- 1Faculty of Agricultural Sciences, Federal University of Grande Dourados, Dourados, Mato Grosso do Sul, Brazil
- 2Kupono Assessoria e Consultoria, Curitiba, Paraná, Brazil
- 3Mato Grosso do Sul State University, Dourados, Mato Grosso do Sul, Brazil
This study investigated the effects of a commercial polyphenol blend on broiler performance, meat quality, carcass traits, and the incidence of pectoral myopathies. Broilers (1–42 days old) were allocated to four treatments: T1 (control, basal diet), T2 (250 g/ton polyphenol blend), T3 (500 g/ton), and T4 (1,000 g/ton), with eight replicates of 40 birds each. All diets were corn-soy based, isonutritional, and formulated to meet age-specific nutritional requirements. Parameters assessed at 21, 28, 35, and 42 days included antioxidant potential, growth performance, myopathy incidence, carcass yield, allometric growth, muscle morphometry, meat quality, and lipid profile. Optimal performance was observed at a supplementation level of 514 g/ton of polyphenols. While carcass yield remained unaffected, birds fed 500 g/ton exhibited delayed breast growth relative to other body parts, suggesting modulated allometric growth. Polyphenol supplementation reduced breast muscle fiber size, increased fiber density, and lowered the severity of wooden breast without influencing the incidence of white striping. Improved meat tenderness was evident through reduced cooking weight loss and enhanced shear force. Antioxidant status improved in plasma, muscle, and liver tissues, and the muscle lipid profile was favorably altered. In conclusion, the polyphenol blend enhanced broiler zootechnical performance, alleviated wooden breast severity, and improved meat quality and tenderness.
1 Introduction
Broiler chicken myopathies, such as wooden breast and white striping, lead to the condemnation of carcasses and prime commercial cuts during slaughter, resulting in significant economic losses for the poultry industry. Wooden breast is characterized by localized or diffuse areas of hardness in the pectoral muscle, sometimes accompanied by viscous material and petechiae (1). White striping manifests as white lines running parallel to the muscle fibers, which are prominently visible in raw meat, primarily affecting the breast and thigh muscles (2, 3).
The myodegeneration associated with white striping and wooden breast is well documented to reduce total protein content and alter protein profiles (4). Histologically, these myopathies are characterized by myodegeneration, necrosis, fibrosis, lipidosis, and regenerative changes in the pectoralis major muscle (5).
These myopathies are strongly correlated with the fast growth rates of broiler chickens (6) and genetic selection for increased breast size, which compromise blood and oxygen supply to the muscle tissue. This leads to severe hypoxia (7–9) and cellular damage, as the removal of metabolic waste products is also impaired (10–12). Evidence suggests that muscle tissue attempts to counteract hypoxia by synthesizing nitric oxide to increase blood flow, but this response can exacerbate and accelerate oxidative stress. This pro-oxidative environment contributes to tissue inflammation and further myodegeneration (7). Although the link between pectoral myopathies and oxidative stress is not yet fully elucidated, studies have reported elevated levels of oxidative markers in chickens affected by both white striping and wooden breast (13, 14). Animals with higher growth rates and accelerated metabolism may be particularly susceptible to oxidative stress due to increased production of reactive oxygen species (ROS) (15).
This study hypothesizes that nutritional strategies may reduce oxidative stress and mitigate myopathy severity. Polyphenols, a diverse group of phytogenic substances found in plant components such as leaves, seeds, flowers, and fruits (16), have demonstrated antioxidant, anti-inflammatory, and anti-mutagenic properties (17, 18). Polyphenols can be categorized into two main groups: non-flavonoids, including lignans, stilbenes, and phenolic acids (e.g., tannins), and flavonoids, such as flavones, flavonols, flavanols, flavanones, isoflavonoids, and anthocyanins (19).
The antioxidant mechanism of polyphenols involves scavenging free radicals or stimulating the synthesis of ROS-removing enzymes, including superoxide dismutase, catalase, and glutathione peroxidase (16, 20, 21). Consequently, polyphenols are promising candidates for poultry nutrition to mitigate myopathies and enhance meat quality. Previous research has shown that polyphenols positively influence the performance and intestinal health of production animals (16, 21, 22).
Therefore, this study aimed to evaluate the effects of different levels of a commercial polyphenol blend in broiler chicken diets on zootechnical performance, the allometric growth of body parts, the incidence of white striping and wooden breast myopathies, meat quality parameters, muscle morphometry, antioxidant potential, and lipid profile.
2 Materials and methods
2.1 Birds and housing
This study was approved by the Ethics Committee on the Use of Animals (CEUA/UFGD) under protocol number 02/2022. The experiment was conducted in the poultry house of the School of Agricultural Sciences at the Federal University of Grande Dourados (FCA/UFGD). The facility was equipped with a negative pressure system, pendulum drinkers, tubular feeders, and 250 W infrared lamps. For the first week, the light program provided 23 h of continuous light, which was gradually reduced over 11 days. Thereafter, birds were exposed to 18 h of intermittent light per day at an intensity of 22 lumens. Temperature and humidity were monitored every 15 min using the RC-51 data logger (Elitech Brasil).
The study included 1,280 one-day-old male Ross AP91® chicks raised at a density of 14 birds/m2. Birds were allocated to a completely randomized design comprising four experimental diets with eight replicates of 40 birds each: T1 (control, basal diet), T2 (250 g/ton of a commercial polyphenol blend, Silvateam ATX), T3 (500 g/ton of ATX), and T4 (1,000 g/ton of ATX).
The polyphenol blend (Silvafeed ATX®) was a powdered additive rich in polyphenols, flavonoids, and tannins, produced by SilvaTeam (San Michele Mondovì CN, Italy). Diets were formulated using corn and soybean meal to meet nutritional requirements based on Rostagno et al. (23). Feed was provided ad libitum in four phases according to age: pre-starter (1–7 days), starter (8–21 days), grower (22–35 days), and finisher (36–42 days) (Table 1). Management practices followed the guidelines specified in the Ross manual.
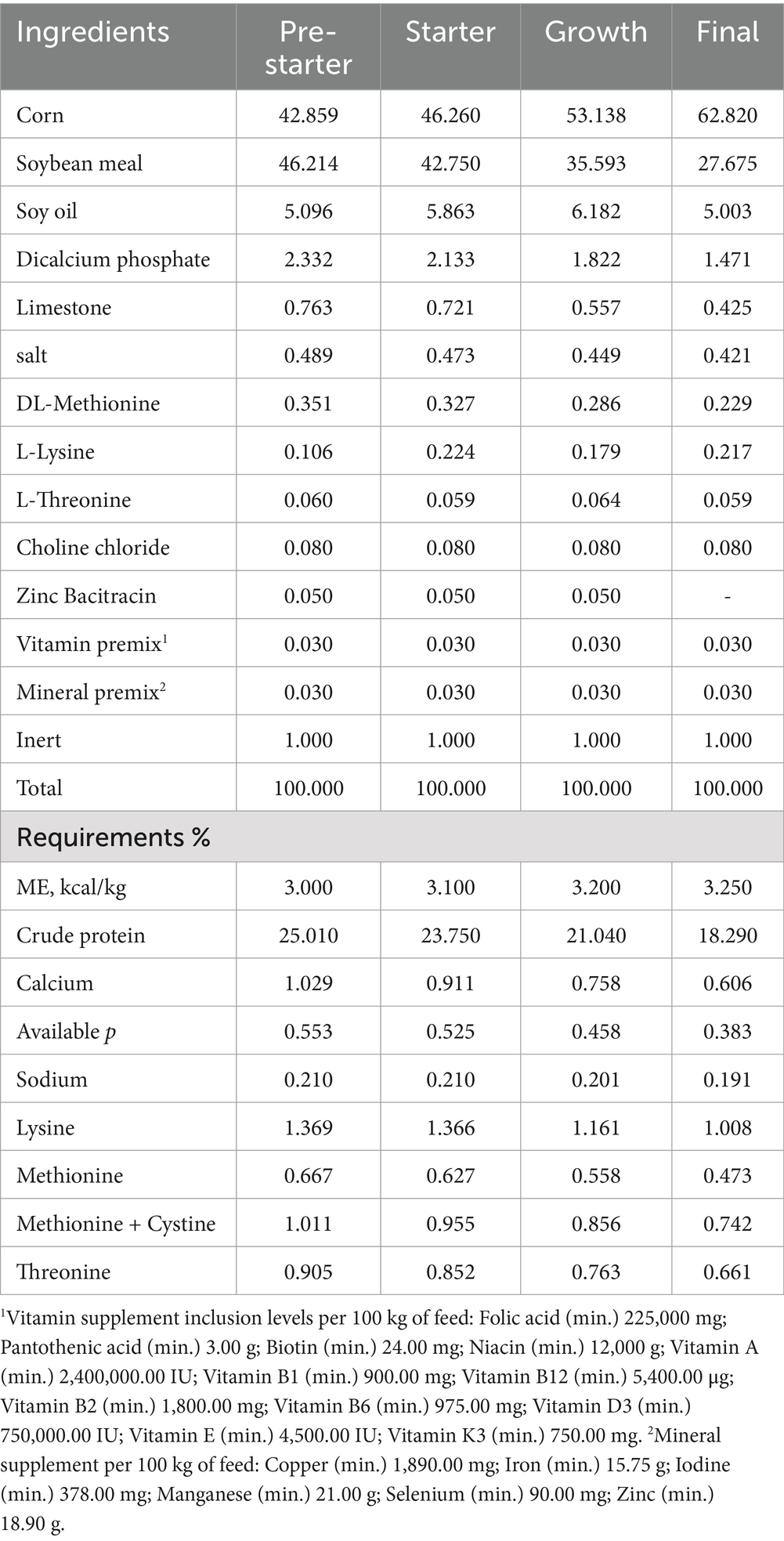
Table 1. Composition of experimental diets according to the productive phase in meeting the nutritional requirements for male broilers in 100 kg of feed.
2.2 Zootechnical performance
Zootechnical performance was evaluated during the 1–7, 1–21, 1–35, and 1–42 days periods, with parameters including feed intake (FI), weight gain (WG), feed conversion ratio (FCR), and the European Broiler Production Efficiency Index (EBI). All birds were weighed weekly to determine WG, while feed supplied and leftover feed were recorded to calculate FI. The FCR was derived from the ratio of FI to WG. The EBI was calculated as:
2.3 Carcass and cut yield and calculation of the allometric coefficient
All birds were weighed at 21, 28, 35, and 42 days of age. Two birds per replicate (16 birds per treatment), representing the average pen weight (±5%), were selected to evaluate carcass and cut yield, as well as meat quality and pectoral myopathy incidence (white striping and wooden breast).
The birds were fasted for 8 h, slaughtered via cervical dislocation, and exsanguinated by severing the jugular veins and carotid arteries. Carcasses underwent scalding, plucking, evisceration, pre-cooling (10–18°C for 12 min), cooling (0–2°C for 18 min), and dripping (5 min). Carcasses were weighed post-evisceration (hot), post-dripping (chilled), and then processed for cuts.
Yields (%) were calculated based on live weight for hot and chilled carcasses (excluding feet and head) and based on chilled carcass weight for cuts (bone-in breast, boneless skinless breast, thighs and drumsticks, wings, and back).
The relative growth or allometric coefficient of commercial cuts and chilled carcass weight was determined using the methodology described by Castilho et al. (24).
2.4 Classification of myopathies and meat quality analysis
Boneless breast filets were classified for myopathy severity at 21, 28, 35, and 42 days of age. White striping was scored using the four-point scale established by Kuttappan et al. (25), while wooden breast was classified by manual palpation, following the guidelines of Sihvo et al. (12). A trained evaluator conducted the classifications.
Filets were stored at 5°C for 24 h before meat quality analyses, which included dimensions (length, width, thickness) (26), pH (after 24 h), objective color (27), water holding capacity (28), drip loss (29) weight loss from thawing/cooking (30), and shear force (31). Sampling for each filet was standardized according to Castilho et al. (24).
2.5 Muscle morphometry
Fragments of the pectoralis major muscle were collected from one bird per replicate (eight birds per treatment) at 21, 28, 35, and 42 days of age for morphometric analysis. Samples (~200 mg) were cut into parallelepiped shapes, oriented parallel to the muscle fibers, and had their surfaces removed. Samples were immediately frozen in liquid nitrogen and stored at −80°C (96).
Frozen samples were sectioned using a Lupetec® CM 2850 cryostat microtome at −20°C. Samples were fixed onto the cryostat’s metallic support using a special resin. Histological sections were obtained, considering two slides per bird and 10 sections per slide, with a thickness of 10 μm. The sections were then subjected to Hematoxylin–Eosin (HE) staining to analyze the general morphometry of muscle fibers.
A total of 10 photomicrographs per sample were obtained using a Precision® P207BI binocular microscope equipped with a BEL Engineering® Eurekam 1.3 camera, connected to a computer, and analyzed with the Image Pro-Plus® software. The images were captured under 10x objective magnification, combined with a 10x ocular lens, resulting in a total magnification of 100x, allowing for a detailed evaluation of the muscle fiber structure. The analyses included fiber count per field, representing the number of fibers in the cross-sectional area; fiber diameter (μm), which corresponds to the mean measurement of the largest diameter of each muscle fiber per field; fiber area (μm2), represented by the mean measurement of each muscle fiber’s area per field; and the fiber area per field (%), which represents the percentage of the cross-sectional area occupied by muscle fibers, excluding free spaces, as described by Castilho et al. (32).
