- 1Department of Biological Engineering, Inha University, Incheon, South Korea
- 2STR Biotech Co., Ltd., Chuncheon-si, South Korea
- 3Department of Molecular Bio-Science, Kangwon National University, Chuncheon-si, South Korea
- 4Green Chemistry and Materials Group, Korea Institute of Industrial Technology, Cheonan-si, South Korea
- 5Green Process and System Engineering Major, Korea University of Science and Technology (UST), Daejeon, South Korea
3-Dehydroshikimate (DHS) is a useful starting metabolite for the biosynthesis of muconic acid (MA) and shikimic acid (SA), which are precursors of various valuable polymers and drugs. Although DHS biosynthesis has been previously reported in several bacteria, the engineered strains were far from satisfactory, due to their low DHS titers. Here, we created an engineered Escherichia coli cell factory to produce a high titer of DHS as well as an efficient system for the conversion DHS into MA. First, the genes showing negative effects on DHS accumulation in E. coli, such as tyrR (tyrosine dependent transcriptional regulator), ptsG (glucose specific sugar: phosphoenolpyruvate phosphotransferase), and pykA (pyruvate kinase 2), were disrupted. In addition, the genes involved in DHS biosynthesis, such as aroB (DHQ synthase), aroD (DHQ dehydratase), ppsA (phosphoenolpyruvate synthase), galP (D-galactose transporter), aroG (DAHP synthase), and aroF (DAHP synthase), were overexpressed to increase the glucose uptake and flux of intermediates. The redesigned DHS-overproducing E. coli strain grown in an optimized medium produced ~117 g/L DHS in 7-L fed-batch fermentation, which is the highest level of DHS production demonstrated in E. coli. To accomplish the DHS-to-MA conversion, which is originally absent in E. coli, a codon-optimized heterologous gene cassette containing asbF, aroY, and catA was expressed as a single operon under a strong promoter in a DHS-overproducing E. coli strain. This redesigned E. coli grown in an optimized medium produced about 64.5 g/L MA in 7-L fed-batch fermentation, suggesting that the rational cell factory design of DHS and MA biosynthesis could be a feasible way to complement petrochemical-based chemical processes.
Introduction
Aromatic amino acid biosynthetic pathways have long been a potential source for commercially relevant chemicals with diverse industrial applications (Tzin and Galili, 2010). One of the core metabolites that facilitate the production of useful intermediates in aromatic amino acid biosynthetic pathways is 3-dehydroshikimate (DHS), a key intermediate in the biosynthesis of shikimic acid (SA), muconic acid (MA), vanillin, and protocatechuate (PCA) (Li et al., 2019). This group of chemicals has drawn much attention for the major feedstock for food, drugs and commodity chemicals (Chen and Nielsen, 2013; Becker and Wittmann, 2015). Bio-based production of these compounds is a promising alternative to the current petrochemical-based production routes that are a cause of environment and energy concerns (Jan and Cavallaro, 2015). MA is easily converted into adipic acid and terephthalic acid, which find universal application in the synthesized nylons and polymer polyethylene terephthalate (PET) industries (Draths and Frost, 1994; Kruyer and Peralta-Yahya, 2017). In addition, SA is an initial precursor for the production of a well-known anti-virial compound named oseltamivir phosphate, also known as Tamiflu®.
DHS has a six-member carbon ring with two asymmetric carbons, which is difficult and costly to synthesize chemically (Richman et al., 1996; Nishikura-Imamura et al., 2014). On the other hand, DHS is a natural metabolite found in the shikimate pathway in plants, fungi, and bacteria, making its production feasible through microbial fermentation (Li et al., 1999). DHS biosynthesis in E. coli starts from glucose, via the glycolysis pathway, a central carbon metabolism (Figure 1). Two intermediates, phosphoenolpyruvate (PEP) and erythrose-4-phosphate (E4P), are converted to DHS via 3-deoxy-D-arabino-heptulosonate-7-phosphate (DAHP) and dehydroquinate (DHQ) by DAHP synthase (aroG, aroF, and aroH), DHQ synthase (aroB), and DHQ dehydratase (aroD).
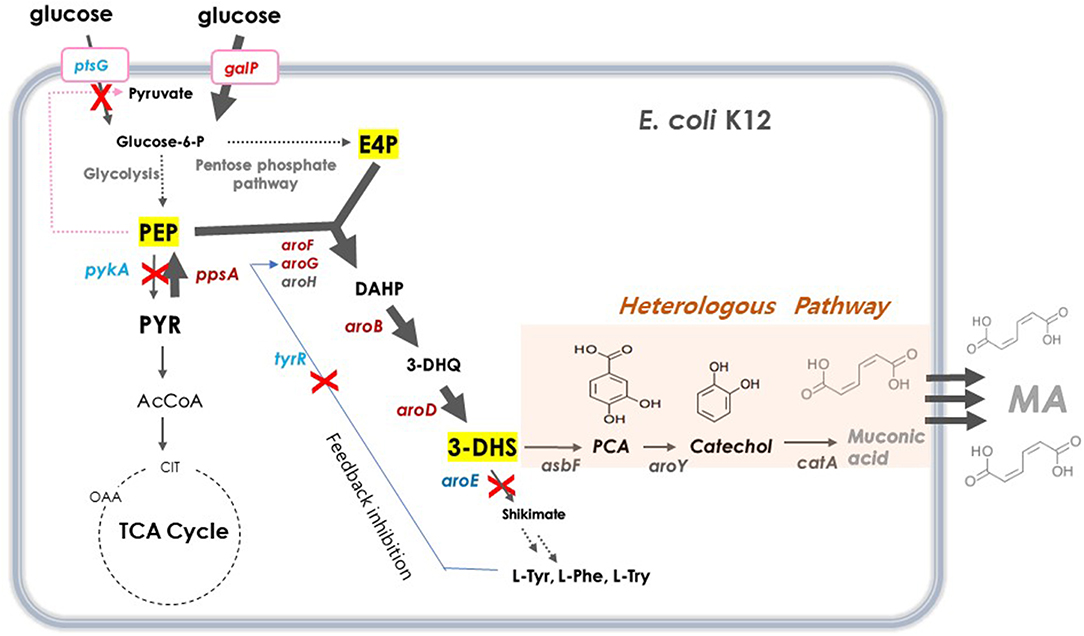
Figure 1. Schematic overview of metabolic pathway for DHS biosynthesis in E. coli. Red crosses denote disrupted genes and bold blue arrows denote steps that are overexpressed. Dashed arrows represent two or more steps. G6P, glucose-6-phosphate; PEP, phosphoenolpyruvate; PYR, pyruvate; AcCoA, acetyl-coenzyme A; OAA, oxaloacetate; CIT, citrate; E4P, erythrose4-phosphate; DAHP, 3-deoxy-D-arabinoheptulosonate-7-phosphate; DHQ, 3-de-hydroquinate; DHS, 3-dehydroshikimate; SA, shikimic acid; PCA, protocatechuate; CA, catechol, MA, muconic acid. ptsG, glucose specific sugar: phosphoenolpyruvate phosphotransferase; galP, D-galactose transporter; zwf, glucose-6-phosphate 1-dehydrogenase; tktA, transketolase; tyrR, tyrosine dependent transcriptional regulator; pykF, pyruvate kinase 1; pykA, pyruvate kinase 2; ppsA, phosphoenolpyruvate synthase; pckA, phosphoenolpyruvate carboxykinase; aroG, DAHP synthase; aroF, DAHP synthase; aroB, DHQ synthase; aroD, DHQ dehydratase; aroE, shikimate dehydrogenase: asbFEopt, 3-dehydroshikimate(DHS) dehydratase; pcaG/H, codon-optimized PCA deoxygenase; aroYEopt, codon-optimized PCA decarboxylase; catAEopt, codon-optimized CA 1,2 -dioxygenase.
