- 1Center of Advanced European Studies and Research, Department of Molecular Sensory Systems, Bonn, Germany
- 2Institute of Innate Immunity, Department of Biophysical Imaging, Medical Faculty, University of Bonn, Bonn, Germany
- 3Center of Reproductive Medicine and Andrology, University Hospital Münster, University of Münster, Münster, Germany
- 4Biochemie der Pflanzen, Ruhr-Universität Bochum, Bochum, Germany
- 5Department of Biosciences and Nutrition, Karolinska Institutet, Solna, Sweden
- 6Department of Obstetrics and Gynecology, University of Massachusetts Medical School Worcester, Worcester, MA, United States
- 7Life and Medical Sciences Institute, University of Bonn, Bonn, Germany
Mammalian oocytes are enveloped by the zona pellucida (ZP), an extracellular matrix of glycoproteins. In sperm, stimulation with ZP proteins evokes a rapid Ca2+ influx via the sperm-specific, pH-sensitive Ca2+ channel CatSper. However, the physiological role and molecular mechanisms underlying ZP-dependent activation of CatSper are unknown. Here, we delineate the sequence of ZP-signaling events in mouse sperm. We show that ZP proteins evoke a rapid intracellular pHi increase that rests predominantly on Na+/H+ exchange by NHA1 and requires cAMP synthesis by the soluble adenylyl cyclase sAC as well as a sufficiently negative membrane potential set by the spem-specific K+ channel Slo3. The alkaline-activated CatSper channel translates the ZP-induced pHi increase into a Ca2+ response. Our findings reveal the molecular components underlying ZP action on mouse sperm, opening up new avenues for understanding the basic principles of sperm function and, thereby, mammalian fertilization.
Introduction
The zona pellucida (ZP) serves as an important check-point during fertilization, allowing sperm from the same (homologous) species to penetrate the ZP and fuse with the oocyte, while preventing the penetration of sperm from a different (heterologous) species. Fertilization requires sperm capacitation, a maturation process occurring in the female genital tract (Chang, 1951; Austin, 1952; Braden et al., 1954; Avella et al., 2014; Fahrenkamp et al., 2020). After fertilization, the ZP becomes impenetrable also to homologous sperm, avoiding the fertilization of the oocyte by more than one sperm cell, called polyspermy (Avella et al., 2013; Gupta, 2015). Moreover, in vitro, binding of sperm to the ZP changes swimming behavior (Katz and Yanagimachi, 1981; Baltz et al., 1988; Drobnis et al., 1988; Sumigama et al., 2015), and stimulation of sperm with solubilized ZP proteins evokes acrosomal exocytosis (Florman and Storey, 1982; Bleil and Wassarman, 1983; Baltz et al., 1988; Cross et al., 1988; Florman et al., 1989; Arnoult et al., 1996a, b), which is also evoked upon binding of sperm to isolated, intact ZPs (Jin et al., 2011; Sumigama et al., 2015). The significance and role of the ZP-induced acrosome reaction for fertilization are, however, unclear and might vary between mammalian species (Jin et al., 2011; La Spina et al., 2016; Muro et al., 2016; Hirohashi and Yanagimachi, 2018). Furthermore, the signaling pathways underlying the action of ZP proteins on sperm are only ill-defined (Florman et al., 2008).
Swimming behavior and acrosomal exocytosis are controlled by changes in the intracellular Ca2+ concentration ([Ca2+]i) (Ho and Suarez, 2001; Eisenbach and Giojalas, 2006; Florman et al., 2008; Kaupp et al., 2008; Publicover et al., 2008). In mouse sperm, ZP proteins evoke a Ca2+influx (Arnoult et al., 1996a, 1999; Florman et al., 2008) via the sperm-specific Ca2+ channel CatSper (Xia and Ren, 2009). How ZP proteins activate CatSper is not known. In general, CatSper is activated upon depolarization of the membrane potential (Vm) and alkalization of the intracellular pH (pHi) (Kirichok et al., 2006; Lishko and Kirichok, 2010; Lishko et al., 2011; Strünker et al., 2011; Seifert et al., 2015). ZP proteins evoke a pHi increase, which might stimulate alkaline-evoked Ca2+ influx via CatSper (Arnoult et al., 1996b, 1999), The sperm-specific Na+/H+ exchanger sNHE (Slc9c1) (Wang et al., 2003) has been proposed to mediate the pHi increase (Chavez et al., 2014). Finally, ZP-evoked Ca2+ influx in mouse sperm requires a sufficiently negative membrane potential (Vm), set by the sperm-specific K+ channel Slo3 (Kcnu1, Table 1) (Chavez et al., 2014). However, the mechanism underlying the Vm-control of the ZP action has remained enigmatic.
Here, we study the action of solubilized ZP proteins on mouse sperm. We show that the ZP-induced pHi increase is required to evoke Ca2+ influx via CatSper. The pHi increase is abolished upon depolarization, which underlies the Vm-control of CatSper activation by ZP proteins. Moreover, we show that the ZP-induced pHi increase rests on Na+/H+ exchange by NHA1, but not by sNHE, and requires cAMP synthesis by the soluble adenylyl cyclase sAC. Altogether, our findings answer long-standing questions about the molecular mechanisms underlying ZP action on mouse sperm.
Results
ZP-Induced Ca2+- and pHi-Signaling Events in Mouse Sperm
We studied the action of solubilized ZP proteins on mouse sperm. In mice, the ZP consists of three glycoproteins (mZP1-3). In line with previous studies (Avella et al., 2014), staining of isolated oocytes with antibodies against mZP1, mZP2, and mZP3 labeled the ZP surrounding the oocyte (Figure 1A). The specificity of the anti-ZP antibodies was confirmed by detection of heterologously expressed mZP1, mZP2, and mZP3 in both immunocytochemistry and Western blots (Supplementary Figure S1 and Figure 1C). On Western blots of solubilized ZPs isolated from mouse oocytes, the antibodies detected proteins with apparent molecular weights (Mw) of about 150, 100, and 83 kDa for mZP1, mZP2, and mZP3, respectively (Figure 1B), similar to what has been shown previously (Bleil and Wassarman, 1980; Wassarman, 1988; Thaler and Cardullo, 1996). Treatment with PNGase F decreased the Mw, demonstrating that glycosylation of ZP proteins was preserved during isolation (Figure 1B).
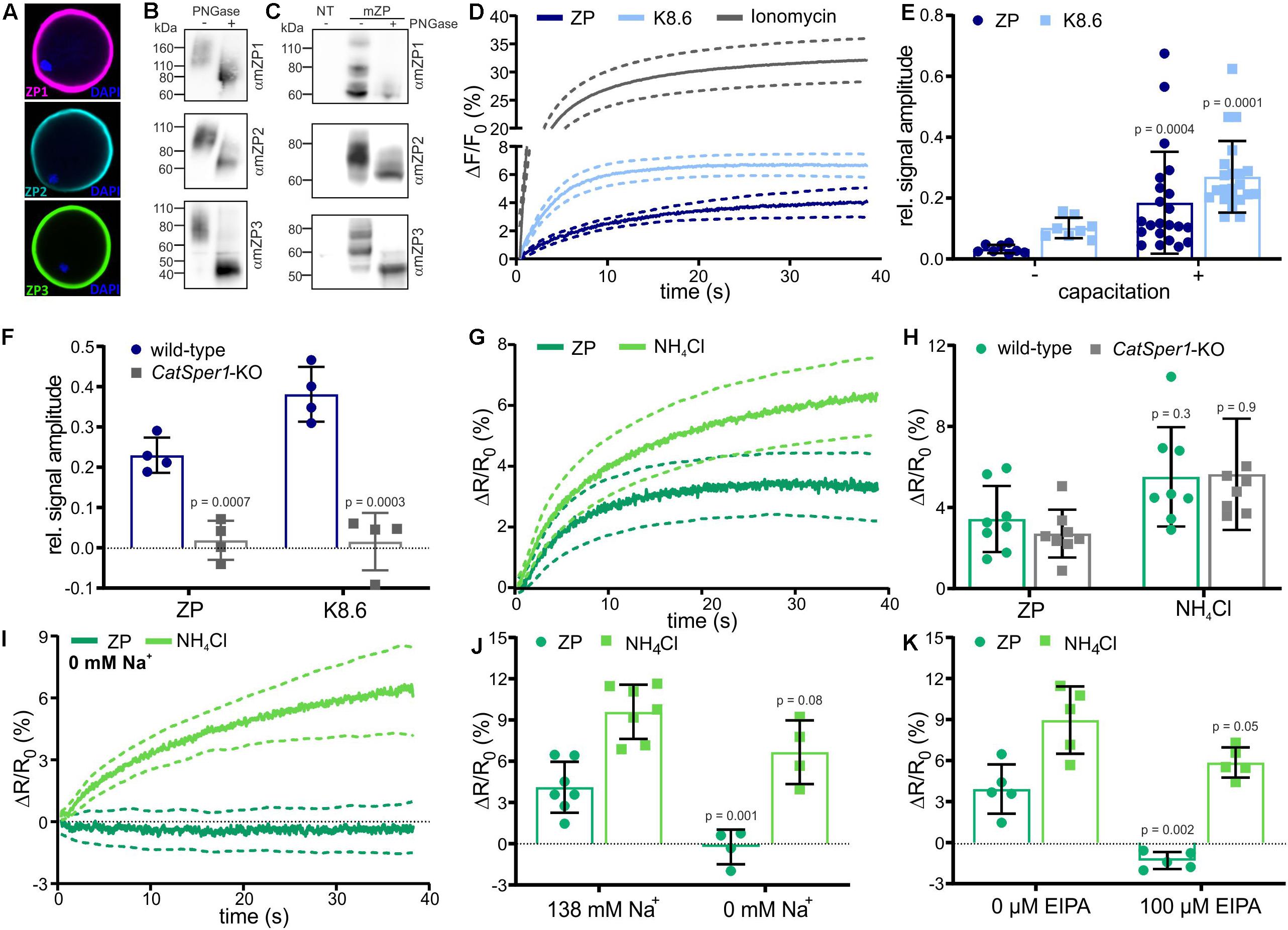
Figure 1. Changes in pHi and [Ca2+]i stimulated by solubilized zona pellucida (ZP) glycoproteins in mouse sperm. (A) Mouse oocytes labeled with antibodies directed against mZP1 (purple), mZP2 (cyan), and mZP3 (green); the DNA was labeled using DAPI (blue). (B) Western blot of solubilized ZP glycoproteins before (–) and after (+) PNGase-F treatment. The blots were probed with ZP isoform-specific antibodies. (C) Western blot of heterologously-expressed mouse ZP glycoproteins before (–) and after (+) PNG-F treatment, probed with mZP isoform-specific antibodies; NT: non-transfected cells. (D) Ca2+ responses in populations of sperm evoked by mixing with 1 ZP/μl, K8.6 buffer, or 2 μM ionomycin; shown are the averages (solid lines, n = 7) and the 95% confidence interval (dashed lines). Shown is the percent change in fluorescence (ΔF) with respect to the mean of the first three data points recorded immediately after mixing (F0). The control ΔF/F0 signal observed upon mixing with buffer (control) was subtracted from K8 6-, ZP-, or ionomycin-induced signals, setting the control-signal level to ΔF/F (%) = 0. (E) Relative amplitude of the Ca2+ responses evoked by mixing with 1 ZP/μl or K8.6 (mean ± SD of the average of the last three data points, n≥7) in non-capacitated (–) and capacitated (+) sperm, normalized to the ionomycin-evoked Ca2+ responses. (F) Relative amplitude of Ca2+ responses in wild-type and Catsper1-KO sperm (n = 4). (G) pHi responses evoked by mixing with 1 ZP/μl or 10 mM NH4Cl in mouse sperm populations; shown are averages (solid lines, n = 7) and the 95% confidence interval (dashed lines). Shown is the percent change in fluorescence ratio (ΔR) with respect to the mean of the first three data points recorded immediately after mixing (R0). The control ΔR/R0 signal observed upon mixing with buffer (control) was subtracted, setting the control-signal level to ΔR/R0 (%) = 0. (H) Amplitude of pHi responses (mean ± SD of the average of the last three data points, n≥ 7) evoked by mixing with 1 ZP/μl or 10 mM NH4Cl in wild-type and Catsper1-KO sperm (n = 4). (I) pHi responses in sperm bathed in 0 mM Na+ buffer (n = 4). (J) Amplitude of pHi responses at 138 or 0 mM extracellular Na+ (mean ± SD, n = 4). (K) Amplitude of pHi responses in the absence (–) or presence (+) of 100 μM EIPA (mean ± SD, n = 4). Statistical significance between two groups was determined using two-tailed, unpaired t-test with Welch’s correction; p-values are indicated.