2.6 Antioxidant activity
Plasma, 3 g of liver, and Pectoralis major muscle samples were collected from five birds per treatment at 21 and 42 days of age. Plasma was obtained by collecting blood samples from the ulnar vein in tubes containing an anticoagulant, followed by centrifugation to separate the blood components immediately after collection. The samples were centrifuged at 10,000 g for 15 min at 4°C, allowing the separation of plasma, which was carefully collected and stored at an appropriate temperature (−80°C) until analysis. Liver and muscle samples were stored at −80°C and subsequently lyophilized. To evaluate antioxidant potential, liver and muscle samples were freeze-dried, pulverized, and extracted with 5 mL of hexane for 30 min using ultrasound. Following extraction, the hexane phase was filtered and evaporated under a fume hood. The remaining solid residue underwent further sequential extraction using ethyl acetate and ethanol under the same conditions. The resulting filtrates were combined, dried, and solubilized in ethanol to form a single extract. This extract was analyzed for antioxidant activity using the DPPH (2,2-diphenyl-1-picrylhydrazyl) assay. Plasma samples were analyzed directly without prior extraction.
To assess the DPPH inhibition capacity, 3 mL of 0.1 mM DPPH solution was mixed with triplicate sample aliquots at varying concentrations. Absorbance at 517 nm was measured using a spectrophotometer. The percentage of inhibition was calculated following the method described by Kumaran (33).
2.7 Determination of fatty acid profile
The lipid fraction of Pectoralis major muscle samples from 42-day-old birds was extracted following the method of Bligh and Dyer (34). The lipid extract was weighed, and 60 mg of it was methylated according to Maia and Rodriguez-Amaya (35) for gas chromatography analysis.
Fatty acid methyl ester analysis was conducted using a gas chromatograph (Thermo) equipped with a flame ionization detector, a “split/splitless” injector, and a fused silica capillary column with polyethylene glycol as the stationary phase (DB-Wax, 30 m × 0.25 mm, J&W Scientific). Chromatographic conditions were as follows: injector temperature at 250°C; column temperature at 180°C for 20 min, followed by a programmed increase of 2°C/min to 220°C; detector temperature at 260°C; hydrogen carrier gas at a flow rate of 1.0 mL/min; nitrogen make-up gas at 20 mL/min; and injection volume of 1 μL.
Fatty acid identification was based on retention time comparisons with methyl ester standards (Sigma-Aldrich). Quantification was performed via area normalization, and results were expressed as the percentage of each fatty acid relative to the total fatty acid area.
2.8 Serum biochemical profile
Blood samples (5 mL/bird) were collected from the ulnar vein of one bird per replicate (eight birds per treatment) using heparinized syringes at 42 days of age. Samples were collected at the experimental poultry house, ensuring that they were obtained under standardized conditions. Samples were centrifuged at 4,000 rpm for 10 min to separate the serum, which was subsequently stored at −80°C until analysis.
To assess clinical markers of muscle damage, serum alanine aminotransferase (ALT), aspartate aminotransferase (AST), and lactate levels were evaluated. ALT and AST were analyzed using a spectrophotometer (BioPlus 200) as per the manufacturer’s instructions (GoldAnalisa®). Lactate levels were measured with the Accutrend Plus Roche® device and BM-Lactate® test strips.
2.9 Statistical analysis
The normality of residuals and homogeneity of variances were assessed using the Shapiro–Wilk and Levene tests, respectively. Subsequently, the data were subjected to analysis of variance (ANOVA) using the SAS MIXED procedure (SAS, version 9.4; SAS Institute Inc., Cary, NC, United States). For performance and live weight data, the mathematical model included covariates to adjust for initial conditions. When the statistical model was significant, polynomial regression analysis was used to estimate the effects of polyphenol blend inclusion levels.
Regression models were evaluated based on the significance of parameters, the coefficient of determination (R2), and the alignment of estimated levels with biological plausibility. Coefficients of determination were classified as high (0.70–1.00), moderate (0.40–0.69), or low (0.00–0.39). Statistical significance was set at a 5% level (p < 0.05).
Since the results for myopathy assessments were not normally distributed, generalized linear models (GLMs) were applied following the methodology of Nelder and Wedderburn (36) using the SAS GLIMMIX procedure (97). Data distributions were modeled as gamma (GAMMA) if residuals exhibited exponential behavior. Regression coefficients and intercepts were obtained using the SOLUTION option of the GLIMMIX procedure. To compare means, “lsmeans” were calculated using the “inverse link” function, with adjustments made using the pdiff ilink lines statement in the GLIMMIX procedure.
The study of relative growth of body cuts was analyzed using the power equation Y = aXbY = a Xb, which was transformed logarithmically into a linear model, lnY = lna + b lnX + lnei (24, 37, 38).
3 Results
3.1 Environmental conditions and zootechnical performance, carcass and cut yield, and allometry
During the study, temperature and humidity were within the expected range for the age of the animals (Table 2). However, from 11 to 42 days, daily temperature variations ranged from 7 to 10°C.
Feed intake demonstrated a linear decrease over the cumulative periods of 1–21, 1–35, and 1–42 days of age. Specifically, higher levels of polyphenol blend in the diet were associated with lower feed consumption (Figure 1).
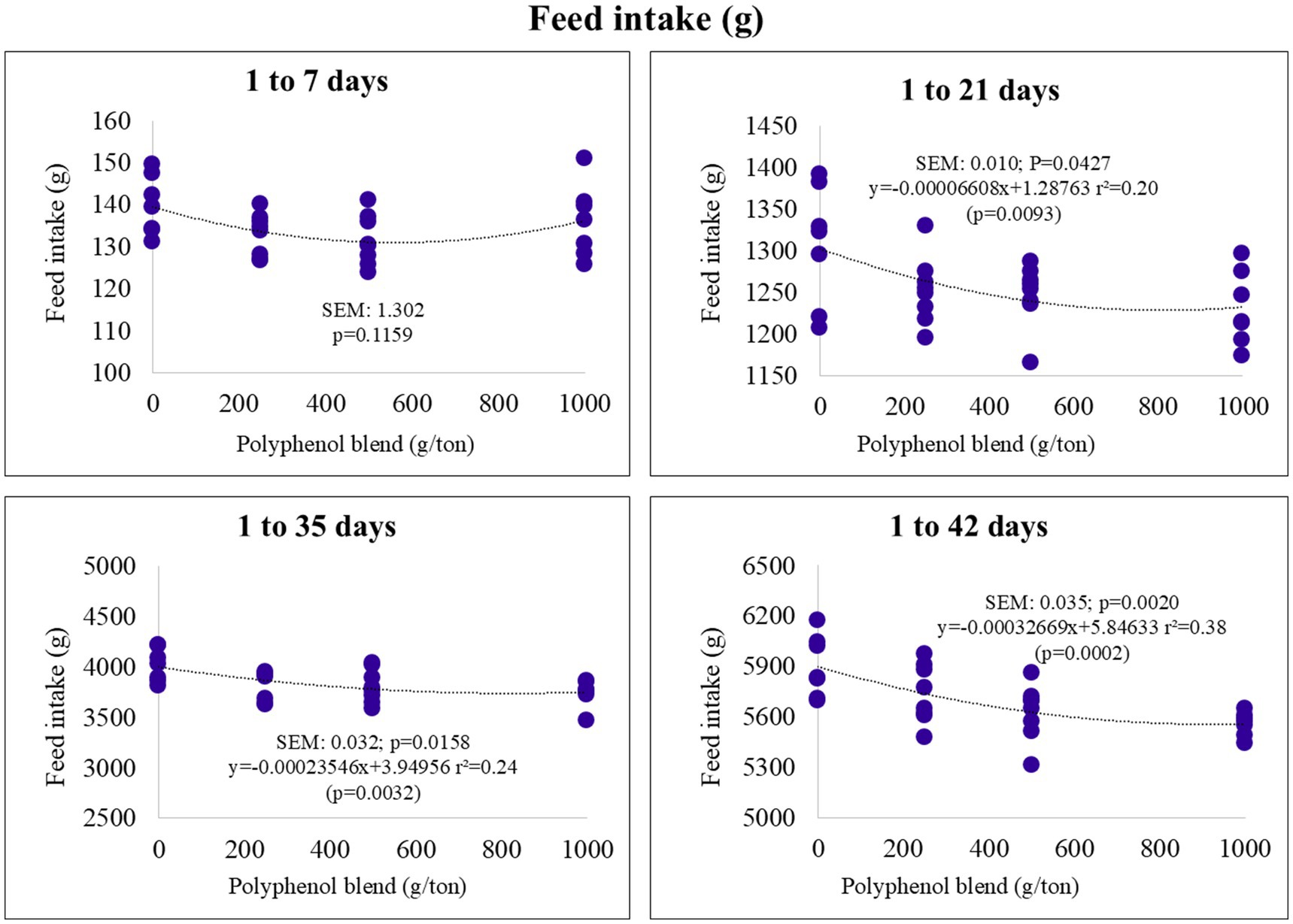
Figure 1. Feed intake (g) of broiler chickens fed on diets containing different levels of polyphenol blend.
In the starter phase (1–21 days), weight gain exhibited a linear increase with higher polyphenol inclusion. In the final phase (1–42 days), it followed a quadratic trend, with an estimated maximum of 3.620 kg at 664 g/ton of polyphenol inclusion (Figure 2).
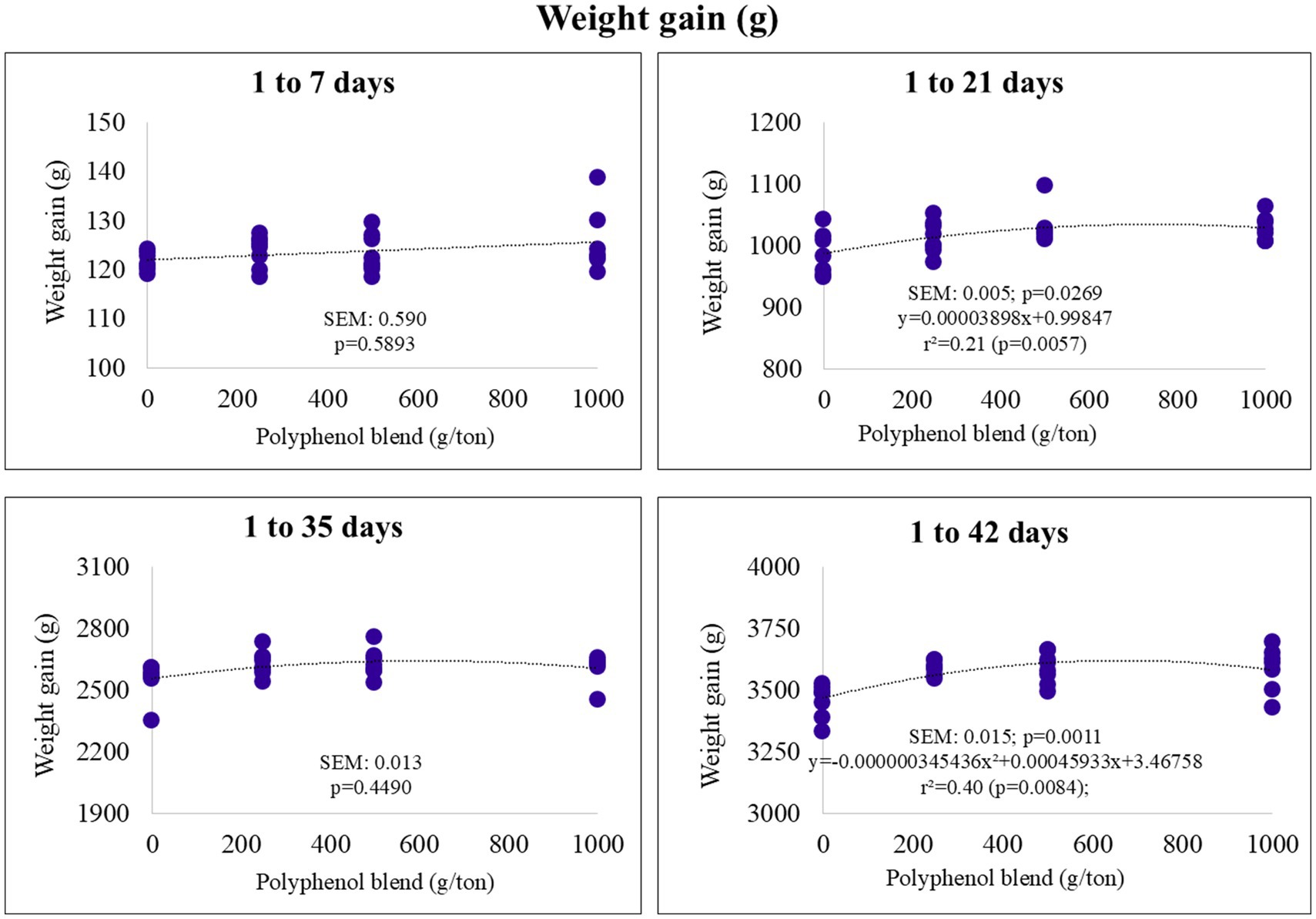
Figure 2. Weight gain (g) of broiler chickens fed on diets containing different levels of polyphenol blend.
The feed conversion ratio (FCR) exhibited a quadratic response, with minimum points observed at polyphenol blend inclusion levels of 633, 778, 722, and 763 g/ton for the pre-starter, starter, growth, and final phases, respectively. The corresponding FCR values were estimated at 1.09, 1.18, 1.41, and 1.53 (Figure 3).