For several decades, many approaches have been applied to increase the carbon flux toward shikimate biosynthetic pathway for the production of several aromatic compounds. For example, there was an attempt to solve the feedback inhibition issue of DAHP synthase to improve the production of aromatic compounds (Pittard and Yang, 2008; Wang et al., 2013). Another strategy involved the inactivation of the PEP-consuming PTS system and use of another PEP-independent glucose uptake pathway, as PEP is an important precursor of an aromatic compound (Patnaik et al., 1995; Chandran et al., 2003; Johansson et al., 2005). Yet another approach was to eliminate the pyruvate kinases involved in PEP conversion to pyruvate, thereby enhancing the pathway that converts pyruvate to PEP (Patnaik et al., 1995; Chandran et al., 2003; Johansson et al., 2005; Meza et al., 2012; Rodriguez et al., 2013). Combinations of these strategies are typically used to improve the productivity of aromatic compounds (Yi et al., 2002; Wang et al., 2013; Rodriguez et al., 2014; Martínez et al., 2015; Lee et al., 2018).
Recently, we engineered a Corynebacterum glutamicum strain to overproduce MA from glucose, through redesign of the aromatic amino acid biosynthetic pathway (Lee et al., 2018). Through heterologous expression of a codon-optimized PCA decarboxylase gene cluster under a strong promoter, and deletions of several key genes involved in MA intermediate bypass routes, an optimized pathway for DHS-PCA-CA-MA was successfully constructed in C. glutamicum. Optimization of culture media and processes using the MA-producing C. glutamicum strain were performed to achieve a significantly increased titer of MA (Lee et al., 2018).
In this study, we generated engineered E. coli strains to overproduce both DHS and MA, through manipulation of several key genes involved in DHS biosynthesis and heterologous expression of codon-optimized foreign genes involved in DHS-to-MA conversion. These redesigned DHS-overproducing and MA-overproducing E. coli strains, grown in optimized media, produced the highest levels of both DHS and MA production in 7L fed-batch fermentations, indicating that the rational microbial cell factory design of DHS and MA biosynthesis could be an alternative way to complement petrochemical-based chemical processes.
Materials and Methods
Bacterial Strains, Media, and Culture Conditions
The bacterial strains and plasmids used in this study are listed in Table 1. E. coli DH5a was used as the cloning host, and E. coli K12 & AB2834 were used as parent strains. E. coli strains for genetic manipulations were grown in Luria-Bertani (LB) medium at 37 or 30°C. For DHS production in small-scale culture, a single colony was inoculated in LB medium at 30°C for 15 h, and secondary culture was inoculated with 1% (v/v) in the same medium at 30°C for 6 h. The miniature culture was grown using 1.3 mL of E. coli production medium (EPM) in a 24-well cell culture plate, at 220 rpm under 30°C, for 4 days. The composition of the EPM for DHS and MA production was: glucose (5 g/L), glycerol (10 g/L), yeast extract (2.5 g/L), tryptone (2.5 g/L), KH2PO4 (7.5 g/L), MgSO4 (0.5 g/L), (NH4)2SO4 (3.5 g/L), NH4Cl (2.7 g/L), Na2SO4 (0.7 g/L), Na2HPO4•12H2O (9 g/L), and a trace metal solution (1 mL/L). The trace metal solution contained FeSO4•7H2O (10 g/L), CaCl2•2H2O (2 g/L), ZnSO4•7H2O (2.2 g/L), MnSO4•4H2O (0.5 g/L), CuSO4•5H2O (1 g/L), (NH4)6Mo7O24•4H2O (0.1 g/L), and Na2B4O7•10H2O (0.02 g/L).
Construction of Plasmids and Strains
All constructed plasmids for integration and disruption are listed in Table 1. Plasmid DNA extraction and purification were performed using a commercial kit (TIANGEN). For PCR, the Pfu DNA polymerase and HIFI DNA polymerase (TransGen) were used. For disruption and substitution of chromosomal genes, suicide vector pKOV (Addgene, USA) was used, which has the sacB gene for providing a markerless system. The constructed plasmids were transformed into E. coli AB2834 by electroporation.
For disruption of tyrR, ptsG, pykA, and lacI genes, homologous DNA fragments were PCR-amplified with primer sets R1-R4, G1-G4, A1-A4, and I1-I4, respectively, which were then inserted into the pKOV vector, generating pKOV-R, pKOV-G, pKOV-A, and pKOV-I, respectively. For overexpression of several genes, T-lac was generated by inserting the lac promoter and RBS into T-vector. The lac promoter and RBS were amplified with primer sets Plac1-Plac2 and RBS1-RBS2 from pUC18 and the pET-21b(+) vector. DNA fragments containing E. coli aroB, aroD, galP, ppsA, aroG, and aroF genes were amplified with primer sets B1-B2, D1-D2, P1-P2, A3-A4, G3-G4, and F1-F2, respectively, and the amplified DNA fragments were inserted into the T-lac vector, generating T-BD, T-PA, and T-GF. Subsequently, Plac_aroB_aroD cassette was amplified with primer sets BD1-BD2 and T-BD as a template, and the amplified cassette was inserted into pKOV-I, generating pKOV-BD. The Plac_aroB_aroD and Plac_galP_ppsA cassettes were amplified with primer sets BD1-BD3 and PA1-PA2, using T-BD and T-PA as a template, respectively, and the amplified cassettes were inserted into pKOV-I, generating pKOV-PABD. The Plac_aroG_aroF cassette was amplified with primer sets GF1-GF2, using T-GF as a template, and the amplified cassette was inserted into pKOV-PABD, generating pKOV-PAGFBD (Supplementary Table 1).
A series of E. coli DHS-producing strains were constructed using the host strain AB2834, which was constructed by the markerless disruption of the aroE gene. The tyrR gene in AB2834 was disrupted using plasmid pKOV-R, generating the Inha 24 strain. Subsequently, the ptsG and pykA genes were disrupted from Inha 24 using plasmids pKOV-G and pKOV-A, generating strains Inha 29 and Inha 52, respectively. Markerless integration of aroB and aroD genes, under the control of the lac promoter, were integrated at LacI gene locus into strain Inha 52 using plasmid pKOV-BD, generating the strain Inha 99 (Figure 2A). Using the plasmid pKOV-PABD, the aroB, aroD, ppsA, and galP genes, under the control of the lac promoter, were integrated into strain Inha 52, generating strain Inha 95. The aroB, aroD, ppsA, galP, aroG, and aroF genes, under the control of the lac promoter, were chromosome-integrated using plasmid pKOV-PAGFBD in Inha 52 strain, generating strain Inha 103.
