- 1Modern Research Center for Traditional Chinese Medicine, School of Chinese Materia Medica, Beijing University of Chinese Medicine, Beijing, China
- 2Department of Molecular Orthopaedics, Beijing Institute of Traumatology and Orthopaedics, Beijing Jishuitan Hospital, Beijing, China
Hepatocellular carcinoma (HCC) is one of the most prevalent malignancies, which ranks the third leading cause of cancer-related death worldwide. The screening of anti-HCC drug with high efficiency and low toxicity from traditional Chinese medicine (TCM) has attracted more and more attention. As a TCM, Chinese dragon’s blood has been used for the treatment of cardiovascular illness, gynecological illness, skin disorder, otorhinolaryngological illness, and diabetes mellitus complications for many years. However, the anti-tumor effect and underlying mechanisms of Chinese dragon’s blood remain ill-defined. Herein we have revealed that Chinese dragon’s blood EtOAc extract (CDBEE) obviously suppressed the growth of human hepatoma HepG2 and SK-HEP-1 cells. Moreover, CDBEE inhibited the migration and invasion of HepG2 and SK-HEP-1 cells. Additionally, CDBEE displayed good in vitro anti-angiogenic activity. Importantly, CDBEE treatment significantly blunted the oncogenic capability of HepG2 cells in nude mice. Mechanistically, CDBEE inhibited Smad3 expression in human hepatoma cells and tumor tissues from nude mice. Using RNA interference, we demonstrated that CDBEE exerted anti-hepatoma activity partially through down-regulation of Smad3, one of major members in TGF-β/Smad signaling pathway. Therefore, CDBEE may be a promising candidate drug for HCC treatment, especially for liver cancer with aberrant TGF-β/Smad signaling pathway.
Introduction
Cancer is the second leading cause of death worldwide and seriously threatens human health (Bray et al., 2018). Hepatocellular carcinoma (HCC) is one of the most common malignant tumors in the world, which ranks the third in cancer mortality (Ghouri et al., 2017). The research and development of anti-HCC drug is urgently needed. The anti-tumor effects of traditional Chinese medicine (TCM) has attracted increasing attention in recent years (Guo et al., 2015b; Xu et al., 2015; Xiang et al., 2019).
Chinese dragon’s blood is the red resin of Dracaena cochinchinensis (Lour.) S. C. Chen (Pang et al., 2016). As a TCM, Chinese dragon’s blood has been used for the treatment of several types of diseases in China for many years, including cardiovascular illness, gynecological illness, skin disorder, otorhinolaryngological illness, and diabetes mellitus complications (Yuan et al., 2013). It has been reported that the 75% ethanol extract from Chinese dragon’s blood suppressed cell proliferation and promoted apoptosis in human cholangiocarcinoma cells (Wen et al., 2016).
TGF-β/Smad pathway plays a critical role in proliferation, apoptosis, angiogenesis, and metastasis in cancer (Massague et al., 2000; Hata and Chen, 2016). Accumulating evidence has shown that TGF-β/Smad pathway is frequently hyperactivation in HCC (Giannelli et al., 2014; Yang et al., 2016b; Yoshida et al., 2018). Smad3, one of members in TGF-β/Smad pathway, is reported to be an important pro-oncogenic gene in cancer growth (Lu et al., 2007; Millet and Zhang, 2007; Tang et al., 2017). Thus, it is of significance to find anti-tumor drugs targeting Smad3 or TGF-β/Smad pathway from TCM.
In this study, it was first reported that Chinese dragon’s blood EtOAc extract (CDBEE) displayed anti-hepatoma activity. We demonstrated that CDBEE suppressed the growth and metastasis of human hepatoma HepG2 and SK-HEP-1 cells in vitro, and showed good anti-angiogenic activity. Moreover, CDBEE inhibited the tumorigenesis of human hepatoma cells in nude mouse xenografts. Intriguingly, suppression of TGF-β/Smad pathway by downregulating Smad3 may be partially responsible for the inhibition of proliferation and metastasis of human hepatoma cells induced by CDBEE.
Materials and Methods
Reagents and Antibodies
Dulbecco’s Modified Eagle’s Medium (DMEM), fetal bovine serum (FBS), penicillin-streptomycin mixed solution, 0.25% trypsin, Matrigel, and Transwell chambers were obtained from Corning Life Sciences (Corning, NY, USA). Opti-MEM medium and Lipofectamine 2000 were from Invitrogen (Invitrogen, Carlsbad, CA). Hoechst 33258 was from Biyuntian Biotechnology (Shanghai, China). DMSO was from Sigma-Aldrich (St. Louis, MO, USA). The enhanced chemiluminescence (ECL) was obtained from GE Healthcare (Pittsburgh, PA, USA). β−actin (sc-47778) antibody was purchased from Santa Cruz Biotechnology (Santa Cruz, CA, USA). p38 (cat. no. 8690T), p-p38 (cat. no. 4511T), ERK (cat. no. 4695T), p-ERK (cat. no. 4370T), N-cadherin (13116T), Slug (9585T), P21 (2947T), CyclinD1 (2978T), and Smad3 (9523T) antibodies were purchased from Cell Signaling Technology (Danvers, MA, USA).
Preparation of CDBEE
The original medicinal materials of the Chinese dragon’s blood, which is the red resins of Dracaena cochinchinensis (Lour.) S.C.Chen, were purchased from Guangxi University of Chinese Medicine Pharmaceutical Factory (Guangxi, China, 20120404). The Chinese dragon’s blood (950 g) was refluxed with petroleum ether (8 L × 3, 2 h each), and then refluxed with ethyl acetate using the same method to produce Chinese dragon’s blood EtOAc extract (600 g, ethyl acetate fraction). The extraction yield of Chinese dragon’s blood EtOAc extract was 63.16%. The chemical composition analysis of CDBEE was attempted using HPLC-DAD-IT-TOF-MS (Supplementary Figure S1). CDBEE was dissolved in DMSO to obtain a 40 mg/ml stock solution, then stored at −20˚C for short-term use. The sample of CDBEE is stored at the Modern Research Center for TCM, School of Chinese Materia Medica, Beijing University of Chinese Medicine.
Cell Culture
Human hepatoma cell lines HepG2 and SK-HEP-1 were obtained from American Type Culture Collection. Human umbilical vein endothelial cells (HUVECs) were obtained from the Cell Culture Center of the Institute of Basic Medical Sciences of the Chinese Academy of Medical Sciences (Beijing, China). Cells were maintained in DMEM containing 10% FBS and 1% penicillin-streptomycin at 37°C and 5% CO2.
Cell Viability Assay
Cells were seeded in 96-well plate at a density of 2,500–3,500 cells/well. Twenty-four hours later, cells were treated with CDBEE at the indicated concentrations for different time. Then MTT was added into the 96-well plate and incubated at 37˚C for 4 h. Next 150 μl of DMSO was added into each well and gently shaked for 10 min. Finally, the optical density was measured with a microplate reader at 490 nm.
Flow Cytometry Analyses
HepG2, SK-HEP-1, and HUVECs were seeded in six-well plates. Twenty-four hours later, cells were treated with CDBEE at the indicated concentrations for 48 h. Next cells were collected for apoptosis analysis by using an Annexin V-FITC apoptosis detection kit (BD Pharmingen™) according to the manufacturer’s instructions described previously (Yang et al., 2016a).
Hoechst Staining
HepG2, SK-HEP-1, and HUVECs were seeded in six-well plates. Twenty-four hours later, cells were treated with CDBEE at the indicated concentrations for 48 h. Then cells were washed twice with PBS and fixed with 4% paraformaldehyde for 12 min, and stained with Hoechst 33258 for 30 min at room temperature in the dark. Finally, cells were observed under an inverted fluorescence microscope (Leica Microsystems GmbH).
Wound Healing Assay
HepG2, SK-HEP-1, and HUVECs were seeded in 12-well plates. The scratch assay was performed as described previously (Hu et al., 2016). The migration distances were analyzed quantitatively.
Cell Invasion Assay
HepG2, SK-HEP-1, and HUVECs cultured in serum-free medium for 12 h were subjected to Transwell assay described previously (Hu et al., 2016). The number of successfully invaded cells was analyzed quantitatively.
