- 1Department of Developmental Neuroscience, IRCCS Stella Maris Foundation, Pisa, Italy
- 2Department of Clinical and Experimental Medicine, University of Pisa, Pisa, Italy
- 3National Research Council (CNR), Institute of Neuroscience, Pisa, Italy
- 4Department of Neurology, Boston Children's Hospital, F. M. Kirby Neurobiology Center, Harvard Medical School, Boston, MA, United States
Neurodevelopmental disorders (NDDs) cause profound intellectual and physical impairment, yet therapeutic progress remains hindered by the lack of quantitative, unbiased, and non-invasive biomarkers to monitor disease onset and progression. Visual evoked potentials (VEPs) have emerged as promising functional biomarkers for X-linked NDDs, with reduced VEP amplitudes correlating with disease severity. Complementary approaches, like functional Near Infrared Spectroscopy (fNIRS), offer a non-invasive additional tool to assess brain metabolism, monitor disease progression, and evaluate therapeutic responses. This perspective explores the potential of fNIRS in studying visually evoked hemodynamic responses (vHDR) across different age groups, demonstrating its reliability in capturing task-specific cortical activation and tracking brain maturation even in challenging populations. Notably, fNIRS identifies unique vHDR patterns in conditions like optic neuritis, myopia, glaucoma, and migraines, validating its role as a biomarker for disease severity and treatment efficacy. Moreover, fNIRS has proven effective in detecting early neural deficits in high-risk infants, including pre-term newborns. Preclinical studies support that visually induced hemodynamic changes can differentiate healthy from pathological conditions in X-linked NDDs. However, direct evidence from human cohorts with X-linked NDDs remains limited, highlighting the urgent need for further research to validate the potential of visual fNIRS as a reliable functional biomarker in clinical settings. To enhance clinical relevance, the development of standardized protocols, engaging stimuli, and age-stratified analyses is also crucial for improving diagnostic accuracy, tracking neurodevelopmental trajectories, and evaluating therapeutic interventions.
1 Introduction
Neurodevelopmental disorders (NDDs) pose a significant global public health challenge, impacting millions of children and their families across the world. These chronic and heterogeneous conditions are often underdiagnosed, with an estimated prevalence exceeding 1.5% of the global population (Chung et al., 2022). Over 90 genes on the X chromosome have been linked to NDDs, which encompass rare disorders characterized by severe intellectual and physical disabilities (Lumsden and Urv, 2023). Despite the urgent need for effective treatments, progress in therapeutic development remains constrained by the lack of robust translational endpoints and reliable, non-invasive biomarkers essential for accelerating preclinical and clinical testing (Kearns and Artman, 2015; Budimirovic et al., 2017; Krainc et al., 2023).
Recent animal model studies have demonstrated that the visual system serves as a powerful proxy for assessing overall brain function (Durand et al., 2012; Della Sala and Pizzorusso, 2014; Mazziotti et al., 2020). Visual evoked potential (VEP) electrophysiological recordings have revealed significantly reduced VEP amplitudes and diminished visual acuity in Mecp2 mutant mice compared to their wild-type (WT) littermates. Notably, the reduction in VEP amplitude is most pronounced in animals with greater phenotypic severity and more advanced RTT-like symptoms, suggesting that VEP amplitude reflects overall neurological health and disorder progression (LeBlanc et al., 2015). Importantly, similar findings have been reported in RTT patients, who exhibit significantly diminished P1 amplitudes, which worsen as the disorder advances (Stauder et al., 2006; LeBlanc et al., 2015; Saby et al., 2021). These data underscore the potential clinical utility of P1 amplitude as a translatable biomarker. Furthermore, recent analyses have extended these observations to other X-linked disorders, with similar results replicated in both animal models and patients with CDKL5 Deficiency (Mazziotti et al., 2017; Saby et al., 2022), and MECP2 duplication syndrome (Saby et al., 2023).
These findings highlight neurophysiological measures, such as VEPs, as valuable, quantitative, and unbiased biomarkers for assessing disease severity and progression in X-linked NDDs. Their potential for repeated, non-invasive longitudinal assessments also makes them highly suitable for clinical trial readiness (LeBlanc et al., 2015; McPartland, 2016; Sidorov et al., 2017). However, the development of additional biomarkers is critical for advancing our understanding of these disorders and refine therapeutic strategies. Given that disruptions in energy metabolism are a key pathological mechanism in many X-linked disorders (Ghirardini et al., 2021; Oyarzábal et al., 2021; van Bergen et al., 2021), assessing cerebral blood flow and oxygen consumption offers a complementary and highly sensitive approach for quantifying functional changes in neural circuits. This strategy provides crucial insights into neurovascular coupling, enhancing our ability to monitor disease progression and therapeutic efficacy.
The functional Near Infrared Spectroscopy (fNIRS) is a non-invasive optical imaging technique that has gained increasing recognition for analyzing energy metabolism in the brain (Strangman et al., 2002; Cui et al., 2011; Chen et al., 2020). By detecting hemodynamic responses (HDR) associated with cortical activation, fNIRS measures increase in total hemoglobin (THb) and oxygenated hemoglobin (OHb) levels while recording decreases in deoxygenated hemoglobin (DHb) concentrations in regions of interest (ROIs) (van de Rijt et al., 2016). Although fNIRS was introduced into clinical care nearly 40 years ago (Villringer et al., 1993; Chen et al., 2020), its application in studying brain development and NDDs has only recently gained attention (Vanderwert and Nelson, 2014). To date, fNIRS has primarily been used to investigate typical developmental processes such as speech perception and language, sensory and motor functions, social communication, and object and action processing in toddlers and children (Lloyd-Fox et al., 2010; Gervain et al., 2011). Its ability to capture brain activation from the earliest stages of development (Cabrera and Gervain, 2020) makes it particularly well-suited for studying NDDs.
This perspective paper explores the potential of fNIRS for analyzing visually-evoked hemodynamic responses (vHDR), assessing its viability as biomarker for brain function across both pediatric and adult populations.
2 The application of fNIRS in the study of the visual system
This section reviews key findings from studies employing fNIRS to analyze vHDR. The discussion is divided into two subsections: studies in adults and those in children. We summarize major findings, methodological approaches, and clinical applications while addressing the limitations of current research. Detailed technical information—including equipment, participant characteristics, and experimental paradigms—is systematically presented in Table 1 (adults) and Table 2 (children).
