- 1Centre for Organelle Research, University of Stavanger, Stavanger, Norway
- 2Plant Biochemistry and Infection Biology, Institute for Plant Science and Microbiology, Universität Hamburg, Hamburg, Germany
The stramenopile alga Nannochloropsis evolved by secondary endosymbiosis of a red alga by a heterotrophic host cell and emerged as a promising organism for biotechnological applications, such as the production of polyunsaturated fatty acids and biodiesel. Peroxisomes play major roles in fatty acid metabolism but experimental analyses of peroxisome biogenesis and metabolism in Nannochloropsis are not reported yet. In fungi, animals, and land plants, soluble proteins of peroxisomes are targeted to the matrix by one of two peroxisome targeting signals (type 1, PTS1, or type 2, PTS2), which are generally conserved across kingdoms and allow the prediction of peroxisomal matrix proteins from nuclear genome sequences. Because diatoms lost the PTS2 pathway secondarily, we investigated its presence in the stramenopile sister group of diatoms, the Eustigmatophyceae, represented by Nannochloropsis. We detected a full-length gene of a putative PEX7 ortholog coding for the cytosolic receptor of PTS2 proteins and demonstrated its expression in Nannochloropsis gaditana. The search for predicted PTS2 cargo proteins in N. gaditana yielded several candidates. In vivo subcellular targeting analyses of representative fusion proteins in different plant expression systems demonstrated that two predicted PTS2 domains were indeed functional and sufficient to direct a reporter protein to peroxisomes. Peroxisome targeting of the predicted PTS2 cargo proteins was further confirmed in Nannochloropsis oceanica by confocal and transmission electron microscopy. Taken together, the results demonstrate for the first time that one group of stramenopile algae maintained the import pathway for PTS2 cargo proteins. To comprehensively map and model the metabolic capabilities of Nannochloropsis peroxisomes, in silico predictions needs to encompass both the PTS1 and the PTS2 matrix proteome.
Introduction
Peroxisomes are small organelles of 0.1–1.7 μm in diameter, surrounded by a single membrane and able to detoxify reactive oxygen species generated by diverse peroxisomal enzymes. Common peroxisomal functions of animals and plants are the glyoxylate cycle, amino acid metabolism, and polyamine oxidation. Plant peroxisomes are furthermore capable of contributing to photorespiration and to several biosynthetic functions, such as jasmonate, phylloquinone, and biotin biosynthesis (Reumann and Bartel, 2016; Pan et al., 2020). Importantly, peroxisomes play vital roles in lipid metabolism in many organisms. While in animals fatty acid β-oxidation occurs in both mitochondria and peroxisomes (Wanders and Waterham, 2006), in plants the process takes place exclusively in peroxisomes (Graham and Eastmond, 2002). Hence, peroxisomal metabolism determines the steady state levels of total cellular fatty acids. In mammals, peroxisomes are additionally involved in the biosynthesis of docosahexaenoic acid (C22:6n-3) and eicosapentaenoic acid (EPA; C20:5n-3) by partial degradation of polyunsaturated fatty acids (PUFAs) with longer chains, such as C24:6n-3 (Sprecher, 2000). Knowledge of the metabolic capabilities of peroxisomes from plants, yeast, and fungi in lipid metabolism is prerequisite for successful biotechnological applications and genetic engineering to increase PUFA and biodiesel productivity.
All peroxisomal matrix proteins are encoded in the nuclear genome, synthesized on cytosolic ribosomes and are transported into peroxisomes with the help of peroxins (PEX proteins). Matrix proteins are targeted to peroxisomes by two major import pathways, depending on the possession of two distinct signals, called the peroxisomal targeting signal type 1 (PTS1) or type 2 (PTS2) (Gould et al., 1989; Swinkels et al., 1992). The PTS1 pathway predominates in all organisms studied to date and relies on the presence of a conserved C-terminal tripeptide. The twelve PTS1 tripeptides included in the plant consensus sequence [SA]-[KR]-[LMI]> have the highest peroxisomal targeting probability, but many additional non-canonical tripeptides have been characterized particularly in plants (Lingner et al., 2011). Additional residues upstream of the C-terminal tripeptide often contribute to peroxisome targeting (Brocard and Hartig, 2006; Lingner et al., 2011). Generally, the PTS2 nonapeptide is located within the first 40 amino acid (aa) residues and is cleaved upon transport into peroxisomes by the DEG15 protease (Helm et al., 2007; Schuhmann et al., 2008). Four amino acid residues are most conserved in canonical PTS2 nonapeptides included in the motif [RK]-[LVI]-x5-[HQ]-[LAF], where x5 denotes four variable amino acid residues and one hydrophobic residue in the middle (Osumi et al., 1992; Swinkels et al., 1992; Petriv et al., 2004; Kunze et al., 2011; Kunze, 2020). Two cytosolic receptors, PEX5 and PEX7, recognize the PTS1 and PTS2 of soluble proteins, respectively, and transport them to the peroxisomal membrane (Kragler et al., 1998; Gatto et al., 2000). PEX5 possesses at least two functional domains, namely the C-terminal half with seven tetratricopeptide (34-amino acid) repeats, which form the PTS1 binding pocket, and the N-terminal half bearing several diaromatic WxxxF/Y motifs that bind PEX14. PEX5 homologs of basidiomycetes, animals and plants additionally contain a PEX7 binding domain of approximately 37 amino acid residues in the N-terminal domain and also function as PEX7 co-receptors (Dodt et al., 2001; Woodward and Bartel, 2005). The docking complex proteins, PEX13 and PEX14, are responsible for initial PEX5-PEX7-cargo binding to the peroxisomal membrane (Bartel et al., 2014).
Peroxisomes are present in nearly all eukaryotes (Tolbert and Essner, 1981; Gabaldón, 2010). Particularly in eukaryotic microorganisms, such as algae and protists, basic research data are scarce regarding the biogenesis mechanisms and physiological functions of peroxisomes. In Closterium ehrenbergii, a charophyte alga of the family of Zygnematophyceae, peroxisomes have been visualized by H2O2/diaminobenzidine staining (Shinozaki et al., 2009; Hayashi and Shinozaki, 2012). In the chlorophyte alga Chlamydomonas, all enzymes associated with the glyoxylate cycle except for isocitrate lyase were located in punctate structures indicative of peroxisomes (Lauersen et al., 2016). In chromalveolates (organisms containing complex plastids of red algal ancestry), peroxisomes have mainly been studied in diatoms and alveolates (Gonzalez et al., 2011; Moog et al., 2017; Ludewig-Klingner et al., 2018). Alveolates, which include apicomplexa, dinoflagellates and ciliates, were long thought to lack peroxisomes, but coccidian apicomplexa, such as Toxoplasma, have recently been shown to possess peroxisomes, while other groups (e.g., Plasmodium) indeed lack this organelle (Moog et al., 2017). In the cryptophyte Guillardia theta, which possesses two phylogenetically different nuclei of host and endosymbiotic origin, a complete set of peroxins including PEX7 was identified and some of them were heterologously localized in peroxisomes as green fluorescent protein (GFP) fusions in Phaeodactylum tricornutum (Mix et al., 2018). In the same study, genome analyses indicated the presence of peroxins and, hence, peroxisomes in three stramenopile algae (Nannochloropsis gaditana, Aureococcus anophagefferens, and the brown alga, Ectocarpus siliculosus). In contrast, two haptophytes (Emiliania huxleyi and Chrysochromulina tobin) appeared to lack essential peroxins (Mix et al., 2018). Experimental analyses of peroxisomes in Nannochloropsis by microscopy or biochemistry have not been reported to date.
While the PTS1 targeting pathway is ubiquitous in all organisms that possess peroxisomes, the PTS2 targeting pathway is absent in specific organisms, such as Caenorhabditis elegans (Motley et al., 2000), Drosophila melanogaster (Faust et al., 2012; Baron et al., 2016) and few microalgae. As revealed by genome sequencing of the red alga Cyanidioschyzon merolae, the PEX7 receptor and PTS2 containing cargo proteins are absent (Matsuzaki et al., 2004; Shinozaki et al., 2009). The most comprehensive studies have been reported for the stramenopile diatom, P. tricornutum, which accordingly lacks PEX7 and predicted PTS2 proteins and is not capable of importing foreign PTS2 cargo proteins into the peroxisomal matrix (Gonzalez et al., 2011).
Stramenopiles (or heterokonts) form a large and diverse subgroup of chromalveolates that possess two morphologically different flagella and share the same evolutionary history of secondary endosymbiosis between a eukaryotic red alga and a heterotrophic eukaryotic host cell. Stramenopiles include both photosynthetic members (the ochrophytes) with complex plastids as well as aplastidic, non-photosynthetic members (e.g., oomycetes, Supplementary Figure 1). As a relic of evolution and phagocytosis, the plastids of photosynthetic stramenopiles are often still surrounded by four membranes (McFadden, 2001; Barsanti and Gualtieri, 2014). The photosynthetic group of stramenopiles includes many ecologically important lineages (diatoms, brown algae, pelagophytes) as well as the Eustigmatophyceae (e.g., Nannochloropsis) and forms the most significant component of eukaryotic marine phytoplankton (Guiry, 2012; Qiu et al., 2013; Dorrell et al., 2017).
Nannochloropsis is the best known representative of the Eustigmatophyceae and recently attracted increasing attention due to its high lipid accumulation of up to 60% of biomass dry weight (Rodolfi et al., 2009) and its outstanding EPA productivity [up to 4.3% (w/w) of biomass dry weight, Camacho-Rodriguez et al., 2014]. Therefore, Nannochloropsis species are considered promising candidates for the production of algal biomass and lipids for biofuels and high-value products (Ma et al., 2016). The genome sequences of the first Nannochloropsis strains have been published, including N. gaditana CCMP526 (Radakovits et al., 2012), N. gaditana B-31 (Corteggiani Carpinelli et al., 2014), and N. oceanica CCMP1779 and IMET-1 (Vieler et al., 2012; Wang et al., 2014). In silico analyses indicated the presence of genes encoding a putative PEX5 ortholog and putative PTS1 proteins. Due to the close phylogenetic relationship between red algae, diatoms and Eustigmatophyceae, the absence of the PTS2 pathway in Nannochloropsis was considered likely (Lauersen et al., 2016). In fact, not any PTS2 containing protein has been identified computationally or experimentally in this genus to date despite the availability of sequencing data for several Nannochloropsis species.
In the present study, we verified the expression of a putative PEX7 gene from N. gaditana. By comprehensive computational analyses, we predicted putative PTS2 cargo proteins in Nannochloropsis and identified more than a dozen of candidate proteins. We demonstrated that several PTS2-carrying cargo proteins of N. gaditana were indeed imported into peroxisomes in two plant expression systems and also in Nannochloropsis itself. Taken together, the results demonstrate for the first time experimentally that this stramenopile alga has maintained the PTS2 protein import pathway, even though the same trafficking route has been lost secondarily in the stramenopile sister group of diatoms.
Materials and Methods
Computational Analyses
For the identification of putative orthologs of Arabidopsis PEX and PTS2 proteins, the Arabidopsis proteins were used as queries in homology searches (BLASTp at NCBI, BLOSUM62 matrix, and standard parameters, McGinnis and Madden, 2004; Boratyn et al., 2013) against the non-redundant database of proteins of N. gaditana strains B-31 and CCMP526. Proteins with significant similarity (E-value <0.001, >30% identity, >50% of query length) were analyzed for the presence of a predicted PTS1 (PredPlantPTS1, Reumann et al., 2012) and a PTS2 included in the motif [RK]-[LVI]-x5-[HQ]-[LAF] (manual analyses, Kunze et al., 2011).
To identify yet unknown PTS2 proteins, the predicted protein sequences of N. gaditana B-31 (version 1.0, accessed on October 2, 2014) and CCMP526 (version 1.1, accessed on July 14, 2014) were downloaded. The sequences were analyzed for canonical PTS2 nonapeptides (see above) by applying the search algorithm “advanced find” of Microsoft Word. In N. gaditana B-31 more than 50 proteins were found, 13 of which contained the PTS2 motif in the N-terminal 40-amino acid domain (Table 1). For analyses of PTS2 conservation, homologous sequences were aligned by COBALT (Papadopoulos and Agarwala, 2007) and sequence conservation labeled by Boxshade.
Phylogenetic analysis of putative peroxisomal proteins was conducted on the platform Phylogeny.fr (Dereeper et al., 2008). Multiple sequence alignments were created by the MUSCLE algorithm (v3.8.31, Edgar, 2004). Phylogenetic trees were reconstructed using the Bayesian inference method, which is based on the Poisson model implemented in the MrBayes program (v3.2.6, Ronquist et al., 2012). The rate variation across sites was fixed to “invgamma”, and the number of substitution types was set to 6. Four Markov Chain Monte Carlo chains were run for 10,000 generations (sampling every 10 generations) with the first 250 sampled trees discarded as “burn-in”. Last, a “50% majority rule consensus tree” was constructed. The percentage of posterior probabilities of replicate trees, in which the associated taxa clustered together in the support test, is shown next to the branches (see Figure 1 and Supplementary Figures 5, 9). Graphical representation and formatting of the phylogenetic trees were performed with MEGA X (Kumar et al., 2018).
