- Joint Department of Biomedical Engineering, University of North Caroline Chapel Hill and North Carolina State University, Chapel Hill, NC, United States
The biosensors are generally described as analytical devices that transduce a signal derived from molecular recognition by biological recognition element (BRE) to quantify analytes or species in samples by combining varieties of transducers with different detection modalities. Therefore, the characteristics of biosensors are highly dependent on BREs. BREs are categorized into two types; a biocatalytic type BRE (BioCat-BREs) and a bioaffinity type BRE (BioAff-BREs). Following the currently most successful biosensors, the sensors for continuous glucose monitors (CGMs) for diabetes management, the development of biosensors for continuous sensing of biomarkers and drugs, such as small molecule drugs, peptides and proteins such as therapeutic antibodies is of increasing interest to both researchers and clinicians. However, unlike glucose oxidoreductases and the concentration of glucose in the mM range, the target molecules for the future continuous monitoring system require the development of innovative BioCat-BREs, which ideally are direct transfer type oxidoreductases, and BioAff-BREs which maintain their high affinity and specificity to the target while their binding site is regenerable under in vivo sensor operating condition, as well as the development of new modalities and devices to detect targets in the µM - pM range.
1 Introduction; why glucose enzymatic sensor has been so successful?
The definition of biosensors can be found in several resources, including but not limited such as IUPAC definition (Labuda et al., 2018), IEEE definition (IEEE Technology Navigator, 2025), and research journals (Thévenot et al., 2001; Turner et al., 1987), where a biosensor is defined as (quote from IUPAC recommendation)
“Measuring instrument requiring no additional reagents and providing selective qualitative and/or quantitative analytical information using a biological recognition element (BRE), which is retained in direct spatial contact with a transduction element (transducer)”, following “Note 1: The biological recognition system, mediated by isolated enzymes, immunosystems, tissues, organelles, whole cells, or others, translates information from the biochemical domain, usually an analyte concentration, into a chemical or physical (electrical, thermal or optical) output signal with a defined sensitivity. Note 2: As a biosensor is a self-contained integrated receptor– transducer device, it should be clearly distinguished from an analytical system that incorporates additional separation steps, such as high performance liquid chromatography (HPLC) or additional hardware and/or sample processing, such as specific reagent introduction, e.g. flow injection analysis (FIA). Thus, a biosensor should be a reagentless analytical device, although the presence of ambient cosubstrates, such as water for hydrolases or oxygen for oxidoreductases, may be required for the analyte determination. HPLC or FIA system may incorporate a biosensor as a detecting device.”
In short, biosensors are generally described as analytical devices that transduce a signal derived from molecular recognition by BRE to quantify analytes or species in samples by combining varieties of transducers with different detection modalities. Therefore, the characteristics of biosensors are highly dependent on BREs. BREs are categorized into two types; a biocatalytic type BRE (BioCat-BREs) and a bioaffinity type BRE (BioAff-BREs). The representative BioCat-BREs are enzymes, especially oxidoreductases such as glucose oxidoreductases.
The currently most successful biosensors are the sensor for continuous glucose monitors (CGMs) for diabetes management, using a biosensor inserted or implanted under the skin to measure interstitial fluid (ISF) glucose concentrations (Lee et al., 2021). The advent of CGMs enabled the development of an automated insulin delivery (AID) system, in which a subcutaneous insulin pump communicates with a CGM and injects insulin based on both real-time and predicted glucose levels. Focusing on the success in realizing the closed-loop system in the use of biosensors to improve therapeutic outcomes for patients with diabetes over the past decade, the development of biosensors for continuous sensing of biomarkers and drugs, such as small molecule drugs, peptides and proteins such as therapeutic antibodies, is of emerging interest to both researchers and clinicians.
What are the opportunities and challenges in developing biosensors for continuous monitoring other than glucose? Is the success of CGM an exception or a representative case/model? The answer is yes and no. Before diving into the main topics of this article, we should summarize and recall how and why enzymatic glucose sensors were developed and were so successful.
The history of biosensor research has been initiated by the development of enzymatic glucose sensor using an oxidoreductase, glucose oxidase, as the first BRE by the late Prof. Clark, which was based on potentiometric principle by the combination of pH monitoring electrode and amperometric principle by the combination of oxygen electrode (Clark and Lyons, 1962). After the advent of enzymatic glucose sensors, various biosensor principles have been proposed one after another, and biosensor research has been promoted. Over the past more than 60 years, enzymatic glucose sensors have led the science and technology of biosensors, such as the combinations of BREs and varieties of sensing principle modalities and transducers. Enzymatic glucose sensors have also led the applications of biosensors industrialization, especially in the field of diabetes management; as analytical instruments for central laboratory testing, for professional such as for point-of-care testing, and for personal use, from single-use disposable sensors, repeated use, and to the sensors for in vivo continuous monitoring, targeting varieties of biological samples such as whole blood, serum, ISF, urine, saliva, tears and sweat.
