- 1Department of Translational Medicine, The Scripps Research Institute, La Jolla, CA, United States
- 2Department of Pharmacology, Universidade Federal de São Paulo, São Paulo, Brazil
- 3Animal Models Core, The Scripps Research Institute, La Jolla, CA, United States
Introduction: Stress is a risk factor for ethanol use disorders, which has been modeled by chronic intermittent ethanol (CIE) vapor exposure. Repeated stress alters CB1 receptor signaling, which could influence ethanol-related behaviors. Striatal CB1 receptors regulate D1-medium spiny neurons (D1-MSNs), involved in goal-directed behaviors and stress responses. This study tested the hypothesis that predator odor stress interacts with CIE exposure to: (1) increase or accelerate CIE-induced escalation in ethanol intake, (2) increase plasma corticosterone levels, and (3) increase the expression or co-localization of CB1 receptors, D1-MSNs, and Fos neuronal activation marker in the nucleus accumbens (NAc), dorsomedial (DMS), and dorsolateral (DLS) striatum.
Methods: Male C57BL/6J mice underwent three cycles of 4 days CIE or air exposure, alternated with 5 days ethanol access. During the last two cycles, mice were exposed to predator odor or control bedding before each drinking session. Following the last stressor, brains were processed for RNAscope to label Cnr1 (encodes CB1), Drd1 (D1), and Fos (Fos).
Results: As hypothesized, predator odor accelerated the CIE-induced increase in ethanol intake. Contrary to our expectations, CIE did not alter corticosterone levels after the final stressor. Compared to control bedding, predator odor reduced the percentage of Fos+ and triple-labeled Cnr1/Drd1/Fos+ cells in NAc, but not dorsal striatum. In addition, CIE vs. Air exposure, increased percentages of Fos+, double-labeled Cnr1/Fos+, Drd1/Fos+, and triple Cnr1/Drd1/Fos+ cells in the NAc, but not DMS or DLS.
Discussion: Thus, CIE and stress elicited opposite neuroactivational effects on CB1-regulated D1-MSNs of the NAc. The role of these changes in stress- and CIE-augmented drinking warrants further investigation.
1 Introduction
Ethanol is the most widely consumed addictive substance worldwide, and its harmful use is a risk factor for disease, disability, and mortality (World Health Organization [WHO], 2018). Chronic stress is a vulnerability factor for ethanol use disorders (Sinha, 2001, 2008). Perceived threats to safety are considered a fundamental source of stress for humans (Maslow, 1943; Zheng et al., 2016) and are linked to subsequently increased alcohol drinking and stress-related disorders (Grieger et al., 2003; Fullerton et al., 2015; Bradford et al., 2024). Animal models of perceived threats to safety, such as shock, social defeat, maternal separation, and predator stimuli have been widely used to identify the key determinants and underlying mechanisms of negative emotional behaviors and drinking associated with threat-related stress (Volpicelli et al., 1982; van Erp and Miczek, 2001; Logrip and Zorrilla, 2012; Logrip et al., 2012; Steinman et al., 2021; Finn et al., 2024; Ornelas and Besheer, 2024). Here, we employed a naturalistic predator odor exposure model that can increase ethanol intake in mice (Cozzoli et al., 2014; Finn et al., 2018; Finn et al., 2024) to identify striatal neuroadaptations that result from repeated threat.
Chronic intermittent ethanol (CIE) vapor exposure is another predictive model for long-term ethanol-related addictive behaviors (O’Dell et al., 2004; Vendruscolo and Roberts, 2014; Favoretto et al., 2024). Thus, CIE vapor exposure enhances ethanol self-administration in both mice and rats (Valdez et al., 2002; Chu et al., 2007). Additionally, withdrawal from CIE vapor is associated with increased anxiety- and depressive-like behaviors (Zhao et al., 2007; Serrano et al., 2018; Ferragud et al., 2021), as well as heightened aggression in rodents (Kimbrough et al., 2017; Somkuwar et al., 2017), resembling symptoms during ethanol abstinence in humans (Heilig et al., 2010).
Notably, the CIE model can be used to examine how ethanol exposure and stress interact. For example, exposure to repeated forced swim stress increased voluntary ethanol intake in mice subjected to CIE, but not in Air vapor controls (Lopez et al., 2016). Piggott et al. (2020) similarly found that while single-prolonged stress alone did not affect ethanol intake, mice subjected to both stress and CIE exhibited sustained increased ethanol consumption compared to controls. Here, we test the hypothesis that exposure to rat predator odor stress promotes increased ethanol intake in mice exposed to CIE. We also investigated whether a history of CIE affected plasma corticosterone response levels after the final session of predator odor stress.
The endocannabinoid (eCB) system consists of endocannabinoids, such as the fatty acid neuromodulators N-arachidonoylethanolamine (AEA) and 2-arachidonoylglycerol (2-AG); their typically Gi/o protein-coupled receptors, CB1 and CB2; and the respective enzymes for their biosynthesis and degradation (Navarrete et al., 2020). CB1 receptors, primarily located in axon terminals, mediate retrograde endocannabinoid signaling (Vaughan and Christie, 2005) and are highly expressed in brain regions associated with drug rewards, reinforcement, and addiction, including the striatum (Glass et al., 1997; Wang et al., 2003; Flores et al., 2013).
Chronic ethanol exposure impacts endocannabinoid-CB1-mediated pathways (DePoy et al., 2013; Serrano et al., 2018), neuroadaptations that may alter subsequent consummatory, addictive-like, or stress-related behavior (Parylak et al., 2012; Vozella et al., 2023). Stressors activate CB1 signaling to mitigate the physiological and behavioral consequences of acute stress exposure (Blasio et al., 2013; Hillard, 2014). Thus, like ethanol, repeated exposure to stress may also alter CB1-mediated endocannabinoid signaling, leading to long-term behavioral changes (Romano-López et al., 2012; Amancio-Belmont et al., 2019).
Within the striatum, CB1 receptors are found on various neuronal subpopulations, including dopamine D1- and D2-receptor positive medium spiny neuron (MSN) axon collaterals (Fitzgerald et al., 2012). D1-MSNs are implicated in promoting alcohol intake (Cheng et al., 2017), the reinforcing effects of addictive drugs, and responses to stress (Lobo and Nestler, 2011; Francis and Lobo, 2017; Favoretto et al., 2023). Exposure to cycles of voluntary alcohol intake, other addictive substances, and stressors can induce cellular and molecular changes in these subpopulations of striatal neurons that correlate with lasting behavioral outcomes (Lobo and Nestler, 2011; Lobo et al., 2013; Jeanes et al., 2014; Wang et al., 2015; Cheng et al., 2017; Francis and Lobo, 2017; Renteria et al., 2017, 2018). The subregional effects of CIE vapor and stress exposure on CB1 expression within striatal D1-MSNs are poorly understood. Thus, we here also aimed to test the hypothesis that exposure to CIE vapor and/or predator odor stress influences immediate-early gene and CB1 gene expression in D1-MSNs within the nucleus accumbens (NAc), dorsomedial striatum (DMS), and dorsolateral striatum (DLS). For that, we used RNAscope to label mRNAs that encode for CB1, D1, and Fos (Cnr1, Drd1, and Fos, respectively).
2 Materials and methods
2.1 Animals
Forty adult male C57BL/6J mice (9 weeks old) were purchased from Jackson Laboratories (Bar Harbor, ME) and group-housed (up to five mice per cage) in polycarbonate cages within a temperature-controlled room, maintained on a reverse 12 h light/dark cycle (lights on at 8:00 a.m.). Water and food (Envigo, Teklad Global 2018, 3.1 kcal/g; 24% kcal protein) were provided ad libitum, unless otherwise noted. Experimental procedures were carried out after approval by the Institutional Animal Care and Use Committee and met the NIH Guide for the Care and Use of Laboratory Animals.
2.2 Experimental design overview—2-bottle choice, CIE, and predator odor stress
Mice were first evaluated for baseline voluntary ethanol intake over 3 weeks in test cages (2 h/day, 5 days/week) using a limited access, two-bottle choice (2BC) paradigm [voluntary “choice” between 15% (w/v) ethanol and water]. For this, mice were individually studied in test cages (not their home cages), equipped with two graduated serological pipets with sipper tubes: one contained 15% ethanol (w/v) diluted in water and the other water. Liquid volumes were visualized before and after each session to calculate ethanol consumption and preference. Drinking sessions began 3–4 h into the dark cycle, and ethanol solutions were prepared weekly. By cage, mice were then assigned to either CIE or air vapor exposure, matched for ethanol intake (g/kg) during the 3rd baseline week.
After receiving a first cycle of 4 days ethanol vapor or air exposure, followed by a 5 days 2BC procedure for voluntary intake, mice were further subdivided into four groups: Control-Air, Stress-Air, Control-CIE, and Stress-CIE (n = 8–10 per group; two Stress-CIE mice died, reducing the final sample size of that group to eight) [respective assignments to Stress vs. Control conditions were matched based on ethanol intake (g/kg) during the 1st post-CIE cycle]. They then underwent two additional cycles of ethanol vapor or air exposure, followed by the 2BC procedure. During these 2nd and 3rd cycles, mice in the Stress-Air and Stress-CIE groups were exposed to predator odor stress for 30 min immediately before each 2BC session. Mice in the Control-Air and Control-CIE groups instead were placed in cages with clean bedding for 30 min before the 2BC tests. After the final stress session, mice were rapidly isoflurane-anesthetized and euthanized. Brains were flash-frozen in isopentane under dry ice and stored at −80°C. Trunk blood was collected, and plasma was stored at −80°C until ELISA for corticosterone levels [n = 9–10/group; all mice were included except for one Control-Air animal, which was omitted due to technical limitation (i.e., lack of space on the EIA plate)]. Brain tissue was sectioned using a cryostat, and slices were processed for RNAscope in situ hybridization to label mRNAs encoding for CB1, D1, and Fos (Cnr1, Drd1, and Fos, respectively), as shown in Figure 1A. For RNAscope assay, 4 mice per group (n = 4) were randomly selected, and their behavior was representative of the larger cohort (see Supplementary materials, page 2).