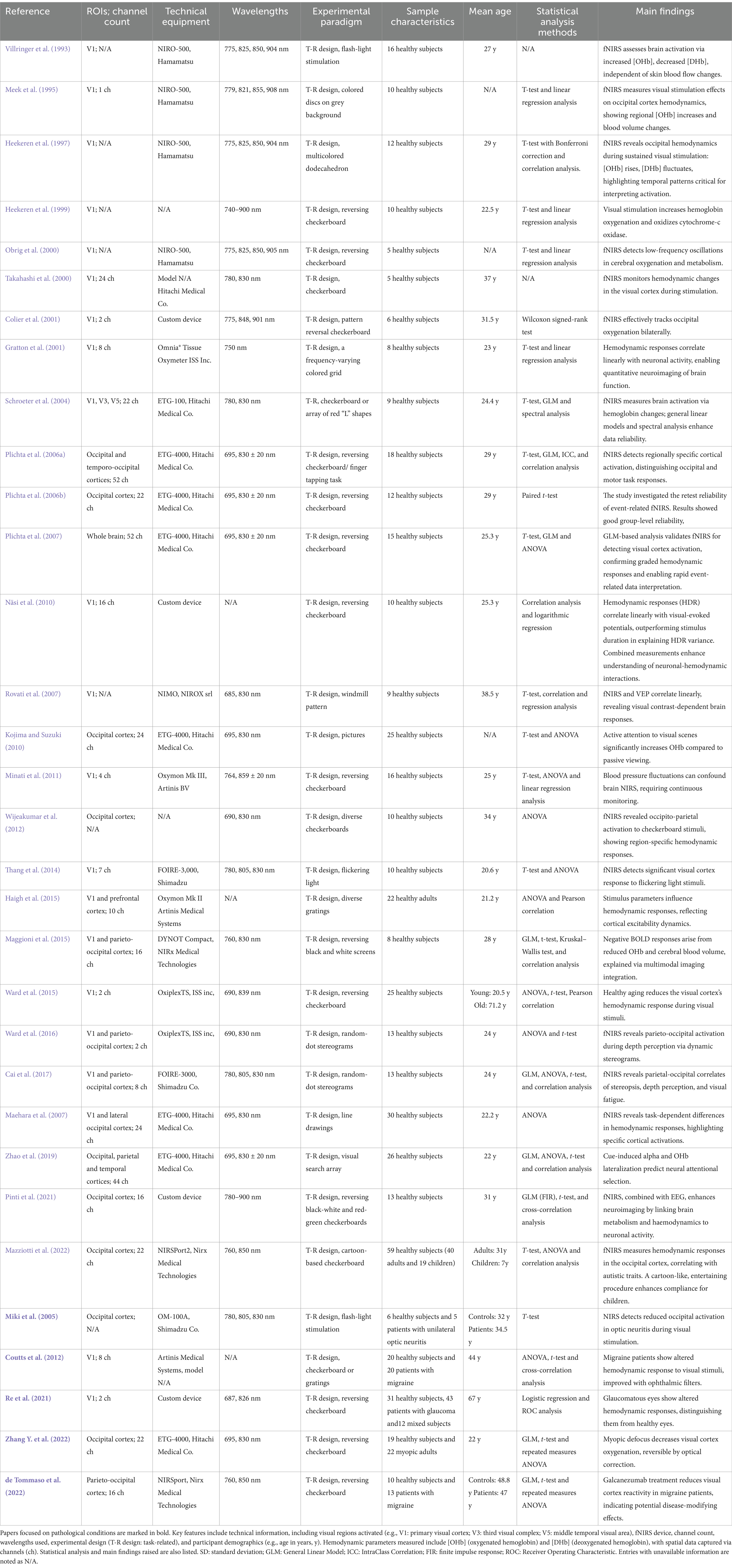
Table 1. The table summarizes fNIRS studies investigating visual processing in adults, presented chronologically to illustrate the evolving complexity of the visual paradigms utilized over time.
2.1 Visual cortical processing through fNIRS: methodological insights and clinical applications in adults
Early studies in the 1990s used the visual system as a model to investigate cortical HDR during task-related experiments in healthy adults. Villringer’s and Zeki’s group employed various visual stimuli- including flashes, pictures, and moving colored shapes- to examine occipital cortex activation. These studies revealed a distinct HDR pattern, with increased OHb levels and a smaller-magnitude decrease in DHb, peaking approximately 5 s after stimulus onset. This pattern underscored fNIRS’s potential as a bedside tool for monitoring brain activity with minimal interference from superficial blood flow (Villringer et al., 1993; Meek et al., 1995). Subsequent research explored the temporal dynamics of vHDR during sustained stimulation, showing that OHb levels rise and remain elevated, while DHb initially decreases before returning to baseline and exhibiting a post-stimulus overshoot (Heekeren et al., 1997). Multi-channel fNIRS studies confirmed the regional specificity of these responses, with activation localized to the contralateral occipital cortex reinforcing the stimulus-specific nature of vHDR (Colier et al., 2001; Plichta et al., 2006a; Wijeakumar et al., 2012).
Over the years, experimental paradigms have refined visual stimulation parameters- such as shape, contrast, chromaticity, and frequency- to optimize vHDR reliability (Gratton et al., 2001; Rovati et al., 2007; Wijeakumar et al., 2012; Thang et al., 2014; Haigh et al., 2015). High-contrast black-and-white checkerboard and pattern-reversal gratings have proven the most effective and are now widely used in fNIRS studies of visual processing (Heekeren et al., 1999; Obrig et al., 2000; Takahashi et al., 2000; Wijeakumar et al., 2012; Haigh et al., 2015). Higher stimulus frequencies produce linear increases in vHDR amplitude, whereas greater contrast elicits logarithmic changes in OHb and DHb levels (Gratton et al., 2001; Rovati et al., 2007). These findings highlight how optimizing visual stimulation parameters can enhance the reliability and robustness of fNIRS measurements in capturing brain activity.
Recent studies have expanded beyond primary visual processing to examine associative pathways in extrastriate cortices. Tasks involving depth perception, selective attention, and shape recognition have provided insights into the broader visual networks and their roles in higher-order cognitive functions (Ward et al., 2016; Cai et al., 2017; Maehara et al., 2007; Zhao et al., 2019). Advanced analytical methods, including general linear models and spatially resolved spectral techniques, have further enhanced fNIRS capability to detect cortical activity (Schroeter et al., 2004; Plichta et al., 2007). Additionally, multi-modal approaches integrating fNIRS with EEG or fMRI have validated fNIRS’s reliability by demonstrating strong signal consistency across modalities (Rovati et al., 2007; Näsi et al., 2010; Maggioni et al., 2015; Chen et al., 2017; Zhao et al., 2019; Pinti et al., 2021).
Building from the data collected in young adults, studies on healthy aging have revealed increased variability in vHDR, with OHb and DHb responses becoming less distinct between stimulation and rest in older adults. This pattern likely reflects age-related declines in neural efficiency and vascular responsiveness (Ward et al., 2015).