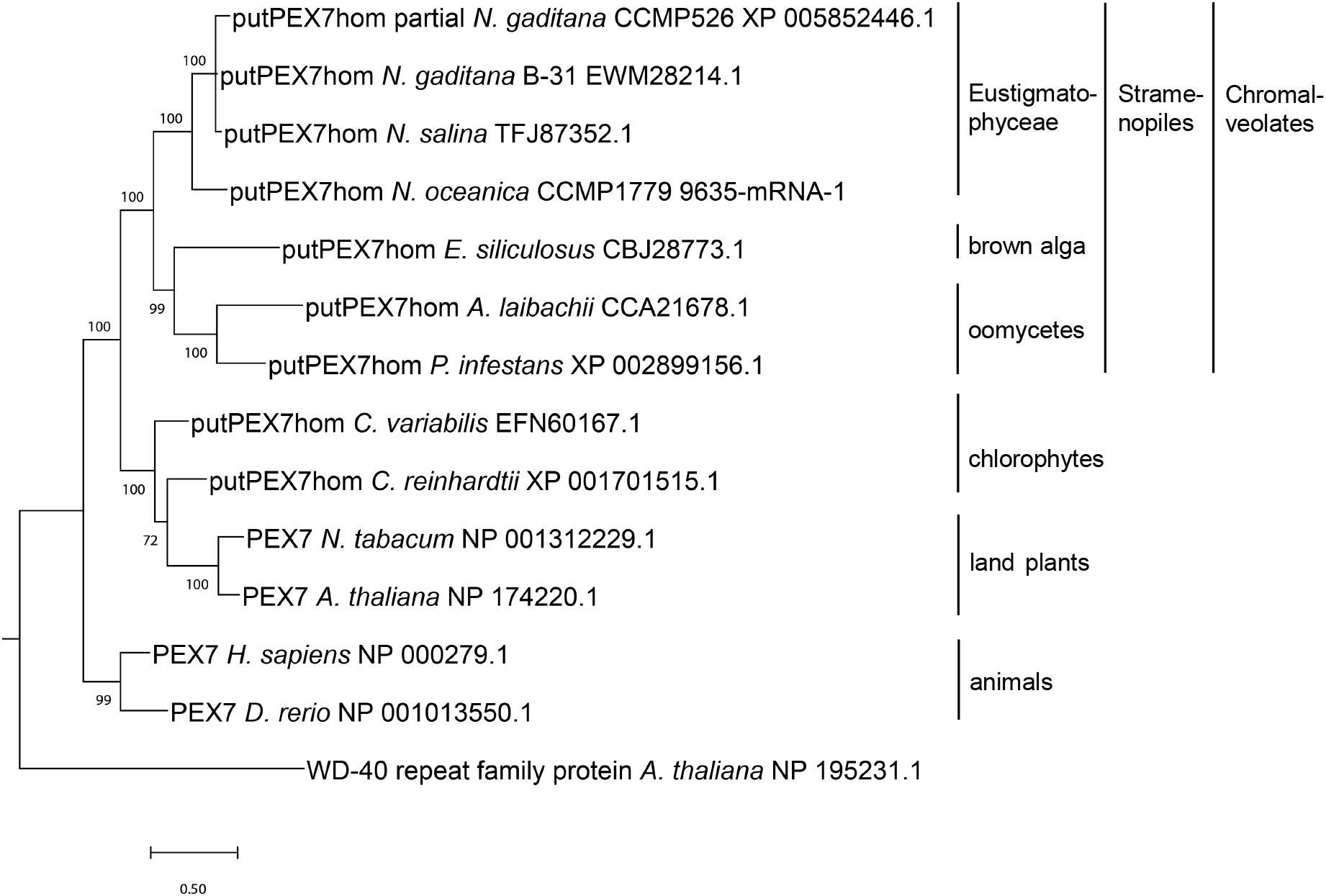
Figure 1. Phylogenetic analysis of PEX7 homologs detected in stramenopiles and related organisms. Sequences were aligned by MUSCLE (Edgar, 2004) and the phylogenetic tree was constructed using the Bayesian inference method (Ronquist et al., 2012). The branch support values were calculated as Bayesian posterior probabilities and are shown next to the branches. The platform Phylogeny.fr (Dereeper et al., 2008) was used for phylogenetic analysis, and the tree was visualized by MEGA X (Kumar et al., 2018).
Molecular Cloning
Genomic DNA was isolated from N. gaditana strain CCMP526 as described (Vieler et al., 2012) with the DNAeasy® Mini Kit (Qiagen, Germany). The N-terminal domains of NgMLS2 (B-31, EWM30341.1, 44 aa including RIx5HL), PKT (EWM24705.1, 24 aa including RLx5HL), and histidine triad family protein 1 (HIT1, EWM29206.1, 68 aa including RLx5HL) were amplified with primers including specific restriction sites (Supplementary Table 3) and inserted directly into the pCAT vector upstream of enhanced yellow fluorescent protein (EYFP) (Ma et al., 2006). Amplified DNA was sequenced to verify the identity. Plasmid DNA for in vivo subcellular targeting analyses was extracted with the GeneJET Plasmid Miniprep Kit (Thermo Fisher ScientificTM, United States).
For subcellular localization studies in Nannochloropsis, the above-mentioned N-terminal domains of NgMLS2 and NgHIT1 and the full-length CDS of AtpMDH1 (At2g22780) were re-amplified from available pCAT vectors and ABRC clones, respectively (Supplementary Table 3), and inserted upstream of the Venus fluorophore in the N. oceanica transformation vector pNoc ox Venus (Zienkiewicz et al., 2017). To create a peroxisomal marker for N. oceanica, the original pNoc ox Venus vector was modified by exchanging the hygromycin resistance gene and the nopaline synthase terminator against the blasticidin resistance gene and a cauliflower mosaic virus (CaMV) 35S terminator. The latter elements were reamplified from an episomal CRISPR/Cas9 vector (Addgene accession number: #101009, Poliner et al., 2018; Supplementary Table 3) and subcloned by ApaI and NotI. The Venus reporter gene was replaced by the blue fluorescent reporter gene mCerulean extended by a C-terminal PTS1 (Falter et al., 2019) using the given primer pairs (Supplementary Table 3) and the restriction enzymes SgsI and SacI.
For PEX7 expression analyses, RNA was isolated from N. gaditana CCMP526 as described (Vieler et al., 2012) using TrizolTM (InvitrogenTM, United States) and the RNeasy® Mini Kit with DNase digest (Qiagen, Germany). Total single strand cDNA was generated using the RevertAid First Strand cDNA Synthesis Kit and an oligo-dT primer (Thermo ScientificTM, United States), followed by PCR amplification of PEX7 with specific primers (B-31, EWM28214.1; CCMP526, NGA_0680400; Supplementary Table 3). The specific 460-bp amplicon obtained with the primer pair of fw2 and rv1 was sequenced, which confirmed the cDNA identity.
In vivo Subcellular Targeting Analyses
Onion (Allium cepa L.) epidermal cells were transformed biolistically as described (Falter et al., 2019), followed by microscopic analyses 1–7 days post transformation (dpt). Arabidopsis protoplasts were transiently transformed as described (Yoo et al., 2007) and analyzed 1–2 dpt. For N. oceanica CCMP1779 transformation, the wild-type cells were grown under standard growth conditions in f/2 medium (22°C, 75 μmol photons m–2 s–1, 16/8 h light/dark) to mid-exponential growth phase and transformed by electroporation with 3 μg of vector (linearized by AhdI), and 30 μg of salmon sperm DNA (Invitrogen), as described (Vieler et al., 2012; de Grahl et al., 2020). Single colonies were grown on selective plates containing 50 μg/ml hygromycin, transferred to 96-well plates containing 200 μl of f/2 medium with hygromycin and were incubated for 7–10 days under standard growth conditions (de Grahl et al., 2020) for subcellular analysis by confocal microscopy.
Confocal and Transmission Electron Microscopy
For confocal microscopy, a Leica DMi8 inverted microscope was used coupled to a confocal spinning disc unit CSU X1 (Yokogawa Electric Corporation; Musashino, Japan). The system was equipped with a 445-nm laser for excitation of CFP and monomeric Cerulean and a 515-nm laser for excitation of EYFP and Venus with the corresponding emission filters (ET480/40m and ET535/30m, respectively). Image acquisition was performed using the VisiView software (Visitron Systems, Puchheim, Germany). Confocal images were captured as single planes with a QImaging OptiMOS sCMOS camera system.
For transmission electron microscopy (TEM), stable N. oceanica CCMP1779 transformants expressing NgMLS2-Venus were harvested in mid-exponential growth phase by centrifugation and were fixed with a mixture of 5 ml osmium tetroxide (1% in 0.1 M cacodylate buffer, pH 7.0), 2 ml sucrose [20 mM in 0.25× salinity artificial sea water (ASW)], 0.8 ml paraformaldehyde (16%) and 0.02 ml glutaraldehyde by incubation on ice for 1.5 h (Karlson et al., 1996). After four times washing with 0.25× salinity ASW, the cells were imbedded in 2% agarose. After dehydration with a graded ethanol series and LR-white-infiltration, ultrathin sections were obtained with an ultramicrotome (Ultracut E, Leica-Reichert-Jung, Nußloch, Germany). For immunogold labeling, a section containing the embedded algae was incubated with MSB (100 mM PIPES, 10 mM EDTA, 5 mM magnesium sulfate, pH 6.8) for 30 min and blocked with 3% BSA and 0.2% BSA-C in MSB for 30 min. After incubation with the primary anti-GFP antibody from rabbit (dilution 1:200; Abcam, Cambridge, United Kingdom), the section was washed five times with 1% BSA and 0.07% BSA-C in MSB and incubated with the secondary antibody [anti-rabbit IgG-Gold (10 nm), 1:50 dilution; Sigma Life Science, Taufkirchen, Germany] for 1 h and washed again. After a treatment with 1% glutaraldehyde and washing with water (three times), sections were incubated with 2% uranyl acetate for 10 s, washed with water once again and incubated with 0.2% lead citrate for 15 s. Sections were viewed with a LEO 906 E TEM (LEO, Oberkochen, Germany) equipped with the MultiScan CCD Camera (Model 794) of Gatan (Munich, Germany) using the Digital Micrograph software version 2.0.2. from Gatan to acquire, visualize, analyze, and process the image data.
Results
Prediction and Expression Analysis of a PEX7 Ortholog of N. gaditana
In stramenopile algae, experimental studies investigating peroxisome biogenesis and functions are presently restricted to the diatom Phaeodactylum, which was shown to lack the PTS2 import pathway (Gonzalez et al., 2011). To investigate whether the absence of the PTS2 pathway is a general feature of stramenopiles or specific to one or several subgroups, we chose the family of Eustigmatophyceae and Nannochloropsis as a representative genus (Supplementary Figure 1). To identify PEX proteins encoded in the N. gaditana genome, those of Arabidopsis thaliana (Supplementary Table 1) were used as queries for homology searches. Orthologs of nearly all A. thaliana PEX proteins were identified in N. gaditana (Supplementary Table 1), suggesting that the fundamental mechanisms of peroxisome biogenesis and proliferation are largely conserved in Eustigmatophyceae. The detection of Nannochloropsis homologs for the PTS2 protein receptor, PEX7, as well as the docking complex proteins, PEX13 and PEX14, supported the existence of the import pathway for PTS2-carrying matrix proteins in this microalga. To investigate whether the identified Nannochloropsis protein was a true PEX7 ortholog, we performed phylogenetic analysis of this protein in comparison to other PEX7 orthologs. Indeed, the putative Nannochloropsis PEX7 homologs shared a common ancestor with PEX7 homologs of other stramenopiles, such as brown algae (Ectocarpus), Pelagophyceae (e.g., Aureococcus) and oomycetes (e.g., Phytophthora, Figure 1). The tree topology also showed that PEX7 of stramenopiles is more closely related to PEX7 orthologs from green algae and land plants than to animal PEX7. Similar to Arabidopsis, Nannochloropsis PEX7 contains seven predicted WD40 repeats (Figure 2A).
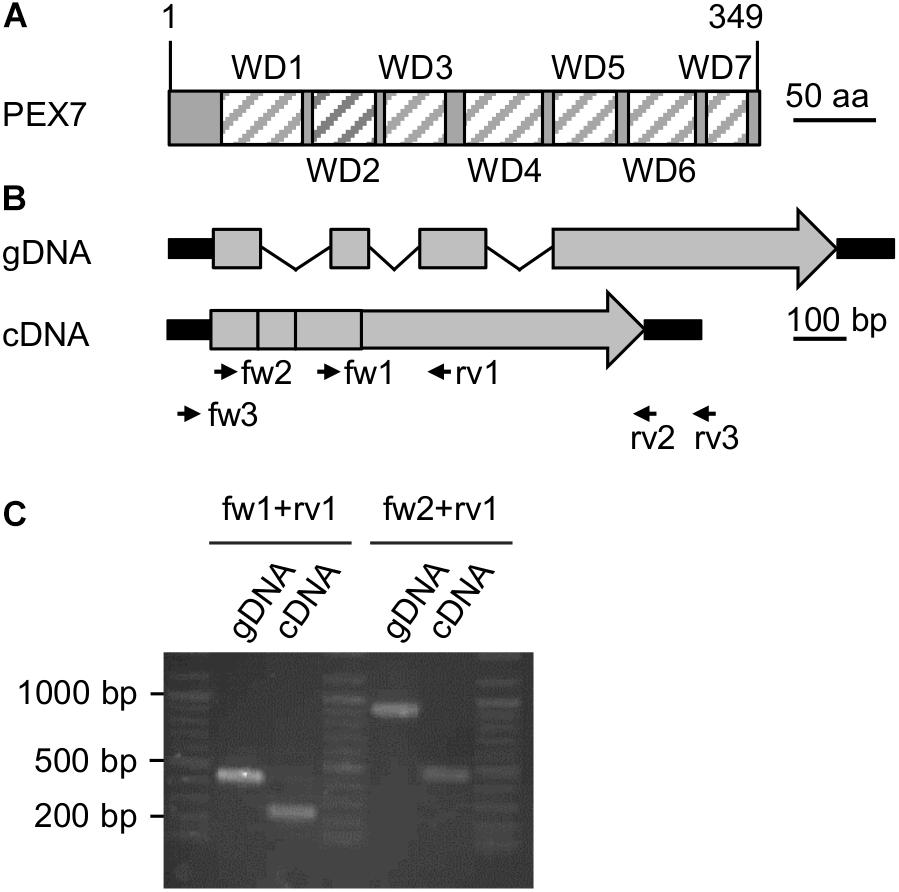
Figure 2. Expression analysis of a putative PEX7 ortholog from Nannochloropsis. (A) Domain structure of the predicted PEX7 protein from N. gaditana with seven predicted WD40 domains (striped boxes, WD1-7). (B) Predicted gene and cDNA structure of PEX7 from N. gaditana. Exons (gray boxes), introns (thin black lines) and UTRs (thick black lines) are indicated. The amplicon sizes were calculated for the primers fw1 + rv1 (gDNA: 420 bp; cDNA 240 bp) and for fw2 + rv1 (gDNA: 900 bp; cDNA: 460 bp). (C) PCR product size determination for gDNA and cDNA isolated from N. gaditana. The apparent sizes were consistent with the calculated sizes. Sequence analysis of the 460-bp amplicon obtained with fw2 and rv1 from cDNA confirmed its identity with the predicted cDNA and, hence, expression of the PEX7 gene in N. gaditana.