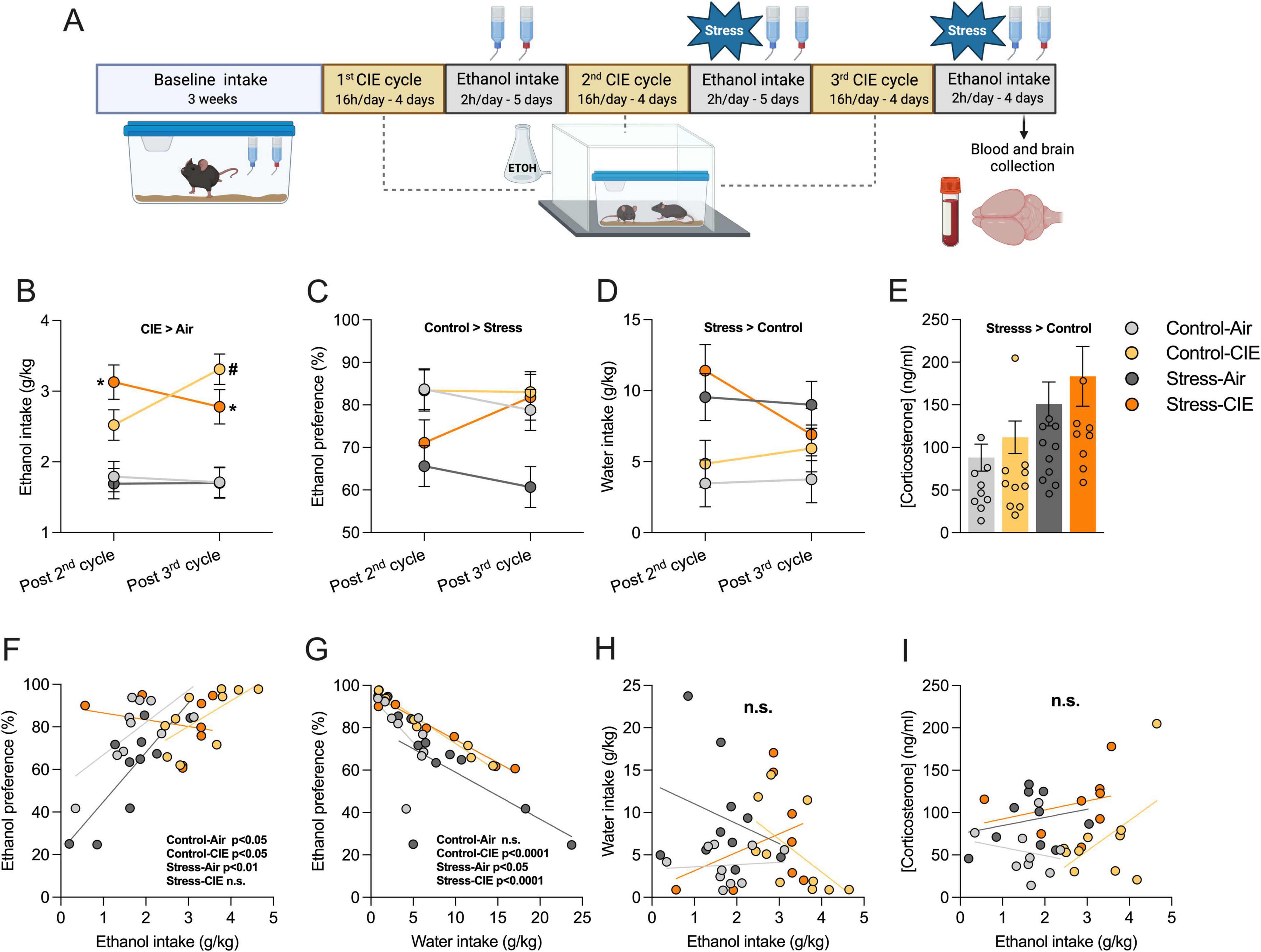
Figure 1. Predator odor stress accelerated chronic intermittent ethanol (CIE)-induced escalation of ethanol intake, reduced ethanol preference, enhanced water intake, and increased plasma corticosterone regardless of CIE. (A) Adult male C57BL/6J mice (n = 8–10/group) were subjected to 3 cycles of 4 days exposure to CIE vapor or air, alternated with 5 days access to 2-bottle choice voluntary ethanol intake (15% w/v). During the last 2 drinking cycles, mice received 30 min of predator odor stress or control cage exposure immediately before each drinking session. Immediately after the last stress/control episode, mice were anesthetized and sacrificed. Brain and blood samples were collected. Brains were sliced and processed for RNAscope in situ hybridization. Plasma corticosterone concentrations were determined using an ELISA assay (Created with BioRender.com). (B) Estimated marginal means for average ethanol intake (g/kg) following 2nd and 3rd CIE vapor cycles. Mixed Models analysis for CIE (CIE × Air), Stress (Stress × Control), and Time (post 2nd x post 3rd cycle) factors, and average baseline ethanol intake (g/kg) as a covariate detected a main CIE effect (p < 0.05; CIE > Air) and CIE × Stress × Time interaction. Post hoc test revealed that CIE exposure increased ethanol intake by the 3rd cycle in Stress-CIE (*p < 0.05) and Control-CIE (#p < 0.05) groups relative to their respective Air controls. Also, stressed CIE mice presented increased ethanol intake vs. their stressed Air controls earlier - by the 2nd cycle (*p < 0.05). (C) Estimated marginal means for average preference for ethanol solution (%). Mixed Models showed main effect of Stress (p < 0.05; Control > Stress). (D) Estimated marginal means for average water intake (g/kg). Mixed Models detected a significant main Stress effect (p < 0.05; Stress > Control). (E) Corticosterone concentration (ng/ml) following the 10th stress or control condition episode in mice exposed to CIE or Air. Generalized Linear Models analysis showed a main Stress effect (p < 0.05; Stress > Control). (F–I) Pearson correlation analyses showing relationships between ethanol intake, ethanol preference, water intake, and corticosterone levels. Significant correlations are indicated. Data are shown as estimated marginal means ± pooled standard errors (B–D). Individual data points are also shown in panel (E).
2.3 Chronic intermittent ethanol (CIE) vapor exposure and 2BC intake
Vapor chambers consisted of acrylic cages holding up to five mice (La Jolla Alcohol Research Inc., La Jolla, CA, United States). Ethanol vapor was generated by introducing 95% ethanol into a vacuum flask at 50°C, with air blowing over it at 11 L/min. Ethanol vapor exposure aimed to achieve blood ethanol concentrations of 100–250 mg/dl. Before exposure, the CIE group received an intraperitoneal (i.p.) injection of ethanol at the dose of 1.75 g/kg and pyrazole at the dose of 68.1 mg/kg (ethanol dehydrogenase inhibitor, Sigma-Aldrich) to reach pharmacologically relevant blood ethanol levels. The Air groups received pyrazole diluted in saline. In each exposure cycle, mice were exposed to vapor for 16 h (3:00 p.m. – 7:00 a.m.; with 8 h off) over 4 consecutive days, followed by 3 days undisturbed in their home cages. In the following days, mice were then given 2 h access to the 2BC procedure (15% ethanol vs. water) for 5 consecutive days. This 4 days CIE or air exposure protocol, followed by the 5 days 2BC procedure, was repeated for 3 cycles (Becker and Lopez, 2004; Lopez and Becker, 2005; Griffin et al., 2009a,2009b; Huitron-Resendiz et al., 2018), with repeated predator stress beginning during the 2nd cycle. Tail blood alcohol levels (BALs) at session end were measured using gas chromatography after each ethanol vapor exposure week. In the Control-CIE group, blood alcohol levels [BALs (mg/dl); M ± SEM] were 114.6 ± 6.2, 153.4 ± 8.5, and 222.6 ± 10.3 for the 1st, 2nd, and 3rd cycles, respectively. In the Stress-CIE group, averages were 90.2 ± 8.6, 150.7 ± 11.7, and 216.2 ± 15.9, respectively.
2.4 Exposure to predator odor stress
For the predator odor stress procedure, the experimental mice were removed from their home cages and introduced into polycarbonate cages filled with soiled shavings from adult rats for 30 min, without food and water (Cozzoli et al., 2014). The soiled rat bedding was collected from the cages of adult male and female rats, both same-sex and mate-housed, that had been unchanged for 1 week (most were Wistar strain rats). Control mice were placed in identical cages filled with clean shavings (Envigo/Inotiv 7090 Aspen Sani Chip Bedding) for 30 min, without food and water.
2.5 Determination of plasma corticosterone concentrations
Immediately after the final (10th) predator bedding or control cage exposure, mice were rapidly anesthetized with isoflurane, decapitated, and trunk blood was collected into wet-ice chilled, EDTA-coated plastic tubes. The blood was centrifuged for 15 min at 3,000 × g (4°C) to separate the plasma, which was then stored at −80°C until analysis. Corticosterone concentrations were measured in duplicate using the ELISA DetectX® Corticosterone Enzyme Immunoassay Kit (Arbor Assays, Ann Arbor, MI, #K014-H), according to the manufacturer’s instructions. The kit has an intra-assay coefficient of variation (CV) of less than 10%. Plasma samples were diluted 1:400 with the assay buffer provided in the kit.
2.6 In situ hybridization (RNAscope™)
At sacrifice, brains were also collected, snap-frozen in dry-ice chilled isopentane, and stored at −80°C. Brains for a random, behaviorally-representative subset of subjects (n = 4/group; see Supplementary materials) were coronally cryosectioned (−20°C, 20 μm) and mounted directly on Super Frost Plus slides (Fisher Scientific). Slides were at −20°C for ∼10 min and stored at −80°C until the in situ hybridization assay. For the assay, slides containing brain slices were immersed in 10% neutral buffered formalin (Fisher Scientific) for 20 min at 4°C, washed twice (∼15 s, 1x PBS), and successively dehydrated (5 min/concentration) in 50%, 70%, then 100% ethanol (the latter twice). Slides were dried at room temperature (10 min, ∼22°C), and a hydrophobic pen (ImmEdger Hydrophobic Barrier Pen, Vector Laboratories) was used to make a physical barrier around the brain sections to contain the RNAscope assay solution. We used the Advanced Cell Diagnostics HybEZ Hybridization System. The protease solution (pretreatment solution four) was incubated with sections at room temperature for 20 min. After washing, the sections were incubated with target probes for specific mRNAs (Fos, Cnr1, and Drd1; catalog #316921, 420721-C2, 461901-C3, respectively) at 40°C, for 2 h, in the HybEZ oven. The sections were then incubated with pre-amplification and amplification probes applying AMP1 (40°C for 30 min), AMP2 (40°C for 30 min), and AMP3 (40°C for 15 min). Then, the sections were incubated with the fluorescently labeled probes far red (TSA vivid fluorophore 650 nm), red (TSA vivid fluorophore 570 nm), and green (TSA vivid fluorophore 520 nm). Finally, sections were incubated for 20 s with DAPI to label nuclei (blue), mounted with ProLong Gold Antifade Mounting medium (Thermo Fisher Scientific, #P36930), and kept at 4°C. Positive and negative control sections were also run.
For DMS and DLS subregions, coronal sections ranging approximately from anteroposterior coordinates +1.1 to 0.38 mm (from bregma, per Franklin and Paxinos, The Mouse Brain in Stereotaxic Coordinates, 3rd edition) were imaged. For the NAc subregion, a narrower interval was considered (+1.1 to 0.62 mm due to the more anterior limit of this structure). Four images (two coronal slices with two brain hemispheres each) were acquired per animal using a laser scanning confocal microscope (Zeiss LSM 710) with the objective of 40 × magnification. Each image comprised four tiles and seven z-stacks, each stack with 0.85 μm of thickness. Image acquisition parameters were kept the same across all images. Analysis was performed using QuPath software (Bankhead et al., 2017) - a Random trees (Rtrees) classifier was trained by annotating cells as positive or negative, trained on the selected feature Nucleus: channel# Mean. Classifier performance was then applied to all images in batch mode. Cells were identified based on nuclei labeled with DAPI. The percentage of positive cells for each probe (or a combination of probes) was obtained by dividing the number of cells expressing the probe by the number of nuclei stained with DAPI.