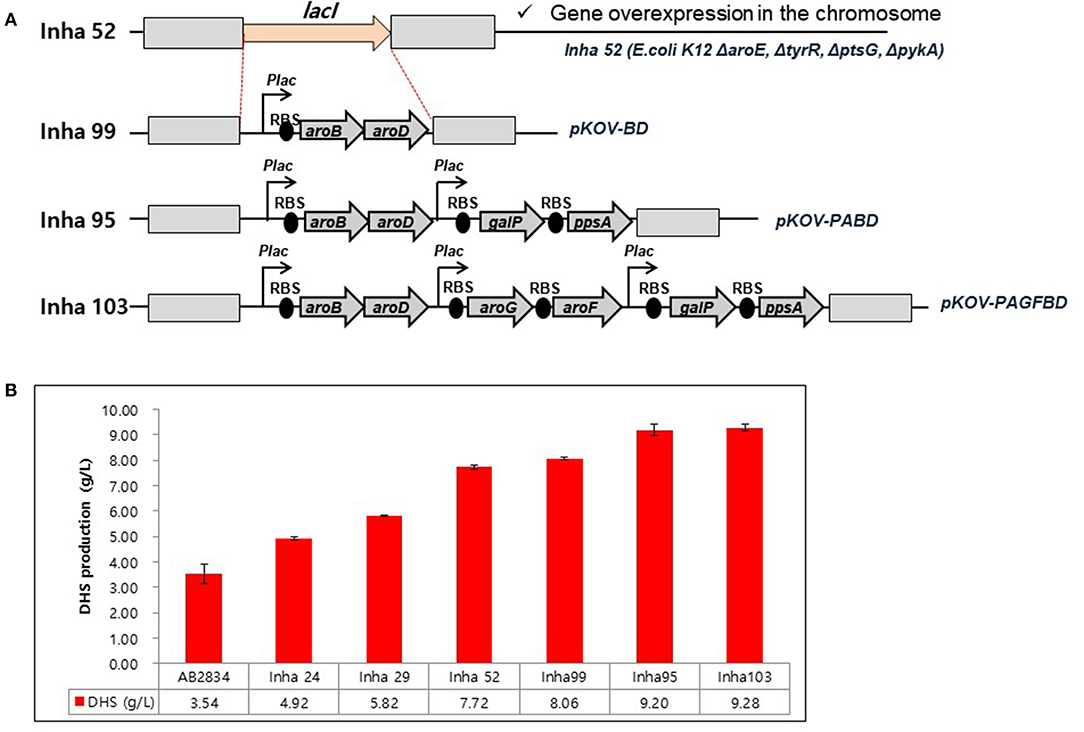
Figure 2. (A) Construction of E. coli mutants for overproduction of DHS. (B) HPLC analysis of DHS production yields in DHS-producing E. coli strains. E. coli cells were cultured in 1.3 mL of EPM at 30°C for 96 h. Data represent averages and standard deviations from two independent experiments.
RNA Sequencing
For RNA sequencing, E. coli strains AB2834 and Inha52 were cultured in LB media for seeding, and in 500 mL of EPM media for the main production. Growth curves were generated, and each strain was sampled at the early stage of exponential phase (AB2834, 4 h; Inha52, 7 h) as well as the early stage of stationary phase (AB2834, 9 h; Inha52, 12 h). RNeasy mini prep kit (Qiagen, CA) was used for total RNA isolation, according to the manufacturer's instructions. DNaseI (TaKaRa, Japan) was used to eliminate any potential chromosomal DNA contamination. RNA-Seq was performed by ChunLab Inc., Korea.
Metabolite Analysis
The E. coli cells were removed from cultures by centrifugation and culture supernatant was filtered using a membrane filter. The metabolites were separated by HPLC using an Aminex HPX-87H column (Bio-rad, Japan). The mobile phase was 2.5 mM H2SO4, and a flow rate of 0.6 mL/min was used for metabolites. The column had to be heated at a temperature of 65°C for detection of metabolites. MA and DHS were analyzed at wavelengths of 262 and 236 nm, respectively. Organic acids, including succinic acid, acetic acid, formic acid, and lactic acid, were detected at wavelengths of 210 nm.
Fed-Batch Fermentation
The first growth culture was carried out in a conical tube containing 5 mL of LBG medium (30°C and 200 rpm). After 12 h of culture, 0.2 mL of the culture broth was inoculated in a 250-mL baffled flask containing 20 mL of culture medium, and cultured at 30°C and 200 rpm in an incubator. After 6 h, the second culture broth was inoculated into a 7-L fermenter (1% v/v inoculum). Production culture was carried out using the PB4-md5 medium in a 7-L fermenter (working volume: 2 L). The PB4-md5 medium includes: 30 g/L glucose, 10 g/L glycerol, 15.75 g/L yeast extract, 21.375 g/L tryptone, 5.25 g/L KH2PO4, 1 g/L MgSO4 · 7H2O, 0.8 g/L citric acid, 1 mL/L trace metals, and 200 μg/L thiamine hydrochloride. The feeding medium includes: 600 g/L glucose, 100 g/L yeast extract, 20 g/L MgSO4 · 7H2O, and 5 mL/L trace metal. Phosphate was not added to the feeding medium, to allow for the regulation of cell growth. The pH levels were 7.0 in each culture (10N NaOH, 3M HCl), and the DO level was maintained above at 30% by controlling the agitation, aeration, and feeding rates. The configuration of the fermenter was as follows: two impellers with turbine and marine impeller, ring-type sparger with 12 holes, top-driven, and 160 mm tank diameter. The feeding medium was supplied when glucose was depleted, using a peristaltic pump.
Construction of a Gene Cassette Involved in DHS-to-MA Conversion
E. coli Inha M101 was prepared by transformation of a plasmid pMESK1 into Inha M103. pMESK1 was prepared by ligation of an AsbFEopt -aroYEopt -catAEopt cassette to pUC18. Codon-optimized gene versions for increased protein expression in E. coli were synthesized from Cosmo Genetech, Korea. Digestion of pUC57_M1 with XbaI and HindIII liberated a 3.3-kb asbFEopt -aroYEopt -catAEopt fragment. Plasmid pUC18 was digested at the same restriction site. Subsequent ligation of asbFEopt -aroYEopt -catAEopt to pUC18 resulted in pMESK1. The ligation of the asbFEopt -aroYEopt -catAEopt cassette was verified by restriction enzyme digestion analysis. To select the promoter for expression of heterologous gene cluster, the intergenic region of rnpB, fusA, oppA, dps, and rmf genes (upstream region of the start codon) was amplified by PCR and cloned into pMESK1 digested by EcoRI and XbaI.
Results
Redesign of 3-Dehydroshikimate Biosynthetic Pathway in Escherichia coli
We used the E. coli AB2834 strain as a parental host for DHS accumulation, which is an aroE mutant strain lacking shikimate dehydrogenase (Draths and Frost, 1994). To further improve DHS accumulation, we first disrupted the key genes involved in DHS accumulation in E. coli AB2834. The tyrR repressor, which exerts negative control on the transcription of aroG and aroF genes with two aromatic amino acids (L-phenylalanine and L-tyrosine), was disrupted (Bongaerts et al., 2001). The tyrR-disruption mutant strain (named Inha 24) accumulated 4.92 g/L of DHS in the culture medium after 96 h, whereas the parental strain produced 3.54 g/L of DHS under the same culture condition. Next, the ptsG gene, one of the phosphotransferase system genes that consume PEP for glucose uptake, was disrupted in the Inha 24 strain (named Inha 29) to increase the availability of PEP, a DHS precursor. The Inha 29 strain produced 5.82 g/L of DHS under the same culture condition. Finally, the pykA gene, encoding a pyruvate kinase, was also disrupted in the Inha 29 strain (named Inha 52), leading to a production of 7.72 g/L of DHS, signifying a more than 2-fold improvement in production yield relative to the parental strain (Figure 2B).