Tube Formation Assay
HUVECs were seeded in a six-well plate. Twenty-four hours later, cells were treated with CDBEE at the indicated concentrations for 24 h. Matrigel was added into 96-well plate (70 μl/well) and maintained at 37°C for 30 min. HUVECs were collected and resuspended with complete medium at a density of 1× 105 cells/ml, and then 200 μl of the medium was added into 96-well plate. Next the plate was incubated at 37°C for 10 h. The microtubule structure was observed and photographed on five random fields under an inverted microscope.
Western Blotting
HepG2 or SK-HEP-1 cells were treated with CDBEE at the indicated concentrations for 24 h and harvested with lysis buffer (10 mM Tris (pH 6.8), 2% SDS, 10% glycerol, 100 mM DTT), then boiled at 98˚C for 10 min. The protein levels were detected by immunoblotting described previously (Hu et al., 2015).
RNA Sequence and Data Analysis
Total RNA was extracted from HepG2 cells treated with or without CDBEE (40 μg/ml) for 24 h by using E.Z.N.A.® Total RNA Kit I (Omega Bio-Tek, Norcross, GA, USA) according to the manufacturer’s protocol. RNA sequencing was performed on the Illumina Hiseq x Ten by Shanghai Biotechnology Corporation (Shanghai, China). Data analysis was conducted using the Hisat2 and Stringtie software.
Quantitative Real-Time PCR
Total RNA was extracted from HepG2 or SK-HEP-1 cells treated with or without CDBEE at the indicated concentrations for 24 h by using E.Z.N.A.® Total RNA Kit I (Omega Bio-Tek, Norcross, GA, USA), and then was converted into cDNA by using the PrimeScript RT Reagent Kit (TaKaRa, Dalian, China) according to manufacturer’s protocol. Four μl of cDNA was used as a template for the quantitative PCR by using the TransStart Tip Green qPCR Kit (TransGen Biotech, Beijing, China). The primer sequences were as follows:
Smad3 forward: 5′-GCGTGCGGCTCTACTACATC-3′, Smad3 reverse: 5′-GCACATTCGGGTCAACTGGTA-3′; GAPDH forward: 5′-TCAAGAAGGTGGTGAAGCAG-3′, GAPDH reverse: 5′-TCGCTGTTGAAGTCAGAGGA-3′.
RNA Interference
Smad3 siRNAs (sense, 5′-GCCUGGUCAAGAAACUCAATT-3′) and negative control siRNAs (sense, 5′-UUCUCCGAACGUGUCACGUTT-3′) oligonucleotides were synthesized by Suzhou GenePharma Co., Ltd. (Suzhou, China). HepG2 or SK-HEP-1 cells were seeded in six-well plates. Next day cells were transfected with siRNAs against Smad3 with Lipofectamine 2000 according to the manufacturer’s protocol.
Experimental Animal
The male BALB/c nude mice (4–5 weeks old) were purchased from Beijing Vital River Laboratory Animal Technology Co, Ltd. A total of 2×106 HepG2 cells in 200 μl of DMEM were subcutaneously inoculated into the right posterior back region of nude mouse. The nude mice were randomized into three different groups: (i) PBS, once daily, intragastric administration; (ii) 250 mg/kg CDBEE, once daily, intragastric administration; (iii) 30 mg/kg 5-FU, three times per week, intraperitoneal injection. Tumor growth was measured and normalized to the initial volumes. The animal experiment was performed in accordance with guidelines for the use and care of animals approved by the Beijing University of Chinese Medicine Committee of Ethics. The subcutaneous tumor model was established as described previously (Sun et al., 2011).
Immunohistochemistry (IHC) Analysis
Immunohistochemical staining was performed as described previously (Jin et al., 2017). In brief, tumor tissues of nude mice treated with or without CDBEE were fixed in 4% paraformaldehyde and were embedded in paraffin. And then the paraffin sections were subjected to hematoxylin and eosin (H&E) staining and immunohistochemical staining of indicated proteins.
Statistics
Data are presented as mean ± SEM from three independent experiments and differences between two groups are compared with the two-tailed Student t-test in GraphPad Prism 5.0 software. P < 0.05 is considered to be statistically significant.
Results
CDBEE Suppressed the Proliferation and Induced the Apoptosis in Human Hepatoma HepG2 and SK-HEP-1 Cells
To measure the effects of CDBEE on the proliferation of HepG2 and SK-HEP-1 cells, we treated cells with CDBEE at the concentrations of 0, 10, 20, 40, and 80 μg/ml for 24, 48, and 72 h, respectively. CDBEE treatment significantly inhibited the cell viability of HepG2 and SK-HEP-1 cells in a time- and dose-dependent manner (Figure 1A), and the IC50 values of CDBEE for 48 h in HepG2 and SK-HEP-1 cells were 27.84 and 32.06 μg/ml, respectively. Subsequently, we investigated the effect of CDBEE on apoptosis of human hepatoma cells by using Hoechst staining assay and flow cytometry analysis. Hoechst 33258 dye could be used for staining of apoptotic cells (Guo et al., 2015a). The nuclei of HepG2 and SK-HEP-1 cells treated with CDBEE exhibited more bright blue fluorescence (Figure 1B), indicating that the apoptosis of HepG2 and SK-HEP-1 cells was promoted by CDBEE. Moreover, the result of flow cytometry analysis showed that CDBEE treatment significantly increased the apoptosis rate of HepG2 and SK-HEP-1 cells (Figure 1C). The apoptosis rate of HepG2 and SK-HEP-1 cells exposed to CDBEE (40 μg/ml) were (35.87 ± 2.05) % and (33.93 ± 2.34) %, respectively. The appearance of shear bands of PARP is considered as the main marker of apoptotic cells (Soldani and Scovassi, 2002). Immunoblotting results showed that the protein level of cleaved PARP was upregulated in HepG2 and SK-HEP-1 cells treated with CDBEE (Figure 1D). Mitogen activated protein kinases (MAPKs), including c-Jun N-terminal kinase (JNK), p38, and extracellular signal-regulated kinase (ERK), play a crucial role in apoptosis induced by various cellular stresses or chemotherapeutic agents (Johnson and Lapadat, 2002; Dhillon et al., 2007). We found that CDBEE upregulated MAPK signaling pathway, indicated by the increased phosphorylation of ERK and p38 in HepG2 and SK-HEP-1 cells (Supplementary Figure S2). In addition, we also investigated the effect of CDBEE on the cell cycle of HepG2 and SK-HEP-1 cells by flow cytometry analysis. As shown in Figure 1E, CDBEE induced G2/M cell cycle arrest of HepG2 and SK-HEP-1 cells. Furthermore, we demonstrated that CDBEE treatment increased P21 expression and reduced CyclinD1 expression in HepG2 and SK-HEP-1 cells (Figure 1F).