Results are presented as means of the variables of interest calculated for each animal based on the data obtained from each image. An experimenter, blind to treatment groups, excluded images of questionable quality, such as those with high background or channel bleed-through that led to inaccurate cell classification or false-positive signal detection. For the NAc subregion, all available images from four mice were deemed questionable image quality (n = 2) or too posterior (n = 2) (implicating that the NAc subregion was not present at that coordinate level) and technically excluded from the NAc analysis. Sensitivity analysis showed that exclusion of these mice from the dorsal striatum analysis did not substantively change inferential results (not shown).
Additionally, images of the cingulate cortex (one tile and seven z-stacks, each stack with 0.85 μm of thickness), a region reciprocally connected to the dorsal striatum and NAc, that links emotional and motivational states with action selection and motor control (Reep et al., 2003; Tewari et al., 2016), were analyzed for percentage of Cnr1+, Fos+, and double-labeled (dual) Cnr1/Fos+ cells. Respective results are presented in Supplementary materials (page 1). Representative images shown in Figures 2–4 and Supplementary Figure 1 were uniformly adjusted for brightness for visualization purposes only. Raw images were used for quantitative analyses.
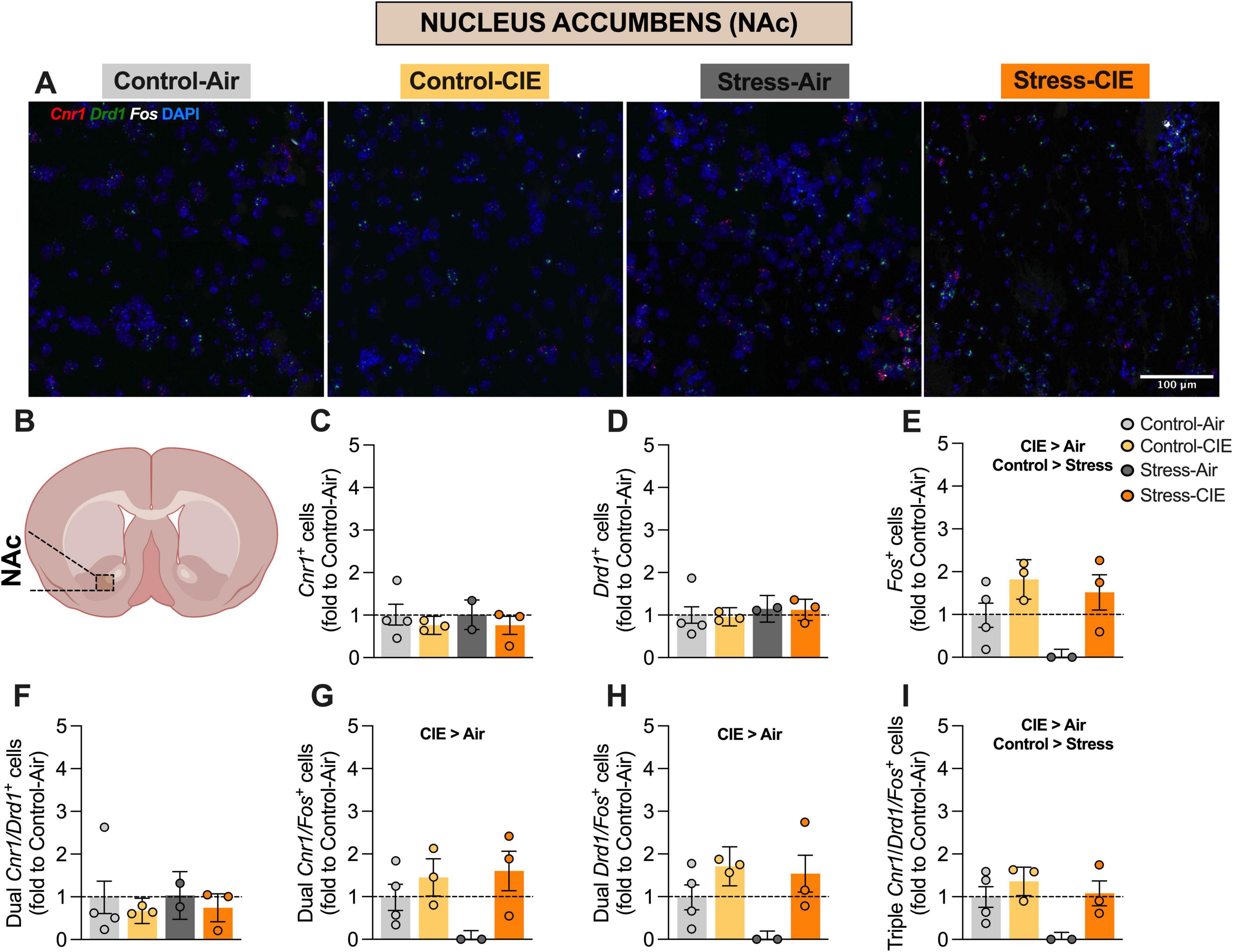
Figure 2. Predator odor stress decreased the percentage of Fos+ and Cnr1/Drd1/Fos+ cells, while chronic intermittent ethanol (CIE) increased the percentage of Fos+, Cnr1/Fos+, Drd1/Fos+, and triple-labeled cells in the nucleus accumbens (NAc). (A) Representative images of NAc cells from the different experimental groups expressing Cnr1 (in red), Drd1 (in green), and Fos (in white), plus DAPI (in blue). (B) Diagram of a mouse brain, with NAc highlighted (Created with BioRender.com). Percentage (fold to Control-Air) of Cnr1+cells (C), Drd1+ cells (D), Fos+ cells (E), dual Cnr1/Drd1+ cells (F), dual Cnr1/Fos+ cells (G), dual Drd1/Fos+ cells (H), and triple Cnr1/Drd1/Fos+ cells (I). Generalized Linear Model Analysis found a significant main effect of CIE (CIE > Air) for the percentage of Fos+ (E), dual Cnr1/Fos+ (G), dual Drd1/Fos+ (H), and triple Cnr1/Drd1/Fos+ (I) cells. Additionally, a main effect of Stress was detected for the percentage of Fos+ (E) and of triple Cnr1/Drd1/Fos+ cells (I). Data are shown as estimated marginal means ± pooled standard errors, with individual data points overlaid as scatter plots.
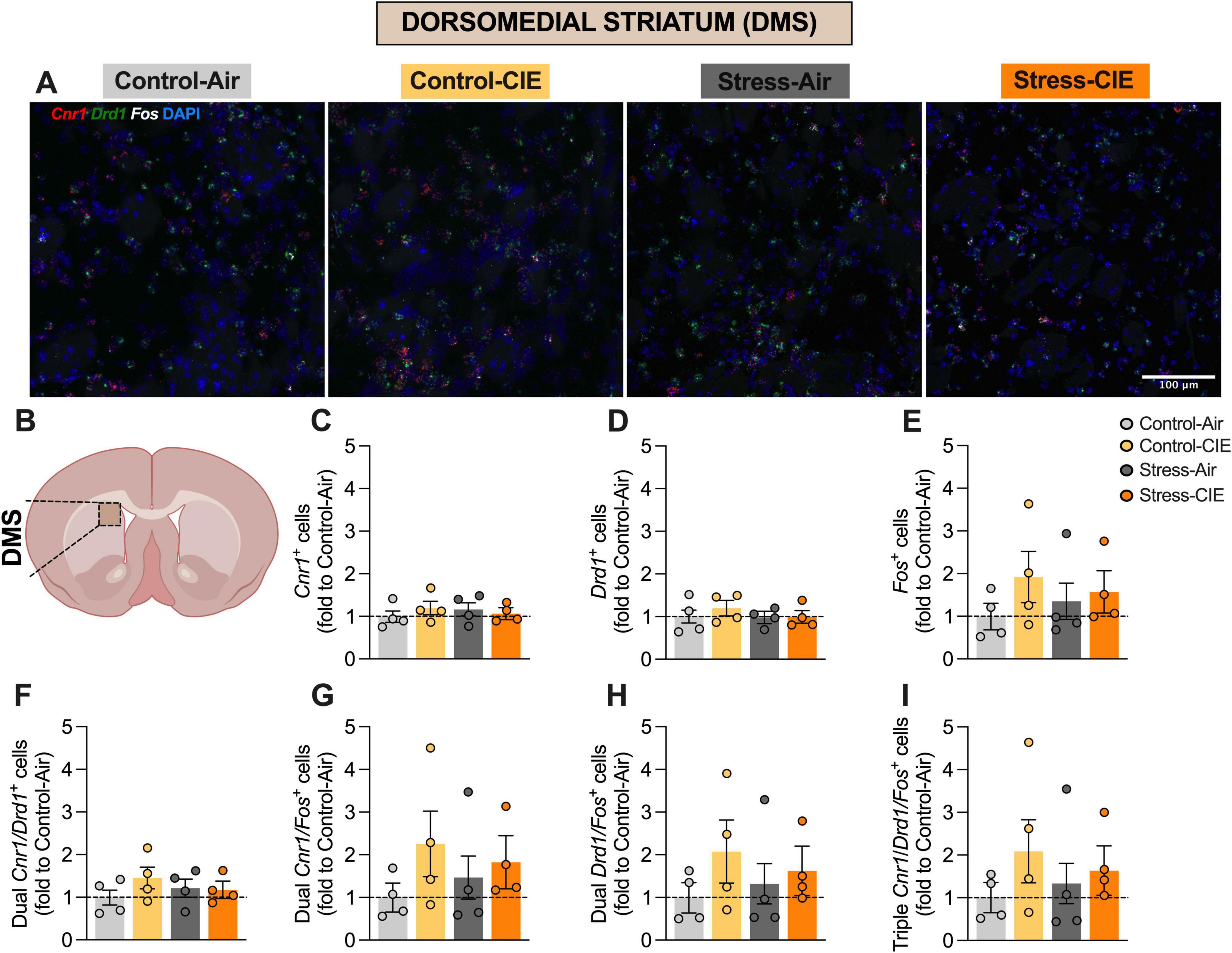
Figure 3. Neither predator odor stress nor chronic intermittent ethanol (CIE) exposure altered the percentage of Cnr1+, Drd1+, or Fos+ cells, or their colocalizations, in the dorsomedial striatum (DMS). (A) Representative images of DMS cells from the different experimental groups expressing Cnr1 (in red), Drd1 (in green), and Fos (in white), plus DAPI (in blue). (B) Diagram of a mouse brain, with DMS highlighted (Created with BioRender.com). Percentage (fold to Control-Air) of Cnr1+ cells (C), Drd1+ cells (D), Fos+ cells (E), dual Cnr1/Drd1+ cells (F), dual Cnr1/Fos+ cells (G), dual Drd1/Fos+ cells (H), and triple Cnr1/Drd1/Fos+ cells (I). Generalized Linear Model Analysis did not detect any significant effects involving CIE or Stress for any of the assessed variables. Data are shown as estimated marginal means ± pooled standard errors, with individual data points overlaid as scatter plots.