We analyzed the action of ZP proteins on mouse sperm using a stopped-flow apparatus and fluorescent probes for Ca2+ and pHi. Mixing of capacitated sperm (incubated for 90 min in buffer containing 3 mg/ml BSA and 25 mM at pH 7.4) with ZP proteins evoked a rapid Ca2+ increase that reached a plateau within 30–40 s (Figure 1D); the control signal evoked by mixing with buffer alone was subtracted, setting the control-signal level consistently to ΔF/F0 (%) = 0. Simultaneous alkalization and depolarization of sperm by mixing with buffer adjusted to pH 8.6 and containing a high K+ and a low Na+ concentration (dubbed K8.6 buffer) (Babcock and Pfeiffer, 1987) also evoked a rapid Ca2+ increase that reached a slightly higher [Ca2+]i (Figure 1D). As a reference for the maximal signal amplitude at saturation of the Ca2+ indicator, we recorded the Ca2+ response evoked by the Ca2+ ionophore ionomycin (Figure 1D). ZP proteins, K8.6 buffer, and ionomycin also evoked Ca2+ responses in non-capacitated sperm (Figure 1E and Supplementary Figures S2a,b). Yet, relative to the reference signal evoked by ionomycin, the amplitudes of ZP- and K8.6-evoked Ca2+ response were enhanced upon capacitation (Figure 1E and Supplementary Figure S2b). To verify that the Ca2+ responses are carried by CatSper, we measured Ca2+ responses in wild-type and CatSper1-deficient mice. Indeed, the Ca2+ responses were abolished in sperm from Catsper1 deficient-mice (Catsper1-KO, Figure 1F) (Xia and Ren, 2009).
Next, we studied ZP-induced pHi responses. Mixing of capacitated sperm with ZP proteins evoked a rapid pHi increase that reached a plateau after 20–30 s (Figure 1G). Mixing of sperm with the weak base NH4Cl as a positive control also evoked a rapid pHi increase that reached a slightly higher pHi (Figure 1G). The ZP- and NH4Cl-evoked pHi responses were similar in wild-type and Catsper1-KO sperm (Figure 1H).
Altogether, these results confirm that ZP action on sperm involves an increase of pHi (Arnoult et al., 1996b) and a Ca2+ influx via CatSper (Xia and Ren, 2009), and that capacitation enhances the Ca2+ response (Arnoult et al., 1999). Moreover, because the pHi increase is preserved in Catsper1-KO mice, we conclude that the pHi increase is not evoked by Ca2+ influx via CatSper. Instead, the ZP-induced pHi increase might underlie the ZP-activation of CatSper.
The ZP-Induced Alkalization Does Not Involve the Na+/H+ Exchanger sNHE
We aimed to unravel the molecular players underlying the ZP-evoked alkalization. Na+/H+ exchange via sNHE has been proposed to take part in the ZP-evoked alkalization (Chavez et al., 2014). In addition to sNHE, two members of the Na+/H+ antiporter (NHA) subfamily, NHA1 and NHA2 (encoded by the Slc9b1 and Slc9b2 genes, respectively), have been identified in mouse sperm (Liu et al., 2010; Chen et al., 2016). Thus, NHA1 and NHA2 are also candidates to mediate the ZP-induced alkalization by Na+/H+ exchange.
We first probed the role of Na+/H+ exchange in the ZP-induced alkalization using Na+ substitution and pharmacology. Indeed, the ZP-induced pHi response was abolished by substitution of extracellular Na+ by NMDG (N-methyl-D-glucamine) or addition of EIPA, a commonly used non-selective inhibitor of Na+/H+ exchangers (Shi et al., 2017). The NH4Cl-induced pHi response was, however, similar in the absence or presence of Na+ or EIPA (Figures 1I–K). These results confirm that the ZP-induced pHi response depends on Na+/H+ exchange.
We examined if ZP-induced Na+/H+ exchange is mediated by sNHE using Slc9c1 knockout-mice (Slc9c1-KO) (Wang et al., 2003). In Slc9c1-KO sperm, the ZP-induced pHi and Ca2+ responses were abolished, whereas the pHi and Ca2+ response evoked by NH4Cl and K8.6, respectively, was preserved (Figures 2A,B). However, sNHE interacts with the soluble adenylyl cyclase sAC, encoded by Adcy10 (Wang et al., 2007), which constitutes the principal source of cAMP in mammalian sperm (Esposito et al., 2004; Hess et al., 2005; Xie et al., 2006); Slc9c1-KO sperm lack functional sAC and, therefore, cAMP synthesis (Wang et al., 2007). We wondered whether the failure of ZP proteins to increase [Ca2+]i and pHi in Slc9c1-KO is due to the lack of sNHE, sAC, or both. To test this, we used optogenetics and the membrane-permeable cAMP analog db-cAMP to rescue intracellular cAMP levels. Transgenic expression of the photoactivated adenylyl cyclase bPAC in Slc9c1-KO sperm provides a tool to stimulate cAMP synthesis in a light-dependent manner (Jansen et al., 2015). Light-stimulated cAMP synthesis in Slc9c1-KO/bPAC sperm or incubation of Slc9c1-KO sperm in db-cAMP both restored the ZP-induced pHi and Ca2+ response (Figures 2C–F). Thus, the Na+/H+ exchange stimulated by ZP proteins does not require sNHE, but rather cAMP synthesis by sAC. Using sperm that express the FRET-based cAMP biosensor mlCNBD-FRET (Mukherjee et al., 2016), we investigated whether ZP proteins enhance sAC activity and, thereby, control intracellular cAMP synthesis. Mixing of mlCNBD-FRET sperm with ZP proteins did not increase cAMP levels, whereas activation of sAC by using 25 mM NaHCO3 as a control evoked a pronounced cAMP increase (Figure 2G), demonstrating that ZP proteins do not control cAMP levels in sperm. Altogether, we conclude that the ZP-induced Na+/H+ exchange is not mediated by sNHE, but requires cAMP.
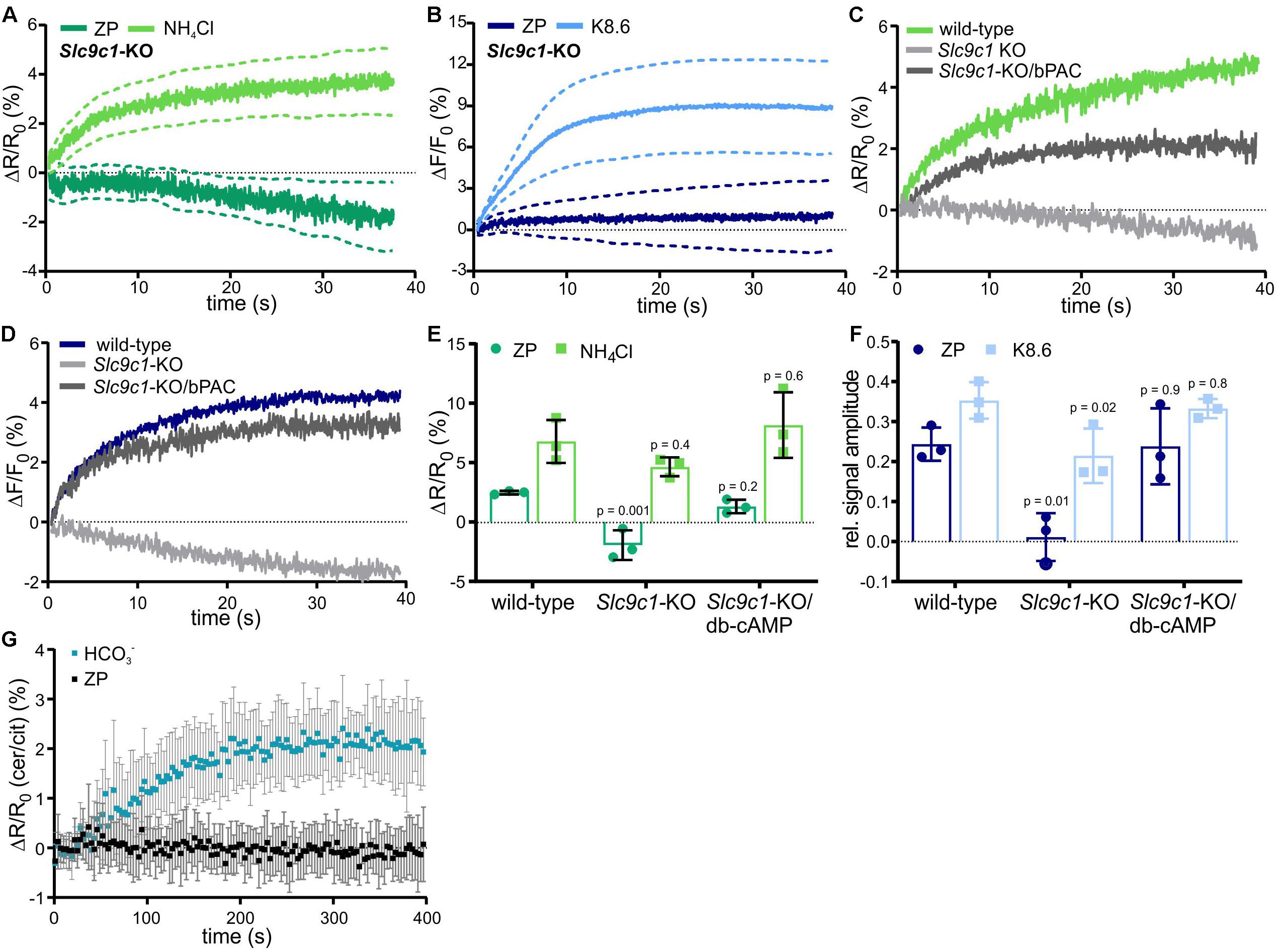
Figure 2. ZP-induced pHi and Ca2+ responses in sperm lacking sNHE. Intracellular pH (A) and Ca2+ (B) responses in Slc9c1-KO sperm mean ± 95% confidence interval (n = 4). (C) Representative pHi responses evoked by 1 ZP/μl in wild-type, Slc9c1-KO, and light-stimulated Slc9c1-KO/bPAC sperm. (D) Representative Ca2+ responses evoked by mixing with 1 ZP/μl in wild-type, Slc9c1-KO, and light-stimulated Slc9c1-KO/bPAC sperm. (E) Amplitudes of pHi and (F) relative amplitudes of Ca2+ responses in wild-type, Slc9c1-KO, and Slc9c1-KO sperm pre-incubated with 5 mM db-cAMP (mean ± SD, n = 4). (G) Changes in the cerulean/citrine FRET ratio in mlCNBD-FRET sperm evoked by mixing with 50 mM NaHCO3 or 1 ZP/μl. An increase of the FRET ratio indicates an increase of free intracellular cAMP; mean ± SD, n = 5. Statistical comparison between multiple groups was performed using one-way ANOVA with Dunnett’s correction; p-values are indicated.
The ZP-Induced Alkalization Involves the Na+/H+ Exchanger NHA1
NHA1, a member of the Na+/H+ antiporter subfamily encoded by the Slc9b1 gene, was identified in the flagellum of mouse sperm (Liu et al., 2010; Chen et al., 2016). Slc9b1-KO sperm suffer from impaired motility, resulting in male subfertility (Chen et al., 2016). To investigate the role of NHA1 in the ZP-induced alkalization, we generated Slc9b1-KO mice. Slc9b1-KO mice were born in Mendelian ratios from heterozygous matings, and the mice were viable without any gross phenotype. The recombinant Slc9b1 locus contains the lacZ gene, expressing beta-galactosidase under the control of the Slc9b1 promoter (Figure 3A). Gene targeting was verified by PCR (Figure 3B). Labeling of beta-galactosidase in wild-type and heterozygous Slc9b1+/lacZ testis sections and on Western blots of Slc9b1+/lacZ testis and sperm lysates demonstrated that the Slc9b1 gene is expressed in developing sperm (Figures 3C,D). Protein mass spectrometry identified NHA1 in wild-type sperm lysates (Supplementary Table S1). Furthermore, an anti-NHA1 antibody labeled the flagellum of wild-type, but not of Slc9b1-KO sperm (Figure 3E) and, as control, HEK293 cells heterologously expressing mouse NHA1 (Figure 3F). Altogether, these results confirm that NHA1 is expressed in wild-type, but not in Slc9b1-KO mouse sperm (Liu et al., 2010; Chen et al., 2016).