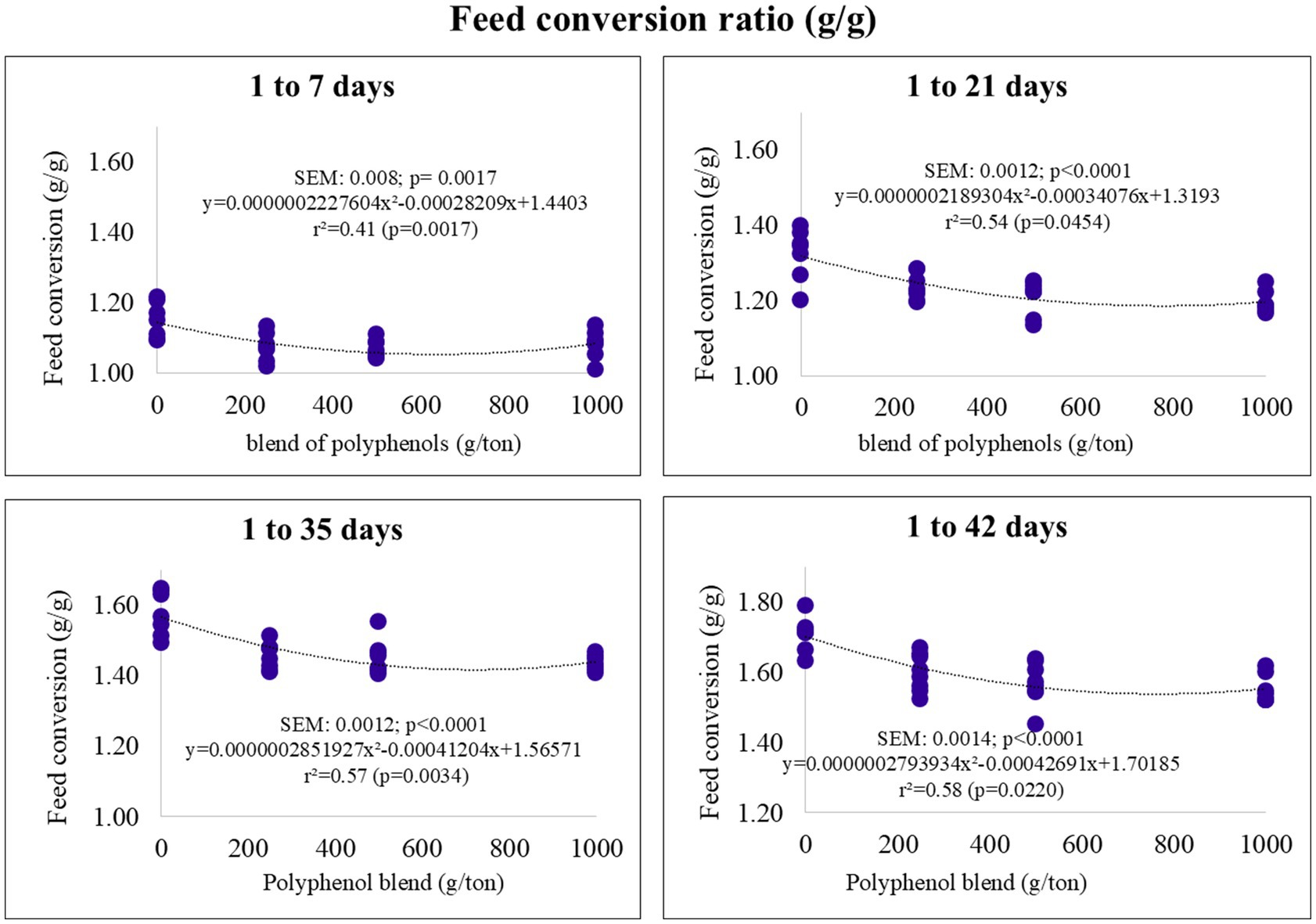
Figure 3. Feed conversion ratio (FCR) of broiler chickens fed on diets containing different levels of polyphenol blend.
The European Production Efficiency Index (EBI) also followed a quadratic trend. The maximum estimated inclusion levels for EBI were 580, 519, and 514 g/ton for the pre-starter, starter, and final phases, respectively (Figure 4). The optimal EBI values were estimated at 157 (pre-starter), 359 (starter), and 477 (final).
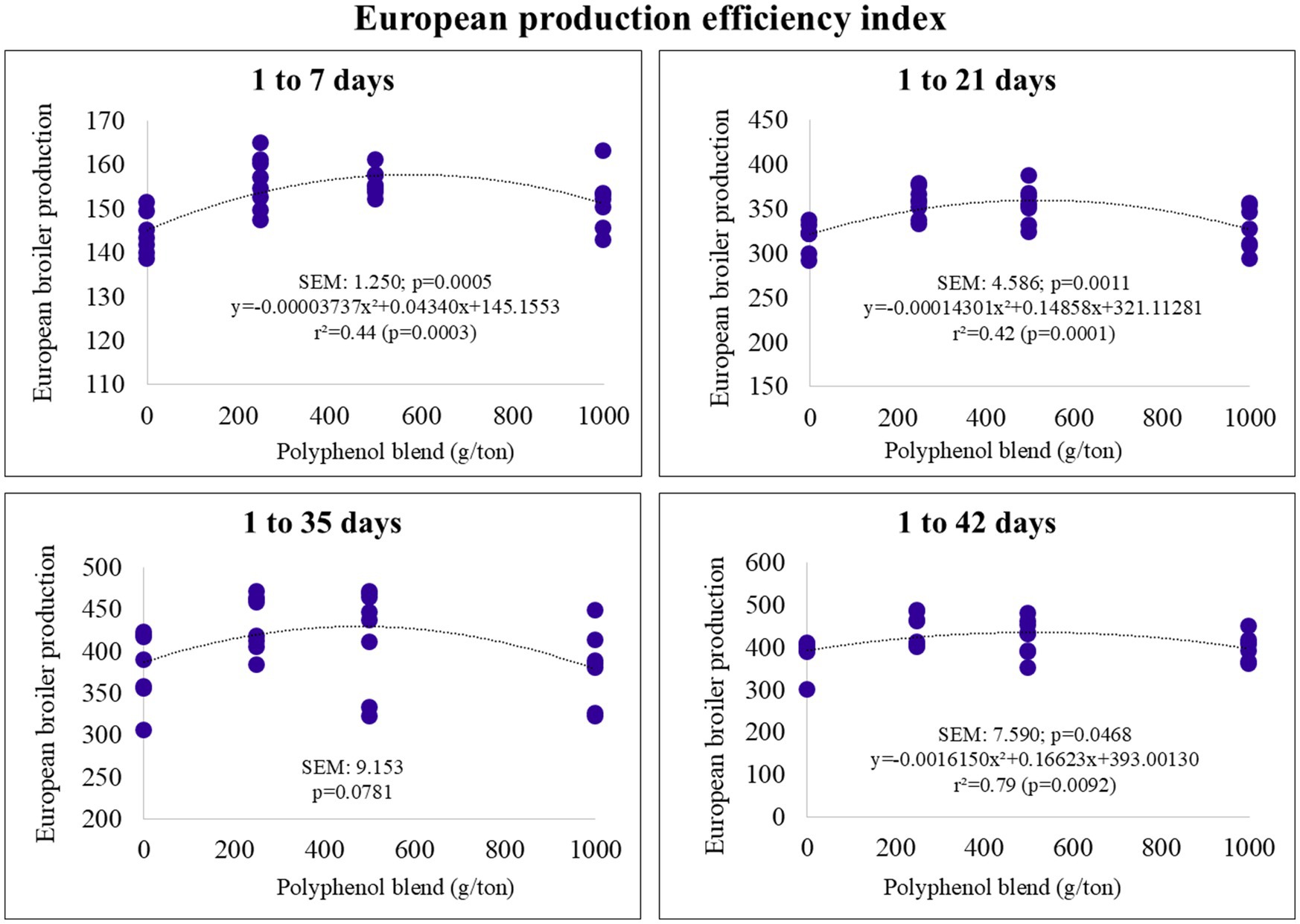
Figure 4. European Production efficiency index of broiler chickens fed on diets containing different levels of polyphenol blend.
Carcass yield and the yield of bone-in and boneless cuts from broilers at 21, 28, 35, and 42 days of age were not significantly influenced by the inclusion of the polyphenol blend, except for back yield at 42 days (Table 3). Back weight showed a linear decrease, but the low coefficient of determination indicated that the regression equation poorly explained the data variation.
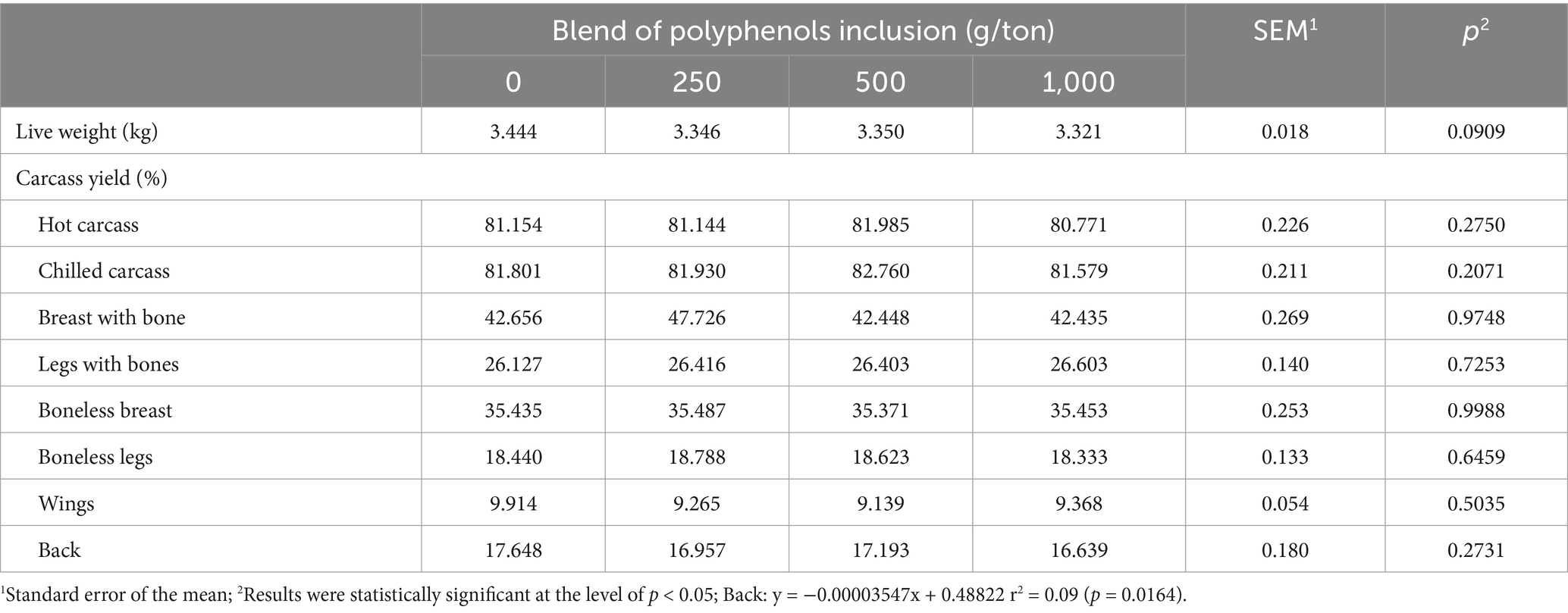
Table 3. Carcass and cut yield of broiler chickens fed on different levels of polyphenol blend at 42 days of age.
For allometric growth coefficients, the control diet (without polyphenol blend inclusion) revealed isogonic growth (b = 1) for the back, indicating similar growth rates to the overall body (Table 4). In contrast, the breast, legs, and wings (except for the inclusion of 250 g/ton) exhibited heterogonic growth (b ≠ 1). The breast and wings displayed early growth (b < 1), while the legs showed late growth (b > 1) in the control treatment.
