- Department of Zoology, College of Science, King Saud University, Riyadh, Saudi Arabia
Background: Malaria is a major public health problem caused by the apicomplexan Plasmodium parasite. Cerebral malaria (CM) is the most critical outcome of Plasmodium infection. It is becoming more difficult to manage, particularly in areas of multi-drug resistance. Scientists are focused on identifying alternative strategies to combat malaria infection. Therefore, this study was designed to evaluate the activity of Juglans regia leaf extract (JRLE) in Plasmodium berghei-infected C57BL/6 mice.
Methods: The J. regia leaf extract (JRLE) was prepared using methanol and characterized by Fourier-transform infrared spectroscopy (FT-IR). Female C57BL/6 mice were divided into six groups (5 mice/group): control, non-infected but JRLE-treated (500 mg/kg), P. berghei-infected non-treated, and P. berghei-infected treated with JRLE (250 or 500 mg/kg) or chloroquine (10 mg/kg). Groups (3–6) were infected intraperitoneally with P. berghei (1 × 10⁵). Treatment (oral JRLE or chloroquine) was administered for 5 days starting on day 4. Parasitemia, survival, and body weight were assessed, and brains were collected on day 9 p.i. for histopathological analysis (H&E staining) and GFAP immunohistochemistry. GABA, glutamate, neurotransmitters (epinephrine, norepinephrine, dopamine, serotonin), and mRNA expression of signaling genes (Chrnb2, Gabbr1, Gnai1, Gria2) were evaluated using ELISA and real-time PCR.
Results: Phytochemical screening by FT-IR demonstrated the presence of 10 functional groups in the JRLE. By day 9 after infection with the P. berghei parasite, the parasitemia was significantly reduced after JRLE treatment with a dose of 500 mg/kg (6.33% ± 1.18%) compared to the infected group (23.84% ± 2.06%) with a positive correlation with body weight. Our data showed that JRLE prolonged the survival curve of the infected mice. JRLE ameliorates the reduction of the brain index caused by P. berghei infection. Furthermore, histological analysis showed that infection with P. berghei exacerbates brain damage as evidenced by degeneration of Purkinje cells, cerebral hemorrhage, intravascular sequestrations of parasitized red blood corpuscles (pRBCs), and infiltration of lymphocytes. At the same time, treatment with JRLE mitigates the brain injury induced by the infection. JRLE reduced the level of GFAP expression in the brain tissue of the infected mice. Additionally, treatment with JRLE ameliorates the brain neurotransmitter disbalance (i.e., epinephrine, norepinephrine, dopamine, and serotonin) after Plasmodium infection. Upon JRLE treatment, Chrnb2, Gnai1, and Gabbr1 mRNA expression were down-regulated in the brain tissues derived from infected female C57BL/6 mice. Meanwhile, mRNA expression of Gria2 was up-regulated after JRLE inoculation. Our study proved that JRLE significantly ameliorated the neurotransmitter markers by increasing GABA levels and decreasing the glutamate level in the brain of P. berghei-infected mice.
Conclusion: Taken together, the data reported here illustrate that J. regia leaf extracts possess potent antimalarial effects and may offer a potential drug lead for developing a safe, effective, and affordable antimalarial therapy. Further studies are recommended to include the broader organ-specific effects of plant extract.
Introduction
Malaria is a life-threatening malady caused by mosquito-borne parasites belonging to the genus Plasmodium (phylum Apicomplexa) that have complex life cycles involving two hosts (female Anopheles mosquito and vertebrate host) (1). Four types of Plasmodium cause malaria in humans (Plasmodium ovale, Plasmodium vivax, Plasmodium falciparum, and Plasmodium malariae) (2). Among these four types, the P. falciparum that causes malaria falciparum is the most dangerous type because it can cause serious complications that lead to death (3). Rodent-specific Plasmodium species including Plasmodium berghei, Plasmodium yoelii, Plasmodium chabaudi, and Plasmodium vinckei, are commonly used in mouse models to study malaria (4).
Despite significant progress in the fight against malaria, about a billion people worldwide are at risk of malaria infection (5). In 2022, a total of 241 million cases of malaria and 627,000 deaths were reported globally (6). In Saudi Arabia, the highest malaria rates are mostly found in the southwestern parts of the country with sporadic malaria occurrence (7). The outcome of the Plasmodium infection can vary from a symptomatic infection to mild febrile disease, to severe malaria, the deadliest form of which is cerebral malaria (CM) (8). CM is the most severe neurological complication of malaria, and cognitive and behavioral deficits are commonly reported in surviving cases (9). One of the major hypotheses in CM is the sequestration of parasitized erythrocytes (pRBCs) in the cerebral microvascular endothelium leading to flow obstruction and decreased tissue perfusion, thereby compromising the function of the central nervous system (10). Due to the high degree of reproducibility, easily manageable characteristics, and development of histopathological and neurological signs typical of human CM, the murine model using P. berghei has been widely used to understand this condition better (11).
Active malaria chemotherapy helps to treat malaria in conjunction with blocking the vector’s parasite infectivity by exploiting the metabolic differences between Plasmodium and the host. Factors that include parasite resistance and unspecific drug toxicity restrict the therapeutic efficacy of the drugs (12). Antimalarial drugs may be divided into causal prophylaxis (act on the pre-erythrocytic forms), gametocytocides (act on gametocytes), sporontocides (inhibit the sporogonic phase of development of the parasite in the mosquito), and schizontocides (act on the asexual erythrocytic forms of all species of malaria parasites) (13). Chloroquine (CQ) is a schizontocidal drug that is strong and rapid for treating and preventing clinical symptoms (14).
Owing to the presence of antiparasitic drug resistance, experts are looking to find alternate sources of protection (15). Current frontline antimalarial drugs are either derived directly from plants or are synthetically produced from a plant-derived chemical compound as a template (16). Several studies have been conducted on various plants to find new natural antimalarial medicine in Saudi Arabia (17–20). The reliance on herbal medicine is mainly attributed to factors such as affordability, availability, and accessibility (21). Increasing research data has demonstrated the potential of herbal drugs owing to the many bioactive compounds they contain (22).
The studied plant, Juglans regia, belongs to the genus Juglans (family Juglandaceae). Walnuts (J. regia) have a significant economic value and medicinal importance for human health because of their biochemical composition of polyunsaturated fatty acids (23), fiber, minerals (potassium, calcium, and magnesium) (24), amino acids and proteins (25), and phenolic compounds and saponins (26–28). Among J. regia parts, leaves are highly effective in acting as an alternative therapy. Ethnopharmacological studies of walnuts indicate that it is a rich source of phytochemicals that shown to have therapeutic effects, including gastro-protective (29), antibacterial (30), anticancer (31), hepatoprotective and anti-inflammatory (32), antioxidant (33), anti-diabetic (33), antimicrobial (34), and antifungal (35). Based on the above ethnobotanical and bio-activity studies, the current study aims to investigate the in vivo anti-malarial effect of the methanolic J. regia leaf extracts (JRLE) in P. berghei-infected mice for the first time.
Materials and methods
Plant collection and commercial drug
Leaves of Juglans regia (walnut) were collected from Al Bahah City, Saudi Arabia. Authentication of the plant with voucher number KSU-21595 was carried out in the herbarium of the Botany Department, College of Science, King Saud University. Chloroquine diphosphate salt (Sigma-Aldrich, St. Louis, United States) was used as a commercially available standard antimalarial drug.
Preparation of Juglans regia leaf extracts
The leaves of J. regia were washed with water, shade-dried, and coarsely powdered in a grinder. Walnut leaf extracts were prepared according to the method described by Manikandan et al. (36). In brief, the air-dried powder (100 g) of walnut leaves was extracted by percolation with 70% methanol for higher extraction efficiency and kept in the refrigerator for 24 h, filtered, and concentrated under reduced pressure in a rotatory evaporator at 55°C. To evaporate the alcohol and obtain a dry extract, it was placed at 37°C for 15 h. The residue was collected and placed in sealed bottles. The freeze-dried extracts were kept at −20°C until used for antimalarial testing.
Fourier-transform infrared spectroscopy
A small quantity of JRLE was mixed with potassium bromide as 1: 99 wt % and ground to form a uniform consistency, then pulverized and analyzed using Thermo Scientific’s optical spectrometer NICOLET 6700 (Waltham, United States), according to the recommended protocol of Negrea et al. (37). Maximum absorption was reported in the number of waves in a range of 4,000–400 cm−1 at 25°C.
Activation of Plasmodium parasite
Cryopreserved P. berghei parasite was passaged four times in laboratory mice (Mus musculus, n = 5). Parasitemia of infected mice was measured by staining thin tail blood smears with 10% Giemsa solution (Sigma-Aldrich; diluted in PBS), using the method of Hilou et al. (38). Parasitemia (% of pRBCs) was evaluated by microscopic count and calculated as follows: [(number of pRBCs)/(total numbers of RBCs counted)] × 100. When parasitemia reached about 20%, parasitized blood was collected in a heparinized tube and diluted in 200 μL of phosphate-buffered saline (PBS; pH 7.4) to 1 × 105 parasitized erythrocytes/ml (39). The infection of C57BL/6 female mice (experimental animals) was initiated by the passage of the P. berghei parasite via an intraperitoneal injection (40). Each mouse received 100 μL of dilute infected whole blood containing 1 × 105 parasitized erythrocytes.
Experimental animals
Female mice of the C57BL/6 strain (n = 30) were used in this study. Animals were obtained from the animal facility in the College of Pharmacy (King Saud University, Saudi Arabia). Mice were approximately 9–12 weeks old at the start of the study (weights in the 20–25 g). They were housed in groups of 5 mice per polypropylene cage for 7 days before being used in the experiment. Mice were maintained at room temperature (23°C ± 2°C) in a 12 h light/dark cycle, given free access to standard laboratory animal food and water ad libitum. This study was approved by the Research Ethics Committee (REC) for Laboratory Animal Care at King Saud University (KSU-SE-24-74).
Experimental design
Experimental mice were divided into six groups of five each, as follows:
Group 1: Non-infected and non-treated (negative control).
Group 2: Non-infected and treated with the potentially effective JRLE dose with low parasitemia (%).
Group 3: Infected and non-treated (positive control).
Group 4: Infected and treated with JRLE at 250 mg/kg of body weight (B.W.).
Group 5: Infected and treated with JRLE at 500 mg/kg of B.W.
Group 6: Infected and treated with the antimalarial drug, chloroquine (CQ), at 10 mg/kg of B.W.
All groups, except groups (1) and (2), were intraperitoneally injected with 1 × 105 P. berghei-parasitized erythrocytes, according to Timms et al. (41). The mice were then left for 3 days post-infection. On day 4, treatments were administered orally as follows: groups (4 and 5) were orally treated with different concentrations of JRLE, according to Sharif et al. (42), and group (6) was treated with chloroquine, according to Abay et al. (43). Also, each mouse was weighed on day 9 and the difference between the pre- (day 1) and post-infection with P. berghei (day 9) body weights was calculated according to Dkhil et al. (44). On day 9 p.i., all mice were sacrificed by CO2 asphyxiation, dissected, and then samples were obtained.
Parasitemia and survival rates
From the 3rd-day post-infection (dpi) onwards, the parasitemia of each infected experimental mouse was measured by thin blood smear, as earlier described. Parasitemia (%) was determined as described above. P. berghei-infected, infected-JRLE, and infected-CQ mice were monitored daily, and the time of death (days) was promptly registered to determine the survival rate curve, according to Ezike et al. (45). Each group’s survival time was calculated using the following equation: [total survival time of animals in the group/number of animals in the group].
Sample collection
After the scarification of mice on day 9, the whole brain was removed from the mouse skull. Brain index was calculated using the equation: [(Brain weight.gm/mouse weight) × 100]. The brain was divided into two halves along the midline, according to Al-Shaebi et al. (39), and then preserved: (i) In neutral buffered formalin (NBF), it was used for histopathological examination. (ii) it was wrapped and stored at −80°C for neurotransmitter estimation in clean plastic films. (iii) In RNA later, it was stored at −80°C for later use in gene expression assay.
Histopathological examination
According to Ogundolie et al. (46), the fixed brains in 10% NBF were used for histopathology analysis. The tissues were dehydrated in graded ethyl alcohol, treated with xylene, socked in paraffin wax, and finally cut into 5 μm thickness using a microtome machine. These sections were stained with hematoxylin and eosin (H&E) using standard protocols. Sections were examined and photographed using an Olympus B × 61 microscope (Tokyo, Japan).