In clinical settings, fNIRS has been applied to ophthalmological conditions such as optic neuritis, myopia, and glaucoma, identifying distinct HDR alterations as potential biomarkers of visual impairment (Miki et al., 2005; Zhang Y. et al., 2022; Re et al., 2021). For example, optic neuritis patients exhibit reduced occipital HDR during affected-eye stimulation, despite normal visual acuity recovery, suggesting persistent neural deficits (Miki et al., 2005). Additionally, variations in vHDR patterns have been associated with disease severity in glaucoma and myopia, underscoring the diagnostic potential of fNIRS (Zhang Y. et al., 2022; Re et al., 2021).
The fNIRS has also offered valuable insights into neurological disorders. Studies in migraine patients have shown increased OHb levels during visual stimulation, which normalized after treatment with Galcanezumab, correlating with symptom relief (Coutts et al., 2012; de Tommaso et al., 2022). These findings highlight the potential of fNIRS in monitoring treatment efficacy and investigating changes in visual processing across a range of neurological conditions.
2.2 fNIRS for assessing visual processing and cortical development in infants and children
The functional Near Infrared Spectroscopy has become a key tool for investigating brain activity and development in infants and children, offering insights into functional specialization, cortical maturation, and sensory processing (Lloyd-Fox et al., 2010; Gervain et al., 2011).
Hemodynamic responses to visual stimuli have been observed from the earliest stages of life. Studies using checkerboard patterns in awake infants aged 3 days to 16 weeks have demonstrated regionally specific OHb and DHb changes (Meek et al., 1998; Taga et al., 2003a; Liao et al., 2010). These findings highlight fNIRS’s high spatial specificity, even in very young subjects, and suggest the presence of early cerebrovascular coupling that closely resembles adult patterns.
However, interpreting infant visual fNIRS data requires careful consideration. Unlike adults, neonates often exhibit increased DHb levels during stimulation likely due to immature neurovascular coupling, which typically stabilizes by 3–4 years of age (Meek et al., 1998; Kusaka et al., 2004). Additionally, factors such as consciousness state and stimulus characteristics influence HDR reliability. Checkerboard patterns consistently evoke robust responses, whereas less structured stimuli yield weaker activations (Watanabe et al., 2012). Furthermore, early sensory processing in neonates involves prefrontal cortical contributions, with neural circuits progressively maturing into an adult-like configuration over time (Taga et al., 2003a,b; Karen et al., 2008).
Building on these foundational studies, research has explored functional differentiation within visual cortical circuits. Studies in 3-month-old infants revealed that primary visual regions respond similarly to both object (e.g., moving objects) and non-object (e.g., checkerboard) stimuli, whereas the lateral occipital cortex selectively activates in response to object stimuli. The same pattern is replicated in preschool children (Watanabe et al., 2008; Remijn et al., 2011; Hirshkowitz et al., 2018). By 5–6 months of age, the inferior temporal and the lateral occipital cortices support feature-based object recognition, with infants distinguishing objects by shape and size before incorporating color clues (Wilcox, 1999; Wilcox et al., 2005; Wilcox et al., 2008; Emberson et al., 2017). These findings further indicate that stable functional segregation within the visual cortex is present from an early age. Longitudinal studies further indicate that functional specialization follows a developmental trajectory, shifting from broad, undifferentiated cortical activation at 2 months to region-specific processing by 3 months of age (Watanabe et al., 2010).
Recent research has also explored the emergence of multisensory integration in infants. Audiovisual stimuli enhance activation in both sensory-specific areas and strengthen functional connectivity between the occipital and temporal cortices, emphasizing the role of early multisensory experience in shaping neuronal networks (Watanabe et al., 2013; Emberson et al., 2015; Werchan et al., 2018 but see also Bortfeld et al., 2007).
Beyond typical development, fNIRS has been valuable for studying high-risk populations, such as preterm infants. Although research on pathological visual processing using fNIRS are limited, recent studies suggest that prematurity may disrupt functional connectivity and top-down modulation, leading to inefficient information transfer and potential learning difficulties (Boldin et al., 2018). These findings highlight fNIRS potential for early cerebral dysfunction detection, enabling timely interventions to mitigate developmental challenges.
3 Discussion
This perspective paper provides a comprehensive review of visual paradigms employed in fNIRS studies across adult and pediatric populations, emphasizing its potential as a biomarker for monitoring brain function. By capturing vHDR, fNIRS emerges as a promising tool in diagnosing and tracking NDDs and assessing treatment responses, advancing the field of personalized medicine.
Seminal studies have established that fNIRS reliably detects task-specific cortical activation across ages, with checkerboard stimuli consistently eliciting robust and reproducible vHDR in both adults and children (Villringer et al., 1993; Meek et al., 1995; Meek et al., 1998; Taga et al., 2003a). These foundational findings set the stage for its use in mapping developmental trajectories and revealing neural alterations in both research and clinical settings. More recent studies have applied fNIRS to pathological conditions such as migraines, visual impairments, and prematurity (Miki et al., 2005; Boldin et al., 2018; Re et al., 2021; Zhang Y. et al., 2022a; de Tommaso et al., 2022), demonstrating its ability to distinguish pathological states, predict disease severity, and monitor treatment efficacy. For example, studies in glaucoma and migraine patients have shown that fNIRS can detect disease-specific hemodynamic alterations and track therapeutic responses (Re et al., 2021; de Tommaso et al., 2022). Additionally, research on prematurity has highlighted how early disruptions in neural connectivity affect sensory processing and cognitive development, reinforcing fNIRS’s potential in detecting and addressing developmental vulnerabilities (Boldin et al., 2018).
In the study of NDDs, fNIRS has gained traction as a tool for identifying divergent hemodynamic patterns in conditions such as Attention Deficit Hyperactivity Disorder (ADHD), Autism Spectrum Disorder (ASD), and Developmental Coordination Disorder (DCD) (Conti et al., 2022; Scaffei et al., 2023; Su et al., 2023; Huang et al., 2024; Kurane et al., 2024). Resting-state and longitudinal connectivity studies have revealed abnormalities in the temporal dynamics of brain activity, shedding insights on developmental deviations in children with NDDs (e.g., Keehn et al., 2013; Cao et al., 2021; Zhang F. et al., 2022). Event-related studies have further identified distinct alterations in HDR, such as atypical prefrontal cortex activation in ADHD (Ishii et al., 2017; Yasumura et al., 2019) and reduced vHDR amplitude in preschool females with high-functioning ASD (Scaffei et al., 2023), reinforcing the potential of task-related fNIRS as a non-invasive and accessible diagnostic and monitoring tool. However, the methodological variability in data collection and analysis presents significant challenges, limiting the comparability of results across studies and hindering the broader clinical application of fNIRS. To overcome these limitations, there is an urgent need for standardized protocols that encompass every stage of the experimental process. These protocols should provide clear guidelines for stimulation paradigms, as well as establish specific requirements for fNIRS equipment, including wavelength, source-detector distance, and channel configuration. In addition, robust signal processing procedures must be defined, with a particular focus on motion artifact correction techniques, such as short-channel regression or independent component analysis, along with appropriate filtering and data analysis methods. Finally, implementing rigorous reporting standards is essential to guarantee transparency, reproducibility, and the ability to effectively compare findings across studies.