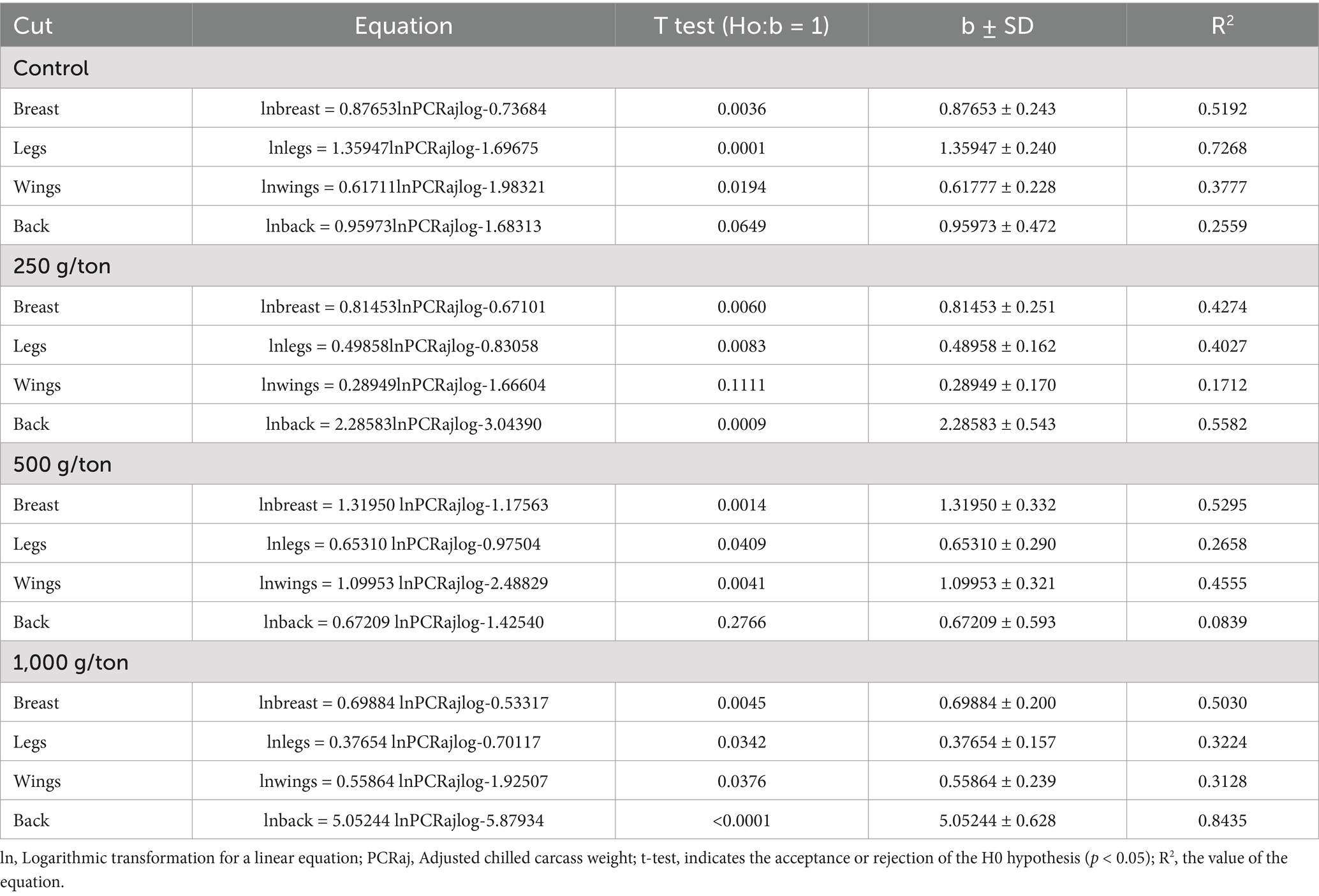
Table 4. Allometric coefficient of broiler chickens slaughtered at 42 days of age fed on diets containing different levels of polyphenol blend.
The inclusion of 250 g/ton of the polyphenol blend resulted in early growth for the breast and legs (negative heterogonic growth), late growth for the back (positive heterogonic growth), and isogonic growth for the wings (b = 1). At 500 g/ton, breasts and wings showed late growth, legs showed early growth, and back exhibited isogonic growth. At 1,000 g/ton, early growth was observed for the breast, legs, and wings, while the back showed late growth.
3.2 Quantification of myopathies, meat quality, and muscle morphometry
At 21 days, wooden breast severity decreased linearly with increasing levels of the polyphenol blend (Figure 5). The polyphenol blend did not affect the incidence of white striping (data not shown).
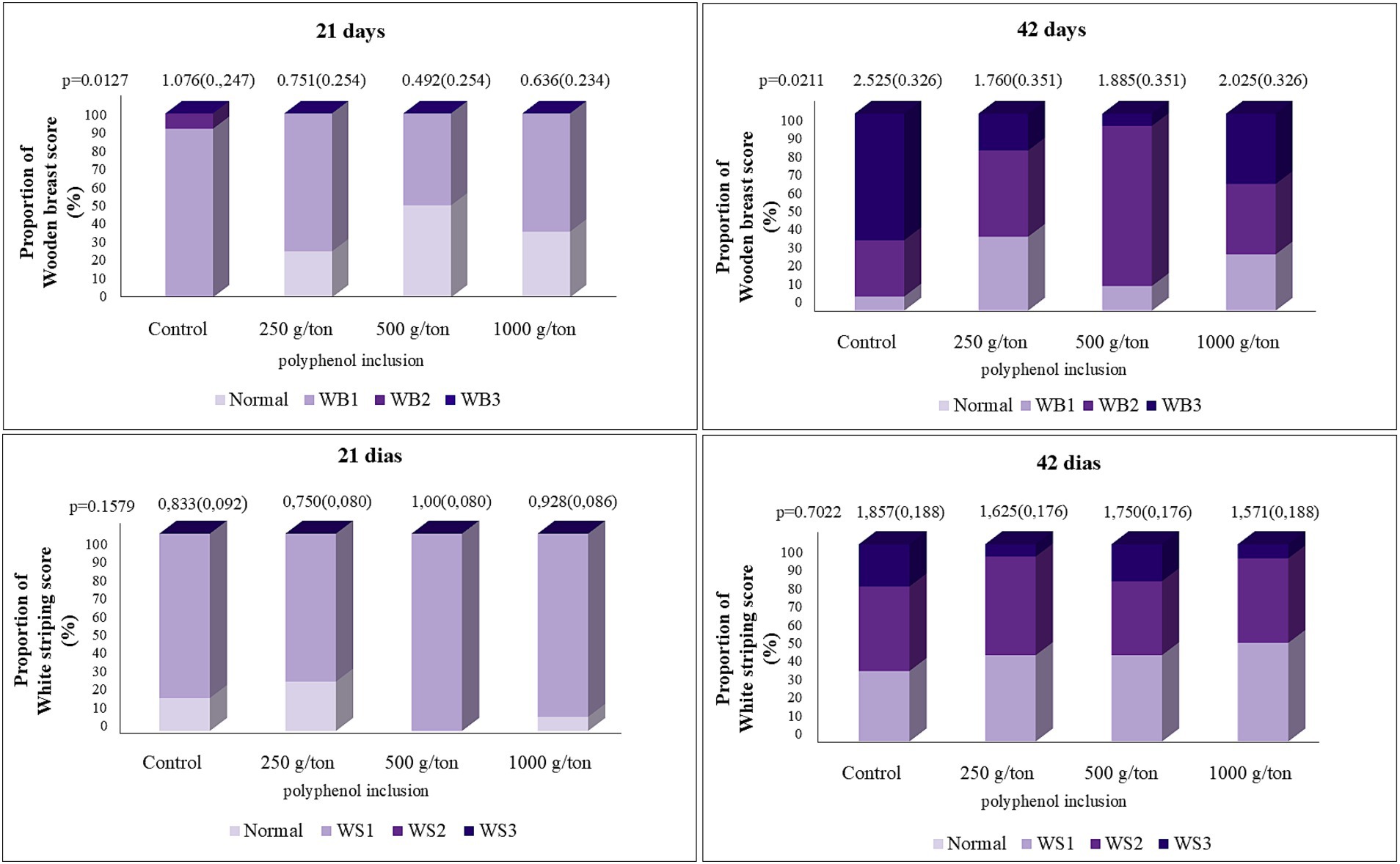
Figure 5. Quantification of pectoral myopathy scores in broiler chickens fed on different levels of polyphenol blend. Average estimated by the mathematical model. WB, wooden breast (21 days): Y = −0.00038X + 0.8967 a/b (SEM); WB (42 days): y = 0.0000009516×2 − 0.00126X + 1.8876 a/b (SEM).
At 42 days, wooden breast myopathy exhibited a quadratic trend, with the minimum severity estimated at 652 g/ton of polyphenol blend inclusion. No significant differences were observed for wooden breast severity at 28 and 35 days. No statistically significant differences were found for the evaluation of white striping.
Meat quality parameters evaluated at 21, 28, and 35 days were not influenced by the polyphenol blend (results not shown). However, at 42 days, cooking weight loss (CPP) decreased linearly as polyphenol inclusion increased (Table 5). Shear force exhibited a quadratic response, with the minimum shear force estimated at 609 g/ton of polyphenol blend inclusion.
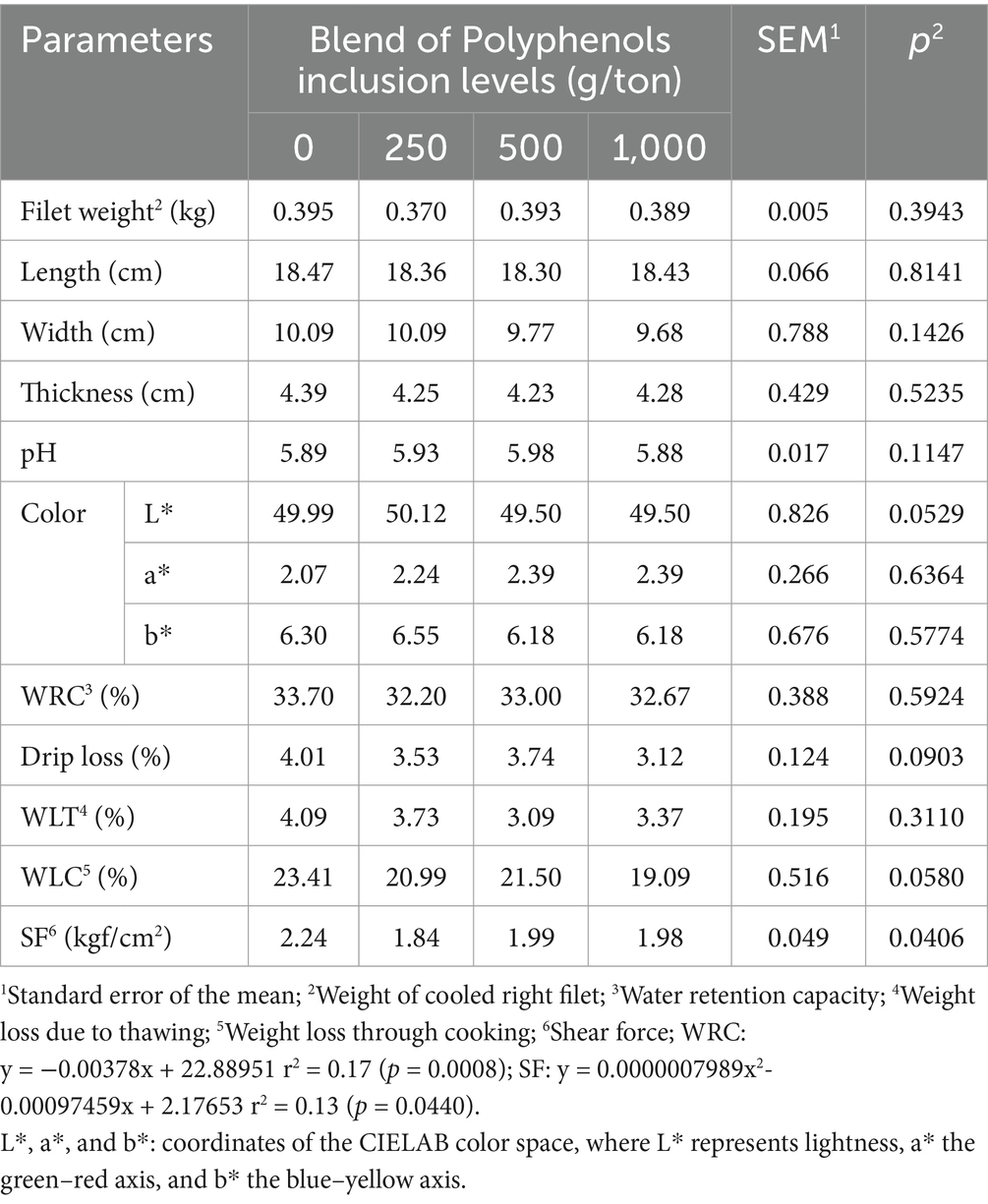
Table 5. Meat quality parameters of broiler chickens slaughtered at 42 days of age fed on different levels of polyphenol blend.
Regarding muscle tissue morphometry, at 21 days, fiber number per field showed a linear increase with increasing polyphenol inclusion, while fiber diameter and area decreased linearly (Table 6). At 42 days, the number of muscle fibers continued to increase linearly with polyphenol levels, while fiber area decreased. No significant differences in muscle morphometry were observed at 28 and 35 days of age.