RNA sequencing-based comparative transcriptome analysis between the parental strain and Inha52 revealed that the transcription levels of the DHS biosynthetic pathway genes such as aroB (encoding DHQ synthease) and aroD (encoding DHQ dehydratase) were significantly reduced in the Inha52 strain (data not shown). To overcome this limitation, both aroB and aroD genes were overexpressed under the control of the lac promoter via an integration plasmid pKOV-BD, generating the strain Inha 99 (Figure 2A). The Inha 99 strain, harboring an extra copy of aroB and aroD genes, produced 8.06 g/L of DHS (Figure 2B). In addition, Inha 95 was constructed using pKOV-PABD to overexpress the galP and ppsA genes as well as aroB and aroD genes in the Inha 52 strain (Figure 2A). The galP gene, encoding D-galactose transporter, was overexpressed to improve the level of glucose uptake. The ppsA gene, encoding a phosphoenolpyruvate synthase, was also overexpressed to increase the availability of PEP. Inha 95, overexpressing the aroB, aroD, galP, and ppsA genes, produced 9.20 g/L of DHS (Figure 2B). Subsequently, aroG and aroF genes, encoding DAHP synthase, were additionally overexpressed in strain Inha 95, generating the Inha 103 strain (Figure 2A). The Inha 103 strain, harboring extra copies of aroB, aroD, galP, ppsA, aroG, and aroF genes under the control of the lac promoter, produced 9.28 g/L of DHS, which is 20% higher DHS yield than that of strain Inha 52, and 2.6-fold higher than that of the parental strain (Figure 2B; Supplementary Table 2). Thus, the DHS production yield of our recombinant strains increased gradually through serial disruptions and overexpression of the genes involved in DHS synthesis.
Fed-Batch Fermentation for DHS Production
During the statistical medium optimization process in shake-flask cultures using the metabolically engineered E. coli cells, composition of the production medium was found to be a very important factor for enhanced DHS production. Sufficient supply of dissolved oxygen during the entire fermentation period was also observed to be crucial, as revealed by higher DHS production yield according to increase in oxygen mass transfer rate (kLa) through increment of agitation speed (rpm). Therefore, we intended to develop a fed-batch operation process that could efficiently control the producers' fermentation physiology, simultaneously overcoming the oxygen-limited culture conditions caused by high cell density in bioreactor fermentations. The fed-batch culture was carried out using the abovementioned four strains, i.e., Inha 24, Inha 29, Inha 52, and Inha 103. The optimized medium from flask culture was again optimized for the C/N ratio in the fermenter. The DHS production yield was confirmed by fed-batch culture based on this medium. Scaled-up operations from shake flasks to fermenters are largely affected by the operating conditions such as gas-liquid mass transfer, shear stress, mixing time, antifoam, pH regulation, and power input. A phosphate-limited fed-batch culture was performed to regulate cell growth. After the glucose level were almost depleted in the fermentation broth, feeding medium was continuously injected using a peristaltic pump to maintain below 10 g/L to prevent the production of organic acids by residual glucose. As shown in Figure 3, DO levels could be successfully maintained above 30% during the whole fermentation time in the respective fed-batch fermentation, thereby leading to overcoming the undesirable oxygen-limited conditions due to relatively high cell density. It is well-known that when E. coli cells become oxygen-limited, cellular metabolism rapidly changes, producing proteases that can degrade foreign proteins, thus lowering the production ability of the transformed E. coli cells.
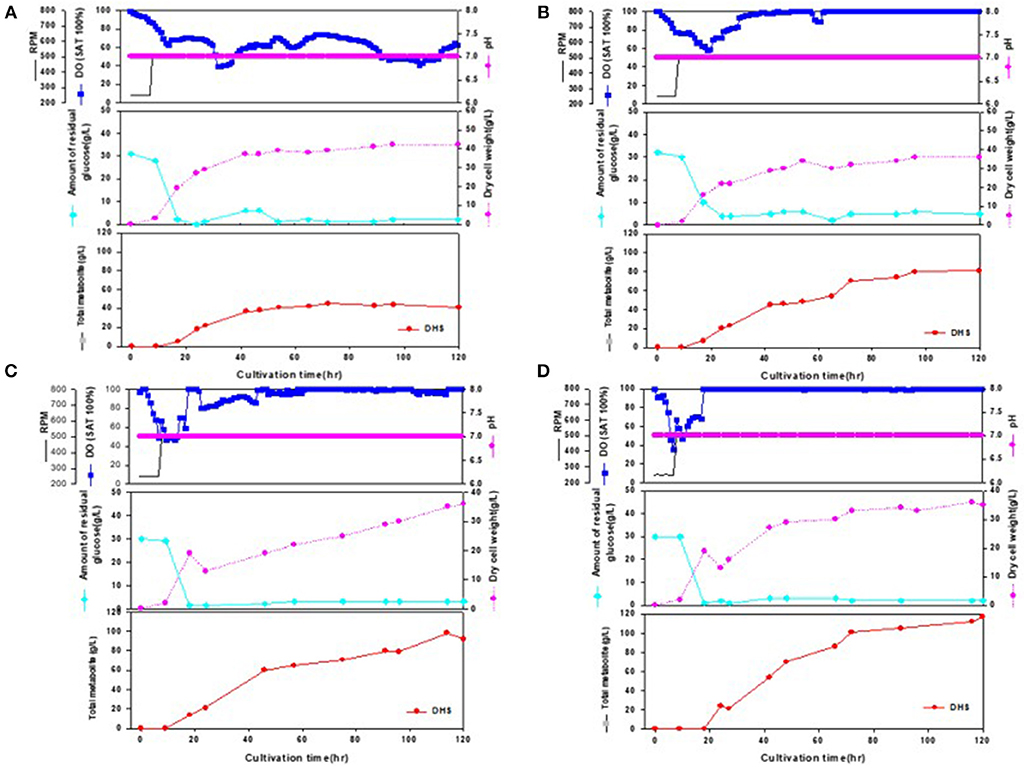
Figure 3. Time-course profiles of cell growth (DCW), glucose, organic acid, and metabolite production by strains in the 7-L fermenter. (A) Inha 24: The feeding medium was sequentially injected at culture periods of 17, 19, 24, 27, 42, 47, and 54 h, at a rate of 0.1176, 0.1568, 0.1176, 0.1568, 0.0784, 0.0522, and 0.1176 mL/min, respectively. (B) Inha 29: The feeding medium was sequentially injected at culture periods of 17, 19, 24, 42, 47, 54, and 89 h, at a rate of 0.0392, 0.1176, 0.1568, 0.0784, 0.0522, 0.1176, and 0.0784 mL/min, respectively. (C) Inha 52: The feeding medium was sequentially injected at culture periods of 17, 19, 42, 47, 54, 65, and 89 h, at a rate of 0.0392, 0.1176, 0.0784, 0.0522, 0.1568, 0.1176, and 0.0522 mL/min, respectively. (D) Inha 103: The feeding medium was sequentially injected at culture periods of 18, 24, 42, and 66 h at a rate of 0.1306, 0.1568, 0.1306, and 0.1045 mL/min, respectively.