Immunohistochemical study
Detection of Glial Fibrillary Acidic Protein (GFAP) was performed according to Varma et al. (47). Brain sections (5 μm) were deparaffinized as per standard protocol. Sections were rehydrated in descending alcohol series. Antigen retrieval of all slides was done, after which each slide was treated with methanol containing 4% H2O2 for 30 min followed by placement for 10 min in 0.05 M Tris–HCl buffer (pH 7.4). Sections were covered with the primary antibody of mouse anti-GFAP (BioGenex, United States) in a 1:200 dilution in phosphate-buffered saline (PBS) and incubated for 45 min in a humid chamber at room temperature. Following removal of the primary antibodies and repetitive rinsing in PBS, sections were incubated with a biotin-conjugated secondary antibody of anti-mouse antiglobulin in PBS (Santa Cruz Biotechnology) containing carrier protein and sodium azide (15 mmol/L) large volume (Universal BioGenex kit). Horseradish peroxidase (HRP) conjugated streptavidin was used to cover the brain sections at room temperature and incubated for 30 min. After rinsing, slides were covered with substrate chromogen solution and incubated at room temperature until the optimum brown color peroxidase product was developed. Counter staining was done with hematoxylin (Sigma Chemical Co.). All sections were photographed using an Olympus B × 61 microscope (Tokyo, Japan).
Neurotransmitters estimation
Contents of epinephrine (E), norepinephrine (NE), dopamine (DA), and serotonin (5-HT) were determined in the isolated brain samples, according to the method of Ciarlone (48). In brief, 0.3 mL of tissue homogenate was combined with 0.1 N hydrochloric acid and 3 mL of butanol to prepare duplicate internal standard tubes for neurotransmitter analysis. After centrifugation at 1,000 × g for 5 min, 2.5 mL of the supernatant was mixed with 1.6 mL of 0.1 N hydrochloric acid and 5 mL of heptane, vortexed for 2 min, and re-centrifuged. The organic phase was discarded, with 0.2 mL of the aqueous phase reserved for 5-HT analysis and 1 mL for E, NE, and DA analysis, kept on ice. For 5-HT external standards, 0.2 mL samples in 0.1 N hydrochloric acid had 1.2 mL of o-Phthalaldehyde added, mixed, and heated in a water bath for 10 min before spectrophotometric reading at 470 nm. For E, NE, and DA, 300 mg of neutral alumina was added, followed by shaking and centrifugation. The supernatant was discarded, and 4 mL of distilled water was added, followed by elution with 1.5 mL of 0.2 N acetic acid. External standards for E, NE, and DA were made in 0.2 N acetic acid with 0.2 mL EDTA added. A reagent blank was prepared with alkaline sulfite, iodine, and acetic acid. After heating, fluorescence was measured for E and NE at 480 nm and DA at 375 nm.
Quantitative real-time polymerase chain reaction
RNA was extracted from the brain tissues tissue using the Qiagen RNeasy Plus Mini kit. Concentration and purity for each RNA sample were detected by NanoDrop ND-1000 Spectrophotometer (NanoDrop Technologies, Wilmington, Delaware, United States) using 260 and 260/280 nm ratios. The RNA samples were reverse-transcribed using a high-capacity RNA-to-cDNA Master Mix kit to prepare cDNA. The primers used in the present study were designed using Primer-BLAST, and amplification was done using the specific PCR primers for different genes involved in neurotransmitter signaling and Thermo Scientific Maxima® SYBR Green/ROXqPCR Master Mix (2×) by Rotor-Gene Q (Qiagen, United States) (Table 1). The resulting Threshold Cycle (Ct) value determined the 2−ΔΔCt of mRNA expression of the different genes (49). The reference gene was beta-actin (β-actin).
Sandwich enzyme-linked immunosorbent assay
Using mouse enzyme-linked immunosorbent assay (ELISA) kits, the level of neurotransmitter markers, including Gamma-Aminobutyric Acid (GABA) (Cat. MBS269152, MyBioSource, United States) and Glutamate (Cat. KA1909, Abnova, United States), was investigated following the protocol instructions. Optical densities (OD) of outcomes from the brain samples were measured using the Bio-Rad IMark Microplate Reader SW 1.04.02.E. Based on a standard curve, OD values were converted to concentrations and presented as ng/mg (for GABA) and ug/mg (for glutamate).
Statistical analysis
The data from individual experiments was presented as mean ± SD. Differences between groups were analyzed using one-way analysis of variance (ANOVA) through the SPSS v.18 software program (SPSS Inc., Chicago, Illinois, United States). The minimum criterion for statistical significance was set at a p-value ≤ 0.05 for all group comparisons.
Results
The analysis of JRLE using FT-IR showed major bands at 3,425.03, 2,928.14, 1,615.40, 1,516.13, 1,449.47, 1,282.61, 1,055.11, 817.69, 778.01, and 590.73 cm−1 (Figure 1). O-H stretching was indicated by the band at 3,425.03 cm−1 confirming the presence of alcohol. The band at 2,928.14 cm−1 implied C-H stretching for the presence of alkane. C=C stretching at 1,615.40 cm−1 confirming the presence of α,β-unsaturated ketone. The band at 1,516.13 cm−1 implied N-O stretching for the presence of nitro compound. C-H stretching at the band 1,449.47 cm−1 confirmed the presence of alkane. The band 1,282.61 cm−1 (C-O stretching), 1,055.11 cm−1 (C-O stretching), 817.69 cm−1 (C=C stretching), and 778.01 cm−1 (C-H stretching), 590.73 cm−1 (C-I stretching) confirmed the presence of aromatic ester, primary alcohol, alkene, 1,2,3-trisubstituted, and halo compound, respectively (Table 2).
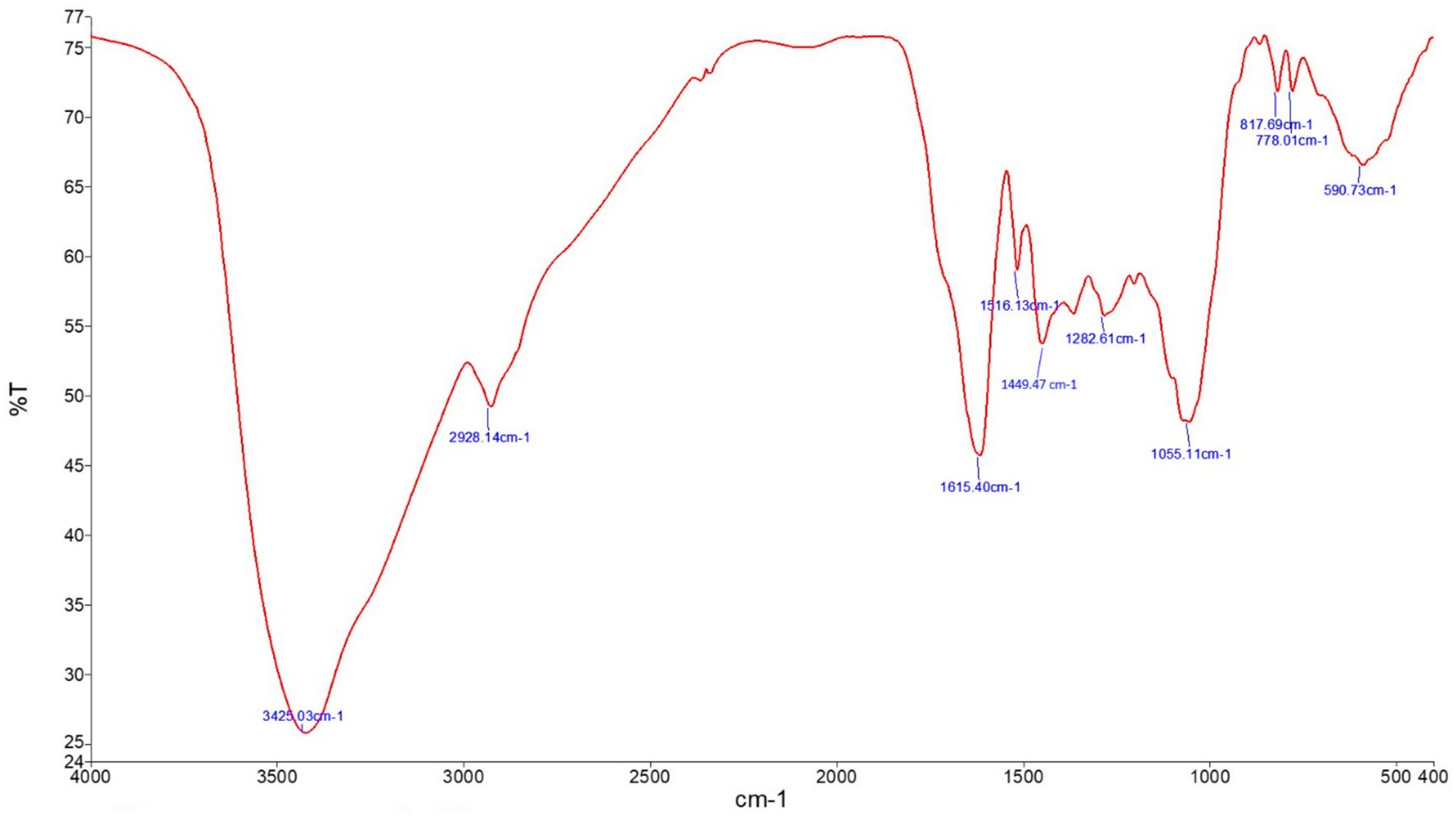
Figure 1. FT-IR of Juglans regia leaf extracts in an aqueous medium showing the functional characteristic of the material.
Parasitemia levels were assessed using Giemsa-stained blood smears (Figure 2). On day 9 post-infection, mice infected with P. berghei at an inoculum of 1 × 10⁵ parasitized erythrocytes exhibited a parasitemia level of 23.84% ± 2.06%. Treatment with plant extracts significantly reduced parasitemia in a dose-dependent manner. Specifically, doses of 250 mg/kg and 500 mg/kg of JRLE reduced parasitemia to 13.25% ± 1.53% and 6.33% ± 1.18%, respectively. In contrast, infected mice treated with chloroquine exhibited the lowest parasitemia level (3.11% ± 0.69%), demonstrating that the standard drug exhibited the highest antiplasmodial activity compared to JRLE.
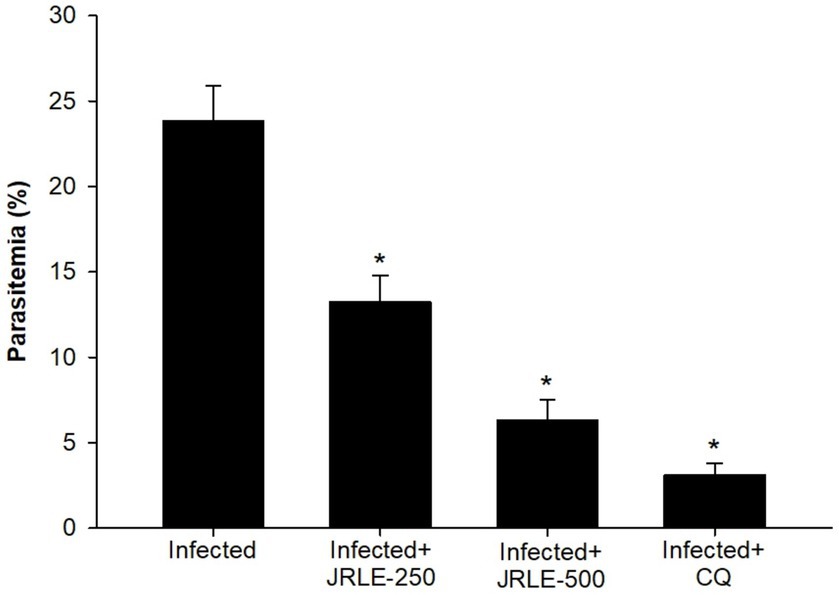
Figure 2. Percentage of parasitemia in mice infected with Plasmodium berghei and treated with various doses of JRLE or chloroquine (CQ). Data are presented as mean ± SD at p ≤ 0.001. *Significance against the infected group.
Figure 3 shows the survival rate of experimental animals in each group concerning the time of infection. Our data showed that during P. berghei infection, mice in the infected group started to die by day 3 p.i. Treatment with JRLE in a dose of 250 mg/kg affected the mortality rate of P. berghei-infected mice, which began to die by day 7 p.i. On the other hand, treatment with 500 mg/kg JRLE improved the clinical outcomes of Plasmodium infection and expanded the survival time until day 9 p.i. (Figure 3). All the mice in the CQ group survived during the experiment (Figure 3).
The mean body weight (BW) analysis over 9 days is illustrated in Figure 4, highlighting significant differences between the studied groups. On day 9 post-infection (p.i.), the control group exhibited a significant BW increase of 9.62%. In contrast, P. berghei infection caused severe weight loss in the infected group, with an average decrease of −7.25%. Treatment with JRLE or chloroquine (CQ) significantly mitigated the weight loss. The JRLE-treated group showed an average BW change of −3.82%, while the CQ-treated group had a slightly greater reduction in BW, averaging −4.34%. These results indicate that both treatments improved weight retention, although chloroquine had a slightly lower effect compared to JRLE.
