Role of Non-coding RNAs on the Radiotherapy Sensitivity and Resistance of Head and Neck Cancer: From Basic Research to Clinical Application
- Department of Otolaryngology Head and Neck Surgery, Shengjing Hospital of China Medical University, Shenyang, China
Head and neck cancers (HNCs) rank as the sixth common and the seventh leading cause of cancer-related death worldwide, with an estimated incidence of 600,000 cases and 40–50% mortality rate every year. Radiotherapy is a common local therapeutic modality for HNC mainly through the function of ionizing radiation, with approximately 60% of patients treated with radiotherapy or chemoradiotherapy. Although radiotherapy is more advanced and widely used in clinical practice, the 5-year overall survival rates of locally advanced HNCs are still less than 40%. HNC cell resistance to radiotherapy remains one of the major challenges to improve the overall survival in HNC patients. Non-coding RNAs (ncRNAs) are newly discovered functional small RNA molecules that are different from messenger RNAs, which can be translated into a protein. Many previous studies have reported the dysregulation and function of ncRNAs in HNC. Importantly, researchers reported that several ncRNAs were also dysregulated in radiotherapy-sensitive or radiotherapy-resistant HNC tissues compared with the normal cancer tissues. They found that ectopically elevating or knocking down expression of some ncRNAs could significantly influence the response of HNC cancer cells to radiotherapy, indicating that ncRNAs could regulate the sensitivity of cancer cells to radiotherapy. The implying mechanism for ncRNAs in regulating radiotherapy sensitivity may be due to its roles on affecting DNA damage sensation, inducing cell cycle arrest, regulating DNA damage repair, modulating cell apoptosis, etc. Additionally, clinical studies reported that in situ ncRNA expression in HNC tissues may predict the response of radiotherapy, and circulating ncRNA from body liquid serves as minimally invasive therapy-responsive and prognostic biomarkers in HNC. In this review, we aimed to summarize the current function and mechanism of ncRNAs in regulating the sensitivity of HNC cancer cells to radiotherapy and comprehensively described the state of the art on the role of ncRNAs in the prognosis prediction, therapy monitoring, and prediction of response to radiotherapy in HNC.
Introduction
Head and neck cancers (HNCs) rank as the sixth common and the seventh leading cause of cancer-related death worldwide, with an estimated incidence of 600,000 cases and 40–50% mortality rate every year (Ferlay et al., 2015; Economopoulou et al., 2016). HNCs are presented mainly by the squamous cell carcinoma originating from the epithelial cells of the oral cavity, nasal cavity, oropharynx, larynx, or hypopharynx (Leemans et al., 2018).
Radiotherapy is a common local therapeutic modality for cancers mainly through the function of ionizing radiation. Ionizing radiation could kill cancer cells via directly damaging the DNA strands of cancer cells to end the infinite proliferative capacity, and also indirectly causes DNA damages in cancer cell through ionizing water to generating highly reactive oxygen species. Radiotherapy is one of the major treatment modalities for HNC, with approximately 60% of patients treated with radiotherapy or chemoradiotherapy (Berrington de Gonzalez et al., 2011). Many high-quality clinical studies have reported that radiotherapy or concurrent chemoradiotherapy could improve the survival for patients with early-stage HNC or locally advanced HNC (Bonner et al., 2010; Caudell et al., 2017; Lacas et al., 2017; Nichols et al., 2019). Although radiotherapy is more advanced and widely used in clinical practice, 5-year overall survival rates of locally advanced HNC are still less than 40% (Pignon et al., 2009). Cancer cell resistance to radiotherapy remains one of the major challenges to improve the overall survival in HNC patients.
Non-coding RNAs (ncRNAs) are newly discovered functional RNA molecules that are different from messenger RNAs, which can be translated into a protein (Mattick and Makunin, 2006; Feng et al., 2019; Dai et al., 2020; Yu et al., 2020). Over the past decades, researchers have discovered multiple kinds of ncRNAs, showing that ncRNAs constitute more than 90% of RNAs transcribed from the human genome DNA (Slack, 2006). Such discovery of numerous ncRNAs has opened up brand-new directions for understanding the normal physiology and the development of diseases. Based on the function difference, ncRNAs are usually divided into two categories: housekeeping and regulatory ncRNAs. The regulatory ncRNAs are mainly composed of miRNAs, circRNAs, and long non-coding RNAs (lncRNA; Chen, 2016; Wu et al., 2017; Yao et al., 2019). In recent years, regulatory RNAs were extensively researched and are revealed to participate in regulating the expression of protein-coding genes at transcriptional, post-transcriptional, as well as translational levels. Emerging studies have reported the key roles of regulatory ncRNAs in various biological processes, disease occurrence, and development.
Many previous studies have reported the dysregulation and function of ncRNAs in HNC (Wang et al., 2018; Jiang et al., 2019; Vo et al., 2019). NcRNAs could regulate the expression of genes associated with cell cycle, cell apoptosis, invasion, and migration, and eventually affect the proliferation, invasion, and metastasis of HNC (Feng et al., 2020; Gu et al., 2020; Huang et al., 2020; Sur et al., 2020; Wang et al., 2020; Zhang et al., 2020). Additionally, researchers reported that several ncRNAs were also dysregulated in radiotherapy-sensitive or radiotherapy-resistant HNC tissues compared with the normal cancer tissues (Zhang et al., 2013; de Jong et al., 2015; Qu et al., 2015a,b; Suh et al., 2015; Xu et al., 2015; Gao et al., 2017a; Hess et al., 2017; Chen et al., 2019; Vahabi et al., 2019; Pasi et al., 2020). They found that ectopically elevating or knocking down expression of some ncRNA could significantly influence the response of HNC cancer cells to radiotherapy, indicating that ncRNAs could regulate the sensitivity of cancer cells to radiotherapy. In this review, we aimed to summarize the current function and mechanism of ncRNAs in regulating the sensitivity of HNC cancer cells to radiotherapy.
Radiation-Induced Cell Response and Mechanism of Radiotherapy Resistance
Since the discovery of ionizing radiation in 1895, the concept of radiation-based therapy modality was prompted and has been regarded as a major treatment for many types of cancers (Wills, 1904; Alsahafi et al., 2020; Ampil et al., 2020; Plavc et al., 2020; Tao et al., 2020; Sher et al., 2020a, b). Especially, radiotherapy is a crucial treatment for HNC. The radiation-induced cell death is regarded to originate from the damage of two cellular components: DNA and cell membrane.
Upon exposure to radiation, the radiation passed directly through the cell and ionized the DNA, causing lethal DNA damage. Additionally, the radiation can also result in DNA damage through ionizing the intracellular water and inducing the generation of reactive oxygen species (Rothkamm and Löbrich, 2003). These reactive oxygen species could bring various injuries to cells, among which are the DNA double-strand breaks (DSBs), which are one of the most cytotoxic injuries to cells. DSBs, involving breaks in the phosphodiester backbone of both strands of DNA, increase positively with the radiation dose (Hanai et al., 1998; Rothkamm and Löbrich, 2003). It is estimated that each gray unit of radiation could produce 105 ionizations per cell, which cause about 40 DSBs, 2,000 single-strand breaks (SSBs), as well as other types of damages in the DNA (Lewanski and Gullick, 2001). When recognizing these complex radiation-induced damages, the cells respond to these damages through multiple signaling pathways. These pathways are involved in modulating important cellular activities such as DNA damage repair, cell-cycle arrest, and apoptosis. In addition, radiation could also function on the cell membrane to mediate cell apoptosis. Mechanistically, radiation activates the enzyme sphingomyelinase, which could hydrolyze plasma-derived sphingomyelin and produce ceramide. Through this process, ceramide could be produced within seconds of radiation exposure. The accumulation of ceramide in cells could initiate cell apoptosis (Obeid et al., 1993; Haimovitz-Friedman et al., 1994; Jarvis et al., 1994a, b). However, the mechanism on how ceramide initiates apoptosis is still unclear.
In response to radiation exposure, most cells immediately initiate cell apoptosis because of severe DNA damage or the accumulation of ceramide in cells. Such programmed death mechanism could protect cells from propagating genetic mutations to the next generation. Additionally, cells will also initiate the DNA damage repair system, which was developed during the evolution of species. There are two major DNA repair pathways: homologous recombination (HR) and non-homologous end joining (NHEJ; Burgess et al., 2020; Nastasi et al., 2020; Zhao et al., 2020).