During these challenges, we learned a lot of technological information including but not limited to the representative configurations of biosensors for both disposable and reusable/continuous monitoring, the issues related to biosensors for in vivo use such as cellular inflammation, the impact of endogenous or exogenous interferents on sensor responses, the required stability of biosensors, the differences between different biological matrices such as whole blood and ISF.
1.1 Why have enzymatic glucose sensors been so successful?
We believe there have been the following 3 “form factors” for this success; 1) the BRE; glucose oxidase is a “catalyst” and a relatively stable enzyme that is easy to handle even for those not familiar with fragile biological materials, 2) the target concentration in the biological fluid; the glucose concentration in the target biological fluid is high (2–40 mM), and importantly, 3) the need; the development of enzymatic glucose sensors has been driven by both academic and market needs, supported by the obvious clinical importance. In this article, I will refer to 1) and 2), but I do not plan to discuss 3) in this article, although we will add our comment that the success of enzymatic glucose sensors is strongly supported by market-driven technology development.
Therefore, to realize biosensors for continuous monitoring system other than glucose, we should understand and acknowledge the advantages and disadvantages of BREs, which will be selected depending on the concentration range of target molecules and potential interferants/ingredients that may affect the recognition and sensing signals of BREs. Then, we can address how to overcome potential problems to develop biosensors for continuous monitoring systems. In Table 1, we have summarized the promising BREs with their features and challenges for the following discussion.

Table 1. Comparison of recognition element types for biosensors: Affinity constants, in vivo regeneration properties, and development challenges.
2 The challenges and opportunities in the BioCat-BREs based sensor for continuous monitoring
The catalytic sites of these BioCat-BREs, which are continuously regenerated after recognition, provide constant signals that can be detected by transducers suitable for designing sensors for continuous monitoring systems. BioCat-BREs include enzymes, cells (prokaryotes and eukaryotes) for both wild-type, genetically engineered or “synthetic” organisms, and enzyme-mimicking catalysts (molecularly imprinted catalysts). Considering the in vivo application of the sensor for a continuous monitoring system, enzymes are the main BioCat BREs to be discussed here. Based on the reactions catalyzed by the enzymes, they are categorized into 7 classification, based on International Union of Biochemistry and Molecular Biology (IUBMB) Enzyme nomenclature and classification (McDonald and Tipton, 2023). Among these types of enzymes, oxidoreductases (EC1) are the most commonly used enzymes for biosensor construction, considering their combination with electrochemical sensors and colorimetric sensing principle with the combination of redox dyes. Oxidoreductases harbor redox cofactors that are reduced (oxidized) while the substrates are oxidized (reduced), while the regeneration of redox cofactors usually provides the signals that can be detected by the transducers. As introduced above, glucose oxidoreductases are the representative redox enzymes that catalyze the oxidation of glucose in the reductive half reaction and their redox cofactors, such as flavin adenine dinucleotide (FAD), pyrroloquinoline quinone (PQQ) or nicotine adenine dinucleotide (phosphate) (NAD or NADP), are reduced (Ferri et al., 2011). The regeneration of the catalytic site is accompanied by the oxidation of reduced redox cofactors in the oxidative half of the reaction, when the electron is transferred to the appropriate electron acceptors. The principles of electrochemical enzymatic sensors using oxidoreductases are then categorized into the 3 generations, depending on the electron acceptors during the oxidative half reaction; 1st generation uses oxygen, 2nd generation uses synthetic electron acceptors, and 3rd generation uses electrode directly referred to as direct electron transfer or DET. Current enzymatic sensors for CGM use 1st and 2nd generation principles. For optimal sensor design, the use of minimal number of components and reactions is essential. Furthermore, avoiding potentially biologically harmful synthetic materials is critical for in vivo applications. The 3rd generation principle, which utilizes enzymes capable of direct electron transfer (DET) with electrodes, represents an ideal approach for continuous in vivo monitoring. However, the availability of oxidoreductases capable of DET with electrodes is limited. Therefore, the creation of engineered BioCat-BREs capable of DET with electrode is an emerging challenge.
Since oxidoreductases originally utilize a variety of biologically derived electron transfer molecules, including electron transfer redox proteins represented by heme b and heme c, the combinations of redox proteins have been investigated, including the construction of genetically engineered fusion proteins between redox enzymes and electron transfer proteins (Sowa et al., 2024). Alternatively, redox enzymes can be modified with redox mediators to achieve a quasi-DET reaction with an electrode (Hatada et al., 2018; Hiraka et al., 2020; Suzuki et al., 2020; Hatada et al., 2021).
What will be the next targets for enzymatic sensors for continuous monitoring? Considering the concentration range of the targets and the availabilities of oxidoreductases, metabolites, nutrients and pharmaceutical synthetic organic molecules are the potential future targets of enzymatic sensors for continuous monitoring, but not proteins/peptides. The enzymes that can catalyze the reactions of proteins/peptides are mainly hydrolases or kinases, which do not produce signals or molecules that can be directly detected optically or electrochemically, in the configurations particularly suitable for the continuous monitoring systems. In addition, the target concentration ranges for proteins/peptides in the in vivo monitoring are in the nM-pM range, while such enzymes have Km values in the µM-mM range.