For X-linked NDDs, preclinical research has demonstrated that visually evoked hemodynamic changes can distinguish between healthy and pathological conditions (Mazziotti et al., 2017; Mazziotti et al., 2020). These findings suggest that vHDR could serve as a non-invasive tool for early diagnosis and disease monitoring in clinical populations. However, it is important to note that the current evidence is primarily derived from animal models. Therefore, future research should prioritize examining vHDR in human cohorts with X-linked NDD to validate the translational potential of fNIRS. Additionally, to fully realize the potential of visual fNIRS as a functional biomarker, it is essential to broaden its application across a wide range of NDDs. Investigating fNIRS biomarkers in various NDDs—encompassing genetic, idiopathic, and environmentally influenced disorders—will deepen our understanding of both shared and disorder-specific mechanisms. This expanded focus will ultimately enhance diagnostic precision and support the development of more personalized intervention strategies.
Despite its promises, several critical factors influence the reliability and clinical utility of fNIRS as a biomarker, including its restricted brain penetration, feasibility, test–retest reliability, age-related variations in response amplitudes, and engagement-dependent fluctuations in hemodynamic responses. fNIRS has proven to be highly feasible even in challenging populations, including infants and children with NDDs (Vanderwert and Nelson, 2014). However, participant engagement significantly impacts the quality of vHDR recordings, as active tasks tend to produce stronger responses compared to passive viewing (Kojima and Suzuki, 2010). To improve compliance, particularly in young children, innovative protocols combining animated stimuli with traditional checkerboard paradigms have been developed, enhancing both engagement and data quality (Mazziotti et al., 2022).
Age-related anatomical differences, such as thinner skulls and scalps in children, influence light propagation during fNIRS measurements, highlighting the need for age-stratified interpretations of biomarkers (Remijn et al., 2011; Mazziotti et al., 2022). Additionally, while short-term reproducibility of visual event-related hemodynamic responses has been demonstrated in adults (Plichta et al., 2006b), further research is required to assess the long-term stability and circadian effects on fNIRS measurements. Moreover, controlling for systemic physiological factors, such as blood pressure and heart rate fluctuations, is essential to improve the precision of fNIRS-based biomarkers (Minati et al., 2011).
In conclusion, fNIRS, particularly in visual paradigms, is a powerful, non-invasive tool for assessing brain function, complementing existing technologies such as fMRI and EEG, in both typical and atypical development. Expanding standardized protocols and refining vHDR analysis will strengthen its clinical applications, enabling earlier and more accurate diagnoses, tracking neurodevelopmental trajectories, and optimizing therapeutic strategies. Given the urgent need for reliable functional biomarkers in X-linked NDDs, expanding fNIRS studies across a broader spectrum of conditions will be essential. Furthermore, integrating fNIRS into a multimodal framework, such as combining it with EEG, could provide transformative insights into brain function. EEG offers high temporal resolution complementing fNIRS’s spatial information, thus enabling a more comprehensive assessment of neural activity. The potential of multimodal imaging is also evident in recent studies that simultaneously collect fNIRS and fMRI data to investigate the relationship between cortical hemodynamics and deeper brain activity. For example, the feasibility of using fMRI-measured cortical activity has been demonstrated to predict deep-brain activity, showing a strong correlation with fNIRS measurements. Since many deep brain regions are anatomically and functionally connected with cortical surface regions (Bullmore and Sporns, 2009), this suggests that fNIRS, when combined with fMRI-informed models, can extend its utility beyond its typical limitation of superficial cortical sensitivity (Liu et al., 2015; Balters et al., 2023).
Future research should also focus on validating fNIRS measures, such as vHDR, against established clinical endpoints, including cognitive assessments (e.g., Bayley Scales of Infant Development, Wechsler Intelligence Scale for Children, Vineland Adaptive Behavior Scale, etc.) and motor function scales (e.g., Gross Motor Function Measure). This validation is essential for confirming the clinical relevance of fNIRS biomarkers and for establishing their ability to predict disease severity and treatment outcomes.
Data availability statement
The original contributions presented in the study are included in the article/supplementary material, further inquiries can be directed to the corresponding author.
Author contributions
ES: Conceptualization, Data curation, Methodology, Writing – original draft, Writing – review & editing. CB: Writing – original draft, Writing – review & editing. RB: Funding acquisition, Supervision, Writing – original draft, Writing – review & editing. MF: Funding acquisition, Supervision, Writing – original draft, Writing – review & editing. LB: Conceptualization, Data curation, Funding acquisition, Methodology, Supervision, Writing – original draft, Writing – review & editing.
Funding
The author(s) declare that financial support was received for the research and/or publication of this article. This work was supported by EJP RD JTC 2022 “Development of new analytic tools and pathways to accelerate diagnosis and facilitate diagnostic monitoring of rare diseases” under the EJP-Cofund Action on PM - N° 825575 and Italian Ministry of Health.
Conflict of interest
The authors declare that the research was conducted in the absence of any commercial or financial relationships that could be construed as a potential conflict of interest.
The author(s) declared that they were an editorial board member of Frontiers, at the time of submission. This had no impact on the peer review process and the final decision.
Generative AI statement
The authors declare that no Gen AI was used in the creation of this manuscript.
Publisher’s note
All claims expressed in this article are solely those of the authors and do not necessarily represent those of their affiliated organizations, or those of the publisher, the editors and the reviewers. Any product that may be evaluated in this article, or claim that may be made by its manufacturer, is not guaranteed or endorsed by the publisher.
