- 1Institut de Génétique et de Biologie Moléculaire et Cellulaire, Illkirch, France
- 2Centre National de la Recherche Scientifique, UMR7104, Illkirch, France
- 3Institut National de la Santé et de la Recherche Médicale, U964, Illkirch, France
- 4Strasbourg University, Illkirch, France
- 5I-Stem, Evry, France
- 6Genetic Diagnosis Laboratory, Strasbourg University Hospital, Strasbourg, France
- 7Institut Universitaire de France, Paris, France
Introduction: Mutations in dual-specificity tyrosine phosphorylation-regulated kinase 1A (DYRK1A) represent one of the most prevalent monogenic causes of neurodevelopmental disorders (NDDs), often associated with intellectual developmental disorder and autism spectrum disorder. DYRK1A encodes a dual-specificity kinase (tyrosine and serine/threonine) that plays a key role in various cellular processes and is a critical regulator of nervous system development.
Methods: For the first time, we have characterized the DYRK1A interactome and study the consequences of DYRK1A depletion in human neural stem cells (hNSCs).
Results: We identified 35 protein partners of DYRK1A involved in essential pathways such as cell cycle regulation and DNA repair. Notably, five of these interactors are components of the anaphase-promoting complex (APC), and one is an additional ubiquitin ligase, RNF114 (also known as ZNF313), which is known to target p21. Many of these identified partners are also linked to other human NDDs, and several others (e.g., DCAF7 and GSPT1) may represent novel candidate genes for NDDs. DYRK1A knockdown (KD) in hNSCs using siRNA revealed changes in the expression of genes encoding proteins involved in extracellular matrix composition and calcium binding (e.g., collagens, TGFβ2 and UNC13A). While the majority of genes were downregulated following DYRK1A depletion, we observed an upregulation of early growth factors (EGR1 and EGR3), as well as E2F2 and its downstream targets. In addition, DYRK1A-KD led to a reduction in p21 protein levels, despite an increase in the expression of a minor transcript variant for this gene, and a decrease in ERK pathway activation.
Discussion: Together, the DYRK1A interactome in hNSCs and the gene expression changes induced by its depletion highlight the significant role of DYRK1A in regulating hNSC proliferation. Although the effects on various growth signaling pathways may appear contradictory, the overall impact is a marked reduction in hNSC proliferation. This research underscores the pivotal role of DYRK1A in neurodevelopment and identifies, among DYRK1A’s protein partners and differentially expressed genes, potential novel candidate genes for NDDs and promising therapeutic targets for DYRK1A syndrome.
Introduction
Intellectual developmental disorder (IDD) and autism spectrum disorder (ASD) are two groups of neurodevelopmental disorders (NDDs) that share a significant genetic contribution and exhibit strong overlap both at the clinical and genetic levels. Single genetic events account for an important part of IDD cases and a non-negligible part of ASD cases. More than a thousand genes have been implicated in monogenic forms of IDD or ASD. For the majority of them, little is known about the pathophysiological mechanisms leading to IDD and ASD. Some genes are more frequently mutated than others, such as dual-specificity tyrosine phosphorylation-regulated kinase 1A (DYRK1A; Gonzalez-Mantilla et al., 2016). The first disruptions of DYRK1A were identified in individuals with intrauterine growth retardation, primary microcephaly, and epilepsy (Møller et al., 2008). The clinical spectrum associated with heterozygote mutations in DYRK1A was further refined with the publication of additional patient cases. This spectrum includes IDD, feeding difficulties, poor or absent language, microcephaly, autistic traits, epilepsy, and a typical facial gestalt (Courraud et al., 2021; van Bon et al., 2016; Bronicki et al., 2015). Loss-of-function mutations were also identified in cohorts of ASD individuals (O’Roak et al., 2012; Iossifov et al., 2014), but all have IDD. Interestingly, an increased dosage of DYRK1A, located on chromosome 21, is thought to participate in the cognitive manifestations of Down syndrome (Altafaj et al., 2001), suggesting that a correct balance of DYRK1A dosage is essential for brain development and cognitive function.
The DYRK1A gene codes a dual tyrosine-serine/threonine (Tyr-Ser/Thr) kinase protein that belongs to the DYRKs kinase family. Its main isoform comprises 753 amino acids (Becker, 2011). DYRK1A has the general structure of the DYRKs kinase family, including a DYRK Homology-box (DH) domain, two Nuclear Localization Signal sequences (NLS), and a central catalytic domain. The catalytic domain contains Tyrosine 321, which is involved in the activation of DYRK1A through autophosphorylation. Additionally, DYRK1A features a leucine zipper (bZIP) domain, suggesting it can form dimers or multimers with other nuclear proteins, such as transcription. It also includes Ser/Thr repeats that facilitate interaction with target proteins, a poly-histidine sequence that directs it to nuclear speckle compartments, and a PEST domain essential for its degradation and precise regulation of its cellular concentration. DYRK1A is ubiquitously expressed during embryonic development and in adults. Its location is both cytoplasmic and nuclear depending on the cell type and stage of development (Hämmerle et al., 2008). By the number and the diversity of its protein targets, DYRK1A regulates numerous cellular functions (Tejedor and Hämmerle, 2011; Duchon and Herault, 2016). DYRK1A regulates cytoskeleton-associated proteins, such as TAU, MAP1B, β-tubulin, and others, and has been shown to play a role in the regulation of dendritic morphogenesis in rodents and Drosophila (Ori-McKenney et al., 2016; Ryoo et al., 2007). DYRK1A modulates synaptic plasticity by regulating NMDA receptor expression at the membrane surface (Grau et al., 2014). DYRK1A interacts with proteins involved in endocytosis such as Dynamin1a or Amphiphysin1 (Murakami et al., 2006) and interacts with the light chain of Clathrin and Endophilin A1 (Murakami et al., 2009; Huang et al., 2004). DYRK1A phosphorylates several proteins involved in cell cycle regulation, such as cyclin D1 or p27Kip1, and therefore is involved in the regulation of mouse neural progenitor proliferation (Hämmerle et al., 2008). In addition to its cytoplasmic targets, DYRK1A also regulates numerous nuclear proteins: It interacts with and phosphorylates transcription factors such as acetyltransferases CBP and p300 (Li et al., 2018) and binds the chromatin remodeling SWI/SNIF complex (Lepagnol-Bestel et al., 2009), Histone H3 (Jang et al., 2014), and RNA polymerase type II (Di Vona et al., 2015). On the one hand, DYRK1A positively regulates CREB (Yang et al., 2001) and GLI1 (Ehe et al., 2017) activity, and the nuclear translocation of NFAT (Gwack et al., 2006) and, on the other hand, negatively regulates REST complex stability (Lu et al., 2011). We recently demonstrated that loss-of-function variants in DYRK1A induce specific changes in DNA methylation in blood, consistent with a role in chromatin remodeling (Courraud et al., 2021). Other nuclear substrates of DYRK1A have been reported, including splicing factors (Shi et al., 2008).
Although numerous studies have unraveled some of the functions of DYRK1A, the majority of the work about its role in the brain has been performed in mice (Fotaki et al., 2002; Fotaki et al., 2004), Drosophila (Fischbach and Heisenberg, 1981; Tejedor et al., 1995), or, more recently, in Xenopus models (Blackburn et al., 2019; Willsey et al., 2020). Therefore, little is known about its role in human neural cells and about which of its cellular functions are critical for early human brain development and functioning. To characterize the role of DYRK1A in human neuronal progenitors, we performed proteomic studies to identify its interactome as well as transcriptomic analysis to identify changes in gene expression occurring after its knockdown (KD). We also analyzed the effect of DYRK1A-KD on hNSC ability to proliferate.
Materials and methods
Cell culture, transfection, and proliferation assay
Human neuronal stem cells (hNSCs) from two genetic backgrounds (SA001/hNSC1 and GMO1869/hNSC2) were obtained from I-Stem and are described in previous studies (Boissart et al., 2012; Courraud et al., 2023). hNSCs were seeded on poly-ornithine-and laminin-coated dishes and maintained in N2B27 medium (DMEM/F12 and Neurobasal medium [1:1] supplemented with N2, B27, 2-mercaptoethanol [all from Invitrogen]), BDNF (20 ng/mL), FGF-2 (10 ng/mL; both from PeproTech), and EGF (R&D Systems; 10 ng/mL). Culture and quality controls of hNSCs were performed as described in Quartier et al. (2018). hNSCs were transfected using INTERFERin reverse transfection protocol (Polyplus-transfection), with Scramble siRNA, a pool of DYRK1A siRNA (120 nM final concentration), or the transfecting agent only. Cells were harvested 48 h after transfection for RNA and protein extractions. All cell lines were grown at 37°C in 5% CO2. For proliferation assay, reverse transfection of hNSCs (20,000 cells/cm2) was performed in 96-well plates using INTERFERin reagent and siRNA according to the manufacturer’s recommendations. Each day, a plate was fixed and stained with DAPI, and the number of nuclei in each condition was counted using CellInsight automated microscope and HCS Studio software (Thermo Fisher Scientific).