All four strains consumed glucose for 17 h and started feeding from this time onwards. As a result, in the Inha 24 strain, the cells showed steady growth, and the DCW (Dry cell weight) and production yield declined 48 h after the incubation; the DCW was about 42 g/L and the DHS was about 45 g/L. In the same culture condition, the Inha 29 strain exhibited the maximum cell dry weight of about 37 g/L and a DHS level of 74 g/L. In the Inha 52 strain, DCW was lower than that of the Inha 29 strain, but DHS production yield was as high as 81 g/L, implying that there is a competitive relationship between cell growth and DHS production. Although DHS is a primary metabolite, its production in the engineered strains exhibited typical secondary metabolite patterns (Figure 3). In aromatic amino acid processes, E. coli and C. glutamicum have characteristic metabolites that suppress cell density at certain concentrations (Cheng et al., 2012; Rodriguez et al., 2014; Lee et al., 2018). Finally, in the Inha 103 strain with enhanced flux to DHS, the DCW was about 35 g/L, slightly lower than that of Inha 52, while the production yield of DHS had increased by 44% to 117 g/L. The specific production yield (Yp/x) was 1.07 g DHS/g DCW for the Inha 24 strain, 2 g DHS/g DCW for the Inha 29 strain, 2.25 g DHS/g DCW for the Inha 52 strain, and 3.34 g DHS/g DCW for the Inha 103 strain. The specific production yield of the Inha 103 strain was 3.1-times higher than that of Inha 24 (Table 2; Supplementary Figure 3).
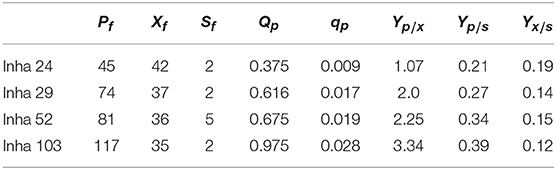
Table 2. A, 7-L lab-scale fermentation; B, 50-L pilot-scale fermentation; Pf, maximum DHS production (g IA/L); Xf, maximum dry cell weight (g DCW/L); Sf, final residual glucose concentration (g glucose/L); Qp, average volumetric DHS production rate (g DHS/L/h); qp, average specific DHS production rate (g DHS/g DCW/h); Yp/x, specific DHS production (g DHS/g DCW); Yp/s, DHS production yield based on glucose (g DHS/g glucose); Yx/s, DCW yield based on glucose (g DCW/g glucose).
Heterologous Expression of the Genes Involved in DHS-to-MA Conversion
We tried to produce muconic acid, another valuable compound, through heterologous expression of the genes involved in DHS-to-MA conversion in the DHS-overproducing E. coli strain. Previously, three foreign genes (aroZ, aroY, and catA) were reported to be responsible for the DHS-to-MA conversion in E. coli (Draths and Frost, 1994, Figure 1). Similarly, we generated a codon-optimized single operon construct containing three genes, asbF from Bacillus thuringiensis (encoding DHS dehydratase for DHS-to-PCA), aroY from Klebsiella pneumoniae (encoding PCA decarboxylase for PCA-to-CA), and catA from Acinetobacter calcoaceticus (encoding catechol 1,2-dioxygenase for CA-to-MA). Genes asbFEopt, aroYEopt, and catAEopt were cloned into modified pUC18 under the control of the lac promoter, thus generating pMESK1. To optimize the heterologous foreign genes expression in a DHS-overproducing E. coli strain, we searched for a naturally-suitable promoter via comparative transcriptome analysis between the parental strain and Inha 52 (Supplementary Figure 1). Among the gene expression profiles during the culture duration, five promoters were selected according to their transcription strength, and each selected promoter was cloned to control the foreign gene cassette (Supplementary Figure 2A). The E. coli strains expressing each promoter from pMESK4 to pMESK8 were named E. coli Inha M104–M108, respectively, and were tested to determine whether MA accumulated in their culture broths. The production level of MA varied, depending on the promoter, as shown in Supplementary Figures 2B and 4. The highest MA titer was observed in strain Inha 103, expressing oppA gene promoter, which encodes periplasmic oligopeptides-binding protein in E. coli. It yielded up to 1,789 mg/L of MA, an 8-fold increase compared with strain Inha M101 (Plac_ asbFEopt -aroYEopt -catAEopt), which showed an MA titer of 223 mg/L. DHS was accumulated in relatively large amounts in two strains (Plac and Pdps), and only a small amount of MA was produced in the other two strains (Prmf and PrmpB). In the 7-L fermenter, the Inha 103 strain containing the asbFEopt -aroYEopt -catAEopt operon cassette under the control of oppA promoter produced 64.5 g/L MA with little accumulation of other intermediates (Figure 4).
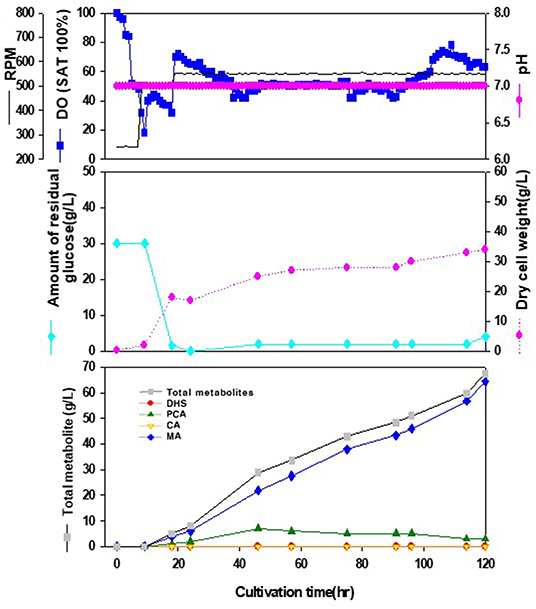
Figure 4. Time-course profiles of cell growth (DCW, pink closed diamond), glucose (cyan closed diamond), organic acid, and metabolite production by the engineered E. coli InhaM104 in the 7-L fermenter. The feeding medium was sequentially injected at culture periods of 18, 24, and 46 h, at a rate of 0.0392, 0.0784, and 0.0653 mL/min, respectively. Closed gray square, total metabolite; red closed diamond, DHS (3-dehydroshikimic acid); green closed diamond, PCA (protocatechuate); yellow closed triangle, CA (catechol); blue closed diamond, MA (cis-cis muconic acid).
Discussion
In general, strategies to redesign metabolic pathways constitute the primary goal of metabolic engineering, which is a prerequisite to produce certain valuable compounds. This strategy has been used to engineer the shikimate pathway for producing a number of useful aromatic products in E. coli. For example, Chandran et al. reported an engineered E. coli strain that achieved 84 g/L of shikimate with a yield of 0.33 mol/mol glucose (Chandran et al., 2003). In addition, Wang et al. reported a 48 g/L yield of L-tryptophan, with a conversion ratio of 21.87% from glucose (Wang et al., 2013). It was previously reported that the introduction of heterologous biosynthetic genes such as aroZ, aroY, and catA into the aroE-deleted E. coli led to the accumulation of MA up to 36 g/L via benzene-independent pathway (Niu et al., 2002). In addition, various microbial cell factory strategies were also purposed to enhance production of MA or shikimic acid (Table 3) (Noda and Kondo, 2017; Bilal et al., 2018a).
