- 1Department of Molecular Pathobiology and Cell Adhesion Biology, Mie University Graduate School of Medicine, Tsu, Japan
- 2Department of Cardiothoracic and Vascular Surgery, Mie University Graduate School of Medicine, Tsu, Japan
- 3Department of Emergency and Disaster Medicine, Mie University Graduate School of Medicine, Tsu, Japan
Extracellular vesicles (EVs) have emerged as key players of intercellular communication and mediate crosstalk between tissues. Metastatic tumors release tumorigenic EVs, capable of pre-conditioning distal sites for organotropic metastasis. Growing evidence identifies muscle cell-derived EVs and myokines as potent mediators of cellular differentiation, proliferation, and metabolism. Muscle-derived EVs cargo myokines and other biological modulators like microRNAs, cytokines, chemokines, and prostaglandins hence, are likely to modulate the remodeling of niches in vital sites, such as liver and adipose tissues. Despite the scarcity of evidence to support a direct relationship between muscle-EVs and cancer metastasis, their indirect attribution to the regulation of niche remodeling and the establishment of pre-metastatic homing niches can be put forward. This hypothesis is supported by the role of muscle-derived EVs in findings gathered from other pathologies like inflammation and metabolic disorders. In this review, we present and discuss studies that evidently support the potential roles of muscle-derived EVs in the events of niche pre-conditioning and remodeling of metastatic tumor microenvironment. We highlight the potential contributions of the integrin-mediated interactions with an emerging myokine, irisin, to the regulation of EV-driven microenvironment remodeling in tumor metastasis. Further research into muscle-derived EVs and myokines in cancer progression is imperative and may hold promising contributions to advance our knowledge in the pathophysiology, progression and therapeutic management of metastatic cancers.
Introduction
Extracellular vesicles (EVs) are secreted by all cell types of the body, including tumor cells and can be isolated from various biological fluids (Neven et al., 2017). Initially thought to be cellular “trash bins” containing unwanted excretes, EVs in recent times have insightfully been recognized as significant players in proximal and distal intercellular communication. A peculiar characteristic of EVs is their ability to cargo several biologic materials, capable of influencing physiological and pathological processes (Frühbeis et al., 2013; Regev-Rudzki et al., 2013; Abels and Breakefield, 2016) (Figures 1, 2). In recent times, rapid progress is being made with regards to the implementation of EVs in therapeutic interventions in the areas of inflammation, metabolic disorders, vaccination and drug delivery (Viaud et al., 2010; Lee et al., 2012; Hagiwara et al., 2014). Muscle-derived EVs have gained attention owing to their beneficial function in modulating metabolism, cell differentiation and regeneration (Choi et al., 2016; Takafuji et al., 2020a). It remains yet to be confirmed, the direct role of muscle-derived EVs in regulating cancer progression and metastasis, however, the evidence of regulatory properties of muscle EVs and myokines on other cell types hold promising cues for their potential role in tumor spread (Gannon et al., 2015; Zhang et al., 2018). In this review, we summarize the biogenesis, characteristics and functions, as well as recent findings on how muscle-derived EVs mitigate or aggravate disease conditions. We also attempt to spotlight the potential role of muscle EVs in remodeling metastatic pre-conditioning events via irisin-triggered integrin signaling.