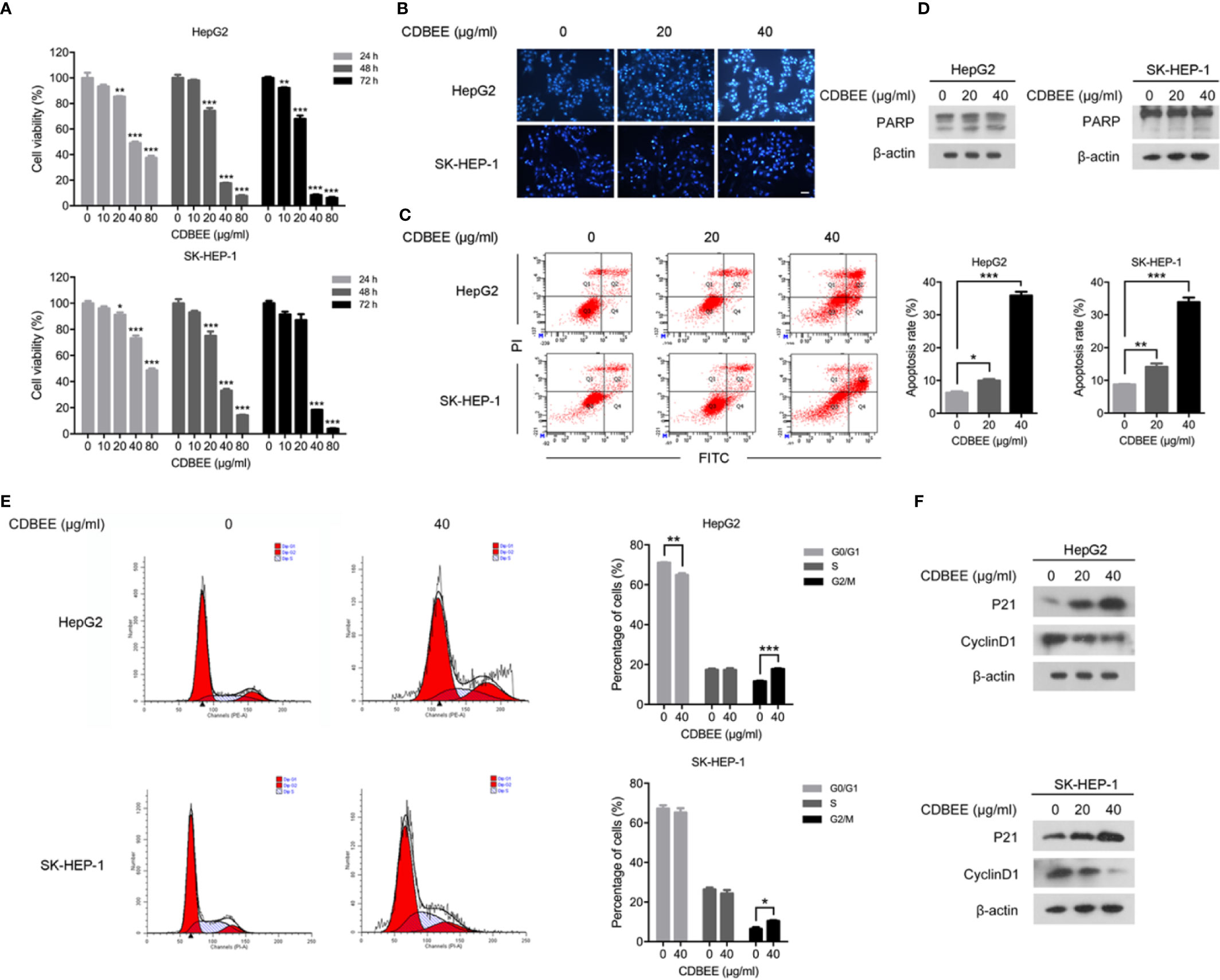
Figure 1 Chinese dragon’s blood EtOAc extract (CDBEE) treatment led to inhibition of proliferation and induction of apoptosis in HepG2 and SK-HEP-1 cells. (A) HepG2 or SK-HEP-1 cells were treated with CDBEE at the concentrations of 0, 10, 20, 40, and 80 μg/ml for 24, 48, and 72 h, respectively. The cell viability was evaluated by the MTT assay. (B) HepG2 or SK-HEP-1 cells treated with CDBEE at the concentrations of 0, 20, and 40 μg/ml for 48 h were stained with Hoechst 33258. Representative images are presented (100×). (C) HepG2 or SK-HEP-1 cells treated with CDBEE at the concentrations of 0, 20, and 40 μg/ml for 48 h were subjected to apoptosis analysis by flow cytometry. (D) Total cell lysates harvested from HepG2 or SK-HEP-1 cells treated with CDBEE at the concentrations of 0, 20, and 40 μg/ml for 24 h were subjected to immunoblotting. (E) Cell cycle distribution of HepG2 or SK-HEP-1 cells treated with CDBEE (40 μg/ml) for 48 h were evaluated by flow cytometry. (F) Total cell lysates harvested from HepG2 or SK-HEP-1 cells treated with CDBEE at the concentrations of 0, 20, and 40 μg/ml for 24 h were subjected to immunoblotting. *P < 0.05, **P < 0.01, ***P < 0.001.
CDBEE Inhibited the Metastatic Potential of HepG2 and SK-HEP-1 Cells
We explored the effect of CDBEE on the metastatic ability of human hepatoma cells in vitro. Wound healing assay showed that CDBEE treatment reduced the migration distance of HepG2 and SK-HEP-1 cells (Figure 2A), indicating that CDBEE inhibited the migration capacity of HepG2 and SK-HEP-1 cells. Moreover, Transwell assay revealed that the number of HepG2 and SK-HEP-1 cells invading through the Matrigel-coated membrane was significantly decreased in the presence of CDBEE, suggesting that CDBEE suppressed the invasive capacity of HepG2 and SK-HEP-1 cells (Figure 2B). Additionally, we explored the effect of CDBEE on epithelial-mesenchymal transition (EMT) in HepG2 and SK-HEP-1 cells. Immunoblotting results showed that CDBEE reduced the expression of mesenchymal markers N-cadherin and Slug in HepG2 and SK-HEP-1 cells (Figure 2C). Thus, CDBEE suppressed the metastatic capacity of HepG2 and SK-HEP-1 cells via inhibiting EMT.
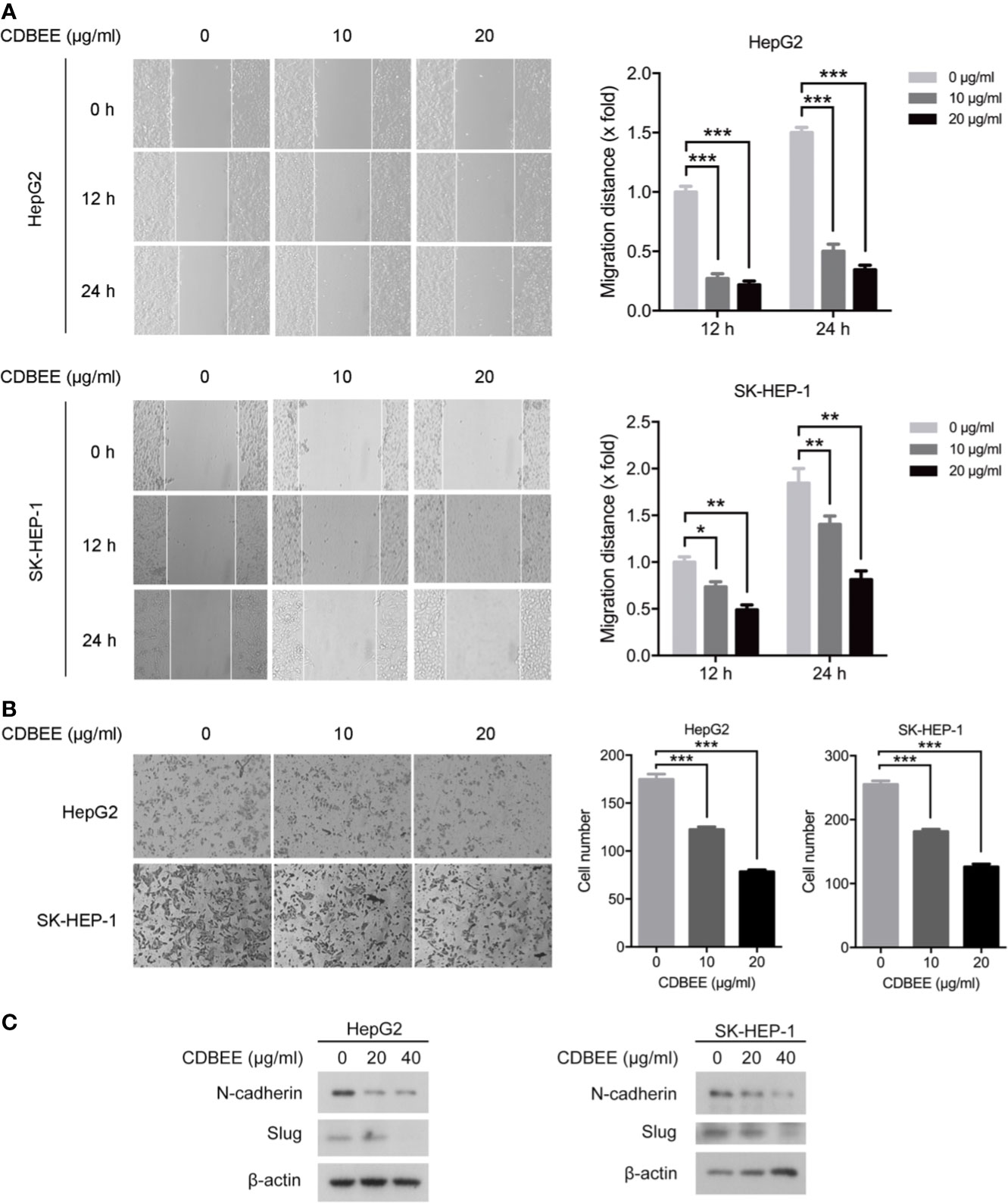
Figure 2 Chinese dragon’s blood EtOAc extract (CDBEE) inhibited the metastatic potential of HepG2 and SK-HEP-1 cells. (A) HepG2 or SK-HEP-1 cells treated with CDBEE at the concentrations of 0, 10, and 20 μg/ml were subjected to the scratch assay and observed at 0, 12, and 24 h, respectively. Left panel: representative images (100×); right panel: quantitative data. (B) HepG2 or SK-HEP-1 cells treated with CDBEE at the concentrations of 0, 10, and 20 μg/ml for 24 h were subjected to cell invasion assay. Left panel: representative images (100×); right panel: quantitative data. (C) Total cell lysates harvested from HepG2 or SK-HEP-1 cells treated with CDBEE at the concentrations of 0, 20, and 40 μg/ml for 24 h were subjected to immunoblotting. *P < 0.05, **P < 0.01, ***P < 0.001.