Immunoprecipitation coupled to mass spectrometry
Proteins extracted from untreated hNSC1 were immunoprecipitated with DYRK1A antibodies targeting the N-terminal and C-terminal regions of the protein, respectively (Cohesion Biosciences #CPA1357, Immunogen sequence aa 39-51 and Abnova H00001859-M01, aa 674–763). As negative controls, we used free beads without antibodies as well as mouse anti-rabbit (MAR, 211–002-171, Jackson ImmunoResearch) and rabbit anti-mouse (RAM, 315–005-044, Jackson ImmunoResearch) antibodies as described (Mattioli et al., 2019). Immunoprecipitations were validated using Western blot as previously described (Mattioli et al., 2019) before the mass spectrometry analyses (Proteomic platform, IGBMC). In brief, the samples were treated with LysC/trypsin for digestion and injected into Orbitrap ELITE/C18 Accucore 50 cm (20 μL 0.1%TFA/1 μL) for 2 h runs in triplicate. Data were processed using Proteome Discoverer 2.2 software with Homosapiens_190716_reviewed. fasta and contaminants_190528.fasta databases. Thresholds were set at 1% FDR with a minimum of two unique peptides per protein. To consider a protein as a candidate interactor, we applied the thresholds to keep only proteins with (1) peptide-spectrum matching (PSM) sum of any of the control conditions (Beads, MAR, GAR) inferior to 5, (2) a sum of PSM for the three replicates of the test conditions (DYRK1A C-term or DYRK1A N-term) greater or equal to 5 with a positive value for each replicate, and (3) a ratio of NSAF between anti-DYRK1A and control conditions ≥1.5. Enrichment analysis was realized using Database for Annotation, Visualization, and Integrated Discovery (DAVID) using proteins detected in the mass spectrometry experiments (minimum of two peptides per protein) as background. The list of already known DYRK1A interactors was obtained from BioGRID and from previously published proteomic studies (Varjosalo et al., 2013; Li et al., 2015; Menon et al., 2019; Roewenstrunk et al., 2019; Guard et al., 2019; Huttlin et al., 2021). Network analysis was performed using Poteo3Dnet.1 Information about pathologies caused by mutations in genes encoding DYRK1A interactors was retrieved using Human Phenotype Ontology. The probability of the genes being intolerant to the loss-of-function variant was obtained from GnomAD (pLI). Gene expression profiles during brain development were obtained from BrainSpan data and were correlated with the DYRK1A expression profile (Pearson’s correlation).
Western blot
Cells were lysed in RIPA buffer (50 mM Tris–HCl pH 7.5, 150 mM NaCl, 0.25% sodium deoxycholate, 1% NP-40) supplemented with protease inhibitor cocktail and phosphatase inhibitor cocktail. A total of 5 to 50 μg of protein lysate was separated on 10% SDS-PAGE and transferred to a polyvinylidene fluoride (PVDF) membrane. Membranes were blocked in 5% non-fat dry milk diluted in tris-buffered saline with tween 20 (50 mM Tris, 150 mM NaCl, 0.05% Tween 20) and probed using the antibodies overnight at 4°C. GAPDH was used as a loading control. Incubation with appropriate HRP-conjugated secondary antibody was followed by detection using Immobilon Western Chemiluminescent HRP Substrate (Merck Millipore, Darmstadt, Germany). The antibodies used were anti-GPADH (G9545 Sigma Aldrich), anti-DYRK1A N-ter (CPA1357 Cohesion Biosciences), anti-DYRK1A C-ter (H00001859-M01 Abnova), pan ERK1/2 (4696S Cell signaling technology), p21/CDKN1A (2947S Cell signaling technology), PTBP2 (H00058155-M01 VWR International), RNF114 (Fisher Scientific, PA5-112261), phospho ERK1/2 (9,101 Cell signaling technology), pan mTOR (2,972 Cell signaling technology), phospho mTOR (2,974 Cell signaling technology), pan PKC (P5704 clone MC5 Sigma), and phospho PKCα (sc-208 Santa Cruz).
RNA sequencing and RT-qPCR
RNAs were extracted from two series (biological replicates) of hNSC1 treated with siRNA targeting DYRK1A, Scramble siRNA, or transfection agent alone for 48 h and sequenced as previously described (Mattioli et al., 2020). DESeq was used to detect changes in gene expression, and splicing changes were identified using LeafCutter. Genes with a change in mRNA level with an adjusted p-value of <0.1 were considered differentially expressed genes (DEG). Gene ontology enrichment was performed using DAVID tools on all the DEG using all the genes expressed in hNSCs as background (number of reads normalized and divided by the median of transcripts length in kb >50). A list of E2F and TGFB1 targets was retrieved from the MSigDB Molecular Signatures Database.2 Expression changes were confirmed for the most significant DEG in a third series of hNSC1 and in three series (biological replicates) of hNSC2: After RNA isolation, reverse transcription reactions (Invitrogen SuperScript IV) were performed using 200–500 ng RNA, followed by qPCR on cDNA on LightCycler 480 II (Roche) using the QuantiTect SYBR Green PCR Master Mix (Qiagen) or RT-qPCR multiplex using 48*48 array Fluidigm Biomark technology (GenomEast platform). A list of primers is available upon request.
SA-β galactosidase assay and cell irradiation
Cells were fixed 4 days after siRNA transfections with 0.5% glutaraldehyde solution for 15 min at room temperature and washed twice with PBS/MgCl2 pH 5.95. Fixed cells were incubated with freshly prepared X-gal staining solution (1 mg/mL X-gal), 5 mM potassium ferrocyanide, and 5 mM potassium ferricyanide in PBS/MgCl2 pH 6 at 37°C for 20 to 48 h. Cells were washed three times with H2O and pictured in a bright field (20X magnification). Counting was done manually using the Cell Counter plugin in FIJI software, and 1,400 to 3,200 cells were counted for each condition. As a positive control of SA-β galactosidase labeling, a batch of untreated cells was irradiated at 8Gy using the “CellRad System Precision,” then fixed and labeled as previously described.
Results
Characterization of DYRK1A interactome in human neural stem cells
DYRK1A interactome has been studied in different cell types, including HeLa, HEK293T, human glioblastoma T98G, and human neuroblastoma SH-SY-5Y cell lines (Varjosalo et al., 2013; Li et al., 2015; Menon et al., 2019; Roewenstrunk et al., 2019; Guard et al., 2019; Huttlin et al., 2021), which led to a list of 552 interactors (BioGRID). To identify protein partners of DYRK1A in human neural stem cells (hNSC1), we performed immunoprecipitations on endogenous DYRK1A using antibodies recognizing either the C-terminal (Cter) or the N-terminal (Nter) parts of the protein. Mass spectrometry analysis of immunoprecipitated proteins (IP-MS) revealed, in addition to DYRK1A, 35 protein interactors with 7 detected with both antibodies. Among them, 15 had previously been identified as DYRK1A partners in other cell types in the literature, and 20 were novel interactors (Table 1; Figure 1A). Phosphorylation analysis on IP-MS data identified phosphorylation events on DYRK1A (Tyr321) and in three partners: FAM117B (Ser106), GLCCI1 (Ser30 and Ser303), and FAM53C (Ser324). The partners identified were significantly enriched in proteins involved in the regulation of cell cycle and protein ubiquitination (Figure 1B), such as for instance members of the anaphase-promoting complex (APC) but also RNF114, alias ZNF313, a zinc-finger E3 ligase involved in cell cycle progression and senescence repression known to ubiquitinate p21/CDKN1A. Among the five members of the APC identified as DYRK1A’s partners in hNSC, three (CDC27/ANAPC3, ANAPC4, and CDC23/ANAPC8) had already been identified in previous studies (Lepagnol-Bestel et al., 2009; Li et al., 2018) but two (CDC16/ANAPC6 and ANAPC7) had not previously been described as DYRK1A interactors (Figure 1A; Supplementary Figure S1). Given that all these proteins are components of the APC complex, immunoprecipitation likely captures the entire complex rather than reflecting direct interactions between DYRK1A and each subunit. Apart from APC, additional clusters of interactions of proteins involved in DNA repair, mRNA regulation at the transcriptional and posttranscriptional level, and intracellular signaling pathways were also identified (Supplementary Figure S1).