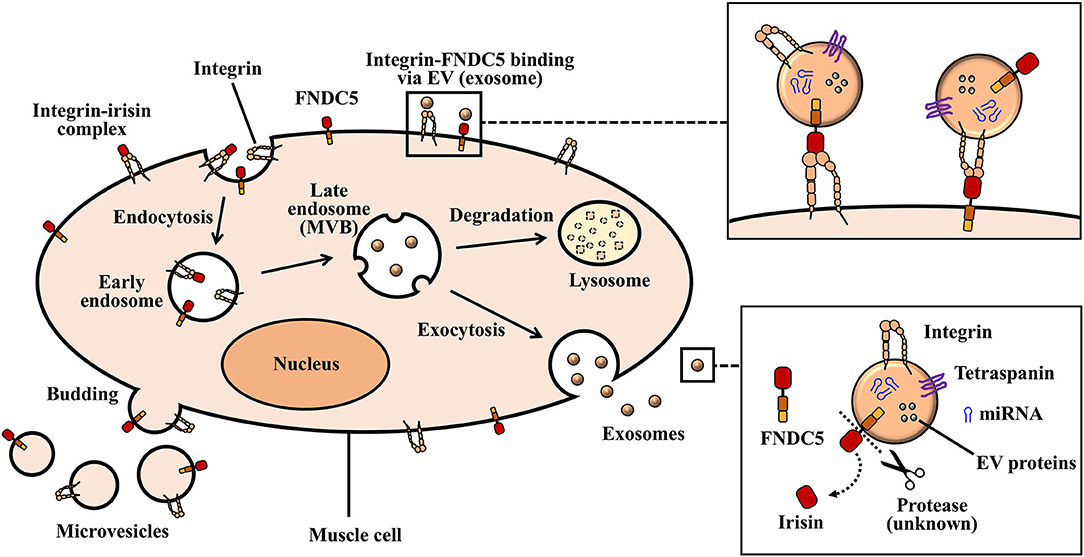
Figure 1. Biogenesis of exosomes and microvesicles. Inward protrusions from the plasma membrane into the cytoplasm allows for the internalization of extracellular and transmembrane proteins in the form of early endosomes. Multivesicular bodies (MVBs), as well as intraluminal vesicles originate from invaginated early endosomes. Large MVBs fuse with the plasma membrane and release exosomes into extracellular space. Vesicles that arise from the outward budding and fusion of plasma membrane make up ectosomes (microvesicles).
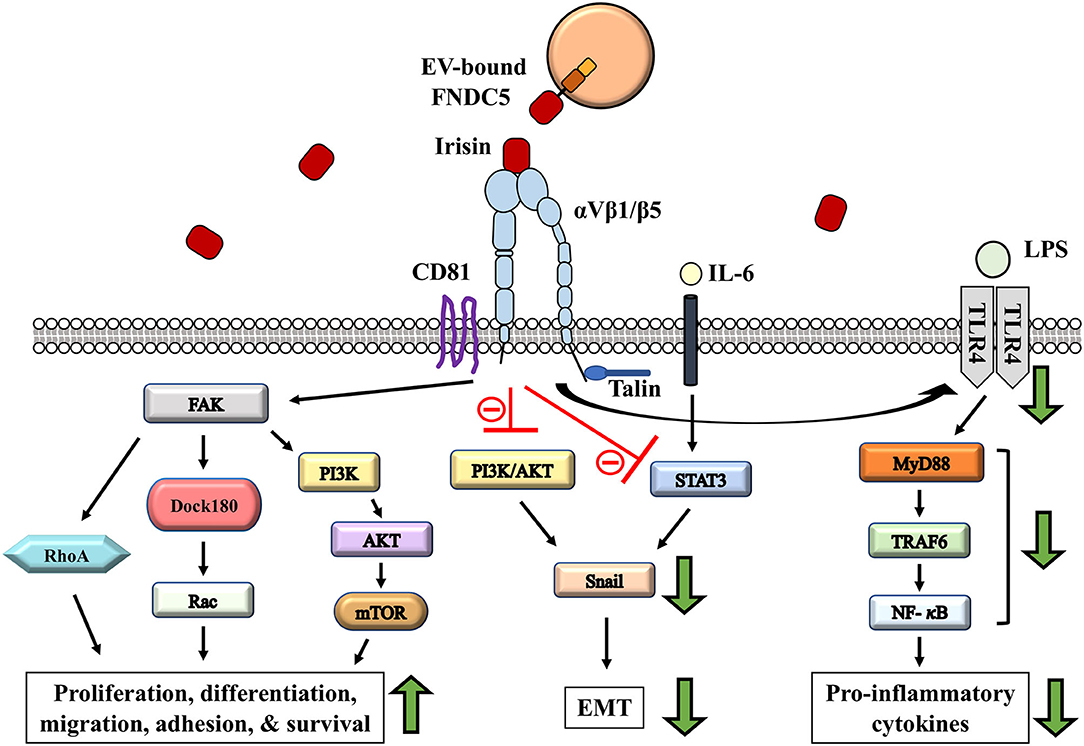
Figure 2. Potential role of irisin-involved integrin signaling cascade in modulating tumor microenvironment remodeling. Irisin exerts anti-inflammatory and cancer suppressing effects through the reduction in proinflammatory cytokines; via regulation of TLR4/MyD88 downstream signaling (Mazur-Bialy et al., 2017a,b). IL-6 induced EMT is regulated by irisin through STAT3/Snail signaling pathways in osteosarcoma (Kong et al., 2017). EMT inhibition is mediated by irisin signaling via the inhibition of PI3K/AKT pathway in lung cancer cells (Shao et al., 2017). Activation of CD81/integrin-mediated FAK signaling in response to irisin regulate cell survival, proliferation and migration (Oguri et al., 2020).