We next investigated experimentally whether the PEX7 gene was indeed expressed and involved in peroxisome biogenesis or represented an untranscribed pseudogene without protein function in vivo. Total RNA was isolated from N. gaditana strain CCMP526 grown to logarithmic growth phase and converted to single strand cDNA using an oligo-dT primer for subsequent PCR amplification by three different PEX7 primer pairs (Supplementary Table 3). While the full-length cDNA or CDS was not obtained, two different smaller fragments could indeed be amplified (Figure 2C). One primer pair binding to exons 3 and 4 amplified a 240-bp fragment from cDNA that clearly differed in size from the longer 420-bp fragment from genomic DNA (Figure 2B). The largest PEX7 CDS fragment of 460 bp spanned from the beginning of exon 1 to the middle of the 4th and last exon. Sequence analysis confirmed its identity with PEX7 and the lack of introns. The data demonstrated that PEX7 was indeed expressed and indicated that the PTS2 protein import pathway was functional in N. gaditana.
Prediction of PTS2 Cargo Proteins in N. gaditana
We next predicted the presence of putative PTS2 proteins by in silico analysis of the available genomes of N. gaditana strains and their deduced proteomes. We experienced that the protein predictions of N. gaditana strain B-31 were more reliable compared to those of N. gaditana CCMP526 and focused on the former. In brief, PTS2 containing proteins were identified in N. gaditana B-31 by a direct PTS2 search using a relatively stringent PTS2 motif, [RK][LVI]x5[HQ][LAF] (Kunze et al., 2011). More than 50 protein sequences were detected, 13 of which contained the PTS2 motif in the typical N-terminal 40-amino acid residue domain (Table 1). The putative PTS2 proteins included few homologs of Arabidopsis PTS2 proteins, including one malate synthase (referred to as NgMLS2; EWM30341.1) of the glyoxylate cycle and one (peroxisomal) 3-ketoacyl-CoA thiolase (NgPKT, EWM24705.1) involved in fatty acid β-oxidation. A third protein was homologous to the bifunctional A. thaliana transthyretin-like protein, also referred to as allantoin synthase (ALNS; Reumann et al., 2007; Lamberto et al., 2010). The C-terminal domain acts as a 5-hydroxyisourate hydrolase (HIUase, Supplementary Figure 2). Another Nannochloropsis protein was annotated as “protein kinase c binding protein” (EWM29206.1) and homologous to the Arabidopsis HIT3, which is a PTS2 protein of yet unknown physiological function identified in the peroxisomal proteome of Arabidopsis leaf peroxisomes (Reumann et al., 2009). The N. gaditana protein is referred to as NgHIT1 (Table 1). Hence, out of eleven known PTS2 protein families in Arabidopsis (Supplementary Table 2), four had predicted PTS2 homologs also in Nannochloropsis (Table 1 and Supplementary Table 2), further supporting the presence of PTS2 cargo and the existence of the PTS2 pathway in Nannochloropsis.
Interestingly, most of the known Arabidopsis PTS2 protein families had predicted PTS1- rather than PTS2-carrying homologs in Nannochloropsis (Supplementary Table 2), namely two acyl-CoA oxidases (both ARL>), one aspartate aminotransferase (AHL>), one citrate synthase (ARL>), one long-chain acyl-CoA synthetase (ARL>), and the N-terminal domain of the bifunctional A. thaliana transthyretin-like protein, which decarboxylates 2-oxo-4-hydroxy-4-carboxy-5-ureidoimidazoline (OHCU, SRL>, Supplementary Figure 2) as part of urate catabolism. Hence, key metabolic functions of Arabidopsis PTS2 proteins (glyoxylate cycle, fatty acid β-oxidation) appeared as being conserved in Nannochloropsis peroxisomes but seemed to be carried out by PTS1 homologs instead. Four other Arabidopsis proteins/families had apparent non-peroxisomal homologs in N. gaditana, including (i) malate dehydrogenase (MDH, but SHL> in N. oceanica CCMP1779), (ii) pseudouridine monophosphate glycosylase/indigoidine synthase A (PUMY/IndA), which forms a bifunctional fusion protein together with pseudouridine kinase/6-phosphofructokinase (PUKI/PfkB, Supplementary Figure 3), both of which are involved in (peroxisomal) pseudouridine catabolism in Arabidopsis (Chen and Witte, 2020), (iii) 1,4-dihydroxy-2-naphthoyl-CoA synthase (DHNS) involved in phylloquinone biosynthesis (Babujee et al., 2010), and (iv) one small heat-shock protein with an alpha-crystallin domain (Ma et al., 2006; Supplementary Table 2).
The other N. gaditana proteins with predicted PTS2 (Table 1) were not homologous to known Arabidopsis PTS2 proteins and indicated novel functions of Nannochloropsis peroxisomes. They included, for instance, an α/β-hydrolase domain-containing protein, also annotated as embryogenesis-associated protein EMB8 (EWM21473.1, RLx5QL), which atypically also contained a predicted PTS1 (SRL>).
Similar PTS2 protein predictions were carried out for the second N. gaditana strain CCMP526 and for N. oceanica CCMP1779, both of which further confirmed the PTS2 protein predictions. Atypically and interestingly, one predicted PTS2 protein of N. gaditana B-31 (EWM27137.1, hypothetical protein, RLx5HL) had a PTS1-containing homolog in N. oceanica CCMP1779 (protein ID: 564055, SRL>), strengthening the predicted peroxisome targeting of both orthologs. Predicted peroxisome targeting of these homologs in stramenopiles or other taxa indicated novel peroxisomal proteins and functions.
We validated the PTS2 protein predictions of N. gaditana by different complementary computational analyses, for instance, PTS2 conservation analysis in other photosynthetic stramenopiles, such as Nannochloropsis (N. oceanica, N. salina), Ectocarpus, Aureococcus (Pelagophyceae) and in heterotrophic stramenopiles, such as oomycetes (Supplementary Figure 1). The PTS2 of some proteins was well conserved (Supplementary Figure 4). For NgPKT, for instance, predicted PTS2 nonapeptides were detected in nearly all orthologs of Nannochloropsis, Ectocarpus, oomycetes and even in two early branching species (Hondaea fermentalgiana, slime net, and Cafeteria roenbergensis, Supplementary Figure 4A). In further support of true functional PTS2, all NgPKT orthologs contained hydrophobic residues at position 5 of their nonapeptides (Ile or Leu, Supplementary Figure 4A). In the four diatoms, the orthologs were N-terminally shortened by approximately 20 aa and possessed a PTS1 instead (SRL>, SSL>, and SAL>, data not shown). Hence, PTS2 conservation in stramenopiles outside of Bacillariophyceae (diatoms) together with PTS1 presence in diatoms in many stramenopile orthologs strongly supported the correct PTS2 prediction in NgPKT. By contrast, due to a lack of NgMLS2 orthologs in stramenopiles, PTS2 conservation analyses did not provide further support for correct PTS2 prediction in NgMLS2 (data not shown). Other predicted Nannochloropsis PTS2 proteins either lacked obvious orthologs in other stramenopiles or the PTS2 of the Nannochloropsis protein was not conserved (data not shown).
Because proteins rarely contain simultaneously two different N-terminal targeting signals to both peroxisomes (PTS2) and a second cell organelle (ER, mitochondria, plastids), we also investigated whether any PTS2 proteins were simultaneously predicted to be targeted to any non-peroxisomal cell organelle in stramenopiles, which would weaken our PTS2 prediction (e.g., HECTAR1; Gschloessl et al., 2008). Indeed, many putative PTS2 proteins were not predicted to additionally possess an N-terminal targeting signal for mitochondria, complex plastids or the ER (data not shown) except for NgHIT1 (see below).
In spermatophytes, the PTS2 is cleaved off in the peroxisomal matrix by the protease DEG15 (SKL>, Helm et al., 2007; Schuhmann et al., 2008; Dolze et al., 2013). A putative but weakly conserved homolog of DEG15 was indeed detected in Nannochloropsis (EWM21659). It could be aligned with AtDEG15 and putative stramenopile orthologs and clustered reasonably upon phylogenetic analysis (Supplementary Figures 5A,B). Peroxisome targeting and proteolytic activity of the Nannochloropsis homolog are supported by the presence of a predicted PTS1 (SLL>) and conservation of the catalytic triad (His-Asp-Ser, data not shown), respectively. The cleavage site of PTS2 proteins is characterized by a conserved Cys-containing motif closely downstream of the PTS2 nonapeptide (Kato et al., 1995, 1996). When searching for this feature, both NgPKT and NgHIT1 (but not NgMLS2) indeed contained such Cys (Supplementary Figure 6). Taken together, all these features of the predicted PTS2 cargo proteins and of the DEG15 peptidase further supported the existence of a functional PTS2 protein import pathway in Nannochloropsis.
In vivo Subcellular Targeting Analyses of Predicted PTS2 Proteins in Two Plant Expression Systems
For experimental validations of the predictions, we selected three putative PTS2 proteins (Table 1). To enhance surface exposure of the PTS2 peptide in the fluorescent protein fusion and to avoid oligomerization with endogenous isoforms and co-import by piggy-back mechanism, we restricted the gene fusion to the N-terminal exon (24–68 amino acid residues, Supplementary Figure 6) encoding the predicted PTS2. This domain was amplified from genomic DNA of N. gaditana CCMP526 and cloned into the transient vector, pJET. Sequence analysis verified cloning of the correct exons. The PTS2 domains were subcloned into the plant expression vector pCAT for expression from the strong CaMV 35S promoter and to create C-terminal fusion proteins with EYFP.
In the transient monocotyledon expression system of onion epidermal cells, the predicted PTS2 domain of NgMLS2 (RIx5HL) directed EYFP to small punctate structures that were identical with peroxisomes, as demonstrated by co-expression with the peroxisomal marker, cyan fluorescent protein (CFP-SKL, Figures 3A1, A2). EYFP alone served as negative control (data not shown). Also in the dicotyledon expression system of Arabidopsis protoplasts, the predicted PTS2 domain of NgMLS2 was sufficient to direct EYFP to peroxisomes (Figures 3B1, B2). Despite its canonical PTS2, however, the second PTS2 protein, NgPKT-EYFP (RLx5HL), remained cytosolic in onion epidermal cells even after extended expression times of 7 days (Figure 3A3). In Arabidopsis protoplasts, where weak peroxisome targeting can often be detected with higher sensitivity (Kataya and Reumann, 2010), the same fusion protein was targeted to peroxisomes in several cells, consistent with its predicted PTS2 (Figure 3B3). We concluded that both NgMLS2 and NgPKT were indeed peroxisomal matrix proteins of N. gaditana and were targeted to the organelle by the PTS2 pathway. While NgMLS2 was efficiently imported into peroxisomes in both plant expression systems, NgPKT was weakly imported into plant peroxisomes for yet unknown reasons.
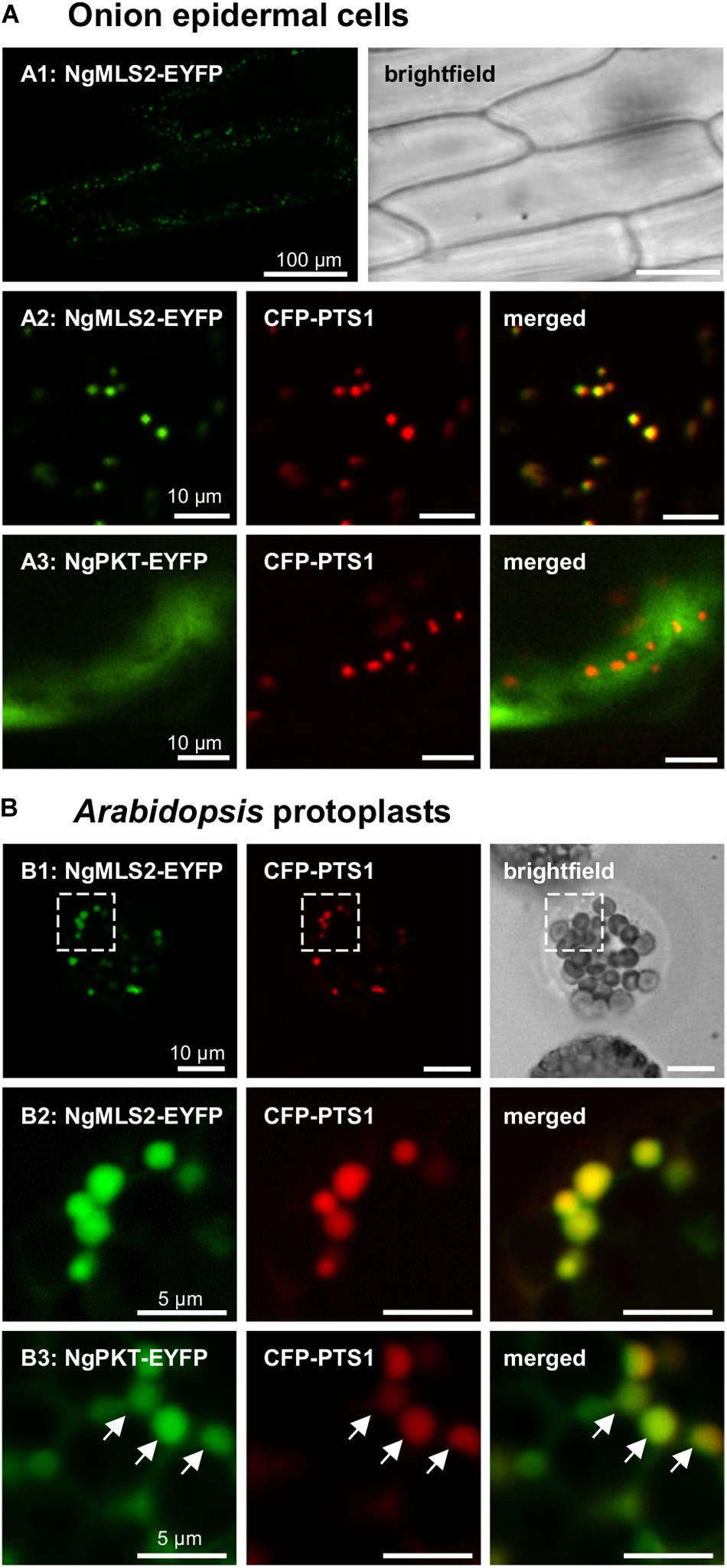
Figure 3. In vivo subcellular targeting analyses of two putative PTS2 proteins from N. gaditana in plant cells. (A) In onion epidermal cells, MLS2-EYFP co-localized with the peroxisome marker, CFP-PTS1 (already 1 dpt). However, NgPKT remained cytosolic, even after long-term expression of 7 dpt. The first exons of the putative PTS2 proteins from N. gaditana were fused C-terminally with EYFP and transiently co-expressed with CFP-PTS1 in onion epidermal cells. (B) In transiently transformed Arabidopsis protoplasts, NgMLS2-EYFP also co-localized with the peroxisomal marker (2 dpt). The boxed region shown in B1 is enlarged in B2. NgPKT-EYFP remained largely cytosolic but showed partial co-localization with the peroxisome marker 2 dpt. The arrows point out peroxisome targeting of NgPKT-EYFP.
