- 1Department of Neurosurgery, UTHealth Houston, McGovern Medical School, Houston, TX, United States
- 2Department of Pediatric Surgery, McGovern Medical School at UTHealth, Houston, TX, United States
- 3Center for Molecular Imaging, The Brown Foundation Institute of Molecular Medicine, UTHealth, Houston, TX, United States
Traumatic Brain Injury (TBI) in children is a profound public health issue with the potential to disrupt cognitive, behavioral, and psychosocial development significantly. This review provides an updated examination of the role of neuroinflammation in pediatric TBI, emphasizing its dual impact on injury progression and recovery. Highlighted is the complex interplay of primary and secondary injury mechanisms, including the critical contributions of neuroinflammatory responses mediated by central and peripheral immune cells. Advances in biomarker identification and imaging techniques are discussed, showcasing how tools like diffusion tensor imaging (DTI) and positron emission tomography (PET) enhance our understanding of neuroinflammatory processes. The review also explores current therapeutic strategies targeting neuroinflammation, underscoring emerging treatments such as pharmacologic agents that modulate immune responses and novel therapies like stem cell interventions. This comprehensive review seeks to deepen the understanding of neuroinflammation’s pathophysiological roles in pediatric TBI and propose directions for future clinical and research efforts.
Introduction
Traumatic brain injury (TBI) remains a critical public health challenge worldwide, particularly as one of the leading causes of disability and mortality among children and adolescents (Jacquens et al., 2024; Lajud et al., 2021; Fraunberger and Esser, 2019). In 2022, the US Centers for Disease Control and Prevention (CDC) reported that 2.3 million children <18 years old had ever received a diagnosis of concussion or brain injury (Elgaddal and Black, 2023). Additionally, a 2017 analysis using data from the CDC’s Mortality and Morbidity Weekly Report (MMWR) reported that the incidence of TBI-related emergency department (ED) visits, hospitalizations, and mortality within the pediatric population was roughly 1% (Taylor et al., 2017). The long-term consequences of TBI in this demographic can severely disrupt cognitive, behavioral, and psychosocial development. While the impacts of pediatric TBI on areas such as executive function and cognitive flexibility are well-documented, the broader literature also extensively discusses the myriad causes of TBI in children, from falls and motor vehicle collisions to sports injuries and child abuse. Each cause presents distinct challenges in clinical management and prognosis (Jacquens et al., 2024; Lajud et al., 2021; Fraunberger and Esser, 2019; Gao et al., 2020; Hon et al., 2019; Emery et al., 2016; Peterson et al., 2019). Additionally, the classification of TBI severity in children has often been extrapolated from the adult literature and is based on the Glasgow Coma Scale (GCS) which is scored from 3 to 15: mild TBI (13–15), moderate TBI (9–12), severe TBI (3–8) (Haydel et al., 2025; Jain and Iverson, 2025).
Substantial research has been conducted on the immediate and secondary injury phases in pediatric TBI, which are characterized by mechanical damage and a complex biochemical cascade, respectively (Jacquens et al., 2024; Lajud et al., 2021; Fraunberger and Esser, 2019; Gao et al., 2020; Kölliker-Frers et al., 2021; Reis et al., 2017; Regner et al., 2018; Sulhan et al., 2020; Xiong et al., 2018; Burda et al., 2016; Pearn et al., 2017; Wolf et al., 2001; Zetterberg et al., 2013; De Souza et al., 2009; Roth et al., 2014; Liddelow et al., 2017; Tang-Schomer et al., 2010; Winkler et al., 2016; Chodobski et al., 2011; Abdelhak et al., 2022; Yue et al., 2019; Pelinka et al., 2004; Sperry et al., 2024; Okonkwo et al., 2020; Thelin et al., 2017; Oris et al., 2023; Park and Hwang, 2018; Cheng et al., 2014; Bandyopadhyay et al., 2005; Wilkinson et al., 2017; Czeiter et al., 2020; Agoston et al., 2017; Rosenberg et al., 1997; Zou et al., 2021; Park, 2018; Xie et al., 2024; Gonzalez Rodriguez et al., 2018; Iczkiewicz et al., 2007). It is evident that neuroinflammation plays a critical dual role. Initially beneficial for debris clearance and neuronal repair, prolonged or dysregulated inflammation can impair recovery and exacerbate long-term outcomes (Jacquens et al., 2024; Hamood et al., 2022). Recent studies have proposed that such sustained inflammation could lead to glymphatic dysfunction, which impedes the brain’s waste-clearance mechanisms, thereby accumulating toxic metabolites and potentially accelerating neurodegeneration (Peters and Lyketsos, 2023). Amid ongoing brain development and heightened neuroplasticity in children, understanding the nuanced responses to brain injury is crucial for improving long-term outcomes. This review acknowledges the robust discussion in existing literature surrounding key inflammatory mediators like cytokines and chemokines (e.g., TNF-α, IL-1β, IL-6, HMGB1), which are central both in the pathology and the resolution of TBI (Abdelhak et al., 2022; Yue et al., 2019; Pelinka et al., 2004; Sperry et al., 2024; Okonkwo et al., 2020; Thelin et al., 2017; Oris et al., 2023; Park and Hwang, 2018; Cheng et al., 2014; Bandyopadhyay et al., 2005; Wilkinson et al., 2017; Czeiter et al., 2020; Agoston et al., 2017; Zou et al., 2021; Park, 2018; Xie et al., 2024; Gonzalez Rodriguez et al., 2018; Iczkiewicz et al., 2007; Rogan et al., 2023; Undén and Romner, 2010; Ergün et al., 1998; Chen et al., 2022). Furthermore, recent emphasis on identifying reliable biomarkers such as plasma osteopontin and glial fibrillary acidic protein highlights advances in diagnosing and predicting the progression of pediatric TBI (Jacquens et al., 2024; Gao et al., 2020; Abdelhak et al., 2022; Yue et al., 2019; Pelinka et al., 2004; Sperry et al., 2024; Iczkiewicz et al., 2007).
This manuscript integrates recent findings to deepen our understanding of neuroinflammation’s pathophysiological roles in pediatric TBI. It strives to expand on the current literature by offering new insights into the inflammatory mechanisms, examining both systemic and localized responses and their effects on neurological health over time. By proposing future directions for clinical and research efforts, this review seeks to fill gaps in our understanding and suggest ways to mitigate the long-term impacts of pediatric TBI.
Pathophysiology of neuroinflammation in pediatric TBI
The inflammatory response to traumatic brain injury (TBI) involves a complex interplay between various central nervous system (CNS) and peripheral immune cells, each contributing to the overall inflammatory cascade. Astrocytes, microglia, pericytes, and mast cells play crucial roles in the CNS response, while neutrophils, monocytes, and T cells drive the peripheral response (Harting et al., 2008). Understanding these mechanisms and their interactions is essential for developing targeted therapies for TBI. While the complexity of outcomes following CNS injuries in the developed brain is extensive, injuries to the developing brain pose a unique and significant challenge. The ongoing processes of synaptogenesis and myelination in the developing brain are crucial for forming neural networks that may be disrupted by an injury that is mild in severity, which can impact behavior and molecular interactions, such as inflammation. One of the significant consequences of TBI-induced neuroinflammation is the subsequent activation of microglia, the brain’s resident immune cells, which serve a multifaceted role in both the injury response and downstream recovery (Donat et al., 2017). While microglia are essential for removing debris and modulating repair mechanisms, overactivation leads to increased synaptic pruning, disrupting normal synaptogenesis and myelination. This dysregulated microglial activity impairs the stability of neural circuits, particularly in white matter structures, contributing to long-term cognitive and motor deficits (Eyolfson et al., 2020).
Traumatic brain injury (TBI) is a leading cause of death and disability across all age groups worldwide (Hon et al., 2019). Due to the ongoing brain development in children, this group is particularly susceptible to both acute and chronic cognitive and behavioral changes following a TBI. These changes include sleep disturbances, impaired attention and memory, emotional instability, and motor and balance issues (Emery et al., 2016). TBI is defined by the Centers for Disease Control (CDC) as a disruption in brain function caused by an external force to the head, otherwise referred to as the “primary” injury (Peterson et al., 2019). The injury-inducing forces can be applied in a linear or non-linear (i.e., rotational) fashion, which may physically damage brain tissue. The pathophysiology of pediatric TBI involves a multifaceted interplay of primary and secondary injury mechanisms.