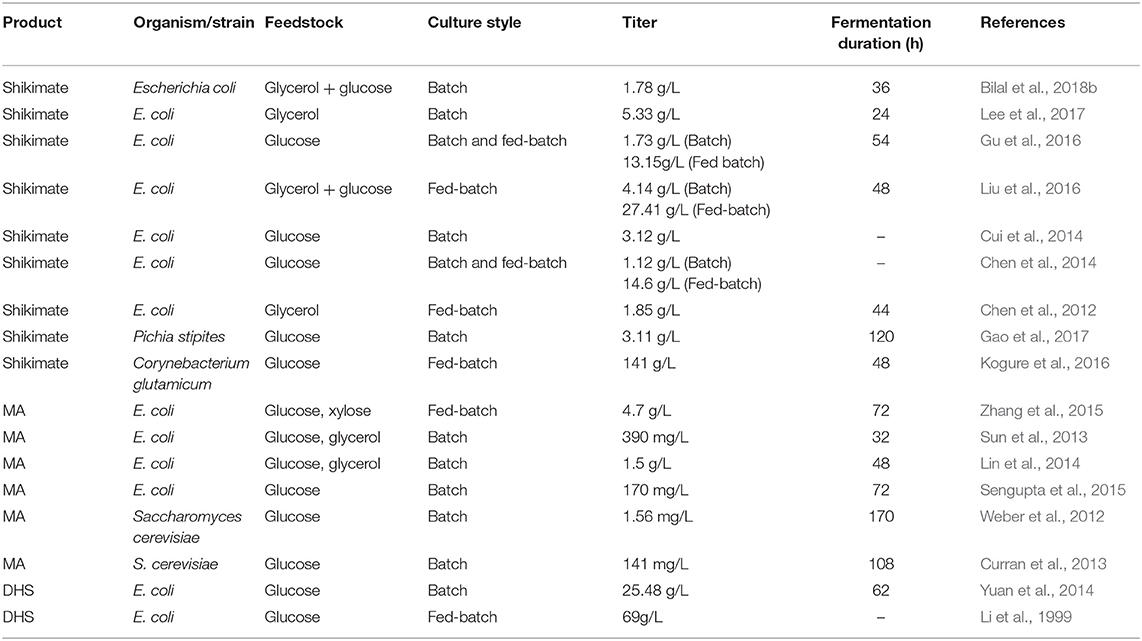
Table 3. Recent overview of engineered microbial strains for enhanced shikimate and MA biosynthesis.
In this study, a phosphate-limited fed-batch fermentation process was successfully developed for enhanced production of DHS using a DHS-overproducing E. coli strain constructed by redesigning its biosynthetic pathway. The phosphate-limited fed-batch operation in the 7 L bioreactor level was observed to strictly regulate the specific growth rate (μ) and the glucose consumption rate and suppresses the production of undesirable byproducts by effectively controlling DO levels. Notably, under this culture conditions, DHS was biosynthesized in a non-growth-associated mode during the later stage of the fed-batch operations, efficiently utilizing higher portion of the supplied nutrients into the DHS biosynthesis rather than cellular growth. As a result, the Inha 103 strain showed significantly high level of DHS production amounting to 117 g/L (at a production yield (Yp/s) of 0.39 g/g glucose) in the 7-L fed-batch bioreactor culture, ~13-fold increase as compared to the parallel flask culture (9 g/L) performed under the identical culture conditions.
It was observed that sufficient amount of dissolved oxygen (DO) should be supplied in the cultivation of the metabolically engineered E. coli cells for enhanced production of DHS: Increase in oxygen mass transfer rate (kLa) through increment of agitation speed (rpm), and expansion of the diameter ratio of the impeller to the fermenter vessel up to 0.46 resulted in higher productivity of DHS. In addition, as described in this paper, by installing a turbine impeller just above the ring-type sparger for efficient break-down of the sparged air, and a marine impeller above the turbine impeller for proficient mixing of the dissolved oxygen, DO levels could be successfully maintained above 50% during the whole fermentation period, thereby leading to overcoming the undesirable oxygen-limited conditions due to relatively high cell density. Notably, with almost the same configuration of this fermenter system, higher production levels of MA (muconic acid) had been observed, maintaining DO levels above 40% during the entire fermentation time, leading to facilitated transfer of DO to the producing cells, and thereby improving MA production (Figure 4). In addition, by carefully controlling the composition and feeding rate of the supplied medium during the fed-batch operation, it was possible to overcome DO-limited conditions, simultaneously minimizing the production of the byproducts caused by high levels of residual glucose in the fermentation broth. Notably, under the phosphate-limited fed-batch fermentations, DHS was observed to be biosynthesized almost in a growth-associated mode, thus resulting in the remarkable enhancement in DHS productivity (i.e., ~6-fold increase as compared to the parallel batch bioreactor fermentation performed under the identical environments).
Such a remarkable production yield could be achieved by applying a combination of several metabolic engineering strategies for DHS production to E. coli strains. We demonstrated that engineering of key genes for DHS production using the host strain E. coli AB2834, in which the aroE-encoded shikimate dehydrogenase is inactivated, gradually increased the DHS production. We inactivated the tyrR-encoded tyrosine-dependent transcriptional regulator, which is a feedback repressor of aroG- and aroF-encoding DAHP synthase, for improving the transcription levels. Among the several approaches to improve PEP availability for promoting DHS production, we generated PTS-inactivated strain by disrupting ptsG, which is a PEP-dependent glucose transporter. Instead, we overexpressed galP-encoding D-galactose transporter, which is another glucose-uptake route. In addition, pykA-encoding pyruvate kinase 2 was removed and ppsA-encoded PEP synthase was overexpressed for reconversion of pyruvate to PEP. Furthermore, we overexpressed the genes on the biosynthetic pathway to complete the DHS-overproducing strain. Construction of an MA-producing E. coli cell factory was carried out by the introduction of a single operon containing asbFEopt -aroYEopt -catAEopt gene cluster, as well as applying a promoter engineering strategy through the transcriptome analysis. Although the current heterologous gene cluster converts MA to about 50% of DHS, strengthening and optimization of the DHS-PCA-CA-MA route in further studies could result in better performing strains with higher DHS-to-MA bioconversion efficiency.
In summary, we report the construction of E. coli strains capable of producing DHS at high concentrations from D-glucose. To accumulate high concentrations of DHS, tyrR, ptsG, and pykA gene were sequentially deleted from E. coli AB2834, in which the aroE gene was mutated to prevent the conversion of DHS to SA. Extra copies of aroB, aroD, galP, ppsA, aroG, and aroF genes involved in DHS biosynthesis were additionally inserted to maximize DHS accumulation. MA was also successfully produced by a heterologous expression pathway for DHS-to-MA bioconversion. A controlled fed-batch operation was performed with a statistically optimized production medium in a 7L bioreactor and the redesigned E. coli strain could convert DHS to MA efficiently, thereby producing about 64.5 g/L MA with almost no accumulation of metabolic intermediates such as PCA, CA, and DHS. This study demonstrates the potential value of E. coli host to produce high level of an intermediate metabolite of aromatic pathways and the rational cell factory design approach to possibly complement petrochemical-based chemical processes.
Data Availability Statement
The datasets generated for this study are available on request to the corresponding author.