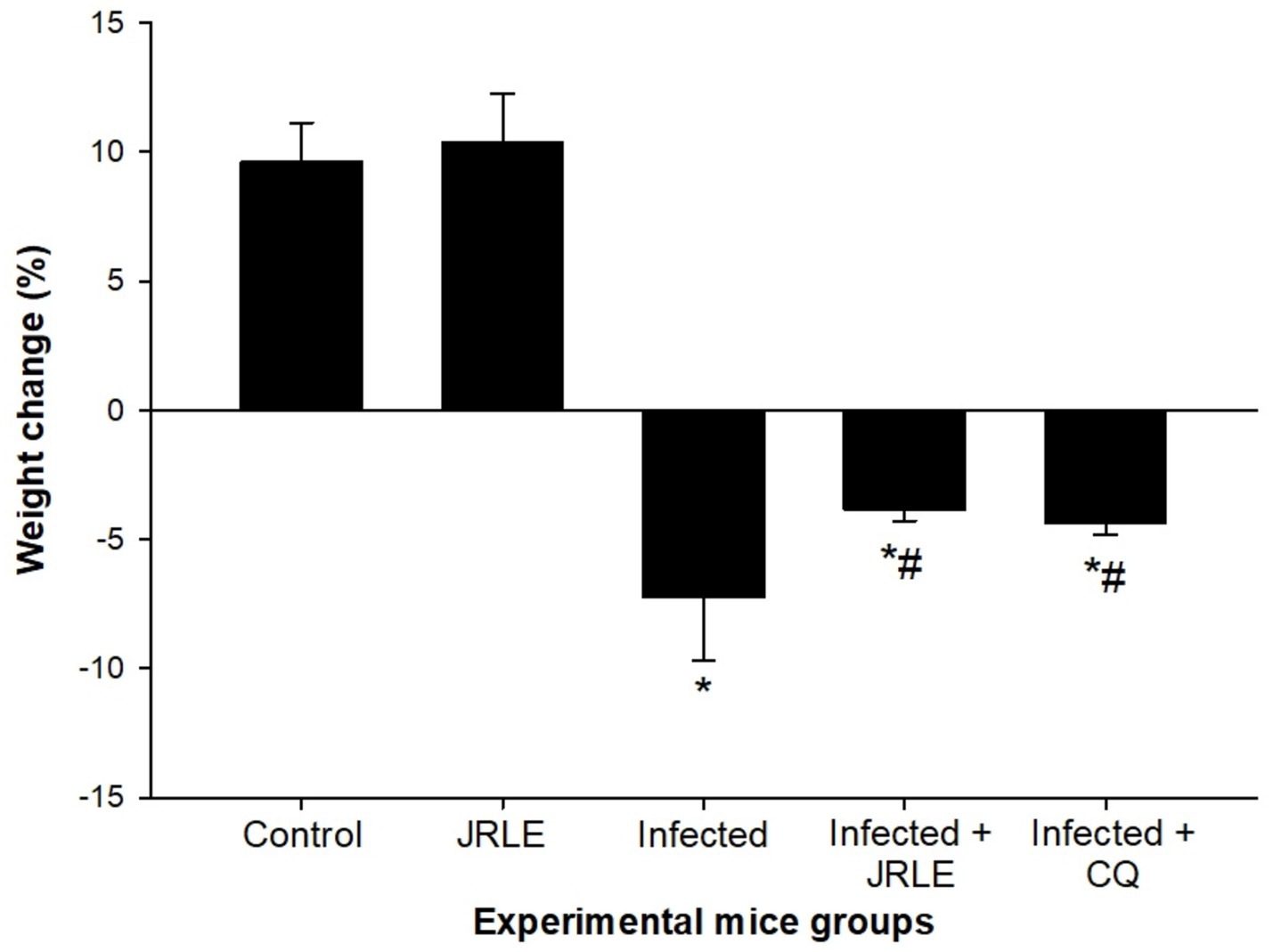
Figure 4. JRLE improved weight loss due to infection with Plasmodium berghei in mice. Values are means ± SEM. *Significance (p ≤ 0.05) against non-infected control group, #Significance (p ≤ 0.05) against infected group.
The brain index, calculated as the ratio of brain weight to body weight, revealed significant differences among the groups of infected mice. The infected group exhibited a notably higher brain weight compared to the control group (Figure 5). By day 9 post-infection (p.i.), the infected mice showed a significant increase in brain weight, averaging 2.59 ± 0.19. Treatment with JRLE effectively reduced the brain weight to 2.08 ± 0.09, closely resembling the brain weight observed in the chloroquine-treated group (2.00 ± 0.07) (Figure 5). These results highlight the potential of JRLE in mitigating the effects of P. berghei infection on brain weight.
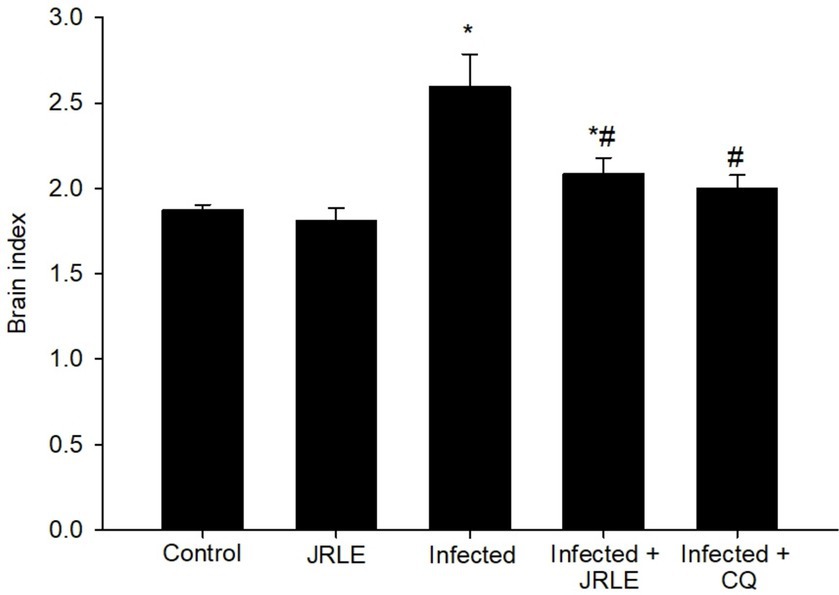
Figure 5. Brain index among the experimental mice groups. Values are means ± SEM. *Significance (p ≤ 0.05) against non-infected control group, #Significance (p ≤ 0.05) against infected group.
To better understand the impact of P. berghei infection on brain status, hematoxylin–eosin staining sections were utilized to characterize histopathological changes in the cerebellum (Figure 6). The findings show that brain tissue sections from control mice, as well as those in the non-infected treated group with 500 mg/kg, exhibited no morphological alterations, which is reassuring. In contrast, the P. berghei-infected animals revealed concerning progressive morphological changes by day 9 p.i. These included degeneration of Purkinje cells, cerebral hemorrhage in dilated sinusoids, intravascular sequestrations of parasitized red blood cells (pRBCs), and lymphocyte infiltration (Figure 6). Notably, the histological analysis of brain tissue from infected mice treated with 500 mg/kg JRLE showed significant improvements, resembling the histological features of the chloroquine-treated group (Figure 6).
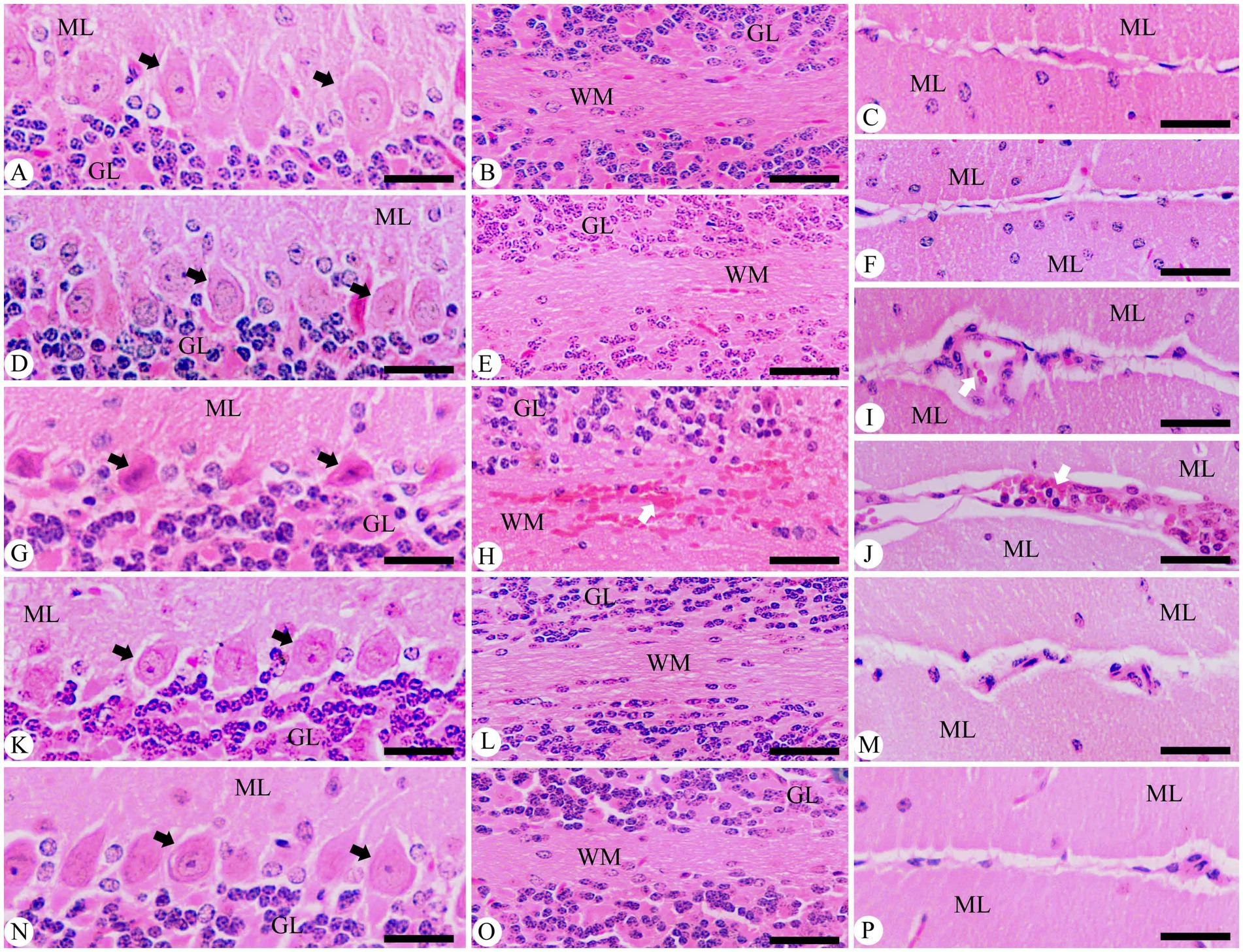
Figure 6. Photomicrographs of the cerebellum in different experimental groups on day 9 p.i. with Plasmodium berghei. (A–C) Control group. (D-F) non-infected-treated group with 500 mg/kg of JRLE. (G–J) Infected group. (K–M) Infected-treated group with 500 mg/kg of JRLE. (N–P) Infected-treated group with chloroquine. ML, molecular layer; GL, granular layer; WM, white matter; Black arrows, Purkinje cell; White arrows, hemorrhage. Sections were stained with hematoxylin and eosin. Scale Bar = 100 μm.
For a deeper understanding, brain sections from different experimental groups underwent immunohistochemical staining to assess GFAP-positive cells in the cerebellum. These cells play a critical role in maintaining structural stability and integrity, alongside supporting the homeostatic functions of astrocytes (Figure 7). In the control group, the presence of GFAP-positive cells was within normal limits. Unfortunately, Plasmodium infection led to an increase in these cells around the Purkinje cell layer and in both the cerebellar molecular and white layers compared to normal levels in control mice (Figure 7). Notably, these changes were more pronounced in areas of neuronal loss. Following treatment with JRLE, GFAP expression significantly decreased in the brains of mice affected by P. berghei, indicating a reduction in cellular reactivity compared to the infected group.