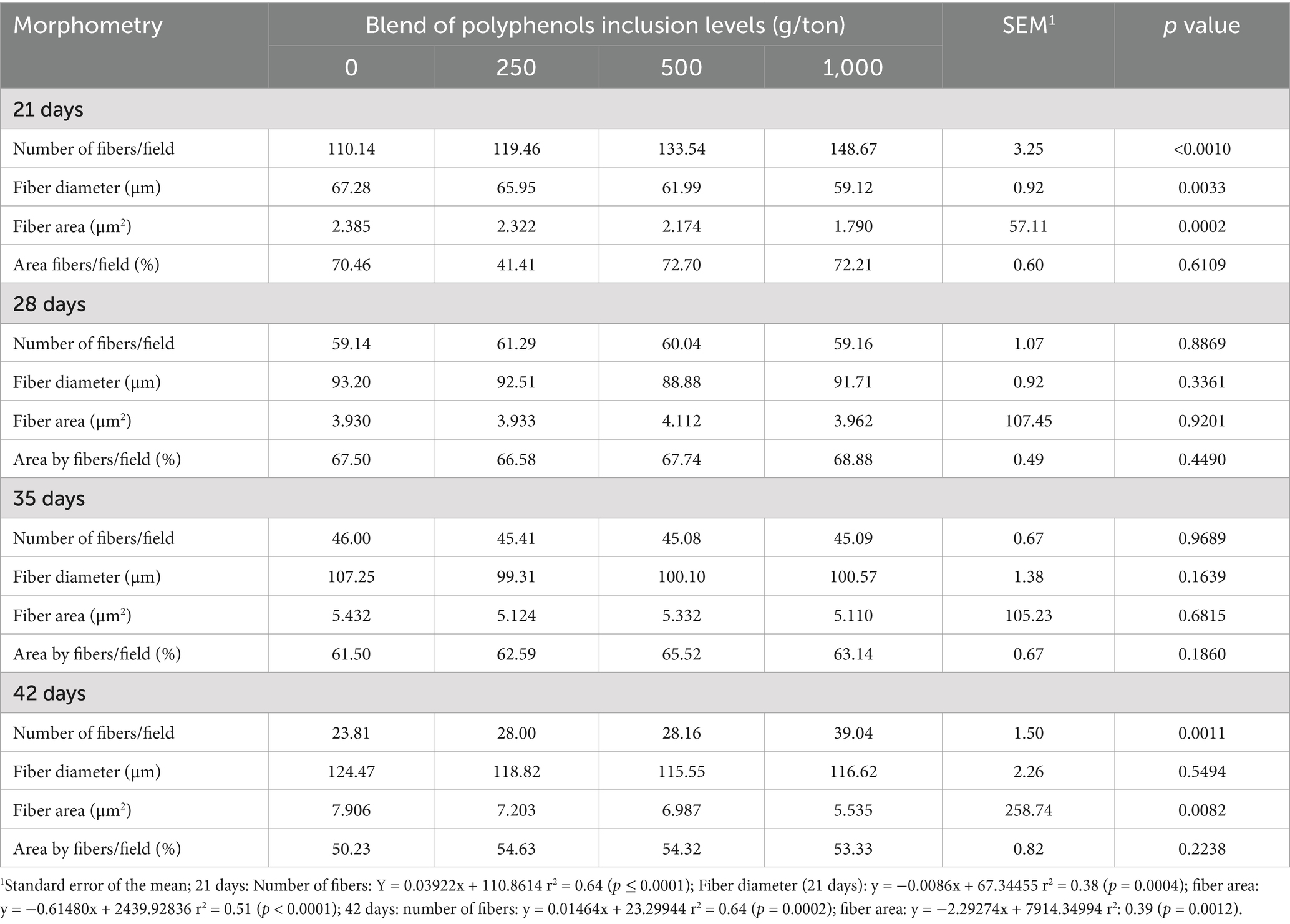
Table 6. Morphometry of muscle fibers from the breast of broiler chickens fed on different levels of polyphenol blend.
3.3 Antioxidant status, lipid profile, and biochemical parameters
Plasma antioxidant potential was significantly affected by polyphenol inclusion, with quadratic responses observed at 21 and 42 days. The maximum estimated inclusion levels were 978 g/ton at 21 days and beyond the tested range at 42 days (Figure 6).
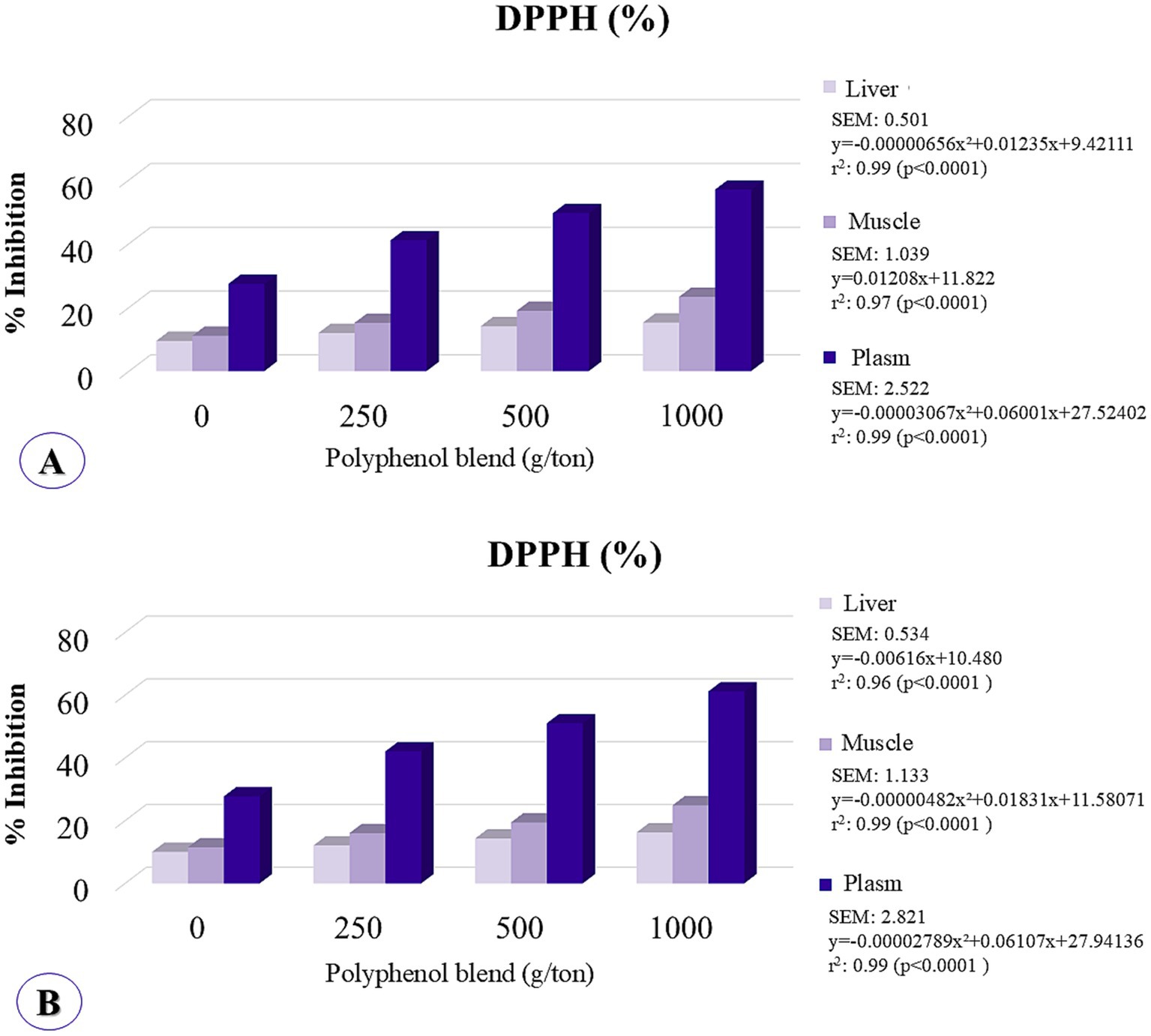
Figure 6. Hepatic, muscular, and serum concentrations of antioxidant potential were analyzed by the method of inhibiting DPPH (2,2-diphenyl-1-picrylhydrazyl) in broiler chickens fed on different levels of polyphenol inclusion. (A) Assessment at 21 days; (B) Assessment at 42 days.
In the breast muscle, DPPH inhibition demonstrated a linear increase at 21 days and exhibited quadratic behavior at 42 days, with the maximum point occurring beyond the tested range. In the liver, antioxidant potential followed a quadratic trend at 21 days, with the maximum inclusion level estimated at 941 g/ton, and displayed linear increasing behavior at 42 days. These findings indicate that higher levels of polyphenol inclusion enhance tissue antioxidant activity.
Capric acid (C10:0) and α-linolenic fatty acid (C18:3 ω3) showed a linear decrease with increasing levels of polyphenol blend inclusion. Conversely, palmitoleic acid (C16:1) exhibited a linear increase, indicating higher concentrations in the muscles of birds fed diets with greater polyphenol inclusion. Dihomo-γ-linolenic fatty acid (C20:3 ω6) followed a quadratic trend, with a maximum point estimated at 460 g/ton of polyphenol blend inclusion based on the regression equation (Table 7). Similarly, conjugated linoleic acid (CLA, C18:2) displayed quadratic behavior, with the optimal dosage estimated at 329 g/ton of polyphenols. Total omega-3 fatty acids exhibited a linear decrease with increasing polyphenol blend inclusion in the diet.
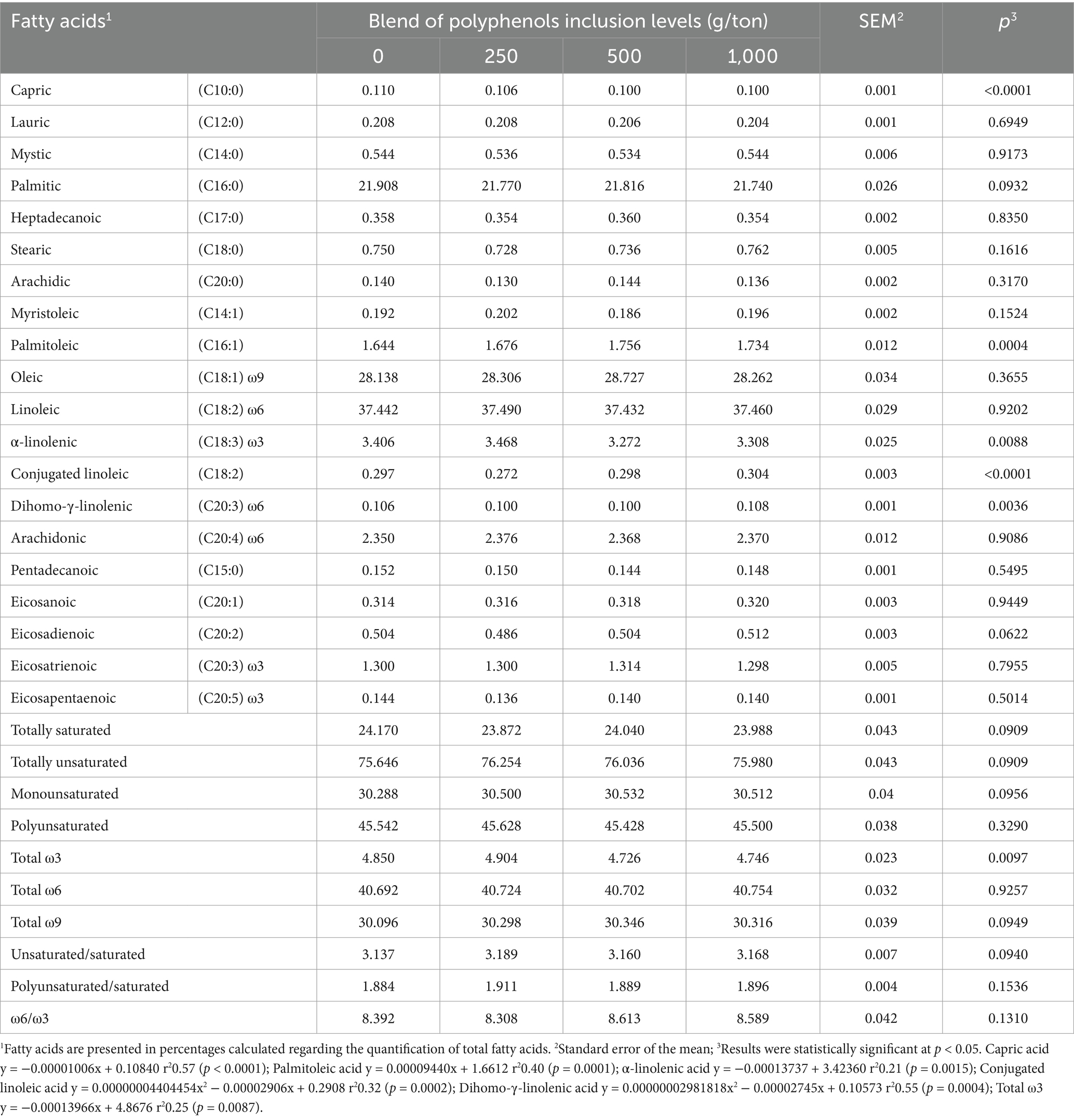
Table 7. Fatty acid profile of the breast muscle tissue (Pectoralis major) of broiler chickens fed with polyphenols and evaluated at 42 days of age.
No significant differences were observed in blood biochemical parameters, including alanine aminotransferase (ALT), aspartate aminotransferase (AST), and lactate levels (data not shown).
4 Discussion
The dietary inclusion of the polyphenol blend did not affect weight gain or feed intake during the pre-starter phase. However, broiler chicks supplemented with the polyphenol blend showed significant improvements in all performance parameters during the starter and growing phases, as well as over the entire period, indicating more efficient nutrient utilization. This finding contrasts with a study by Jamroz et al. (39), which found no significant effects on body weight gain or feed conversion when chickens were supplemented with a tannin compound from Chestnut (Castanea sativa) at concentrations of 250 or 500 mg/kg. Additionally, Zhou et al. (40) demonstrated that the positive effects of flavonoids are dose-dependent. These varying results suggest that the benefits of polyphenols depend on factors such as the specific type of polyphenol, the effective concentrations of bioactive molecules reaching the epithelium, and the metabolites produced by the gut microbiota, which may influence performance. Therefore, the correct dosage of polyphenols in feed is crucial for producing beneficial effects in chickens, and this also depends on the standardization of the plant extract.
The inclusion of the polyphenol blend in the diet from 1 to 42 days of age resulted in favorable production parameters. The optimal inclusion levels were estimated to be between 514 and 664 g/ton for weight gain and productive efficiency index, respectively. Current research supports the idea that appropriate amounts of polyphenols, such as tannic acid in the range of 0.5 g/kg to 5 g/kg, can improve performance and intestinal health due to their potential antimicrobial, antioxidant, and anti-inflammatory effects (41–44). However, higher doses of flavonoids may have adverse effects on performance (40).