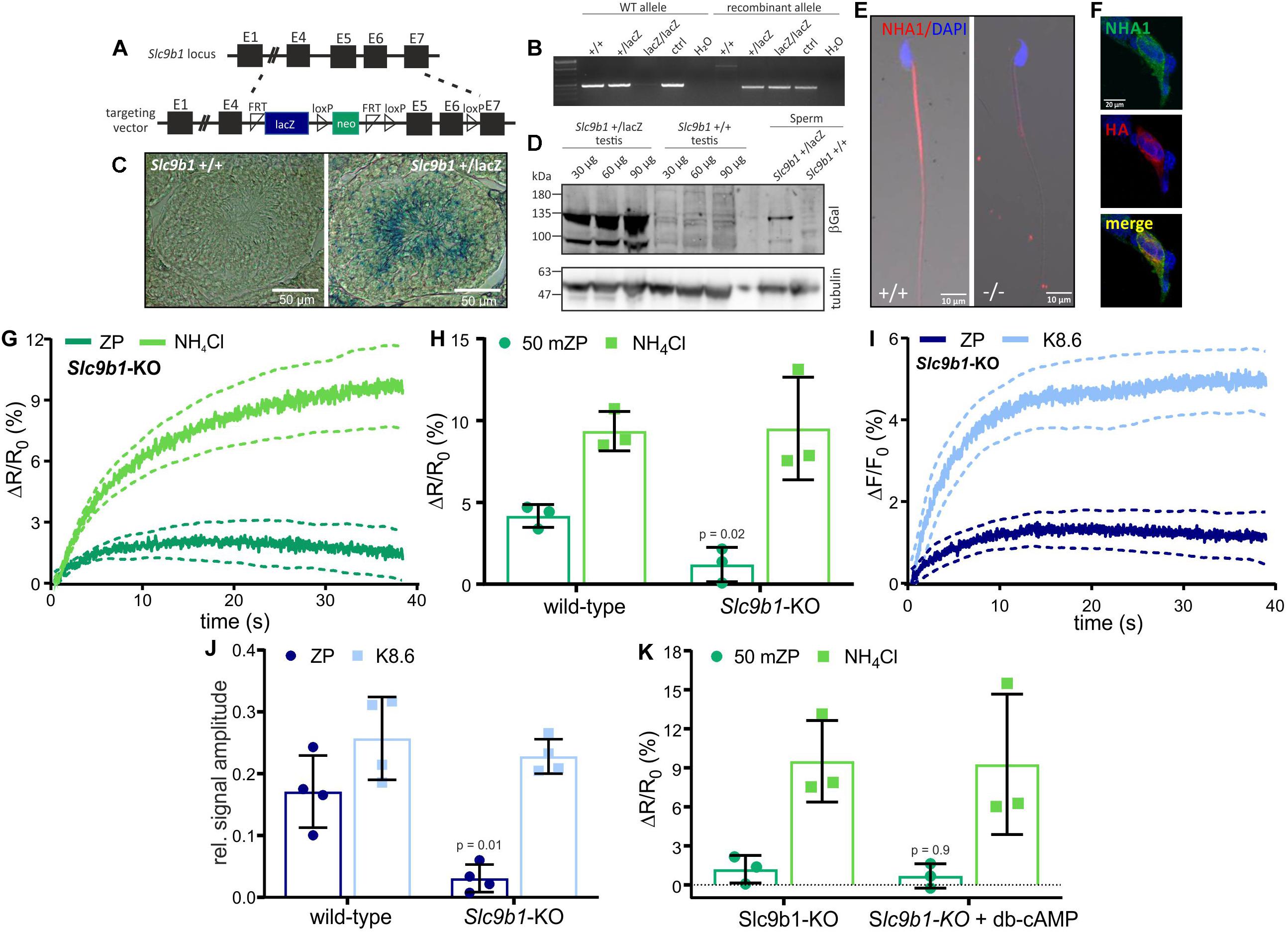
Figure 3. ZP-induced pHi and Ca2+ response in sperm lacking NHA1. (A) Targeting strategy to delete the Slc9b1 gene to generate Slc9b1-KO mice. (B) Genotyping PCR of wild-type (+/+), heterozygous (+ /lacZ), and homozygous (lacZ/lacZ) knockout mice. (C) X-gal staining of Slc9b1+/+ and Slc9b1+/lacZ testis section; scale bar: 50 μm. (D) Western blot of testis and sperm lysates from Slc9b1+/+ and Slc9b1+/lacZ mice, probed with anti-beta-galactosidase and anti-beta tubulin antibodies. (E) Immunostaining of wild-type and Slc9b1-KO sperm with an anti-NHA1 antibody; scale bar: 10 μm. (F) HEK293T cells heterologously-expressing NHA1, stained with an anti-NHA1 (green) and an anti-HA-tag antibody (red), co-localization shown in yellow, scale bar = 20 μm. (G) pHi responses evoked by mixing with 1 ZP/μl or 10 mM NH4Cl in Slc9b1-KO mouse sperm; average ± 95% confidence interval (n = 3). (H) Amplitude of pHi responses in wild-type or Slc9b1-KO mouse sperm; mean ± SD (n = 3). (I) Ca2+ responses evoked by mixing with 1 ZP/μl or K8.6 in Slc9b1-KO mouse sperm; average ± 95% confidence interval (n = 4). (J) Relative amplitude of Ca2+ responses evoked by mixing with 1 ZP/μl or K8.6 in wild-type or Slc9b1-KO mouse sperm; mean ± SD (n = 4). (K) Amplitude of pHi responses in Slc9b1-KO sperm in the absence and presence of 5 mM db-cAMP; mean ± SD (n = 3). Statistical significance between two groups was determined using two-tailed, unpaired t-test with Welch’s correction; p-values are indicated.
We investigated the action of ZP proteins on Slc9b1-KO sperm. The ZP-induced pHi and Ca2+ responses were strongly attenuated, whereas the pHi and Ca2+ responses evoked by NH4Cl or K8.6, respectively, were similar in wild-type and Slc9b1-KO (Figures 3G–J). These results suggest that the ZP-induced alkalization depends predominantly on Na+/H+ exchange via NHA1. However, genetic ablation of NHA1 reportedly affects sAC expression and, thereby, intracellular cAMP levels (Chen et al., 2016). Thus, the phenotype of Slc9b1-KO sperm might be caused by impaired or abolished cAMP synthesis, resembling the Slc9c1-KO phenotype (Wang et al., 2003). Yet, in contrast to Slc9c1-KO sperm, db-cAMP did not restore the ZP-induced pHi response in Slc9b1-KO sperm (Figure 3K). These results indicate that lack of NHA1, rather than impaired cAMP synthesis, underlies the attenuated ZP-induced pHi response in Slc9b1-KO sperm. We surmise that the residual Na+/H+ exchange in Slc9b1-KO mice is either mediated by NHA2, by another unknown Na+/H+ exchanger, or a combination of both.
Genetic Ablation of NHA1 Affects Sperm Motility
Considering that the ZP-induced alkalization is predominantly mediated by NHA1, we analyzed the phenotype of Slc9b1-KO mice in more detail. In line with previous results, the fertility of Slc9b1-KO males was severely impaired (Chen et al., 2016): only 2 out of 21 matings (Slc9b1-KO males with wild-type females) produced offspring (Supplementary Table S2). The testis and epididymis weight and average sperm count were similar in wild-type and Slc9b1-KO mice (Supplementary Table S2), yet Slc9b1-KO sperm largely failed to fertilize oocytes in vitro (Supplementary Table S2), which might be attributed to the severely impaired ZP-induced Ca2+ and pHi signaling. In previous studies, the number of sperm cells that were motile was significantly reduced in Slc9b1-KO mice (Chen et al., 2016). We observed a similar phenotype: only 55 ± 5% of Slc9b1-KO sperm showed progressive motility compared to 86 ± 5% of wild-type sperm. The decrease in motility has been attributed to a reduced sAC protein expression level (Chen et al., 2016). Thus, we investigated sAC function in wild-type versus Slc9b1-KO sperm. To this end, we studied the flagellar beat frequency (Hansen et al., 2018), which is controlled by sAC: activation of sAC by NaHCO3 rapidly increases intracellular cAMP levels and the flagellar beat frequency. In sperm that lack sAC, cAMP synthesis and the action of NaHCO3 is abolished, rendering the sperm immotile (Wennemuth et al., 2003; Esposito et al., 2004; Hess et al., 2005; Xie et al., 2006; Carlson et al., 2007). To investigate whether sAC dysfunction underlies the defect in sperm motility in Slc9b1-KO sperm, we compared the basal flagellar beat frequency, determined at about 60 μm distance from the center of the sperm head, between wild-type and Slc9b1-KO sperm. Under basal conditions, the beat frequency was similar (WT: 11 ± 3 and KO: 13 ± 3 Hz, n ≥ 13), and stimulation with 25 mM NaHCO3 increased the frequency to a similar extent (WT: 20 ± 6% and KO: 22 ± 9%, n ≥ 13). Thus, the lack of NHA1 does not impair the sAC-control of flagellar beat frequency, suggesting that cAMP synthesis is not impaired.
When analyzing the flagellar beat in detail, we noticed that the beat frequency along the flagellum was not uniform in Slc9b1-KO (Figures 4A,B and Supplementary Movies S1–S2). At ≤ 80 μm distance from the sperm head, the flagellar beat frequency was similar in Slc9b1-KO and wild-type sperm, whereas at > 80 μm, the frequency was considerably faster in Slc9b1-KO sperm (Figure 4B). Strikingly, Slc9b1-KO sperm displayed a stiff midpiece, which prevented to reliably determine the beat frequency at the first 20 μm of the flagellum. To describe this defect in quantitative terms, we compared the amplitudes of the curvature angle along the flagellum as a measure for the beat amplitude (Figure 4C) (Hansen et al., 2018). In the midpiece, the amplitude was lower in Slc9b1-KO sperm compared to wild-type sperm, reflecting the restricted movement (Figure 4C). Farther along the flagellum, the amplitude was similar between wild-type and Slc9b1-KO sperm (Figure 4C).
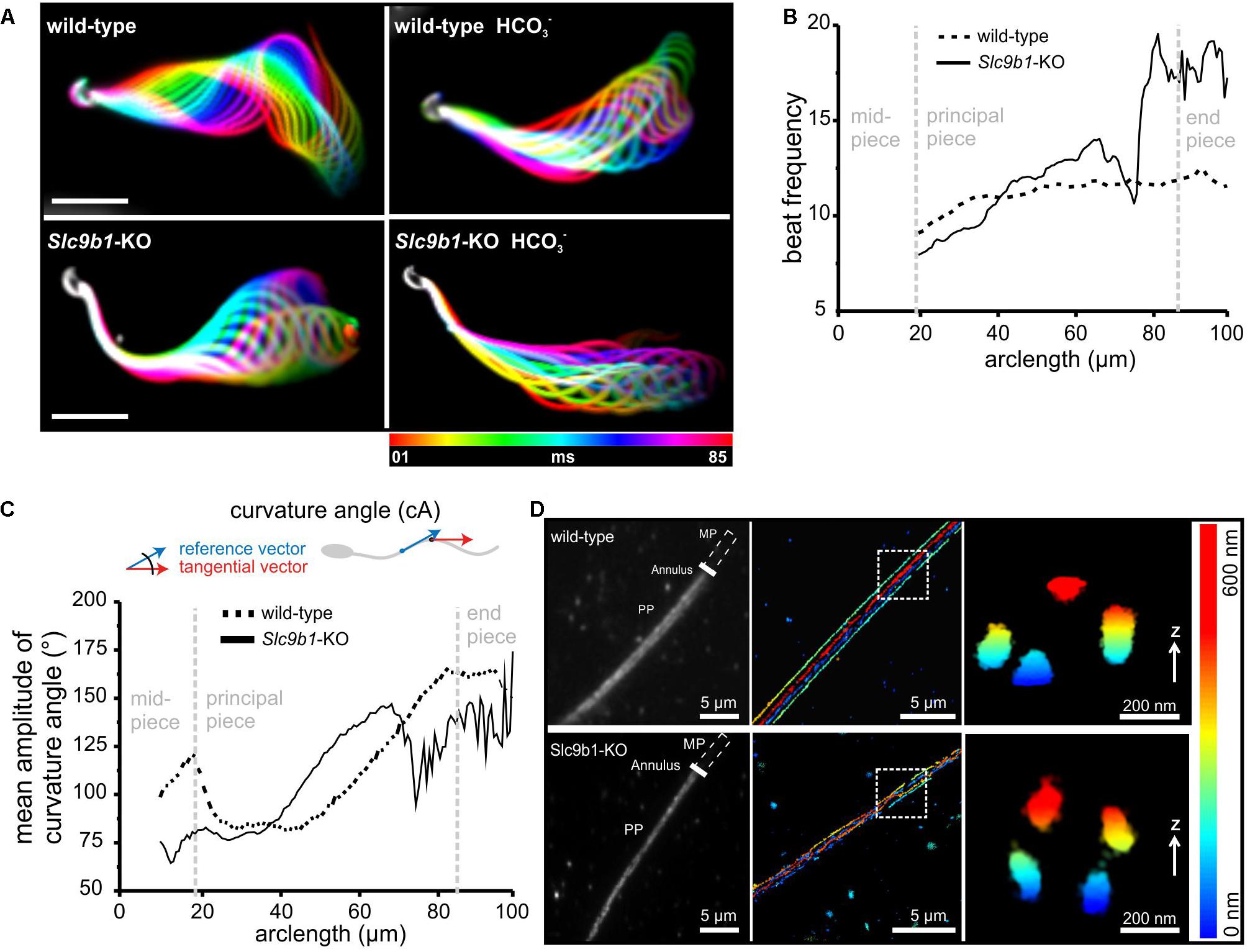
Figure 4. Flagellar beating pattern and ultrastructure in sperm lacking NHA1. (A) Flagellar waveform of wild-type and Slc9b1-KO sperm before and after stimulation with 25 mM NaHCO3. Superimposed color-coded frames taken every 5 ms, illustrating one flagellar beat cycle; scale bar: 30 μm. (B) Mean flagellar beat frequency along the flagellum of wild-type and Slc9b1-KO sperm (n = 5). (C) Mean amplitude of the curvature angle along the flagellum of wild-type and Slc9b1-KO sperm (n = 5). (D) Left: Epifluorescence image of wild-type and Slc9b1-KO sperm labeled with an anti-CatSper1 antibody. Position of the midpiece (MP), the annulus, and the principal piece (PP) is indicated. Middle: 3D-STORM image of the principal piece of wild-type and Slc9b1-KO sperm in x-y projection and color-coded for z; scale bars are indicated. Position of Z cross-section is indicated by white square. Right: Z cross-section of principal piece showing the four CatSper-organized signaling domains along the flagellum of wild-type and Slc9b1-KO sperm.