Anti-Angiogenic Activity of CDBEE
Angiogenesis plays a crucial role in cancer development (Carmeliet and Jain, 2000). Angiogenesis is a complicated process involving multiple steps, including the degradation of vascular basement membrane, the proliferation and migration of vascular endothelial cells, and the formation of new vascular networks (Adair and Montani, 2010). To investigate the effect of CDBEE on angiogenesis, we first carried out cell proliferation assay on HUVECs. CDBEE apparently inhibited the proliferation of HUVECs (Figure 3A). Next, Hoechst staining assay and flow cytometry analysis showed that CDBEE induced apoptosis of HUVECs (Figure 3B). Wound healing assay was used to evaluate the migration ability of HUVECs in vitro. The migration distance of HUVECs was decreased in response to CDBEE treatment (Figure 3C). In addition, Transwell assay revealed that CDBEE dramatically suppressed the invasive capability of HUVECs (Figure 3D). Endothelial cells can form tube-like structures on Matrigel basement membrane matrix, which could serve as an in vitro model to explore the anti-angiogenic activity of drugs (Nam et al., 2001; Su et al., 2016). CDBEE markedly blocked the formation of tube-like structures (Figure 3E). Taken together, CDBEE exerts good anti-angiogenic activity.
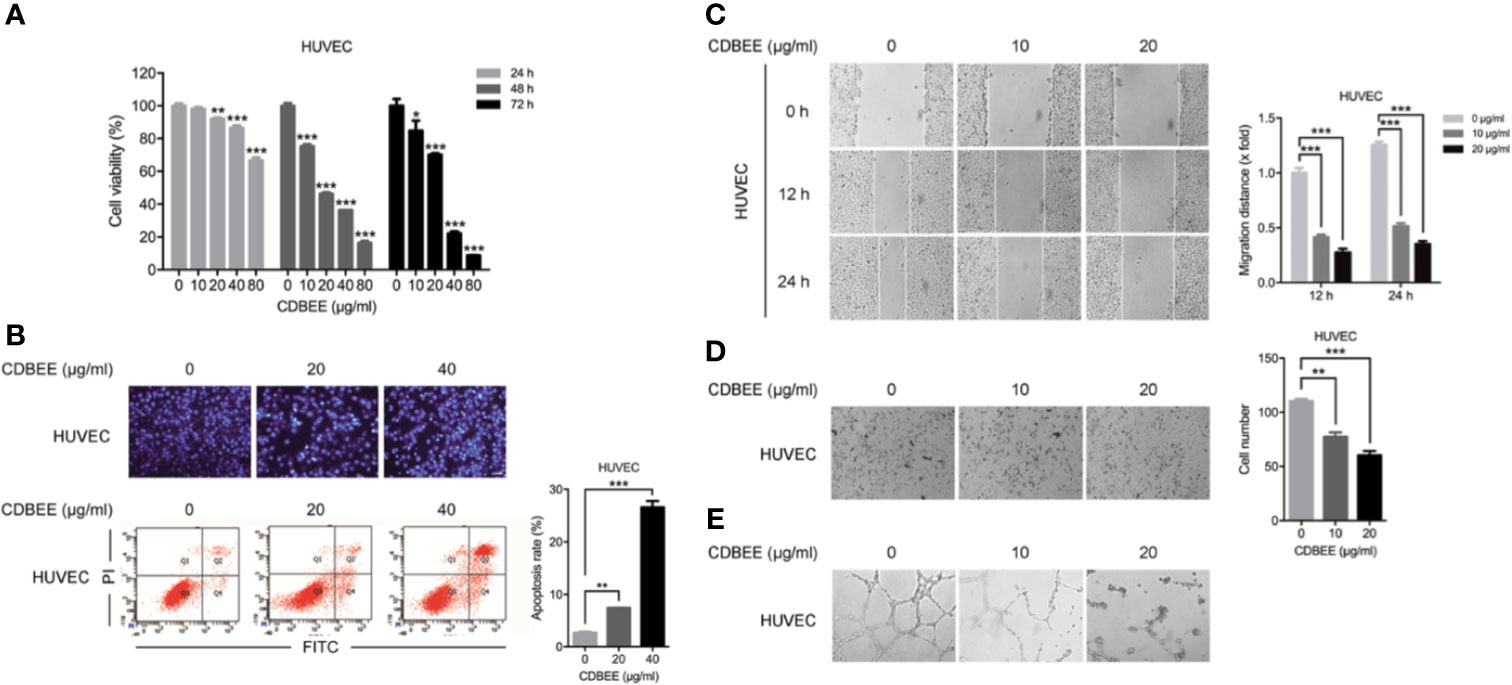
Figure 3 Anti-angiogenic activity of Chinese dragon’s blood EtOAc extract (CDBEE). (A) Human umbilical vein endothelial cells (HUVECs) were treated with CDBEE at the concentrations of 0, 10, 20, 40, and 80 μg/ml for 24, 48, and 72 h, respectively. The cell viability was evaluated by the MTT assay. (B) HUVECs treated with CDBEE at the concentrations of 0, 20, and 40 μg/ml for 48 h were stained with Hoechst 33258 (upper panel) and subjected to apoptosis analysis by flow cytometry (lower panel). (C) HUVECs treated with CDBEE at the concentrations of 0, 10, and 20 μg/ml were subjected to the scratch assay and observed at 0, 12, and 24 h, respectively. Left panel: representative images (100×); right panel: quantitative data. (D) HUVECs treated with CDBEE at the concentrations of 0, 10, and 20 μg/ml for 24 h were subjected to cell invasion assay. Left panel: representative images (100×); right panel: quantitative data. (E) HUVECs treated with CDBEE at the concentrations of 0, 10, and 20 μg/ml for 10 h were subjected to tube formation assay. *P < 0.05, **P < 0.01, ***P < 0.001.
Decreased Smad3 Was Partially Responsible for the Suppression of Proliferation in Human Hepatoma Cells Treated by CDBEE
Next we performed RNA sequencing on HepG2 cells treated with or without CDBEE. As depicted in Table 1, the expression abundance of Smad3 was decreased in HepG2 cells treated with CDBEE compared with the control group. Moreover, the results from quantitative real-time PCR and immunoblotting showed that CDBEE treatment reduced the mRNA and protein levels of Smad3 in HepG2 and SK-HEP-1 cells (Figures 4A, B). To investigate whether Smad3 was implicated in the anti-proliferative effect of CDBEE, we knocked down Smad3 expression with siRNAs in HepG2 and SK-HEP-1 cells (Figure 4C). Reduction of Smad3 expression inhibited the proliferation of HepG2 cells. Furthermore, depletion of Smad3 attenuated the suppressive proliferation of HepG2 cells induced by CDBEE (Figure 4D). Collectively, the down-regulation of Smad3 expression at least partially contributed to the anti-proliferative effect of CDBEE in human hepatoma cells.