The primary injury in TBI occurs immediately following the impact that results in physical disruption of brain tissue, including neurons, microglia, and endothelial cells that makeup the blood–brain barrier (BBB) (Reis et al., 2017). The effects following the initial insult trigger a cascade of secondary injury processes characterized by ischemia, hypoxia, cerebral edema, raised intracranial pressure, excitotoxicity, calcium dysregulation, mitochondrial dysfunction, inflammation, and apoptosis (Regner et al., 2018; Sulhan et al., 2020; Xiong et al., 2018). These secondary injury mechanisms evolve over a longer time span, ranging from hours, days, and weeks, following the initial trauma, which exacerbates the primary injury, leading to further neurological damage (Hamood et al., 2022).
The secondary cascade involved in pediatric TBI involves the activation of a neuroinflammatory response involving microglia, astrocytes, and peripheral immune cells, which disrupts the integrity of the BBB. TBI-induced activation of astrocytes results in the stretching of the N-methyl-D-aspartate (NMDA) surface receptors, causing deformity and unregulated ionic changes (Burda et al., 2016). The range of severity in the primary injury determines the degree of cellular membrane damage, which results in the rapid and unregulated influx of sodium coupled with potassium efflux and increases in intra-axonal calcium (Pearn et al., 2017; Wolf et al., 2001; Zetterberg et al., 2013). Simultaneous ATP release initiates autocrine and paracrine calcium influx, causing additional ATP, glutamate, and S100B release through MAPK signaling (Jacquens et al., 2024; Pearn et al., 2017). This process leads to the activation of astrocytes and microglia recruitment to the site of injury, which secrete IL-1a, TNFa, and C1q, stimulating a phenotypic conversion of astrocytes to an A1 “neurotoxic” profile, demonstrating the reciprocal activation of these glial cells in TBI (Roth et al., 2014; Liddelow et al., 2017). In addition to the ionic mechanism changes, rapid acceleration and deceleration upon impact results in extensive shearing of axons, known as diffuse axonal injury. Structural analysis of axons following injury shows microtubule breakage, eventually leading to the progressive disassembly of microtubules (Tang-Schomer et al., 2010). The damage to these structures contributes to the accumulation of protein products, interruption of axonal transport, axonal swelling, and degeneration (Tang-Schomer et al., 2010). Figure 1 illustrates the role of microglia and the immune system in promoting neurotoxicity.
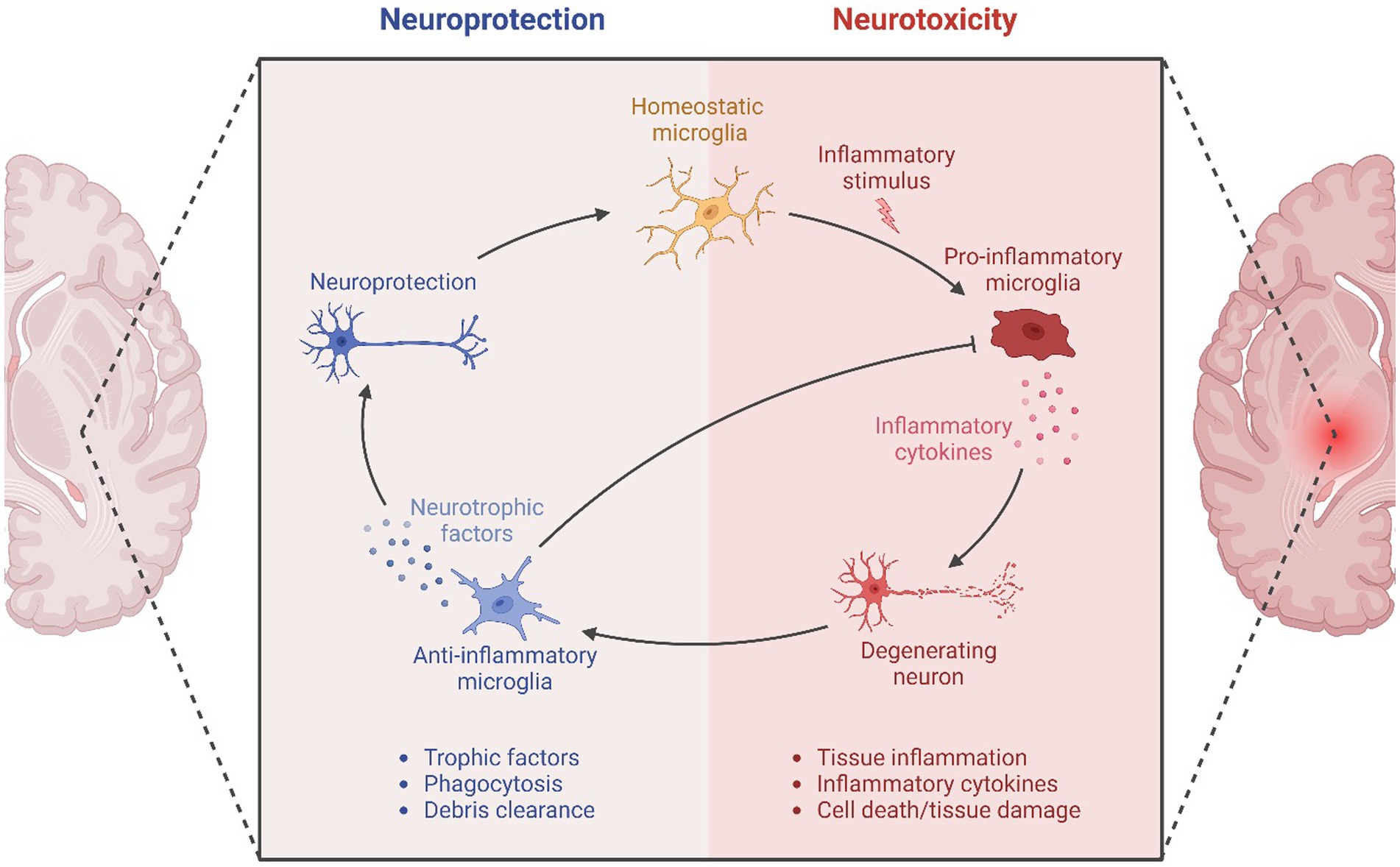
Figure 1. Shows the process of neuroinflammation and the role of the immune system in promoting neuroprotection and neurotoxicity (https://biorender.com/e81a861). Modified from https://app.biorender.com/biorender-templates/figures/all/t-63a4bc394320eed93210b91b-roles-of-microglia-in-neuroinflammation.
Peripheral immune cells play a pivotal role in the inflammatory response following TBI in the pediatric population. Previous studies have indicated the differences in peripheral immune cell composition in adolescents compared to adults for up to 18 years. These differences include, for example, lymphocytes slowly increasing in children from ages 6 months to 18 years old, while neutrophils and eosinophils decrease in ages 14–18 (Aldrimer et al., 2013). This is an important indication as the cells responding to the site of injury will differ between the pediatric and adult populations, greatly altering the immune response and subsequent inflammation. The disruption of BBB permeability following TBI leads to the recruitment of macrophages, neutrophils, and lymphocytes to the injury site, further contributing to the inflammatory response. Injured brain parenchyma increases the expression of leukocyte adhesion molecules on the brain endothelium early in TBI (Worthylake and Burridge, 2001). Through this cascade, leukocytes further disrupt the BBB by generating reactive oxygen species, activating proteolytic enzymes, and secreting cytokines and chemokines (Winkler et al., 2016; Chodobski et al., 2011). Additionally, the chemokines secreted attract more inflammatory cells, allowing for their extravasation across the vascular membrane, furthering the neuroinflammatory response and damage to brain tissue. Understanding the pathophysiology of pediatric TBI requires consideration of both the unique aspects of the developing brain and the complex interplay of inflammatory and immune responses. Further research is needed to develop effective treatments that address these intricate mechanisms.