Author Contributions
SK, SL, G-TC, and E-SK designed the research and provided the improvement of the manuscript. S-SC, S-YS, S-OP, H-NL, JS, JK and J-HP performed the experiments, as well as data collection and analysis. JS and JK performed RNA-seq analysis. S-SC and H-NL performed genetic engineering. S-YS and S-OP performed medium optimization and fermentation. S-SC and S-YS wrote the article.
Conflict of Interest
S-YS, S-OP, H-NL, and SL were employed by the company STR Biotech Co., Ltd.
The remaining authors declare that the research was conducted in the absence of any commercial or financial relationships that could be construed as a potential conflict of interest.
Acknowledgments
This work was carried out with the support of (1) Cooperative Research Program for Agriculture Science and Technology Development (Project No. PJ01318701) Rural Development Administration, (2) Agricultural Microbiome R&D Program, Ministry of Agriculture, Food and Rural Affairs, Republic of Korea [as part of the (multi-ministerial) Genome Technology to Business Translation Program] No. 918008-04 and (3) partially by the 2015 Research Grant Program (520150308) from Kangwon National University, Republic of Korea.
Supplementary Material
The Supplementary Material for this article can be found online at: https://www.frontiersin.org/articles/10.3389/fbioe.2019.00241/full#supplementary-material
References
Becker, J., and Wittmann, C. (2015). Advanced biotechnology: metabolically engineered cells for the bio-based production of chemicals and fuels, materials, and health-care products. Angew. Chem. Int. Ed Engl. 54, 3328–3350. doi: 10.1002/anie.201409033
Bilal, M., Wang, S., Iqbal, H. M. N., Zhao, Y., Hu, H., Wang, W., et al. (2018a). Metabolic engineering strategies for enhanced shikimate biosynthesis: current scenario and future developments. Appl. Microbiol. Biotechnol. 102, 7759–7773. doi: 10.1007/s00253-018-9222-z
Bilal, M., Yue, S., Hu, H., Wang, W., and Zhang, X. (2018b). Systematically engineering Escherichia coli for enhanced shikimate biosynthesis co-utilizing glycerol and glucose. Biofuels Bioprod. Biorefin. 12, 348–361. doi: 10.1002/bbb.1867
Bongaerts, J., Krämer, M., Müller, U., Raeven, L., and Wubbolts, M. (2001). Metabolic engineering for microbial production of aromatic amino acids and derived compounds. Metab. Eng. 3, 289–300. doi: 10.1006/mben.2001.0196
Chandran, S. S., Yi, J., Draths, K. M., von Daeniken, R., Weber, W., and Frost, J. W. (2003). Phosphoenolpyruvate availability and the biosynthesis of shikimic acid. Biotechnol. Prog. 19, 808–814. doi: 10.1021/bp025769p
Chen, K., Dou, J., Tang, S., Yang, Y., Wang, H., Fang, H., et al. (2012). Deletion of the aroK gene is essential for high shikimic acid accumulation through the shikimate pathway in E. coli. Bioresour. Technol. 119, 141–147. doi: 10.1016/j.biortech.2012.05.100
Chen, X., Li, M., Zhou, L., Shen, W., Algasan, G., Fan, Y., et al. (2014). Metabolic engineering of Escherichia coli for improving shikimate synthesis from glucose. Bioresour. Technol. 166, 64–71. doi: 10.1016/j.biortech.2014.05.035
Chen, Y., and Nielsen, J. (2013). Advances in metabolic pathway and strain engineering paving the way for sustainable production of chemical building blocks. Curr. Opin. Biotechnol. 24, 965–972. doi: 10.1016/j.copbio.2013.03.008
Cheng, L., Wang, J., Xu, Q., Xie, X., Zhang, Y., Zhao, C., et al. (2012). Effect of feeding strategy on L-tryptophan production by recombinant Escherichia coli. Ann. Microbiol. 62, 1625–1634. doi: 10.1007/s13213-012-0419-6
Cui, Y. Y., Chen, L., Zhang, Y. Y., Jian, H., and Liu, J. Z. (2014). Production of shikimic acid from Escherichia coli through chemically inducible chromosomal evolution and cofactor metabolic engineering. Microb. Cell Fact. 13:21. doi: 10.1186/1475-2859-13-21
Curran, K. A., Leavitt, J. M., Karim, A. S., and Alper, H. S. (2013). Metabolic engineering of muconic acid production in Saccharomyces cerevisiae. Metab. Eng. 15, 55–66. doi: 10.1016/j.ymben.2012.10.003
Draths, K. M., and Frost, J. W. (1994). Environmentally compatible synthesis of adipic acid from D-glucose. J. Am. Chem. Soc. 116, 399–400. doi: 10.1021/ja00080a057
Gao, M., Cao, M., Suástegui, M., Walker, J., Rodriguez Quiroz, N., Wu, Y., et al. (2017). Innovating a nonconventional yeast platform for producing shikimate as the building block of high-value aromatics. ACS. Synth. Biol. 6, 29–38. doi: 10.1021/acssynbio.6b00132
Gu, P., Su, T., Wang, Q., Liang, Q., and Qi, Q. (2016). Tunable switch mediated shikimate biosynthesis in an engineered non-auxotrophic Escherichia coli. Sci. Rep. 6:29745. doi: 10.1038/srep29745
Jan, C. J. B., and Cavallaro, S. (2015). Transiting from adipic acid to bioadipic acid. 1, Petroleum-based processes. Ind. Eng. Chem. Res. 54, 1–46. doi: 10.1021/ie5020734
Johansson, L., Lindskog, A., Silfversparre, G., Cimander, C., Nielsen, K. F., and Lidén, G. (2005). Shikimic acid production by a modified strain of E. coli (W3110.shik1) under phosphate-limited and carbon-limited conditions. Biotechnol. Bioeng. 92, 541–552. doi: 10.1002/bit.20546
Kogure, T., Kubota, T., Suda, M., Hiraga, K., and Inui, M. (2016). Metabolic engineering of Corynebacterium glutamicum for shikimate overproduction by growth-arrested cell reaction. Metab. Eng. 38, 204–216. doi: 10.1016/j.ymben.2016.08.005
Kruyer, N. S., and Peralta-Yahya, P. (2017). Metabolic engineering strategies to bio-adipic acid production. Curr. Opin. Biotechnol. 45,136–143. doi: 10.1016/j.copbio.2017.03.006
Lee, H. N., Shin, W. S., Seo, S. Y., Choi, S. S., Song, J. S., Kim, J. Y., et al. (2018). Corynebacterium cell factory design and culture process optimization for muconic acid biosynthesis. Sci. Rep. 8:18041. doi: 10.1038/s41598-018-36320-4
Lee, M. Y., Hung, W. P., and Tsai, S. H. (2017). Improvement of shikimic acid production in Escherichia coli with growth phase-dependent regulation in the biosynthetic pathway from glycerol. World J. Microbiol. Biotechnol. 33:25. doi: 10.1007/s11274-016-2192-3
Li, K., Mikola, M. R., Draths, K. M., Worden, R. M., and Frost, J. W. (1999). Fed-batch fermentor synthesis of 3-dehydroshikimic acid using recombinant Escherichia coli. Biotechnol. Bioeng. 64, 61–733. doi: 10.1002/(SICI)1097-0290(19990705)64:1<61::AID-BIT7>3.0.CO;2-G