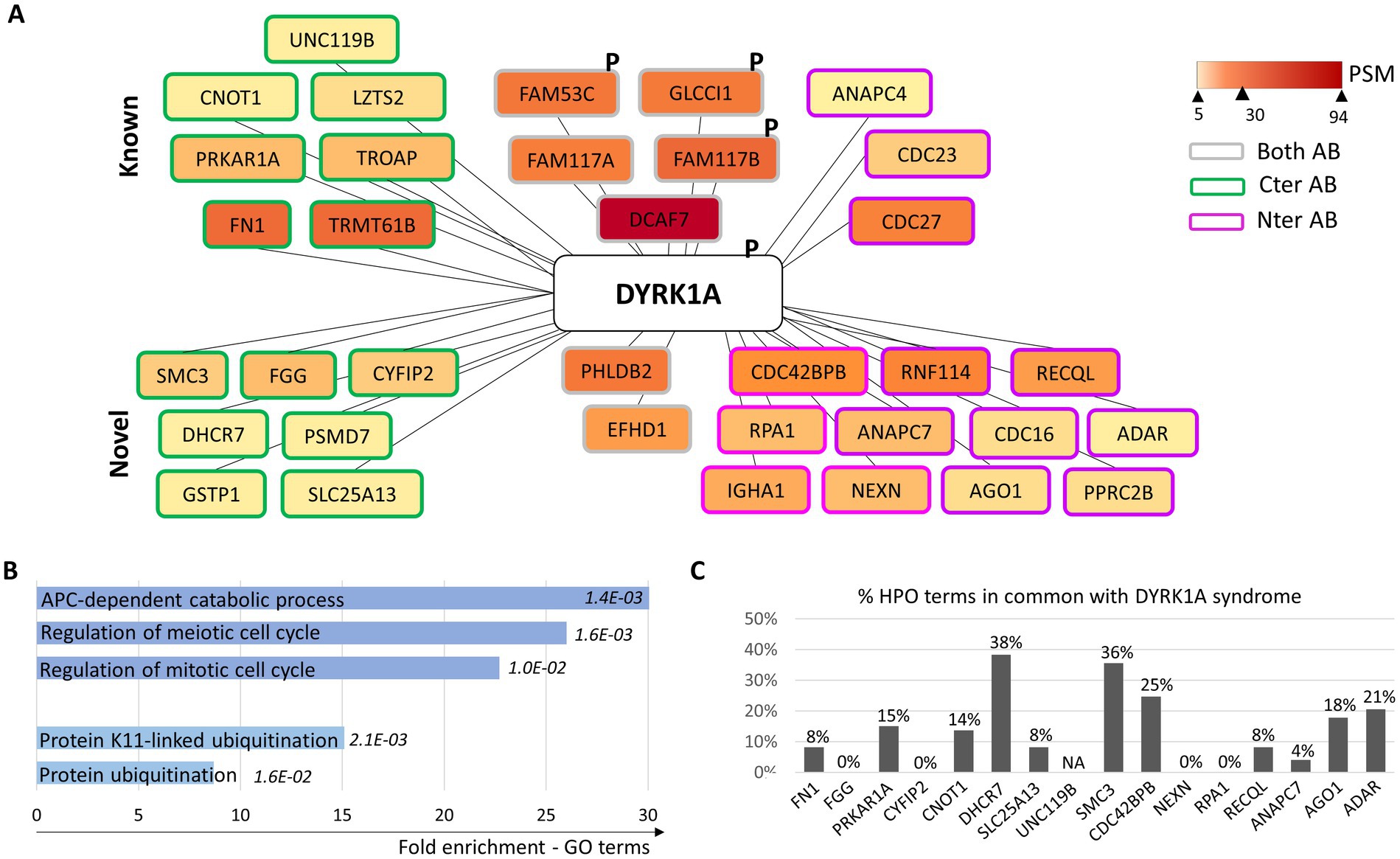
Figure 1. Representation of DYRK1A protein interactome in human neural stem cells. (A) Protein interactome of DYRK1A: boxes are filled with a yellow to dark red gradient corresponding to the number of peptide-spectrum matching (PSM) values (see Supplementary Table S1); proteins found using the anti-DYRK1A antibody directed against the C-terminal part of the protein are circled in green, those found using the one directed against the N-terminal part in pink, and those found with both antibodies in gray; “P” indicates that phosphorylation events have been identified. (B) Significant enrichment in GO terms among DYRK1A interactors. (C) Number of Human Phenotype Ontology (HPO) terms of DYRK1A syndrome reported in the diseases associated with DYRK1A’s partners.
We compared the expression of DYRK1A and its partners during brain development across the different brain structures (data from BrainSpan) and found a moderate-to-high positive correlation (Pearson’s coefficient) for one-third of them (11/35; Table 1). Half of DYRK1A’s partners identified in hNSCs (16/35) are known to be involved in human diseases, with neurodevelopmental manifestations for nine of them (PRKAR1A, CYFIP2, CNOT1, DHCR7, SMC3, CDC42BPB, ANAPC7, AGO1, and ADAR; Table 1). Interestingly, of the 73 clinical signs listed in the Human Phenotype Ontology (HPO) as part of DYRK1A syndrome, 80% are also described in diseases caused by mutations in partners of DYRK1A (Supplementary Figure S2). The diseases caused by pathogenic variants in DHCR7, SMC3, and CDC42BPB show the strongest overlap as they share more than 25% of the clinical signs of DYRK1A syndrome (Figure 1C). Among DYRK1A’s newly described partners that are not yet linked to human pathology, some exhibit high intolerance to loss-of-function (LoF) variants (high pLI in the gnomAD database) and present an expression profile during brain development that strongly correlates with DYRK1A. This suggests that these partners could be relevant candidates for NDDs and may contribute to the pathophysiology of DYRK1A syndrome. Notable examples include the well-known partner DCAF7 (pLI = 0.93, Pearson’s correlation = 0.81) as well as GSPT1, a GTP-binding protein involved in translation termination and the regulation of cell cycle progression (1; 0.85).
DYRK1A KD in human neural stem cells affects the expression of genes encoding extracellular matrix proteins
DYRK1A has previously been described as both a direct and indirect transcriptional regulator. Indeed, DYRK1A has been shown to modulate chromatin remodeling complexes and epigenetic marks (Lepagnol-Bestel et al., 2009; Li et al., 2018) and also interacts with various transcription factors such as NFAT (Gwack et al., 2006) and GLI1 (Ehe et al., 2017) and with RNA Pol II (Yu et al., 2019). To identify the effect of DYRK1A loss on the regulation of gene expression in hNSCs, we used a pool of siRNAs to knock down (KD) DYRK1A expression in hNSC1 (Supplementary Figure S3) and performed mRNA sequencing. This transcriptomic analysis revealed 91 significantly deregulated protein-coding genes (DEG) following DYRK1A-KD compared to the control condition, with 85 of these genes being downregulated; DYRK1A was the second most significantly downregulated gene (log2 fold change = −0.87, adjusted p-value =1.10E-05; Figure 2A; Supplementary Tables S2, S3). In comparison, no DEGs were found in hNSC1 treated with non-specific siRNA (Scramble). Uniprot and GO terms analyses revealed an enrichment of genes related to extracellular matrix (ECM) components and the plasma membrane (e.g., COL6A1, COL6A3, LUM, and THBS2), as well as calcium binding (e.g., CALB1, SGC2, and UNC13A) among the DEGs (Figure 2B). Only six genes (EGR1, EGR3, E2F2, HMRB2 and TYMS) were found to be significantly upregulated. Among them, two were members of the early growth factor (EGR) transcription factors families, EGR1 and EGR3. We then checked the expression of the most significant DEGs using multiplex RT-qPCR in a new series of the same hNSC line (hNSC1) and three series of an additional hNSC line from another genetic background (hNSC2) treated by DYRK1A siRNA. We confirmed significant changes in gene expression in both cell lines for 23 genes (Figure 2C). No significant motif enrichment was found in the promoter region (−1,000/+100 bp) of the DEGs using the Analysis of Motif Enrichment, version 5.1.1 (AME) tool. Among the DEGs, strong evidence of involvement in neurodevelopmental disorders was reported for 15 of them (“definitive” gene list from SysNDD, e.g., PLP1, RGS6, and SCN3A) and moderate evidence for 10 more (“limited” gene list from SysNDD, e.g., UNC13A, NMNAT2, and CHL1). Some of the DEG are not yet associated with human disease but are highly intolerant to LoF. Among them, PTBP2 has a high pLI and exhibits a strong expression correlation with DYRK1A during brain development (Pearson’s correlation = 0.8), suggesting that it could be a novel candidate gene for NDDs. Interestingly, if an increase of PTPB2 mRNA was observed after DYRK1A KD in both hNSC lines, a significant decrease in protein level was observed (Supplementary Figure S4). The same observation was made for the gene CDKN1A, which encodes the p21 protein, as a decrease in protein level was detected after DYRK1A KD, while an increase of mRNA was detected using Leafcutter, a program designed to detect changes in exon/intron ratios (Supplementary Table S5; Figure 3A; Supplementary Figure S5), affecting a weakly expressed non-canonical isoform with unknown function (NM_001291549.1). An overexpression of E2F2 mRNA was observed, which prompted us to analyze the expression of all known target genes of the E2F signaling pathway. Among the 200 genes belonging to this molecular signature, 91% were overexpressed in the DYRK1A-KD condition (Supplementary Figure S6), suggesting incomplete repression of E2F-mediated gene transcription, similar to what has been reported in human lymphocytes (Thompson et al., 2015). In contrast, we checked the expression of TGFB target genes as both TGFB2 and TGFBI mRNA were found to significantly decrease after DYRK1A-KD and observed that they are mainly downregulated (42/54 with a log2FC <0), highlighting repression of the TGFβ pathway after DYRK1A loss.