Biomarkers and neuroinflammation
In the evolving landscape of traumatic brain injury (TBI) research, molecular biomarkers are paramount in advancing our understanding of the complex pathology that characterizes TBI. Traditional biomarkers such as Glial fibrillary acidic protein (GFAP), S100B, and neuron-specific enolase (NSE) are well-documented in their roles in parenchymal trauma. GFAP, a protein predominantly found in astrocytes, is utilized to evaluate astrocytic injury and has been correlated with injury severity and patient outcomes, often serving as a reliable indicator of the structural impact of TBI (Abdelhak et al., 2022; Yue et al., 2019; Pelinka et al., 2004; Sperry et al., 2024; Okonkwo et al., 2020). S100B serves a dual role, primarily reflecting acute neuronal damage due to its presence in mature perivascular astrocytes and its involvement in calcium signaling and regulation. It has been effectively used in clinical settings to predict neurological outcomes following TBI (Thelin et al., 2017; Oris et al., 2023; Park and Hwang, 2018; Rogan et al., 2023; Undén and Romner, 2010). NSE, highly specific to neurons, is another critical marker for assessing the extent of neuronal damage and is often utilized to gauge the severity of brain injuries and to predict long-term recovery trajectories (Cheng et al., 2014; Bandyopadhyay et al., 2005; Wilkinson et al., 2017; Ergün et al., 1998; Chen et al., 2022). In addition to these markers of primary injury, or focal biomarkers, UCHL-1, total tau, NF-L and MBP are also being studied as potential focal biomarkers (Sperry et al., 2024; Czeiter et al., 2020; Agoston et al., 2017).
While focal biomarkers offer valuable insights into the extent of structural damage, they fall short in providing direct measures of the neuroinflammatory processes that play a critical role in the secondary injury mechanisms following TBI. This gap has led to increased research into biomarkers that can specifically quantify neuroinflammation, or global biomarkers.
Among the emerging global biomarkers, Osteopontin (OPN) and Syndecan-1 (sdc1) have shown promise in specifically reflecting the inflammatory response in TBI. OPN is involved in regulating immune processes and is upregulated in response to trauma, particularly in microglial cells. Elevated levels of OPN not only correlate with the severity of the injury but also with inflammatory processes that may affect recovery and long-term outcomes (Gao et al., 2020). This biomarker offers a unique perspective on the inflammatory landscape following TBI, providing insights that are not captured by traditional biomarkers focused on structural damage. Syndecan-1, a component of the endothelial extracellular matrix, is crucial for vascular integrity and is implicated in the systemic inflammatory response following TBI. Studies have shown that elevated levels of sdc1 after TBI indicate endothelial damage and a broader inflammatory response that may worsen neurological outcomes, particularly in patients with concurrent extracranial injuries (Rosenberg et al., 1997; Zou et al., 2021; Park, 2018; Xie et al., 2024; Gonzalez Rodriguez et al., 2018). The measurement of sdc1 levels provides a window into the systemic effects of TBI and highlights the interconnectedness of inflammatory pathways that extend beyond the primary site of injury.
Furthermore, the exploration of biomarkers like High Mobility Group Box 1 (HMGB1) and Interleukin-6 (IL-6) is expanding our understanding of the molecular dynamics at play in TBI. HMGB1, a nuclear protein released during cellular stress and necrosis, acts as a pro-inflammatory mediator in the brain, exacerbating tissue damage following TBI. Its role as a biomarker is significant in delineating the mechanisms of secondary injury and inflammation (Iczkiewicz et al., 2007; Wozniak et al., 2007; Dennis et al., 2018; Bodanapally et al., 2019; Bodanapally et al., 2019). Similarly, IL-6 is rapidly upregulated following TBI and has been studied for its dual role as both a pro-inflammatory cytokine and a potential marker for therapeutic targets, especially in modulating the acute inflammatory response to improve patient outcomes.
While considering the potential for clinical utility of biomarkers in the context of TBI, it is important that their limitations be given due consideration. NSE, which is also found in erythrocytes, loses specificity in settings where hemolysis can significantly confound its interpretation. Although S100B is immune to this interference, its short half-life makes its sensitivity heavily dependent on the delay between initial injury and its measurement (Oris et al., 2023). Sdc1, given its global expression in vasculature throughout the body, loses specificity in polytrauma settings when extracranial injuries can contribute to elevations in its levels (Xie et al., 2024).
Though the utility and significance of various biomarkers in TBI is an area of active research, there have already been promising clinical applications. Of particular interest are GFAP and UCHL-1, markers of glial and neuronal injury, respectively. These biomarkers, in tandem, have demonstrated significant diagnostic and prognostic value for the management of intracranial injury (Bazarian et al., 2018; Posti et al., 2016). Furthermore, a rapid blood test to assay them has recently gained FDA approval, facilitating their transition from the bench to the bedside. In the pediatric population, this tool was demonstrated to have a sensitivity and negative predictive value of 100% for the detection of clinically important traumatic brain injuries (Puravet et al., 2025). Though significant, this work alludes to the greater promise that biomarkers hold for guiding clinical management and prognostication. Further work directed toward the integration of these biomarkers into clinical practice is crucial for developing targeted therapies that address both the immediate and secondary injuries associated with TBI. By better understanding the specific roles of these biomarkers, particularly those involved in neuroinflammation, clinicians and researchers can tailor treatments that not only mitigate the initial impact of TBI but also the prolonged inflammatory responses that can dictate patient recovery and quality of life.
Imaging techniques
Advancements in imaging techniques have significantly improved our understanding of neuro-inflammatory changes following pediatric traumatic brain injury (TBI). While MRI, including diffusion tensor imaging (DTI), has been widely recognized for its sensitivity to white matter integrity and axonal damage, other advanced technologies such as dual-energy computed tomography (DECT) also play a crucial role in neuro-inflammation research.
DTI is invaluable for its ability to measure the diffusion properties of water molecules within brain tissue, providing detailed insights into white matter disruptions indicative of neuro-inflammatory responses. This MRI technique uses parameters such as fractional anisotropy (FA) and mean diffusivity (MD) to offer a nuanced view of the microstructural changes that are characteristic of inflammation following TBI. DTI’s sensitivity to axonal integrity makes it an excellent tool for detecting diffuse axonal injury (DAI), a common feature in TBI that is closely associated with inflammatory processes (Wozniak et al., 2007; Dennis et al., 2018; Bodanapally et al., 2019; Bodanapally et al., 2019; Basser and Jones, 2002; Basser et al., 1994; Wallace et al., 2018; Arfanakis et al., 2002; Hulkower et al., 2013; Huisman et al., 2004; Roberts et al., 2016; Roberts et al., 2014; Wilde et al., 2008).
DECT, as an advanced imaging technology, extends beyond traditional applications by offering unique capabilities in assessing material composition and structural integrity within the brain. This technique is particularly useful for detecting variations in brain tissue that may signify inflammation, such as the presence of hemorrhagic contusions, which are often associated with traumatic brain injuries. DECT’s ability to image at two different energy levels allows for superior differentiation of tissue types and provides a clearer depiction of the brain’s condition post-injury (Gibney et al., 2020; Hamid et al., 2021; Hu et al., 2016; Tanzil et al., 2023; Bodanapally et al., 2019; Lee et al., 2023).
Furthermore, the use of positron emission tomography (PET) with probes such as the mitochondrial translocator protein 18-kDa (TSPO) enriches our capability to visualize and quantify neuro-inflammation directly. TSPO, released by activated microglia and astrocytes, is a critical marker in neuro-inflammatory studies. Research continues to enhance the specificity of TSPO PET tracers and refine imaging protocols, aiming to provide more precise diagnostic and therapeutic outcomes (Chen et al., 2021; Zhang et al., 2021; Schubert et al., 2021; Wimberley et al., 2021; Nikam et al., 2021). Tau deposits are also a core pathological feature of TBI (Wang et al., 2018; Sarkis et al., 2021). Tau PET imaging with tracers such as 11C-PBB3 has demonstrated that increased tracer binding in neocortical and white matter regions in long-term TBI survivors correlates with late-onset neuropsychiatric symptoms, including psychosis (Takahata et al., 2019). Notably, patients with traumatic encephalopathy syndrome show significantly higher 11C-PBB3 binding in the frontal white matter, and in vitro assays confirm that this binding reflects tau lesions, particularly at the depths of neocortical sulci. The development of new PET probes targeting other elements of the inflammatory pathway such as cyclooxygenase-1 (COX-1), cyclooxygenase-2 (COX-2), and cannabinoid type 2 (CB2) receptors is also promising, potentially offering deeper insights into the complex inflammatory responses in pediatric TBI (Kim et al., 2020; Prabhakaran et al., 2021; Haider et al., 2019; Bedi et al., 2023).
The integration of DTI, DECT, and PET into the diagnostic process not only enriches our understanding of the structural damages associated with TBI but also illuminates the extensive neuro-inflammatory reactions that are critical to developing effective treatment strategies.