Li, L., Tu, R., Song, G., Cheng, J., Chen, W., Li, L., et al. (2019). Development of a synthetic 3-dehydroshikimate biosensor in Escherichia coli for metabolite monitoring and genetic screening. ACS Synth. Biol. 8, 297–306. doi: 10.1021/acssynbio.8b00317
Lin, Y., Sun, X., Yuan, Q., and Yan, Y. (2014). Extending shikimate pathway for the production of muconic acid and its precursor salicylic acid in Escherichia coli. Metab. Eng. 23, 62–69. doi: 10.1016/j.ymben.2014.02.009
Liu, X., Lin, J., Hu, H., Zhou, B., and Zhu, B. (2016). Site-specific integration and constitutive expression of key genes into Escherichia coli chromosome increases shikimic acid yields. Enzyme Microb. Technol. 82, 96–104. doi: 10.1016/j.enzmictec.2015.08.018
Martínez, J. A., Bolívar, F., and Escalante, A. (2015). Shikimic acid production in Escherichia coli: from classical metabolic engineering strategies to omics applied to improve its production. Front. Bioeng. Biotechnol. 3:145. doi: 10.3389/fbioe.2015.00145
Meza, E., Becker, J., Bolivar, F., Gosset, G., and Wittmann, C. (2012). Consequences of phosphoenolpyruvate sugar phosphotranferase system and pyruvate kinase isozymes inactivation in central carbon metabolism flux distribution in Escherichia coli. Microb. Cell Fact. 11:127. doi: 10.1186/1475-2859-11-127
Nishikura-Imamura, S., Matsutani, M., Insomphun, C., Vangnai, A., Toyama, H., Yakushi, T., et al. (2014). Overexpression of a type II 3-dehydroquinate dehydratase enhances the biotransformation of quinate to 3-dehydroshikimate in Gluconobacter oxydans. Appl. Microbiol. Biotechnol. 98, 2955–2963. doi: 10.1007/s00253-013-5439-z
Niu, W., Draths, K. M., and Frost, J. W. (2002). Benzene-free synthesis of adipic acid. Biotechnol. Prog. 18, 201–211. doi: 10.1021/bp010179x
Noda, S., and Kondo, A. (2017). Recent advances in microbial production of aromatic chemicals and derivatives. Trends Biotechnol. 35, 785–796. doi: 10.1016/j.tibtech.2017.05.006
Patnaik, R., Spitzer, R. G., and Liao, J. C. (1995). Pathway engineering for production of aromatics in Escherichia coli: confirmation of stoichiometric analysis by independent modulation of AroG, TktA, and Pps activities. Biotechnol. Bioeng. 46, 361–370. doi: 10.1002/bit.260460409
Pittard, J., and Yang, J. (2008). Biosynthesis of the aromatic amino acids. EcoSal Plus 3, 1–39. doi: 10.1128/ecosalplus.3.6.1.8
Richman, J. E., Chang, Y., Kambourakis, S., Draths, K. M., Almy, E., Snell, K. D., et al. (1996). Reaction of 3-dehydroshikimic acid with molecular oxygen and hydrogen peroxide: products, mechanism, and associated antioxidant activity. J. Am. Chem. Soc. 118, 11587–11591. doi: 10.1021/ja952317i
Rodriguez, A., Martínez, J. A., Báez-Viveros, J. L., Flores, N., Hernández-Chávez, G., Ramírez, O. T., et al. (2013). Constitutive expression of selected genes from the pentose phosphate and aromatic pathways increases the shikimic acid yield in high-glucose batch cultures of an Escherichia coli strain lacking PTS and pykF. Microb. Cell Fact. 12:8. doi: 10.1186/1475-2859-12-86
Rodriguez, A., Martínez, J. A., Flores, N., Escalante, A., Gosset, G., and Bolivar, F. (2014). Engineering Escherichia coli to overproduce aromatic amino acids and derived compounds. Microb. Cell Fact. 13:126. doi: 10.1186/s12934-014-0126-z
Sengupta, S., Jonnalagadda, S., Goonewardena, L., and Juturu, V. (2015). Metabolic engineering of a novel muconic acid biosynthesis pathway via 4-hydroxybenzoic acid in Escherichia coli. Appl. Environ. Microbiol. 81, 8037–8043. doi: 10.1128/AEM.01386-15
Sun, X., Lin, Y., Huang, Q., Yuan, Q., and Yan, Y. (2013). A novel muconic acid biosynthesis approach by shunting tryptophan biosynthesis via anthranilate. Appl. Environ. Microbiol. 79, 4024–4030. doi: 10.1128/AEM.00859-13
Tzin, V., and Galili, G. (2010). The biosynthetic pathways for shikimate and aromatic amino acids in Arabidopsis thaliana. Arabidopsis Book 8:e0132. doi: 10.1199/tab.0132
Wang, J., Cheng, L., Wang, J., Liu, Q., Shen, T., and Chen, N. (2013). Genetic engineering of Escherichia coli to enhance production of L-tryptophan. Appl. Microbiol. Biotechnol. 97, 7587–7596. doi: 10.1007/s00253-013-5026-3
Weber, C., Brückner, C., Weinreb, S., Lehr, C., Essl, C., and Boles, E. (2012). Biosynthesis of cis,cis-muconic acid and its aromatic precursors, catechol and protocatechuic acid, from renewable feedstocks by Saccharomyces cerevisiae. Appl. Environ. Microbiol. 78, 8421–8430. doi: 10.1128/AEM.01983-12
Yi, J., Li, K., Draths, K. M., and Frost, J. W. (2002). Modulation of phosphoenolpyruvate synthase expression increases shikimate pathway product yields in E. coli. Biotechnol. Prog. 18, 1141–1148. doi: 10.1021/bp020101w
Yuan, F., Chen, W., Jia, S., and Wang, Q. (2014). Improving 3-dehydroshikimate production by metabolically engineered Escherichia coli. Sheng Wu Gong Cheng Xue Bao 30, 1549–1560. doi: 10.13345/j.cjb.140019
Keywords: dehydroshikimate, cell factory design, Escherichia coli, culture process, production optimization
Citation: Choi S-S, Seo S-Y, Park S-O, Lee H-N, Song J, Kim J, Park J-H, Kim S, Lee SJ, Chun G-T and Kim E-S (2019) Cell Factory Design and Culture Process Optimization for Dehydroshikimate Biosynthesis in Escherichia coli. Front. Bioeng. Biotechnol. 7:241. doi: 10.3389/fbioe.2019.00241
Received: 29 June 2019; Accepted: 11 September 2019;
Published: 09 October 2019.
Edited by:
Nils Jonathan Helmuth Averesch, Stanford University, United StatesReviewed by:
Zhen Chen, Tsinghua University, ChinaZhi-Gang Jeff Qian, Shanghai Jiao Tong University, China
Copyright © 2019 Choi, Seo, Park, Lee, Song, Kim, Park, Kim, Lee, Chun and Kim. This is an open-access article distributed under the terms of the Creative Commons Attribution License (CC BY). The use, distribution or reproduction in other forums is permitted, provided the original author(s) and the copyright owner(s) are credited and that the original publication in this journal is cited, in accordance with accepted academic practice. No use, distribution or reproduction is permitted which does not comply with these terms.
*Correspondence: Gie-Taek Chun, Z3RjaHVuODU0N0BnbWFpbC5jb20=; Eung-Soo Kim, ZXVuZ3Nvb0BpbmhhLmFjLmty
†These authors have contributed equally to this work