Importantly, polyphenol blend supplementation influenced the growth pattern of various body parts in broiler chickens (Table 3). At 500 g/ton, there was a delay in breast growth compared to leg growth. However, no difference was observed in breast yield at 42 days of age (Table 2), suggesting that there was no reduction in protein deposition in the breast muscle, but rather an adjustment in the timing of growth for different body parts. This may also be related to the findings on Wooden breast myopathy, where an inclusion level of 654 g/ton led to a reduction in the severity of this myopathy. The results observed in the growth and development of muscle tissue can be explained by the myoprotective properties attributed to polyphenolic compounds, including: (1) enhancement of mitochondrial function and biogenesis; (2) induction of antioxidant enzymes and protection against mitochondrial dysfunction; (3) promotion of muscle angiogenesis, energy capacity, and contractile function; and (4) stimulation of protein synthesis, myotube differentiation, and hypertrophy of muscle fibers (45–47). While the exact biochemical mechanisms through which polyphenolic compounds protect skeletal muscle cells are not fully understood (48), their protective actions may occur at the intracellular level, through direct or indirect interactions with transcription factors such as peroxisome proliferator-activated receptor gamma coactivator 1-alpha (PGC-1α), Nuclear Respiratory Factor 1 (Nrf1), mitochondrial transcription factor A (TFAM), or myogenic regulators such as myogenin, Myogenic Factor 5 (Myf5), and Myoblast Determination Protein 1 (MyoD) (49).
The polyphenol blend used in this study contains different plant extracts, and its effects may arise from the combination of various molecules, such as condensed hydrolyzable tannins and flavonoids. Notably, ellagitannins, a class of hydrolyzable tannins, release a sugar molecule and several gallic acid and ellagic acid molecules upon hydrolysis (50). Gallic acid is easily absorbed into the bloodstream (51), while ellagic acid has low bioavailability due to its strong affinity for proteins and poor absorption (52). However, as Espín et al. (53) noted, ellagitannins undergo hydrolysis in the jejunum, increasing the amounts of ellagic acid and its derivatives, which are then metabolized by the intestinal microbiota. This metabolism leads to the production of compounds such as urolithins, which enhance cellular health and promote mitophagy and mitochondrial function, in addition to combating disorders related to muscles and the brain (54). Furthermore, intestinal metabolites of ellagic acid can produce urolithin A or urolithin B (55), both of which have myoprotective effects (56–58). Urolithin A has been shown to improve muscle strength and endurance (56, 57). The biological functions of bioactive compounds depend on their metabolites produced through intestinal and hepatic metabolism (59), highlighting the importance of intestinal health in the utilization of these compounds. To benefit from the muscle-related effects of ellagitannin supplements, individuals must possess an intestinal microbiome that includes representative amounts of Akkermansia muciniphila and Lactobacillus spp. (60, 61). Akkermansia muciniphila is associated with muscle development (62), while Lactobacillus spp. is linked to increased muscle mass and strength (63). Given these findings, further research should investigate the effects of polyphenol blend administration on the intestinal microbiome.
Studies have demonstrated that urolithin B has an androgenic effect on skeletal muscle without negatively impacting the development of other body tissues (58, 64). In vitro studies further show that urolithin B stimulates protein synthesis while reducing protein degradation (58). Additionally, this metabolite of ellagic acid exhibits anti-inflammatory effects by decreasing the expression of several cytokines, including interleukin-6 (IL-6), interferon-γ (IFN-γ), tumor necrosis factor-α (TNF-α), interleukin-4 (IL-4), and interleukin-1β (IL-1β) (65). Xing et al. (66) found that IL-6, IL-1β, and TNF-α mRNA expressions were elevated in muscles affected by Wooden breast myopathy. For instance, TNF-α contributes to muscle loss by inhibiting muscle regeneration, maintaining satellite cells in the proliferation stage and preventing their differentiation (67). The presence of IL-1β acts as a predisposing factor for impaired muscle regeneration, and its inhibition promotes muscle differentiation (68). Therefore, modulating the expression of inflammatory cytokines can improve inflammatory tissue damage and facilitate the regenerative phase of muscle affected by Wooden breast.
Furthermore, ellagitannins and ellagic acid possess phenolic hydroxyl groups, which contribute to the stabilization of reactive oxygen species (ROS) due to their ability to donate H+ radicals, thus remaining stable. As a result, they exhibit potent and sustained antioxidant effects both in vitro and in vivo, providing significant protection against ROS (69–71). Polyphenols can selectively induce the expression of antioxidant enzymes through the modulation of redox-sensitive signaling pathways, inhibiting lipid peroxidation, neutralizing free radicals (56, 72), and influencing muscle development as well as pectoral myopathies. Moreover, oxidative stress plays a pathogenic role in inflammatory diseases (73), including muscle conditions such as White striping and Wooden breast. Thus, evaluating the effects of polyphenols on the antioxidant capacity of broiler chickens is critical. As previously hypothesized, this study suggests that the polyphenol blend could positively impact the reduction of pectoral myopathies by modulating oxidative stress. At the molecular level, gene expression and metabolomics studies confirm that oxidative stress is a key factor in the development of pectoral myopathies (8, 13, 74). Accordingly, the use of a polyphenol blend in the diet positively influenced the antioxidant potential of plasma, muscle, and liver in chickens, as evaluated using the DPPH inhibition method (Figure 5). The DPPH radical is a widely used indicator for measuring antioxidant potential in various tissues and substances, including plant extracts, chemical compounds, and foods (75). It is employed to assess the capacity to neutralize free radicals, which are strongly associated with cellular aging and tissue damage (76). Polyphenols possess strong antioxidant properties due to the presence of one or more aromatic rings with hydroxyl groups that can interact with free radicals to form phenoxyl radicals, a structure responsible for their antioxidant activity (77–79). Therefore, the polyphenol blend may also mitigate lipid oxidation by inhibiting the formation of reactive oxygen species and acting as metal ion chelators, which catalyze oxidation reactions (80).
The morphometry of the pectoral muscle tissue further supports the reduction in the severity of myopathies. It reveals that higher levels of polyphenol blend inclusion lead to smaller muscle fiber areas, compensating for this reduction in fiber diameter with an increased fiber count per unit area. These findings explain why there were no losses in carcass and breast yield in birds at 42 days of age. This is likely due to the myoprotective action of compounds derived from the hydrolysis of previously described components. Therefore, the factors discussed may be correlated with the development of the pectoral muscle, with improvements in meat tenderness and a reduction in cooking-induced weight loss. A significant decrease in protein content and an increase in collagen in muscles affected by Wooden breast myopathy have been widely reported. These changes are likely related to progressive myodegeneration, deposition of interstitial connective tissue, and fibrosis (1, 14, 81). This finding corroborates several studies that have shown that severe Wooden breast increases meat toughness, drip loss, and moisture loss during cooking (82–85). Furthermore, relatively large muscle fibers are responsible for the coarse and undesirable texture of cross-cut meat (86).
When considering the quality of chicken products, fatty acids also play a significant role, as the use of natural food additives can alter the lipid profile of intramuscular fat (87) and directly impact meat quality and organoleptic properties. The administration of polyphenols has been shown to modify the lipid composition of animal products (88, 89). The analysis in this study demonstrated an effect on specific fatty acids, such as palmitoleic acid (C16:1), α-linolenic acid (C18:3), conjugated linoleic acid (C18:2), and dihomo-γ-linolenic acid (C20:3). Furthermore, a decrease in omega-3 fatty acid concentrations was observed as the inclusion levels of the polyphenol blend increased (Table 7). These variations may be attributed to the interaction between the metabolic processes involved in the synthesis of these fatty acids and the compounds in the polyphenol extract, as well as their capacity to influence lipid absorption. However, the reduction of omega-3 fatty acids may contribute to an extended shelf life for meat products by decreasing the presence of long-chain fatty acids, which are more prone to oxidation. These findings suggest that further studies on metabolic pathways could provide a deeper understanding of the effects of these compounds on the animal organism. Tannins and flavonoids are known to influence lipid metabolism in various ways. For example, tannins can reduce lipid absorption in the intestine by forming tannin-lipid complexes (90), thereby altering the physical properties of lipids and potentially affecting their bioavailability and functionality. The hypotriglyceridemic effect of proanthocyanidins found in chestnut wood, for example, is associated with reduced lipid absorption by enterocytes, leading to increased fecal excretion of cholesterol (91). Moreover, polyphenolic compounds can modulate lipid metabolism by influencing gene expression and enzymatic activity involved in lipogenesis, lipolysis, and cholesterol synthesis (92, 93). Another hypothesis is that bioactive compounds may directly influence the intestinal microbiota, which in turn could modulate the absorption, bioavailability, and biotransformation of fatty acids (94). Certain microbial species, such as Bacillus proteus or Lactobacillus plantarum, have been shown to convert α-linolenic acid (C18:3n-3), a precursor of omega-3 polyunsaturated fatty acids (PUFAs), into conjugated linoleic acid (C18:2), which is then further hydrogenated to saturated fatty acids like stearic acid (C18:0), thereby reducing the composition of omega-3 PUFAs (95).
Based on what has been explained so far, polyphenol blend reduced the incidence of Wooden Breast myopathy at both 21 and 42 days of age, likely due to its antioxidant potential, as observed in various tissues, and the modulation of muscle fiber size in the breast. This is associated with improved meat tenderness and reduced weight loss during cooking. Additionally, in birds fed with 500 g/ton of polyphenol blend, breast growth was delayed compared to other body parts, suggesting a modulation of allometric growth.
Supplementation with the polyphenol blend also improved the animals’ zootechnical performance, including feed consumption, weight gain, and feed conversion. The best value for the European productive efficiency index was observed at a dose of 514 g/ton of extract at 42 days of age.
Data availability statement
The raw data supporting the conclusions of this article will be made available by the authors, without undue reservation.
Ethics statement
The animal study was approved by Ethics Committee on the Use of Animals (CEUA/UFGD) under protocol number 02/2022. The study was conducted in accordance with the local legislation and institutional requirements.
Author contributions
VC: Conceptualization, Data curation, Formal analysis, Investigation, Methodology, Writing – original draft, Writing – review & editing. MB: Conceptualization, Data curation, Formal analysis, Methodology, Visualization, Writing – review & editing. BP: Writing – review & editing. LG: Investigation, Methodology, Writing – review & editing. JV: Investigation, Methodology, Writing – review & editing. RG: Investigation, Methodology, Writing – review & editing. FC: Investigation, Methodology, Writing – review & editing. ES: Data curation, Validation, Visualization, Writing – review & editing. CC: Investigation, Methodology, Validation, Writing – review & editing. CK: Conceptualization, Data curation, Funding acquisition, Investigation, Methodology, Resources, Supervision, Validation, Visualization, Writing – original draft, Writing – review & editing.
Funding
The author(s) declare that financial support was received for the research and/or publication of this article. This work was supported by Coordenação de Aperfeiçoamento de Pessoal de Nível Superior—Brasil (CAPES) and Fundação de Apoio ao Desenvolvimento do Ensino, Ciência e Tecnologia do Estado de Mato Grosso do Sul (FUNDECT)—Código Financeiro 001.
Conflict of interest
ES was employed by Kupono Assessoria e Consultoria.
The remaining authors declare that the research was conducted in the absence of any commercial or financial relationships that could be construed as a potential conflict of interest.
Generative AI statement
The authors declare that no Gen AI was used in the creation of this manuscript.
Publisher’s note
All claims expressed in this article are solely those of the authors and do not necessarily represent those of their affiliated organizations, or those of the publisher, the editors and the reviewers. Any product that may be evaluated in this article, or claim that may be made by its manufacturer, is not guaranteed or endorsed by the publisher.