Therapeutic options
In addressing the treatment of pediatric traumatic brain injury (TBI), a focused discussion on interventions targeting neuro-inflammation is critical given its pivotal role in both the progression and outcome of TBI. Pharmacologic treatments such as Minocycline and Intravenous Immunoglobulin (IVIG) modulate immune responses and reduce microglial activation, crucial in the secondary injury cascade. These agents have shown promise in mitigating neurodegeneration following acute neuro-inflammatory responses, although further research is needed to fully ascertain their efficacy (Liu et al., 2023; Koulaeinejad et al., 2019; Thom et al., 2017). Antioxidants like N-acetylcysteine, vitamin C, and vitamin E combat oxidative stress—an aftermath of increased reactive oxygen species and lipid peroxidation post-TBI, helping to protect against further cellular injury and support recovery from neuro-inflammatory damage (Davis and Vemuganti, 2022).
Emerging therapies, including stem cell treatments, represent a novel frontier in TBI management. Research on neural stem cells (NSCs) and mesenchymal stem cells (MSCs) has shown potential in regenerating damaged neural tissue and aiding recovery from neurodegenerative impacts of sustained neuro-inflammation (Schepici et al., 2020; Weston and Sun, 2018; Walker et al., 2012; Walker et al., 2011; Savitz and Cox, 2023; Cox et al., 2024; Caplan et al., 2021). These therapies, however, remain largely experimental and require more rigorous trials to establish their safety and effectiveness in pediatric populations.
Surgical interventions such as decompressive craniectomy are essential for managing severe cases where pharmacologic and non-invasive methods are insufficient. Primary decompressive craniectomy is performed urgently to mitigate acute brain swelling and prevent herniation in cases where there is significant brain compression or a deteriorating neurological examination due to an expanding hematoma (Sy et al., 2021; Trimble et al., 2020). Secondary decompressive craniectomy, on the other hand, is considered for late refractory intracranial pressure (ICP) elevation that is unresponsive to other treatments. This surgical approach is only recommended when ICP remains dangerously high despite exhaustive medical interventions, providing a necessary relief to preserve brain function and manage inflammation effectively (Hawryluk et al., 2020). These procedures, particularly when paired with less invasive interventions such as ICP monitoring and CSF drainage, form a comprehensive treatment strategy that addresses both the immediate and secondary impacts of neuro-inflammation in pediatric TBI. By integrating a range of therapeutic approaches—from medical management to surgical intervention—treatment plans can be tailored to effectively manage the complex challenges posed by severe TBI, ultimately improving outcomes and prognoses for affected children. However, it is important to note, that only a small fraction of pediatric TBI is severe enough to necessitate surgical intervention (Dewan et al., 2016).
A vast majority of pediatric TBI is classified as mild or moderate with negative imaging (Dewan et al., 2016; Greenberg et al., 2019; Venkataraman et al., 2018). Management of these children requires a multifaceted approach, encompassing both medical and rehabilitative strategies to optimize recovery and prevent long-term complications. The initial phase of management focuses on accurate diagnosis and symptom monitoring, as persistent post-concussive symptoms can impact cognitive, emotional, and physical functioning (Lumba Brown et al., 2018). Early intervention with cognitive and physical rest is essential, but recent guidelines emphasize a gradual return to activity rather than prolonged restriction, as excessive inactivity may exacerbate symptoms and prolong recovery (Levin and Diaz-Arrastia, 2015; Kirkwood et al., 2008). Non-pharmacological therapies, including cognitive rehabilitation, speech therapy, occupational therapy, and physical therapy, are crucial for managing deficits in attention, memory, balance, and motor function, especially in cases where symptoms persist beyond the acute phase (Kirkwood et al., 2008). Additionally, emotional and psychological support is necessary, as mTBI can contribute to mood disorders, anxiety, and behavioral changes (Lumba Brown et al., 2018). Parental education plays a key role in reinforcing symptom monitoring and ensuring adherence to activity restrictions and rehabilitation plans (Levin and Diaz-Arrastia, 2015). While most children recover within weeks to months, a subset may experience prolonged symptoms requiring specialized interventions, including neuropsychological assessment and targeted therapy (Lumba Brown et al., 2018; Kirkwood et al., 2008).
Overall, these approaches highlight the importance of individualized and multifaceted strategies that incorporate both innovative and traditional treatments to combat the diverse manifestations of TBI in the pediatric population and improve long-term functional outcomes.
Conclusion
This review has articulated the critical roles neuroinflammation plays in the pathophysiology of pediatric traumatic brain injury (TBI), delineating its profound impact on long-term outcomes. We have explored the nuanced interactions between mechanical injury and subsequent biochemical cascades, emphasizing how these interactions lead to persistent inflammatory responses that can drastically alter neurological development and recovery. Advancements in biomarker technology and neuroimaging have been pivotal in enhancing our understanding of these complex processes. The identification of specific biomarkers like OPN and sdc and emerging PET probes tailored to neuroinflammatory markers offers promising avenues for more precise diagnosis and monitoring of TBI progression. Furthermore, imaging modalities such as DTI and PET have provided unparalleled insights into the microstructural and metabolic changes associated with TBI, allowing for a more targeted approach in clinical assessments and interventions.
Despite these advancements, the treatment of pediatric TBI remains challenging, with current therapeutic strategies primarily focusing on mitigating immediate risks and stabilizing the patient. The exploration of new treatments, particularly those targeting the inflammatory and degenerative components of TBI, such as the use of minocycline, IVIG, and stem cell therapies, opens new doors for potentially transformative outcomes. However, the efficacy of these novel interventions requires robust validation through clinical trials to ensure they are both safe and effective for pediatric use. As we move forward, it is imperative that the research community continues to develop and refine strategies that address both the acute and chronic aspects of neuroinflammation in pediatric TBI. Collaborative efforts, including multi-center trials and longitudinal studies, will be essential in overcoming the current limitations in treatment and improving the prognosis for affected children. Ultimately, by deepening our understanding of neuroinflammation’s mechanisms and consequences, we can pave the way for innovative therapeutic approaches that significantly enhance recovery and quality of life for young TBI patients.
Author contributions
SS: Conceptualization, Investigation, Methodology, Writing – original draft, Writing – review & editing. AS: Writing – original draft, Writing – review & editing. DJ: Writing – original draft, Writing – review & editing. CH: Writing – original draft, Writing – review & editing. BO’R: Writing – original draft, Writing – review & editing. MSk: Writing – original draft, Writing – review & editing. AM: Conceptualization, Investigation, Methodology, Writing – original draft, Writing – review & editing. BZ: Funding acquisition, Writing – original draft, Writing – review & editing. ES-M: Funding acquisition, Writing – original draft, Writing – review & editing. JJ: Writing – original draft, Writing – review & editing. CC: Writing – original draft, Writing – review & editing. MSh: Conceptualization, Funding acquisition, Investigation, Methodology, Supervision, Writing – original draft, Writing – review & editing.
Funding
The author(s) declare that financial support was received for the research and/or publication of this article. MSh, BZ, and ES-M are funded by the NIH R01NS126437.
Conflict of interest
The authors declare that the research was conducted in the absence of any commercial or financial relationships that could be construed as a potential conflict of interest.
Generative AI statement
The authors declare that no Gen AI was used in the creation of this manuscript.
Publisher’s note
All claims expressed in this article are solely those of the authors and do not necessarily represent those of their affiliated organizations, or those of the publisher, the editors and the reviewers. Any product that may be evaluated in this article, or claim that may be made by its manufacturer, is not guaranteed or endorsed by the publisher.
References
Abdelhak, A., Foschi, M., Abu-Rumeileh, S., Yue, J. K., D'Anna, L., Huss, A., et al. (2022). Blood GFAP as an emerging biomarker in brain and spinal cord disorders. Nat. Rev. Neurol. 18, 158–172. doi: 10.1038/s41582-021-00616-3
Agoston, D. V., Shutes-David, A., and Peskind, E. R. (2017). Biofluid biomarkers of traumatic brain injury. Brain Inj. 31, 1195–1203. doi: 10.1080/02699052.2017.1357836
Aldrimer, M., Ridefelt, P., Rödöö, P., Niklasson, F., Gustafsson, J., and Hellberg, D. (2013). Population-based pediatric reference intervals for hematology, iron and transferrin. Scand. J. Clin. Lab. Invest. 73, 253–261. doi: 10.3109/00365513.2013.769625
Arfanakis, K., Haughton, V. M., Carew, J. D., Rogers, B. P., Dempsey, R. J., and Meyerand, M. E. (2002). Diffusion tensor MR imaging in diffuse axonal injury. AJNR Am. J. Neuroradiol. 23, 794–802.