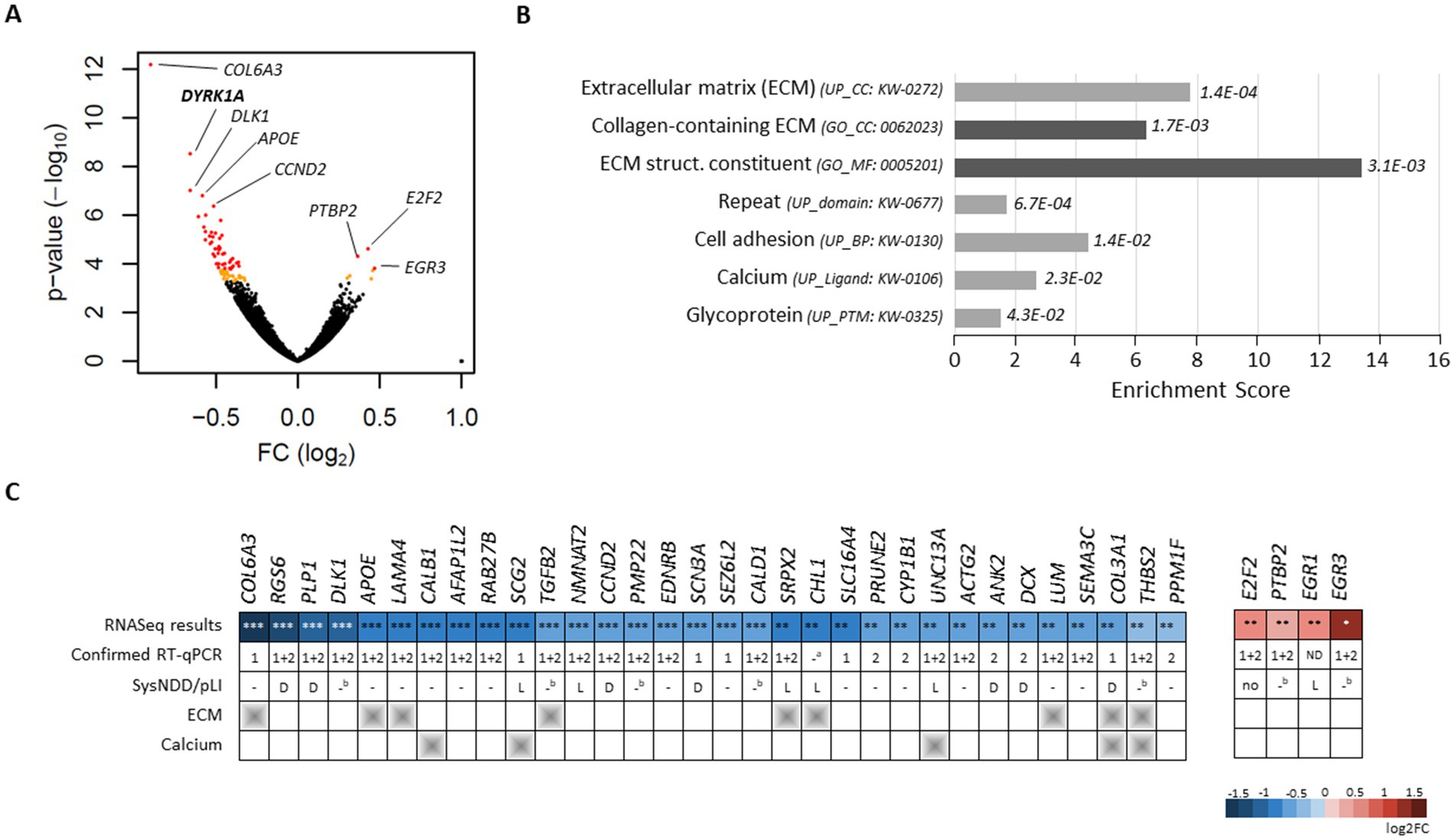
Figure 2. Transcriptomic changes induced by DYRK1A knockdown in human neural stem cells. (A) Volcano plot showing RNA sequencing data (control vs. siDYRK1A treated hNSC1) with the significance (−log10 of the p-value) as a function of the log2 fold change. Genes not significantly deregulated are shown in black; genes significantly deregulated (DEG) with an adjusted p-value between 0.05 and 0.1 are shown in orange, while genes with an adjusted p-value <0.05 are shown in red. (B) Pathways analysis using Uniprot and Gene Ontology terms revealed significant enrichment in proteins from the extracellular matrix (ECM), involved in cell adhesion and binding calcium; CC, cellular component; MF, molecular function, BP, biological process; PTM, posttranslational modification. (C) Representation of RNA-Seq results for a subset of DEG: RNAseq results including adjusted p-value (*p-value<0.1, **p-value<0.05, and ***p-value<0.01) and log2 fold change (color intensity), RT-qPCR analysis performed in a third series of hNSC1 (n = 3) and in three series of hNSC2 (n = 9; normalized on GAPDH and YWHAZ); significant change in gene expression confirmed in hNSC1 (1), hNSC2 (2), or both (1 + 2). Genes involved in neurodevelopmental disorders (NDD) are retrieved from the “Definitive” (D) and “Limited” (L) gene lists of SysNDD database. b, gene not already associated with an NDD but having a high intolerance to loss-of-function in the gnomAD database (pLI > 0.9).