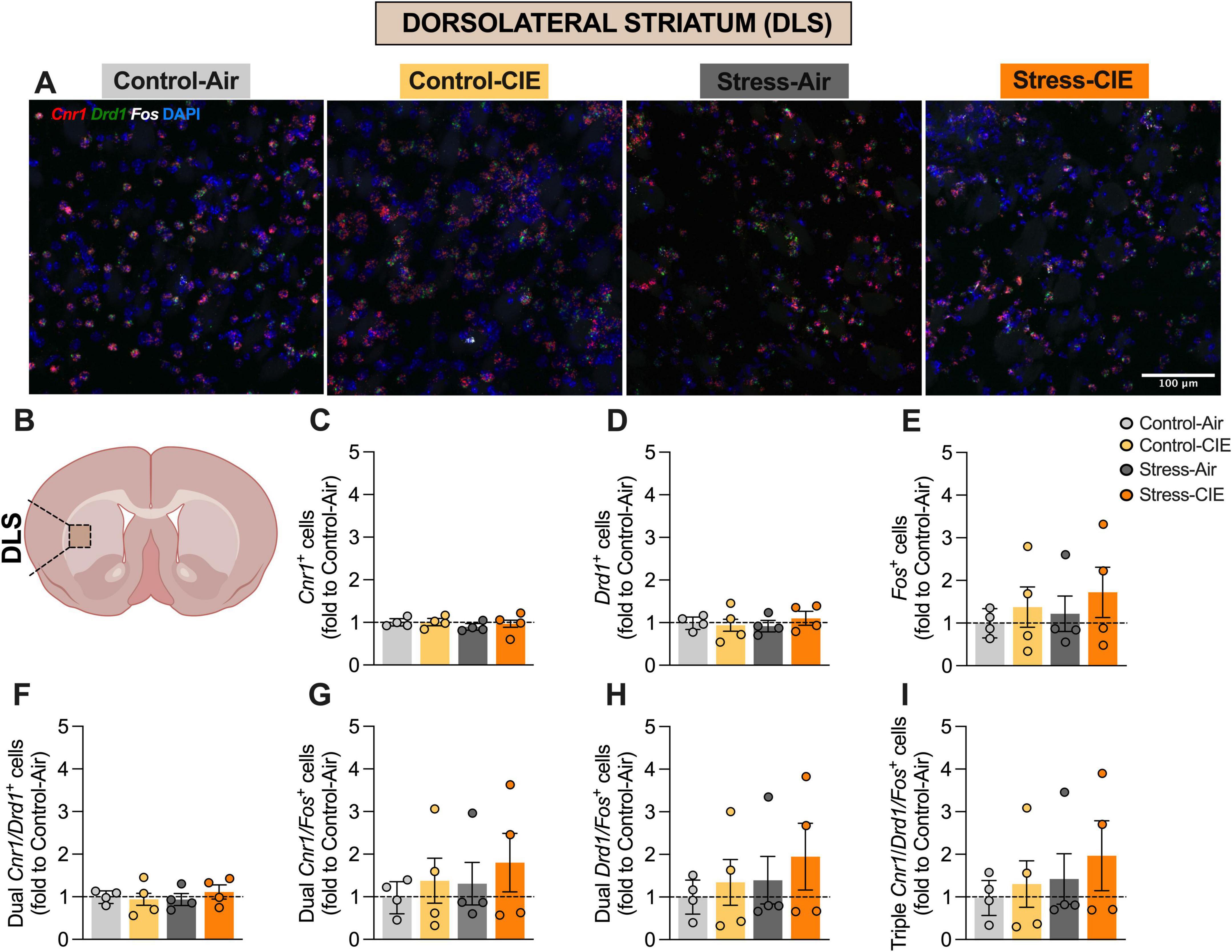
Figure 4. Neither predator odor stress nor chronic intermittent ethanol (CIE) exposure altered the percentage of Cnr1+, Drd1+, or Fos+ cells, or their colocalizations, in the dorsolateral striatum (DLS). (A) Representative images of DLS cells from the different experimental groups expressing Cnr1 (in red), Drd1 (in green), and Fos (in white), plus DAPI (in blue). (B) Diagram of a mouse brain, with DLS highlighted (Created with BioRender.com). Percentage (fold to Control-Air) of Cnr1+ cells (C), Drd1+ cells (D), Fos+ cells (E), dual Cnr1/Drd1+ cells (F), dual Cnr1/Fos+ cells (G), dual Drd1/Fos+ cells (H), and triple Cnr1/Drd1/Fos+ cells (I). Generalized Linear Model Analysis did not detect any effects of CIE, Stress, or interaction between these factors for any of the assessed variables. Data are shown as estimated marginal means ± pooled standard errors, with individual data points overlaid as scatter plots.
2.7 Statistical analysis
Behavioral data were analyzed using Mixed Models to assess the effects of CIE, Stress, and Time, with baseline intake or preference included as a covariate and subject treated as a cluster variable. Significant interactions identified through Mixed Models were further analyzed using Holm post hoc tests. Corticosterone and RNAscope data were analyzed using Generalized Linear Models, with CIE and Stress as fixed factors, employing a gamma distribution with a log link function. For RNAscope data, dependent variables were calculated as fold-change (vs. Control-Air) percentages for Cnr1+, Drd1+, Fos+, double-labeled (dual Cnr1/Drd1+, Cnr1/Fos+, and Drd1/Fos+), and triple-labeled (triple Cnr1/Drd1/Fos+) cells. A constant value (1) was added to variables containing zero values to allow modeling with the gamma distribution. Following Schmettow (2021), the choice of distribution and link function was based on the data structure — specifically, the use of gamma distribution was appropriate for continuous, non-negative skewed data without upper bounds but with lower boundaries = zero. The gamma distribution yielded a smaller AIC than the Gaussian in 21 out of 25 cases (the 25 comparisons included all RNAscope variables for the NAc, DMS, DLS, and cingulate cortex, as well as corticosterone levels) which is significantly more frequent than the 50% expected by chance (z = 3.4, p < 0.001; exact binomial p < 0.001). Moreover, for each of the striatal subregions, t-tests comparing AIC values obtained from Generalized Linear Model analyses that used gamma vs. Gaussian distributions showed that, overall, the mean AIC across analyses for the gamma distribution was significantly lower than that for the Gaussian distribution. This result supports that the cross-sectional data were modeled better by a gamma than Gaussian distribution.
Pearson correlations were performed to evaluate relationships amongst intake, preference, and corticosterone levels. Correlations were conducted in GraphPad Prism (version 10). All other statistical analyses were performed in Jamovi (version 2.3). Statistical significance was defined as p ≤ 0.05.
3 Results
Predator odor stress accelerated CIE-induced increase of ethanol intake: A three-way (CIE, Stress, Time) mixed models analysis covarying for baseline ethanol intake, revealed a significant main effect of CIE [B = 1.21, 95% CI (0.83, 1.60), p < 0.001, CIE > Air] and a significant CIE × Stress × Time interaction [B = −1.22, 95% CI (−2.03, −0.42), p = 0.005]. The model equation was: Y = 2.33 + 1.21⋅(CIE)–0.01⋅(Stress) + 0.09⋅(Time) + 0.55⋅(Baseline) + 0.1⋅(CIE × Stress) + 0.25⋅(CIE × Time)–0.52⋅(Stress × Time)–1.22⋅(CIE × Stress × Time). Holm post hoc tests to interpret the interaction showed that whereas CIE exposure increased ethanol intake vs. respective Air controls by the 3rd cycle in both the Stress-CIE (p = 0.030) and Control-CIE (p < 0.001) groups, only Stress-CIE mice exhibited increased ethanol intake compared to Stress-Air by the 2nd cycle (p = 0.001). Additionally, Control-CIE mice had higher ethanol intake post-3rd cycle compared to post-2nd cycle (p = 0.008), as shown in Figure 1B. Of note, ethanol consumption following the 2nd and 3rd CIE cycles was not compared to drinking after the 1st CIE cycle because exposure to predator odor or control cages only began after the 2nd CIE cycle.
Predator stress reduced ethanol preference by increasing water intake even more than ethanol intake: In terms of ethanol preference (%), a three-way mixed models test covarying for baseline ethanol preference revealed significant main effect of Stress [B = −12.43, 95% CI (−20.39, −4.47), p = 0.004; Control > Stress], as shown in Figure 1C. The model equation was: Y = 75.99 + 7.62⋅ (CIE)–12.43⋅ (Stress) + 0.11⋅ (Time) + 0.32⋅ (Baseline) + 11.34⋅ (CIE × Stress) + 10.09⋅ (CIE × Time) + 5.55⋅ (Stress × Time) + 11.17⋅ (CIE × Stress × Time). Finally, three-way mixed models covarying for baseline water intake also detected a significant main effect of Stress on water intake [B = 4.72, 95% CI (1.98, 7.46), p = 0.002; Stress > Control], as depicted in Figure 1D. The model equation was: Y = 6.86 + 0.83⋅ (CIE) + 4.72⋅ (Stress)–0.92⋅ (Time) + 0.35⋅ (Baseline)–1.90⋅ (CIE × Stress)–1.59⋅ (CIE × Time)−3.19⋅ (Stress × Time)–4.77⋅ (CIE × Stress × Time).
Because ethanol preference and water intake were not normally distributed (p < 0.05 Shapiro-Wilk for normality of residuals), we also analyzed data after applying a Box-Cox transformation to ethanol preference and a Log10 transformation to water intake - both resulting in normally distributed residuals (Shapiro-Wilk p > 0.05). The inferential outcomes were substantively unchanged, with a main effect of Stress still observed for both measures.
Predator odor stress exposed mice had increased plasma corticosterone levels, regardless of CIE history: A two-way Generalized Linear Model (factors: CIE, Stress) revealed a significant main effect of Stress [B = −0.52, 95% CI (−0.87, −0.17), p = 0.007]. The model equation was: Y = 4.35–0.52⋅Stress + 0.22⋅CIE + 0.04⋅(Stress × CIE). This indicates that stressed mice, regardless of prior CIE or air exposure, exhibited higher mean corticosterone levels after the 10th stress episode compared to control mice (Figure 1E).
Stress-CIE disrupted the direct correlation of ethanol preference to intake: Ethanol intake correlated significantly and directly with ethanol preference (Figure 1F) in the Control-Air (r = 0.68; p = 0.029), Control-CIE (r = 0.66; p = 0.037), and Stress-Air (r = 0.81; p = 0.004), but not Stress-CIE group (r = −0.23; p = 0.587). Pairwise comparisons of correlations from independent samples showed that the magnitude of correlation within Stress-CIE mice differed significantly from other groups (Table 1).