A stiff midpiece has also been observed in sperm lacking calcineurin or the CatSper-channel subunit CatSper ζ (Miyata et al., 2015; Chung et al., 2017). The CatSper-channel complex forms Ca2+ signaling domains along the flagellum that are organized in four longitudinal columns (Chung et al., 2014). Loss of the channel complex disrupts this organization (Chung et al., 2014, 2017). To examine whether a defect in the Ca2+ signaling domains might underlie the stiff midpiece in Slc9b1-KO sperm, we used super-resolution microscopy (3D-STORM). In wild-type and Slc9b1-KO sperm, an anti-CatSper1 antibody labeled four distinct columns aligned longitudinally along the flagellum, as previously described for wild-type sperm using the same antibody (Chung et al., 2014), demonstrating that the Ca2+-signaling domains are preserved in Slc9b1-KO sperm (Figure 4D). Furthermore, the density distribution of CatSper1 labeling along the flagellum was not different between wild-type and Slc9b1-KO sperm (Supplementary Figure S3). Thus, the molecular mechanism underlying the motility defect of Slc9b1-KO sperm remains unclear, but we propose that a combination of defective ZP signaling and altered flagellar beat pattern in Slc9b1-KO sperm underlies the sub-fertility of male Slc9b1-KO mice.
ZP-Induced Signaling Requires a Sufficiently Negative Membrane Potential
Although it is unknown how ZP proteins activate Na+/H+ exchange via NHA1, the membrane potential seems to be an important factor (Zeng et al., 1995; Arnoult et al., 1999; De La Vega-Beltran et al., 2012; Chavez et al., 2014). Thus, we tested the role of the Vm in ZP signaling. In wild-type sperm, that were depolarized by incubation in high extracellular potassium ([K+]o = 138 mM), the ZP-induced pHi and Ca2+ responses were abolished, whereas the pHi and Ca2+ responses evoked by NH4Cl or K8.6, respectively, were preserved (Figures 5A–D). This suggests that the ZP-induced pHi increase requires a more negative Vm. Slo3 and its auxiliary subunit Lrrc52 form the principal K+ channel in mouse (Santi et al., 2010; Yang et al., 2011; Zeng et al., 2011, 2015) and human sperm (Brenker et al., 2014). We studied the action of ZP proteins on Kcnu1- and Lrrc52-KO sperm, which both feature a depolarized Vm (Santi et al., 2010; Yang et al., 2011; Zeng et al., 2011, 2015). In Kcnu1-KO and Lrrc52-KO sperm, the ZP-evoked pHi and Ca2+ responses were abolished, whereas the pHi and Ca2+ responses evoked by NH4Cl and K8.6, respectively, were preserved (Figures 5E,F,I–L). Remarkably, hyperpolarizing Kcnu1- and Lrrc52-KO sperm using the K+ ionophore valinomycin (Santi et al., 2010; Chavez et al., 2014) restored the ZP-induced pHi and Ca2+ responses (Figures 5G–I,L), supporting the notion that ZP signaling requires a sufficiently negative Vm. Altogether, these results corroborate that a negative Vm, set by Slo3, enables the ZP-induced pHi increase and, thereby, ZP-induced Ca2+ influx via CatSper. However, the mechanism underlying the Vm-control of NHA1 remains to be elucidated.
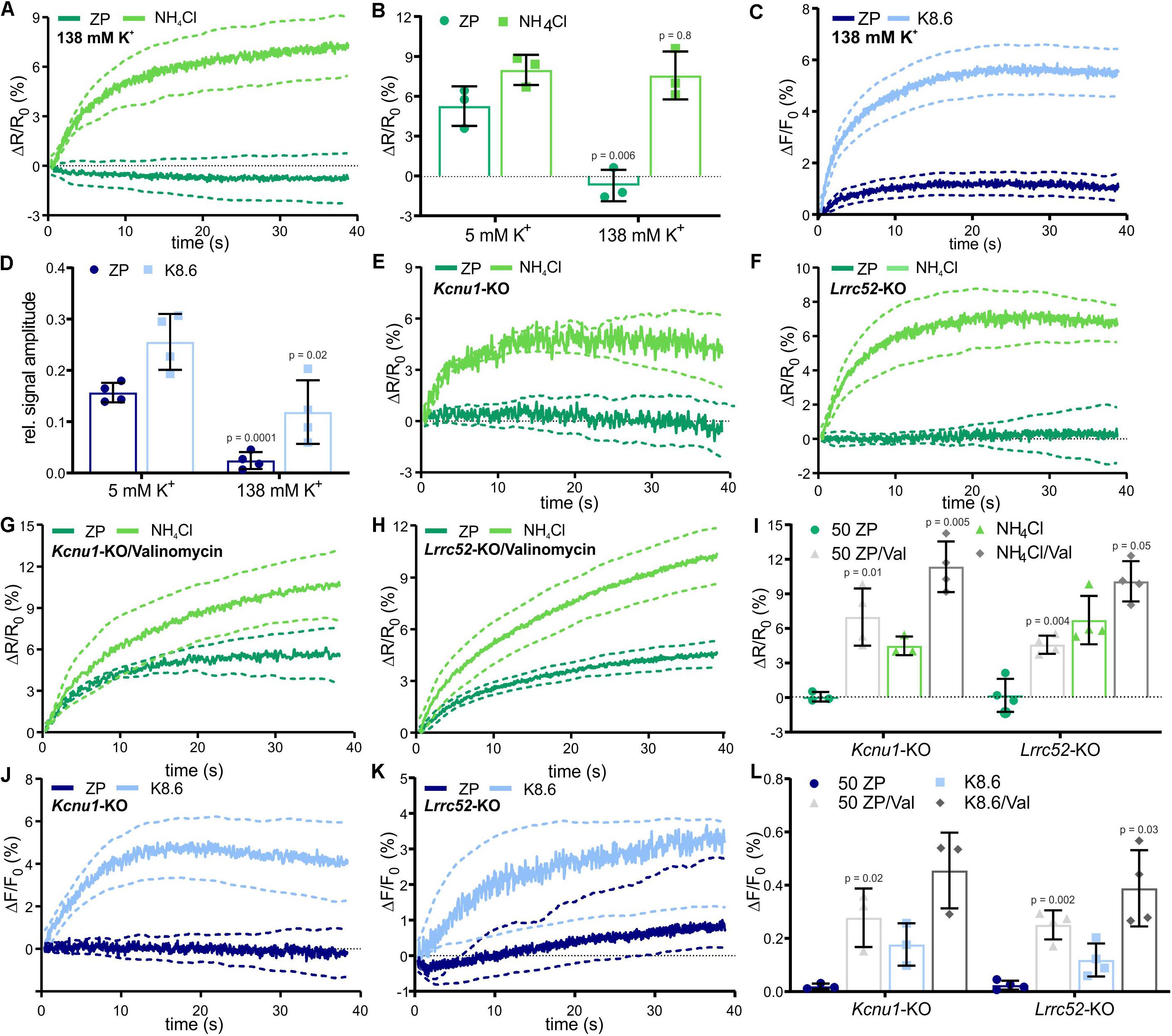
Figure 5. ZP-evoked pHi and Ca2+ responses in mouse sperm lacking Slo3 or its auxiliary subunit Lrrc52. (A) pHi responses evoked by mixing with 1 ZP/μl or 10 mM NH4Cl in sperm bathed in 138 mM K+ buffer; average ± 95% confidence interval (n = 3). (B) Amplitude of pHi responses evoked by mixing with 1 ZP/μl or 10 mM NH4Cl in sperm bathed in 5 mM or 138 mM K+ buffer; mean ± SD (n = 3). (C) Ca2+ responses evoked by mixing with 1 ZP/μl or K8.6 in sperm bathed in 138 mM K+ buffer; average ± 95% confidence interval (n = 3). (D) Relative amplitude of Ca2+ responses evoked by mixing with 1 ZP/μl or K8.6 in sperm bathed in 5 or 138 mM K+ buffer; mean ± SD (n = 3). (E,F) pHi responses evoked by mixing with 1 ZP/μl or 10 mM NH4Cl in Kcnu1-KO (E) and Lrrc52-KO (F) sperm; average ± 95% confidence interval (n = 3). (G,H) pHi responses evoked by mixing with 1 ZP/μl or 10 mM NH4Cl in Kcnu1-KO (G) and Lrrc52-KO (H) sperm bathed in 2 μM valinomycin; average ± 95% confidence interval (n = 3). (I) Amplitude of pHi responses evoked by mixing with 1 ZP/μl or 10 mM NH4Cl in Kcnu1-KO and Lrrc52-KO sperm in the absence and presence of 2 μM valinomycin; mean ± SD (n = 3). (J,K) Ca2+ responses evoked by mixing with 1 ZP/μl and K8.6 in Kcnu1-KO (J) and Lrrc52-KO (K) sperm; average ± 95% confidence interval (n = 3). (L) Relative amplitude of Ca2+ evoked by mixing with 1 ZP/μl or K8.6 in Kcnu1-KO and Lrrc52-KO sperm in the absence and presence of 2 μM valinomycin; mean ± SD (n = 3). Statistical significance between two groups was determined using two-tailed, unpaired t-test with Welch’s correction; p-values are indicated.
Discussion
The function of mammalian sperm is controlled by external cues that engage various signaling molecules. How these molecules are integrated into signaling pathways is not well-understood. Here, we show that the synthesis of cAMP and a sufficiently negative membrane potential prime mouse sperm to transduce binding of ZP proteins into rapid H+ and Ca2+ signaling events (Figure 6). This ZP-induced Ca2+ increase might be involved in the control of swimming behavior and acrosomal exocytosis.
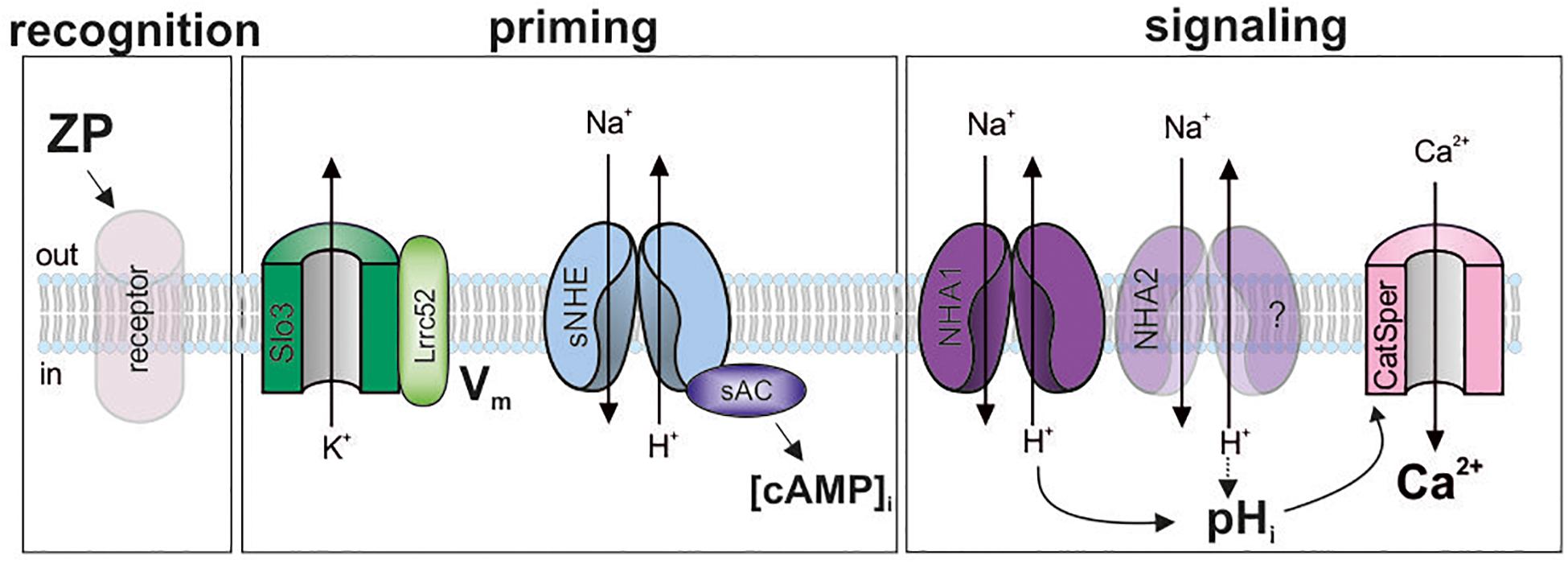
Figure 6. Model of the ZP-signaling pathway in mouse sperm. ZP glycoproteins bind to a yet unknown receptor (recognition), which initiates a signaling cascade. This cascade requires a hyperpolarized membrane potential (Vm), set by the Slo3/Lrrc52-channel complex, and cAMP synthesis by sAC (priming). Thereby, a pHi response, resting on Na+/H+ exchange by NHA1, and probably NHA2, is enabled that promotes Ca2+ influx through CatSper (signaling). Shaded objects indicate that the signaling mechanism and function of the molecules has not been established yet.