The HR repairing system depends on homologous DNA sequences from sister chromatids. This makes HR restricted to phases of the cell cycle where homologous sister chromatids coexist (Zhao et al., 2017). In contrast, NHEJ is a promiscuous repair system that directly ligates two broken ends independent of sequence homology. Hence, NHEJ is not cell cycle dependent and could be initiated at any cell cycle phase (Emerson and Bertuch, 2016). Another important response to DNA damage is cell cycle arrest. Normally, cell cycles pass through the G0 phase, G1 phase, S phase, G2 phase, and M phase. The cell cycle checkpoint pathway regulated the progression of the cell cycle. When sensing DNA damages, cells will stop the cell cycle progression to spare more time for DNA repairing. Overall, these complex cell responses to radiation-induced injury depend on the modulation of various genes.
The sensitivity of cancer cells to radiotherapy depends on the intensity of DNA damage with cells, the cells’ ability to balance the expression of genes associated with apoptosis promotion and inhibition, the expression level of genes regulating the induction of cell cycle arrest, and the DNA repair system. Radiotherapy-resistant cancer cells showed obvious tendency to inhibit cell apoptosis and augment the DNA damage repair rate. This adaptation to radiotherapy is closely related with the dysregulated expression of genes in resistant cells. NcRNAs, especially the regulatory ncRNAs, are characterized for their role on regulating gene expression in cancer cells. Because of such an important role, ncRNAs are believed to be associated with radiotherapy resistance and could regulate cell sensitivity to radiotherapy.
Ionizing Radiation Modulates Ncrna Expression Profiling
The role of ncRNAs on cancer development and progression is one of the hottest topics in cancer cell biology research. With the help of microarray and next-generation technology, researchers have discovered an amount of ncRNAs, such as miRNAs, lncRNAs, and circRNAs, which was differently expressed in cancer tissues compared with the normal tissues. Many of these ncRNAs could significantly regulate the biological behaviors of cancer cell. In HNC, many researchers also focus on the expression and function of ncRNAs. Numerous ncRNAs are reported to modulate HNC proliferation, invasion, metastasis, apoptosis, and other biological processes (Wu et al., 2015; Bao et al., 2018; Vo et al., 2019; Wang S. S. et al., 2019). Recently, researchers also found that these oncogenic or tumor-suppressive ncRNAs could also regulate the sensitivity of cancer cells to radiotherapy. Moreover, they also explore dysregulated ncRNAs expression through comparing radiotherapy-resistant, radiotherapy-sensitive, and normal cancer tissues or cells, so as to discover key ncRNAs that could regulate radiotherapy sensitivity. We summarized the differently expressed ncRNAs in radiotherapy-resistant, radiotherapy-sensitive, and normal cancer tissues or cells as shown in Table 1 (Qu et al., 2012, 2015a, b; Li et al., 2013; Li B. Y. et al., 2017; Li L. N. et al., 2017; Li et al., 2020; Shiiba et al., 2013; Zhang et al., 2013; Wang et al., 2014, 2016, Wang Z. et al., 2019; de Jong et al., 2015; Lin et al., 2015; Maia et al., 2015; Suh et al., 2015; Sun et al., 2015; Xu et al., 2015; Zhao et al., 2015; Huang et al., 2016, 2018, 2019; Kang et al., 2016; Weng et al., 2016; Wu et al., 2016, 2018; Gao et al., 2017a, b; Han et al., 2017; Hu et al., 2017; Chen H. et al., 2018; Chen et al., 2019; Feng et al., 2018; He et al., 2018; Yang et al., 2018; Kong et al., 2019; Tian et al., 2019; Vahabi et al., 2019; Yi et al., 2019; Gou et al., 2020; Kangboonruang et al., 2020; Shuai and Huang, 2020).
ncRNAs Directly Regulate Radiotherapy Sensitivity by Modulating Specific Processes
The efficacy of radiotherapy depends mainly on the sensitivity of cancers to radiotherapy. Many factors are found to influence the radiotherapy sensitivity of cancers. Several research groups have discovered that some differentially expressed ncRNAs in HNC could affect radiotherapy sensitivity. Meanwhile, several research groups also discovered differentially expressed ncRNAs in radiation-sensitive and radiation-resistant HNC cancer cells by sequencing and microarray analysis. Some high-regulated ncRNAs in radiation-resistant cancers could decrease the sensitivity of cancer cells to radiotherapy, whereas ncRNAs down-regulated in radiation-resistant cancers could enhance the sensitivity of cancer cells to radiotherapy. These ncRNAs modulate the response of cancer cells to radiotherapy mainly through regulating different genes involved in important processes that are tightly associated with radiotherapy sensitivity. In this section, we mainly describe the effect and molecular mechanisms of ncRNA on regulating radiotherapy sensitivity of HNC cells by DNA damage sensing, cell cycle progression, DNA damage repair, and cell apoptosis of HNC cells.
Affecting DNA Damage Sensation and Inducing Cell Cycle Arrest
In response to DNA damage, cells have evolutionarily developed a mechanism to sense the DNA damage and initiate DNA damage repair. In the process, the ataxia telangiectasia mutated (ATM), an important Ser/Thr kinase, plays a major role in sensing DNA DSBs and initiating a series of cascade responses leading to cell cycle checkpoint activation and DNA repair (Weber and Ryan, 2015; Carrassa and Damia, 2017; Figures 1, 2). After sensing the radiation-induced DNA damage, the main sensor ATM and the ataxia telangiectasia mutated and Rad3 related (ATR) were activated and subsequently activated the cell cycle checkpoint kinases. Usually, ATM activates the checkpoint kinase 2 (CHK2), and ATR is responsible for the activation of CHK1. The activation of CHK1/2 could lead to phosphorylation and inactivation of CDC25A and CDC25C, both of which were involved in dephosphorylation and activation of CDK2 and CDK1, respectively. Inactivation of CDC25A and CDC25C consequently leads to maintenance of CDKs in the phosphorylated and inactivated form, thus inhibiting S phase and M phase entry and eventually inducing cell cycle arrest. Overall, ATM/CHK2 and ATR/CHK1 pathways work coordinately and cooperate to mediating cellular responses to DNA damage and are responsible for the maintenance of genomic stability by inducing cell cycle arrest. In HNC, the function of ATM was reported to be directly and indirectly regulated by several ncRNAs including miRNA and lncRNA. Mansour et al. (2013) reported that miR-421 could directly inhibit the expression of ATM in HNC through binding the ATM’ S 3’-untranslated region (UTR). Forced expression of this miRNA could result in a significant cellular ATM deficiency and defect DNA damage repair, and eventually promote the sensitivity of HNC cells to radiotherapy. LncRNA PVT1 was found to be overexpressed in HNC tissues and cell line, and knockdown of PVT1 enhances the radiosensitivity of nasopharyngeal carcinoma (NPC) cell lines (He et al., 2018). Mechanically, knockdown of PVT1 significantly decreased the phosphorylation levels of ATM, p53, and Chk2, causing a decrease in the DNA repair ability of NPC cells after radiotherapy and enhancing their radiosensitivity (He et al., 2018).
Regulating DNA Damage Repair
When DSBs, the most harmful type of DNA damage, occurred in cells, the histone variant H2AX was immediately phosphorylated by the activated ATM (Jackson, 2002; Symington and Gautier, 2011). Therefore, phosphorylated H2AX (γ-H2AX) can be a useful indicator for DSB DNA damage. More importantly, γ-H2AX is responsible for the recruitment of DNA repair associated proteins to initiate the NHEJ or HR repair processes. In NPC cancer, miR-24 has been revealed to directly target H2AX (Wang et al., 2014). It demonstrated that the inhibition of miR-24 results in significant up-regulation of H2AX and thereby renders cancer cells resistant to radiation-induced DNA damage (Wang et al., 2014). Meanwhile, researchers also reported that miR-24 can inhibit the DNA DSB repair by targeting a DNA damage repair associated protein c-Jun activation domain binding protein-1 (Jab1; Wang et al., 2016). Jab 1 was crucial for DSB repair, and deletion of Jab1 resulted in spontaneous DNA fragmentation and increased expression of the γ-H2AX level (Doronkin et al., 2002; Pan et al., 2012, 2013).
Modulation of Cell Apoptosis
When the radiation-induced DNA damage is too lethal to be repaired, cells would initiate an automatic death program like apoptosis to protect genome stability. Therefore, the flexibility of cancer cells to initiate cell apoptosis sometimes may determine whether cancer cells are sensitive to radiotherapy or resistant to it. Some ncRNAs have been reported to influence the sensitivity of cancer cells to radiotherapy through modulation of cell apoptosis. Mechanistically, these ncRNAs mainly function through regulating the expression of anti-apoptosis or pro-apoptosis members (Table 2).