References
Balters, S., Schlichting, M. R., Foland-Ross, L., Brigadoi, S., Miller, J. G., Kochenderfer, M. J., et al. (2023). Towards assessing subcortical “deep brain” biomarkers of PTSD with functional near-infrared spectroscopy. Cereb. Cortex 33, 3969–3984. doi: 10.1093/cercor/bhac320
Boldin, A. M., Geiger, R., and Emberson, L. L. (2018). The emergence of top-down, sensory prediction during learning in infancy: a comparison of full-term and preterm infants. Dev. Psychobiol. 60, 544–556. doi: 10.1002/dev.21624
Bortfeld, H., Wruck, E., and Boas, D. A. (2007). Assessing infants’ cortical response to speech using near-infrared spectroscopy. NeuroImage 34, 407–415. doi: 10.1016/j.neuroimage.2006.08.010
Budimirovic, D. B., Berry-Kravis, E., Erickson, C. A., Hall, S. S., Hessl, D., Reiss, A. L., et al. (2017). Updated report on tools to measure outcomes of clinical trials in fragile X syndrome. J. Neurodev. Disord. 9:14. doi: 10.1186/s11689-017-9193-x
Bullmore, E., and Sporns, O. (2009). Complex brain networks: graph theoretical analysis of structural and functional systems. Nat. Rev. Neurosci. 10, 186–198. doi: 10.1038/nrn2575
Cabrera, L., and Gervain, J. (2020). Speech perception at birth: the brain encodes fast and slow temporal information. Sci. Adv. 6:eaba7830. doi: 10.1126/sciadv.aba7830
Cai, T., Zhu, H., Xu, J., Wu, S., Li, X., and He, S. (2017). Human cortical neural correlates of visual fatigue during binocular depth perception: an fNIRS study. PLoS One 12:e0172426. doi: 10.1371/journal.pone.0172426
Cao, W., Zhu, H., Li, Y., Wang, Y., Bai, W., Lao, U., et al. (2021). The development of brain network in males with autism Spectrum disorders from childhood to adolescence: evidence from fNIRS study. Brain Sci. 11:120. doi: 10.3390/brainsci11010120
Chen, L. C., Stropahl, M., Schönwiesner, M., and Debener, S. (2017). Enhanced visual adaptation in cochlear implant users revealed by concurrent EEG-fNIRS. NeuroImage 146, 600–608. doi: 10.1016/j.neuroimage.2016.09.033
Chen, W.-L., Wagner, J., Heugel, N., Sugar, J., Lee, Y.-W., Conant, L., et al. (2020). Functional near-infrared spectroscopy and its clinical application in the field of neuroscience: advances and future directions. Front. Neurosci. 14:724. doi: 10.3389/fnins.2020.00724
Chung, C. C. Y., Chu, A. T. W., and Chung, B. H. Y. (2022). Rare disease emerging as a global public health priority. Front. Public Health 10:1028545. doi: 10.3389/fpubh.2022.1028545
Colier, W. N., Quaresima, V., Wenzel, R., van der Sluijs, M. C., Oeseburg, B., Ferrari, M., et al. (2001). Simultaneous near-infrared spectroscopy monitoring of left and right occipital areas reveals contra-lateral hemodynamic changes upon hemi-field paradigm. Vis. Res. 41, 97–102. doi: 10.1016/s0042-6989(00)00219-4
Conti, E., Scaffei, E., Bosetti, C., Marchi, V., Costanzo, V., Dell’Oste, V., et al. (2022). Looking for “fNIRS signature” in autism Spectrum: a systematic review starting from preschoolers. Front. Neurosci. 16:785993. doi: 10.3389/fnins.2022.785993
Coutts, L. V., Cooper, C. E., Elwell, C. E., and Wilkins, A. J. (2012). Time course of the haemodynamic response to visual stimulation in migraine, measured using near-infrared spectroscopy. Cephalalgia 32, 621–629. doi: 10.1177/0333102412444474
Cui, X., Bray, S., Bryant, D. M., Glover, G. H., and Reiss, A. L. (2011). A quantitative comparison of NIRS and fMRI across multiple cognitive tasks. NeuroImage 54, 2808–2821. doi: 10.1016/j.neuroimage.2010.10.069
de Tommaso, M., La Rocca, M., Quitadamo, S. G., Ricci, K., Tancredi, G., Clemente, L., et al. (2022). Central effects of galcanezumab in migraine: a pilot study on steady state visual evoked potentials and occipital hemodynamic response in migraine patients. J. Headache Pain 23, 52–14. doi: 10.1186/s10194-022-01421-z
Della Sala, G., and Pizzorusso, T. (2014). Synaptic plasticity and signaling in Rett syndrome. Dev. Neurobiol. 74, 178–196. doi: 10.1002/dneu.22114
Durand, S., Patrizi, A., Quast, K. B., Hachigian, L., Pavlyuk, R., Saxena, A., et al. (2012). NMDA receptor regulation prevents regression of visual cortical function in the absence of Mecp2. Neuron 76, 1078–1090. doi: 10.1016/j.neuron.2012.12.004
Emberson, L. L., Crosswhite, S. L., Richards, J. E., and Aslin, R. N. (2017). The lateral occipital cortex is selective for object shape, not texture/color, at six months. J. Neurosci. 37, 3698–3703. doi: 10.1523/JNEUROSCI.3300-16.2017
Emberson, L. L., Richards, J. E., and Aslin, R. N. (2015). Top-down modulation in the infant brain: learning-induced expectations rapidly affect the sensory cortex at 6 months. Proc. Natl. Acad. Sci. USA 112, 9585–9590. doi: 10.1073/pnas.1510343112
Gervain, J., Mehler, J., Werker, J. F., Nelson, C. A., Csibra, G., Lloyd-Fox, S., et al. (2011). Near-infrared spectroscopy: a report from the McDonnell infant methodology consortium. Dev. Cogn. Neurosci. 1, 22–46. doi: 10.1016/j.dcn.2010.07.004
Ghirardini, E., Calugi, F., Sagona, G., Di Vetta, F., Palma, M., Battini, R., et al. (2021). The role of preclinical models in Creatine transporter deficiency: neurobiological mechanisms, biomarkers and therapeutic development. Genes 12:123. doi: 10.3390/genes12081123
Gratton, G., Goodman-Wood, M. R., and Fabiani, M. (2001). Comparison of neuronal and hemodynamic measures of the brain response to visual stimulation: an optical imaging study. Hum. Brain Mapp. 13, 13–25. doi: 10.1002/hbm.1021
Haigh, S. M., Cooper, N. R., and Wilkins, A. J. (2015). Cortical excitability and the shape of the haemodynamic response. NeuroImage 111, 379–384. doi: 10.1016/j.neuroimage.2015.02.034
Heekeren, H. R., Kohl, M., Obrig, H., Wenzel, R., von Pannwitz, W., Matcher, S. J., et al. (1999). Noninvasive assessment of changes in cytochrome-c oxidase oxidation in human subjects during visual stimulation. J. Cereb. Blood Flow Metab. 19, 592–603. doi: 10.1097/00004647-199906000-00002