The CatSper channel is the principal pathway for Ca2+ entry into mammalian sperm (Quill et al., 2001; Ren et al., 2001; Kirichok et al., 2006; Lishko and Kirichok, 2010). We propose that the action of ZP proteins on CatSper is indirect, mediated by a pHi-signaling pathway (Figure 6): any experimental condition that abolishes the ZP-induced pHi increase abolishes the Ca2+ influx, and vice versa, conditions that restore the pHi response also restore the Ca2+ influx. This result is consistent with earlier reports, demonstrating a ZP-evoked increase of pHi and [Ca2+]i in mouse sperm (Bailey and Storey, 1994; Murase and Roldan, 1996). In patch-clamp experiments, ZP proteins did not enhance monovalent CatSper currents recorded from sperm isolated from the corpus of the ductus epididymis (Xia and Ren, 2009). Yet, sperm from this region of the epididymis are unable to undergo capacitation (Yanagimachi, 1994), which potentiates the Ca2+ response (see Figure 1D). Thus, the use of non-capacitated sperm might have hampered the detection of a direct ZP protein action on CatSper. Nevertheless, these results support our model that in mouse sperm, ZP proteins activate CatSper via intracellular alkalization (Figure 6). The ZP-induced pHi increase rests on Na+/H+ exchange that is predominantly mediated by NHA1 (Figure 6). The residual pHi response in Slc9b1-KO might be carried by NHA2; future work needs to address this question using Scl9b1/2 knockout mice. The pHi response is only observed at the negative Vm set by Slo3 (Santi et al., 2010; Zeng et al., 2011, 2015). It remains to be elucidated how the Vm dependence is integrated into the ZP-signaling pathway. In mouse sperm, K+ currents and, thus, Vm are strongly controlled by pHi (Navarro et al., 2007; Zeng et al., 2011, 2013). The control of the ZP-induced pHi response by Vm, the control of Vm by Ca2+ and pHi, and the interplay of CatSper and Slo3 during ZP signaling deserve further studies.
We identified cAMP as a key player in the ZP-signaling pathway in mouse sperm: basal cAMP synthesis by sAC is required for the pHi response and ensuing CatSper activation. sNHE, which might form a physical or functional complex with sAC, is however not responsible for the ZP-induced alkalization. It has been proposed that NHA1 and NHA2 control sAC expression and, thereby, cAMP synthesis and motility (Chen et al., 2016). We also find Slc9b1-KO males are infertile and feature a significantly reduced number of motile sperm. However, we show that sAC function is unaffected in NHA1-KO sperm as the control of the flagellar beat frequency by remains unchanged. Thus, the pathomechanism underlying the reduced number of motile sperm in Slc9b1-KO males remains to be elucidated. Yet, the loss of NHA1 alters the flagellar beat pattern along the flagellum with the midpiece being stiff and the rest of the flagellum being more flexible. Although the ZP-induced alkalization via NHA1 activates CatSper, the exchanger is not required for the organization of CatSper in quadrilateral columns. Future studies are required to elucidate whether NHA1 is part of these columns to form nanodomains of pH and Ca2+ signaling. Whether the loss of NHA1 affects other downstream processes that control the flagellar beat pattern or if the axonemal structure/dynein function is altered is not known and warrants further studies.
Sperm must undergo the acrosome reaction to penetrate through the ZP. Across species, binding of sperm to the native ZP, to isolated ZPs, as well as to solubilized ZP proteins evokes the acrosome reaction in sperm (Florman and Storey, 1982; Bleil and Wassarman, 1983; Cherr et al., 1986; O’Rand and Fisher, 1987; Cross et al., 1988; Florman and First, 1988; Uto et al., 1988; Arnoult et al., 1996a; Schroer et al., 2000; Tollner et al., 2003; Gupta et al., 2012). This suggested that in vivo, sperm undergo the acrosome reaction primarily upon binding to the ZP (Hirohashi and Yanagimachi, 2018). Recent studies utilizied fluorescently-labeled sperm from transgenic mouse models to determine the acrosomal status and acrosome reaction in live mouse sperm upon fertilization in vitro and ex vivo within the oviduct. These experiments revealed that at least in mice, most sperm undergo the acrosomal exocytosis during their journey across the oviduct or within the cumulus cell-matrix rather than upon binding to the zona pellucida (Jin et al., 2011; Hino et al., 2016; La Spina et al., 2016; Muro et al., 2016). Moreover, acrosome-reacted mouse sperm seem to reach the surface of the oocyte more readily than acrosome-intact sperm (Hildebrand et al., 2010), and mouse sperm can bind to the ZP without undergoing the acrosome reaction (Baibakov et al., 2007). Thus, the significance of the acrosomal exocytosis and signaling events evoked by binding of sperm to the ZP for fertilization in mice or other mammalian species still remain to be elucidated.
In summary, our study provides new insights and at the same time, raises new questions about the action of ZP proteins on mammalian sperm. The identification of the ZP receptor(s) and binding site(s) on sperm is certainly essential to delineate the whole ZP-signaling pathway. However, this question has remained unanswered since the initial characterization of ZP-sperm interaction (Bleil and Wassarman, 1980). Our results present NHA1, sAC, and Slo3/Lrrc52 as new players in the sperm ZP- signaling pathway. This might be the basis for future approaches, unraveling the molecular mechanisms underlying infertility and the design of new contraceptives.
Materials and Methods
Nomenclature
For most of the proteins, we have used the alias in the text. The official nomenclature is indicated in Table 1.
Generation of Slc9b1-KO Mice
Slc9b1-KO mice were generated by blastocyst injection of Slc9b1 mutant embryonic stem (ES) cells (EPD0187_1_D11, EUCOMM) into albino C57BL/6Tyr females (Transgenic Service, LIMES institute, University of Bonn) and backcrossed to C57Bl/6N. The offspring was genotyped by PCR using Slc9b1-specific primers (wild-type allele: 507 bp using #1: 5′-TAGGTTGAGTTCTACTACAATG-3′, #2: 5′-TAGAGTCCATAGTGCAATGAG-3′; floxed allele: 673 bp using #1/2; lacZ allele: 427 bp using #1 and #3: 5′-AGTCTTCCTGTCCAGG-3′). Mice used in this study were 2–5 months of age. Animal experiments were in accordance with the relevant guidelines and regulations and approved by the local authorities (LANUV) AZ 84-02.04.2012.A192 [intern BCI_10]. Fertility was tested using timed matings (wild-type females mated with Slc9b1-KO males overnight and separated after plug check the following morning). All females were plug positive, but only 2/21 Slc9b1-KO males produced offspring.
Transgenic and Knockout Mice
Catsper1-KO (Ren et al., 2001) and Slc9c1-KO mice (Wang et al., 2003) were provided by David Clapham (Janelia Research Campus, United States) and the Jackson laboratory, respectively. Kcnu1-KO and Lrrc52-KO mice were provided by Christopher Lingle (Yang et al., 2011; Zeng et al., 2015). Prm1-bPAC/Slc9c1-KO mice have been described before (Jansen et al., 2015).
Sperm Preparation
Mouse sperm were isolated as previously described (Raju et al., 2015). In brief, sperm were isolated by incision of the cauda epididymis followed by a swim-out in modified TYH medium (in mM: 135 NaCl, 4.8 KCl, 2 CaCl2, 1.2 KH2PO4, 1 MgSO4, 5.6 glucose, 0.5 sodium pyruvate, 10 lactic acid, 10 HEPES, pH 7.4 adjusted at 37°C with NaOH). After 15–30 min swim-out at 37°C, sperm were collected and counted. For capacitation, sperm were incubated for 90 min in TYH containing 3 mg/ml BSA and 25 mM NaHCO3 substituting 25 mM of the NaCl; the pH was adjusted to 7.4. Measurements under depolarized membrane potential were performed in TYH with 135 mM KCl and 5 mM NaCl at pH 7.4. For experiments in the absence of extracellular Na+, Na+ was substituted by N-methyl-D-glucamine (M2004, Sigma-Aldrich) and the pH was adjusted with HCl. Valinomycin and db-cAMP were added after swim-out and were present throughout the experiment. Prm1-bPAC/Slc9c1-KO sperm were isolated under dim red light. Light-activation of Prm1-bPAC/Slc9c1-KO sperm was performed in a custom-made rack equipped with blue LEDs during sperm capacitation. Experiments were performed with capacitated sperm unless otherwise indicated. The cAMP analogs (db-cAMP) were already added during the capacitation phase for 90 min.
Isolation of Mouse Zona pellucidae
For ZP isolation, wild-type female mice were super-ovulated by intraperitoneal injection of 10 I.U. hCG (human Chorionic Gonadotropin; ProSpec, Rehovot, Israel) 3 days before the experiment. 14 h before oocyte isolation, mice were injected with 10 I.U. PMSG (Pregnant Mare’s Serum Gonadotropin; ProSpec). Mice were killed by cervical dislocation and oviducts were dissected. Cumulus-enclosed oocytes were prepared from the oviducts in TYH buffer containing 300 μg/ml hyaluronidase (Sigma). After 15 min, cumulus-free oocytes were transferred into fresh buffer and washed twice. Zonae pellucidae and oocytes were separated by shear forces generated by expulsion from 50 nm pasteur pipettes. Zona pellucidae were counted, transferred into fresh buffer, diluted to a concentration of 1 ZP per ul, and solubilized by incubation at 75°C for 15 min (Thaler and Cardullo, 1996). Animal experiments were performed in accordance with the relevant guidelines and regulations and approved by the local authorities (LANUV) AZ84-02.05.40.13.127.
Heterologous Expression of ZP Glycoproteins
The cDNA sequence of mZP1, mZP2, and mZP3 was amplified via PCR. A hexa-histidine tag was inserted upstream of the conserved furin cleavage site, an AgeI restriction site was added to the 5′ end, and a Kpnl restriction site to the 3′ end by nested PCR. The PCR product was cloned into a pHLsec vector (kindly provided by Prof. Yvonne Jones, Wellcome Trust Center for Human Genetics, University of Oxford, United Kingdom) using AgeI and Kpnl. pHLsec-mZP1, pHLsec-mZP2 and pHLsec-mZP3 were transiently transfected in HEK293T cells (ATCC-CRL-3216) using polyethyleneimine (Life Technologies, Carlsbad, United States).
Western Blot Analysis
Total protein lysates were obtained by homogenizing the cells in lysis buffer (10 mM Tris/HCl, pH 7.6, 140 mM NaCl, 1 mM EDTA, 1% Triton X-100, mPIC protease inhibitor cocktail 1:500). Samples were incubated for 30 min on ice and centrifuged at 10,000 g for 5 min at 4°C. The protein concentration was determined by BCA assay. Prior to separation by SDS-PAGE, 50 isolated ZPs, cells, or tissue lysates were mixed with 4 × SDS loading buffer [200 mM Tris/HCl, pH 6.8, 8% SDS (w/v), 4% β-mercaptoethanol (vol/vol), 50% glycerol, 0.04% bromophenol blue] and heated for 5 min at 95°C. For Western blot analysis, proteins were transferred onto PVDF membranes (Merck Millipore, Billerica, United States), probed with antibodies, and analyzed using a chemiluminescence detection system. For deglycosylation, 50 ZPs were incubated for 1 h with PNGase-F (New England Biolabs) according to the manufacturer’s instructions.