Many ncRNAs could enhance HNC cancer cell sensitivity to radiotherapy through promoting cell apoptosis. MiR-29c was found to target the classical anti-apoptotic proteins B cell lymphoma 2 (Bcl-2) family including myeloid cell leukemia 1 (MCL1) and Bcl-2 itself in human NPC. In vitro and in vivo studies illustrated that miR-29c could promote cell apoptosis, through which ectopic restoration of miR-29C substantially enhanced the sensitivity of NPC cells to radiotherapy (Zhang et al., 2013). MiR-378g was reported to enhance radiosensitivity, promoting apoptosis in NPC cells via directly targeting SHP-1 (Lin et al., 2015). Overexpression of SHP-1 partially reversed the effect of miR-378g mimics on cell apoptosis and radiosensitivity. MiR-185-3p and miR-324-3p can inhibit NPC cell growth and promote apoptosis partly through targeting SMAD7 (Xu et al., 2015; Figure 3).
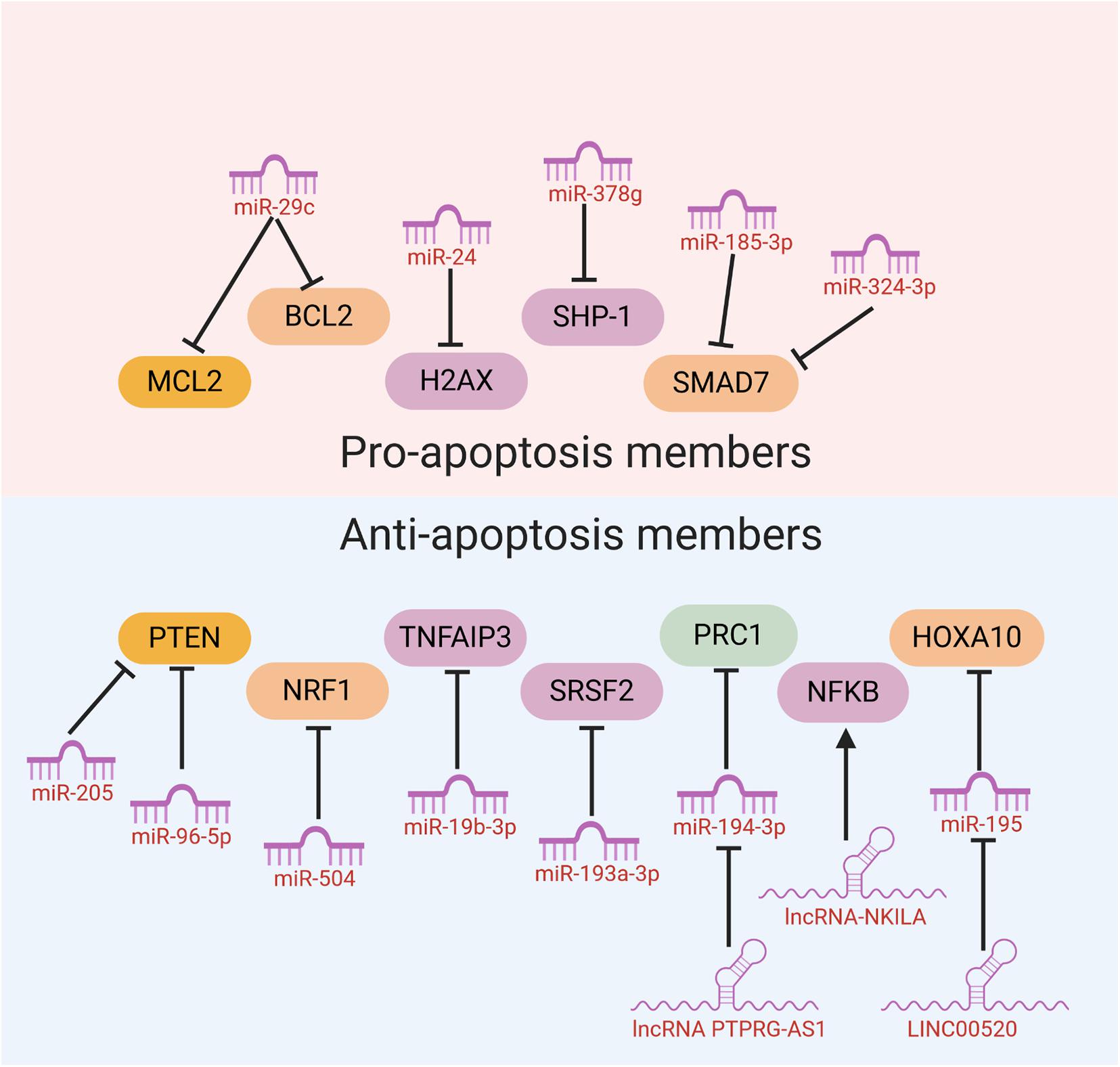
Figure 3. NcRNAs could affect HNC cancer cell sensitivity to radiotherapy through modulation of cell apoptosis.
On the other hand, several miRNAs were reported to inhibit cell apoptosis and enhance HNC cancer cell resistance to radiotherapy. Evidence has shown that PTEN is involved in regulating cell response to radiation-induced cell apoptosis and cell cycle arrest through inhibiting radiation-induced activation of the PI3K-Akt signaling pathway. Several miRNAs including miR-205 and miR-96-5p were reported to be elevated in HNC tissues from NPC patients after radiotherapy, and target PTEN to mediate resistance of HNC cells to radiotherapy (Qu et al., 2012; Vahabi et al., 2019). MiR-504 is found to be up-regulated in NPC radioresistant cells and could directly inhibit the expression of NRF1 and lead to radioresistance in NPC cells. NRF1 inhibition by miR-504 disturbed mitochondrial-mediated oxidative responses, which influence apoptosis of HNC cells and contribute to the resistance of cancer cells to radiation (Zhao et al., 2015). Huang et al. revealed that miR-19b-3p is up-regulated in NPC and could activate NF-κB activity by targeting TNFAIP3. This miR-19b-3p/TNFAIP3/NF-κB axis could eventually inhibit cancer cell apoptosis and lead to NPC cell radioresistance (Huang et al., 2016). In addition, miR-210, miR-193a-3p, and miR-17-5p were reported to inhibit HNC cell apoptosis and promote cancer cell resistance to radiotherapy (Wu et al., 2016; Li B. Y. et al., 2017; Kong et al., 2019; Figure 3).
Recently, evidences have discovered that several lncRNAs and circRNAs could influence cancer cell sensitivity to radiotherapy through modulation of cell apoptosis. These lncRNAs and circRNAs function mainly through alleviating the role of certain miRNAs, which could be bound by lncRNAs and circRNAs through the base-pairing principles. For example, lncRNA ANRIL could function as a miR-125a sponge and negatively modulate miR-125a expression. ANRIL could reverse the inhibited proliferation, induced apoptosis, and enhanced radiosensitivity triggered by miR-125a overexpression (Hu et al., 2017). PTPRG-AS1 was found to specifically bind to miR-194-3p as a competing endogenous RNA, and miR-194-3p negatively regulates PRC1. Silencing PTPRG-AS1 could release the expression of miR-194-3p and resulted in enhanced sensitivity to radiotherapy and cell apoptosis (Yi et al., 2019). The LINC00520 could promote cell resistance to radiotherapy through reversing miR-195/HOXA10 in HNC (Li et al., 2020). CircRNA_000543 could serve as a sponge for miR-9 in NPC. Silencing circRNA_000543 sensitizes NPC cells to radiation by targeting the miR-9/PDGFRB axis (Chen et al., 2019). Additionally, NF-κB interacting lncRNA (NKILA) has been reported to be down-regulated in laryngeal cancer and could enhance the cytotoxicity of radiation through promoting cell apoptosis. Mechanically, lncRNA NKILA functions through combining with NF-κB: IκB complex to inhibit IκB phosphorylation, inhibits p65 nuclear translocation, and finally inhibits NF-κB activation (Yang et al., 2018; Figure 3).
Activation of EGFR Signaling
Ionizing radiation activates the epidermal growth factor receptor (EGFR) family of receptor tyrosine kinases, which, in turn, can initiate PI3K/AKT or MAPK pathways.
Through activating the PI3K-AKT pathway, EGFR signaling can prevent radiation-induced apoptosis. EGFR signaling can also promote cancer cell growth by inducing cell cycle progression, which was driven by activation of the retrovirus associated DNA sequence (RAS)–rapidly accelerated fibrosarcoma (RAF)–mitogen/extracellular signal-regulated kinase (MEK)–ERK pathways. Published data demonstrate that miR-203 is a critical determinant of NPC cells’ response to radiotherapy, and reduced miR-203 could promote NPC cell radioresistance by activating IL8/AKT signaling (Qu et al., 2015b). In addition, PTEN is a common inhibitor of AKT and is also a direct target of several miRNAs in HNC, such as miR-205 and miR-96-5p (Figure 4).