Here are some example for the future targets and examples for enzymatic sensors for continuous monitoring.
Recent advances in the use of AID systems for diabetic patients are encouraging multimodal parameter monitoring of metabolites, not only glucose but also ketone bodies and lactate, in order to pinpoint the cause of glycemic variability. Monitoring of ketones, especially 3-hydroxybutyrate (β-hydroxybutyrate; BHB), is specifically targeted as the next target for continuous monitoring, continuous ketone monitoring or CKM. The development of CKM is urgently needed for the realization of medical devices to prevent acute diabetic ketoacidosis (DKA), which is a fatal condition not only for type 1 diabetics but also for type 2 diabetics. Sodium-glucose cotransporter-2 (SGLT-2) inhibitors have been widely used in patients with type 2 diabetes, but they can increase the risk of DKA. These clinical needs prompted the development of the CKM system. The challenges in realizing biosensors for CKM are the limited availability of enzymes. The disposable, single-use type BHB enzymatic sensor for personal or professional use has been successively commercialized and utilized using BHB dehydrogenase (BHBDh). BHBDh (EC 1.1.1.30) is an NAD+-dependent oxidoreductase that catalyzes the reversible reaction between BHB and acetoacetate, coupled with NAD+/NADH conversion. BHBDh is currently the only enzyme used to detect BHB for ketone monitoring. The direct oxidation of NADH on the electrode has been one of the major challenges in electrochemical research because it requires high overpotential (>1V vs. Ag/AgCl) and results in inactive form of NAD+ including dimerized NAD+ molecules on the electrode surface. Therefore, the use of appropriate mediator together with NAD+ is essential to construct BHBDh based enzymatic sensors.
The use of Os-complex based “wired technology” is a representative example that is being commercialized, but the availability of such a redox-mediator modified hydrogel is limited considering the unique intellectual property. Several authors also published BHBDh based sensors aimed for CKM application (Teymourian et al., 2020; Alva et al., 2021; Zhang et al., 2022). The authors reported the development of quasi-DET type BHB sensor focusing on its use for CKM, by engineering BHBDh to identify the position of redox mediator direct modification on the surface of enzyme (Ikegai et al., 2024). The further engineering approaches will realize enzymatic sensors for CKM system.
The continuous monitoring of pharmaceutical compounds is also a great opportunity for the use of enzymatic biosensors. Levodopa (L-DOPA) is the gold standard drug for treating patients with Parkinson’s disease (PD), a neurodegenerative disease characterized by the loss of dopaminergic neurons in the substantia nigra. PD is a progressive neurodegenerative disease and patients are typically treated with levodopa, a dopamine precursor that can cross the blood-brain barrier and improve motor symptoms. Fluctuations in levodopa in vivo concentration outside the therapeutic range can lead to significant side effects; lower levodopa levels cause off periods that lead to the return of debilitating symptoms, and higher levodopa levels cause levodopa-induced dyskinesia. Therefore, the development of continuous levodopa monitoring is highly desirable to improve the management of patients with Parkinson’s disease (Probst et al., 2024).
However, the levodopa sensor has yet to be developed. Two major principles have been reported for levodopa sensing: direct electrochemical oxidation of levodopa and oxidoreductase. Direct oxidation of levodopa to dopaquinone is possible at potentials greater than 0.34 V (vs. Ag/AgCl). Because this principle does not use a fragile biocatalyst, it offers long-term stability, although it is inherently limited by poor specificity. The enzymatic principle has been reported using an enzyme, tyrosinase, which oxidizes levodopa; however, tyrosinase can also oxidize several polyphenolic compounds, such as levodopa, and lacks substrate specificity. Although tyrosinase is a DET enzyme when it is used for the cathodic reaction where oxygen is reduced, its DET ability in the anodic reaction when levodopa is oxidized is strongly suppressed because its primary electron acceptor is oxygen under ambient conditions. To overcome this limitation, we recently reported the construction of an engineered DET-type enzyme for levodopa monitoring (Batchu et al., 2025). The enzyme is specific for levodopa and hardly reacts with components and levodopa metabolites as well as co-administered drugs (carbidopa; inhibitor of levodopa degrading enzyme), the sensor using this engineered enzyme can continuously monitor levodopa with the 3rd generation principle. The development of the innovative Bio-CatBRE for levodopa monitoring will realize the sensor for the future in vivo continuous monitoring of levodopa, which will realize the closed-loop therapy together with the combination of continuous levodopa infusion system.
BioCatBREs, especially DET-type oxidoreductase based biosensors, are well suited for the future development of continuous monitoring systems. The key to success will be the targeting of oxidoreductases to detect physiologically relevant concentrations of clinically relevant targets, as demonstrated by the success of glucose enzymatic sensors.