Bandyopadhyay, S., Hennes, H., Gorelick, M. H., Wells, R. G., and Walsh-Kelly, C. M. (2005). Serum neuron-specific enolase as a predictor of short-term outcome in children with closed traumatic brain injury. Acad. Emerg. Med. 12, 732–738. doi: 10.1197/j.aem.2005.02.017
Basser, P. J., and Jones, D. K. (2002). Diffusion-tensor MRI: theory, experimental design and data analysis - a technical review. NMR Biomed. 15, 456–467. doi: 10.1002/nbm.783
Basser, P. J., Mattiello, J., and LeBihan, D. (1994). MR diffusion tensor spectroscopy and imaging. Biophys. J. 66, 259–267. doi: 10.1016/S0006-3495(94)80775-1
Bazarian, J. J., Biberthaler, P., Welch, R. D., Lewis, L. M., Barzo, P., Bogner-Flatz, V., et al. (2018). Serum GFAP and UCH-L1 for prediction of absence of intracranial injuries on head CT (ALERT-TBI): a multicentre observational study. Lancet Neurol. 17, 782–789. doi: 10.1016/S1474-4422(18)30231-X
Bedi, S. S., Scott, M. C., Skibber, M. A., Kumar, A., Caplan, H. W., Xue, H., et al. (2023). PET imaging of microglia using PBR28suv determines therapeutic efficacy of autologous bone marrow mononuclear cells therapy in traumatic brain injury. Sci. Rep. 13:16142. doi: 10.1038/s41598-023-43245-0
Bodanapally, U. K., Archer-Arroyo, K. L., Dreizin, D., Shanmuganathan, K., Schwartzbauer, G., Li, G., et al. (2019). Dual-energy computed tomography imaging of head: virtual high-energy monochromatic (190 keV) images are more reliable than standard 120 kV images for detecting traumatic intracranial hemorrhages. J. Neurotrauma 36, 1375–1381. doi: 10.1089/neu.2018.5985
Bodanapally, U. K., Shanmuganathan, K., Parikh, G. Y., Schwartzbauer, G., Kondaveti, R., and Feiter, T. R. (2019). Quantification of iodine leakage on dual-energy CT as a marker of blood-brain barrier permeability in traumatic hemorrhagic contusions: prediction of surgical intervention for intracranial pressure management. AJNR Am. J. Neuroradiol. 40, 2059–2065. doi: 10.3174/ajnr.A6316
Bodanapally, U. K., Shanmuganathan, K., Ramaswamy, M., Tsymbalyuk, S., Aarabi, B., Parikh, G. Y., et al. (2019). Iodine-based dual-energy CT of traumatic hemorrhagic contusions: relationship to in-hospital mortality and short-term outcome. Radiology 292, 730–738. doi: 10.1148/radiol.2019190078
Burda, J. E., Bernstein, A. M., and Sofroniew, M. V. (2016). Astrocyte roles in traumatic brain injury. Exp. Neurol. 275, 305–315. doi: 10.1016/j.expneurol.2015.03.020
Caplan, H. W., Prabhakara, K. S., Toledano Furman, N. E., Zorofchian, S., Kumar, A., Martin, C., et al. (2021). Combination therapy with Treg and mesenchymal stromal cells enhances potency and attenuation of inflammation after traumatic brain injury compared to monotherapy. Stem Cells 39, 358–370. doi: 10.1002/stem.3320
Chen, Z., Haider, A., Chen, J., Xiao, Z., Gobbi, L., Honer, M., et al. (2021). The repertoire of small-molecule PET probes for Neuroinflammation imaging: challenges and opportunities beyond TSPO. J. Med. Chem. 64, 17656–17689. doi: 10.1021/acs.jmedchem.1c01571
Chen, W., Wang, G., Yao, C., Zhu, Z., Chen, R., Su, W., et al. (2022). The ratio of serum neuron-specific enolase level to admission Glasgow coma scale score is associated with diffuse axonal injury in patients with moderate to severe traumatic brain injury. Front. Neurol. 13:887818. doi: 10.3389/fneur.2022.887818
Cheng, F., Yuan, Q., Yang, J., Wang, W., and Liu, H. (2014). The prognostic value of serum neuron-specific enolase in traumatic brain injury: systematic review and meta-analysis. PLoS One 9:e106680. doi: 10.1371/journal.pone.0106680
Chodobski, A., Zink, B. J., and Szmydynger-Chodobska, J. (2011). Blood-brain barrier pathophysiology in traumatic brain injury. Transl. Stroke Res. 2, 492–516. doi: 10.1007/s12975-011-0125-x
Cox, C. S. Jr., Notrica, D. M., Juranek, J., Miller, J. H., Triolo, F., Kosmach, S., et al. (2024). Autologous bone marrow mononuclear cells to treat severe traumatic brain injury in children. Brain 147, 1914–1925. doi: 10.1093/brain/awae005
Czeiter, E., Amrein, K., Gravesteijn, B. Y., Lecky, F., Menon, D. K., Mondello, S., et al. (2020). Blood biomarkers on admission in acute traumatic brain injury: relations to severity, CT findings and care path in the CENTER-TBI study. EBioMedicine 56:102785. doi: 10.1016/j.ebiom.2020.102785
Davis, C. K., and Vemuganti, R. (2022). Antioxidant therapies in traumatic brain injury. Neurochem. Int. 152:105255. doi: 10.1016/j.neuint.2021.105255
De Souza, D. F., Leite, M. C., Quincozes-Santos, A., Nardin, P., Tortorelli, L. S., Rigo, M. M., et al. (2009). S100B secretion is stimulated by IL-1beta in glial cultures and hippocampal slices of rats: likely involvement of MAPK pathway. J. Neuroimmunol. 206, 52–57. doi: 10.1016/j.jneuroim.2008.10.012
Dennis, E. L., Babikian, T., Giza, C. C., Thompson, P. M., and Asarnow, R. F. (2018). Neuroimaging of the injured pediatric brain: methods and new lessons. Neuroscientist 24, 652–670. doi: 10.1177/1073858418759489
Dewan, M. C., Mummareddy, N., Wellons, J. C., and Bonfield, C. M. (2016). Epidemiology of global pediatric traumatic brain injury: qualitative review. World Neurosurg. 91, 497–509.e1. doi: 10.1016/j.wneu.2016.03.045
Donat, C. K., Scott, G., Gentleman, S. M., and Sastre, M. (2017). Microglial activation in traumatic brain injury. Front. Aging Neurosci. 9:208. doi: 10.3389/fnagi.2017.00208
Elgaddal, N., and Black, L. I. (2023). QuickStats: percentage* of children and adolescents aged ≤17 years who had ever received a diagnosis of concussion or brain injury,† by sex and age group - National Health Interview Survey,§ United States, 2022. MMWR Morb. Mortal Wkly. Rep. 72:899. doi: 10.15585/mmwr.mm7233a5
Emery, C. A., Barlow, K. M., Brooks, B. L., Max, J. E., Villavicencio-Requis, A., Gnanakumar, V., et al. (2016). A systematic review of psychiatric, psychological, and Behavioural outcomes following mild traumatic brain injury in children and adolescents. Can. J. Psychiatr. 61, 259–269. doi: 10.1177/0706743716643741
Ergün, R., Bostanci, U., Akdemir, G., Beşkonakli, E., Kaptanoğlu, E., Gürsoy, F., et al. (1998). Prognostic value of serum neuron-specific enolase levels after head injury. Neurol. Res. 20, 418–420. doi: 10.1080/01616412.1998.11740541
Eyolfson, E., Carr, T., Khan, A., Wright, D. K., Mychasiuk, R., and Lohman, A. W. (2020). Repetitive mild traumatic brain injuries in mice during adolescence cause sexually dimorphic behavioral deficits and neuroinflammatory dynamics. J. Neurotrauma 37, 2718–2732. doi: 10.1089/neu.2020.7195
Fraunberger, E., and Esser, M. J. (2019). Neuro-inflammation in pediatric traumatic brain injury-from mechanisms to inflammatory networks. Brain Sci. 9. doi: 10.3390/brainsci9110319
Gao, N., Zhang-Brotzge, X., Wali, B., Sayeed, I., Chern, J. J., Blackwell, L. S., et al. (2020). Plasma osteopontin may predict neuroinflammation and the severity of pediatric traumatic brain injury. J. Cereb. Blood Flow Metab. 40, 35–43. doi: 10.1177/0271678X19836412
Gibney, B., Redmond, C. E., Byrne, D., Mathur, S., and Murray, N. (2020). A review of the applications of dual-energy CT in acute neuroimaging. Can. Assoc. Radiol. J. 71, 253–265. doi: 10.1177/0846537120904347
Gonzalez Rodriguez, E., Cardenas, J. C., Cox, C. S., Kitagawa, R. S., Stensballe, J., Holcomb, J. B., et al. (2018). Traumatic brain injury is associated with increased syndecan-1 shedding in severely injured patients. Scand. J. Trauma Resusc. Emerg. Med. 26:102. doi: 10.1186/s13049-018-0565-3
Greenberg, J. K., Jeffe, D. B., Carpenter, C. R., Yan, Y., Pineda, J. A., Lumba-Brown, A., et al. (2019). North American survey on the post-neuroimaging management of children with mild head injuries. J. Neurosurg. Pediatr. 23, 227–235. doi: 10.3171/2018.7.PEDS18263