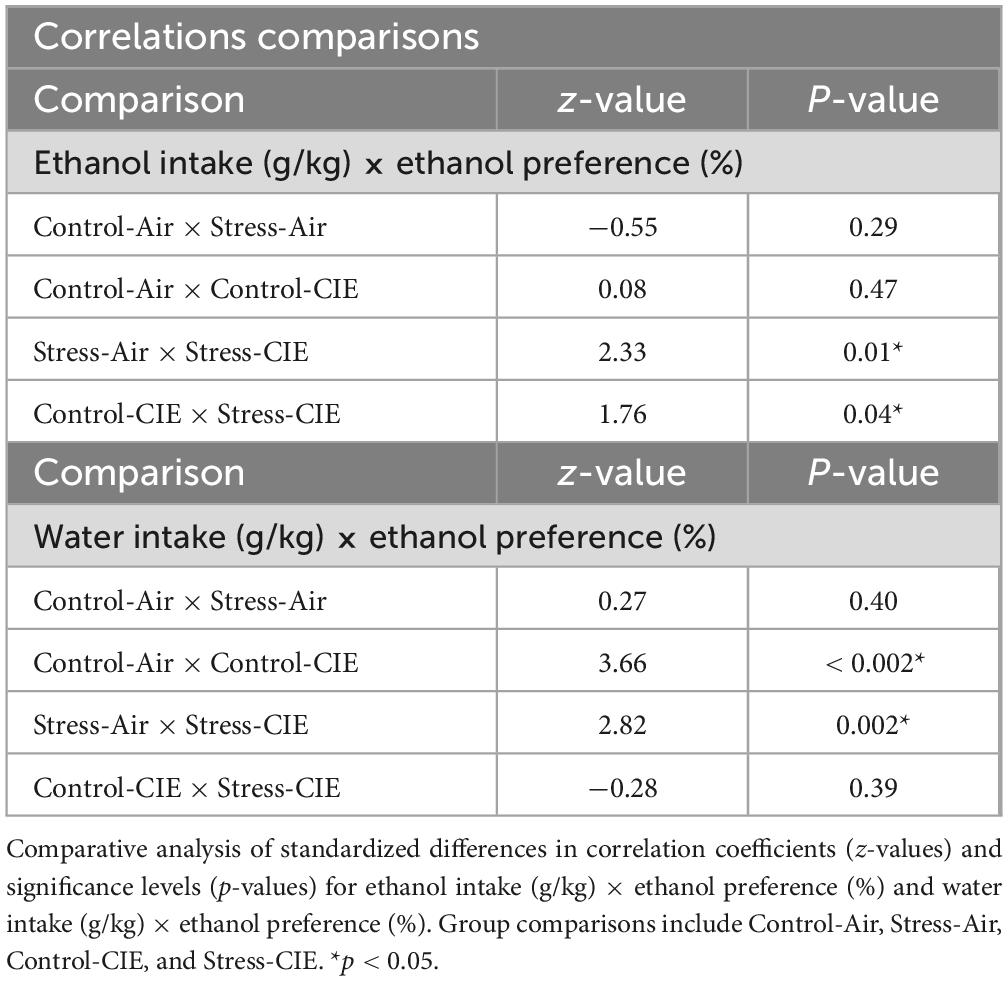
Table 1. Chronic intermittent ethanol (CIE) and Stress history alter correlations of ethanol preference to ethanol and water intake.
CIE strengthened the inverse correlation of ethanol preference to water intake: Water intake and ethanol preference (Figure 1G) were strongly inversely correlated in CIE groups (Control-CIE, r = −0.99; p < 0.0001; Stress-CIE, r = −0.99; p < 0.0001) and moderately in Air groups (Stress-Air, r = −0.66; p = 0.039; Control-Air group, r = −0.57; p = 0.086). Pairwise comparisons of correlations from independent samples showed that the strength of correlation within CIE groups was significantly greater than in their respective Air control group (Table 1).
Ethanol intake not correlated to water or corticosterone levels: Ethanol and water intakes (Figure 1H) did not correlate significantly in any group. There was no significant correlation of ethanol intake (Figure 1I) or preference (not shown) with corticosterone concentration in any group.
Predator odor stress reduced, while CIE exposure increased, the percentage of Fos+ and triple Cnr1/Drd1/Fos+ cells in the NAc, but not dorsal striatum: For NAc data, Generalized Linear Model revealed significant main effects of CIE [B = 0.64, 95% CI (0.31, 0.97), p = 0.005] and Stress [B = −0.40, 95% CI (−0.73, −0.07), p = 0.044] for the percentage of Fos+ cells [model equation: Y = 0.67–0.40⋅(Stress) + 0.64⋅(CIE) + 0.58⋅(Stress × CIE)]. For the percentage of dual Cnr1/Fos+ cells, Generalized Linear Model analysis found a CIE effect [B = 0.59, 95% CI (0.22, 0.94), p = 0.013, equation: Y = 0.64–0.32⋅(Stress) + 0.59⋅(CIE) + 0.75⋅(Stress × CIE)]. Considering the percentage of dual Drd1/Fos+ cells, analysis also detected a main CIE effect [B = 0.63, 95% CI (0.28, 0.97), p = 0.007, equation: Y = 0.66–0.38⋅(Stress) + 0.63⋅(CIE) + 0.63⋅(Stress × CIE)]. Finally, for the percentage of triple Cnr1/Drd1/Fos+ cells, Generalized Linear Model analysis revealed main effects of CIE [B = 0.45, 95% CI (0.17, 0.74), p = 0.014] and Stress [B = −0.41, 95% CI (−0.69, −0.12), p = 0.022]. The model equation was Y = 0.57–0.41⋅(Stress) + 0.45⋅(CIE) + 0.57⋅(Stress × CIE). In all cases, CIE increased expression of the relevant cell population, while exposure to predator odor stress decreased this percentage as compared to control bedding exposure (Figure 2).
No significant effects were detected for the percentage of Cnr1+, Drd1+ cells, or dual Cnr1/Drd1+ cells in the NAc. No significant effects involving CIE or Stress were observed in the DMS or DLS striatal subregions (Figures 3, 4).
No significant effects involving CIE or Stress were seen on the percentage of Cnr1+, Fos+, and dual Cnr1/Fos+ cells in the cingulate cortex (Supplementary Figure 1).
4 Discussion
The present study found that predator odor stress accelerated CIE-induced escalation of ethanol intake and reduced the percentage of NAc Fos+ and triple-labeled Cnr1/Drd1/Fos+ cells. In contrast, CIE vapor exposure increased proportions of Fos+, dual Cnr1/Fos+ and Drd1/Fos+, and triple Cnr1/Drd1/Fos+ cells in the NAc. As expected and consistent with previous reports (Griffin et al., 2023; Macedo et al., 2023; Okhuarobo et al., 2020; Xiao et al., 2023), control mice that were not exposed to predator stress developed increased ethanol intake after three cycles of CIE vapor exposure. Supporting our hypothesis, predator odor exposure accelerated the CIE-induced increase in ethanol consumption. Specifically, the Stress-CIE group, but not the Control-CIE group, showed significantly higher ethanol intake compared to the Stress-Air mice after only two cycles of CIE exposure, a difference reflected in a CIE × Stress × Time interaction. This result aligns with findings by Finn et al. (2018), who observed that rat bedding stress exacerbated ethanol intake in male mice with a history of binge drinking, but not in control mice not previously exposed to ethanol. Also similar to the present findings, Rodberg et al. (2017) reported that forced swim stress increased ethanol intake only in mice subjected to CIE vapor, but not in Air controls.
Whereas RNAscope in situ hybridization showed that CIE increased the proportion of Fos+, double Cnr1/Fos+ and Drd1/Fos+, and triple Cnr1/Drd1/Fos+ NAc cells, Stress specifically decreased Fos+ and the triple-labeled population. This result suggests that repeated predator odor may have differentially reduced the activation of the aggregate neuronal population as well as CB1-positive D1-MSNs, potentially highlighting a unique role for this neuronal subpopulation in stress-related neural adaptations. There is a relative gap in research that addresses the effects of Stress and CIE on CB1 receptor-expressing striatal D1-MSNs. This is notable given the key role of D1-MSNs in modulating rewards-related and stress-associated behaviors through dopaminergic and endocannabinoid signaling (Lobo and Nestler, 2011; Francis and Lobo, 2017; Favoretto et al., 2023). We found that CIE increased and repeated predator odor stress decreased overall Fos expression, as well as Fos expression in Cnr1/Drd1+ cells in the NAc. In contrast, neither manipulation reliably altered Fos expression or colocalization with putative D1-MSNs or CB1-expressing cells in the dorsal striatum, as defined by (co-)expression of Fos, Cnr1, and/or Drd1 mRNAs in the DMS or DLS. These results suggest a striatal subregion-specific effect of CIE and predator odor exposure on activation of aggregate neuronal population as well as endocannabinoid-modulated D1-MSNs, with a differential impact in the NAc.
It is important to note that this was an exploratory study with a limited sample size. As such, the conclusions should be interpreted with caution and not overstated. We acknowledge that the present study may be underpowered, and therefore we cannot rule out the possibility that effects may become evident in the dorsal striatum or that findings in the NAc could result in CIE × Stress interactions with a larger sample size. Nevertheless, the Bayes Factor scores (BF10) for the dorsal striatum were below 1, providing evidence in favor of the null hypothesis in this region (see Supplementary materials). Confirmatory studies with larger cohorts are necessary to validate or refute our findings.
In contrast to positive findings with Fos-colocalization in this cellular subpopulation, predator odor and CIE did not reliably change the percentage of cells observed to express Cnr1 mRNA or co-express Drd1/Cnr1 in any striatal subregion. Previous stressor paradigms have reportedly yielded both decreases (Hill et al., 2008; Marco et al., 2014; Vangopoulou et al., 2018) and increases (Papilloud et al., 2018; Amancio-Belmont et al., 2019) in Cnr1 expression or CB1 expression/binding within the dorsal and ventral striatum. Few studies have examined predator odor stress effects. Tyler et al. (2020) found with qPCR of NAc punches that a single exposure to TMT (2,4,5-trimethylthiazoline) predator odor reduced Cnr1 gene expression 4 weeks later in rats. This finding contrasts with our negative results for Cnr1 cellular localization, perhaps reflecting study differences in the chronicity and nature of the predator odor, species, power, timepoint, Cnr1 readout, or other experimental conditions.
Prior literature also shows inconsistent effects of ethanol manipulations on striatal CB1 or Cnr1 expression. Increases (Oliva and Manzanares, 2007), decreases (Ortiz et al., 2004; Martín-Llorente et al., 2023), and no changes have been reported (Rubio et al., 2009; Thanos et al., 2011; Favoretto et al., 2025). Here, we found no differences between CIE and Air control mice. Importantly, all mice in our study had a history of 2-bottle choice alcohol drinking, and we cannot rule out that effects of CIE or predator stress on Cnr1 or Drd1 expression might have been observed in comparison to alcohol-naïve controls.
Despite increasing ethanol intake, predator odor stress reduced ethanol preference ratios in mice, reflecting an even larger, concurrent, stress-induced increase in water intake. This result resembles findings of Cozzoli et al. (2014), who found that rat bedding stress increased water intake and reduced ethanol preference on the stress exposure day in mice. Thus, the increased drinking was not behaviorally-specific for ethanol, but the mice nonetheless drank more ethanol. Ethanol and water intakes did not correlate with one another, but predator stress and CIE altered the strengths of the correlations of ethanol and water intake to ethanol preference. Specifically, ethanol intake was directly correlated with ethanol preference in all groups except the Stress-CIE group. Conversely, water intake was inversely correlated with ethanol preference more strongly in both CIE groups than in their respective Air controls. The results indicate that predator stress and CIE differently and interactively influence voluntary 2-bottle choice drinking behavior patterns. This may reflect their influences on stress responses, behavioral activation, polydipsia, fluid balance, or other mechanisms (Goto et al., 2014; Gross and Pinhasov, 2016; Zhang et al., 2016).
As expected, corticosterone levels after the 10th stress session were higher than those after the 10th control cage exposure. Cozzoli et al. (2014) previously showed that acute predator odor stress increased corticosterone levels to a comparable degree as restraint stress and tail suspension. Contrary to our initial hypothesis, prior CIE did not potentiate corticosterone levels after the final stress session. Similarly, Finn et al. (2018) found that prior binge drinking did not alter the corticosterone response to predator odor stress. However, a previous study reported that prior CIE exposure enhanced corticosterone responses to a different stressor, forced swim stress, during acute (8 h) but not post-acute (72 h) alcohol withdrawal (Maldonado-Devincci et al., 2016).