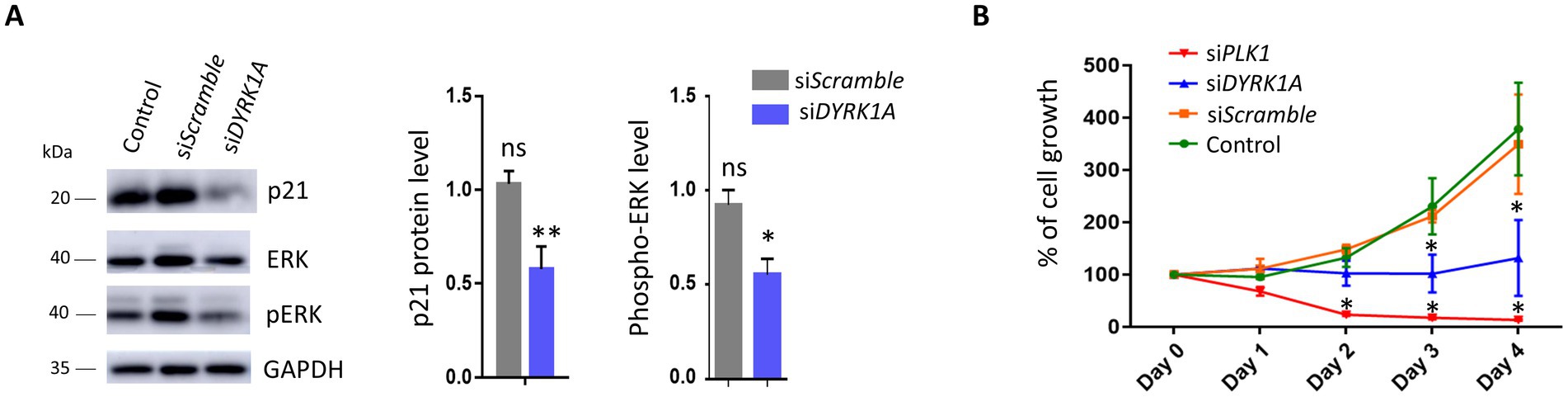
Figure 3. DYRK1A knockdown affects human neural stem cell proliferation. (A) Western blot analysis on total protein extract from hNSC1 treated with lipofectant alone (Control) or transfected with Scramble or DYRK1A siRNA for 48 h. The level of p21 protein (n = 3) and ERK and phosphoERK (pERK; n = 3) was normalized to the GAPDH level. Multiple comparison tests were performed using a one-way ANOVA test with Dunnett’s correction: ns: not significant; **: adjusted p-value<0.01; *: adjusted p-value<0.05; errors bar represent standard error of the mean (SEM); (B) proliferation assay performed on hNSC1 treated with lipofectant alone (Control), transfected with Scramble siRNA, DYRK1A siRNA (siDYRK1A). A treatment with PLK1 siRNA (siPLK1) was used as a positive control, leading to cell apoptosis. At each time point (days 0, 1, 2, 3, and 4), cells were counted and data normalized with day 0 (n = 3 per line). Student’s t-test comparison was done comparing to Control condition: *p-value<0.05; error bars represent SEM.
DYRK1A inactivation reduces human neural stem cell proliferation and ERK activation
The interaction of DYRK1A with APC members and other proteins involved in cell cycle regulation, as well as the changes in expression of p21, E2F, and others identified after DYRK1A-KD suggest a role of DYRK1A in the proliferation of hNSCs, consistent with what was observed in other cell types (Park et al., 2010). Therefore, we analyzed the ability of hNSCs to proliferate after DYRK1A-KD and observed a significant decrease in cell proliferation 72 h following DYRK1A-KD in hNSCs, compared to a control condition or Scramble siRNA (Figure 3B; Supplementary Figure S7A). Because of the implication of p21/CDKN1A in cellular senescence, we tested whether DYRK1A-KD affects the number of cells undergoing senescence using the SA-β galactosidase assay 4 days post-transfection. Counting revealed that few cells turned positive after SA-β galactosidase labeling and that neither siScramble nor siDYRK1A treatments appeared to increase the number of senescent cells (Supplementary Figure S8). As one of the novel DYRK1A partners we identified, RNF114 is a ubiquitin ligase that regulates cell cycle progression by degrading p21 (Han et al., 2013). We tested the consequences of its KD in hNSCs (Supplementary Figure S9A). However, if a decrease in p21 protein levels was observed after RNF114-KD (Supplementary Figure S9B), no obvious effect on proliferation was observed (Supplementary Figure S8C), suggesting that the decrease in hNSC proliferation caused by DYRK1A-KD is not mediated by its interaction with RNF114. Recently, a study conducted in a conditional Dyrk1a heterozygous mouse model identified several alterations in growth signaling cascades, such as ERK and mTOR (Levy et al., 2021). Interestingly, a significant decrease in ERK phosphorylation was observed in hNSCs 48 h after treatment with siDYRK1A (Figure 3A).
Discussion
Our study contributes to a growing understanding of the molecular role of DYRK1A in human neural development, using the model of human neural stem cells (hNSCs), by characterizing its interactome, its impact on gene expression, and its regulatory functions on cellular processes such as proliferation. The findings shed light on how DYRK1A interacts with proteins or regulates the expression of genes known to be involved in neurodevelopmental disorders (NDDs). Additionally, they highlight other genes that, due to their intolerance to loss-of-function and expression profiles during brain development, emerge as strong candidates for involvement in NDDs.
Through immunoprecipitation and mass spectrometry (IP-MS), we identified 35 DYRK1A interactors in human neural stem cells, with an important overlap with those previously identified in other cell types (15/35), highlighting a strong conservation of DYRK1A interactions across different tissues. These findings show that DYRK1A engages with multiple proteins involved in cell cycle regulation, transcriptional control, and signaling pathways critical to neurodevelopment. At the intersection of these different biological processes lies the large E3 ubiquitin ligase machinery of the anaphase-promoting complex (APC; Pal and Summers, 2017; Skaar and Pagano, 2009), with five of its members (ANAPC3, 4, 6, 7, and 8) identified as interacting with DYRK1A. Recently, another study also highlighted DYRK1A interaction with three APC factors (ANAPC3/CDC27, ANAPC4, and ANAPC8/CDC23; Guard et al., 2019), which we validated in this study. Interestingly, four of these core subunits (ANAPC3-6-7 and 8) compose the tetratricopeptide repeat (TPR) lobe APC subcomplex, playing a role in the global scaffolding of the complex and substrate recognition (Sivakumar and Gorbsky, 2015). This would suggest that DYRK1A may interact with APC through the TPR lobe and given the kinase activity of DYRK1A, could possibly phosphorylate the subunit and therefore play a role in the global activity of the complex. The pronounced enrichment of ANAPC3/CDC27 suggests it may be the primary interaction partner of DYRK1A. Another ubiquitin E3 ligase, RNF114/ZNF313, playing a role in the regulation of cell cycle and repression of senescence, was detected in our study. Interestingly, although this gene is not directly involved in NDD, a polymorphism affecting its expression is associated with an increased risk of developing psoriasis (Capon et al., 2008). Notably, we recently showed that inflammatory skin conditions are frequent in individuals with DYRK1A syndrome (Courraud et al., 2021). RNF114 ubiquitinates p21/CDKN1A, leading to its degradation (Han et al., 2013). Surprisingly, its knockdown in hNSCs leads to a decrease in p21 protein levels, like what is observed with DYRK1A knockdown, while, unlike DYRK1A knockdown, it has no effect on the proliferation of hNSCs. These results indicate that the downregulation of RNF114 and p21 proteins alone is not sufficient to cause the decreased proliferation identified in the DYRK1A-KD condition.
Importantly, several DYRK1A interactors identified in our study exhibit significant expression correlation with DYRK1A during brain development. Some are mutated in NDDs, supporting their relevance in neurodevelopmental pathways. The dysfunction of these interacting proteins may contribute to the shared clinical manifestations of DYRK1A-related disorders (such as short stature, microcephaly, strabismus, and scoliosis). Others are not associated yet with any human disease but have a high intolerance to loss-of-function (LoF) variants, which warrants further investigation for their potential implication in NDDs. It is the case for instance for GSPT1 or DCAF7, for which de novo missense variants classified as “probably deleterious” have been identified in individuals with NDDs (Supplementary Table S5). The identification of additional patients will be helpful in confirming these genes as novel NDD genes.
We identified genes differentially expressed in hNSCs after a transient DYRK1A knockdown (KD) showing little overlap with previous transcriptomic studies conducted in murine B and T cells (Thompson et al., 2015), zebrafish brain (Cho et al., 2019), xenopus brain (Willsey et al., 2020), and human HeLa cells (Vona et al., 2015). In hNSCs, DYRK1A-KD resulted in the dysregulation of genes encoding proteins associated with the extracellular matrix (ECM) and calcium-binding proteins. Many of these calcium-binding ECM proteins, such as COL3A1 and THBS2, play roles in cellular adhesion and structure, potentially impacting neural cell interactions and therefore the balance between proliferation and differentiation during brain development. Interestingly, mutations in these two genes are responsible for a disorder of connective tissues, Ehlers-Danlos syndrome, a connective tissue disorder characterized by fragile skin, which is also frequently observed in DYRK1A syndrome.