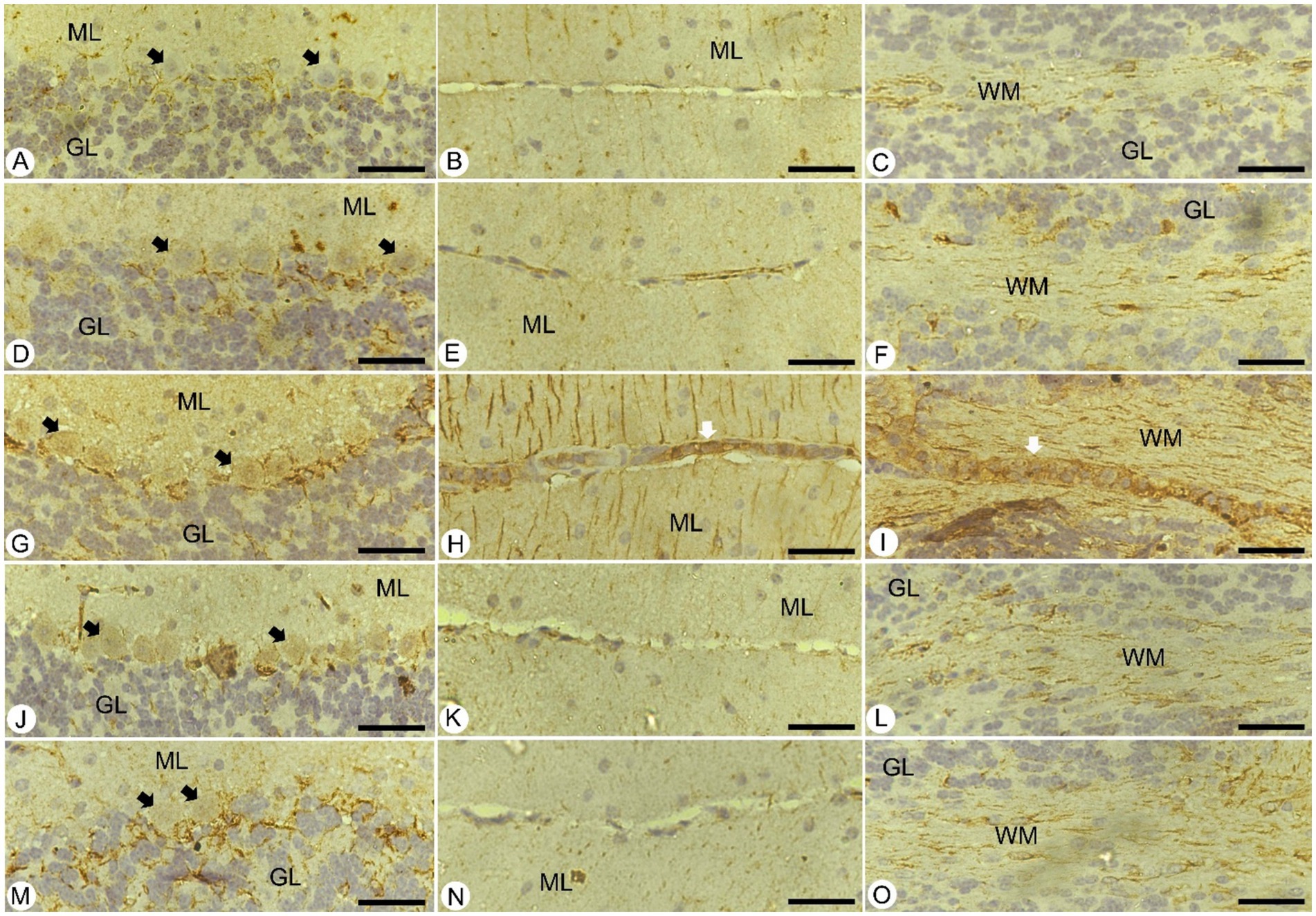
Figure 7. Immunohistochemical localization of GFAP in the brain of mice. (A–C) Control group. (D–F) non-infected-treated group with 500 mg/kg of JRLE. (G–I) Infected group. (J–L) Infected-treated group with 500 mg/kg of JRLE. (M–O) Infected-treated group with chloroquine. ML, molecular layer; GL, granular layer; WM, white matter; Black arrows, Purkinje cell; White arrows, hemorrhage. Scale Bar = 100 μm.
A concerning aspect of the infection was the significant decrease in the levels of neurotransmitters like epinephrine (14.15 ± 2.74 ng/mg/protein), norepinephrine (28.93 ± 4.11 ng/mg/protein), dopamine (23.48 ± 2.50 ng/mg/protein), and serotonin (12.90 ± 2.23 ng/mg/protein) in the brains of the infected mice when compared to the control group (Figure 8). Upon treatment with JRLE, there was a notable increase in the neurotransmitter levels (72.95 ± 2.47, 115.43 ± 3.12, 61.82 ± 4.38, and 54.05 ± 1.38 ng/mg/protein, respectively) in the brain of infected mice compared to those that were merely infected (Figure 8).
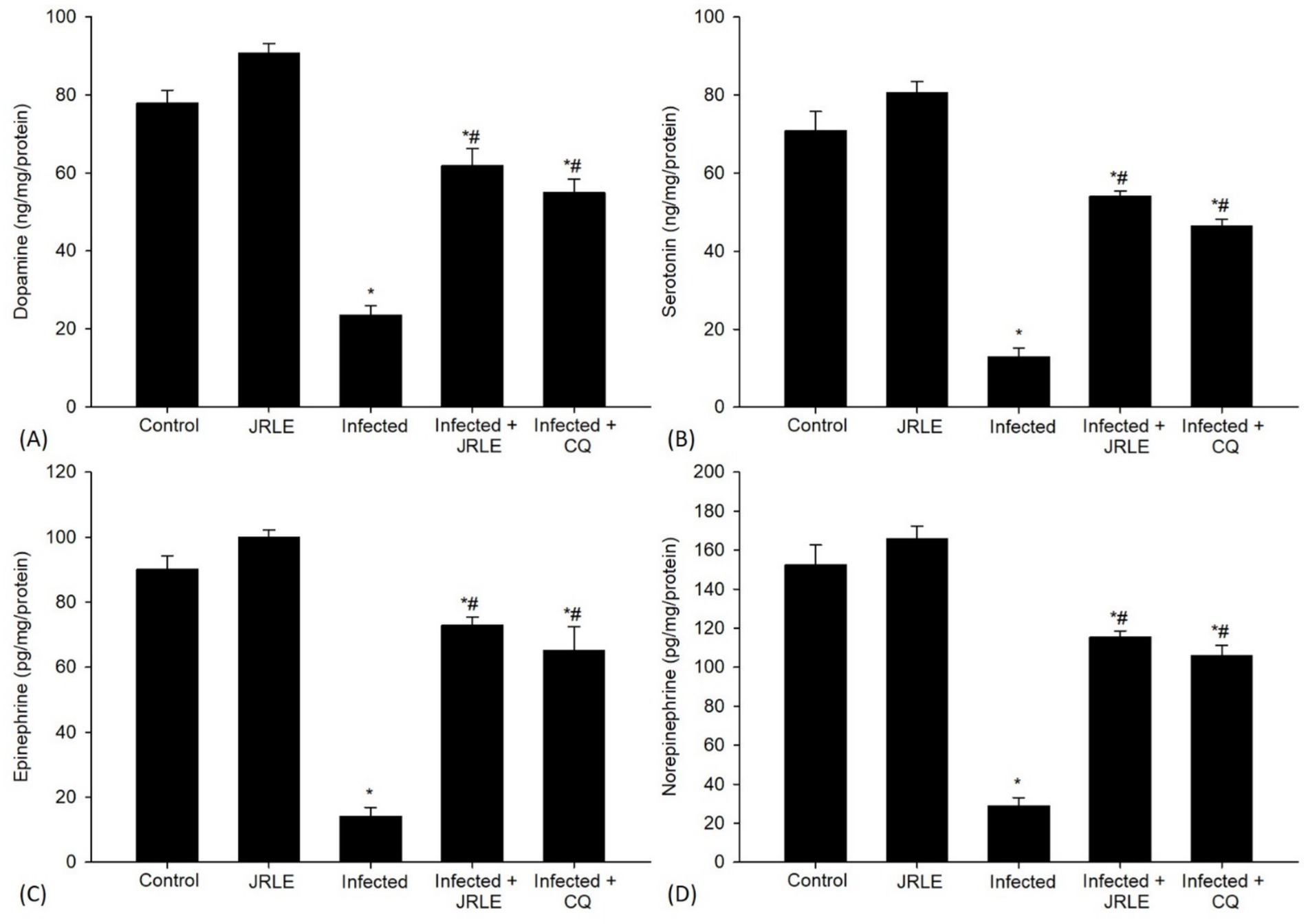
Figure 8. Neurotransmitters in the brain samples from different experimental mice groups in the 9th day p.i. (A) Dopamine. (B) Serotonin. (C) Epinephrine. (D) Norepinephrine. *Significant change concerning the control group, #Significance change concerning the infected group.
Quantitative RT-PCR (qRT-PCR) was utilized to examine changes in the mRNA expression levels of neurotransmitter signaling genes in the mouse brain (Figures 9A–D). The P. berghei infection led to a significant upregulation of the Gabbr1 gene expression, approximately 3.63-fold higher than the control group (Figure 9A). Treatment with JRLE brought a significant downregulation of this gene by about 1.74-fold, closely resembling the reference drug (1.71-fold) (Figure 9A). Moreover, P. berghei infection resulted in a significant upregulation of the nicotinic acetylcholine receptor subunit Chrnb2 (Figure 9B), with a 4.33-fold increase compared to the control group (Figure 9B). JRLE treatment showed significant downregulation of 1.99-fold for this receptor compared to the reference drug’s 1.68-fold (Figure 9B). The study also documented upregulation of the Gnai1 gene after infection, with an elevation of about 3.99-fold compared to the control group (1.00-fold) (Figure 9C). Treatment with JRLE significantly downregulated this gene by about 1.87-fold in comparison to the reference drug (1.75-fold) (Figure 9C). The infection led to a significant downregulation of the Gria2 gene expression to about 0.13-fold, but there was significant improvement after JRLE treatment, recording an upregulation of 0.79-fold compared to the chloroquine group (0.91-fold) (Figure 9D).
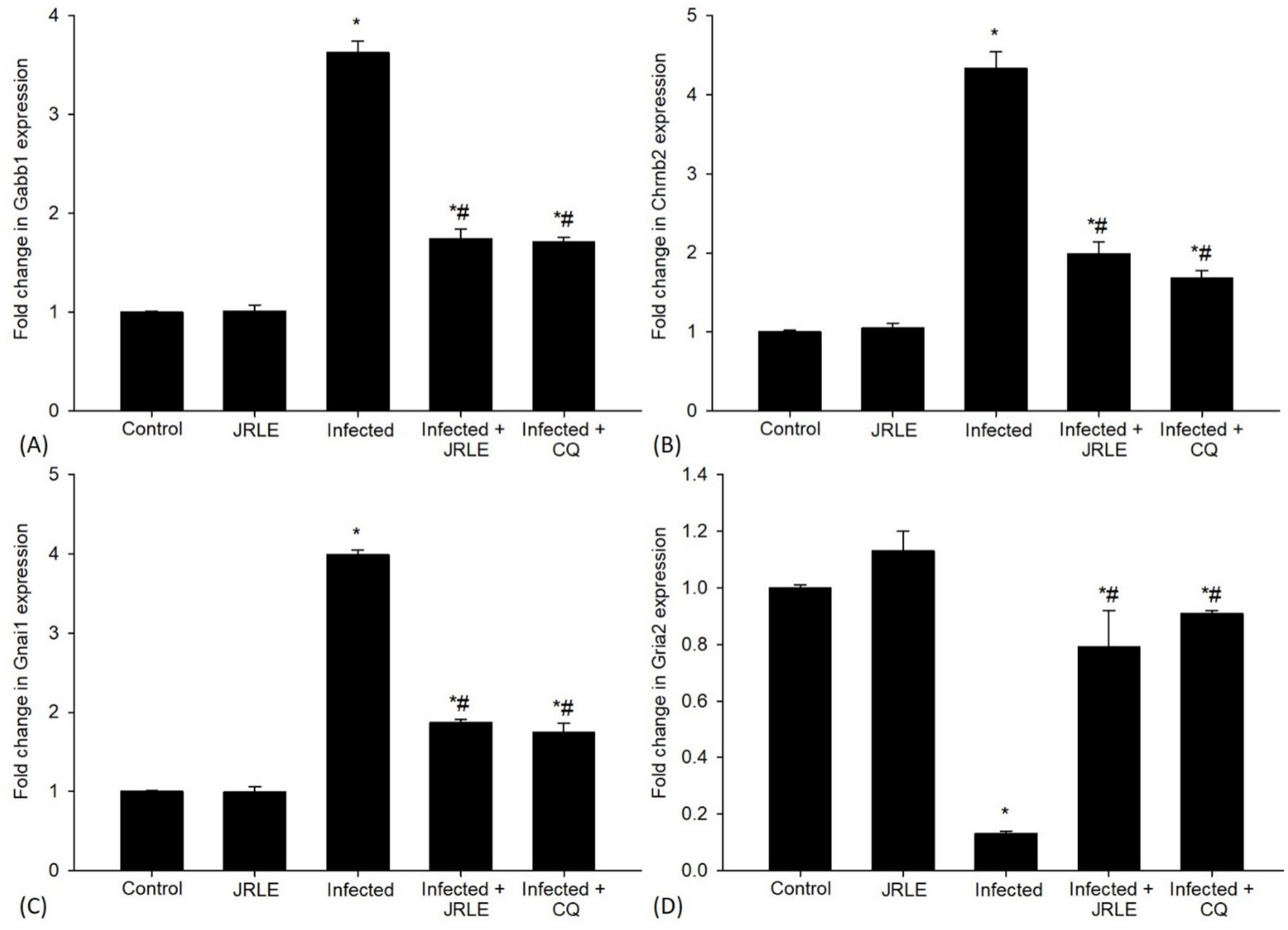
Figure 9. Effect of JRLE on the mRNA expression of some selected genes in the brain samples from different experimental mice groups in the 9th-day p.i. (A) Gabb1. (B) Chrnb2. (C) Gnai1. (D) Gria2. *Significant change concerning the control group, #Significance change concerning the infected group.