Primary antibodies: anti-β-galactosidase (1:1000; Molecular Probes), anti-α-tubulin (1:5000; Sigma-Aldrich), antibodies against mouse ZP glycoproteins were isolated from the supernatant of hybridoma cell lines (mZP1: ATCC CRL-2464, mZP2: ATCC CRL-2463, mZP3: ATCC CRL-2463) and diluted 1:1000. Secondary antibody: goat-anti-rat, HRP conjugated (1:5000, Dianova), donkey-anti-rabbit, HRP conjugated (1:5000, Dianova).
Immunocytochemistry
Sperm smeared on positively charged microscope slides were dried at room temperature (RT). For antigen retrieval, sperm were incubated in citrate buffer (10 mM sodium citrate, 0.05% Tween-20, pH 6) in a steamer at 99°C for 20 min and washed in PBS for 15 min at RT. Immunocytochemical analysis of CatSper1 expression was performed without antigen retrieval. Transiently transfected HEK293T cells, oocytes, or sperm were incubated in 4% PFA for 15 min and washed in PBS. To block unspecific binding sites, samples were incubated for 1 h with blocking buffer [0.5% Triton X-100 and 5% ChemiBLOCKER (Merck Millipore) in 0.1M phosphate buffer, pH 7.4]. The primary antibody was diluted in blocking buffer and incubated overnight. The fluorescent secondary antibody was diluted in blocking buffer containing 0.5 mg/ml DAPI (Life Technologies) and incubated for 1 h. Images were taken on a confocal microscope (FV1000; Olympus). Primary antibodies: anti-NHA1 (1:100; Biorbyt), anti-CatSper1 (1:250; Santa Cruz, sc-21180) (Chung et al., 2014), anti-His (1:100, Millipore); primary antibodies against mouse ZP glycoproteins were isolated from the supernatant of hybridoma cell lines (mZP1: ATCC CRL-2464, mZP2: ATCC CRL-2463, mZP3: ATCC CRL-2463) and diluted 1:100; anti-HA antibody (Roche). Secondary antibodies: donkey anti-rat Alexa488 (1:500; Dianova), donkey anti-rat A647 (1:1000; Life technologies), donkey anti-mouse Cy5 (1:500; Dianova).
STORM Imaging and Analysis of Sperm Flagellar Proteins
STORM imaging experiments were performed in an imaging buffer (50 mM Tris, pH 8, 10 mM NaCl) with an oxygen scavenging system (0.5 mg/mL glucose oxidase, 40 μg/ml catalase, 10% glucose, and 10 mM 2-aminoethanethiol). 10.000–60.000 frames were acquired per data set using 647 nm excitation at 100 mW. A 405 nm laser was used to maintain an adequate number of localizations per frame. A cylindrical lens was introduced in the detection path for astigmatism 3D STORM acquisition. Perfect focus system from Nikon was used to minimize axial drift and a vibration isolation table was used to minimize lateral drift. STORM movies were analyzed as described previously using the Nikon software package based on a technology developed by Dr. Xiaowei Zhuang (Huang et al., 2008). Briefly, fluorescence peaks corresponding to individual molecules were identified in each frame and fit using least-squares or maximum-likelihood estimation with a two-dimensional Gaussian to determine the (x,y) position of each molecule. For 3D imaging, the ellipticity of the Gaussian fit was used to assign a z coordinate. Drift correction was applied using cross-correlation.
STORM images were rendered with each localization plotted as a Gaussian. Images were filtered to reject molecules with low photon number (below 500 photons). Molecules with aspect ratio higher than 1.5 for 2D and 2.5 for 3D datasets were rejected. Moreover, molecules that appear for > 10 consecutive frames were rejected. Non-specifically bound antibodies can give background in the STORM images, which appears as scattered localizations with low local densities. This background noise was removed by a local density filter. Low-density localizations were filtered out by removing a molecule if it was surrounded by fewer than 10 localizations in the 80 × 80 nm region.
Beta-Galactosidase Staining of Testis Sections
Testis of adult male mice were isolated, punctured twice with a cannula and incubated overnight at RT in 4% PFA. After a single washing step in PBS for 10 min, testis were transferred into a 10% sucrose solution for 1 h and subsequently incubated overnight in 30% sucrose. On the next day, testis were embedded in Tissue TEK (Sakura Finetek) and stored at −80°C. The testis was sectioned in 16 μm thick cross-sections using a 2800 Frigocut-E cryostat (Reichert-Jung, Nußloch) at a knive temperature of −22°C. Sectioned tissue was washed three times for 5 min at RT with LacZ wash solution (100 mM NaH2PO4, 1.25 mM MgCl2, 2 mM EGTA, 0.1% w/v Deoxycholate, 0.2% w/v Nonidet P40, pH 7.4). Sections were incubated overnight at 37°C in LacZ substrate solution (100 mM NaH2PO4, 1.25 mM MgCl2, 2 mM EGTA, 50 mM K3[Fe(CN)6], 50 mM K4[Fe(CN)6], 8% w/v X-Gal, pH 7.4) and washed twice with H2O before being mounted on coverslips using Aqua-Poly/Mount (Polyscience).
Measurement of Changes in Intracellular Ca2+ and pH in Mouse Sperm
Changes in [Ca2+]i and pHi in mouse sperm were recorded in a rapid-mixing device in the stopped-flow mode (SFM400; Bio-Logic, Claix, France) after loading with the fluorescent Ca2+ indicator Cal-520-AM (AAT Bioquest, Sunnyvale, United States) or the fluorescent pH indicator BCECF-AM (Thermo Fisher), respectively. Changes in [Ca2+]i were measured as previously described (Strünker et al., 2011) with minor modifications. In brief, sperm were loaded with Cal-520-AM (5 μM) in the presence of Pluronic F-127 (0.02% v/v) at 37°C for 45 min. After incubation, excess dye was removed by three centrifugation steps (700 g, 7 min, RT). The pellet was resuspended in buffer and equilibrated for 5 min at 37°C. The sperm suspension (5 × 106 sperm/ml) was rapidly mixed 1:1 (v/v, 100:100 μl) with the respective stimulant [ZP, K8.6, 2 μM ionomycin (Tocris)] at a flow rate of 0.5 ml/s. Fluorescence was excited by a SpectraX Light Engine (Lumencor, Beaverton, United States), whose intensity was modulated with a frequency of 10 kHz. The excitation light was passed through a BrightLine 475/28 nm filter (Semrock, Rochester, United States) onto the cuvette. Emission light was passed through a BrightLine 536/40 filter (Semrock) and recorded by photomultiplier modules (H10723-20; Hamamatsu Photonics). The signal was amplified and filtered through a lock-in amplifier (7230 DualPhase; Ametek, Paoli, United States). Data acquisition was performed with a data acquisition pad (PCI-6221; National Instruments, Austin, United States) and Bio-Kine software v. 4.49 (Bio-Logic). Ca2+ signal traces are depicted as the percent change in fluorescence (ΔF) with respect to the mean of the first three data points recorded immediately after mixing (F0). Mean ± 95% CI are shown to visualize the true range of the data. The control ΔF/F0 signal observed upon mixing with buffer (control) was subtracted from K8 6-, ZP-, or ionomycin-induced signals, setting the control-signal level to ΔF/F0 (%) = 0. The K8.6 solution (Babcock and Pfeiffer, 1987) contained (in mM: 4.8 NaCl, 138 KCl, 2 CaCl2, 1.2 KH2PO4, 1 MgSO4, 5.6 glucose, 0.5 sodium pyruvate, 10 lactic acid, 10 HEPES, pH 8.6, adjusted with KOH) to depolarize the Vm and simultaneously increase pHi to activate CatSper. Bar graphs show the maximal amplitude of the ZP- or K8.6-evoked Ca2+ response (average of last three data points), normalized to the respective ionomycin-evoked Ca2+ response (average of last three data points) (relative signal amplitude). To measure pH changes in the stopped-flow mode, sperm were loaded with BCECF-AM (10 μM) at 37°C for 15 min. The pellet was resuspended in TYH and equilibrated for 5 min at 37°C. The excitation light was passed through a BrightLine 452/45 nm filter (Semrock) onto the cuvette. Emission light was passed in parallel through a BrightLine 494/20 filter and a BrightLine 540/10 filter (Semrock). pH signals are depicted as the percent change in fluorescence ratio (ΔR) of 494 nm/540 nm with respect to the mean of the first three data points recorded immediately after mixing (R0) when a stable fluorescence signal was observed. The ΔR/R0 signal evoked by mixing with buffer (control) was subtracted from ZP- or NH4Cl-induced signals. Bar graphs show the maximal amplitude of the ZP- or NH4Cl-evoked pH response (average of last three data points).
In vitro Fertilization
Superovulation in females was induced as described above. HTF medium (EmbryoMax Human Tubal Fluid; Merck Millipore) was mixed 1:1 with mineral oil (Sigma-Aldrich) and equilibrated overnight at 37°C. Sperm were capacitated for 90 min in TYH medium supplemented as indicated above. On the day of preparation, 100 μl drops of HTF were covered with the medium/oil mixture and 105 sperm were added to each drop. Cumulus-enclosed oocytes were prepared from the oviducts of superovulated females and added to the drops. After 4 h at 37°C and 5% CO2, oocytes were transferred to fresh HTF. The number of 2-cell stages was evaluated after 24 h.
Sperm Motility Analysis
Freely beating sperm were observed in shallow perfusion chambers with 200 μm depth, which allowed to exchange the buffer during recordings. Sperm were tethered to the glass surface by lowering the BSA to 0.3 mg/ml. An inverted dark-field video microscope (IX71; Olympus) with a 10 x objective (UPlanFL, NA 0.4; Olympus) and an additional 1.6 x magnification lens (16x final amplification) was combined with a high-speed camera (Dimax; PCO). Dark-field videos were recorded with a frame rate of 200 Hz. The temperature was 37°C (Incubator; Life Imaging Services). The flagellar beat was analyzed using SpermQ (Hansen et al., 2018). SpermQ outputs the parameter curvature angle as a measure for flagellar bending. The curvature angle at a given point on the flagellum was determined by the angle between the tangential vector at the given point and the tangential vector at the point 10 μm proximal on the flagellum. The beat frequency at a given point on the flagellum was determined by the highest peak in the frequency spectrum obtained by Fast-Fourier-Transformation of the time-course of the parameter curvature angle at the given flagellar point. The amplitude of the curvature angle for a given point was determined as the absolute difference between the median of the five highest and the median of the five lowest curvature angle values in the entire time-course at the given point.
Measuring cAMP Dynamics in Sperm
Population measurements using a spectrofluorometer (Quantamaster 40, PTI) were performed as previously described (Mukherjee et al., 2016) in PMMA cuvettes at 1 × 105 cells/ml under constant stirring (Spinbar, Bel-art Products, Wayne, NJ, United States).
Identification of NHA1 by Mass Spectrometry
Sperm were isolated from wild-type C57Bl/6 mice and subjected to mass spectrometry as described previously (Raju et al., 2015).
Statistical Analysis
Statistical analyses for graphs shown in figures has been performed in GraphPad Prism. Statistical significance between two groups was determined using two-tailed, unpaired t-test with Welch’s correction, statistical significance between multiple groups was determined using one-way ANOVA with Dunnett’s correction. The respective details are indicated in the figure legends.
Data Availability Statement
The raw data supporting the conclusions of this article will be made available by the authors, without undue reservation.
Ethics Statement
The animal study was reviewed and approved by Landesamt für Natur, Umwelt und Verbraucherschutz Nordrhein-Westfalen (State Office for Nature, Environment and Consumer Protection North Rhine-Westphalia) [AZ 84-02.04.2012.A192 and AZ 84-02.05.40.13.127].
Author Contributions
MB established and performed the pHi and Ca2+ fluorimetry, prepared the native ZPs, and generated and analyzed the NHA1-KO. CS also performed pHi and Ca2+ fluorimetry. HH performed STORM imaging. JJ and JH analyzed sperm motility. CT performed the mass spectrometry. PN performed genotyping of knockout mice. HF provided protocols for ZP isolation and analysis. HF, UK, CB, LJ, and LH analyzed and/or interpreted data, participated in drafting the manuscript, and revised the manuscript critically for important intellectual content. TS and DW conceived the project, designed and coordinated the experiments, analyzed and/or interpreted data, and wrote the manuscript. All authors contributed to the article and approved the submitted version.
Funding
The work was funded by the Deutsche Forschungsgemeinschaft (DFG, German Research Foundation) under Germany’s Excellence Strategy – EXC2151 – 390873048 (DW), the Cells-in-Motion (CiM) Cluster of Excellence, Münster (FF-2016-17; TS), SPP1926 (DW), TRR83 (DW), SPP1726 (DW and UK), CRU326 (TS and CB), and the NIH (HD046948, HF). JH was a Ph.D. fellow of the Boehringer Ingelheim Foundation.
Conflict of Interest
The authors declare that the research was conducted in the absence of any commercial or financial relationships that could be construed as a potential conflict of interest.