Only a few genes were found significantly upregulated after DYRK1A-KD. Among them, PTBP2, an RNA-binding protein, regulates alternative splicing, polyadenylation, mRNA stability, and translation and plays a role in neural development by regulating alternative splicing in brain tissues (Hu et al., 2018). Interestingly, despite the significant upregulation of PTBP2 mRNA following DYRK1A-KD, we observed a marked decrease in PTBP2 protein expression. Similar results were obtained for CDKN1A, where the mRNA level of a long minor isoform (potentially encoding a protein that is 34 amino acids longer than the main isoform, with an unknown function) increased after DYRK1A-KD, while the amount of P21/CDKN1A protein was significantly reduced. These findings suggest that DYRK1A-KD may lead to decreased protein stability or degradation, triggering compensatory mechanisms that result in increased transcription of the corresponding mRNA.
On the one hand, DYRK1A interacts with proteins and complexes involved in cell proliferation regulation, such as APC; conversely, DYRK1A-KD impacts several signaling pathways critical for cell proliferation. These include increased expression of the transcription factor E2F2 and their target genes, elevated expression of EGR genes, reduced expression of the growth factor TGFβ, decreased levels of the p21 protein, and diminished ERK activation. Although these effects on distinct growth signaling pathways appear contradictory, we demonstrated that the overall impact of DYRK1A-KD is a significant reduction in hNSC proliferation. The majority of previous published studies report decreased proliferation associated with DYRK1A-KD, as observed in Drosophila (Tejedor and Hämmerle, 2011) or in mouse models (Laguna et al., 2013; Guedj et al., 2012; Barallobre et al., 2014). DYRK1A’s role in neuronal precursor proliferation has been recognized since its discovery in the Drosophila mnb model (Fischbach and Heisenberg, 1981), where reduced cell numbers and brain size were observed in mutant flies. While DYRK1A has been identified as both a positive and negative regulator of cellular proliferation depending on the context, reduced DYRK1A expression generally results in decreased cell proliferation across various models. For example, heterozygous KO Dyrk1a +/− drosophila, mice, zebrafish, or xenopus showed reduced brain size (Fotaki et al., 2002; Willsey et al., 2020; Tejedor et al., 1995; Kim et al., 2017) attributed to decreased neuronal precursor proliferation during neurogenesis. The effect of DYRK1A inhibition on hNSC proliferation had not been explored prior to our study. However, Bellmaine et al. highlighted that DYRK1A inhibition disrupts neural specification in human embryonic stem cells (ESCs; Bellmaine et al., 2017). Future studies employing non-transient inactivation of DYRK1A, such as hNSCs differentiated from induced pluripotent stem cells with a loss-of-function mutation in DYRK1A, could provide further insights into how DYRK1A loss affects hNSC proliferation (cell cycle elongation, increase of apoptosis, etc.) and differentiation.
In conclusion, this study enhances the understanding of DYRK1A’s multifaceted role in human neurodevelopment. By characterizing DYRK1A’s interactome in human neural stem cells, its effects on gene expression, and its functional impact on proliferation, we reveal critical insights into its role in brain development and the molecular basis of associated neurodevelopmental disorder. Future research could explore therapeutic modulation of DYRK1A activity or its interactors, providing new avenues for intervention in DYRK1A syndrome. Our findings emphasize the importance of balanced DYRK1A expression in neurodevelopment and support its continuing study as a central player in the complex network underlying cognitive development and function.
Data availability statement
The datasets presented in this study can be found in online repositories Gene Expression Omnibus: GSE247739. The names of the repository/repositories and accession number(s) can be found in the article/Supplementary material.
Ethics statement
The studies involving humans were approved by the cells are coming from Istem, this research is authorized by ABM. The studies were conducted in accordance with the local legislation and institutional requirements. The human samples used in this study were acquired from gifted from another research group. Written informed consent for participation was not required from the participants or the participants’ legal guardians/next of kin in accordance with the national legislation and institutional requirements.
Author contributions
JC: Conceptualization, Formal analysis, Investigation, Methodology, Writing – original draft. AQ: Formal analysis, Methodology, Writing – review & editing. ND: Formal analysis, Writing – review & editing. IZ-B: Formal analysis, Writing – review & editing. JG: Formal analysis, Writing – review & editing. AB: Writing – review & editing, Methodology, Resources. J-LM: Writing – review & editing, Investigation. AP: Investigation, Writing – review & editing, Conceptualization, Data curation, Formal analysis, Funding acquisition, Methodology, Project administration, Resources, Validation, Writing – original draft.
Funding
The author(s) declare that financial support was received for the research and/or publication of this article. This study received funding from the Fondation de France and Fondation APLM.
Acknowledgments
The authors would like to thank the families for their participation and support. The authors also thank the Fondation de France and Fondation APLM for financial support. We also thank people from the GenomEast platform, especially Céline Keime, Damien Plassard, Christele Thibaut-Carpentier, and Bernard Jost, as well as Bastien Morlet from the mass spectrometry and high-throughput screening platforms.
Conflict of interest
The authors declare that the research was conducted in the absence of any commercial or financial relationships that could be construed as a potential conflict of interest.
Generative AI statement
The authors declare that Gen AI was used in the creation of this manuscript. ChatGPT to correct errors in English language.
Publisher’s note
All claims expressed in this article are solely those of the authors and do not necessarily represent those of their affiliated organizations, or those of the publisher, the editors and the reviewers. Any product that may be evaluated in this article, or claim that may be made by its manufacturer, is not guaranteed or endorsed by the publisher.
Supplementary material
The Supplementary material for this article can be found online at: https://www.frontiersin.org/articles/10.3389/fnins.2025.1533253/full#supplementary-material
Footnotes
References
Altafaj, X., Dierssen, M., Baamonde, C., Martí, E., Visa, J., Guimerà, J., et al. (2001). Neurodevelopmental delay, motor abnormalities and cognitive deficits in transgenic mice overexpressing Dyrk1A (Minibrain), a murine model of Down’s syndrome. Hum. Mol. Genet. 10, 1915–1923. doi: 10.1093/hmg/10.18.1915
Barallobre, M. J., Perier, C., Bové, J., Laguna, A., Delabar, J. M., Vila, M., et al. (2014). DYRK1A promotes dopaminergic neuron survival in the developing brain and in a mouse model of Parkinson’s disease. Cell Death Dis. 5:e1289. doi: 10.1038/cddis.2014.253
Becker, W. (2011). Recent insights into the function of DYRK1A. FEBS J. 278:222. doi: 10.1111/j.1742-4658.2010.07953.x
Bellmaine, S. F., Ovchinnikov, D. A., Manallack, D. T., Cuddy, C. E., Elefanty, A. G., Stanley, E. G., et al. (2017). Inhibition of DYRK1A disrupts neural lineage Specificationin human pluripotent stem cells. eLife 6:e24502. doi: 10.7554/eLife.24502