Biogenesis, Characteristics, and Function of Extracellular Vesicles
Extracellular vesicles (EVs) describe the heterogenous collection of a highly conserved system for intercellular communication, within which cells are able to exchange information in the form of biologically functional components: nucleic acids, proteins, and lipids (Stahl and Raposo, 2018; Wiklander et al., 2019). EVs are released by all cell types as membrane-bound spherical structures that originate from the endosome or plasma membrane, and are present in many body fluids, such as blood, urine, semen, saliva, and breast milk (Neven et al., 2017). The term, “extracellular vesicles” is generic and hence specific properties, such as the origin/source, physical characteristics and biochemical properties are required to adequately define the various subtypes (Théry et al., 2018).
Classically, EVs are broadly categorized into three vesicular types depending on their biogenesis; outward budding or fusion of multivesicular bodies (MVB) with plasma membrane. This classification gives rise to three distinct vesicle types, namely, Exosomes (EXOs), microvesicles (MVs), and apoptotic bodies (Akers et al., 2013) (Figure 1). EVs are isolated from biological samples via a myriad of isolation methods, such as precipitation, filtration, centrifugation, and a combination of different method. However, specificity and recovery of EVs may greatly vary per the isolation methods used to obtain them. Additionally, the presence of EVs in biological samples are confirmed by specific protein and lipid markers (present or absent) on the various subtypes. Well-documented guidelines outlining the recommended protocols for EVs and their documentation (provided by the International Society of Extracellular Vesicles-ISEV) exist. These guidelines encompass protocols and recommendations designed for the efficient characterization, isolation, and functional studies of EVs (Théry et al., 2018).
Exosomes
Exosomes, which are nano-sized vesicles ranging between small diameters of about 30–150 nm, are derived from the endosomal system. The formation and secretion of exosomes begin with the invagination of the plasma membrane (endocytosis) to form a cup-like structure harboring cell-surface proteins and extracellular deposits (Kalluri and LeBleu, 2020). This invagination forms an early endosome which then mature to form late endosomes. Subsequently, intraluminal vesicles (ILVs) are formed by the progressive, inward budding of the late endosomal membrane. The accumulation of ILVs in the late endosome generates multivesicular bodies (MVBs) (Kalluri and LeBleu, 2020; Yue et al., 2020). At this stage, MVBs can fuse with autophagosomes or lysosomes for the degradation of their endosomal contents. Degraded components can then be recycled by the cell. Alternatively, MVBs (containing mature or late endosomes) that do not traffic to lysosomes move toward the cytoplasmic side of the plasma membrane with the aid of the cellular cytoskeletal and microtubule network (Kalluri and LeBleu, 2020). Here, MVBs are able to dock via MVB-docking proteins and fuse with the plasma membrane as exocytic MVBs. Exocytic MVBs release their lipid bi-layered ILV contents to the extracellular space as exosomes (Kalra et al., 2016) (Figure 1).