Haider, A., Kretz, J., Gobbi, L., Ahmed, H., Atz, K., Bürkler, M., et al. (2019). Structure-activity relationship studies of pyridine-based ligands and identification of a fluorinated derivative for positron emission tomography imaging of cannabinoid type 2 receptors. J. Med. Chem. 62, 11165–11181. doi: 10.1021/acs.jmedchem.9b01280
Hamid, S., Nasir, M. U., So, A., Andrews, G., Nicolaou, S., and Qamar, S. R. (2021). Clinical applications of dual-energy CT. Korean J. Radiol. 22, 970–982. doi: 10.3348/kjr.2020.0996
Hamood, Y., Abdullah, M., El Ghoul, H., Saad, N., Dysko, R. C., and Zhang, Z. (2022). Sex specific effects of buprenorphine on behavior, astrocytic opioid receptor expression and neuroinflammation after pediatric traumatic brain injury in mice. Brain Behav. Immun. Health 22:100469. doi: 10.1016/j.bbih.2022.100469
Harting, M. T., Jimenez, F., Adams, S. D., Mercer, D. W., and Cox, C. S. (2008). Acute, regional inflammatory response after traumatic brain injury: implications for cellular therapy. Surgery 144, 803–813. doi: 10.1016/j.surg.2008.05.017
Hawryluk, G. W. J., Rubiano, A. M., Totten, A. M., O'Reilly, C., Ullman, J. S., Bratton, S. L., et al. (2020). Guidelines for the Management of Severe Traumatic Brain Injury: 2020 update of the decompressive craniectomy recommendations. Neurosurgery 87, 427–434. doi: 10.1093/neuros/nyaa278
Haydel, MJ, Weisbrod, LJ, and Saeed, W. Pediatric head trauma. Treasure Island (FL): StatPearls Publishing. (2025).
Hon, K. L., Leung, A. K. C., and Torres, A. R. (2019). Concussion: a global perspective. Semin. Pediatr. Neurol. 30, 117–127. doi: 10.1016/j.spen.2019.03.017
Hu, R., Besheli, L. D., Young, J., Wu, M., Pomerantz, S., Lev, M. H., et al. (2016). Dual-energy Head CT enables accurate distinction of Intraparenchymal hemorrhage from calcification in emergency department patients. Radiology 280, 177–183. doi: 10.1148/radiol.2015150877
Huisman, T. A. G. M., Schwamm, L. H., Schaefer, P. W., Koroshetz, W. J., Shetty-Alva, N., Ozsunar, Y., et al. (2004). Diffusion tensor imaging as potential biomarker of white matter injury in diffuse axonal injury. AJNR Am. J. Neuroradiol. 25, 370–376.
Hulkower, M. B., Poliak, D. B., Rosenbaum, S. B., Zimmerman, M. E., and Lipton, M. L. (2013). A decade of DTI in traumatic brain injury: 10 years and 100 articles later. AJNR Am. J. Neuroradiol. 34, 2064–2074. doi: 10.3174/ajnr.A3395
Iczkiewicz, J., Rose, S., and Jenner, P. (2007). Osteopontin expression in activated glial cells following mechanical- or toxin-induced nigral dopaminergic cell loss. Exp. Neurol. 207, 95–106. doi: 10.1016/j.expneurol.2007.05.030
Jacquens, A., Csaba, Z., Soleimanzad, H., Bokobza, C., Delmotte, P.-R., Userovici, C., et al. (2024). Deleterious effect of sustained neuroinflammation in pediatric traumatic brain injury. Brain Behav. Immun. 120, 99–116. doi: 10.1016/j.bbi.2024.04.029
Kim, M.-J., Lee, J.-H., Anaya, F. J., Hong, J., Miller, W., Telu, S., et al. (2020). First-in-human evaluation of [11C]PS13, a novel PET radioligand, to quantify cyclooxygenase-1 in the brain. Eur. J. Nucl. Med. Mol. Imaging 47, 3143–3151. doi: 10.1007/s00259-020-04855-2
Kirkwood, M. W., Yeates, K. O., Taylor, H. G., Randolph, C., McCrea, M., and Anderson, V. A. (2008). Management of pediatric mild traumatic brain injury: a neuropsychological review from injury through recovery. Clin. Neuropsychol. 22, 769–800. doi: 10.1080/13854040701543700
Kölliker-Frers, R., Udovin, L., Otero-Losada, M., Kobiec, T., Herrera, M. I., Palacios, J., et al. (2021). Neuroinflammation: an integrating overview of reactive-Neuroimmune cell interactions in health and disease. Mediat. Inflamm. 2021:9999146. doi: 10.1155/2021/9999146
Koulaeinejad, N., Haddadi, K., Ehteshami, S., Shafizad, M., Salehifar, E., Emadian, O., et al. (2019). Effects of minocycline on neurological outcomes in patients with acute traumatic brain injury: a pilot study. Iran J. Pharm. Res. 18, 1086–1096. doi: 10.22037/ijpr.2019.1100677
Lajud, N., Roque, A., Cheng, J. P., Bondi, C. O., and Kline, A. E. (2021). Early life stress preceding mild pediatric traumatic brain injury increases Neuroinflammation but does not exacerbate impairment of cognitive flexibility during adolescence. J. Neurotrauma 38, 411–421. doi: 10.1089/neu.2020.7354
Lee, J., Park, S. T., Hwang, S. C., Kim, J. Y., Lee, A. L., and Chang, K. H. (2023). Dual-energy computed tomography material decomposition improves prediction accuracy of hematoma expansion in traumatic intracranial hemorrhage. PLoS One 18:e0289110. doi: 10.1371/journal.pone.0289110
Levin, H. S., and Diaz-Arrastia, R. R. (2015). Diagnosis, prognosis, and clinical management of mild traumatic brain injury. Lancet Neurol. 14, 506–517. doi: 10.1016/S1474-4422(15)00002-2
Liddelow, S. A., Guttenplan, K. A., Clarke, L. E., Bennett, F. C., Bohlen, C. J., Schirmer, L., et al. (2017). Neurotoxic reactive astrocytes are induced by activated microglia. Nature 541, 481–487. doi: 10.1038/nature21029
Liu, N., Li, Y., Jiang, Y., Shi, S., Niamnud, A., Vodovoz, S. J., et al. (2023). Establishment and application of a novel in vitro model of microglial activation in traumatic brain injury. J. Neurosci. 43, 319–332. doi: 10.1523/JNEUROSCI.1539-22.2022
Lumba Brown, A., Yeates, K. O., Sarmiento, K., Breiding, M. J., Haegerich, T. M., Gioia, G. A., et al. (2018). Diagnosis and Management of Mild Traumatic Brain Injury in children: a systematic review. JAMA Pediatr. 172:e182847. doi: 10.1001/jamapediatrics.2018.2847
Nikam, R. M., Yue, X., Kandula, V. V., Paudyal, B., Langhans, S. A., Averill, L. W., et al. (2021). Unravelling neuroinflammation in abusive head trauma with radiotracer imaging. Pediatr. Radiol. 51, 966–970. doi: 10.1007/s00247-021-04995-z
Okonkwo, D. O., Puffer, R. C., Puccio, A. M., Yuh, E. L., Yue, J. K., Diaz-Arrastia, R., et al. (2020). Point-of-care platform blood biomarker testing of glial fibrillary acidic protein versus S100 calcium-binding protein B for prediction of traumatic brain injuries: a transforming research and clinical knowledge in traumatic brain injury study. J. Neurotrauma 37, 2460–2467. doi: 10.1089/neu.2020.7140
Oris, C., Kahouadji, S., Durif, J., Bouvier, D., and Sapin, V. (2023). Actor and biomarker of mild traumatic brain injury. Int. J. Mol. Sci. 24. doi: 10.3390/ijms24076602
Park, P. W. (2018). Isolation and functional analysis of syndecans. Methods Cell Biol. 143, 317–333. doi: 10.1016/bs.mcb.2017.08.019
Park, S. H., and Hwang, S. K. (2018). Prognostic value of serum levels of S100 calcium-binding protein B, neuron-specific enolase, and Interleukin-6 in pediatric patients with traumatic brain injury. World Neurosurg. 118, e534–e542. doi: 10.1016/j.wneu.2018.06.234
Pearn, M. L., Niesman, I. R., Egawa, J., Sawada, A., Almenar-Queralt, A., Shah, S. B., et al. (2017). Pathophysiology associated with traumatic brain injury: current treatments and potential novel therapeutics. Cell. Mol. Neurobiol. 37, 571–585. doi: 10.1007/s10571-016-0400-1
Pelinka, L. E., Kroepfl, A., Schmidhammer, R., Krenn, M., Buchinger, W., Redl, H., et al. (2004). Glial fibrillary acidic protein in serum after traumatic brain injury and multiple trauma. J. Trauma 57, 1006–1012. doi: 10.1097/01.ta.0000108998.48026.c3
Peters, M. E., and Lyketsos, C. G. (2023). The glymphatic system’s role in traumatic brain injury-related neurodegeneration. Mol. Psychiatry 28, 2707–2715. doi: 10.1038/s41380-023-02070-7
Peterson, AB, Xu, L, and Daugherty, J. Surveillance report of traumatic brain injury-related emergency department visits, hospitalizations, and deaths, United States, 2014. (2019). CDC.