The third representative protein, NgHIT1, showed a different subcellular targeting contrary to the PTS2 predictions. In onion epidermal cells, NgHIT1-EYFP labeled punctate structures that were not identical with and larger than peroxisomes. These structures had terminal extensions that were reminiscent of plastidic stromuli (Figures 4A1, A2). The fluorescent pattern of NgHIT1-EYFP was similar to that of Arabidopsis 6-phosphogluconolactonase 3 (AtPGL3-EYFP), which localizes to leucoplasts in onion epidermal cells and labels their stromuli extensions, as reported earlier (Figure 4A3; Reumann et al., 2004, 2007; Holscher et al., 2014). Consistent with the prediction of a mitochondrial presequence by HECTAR (score: 0.8, Gschloessl et al., 2008), NgHIT1-EYFP was additionally directed to mitochondria labeled with COXIV-CFP (Figure 4A2). Similar results of dual protein targeting to both mitochondria (Figures 4B1, B2) and plastids (Figure 4B3) were obtained in Arabidopsis protoplasts, where plastid targeting was even better detectable due to the larger plastids and their chlorophyll autofluorescence. In conclusion, two (out of three) predicted and experimentally investigated Nannochloropsis PTS2 proteins were indeed imported into peroxisomes in Arabidopsis protoplasts, confirming the existence of PTS2 cargo proteins in N. gaditana.
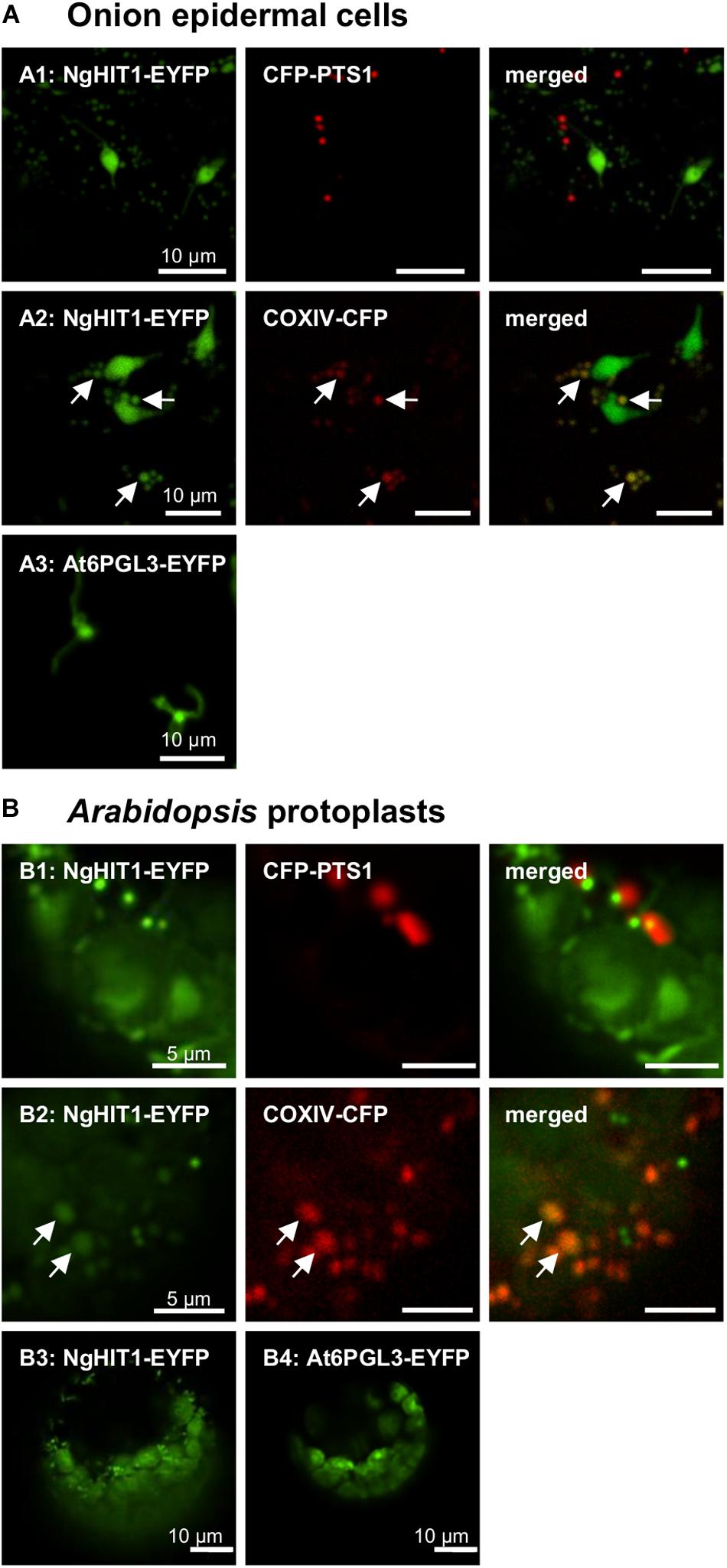
Figure 4. In vivo subcellular targeting analyses of a HIT homolog from N. gaditana in plant cells. (A) In onion epidermal cells, the putative PTS2 protein NgHIT1 was targeted to punctate structures of different sizes, some of which were larger than and not identical with peroxisomes labeled by CFP-PTS1 (A1). Yellow fluorescent organelle extensions were reminiscent of leucoplast stromuli (A1). Co-expression with a mitochondrial marker, COXIV-CFP, showed that NgHIT1 was partly targeted to mitochondria (A2). A known plastidic protein from Arabidopsis, At6PGL3-EYFP, labeled leucoplasts and their stromuli (A3; Reumann et al., 2007), showing that NgHIT1-EYFP was dually targeted to both plastids and mitochondria. (B) Likewise, in Arabidopsis protoplasts, NgHIT1 was targeted to small organelle-like structures, not identical with but frequently found in close proximity to peroxisomes (B1). Co-expression with the mitochondrial marker (COXIV-CFP) identified the organelles as mitochondria (B2). As in onion epidermal cells, NgHIT1-EYFP was also targeted to plastids (chloroplasts, B3), as was At6PGL3-EYFP (Reumann et al., 2007). Images were taken 2 dpt. The arrows point out mitochondrial targeting of NgHIT1-EYFP.
Peroxisome Targeting Analyses in Nannochloropsis
In order to investigate peroxisome targeting of predicted PTS2 proteins also in the homologous system of Nannochloropsis, selected PTS2 domains were subcloned behind the endogenous strong and constitutive promoter of elongation factor and in front of the Venus gene in an appropriate N. oceanica expression vector (pNoc ox Venus, Supplementary Table 3; Zienkiewicz et al., 2017; de Grahl et al., 2020). As a well-known heterologous PTS2 cargo protein, we used Arabidopsis peroxisomal malate dehydrogenase 1 (pMDH1, At2g22780). N. oceanica CCMP1779 was transformed by electroporation, and transformants were selected by hygromycin resistance. Single transformants were grown under standard growth conditions in liquid cultures in 96-well plates and were analyzed after 6–8 days by spinning disk confocal microscopy. Not only AtpMDH1 but also NgMLS2 directed Venus to small punctae (approximately 1–2 per cell) of high fluorescence, consistent with peroxisome targeting (Figures 5A,C). Punctae of similar size and shape were detected for a peroxisomal marker, i.e., monomeric blue fluorescent Cerulean, extended C-terminally by a PTS1 (mCer-SKL, Figure 5B). The small punctae labeled by NgHIT1-Venus, however, were more numerous per cell (Figure 5D). Hence, the punctae labeled by AtMDH1 and NgMLS2 provided additional indirect evidence for maintenance of the PTS2 protein import pathway in Nannochloropsis.
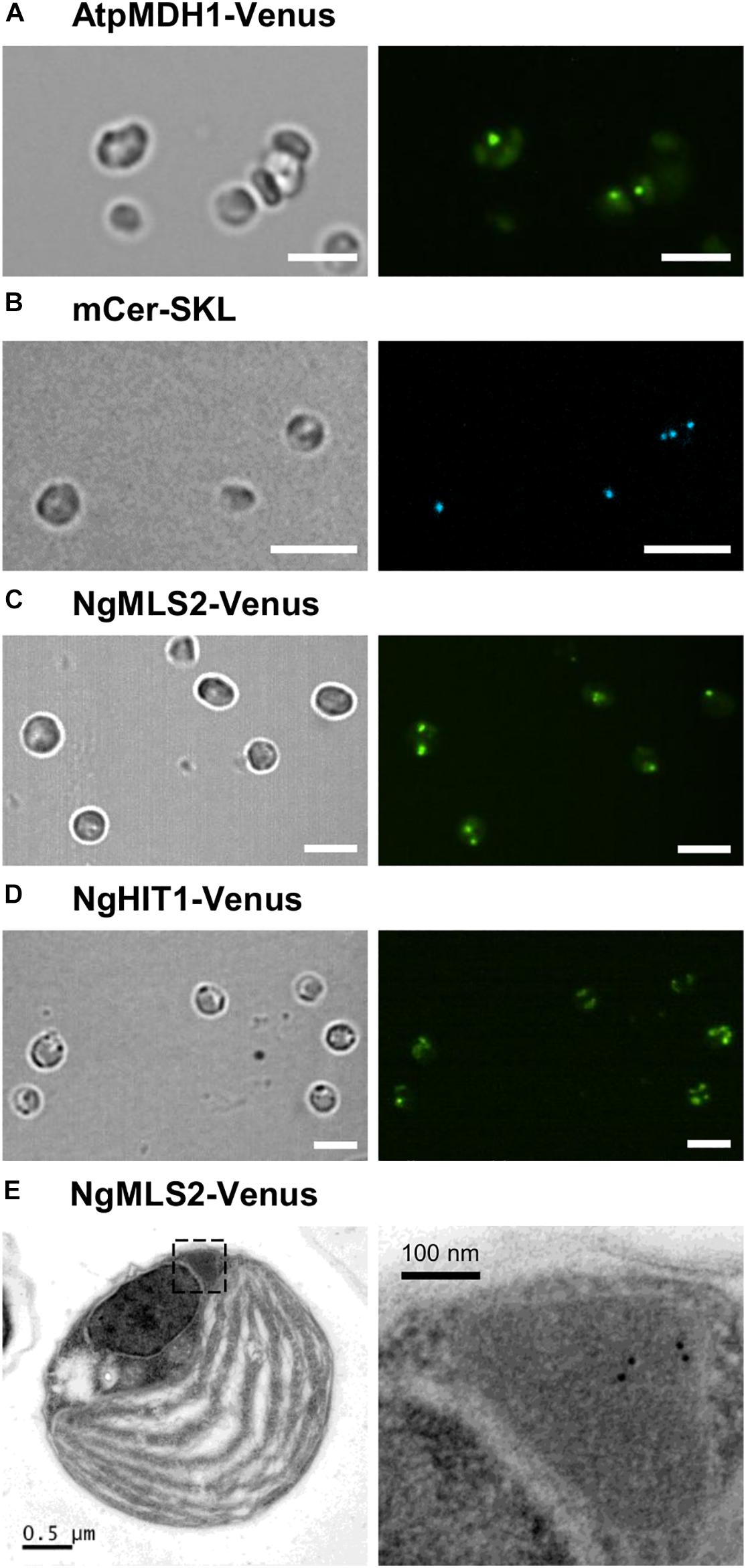
Figure 5. Peroxisome targeting analysis of representative Arabidopsis and Nannochloropsis PTS2 proteins in N. oceanica. (A) As positive control, the PTS2 protein, pMDH1 from A. thaliana (AtpMDH1), was C-terminally fused to Venus in the expression vector pNoc ox Venus. Wild-type N. oceanica was transformed by electroporation, and stable transformants were generated. Venus fluorescence was visible as small puncta indicative of AtpMDH1 targeting to peroxisomes. (B) To label peroxisomes, N. oceanica wt cells were transformed with an expression vector encoding blue fluorescent monomeric Cerulean extended by SKL> (mCer-SKL). (C) The first exon of NgMLS2 containing the predicted PTS2 was chosen representatively and fused similarly with Venus. In the corresponding N. oceanica transformants, Venus fluorescence was visible in a single small punctate structure, consistent with Venus targeting to Nannochloropsis peroxisomes. In some transformants, two or more punctate fluorescent structures were visible. Scale bar 5 μm. (D) NgHIT1-Venus was also targeted to small punctate structures, but additionally to larger round structures reminiscent of chloroplasts. Scale bars 5 μm in (A–D). (E) For validation of NgMLS2-Venus targeting to peroxisomes, TEM was carried out combined with immunogold-labeling using a primary antibody against GFP. Gold particles (10 nm) were specifically detected in peroxisomes. Scale bars as indicated.
To further validate PTS2 cargo targeting to peroxisomes by the PTS2 import pathway in N. oceanica, we investigated peroxisome targeting of NgMLS2-Venus by TEM, using primary antibodies against GFP and gold-labeled secondary antibodies. Peroxisomes were well distinguishable from chloroplasts, mitochondria, lipid bodies, and vacuoles by their size, the lack of internal membranes and by their protein-rich matrix. Approximately 60% of the visible algal peroxisomes were labeled at least by one gold particle per peroxisome, and approximately half of these contained several gold particles (Figure 5E). In contrast, no gold-labeled peroxisomes could be identified in the untransformed wild-type strain. Although a minor background of gold labeling was observed, no EYFP targeting to any other organelles like mitochondria or chloroplasts was visible. Thus, the TEM results provided complementary support for the identification of NgMLS2 as a true PTS2 cargo and functionality of the PTS2 protein import pathway in Nannochloropsis.
Taken together, by (i) verifying the expression of the cytosolic PEX7 receptor, (ii) predicting more than a dozen of PTS2 cargo proteins, and (iii) experimentally validating peroxisome targeting of representative PTS2 candidates in plants and Nannochloropsis, we concluded that the PTS2 protein import pathway of peroxisomes has been maintained in full functionality in the stramenopile alga Nannochloropsis, which contrasts peroxisome biogenesis in the sister group of diatoms (Figure 6).