In our ongoing efforts to understand the effects of JRLE, we also investigated levels of GABA and glutamate using ELISA (Figure 10A). The results striking; compared to the control group, Plasmodium infection resulted in a significant decrease in GABA level (80.89 ± 4.26 ng/mg). Yet, it was uplifting to observe that JRLE treatment significantly elevated the P. berghei-induced decrease in GABA level (139.77 ± 2.70 ng/mg) compared to the infected group. Additionally, glutamate levels increased dramatically after Plasmodium infection (11.46 ± 0.67 ug/mg), correlating with behavioral changes when compared to the control group. The infected-treated group that received 500 mg/kg JRLE displayed a significant decrease in glutamate levels (6.60 ± 0.31 ug/mg) compared to the P. berghei-infected mice (Figure 10B).
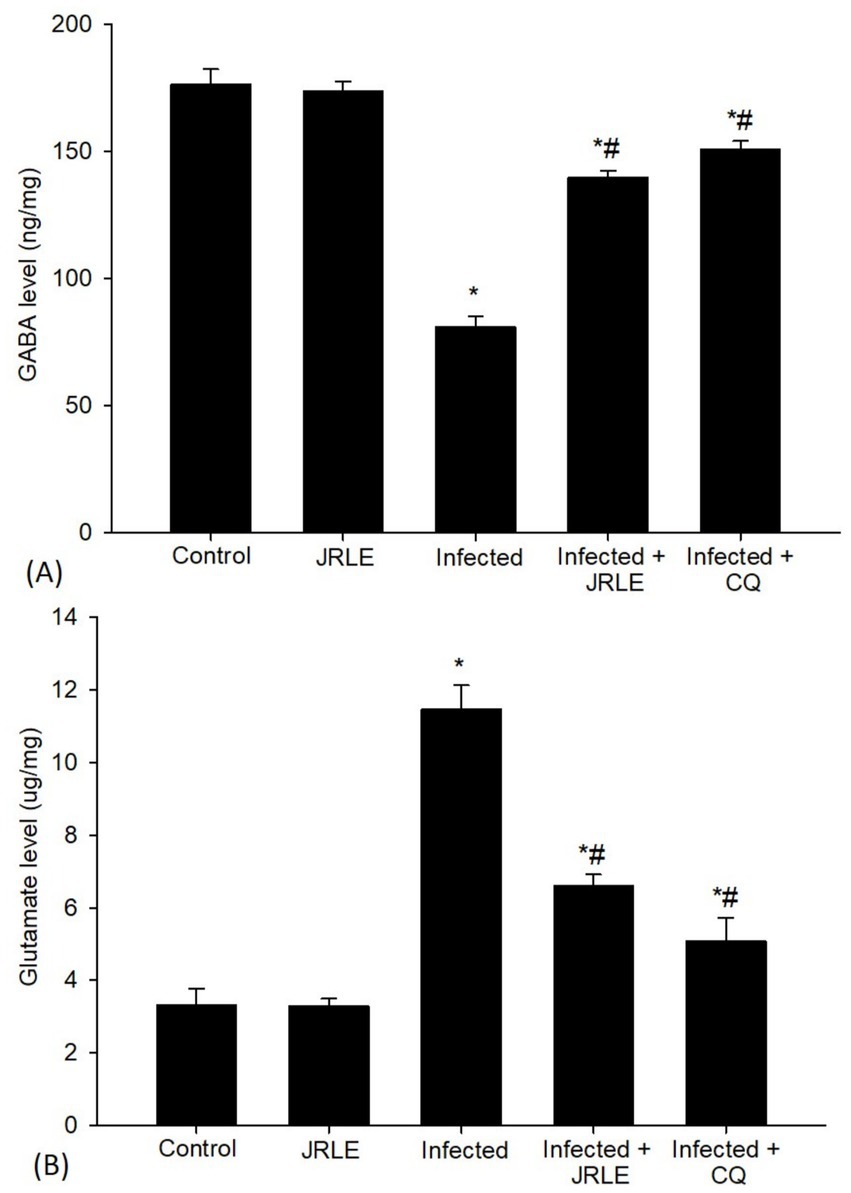
Figure 10. Levels of neurotransmitters of (A) GABA and (B) Glutamate in the brain samples from the different experimental mice groups. *Significant change (p ≤ 0.05) concerning the control group, #Significance change (p ≤ 0.05) concerning the infected group with Plasmodium berghei.
Discussion
Malaria, caused by an apicomplexan protozoan parasite of the genus Plasmodium, is a major global health problem, particularly in tropical and subtropical regions (5). The emergence of drug-resistant strains for Plasmodium underscores the urgent need for novel therapeutic strategies (50, 51). Developing new antimalarial compounds from different sources, especially traditional medicinal plants, offers a promising approach to addressing parasite resistance. Therefore, the present in vivo study evaluated the antimalarial activity of J. regia leaf extracts (JRLE) against P. berghei infection.
Infection of the mouse strain, C57BL/6, with P. berghei affords an experimental model of cerebral malaria that shares some aspects with human cerebral malaria (52). In this study, the course of infection in the infected mice reached a peak level of infection (23.84% ± 2.06%) on day 9. This result agreed with Rajan et al. (53) and Badejo et al. (54) reported the peak parasitemia achieved on day 9 during P. berghei infection. This study showed that JRLE has a dose-dependent in vivo antimalarial effect against the Plasmodium parasite. When JRLE (500 mg/kg) was administrated, the parasitemia was significantly reduced to 6.33 ± 1.18%. These results might be due to the bioactive compounds of JRLE (i.e., alkaloids, flavonoids, phenolic compounds, and terpenoids) which might be implicated in antiplasmodial activity. Flavonoids inhibit the biosynthesis of fatty acids in the parasite metabolism which is required for the development of Plasmodium parasites during the erythrocytic phase, this is agreed with data obtained by Zhao et al. (55). Moreover, tannins in JRLE may play a role in inhibiting the Plasmodium protease enzyme, so that its growth and development are inhibited and prevent the invasion of new erythrocytes, consistent with Kaushik et al. (56). Similarly, Serakta et al. (57) reported the antiparasitic activity of the J. regia hydroalcoholic extract that significantly inhibits the growth of the promastigotes of leishmania major.
In this study, P. berghei-infected mice died showing typical signs of cerebral malaria (i.e., hemiplegia, ataxia, epilepsy, and blindness), which agreed with the previous studies by de Souza and Riley (58). The JRLE affected the blood and tissue stages of P. berghei significantly. The JRLE also prolonged the survival time of the treated mice which is an indication of significant antimalarial activity associated with the presence of some bioactive secondary metabolites in the plant leaf extracts. Flavonoids in JRLE could counter the redox imbalance associated with Plasmodium infection, increasing the mean survival time of the infected treated mice. As previously reported, plant’s bioactive principles possess antiparasitic activity (59).
After infection, the weight loss exhibited by animals following P. berghei infection was correlated with high parasitemia due to the disturbances in the mice’s metabolism and the loss of mice’s appetite that might have resulted from parasite proliferation, which agreed with previous studies (44, 60–65). Treatment with JRLE induced the body weight of the infected mice. This might be due to the presence of bioactive components (i.e., sterols in the plant extract) which could enhance nutrient absorption and aid in animal digestion, this is agreed with Thakur and Singh (66) and Rock et al. (67).
In the brains of the infected mice, there were some histopathological alterations in the cerebellum including degeneration of Purkinje cells, hemorrhagic damage, infiltration of lymphocytes, and intravascular sequestrations of pRBCs which is consistent with the wide-ranging patterns of reported neurocognitive sequelae after cerebral malaria infection. These results agreed with previous studies by Bopp et al. (68), Dkhil et al. (44), Al-Shaebi et al. (39), Mohanty et al. (69), and Coughlan et al. (70). According to Maung and Than (71), during P. berghei infection, extravasation of RBCs is not only due to the rupture of blood vessels but also an increase in vascular permeability which results in the release of RBCs, both uninfected RBCs and infected with trophozoite. After the Plasmodium infection, a significant elevation of the brain index was observed in the infected group. This may be due to the presence of brain edema during cerebral malaria (72). Upon treatment, the JRLE not only inhibited the growth of the Plasmodium parasite but also caused a decrease in the overall pathologic effect of the parasite in the mice through the improvement in the brain tissue of the infected mice. This data is consistent with Aydın et al. (73), Ansari et al. (74), Rusu et al. (75), and Sharma et al. (76). Moreover, Edenharder and Grünhage (77) and Salter et al. (78) reported that flavonoids in J. regia could protect cells by acting as free radical scavengers, inhibiting DNA damage and mutagenicity.
GFAP is a brain-specific protein that functions as the major integral component of the cytoskeleton of astrocytes (79). Although few gross histopathological changes were observed, a significant increase in overall GFAP staining was observed around the Purkinje cell layer and in both the cerebellar molecular and white layers, indicating the dysfunction of astrocytes after Plasmodium infection. This is agreed with Missler et al. (80) and Herbas et al. (81) reported that GFAP was significantly increased after the infection in C57BL/6 mice with P. berghei ANKA. According to Carter et al. (82), astrocytes are crucial in maintaining the blood–brain barrier and the neurovascular unit. Their dysfunction could release GFAP, explaining its elevations using immunohistochemistry. These results agreed with previous studies by Chen and Swanson (83), and Sofroniew (84) proposed that GFAP expression is a cardinal feature of many pathological conditions of the central nervous system (CNS) and astrocytes. Treatment with JRLE induced protection of the mice’s brain from cerebral injury by inhibiting GFAP expression. Similarly, to our study, they investigated a strong neuroprotective effect of JRLE against cognitive deficits (74, 76).
Several biomarkers associated with cerebral injury were examined in the P. berghei-infected mice. Neurotransmitters are chemicals found in nerve cells that facilitate communication between cells at synapses, playing a critical role in signaling regulation (85). Our results indicated that the brains of the infected mice exhibited reduced contents of neurotransmitters, specifically epinephrine, norepinephrine, dopamine, and serotonin. This finding is consistent with Zai et al. (86), who suggested that the GABAB receptor plays a physiological role in modulating GABA release through an autoreceptor, as well as influencing the release of other neurotransmitters such as noradrenaline (87) and serotonin (88), cholecystokinin (89), and Somatostatin (90). The decrease in neurotransmitter levels may contribute to elevated body temperature, increasing malaria risk (91). Oyewole et al. (92) reported an increase in serotonin levels in the brains of infected mice following the lysis of platelet cells. Generally, some parasitic infections, such as Toxoplasma gondii (93), Schistosoma mansoni (94), Toxocara canis and Trichinella spiralis (95), lead to alterations in neurotransmitter levels. In our study, oral treatment with JRLE significantly increased neurotransmitter levels. This may be attributed to the role of juglone, as stated by Thakur (96) and Cintesun et al. (97).