Posti, J. P., Takala, R. S. K., Runtti, H., Newcombe, V. F., Outtrim, J., Katila, A. J., et al. (2016). The levels of glial fibrillary acidic protein and ubiquitin C-terminal hydrolase-L1 during the first week after a traumatic brain injury: correlations with clinical and imaging findings. Neurosurgery 79, 456–464. doi: 10.1227/NEU.0000000000001226
Prabhakaran, J., Molotkov, A., Mintz, A., and Mann, J. J. (2021). Progress in PET imaging of Neuroinflammation targeting COX-2 enzyme. Molecules 26. doi: 10.3390/molecules26113208
Puravet, A., Oris, C., Pereira, B., Kahouadji, S., Gonzalo, P., Masson, D., et al. (2025). Serum GFAP and UCH-L1 for the identification of clinically important traumatic brain injury in children in France: a diagnostic accuracy substudy. Lancet Child Adolesc. Health 9, 47–56. doi: 10.1016/S2352-4642(24)00295-5
Regner, A., Meirelles Da, S. L., and Simon, D. (2018). Traumatic penumbra: opportunities for neuroprotective and Neurorestorative processes. Traumatic Brain Injury Pathobiol. Advan. Diagn. Acute Manag. doi: 10.5772/intechopen.72156
Reis, C., Gospodarev, V., Reis, H., Wilkinson, M., Gaio, J., Araujo, C., et al. (2017). Traumatic brain injury and stem cell: pathophysiology and update on recent treatment modalities. Stem Cells Int. 2017:6392592. doi: 10.1155/2017/6392592
Roberts, R. M., Mathias, J. L., and Rose, S. E. (2014). Diffusion tensor imaging (DTI) findings following pediatric non-penetrating TBI: a meta-analysis. Dev. Neuropsychol. 39, 600–637. doi: 10.1080/87565641.2014.973958
Roberts, R. M., Mathias, J. L., and Rose, S. E. (2016). Relationship between diffusion tensor imaging (DTI) findings and cognition following pediatric TBI: a Meta-analytic review. Dev. Neuropsychol. 41, 176–200. doi: 10.1080/87565641.2016.1186167
Rogan, A., Sik, A., Dickinson, E., Patel, V., Peckler, B., McQuade, D., et al. (2023). Diagnostic performance of S100B as a rule-out test for intracranial pathology in head-injured patients presenting to the emergency department who meet NICE Head injury guideline criteria for CT-head scan. Emerg. Med. J. 40, 159–166. doi: 10.1136/emermed-2022-212549
Rosenberg, R. D., Shworak, N. W., Liu, J., Schwartz, J. J., and Zhang, L. (1997). Heparan sulfate proteoglycans of the cardiovascular system. Specific structures emerge but how is synthesis regulated? J. Clin. Invest. 100, S67–S75.
Roth, T. L., Nayak, D., Atanasijevic, T., Koretsky, A. P., Latour, L. L., and McGavern, D. B. (2014). Transcranial amelioration of inflammation and cell death after brain injury. Nature 505, 223–228. doi: 10.1038/nature12808
Sarkis, G. A., Zhu, T., Yang, Z., Li, X., Shi, Y., Rubenstein, R., et al. (2021). Characterization and standardization of multiassay platforms for four commonly studied traumatic brain injury protein biomarkers: a TBI endpoints development study. Biomark. Med 15, 1721–1732. doi: 10.2217/bmm-2021-0284
Savitz, S. I., and Cox, C. S. (2023). Cell-based therapies for neurological disorders - the bioreactor hypothesis. Nat. Rev. Neurol. 19, 9–18. doi: 10.1038/s41582-022-00736-4
Schepici, G., Silvestro, S., Bramanti, P., and Mazzon, E. (2020). Traumatic brain injury and stem cells: an overview of clinical trials, the current treatments and future therapeutic approaches. Medicina 56. doi: 10.3390/medicina56030137
Schubert, J., Tonietto, M., Turkheimer, F., Zanotti-Fregonara, P., and Veronese, M. (2021). Supervised clustering for TSPO PET imaging. Eur. J. Nucl. Med. Mol. Imaging 49, 257–268. doi: 10.1007/s00259-021-05309-z
Sperry, J. L., Luther, J. F., Okonkwo, D. O., Vincent, L. E., Agarwal, V., Cotton, B. A., et al. (2024). Early GFAP and UCH-L1 point-of-care biomarker measurements for the prediction of traumatic brain injury and progression in patients with polytrauma and hemorrhagic shock. J. Neurosurg. 10, 1–10. doi: 10.3171/2024.1.JNS232569
Sulhan, S., Lyon, K. A., Shapiro, L. A., and Huang, J. H. (2020). Neuroinflammation and blood-brain barrier disruption following traumatic brain injury: pathophysiology and potential therapeutic targets. J. Neurosci. Res. 98, 19–28. doi: 10.1002/jnr.24331
Sy, E. H. C. N., Cisse, Y., Thiam, A. B., Barry, L. F., Mbaye, M., Diop, A., et al. (2021). Decompressive craniectomy: indications and results of 24 cases at the neurosurgery clinic of Fann university hospital of Dakar. Pan Afr. Med. J. 38:399. doi: 10.11604/pamj.2021.38.399.27571
Takahata, K., Kimura, Y., Sahara, N., Koga, S., Shimada, H., Ichise, M., et al. (2019). PET-detectable tau pathology correlates with long-term neuropsychiatric outcomes in patients with traumatic brain injury. Brain 142, 3265–3279. doi: 10.1093/brain/awz238
Tang-Schomer, M. D., Patel, A. R., Baas, P. W., and Smith, D. H. (2010). Mechanical breaking of microtubules in axons during dynamic stretch injury underlies delayed elasticity, microtubule disassembly, and axon degeneration. FASEB J. 24, 1401–1410. doi: 10.1096/fj.09-142844
Tanzil, S. M., Qadri, S. A. K., Ali, S. S., Batchu, V., and Fatima, N. (2023). Computed tomography as a diagnostic imaging tool for pediatric traumatic brain injury: a review. Int. J. Innov. Sci. Res. Technol. 8, 139–144. doi: 10.5281/zenodo.8255947
Taylor, C. A., Bell, J. M., Breiding, M. J., and Xu, L. (2017). Traumatic brain injury-related emergency department visits, hospitalizations, and deaths - United States, 2007 and 2013. MMWR Surveill. Summ. 66, 1–16. doi: 10.15585/mmwr.ss6609a1
Thelin, E. P., Nelson, D. W., and Bellander, B. M. (2017). A review of the clinical utility of serum S100B protein levels in the assessment of traumatic brain injury. Acta Neurochir. 159, 209–225. doi: 10.1007/s00701-016-3046-3
Thom, V., Arumugam, T. V., Magnus, T., and Gelderblom, M. (2017). Therapeutic potential of intravenous immunoglobulin in acute brain injury. Front. Immunol. 8:875. doi: 10.3389/fimmu.2017.00875
Trimble, D. J., Parker, S. L., Zhu, L., Cox, C. S., Kitagawa, R. S., Fletcher, S. A., et al. (2020). Outcomes and prognostic factors of pediatric patients with a Glasgow coma score of 3 after blunt head trauma. Childs Nerv. Syst. 36, 2657–2665. doi: 10.1007/s00381-020-04637-z