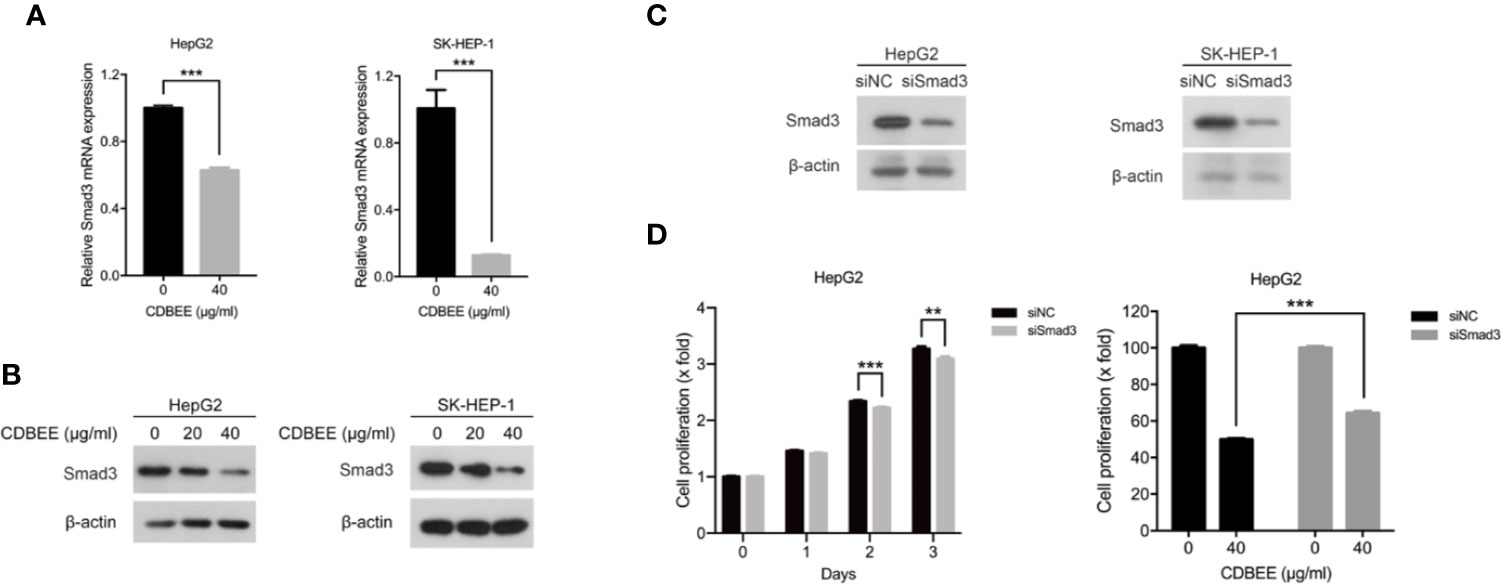
Figure 4 Reduced Smad3 was partially responsible for the inhibition of proliferation of human hepatoma cells exposed to Chinese dragon’s blood EtOAc extract (CDBEE). (A) Total RNA extracted from HepG2 or SK-HEP-1 cells treated with or without CDBEE (40 μg/ml) for 24 h was subjected to quantitative real-time PCR. (B) Total cell lysates harvested from HepG2 or SK-HEP-1 cells treated with CDBEE at the concentrations of 0, 20, and 40 μg/ml for 24 h were subjected to immunoblotting. (C) HepG2 or SK-HEP-1 cells were transfected with Smad3 siRNAs for 48 h and then subjected to immunoblotting for Smad3 expression. (D) HepG2 cells transfected with Smad3 siRNAs or negative control siRNAs were treated with or without CDBEE. The cell viability was measured by MTT assay. **P < 0.01, ***P < 0.001.
Smad3 Was Partially Involved in the Inhibition of Migration and Invasion of Human Hepatoma Cells Induced by CDBEE
Next HepG2 and SK-HEP-1 cells transfected with Smad3 siRNAs were subjected to migration and invasion assay. The migration ability of HepG2 and SK-HEP-1 cells was inhibited by knockdown of Smad3 (Supplementary Figure S3A). Moreover, inhibition of Smad3 expression impaired the suppression of migration ability of HepG2 and SK-HEP-1 cells induced by CDBEE (Figure 5A). In addition, transfection of Smad3 siRNAs restrained the invasion ability of HepG2 and SK-HEP-1 cells (Supplementary Figure S3B), and CDBEE-induced inhibition of invasion of HepG2 and SK-HEP-1 cells was weakened by reduction of Smad3 expression (Figure 5B). Thus, anti-migration and -invasion effect of CDBEE was partially mediated by Smad3 in human hepatoma cells.
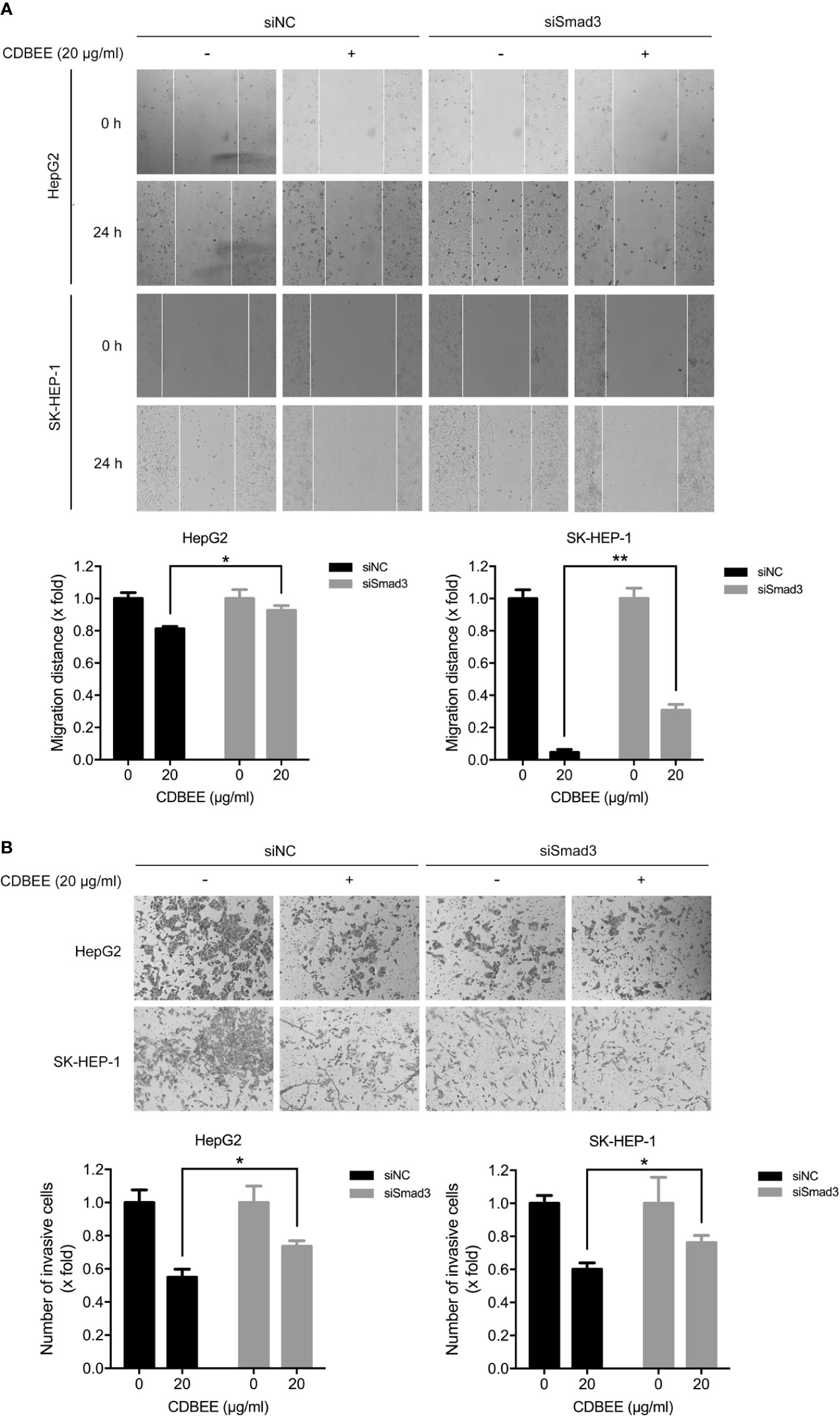
Figure 5 Smad3 was involved in Chinese dragon’s blood EtOAc extract (CDBEE)-induced inhibition of migration and invasion of human hepatoma cells. (A) HepG2 or SK-HEP-1 cells transfected with Smad3 siRNAs or negative control siRNAs were treated with or without CDBEE (20 μg/ml) for 24 h. The cells were subjected to the scratch assay and observed at 0 and 24 h. (B) HepG2 or SK-HEP-1 cells transfected with Smad3 siRNAs or negative control siRNAs were treated with or without CDBEE (20 μg/ml) for 24 h. The cells were subjected to cell invasion assay. *P < 0.05, **P < 0.01.