It still remains to be completely understood, the processes that govern the formation of ILVs in MVBs and their further release as exosomes. However, a proposed mechanism implicated in this process involves the Endosomal Sorting Complex Required for Transport (ESCRT). ESCRTs comprise of several proteins that assemble into four ESCRT complexes: namely ESCRT-0, -I, -II, and -III, in association with others like ALIX and vacuolar protein sorting-associated proteins (VPS4, VTA 1) (Colombo et al., 2013). Initiating this process is the organization of endosomal membranes into specialized units that are highly enriched for the tetraspanin class of membrane proteins. Tetraspanins (e.g., CD63, CD9, and CD81) function to cluster the required proteins for ILV formation and are considered common markers for the identification of exosomal vesicles (Pols and Klumperman, 2009). ESCRTs are then recruited to the site of ILV formation where ESCRT-0 recognizes and binds to ubiquitinated proteins present on the outside of the endosomal membrane. ESCRTs-I and -II are then assembled to the cytosolic area to initiate and drive intraluminal membrane budding. The assembling of ESCRT-I/II is reportedly stimulated by some factors, such as the abundance of phosphatidylinositol 3-phosphate (PIP3) in the membrane early endosomes, ESCRT-0 proteins (e.g., hepatocyte growth factor-regulated tyrosine kinase substrate-HRS) and the ubiquitination of the cytosolic tail of endocytosed proteins and/or their curved membrane topology (Henne et al., 2013; Abels and Breakefield, 2016). Upon activation of ESCRT-II, ESCRT-III is recruited through an associated protein, ALIX. ALIX binds to the tumor susceptibility gene 101 (TSG101) component of ESCRT-I complex and also to the charged MVB protein 4A (CHMP4A) components of ESCRT-III, thus, serving as an intermediate between ESCRT-III and ESCRT-I association (McCullough et al., 2008). ESCRT-III in conjunction with deubiquitinating enzymes then finalize the processes that involve vesicle closure and the detachment of ILVs from the membrane. ESCRT-III forms structures that constrict budding neck followed by scissoring of the membrane (driven by accessory proteins, such as VPS4) and finally, the dissociation and recycling of the ESCRT machinery (Kalra et al., 2016; Schöneberg et al., 2017).
Recent studies identify an alternate ESCRT-independent pathway for ILV formation which involves clustering of sphingomyelin in lipid rafts where it is converted to ceramide by sphingomyelinases. The accumulation of ceramide subsequently induces the merging of microdomain, thus, triggering ILV formation (Stuffers et al., 2009; Kalra et al., 2016). As such, exosomes presumed to be formed through this pathway may lack the expression of common markers, such as ALIX and TSG 101 involved in the endosomal pathway. Small RAB GTPases facilitate the docking, fusion and secretion of exosomes. For example, RAB27A promotes docking of MVBs and fusion to the plasma membrane, whiles RAB27B is involved in vesicle transfer from the Golgi to MVBs, and mobilization of MVBs to the actin-rich cortex under the plasma membrane (Ostrowski et al., 2010; Kalra et al., 2016).
Microvesicles
Vesicles that arise through direct outward budding and fission of the plasma membrane are known as microvesicles (MVs) or ectosomes (Figure 1). These range between relatively larger diameters of about 50–2,000 nm (Akers et al., 2013). Ectosome biogenesis involves plasma membrane phospholipid re-arrangements/redistribution, as well as, contractions of cytoskeletal proteins.
Enzymes (collectively described as aminophospholipid translocases) involved in the exchange of lipids between the inner and outer leaflet of the cell membrane for asymmetric maintenance are activated to induce changes within the bilayer to allow for budding and membrane abscission. Flippases are translocases required for the transfer of phospholipids from the outer leaflet to the inner leaflet and floppases, on the other hand, transfer phospholipids from within out. Following this, actin and myosin interactions within the cytosol induce the contraction of cytoskeletal structures; a mechanism that underlies bud formation and subsequent release of ectosome portions. Microvesicles, like exosomes, can be highly enriched in sets of proteins and are particularly identified with markers, such as vesicle-associated membrane protein 3 (VAMP3) and ADP-ribosylation factor 6 (ARF6) (Hugel et al., 2005; Muralidharan-Chari et al., 2009).
Apoptotic Bodies
During programmed cell death, apoptotic cells undergo several stages. These stages begin with condensation of the nuclear chromatin and then membrane blebbing. Cellular components that undergo disintegration are then processed into distinct membrane-enclosed vesicles. These vesicles comprise of a type of EV known as apoptotic bodies that range in sizes between 500 and 4,000 nm. Apoptotic bodies pack organelles or cytosolic components and are capable of transferring genetic information from cell to cell via their uptake (Bergsmedh et al., 2001; Cocucci et al., 2009; Kalra et al., 2016).