Undén, J., and Romner, B. (2010). Can low serum levels of S100B predict normal CT findings after minor head injury in adults?: an evidence-based review and meta-analysis. J. Head Trauma Rehabil. 25, 228–240. doi: 10.1097/HTR.0b013e3181e57e22
Venkataraman, S. S., Cox, C. S., and Shah, M. N. (2018). Judicious angiographic imaging and conservative management should be the standard for pediatric patients at risk for blunt cerebrovascular injury. J. Trauma Acute Care Surg. 85, 651–652. doi: 10.1097/TA.0000000000001948
Walker, P. A., Letourneau, P. A., Bedi, S., Shah, S. K., Jimenez, F., and Cox, C. S. (2011). Progenitor cells as remote “bioreactors”: neuroprotection via modulation of the systemic inflammatory response. World J. Stem Cells 3, 9–18. doi: 10.4252/wjsc.v3.i2.9
Walker, P. A., Shah, S. K., Jimenez, F., Aroom, K. R., Harting, M. T., and Cox, C. S. (2012). Bone marrow-derived stromal cell therapy for traumatic brain injury is neuroprotective via stimulation of non-neurologic organ systems. Surgery 152, 790–793. doi: 10.1016/j.surg.2012.06.006
Wallace, E. J., Mathias, J. L., and Ward, L. (2018). The relationship between diffusion tensor imaging findings and cognitive outcomes following adult traumatic brain injury: a meta-analysis. Neurosci. Biobehav. Rev. 92, 93–103. doi: 10.1016/j.neubiorev.2018.05.023
Wang, K. K., Yang, Z., Zhu, T., Shi, Y., Rubenstein, R., Tyndall, J. A., et al. (2018). An update on diagnostic and prognostic biomarkers for traumatic brain injury. Expert. Rev. Mol. Diagn. 18, 165–180. doi: 10.1080/14737159.2018.1428089
Weston, N. M., and Sun, D. (2018). The potential of stem cells in treatment of traumatic brain injury. Curr. Neurol. Neurosci. Rep. 18:1. doi: 10.1007/s11910-018-0812-z
Wilde, E. A., SR, M. C., Hunter, J. V., Bigler, E. D., Chu, Z., Wang, Z. J., et al. (2008). Diffusion tensor imaging of acute mild traumatic brain injury in adolescents. Neurology 70, 948–955. doi: 10.1212/01.wnl.0000305961.68029.54
Wilkinson, A. A., Dennis, M., Simic, N., Taylor, M. J., Morgan, B. R., Frndova, H., et al. (2017). Brain biomarkers and pre-injury cognition are associated with long-term cognitive outcome in children with traumatic brain injury. BMC Pediatr. 17:173. doi: 10.1186/s12887-017-0925-6
Wimberley, C., Lavisse, S., Hillmer, A., Hinz, R., Turkheimer, F., and Zanotti-Fregonara, P. (2021). Kinetic modeling and parameter estimation of TSPO PET imaging in the human brain. Eur. J. Nucl. Med. Mol. Imaging 49, 246–256. doi: 10.1007/s00259-021-05248-9
Winkler, E. A., Minter, D., Yue, J. K., and Manley, G. T. (2016). Cerebral edema in traumatic brain injury: pathophysiology and prospective therapeutic targets. Neurosurg. Clin. N. Am. 27, 473–488. doi: 10.1016/j.nec.2016.05.008
Wolf, J. A., Stys, P. K., Lusardi, T., Meaney, D., and Smith, D. H. (2001). Traumatic axonal injury induces calcium influx modulated by tetrodotoxin-sensitive sodium channels. J. Neurosci. 21, 1923–1930. doi: 10.1523/JNEUROSCI.21-06-01923.2001
Worthylake, R. A., and Burridge, K. (2001). Leukocyte transendothelial migration: orchestrating the underlying molecular machinery. Curr. Opin. Cell Biol. 13, 569–577. doi: 10.1016/s0955-0674(00)00253-2
Wozniak, J. R., Krach, L., Ward, E., Mueller, B. A., Muetzel, R., Schnoebelen, S., et al. (2007). Neurocognitive and neuroimaging correlates of pediatric traumatic brain injury: a diffusion tensor imaging (DTI) study. Arch. Clin. Neuropsychol. 22, 555–568. doi: 10.1016/j.acn.2007.03.004
Xie, W. W., Ding, Y. J., Bhandari, S., Li, H., Chen, H., Jin, S., et al. (2024). Clinical value of syndecan-1 levels in trauma brain injury: a meta-analysis. Shock 61, 49–54. doi: 10.1097/SHK.0000000000002255
Xiong, Y., Mahmood, A., and Chopp, M. (2018). Current understanding of neuroinflammation after traumatic brain injury and cell-based therapeutic opportunities. Chin. J. Traumatol. 21, 137–151. doi: 10.1016/j.cjtee.2018.02.003
Yue, J. K., Yuh, E. L., Korley, F. K., Winkler, E. A., Sun, X., Puffer, R. C., et al. (2019). Association between plasma GFAP concentrations and MRI abnormalities in patients with CT-negative traumatic brain injury in the TRACK-TBI cohort: a prospective multicentre study. Lancet Neurol. 18, 953–961. doi: 10.1016/S1474-4422(19)30282-0
Zetterberg, H., Smith, D. H., and Blennow, K. (2013). Biomarkers of mild traumatic brain injury in cerebrospinal fluid and blood. Nat. Rev. Neurol. 9, 201–210. doi: 10.1038/nrneurol.2013.9
Zhang, L., Hu, K., Shao, T., Hou, L., Zhang, S., Ye, W., et al. (2021). Recent developments on PET radiotracers for TSPO and their applications in neuroimaging. Acta Pharm. Sin. B 11, 373–393. doi: 10.1016/j.apsb.2020.08.006
Keywords: traumatic brain injury, pediatric TBI, neuroinflammation, biomarkers, neuroimaging, brain injury
Citation: Shah SS, Shetty AJ, Johnston DT, Hanan CL, O’Reilly BT, Skibber MA, Massoud AT, Zhu B, Sevick-Muraca EM, Juranek J, Cox CS Jr and Shah MN (2025) Implications and pathophysiology of neuroinflammation in pediatric patients with traumatic brain injury: an updated review. Front. Neurosci. 19:1587222. doi: 10.3389/fnins.2025.1587222
Edited by:
Karen L. Lankford, Yale University, United StatesReviewed by:
Arman Fesharaki-Zadeh, Yale Medicine, United StatesCopyright © 2025 Shah, Shetty, Johnston, Hanan, O’Reilly, Skibber, Massoud, Zhu, Sevick-Muraca, Juranek, Cox and Shah. This is an open-access article distributed under the terms of the Creative Commons Attribution License (CC BY). The use, distribution or reproduction in other forums is permitted, provided the original author(s) and the copyright owner(s) are credited and that the original publication in this journal is cited, in accordance with accepted academic practice. No use, distribution or reproduction is permitted which does not comply with these terms.
*Correspondence: Ahmed T. Massoud, QWhtZWQudC5tYXNzb3VkQHV0aC50bWMuZWR1