In Vivo Anti-Hepatoma Activity of CDBEE
To evaluate anti-hepatoma effect of CDBEE in vivo, we established a xenograft tumor model with human hepatoma HepG2 cells. CDBEE treatment hindered the tumorigenicity of human hepatoma HepG2 cells in nude mice (Figure 6A), and led to no significant weight loss of nude mice (Figure 6B). Results from H&E staining indicated that CDBEE exhibited no obvious toxicity on the major organs of nude mice, including heart, liver, spleen, lung, and kidney (Figure 6C). Moreover, immunohistochemical analysis for Smad3, Ki67 (a proliferation marker)(Scholzen and Gerdes, 2000), TUNEL (apoptosis analysis)(Kyrylkova et al., 2012), and MMP9 (a metastasis marker)(Deryugina and Quigley, 2006) demonstrated that CDBEE downregulated Smad3 expression, suppressed the proliferation, promoted the apoptosis, and inhibited the metastatic potential of HepG2 cells in nude mice (Figure 6D).
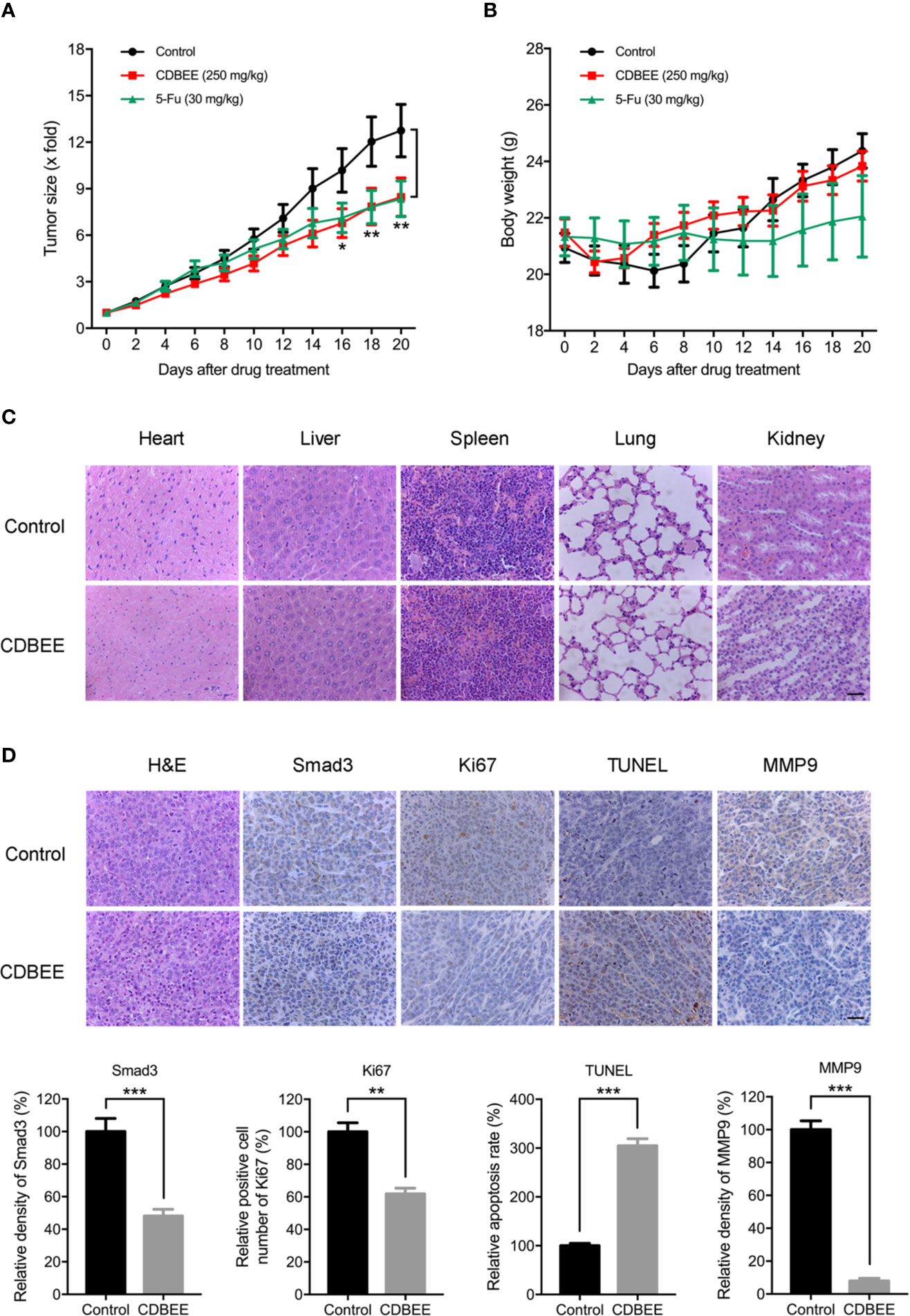
Figure 6 Chinese dragon’s blood EtOAc extract (CDBEE) blunted the oncogenic capability of HepG2 cells in nude mouse xenografts. (A) Nude mice bearing HepG2 tumor xenografts were treated with CDBEE (250 mg/kg, once daily, intragastric administration) or 5-FU (30 mg/kg, three times per week, intraperitoneal injection). (B) Body weight of nude mice. (C) H&E staining of major organs (heart, liver, spleen, lung, and kidney) from nude mice treated with or without CDBEE. (D) Immunohistochemical staining of tumor tissues from nude mice treated with or without CDBEE for Smad3, Ki67, TUNEL, and MMP9, and quantitative analysis. Scale bar, 50 μm. *P < 0.05, **P < 0.01, ***P < 0.001.
Discussion
The research of anti-HCC drugs from TCM has attracted more and more attention (Xi and Minuk, 2018). In this study, we have demonstrated that CDBEE exhibited good anti-hepatoma activity in vitro and in vivo, which was partially mediated by downregulation of Smad3 expression.
The maintenance of malignant phenotypes in cancers remain mainly dependent on some pivotal genes or signaling pathways. Hence, pharmacological intervention of these aberrant signaling pathways may be an effective strategy for cancer therapy. Smad3, as a major intracellular mediator of TGF-β signaling, plays an important role in tumorigenesis (Lu et al., 2007; Millet and Zhang, 2007; Tang et al., 2017). Activation of TGF-β/Smad pathway increased angiogenesis and metastasis in HCC (Reichl et al., 2012). TGF-β/Smad signaling pathway exerts a pro-oncogenic role in the late stage of HCC (Lee et al., 2014). Thus, blockage of TGF-β/Smad pathway may be an important strategy for HCC therapy (Mazzocca et al., 2009). Based on the results of RNA-sequencing, the further investigation revealed that CDBEE inhibited Smad3 expression in human hepatoma cells and tumor tissues. Moreover, decreased Smad3 contributed to the inhibition of proliferation, migration and invasion of human hepatoma cells exposed to CDBEE. Therefore, anti-hepatoma activity of CDBEE was partially mediated by TGF-β/Smad signaling pathway.
Extracellular signal-regulated kinase (ERK), c-Jun NH2-terminal kinase (JNK), and p38 are MAPK family members. MAPK signaling pathway plays a crucial role in cancer (Johnson and Lapadat, 2002; Dhillon et al., 2007). It has been reported that activation of MAPK signaling pathway inhibits the proliferation of cancer cells, and MAPKs play important role in the induction of apoptosis (Wada and Penninger, 2004; Yao et al., 2008). Our study showed that CDBEE treatment activated MAPK signaling pathway in HepG2 and SK-HEP-1 cells. Collectively, up-regulation of MAPK signaling pathway was partially responsible for the induction of apoptosis of human hepatoma cells by CDBEE treatment.