Blackburn, A. T. M., Bekheirnia, N., Uma, V. C., Corkins, M. E., Yuxiao, X., Rosenfeld, J. A., et al. (2019). DYRK1A-related intellectual disability: a syndrome associated with congenital anomalies of the kidney and urinary tract. Genetics Med.: Official J. American College of Medical Genetics 21, 2755–2764. doi: 10.1038/s41436-019-0576-0
Boissart, C., Nissan, X., Giraud-Triboult, K., Peschanski, M., and Benchoua, A. (2012). MiR-125 potentiates early neural specification of human embryonic stem cells. Development 139, 1247–1257. doi: 10.1242/dev.073627
Bronicki, L. M., Redin, C., Drunat, S., Piton, A., Lyons, M., Passemard, S., et al. (2015). Ten new cases further delineate the syndromic intellectual disability phenotype caused by mutations in DYRK1A. European J. Human Genetics: EJHG 23, 1482–1487. doi: 10.1038/ejhg.2015.29
Capon, F., Bijlmakers, M.-J., Wolf, N., Quaranta, M., Huffmeier, U., Allen, M., et al. (2008). Identification of ZNF313/RNF114 as a novel psoriasis susceptibility gene. Hum. Mol. Genet. 17, 1938–1945. doi: 10.1093/hmg/ddn091
Cho, H.-J., Lee, J.-G., Kim, J.-H., Kim, S.-Y., Huh, Y. H., Kim, H.-J., et al. (2019). Vascular defects of DYRK1A knockouts are ameliorated by modulating calcium signaling in zebrafish. Dis. Model. Mech. 12:dmm037044. doi: 10.1242/dmm.037044
Courraud, E. C.-D., Durand, B., and Vincent, M. (2021). Integrative approach to interpret DYRK1A variants, leading to a frequent neurodevelopmental disorder. medRxiv 2021:21250155. doi: 10.1101/2021.01.20.21250155
Courraud, J., Engel, C., Quartier, A., Drouot, N., Houessou, U., Plassard, D., et al. (2023). Molecular consequences of PQBP1 deficiency, involved in the X-linked Renpenning syndrome. Molecular Psychiatry 29, 287–296. doi: 10.1038/s41380-023-02323-5
Duchon, A., and Herault, Y. (2016). DYRK1A, a dosage-sensitive gene involved in neurodevelopmental disorders, is a target for drug development in down syndrome. Front. Behav. Neurosci. 10:104. doi: 10.3389/fnbeh.2016.00104
Ehe, B. K., Lamson, D. R., Tarpley, M., Onyenwoke, R. U., Graves, L. M., and Williams, K. P. (2017). Identification of a DYRK1A-mediated phosphorylation site within the nuclear localization sequence of the hedgehog transcription factor GLI1. Biochem. Biophys. Res. Commun. 491, 767–772. doi: 10.1016/j.bbrc.2017.07.107
Fischbach, K. F., and Heisenberg, M. (1981). Structural brain mutant of Drosophila Melanogaster with reduced cell number in the medulla cortex and with Normal Optomotor yaw response. Proc. Natl. Acad. Sci. USA 78, 1105–1109. doi: 10.1073/pnas.78.2.1105
Fotaki, V., Dierssen, M., Alcántara, S., Martínez, S., Martí, E., Casas, C., et al. (2002). Dyrk1A Haploinsufficiency affects viability and causes developmental delay and abnormal brain morphology in mice. Mol. Cell. Biol. 22, 6636–6647. doi: 10.1128/mcb.22.18.6636-6647.2002
Fotaki, V., Martínez De Lagrán, M., Estivill, X., Arbonés, M., and Dierssen, M. (2004). Haploinsufficiency of Dyrk1A in mice leads to specific alterations in the development and regulation of motor activity. Behav. Neurosci. 118, 815–821. doi: 10.1037/0735-7044.118.4.815
Gonzalez-Mantilla, A. J., Moreno-De-Luca, A., Ledbetter, D. H., and Martin, C. L. (2016). A cross-disorder method to identify novel candidate genes for developmental brain disorders. JAMA Psychiatry 73, 275–283. doi: 10.1001/jamapsychiatry.2015.2692
Grau, C., Arató, K., Fernández-Fernández, J. M., Valderrama, A., Sindreu, C., Fillat, C., et al. (2014). DYRK1A-mediated phosphorylation of GluN2A at Ser(1048) regulates the surface expression and channel activity of GluN1/GluN2A receptors. Front. Cell. Neurosci. 8:331. doi: 10.3389/fncel.2014.00331
Guard, S. E., Poss, Z. C., Ebmeier, C. C., Pagratis, M., Simpson, H., Taatjes, D. J., et al. (2019). The nuclear Interactome of DYRK1A reveals a functional role in DNA damage repair. Sci. Rep. 9:6539. doi: 10.1038/s41598-019-42990-5
Guedj, F., Pereira, P. L., Najas, S., Barallobre, M.-J., Chabert, C., Souchet, B., et al. (2012). DYRK1A: a master regulatory protein controlling brain growth. Neurobiol. Dis. 46, 190–203. doi: 10.1016/j.nbd.2012.01.007
Gwack, Y., Sharma, S., Nardone, J., Tanasa, B., Iuga, A., Srikanth, S., et al. (2006). A genome-wide Drosophila RNAi screen identifies DYRK-family kinases as regulators of NFAT. Nature 441, 646–650. doi: 10.1038/nature04631
Hämmerle, B., Elizalde, C., and Tejedor, F. J. (2008). The Spatio-temporal and subcellular expression of the candidate down syndrome gene Mnb/Dyrk1A in the developing mouse brain suggests distinct sequential roles in neuronal development. Eur. J. Neurosci. 27, 1061–1074. doi: 10.1111/j.1460-9568.2008.06092.x
Han, J., Kim, Y.-L., Lee, K.-W., Her, N.-G., Ha, T.-K., Yoon, S., et al. (2013). ZNF313 is a novel cell cycle activator with an E3 ligase activity inhibiting cellular senescence by destabilizing P21(WAF1.). Cell Death Differ. 20, 1055–1067. doi: 10.1038/cdd.2013.33
Hu, J., Qian, H., Xue, Y., and Xiang-Dong, F. (2018). PTB/NPTB: master regulators of neuronal fate in mammals. Biophys. Rep. 4, 204–214. doi: 10.1007/s41048-018-0066-y
Huang, Y., Chen-Hwang, M.-C., Dolios, G., Murakami, N., Padovan, J. C., Wang, R., et al. (2004). Mnb/Dyrk1A phosphorylation regulates the interaction of dynamin 1 with SH3 domain-containing proteins. Biochemistry 43, 10173–10185. doi: 10.1021/bi036060+
Huttlin, E. L., Bruckner, R. J., Navarrete-Perea, J., Cannon, J. R., Baltier, K., Gebreab, F., et al. (2021). Dual proteome-scale networks reveal cell-specific remodeling of the human Interactome. Cell 184, 3022–3040.e28. doi: 10.1016/j.cell.2021.04.011
Iossifov, I., O’Roak, B. J., Sanders, S. J., Ronemus, M., Krumm, N., Levy, D., et al. (2014). The contribution of de novo coding mutations to autism Spectrum disorder. Nature 515, 216–221. doi: 10.1038/nature13908
Jang, S. M., Saliha, A., and Guillaume, S.. (2014). DYRK1A Phoshorylates Histone H3 to Differentially Regulate the Binding of HP1 Isoforms and Antagonize HP1-Mediated Transcriptional Repression. EMBO Reports 15, 686–94. doi: 10.15252/embr.201338356
Kim, O.-H., Cho, H.-J., Han, E., Hong, T. I., Ariyasiri, K., Choi, J.-H., et al. (2017). Zebrafish knockout of down syndrome gene, DYRK1A, shows social impairments relevant to autism. Mol. Autism. 8:50. doi: 10.1186/s13229-017-0168-2
Laguna, A., Barallobre, M.-J., Marchena, M.-Á., Mateus, C., Ramírez, E., Martínez-Cue, C., et al. (2013). Triplication of DYRK1A causes retinal structural and functional alterations in down syndrome. Hum. Mol. Genet. 22, 2775–2784. doi: 10.1093/hmg/ddt125
Lepagnol-Bestel, A.-M., Zvara, A., Maussion, G., Quignon, F., Ngimbous, B., Ramoz, N., et al. (2009). DYRK1A interacts with the REST/NRSF-SWI/SNF chromatin Remodelling complex to deregulate gene clusters involved in the neuronal phenotypic traits of down syndrome. Hum. Mol. Genet. 18, 1405–1414. doi: 10.1093/hmg/ddp047
Levy, J. A., LaFlamme, C. W., Tsaprailis, G., Crynen, G., and Page, D. T. (2021). Dyrk1a mutations cause undergrowth of cortical pyramidal neurons via dysregulated growth factor signaling. Biological Psychiatry 90, 295–306. doi: 10.1016/j.biopsych.2021.01.012
Li, S., Chu, X., Yinkun, F., Lei, P.-J., Yao, Y., Yang, W., et al. (2018). DYRK1A interacts with histone acetyl transferase P300 and CBP and localizes to enhancers. Nucleic Acids Res. 46, 11202–11213. doi: 10.1093/nar/gky754
Li, J., Ma, Z., Shi, M., Malty, R. H., Aoki, H., Minic, Z., et al. (2015). Identification of human neuronal protein complexes reveals biochemical activities and convergent mechanisms of action in autism Spectrum disorders. Cell Systems 1, 361–374. doi: 10.1016/j.cels.2015.11.002