Regarding gene expression, cerebral malaria is fundamentally a neurological disease, as evidenced by substantial changes in gene expression in the brain (98). Several gene expressions recorded in this study included Chrnb2, Gabbr1, Gnai1, and Gria2, all showing significant alterations compared to those in the control mice. This aligns with findings from Desruisseaux et al. (98) and Mubaraki et al. (99), who reported changes in many encoding proteins in mice infected with P. berghei ANKA that exhibited neuropathology. The Chrnb2 gene encodes a protein that forms a subunit of a larger protein known as a neuronal nicotinic acetylcholine receptor (nAChR), which is part of a superfamily of ligand-gated ion channels and is crucial for fast transmission at synapses (100). Our findings demonstrated high expression levels of the Chrnb2 gene associated with damaged Purkinje cells in the P. berghei-infected mice group compared to the control group. Additionally, the Gria2 gene, which is part of the family of glutamate receptors sensitive to alpha-amino-3-hydroxy-5-methyl-4-isoxazole propionate (AMPA), functions as ligand-activated cation channels (101). However, Gria2 expression was lower in P. berghei-infected mice compared to the control group. Given the physiologic significance of the GABAB receptor in neurobiology, Gabbr1 is considered an attractive candidate gene for neuro-behavioral disorders (86). The Gabbr1 gene was found to be significantly over-expressed in the infected group that had been induced by cerebral malaria. According to Priya et al. (102), there is a close relationship between neuronal activity and energy metabolism. In this study, the G-proteins inhibitory α subunits represented by Gnai1 were overexpressed at 9 days post-infection (p.i.) in the brain of infected mice. Narzi et al. (103) noted that Gnai1 is associated with an inhibition mechanism of ATP-bound adenylyl cyclase type 5. The upregulation of Gnai1 was linked to decreased relapse-free survival, consistent with Li et al. (104). Generally, JRLE treatment significantly reduced the expression of Chrnb2, Gabbr1, and Gnai1 genes in the infected mice except for the elevation of Gria2. This finding highlights the ability of JRLE to relieve the burden of malaria pathogenesis suggesting that this plant extract may have neuroprotective properties as noted by Ansari et al. (74), Liu et al. (105), Sharma et al. (76), and Yeh et al. (106).
Our results indicated that the brains of the infected mice had lower levels of GABA. This reduction may be due to the Plasmodium parasite, which affects the action of GABA by inhibiting the permeability of nerve cell membranes to chloride ions (Cl−) from the extracellular environment. This process leads to membrane depolarization and results in physiological disruption. This data supports the findings of Kantrowitz et al. (107) and Heuer and Grosell (108), which indicated that dysfunction in the GABAergic system and the resulting reduction in GABA’s inhibitory effects can lead to various pathological processes and the development of diseases. After treatment, the active constituents of the plant extract play a significant role in mitigating GABA’s effects and restoring its function in neurons, which agrees with Ansari et al. (74) and Adarmanabadi et al. (109). Furthermore, glutamate is a major neurotransmitter, and brain neurons have a unique glutamine metabolism (110). The amount of glutamate in the brain of the infected mice was significantly increased at 9 p.i. This is consistent with Parekh et al. (111) and Miranda et al. (112) reported an increase of glutamine levels in the metabolite pool from brain extracts of P. berghei-infected mice associated with neurological and behavioral changes. These studies suggest that an imbalance in glutamate/glutamine metabolism may be relevant to cerebral malaria pathogenesis. Upon treatment, JRLE ameliorates the level of glutamine-glutamate relationship by enhancing the activation of glutamine transporter function in astrocytes. This finding highlights the neuroprotective properties of JRLE as noted by Sharma et al. (76), Liu et al. (105), and Yeh et al. (106).
Conclusion
This study demonstrated that P. berghei infection leads to chronic infection and brain damage in the C57BL/6 strain of mice. Treatment with Juglans regia leaf extracts (JRLE) provided significant protection against brain injury and influenced the disease outcome positively. However, the study primarily focused on the brain, and the impact of JRLE on other organs remains to be explored. Future research should investigate the broader organ-specific effects of JRLE, as well as the molecular mechanisms underlying the parasite–host interaction. Additionally, studies evaluating the long-term safety and efficacy of JRLE in different Plasmodium models would be crucial for assessing its therapeutic potential in malaria treatment.
Data availability statement
The raw data supporting the conclusions of this article will be made available by the authors, without undue reservation.
Ethics statement
The animal study was approved by the Research Ethics Committee (REC) at King Saud University (approval number KSU-SE-24-74). The study was conducted in accordance with the local legislation and institutional requirements.
Author contributions
AA: Conceptualization, Data curation, Formal analysis, Funding acquisition, Investigation, Methodology, Project administration, Resources, Software, Supervision, Validation, Visualization, Writing – original draft, Writing – review & editing. SA: Conceptualization, Data curation, Formal analysis, Funding acquisition, Investigation, Methodology, Project administration, Resources, Software, Supervision, Validation, Visualization, Writing – original draft, Writing – review & editing. EA-S: Conceptualization, Data curation, Formal analysis, Funding acquisition, Investigation, Methodology, Project administration, Resources, Software, Supervision, Validation, Visualization, Writing – original draft, Writing – review & editing. SQ: Conceptualization, Data curation, Formal analysis, Funding acquisition, Investigation, Methodology, Project administration, Resources, Software, Supervision, Validation, Visualization, Writing – original draft, Writing – review & editing. RA-G: Conceptualization, Data curation, Formal analysis, Funding acquisition, Investigation, Methodology, Project administration, Resources, Software, Supervision, Validation, Visualization, Writing – original draft, Writing – review & editing.
Funding
The author(s) declare that financial support was received for the research and/or publication of this article. This study was supported by the Researchers Supporting Project (RSP2024R25), King Saud University, Riyadh, Saudi Arabia.
Conflict of interest
The authors declare that the research was conducted in the absence of any commercial or financial relationships that could be construed as a potential conflict of interest.
Generative AI statement
The authors declare that no Gen AI was used in the creation of this manuscript.
Publisher’s note
All claims expressed in this article are solely those of the authors and do not necessarily represent those of their affiliated organizations, or those of the publisher, the editors and the reviewers. Any product that may be evaluated in this article, or claim that may be made by its manufacturer, is not guaranteed or endorsed by the publisher.
References
1. Mehlhorn, H. Encyclopedic reference of parasitology. 4th ed. Berlin, Heidelberg: Springer-Verlag (2014).
2. Cox-Singh, J, Davis, TM, Lee, KS, Shamsul, SS, Matusop, A, Ratnam, S, et al. Plasmodium knowlesi malaria in humans is widely distributed and potentially life threatening. Clin Infect Dis. (2008) 46:165–71. doi: 10.1086/524888
3. Marteau, A, Ouedraogo, E, Van der Meersch, G, Akhoundi, M, Souhail, B, Cohen, Y, et al. Severe long-delayed malaria caused by plasmodium malariae in an elderly French patient. Malar J. (2021) 20:337. doi: 10.1186/s12936-021-03870-4
4. Huang, BW, Pearman, E, and Kim, CC. Mouse models of uncomplicated and fatal malaria. Bio Protoc. (2015) 5:e1514. doi: 10.21769/BioProtoc.1514
5. Talapko, J, Škrlec, I, Alebić, T, Jukić, M, and Včev, A. Malaria: the past and the present. Microorganisms. (2019) 7:179. doi: 10.3390/microorganisms7060179
6. World Health Organization (WHO). Available online at: https://www.who.int/teams/global-malaria-programme/reports/world-malaria-report-2022. (2022) (Accessed October 25, 2024).
7. Elagali, A, Shubayr, M, Noureldin, E, Alene, KA, and Elagali, A. Spatiotemporal distribution of malaria in the Kingdom of Saudi Arabia. Trop Med Infect Dis. (2024) 9:16. doi: 10.3390/tropicalmed9010016
8. Lima, MN, Oliveira, HA, Fagundes, PM, Estato, V, Silva, AYO, Freitas, RJRX, et al. Mesenchymal stromal cells protect against vascular damage and depression-like behavior in mice surviving cerebral malaria. Stem Cell Res Ther. (2020) 11:367. doi: 10.1186/s13287-020-01874-6
9. Riggle, BA, Manglani, M, Maric, D, Johnson, KR, Lee, MH, Neto, OLA, et al. CD8+ T cells target cerebrovasculature in children with cerebral malaria. J Clin Invest. (2020) 130:1128–38. doi: 10.1172/JCI133474
10. Idro, R, Jenkins, NE, and Newton, CRJC. Pathogenesis, clinical features, and neurological outcome of cerebral malaria. Lancet Neurol. (2005) 4:827–40. doi: 10.1016/S1474-4422(05)70247-7
11. Martins, YC, Freeman, BD, Akide Ndunge, OB, Weiss, LM, Tanowitz, HB, and Desruisseaux, MS. Endothelin-1 treatment induces an experimental cerebral malaria-like syndrome in C57BL/6 mice infected with plasmodium berghei NK65. Am J Pathol. (2016) 186:2957–69. doi: 10.1016/j.ajpath.2016.07.020
12. Capela, R, Moreira, R, and Lopes, F. An overview of drug resistance in protozoal diseases. Int J Mol Sci. (2019) 20:5748. doi: 10.3390/ijms20225748
13. Baird, JK. Effectiveness of antimalarial drugs. N Engl J Med. (2005) 352:1565–77. doi: 10.1056/nejmra043207
14. Bruce-Chwatt, LJ. Classification of anti-malarial drugs in relation to different stages in the life cycle of the parasite: commentary on a diagram. Bull World Health Organ. (1962) 27:287–90.
15. Moelyadi, F, Utami, PD, and Dikman, IM. Inhibitory effect of active substances of Lollyfish (Holothuria atra) against the development of plasmodium falciparum based on in silico study. Indones J Marine Sci. (2020) 25:135–42. doi: 10.14710/ik.ijms.25.4.135-142
16. Atanu, FO, Idih, FM, Nwonuma, CO, Hetta, HF, Alamery, S, and El-Saber Batiha, G. Evaluation of antimalarial potential of extracts from Alstonia boonei and Carica papaya in plasmodium berghei-infected mice. Evid Based Complement Alternat Med. (2021) 2021:2599191. doi: 10.1155/2021/2599191
17. Dkhil, MA, Lubbad, MY, Al-Shaebi, EM, Delic, D, and Al-Quraishy, S. The antiplasmodial and spleen protective role of crude Indigofera oblongifolia leaf extract traditionally used in the treatment of malaria in Saudi Arabia. Drug Des Devel Ther. (2015) 9:6235–46. doi: 10.2147/DDDT.S94673
18. Al-Shaebi, EM, Taib, NT, Mubaraki, MA, Hafiz, TA, Lokman, MS, Al-Ghamdy, AO, et al. Indigofera oblongifolia leaf extract regulates spleen macrophage response during plasmodium chabaudi infection. Saudi J Biol Sci. (2017) 24:1663–6. doi: 10.1016/j.sjbs.2017.06.006
19. Aljawdah, HMA, Abdel-Gaber, R, Al-Shaebi, EM, Thagfan, FA, Al-Quraishy, S, Qasem, MAA, et al. Hepatoprotective activity of Eucalyptus camaldulensis extract in murine malaria mediated by suppression of oxidative and inflammatory processes. Front Cell Infect Microbiol. (2022) 12:955042. doi: 10.3389/fcimb.2022.955042
20. Dkhil, MA, Aljawdah, HMA, Abdel-Gaber, R, Thagfan, FA, Delic, D, and Al-Quraishy, S. The effect of Eucalyptus camaldulensis leaf extracts from different environmental harvesting locations on plasmodium chabaudi-induced malaria outcome. Food Sci Technol. (2023) 43:e006723. doi: 10.1590/fst.006723
21. Olowokudejo, JD, Kadiri, AB, and Travih, VA. An ethnobotanical survey of herbal markets and medicinal plants in Lagos state of Nigeria. Ethnobotanical Leaflets. (2008) 1:116.
22. Enechi, OC, Amah, CC, Okagu, IU, Ononiwu, CP, Azidiegwu, VC, Ugwuoke, EO, et al. Methanol extracts of Fagara zanthoxyloides leaves possess antimalarial effects and normalizes haematological and biochemical status of plasmodium berghei-passaged mice. Pharm Biol. (2019) 57:577–85. doi: 10.1080/13880209.2019.1656753
23. Elouafy, Y, El Yadini, A, El Moudden, H, Harhar, H, Alshahrani, MM, Awadh, AAA, et al. Influence of the extraction method on the quality and chemical composition of walnut (Juglans regia L.) oil. Molecules. (2022) 27:7681. doi: 10.3390/molecules27227681
24. Kafkas, E, Burgut, A, Ozcan, H, Ozcan, A, Sutyemez, M, Kafkas, S, et al. Fatty acid, total phenol and tocopherol profiles of some walnut cultivars: a comparative study. Food Nutr Sci. (2017) 8:1074–84. doi: 10.4236/fns.2017.812079
25. Simsek, M, Gulsoy, E, Beyhan, O, Osmanoglu, A, and Turgut, Y. Determination of some botanical, phenological, physical and chemical characteristics of walnut (Juglans regia L.) genotypes grown in Turkey. Appl Ecol Environ Res. (2017) 15:1279–91. doi: 10.15666/aeer/1503_12791291
26. Fukuda, T, Ito, H, and Yoshida, T. Antioxidative polyphenols from walnuts (Juglans regia L.). Phytochemistry. (2003) 63:795–801. doi: 10.1016/s0031-9422(03)00333-9
27. Bujdosó, G, Végvári, G, Hajnal, V, Ficzek, G, and Tóth, M. Phenolic profile of the kernel of selected Persian walnut (Juglans regia L.) cultivars. Not Bot Horti Agrobo. (2014) 42:24–9. doi: 10.15835/nbha4219400
28. Aja, PM, Ugwu Okechukwu, PC, Nwobasi, CS, Alum, EU, and Ekpono, EU. Phytochemical and anti-nutrient compositions of Juglas regia seeds. J Appl Sci. (2017) 2:92–113.