Heekeren, H. R., Obrig, H., Wenzel, R., Eberle, K., Ruben, J., Villringer, K., et al. (1997). Cerebral haemoglobin oxygenation during sustained visual stimulation--a near-infrared spectroscopy study. Philos. Trans. R. Soc. Lond. Ser. B Biol. Sci. 352, 743–750. doi: 10.1098/rstb.1997.0057
Hirshkowitz, A., Biondi, M., and Wilcox, T. (2018). Cortical responses to shape-from-motion stimuli in the infant. Neurophotonics 5:011014:1. doi: 10.1117/1.NPh.5.1.011014
Huang, Q., Yeung, M. K., Fong, K. N. K., and Chien, C.-W. (2024). Cortical activity during online motor control in children with and without developmental coordination disorder: a cross-sectional functional near-infrared spectroscopy study. J. Neuroeng. Rehabil. 21:160. doi: 10.1186/s12984-024-01465-z
Ishii, S., Kaga, Y., Tando, T., Aoyagi, K., Sano, F., Kanemura, H., et al. (2017). Disinhibition in children with attention-deficit/hyperactivity disorder: changes in [oxy-Hb] on near-infrared spectroscopy during “rock, paper, scissors” task. Brain Dev. 39, 395–402. doi: 10.1016/j.braindev.2016.12.005
Karen, T., Morren, G., Haensse, D., Bauschatz, A. S., Bucher, H. U., and Wolf, M. (2008). Hemodynamic response to visual stimulation in newborn infants using functional near-infrared spectroscopy. Hum. Brain Mapp. 29, 453–460. doi: 10.1002/hbm.20411
Kearns, G. L., and Artman, M. (2015). Functional biomarkers: an approach to bridge pharmacokinetics and pharmacodynamics in pediatric clinical trials. Curr. Pharm. Des. 21, 5636–5642. doi: 10.2174/1381612821666150901105337
Keehn, B., Wagner, J. B., Tager-Flusberg, H., and Nelson, C. A. (2013). Functional connectivity in the first year of life in infants at-risk for autism: a preliminary near-infrared spectroscopy study. Front. Hum. Neurosci. 7:444. doi: 10.3389/fnhum.2013.00444
Kojima, H., and Suzuki, T. (2010). Hemodynamic change in occipital lobe during visual search: visual attention allocation measured with NIRS. Neuropsychologia 48, 349–352. doi: 10.1016/j.neuropsychologia.2009.09.028
Krainc, D., Martin, W. J., Casey, B., Jensen, F. E., Tishkoff, S., Potter, W. Z., et al. (2023). Shifting the trajectory of therapeutic development for neurological and psychiatric disorders. Sci. Transl. Med. 15:eadg4775. doi: 10.1126/scitranslmed.adg4775
Kurane, K., Lin, N., Dan, I., Tanaka, H., Tsuji, Y., Ito, W., et al. (2024). Visualizing changes in cerebral hemodynamics in children with ADHD who have discontinued methylphenidate: a pilot study on using brain function for medication discontinuation decisions. Brain Dev. 46, 373–382. doi: 10.1016/j.braindev.2024.09.004
Kusaka, T., Kawada, K., Okubo, K., Nagano, K., Namba, M., Okada, H., et al. (2004). Noninvasive optical imaging in the visual cortex in young infants. Hum. Brain Mapp. 22, 122–132. doi: 10.1002/hbm.20020
LeBlanc, J. J., DeGregorio, G., Centofante, E., Vogel-Farley, V. K., Barnes, K., Kaufmann, W. E., et al. (2015). Visual evoked potentials detect cortical processing deficits in Rett syndrome. Ann. Neurol. 78, 775–786. doi: 10.1002/ana.24513
Liao, S. M., Gregg, N. M., White, B. R., Zeff, B. W., Bjerkaas, K. A., Inder, T. E., et al. (2010). Neonatal hemodynamic response to visual cortex activity: high-density near-infrared spectroscopy study. J. Biomed. Opt. 15:026010. doi: 10.1117/1.3369809
Liu, N., Cui, X., Bryant, D. M., Glover, G. H., and Reiss, A. L. (2015). Inferring deep-brain activity from cortical activity using functional near-infrared spectroscopy. Biomed. Opt. Express 6, 1074–1089. doi: 10.1364/BOE.6.001074
Lloyd-Fox, S., Blasi, A., and Elwell, C. E. (2010). Illuminating the developing brain: the past, present and future of functional near infrared spectroscopy. Neurosci. Biobehav. Rev. 34, 269–284. doi: 10.1016/j.neubiorev.2009.07.008
Lumsden, J. M., and Urv, T. K. (2023). The rare diseases clinical research network: a model for clinical trial readiness. Ther. Adv. Rare Dis. 4:26330040231219272. doi: 10.1177/26330040231219272
Maehara, G., Taya, S., and Kojima, H. (2007). Changes in hemoglobin concentration in the lateral occipital regions during shape recognition: a near-infrared spectroscopy study. J. Biomed. Opt. 12:062109. doi: 10.1117/1.2815720
Maggioni, E., Molteni, E., Zucca, C., Reni, G., Cerutti, S., Triulzi, F. M., et al. (2015). Investigation of negative BOLD responses in human brain through NIRS technique a visual stimulation study. NeuroImage 108, 410–422. doi: 10.1016/j.neuroimage.2014.12.074
Mazziotti, R., Cacciante, F., Sagona, G., Lupori, L., Gennaro, M., Putignano, E., et al. (2020). Novel translational phenotypes and biomarkers for creatine transporter deficiency. Brain Commun. 2:fcaa089. doi: 10.1093/braincomms/fcaa089
Mazziotti, R., Lupori, L., Sagona, G., Gennaro, M., Della Sala, G., Putignano, E., et al. (2017). Searching for biomarkers of CDKL5 disorder: early-onset visual impairment in CDKL5 mutant mice. Hum. Mol. Genet. 26, 2290–2298. doi: 10.1093/hmg/ddx119
Mazziotti, R., Scaffei, E., Conti, E., Marchi, V., Rizzi, R., Cioni, G., et al. (2022). The amplitude of fNIRS hemodynamic response in the visual cortex unmasks autistic traits in typically developing children. Transl. Psychiatry 12, 53–16. doi: 10.1038/s41398-022-01820-5
McPartland, J. C. (2016). Considerations in biomarker development for neurodevelopmental disorders. Curr. Opin. Neurol. 29, 118–122. doi: 10.1097/WCO.0000000000000300
Meek, J. H., Elwell, C. E., Khan, M. J., Romaya, J., Wyatt, J. S., Delpy, D. T., et al. (1995). Regional changes in cerebral haemodynamics as a result of a visual stimulus measured by near infrared spectroscopy. Proc. Biol. Sci. 261, 351–356. doi: 10.1098/rspb.1995.0158