Cellular Microenvironments (Niches) and Extracellular Vesicles
Accumulating evidence has revealed that EVs are involved in the inter-cellular communication of a wide range of physiology and pathophysiology (Park et al., 2019a; Wortzel et al., 2019; Kalluri and LeBleu, 2020). In the following sections, we focus on an emerging topic (Myint et al., 2020): the roles of EVs in the regulations of microenvironments supporting the trafficking of normal (lymphocytes) and transformed (e.g., cancer) cells in sections ‘Cellular Microenvironments (Niches) and Extracellular Vesicles' and ‘EV-Mediated Remodeling of Cancer Microenvironment', respectively.
Efficient migration and retention of specific cell types in various compartments of the body are supported by a special microenvironment (homing niche). Such microenvironments have the ability to maintain specific cell populations away from apoptotic and differentiation signals, as well as provide an ambience (cellular structures, extracellular proteins and soluble factors) for proliferation (Moore and Lemischka, 2006).
EVs in Lymphocyte Homing
The regulated migration of lymphocytes is necessary for their effective function. This regulation phenomenon termed, “lymphocyte homing” enables for the trafficking of lymphocytes to specific sites of the body. Lymphocyte homing has been particularly demonstrated in gut immunology. Antigen-primed naïve T lymphocytes in the gut lymph nodes are imprinted to allow them home back into gut tissues as effector or memory T lymphocytes: a necessity for efficient adaptive immunity in the gut mucosa (Myint et al., 2020). High endothelial venules (HEV) of the gut associated lymphoid tissues (GALT) highly express mucosal addressin cell adhesion molecule-1 (MAdCAM-1) that engages the receptor integrin α4β7 expressed on activated lymphocytes. This interaction enables for the homing of antigen-specific B and T lymphocytes into gut tissues (Streeter et al., 1988; Berlin et al., 1995). The inability of β7 integrin deficient mice to mount antigen-mediated humoral responses in the gut affirms the critical role of integrin α4β7 in gut mucosa B-cell immune responses (Schippers et al., 2012). Additionally, HEVs in GALT chemo-attract chemokine receptor 7 (CCR7)-bearing naïve and central memory T lymphocytes via CCL21 (Okada et al., 2002). Gut-tropic lymphocytes expressing chemokine receptor 9 (CCR9) are signaled by CCL25 and integrin α4β7/MADCAM-1 expressing epithelial cells of HEV to facilitate their migration and homing to the gut (Myint et al., 2020). Lymphocyte homing niche in the gut is physiologically regulated. The expression of MAdCAM-1 in the gut is stabilized in limited levels to maintain homeostasis (Pabst et al., 2000). Elevated signal from inflammatory mediators as seen in gut inflammatory diseases upregulate MAdCAM-1 expression and promote the accumulation of activated T lymphocytes that abundantly express integrin α4β7 (Myint et al., 2020).
Remodeling of homing niches to regulate the tissue-specific migration and recruitment of activated lymphocytes has been demonstrated in recent studies. One mechanism by which the homing niche is remodeled appears to be through gut-tropic derived exosomal secretions, demonstrated by Park et al. (2019b). Activated gut-tropic T lymphocytes secrete exosomes that highly express functional integrin α4β7 with the ability to bind MAdCAM-1 and preferentially home to the gut mucosa. These α4β7 expressing exosomes function to suppress MAdCAM-1 through the cargo and delivery of microRNA milieu that target the transcriptional factor, Nirenberg-Kim (NK) 2 homeobox 3 (NKX2.3) necessary for MAdCAM-1 regulation. Consequently, other homing niche factors, such as the chemokines CCL25 and CCL28 were suppressed by gut-tropic lymphocyte-derived exosomes (Park et al., 2019b). It is therefore not far-fetched to consider exosomes of lymphocytes and other host cells as key remodeling regulators of homing niches to maintain homeostasis.