References
1. Sihvo, HK, Immonen, K, and Puolanne, E. Myodegeneration with fibrosis and regeneration in the pectoralis major muscle of broilers. Vet Pathol. (2014) 51:619–23. doi: 10.1177/0300985813497488
2. Kuttappan, VA, Hargis, BM, and Owens, CM. White striping and woody breast myopathies in the modern poultry industry: a review. Poult Sci. (2016) 95:2724–33. doi: 10.3382/ps/pew216
3. Cruz, RFA, Vieira, SL, Kindlein, L, Kipper, M, Cemin, HS, and Rauber, SM. Occurrence of white striping and wooden breast in broilers fed grower and finisher diets with increasing lysine levels. Poult Sci. (2017) 96:501–10. doi: 10.3382/ps/pew310
4. Tasoniero, G, Zhuang, H, Gamble, GR, and Bowker, BC. Effect of spaghetti meat abnormality on broiler chicken breast meat composition and technological quality. Poult Sci. (2020) 99:1724–33. doi: 10.1016/j.psj.2019.10.069
5. Kuttappan, VA, Shivaprasad, HL, Shaw, DP, Valentine, BA, Hargis, BM, Clark, FD, et al. Pathological changes associated with white striping in broiler breast muscles. Poult Sci. (2013) 92:331–8. doi: 10.3382/ps.2012-02646
6. Petracci, M, Soglia, F, Madruga, M, Carvalho, L, Ida, E, and Estévez, M. Wooden-breast, white striping, and spaghetti meat: causes, consequences and consumer perception of emerging broiler meat abnormalities. Compr Rev Food Sci Food Saf. (2019) 18:565–83. doi: 10.1111/1541-4337.12431
7. Boerboom, G, van Kempen, T, Navarro-Villa, A, and Pérez-Bonilla, A. Unraveling the cause of white striping in broilers using metabolomics. Poult Sci. (2018) 97:3977–86. doi: 10.3382/ps/pey266
8. Papah, MB, Brannick, EM, Schmidt, CJ, and Abasht, B. Gene expression profiling of the early pathogenesis of wooden breast disease in commercial broiler chickens using RNA-sequencing. PLoS One. (2018) 13:e0207346. doi: 10.1371/journal.pone.0207346
9. Sihvo, HK, Airas, N, Lindén, J, and Puolanne, E. Pectoral vessel density and early ultrastructural changes in broiler chicken wooden breast myopathy. J Comp Pathol. (2018) 161:1–10. doi: 10.1016/j.jcpa.2018.04.002
10. Velleman, SG. Relationship of skeletal muscle development and growth to breast muscle myopathies: a review. Avian Dis. (2015) 59:525–31. doi: 10.1637/11223-063015-Review.1
11. Papah, MB, Brannick, EM, Schmidt, CJ, and Abasht, B. Evidence and role of phlebitis and lipid infiltration in the onset and pathogenesis of wooden breast disease in modern broiler chickens. Avian Pathol. (2017) 46:623–43. doi: 10.1080/03079457.2017.1339346
12. Sihvo, HK, Lindén, J, Airas, N, Immonen, K, Valaja, J, and Puolanne, E. Wooden breast myodegeneration of pectoralis major muscle over the growth period in broilers. Vet Pathol. (2017) 54:119–28. doi: 10.1177/0300985816658099
13. Abasht, B, Mutryn, MF, Michalek, RD, and Lee, WR. Oxidative stress and metabolic perturbations in wooden breast disorder in chickens. PLoS One. (2016) 11:e0153750. doi: 10.1371/journal.pone.0153750
14. Soglia, F, Mudalal, S, Babini, E, Di Nunzio, M, Mazzoni, M, Sirri, F, et al. Histology, composition, and quality traits of chicken pectoralis major muscle affected by wooden breast abnormality. Poult Sci. (2016) 95:651–9. doi: 10.3382/ps/pev353
15. Estevez, M, and Petracci, M. Benefits of magnesium supplementation to broiler subjected to dietary and heat stress: improved redox status, breast quality and decreased myopathy incidence. Antioxidants. (2019) 8:456. doi: 10.3390/antiox8100456
16. Abdel-Moneim, AME, Shehata, AM, Alzahrani, SO, Shafi, ME, Mesalam, NM, Taha, AE, et al. The role of polyphenols in poultry nutrition. J Anim Physiol Anim Nutr. (2020) 104:1851–66. doi: 10.1111/jpn.13455
17. Panche, AN, Diwan, AD, and Chandra, SR. Flavonoids: an overview. J Nutr Sci. (2016) 5:e47. doi: 10.1017/jns.2016.41
18. Gouveia, HJ, Urquiza-Martínez, MV, Manhães-de-Castro, R, Costa-de-Santana, BJ, Villarreal, JP, Mercado-Camargo, R, et al. Effects of the treatment with flavonoids on metabolic syndrome components in humans: a systematic review focusing on mechanisms of action. Int J Mol Sci. (2022) 23:8344. doi: 10.3390/ijms23158344
19. Surai, PF. Polyphenol compounds in the chicken/animal diet: from the past to the future. J Anim Physiol Anim Nutr. (2014) 98:19–31. doi: 10.1111/jpn.12070
20. Basiouni, S, Tellez-Isaias, G, Latorre, JD, Graham, BD, Petrone-Garcia, VM, El-Seedi, HR, et al. Anti-inflammatory and Antioxidative phytogenic substances against secret killers in poultry: current status and prospects. Vet Sci. (2023) 10:55. doi: 10.3390/vetsci10010055
21. Batiha, GES, Magdy Beshbishy, A, Wasef, L, Elewa, YH, Abd El-Hack, ME, Taha, AE, et al. Uncaria tomentosa (Willd. Ex Schult.) DC.: a review on chemical constituents and biological activities. Appl Sci. (2020) 10:2668. doi: 10.3390/app10082668
22. Choi, J, Liu, G, Goo, D, Wang, J, Bowker, B, Zhuang, H, et al. Effects of tannic acid supplementation on growth performance, gut health, and meat production and quality of broiler chickens raised in floor pens for 42 days. Front Physiol. (2022) 13:2661. doi: 10.3389/fphys.2022.1082009
23. Rostagno, HS, Albino, LFT, Hannas, MI, Donzele, JL, Sakomura, NK, Perazzo, FG, et al. Tabelas brasileiras para aves e suínos: composição de alimentos e exigências nutricionais de aves e suínos. Viçosa, MG: UFV (2017). 488 p.
24. Castilho, VAR, Komiyama, CM, Burbarelli, MFC, Fernandes, AM, Garcia, RG, Seno, LO, et al. Precision technologies for predictive diagnosis and study of the allometric growth of broiler chickens with breast myopathies. Br Poult Sci. (2022) 64:204–13. doi: 10.1080/00071668.2022.2128989
25. Kuttappan, VA, Brewer, VB, Apple, JK, Waldroup, PW, and Owens, CM. Influence of growth rate on the occurrence of white striping in broiler breast fillets. Poult Sci. (2012) 91:2677–85. doi: 10.3382/ps.2012-02259
26. Mudalal, S, Babini, E, Cavani, C, and Petracci, M. Quantity and functionality of protein fractions in chicken breast fillets affected by white striping. Poult Sci. (2014) 93:2108–16. doi: 10.3382/ps.2014-03911
27. Van Laack, RLJM, Liu, CH, Smith, MO, and Loveday, HD. Characteristics of pale, soft, exudative broiler breast meat. Poult Sci. (2000) 79:1057–61. doi: 10.1093/ps/79.7.1057
28. Hamm, R. Biochemistry of meat hydratation. Adv Food Res. (1960) 10:355–463. doi: 10.1016/S0065-2628(08)60141-X
29. Rasmussen, A. J., and Andersson, M. New method for determination of drip loss in pork muscles. In Proceedings 42nd international congress of meat science and technology, Lillehammer (pp. 286–287), 1–6. (1996).
30. Honikel, KO. Water-holding capacity of meat In: Muscle development of livestock animals: Physiology, genetics and meat quality. Wallingford UK: Springer (2004) 389–400.
31. Komiyama, CM, Mendes, AA, Takahashi, SE, Moreira, J, Borba, HBA, Leonel, FR, et al. Qualitative characteristics of products produced with pale and normal broiler chicken meat. Food Sci Technol. (2009) 29:38–45. doi: 10.1590/S0101-20612009000100007
32. Castilho, VARD, Komiyama, CM, Burbarelli, MFC, Fernandes, ARM, Garcia, RG, Barbosa, DK, et al. Techniques for in vivo assessment of myopathies in broiler chicken breasts using a biopsy as a support tool. Avian Pathol. (2021) 50:477–89. doi: 10.1080/03079457.2021.1970107
33. Kumaran, A. Antioxidant and free radical scavenging activity of an aqueous extract of Coleus aromaticus. Food Chem. (2006) 97:109–14. doi: 10.1016/j.foodchem.2005.03.032
34. Bligh, EG, and Dyer, WJ. A rapid method of total lipid extraction and purification. Can J Biochem Physiol. (1959) 37:911–7.
35. Maia, EL, and Rodriguez-Amaya, DB. Evaluation of a simple and inexpensive method for the methylation of fatty acid with lipids of various fish species. Rev Inst Adolfo Lutz. (1993) 53:982. doi: 10.53393/rial.1993.53.35982
36. Nelder, JA, and Wedderburn, RW. Generalized linear models. J Royal Stat Soc Series A. (1972) 135:370–84. doi: 10.2307/2344614
37. Huxley, J. Problems of relative growth. Found Nat History. (1993) 16:276–21. doi: 10.56021/9780801846595
38. Santos, FA, Komiyama, CM, Castilho Heiss, VA, Burbarelli, MF, Garcia, RG, Barbosa, DK, et al. Allometric coefficient in broilers and development of white striping and wooden breast myopathies. Czeh J Anim Sci. (2023) 68:212–21. doi: 10.17221/182/2022-CJAS
39. Jamroz, D, Wiliczkiewicz, A, Skorupińska, J, Orda, J, Kuryszko, J, and Tschirch, H. Effect of sweet chestnut tannin (SCT) on the performance, microbial status of intestine and histological characteristics of intestine wall in chickens. Br Poult Sci. (2009) 50:687–99. doi: 10.1080/00071660903191059
40. Zhou, Y, Mao, S, and Zhou, M. Effect of the flavonoid baicalein as a feed additive on the growth performance, immunity, and antioxidant capacity of broiler chickens. Poult Sci. (2019) 98:2790–9. doi: 10.3382/ps/pez071
41. Kubena, LF, Byrd, JA, Young, CR, and Corrier, DE. Effects of tannic acid on cecal volatile fatty acids and susceptibility to Salmonella typhimurium colonization in broiler chicks. Poult Sci. (2001) 80:1293–8. doi: 10.1093/ps/80.9.1293
42. Mašek, T, Starčević, K, and Mikulec, Ž. The influence of the addition of thymol, tannic acid or gallic acid to broiler diet on growth performance, serum malondialdehyde value and cecal fermentation. Eur Poultry Sci. (2014) 78:64. doi: 10.1399/eps.2014.64
43. Starčević, K, Krstulović, L, Brozić, D, Maurić, M, Stojević, Z, Mikulec, Ž, et al. Production performance, meat composition and oxidative susceptibility in broiler chicken fed with different phenolic compounds. J Sci Food Agric. (2015) 95:1172–8. doi: 10.1002/jsfa.6805
44. Cengiz, Ö, Köksal, BH, Tatlı, O, Sevim, Ö, Ahsan, U, Bilgili, SF, et al. Effect of dietary tannic acid supplementation in corn-or barley-based diets on growth performance, intestinal viscosity, litter quality, and incidence and severity of footpad dermatitis in broiler chickens. Livest Sci. (2017) 202:52–7. doi: 10.1016/j.livsci.2017.05.016
45. Daglia, M, Di Lorenzo, A, Seyed, FN, Zeliha, ST, and Seyed, MN. Polyphenols: well beyond the antioxidant capacity: gallic acid and related compounds as neuroprotective agents: you are what you eat! Curr Pharm Biotechnol. (2014) 15:362–72. doi: 10.2174/138920101504140825120737
46. Cione, E, La Torre, C, Cannataro, R, Caroleo, MC, Plastina, P, and Gallelli, L. Quercetin, epigallocatechin gallate, curcumin, and resveratrol: from dietary sources to human microRNA modulation. Molecules. (2019) 25:63. doi: 10.3390/molecules25010063
47. Sharifi-Rad, J, Quispe, C, Castillo, CMS, Caroca, R, Lazo-Vélez, MA, Antonyak, H, et al. Ellagic acid: a review on its natural sources, chemical stability, and therapeutic potential. Oxidative Med Cell Longev. (2022) 2022:3848084. doi: 10.1155/2022/3848084
48. Ticinesi, A, Nouvenne, A, Cerundolo, N, Parise, A, and Meschi, T. Accounting gut microbiota as the mediator of beneficial effects of dietary (poly) phenols on skeletal muscle in aging. Nutrients. (2023) 15:2367. doi: 10.3390/nu15102367
49. Nikawa, T, Ulla, A, and Sakakibara, I. Polyphenols and their effects on muscle atrophy and muscle health. Molecules. (2021) 26:4887. doi: 10.3390/molecules26164887
50. Lamy, E, Pinheiro, C, Rodrigues, L, Capela-Silva, F, Lopes, O, Tavares, S, et al. Determinants of tannin-rich food and beverage consumption: oral perception vs. psychosocial aspects In: CA Combs, editor. Tannins: Biochemistry, Food Sources and Nutritional Properties : Nova Science Publishers Inc (2016). 29–58. Available at: http://hdl.handle.net/10174/18018
51. Manach, C, Williamson, G, Morand, C, Scalbert, A, and Rémésy, C. Bioavailability and bioefficacy of polyphenols in humans. I. Review of 97 bioavailability studies. Am J Clin Nutr. (2005) 81:230S–42S. doi: 10.1093/ajcn/81.1.230S
52. Seeram, NP, Lee, R, and Heber, D. Bioavailability of ellagic acid in human plasma after consumption of ellagitannins from pomegranate (Punica granatum L.) juice. Clin Chim Acta. (2004) 348:63–8. doi: 10.1016/j.cccn.2004.04.029