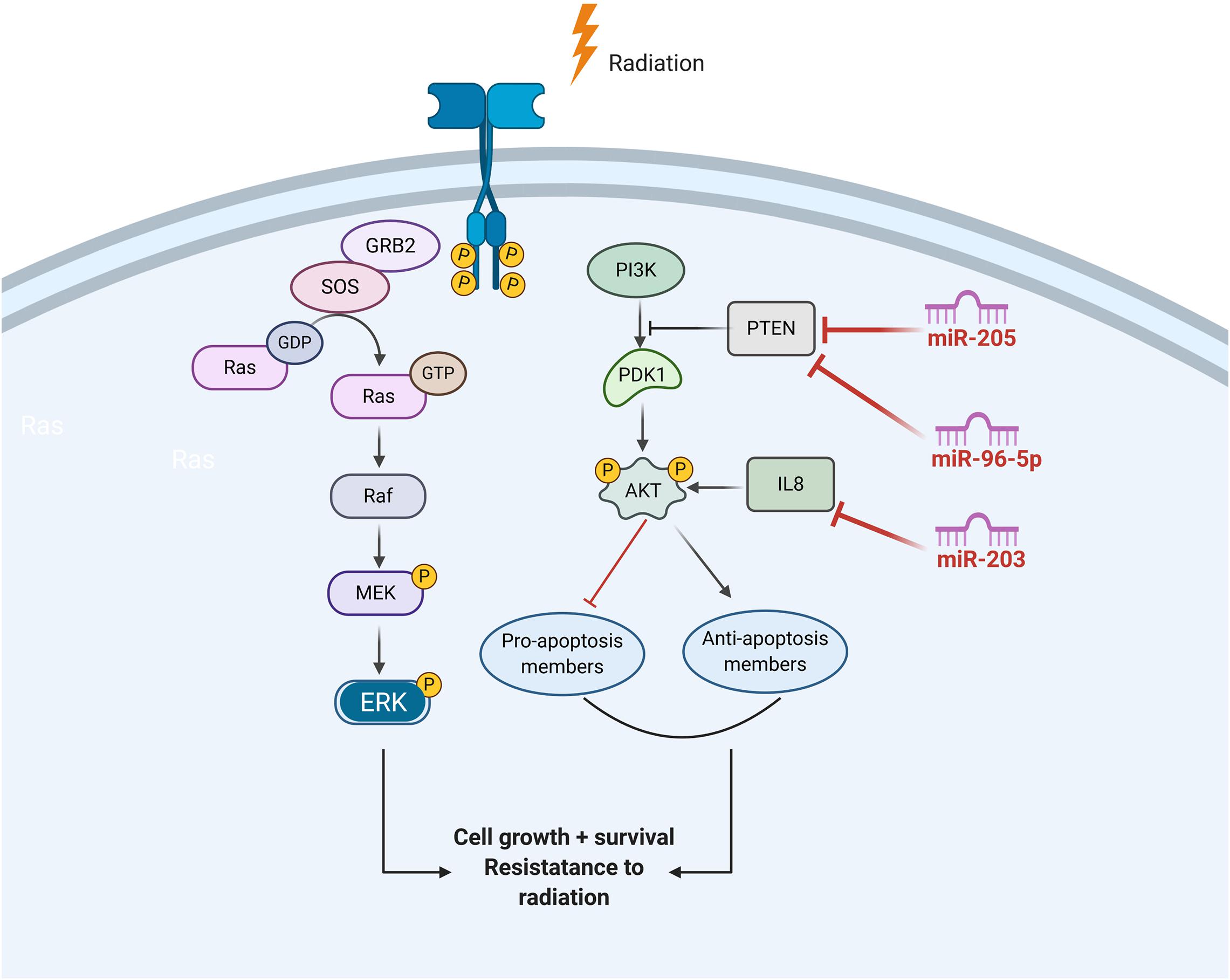
Figure 4. The role of ncRNAs on the regualtion of EGFR signalling in response to radiation induced DNA damage.
Epithelial-to-Mesenchymal Transition
Epithelial-to-mesenchymal transition (EMT) is a phenotypic change in which the epithelial cancer cell acquired a fibroblastoid-like morphology. Such transition could result in enhanced tumor cell motility and invasiveness, increased metastatic potential, as well as resistance to radiotherapy or chemotherapy (Marie-Egyptienne et al., 2013). ZEB1, an important EMT marker, could also be regulated by lncRNA NEAT1 in NPC. lncRNA NEAT1 knockdown could sensitize NPC cells to radiation through releasing the expression of miR-204, and eventually enhances the expression of ZEB1, a downstream target of miR-204.
Clinical Application of ncRNAs as Biomarkers to Radiotherapy
The in situ ncRNA Expression in HNC Tissues Predicting Response of Radiotherapy
It is well known that ncRNAs have shown an important regulatory role on the sensitivity or resistance of HNC cells to radiotherapy. Based on this, ncRNAs expression can be useful biomarkers to identify HNC patients who will be sensitive to radiotherapy and to predict the survival outcomes of HNC patients receiving radiotherapy in clinical practice. One study explored the associations between miR-200b and miR-155 expression in HNC tissues and outcome, and confirmed the prognosis predictive value of candidate miRNAs (Hess et al., 2017). Additionally, some researchers began to build a predictive panel consisting of multiple markers in an attempt to better improve the predictive value. Chen L. et al. (2018) analyzed a large scale of miRNA array profiles and the corresponding clinical records for HNSCC patients (including 509 carcinomas and 44 normal mucosa specimens) from the TCGA. They established a 5-miRNA signature including miR-99a, miR-31, miR-410, miR-424, and miR-495. Their results showed that this 5-miRNA signature could predict clinical outcomes, and the 5-miRNA signature-based nomogram is useful in predicting radiotherapy response and survival in HNSCC, implying that it might become a promising tool to optimize radiation strategies.
Circulating ncRNAs Serve as Minimally Invasive Therapy-Responsive and Prognostic Biomarkers
Circulating biomarkers in the peripheral blood, such as the biomarker HSP70 in HNSCC patients (Gehrmann et al., 2014), could provide a minimally invasive way to predict therapy response and survival outcomes, as well as monitor the therapy. NcRNAs, especially miRNAs, were reported to show high stability in blood plasma and resistance to RNase activity (Mitchell et al., 2008). This characteristic of miRNA combined with the minimally invasive accessibility of blood sample makes circulating miRNAs useful and attractive biomarkers. Nakashima et al. (2019) reported that the expression of miR-1290 was significantly down-regulated in the plasma of oral squamous cell carcinoma patients as compared to that in healthy volunteers. The patients who showed a poor pathological response to chemoradiotherapy presented a high proportion of miR-1290 down-regulation (Nakashima et al., 2019), suggesting that miR-1290 expression may be useful for guiding treatment decisions in oral squamous cell carcinoma patients receiving radiotherapy. Another research identified eight plasma miRNAs that differentiated significantly between HNC patients and the healthy donors. These candidate miRNAs also showed well therapy-response features and significantly decreased after receiving radiotherapy.
Conclusion and Prospective
Radiotherapy is a common therapeutic modality for HNC. Decreased sensitivity or resistance to radiotherapy is still a significant challenge in clinical practice and a barrier to improve the prognosis of HNC patients. With the development of molecular biology and sequencing technology, mounting evidence revealed the important role of ncRNAs on the carcinogenesis, development, and therapy resistance of HNC. These findings were of great significance for the following reasons. First, they highlight the potential of intervening relevant ncRNAs to overcome resistance and re-sensitize cancer cells to the effects of radiotherapy. Second, the ncRNAs could be used as circulating biomarkers in the peripheral blood to predict therapy response and survival outcomes, as well as monitor the therapy.
In the future, more studies are required to further elucidate the potential roles of novel ncRNAs like circRNAs and piRNAs in regulating the sensitivity of HNC cells to radiotherapy. Furthermore, it is still unclear and less studied whether the tumor cells or tumor mesenchymal cells-derived exosomal ncRNAs confer HNC cell resistance characteristics to radiotherapy. These topics will be very interesting and meaningful because circRNAs were more stable than linear RNAs due to their covalently closed loop structures, and exosome encapsulated ncRNAs were less easy to be degraded in the circulation system. These characteristics make circRNAs and exosomal ncRNAs potential biomarker candidates for prognosis prediction and therapy response monitoring. Additionally, with the development of bioinformatics, the integration of ncRNA signature profiling into HNC screening algorithms may help in increasing the specificity of screening patients who will benefit from radiotherapy and improving the prognosis of HNC patients.
Author Contributions
JY designed the review. XZ and JY collected the related manuscript. XZ and JY drafted and revised the manuscript. Both authors read and approved the final manuscript.