HCC commonly metastasizes to lungs, lymph nodes, bone, adrenal glands, peritoneum, and brain (Katyal et al., 2000; Becker et al., 2014). The prognosis for patients with metastatic HCC is poor (Uchino et al., 2011). Thus, the inhibition of metastasis is an effective strategy for HCC treatment. In this study, we demonstrated that CDBEE inhibited metastatic capability of human hepatoma cells. Numerous studies have shown that angiogenesis is required for tumor growth and metastasis (Carmeliet and Jain, 2000; Folkman, 2002). Inhibition of tumor angiogenesis is a promising approach for cancer treatment (Folkman, 1995). Anti-angiogenic activity could be evaluated through investigating multiple steps involved in angiogenesis, including proliferation, migration, and invasion of endothelial cells. Furthermore, in vitro tubular structure model of endothelial cells is a reliable experimental model for assessing angiogenesis capacity. Here we have demonstrated that CDBEE showed good anti-angiogenic activity supported by the data from cell proliferation assay, apoptosis assay, migration assay, invasion assay, and tube formation assay in HUVECs. Taken together, CDBEE may serve as a promising anti-metastatic drug in HCC treatment.
In summary, this study has identified the anti-hepatoma activity of CDBEE in vitro and in vivo. Mechanistically, CDBEE exerted anti-HCC activity partially through down-regulation of Smad3, one of major members in TGF-β/Smad pathway. Therefore, CDBEE may be a promising candidate drug for HCC treatment, especially for liver cancer with aberrant TGF-β/Smad signaling pathway.
Data Availability Statement
The raw data supporting the conclusions of this article are available on request to the corresponding authors.
Ethics Statement
The animal study was reviewed and approved by the Beijing University of Chinese Medicine Committee of Ethics.
Author Contributions
XC and YNZ performed the experiments, analyzed data, and wrote the manuscript. AY, YT, DP, JS, LT, and HH performed the experiments. YW, YFZ, and PT analyzed data and revised the manuscript. ZH and JL designed experiments, supervised the study, and revised the manuscript. All authors read and approved the final manuscript.
Funding
This study was financially supported by the Beijing Nova Program of Science and Technology (Z191100001119083), the Young Elite Scientists Sponsorship Program by China Association of Chinese Medicine (CACM-2018-(QNRC2-B05)), the Fundamental Research Funds for the Central Universities (2015-JYB-XYQ-004), and National Natural Science Foundation of China (81873044, 81573572).
Conflict of Interest
The authors declare that the research was conducted in the absence of any commercial or financial relationships that could be construed as a potential conflict of interest.
Supplementary Material
The Supplementary Material for this article can be found online at: https://www.frontiersin.org/articles/10.3389/fphar.2020.00669/full#supplementary-material
Abbreviations
CDBEE, Chinese dragon’s blood EtOAc extract; HUVECs, human umbilical vein endothelial cells; DMSO, dimethyl sulfoxide; 5-FU, 5-Fluorouracil; DMEM, Dulbecco’s Modified Eagle’s Medium; FBS, fetal bovine serum; ECL, enhanced chemiluminescence; IHC, immunohistochemistry; H&E, hematoxylin and eosin; HCC, hepatocellular carcinoma; TCM, traditional Chinese medicine; MAPKs, mitogen activated protein kinases; TGF-β, transforming growth factor-β; MMP9, matrix Metalloproteinase-9; PARP, poly ADP-ribose polymerase; JNK, c-Jun N-terminal kinase; ERK, extracellular signal-regulated kinase; EMT, epithelial-mesenchymal transition.
References
Becker, A. K., Tso, D. K., Harris, A. C., Malfair, D., Chang, S. D. (2014). Extrahepatic metastases of hepatocellular carcinoma: A spectrum of imaging findings. Can. Assoc. Radiol. J. 65 (1), 60–66. doi: 10.1016/j.carj.2013.05.004
Bray, F., Ferlay, J., Soerjomataram, I., Siegel, R. L., Torre, L. A., Jemal, A. (2018). Global cancer statistics 2018: GLOBOCAN estimates of incidence and mortality worldwide for 36 cancers in 185 countries. CA Cancer J. Clin. 68 (6), 394–424. doi: 10.3322/caac.21492
Carmeliet, P., Jain, R. K. (2000). Angiogenesis in cancer and other diseases. Nature 407 (6801), 249–257. doi: 10.1038/35025220
Deryugina, E. I., Quigley, J. P. (2006). Matrix metalloproteinases and tumor metastasis. Cancer Metastasis Rev. 25 (1), 9–34. doi: 10.1007/s10555-006-7886-9
Dhillon, A. S., Hagan, S., Rath, O., Kolch, W. (2007). MAP kinase signalling pathways in cancer. Oncogene 26 (22), 3279–3290. doi: 10.1038/sj.onc.1210421
Folkman, J. (1995). Angiogenesis in cancer, vascular, rheumatoid and other disease. Nat. Med. 1 (1), 27–31. doi: 10.1038/nm0195-27
Folkman, J. (2002). Role of angiogenesis in tumor growth and metastasis. Semin. Oncol. 29 (6 Suppl 16), 15–18. doi: 10.1053/sonc.2002.37263
Ghouri, Y. A., Mian, I., Rowe, J. H. (2017). Review of hepatocellular carcinoma: Epidemiology, etiology, and carcinogenesis. J. Carcinog. 16, 1. doi: 10.4103/jcar.JCar_9_16
Giannelli, G., Villa, E., Lahn, M. (2014). Transforming growth factor-beta as a therapeutic target in hepatocellular carcinoma. Cancer Res. 74 (7), 1890–1894. doi: 10.1158/0008-5472.CAN-14-0243
Guo, C., Liu, S., Dong, P., Zhao, D., Wang, C., Tao, Z., et al. (2015a). Akbu-LAAO exhibits potent anti-tumor activity to HepG2 cells partially through produced H2O2viaTGF-β signal pathway. Sci. Rep. 5, 18215. doi: 10.1038/srep18215
Guo, Q., Li, J., Lin, H. (2015b). Effect and Molecular Mechanisms of Traditional Chinese Medicine on Regulating Tumor Immunosuppressive Microenvironment. BioMed. Res. Int. 2015, 261620. doi: 10.1155/2015/261620
Hata, A., Chen, Y. G. (2016). TGF-beta Signaling from Receptors to Smads. Cold Spring Harb. Perspect. Biol. 8 (9), 1–21. doi: 10.1101/cshperspect.a022061
Hu, Z., Wang, Y., Huang, F., Chen, R., Li, C., Wang, F., et al. (2015). Brain-expressed X-linked 2 Is Pivotal for Hyperactive Mechanistic Target of Rapamycin (mTOR)-mediated Tumorigenesis. J. Biol. Chem. 290 (42), 25756–25765. doi: 10.1074/jbc.M115.665208
Hu, Z., Yang, A., Su, G., Zhao, Y., Wang, Y., Chai, X., et al. (2016). Huaier restrains proliferative and invasive potential of human hepatoma SKHEP-1 cells partially through decreased Lamin B1 and elevated NOV. Sci. Rep. 6, 31298. doi: 10.1038/srep31298
Jin, F., Jiang, K., Ji, S., Wang, L., Ni, Z., Huang, F., et al. (2017). Deficient TSC1/TSC2-complex suppression of SOX9-osteopontin-AKT signalling cascade constrains tumour growth in tuberous sclerosis complex. Hum. Mol. Genet. 26 (2), 407–419. doi: 10.1093/hmg/ddw397
Johnson, G. L., Lapadat, R. (2002). Mitogen-activated protein kinase pathways mediated by ERK, JNK, and p38 protein kinases. Science 298 (5600), 1911–1912. doi: 10.1126/science.1072682