Similar to Cozzoli et al. (2014), we found no cross-sectional relationship of corticosterone to ethanol intake or preference on the final day of predator odor stress. Interestingly, Cozzoli et al. (2014) reported that corticosterone levels did instead predict ethanol intake the day after the stressor. Given implicated potential for anticorticosteroid treatments to reduce drinking (Vendruscolo et al., 2012; Somkuwar et al., 2017) further research on temporal dynamics of glucocorticoid responses with ethanol consumption could provide valuable insights.
Several caveats should be considered in interpretation. First, mice were sacrificed 24 h after their last alcohol access, so acute withdrawal may have potentiated or masked effects of predator stress on gene co-localization or corticosterone levels. Indeed, C57BL/6J mice previously showed increased Fos expression in the NAc, but not dorsal striatum during acute alcohol withdrawal (Kozell et al., 2005; Smith et al., 2020). Alcohol withdrawal also reportedly reduced Cnr1 expression in the dorsal striatum of rats (Marszalek-Grabska et al., 2021) and the NAc of mice (Gasparyan et al., 2023). Future studies that also include alcohol-naïve controls can help determine if access to voluntary 2-bottle choice alcohol drinking modulated effects of predator stress. Our primary rationale for the chosen timepoint of sacrifice was to collect brain and blood samples immediately after the final stress/control cage exposure, which occurred 30 min after the onset of stress. We were aware that both Stress and CIE effects on corticosterone levels and RNAscope outcomes could be influenced by the amount of ethanol consumed, which varied across animals and groups. To minimize this potential confound, we opted to collect samples immediately after the standardized stress/control cage exposure. This ensured that all animals did not acutely have ethanol access at the time of sacrifice, since ongoing ethanol intake (which, as we confirmed, differed across groups) could have introduced bias into our corticosterone and RNAscope data.
Second, we subjected mice to 10 predator odor sessions, so gene expression changes or corticosterone responses may have habituated or sensitized by the final session. Indeed, unlike here, acute exposure to cat odor did increase the number of Fos-expressing cells in the rat caudate-putamen (Staples et al., 2008). On the other hand, Finn et al. (2018) reported no significant changes in corticosterone responses between a 1st and 4th rat bedding stress exposure. Third, other striatal cell types, including Drd2/Adora2a+ MSNs, other Drd1+ MSN subpopulations, interneurons, and glial populations were not studied. Fourth, only RNAs and not proteins were measured. Fifth, the control group used here was an active novel cage change control group, that may itself be stressful; so, other Stress effects might have been seen in comparison to unhandled home cage controls. Sixth, low power due to small size might have yielded false negative findings. Arguing against this, Bayes Factor scores (Keysers et al., 2020) were low for the negative dorsal striatum findings (BFs lower than 0.9, indicating support for the null hypothesis).
In summary, predator odor stress accelerated CIE-induced escalation of ethanol intake but did not potentiate corticosterone response. Repeated predator stress differentially reduced the percentage of NAc, but not dorsal striatum, Fos+ and triple-labeled Cnr1/Drd1/Fos+ cells. In contrast, CIE vapor exposure more generally increased proportions of Fos+, dual Cnr1/Fos+ and Drd1/Fos+, and triple Cnr1/Drd1/Fos+ cells in the NAc.
Collectively, these findings indicate that stress and alcohol exposure differentially altered activation of accumbal neuronal circuitry, including the CB1-regulated D1-MSNs population, which could influence rewards-related and appetitive behaviors involved in the development of problematic alcohol use. However, further investigation is needed to confirm these findings and to determine the causal role of these neuroadaptations in driving behavioral changes.
Data availability statement
The raw data supporting the conclusions of this article will be made available by the authors upon request, without undue reservation.
Ethics statement
The animal study was approved by Institutional Animal Care and Use Committee (IACUC). The study was conducted in accordance with the local legislation and institutional requirements.
Author contributions
CF: Conceptualization, Data curation, Formal Analysis, Funding acquisition, Investigation, Methodology, Project administration, Writing – original draft, Writing – review and editing. AN: Investigation, Writing – review and editing. GC: Investigation, Writing – review and editing. AR: Investigation, Writing – review and editing. TN: Investigation, Writing – review and editing. SR: Investigation, Writing – review and editing. LB: Investigation, Writing – review and editing. FC: Conceptualization, Funding acquisition, Methodology, Writing – review and editing. EZ: Conceptualization, Funding acquisition, Methodology, Project administration, Supervision, Writing – original draft, Writing – review and editing.
Funding
The author(s) declare that financial support was received for the research and/or publication of this article. This work was supported by the National Institutes of Health (NIH Grants R01 AA028879, P60 AA006420, T32 AA007456, R21 AA029498), the São Paulo Research Foundation (FAPESP, Grant No. 2022/10168-5), and the Pearson Center for Alcoholism and Addiction Research.
Conflict of interest
The authors declare that the research was conducted in the absence of any commercial or financial relationships that could be construed as a potential conflict of interest.
The author(s) declared that they were an editorial board member of Frontiers, at the time of submission. This had no impact on the peer review process and the final decision.
Generative AI statement
The author(s) verify and take full responsibility for the use of generative AI in the preparation of this manuscript. Generative AI was used during the preparation of this manuscript, generative AI tools (Grammarly, ChatGPT) were used to enhance readability and language. The author(s) thoroughly reviewed and edited the content as needed and take full responsibility for the final published article.
Publisher’s note
All claims expressed in this article are solely those of the authors and do not necessarily represent those of their affiliated organizations, or those of the publisher, the editors and the reviewers. Any product that may be evaluated in this article, or claim that may be made by its manufacturer, is not guaranteed or endorsed by the publisher.
Supplementary material
The Supplementary Material for this article can be found online at: https://www.frontiersin.org/articles/10.3389/fnins.2025.1568952/full#supplementary-material
References
Amancio-Belmont, O., Meléndez, A. L. B., Ruiz-Contreras, A. E., Méndez-Díaz, M., and Prospéro-García, O. (2019). Opposed cannabinoid 1 receptor (CB1R) expression in the prefrontal cortex vs. nucleus accumbens is associated with alcohol consumption in male rats. Brain Res. 1725:146485. doi: 10.1016/j.brainres.2019.146485
Bankhead, P., Loughrey, M. B., Fernández, J. A., Dombrowski, Y., McArt, D. G., Dunne, P. D., et al. (2017). QuPath: Open source software for digital pathology image analysis. Sci. Rep. 7:16878. doi: 10.1038/s41598-017-17204-5
Becker, H. C., and Lopez, M. F. (2004). Increased ethanol drinking after repeated chronic ethanol exposure and withdrawal experience in C57BL/6 mice. Alcohol Clin. Exp. Res. 28, 1829–1838. doi: 10.1097/01.alc.0000149977.95306.3a
Blasio, A., Iemolo, A., Sabino, V., Petrosino, S., Steardo, L., Rice, K. C., et al. (2013). Rimonabant precipitates anxiety in rats withdrawn from palatable food: Role of the central amygdala. Neuropsychopharmacology 38, 2498–2507. doi: 10.1038/npp.2013.153
Bradford, D. E., Dev, A. S., DeFalco, A., Whittaker, F., and Timpano, K. R. (2024). Increasing ecological validity in neuropsychopharmacology research on the relationship between alcohol use and uncertain stressors. Neuropsychopharmacology 49, 353–354. doi: 10.1038/s41386-023-01672-7
Cheng, Y., Huang, C. C. Y., Ma, T., Wei, X., Wang, X., Lu, J., et al. (2017). Distinct synaptic strengthening of the striatal direct and indirect pathways drives alcohol consumption. Biol. Psychiatry 81, 918–929. doi: 10.1016/j.biopsych.2016.05.016
Chu, K., Koob, G. F., Cole, M., Zorrilla, E. P., and Roberts, A. J. (2007). Dependence-induced increases in ethanol self-administration in mice are blocked by the CRF1 receptor antagonist antalarmin and by CRF1 receptor knockout. Pharmacol. Biochem. Behav. 86, 813–821. doi: 10.1016/j.pbb.2007.03.009
Cozzoli, D. K., Tanchuck-Nipper, M. A., Kaufman, M. N., Horowitz, C. B., and Finn, D. A. (2014). Environmental stressors influence limited-access ethanol consumption by C57BL/6J mice in a sex-dependent manner. Alcohol 48, 741–754. doi: 10.1016/j.alcohol.2014.07.015
DePoy, L., Daut, R., Brigman, J. L., MacPherson, K., Crowley, N., Gunduz-Cinar, O., et al. (2013). Chronic alcohol produces neuroadaptations to prime dorsal striatal learning. Proc. Natl. Acad. Sci. U. S. A. 110, 14783–14788. doi: 10.1073/pnas.1308198110
Favoretto, C. A., Bertagna, N. B., Anjos-Santos, A., Loss, C. M., Rodolpho, B. T., Righi, T., et al. (2025). Impacts of maternal separation stress on ethanol intake and endocannabinoid system in adolescent mice. Neuroscience 565, 124–137. doi: 10.1016/j.neuroscience.2024.11.037
Favoretto, C. A., Pagliusi, M., and Morais-Silva, G. (2023). Involvement of brain cell phenotypes in stress-vulnerability and resilience. Front. Neurosci. 17:1175514. doi: 10.3389/fnins.2023.1175514
Favoretto, C. A., Righi, T., Fernandes, G. J. D., Bertagna, N. B., Rodolpho, B. T., Janisset, N. D. R. L. L., et al. (2024). Animal models for studying therapeutic targets and treatments for alcohol use disorder. Int. Rev. Neurobiol. 178, 355–381. doi: 10.1016/bs.irn.2024.08.004