Funding
This study was supported by the 345 Talent project from the Shengjing Hospital of China Medical University, the Natural Science Foundation of Liaoning Province (2014021059).
Conflict of Interest
The authors declare that the research was conducted in the absence of any commercial or financial relationships that could be construed as a potential conflict of interest.
Acknowledgments
We would like to acknowledge that figures were created using the © BioRender-biorender.com platform. We would also like to thank members from the Department of Otolaryngology Head and Neck Surgery, Shengjing Hospital of China Medical University for their help on our manuscript.
Supplementary Material
The Supplementary Material for this article can be found online at: https://www.frontiersin.org/articles/10.3389/fcell.2020.637435/full#supplementary-material
Abbreviations
ATM, ataxia telangiectasia mutated; ATR, the ataxia telangiectasia mutated and Rad3 related; Bcl-2, B cell lymphoma 2; CHK2, checkpoint kinase 2; DSBs, DNA double-strand breaks; EGFR, epidermal growth factor receptor; EMT, epithelial-to-mesenchymal transition; γ-H 2AX, phosphorylated H2AX; HNCs, head and neck cancers; HR, homologous recombination; Jab1, c-Jun activation domain binding protein-1; lncRNA, long non-coding RNAs; MCL1, myeloid cell leukemia 1; ncRNAs, non-coding RNAs; NHEJ, non-homologous end joining; NKILA, NF- κ B interacting lncRNA; NPC, nasopharyngeal carcinoma; SSBs, single-strand breaks; UTR, untranslated region.
References
Alsahafi, E. N., Thavaraj, S., Sarvestani, N., Novoplansky, O., Elkabets, M., Ayaz, B., et al. (2020). EGFR overexpression increases radiotherapy response in HPV-positive head and neck cancer through inhibition of DNA damage repair and HPV E6 downregulation. Cancer Lett. 498, 80–97.
Ampil, F. L., Nathan, C. A., and Asarkar, A. (2020). Long-term survival in patients with intermediate-risk head and neck cancer treated with adjuvant radiotherapy. Oral Oncol. doi: 10.1016/j.oraloncology.2020.105071 [Epub ahead of print].
Bao, L., You, B., Shi, S., Shan, Y., Zhang, Q., Yue, H., et al. (2018). Metastasis-associated miR-23a from nasopharyngeal carcinoma-derived exosomes mediates angiogenesis by repressing a novel target gene TSGA10. Oncogene 37, 2873–2889. doi: 10.1038/s41388-018-0183-186
Berrington de Gonzalez, A., Curtis, R. E., Kry, S. F., Gilbert, E., Lamart, S., et al. (2011). Proportion of second cancers attributable to radiotherapy treatment in adults: a cohort study in the US SEER cancer registries. Lancet Oncol. 12, 353–360. doi: 10.1016/s1470-2045(11)70061-70064
Bonner, J. A., Harari, P. M., Giralt, J., Cohen, R. B., Jones, C. U., Sur, R. K., et al. (2010). Radiotherapy plus cetuximab for locoregionally advanced head and neck cancer: 5-year survival data from a phase 3 randomised trial, and relation between cetuximab-induced rash and survival. Lancet Oncol. 11, 21–28. doi: 10.1016/s1470-2045(09)70311-70310
Burgess, J. T., Rose, M., Boucher, D., Plowman, J., Molloy, C., Fisher, M., et al. (2020). The therapeutic potential of DNA Damage repair pathways and genomic stability in lung Cancer. Front. Oncol. 10:1256. doi: 10.3389/fonc.2020.01256
Carrassa, L., and Damia, G. (2017). DNA damage response inhibitors: mechanisms and potential applications in cancer therapy. Cancer Treat Rev. 60, 139–151. doi: 10.1016/j.ctrv.2017.08.013
Caudell, J. J., Torres-Roca, J. F., Gillies, R. J., Enderling, H., Kim, S., Rishi, A., et al. (2017). The future of personalised radiotherapy for head and neck cancer. Lancet Oncol. 18, e266–e273. doi: 10.1016/s1470-2045(17)30252-30258
Chen, H., Jin, L., Zhou, L., and Huang, J. M. (2018). Overexpressed miR-128a enhances chemoradiotherapy to laryngeal cancer cells and its correlation with BMI1. Future Oncol. 14, 611–620. doi: 10.2217/fon-2017-2542
Chen, L., Wen, Y., Zhang, J., Sun, W., Lui, V. W. Y., Wei, Y., et al. (2018). Prediction of radiotherapy response with a 5-microRNA signature-based nomogram in head and neck squamous cell carcinoma. Cancer Med. 7, 726–735. doi: 10.1002/cam4.1369
Chen, L., Zhou, H., and Guan, Z. (2019). CircRNA_000543 knockdown sensitizes nasopharyngeal carcinoma to irradiation by targeting miR-9/platelet-derived growth factor receptor B axis. Biochem. Biophys. Res. Commun. 512, 786–792. doi: 10.1016/j.bbrc.2019.03.126
Chen, L. L. (2016). The biogenesis and emerging roles of circular RNAs. Nat. Rev. Mol. Cell Biol. 17, 205–211. doi: 10.1038/nrm.2015.32
Dai, F., Dai, L., Zheng, X., Guo, Y., Zhang, Y., Niu, M., et al. (2020). Non-coding RNAs in drug resistance of head and neck cancers: a review. Biomed. Pharmacother 127:110231. doi: 10.1016/j.biopha.2020.110231
de Jong, M. C., Ten Hoeve, J. J., Grénman, R., Wessels, L. F., Kerkhoven, R., Te Riele, H., et al. (2015). Pretreatment microRNA expression impacting on epithelial-to-mesenchymal transition predicts intrinsic radiosensitivity in head and neck cancer cell lines and patients. Clin. Cancer Res. 21, 5630–5638. doi: 10.1158/1078-0432.Ccr-15-0454
Doronkin, S., Djagaeva, I., and Beckendorf, S. K. (2002). CSN5/Jab1 mutations affect axis formation in the Drosophila oocyte by activating a meiotic checkpoint. Development 129, 5053–5064.