3 BioAff-BREs based continuous monitoring; antibodies, aptamers, receptors, binding proteins, MIPs
BioAff-BREs include antibodies, binding proteins, aptamers, receptors, and synthetic receptors such as molecularly imprinted polymers (MIPs). Considering that the nature of BioAff-BREs is to bind to the target and not to catalyze any reaction, the regeneration of the target binding site is strongly dependent on the dissociation (association) constant; Kd (Ka). In addition, BioAff-BREs do not inherently generate a signal suitable for monitoring by conventional transducers used for enzymatic sensors. Including these considerations, the challenges are 1) in situ regeneration of binding sites of BioAff-BREs, 2) generation of signals for detection or selection of transducers to detect the signals, and 3) method of robust immobilization method suitable for in vivo monitoring and preserving their sensitive selectivity and stabilities.
For the detection of metabolites or pharmaceutical compounds present in the mM - µM range, and when enzymes are not available as the BREs to construct biosensors, binding proteins and aptamers with dissociation constant (Kd) of 10−3 to 10−6 M (mM to µM) level can be used. Aptamers in particular have enabled significant progress in the development of biosensors for in vivo and continuous monitoring of small molecules and metabolites (Downs and Plaxco, 2022; Dauphin-Ducharme et al., 2019). These aptamer-based systems are effective due to several factors: the typically high in vivo concentration of the target compounds, the pseudo-reversibility of affinity-type recognition elements with large apparent dissociation constants (high µM), the transient and dynamic nature of small molecule interactions, and the ability to transduce a signal via conformational changes (Yoo et al., 2020; Zhou et al., 2014).
In these systems, the target binding sites of BioAff-BREs regenerate rapidly, which can follow the change of target concentration even the concentration decreases. The principles of detection using binding proteins and aptamers can recognize their inherent features that the structure of BREs are flexible and change their conformation between ligand-bound and unbound states. These molecular conformational changes are monitored by the modification of BioAff-BREs with redox-probe or by environmentally sensitive fluorescent probes, which can be detected and correlated the values with target concentration by electrochemical (e.g., voltammetry, electrochemical impedance spectroscopy) or by fluorometric methods. The challenge for the application of BioAff-BRE to the sensor for continuous monitoring in this case, for the detection of metabolites or pharmaceutical compounds in the mM - µM range, is therefore availability of cognate BioAff-BREs suitable for the target.
As described above, the development of CKM system is one of the great challenges and opportunities in biosensor research, but the limited availability of BRE, including BioCat-BRE and BioAff-BRE, such as ketone binding proteins, prevents the further progress of this task. Our group has recently discovered a novel group of binding proteins, BHB-binding proteins, which specifically recognize and bind BHB and change their conformation upon binding to BHB (Kane et al., 2024). This discovery accelerates the development of electrochemical binding protein based sensor with redox probe modified BHB binding protein to realize CKM system.
For the detection of protein/peptide biomarkers in picomolar (pM) to nanomolar (nM) concentrations, current biosensor principles use BioAff-BREs such as antibodies and aptamers (Downs and Plaxco, 2022). This is due to their high binding constants (Kd = 10−8 to 10−10 M). The advantages of using such highly sensitive and selective bioaffinity recognition elements are at the same time the disadvantages of using them to create a continuous monitoring system. Namely, the target analyte molecules bind so tightly to the bioaffinity recognition elements that regeneration of these biosensing elements is only possible under harsh chemical conditions or by increasing temperature. In addition, these harsh conditions strip not only the target but also the BRE, especially proteins such as antibodies, from the sensor surface, making re-functionalization of the sensor surface necessary (Figure 1A). Therefore, the most challenging task to realize biosensors for continuous in vivo monitoring of peptides/proteins using BioAff-BREs is the in situ regeneration of biosensors, just releasing the target from BioAff-BREs (Wilson et al., 2023) (Figure 1B).
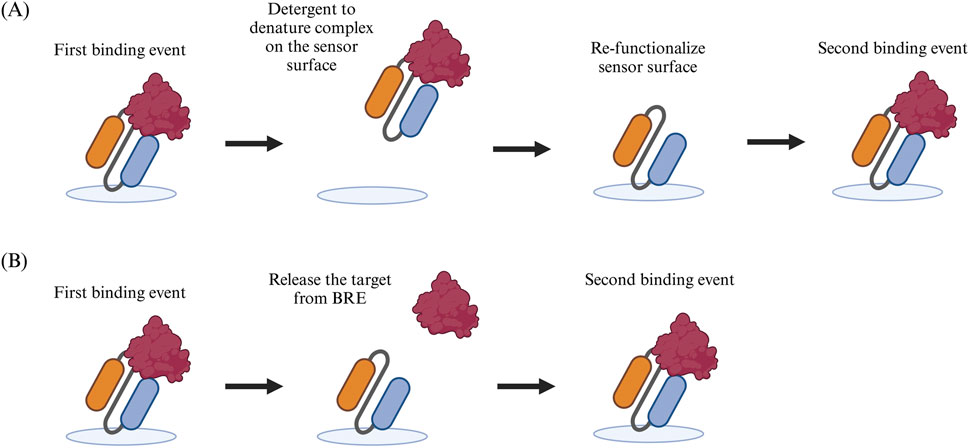
Figure 1. Regeneration strategies for BioAff-BRE sensors (A) The current regeneration method of BioAff-BRE-based sensor. (B) Target-specific release required for in vivo continuous monitoring.