Meek, J. H., Firbank, M., Elwell, C. E., Atkinson, J., Braddick, O., and Wyatt, J. S. (1998). Regional hemodynamic responses to visual stimulation in awake infants. Pediatr. Res. 43, 840–843. doi: 10.1203/00006450-199806000-00019
Miki, A., Nakajima, T., Takagi, M., Usui, T., Abe, H., Liu, C.-S. J., et al. (2005). Near-infrared spectroscopy of the visual cortex in unilateral optic neuritis. Am. J. Ophthalmol. 139, 353–356. doi: 10.1016/j.ajo.2004.07.042
Minati, L., Kress, I. U., Visani, E., Medford, N., and Critchley, H. D. (2011). Intra- and extra-cranial effects of transient blood pressure changes on brain near-infrared spectroscopy (NIRS) measurements. J. Neurosci. Methods 197, 283–288. doi: 10.1016/j.jneumeth.2011.02.029
Näsi, T., Kotilahti, K., Noponen, T., Nissilä, I., Lipiäinen, L., and Meriläinen, P. (2010). Correlation of visual-evoked hemodynamic responses and potentials in human brain. Exp. Brain Res. 202, 561–570. doi: 10.1007/s00221-010-2159-9
Obrig, H., Wenzel, R., Kohl, M., Horst, S., Wobst, P., Steinbrink, J., et al. (2000). Near-infrared spectroscopy: does it function in functional activation studies of the adult brain? Int. J. Psychophysiol. 35, 125–142. doi: 10.1016/s0167-8760(99)00048-3
Oyarzábal, A., Musokhranova, U., Lf, B., and García-Cazorla, A. (2021). Energy metabolism in childhood neurodevelopmental disorders. EBioMedicine 69:103474. doi: 10.1016/j.ebiom.2021.103474
Pinti, P., Siddiqui, M. F., Levy, A. D., Jones, E. J. H., and Tachtsidis, I. (2021). An analysis framework for the integration of broadband NIRS and EEG to assess neurovascular and neurometabolic coupling. Sci. Rep. 11, 1–20. doi: 10.1038/s41598-021-83420-9
Plichta, M. M., Heinzel, S., Ehlis, A.-C., Pauli, P., and Fallgatter, A. J. (2007). Model-based analysis of rapid event-related functional near-infrared spectroscopy (NIRS) data: a parametric validation study. NeuroImage 35, 625–634. doi: 10.1016/j.neuroimage.2006.11.028
Plichta, M. M., Herrmann, M. J., Baehne, C. G., Ehlis, A.-C., Richter, M. M., Pauli, P., et al. (2006b). Event-related functional near-infrared spectroscopy (fNIRS): are the measurements reliable? NeuroImage 31, 116–124. doi: 10.1016/j.neuroimage.2005.12.008
Plichta, M. M., Herrmann, M. J., Ehlis, A.-C., Baehne, C. G., Richter, M. M., and Fallgatter, A. J. (2006a). Event-related visual versus blocked motor task: detection of specific cortical activation patterns with functional near-infrared spectroscopy. Neuropsychobiology 53, 77–82. doi: 10.1159/000091723
Re, R., Messenio, D., Marano, G., Spinelli, L., Pirovano, I., Contini, D., et al. (2021). Monitoring the haemodynamic response to visual stimulation in glaucoma patients. Sci. Rep. 11, 13567–13511. doi: 10.1038/s41598-021-92857-x
Remijn, G. B., Kikuchi, M., Yoshimura, Y., Shitamichi, K., Ueno, S., Nagao, K., et al. (2011). Hemodynamic responses to visual stimuli in cortex of adults and 3- to 4-year-old children. Brain Res. 1383, 242–251. doi: 10.1016/j.brainres.2011.01.090
Rovati, L., Salvatori, G., Bulf, L., and Fonda, S. (2007). Optical and electrical recording of neural activity evoked by graded contrast visual stimulus. Biomed. Eng. Online 6:28. doi: 10.1186/1475-925X-6-28
Saby, J. N., Benke, T. A., Peters, S. U., Standridge, S. M., Matsuzaki, J., Cutri-French, C., et al. (2021). Multisite study of evoked potentials in Rett syndrome. Ann. Neurol. 89, 790–802. doi: 10.1002/ana.26029
Saby, J. N., Mulcahey, P. J., Zavez, A. E., Peters, S. U., Standridge, S. M., Swanson, L. C., et al. (2022). Electrophysiological biomarkers of brain function in CDKL5 deficiency disorder. Brain Commun. 4:fcac197. doi: 10.1093/braincomms/fcac197
Saby, J. N., Peters, S. U., Benke, T. A., Standridge, S. M., Swanson, L. C., Lieberman, D. N., et al. (2023). Comparison of evoked potentials across four related developmental encephalopathies. J. Neurodev. Disord. 15:10. doi: 10.1186/s11689-023-09479-9
Scaffei, E., Mazziotti, R., Conti, E., Costanzo, V., Calderoni, S., Stoccoro, A., et al. (2023). A potential biomarker of brain activity in autism Spectrum disorders: a pilot fNIRS study in female preschoolers. Brain Sci. 13:951. doi: 10.3390/brainsci13060951
Schroeter, M. L., Bücheler, M. M., Müller, K., Uludağ, K., Obrig, H., Lohmann, G., et al. (2004). Towards a standard analysis for functional near-infrared imaging. NeuroImage 21, 283–290. doi: 10.1016/j.neuroimage.2003.09.054
Sidorov, M. S., Deck, G. M., Dolatshahi, M., Thibert, R. L., Bird, L. M., Chu, C. J., et al. (2017). Delta rhythmicity is a reliable EEG biomarker in Angelman syndrome: a parallel mouse and human analysis. J. Neurodev. Disord. 9:17. doi: 10.1186/s11689-017-9195-8
Stauder, J. E. A., Smeets, E. E. J., van Mil, S. G. M., and Curfs, L. G. M. (2006). The development of visual- and auditory processing in Rett syndrome: an ERP study. Brain Dev. 28, 487–494. doi: 10.1016/j.braindev.2006.02.011
Strangman, G., Culver, J. P., Thompson, J. H., and Boas, D. A. (2002). A quantitative comparison of simultaneous BOLD fMRI and NIRS recordings during functional brain activation. NeuroImage 17, 719–731. doi: 10.1006/nimg.2002.1227
Su, W.-C., Colacot, R., Ahmed, N., Nguyen, T., George, T., and Gandjbakhche, A. (2023). The use of functional near-infrared spectroscopy in tracking neurodevelopmental trajectories in infants and children with or without developmental disorders: a systematic review. Front. Psych. 14:1210000. doi: 10.3389/fpsyt.2023.1210000
Taga, G., Asakawa, K., Hirasawa, K., and Konishi, Y. (2003b). Hemodynamic responses to visual stimulation in occipital and frontal cortex of newborn infants: a near-infrared optical topography study. Early Hum. Dev. 75, 203–210. doi: 10.1016/j.earlhumdev.2003.08.023
Taga, G., Asakawa, K., Maki, A., Konishi, Y., and Koizumi, H. (2003a). Brain imaging in awake infants by near-infrared optical topography. Proc. Natl. Acad. Sci. USA 100, 10722–10727. doi: 10.1073/pnas.1932552100