Economopoulou, P., Kotsantis, I., and Psyrri, A. (2016). The promise of immunotherapy in head and neck squamous cell carcinoma: combinatorial immunotherapy approaches. ESMO Open 1:e000122. doi: 10.1136/esmoopen-2016-2122
Emerson, C. H., and Bertuch, A. A. (2016). Consider the workhorse: nonhomologous end-joining in budding yeast. Biochem. Cell Biol. 94, 396–406. doi: 10.1139/bcb-2016-2011
Feng, L., Zhang, J., Sun, M., Qiu, F., Chen, W., and Qiu, W. (2020). Tumor suppressor LINC02487 inhibits oral squamous cell carcinoma cell migration and invasion through the USP17-SNAI1 Axis. Front. Oncol. 10:559808. doi: 10.3389/fonc.2020.559808
Feng, Q., Zhang, H., Yao, D., Chen, W. D., and Wang, Y. D. (2019). Emerging role of non-coding RNAS in esophageal squamous cell carcinoma. Int. J. Mol. Sci. 21:258. doi: 10.3390/ijms21010258
Feng, X., Lv, W., Wang, S., and He, Q. (2018). miR-495 enhances the efficacy of radiotherapy by targeting GRP78 to regulate EMT in nasopharyngeal carcinoma cells. Oncol. Rep. 40, 1223–1232. doi: 10.3892/or.2018.6538
Ferlay, J., Soerjomataram, I., Dikshit, R., Eser, S., Mathers, C., Rebelo, M., et al. (2015). Cancer incidence and mortality worldwide: sources, methods and major patterns in GLOBOCAN 2012. Int. J. Cancer 136, E359–E386. doi: 10.1002/ijc.29210
Gao, W., Lam, J. W., Li, J. Z., Chen, S. Q., Tsang, R. K., Chan, J. Y., et al. (2017a). MicroRNA-138-5p controls sensitivity of nasopharyngeal carcinoma to radiation by targeting EIF4EBP1. Oncol. Rep. 37, 913–920. doi: 10.3892/or.2017.5354
Gao, W., Li, Z. H., Chen, S., Chan, J. Y., Yin, M., Zhang, M. J., et al. (2017b). Epstein-Barr virus encoded microRNA BART7 regulates radiation sensitivity of nasopharyngeal carcinoma. Oncotarget 8, 20297–20308. doi: 10.18632/oncotarget.15526
Gehrmann, M., Specht, H. M., Bayer, C., Brandstetter, M., Chizzali, B., Duma, M., et al. (2014). Hsp70–a biomarker for tumor detection and monitoring of outcome of radiation therapy in patients with squamous cell carcinoma of the head and neck. Radiat Oncol. 9:131. doi: 10.1186/1748-717x-9-131
Gou, C., Han, P., Li, J., Gao, L., Ji, X., Dong, F., et al. (2020). Knockdown of lncRNA BLACAT1 enhances radiosensitivity of head and neck squamous cell carcinoma cells by regulating PSEN1. Br. J. Radiol. 93:20190154. doi: 10.1259/bjr.20190154
Gu, X., Wang, L., Coates, P. J., Boldrup, L., Fåhraeus, R., Wilms, T., et al. (2020). Transfer-RNA-Derived fragments are potential prognostic factors in patients with squamous cell carcinoma of the head and neck. Genes (Basel) 11:1344. doi: 10.3390/genes11111344
Haimovitz-Friedman, A., Kan, C. C., Ehleiter, D., Persaud, R. S., McLoughlin, M., Fuks, Z., et al. (1994). Ionizing radiation acts on cellular membranes to generate ceramide and initiate apoptosis. J. Exp. Med. 180, 525–535. doi: 10.1084/jem.180.2.525
Han, Q., Li, L., Liang, H., Li, Y., Xie, J., and Wang, Z. (2017). Downregulation of lncRNA X Inactive Specific Transcript (XIST) suppresses cell proliferation and enhances radiosensitivity by upregulating mir-29c in nasopharyngeal carcinoma cells. Med. Sci. Monit. 23, 4798–4807. doi: 10.12659/msm.905370
Hanai, R., Yazu, M., and Hieda, K. (1998). On the experimental distinction between ssbs and dsbs in circular DNA. Int. J. Radiat. Biol. 73, 475–479. doi: 10.1080/095530098142013
He, Y., Jing, Y., Wei, F., Tang, Y., Yang, L., Luo, J., et al. (2018). Long non-coding RNA PVT1 predicts poor prognosis and induces radioresistance by regulating DNA repair and cell apoptosis in nasopharyngeal carcinoma. Cell Death Dis. 9:235. doi: 10.1038/s41419-018-0265-y
Hess, A. K., Müer, A., Mairinger, F. D., Weichert, W., Stenzinger, A., Hummel, M., et al. (2017). MiR-200b and miR-155 as predictive biomarkers for the efficacy of chemoradiation in locally advanced head and neck squamous cell carcinoma. Eur. J. Cancer 77, 3–12. doi: 10.1016/j.ejca.2017.02.018
Hu, X., Jiang, H., and Jiang, X. (2017). Downregulation of lncRNA ANRIL inhibits proliferation, induces apoptosis, and enhances radiosensitivity in nasopharyngeal carcinoma cells through regulating miR-125a. Cancer Biol. Ther. 18, 331–338. doi: 10.1080/15384047.2017.1310348
Huang, N. S., Lei, B. W., Tan, L. C., Yu, P. C., Shi, X., Wang, Y., et al. (2020). Mitotically associated long non-coding RNA is a tumor promoter in anaplastic thyroid cancer. Ann. Transl. Med. 8:1226. doi: 10.21037/atm-20-4530
Huang, S., Zhan, Z., Li, L., Guo, H., Yao, Y., Feng, M., et al. (2019). LINC00958-MYC positive feedback loop modulates resistance of head and neck squamous cell carcinoma cells to chemo- and radiotherapy in vitro. Onco Targets Ther. 12, 5989–6000. doi: 10.2147/ott.S208318
Huang, T., Yin, L., Wu, J., Gu, J. J., Wu, J. Z., Chen, D., et al. (2016). MicroRNA-19b-3p regulates nasopharyngeal carcinoma radiosensitivity by targeting TNFAIP3/NF-κB axis. J. Exp. Clin. Cancer Res. 35:188. doi: 10.1186/s13046-016-0465-461
Huang, Y., Tan, D., Xiao, J., Li, Q., Zhang, X., and Luo, Z. (2018). miR-150 contributes to the radioresistance in nasopharyngeal carcinoma cells by targeting glycogen synthase kinase-3β. J. Cancer Res. Ther. 14, 111–118. doi: 10.4103/jcrt.JCRT_682_17
Jackson, S. P. (2002). Sensing and repairing DNA double-strand breaks. Carcinogenesis 23, 687–696. doi: 10.1093/carcin/23.5.687
Jarvis, W. D., Fornari, F. A. Jr., Browning, J. L., Gewirtz, D. A., Kolesnick, R. N., et al. (1994a). Attenuation of ceramide-induced apoptosis by diglyceride in human myeloid leukemia cells. J. Biol. Chem. 269, 31685–31692. doi: 10.1016/s0021-9258(18)31750-2
Jarvis, W. D., Kolesnick, R. N., Fornari, F. A., Traylor, R. S., Gewirtz, D. A., and Grant, S. (1994b). Induction of apoptotic DNA damage and cell death by activation of the sphingomyelin pathway. Proc. Natl. Acad. Sci. U S A. 91, 73–77. doi: 10.1073/pnas.91.1.73
Jiang, Y., Cao, W., Wu, K., Qin, X., Wang, X., Li, Y., et al. (2019). LncRNA LINC00460 promotes EMT in head and neck squamous cell carcinoma by facilitating peroxiredoxin-1 into the nucleus. J. Exp. Clin. Cancer Res. 38:365. doi: 10.1186/s13046-019-1364-z
Kang, M., Xiao, J., Wang, J., Zhou, P., Wei, T., Zhao, T., et al. (2016). MiR-24 enhances radiosensitivity in nasopharyngeal carcinoma by targeting SP1. Cancer Med. 5, 1163–1173. doi: 10.1002/cam4.660
Kangboonruang, K., Wongtrakoongate, P., Lertsuwan, K., Khachonkham, S., Changkaew, P., Tangboonduangjit, P., et al. (2020). MALAT1 decreases the sensitivity of head and neck squamous cell carcinoma cells to radiation and cisplatin. Anticancer Res. 40, 2645–2655. doi: 10.21873/anticanres.14235
Kong, L., Wei, Q., Hu, X., Chen, L., and Li, J. (2019). miR-193a-3p promotes radio-resistance of nasopharyngeal cancer cells by targeting SRSF2 gene and hypoxia signaling pathway. Med. Sci. Monit. Basic Res. 25, 53–62. doi: 10.12659/msmbr.914572
Lacas, B., Bourhis, J., Overgaard, J., Zhang, Q., Grégoire, V., Nankivell, M., et al. (2017). Role of radiotherapy fractionation in head and neck cancers (MARCH): an updated meta-analysis. Lancet Oncol. 18, 1221–1237. doi: 10.1016/s1470-2045(17)30458-30458
Leemans, C. R., Snijders, P. J. F., and Brakenhoff, R. H. (2018). The molecular landscape of head and neck cancer. Nat. Rev. Cancer 18, 269–282. doi: 10.1038/nrc.2018.11
Lewanski, C. R., and Gullick, W. J. (2001). Radiotherapy and cellular signalling. Lancet Oncol. 2, 366–370. doi: 10.1016/s1470-2045(00)00391-390