To realize the in situ regeneration of BioAff-BREs, there are the following three approaches; 1) designing the devices or principle that realize the dissociation or control the binding constant of BioAff-BREs, 2) engineering of BioAff-BREs that can be regenerated or their binding constant controlled by commonly available devices and methods, and 3) the combination of 1) and 2). Recent achievements reported by Dr. Kelly’s group based on their molecular pendulum strategies, demonstrated the feasibility of the methods called “oscillation-based sensor regeneration and active reset” and the devices designed for this method to realize real-time continuous monitoring of protein biomarkers using aptamers and antibodies as BioAff-BREs (Zargartalebi et al., 2024). Dr. Daniele’s group reported the electrochemical method and device for regeneration of BioAff-BRE based sensor (Sharkey et al., 2023). They developed a method for electronically pH control without additional reagents using palladium electrode, to induce pH change, which resulted in dissociation of antibody based affinity-binding complex. The number of approaches and challenges in the engineering of BioAff-BREs to create suitable molecule for continuous monitoring are still limited.
Acknowledging the versatility of designing and flexibility and ease in the selective chemical modification of aptamers, some challenges in the design and characterization of in situ regenerable aptamers were reported. To address these challenges, several approaches have been developed. Previous studies have shown that pH affects the affinity of aptamers (Hianik et al., 2007). A noteworthy work on aptamer regeneration using pH changes was reported by Shastri (Shastri et al., 2015). In their work, they combined a thrombin aptamer that changes its binding affinity with pH and a hydrogel that changes its volume to achieve repeatable thrombin binding/release cycles. Additionally, i-motif is a notable example of a structure that undergoes clear pH-dependent conformational changes. Li et al. developed a pH-responsive structure-switchable aptamer by utilizing the fact that i-motif maintains its structure under acidic conditions (Li et al., 2018). This aptamer was combined with a PTK-7 recognition aptamer and split i-motif. Under physiological pH, i-motif cannot maintain its structure, which leads to disruption of the PTK-7 aptamer structure; therefore, this aptamer loses its binding ability to the target. Conversely, by forming an i-motif structure under acidic conditions, this switchable aptamer recovers its binding ability.
Recent studies have demonstrated progress in aptamer regeneration using photoirradiation. Azobenzene is a photo-responsive molecule that undergoes reversible trans-cis isomerization upon UV irradiation. Asanuma’s group reported that azobenzene photo-isomerization drives the dissociation of DNA duplexes (Asanuma et al., 2007). Based on this report, the regeneration of electrochemical aptamer-based sensors utilizing azobenzene has been reported (Zhang et al., 2018; Zhang et al., 2020). In these studies, azobenzene-inserted aptamers are utilized as BREs in electrochemical sensing, achieving repeatable target binding/release through UV irradiation.
However, very limited success has been reported in creating antibodies which are designed for continuous monitoring. Dr. Cortie’s group reported the directed evolution of single-chain variable antibody fragment (scFv) to enable continuous protein sensing by modulating dissociation kinetics (Fercher et al., 2021). They engineered scFv with ∼30 times faster dissociation rates compared to the WT without significantly affecting overall affinity and specificity.
Beyond engineering the BREs themselves, researchers have explored system-level approaches to overcome limitations in in vivo biosensing. Several groups have developed innovative algorithms and detection strategies that can enhance analytical performance without modifying the recognition elements (Lubken et al., 2021; Rong et al., 2017; Buskermolen et al., 2022; Krainer et al., 2023; Maganzini et al., 2022; Das et al., 2021). These methods include extending dynamic range by manipulating the apparent binding constant through system geometry optimization and measuring substrate concentration using pre-equilibrium conditions to achieve kinetics-independent detection. While these alternative approaches demonstrate remarkable innovation, they face considerable challenges in practical in vivo applications. Particular difficulties arise from assumptions that are difficult to maintain under physiological conditions, such as purely reaction-limited responses and the use of homogeneous reporting systems. Nevertheless, these system-level strategies provide valuable alternatives and highlight the importance of pursuing multiple technological pathways to achieve reliable continuous biosensing.
There are varieties of proteins/peptides that are the target for continuous monitoring, such as protein/peptide biomarkers and therapeutic molecules. In particular, the monitoring of therapeutic antibodies, and peptides are the targets for continuous monitoring to understand the pharmacokinetics at the personal level to provide valuable information to maximize the dose effect, ultimately to realize a closed-loop system together with continuous infusion of therapeutic proteins/peptides. Unlike enzymes, the preparation of antibodies, including scFvs, is still modest and requires specific skills for their recombinant preparation. Aptamers can be chemically synthesized, but their inherent in vivo instability due to the presence of endogenous nucleases will be the critical issue for their application in the in vivo continuous monitoring system.