Acknowledgments
We thank Prof. Christopher J. Lingle (Washington University, St. Louis, United States) for scientific discussions regarding the project, his help concerning the drafting of the manuscript, and for kindly providing the Kcnu1/Lrrc52-KO mice, Prof. David Clapham (Janelia Research Campus, United States) for kindly providing the Catsper1-KO mice, Jens-Henning Krause, Jessica Görgen, Isabel Lux, Dana Herborn, Mona Völker for technical assistance, the Transgenic Service at the LIMES institute (University of Bonn) for their help with the generation of the Slc9b1-KO mice, and the Monoclonal Antibody Core Facility (Helmholtz Zentrum München) for maintaining the hybridoma cell lines.
Supplementary Material
The Supplementary Material for this article can be found online at: https://www.frontiersin.org/articles/10.3389/fcell.2020.572735/full#supplementary-material
FIGURE S1 | Validation of the specificity of anti-ZP antibodies using heterologously expressed protein. Immunostaining of HEK293T cells heterologously expressing mouse ZP glycoproteins with mZP isoform-specific (green) and His-tag antibodies (red), co-localization shown in yellow, (a) mZP1, (b) mZP2, (c) mZP3; scale bar = 10 μm.
FIGURE S2 | Ca2+ responses in non-capacitated and capacitated mouse sperm. (a) Ca2+ responses evoked by mixing with 2 μM ionomycin in non-capacitated and capacitated sperm; average ± 95% confidence interval (n ≥ 18) (b) Ca2+ responses evoked by mixing with 1 ZP/μl, K8.6 or 2 μM ionomycin in non-capacitated and capacitated sperm; individual data points and mean ± SD (n ≥ 18).
FIGURE S3 | Density distribution of CatSper domains along the flagella. (a) Normalized density profile of one CatSper domain along the arc length of wild-type (black) and Slc9b1-KO sperm flagella (red). (b) Fourier spectrum in dB of the data in (a), wild-type (black) and Slc9b1-KO sperm flagella (red).
TABLE S1 | NHA1 peptide sequences identified in total lysates from mouse sperm by mass spectrometry.
TABLE S2 | Fertility parameters of male wild-type and Slc9b1-KO mice.
MOVIE S1 | Flagellar motility of wild-type sperm before and after perfusion with 25 mM NaHCO3.
MOVIE S2 | Flagellar motility of Slc9b1-KO sperm before and after perfusion with 25 mM NaHCO3.
References
Arnoult, C., Cardullo, R. A., Lemos, J. R., and Florman, H. M. (1996a). Activation of mouse sperm T-type Ca2+ channels by adhesion to the egg zona pellucida. Proc. Natl. Acad. Sci. U.S.A. 93, 13004–13009. doi: 10.1073/pnas.93.23.13004
Arnoult, C., Zeng, Y., and Florman, H. M. (1996b). ZP3-dependent activation of sperm cation channels regulates acrosomal secretion during mammalian fertilization. J. Cell Biol. 134, 637–645. doi: 10.1083/jcb.134.3.637
Arnoult, C., Kazam, I. G., Visconti, P. E., Kopf, G. S., Villaz, M., and Florman, H. M. (1999). Control of the low voltage-activated calcium channel of mouse sperm by egg ZP3 and by membrane hyperpolarization during capacitation. Proc. Natl. Acad. Sci. U.S.A. 96, 6757–6762. doi: 10.1073/pnas.96.12.6757
Austin, C. R. (1952). The capacitation of the mammalian sperm. Nature 170:326. doi: 10.1038/170326a0
Avella, M. A., Baibakov, B., and Dean, J. (2014). A single domain of the ZP2 zona pellucida protein mediates gamete recognition in mice and humans. J. Cell Biol. 205, 801–809. doi: 10.1083/jcb.201404025
Avella, M. A., Xiong, B., and Dean, J. (2013). The molecular basis of gamete recognition in mice and humans. Mol. Hum. Reprod. 19, 279–289. doi: 10.1093/molehr/gat004
Babcock, D. F., and Pfeiffer, D. R. (1987). Independent elevation of cytosolic [Ca2+] and pH of mammalian sperm by voltage-dependent and pH-sensitive mechanisms. J. Biol. Chem. 262, 15041–15047.
Baibakov, B., Gauthier, L., Talbot, P., Rankin, T. L., and Dean, J. (2007). Sperm binding to the zona pellucida is not sufficient to induce acrosome exocytosis. Development 134, 933–943. doi: 10.1242/dev.02752
Bailey, J. L., and Storey, B. T. (1994). Calcium influx into mouse spermatozoa activated by solubilized mouse zona pellucida, monitored with the calcium fluorescent indicator, fluo-3. Inhibition of the influx by three inhibitors of the zona pellucida induced acrosome reaction: tyrphostin A48, pertussis toxin, and 3-quinuclidinyl benzilate. Mol. Reprod. Dev. 39, 297–308. doi: 10.1002/mrd.1080390307
Baltz, J. M., Katz, D. F., and Cone, R. A. (1988). Mechanics of sperm-egg interaction at the zona pellucida. Biophys. J. 54, 643–654. doi: 10.1016/S0006-3495(88)83000-5
Bleil, J. D., and Wassarman, P. M. (1980). Mammalian sperm-egg interaction: identification of a glycoprotein in mouse egg zonae pellucidae possessing receptor activity for sperm. Cell 20, 873–882. doi: 10.1016/0092-8674(80)90334-7
Bleil, J. D., and Wassarman, P. M. (1983). Sperm-egg interactions in the mouse: sequence of events and induction of the acrosome reaction by a zona pellucida glycoprotein. Dev. Biol. 95, 317–324. doi: 10.1016/0012-1606(83)90032-5
Braden, A. W., Austin, C. R., and David, H. A. (1954). The reaction of zona pellucida to sperm penetration. Aust. J. Biol. Sci. 7, 391–409. doi: 10.1071/bi9540391
Brenker, C., Zhou, Y., Müller, A., Echeverry, F. A., Trötschel, C., Poetsch, A., et al. (2014). The Ca2+-activated K+ current of human sperm is mediated by Slo3. eLife 3:e01438. doi: 10.7554/eLife.01438
Carlson, A. E., Hille, B., and Babcock, D. F. (2007). External Ca2+ acts upstream of adenylyl cyclase SACY in the bicarbonate signaled activation of sperm motility. Dev. Biol. 312, 183–192. doi: 10.1016/j.ydbio.2007.09.017
Chang, M. C. (1951). Fertilizing capacity of spermatozoa deposited into the fallopian tubes. Nature 168, 697–698. doi: 10.1038/168697b0
Chavez, J. C., Ferreira, J. J., Butler, A., De La Vega Beltran, J. L., Trevino, C. L., Darszon, A., et al. (2014). SLO3 K+ channels control calcium entry through CATSPER channels in sperm. J. Biol. Chem. 289, 32266–32275. doi: 10.1074/jbc.M114.607556
Chen, S. R., Chen, M., Deng, S. L., Hao, X. X., Wang, X. X., and Liu, Y. X. (2016). Sodium-hydrogen exchanger NHA1 and NHA2 control sperm motility and male fertility. Cell Death Dis. 7:e2152. doi: 10.1038/cddis.2016.65
Cherr, G. N., Lambert, H., Meizel, S., and Katz, D. F. (1986). In vitro studies of the golden hamster sperm acrosome reaction: completion on the zona pellucida and induction by homologous soluble zonae pellucidae. Dev. Biol. 114, 119–131. doi: 10.1016/0012-1606(86)90388-x
Chung, J. J., Miki, K., Kim, D., Shim, S. H., Shi, H. F., Hwang, J. Y., et al. (2017). CatSper zeta regulates the structural continuity of sperm Ca2+ signaling domains and is required for normal fertility. eLife 6:e23082. doi: 10.7554/eLife.23082
Chung, J. J., Shim, S. H., Everley, R. A., Gygi, S. P., Zhuang, X., and Clapham, D. E. (2014). Structurally distinct Ca(2+) signaling domains of sperm flagella orchestrate tyrosine phosphorylation and motility. Cell 157, 808–822. doi: 10.1016/j.cell.2014.02.056
Cross, N. L., Morales, P., Overstreet, J. W., and Hanson, F. W. (1988). Induction of acrosome reactions by the human zona pellucida. Biol. Reprod. 38, 235–244.
De La Vega-Beltran, J. L., Sanchez-Cardenas, C., Krapf, D., Hernandez-Gonzalez, E. O., Wertheimer, E., Trevino, C. L., et al. (2012). Mouse sperm membrane potential hyperpolarization is necessary and sufficient to prepare sperm for the acrosome reaction. J. Biol. Chem. 287, 44384–44393. doi: 10.1074/jbc.M112.393488
Drobnis, E. Z., Yudin, A. I., Cherr, G. N., and Katz, D. F. (1988). Hamster sperm penetration of the zona pellucida: kinematic analysis and mechanical implications. Dev. Biol. 130, 311–323. doi: 10.1016/0012-1606(88)90437-x
Eisenbach, M., and Giojalas, L. C. (2006). Sperm guidance in mammals - an unpaved road to the egg. Nat. Rev. Mol. Cell Biol. 7, 276–285. doi: 10.1038/nrm1893
Esposito, G., Jaiswal, B. S., Xie, F., Krajnc-Franken, M. A. M., and Robben, T. (2004). Mice deficient for soluble adenylyl cyclase are infertile because of a severe sperm-motility defect. Proc. Natl. Acad. Sci. U.S.A. 101, 2993–2998. doi: 10.1073/pnas.0400050101
Fahrenkamp, E., Algarra, B., and Jovine, L. (2020). Mammalian egg coat modifications and the block to polyspermy. Mol. Reprod. Dev. 87, 326–340. doi: 10.1002/mrd.23320
Florman, H. M., and First, N. L. (1988). The regulation of acrosomal exocytosis. I. Sperm capacitation is required for the induction of acrosome reactions by the bovine zona pellucida in vitro. Dev. Biol. 128, 453–463. doi: 10.1016/0012-1606(88)90307-7
Florman, H. M., Jungnickel, M. K., and Sutton, K. A. (2008). Regulating the acrosome reaction. Int. J. Dev. Biol. 52, 503–510. doi: 10.1387/ijdb.082696hf
Florman, H. M., and Storey, B. T. (1982). Mouse gamete interactions: the zona pellucida is the site of the acrosome reaction leading to fertilization in vitro. Dev. Biol. 91, 121–130. doi: 10.1016/0012-1606(82)90015-x
Florman, H. M., Tombes, R. M., First, N. L., and Babcock, D. F. (1989). An adhesion-associated agonist from the zona pellucida activates G protein-promoted elevations of internal Ca2+ and pH that mediate mammalian sperm acrosomal exocytosis. Dev. Biol. 135, 133–146. doi: 10.1016/0012-1606(89)90164-4
Gupta, S. K. (2015). Role of zona pellucida glycoproteins during fertilization in humans. J. Reprod. Immunol. 108, 90–97. doi: 10.1016/j.jri.2014.08.006
Gupta, S. K., Bhandari, B., Shrestha, A., Biswal, B. K., Palaniappan, C., Malhotra, S. S., et al. (2012). Mammalian zona pellucida glycoproteins: structure and function during fertilization. Cell Tissue Res. 349, 665–678. doi: 10.1007/s00441-011-1319-y
Hansen, J. N., Rassmann, S., Jikeli, J. F., and Wachten, D. (2018). SpermQ(-)A simple analysis software to comprehensively study flagellar beating and sperm steering. Cells 8:10. doi: 10.3390/cells8010010
Hess, K. C., Jones, B. H., Marquez, B., Chen, Y., and Ord, T. S. (2005). The soluble adenylyl cyclase in sperm mediates multiple signaling events required for fertilization. Dev. Cell 9, 249–259. doi: 10.1016/j.devcel.2005.06.007
Hildebrand, M. S., Avenarius, M. R., Fellous, M., Zhang, Y., Meyer, N. C., Auer, J., et al. (2010). Genetic male infertility and mutation of CATSPER ion channels. Eur. J. Hum. Genet. 18, 1178–1184. doi: 10.1038/ejhg.2010.108
Hino, T., Muro, Y., Tamura-Nakano, M., Okabe, M., Tateno, H., and Yanagimachi, R. (2016). The behavior and acrosomal status of mouse spermatozoa in vitro, and within the oviduct during fertilization after natural mating. Biol. Reprod. 95:50. doi: 10.1095/biolreprod.116.140400
Hirohashi, N., and Yanagimachi, R. (2018). Sperm acrosome reaction: its site and role in fertilization. Biol. Reprod. 99, 127–133. doi: 10.1093/biolre/ioy045