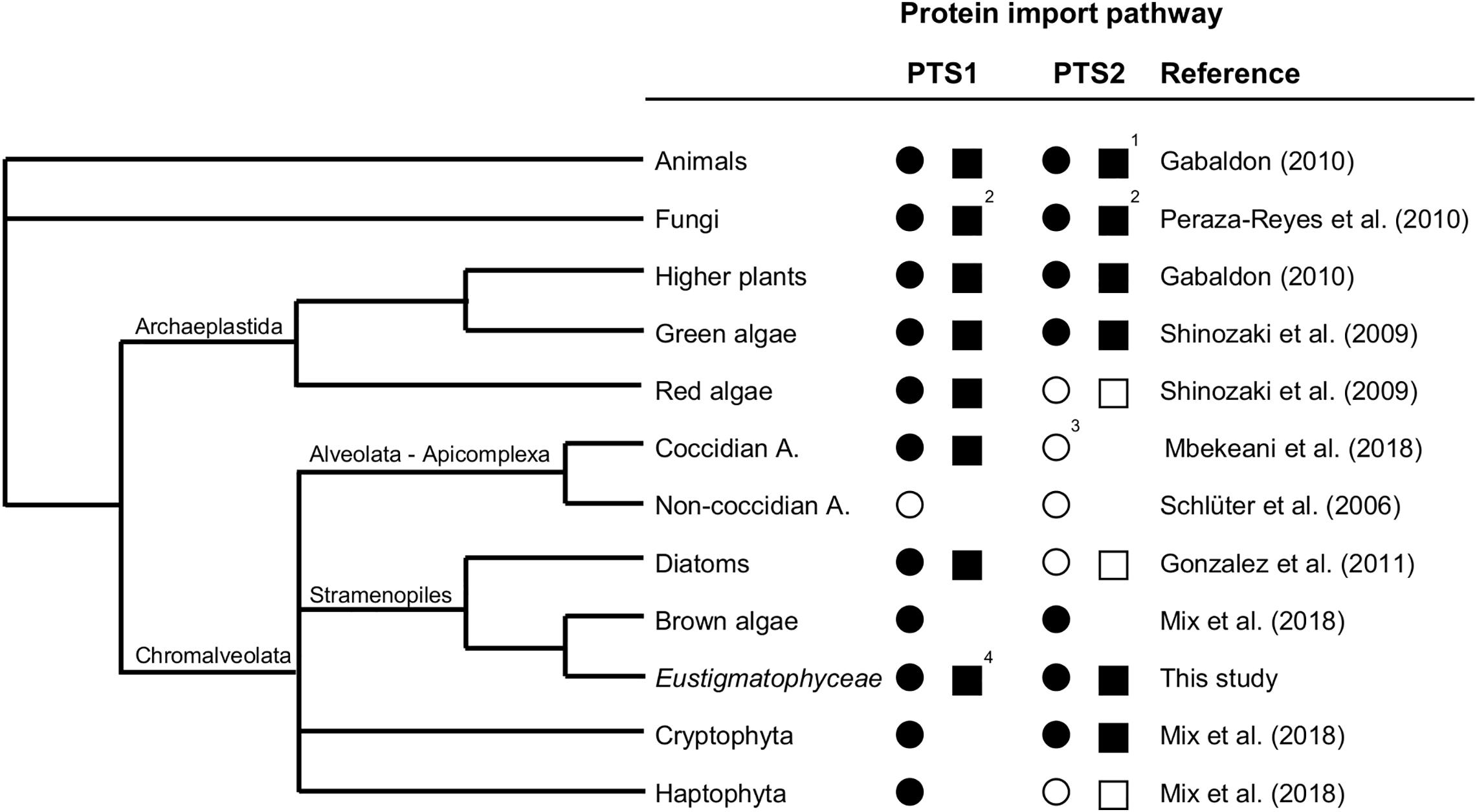
Figure 6. Existence and secondary loss of the peroxisomal targeting pathways for PTS1 and PTS2 proteins in different taxa. The predicted existence of the PTS1 and PTS2 protein import pathways are denoted as a filled circle (present in all representative organisms sequenced to date) or empty circle (absent in all representative organisms sequenced to date). Experimentally verified existence of the PTS1 and PTS2 protein import pathways are denoted as a filled square (presence shown at least for one representative organism) or empty square (absence demonstrated for one representative organism). 1Among animals, the PTS2 pathway has experimentally been shown to be absent in C. elegans and D. melanogaster. 2Among fungi, peroxisomes are missing in the rumen fungi, Neocallimasticalles and Microsporidia. 3In coccidian apicomplexa (e.g., Toxoplasma), which possess peroxisomes contrary to non-coccidian apicomplexa (e.g., Plasmodium), the absence of the PTS2 pathway is indicated by the lack of PEX7, PEX13, and PEX14, even though PEX5 possesses the typical PEX7 binding domain. 4Full functionality of the PTS1 import pathway has been demonstrated (Kechasov, 2017; Kechasov et al., in preparation). A., Apicomplexa.
Discussion
Land plants have an impressive and the largest number of PTS2 proteins that are directed to the peroxisomal matrix by the PTS2 import pathway, as exemplified by 20 PTS2 proteins in A. thaliana (Supplementary Table 2). They include very important enzymes of fatty acid β-oxidation (e.g., three acyl-CoA oxidases, three thiolases and long-chain acyl CoA synthetase 6) and of the glyoxylate cycle (three citrate synthases, two MDHs). Additional PTS2 proteins of Arabidopsis participate, for instance, in purine breakdown (transthyretin/ALNS, Reumann et al., 2007; Lamberto et al., 2010), pseudouridine catabolism (pseudouridine monophosphate glycosylase, Reumann et al., 2009; Reumann, 2011; Chen and Witte, 2020), and phylloquinone biosynthesis (DHNS; Babujee et al., 2010; Reumann, 2013). Given the large number, diversity and importance of these metabolic pathways, knowledge of the existence of the PTS2 import pathway in Nannochloropsis is prerequisite not only to understand evolution, speciation, and peroxisome biogenesis in Eustigmatophyceae, but also to be able to comprehensively map and model all metabolic pathways and competencies of peroxisomes in this genus by computational predictions, followed by experimental validations.
PEX7 is the cytosolic receptor that binds the PTS2 of cargo proteins and shuttles them to the PEX13/14 docking complex in the peroxisomal membrane (Woodward and Bartel, 2005; Ramon and Bartel, 2010). In organisms that lack the PTS2 import pathway, the PEX7 gene is generally absent (e.g., C. elegans, Motley et al., 2000; red algae, Shinozaki et al., 2009; P. tricornutum, Gonzalez et al., 2011). The only exception is D. melanogaster which possesses a functional full-length PEX7 gene (Baron et al., 2016), but lacks PTS2 cargo proteins identifiable by known PTS2 peptides. Our detection of a predicted PEX7 gene in N. gaditana B-31 is consistent with the report of a PEX7 gene in N. gaditana strain CCMP526 (Lauersen et al., 2016) and in N. oceanica CCMP1779 (Mix et al., 2018). The domain structure of the N. gaditana PEX7 homolog shows seven typical WD-40 repeats of approximately 40 amino acid residues that often terminate with Trp-Asp (Neer et al., 1994). The phylogenetic relationship of NgPEX7 with PEX7 orthologs of brown algae (E. siliculosus) and oomycetes confirmed its true PEX7 orthology (Figure 1). The closer relationship of stramenopile PEX7 to orthologs from green algae and higher plants than to animal PEX7 indicates its inheritance from the engulfed red alga upon endosymbiosis rather than from the heterotrophic host genome. Contrary to Phaeodactylum and consistent with maintenance of the PTS2 import pathway in Nannochloropsis, the predicted orthologs of the PTS1 protein receptor, PEX5, from N. gaditana and N. oceanica indeed possess the conserved middle domain of approximately 30 amino acid residues that binds PEX7 in A. thaliana and Homo sapiens (Supplementary Figure 7; Dodt et al., 2001), further indicating maintenance of the PTS2 import pathway. We also found PEX13 and PEX14 orthologs in N. gaditana strain B-31, both of which are required for PTS2 and PTS1 matrix protein import (Cross et al., 2016).
Experimental evidence that the PEX7 gene of Nannochloropsis was indeed expressed had not been reported but is crucial for PTS2 import pathway functionality. We could verify by RT-PCR that the region corresponding to the N-terminal half of PEX7 was indeed expressed in N. gaditana CCMP526 (Figure 2C), but all attempts to amplify the full-length PEX7 CDS from mRNA failed for yet unknown and most likely technical reasons. Since an oligo-dT primer had been used for single-strand cDNA synthesis from mRNA, we consider it very likely that PEX7 is indeed expressed in full length in N. gaditana. PEX7 gene expression in Nannochloropsis is further supported by transcriptomics data of N. gaditana strain B-31 (i.e., Naga_100004g18 in table S17 of Corteggiani Carpinelli et al., 2014) and strain CCMP526 (i.e., Nga06804 in http://nannochloropsis.genomeprojectsolutions-databases.com/Expression_comp.xls for CCMP526, Radakovits et al., 2012). Furthermore, we identified a full-length PEX7 mRNA in N. oceanica strain CCMP1779 in the JGI database (i.e., “583747” in https://mycocosm.jgi.doe.gov/cgi-bin/dispGeneModel?db=Nanoce1779_2&tid=583747, Vieler et al., 2012) that is highly similar to PEX7 of N. gaditana and covers the entire CDS (Supplementary Figure 8). Hence, PEX7 is undoubtedly expressed in full-length in N. oceanica. Taken together, all these PEX7 expression data of both species strengthen the indications that Nannochloropsis possesses a functional PTS2 import pathway.
The computational search for predicted PTS2 proteins in N. gaditana yielded, after filtering for the location of the PTS2 motif in the N-terminal 40-amino acid residue domain, 13 predicted PTS2 proteins. This filter was reasonable because, to the best of our knowledge, only two proteins are currently reported to carry the PTS2 at an internal position, namely ascomycete PEX8 and the plant transthyretin-like protein/ALNS involved in urate catabolism to allantoin (Reumann et al., 2007; Pessoa et al., 2010; Lamberto et al., 2010). Our evolutionary analyses revealed that the bifunctional plant enzyme arose in Viridiplantae shortly after divergence of rhodophytes in the last common ancestor of chlorophyceae and streptophytes by fusion of two single polypeptides, thereby placing the PTS2 internally (Supplementary Figure 2C). Stramenopiles evolved independently different improvements of protein structure and compartmentalization of purine catabolism. In diatoms (lacking the PTS2 pathway), both enzymes were fused but remained cytosolic. The extreme C-terminal four residues of HIUase (YRGS>), which are part of the active site, will not have allowed any PTS1 evolution. In Nannochloropsis, however, both enzymes stayed separated and were directed to peroxisomes (HIUase, RLx5HL; OHCU-DC, SRL>, Table 1 and Supplementary Figure 2D). Despite considerable sequence variation, the PTS2 of NgHIUase is very well conserved in diverse stramenopile orthologs, including a conserved hydrophobic residue at position 5 (Leu, Ile or Val, Supplementary Figure 4C). Hence, even though not investigated experimentally in this study, NgHIUase is most probably another correctly predicted PTS2 protein of N. gaditana.
Interestingly, many predicted PTS2 proteins of Nanno- chloropsis have in common that the PTS2 nonapeptide lies in the short first exon, while the functional protein domain generally starts with the 2nd exon. New PTS2 proteins may have been created by exon shuffling, placing the PTS2 containing first exon in front of novel genes to direct the gene products to peroxisomes. Exon shuffling can also result in alternative splicing of PTS2-encoding genes affecting subcellular localization of the proteins, as documented for A. thaliana (An et al., 2017).
The PTS2 motif [RK]-[LVI]-x5-[HQ][LAF] used for our Nannochloropsis genome screen was rather stringent (Kunze et al., 2011). More relaxed PTS2 motifs have been reported (Petriv et al., 2004; Reumann and Chowdhary, 2018). PTS2 conservation analysis of the newly detected N. gaditana PTS2 proteins in stramenopiles (Supplementary Figure 4) shows that most aligned nonapeptides are included in the motif, but notably not all. For instance, also Met and Val were found at position 2 (RMx5HL in Ectocarpus PKT; RVx5HL in Phytophthora cactorum HIUase) and at position 9 in several species (e.g., in RIx5HM in Achlya PKT, RLx5HV in Saprolegnia HIUase, Supplementary Figures 4A,C). Hence, at least these four nonapeptides are likely additional functional PTS2 nonapeptides in stramenopiles and shall be validated experimentally. Their application in future genome searches might allow the identification of additional PTS2 cargo proteins in Nannochloropsis.
Only few stramenopile microalgae can nowadays be transformed (Rathod et al., 2017). Moreover, in vivo subcellular targeting analyses of N. oceanica require 6–8 weeks from transformation to microscopy of liquid cultures (de Grahl and Rout, personal communication; de Grahl et al., 2020). The PTS1 and PTS2 motifs of both targeting pathways are well conserved in eukaryotes across kingdoms. Therefore, it is reasonable to investigate peroxisome targeting of microalgal proteins in transient expression systems of land plants. Indeed, two predicted PTS2 proteins from N. gaditana were correctly imported into plant peroxisomes (Figures 3A,B), confirming them as functional PTS2 nonapeptides and the proteins as correctly predicted PTS2 cargos. To validate these results in a homologous system, we expressed selected Nannochloropsis constructs also in N. oceanica. Not only Arabidopsis pMDH1 and the peroxisome marker, mCer-SKL, were targeted to small cell organelles, but also NgMLS2 (Figures 5A,B). The new blue fluorescent peroxisome marker, mCer-SKL, created in another expression vector containing a second resistance marker, shall allow in vivo co-localization studies in N. oceanica in future studies. TEM analysis identified the labeled structures as peroxisomes rather than mitochondria (Figure 5E). These results provided another independent line of evidence, leading altogether to the conclusion that the PTS2 import pathway remained fully active and functional in Nannochloropsis.