4 Conclusion and future directions
The success of CGM system prompted the current trends in the development of biosensors for continuous monitoring systems. Please note that CGM system is the biomedical device integrating biosensors with other modules, including controller, sensor inserter, algorithm for monitoring, wireless transmission system to enable wearable controlling/monitoring device. The characterization and performance of the sensor are strongly dependent on the BRE, but to maximize and adapt the sensor performance to the in vivo monitoring conditions with the required sensor lifetime and measurable concentration range with the required resolution, sensor design and configuration, optimization of operating conditions with the development of measurement algorithms are necessary. These efforts are always required to realize biosensors for continuous in vivo monitoring. Thanks to the success of CGM systems, these parameters and form factors can be modified and applied to targets other than glucose. However, unlike glucose oxidoreductases and the concentration of glucose in the mM range, the target molecules for the future continuous monitoring system require the development of innovative BioCat-BREs and BioAff-BREs and new modalities and devices to detect targets in the µM - pM range that can operate under target binding site regeneration. The recent unprecedented progress in the application of AI and machine learning in protein structure prediction and design, as well as data analysis and integration, will accelerate the technology development of BREs and detection modalities. The pioneering technologies introduced above will lead the further development of technology elements to be integrated into biosensors to realize continuous in vivo monitoring.
Data availability statement
The datasets presented in this study can be found in online repositories. The names of the repository/repositories and accession number(s) can be found in the article/supplementary material.
Author contributions
MN: Conceptualization, Writing – original draft, Writing – review and editing. KS: Conceptualization, Funding acquisition, Writing – original draft, Writing – review and editing.
Funding
The author(s) declare that financial support was received for the research and/or publication of this article. This work was financially supported by The Leona M. and Harry B. Helmsley Charitable Trust Foundation and Breakthrough T1D.
Acknowledgments
The authors would like to thank for the financially support by the Joint Department of Biomedical Engineering, the University of North Carolina at Chapel Hill and North Carolina State University.
Conflict of interest
The authors declare that the research was conducted in the absence of any commercial or financial relationships that could be construed as a potential conflict of interest.
Generative AI statement
The author(s) declare that Generative AI was used in the creation of this manuscript. The authors used artificial intelligence (AI) language editing, DeepL, in the grammar-checking process of the revised article. The authors reviewed and edited all content, take full responsibility for the content of the article, and ensure that the data, arguments and discussions accurately reflect the authors' views and work.
Publisher’s note
All claims expressed in this article are solely those of the authors and do not necessarily represent those of their affiliated organizations, or those of the publisher, the editors and the reviewers. Any product that may be evaluated in this article, or claim that may be made by its manufacturer, is not guaranteed or endorsed by the publisher.
References
Alva, S., Castorino, K., Cho, H., and Ou, J. (2021). Feasibility of continuous ketone monitoring in subcutaneous tissue using a ketone sensor. J. Diabetes Sci. Technol. 15 (4), 768–774. doi:10.1177/19322968211008185
Asanuma, H., Liang, X., Nishioka, H., Matsunaga, D., Liu, M., and Komiyama, M. (2007). Synthesis of azobenzene-tethered DNA for reversible photo-regulation of DNA functions: hybridization and transcription. Nat. Protoc. 2 (1), 203–212. doi:10.1038/nprot.2006.465
Batchu, K., Probst, D., Satomura, T., Younce, J., and Sode, K. (2025). The development and application of an engineered direct electron transfer enzyme for continuous levodopa monitoring. Npj Biosensing 2 (1), 1–11. doi:10.1038/s44328-024-00020-z
Buskermolen, A. D., Lin, Y. T., van Smeden, L., van Haaften, R. B., Yan, J., Sergelen, K., et al. (2022). Continuous biomarker monitoring with single molecule resolution by measuring free particle motion. Nat. Commun. 13 (1), 6052. doi:10.1038/s41467-022-33487-3
Clark, Jr. L. C., and Lyons, C. (1962). Electrode systems for continuous monitoring in cardiovascular surgery. Ann. N. Y. Acad. Sci. 102 (1), 29–45. doi:10.1111/j.1749-6632.1962.tb13623.x
Das, J., Gomis, S., Chen, J. B., Yousefi, H., Ahmed, S., Mahmud, A., et al. (2021). Reagentless biomolecular analysis using a molecular pendulum. Nat. Chem. 13 (5), 428–434. doi:10.1038/s41557-021-00644-y