53. Espín, JC, González-Barrio, R, Cerdá, B, López-Bote, C, Rey, AI, and Tomás-Barberán, FA. Iberian pig as a model to clarify obscure points in the bioavailability and metabolism of ellagitannins in humans. J Agric Food Chem. (2007) 55:10476–85. doi: 10.1021/jf0723864
54. D’Amico, D, Andreux, PA, Valdés, P, Singh, A, Rinsch, C, and Auwerx, J. Impact of the natural compound urolithin a on health, disease, and aging. Trends Mol Med. (2021) 27:687–99. doi: 10.1016/j.molmed.2021.04.009
55. García-Villalba, R, Giménez-Bastida, JA, Cortés-Martín, A, Ávila-Gálvez, MÁ, Tomás-Barberán, FA, Selma, MV, et al. Urolithins: a comprehensive update on their metabolism, bioactivity, and associated gut microbiota. Mol Nutr Food Res. (2022) 66:2101019. doi: 10.1002/mnfr.202101019
56. Liu, S, D’Amico, D, Shankland, E, Bhayana, S, Garcia, JM, Aebischer, P, et al. Effect of urolithin a supplementation on muscle endurance and mitochondrial health in older adults: a randomized clinical trial. JAMA Netw Open. (2022) 5:e2144279–9. doi: 10.1001/jamanetworkopen.2021.44279
57. Singh, A, D’Amico, D, Andreux, PA, Fouassier, AM, Blanco-Bose, W, Evans, M, et al. Urolithin a improves muscle strength, exercise performance, and biomarkers of mitochondrial health in a randomized trial in middle-aged adults. Cell Rep Med. (2022) 3:100633. doi: 10.1016/j.xcrm.2022.100633
58. Rodriguez, J, Pierre, N, Naslain, D, Bontemps, F, Ferreira, D, Priem, F, et al. Urolithin B, a newly identified regulator of skeletal muscle mass. J Cachexia Sarcopenia Muscle. (2017) 8:583–97. doi: 10.1002/jcsm.12190
59. Luca, SV, Macovei, I, Bujor, A, Miron, A, Skalicka-Woźniak, K, Aprotosoaie, AC, et al. Bioactivity of dietary polyphenols: the role of metabolites. Crit Rev Food Sci Nutr. (2020) 60:626–59. doi: 10.1080/10408398.2018.1546669
60. Biagi, E, Franceschi, C, Rampelli, S, Severgnini, M, Ostan, R, Turroni, S, et al. Gut microbiota and extreme longevity. Curr Biol. (2016) 26:1480–5. doi: 10.1016/j.cub.2016.04.016
61. Badal, VD, Vaccariello, ED, Murray, ER, Yu, KE, Knight, R, Jeste, DV, et al. The gut microbiome, aging, and longevity: a systematic review. Nutrients. (2020) 12:3759. doi: 10.3390/nu12123759
62. Bressa, C, Bailén-Andrino, M, Pérez-Santiago, J, González-Soltero, R, Pérez, M, Montalvo-Lominchar, MG, et al. Differences in gut microbiota profile between women with active lifestyle and sedentary women. PLoS One. (2017) 12:e0171352. doi: 10.1371/journal.pone.0171352
63. Prokopidis, K, Giannos, P, Kirwan, R, Ispoglou, T, Galli, F, Witard, OC, et al. Impact of probiotics on muscle mass, muscle strength and lean mass: a systematic review and meta-analysis of randomized controlled trials. J Cachexia Sarcopenia Muscle. (2023) 14:30–44. doi: 10.1002/jcsm.13132
64. Rodriguez, J, Caille, O, Ferreira, D, and Francaux, M. Pomegranate extract prevents skeletal muscle of mice against wasting induced by acute TNF-α injection. Mol Nutr Food Res. (2017) 61:1600169. doi: 10.1002/mnfr.201600169
65. Chen, P, Chen, F, Lei, J, and Zhou, B. Gut microbial metabolite urolithin B attenuates intestinal immunity function in vivo in aging mice and in vitro in HT29 cells by regulating oxidative stress and inflammatory signalling. Food Funct. (2021) 12:11938–55. doi: 10.1039/d1fo02440j
66. Xing, T, Pan, X, Zhang, L, and Gao, F. Hepatic oxidative stress, apoptosis, and inflammation in broiler chickens with wooden breast myopathy. Front Physiol. (2021) 12:659777. doi: 10.3389/fphys.2021.659777
67. Coletti, D, Moresi, V, Adamo, S, Molinaro, M, and Sassoon, D. Tumor necrosis factor-α gene transfer induces cachexia and inhibits muscle regeneration. Genesis. (2005) 43:120–8. doi: 10.1002/gene.20160
68. Cohen, TV, Many, GM, Fleming, BD, Gnocchi, VF, Ghimbovschi, S, Mosser, DM, et al. Upregulated IL-1β in dysferlin-deficient muscle attenuates regeneration by blunting the response to pro-inflammatory macrophages. Skelet Muscle. (2015) 5:1–21. doi: 10.1186/s13395-015-0048-4
69. Galano, A, Francisco Marquez, M, and Pérez-González, A. Ellagic acid: an unusually versatile protector against oxidative stress. Chem Res Toxicol. (2014) 27:904–18. doi: 10.1021/tx500065y
70. Karakurt, S, Semiz, A, Celik, G, Gencler-Ozkan, AM, Sen, A, and Adali, O. Contribution of ellagic acid on the antioxidant potential of medicinal plant Epilobium hirsutum. Nutr Cancer. (2016) 68:173–83. doi: 10.1080/01635581.2016.1115092
71. Gontijo, AV, Da, GSA, Koga-Ito, CY, and Salvador, MJ. Biopharmaceutical and antifungal properties of ellagic acid-cyclodextrin using an in vitro model of invasive candidiasis. Future Microbiol. (2019) 14:957–67. doi: 10.2217/fmb-2019-0107
72. Puiggròs, F, Llópiz, N, Ardévol, A, Bladé, C, Arola, L, and Salvadó, MJ. Grape seed procyanidins prevent oxidative injury by modulating the expression of antioxidant enzyme systems. J Agric Food Chem. (2005) 53:6080–6. doi: 10.1021/jf050343m
73. Hussain, T, Tan, B, Yin, Y, Blachier, F, Tossou, MC, and Rahu, N. Oxidative stress and inflammation: what polyphenols can do for us? Oxidative Med Cell Longev. (2016) 2016:797. doi: 10.1155/2016/7432797
74. Clark, DL, and Velleman, SG. Spatial influence on breast muscle morphological structure, myofiber size, and gene expression associated with the wooden breast myopathy in broilers. Poult Sci. (2016) 95:2930–45. doi: 10.3382/ps/pew243
75. Nwachukwu, ID, Sarteshnizi, RA, Udenigwe, CC, and Aluko, RE. A concise review of current in vitro chemical and cell-based antioxidant assay methods. Molecules. (2021) 26:4865. doi: 10.3390/molecules26164865
76. Baliyan, S, Mukherjee, R, Priyadarshini, A, Vibhuti, A, Gupta, A, Pandey, RP, et al. Determination of antioxidants by DPPH radical scavenging activity and quantitative phytochemical analysis of Ficus religiosa. Molecules. (2022) 27:1326. doi: 10.3390/molecules27041326
77. Sieniawska, E. Activities of tannins–from in vitro studies to clinical trials. Nat Prod Commun. (2015) 10:1934578X1501001118. doi: 10.1177/1934578X1501001118
78. Hoyos-Martínez, PL, Merle, J, Labidi, J, and Charrier–El Bouhtoury, F. Tannins extraction: a key point for their valorization and cleaner production. J Clean Prod. (2019) 206:1138–55. doi: 10.1016/j.jclepro.2018.09.243
79. Liu, S, Wang, K, Lin, S, Zhang, Z, Cheng, M, Hu, S, et al. Comparison of the effects between tannins extracted from different natural plants on growth performance, antioxidant capacity, immunity, and intestinal Flora of broiler chickens. Antioxidants. (2023) 12:441. doi: 10.3390/antiox12020441
80. Zeng, X, Du, Z, Sheng, Z, and Jiang, W. Characterization of the interactions between banana condensed tannins and biologically important metal ions (Cu2+, Zn2+ and Fe2+). Food Res Int. (2019) 123:518–28. doi: 10.1016/j.foodres.2019.04.064
81. Baldi, G, Soglia, F, Laghi, L, Tappi, S, Rocculi, P, Tavaniello, S, et al. Comparison of quality traits among breast meat affected by current muscle abnormalities. Food Res Int. (2019) 115:369–76. doi: 10.1016/j.foodres.2018.11.020
82. Chatterjee, D, Zhuang, H, Bowker, BC, Sanchez-Brambila, G, and Rincon, AM. Instrumental texture characteristics of broiler pectoralis major with the wooden breast condition. Poult Sci. (2016) 95:2449–54. doi: 10.3382/ps/pew204
83. Tijare, VV, Yang, FL, Kuttappan, VA, Alvarado, CZ, Coon, CN, and Owens, CM. Meat quality of broiler breast fillets with white striping and woody breast muscle myopathies. Poult Sci. (2016) 95:2167–73. doi: 10.3382/ps/pew129
84. Kuttappan, VA, Owens, CM, Coon, C, Hargis, BM, and Vazquez-Anon, M. Incidence of broiler breast myopathies at 2 different ages and its impact on selected raw meat quality parameters. Poult Sci. (2017) 96:3005–9. doi: 10.3382/ps/pex072
85. Brambila, GS, Bowker, BC, Chatterjee, D, and Zhuang, H. Descriptive texture analyses of broiler breast fillets with the wooden breast condition stored at 4 C and–20 C. Poult Sci. (2018) 97:1762–7. doi: 10.3382/ps/pew327
86. Joo, ST, Kim, GD, Hwang, YH, and Ryu, YC. Control of fresh meat quality through manipulation of muscle fiber characteristics. Meat Sci. (2013) 95:828–36. doi: 10.1016/j.meatsci.2013.04.044
87. Jachimowicz, K, Winiarska-Mieczan, A, and Tomaszewska, E. The impact of herbal additives for poultry feed on the fatty acid profile of meat. Animals. (2022) 12:1054. doi: 10.3390/ani12091054
88. Minieri, S, Buccioni, A, Serra, A, Galigani, I, Pezzati, A, Rapaccini, S, et al. Nutritional characteristics and quality of eggs from laying hens fed on a diet supplemented with chestnut tannin extract (Castanea sativa miller). Br Poult Sci. (2016) 57:824–32. doi: 10.1080/00071668.2016.1216944
89. Liu, HS, Mahfuz, SU, Wu, D, Shang, QH, and Piao, XS. Effect of chestnut wood extract on performance, meat quality, antioxidant status, immune function, and cholesterol metabolism in broilers. Poult Sci. (2020) 99:4488–95. doi: 10.1016/j.psj.2020.05.053
90. Furlan, AL, Jobin, ML, Pianet, I, Dufourc, EJ, and Géan, J. Flavanol/lipid interaction: a novel molecular perspective in the description of wine astringency & bitterness and antioxidant action. Tetrahedron. (2015) 71:3143–7. doi: 10.1016/j.tet.2014.07.106
91. Tebib, K, Bitri, L, Besançon, P, and Rouanet, JM. Polymeric grape seed tannins prevent plasma cholesterol changes in high-cholesterol-fed rats. Food Chem. (1994) 49:403–6. doi: 10.1016/0308-8146(94)90012-4
92. Fang, J, Zeng, L, He, Y, Liu, X, Zhang, T, and Wang, Q. Effects of dietary tannic acid on obesity and gut microbiota in C57BL/6J mice fed with high-fat diet. Food Secur. (2022) 11:3325. doi: 10.3390/foods11213325
93. Molino, S, Francino, MP, and Henares, JÁR. Why is it important to understand the nature and chemistry of tannins to exploit their potential as nutraceuticals? Food Res Int. (2023) 173:113329. doi: 10.1016/j.foodres.2023.113329
94. Druart, C, Bindels, LB, Schmaltz, R, Neyrinck, AM, Cani, PD, Walter, J, et al. Ability of the gut microbiota to produce PUFA-derived bacterial metabolites: proof of concept in germ-free versus conventionalized mice. Mol Nutr Food Res. (2015) 59:1603–13. doi: 10.1002/mnfr.201500014
95. Blanchard, H, Pédrono, F, Boulier-Monthéan, N, Catheline, D, Rioux, V, and Legrand, P. Comparative effects of well-balanced diets enriched in α-linolenic or linoleic acids on LC-PUFA metabolism in rat tissues. Prostaglandins Leukot Essent Fat Acids. (2013) 88:383–9. doi: 10.1016/j.plefa.2013.03.006
96. Bruin, M, Smeulders, MJ, Kreulen, M, Huijing, PA, and Jaspers, RT. Intramuscular connective tissue differences in spastic and control muscle: A mechanical and histological study. Plos one. (2014) 9:e101038.
97. SAS Institute Inc. SAS Institute white paper. “Measuring and Managing Human Capital. Intelligence ”. (2003). Available at: http://www.sas.com/en_us/whitepapers.html
Keywords: condensed tannins, flavonoids, hydrolyzable tannins, meat quality, oxidative stress, pectoral myopathies
Citation: Castilho Heiss VAR, Burbarelli MFC, Przybulinski BB, Garcia LC, Vieira JRRF, Garcia RG, Caldara FR, Santin E, Cardoso CAL and Komiyama CM (2025) Polyphenol blend enhances zootechnical performance, improves meat quality, and reduces the severity of wooden breast in broiler chickens. Front. Vet. Sci. 12:1584897. doi: 10.3389/fvets.2025.1584897
Edited by:
Shereen Basiouni, Johannes Gutenberg University Mainz, GermanyReviewed by:
Alius Pockevičius, Lithuanian University of Health Sciences, LithuaniaWeber Robazza, Santa Catarina State University, Brazil
Copyright © 2025 Castilho Heiss, Burbarelli, Przybulinski, Garcia, Vieira, Garcia, Caldara, Santin, Cardoso and Komiyama. This is an open-access article distributed under the terms of the Creative Commons Attribution License (CC BY). The use, distribution or reproduction in other forums is permitted, provided the original author(s) and the copyright owner(s) are credited and that the original publication in this journal is cited, in accordance with accepted academic practice. No use, distribution or reproduction is permitted which does not comply with these terms.
*Correspondence: Vivian Aparecida Rios de Castilho Heiss, dml2aWFuY2FzdGlsaG9AbGl2ZS5jb20=