Other Homing Niches
EV-mediated regulation of homing niches (by modifying the expression of adhesion molecules and chemokines) may operate in stem cell trafficking, and warrants further research. Here we explain the overview of how integrins and chemokines govern stem cell trafficking. Physiologically, stem cells home to specific tissue areas via chemoattraction. For example, hematopoietic stem cell precursors home to the bone marrow after selectin- mediated braking (E-selectins, P-selectins, and L-selectins) that facilitates migration on adhesion ligands expressed by vascular endothelium (Liesveld et al., 2020). Following braking, intracellular signaling cascades are activated through chemokine- chemokine receptor interactions (e.g., CXCL12/CXCR4) to trigger the activation states of integrins like αLβ2 (LFA-1) and α4β1 (VLA-4). This facilitates the arrest of cells and their subsequent migration via integrin/cell adhesion molecule (CAM) interaction (Schweitzer et al., 1996; Huttenlocher and Horwitz, 2011; Sahin and Buitenhuis, 2012). Increased CXCL12/CXCR4 signaling is implicated in the retention of leukocytes, such as mature neutrophils at inflammatory sites (demonstrated in tissue-damaging inflammatory diseases) (Yamada et al., 2011; Isles et al., 2019), or in the bone marrow (demonstrated in Warts, Hypogammaglobulinemia, Infections, and Myelokathexis (WHIM) syndrome-associated neutropenia) (Kawai and Malech, 2009). Additionally, FoxP3+regulatory T cells trafficking and homing to hematopoietic stem cell niche in the bone marrow involves the signaling of CXCR4 (Zou et al., 2004; Hirata et al., 2018). It has been demonstrated that, loss of adhesion molecules like integrins (e.g., β7-integrins: α4β7, αEβ7) and selectins (e.g., P-selectin) influences stem cell and lymphocyte trafficking (Frenette et al., 1996; Schön et al., 1999; Lucas, 2019).
The subventricular zone (SVZ) of the brain is another example of a host site that serves as a niche for neural stem cells (NSC), with inflammation playing a key role in the homing and recruitment of NSC for CNS regeneration. Increased expression of stromal derived factor 1α (SDF-1α or CXCL12) by astrocytes and endothelial cells of ischemic areas in the brain, as well as their constitutive expression and activation of CXCR4 (receptor of CXCL12), facilitate NSC migration toward ischemic brain explants (Imitola et al., 2004). LeX-positive NSCs (denoting a subpopulation of SVZ adult stem cells that express the extracellular matrix (ECM)-associated carbohydrate, Lewis X) potentially differentiate into cells with neuronal phenotypes following transplantation. These cells acquire enhanced migratory and proliferative characteristics upon exposure to SDF-1α (Corti et al., 2005). Other soluble factors involved in the migration and recruitment of NSCs include vascular endothelial growth factor (VEGF), interleukin-6, hepatocyte growth factor (HGF) and platelet derived growth factor (PDGF) (Garzón-Muvdi and Quiñones-Hinojosa, 2009). Just as with the effect of soluble growth factors on NSC homing, the expression of integrins, such as α6β1 in SVZ neurogenic niche play a vital role in NSC recruitment, demonstrated in a rodent model of cerebral ischemia (Prestoz et al., 2001).
EV-Mediated Remodeling of Cancer Microenvironment
Cancer cells secrete exosomes that remodel homing niches to promote migration, retention and proliferation of tumor cell and/or other cell types, thus, tumor exosomes form a part of the tumor-derived factors necessary for pro-tumor microenvironment formation and metastatic niche pre-conditioning (Hoshino et al., 2015). Exosomes released by breast cancer cells are delivered to distal cells of target organs, such as the lung and liver, where they induce the expression of proinflammatory genes through the activation of Src-kinase signaling. Thus, the initiation of exosome-mediated chronic inflammation contributes to the remodeling of distal tissues to favor tumor metastasis. Concomitantly, breast cancer exosomes highly expressing integrins αvβ5 and α6β4 distribute to the fibronectin-enriched ECM of the liver and laminin-enriched ECM of the lung, respectively. As a result, tissues within which these integrin-directed exosomes are specifically distributed become prone to inflammatory-mediated remodeling to support the cultivation of pre-metastatic niche and consequently, predisposed to metastatic cancers (Hoshino et al., 2015; Myint et al., 2020).