Ferragud, A., Velazquez-Sanchez, C., Minnig, M. A., Sabino, V., and Cottone, P. (2021). Pituitary adenylate cyclase-activating polypeptide (PACAP) modulates dependence-induced alcohol drinking and anxiety-like behavior in male rats. Neuropsychopharmacology 46, 509–518. doi: 10.1038/s41386-020-00904-4
Finn, D. A., Clark, C. D., and Ryabinin, A. E. (2024). Traumatic stress-enhanced alcohol drinking: Sex differences and animal model perspectives. Curr. Addict. Rep. 11, 327–341. doi: 10.1007/s40429-023-00540-0
Finn, D. A., Helms, M. L., Nipper, M. A., Cohen, A., Jensen, J. P., and Devaud, L. L. (2018). Sex differences in the synergistic effect of prior binge drinking and traumatic stress on subsequent ethanol intake and neurochemical responses in adult C57BL/6J mice. Alcohol 71, 33–45. doi: 10.1016/j.alcohol.2018.02.004
Fitzgerald, M. L., Shobin, E., and Pickel, V. M. (2012). Cannabinoid modulation of the dopaminergic circuitry: Implications for limbic and striatal output. Prog. Neuropsychopharmacol. Biol. Psychiatry 38, 21–29. doi: 10.1016/j.pnpbp.2011.12.004
Flores, A., Maldonado, R., and Berrendero, F. (2013). Cannabinoid-hypocretin cross-talk in the central nervous system: What we know so far. Front. Neurosci. 7:256. doi: 10.3389/fnins.2013.00256
Francis, T. C., and Lobo, M. K. (2017). Emerging role for nucleus accumbens medium spiny neuron subtypes in depression. Biol. Psychiatry. 81, 645–653. doi: 10.1016/j.biopsych.2016.09.007
Fullerton, C. S., Herberman Mash, H. B., Benevides, K. N., Morganstein, J. C., and Ursano, R. J. (2015). Distress of routine activities and perceived safety associated with post-traumatic stress, depression, and alcohol use: 2002 Washington, DC, sniper attacks. Disaster Med. Public Health Prep. 9, 509–515. doi: 10.1017/dmp.2015.67
Gasparyan, A., Navarrete, F., Navarro, D., and Manzanares, J. (2023). Cannabidiol regulates behavioral and brain alterations induced by spontaneous alcohol withdrawal. Neuropharmacology 233:109549. doi: 10.1016/j.neuropharm.2023.109549
Glass, M., Dragunow, M., and Faull, R. L. (1997). Cannabinoid receptors in the human brain: A detailed anatomical and quantitative autoradiographic study in the fetal, neonatal and adult human brain. Neuroscience 77, 299–318. doi: 10.1016/s0306-4522(96)00428-9
Goto, T., Kubota, Y., Tanaka, Y., Iio, W., Moriya, N., and Toyoda, A. (2014). Subchronic and mild social defeat stress accelerates food intake and body weight gain with polydipsia-like features in mice. Behav. Brain Res. 270, 339–348. doi: 10.1016/j.bbr.2014.05.040
Grieger, T. A., Fullerton, C. S., and Ursano, R. J. (2003). Posttraumatic stress disorder, alcohol use, and perceived safety after the terrorist attack on the pentagon. Psychiatr. Serv. 54, 1380–1382. doi: 10.1176/appi.ps.54.10.1380
Griffin, W. C., Lopez, M. F., and Becker, H. C. (2009a). Intensity and duration of chronic ethanol exposure is critical for subsequent escalation of voluntary ethanol drinking in mice. Alcohol Clin. Exp. Res. 33, 1893–1900. doi: 10.1111/j.1530-0277.2009.01027.x
Griffin, W. C., Lopez, M. F., Yanke, A. B., Middaugh, L. D., and Becker, H. C. (2009b). Repeated cycles of chronic intermittent ethanol exposure in mice increases voluntary ethanol drinking and ethanol concentrations in the nucleus accumbens. Psychopharmacology (Berl) 201, 569–580. doi: 10.1007/s00213-008-1324-3
Griffin, W. C., Lopez, M. F., Woodward, J. J., and Becker, H. C. (2023). Alcohol dependence and the ventral hippocampal influence on alcohol drinking in male mice. Alcohol 106, 44–54. doi: 10.1016/j.alcohol.2022.10.004
Gross, M., and Pinhasov, A. (2016). Chronic mild stress in submissive mice: Marked polydipsia and social avoidance without hedonic deficit in the sucrose preference test. Behav. Brain Res. 298(Pt B), 25–34. doi: 10.1016/j.bbr.2015.10.049
Heilig, M., Egli, M., Crabbe, J. C., and Becker, H. C. (2010). Acute withdrawal, protracted abstinence and negative affect in alcoholism: Are they linked? Addict. Biol. 15, 169–184. doi: 10.1111/j.1369-1600.2009.00194.x
Hill, M. N., Carrier, E. J., McLaughlin, R. J., Morrish, A. C., Meier, S. E., Hillard, C. J., et al. (2008). Regional alterations in the endocannabinoid system in an animal model of depression: Effects of concurrent antidepressant treatment. J. Neurochem. 106, 2322–2336. doi: 10.1111/j.1471-4159.2008.05567.x
Hillard, C. J. (2014). Stress regulates endocannabinoid-CB1 receptor signaling. Semin. Immunol. 26, 380–388. doi: 10.1016/j.smim.2014.04.001
Huitron-Resendiz, S., Nadav, T., Krause, S., Cates-Gatto, C., Polis, I., and Roberts, A. J. (2018). Effects of withdrawal from chronic intermittent ethanol exposure on sleep characteristics of female and male mice. Alcohol Clin. Exp. Res. 42, 540–550. doi: 10.1111/acer.13584
Jeanes, Z. M., Buske, T. R., and Morrisett, R. A. (2014). Cell type-specific synaptic encoding of ethanol exposure in the nucleus accumbens shell. Neuroscience 277, 184–195. doi: 10.1016/j.neuroscience.2014.06.063
Keysers, C., Gazzola, V., and Wagenmakers, E. J. (2020). Using bayes factor hypothesis testing in neuroscience to establish evidence of absence. Nat. Neurosci. 23, 788–799. doi: 10.1038/s41593-020-0660-4
Kimbrough, A., de Guglielmo, G., Kononoff, J., Kallupi, M., Zorrilla, E. P., and George, O. (2017). CRF1 receptor-dependent increases in irritability-like behavior during abstinence from chronic intermittent ethanol vapor exposure. Alcohol Clin. Exp. Res. 41, 1886–1895. doi: 10.1111/acer.13484
Kozell, L. B., Hitzemann, R., and Buck, K. J. (2005). Acute alcohol withdrawal is associated with c-Fos expression in the basal ganglia and associated circuitry: C57bl/6j and DBA/2J inbred mouse strain analyses. Alcohol Clin. Exp. Res. 29, 1939–1948. doi: 10.1097/01.alc.0000187592.57853.12
Lobo, M. K., and Nestler, E. J. (2011). The striatal balancing act in drug addiction: Distinct roles of direct and indirect pathway medium spiny neurons. Front. Neuroanat. 5:41. doi: 10.3389/fnana.2011.00041
Lobo, M. K., Zaman, S., Damez-Werno, D. M., Koo, J. W., Bagot, R. C., DiNieri, J. A., et al. (2013). ΔFosB induction in striatal medium spiny neuron subtypes in response to chronic pharmacological, emotional, and optogenetic stimuli. J. Neurosci. 33, 18381–18395. doi: 10.1523/JNEUROSCI.1875-13.2013
Logrip, M. L., and Zorrilla, E. P. (2012). Stress history increases alcohol intake in relapse: Relation to phosphodiesterase 10A. Addict. Biol. 17, 920–933. doi: 10.1111/j.1369-1600.2012.00460.x
Logrip, M. L., Zorrilla, E. P., and Koob, G. F. (2012). Stress modulation of drug self-administration: Implications for addiction comorbidity with post-traumatic stress disorder. Neuropharmacology 62, 552–564. doi: 10.1016/j.neuropharm.2011.07.007
Lopez, M. F., Anderson, R. I., and Becker, H. C. (2016). Effect of different stressors on voluntary ethanol intake in ethanol-dependent and nondependent C57BL/6J mice. Alcohol 51, 17–23. doi: 10.1016/j.alcohol.2015.11.010
Lopez, M. F., and Becker, H. C. (2005). Effect of pattern and number of chronic ethanol exposures on subsequent voluntary ethanol intake in C57BL/6J mice. Psychopharmacology (Berl) 181, 688–696. doi: 10.1007/s00213-005-0026-3
Macedo, G. C., Kreifeldt, M., Goulding, S. P., Okhuarobo, A., Sidhu, H., and Contet, C. (2023). Chronic MAP4343 reverses escalated alcohol drinking in a mouse model of alcohol use disorder. Neuropsychopharmacology 48, 821–830. doi: 10.1038/s41386-023-01529-z
Maldonado-Devincci, A. M., Kampov-Polevoi, A., McKinley, R. E., Morrow, D. H., O’Buckley, T. K., and Morrow, A. L. (2016). Chronic intermittent ethanol exposure alters stress effects on (3α,5α)-3-hydroxy-pregnan-20-one (3α,5α-THP) immunolabeling of amygdala neurons in C57BL/6J mice. Front. Cell Neurosci. 10:40. doi: 10.3389/fncel.2016.00040
Marco, E. M., Echeverry-Alzate, V., López-Moreno, J. A., Giné, E., Peñasco, S., and Viveros, M. P. (2014). Consequences of early life stress on the expression of endocannabinoid-related genes in the rat brain. Behav. Pharmacol. 25, 547–556. doi: 10.1097/FBP.0000000000000068
Marszalek-Grabska, M., Smaga, I., Surowka, P., Grochecki, P., Slowik, T., Filip, M., et al. (2021). Memantine prevents the win 55,212-2 evoked cross-priming of ethanol-induced Conditioned Place Preference (CPP). Int. J. Mol. Sci. 22:7940. doi: 10.3390/ijms22157940
Martín-Llorente, A., Serrano, M., Bonilla-Del Río, I., Lekunberri, L., Ocerin, G., Puente, N., et al. (2023). Omega-3 recovers cannabinoid 1 receptor expression in the adult mouse brain after adolescent binge drinking. Int. J. Mol. Sci. 24:17316. doi: 10.3390/ijms242417316
Maslow, A. H. (1943). A theory of human motivation. Psychol. Rev. 50, 370–396. doi: 10.1037/h0054346
Navarrete, F., García-Gutiérrez, M. S., Jurado-Barba, R., Rubio, G., Gasparyan, A., Austrich-Olivares, A., et al. (2020). Endocannabinoid system components as potential biomarkers in psychiatry. Front. Psychiatry 11:315. doi: 10.3389/fpsyt.2020.00315
O’Dell, L. E., Roberts, A. J., Smith, R. T., and Koob, G. F. (2004). Enhanced alcohol self-administration after intermittent versus continuous alcohol vapor exposure. Alcohol Clin. Exp. Res. 28, 1676–1682. doi: 10.1097/01.alc.0000145781.11923.4e
Okhuarobo, A., Bolton, J. L., Igbe, I., Zorrilla, E. P., Baram, T. Z., and Contet, C. A. (2020). novel mouse model for vulnerability to alcohol dependence induced by early-life adversity. Neurobiol. Stress 13:100269. doi: 10.1016/j.ynstr.2020.100269
Oliva, J. M., and Manzanares, J. (2007). Gene transcription alterations associated with decrease of ethanol intake induced by naltrexone in the brain of Wistar rats. Neuropsychopharmacology 32, 1358–1369. doi: 10.1038/sj.npp.1301237
Ornelas, L. C., and Besheer, J. (2024). Predator odor stress reactivity, alcohol drinking and the endocannabinoid system. Neurobiol. Stress 30:100634. doi: 10.1016/j.ynstr.2024.100634
Ortiz, S., Oliva, J. M., Pérez-Rial, S., Palomo, T., and Manzanares, J. (2004). Chronic ethanol consumption regulates cannabinoid CB1 receptor gene expression in selected regions of rat brain. Alcohol. Alcohol. 39, 88–92. doi: 10.1093/alcalc/agh036