Dauphin-Ducharme, P., Yang, K., Arroyo-Currás, N., Ploense, K. L., Zhang, Y., Gerson, J., et al. (2019). Electrochemical aptamer-based sensors for improved therapeutic drug monitoring and high-precision, feedback-controlled drug delivery. ACS Sens. 4 (10), 2832–2837. doi:10.1021/acssensors.9b01616
Downs, A. M., and Plaxco, K. W. (2022). Real-time, in vivo molecular monitoring using electrochemical aptamer based sensors: opportunities and challenges. ACS Sens. 7 (10), 2823–2832. doi:10.1021/acssensors.2c01428
Fercher, C., Jones, M. L., Mahler, S. M., and Corrie, S. R. (2021). Recombinant antibody engineering enables reversible binding for continuous protein biosensing. ACS Sens. 6 (3), 764–776. doi:10.1021/acssensors.0c01510
Ferri, S., Kojima, K., and Sode, K. (2011). Review of glucose oxidases and glucose dehydrogenases: a bird’s eye view of glucose sensing enzymes. J. Diabetes Sci. Technol. 5 (5), 1068–1076. doi:10.1177/193229681100500507
Hatada, M., Loew, N., Inose-Takahashi, Y., Okuda-Shimazaki, J., Tsugawa, W., Mulchandani, A., et al. (2018). Development of a glucose sensor employing quick and easy modification method with mediator for altering electron acceptor preference. Bioelectrochemistry 121, 185–190. doi:10.1016/j.bioelechem.2018.02.001
Hatada, M., Saito, S., Yonehara, S., Tsugawa, W., Asano, R., Ikebukuro, K., et al. (2021). Development of glycated peptide enzyme sensor based flow injection analysis system for haemoglobin A1c monitoring using quasi-direct electron transfer type engineered fructosyl peptide oxidase. Biosens. Bioelectron. 177, 112984. doi:10.1016/j.bios.2021.112984
Hianik, T., Ostatná, V., Sonlajtnerova, M., and Grman, I. (2007). Influence of ionic strength, pH and aptamer configuration for binding affinity to thrombin. Bioelectrochemistry 70 (1), 127–133. doi:10.1016/j.bioelechem.2006.03.012
Hiraka, K., Kojima, K., Tsugawa, W., Asano, R., Ikebukuro, K., and Sode, K. (2020). Rational engineering of Aerococcus viridans l-lactate oxidase for the mediator modification to achieve quasi-direct electron transfer type lactate sensor. Biosens. Bioelectron. 151, 111974. doi:10.1016/j.bios.2019.111974
IEEE Technology Navigator (2025). Biosensors. Available online at: https://technav.ieee.org.
Ikegai, K., Okuda-Shimazaki, J., Tran, T. T., Hatada, M., Asano, R., Ikebukuro, K., et al. (2024). The 2.5th generation enzymatic sensors based on the construction of quasi-direct electron transfer type NAD(P)-Dependent dehydrogenases. Biosens. Bioelectron. 255, 116219. doi:10.1016/j.bios.2024.116219
Kane, B. J., Okuda-Shimazaki, J., Andrews, M. M., Kerrigan, Jr J. A., Murphy, K. V., and Sode, K. (2024). Discovery of periplasmic solute binding proteins with specificity for ketone bodies: β-hydroxybutyrate binding proteins. Protein Sci. 33 (7), e5025. doi:10.1002/pro.5025
Krainer, G., Saar, K. L., Arter, W. E., Welsh, T. J., Czekalska, M. A., Jacquat, R. P. B., et al. (2023). Direct digital sensing of protein biomarkers in solution. Nat. Commun. 14 (1), 653. doi:10.1038/s41467-023-35792-x
Labuda, J., Bowater, R. P., Fojta, M., Gauglitz, G., Glatz, Z., Hapala, I., et al. (2018). Terminology of bioanalytical methods (IUPAC Recommendations 2018). Pure Appl. Chem. 90 (7), 1121–1198. doi:10.1515/pac-2016-1120
Lee, I., Probst, D., Klonoff, D., and Sode, K. (2021). Continuous glucose monitoring systems - current status and future perspectives of the flagship technologies in biosensor research -. Biosens. Bioelectron. 181, 113054. doi:10.1016/j.bios.2021.113054
Li, L., Jiang, Y., Cui, C., Yang, Y., Zhang, P., Stewart, K., et al. (2018). Modulating aptamer specificity with pH-responsive DNA bonds. J. Am. Chem. Soc. 140 (41), 13335–13339. doi:10.1021/jacs.8b08047
Lubken, R. M., Bergkamp, M. H., de Jong, A. M., and Prins, M. W. J. (2021). Sensing methodology for the rapid monitoring of biomolecules at low concentrations over long time spans. ACS Sens. 6 (12), 4471–4481. doi:10.1021/acssensors.1c01991
Maganzini, N., Thompson, I., Wilson, B., and Soh, H. T. (2022). Pre-equilibrium biosensors as an approach towards rapid and continuous molecular measurements. Nat. Commun. 13 (1), 7072. doi:10.1038/s41467-022-34778-5
McDonald, A. G., and Tipton, K. F. (2023). Enzyme nomenclature and classification: the state of the art. FEBS J. 290 (9), 2214–2231. doi:10.1111/febs.16274
Probst, D., Batchu, K., Younce, J. R., and Sode, K. (2024). Levodopa: from biological significance to continuous monitoring. ACS Sens. 9 (8), 3828–3839. doi:10.1021/acssensors.4c00602
Rong, G., Corrie, S. R., and Clark, H. A. (2017). In vivo biosensing: progress and perspectives. ACS Sens. 2 (3), 327–338. doi:10.1021/acssensors.6b00834
Sharkey, C., Twiddy, J., Peterson, K. L., Aroche, A. F., Menegatti, S., and Daniele, M. A. (2023). “Towards electrochemical control of pH for regeneration of biosensors,” in 2023 IEEE BioSensors conference (BioSensors). 1–4. Available online at: https://ieeexplore.ieee.org/document/10281061.