29. Dabburu, K, Kondaveeti, SB, and Babu, SK. Evaluation of gastro-protective effect of the hydro-alcoholic extract of Juglans regia L leaves in experimental animals. J Appl Pharmaceut Sci. (2012) 2:79–83. doi: 10.7324/JAPS.2012.21114
30. Zakavi, F, Hagh, LG, Daraeighadikolaei, A, Sheikh, AF, Daraeighadikolaei, A, and Shooshtari, ZL. Antibacterial effect of Juglans regia bark against oral pathologic bacteria. Int J Dentistry. (2013) 2013:854765:1. doi: 10.1155/2013/854765
31. Soto-Maldonado, C, Vergara-Castro, M, Jara-Quezada, J, Caballero-Valdés, E, Müller-Pavez, A, Zúñiga-Hasen, ME, et al. Polyphenolic extracts of walnut (Juglans regia) green husk containing juglone inhibit the growth of HL-60 cells and induce apoptosis. Electron J Biotechnol. (2019) 39:1–7. doi: 10.1016/j.ejbt.2019.02.001
32. Igbayilola, YD, Aina, OS, Ogunkoya, OO, Williams, OD, and Olaoye, FA. Oxidative, hepatoprotective and ant-inflammatory responses to perinatal walnut (Juglans regia L.) supplemented diet in offspring of Sprague-Dawley rats. Int J Biochem Physiol. (2022) 7:1–10. doi: 10.23880/IJBP-16000205
33. Elouafy, Y, El Yadini, A, Mortada, S, Hnini, M, Harhar, H, Khalid, A, et al. Antioxidant, antimicrobial, and α- glucosidase inhibitory activities of saponin extracts from walnut (Juglans regia L.) leaves. Asian Pac J Trop Biomed. (2023) 13:60–9. doi: 10.4103/2221-1691.369610
34. Elouafy, Y, Mortada, S, El Yadini, A, Hnini, M, Aalilou, Y, Harhar, H, et al. Bioactivity of walnut: investigating the triterpenoid saponin extracts of Juglans regia kernels for antioxidant, anti-diabetic, and antimicrobial properties. Progr Microbes Mol Biol. (2023) 6:a0000325. doi: 10.36877/pmmb.a0000325
35. Ara, T, Shafi, S, Ghazwani, M, Mir, JI, Shah, AH, Qadri, RA, et al. In vitro potent anticancer, antifungal, and antioxidant efficacy of walnut (Juglans regia L.) genotypes. Agronomy. (2023) 13:1232. doi: 10.3390/agronomy13051232
36. Manikandan, P, Letchoumy, PV, Gopalakrishnan, M, and Nagini, S. Evaluation of Azadirachta indica leaf fractions for in vitro antioxidant potential and in vivo modulation of biomarkers of chemoprevention in the hamster buccal pouch carcinogenesis model. Food Chem Toxicol. (2008) 46:2332–43. doi: 10.1016/j.fct.2008.03.013
37. Negrea, P, Caunii, A, Sarac, I, and Butnariu, M. The study of infrared spectrum of chitin and chitosan extract as potential sources of biomass. Dig J Nanomater Biostruct. (2015) 10:1129–38.
38. Hilou, A, Nacoulma, OG, and Guiguemde, TR. In vivo antimalarial activities of extracts from Amaranthus spinosus L. and Boerhaavia erecta L. in mice. J Ethnopharmacol. (2006) 103:236–40. doi: 10.1016/j.jep.2005.08.006
39. Al-Shaebi, EM, Mohamed, WF, Al-Quraishy, S, and Dkhil, MA. Susceptibility of mice strains to oxidative stress and neurotransmitter activity induced by plasmodium berghei. Saudi J Biol Sci. (2018) 25:167–70. doi: 10.1016/j.sjbs.2017.01.057
40. David, AF, Philip, JR, Simon, RC, Reto, B, and Solomon, N. Antimalarial drug discovery: efficacy models for compound screening. Nat Rev. (2004) 3:509–20. doi: 10.1038/nrd1416
41. Timms, R, Colegrave, N, Chan, B, and Read, A. The effect of parasite dose on disease severity in the rodent malaria plasmodium chabaudi. Parasitology. (2001) 123:1–11. doi: 10.1017/S0031182001008083
42. Sharif, M, Anjum, I, Shabbir, A, Khurram Syed, S, Mobeen, I, Hassaan Shahid, M, et al. Amelioration of ovalbumin-induced allergic asthma by Juglans regia via downregulation of inflammatory cytokines and upregulation of aquaporin-1 and aquaporin-5 in mice. J Trop Med. (2022) 2022:6530095. doi: 10.1155/2022/6530095
43. Abay, SM, Lucantoni, L, Dahiya, N, Dori, G, Dembo, EG, Esposito, F, et al. Plasmodium transmission blocking activities of Vernonia amygdalina extracts and isolated compounds. Malar J. (2015) 14:1–19. doi: 10.1186/s12936-015-0812-2
44. Dkhil, MA, Al-Shaebi, EM, Lubbad, MY, and Al-Quraishy, S. Impact of sex differences in brain response to infection with plasmodium berghei. Parasitol Res. (2016) 115:415–22. doi: 10.1007/s00436-015-4803-6
45. Ezike, AC, Okonkwo, CH, Akah, PA, Okoye, TC, Nworu, CS, Mbaoji, FN, et al. Landolphia owariensis leaf extracts reduce parasitemia in plasmodium berghei-infected mice. Pharm Biol. (2016) 54:2017–25. doi: 10.3109/13880209.2016.1138970
46. Ogundolie, OO, Dada, EO, Osho, IB, and Oloruntol, DA. Effect of raw ethanolic seed extract of Tetracarpidium conophorum on heamatological parameters in swiss albino mice infected with P. Berghei. J Appl Life Sci Int. (2017) 12:1103–234. doi: 10.9734/JALSI/2017/33244
47. Varma, AV, Gupta, G, Gupta, J, and Gupta, S. GFAP expression in neuroglial tumours-immunohistochemical confirmation for diagnosis and grading. J Evolut Med Dent Sci. (2018) 7:5034–8. doi: 10.14260/jemds/2018/1120
48. Ciarlone, AE. Modification of a spectrophotofluorometric method of analyzing serotonin, norepinephrine, and dopamine in one brain sample. Microchem J. (1976) 21:349–54. doi: 10.1016/0026-265X(76)90054-0
49. Livak, KJ, and Schmittgen, TD. Analysis of relative gene expression data using real-time quantitative PCR and the 2(-Delta Delta C(T)) method. Methods. (2001) 25:402–8. doi: 10.1006/meth.2001.1262
50. Korenromp, EL, Williams, BG, Gouws, E, Dye, C, and Snow, RW. Measurement of trends in childhood malaria mortality in Africa: an assessment of progress toward targets based on verbal autopsy. Lancet Infect Dis. (2003) 3:349–58. doi: 10.1016/S1473-3099(03)00657-1
51. Petersen, I, Eastman, R, and Lanzer, M. Drug-resistant malaria: molecular mechanisms and implications for public health. FEBS Lett. (2011) 585:1551–62. doi: 10.1016/j.febslet.2011.04.042
52. Schofield, L, and Grau, GE. Immunological processes in malaria pathogenesis. Nat Rev Immunol. (2005) 5:722–35. doi: 10.1038/nri1686
53. Rajan, S, Baek, K, and Yoon, HS. C-h O hydrogen bonds in FK506-binding protein-ligand interactions. J Mol Recognit. (2013) 26:550–5. doi: 10.1002/jmr.2299
54. Badejo, JA, Abiodun, OO, Akinola, O, Happi, CT, Sowunmi, A, and Gbotosho, GO. Interaction between rifampicin, amodiaquine and artemether in mice infected with chloroquine resistant plasmodium berghei. Malar J. (2014) 13:299. doi: 10.1186/1475-2875-13-299
55. Zhao, MH, Jiang, ZT, and Li, R. Flavonoids in Jugalns regia L. leaves and evaluation of in vitro antioxidant activity via intracellular and chemical methods. Sci World J. (2014) 2014:303878. doi: 10.1155/2014/303878
56. Kaushik, NK, Bagavan, A, Rahuman, AA, Zahir, AA, Kamaraj, C, Elango, G, et al. Evaluation of antiplasmodial activity of medicinal plants from north Indian Buchpora and south Indian eastern Ghats. Malar J. (2015) 14:65. doi: 10.1186/s12936-015-0564-z
57. Serakta, M, Djerrou, Z, Mansour-Djaalab, H, Kahlouche-Riachi, F, Hamimed, S, Trifa, W, et al. Antileishmanial activity of some plants growing in Algeria: Juglans regis, Lawsonia inermis and Salvia officinalis. Afr J Tradit Complement Altern Med. (2013) 10:427–30. doi: 10.4314/ajtcam.v10i3.7
58. de Souza, JB, and Riley, EM. Cerebral malaria: the contribution of studies in animal models to our understanding of immunopathogenesis. Microbes Infect. (2002) 4:291–300. doi: 10.1016/S1286-4579(02)01541-1
59. Chegeni, AS, Ezatpour, B, Mohebali, M, Mahmoudvand, H, Zibaei, M, Ebrahimzadeh, F, et al. Effect of peel and leaf extract of walnut (Juglans regia L.) on cutaneous leishmaniasis caused by Leishmania major in BALB/c mice. J Chem Pharm Sci. (2016) 9:2490–5.
60. Karney, WW, and Tong, MJ. Malabsorption in plasmodium falciparum malaria. Am J Trop Med Hyg. (1972) 21:1–5. doi: 10.4269/ajtmh.1972.21.1
61. Eling, W, van Zon, A, and Jerusalem, C. The course of a plasmodium berghei infection in six different mouse strains. Z Parasitenkd. (1977) 54:29–45. doi: 10.1007/BF00380634
62. Wilairatana, P, Meddings, JB, Ho, M, Vannaphan, S, and Looareesuwan, S. Increased gastrointestinal permeability in patients with plasmodium falciparum malaria. Clin Infect Dis. (1997) 24:430–5. doi: 10.1093/clinids/24.3.430
63. Sowunmi, A, Balogun, T, Gbotosho, GO, Happi, CT, Adedeji, AA, and Fehintola, FA. Activities of amodiaquine, artesunate, and artesunate-amodiaquine against asexual- and sexual-stage parasites in falciparum malaria in children. Antimicrob Agents Chemother. (2007) 51:1694–9. doi: 10.1128/AAC.00077-07
64. Basir, R, Rahiman, SF, Hasballah, K, Chong, W, Talib, H, Yam, M, et al. Plasmodium berghei ANKA infection in ICR mice as a model of cerebral malaria. Iran J Parasitol. (2012) 7:62–74.
65. Lee, DH, Chu, KB, Kang, HJ, Lee, SH, and Quan, FS. Previous infection with plasmodium berghei confers resistance to toxoplasma gondii infection in mice. Korean J Parasitol. (2019) 57:93–9. doi: 10.3347/kjp.2019.57.2.93
66. Thakur, M, and Singh, K. Walnut (Juglans regia L.) a complete health and brain food. Asian J Bio Sci. (2013) 8:276–88.