Ho, H. C., and Suarez, S. S. (2001). Hyperactivation of mammalian spermatozoa: function and regulation. Reproduction 122, 519–526. doi: 10.1530/rep.0.1220519
Huang, B., Wang, W., Bates, M., and Zhuang, X. (2008). Three-dimensional super-resolution imaging by stochastic optical reconstruction microscopy. Science 319, 810–813. doi: 10.1126/science.1153529
Jansen, V., Alvarez, L., Balbach, M., Strünker, T., Hegemann, P., Kaupp, U. B., et al. (2015). Controlling fertilization and cAMP signaling in sperm by optogenetics. eLife 4:e05161. doi: 10.7554/eLife.05161
Jin, M., Fujiwara, E., Kakiuchi, Y., Okabe, M., Satouh, Y., Baba, S. A., et al. (2011). Most fertilizing mouse spermatozoa begin their acrosome reaction before contact with the zona pellucida during in vitro fertilization. Proc. Natl. Acad. Sci. U.S.A. 108, 4892–4896. doi: 10.1073/pnas.1018202108
Katz, D. F., and Yanagimachi, R. (1981). Movement characteristics of hamster and guinea pig spermatozoa upon attachment to the zona pellucida. Biol. Reprod. 25, 785–791. doi: 10.1095/biolreprod25.4.785
Kaupp, U. B., Kashikar, N. D., and Weyand, I. (2008). Mechanisms of sperm chemotaxis. Annu. Rev. Physiol. 70, 93–117. doi: 10.1146/annurev.physiol.70.113006.100654
Kirichok, Y., Navarro, B., and Clapham, D. E. (2006). Whole-cell patch-clamp measurements of spermatozoa reveal an alkaline-activated Ca2+ channel. Nature 439, 737–740. doi: 10.1038/nature04417
La Spina, F. A., Puga Molina, L. C., Romarowski, A., Vitale, A. M., Falzone, T. L., Krapf, D., et al. (2016). Mouse sperm begin to undergo acrosomal exocytosis in the upper isthmus of the oviduct. Dev. Biol. 411, 172–182. doi: 10.1016/j.ydbio.2016.02.006
Lishko, P. V., Botchkina, I. L., and Kirichok, Y. (2011). Progesterone activates the principal Ca2+ channel of human sperm. Nature 471, 387–391. doi: 10.1038/nature09767
Lishko, P. V., and Kirichok, Y. (2010). The role of Hv1 and CatSper channels in sperm activation. J. Physiol. 588(Pt 23), 4667–4672. doi: 10.1113/jphysiol.2010.194142
Liu, T., Huang, J. C., Zuo, W. L., Lu, C. L., Chen, M., Zhang, X. S., et al. (2010). A novel testis-specific Na+/H+ exchanger is involved in sperm motility and fertility. Front. Biosci. 2:566–581. doi: 10.2741/e115
Miyata, H., Satouh, Y., Mashiko, D., Muto, M., Nozawa, K., Shiba, K., et al. (2015). Sperm calcineurin inhibition prevents mouse fertility with implications for male contraceptive. Science 350, 442–445. doi: 10.1126/science.aad0836
Mukherjee, S., Jansen, V., Jikeli, J. F., Hamzeh, H., Alvarez, L., Dombrowski, M., et al. (2016). A novel biosensor to study cAMP dynamics in cilia and flagella. eLife 5:e14052. doi: 10.7554/eLife.14052
Murase, T., and Roldan, E. R. (1996). Progesterone and the zona pellucida activate different transducing pathways in the sequence of events leading to diacylglycerol generation during mouse sperm acrosomal exocytosis. Biochem. J. 320(Pt 3), 1017–1023. doi: 10.1042/bj3201017
Muro, Y., Hasuwa, H., Isotani, A., Miyata, H., Yamagata, K., Ikawa, M., et al. (2016). Behavior of mouse spermatozoa in the female reproductive tract from soon after mating to the beginning of fertilization. Biol. Reprod. 94:80. doi: 10.1095/biolreprod.115.135368
Navarro, B., Kirichok, Y., and Clapham, D. E. (2007). KSper, a pH-sensitive K+ current that controls sperm membrane potential. Proc. Natl. Acad. Sci. U.S.A. 104, 7688–7692. doi: 10.1073/pnas.0702018104
O’Rand, M. G., and Fisher, S. J. (1987). Localization of zona pellucida binding sites on rabbit spermatozoa and induction of the acrosome reaction by solubilized zonae. Dev. Biol. 119, 551–559. doi: 10.1016/0012-1606(87)90058-3
Publicover, S. J., Giojalas, L. C., Teves, M. E., de Oliveira, G. S., Garcia, A. A., Barratt, C. L., et al. (2008). Ca2+ signalling in the control of motility and guidance in mammalian sperm. Front. Biosci. 13:5623–5637. doi: 10.2741/3105
Quill, T. A., Ren, D., Clapham, D. E., and Garbers, D. L. (2001). A voltage-gated ion channel expressed specifically in spermatozoa. Proc. Natl. Acad. Sci. U.S.A. 98, 12527–12531. doi: 10.1073/pnas.221454998
Raju, D., Schonauer, S., Hamzeh, H., Flynn, K. C., Bradke, F., Vom Dorp, K., et al. (2015). Accumulation of glucosylceramide in the absence of the beta-glucosidase GBA2 alters cytoskeletal dynamics. PLoS Genet. 11:e1005063. doi: 10.1371/journal.pgen.1005063
Ren, D., Navarro, B., Perez, G., Jackson, A. C., Hsu, S., Shi, Q., et al. (2001). A sperm ion channel required for sperm motility and male fertility. Nature 413, 603–609. doi: 10.1038/35098027
Santi, C. M., Martinez-Lopez, P., de la Vega-Beltran, J. L., Butler, A., Alisio, A., Darszon, A., et al. (2010). The SLO3 sperm-specific potassium channel plays a vital role in male fertility. FEBS Lett. 584, 1041–1046. doi: 10.1016/j.febslet.2010.02.005
Schroer, S. C., Yudin, A. I., Myles, D. G., and Overstreet, J. W. (2000). Acrosomal status and motility of guinea pig spermatozoa during in vitro penetration of the cumulus oophorus. Zygote 8, 107–117. doi: 10.1017/s0967199400000885
Seifert, R., Flick, M., Bönigk, W., Alvarez, L., Trötschel, C., Poetsch, A., et al. (2015). The CatSper channel controls chemosensation in sea urchin sperm. EMBO J. 34, 379–392. doi: 10.15252/embj.201489376
Shi, H., Zhao, X., Ding, Z., Han, C., Jiang, Y., Qian, W., et al. (2017). Na+/H+ exchanger regulates amino acid-mediated autophagy in intestinal epithelial cells. Cell Physiol. Biochem. 42, 2418–2429. doi: 10.1159/000480184
Strünker, T., Goodwin, N., Brenker, C., Kashikar, N. D., Weyand, I., Seifert, R., et al. (2011). The CatSper channel mediates progesterone-induced Ca2+ influx in human sperm. Nature 471, 382–386. doi: 10.1038/nature09769
Sumigama, S., Mansell, S., Miller, M., Lishko, P. V., Cherr, G. N., Meyers, S. A., et al. (2015). Progesterone accelerates the completion of sperm capacitation and activates catsper channel in spermatozoa from the rhesus macaque. Biol. Reprod. 93:130. doi: 10.1095/biolreprod.115.129783
Thaler, C. D., and Cardullo, R. A. (1996). The initial molecular interaction between mouse sperm and the zona pellucida is a complex binding event. J. Biol. Chem. 271, 23289–23297. doi: 10.1074/jbc.271.38.23289
Tollner, T. L., Yudin, A. I., Cherr, G. N., and Overstreet, J. W. (2003). Real-time observations of individual macaque sperm undergoing tight binding and the acrosome reaction on the zona pellucida. Biol. Reprod. 68, 664–672. doi: 10.1095/biolreprod.102.009175
Uto, N., Yoshimatsu, N., Lopata, A., and Yanagimachi, R. (1988). Zona-induced acrosome reaction of hamster spermatozoa. J. Exp. Zool. 248, 113–120. doi: 10.1002/jez.1402480115
Wang, D., Hu, J., Bobulescu, I. A., Quill, T. A., McLeroy, P., Moe, O. W., et al. (2007). A sperm-specific Na+/H+ exchanger (sNHE) is critical for expression and in vivo bicarbonate regulation of the soluble adenylyl cyclase (sAC). Proc. Natl. Acad. Sci. U.S.A. 104, 9325–9330. doi: 10.1073/pnas.0611296104
Wang, D., King, S. M., Quill, T. A., Doolittle, L. K., and Garbers, D. L. (2003). A new sperm-specific Na+/H+ exchanger required for sperm motility and fertility. Nat. Cell Biol. 5, 1117–1122. doi: 10.1038/ncb1072
Wassarman, P. M. (1988). Zona pellucida glycoproteins. Annu. Rev. Biochem. 57, 415–442. doi: 10.1146/annurev.bi.57.070188.002215
Wennemuth, G., Carlson, A. E., Harper, A. J., and Babcock, D. F. (2003). Bicarbonate actions on flagellar and Ca2+-channel responses: initial events in sperm activation. Development 130, 1317–1326. doi: 10.1242/dev.00353
Xia, J., and Ren, D. (2009). Egg coat proteins activate calcium entry into mouse sperm via CATSPER channels. Biol. Reprod. 80, 1092–1098. doi: 10.1095/biolreprod.108.074039
Xie, F., Garcia, M. A., Carlson, A. E., Schuh, S. M., and Babcock, D. F. (2006). Soluble adenylyl cyclase (sAC) is indispensable for sperm function and fertilization. Dev. Biol. 296, 353–362. doi: 10.1016/j.ydbio.2006.05.038
Yanagimachi, R. (1994). Fertility of mammalian spermatozoa: its development and relativity. Zygote 2, 371–372. doi: 10.1017/s0967199400002240
Yang, C., Zeng, X. H., Zhou, Y., Xia, X. M., and Lingle, C. J. (2011). LRRC52 (leucine-rich-repeat-containing protein 52), a testis-specific auxiliary subunit of the alkalization-activated Slo3 channel. Proc. Natl. Acad. Sci. U.S.A. 108, 19419–19424. doi: 10.1073/pnas.1111104108
Zeng, X. H., Navarro, B., Xia, X. M., Clapham, D. E., and Lingle, C. J. (2013). Simultaneous knockout of Slo3 and CatSper1 abolishes all alkalization- and voltage-activated current in mouse spermatozoa. J. Gen. Physiol. 142, 305–313. doi: 10.1085/jgp.201311011
Zeng, X. H., Yang, C., Kim, S. T., Lingle, C. J., and Xia, X. M. (2011). Deletion of the Slo3 gene abolishes alkalization-activated K+ current in mouse spermatozoa. Proc. Natl. Acad. Sci. U.S.A. 108, 5879–5884. doi: 10.1073/pnas.1100240108
Zeng, X. H., Yang, C., Xia, X. M., Liu, M., and Lingle, C. J. (2015). SLO3 auxiliary subunit LRRC52 controls gating of sperm KSPER currents and is critical for normal fertility. Proc. Natl. Acad. Sci. U.S.A. 112, 2599–2604. doi: 10.1073/pnas.1423869112
Keywords: zona pellucida, mouse sperm, calcium, sperm motility, sodium-proton exchange
Citation: Balbach M, Hamzeh H, Jikeli JF, Brenker C, Schiffer C, Hansen JN, Neugebauer P, Trötschel C, Jovine L, Han L, Florman HM, Kaupp UB, Strünker T and Wachten D (2020) Molecular Mechanism Underlying the Action of Zona-pellucida Glycoproteins on Mouse Sperm. Front. Cell Dev. Biol. 8:572735. doi: 10.3389/fcell.2020.572735
Received: 15 June 2020; Accepted: 12 August 2020;
Published: 31 August 2020.
Edited by:
Takanari Inoue, Johns Hopkins Medicine, United StatesReviewed by:
Sashko Damjanovski, University of Western Ontario, CanadaMariano G. Buffone, CONICET Instituto de Biología y Medicina Experimental (IBYME), Argentina
Aldebaran Hofer, Harvard Medical School, United States
Copyright © 2020 Balbach, Hamzeh, Jikeli, Brenker, Schiffer, Hansen, Neugebauer, Trötschel, Jovine, Han, Florman, Kaupp, Strünker and Wachten. This is an open-access article distributed under the terms of the Creative Commons Attribution License (CC BY). The use, distribution or reproduction in other forums is permitted, provided the original author(s) and the copyright owner(s) are credited and that the original publication in this journal is cited, in accordance with accepted academic practice. No use, distribution or reproduction is permitted which does not comply with these terms.
*Correspondence: Timo Strünker, dGltby5zdHJ1ZW5rZXJAdWttdWVuc3Rlci5kZQ==; Dagmar Wachten, ZHdhY2h0ZW5AdW5pLWJvbm4uZGU=
†Present address: Melanie Balbach, Department of Pharmacology, Weill Cornell Medical College, New York, NY, United States