Li, B. Y., Luo, Y., Zhao, W. S., Zhang, L., Zhou, H. J., Zou, Y. C., et al. (2017). MicroRNA-210 negatively regulates the radiosensitivity of nasopharyngeal carcinoma cells. Mol. Med. Rep. 16, 1401–1408. doi: 10.3892/mmr.2017.6694
Li, L. N., Xiao, T., Yi, H. M., Zheng, Z., Qu, J. Q., Huang, W., et al. (2017). MiR-125b increases nasopharyngeal carcinoma radioresistance by targeting A20/NF-κB signaling pathway. Mol. Cancer Ther. 16, 2094–2106. doi: 10.1158/1535-7163.Mct-17-0385
Li, G., Liu, Y., Su, Z., Ren, S., Zhu, G., Tian, Y., et al. (2013). MicroRNA-324-3p regulates nasopharyngeal carcinoma radioresistance by directly targeting WNT2B. Eur. J. Cancer 49, 2596–2607. doi: 10.1016/j.ejca.2013.03.001
Li, J., Liu, X., Nan, S., and Xu, C. (2020). Silencing of long non-coding RNA LINC00520 promotes radiosensitivity of head and neck squamous cell carcinoma cells. Free Radic Res. 54, 254–270. doi: 10.1080/10715762.2020.1752373
Lin, T., Zhou, F., Zhou, H., Pan, X., Sun, Z., and Peng, G. (2015). MicroRNA-378g enhanced radiosensitivity of NPC cells partially by targeting protein tyrosine phosphatase SHP-1. Int. J. Radiat. Biol. 91, 859–866. doi: 10.3109/09553002.2015.1096028
Maia, D., de Carvalho, A. C., Horst, M. A., Carvalho, A. L., Scapulatempo-Neto, C., and Vettore, A. L. (2015). Expression of miR-296-5p as predictive marker for radiotherapy resistance in early-stage laryngeal carcinoma. J. Transl. Med. 13:262. doi: 10.1186/s12967-015-0621-y
Mansour, W. Y., Bogdanova, N. V., Kasten-Pisula, U., Rieckmann, T., Köcher, S., Borgmann, K., et al. (2013). Aberrant overexpression of miR-421 downregulates ATM and leads to a pronounced DSB repair defect and clinical hypersensitivity in SKX squamous cell carcinoma. Radiother Oncol. 106, 147–154. doi: 10.1016/j.radonc.2012.10.020
Marie-Egyptienne, D. T., Lohse, I., and Hill, R. P. (2013). Cancer stem cells, the epithelial to mesenchymal transition (EMT) and radioresistance: potential role of hypoxia. Cancer Lett. 341, 63–72. doi: 10.1016/j.canlet.2012.11.019
Mattick, J. S., and Makunin, I. V. (2006). Non-coding RNA. Hum. Mol. Genet. 15, R17–R29. doi: 10.1093/hmg/ddl046
Mitchell, P. S., Parkin, R. K., Kroh, E. M., Fritz, B. R., Wyman, S. K., Pogosova-Agadjanyan, E. L., et al. (2008). Circulating microRNAs as stable blood-based markers for cancer detection. Proc. Natl. Acad. Sci. U S A. 105, 10513–10518. doi: 10.1073/pnas.0804549105
Nakashima, H., Yoshida, R., Hirosue, A., Kawahara, K., Sakata, J., Arita, H., et al. (2019). Circulating miRNA-1290 as a potential biomarker for response to chemoradiotherapy and prognosis of patients with advanced oral squamous cell carcinoma: a single-center retrospective study. Tumour Biol. 41:1010428319826853. doi: 10.1177/1010428319826853
Nastasi, C., Mannarino, L., and D’Incalci, M. (2020). DNA damage response and immune defense. Int. J. Mol. Sci. 21:7504. doi: 10.3390/ijms21207504
Nichols, A. C., Theurer, J., Prisman, E., Read, N., Berthelet, E., Tran, E., et al. (2019). Radiotherapy versus transoral robotic surgery and neck dissection for oropharyngeal squamous cell carcinoma (ORATOR): an open-label, phase 2, randomised trial. Lancet Oncol. 20, 1349–1359. doi: 10.1016/s1470-2045(19)30410-30413
Obeid, L. M., Linardic, C. M., Karolak, L. A., and Hannun, Y. A. (1993). Programmed cell death induced by ceramide. Science 259, 1769–1771. doi: 10.1126/science.8456305
Pan, Y., Zhang, Q., Atsaves, V., Yang, H., and Claret, F. X. (2013). Suppression of Jab1/CSN5 induces radio- and chemo-sensitivity in nasopharyngeal carcinoma through changes to the DNA damage and repair pathways. Oncogene 32, 2756–2766. doi: 10.1038/onc.2012.294
Pan, Y., Zhang, Q., Tian, L., Wang, X., Fan, X., Zhang, H., et al. (2012). Jab1/CSN5 negatively regulates p27 and plays a role in the pathogenesis of nasopharyngeal carcinoma. Cancer Res. 72, 1890–1900. doi: 10.1158/0008-5472.Can-11-3472
Pasi, F., Corbella, F., Baio, A., Capelli, E., De Silvestri, A., Tinelli, C., et al. (2020). Radiation-induced circulating miRNA expression in blood of head and neck cancer patients. Radiat Environ. Biophys. 59, 237–244. doi: 10.1007/s00411-020-00832-833
Pignon, J. P., le Maître, A., Maillard, E., and Bourhis, J. (2009). Meta-analysis of chemotherapy in head and neck cancer (MACH-NC): an update on 93 randomised trials and 17,346 patients. Radiother Oncol. 92, 4–14. doi: 10.1016/j.radonc.2009.04.014
Plavc, G., Jesenko, T., Oražem, M., and Strojan, P. (2020). Challenges in combining immunotherapy with radiotherapy in recurrent/metastatic head and neck cancer. Cancers (Basel) 12:3197. doi: 10.3390/cancers12113197
Qu, C., Liang, Z., Huang, J., Zhao, R., Su, C., Wang, S., et al. (2012). MiR-205 determines the radioresistance of human nasopharyngeal carcinoma by directly targeting PTEN. Cell Cycle 11, 785–796. doi: 10.4161/cc.11.4.19228
Qu, J. Q., Yi, H. M., Ye, X., Li, L. N., Zhu, J. F., Xiao, T., et al. (2015a). MiR-23a sensitizes nasopharyngeal carcinoma to irradiation by targeting IL-8/Stat3 pathway. Oncotarget 6, 28341–28356. doi: 10.18632/oncotarget.5117
Qu, J. Q., Yi, H. M., Ye, X., Zhu, J. F., Yi, H., Li, L. N., et al. (2015b). MiRNA-203 reduces nasopharyngeal carcinoma radioresistance by targeting IL8/AKT signaling. Mol. Cancer Ther. 14, 2653–2664. doi: 10.1158/1535-7163.Mct-15-0461
Rothkamm, K., and Löbrich, M. (2003). Evidence for a lack of DNA double-strand break repair in human cells exposed to very low x-ray doses. Proc. Natl. Acad. Sci. U S A. 100, 5057–5062. doi: 10.1073/pnas.0830918100
Sher, D. J., Pham, N. L., Shah, J. L., Sen, N., Williams, K. A., Subramaniam, R. M., et al. (2020a). Prospective phase II study of radiotherapy dose and volume de-escalation for elective neck treatment of oropharyngeal and laryngeal cancer. Int. J. Radiat. Oncol. Biol. Phys. doi: 10.1016/j.ijrobp.2020.09.063 [Epub ahead of print].
Sher, D. J., Yan, J., Day, A. T., Khan, S., and Zhu, H. (2020b). Comparative cost analysis between definitive radiotherapy and transoral surgery for oropharyngeal squamous cell carcinoma: a SEER-Medicare analysis. Oral Oncol. 112:105029. doi: 10.1016/j.oraloncology.2020.105029
Shiiba, M., Shinozuka, K., Saito, K., Fushimi, K., Kasamatsu, A., Ogawara, K., et al. (2013). MicroRNA-125b regulates proliferation and radioresistance of oral squamous cell carcinoma. Br. J. Cancer 108, 1817–1821. doi: 10.1038/bjc.2013.175
Shuai, M., and Huang, L. (2020). High expression of hsa_circRNA_001387 in nasopharyngeal carcinoma and the effect on efficacy of radiotherapy. Onco Targets Ther. 13, 3965–3973. doi: 10.2147/ott.S249202
Slack, F. J. (2006). Regulatory RNAs and the demise of ‘junk’. DNA. Genome Biolo. 7:328. doi: 10.1186/gb-2006-7-9-328
Suh, Y. E., Raulf, N., Gäken, J., Lawler, K., Urbano, T. G., Bullenkamp, J., et al. (2015). MicroRNA-196a promotes an oncogenic effect in head and neck cancer cells by suppressing annexin A1 and enhancing radioresistance. Int. J. Cancer 137, 1021–1034. doi: 10.1002/ijc.29397
Sun, Q., Liu, T., Zhang, T., Du, S., Xie, G. X., Lin, X., et al. (2015). MiR-101 sensitizes human nasopharyngeal carcinoma cells to radiation by targeting stathmin 1. Mol. Med. Rep. 11, 3330–3336. doi: 10.3892/mmr.2015.3221
Sur, S., Nakanishi, H., Steele, R., Zhang, D., Varvares, M. A., and Ray, R. B. (2020). Long non-coding RNA ELDR enhances oral cancer growth by promoting ILF3-cyclin E1 signaling. EMBO Rep. 21, e51042. doi: 10.15252/embr.202051042