Takahashi, K., Ogata, S., Atsumi, Y., Yamamoto, R., Shiotsuka, S., Maki, A., et al. (2000). Activation of the visual cortex imaged by 24-channel near-infrared spectroscopy. J. Biomed. Opt. 5, 93–96. doi: 10.1117/1.429973
Thang, N. D., Van Toi, V., Tran, L. G., Tam, N. H. M., and Trinh, L. A. (2014). Investigation of human visual cortex responses to flickering light using functional near infrared spectroscopy and constrained ICA. J. Innov. Opt. Health Sci. 7:1450031. doi: 10.1142/s179354581450031x
Van Bergen, N. J., Massey, S., Stait, T., Ellery, M., Reljić, B., Formosa, L. E., et al. (2021). Abnormalities of mitochondrial dynamics and bioenergetics in neuronal cells from CDKL5 deficiency disorder. Neurobiol. Dis. 155:105370. doi: 10.1016/j.nbd.2021.105370
van de Rijt, L. P. H., van Opstal, A. J., Mylanus, E. A. M., Straatman, L. V., Hu, H. Y., Snik, A. F. M., et al. (2016). Temporal cortex activation to audiovisual speech in Normal-hearing and Cochlear implant users measured with functional near-infrared spectroscopy. Front. Hum. Neurosci. 10:48. doi: 10.3389/fnhum.2016.00048
Vanderwert, R. E., and Nelson, C. A. (2014). The use of near-infrared spectroscopy in the study of typical and atypical development. NeuroImage 85, 264–271. doi: 10.1016/j.neuroimage.2013.10.009
Villringer, A., Planck, J., Hock, C., Schleinkofer, L., and Dirnagl, U. (1993). Near infrared spectroscopy (NIRS): a new tool to study hemodynamic changes during activation of brain function in human adults. Neurosci. Lett. 154, 101–104. doi: 10.1016/0304-3940(93)90181-j
Ward, L. M., Aitchison, R. T., Tawse, M., Simmers, A. J., and Shahani, U. (2015). Reduced Haemodynamic response in the ageing visual cortex measured by absolute fNIRS. PLoS One 10:e0125012. doi: 10.1371/journal.pone.0125012
Ward, L. M., Morison, G., Simpson, W. A., Simmers, A. J., and Shahani, U. (2016). Using functional near infrared spectroscopy (fNIRS) to study dynamic stereoscopic depth perception. Brain Topogr. 29, 515–523. doi: 10.1007/s10548-016-0476-4
Watanabe, H., Homae, F., Nakano, T., and Taga, G. (2008). Functional activation in diverse regions of the developing brain of human infants. NeuroImage 43, 346–357. doi: 10.1016/j.neuroimage.2008.07.014
Watanabe, H., Homae, F., Nakano, T., Tsuzuki, D., Enkhtur, L., Nemoto, K., et al. (2013). Effect of auditory input on activations in infant diverse cortical regions during audiovisual processing. Hum. Brain Mapp. 34, 543–565. doi: 10.1002/hbm.21453
Watanabe, H., Homae, F., and Taga, G. (2010). General to specific development of functional activation in the cerebral cortexes of 2- to 3-month-old infants. NeuroImage 50, 1536–1544. doi: 10.1016/j.neuroimage.2010.01.068
Watanabe, H., Homae, F., and Taga, G. (2012). Activation and deactivation in response to visual stimulation in the occipital cortex of 6-month-old human infants. Dev. Psychobiol. 54, 1–15. doi: 10.1002/dev.20569
Werchan, D. M., Baumgartner, H. A., Lewkowicz, D. J., and Amso, D. (2018). The origins of cortical multisensory dynamics: evidence from human infants. Dev. Cogn. Neurosci. 34, 75–81. doi: 10.1016/j.dcn.2018.07.002
Wijeakumar, S., Shahani, U., Simpson, W. A., and McCulloch, D. L. (2012). Localization of hemodynamic responses to simple visual stimulation: an fNIRS study. Invest. Ophthalmol. Vis. Sci. 53, 2266–2273. doi: 10.1167/iovs.11-8680
Wilcox, T. (1999). Object individuation: infants’ use of shape, size, pattern, and color. Cognition 72, 125–166. doi: 10.1016/s0010-0277(99)00035-9
Wilcox, T., Bortfeld, H., Woods, R., Wruck, E., and Boas, D. A. (2005). Using near-infrared spectroscopy to assess neural activation during object processing in infants. J. Biomed. Opt. 10:011010. doi: 10.1117/1.1852551
Wilcox, T., Bortfeld, H., Woods, R., Wruck, E., and Boas, D. A. (2008). Hemodynamic response to featural changes in the occipital and inferior temporal cortex in infants: a preliminary methodological exploration. Dev. Sci. 11, 361–370. doi: 10.1111/j.1467-7687.2008.00681.x
Yasumura, A., Omori, M., Fukuda, A., Takahashi, J., Yasumura, Y., Nakagawa, E., et al. (2019). Age-related differences in frontal lobe function in children with ADHD. Brain Dev. 41, 577–586. doi: 10.1016/j.braindev.2019.03.006
Zhang, Y., Lin, X., Bi, A., Cao, N., Zhang, T., Wang, S., et al. (2022). Changes in visual cortical function in moderately myopic patients: a functional near-infrared spectroscopy study. Ophthalmic Physiol. Opt. 42, 36–47. doi: 10.1111/opo.12921
Zhang, F., Moerman, F., Niu, H., Warreyn, P., and Roeyers, H. (2022). Atypical brain network development of infants at elevated likelihood for autism spectrum disorder during the first year of life. Autism Res. 15, 2223–2237. doi: 10.1002/aur.2827
Keywords: fNIRS, visual stimulation, brain biomarker, neurodevelopmental disorders, X-linked rare disease
Citation: Scaffei E, Bosetti C, Battini R, Fagiolini M and Baroncelli L (2025) fNIRS as a biomarker for X-linked neurodevelopmental disorders: leveraging visual processing to assess brain function? Front. Neurosci. 19:1560786. doi: 10.3389/fnins.2025.1560786
Edited by:
Yusuke Takatsuru, Toyo University, JapanReviewed by:
Dejan Budimirovic, Johns Hopkins University, United StatesXubin Hou, Niigata University, Japan
Copyright © 2025 Scaffei, Bosetti, Battini, Fagiolini and Baroncelli. This is an open-access article distributed under the terms of the Creative Commons Attribution License (CC BY). The use, distribution or reproduction in other forums is permitted, provided the original author(s) and the copyright owner(s) are credited and that the original publication in this journal is cited, in accordance with accepted academic practice. No use, distribution or reproduction is permitted which does not comply with these terms.
*Correspondence: Elena Scaffei, ZWxlbmEuc2NhZmZlaUBmc20udW5pcGkuaXQ=