The enzyme malate synthase (MLS) is part of the peroxisomal glyoxylate cycle and very important for condensation of fatty acid-derived acetyl-CoA with glyoxylate to malate for carbohydrate synthesis (Kornberg and Krebs, 1957). Many organisms, such as plants, fungi, and animals (except for mammals) possess a single and PTS1-carrying MLS isoform. The same MLS isoform had previously been identified in representative genomes of chlorophytes and stramenopiles, but was absent in multicellular rhodophytes (i.e., Chondrus crispus) and haptophytes (i.e., E. huxleyi, Lauersen et al., 2016). While the authors detected only this single, PTS1-carrying MLS isoform in N. gaditana (EWM22958.1, SRL>, NgMLS1), we identified the second PTS2-carrying NgMLS2 in N. gaditana B-31 (NgMLS2, 52% sequence similarity with NgMLS1, Table 1). Due to a lack of orthologous isoforms in stramenopiles, PTS2 conservation analyses did not provide further support for correct PTS2 prediction in NgMLS2 (data not shown). Our experimental analyses demonstrated that NgMLS2 indeed possesses a functional PTS2 (RIx5HL) because the 1st exon of 68 aa in length was sufficient to direct EYFP to peroxisomes in two plant expression systems (Figures 3A,B). Also when expressed in N. oceanica, EYFP was detected in small fluorescent organelles that lacked internal membranes according to TEM analyses and were identified as peroxisomes rather than mitochondria (Figures 5C,E). Hence, NgMLS2 is a second MLS isoform of the peroxisomal glyoxylate cycle and a PTS2 cargo of the PTS2 import pathway in Nannochloropsis.
Interestingly, phylogenetic analysis showed that NgMLS2 and its few orthologs detected in specific oomycetes (e.g., Saprolegnia diclina VS20, Phytophthora parasitica), clusters together with the single (PTS1-containing) MLS isoform of animals in one clade (Supplementary Figure 9). Hence, the PTS2-carrying NgMLS2 is most likely of metazoan origin and stems from the heterotrophic host cell that engulfed the red alga approximately 1260 Mio years ago (Yoon et al., 2002). The 3rd detected MLS isoform (NgMLS3, EWM30541.1) is only distantly related to NgMLS1/2 and is most likely non-peroxisomal due to the lack of any predicted PTS1/2 (Supplementary Figure 9). By contrast, the PTS1-containing MLS1 isoform of N. gaditana is more closely related to those of the green lineage (e.g., chlorophytes, Volvox carteri; A. thaliana; P. tricornutum; and brown alga, E. siliculosus, Supplementary Figure 9). It will be interesting to learn why Nannochloropsis atypically maintained both the metazoan-like NgMLS2 and the plant-like NgMLS1 in peroxisomes and whether both enzymes specialized on slightly different functions.
3-Ketoacyl-CoA thiolase (also called thiolase) is a degradative key enzyme of fatty acid β-oxidation with broad chain-length specificity for its substrates. We found a single ortholog in N. gaditana, while the second N. gaditana thiolase (EWM30137.1) is a biosynthetic enzyme and orthologous to Arabidopsis acetyl-coenzyme A acetyltransferase, which converts two acetyl-CoA to acetoacetyl-CoA in the mevalonate pathway. The PTS2 of NgPKT was well conserved among stramenopile orthologs, which strongly supported the correct PTS2 prediction in NgPKT. However, when investigating peroxisome targeting of NgPKT experimentally in vivo, the N-terminal 22-aa peptide of this protein did not detectably direct EYFP to peroxisomes in onion epidermal cells, even after long-term expression times (Figure 3A). In Arabidopsis protoplasts, however, peroxisome targeting was better and clearly detectable, but notably only in some cells, while the fusion protein remained cytosolic in others. Apparently, in land plants, the PTS2 of NgPKT was indeed functional but rather weak and inefficient in mediating peroxisome import for yet unknown reasons. A striking feature of the PTS2 of nearly all plant 3-ketoacyl-CoA thiolases is their atypical glutamine residue at position 2, replacing the normally hydrophobic residues (i.e., L, V or I; e.g., AtPKT3: RQx5HL). This residue is not only invariant in plant thiolases but also is very specific for thiolases. Hence, plant PEX7 might have evolved specific structural features for efficient recognition, import and possibly prioritization of plant thiolase based on its specific PTS2, and these plant PEX7 features might not be well compatible with the “atypical” PTS2 of NgPKT (RLx5HL). Future studies shall address whether NgPKT is efficiently imported into N. oceanica peroxisomes.
Contrary to NgMLS2 and NgPKT, the predicted PTS2 of NgHIT1 did not direct EYFP to peroxisomes. Consistent with the prediction of a bipartite ER/mitochondrial targeting signal by HECTAR (score: 0.80; Gschloessl et al., 2008), the punctate structures were indeed identified as mitochondria in both plant expression systems, using the presequence of Saccharomyces cerevisiae COXIV and CFP as mitochondrial marker (Fulda et al., 2002). Consistently, structural similarities between PTS2 and mitochondrial presequences have been reported (Osumi et al., 1992; Kunze et al., 2011; Kunze and Berger, 2015). Interestingly, NgHIT1-EYFP was dually targeted to both mitochondria and plastids (Figure 4). A second HIT homolog seemed non-peroxisomal (NgHIT2/3, EWM21969.1 and EWM28795.1, both w/o PTS2/PTS1). Peroxisome targeting of AtHIT3 had been validated by in vivo subcellular targeting analyses (Quan et al., 2010). Arabidopsis HINT4 showed a dual activity in vitro, acting on adenosine 5′-phosphosulfate both as a hydrolase (forming AMP) and as phosphorylase (forming ADP, Guranowski et al., 2010).
In addition to the predicted PTS2 proteins of N. gaditana that are homologs of Arabidopsis PTS2 proteins, nine proteins represent putative novel Nannochloropsis PTS2 proteins. In the protein annotated as “embryogenesis-associated protein EMB8” (EWM21473.1, Table 1) the predicted PTS2 (RLx5QL) was not conserved in closely related homologs and already mutated to a non-PTS2 in N. salina (RLx5RL). Nevertheless, the protein most likely is a true peroxisomal protein because the N. gaditana protein itself additionally possesses a predicted and conserved PTS1 (SRL>), which is also found in the homologs of N. salina (SRL>), Ectocarpus (ARL>), diatoms (Phaeodactylum, Thalassiosira, Pseudo-nitzschia, SRL>, Fistulifera, SKL>) but not in oomycetes. The PTS1 protein belongs to the functionally diverse superfamily of α/β hydrolases that includes diverse enzymes (proteases, lipases, peroxidases, esterases, epoxide hydrolases and dehalogenases), all characterized by a specific catalytic triad (Ser, Glu/Asp, and His). It will be interesting to study which physiological function this α/β hydrolase carries out in peroxisomes of stramenopiles.
The PTS2 of the remaining eight Nannochloropsis proteins, however, was hardly conserved in homologs outside of the genus Nannochloropsis (data not shown, e.g., vacuolar transporter chaperone 4, aldo/keto reductase, didehydrogluconate reductase; acetyl-/succinylornithine aminotransferase; two hypothetical proteins, Table 1). The data indicate that these PTS2 proteins are either specific to Nannochloropsis or represent false predictions.
The presence of peroxisomes in nearly all eukaryotes entails their vital importance in eukaryotes. The physiological significance of peroxisomes is further supported by evolution and maintenance of two conserved import pathways for soluble proteins to the peroxisomal matrix in most organisms. We concluded that N. gaditana maintained a fully functional PTS2 import pathway based on (i) the expression of an N. gaditana PEX7 ortholog, (ii) the detection of 13 predicted PTS2 cargo proteins, (iii) our experimental validation of two proteins as true positives in vivo in diverse expression systems (NgMLS2 and NgPKT), and (iv) due to two additional PTS2 proteins predicted with high probability by complementary computational methodology (NgHIUase and NgEMB8). Due to single genes of these PTS2 cargo proteins in Nannochloropsis, this import pathway is crucial at least for two essential metabolic pathways of peroxisomes, namely fatty acid β-oxidation (NgPKT) and purine (urate) catabolism (NgHIUase), while the glyoxylate cycle might remain functional by activity of the PTS1-carrying MLS1 alone. The number of at least four probable PTS2 cargo proteins is considerable and exceeds, for instance, the number of only three PTS2 proteins in ascomycetes, such as S. cerevisiae.
To summarize the current knowledge about evolution and maintenance of the PTS1 and PTS2 import pathways in different taxa, we present a simplified phylogenetic tree focusing on stramenopiles (Figure 6). The PTS1 targeting pathway is present in all eukaryotes that possess peroxisomes. Generally, animals, fungi, and Viridiplantae (land plants, charophytes, and green algae) possess both PTS1/2 import pathways (Tolbert and Essner, 1981; Gabaldón, 2010) with a few exceptions due to secondary PTS2 pathway loss in specific animals (C. elegans, Drosophila, see section “Introduction”) and the rumen fungi, Neocallimasticalles and Microsporidia (Peraza-Reyes et al., 2010). The PTS2 import pathway is considered the more ancient import route for peroxisomal matrix proteins (Gabaldon et al., 2006; Bolte et al., 2015; Kunze and Berger, 2015; Reumann et al., 2016). By contrast, chromalveolates and particularly stramenopiles are amazingly heterogeneous with respect to both the presence of peroxisomes and the PTS2 import pathway. Non-coccidian apicomplexa (e.g., Plasmodium) lack peroxisomes entirely, while coccidian apicomplexa (e.g., Toxoplasma) possess solely the PTS1 import pathway (Schluter et al., 2006; Mbekeani et al., 2018), similar to nowadays red algae (Matsuzaki et al., 2004; Shinozaki et al., 2009). As shown by this study, some stramenopiles have maintained both pathways (Eustigmatophyceae, brown algae), while diatoms lost the PTS2 pathway entirely and transferred classical PTS2 cargo proteins (e.g., thiolase) to the PTS1 import pathway (Figure 6; Gonzalez et al., 2011). Because several classical PTS2 cargo proteins of animals, plants and amoebozoa (e.g., Dictyostelium) are PTS1 proteins in Nannochloropsis (e.g., citrate synthase, acyl-CoA oxidase, long-chain acyl-CoA synthetase, Supplementary Table 2), Nannochloropsis seems to follow the same evolutionary path of PTS2-to-PTS1 cargo conversion. Hence, PTS2-to-PTS1 cargo transition occurred in Nannochloropsis prior and independent of any mutational PEX7 gene inactivation and while the PTS2 pathway is still fully functional. The driving force and evolutionary advantage for PTS2-to-PTS1 cargo transition remains elusive.
The absence of the PTS2 pathway in several groups of Chromalveolates can partly be explained by their evolutionary history. The organisms evolved undoubtedly by secondary endosymbiosis of a red alga, proven by the presence of chlorophyll c in their plastids (Cavalier-Smith, 1999). Recent publications support a polyphyletic origin of different subgroups of Chromalveolates rather than their common monophyletic origin because many nuclear, mitochondrial and plastidic genes were found to stem from unexpected origins, including even green algae, non-cyanobacterial prokaryotes and genes from sister groups of Chromalveolates (Yoon et al., 2002; Baurain et al., 2010; Keeling, 2010; Qiu et al., 2013; Dorrell et al., 2017). Moreover, Chromalveolates are now known to have gone through several independent endosymbiotic events. Hence, at least the two nuclear genomes, namely that of the heterotrophic host cell (which encoded all PEX and cargo proteins of both peroxisomal import pathways) and that of the red alga (which encoded all PEX and cargo proteins at least of the PTS1 pathway), were merged upon red alga engulfment in Chromalveolates. This new quasi-duplicated chimeric “nuclear genome entity” likely has allowed and favored the gradual reduction of PTS2 cargo numbers up to the complete loss of the PTS2 pathway for several reasons: (i) the evolutionary driving force for size reduction of the duplicated genome, (ii) the advantage that the red alga probably had already established and could contribute its (yet unknown) mechanism of PTS2-to-PTS1 cargo transition, and (iii) the simultaneous presence of two orthologous gene copies of peroxisomal enzymes, allowing PTS2-to-PTS1 cargo conversion of one copy via non-functional cytosolic enzyme intermediates (Reumann et al., 2016). The heterogeneity of the PTS2 pathway presence and its differential usage intensity in stramenopiles (Figure 6) support the hypothesis of an independent loss of the PTS2 pathway in red algae and several groups of Chromalveolates. In any case, the nuclear genome of the red alga definitely had a significant impact on genome enrichment and peroxisome biogenesis in ancestral Chromalveolates, which favored diversification, speciation and the amazing success of nowadays chromalveolates in conquering ecological niches.
Data Availability Statement
The original contributions presented in the study are included in the article/Supplementary Material, further inquiries can be directed to the corresponding author.
Author Contributions
DK and SR conceived and designed the research, and wrote the manuscript with contributions by IG. DK performed the computational analyses and cloned the N. gaditana genes. DK and PE performed the subcellular targeting analyses in plants. IG carried out PEX7 expression and subcellular targeting analyses in N. oceanica. All authors read and approved the manuscript.
Funding
The research was carried out within the project “Microalgae 2021” (project number 226973/E40) funded by the Research Council of Norway (RCN) and supported by start-up funding of the University of Hamburg (Hamburg, Germany).
Conflict of Interest
The authors declare that the research was conducted in the absence of any commercial or financial relationships that could be construed as a potential conflict of interest.
The handling editor is currently organizing a Research Topic with one of the author SR.
Acknowledgments
We acknowledge funding of this research project by the Research Council of Norway (RCN) and the University of Hamburg (Hamburg, Germany). We are grateful to Prof. C. Benning (Michigan State University, East Lansing, United States) for providing the expression vector pNoc ox Venus. We also would like to thank Elke Wölken (Department of Aquatic Ecophysiology and Phycology, University of Hamburg) for analyses of immunogold-labeled N. oceanica transformants by transmission electron microscopy.
Supplementary Material
The Supplementary Material for this article can be found online at: https://www.frontiersin.org/articles/10.3389/fcell.2020.593922/full#supplementary-material
Abbreviations
aa, amino acid; ALNS, allantoin synthase; ASW, artificial sea water; At, Arabidopsis thaliana; CaMV, cauliflower mosaic virus; DC, decarboxylase; DECR, 2,4-dienoyl-CoA reductase; DHNS, 1,4-dihydroxy-2-naphthoyl-CoA synthase; dpt, days post transformation; EMB8, embryogenesis-associated protein 8; EPA, eicosapentaenoic acid; EYFP/GFP, enhanced yellow/green fluorescent protein; HIT, histidine triad family protein; HIUase, 5-hydroxyisourate hydrolase; IndA, indigoidine synthase A; MDH, malate dehydrogenase; MLS, malate synthase; Ng, Nannochloropsis gaditana; OHCU, 2-oxo-4-hydroxy-4-carboxy-5-ureidoimidazoline; PEX, peroxin; PfkB, 6-phosphofructokinase; PGL3, 6-phosphogluconolactonase 3; PKT, peroxisomal 3-ketoacyl thiolase; PTS1/2, peroxisomal targeting signal type 1/2; PUFA, polyunsaturated fatty acid; PUKI, pseudouridine kinase; PUMY, pseudouridine monophosphate glycosylase; TEM, transmission electron microscopy.