Symington, L. S., and Gautier, J. (2011). Double-strand break end resection and repair pathway choice. Annu. Rev. Genet. 45, 247–271. doi: 10.1146/annurev-genet-110410-132435
Tao, Y., Aupérin, A., Sun, X., Sire, C., Martin, L., Coutte, A., et al. (2020). Avelumab-cetuximab-radiotherapy versus standards of care in locally advanced squamous-cell carcinoma of the head and neck: the safety phase of a randomised phase III trial GORTEC 2017-01 (REACH). Eur. J. Cancer 141, 21–29. doi: 10.1016/j.ejca.2020.09.008
Tian, Y., Yan, M., Zheng, J., Li, R., Lin, J., Xu, A., et al. (2019). miR-483-5p decreases the radiosensitivity of nasopharyngeal carcinoma cells by targeting DAPK1. Lab Invest. 99, 602–611. doi: 10.1038/s41374-018-0169-166
Vahabi, M., Pulito, C., Sacconi, A., Donzelli, S., D’Andrea, M., Manciocco, V., et al. (2019). miR-96-5p targets PTEN expression affecting radio-chemosensitivity of HNSCC cells. J. Exp. Clin. Cancer Res. 38:141. doi: 10.1186/s13046-019-1119-x
Vo, D. T., Karanam, N. K., Ding, L., Saha, D., Yordy, J. S., Giri, U., et al. (2019). miR-125a-5p functions as tumor suppressor microRNA And is a marker of locoregional recurrence and poor prognosis in head and neck cancer. Neoplasia 21, 849–862. doi: 10.1016/j.neo.2019.06.004
Wang, R., Ma, Z., Feng, L., Yang, Y., Tan, C., Shi, Q., et al. (2018). LncRNA MIR31HG targets HIF1A and P21 to facilitate head and neck cancer cell proliferation and tumorigenesis by promoting cell-cycle progression. Mol. Cancer 17:162. doi: 10.1186/s12943-018-0916-918
Wang, S., Pan, Y., Zhang, R., Xu, T., Wu, W., Zhang, R., et al. (2016). Hsa-miR-24-3p increases nasopharyngeal carcinoma radiosensitivity by targeting both the 3’UTR and 5’UTR of Jab1/CSN5. Oncogene 35, 6096–6108. doi: 10.1038/onc.2016.147
Wang, S., Zhang, R., Claret, F. X., and Yang, H. (2014). Involvement of microRNA-24 and DNA methylation in resistance of nasopharyngeal carcinoma to ionizing radiation. Mol. Cancer Ther. 13, 3163–3174. doi: 10.1158/1535-7163.Mct-14-0317
Wang, S. S., Lv, Y., Xu, X. C., Zuo, Y., Song, Y., Wu, G. P., et al. (2019). Triptonide inhibits human nasopharyngeal carcinoma cell growth via disrupting Lnc-RNA THOR-IGF2BP1 signaling. Cancer Lett. 443, 13–24. doi: 10.1016/j.canlet.2018.11.028
Wang, Z., Mao, J. W., Liu, G. Y., Wang, F. G., Ju, Z. S., Zhou, D., et al. (2019). MicroRNA-372 enhances radiosensitivity while inhibiting cell invasion and metastasis in nasopharyngeal carcinoma through activating the PBK-dependent p53 signaling pathway. Cancer Med. 8, 712–728. doi: 10.1002/cam4.1924
Wang, Y., Wang, S., Ren, Y., and Zhou, X. (2020). The role of lncRNA crosstalk in leading cancer metastasis of head and neck squamous cell carcinoma. Front. Oncol. 10:561833. doi: 10.3389/fonc.2020.561833
Weber, A. M., and Ryan, A. J. (2015). ATM and ATR as therapeutic targets in cancer. Pharmacol. Therapeut. 149, 124–138. doi: 10.1016/j.pharmthera.2014.12.001
Weng, J. H., Yu, C. C., Lee, Y. C., Lin, C. W., Chang, W. W., and Kuo, Y. L. (2016). miR-494-3p induces cellular senescence and enhances radiosensitivity in human oral squamous carcinoma cells. Int. J. Mol. Sci. 17:1092. doi: 10.3390/ijms17071092
Wills, W. K. (1904). The present position of radiotherapy in therapeutics. Bristol. Med. Chir. J. (1883) 22, 39–46.
Wu, H., Yang, L., and Chen, L. L. (2017). The diversity of long noncoding RNAs and their generation. Trends Genet. 33, 540–552. doi: 10.1016/j.tig.2017.05.004
Wu, H., Zheng, J., Deng, J., Zhang, L., Li, N., Li, W., et al. (2015). LincRNA-uc002yug.2 involves in alternative splicing of RUNX1 and serves as a predictor for esophageal cancer and prognosis. Oncogene 34, 4723–4734. doi: 10.1038/onc.2014.400
Wu, S. Y., Wu, A. T., and Liu, S. H. (2016). MicroRNA-17-5p regulated apoptosis-related protein expression and radiosensitivity in oral squamous cell carcinoma caused by betel nut chewing. Oncotarget 7, 51482–51493. doi: 10.18632/oncotarget.9856
Wu, W., Chen, X., Yu, S., Wang, R., Zhao, R., and Du, C. (2018). microRNA-222 promotes tumor growth and confers radioresistance in nasopharyngeal carcinoma by targeting PTEN. Mol. Med. Rep. 17, 1305–1310. doi: 10.3892/mmr.2017.7931
Xu, J., Ai, Q., Cao, H., and Liu, Q. (2015). MiR-185-3p and miR-324-3p Predict radiosensitivity of nasopharyngeal carcinoma and modulate cancer cell growth and apoptosis by targeting SMAD7. Med. Sci. Monit. 21, 2828–2836. doi: 10.12659/msm.895660
Yang, T., Li, S., Liu, J., Yin, D., Yang, X., and Tang, Q. (2018). lncRNA-NKILA/NF-κB feedback loop modulates laryngeal cancer cell proliferation, invasion, and radioresistance. Cancer Med. 7, 2048–2063. doi: 10.1002/cam4.1405
Yao, R. W., Wang, Y., and Chen, L. L. (2019). Cellular functions of long noncoding RNAs. Nat. Cell Biol. 21, 542–551. doi: 10.1038/s41556-019-0311-318
Yi, L., Ouyang, L., Wang, S., Li, S. S., and Yang, X. M. (2019). Long noncoding RNA PTPRG-AS1 acts as a microRNA-194-3p sponge to regulate radiosensitivity and metastasis of nasopharyngeal carcinoma cells via PRC1. J. Cell Physiol. 234, 19088–19102. doi: 10.1002/jcp.28547
Yu, M., Lu, B., Zhang, J., Ding, J., Liu, P., and Lu, Y. (2020). tRNA-derived RNA fragments in cancer: current status and future perspectives. J. Hematol. Oncol. 13:121. doi: 10.1186/s13045-020-00955-956
Zhang, J. X., Qian, D., Wang, F. W., Liao, D. Z., Wei, J. H., Tong, Z. T., et al. (2013). MicroRNA-29c enhances the sensitivities of human nasopharyngeal carcinoma to cisplatin-based chemotherapy and radiotherapy. Cancer Lett. 329, 91–98. doi: 10.1016/j.canlet.2012.10.033
Zhang, L. M., Su, L. X., Hu, J. Z., Wang, M., Ju, H. Y., Li, X., et al. (2020). Epigenetic regulation of VENTXP1 suppresses tumor proliferation via miR-205-5p/ANKRD2/NF-kB signaling in head and neck squamous cell carcinoma. Cell Death Dis. 11:838. doi: 10.1038/s41419-020-03057-w
Zhao, B., Rothenberg, E., Ramsden, D. A., and Lieber, M. R. (2020). The molecular basis and disease relevance of non-homologous DNA end joining. Nat. Rev. Mol. Cell Biol. 21, 765–781. doi: 10.1038/s41580-020-00297-298
Zhao, L., Tang, M., Hu, Z., Yan, B., Pi, W., Li, Z., et al. (2015). miR-504 mediated down-regulation of nuclear respiratory factor 1 leads to radio-resistance in nasopharyngeal carcinoma. Oncotarget 6, 15995–16018. doi: 10.18632/oncotarget.4138
Keywords: head and neck cancer, radiotherapy, non-coding RNA, miRNA, long non-coding RNA, circRNA
Citation: Zhang X and Yang J (2021) Role of Non-coding RNAs on the Radiotherapy Sensitivity and Resistance of Head and Neck Cancer: From Basic Research to Clinical Application. Front. Cell Dev. Biol. 8:637435. doi: 10.3389/fcell.2020.637435
Received: 03 December 2020; Accepted: 23 December 2020;
Published: 11 February 2021.
Edited by:
José Díaz-Chávez, National Institute of Cancerology (INCAN), MexicoReviewed by:
Zhonghua Wu, The First Affiliated Hospital of China Medical University, ChinaJinxin Shi, The First Affiliated Hospital of China Medical University, China
Copyright © 2021 Zhang and Yang. This is an open-access article distributed under the terms of the Creative Commons Attribution License (CC BY). The use, distribution or reproduction in other forums is permitted, provided the original author(s) and the copyright owner(s) are credited and that the original publication in this journal is cited, in accordance with accepted academic practice. No use, distribution or reproduction is permitted which does not comply with these terms.
*Correspondence: Jing Yang, yangjing7694@126.com