Shastri, A., McGregor, L. M., Liu, Y., Harris, V., Nan, H., Mujica, M., et al. (2015). An aptamer-functionalized chemomechanically modulated biomolecule catch-and-release system. Nat. Chem. 7 (5), 447–454. doi:10.1038/nchem.2203
Sowa, K., Okuda-Shimazaki, J., Fukawa, E., and Sode, K. (2024). Direct electron transfer–type oxidoreductases for biomedical applications. Annu. Rev. Biomed. Eng. 26(Volume 26). 357–382. doi:10.1146/annurev-bioeng-110222-101926
Suzuki, N., Lee, J., Loew, N., Takahashi-Inose, Y., Okuda-Shimazaki, J., Kojima, K., et al. (2020). Engineered glucose oxidase capable of quasi-direct electron transfer after a quick-and-easy modification with a mediator. Int. J. Mol. Sci. 21 (3), 1137. doi:10.3390/ijms21031137
Teymourian, H., Moonla, C., Tehrani, F., Vargas, E., Aghavali, R., Barfidokht, A., et al. (2020). Microneedle-based detection of ketone bodies along with glucose and lactate: toward real-time continuous interstitial fluid monitoring of diabetic ketosis and ketoacidosis. Anal. Chem. 92 (2), 2291–2300. doi:10.1021/acs.analchem.9b05109
Thévenot, D. R., Toth, K., Durst, R. A., and Wilson, G. S. (2001). Electrochemical biosensors: recommended definitions and classification. Biosens. Bioelectron. 16 (1), 121–131. doi:10.1016/s0956-5663(01)00115-4
Turner, A., Karube, I., and Wilson, G. S. (1987). Biosensors: fundamentals and applications. Oxford University Press. Available online at: https://urn.kb.se/resolve?urn=urn:nbn:se:liu:diva-92007.
Wilson, E., Probst, D., and Sode, K. (2023). In vivo continuous monitoring of peptides and proteins: challenges and opportunities. Appl. Phys. Rev. 10 (4), 041309. doi:10.1063/5.0154637
Yoo, H., Jo, H., and Soo Oh, S. (2020). Detection and beyond: challenges and advances in aptamer-based biosensors. Mater Adv. 1 (8), 2663–2687. doi:10.1039/d0ma00639d
Zargartalebi, H., Mirzaie, S., GhavamiNejad, A., Ahmed, S. U., Esmaeili, F., Geraili, A., et al. (2024). Active-reset protein sensors enable continuous in vivo monitoring of inflammation. Science 386 (6726), 1146–1153. doi:10.1126/science.adn2600
Zhang, L., Zhang, X., Feng, P., Han, Q., Liu, W., Lu, Y., et al. (2020). Photodriven regeneration of G-quadruplex aptasensor for sensitively detecting thrombin. Anal. Chem. 92 (11), 7419–7424. doi:10.1021/acs.analchem.0c00380
Zhang, X., Song, C., Yang, K., Hong, W., Lu, Y., Yu, P., et al. (2018). Photoinduced regeneration of an aptamer-based electrochemical sensor for sensitively detecting adenosine triphosphate. Anal. Chem. 90 (8), 4968–4971. doi:10.1021/acs.analchem.7b05442
Zhang, X., Xia, Y., Liu, Y., Mugo, S. M., and Zhang, Q. (2022). Integrated wearable sensors for sensing physiological pressure signals and β-hydroxybutyrate in physiological fluids. Anal. Chem. 94 (2), 993–1002. doi:10.1021/acs.analchem.1c03884
Keywords: biosensors, biological recognition element (BRE), continuous monitoring, in vivo monitoring, enzyme, antibody, binding protein, aptamer
Citation: Nagata M and Sode K (2025) In-vivo continuous monitoring with biosensors based on engineered biological recognition elements: opportunities and challenges. Front. Sens. 6:1579359. doi: 10.3389/fsens.2025.1579359
Received: 19 February 2025; Accepted: 08 April 2025;
Published: 25 April 2025.
Edited by:
Dermot Diamond, Dublin City University, IrelandReviewed by:
Tonghathai Phairatana, Prince of Songkla University, ThailandCopyright © 2025 Nagata and Sode. This is an open-access article distributed under the terms of the Creative Commons Attribution License (CC BY). The use, distribution or reproduction in other forums is permitted, provided the original author(s) and the copyright owner(s) are credited and that the original publication in this journal is cited, in accordance with accepted academic practice. No use, distribution or reproduction is permitted which does not comply with these terms.
*Correspondence: Koji Sode, a3NvZGVAZW1haWwudW5jLmVkdQ==