Lu, M., Zheng, L., Han, B., Wang, L., Wang, P., Liu, H., et al. (2011). REST regulates DYRK1A transcription in a negative feedback loop. J. Biol. Chem. 286, 10755–10763. doi: 10.1074/jbc.M110.174540
Mattioli, F., Hayot, G., Drouot, N., Isidor, B., Courraud, J., Hinckelmann, M.-V., et al. (2020). De novo frameshift variants in the neuronal splicing factor NOVA2 result in a common C-terminal extension and cause a severe form of neurodevelopmental disorder. Am. J. Hum. Genet. 106, 438–452. doi: 10.1016/j.ajhg.2020.02.013
Mattioli, F., Isidor, B., Abdul-Rahman, O., Gunter, A., Huang, L., Kumar, R., et al. (2019). Clinical and functional characterization of recurrent missense variants implicated in THOC6-related intellectual disability. Hum. Mol. Genet. 28, 952–960. doi: 10.1093/hmg/ddy391
Menon, V. R., Ananthapadmanabhan, V., Swanson, S., Saini, S., Sesay, F., Yakovlev, V., et al. (2019). DYRK1A regulates the recruitment of 53BP1 to the sites of DNA damage in part through interaction with RNF169. Cell Cycle (Georgetown, Tex.) 18, 531–551. doi: 10.1080/15384101.2019.1577525
Møller, R. S., Kübart, S., Hoeltzenbein, M., Heye, B., Vogel, I., Hansen, C. P., et al. (2008). Truncation of the down syndrome candidate gene DYRK1A in two unrelated patients with microcephaly. Am. J. Hum. Genet. 82, 1165–1170. doi: 10.1016/j.ajhg.2008.03.001
Murakami, N., Bolton, D., and Hwang, Y.-W. (2009). Dyrk1A binds to multiple endocytic proteins required for formation of Clathrin-coated vesicles. Biochemistry 48, 9297–9305. doi: 10.1021/bi9010557
Murakami, N., Xie, W., Renne Chen, L., Chen-Hwang, M.-C., Wieraszko, A., and Hwang, Y. W. (2006). Phosphorylation of Amphiphysin I by Minibrain kinase/dual-specificity tyrosine phosphorylation-regulated kinase, a kinase implicated in down syndrome. J. Biol. Chem. 281, 23712–23724. doi: 10.1074/jbc.M513497200
O’Roak, B. J., Vives, L., Wenqing, F., Egertson, J. D., Stanaway, I. B., Phelps, I. G., et al. (2012). Multiplex targeted sequencing identifies recurrently mutated genes in autism Spectrum disorders. Science (New York, N.Y.) 338, 1619–1622. doi: 10.1126/science.1227764
Ori-McKenney, K. M., McKenney, R. J., Huang, H. H., Li, T., Meltzer, S., Jan, L. Y., et al. (2016). Phosphorylation of β-tubulin by the down syndrome kinase, Minibrain/DYRK1a, regulates microtubule dynamics and dendrite morphogenesis. Neuron 90, 551–563. doi: 10.1016/j.neuron.2016.03.027
Pal, D., and Summers, M. (2017). “The roles of Cullin RING ligases and the anaphase promoting complex/Cyclosome in the regulation of DNA double Strand break repair” in Ubiquitination Governing DNA Repair-Implications in Health and Disease (Eds. Effrossyni Boutou and Horst-Werner Stürzbecher. (UK: IntechOpen).
Park, J., Yohan, O., Yoo, L., Jung, M.-S., Song, W.-J., Lee, S.-H., et al. (2010). Dyrk1A phosphorylates P53 and inhibits proliferation of embryonic neuronal cells. J. Biol. Chem. 285, 31895–31906. doi: 10.1074/jbc.M110.147520
Quartier, A., Chatrousse, L., Redin, C., Keime, C., Haumesser, N., Maglott-Roth, A., et al. (2018). Genes and pathways regulated by androgens in human neural cells, potential candidates for the male excess in autism Spectrum disorder. Biol. Psychiatry 84, 239–252. doi: 10.1016/j.biopsych.2018.01.002
Roewenstrunk, J., Di Vona, C., Chen, J., Borras, E., Dong, C., Arató, K., et al. (2019). A comprehensive proteomics-based interaction screen that links DYRK1A to RNF169 and to the DNA damage response. Sci. Rep. 9:6014. doi: 10.1038/s41598-019-42445-x
Ryoo, S.-R., Jeong, H. K., Radnaabazar, C., Yoo, J.-J., Cho, H.-J., Lee, H.-W., et al. (2007). DYRK1A-mediated hyperphosphorylation of tau. A functional link between down syndrome and Alzheimer disease. J. Biol. Chem. 282, 34850–34857. doi: 10.1074/jbc.M707358200
Shi, J., Zhang, T., Zhou, C., Chohan, M. O., Xiaosong, G., Wegiel, J., et al. (2008). Increased dosage of Dyrk1A alters alternative splicing factor (ASF)-regulated alternative splicing of tau in down syndrome. J. Biol. Chem. 283, 28660–28669. doi: 10.1074/jbc.M802645200
Sivakumar, S., and Gorbsky, G. J. (2015). Spatiotemporal regulation of the anaphase-promoting complex in mitosis. Nat. Rev. Mol. Cell Biol. 16, 82–94. doi: 10.1038/nrm3934
Skaar, J. R., and Pagano, M. (2009). Control of cell growth by the SCF and APC/C ubiquitin ligases. Curr. Opin. Cell Biol. 21, 816–824. doi: 10.1016/j.ceb.2009.08.004
Tejedor, F., and Hämmerle, B. (2011). MNB/DYRK1A as a multiple regulator of neuronal development. FEBS J. 278, 223–235. doi: 10.1111/j.1742-4658.2010.07954.x
Tejedor, F., Zhu, X. R., Kaltenbach, E., Ackermann, A., Baumann, A., Canal, I., et al. (1995). Minibrain: a new protein kinase family involved in postembryonic neurogenesis in Drosophila. Neuron 14, 287–301. doi: 10.1016/0896-6273(95)90286-4
Thompson, B. J., Bhansali, R., Diebold, L., Cook, D. E., Stolzenburg, L., Casagrande, A.-S., et al. (2015). DYRK1A controls the transition from proliferation to quiescence during lymphoid development by destabilizing cyclin D3. J. Exp. Med. 212, 953–970. doi: 10.1084/jem.20150002
van Bon, B. W. M., Coe, B. P., Bernier, R., Green, C., Gerdts, J., Witherspoon, K., et al. (2016). Disruptive de novo mutations of DYRK1A Lead to a syndromic form of autism and ID. Mol. Psychiatry 21, 126–132. doi: 10.1038/mp.2015.5
Varjosalo, M., Keskitalo, S., Van Drogen, A., Nurkkala, H., Vichalkovski, A., Aebersold, R., et al. (2013). The protein interaction landscape of the human CMGC kinase group. Cell Rep. 3, 1306–1320. doi: 10.1016/j.celrep.2013.03.027
Vona, D., Chiara, D. B., Islam, A. B. M. M. K., Salichs, E., López-Bigas, N., Ossowski, S., et al. (2015). Chromatin-wide profiling of DYRK1A reveals a role as a gene-specific RNA polymerase II CTD kinase. Mol. Cell 57, 506–520. doi: 10.1016/j.molcel.2014.12.026
Willsey, H. R., Xu, Y., Everitt, A., Dea, J., Exner, C. R. T., Willsey, A. J., et al. (2020). The neurodevelopmental disorder risk gene DYRK1A is required for Ciliogenesis and control of brain size in Xenopus embryos. Development 147, 21. doi: 10.1242/dev.189290
Yang, E. J., Ahn, Y. S., and Chung, K. C. (2001). Protein kinase Dyrk1 activates CAMP response element-binding protein during neuronal differentiation in hippocampal progenitor cells. J. Biol. Chem. 276, 39819–39824. doi: 10.1074/jbc.M104091200
Keywords: DYRK1A, human neural stem cells, interactome, gene expression, cell proliferation, RNF114, p21/CDKN1A, neurodevelopmental disorders
Citation: Courraud J, Quartier A, Drouot N, Zapata-Bodalo I, Gilet J, Benchoua A, Mandel J-L and Piton A (2025) DYRK1A roles in human neural progenitors. Front. Neurosci. 19:1533253. doi: 10.3389/fnins.2025.1533253
Edited by:
Yusuke Takatsuru, Toyo University, JapanReviewed by:
Barbara Bardoni, UMR7275 Institut de Pharmacologie Moléculaire et Cellulaire (IPMC), FranceJean Maurice Delabar, Centre National de la Recherche Scientifique (CNRS), France
Copyright © 2025 Courraud, Quartier, Drouot, Zapata-Bodalo, Gilet, Benchoua, Mandel and Piton. This is an open-access article distributed under the terms of the Creative Commons Attribution License (CC BY). The use, distribution or reproduction in other forums is permitted, provided the original author(s) and the copyright owner(s) are credited and that the original publication in this journal is cited, in accordance with accepted academic practice. No use, distribution or reproduction is permitted which does not comply with these terms.
*Correspondence: Amélie Piton, cGl0b25AaWdibWMuZnI=