Katyal, S., Oliver, J., Peterson, M. S., Ferris, J. V., Carr, B. S., Baron, R. L. (2000). Extrahepatic metastases of hepatocellular carcinoma. Radiology 216 (3), 698–703. doi: 10.1148/radiology.216.3.r00se24698
Kyrylkova, K., Kyryachenko, S., Leid, M., Kioussi, C. (2012). Detection of apoptosis by TUNEL assay. Methods Mol. Biol. 887, 41–47. doi: 10.1007/978-1-61779-860-3_5
Lee, I. H., Sohn, M., Lim, H. J., Yoon, S., Oh, H., Shin, S., et al. (2014). Ahnak functions as a tumor suppressor via modulation of TGFbeta/Smad signaling pathway. Oncogene 33 (38), 4675–4684. doi: 10.1038/onc.2014.69
Lu, S., Lee, J., Revelo, M., Wang, X., Lu, S., Dong, Z. (2007). Smad3 is overexpressed in advanced human prostate cancer and necessary for progressive growth of prostate cancer cells in nude mice. Clin. Cancer Res. 13 (19), 5692–5702. doi: 10.1158/1078-0432.CCR-07-1078
Massague, J., Blain, S. W., Lo, R. S. (2000). TGFbeta signaling in growth control, cancer, and heritable disorders. Cell 103 (2), 295–309. doi: 10.1016/s0092-8674(00)00121-5
Mazzocca, A., Fransvea, E., Lavezzari, G., Antonaci, S., Giannelli, G. (2009). Inhibition of transforming growth factor beta receptor I kinase blocks hepatocellular carcinoma growth through neo-angiogenesis regulation. Hepatology 50 (4), 1140–1151. doi: 10.1002/hep.23118
Millet, C., Zhang, Y. E. (2007). Roles of Smad3 in TGF-beta signaling during carcinogenesis. Crit. Rev. Eukaryot. Gene Expr. 17 (4), 281–293. doi: 10.1615/critreveukargeneexpr.v17.i4.30
Nam, S. W., Clair, T., Kim, Y. S., Mcmarlin, A., Schiffmann, E., Liotta, L. A., et al. (2001). Autotaxin (NPP-2), a metastasis-enhancing motogen, is an angiogenic factor. Cancer Res. 61 (18), 6938–6944. doi: 10.1002/1097-0142(20010915)92:6+<1737::AID-CNCR1506>3.0.CO;2-0
Pang, D. R., Su, X. Q., Zhu, Z. X., Sun, J., Li, Y. T., Song, Y. L., et al. (2016). Flavonoid dimers from the total phenolic extract of Chinese dragon’s blood, the red resin of Dracaena cochinchinensis. Fitoterapia 115, 135–141. doi: 10.1016/j.fitote.2016.10.004
Reichl, P., Haider, C., Grubinger, M., Mikulits, W. (2012). TGF-beta in epithelial to mesenchymal transition and metastasis of liver carcinoma. Curr. Pharm. Des. 18 (27), 4135–4147. doi: 10.2174/138161212802430477
Scholzen, T., Gerdes, J. (2000). The Ki-67 protein: from the known and the unknown. J. Cell Physiol. 182 (3), 311–322. doi: 10.1002/(SICI)1097-4652(200003)182:3<311::AID-JCP1>3.0.CO;2-9
Soldani, C., Scovassi, A. I. (2002). Poly(ADP-ribose) polymerase-1 cleavage during apoptosis: an update. Apoptosis 7 (4), 321–328. doi: 10.1023/a:1016119328968
Su, M., Huang, J., Liu, S., Xiao, Y., Qin, X., Liu, J., et al. (2016). The anti-angiogenic effect and novel mechanisms of action of Combretastatin A-4. Sci. Rep. 6, 28139. doi: 10.1038/srep28139
Sun, Q., Chen, X., Ma, J., Peng, H., Wang, F., Zha, X., et al. (2011). Mammalian target of rapamycin up-regulation of pyruvate kinase isoenzyme type M2 is critical for aerobic glycolysis and tumor growth. Proc. Natl. Acad. Sci. U. S. A 108 (10), 4129–4134. doi: 10.1073/pnas.1014769108
Tang, P. M., Zhou, S., Meng, X. M., Wang, Q. M., Li, C. J., Lian, G. Y., et al. (2017). Smad3 promotes cancer progression by inhibiting E4BP4-mediated NK cell development. Nat. Commun. 8, 14677. doi: 10.1038/ncomms14677
Uchino, K., Tateishi, R., Shiina, S., Kanda, M., Masuzaki, R., Kondo, Y., et al. (2011). Hepatocellular carcinoma with extrahepatic metastasis: clinical features and prognostic factors. Cancer 117 (19), 4475–4483. doi: 10.1002/cncr.25960
Wada, T., Penninger, J. M. (2004). Mitogen-activated protein kinases in apoptosis regulation. Oncogene 23 (16), 2838–2849. doi: 10.1038/sj.onc.1207556
Wen, F., Zhao, X., Zhao, Y., Lu, Z., Guo, Q. (2016). The anticancer effects of Resina Draconis extract on cholangiocarcinoma. Tumour Biol. 37 (11), 15203–15210. doi: 10.1007/s13277-016-5393-3
Xi, S. Y., Minuk, G. Y. (2018). Role of traditional Chinese medicine in the management of patients with hepatocellular carcinoma. World J. Hepatol. 10 (11), 799–806. doi: 10.4254/wjh.v10.i11.799
Xiang, Y., Guo, Z., Zhu, P., Chen, J., Huang, Y. (2019). Traditional Chinese medicine as a cancer treatment: Modern perspectives of ancient but advanced science. Cancer Med. 8 (5), 1958–1975. doi: 10.1002/cam4.2108
Xu, H., Zhao, X., Liu, X., Xu, P., Zhang, K., Lin, X. (2015). Antitumor effects of traditional Chinese medicine targeting the cellular apoptotic pathway. Drug Des. Devel. Ther. 9, 2735–2744. doi: 10.2147/DDDT.S80902
Yang, A., Fan, H., Zhao, Y., Zha, X., Zhang, H., Hu, Z., et al. (2016a). Huaier aqueous extract inhibits proliferation and metastasis of tuberous sclerosis complex cell models through downregulation of JAK2/STAT3 and MAPK signaling pathways. Oncol. Rep. 36 (3), 1491–1498. doi: 10.3892/or.2016.4969
Yang, G., Liang, Y., Zheng, T., Song, R., Wang, J., Shi, H., et al. (2016b). FCN2 inhibits epithelial-mesenchymal transition-induced metastasis of hepatocellular carcinoma via TGF-beta/Smad signaling. Cancer Lett. 378 (2), 80–86. doi: 10.1016/j.canlet.2016.05.007
Yao, Y. Q., Ding, X., Jia, Y. C., Huang, C. X., Wang, Y. Z., Xu, Y. H. (2008). Anti-tumor effect of beta-elemene in glioblastoma cells depends on p38 MAPK activation. Cancer Lett. 264 (1), 127–134. doi: 10.1016/j.canlet.2008.01.049
Yoshida, K., Matsuzaki, K., Murata, M., Yamaguchi, T., Suwa, K., Okazaki, K. (2018). Clinico-Pathological Importance of TGF-β/Phospho-Smad Signaling during Human Hepatic Fibrocarcinogenesis. Cancers 10 (6), 183. doi: 10.3390/cancers10060183
Keywords: Dracaena cochinchinensis (Lour.) S. C. Chen, Chinese dragon’s blood EtOAc extract (CDBEE), hepatocellular carcinoma, Smad3, proliferation, metastasis
Citation: Chen X, Zhao Y, Yang A, Tian Y, Pang D, Sun J, Tang L, Huang H, Wang Y, Zhao Y, Tu P, Hu Z and Li J (2020) Chinese Dragon’s Blood EtOAc Extract Inhibits Liver Cancer Growth Through Downregulation of Smad3. Front. Pharmacol. 11:669. doi: 10.3389/fphar.2020.00669
Received: 29 January 2020; Accepted: 23 April 2020;
Published: 13 May 2020.
Edited by:
Jiahong Lu, University of Macau, ChinaReviewed by:
Guoyuan Zhu, Macau University of Science and Technology, MacauLiang Feng, China Pharmaceutical University, China
Copyright © 2020 Chen, Zhao, Yang, Tian, Pang, Sun, Tang, Huang, Wang, Zhao, Tu, Hu and Li. This is an open-access article distributed under the terms of the Creative Commons Attribution License (CC BY). The use, distribution or reproduction in other forums is permitted, provided the original author(s) and the copyright owner(s) are credited and that the original publication in this journal is cited, in accordance with accepted academic practice. No use, distribution or reproduction is permitted which does not comply with these terms.
*Correspondence: Zhongdong Hu, emRodUBidWNtLmVkdS5jbg==; Jun Li, ZHJsajY2NkAxNjMuY29t
†These authors have contributed equally to this work