Footnotes
References
An, C., Gao, Y., Li, J., Liu, X., Gao, F., and Gao, H. (2017). Alternative splicing affects the targeting sequence of peroxisome proteins in Arabidopsis. Plant Cell Rep. 36, 1027–1036. doi: 10.1007/s00299-017-2131-2
Babujee, L., Wurtz, V., Ma, C., Lueder, F., Soni, P., Van Dorsselaer, A., et al. (2010). The proteome map of spinach leaf peroxisomes indicates partial compartmentalization of phylloquinone (vitamin K1) biosynthesis in plant peroxisomes. J. Exp. Bot. 61, 1441–1453. doi: 10.1093/jxb/erq014
Baron, M. N., Klinger, C. M., Rachubinski, R. A., and Simmonds, A. J. (2016). A systematic cell-based analysis of localization of predicted Drosophila peroxisomal proteins. Traffic 17, 536–553. doi: 10.1111/tra.12384
Barsanti, L., and Gualtieri, P. (2014). Algae: Anatomy, Biochemistry, and Biotechnology. Boca Raton, FL: CRC Press.
Bartel, B., Burkhart, S. E., and Fleming, W. A. (2014). “Protein transport in and out of plant peroxisomes”, in Molecular Machines Involved in Peroxisome Biogenesis and Maintenance, eds C. Brocard and A. Hartig (Vienna: Springer), 325–345. doi: 10.1007/978-3-7091-1788-0_14
Baurain, D., Brinkmann, H., Petersen, J., Rodriguez-Ezpeleta, N., Stechmann, A., Demoulin, V., et al. (2010). Phylogenomic evidence for separate acquisition of plastids in cryptophytes, haptophytes, and stramenopiles. Mol. Biol. Evol. 27, 1698–1709. doi: 10.1093/molbev/msq059
Benson, D. A., Karsch-Mizrachi, I., Lipman, D. J., Ostell, J., and Wheeler, D. L. (2005). GenBank. Nucleic Acids Res. 33, D34–D38.
Bolte, K., Rensing, S. A., and Maier, U. G. (2015). The evolution of eukaryotic cells from the perspective of peroxisomes: phylogenetic analyses of peroxisomal beta-oxidation enzymes support mitochondria-first models of eukaryotic cell evolution. Bioessays 37, 195–203. doi: 10.1002/bies.201400151
Boratyn, G. M., Camacho, C., Cooper, P. S., Coulouris, G., Fong, A., Ma, N., et al. (2013). BLAST: a more efficient report with usability improvements. Nucleic Acids Res. 41, W29–W33.
Brocard, C., and Hartig, A. (2006). Peroxisome targeting signal 1: Is it really a simple tripeptide? Biochim. Biophys. Acta 1763, 1565–1573. doi: 10.1016/j.bbamcr.2006.08.022
Camacho-Rodriguez, J., Gonzalez-Cespedes, A. M., Ceron-Garcia, M. C., Fernandez-Sevilla, J. M., Acien-Fernandez, F. G., and Molina-Grima, E. (2014). A quantitative study of eicosapentaenoic acid (EPA) production by Nannochloropsis gaditana for aquaculture as a function of dilution rate, temperature and average irradiance. Appl. Microbiol. Biotechnol. 98, 2429–2440. doi: 10.1007/s00253-013-5413-9
Cavalier-Smith, T. (1999). Principles of protein and lipid targeting in secondary symbiogenesis: euglenoid, dinoflagellate, and sporozoan plastid origins and the eukaryote family tree. J. Eukaryot. Microbiol. 46, 347–366. doi: 10.1111/j.1550-7408.1999.tb04614.x
Chen, M., and Witte, C. P. (2020). A kinase and a glycosylase catabolize pseudouridine in the peroxisome to prevent toxic pseudouridine monophosphate accumulation. Plant Cell 32, 722–739. doi: 10.1105/tpc.19.00639
Corteggiani Carpinelli, E., Telatin, A., Vitulo, N., Forcato, C., D’Angelo, M., Schiavon, R., et al. (2014). Chromosome scale genome assembly and transcriptome profiling of Nannochloropsis gaditana in nitrogen depletion. Mol. Plant 7, 323–335. doi: 10.1093/mp/sst120
Cross, L. L., Ebeed, H. T., and Baker, A. (2016). Peroxisome biogenesis, protein targeting mechanisms and PEX gene functions in plants. Biochim. Biophys. Acta 1863, 850–862. doi: 10.1016/j.bbamcr.2015.09.027
de Grahl, I., Rout, S. S., Maple-Grødem, J., and Reumann, S. (2020). Development of a constitutive and an auto-Inducible high-yield expression system for recombinant protein production in the microalga Nannochloropsis oceanica. Appl. Microbiol. Biotechnol. 104, 8747–8760. doi: 10.1007/s00253-020-10789-4
Dereeper, A., Guignon, V., Blanc, G., Audic, S., Buffet, S., Chevenet, F., et al. (2008). Phylogeny.fr: robust phylogenetic analysis for the non-specialist. Nucleic Acids Res. 36, W465–W469.
Dodt, G., Warren, D., Becker, E., Rehling, P., and Gould, S. J. (2001). Domain mapping of human PEX5 reveals functional and structural similarities to Saccharomyces cerevisiae Pex18p and Pex21p. J. Biol. Chem. 276, 41769–41781. doi: 10.1074/jbc.m106932200
Dolze, E., Chigri, F., Howing, T., Hierl, G., Isono, E., Vothknecht, U. C., et al. (2013). Calmodulin-like protein AtCML3 mediates dimerization of peroxisomal processing protease AtDEG15 and contributes to normal peroxisome metabolism. Plant Mol. Biol. 83, 607–624. doi: 10.1007/s11103-013-0112-6
Dorrell, R. G., Gile, G., Mccallum, G., Meheust, R., Bapteste, E. P., Klinger, C. M., et al. (2017). Chimeric origins of ochrophytes and haptophytes revealed through an ancient plastid proteome. eLife 6:e23717.
Edgar, R. C. (2004). MUSCLE: multiple sequence alignment with high accuracy and high throughput. Nucleic Acids Res. 32, 1792–1797. doi: 10.1093/nar/gkh340
Falter, C., Thu, N. B. A., Pokhrel, S., and Reumann, S. (2019). New guidelines for fluorophore application in peroxisome targeting analyses in transient plant expression systems. J. Integr. Plant Biol. 61, 884–899.
Faust, J. E., Verma, A., Peng, C., and Mcnew, J. A. (2012). An inventory of peroxisomal proteins and pathways in Drosophila melanogaster. Traffic 13, 1378–1392. doi: 10.1111/j.1600-0854.2012.01393.x
Fulda, M., Shockey, J., Werber, M., Wolter, F. P., and Heinz, E. (2002). Two long-chain acyl-CoA synthetases from Arabidopsis thaliana involved in peroxisomal fatty acid beta-oxidation. Plant J. 32, 93–103. doi: 10.1046/j.1365-313x.2002.01405.x
Gabaldón, T. (2010). Peroxisome diversity and evolution. Philos. Trans. R. Soc. Lond. B Biol. Sci. 365, 765–773. doi: 10.1098/rstb.2009.0240
Gabaldon, T., Snel, B., Van Zimmeren, F., Hemrika, W., Tabak, H., and Huynen, M. A. (2006). Origin and evolution of the peroxisomal proteome. Biol. Direct 1:8.
Gatto, G. J. Jr., Geisbrecht, B. V., Gould, S. J., and Berg, J. M. (2000). Peroxisomal targeting signal-1 recognition by the TPR domains of human PEX5. Nat. Struct. Biol. 7, 1091–1095.
Gonzalez, N. H., Felsner, G., Schramm, F. D., Klingl, A., Maier, U. G., and Bolte, K. (2011). A single peroxisomal targeting signal mediates matrix protein import in diatoms. PLoS One 6:e25316. doi: 10.1371/journal.pone.0025316
Gould, S. J., Keller, G. A., Hosken, N., Wilkinson, J., and Subramani, S. (1989). A conserved tripeptide sorts proteins to peroxisomes. J. Cell Biol. 108, 1657–1664. doi: 10.1083/jcb.108.5.1657
Graham, I. A., and Eastmond, P. J. (2002). Pathways of straight and branched chain fatty acid catabolism in higher plants. Prog. Lipid Res. 41, 156–181. doi: 10.1016/s0163-7827(01)00022-4
Gschloessl, B., Guermeur, Y., and Cock, J. M. (2008). HECTAR: a method to predict subcellular targeting in heterokonts. BMC Bioinformatics 9:393. doi: 10.1186/1471-2105-9-393
Guiry, M. D. (2012). How many species of algae are there? J. Phycol. 48, 1057–1063. doi: 10.1111/j.1529-8817.2012.01222.x
Guranowski, A., Wojdyla, A. M., Zimny, J., Wypijewska, A., Kowalska, J., Jemielity, J., et al. (2010). Dual activity of certain HIT-proteins: A. thaliana Hint4 and C. elegans DcpS act on adenosine 5’-phosphosulfate as hydrolases (forming AMP) and as phosphorylases (forming ADP). FEBS Lett. 584, 93–98. doi: 10.1016/j.febslet.2009.11.003
Hayashi, Y., and Shinozaki, A. (2012). Visualization of microbodies in Chlamydomonas reinhardtii. J. Plant Res. 125, 579–586. doi: 10.1007/s10265-011-0469-z
Helm, M., Luck, C., Prestele, J., Hierl, G., Huesgen, P. F., Froehlich, T., et al. (2007). Dual specificities of the glyoxysomal/peroxisomal processing protease Deg15 in higher plants. Proc. Natl. Acad. Sci. U.S.A. 104, 11501–11506. doi: 10.1073/pnas.0704733104
Holscher, C., Meyer, T., and von Schaewen, A. (2014). Dual-targeting of Arabidopsis 6-phosphogluconolactonase 3 (PGL3) to chloroplasts and peroxisomes involves interaction with Trx m2 in the cytosol. Mol. Plant 7, 252–255. doi: 10.1093/mp/sst126
Karlson, B., Potter, D., Kuylenstierna, M., and Andersen, R. A. (1996). Ultrastructure, pigment composition, and 18S rRNA gene sequence for Nannochloropsis granulata sp. nov. (Monodopsidaceae, Eustigmatophyceae), a marine ultraplankter isolated from the Skagerrak, northeast Atlantic Ocean. Phycologia 35, 253–260. doi: 10.2216/i0031-8884-35-3-253.1
Kataya, A. R., and Reumann, S. (2010). Arabidopsis glutathione reductase 1 is dually targeted to peroxisomes and the cytosol. Plant Signal. Behav. 5, 171–175. doi: 10.4161/psb.5.2.10527
Kato, A., Hayashi, M., Kondo, M., and Nishimura, M. (1996). Targeting and processing of a chimeric protein with the N-terminal presequence of the precursor to glyoxysomal citrate synthase. Plant Cell 8, 1601–1611. doi: 10.2307/3870253
Kato, A., Hayashi, M., Mori, H., and Nishimura, M. (1995). Molecular characterization of a glyoxysomal citrate synthase that is synthesized as a precursor of higher molecular mass in pumpkin. Plant Mol. Biol. 27, 377–390. doi: 10.1007/bf00020191
Kechasov, D. (2017). Development of omega-3 fatty acid-rich Nannochloropsis strains. no. 358, University of Stavanger. Available online at: https:// bibsys-almaprimo.hosted.exlibrisgroup.com/permalink/f/7jaidg/BIBSYS_ILS71 547159420002201
Keeling, P. J. (2010). The endosymbiotic origin, diversification and fate of plastids. Philos. Trans. R. Soc. Lond. B Biol. Sci. 365, 729–748. doi: 10.1098/rstb.2009.0103
Kornberg, H. L., and Krebs, H. A. (1957). Synthesis of cell constituents from C2-units by a modified tricarboxylic acid cycle. Nature 179, 988–991. doi: 10.1038/179988a0
Kragler, F., Lametschwandtner, G., Christmann, J., Hartig, A., and Harada, J. J. (1998). Identification and analysis of the plant peroxisomal targeting signal 1 receptor NtPEX5. Proc. Natl. Acad. Sci. U.S.A. 95, 13336–13341. doi: 10.1073/pnas.95.22.13336
Kumar, S., Stecher, G., Li, M., Knyaz, C., and Tamura, K. (2018). MEGA X: molecular evolutionary genetics analysis across computing platforms. Mol. Biol. Evol. 35, 1547–1549. doi: 10.1093/molbev/msy096
Kunze, M. (2020). The type-2 peroxisomal targeting signal. Biochim. Biophys. Acta Mol. Cell Res. 1867:118609.
Kunze, M., and Berger, J. (2015). The similarity between N-terminal targeting signals for protein import into different organelles and its evolutionary relevance. Front. Physiol. 6:259. doi: 10.3389/fphys.2015.00259
Kunze, M., Neuberger, G., Maurer-Stroh, S., Ma, J., Eck, T., Braverman, N., et al. (2011). Structural requirements for interaction of peroxisomal targeting signal 2 and its receptor PEX7. J. Biol. Chem. 286, 45048–45062. doi: 10.1074/jbc.m111.301853
Lamberto, I., Percudani, R., Gatti, R., Folli, C., and Petrucco, S. (2010). Conserved alternative splicing of Arabidopsis transthyretin-like determines protein localization and S-allantoin synthesis in peroxisomes. Plant Cell 22, 1564–1574. doi: 10.1105/tpc.109.070102
Lauersen, K. J., Willamme, R., Coosemans, N., Joris, M., Kruse, O., and Remacle, C. (2016). Peroxisomal microbodies are at the crossroads of acetate assimilation in the green microalga Chlamydomonas reinhardtii. Algal Res. 16, 266–274. doi: 10.1016/j.algal.2016.03.026
Lingner, T., Kataya, A. R., Antonicelli, G. E., Benichou, A., Nilssen, K., Chen, X. Y., et al. (2011). Identification of novel plant peroxisomal targeting signals by a combination of machine learning methods and in vivo subcellular targeting analyses. Plant Cell 23, 1556–1572. doi: 10.1105/tpc.111.084095
Ludewig-Klingner, A. K., Michael, V., Jarek, M., Brinkmann, H., and Petersen, J. (2018). Distribution and evolution of peroxisomes in alveolates (apicomplexa, dinoflagellates, ciliates). Genome Biol. Evol. 10, 1–13. doi: 10.1093/gbe/evx250
Ma, C., Haslbeck, M., Babujee, L., Jahn, O., and Reumann, S. (2006). Identification and characterization of a stress-inducible and a constitutive small heat-shock protein targeted to the matrix of plant peroxisomes. Plant Physiol. 141, 47–60. doi: 10.1104/pp.105.073841
Ma, X. N., Chen, T. P., Yang, B., Liu, J., and Chen, F. (2016). Lipid production from Nannochloropsis. Mar. Drugs 14, 1–18.