Papilloud, A., Guillot de Suduiraut, I., Zanoletti, O., Grosse, J., and Sandi, C. (2018). Peripubertal stress increases play fighting at adolescence and modulates nucleus accumbens CB1 receptor expression and mitochondrial function in the amygdala. Transl. Psychiatry 8:156. doi: 10.1038/s41398-018-0215-6
Parylak, S. L., Cottone, P., Sabino, V., Rice, K. C., and Zorrilla, E. P. (2012). Effects of CB1 and CRF1 receptor antagonists on binge-like eating in rats with limited access to a sweet fat diet: Lack of withdrawal-like responses. Physiol. Behav. 107, 231–242. doi: 10.1016/j.physbeh.2012.06.017
Piggott, V. M., Lloyd, S. C., Perrine, S. A., and Conti, A. C. (2020). Chronic intermittent ethanol exposure increases ethanol consumption following traumatic stress exposure in mice. Front. Behav. Neurosci. 14:114. doi: 10.3389/fnbeh.2020.00114
Reep, R. L., Cheatwood, J. L., and Corwin, J. V. (2003). The associative striatum: Organization of cortical projections to the dorsocentral striatum in rats. J. Comp. Neurol. 467, 271–292. doi: 10.1002/cne.10868
Renteria, R., Baltz, E. T., and Gremel, C. M. (2018). Chronic alcohol exposure disrupts top-down control over basal ganglia action selection to produce habits. Nat. Commun. 9:211. doi: 10.1038/s41467-017-02615-9
Renteria, R., Maier, E. Y., Buske, T. R., and Morrisett, R. A. (2017). Selective alterations of NMDAR function and plasticity in D1 and D2 medium spiny neurons in the nucleus accumbens shell following chronic intermittent ethanol exposure. Neuropharmacology 112(Pt A), 164–171. doi: 10.1016/j.neuropharm.2016.03.004
Rodberg, E. M., den Hartog, C. R., Anderson, R. I., Becker, H. C., Moorman, D. E., and Vazey, E. M. (2017). Stress facilitates the development of cognitive dysfunction after chronic ethanol exposure. Alcohol Clin. Exp. Res. 41, 1574–1583. doi: 10.1111/acer.13444
Romano-López, A., Méndez-Díaz, M., Ruiz-Contreras, A. E., Carrisoza, R., and Prospéro-García, O. (2012). Maternal separation and proclivity for ethanol intake: A potential role of the endocannabinoid system in rats. Neuroscience 223, 296–304. doi: 10.1016/j.neuroscience.2012.07.071
Rubio, M., de Miguel, R., Fernández-Ruiz, J., Gutiérrez-López, D., Carai, M. A., and Ramos, J. A. (2009). Effects of a short-term exposure to alcohol in rats on FAAH enzyme and CB1 receptor in different brain areas. Drug Alcohol Depend. 99, 354–358. doi: 10.1016/j.drugalcdep.2008.08.004
Schmettow, M. (2021). “Generalized linear models,” in New statistics for design researchers. Human-computer interaction series. Cham: Springer. doi: 10.1007/978-3-030-46380-9_7
Serrano, A., Pavon, F. J., Buczynski, M. W., Schlosburg, J., Natividad, L. A., Polis, I. Y., et al. (2018). Deficient endocannabinoid signaling in the central amygdala contributes to alcohol dependence-related anxiety-like behavior and excessive alcohol intake. Neuropsychopharmacology 43, 1840–1850. doi: 10.1038/s41386-018-0055-3
Sinha, R. (2001). How does stress increase risk of drug abuse and relapse? Psychopharmacology (Berl) 158, 343–359. doi: 10.1007/s002130100917
Sinha, R. (2008). Chronic stress, drug use, and vulnerability to addiction. Ann. N. Y. Acad. Sci. 1141, 105–130. doi: 10.1196/annals.1441.030
Smith, R. J., Anderson, R. I., Haun, H. L., Mulholland, P. J., Griffin, W. C., Lopez, M. F., et al. (2020). Dynamic c-Fos changes in mouse brain during acute and protracted withdrawal from chronic intermittent ethanol exposure and relapse drinking. Addict. Biol. 25:e12804. doi: 10.1111/adb.12804
Somkuwar, S. S., Vendruscolo, L. F., Fannon, M. J., Schmeichel, B. E., Nguyen, T. B., Guevara, J., et al. (2017). Abstinence from prolonged ethanol exposure affects plasma corticosterone, glucocorticoid receptor signaling and stress-related behaviors. Psychoneuroendocrinology 84, 17–31. doi: 10.1016/j.psyneuen.2017.06.006
Staples, L. G., McGregor, I. S., Apfelbach, R., and Hunt, G. E. (2008). Cat odor, but not trimethylthiazoline (fox odor), activates accessory olfactory and defense-related brain regions in rats. Neuroscience 151, 937–947. doi: 10.1016/j.neuroscience.2007.11.039
Steinman, M. Q., Kirson, D., Wolfe, S. A., Khom, S., D’Ambrosio, S. R., Spierling Bagsic, S. R., et al. (2021). Importance of sex and trauma context on circulating cytokines and amygdalar GABAergic signaling in a comorbid model of posttraumatic stress and alcohol use disorders. Mol. Psychiatry 26, 3093–3107. doi: 10.1038/s41380-020-00920-2
Tewari, A., Jog, R., and Jog, M. S. (2016). The striatum and subthalamic nucleus as independent and collaborative structures in motor control. Front. Syst. Neurosci. 10:17. doi: 10.3389/fnsys.2016.00017
Thanos, P. K., Gopez, V., Delis, F., Michaelides, M., Grandy, D. K., Wang, G. J., et al. (2011). Upregulation of cannabinoid type 1 receptors in dopamine D2 receptor knockout mice is reversed by chronic forced ethanol consumption. Alcohol Clin. Exp. Res. 35, 19–27. doi: 10.1111/j.1530-0277.2010.01318.x
Tyler, R. E., Weinberg, B. Z. S., Lovelock, D. F., Ornelas, L. C., and Besheer, J. (2020). Exposure to the predator odor TMT induces early and late differential gene expression related to stress and excitatory synaptic function throughout the brain in male rats. Genes Brain Behav. 19:e12684. doi: 10.1111/gbb.12684
Valdez, G. R., Roberts, A. J., Chan, K., Davis, H., Brennan, M., Zorrilla, E. P., et al. (2002). Increased ethanol self-administration and anxiety-like behavior during acute ethanol withdrawal and protracted abstinence: Regulation by corticotropin-releasing factor. Alcohol Clin. Exp. Res. 26, 1494–1501. doi: 10.1097/01.ALC.0000033120.51856.F0
van Erp, A. M., and Miczek, K. A. (2001). Persistent suppression of ethanol self-administration by brief social stress in rats and increased startle response as index of withdrawal. Physiol. Behav. 73, 301–311. doi: 10.1016/s0031-9384(01)00458-9
Vangopoulou, C., Bourmpoula, M. T., Koupourtidou, C., Giompres, P., Stamatakis, A., Kouvelas, E. D., et al. (2018). Effects of an early life experience on rat brain cannabinoid receptors in adolescence and adulthood. IBRO Rep. 5, 1–9. doi: 10.1016/j.ibror.2018.05.002
Vaughan, C. W., and Christie, M. J. (2005). “Retrograde signalling by endocannabinoids,” in Cannabinoids. Handbook of experimental pharmacology, vol 168, ed. R. G. Pertwee (Berlin: Springer). doi: 10.1007/3-540-26573-2_12
Vendruscolo, L. F., Barbier, E., Schlosburg, J. E., Misra, K. K., Whitfield, T. W., Logrip, M. L., et al. (2012). Corticosteroid-dependent plasticity mediates compulsive alcohol drinking in rats. J. Neurosci. 32, 7563–7571. doi: 10.1523/JNEUROSCI.0069-12.2012
Vendruscolo, L. F., and Roberts, A. J. (2014). Operant alcohol self-administration in dependent rats: Focus on the vapor model. Alcohol 48, 277–286. doi: 10.1016/j.alcohol.2013.08.006
Volpicelli, J. R., Tiven, J., and Kimmel, S. C. (1982). The relationship between tension reduction and ethanol consumption in rats. Physiol. Psychol. 10, 114–116. doi: 10.3758/BF03327014
Vozella, V., Cruz, B., Feldman, H. C., Bullard, R., Bianchi, P. C., Natividad, L. A., et al. (2023). Sexually dimorphic effects of monoacylglycerol lipase inhibitor MJN110 on stress-related behaviour and drinking in marchigian sardinian alcohol-preferring rats. Br. J. Pharmacol. 180, 3130–3145. doi: 10.1111/bph.16197
Wang, J., Cheng, Y., Wang, X., Roltsch Hellard, E., Ma, T., Gil, H., et al. (2015). Alcohol elicits functional and structural plasticity selectively in dopamine d1 receptor-expressing neurons of the dorsomedial striatum. J. Neurosci. 35, 11634–11643. doi: 10.1523/JNEUROSCI.0003-15.2015
Wang, L., Liu, J., Harvey-White, J., Zimmer, A., and Kunos, G. (2003). Endocannabinoid signaling via cannabinoid receptor 1 is involved in ethanol preference and its age-dependent decline in mice. Proc. Natl. Acad. Sci. U. S. A. 100, 1393–1398. doi: 10.1073/pnas.0336351100
World Health Organization [WHO] (2018). World health statistics 2018: Monitoring health for the SDG’s (sustainable development goals). [s.l.: s.n.]. Geneva: WHO.
Xiao, T., Chen, Y., Boisvert, A., Cole, M., and Kimbrough, A. (2023). Chronic intermittent ethanol vapor exposure paired with two-bottle choice to model alcohol use disorder. J. Vis. Exp. 10.3791/65320. doi: 10.3791/65320
Zhang, L., Hernández, V. S., Vázquez-Juárez, E., Chay, F. K., and Barrio, R. A. (2016). Thirst is associated with suppression of habenula output and active stress coping: Is there a role for a non-canonical vasopressin-glutamate pathway? Front. Neural Circuits 10:13. doi: 10.3389/fncir.2016.00013
Zhao, Y., Weiss, F., and Zorrilla, E. P. (2007). Remission and resurgence of anxiety-like behavior across protracted withdrawal stages in ethanol-dependent rats. Alcohol Clin. Exp. Res. 31, 1505–1515. doi: 10.1111/j.1530-0277.2007.00456.x
Keywords: alcohol, stress, D1-medium spiny neurons, CB1 cannabinoid receptors, Fos, corticosterone
Citation: Favoretto CA, Nguyen A, Chacon GR, Roberts AJ, Nadav T, Ranjan S, Bertotto LB, Cruz FC and Zorrilla EP (2025) Impacts of chronic intermittent ethanol vapor and predator odor on ethanol intake and striatal D1 and CB1 cannabinoid receptor-expressing medium spiny neurons. Front. Neurosci. 19:1568952. doi: 10.3389/fnins.2025.1568952
Received: 31 January 2025; Accepted: 28 April 2025;
Published: 27 May 2025.
Edited by:
Gabriele Floris, Yale University, United StatesReviewed by:
Yvan Nicolas Peterschmitt, University of Franche-Comté, FranceRuixiang Wang, University of Iowa, United States
Robert C. Liu, Emory University, United States
Copyright © 2025 Favoretto, Nguyen, Chacon, Roberts, Nadav, Ranjan, Bertotto, Cruz and Zorrilla. This is an open-access article distributed under the terms of the Creative Commons Attribution License (CC BY). The use, distribution or reproduction in other forums is permitted, provided the original author(s) and the copyright owner(s) are credited and that the original publication in this journal is cited, in accordance with accepted academic practice. No use, distribution or reproduction is permitted which does not comply with these terms.
*Correspondence: Eric P. Zorrilla, ZXpvcnJpbGxhQHNjcmlwcHMuZWR1