, M. J., Rinkel, G. J., van der Tweel, I., Algra, A., and Klijn, C. J. (2010). Incidence, case fatality, and functional outcome of intracerebral haemorrhage over time, according to age, sex, and ethnic origin: a systematic review and meta-analysis. Lancet Neurol. 9, 167–176. doi: 10.1016/S1474-4422(09)70340-0
Weerink, L. B., Appelman, A. P., Kloet, R. W., and Van der Hoorn, A. (2023). Susceptibility-weighted imaging in intracranial hemorrhage: not all bleeds are black. Br. J. Radiol. 96:20220304. doi: 10.1259/bjr.20220304
Wijman, C. A., Venkatasubramanian, C., Bruins, S., Fischbein, N., and Schwartz, N. (2010). Utility of early MRI in the diagnosis and management of acute spontaneous intracerebral hemorrhage. Cerebrovasc. Dis. 30, 456–463. doi: 10.1159/000316892
Wilson, M., Wang, J. Y., Andreev, A., Katsanos, A. H., Selim, M., and Lioutas, V. A. (2023). Diagnostic utility of brain MRI in spontaneous intracerebral hemorrhage: a retrospective cohort study and meta-analysis. Eur. Stroke J. 8, 1089–1096. doi: 10.1177/23969873231192761
Wu, B., Yao, X., Lei, C., Liu, M., and Selim, M. H. (2015). Enlarged perivascular spaces and small diffusion-weighted lesions in intracerebral hemorrhage. Neurology 85, 2045–2052. doi: 10.1212/WNL.0000000000002169
Xu, X., Peng, S., Zhou, Y., Li, J., Tong, L., and Gao, F. (2022). Remote diffusion-weighted imaging lesions and blood pressure variability in primary intracerebral hemorrhage. Front. Neurol. 13:950056. doi: 10.3389/fneur.2022.950056
Keywords: intracerebral hemorrhage, magnetic resonance imaging, neuroimaging, computed tomography, hematoma
Citation: Penckofer M, Kazmi KS, Thon J, Tonetti DA, Ries C and Rajagopalan S (2024) Neuro-imaging in intracerebral hemorrhage: updates and knowledge gaps. Front. Neurosci. 18:1408288. doi: 10.3389/fnins.2024.1408288
Edited by:
Lauren Koffman, Temple University Hospital, United StatesReviewed by:
Ahmad Riad Ramadan, Henry Ford Health System, United StatesCopyright © 2024 Penckofer, Kazmi, Thon, Tonetti, Ries and Rajagopalan. This is an open-access article distributed under the terms of the Creative Commons Attribution License (CC BY). The use, distribution or reproduction in other forums is permitted, provided the original author(s) and the copyright owner(s) are credited and that the original publication in this journal is cited, in accordance with accepted academic practice. No use, distribution or reproduction is permitted which does not comply with these terms.
*Correspondence: Swarna Rajagopalan, cmFqYWdvcGFsYW4tc3dhcm5hQENvb3BlckhlYWx0aC5lZHU=