67. Rock, CL, Flatt, SW, Barkai, HS, Pakiz, B, and Heath, DD. Walnut consumption in a weight reduction intervention: effects on body weight, biological measures, blood pressure and satiety. Nutr J. (2017) 16:76. doi: 10.1186/s12937-017-0304-z
68. Bopp, SER, Rodrigo, E, González-Páez, GE, Frazer, M, Barnes, SW, Valim, C, et al. Identification of the plasmodium berghei resistance locus 9 linked to survival on chromosome. Malar J. (2013) 9:316. doi: 10.1186/1475-2875-12-316
69. Mohanty, S, Sahu, PK, Pattnaik, R, Majhi, M, Maharana, S, Bage, J, et al. Evidence of brain alterations in noncerebral falciparum malaria. Clin Infect Dis. (2022) 75:11–8. doi: 10.1093/cid/ciab907
70. Coughlan, C, Jäger, HR, Brealey, D, Carletti, F, Hyare, H, Pattnaik, R, et al. Adult cerebral malaria: acute and subacute imaging findings, long-term clinical consequences. Clin Infect Dis. (2024) 78:457–60. doi: 10.1093/cid/ciad651
71. Maung, M, and Than, T. The pathology of human cerebral malaria. Amr J Trop Med Hyg. (1989) 43:30–7. doi: 10.4269/ajtmh.1990.43.30
72. Promeneur, D, Lunde, LK, Amiry-Moghaddam, M, and Agre, P. Protective role of brain water channel AQP4 in murine cerebral malaria. Proc Natl Acad Sci USA. (2013) 110:1035–40. doi: 10.1073/pnas.1220566110
73. Aydın, S, Gökçe, Z, and Yılmaz, Ö. The effects of Juglans regia L. (walnut) extract on certain biochemical parameters and in the prevention of tissue damage in brain, kidney, and liver in CCl4 applied Wistar rats. Turk J Biochem. (2015) 40:241–50doi: 10.1515/tjb-2015-0009
74. Ansari, JM, Eftekhar-Vaghefi, SH, Shahrokhi, N, Basiri, M, Pour, FM, and Asadi-Shekaari, M. Pre-treatment effects of walnut kernel (Juglans regia) on brain edema, neuronal death and neurological scores in male rat after traumatic brain injury. J Appl Pharmaceut Sci. (2016) 6:102–6. doi: 10.7324/JAPS.2016.601014
75. Rusu, ME, Georgiu, C, Pop, A, Mocan, A, Kiss, B, Vostinaru, O, et al. Antioxidant effects of walnut (Juglans regia L.) kernel and walnut septum extract in a D-galactose-induced aging model and in naturally aged rats. Antioxidants. (2020) 9:424. doi: 10.3390/antiox9050424
76. Sharma, P, Verma, PK, Sood, S, Pankaj, NK, Agarwal, S, and Raina, R. Neuroprotective potential of hydroethanolic hull extract of Juglans regia L. on isoprenaline induced oxidative damage in brain of Wistar rats. Toxicol Rep. (2021) 8:223–9. doi: 10.1016/j.toxrep.2021.01.006
77. Edenharder, R, and Grünhage, D. Free radical scavenging abilities of flavonoids as mechanism of protection against mutagenicity induced by tert-butyl hydroperoxide or cumene hydroperoxide in Salmonella typhimurium TA102. Mutat Res. (2003) 540:1–18. doi: 10.1016/s1383-5718(03)00114-1
78. Salter, L, Clifford, T, Morley, N, Gould, D, Campbell, S, and Curnow, A. The use of comet assay data with a simple reaction mechanism to evaluate the relative effectiveness of free radical scavenging by quercetin, epigallocatechin gallate, and N-acetylcysteine in UV-irradiated MRC5 lung fibroblasts. J Photochem Photo Biol B: Biol. (2004) 75:57–61. doi: 10.1016/j.jphotobiol.2004.05.007
79. Lei, J, Gao, G, Feng, J, Jin, Y, Wang, C, Mao, Q, et al. Glial fibrillary acidic protein as a biomarker in severe traumatic brain injury patients: a prospective cohort study. Crit Care. (2015) 19:362. doi: 10.1186/s13054-015-1081-8
80. Missler, U, Wiesmann, M, Wittmann, G, Magerkurth, O, and Hagenström, H. Measurement of glial fibrillary acidic protein in human blood: analytical method and preliminary clinical results. Clin Chem. (1999) 45:138–41.
81. Herbas, MS, Okazaki, M, Terao, E, Xuan, X, Arai, H, and Suzuki, H. α-Tocopherol transfer protein inhibition is effective in the prevention of cerebral malaria in mice. Am J Clin Nutr. (2010) 91:200–7. doi: 10.3945/ajcn.2009.28260
82. Carter, SF, Herholz, K, Rosa-Neto, P, Pellerin, L, Nordberg, A, and Zimmer, ER. Astrocyte biomarkers in Alzheimer’s disease. Trends Mol Med. (2019) 25:77–95. doi: 10.1016/j.molmed.2018.11.006
83. Chen, Y, and Swanson, RA. Astrocytes and brain injury. J Cereb Blood Flow Metab. (2003) 23:137–49. doi: 10.1097/01.WCB.0000044631.80210.3C
84. Sofroniew, MV. Reactive astrocytes in neural repair and protection. Neuroscientist. (2005) 5:400–7. doi: 10.1177/1073858405278321
85. Mele, T, Carman-Krzan, M, and Juric, DM. Regulation role of monoamine neurotransmitters in astrocytic NT-3 synthesis. Int J Dev Neurosci. (2010) 28:13–9. doi: 10.1016/j.ijdevneu.2009.10.003
86. Zai, G, King, N, Wong, HWH, Barr, CL, and Kennedy, JL. Possible association between the gamma-aminobutyric acid type B receptor 1 (GABBR1) gene and schizophrenia. Eur Neuropsychopharmacol. (2005) 15:347–52. doi: 10.1016/j.euroneuro.2004.12.006
87. Bowery, NG, Hill, DR, Hudson, AL, Doble, A, Middlemiss, DN, Shaw, J, et al. (−) baclofen decreases neurotransmitter release in the mammalian CNS by an action at a novel GABA receptor. Nature. (1980) 283:92–4. doi: 10.1038/283092a0
88. Gray, JA, and Green, AR. GABAB-receptor mediated inhibition of potassium-evoked release of endogenous 5-hydroxytryptamine from mouse frontal cortex. Br J Pharmacol. (1987) 91:517–22.
89. Conzelmann, U, Meyer, DK, and Sperk, G. Stimulation of receptors of γ-aminobutyric acid modulates the release of cholecystokinin-like immunoreactivity from slices of rat neostriatum. Br J Pharmac. (1986) 89:845–52. doi: 10.1111/j.1476-5381.1986.tb11190.x
90. Bonanno, G, Gemignani, A, Fedele, E, Fontana, G, and Raiteri, M. γ-Aminobutyric acidB receptors mediate inhibition of somatostatin release from cerebrocortex nerve terminals. J Pharmacol Exp Ther. (1991) 259:1153–7.
91. Dascombe, MJ, and Sidara, JY. The absence of fever in rat malaria is associated with increased turnover of 5-hydroxytryptamine in the brain. In: Temperature Regulation (1994). pp. 47–52.
92. Oyewole, AL, Akinola, O, and Owoyele, BV. Plasmodium berghei-induced malaria decreases pain sensitivity in mice. Onderstepoort J Vet Res. (2021) 88:a1871. doi: 10.4102/ojvr.v88i1.1871
93. Gatkowska, J, Wieczorek, M, Dziadek, B, Dzitko, K, and Dlugonska, H. Sex-dependent neurotransmitter level changes in brains of toxoplasma gondii infected mice. Exp Parasitol. (2013) 133:1–7. doi: 10.1016/j.exppara.2012.10.005
94. Bauomy, AA, Diab, MS, Abdel Moneim, AE, Dkhil, MA, and Al-Quraishy, S. Neuronal activities of berberine in Schistosoma mansoni-infected mice. Afr J Pharm Pharmacol. (2013) 7:368–74. doi: 10.5897/AJPP12.1179
95. Abdel Ghafar, AE, Elkowrany, SE, Salem, SA, Menaisy, AA, Fadel, WA, and Awara, WM. Effect of some parasitic infection on neurotransmitters in the brain of experimentally infected mice before and after treatment. J Egypt Soc Parasitol. (1996) 26:497–508.
96. Thakur, A. Juglone: a therapeutic phytochemical from Juglans regia L. J Medic Plants Res. (2011) 5:5324–30.
97. Cintesun, S, Ozman, Z, Kocyigit, A, Mansuroglu, B, and Kocacaliskan, I. Effects of walnut (Juglans regia L.) kernel extract and juglone on dopamine levels and oxidative stress in rats. Food Biosci. (2023) 51:102327. doi: 10.1016/j.fbio.2022.102327
98. Desruisseaux, MS, Iacobas, DA, Iacobas, S, Mukherjee, S, Weiss, LM, Tanowitz, HB, et al. Alterations in the brain transcriptome in plasmodium berghei ANKA infected mice. J Neuro-Oncol. (2010) 1:55–60. doi: 10.4303/jnp/N100803
99. Mubaraki, MA, Hafiz, TA, Al-Quraishy, S, and Dkhil, MA. Oxidative stress and genes regulation of cerebral malaria upon Zizyphus spina-christi treatment in a murine model. Microb Pathog. (2017) 107:69–74. doi: 10.1016/j.micpath.2017.03.017
100. Conti, DV, Lee, W, Li, D, Liu, J, Van Den Berg, D, Thoams, PD, et al. Nicotinic acetylcholine receptor β2 subunit gene implicated in a systems-based candidate gene study of smoking cessation. Hum Mol Genet. (2008) 17:2834–48. doi: 10.1093/hmg/ddn181
101. Latsko, MS, Koboldt, DC, Franklin, SJ, Hickey, S, Williamson, RK, Garner, S, et al. Do novo missense variant in GRIA2 in a patient with global developmental delay, autism spectrum disorder, and epileptic encephalopathy. Cold Spring Harb Mol Case Stud. (2022) 8:a006172. doi: 10.1101/mcs.a006172
102. Priya, A, Johar, K, Nair, B, and Wong-Riley, MTT. Nuclear respiratory factor 2 regulates the transcription of AMPA receptor subunit GLUA2 (Gria2). Biochim Biophys Acta. (2014) 1843:3018–28. doi: 10.1016/j.bbamcr.2014.09.006
103. Narzi, D, van Keulen, SC, and Röthlisberger, U. Gαi1 inhibition mechanism of ATP-bound adenylyl cyclase type 5. PLoS One. (2021) 16:e0245197. doi: 10.1371/journal.pone.0245197
104. Li, ZW, Sun, B, Gong, T, Guo, S, Zhang, J, Wang, J, et al. GNAI1 and GNAI3 reduce colitis-associated tumorigenesis in mice by blocking IL6 signaling and Down-regulating expression of GNAI2. Gastroenterology. (2019) 156:2297–312. doi: 10.1053/j.gastro.2019.02.040
105. Liu, M, Yang, S, Yang, J, Lee, Y, Kou, J, and Wang, C. Neuroprotective and memory-enhancing effects of antioxidant peptide from walnut (Juglans regia L.) protein hydrolysates. Nat Prod Commun. (2019) 14:1–9. doi: 10.1177/1934578X19865838
106. Yeh, AL, Chao, CL, Huang, WF, Lin, HC, and Wang, CJ. Walnut (Juglans regia L.) oligopeptide effects on enhancing memory, cognition and improving sleep quality in teenagers and elderly people in a randomized double-blind controlled trial. Nat Product Commun. (2022) 17:1–8. doi: 10.1177/1934578X221089065
107. Kantrowitz, J, Citrome, L, and Javitt, D. GABA(B) receptors, schizophrenia and sleep dysfunction: a review of the relationship and its potential clinical and therapeutic implications. CNS Drugs. (2009) 23:681–91. doi: 10.2165/00023210-200923080-00005
108. Heuer, RM, and Grosell, M. Physiological impacts of elevated carbon dioxide and ocean acidification on fish. Am J Physiol Regul Integr Comp Physiol. (2014) 307:R1061–84. doi: 10.1152/ajpregu.00064.2014
109. Adarmanabadi, SMHH, Gilavand, HK, Taherkhani, A, Rafiei, SK, Shahrokhi, M, Faaliat, S, et al. Pharamacotherapeutic potential of walnut (Juglans spp.) in age-related neurological disorders. IBRO Neurosci Rep. (2022) 14:1–20. doi: 10.1016/j.ibneur.2022.10.015
110. Erecińska, M, and Silver, IA. Metabolism and role of glutamate in mammalian brain. Prog Neurobiol. (1990) 35:245–96. doi: 10.1016/0301-0082(90)90013-7
111. Parekh, SB, Bubb, WA, Hunt, NH, and Rae, C. Brain metabolic markers reflect susceptibility status in cytokine gene knockout mice with murine cerebral malaria. Int J Parasitol. (2006) 36:1409–18. doi: 10.1016/j.ijpara.2006.07.004
Keywords: malaria, Plasmodium berghei, biological criteria, cerebral alterations, neurotransmitters
Citation: Alharbi A, Albasyouni S, Al-Shaebi E, Al Quraishy S and Abdel-Gaber R (2025) Neuroprotective and antimalarial effects of Juglans regia leaf extracts in a murine model of cerebral malaria. Front. Vet. Sci. 12:1537686. doi: 10.3389/fvets.2025.1537686
Edited by:
José Fernandes Vieira, Federal University of Pará, BrazilReviewed by:
Roberto Senas Cuesta, University of Arkansas, United StatesEbunoluwa Asenuga, University of Benin, Nigeria
Copyright © 2025 Alharbi, Albasyouni, Al-Shaebi, Al Quraishy and Abdel-Gaber. This is an open-access article distributed under the terms of the Creative Commons Attribution License (CC BY). The use, distribution or reproduction in other forums is permitted, provided the original author(s) and the copyright owner(s) are credited and that the original publication in this journal is cited, in accordance with accepted academic practice. No use, distribution or reproduction is permitted which does not comply with these terms.
*Correspondence: Rewaida Abdel-Gaber, cmFiZGVsZ2FiZXJAa3N1LmVkdS5zYQ==