Matsuzaki, M., Misumi, O., Shin, I. T., Maruyama, S., Takahara, M., Miyagishima, S. Y., et al. (2004). Genome sequence of the ultrasmall unicellular red alga Cyanidioschyzon merolae 10D. Nature 428, 653–657.
Mbekeani, A. J., Stanley, W. A., Kalel, V. C., Dahan, N., Zalckvar, E., Sheiner, L., et al. (2018). Functional analyses of a putative, membrane-bound, peroxisomal protein import mechanism from the apicomplexan protozoan Toxoplasma gondii. Genes 9:434. doi: 10.3390/genes9090434
McFadden, G. I. (2001). Primary and secondary endosymbiosis and the origin of plastids. J. Phycol. 37, 951–959. doi: 10.1046/j.1529-8817.2001.01126.x
McGinnis, S., and Madden, T. L. (2004). BLAST: at the core of a powerful and diverse set of sequence analysis tools. Nucleic Acids Res. 32, W20–W25.
Mix, A. K., Cenci, U., Heimerl, T., Marter, P., Wirkner, M. L., and Moog, D. (2018). Identification and localization of peroxisomal biogenesis proteins indicates the presence of peroxisomes in the cryptophyte Guillardia theta and other “chromalveolates”. Genome Biol. Evol. 10, 2834–2852.
Moog, D., Przyborski, J. M., and Maier, U. G. (2017). Genomic and proteomic evidence for the presence of a peroxisome in the apicomplexan parasite Toxoplasma gondii and other coccidia. Genome Biol. Evol. 9, 3108–3121. doi: 10.1093/gbe/evx231
Motley, A. M., Hettema, E. H., Ketting, R., Plasterk, R., and Tabak, H. F. (2000). Caenorhabditis elegans has a single pathway to target matrix proteins to peroxisomes. EMBO Rep. 1, 40–46. doi: 10.1093/embo-reports/kvd010
Neer, E. J., Schmidt, C. J., Nambudripad, R., and Smith, T. F. (1994). The ancient regulatory-protein family of WD-repeat proteins. Nature 371, 297–300. doi: 10.1038/371297a0
Osumi, T., Tsukamoto, T., and Hata, S. (1992). Signal peptide for peroxisomal targeting: replacement of an essential histidine residue by certain amino acids converts the amino-terminal presequence of peroxisomal 3-ketoacyl-CoA thiolase to a mitochondrial signal peptide. Biochem. Biophys. Res. Commun. 186, 811–818. doi: 10.1016/0006-291x(92)90818-6
Pan, R., Liu, J., Wang, S., and Hu, J. (2020). Peroxisomes: versatile organelles with diverse roles in plants. New Phytol. 225, 1410–1427. doi: 10.1111/nph.16134
Papadopoulos, J. S., and Agarwala, R. (2007). COBALT: constraint-based alignment tool for multiple protein sequences. Bioinformatics 23, 1073–1079. doi: 10.1093/bioinformatics/btm076
Peraza-Reyes, L., Espagne, E., Arnaise, S., and Berteaux-Lecellier, V. (2010).“Peroxisomes in filamentous fungi,” in Cellular and Molecular Biology of Filamentous Fungi, ed. K. A. Borkovich and D. J. Ebbole (Washington, DC: American Society of Microbiology), 191–206. Available online at: http://doi.org/10.1128/9781555816636.ch15
Pessoa, J., Sarkany, Z., Ferreira-Da-Silva, F., Martins, S., Almeida, M. R., Li, J., et al. (2010). Functional characterization of Arabidopsis thaliana transthyretin-like protein. BMC Plant Biol. 10:30. doi: 10.1186/1471-2229-10-30
Petriv, O. I., Tang, L., Titorenko, V. I., and Rachubinski, R. A. (2004). A new definition for the consensus sequence of the peroxisome targeting signal type 2. J. Mol. Biol. 341, 119–134. doi: 10.1016/j.jmb.2004.05.064
Poliner, E., Pulman, J. A., Zienkiewicz, K., Childs, K., Benning, C., and Farre, E. M. (2018). A toolkit for Nannochloropsis oceanica CCMP1779 enables gene stacking and genetic engineering of the eicosapentaenoic acid pathway for enhanced long-chain polyunsaturated fatty acid production. Plant Biotechnol. J. 16, 298–309. doi: 10.1111/pbi.12772
Qiu, H., Yoon, H. S., and Bhattacharya, D. (2013). Algal endosymbionts as vectors of horizontal gene transfer in photosynthetic eukaryotes. Front. Plant Sci. 4:366. doi: 10.3389/fpls.2013.00366
Quan, S., Switzenberg, R., Reumann, S., and Hu, J. (2010). In vivo subcellular targeting analysis validates a novel peroxisome targeting signal type 2 and the peroxisomal localization of two proteins with putative functions in defense in Arabidopsis. Plant Signal. Behav. 5, 151–153. doi: 10.4161/psb.5.2.10412
Radakovits, R., Jinkerson, R. E., Fuerstenberg, S. I., Tae, H., Settlage, R. E., Boore, J. L., et al. (2012). Draft genome sequence and genetic transformation of the oleaginous alga Nannochloropis gaditana. Nat. Commun. 3:686.
Ramon, N. M., and Bartel, B. (2010). Interdependence of the peroxisome-targeting receptors in Arabidopsis thaliana: PEX7 facilitates PEX5 accumulation and import of PTS1 cargo into peroxisomes. Mol. Biol. Cell 21, 1263–1271. doi: 10.1091/mbc.e09-08-0672
Rathod, J. P., Gade, R. M., Rathod, D. R., and Dudhare, M. (2017). A review on molecular tools of microalgal genetic transformation and their application for overexpression of different genes. Int. J. Curr. Microbiol. App. Sci. 6, 3191–3207. doi: 10.20546/ijcmas.2017.612.373
Reumann, S. (2011). Toward a definition of the complete proteome of plant peroxisomes: Where experimental proteomics must be complemented by bioinformatics. Proteomics 11, 1764–1779. doi: 10.1002/pmic.201000681
Reumann, S. (2013). “Biosynthesis of vitamin K1 (phylloquinone) by plant peroxisomes and its integration into signaling molecule synthesis pathways,” in Peroxisomes and their Key Role in Cellular Signaling and Metabolism. Subcellular Biochemistry, Vol. 69, ed. L. del Río (Dordrecht: Springer), 213–229. doi: 10.1007/978-94-007-6889-5_12
Reumann, S., Babujee, L., Ma, C., Wienkoop, S., Siemsen, T., Antonicelli, G. E., et al. (2007). Proteome analysis of Arabidopsis leaf peroxisomes reveals novel targeting peptides, metabolic pathways, and defense mechanisms. Plant Cell 19, 3170–3193. doi: 10.1105/tpc.107.050989
Reumann, S., and Bartel, B. (2016). Plant peroxisomes: recent discoveries in functional complexity, organelle homeostasis, and morphological dynamics. Curr. Opin. Plant Biol. 34, 17–26. doi: 10.1016/j.pbi.2016.07.008
Reumann, S., Buchwald, D., and Lingner, T. (2012). PredPlantPTS1: a web server for the prediction of plant peroxisomal proteins. Front. Plant Sci. 3:194. doi: 10.3389/fpls.2012.00194
Reumann, S., and Chowdhary, G. (2018). “Prediction of peroxisomal matrix proteins in plants,” in Proteomics of Peroxisomes. Subcellular Biochemistry, Vol. 89,, ed. L. del Río (Singapore: Springer), 125–138. doi: 10.1007/978-981-13-2233-4_5
Reumann, S., Chowdhary, G., and Lingner, T. (2016). Characterization, prediction and evolution of plant peroxisomal targeting signals type 1 (PTS1s). Biochim. Biophys. Acta 1863, 790–803. doi: 10.1016/j.bbamcr.2016.01.001
Reumann, S., Ma, C., Lemke, S., and Babujee, L. (2004). AraPerox. A database of putative Arabidopsis proteins from plant peroxisomes. Plant Physiol. 136, 2587–2608. doi: 10.1104/pp.104.043695
Reumann, S., Quan, S., Aung, K., Yang, P., Manandhar-Shrestha, K., Holbrook, D., et al. (2009). In-depth proteome analysis of Arabidopsis leaf peroxisomes combined with in vivo subcellular targeting verification indicates novel metabolic and regulatory functions of peroxisomes. Plant Physiol. 150, 125–143. doi: 10.1104/pp.109.137703
Rodolfi, L., Chini Zittelli, G., Bassi, N., Padovani, G., Biondi, N., Bonini, G., et al. (2009). Microalgae for oil: strain selection, induction of lipid synthesis and outdoor mass cultivation in a low-cost photobioreactor. Biotechnol. Bioeng. 102, 100–112. doi: 10.1002/bit.22033
Ronquist, F., Teslenko, M., Van Der Mark, P., Ayres, D. L., Darling, A., Hohna, S., et al. (2012). MrBayes 3.2: efficient Bayesian phylogenetic inference and model choice across a large model space. Syst. Biol. 61, 539–542. doi: 10.1093/sysbio/sys029
Schluter, A., Fourcade, S., Ripp, R., Mandel, J. L., Poch, O., and Pujol, A. (2006). The evolutionary origin of peroxisomes: an ER-peroxisome connection. Mol. Biol. Evol. 23, 838–845. doi: 10.1093/molbev/msj103
Schuhmann, H., Huesgen, P. F., Gietl, C., and Adamska, I. (2008). The DEG15 serine protease cleaves peroxisomal targeting signal 2-containing proteins in Arabidopsis. Plant Physiol. 148, 1847–1856. doi: 10.1104/pp.108.125377
Shinozaki, A., Sato, N., and Hayashi, Y. (2009). Peroxisomal targeting signals in green algae. Protoplasma 235, 57–66. doi: 10.1007/s00709-009-0031-1
Sprecher, H. (2000). Metabolism of highly unsaturated n-3 and n-6 fatty acids. Biochim. Biophys. Acta 1486, 219–231.
Swinkels, B. W., Gould, S. J., Bodnar, A. G., Rachubinski, R. A., and Subramani, S. (1992). A novel, cleavable peroxisomal targeting signal at the amino-terminus of the rat 3-ketoacyl-coA thiolase. EMBO J. 10, 3255–3265. doi: 10.1002/j.1460-2075.1991.tb04889.x
Tolbert, N. E., and Essner, E. (1981). Microbodies: peroxisomes and glyoxysomes. J. Cell Biol. 91, 271s–283s.
Vieler, A., Wu, G., Tsai, C. H., Bullard, B., Cornish, A. J., Harvey, C., et al. (2012). Genome, functional gene annotation, and nuclear transformation of the heterokont oleaginous alga Nannochloropsis oceanica CCMP1779. PLoS Genet. 8:e1003064. doi: 10.1371/journal.pgen.1003064
Wanders, R. J., and Waterham, H. R. (2006). Biochemistry of mammalian peroxisomes revisited. Annu. Rev. Biochem. 75, 295–332. doi: 10.1146/annurev.biochem.74.082803.133329
Wang, D., Ning, K., Li, J., Hu, J., Han, D., Wang, H., et al. (2014). Nannochloropsis genomes reveal evolution of microalgal oleaginous traits. PLoS Genet. 10:e1004094. doi: 10.1371/journal.pgen.1004094
Woodward, A. W., and Bartel, B. (2005). The Arabidopsis peroxisomal targeting signal type 2 receptor PEX7 is necessary for peroxisome function and dependent on PEX5. Mol. Biol. Cell 16, 573–583. doi: 10.1091/mbc.e04-05-0422
Yoo, S. D., Cho, Y. H., and Sheen, J. (2007). Arabidopsis mesophyll protoplasts: a versatile cell system for transient gene expression analysis. Nat. Protoc. 2, 1565–1572. doi: 10.1038/nprot.2007.199
Yoon, H. S., Hackett, J. D., Pinto, G., and Bhattacharya, D. (2002). The single, ancient origin of chromist plastids. Proc. Natl. Acad. Sci. U.S.A. 99, 15507–15512. doi: 10.1073/pnas.242379899
Keywords: peroxisome biogenesis, microalgae (unicellular eukaryotic algae), evolution, malate synthase, thiolase, PTS2 protein import pathway
Citation: Kechasov D, de Grahl I, Endries P and Reumann S (2020) Evolutionary Maintenance of the PTS2 Protein Import Pathway in the Stramenopile Alga Nannochloropsis. Front. Cell Dev. Biol. 8:593922. doi: 10.3389/fcell.2020.593922
Received: 11 August 2020; Accepted: 13 October 2020;
Published: 19 November 2020.
Edited by:
Maya Schuldiner, Weizmann Institute of Science, IsraelReviewed by:
Richard Rachubinski, University of Alberta, CanadaMarkus Kunze, Medical University of Vienna, Austria
Marek Skoneczny, Institute of Biochemistry and Biophysics (PAN), Poland
Copyright © 2020 Kechasov, de Grahl, Endries and Reumann. This is an open-access article distributed under the terms of the Creative Commons Attribution License (CC BY). The use, distribution or reproduction in other forums is permitted, provided the original author(s) and the copyright owner(s) are credited and that the original publication in this journal is cited, in accordance with accepted academic practice. No use, distribution or reproduction is permitted which does not comply with these terms.
*Correspondence: Sigrun Reumann, c2lncnVuLnJldW1hbm5AdW5pLWhhbWJ1cmcuZGU=
†Present address: Dmitry Kechasov, Norwegian Institute of Bioeconomy Research, Klepp Stasjon, Norway