Alluring to the evidence that non-tumor cells and their exosomes function to tightly regulate trafficking and homing to specific niches for regeneration, repair and homeostasis (Prestoz et al., 2001; Park et al., 2019b), cancer cells and cancer-derived exosomes on the other hand play a contributory role in remodeling niches to support cancer metastasis (via chronic inflammation, angiogenesis, increased vascular permeability, etc.) (Hoshino et al., 2015; Myint et al., 2020).
Muscle–Derived EVs and Myokines in Microenvironment Remodeling
Growing evidence suggests that major risk factors of cancer development and progression include metabolic disorders that facilitate chronic inflammation, thus, metabolic syndrome is consistently associated with increased risk of common cancers (Esposito et al., 2012). Although mechanisms underlying the link between metabolic syndrome and cancer risk are not fully understood, metabolic syndrome represents a proxy marker for several risk factors, such as lack of physical activity, high dietary fat intake and oxidative stress (Alberti et al., 2009). Adipocytes and infiltrating immune cells induce a systemic low-grade chronic state of inflammation by producing inflammatory mediators/immune cell chemo-attractants (interleukin-1β [IL-lβ], interleukin-6 [IL-6], tumor necrosis factor-α [TNF-α], and monocyte chemoattractant protein-1[MCP-1]) and increasing the circulation of free fatty acids to promote the establishment of tumorigenic microenvironment. This is evident in excess adiposity, insulin resistance, aberrant glucose metabolism and particularly, central obesity (visceral adiposity) (Harvey et al., 2011). Hyperglycemia, a hallmark of metabolic syndrome, is implicated in the dysregulation of growth factors and metabolic hormones like insulin and Insulin-like growth factor (IGF-1). Hyperglycemia-induced suppression of IGF-binding protein synthesis and the promotion of IGF-1 synthesis increase the amounts of bioavailable IGF-1 in circulation; an established critical risk factor for several malignancies (Pollak, 2008). Downstream signaling pathways of activated receptor tyrosine kinases, such as IGF-1 receptor activate the Akt cascades which are commonly altered in epithelial cancers. Typical in tumors and tissues of diabetic rats, is the increased activation of downstream mediators, such as mammalian target of rapamycin (mTOR) (Wong et al., 2010; De Angel et al., 2013).
The association between obesity and physical inactivity has been shown in several studies (Gustat et al., 2002; Golbidi et al., 2012). Evidently, exercise and muscle training prove to be beneficial in the prevention and management of obesity or other related metabolic distress. Decreased daily physical activity in healthy individuals is linked to undesired consequences in metabolism, such as reduced insulin sensitivity and elevated adiposity (Olsen et al., 2008). Exercise induces anti-inflammatory effects, boosts antioxidant capacity, and regulates glucose/fat metabolism. Skeletal muscle activity has been found to be beneficial in regulating metabolism and inflammation within several tissues, including adipose tissues. Contracting muscles release myokines, such as interleukin 6 (IL-6) that positively impact inflammation. IL-6 acts as a pro-inflammatory and an anti-inflammatory mediator, however, studies have shown that exercise-induced muscle-derived IL-6 exerts anti-inflammatory effects. The anti-inflammatory property of IL-6 is shown by way of an inhibitory effect on TNF-α, IL-10, and IL-1β, to protect against TNF-induced insulin resistance (Festa et al., 2000; Febbraio and Pedersen, 2005). Myokines secreted by muscle tissues into the extracellular space are capable of modulating homeostasis in the bone, pancreas and adipose tissues. Such myokines like IL-6, irisin, myostatin, and interleukin-15 (IL-15) play a key role in the crosstalk between muscle and adipose tissues (Leal et al., 2018). Recent articles, such as that of Mika et al., review the potential benefits of exercise-induced release of myokines via the alterations in adipose tissue fatty acid metabolism (e.g., increased lipolysis and reduced fatty acid uptake). Chronic exercise impacts the profile of adipokines released from adipose tissues, as well as promote the “beiging” of adipocytes (Mika et al., 2019).
Intriguingly, the provision of beneficial myokines is not confined to muscle cells. Muscle-derived extracellular vesicles (EVs) cargo and distribute myokines, muscle specific microRNAs (myomiRs) and other soluble factors to proximal and/or distal tissues, thus, crosstalk between muscle and other tissues is at least in part, mediated by EVs (Whitham et al., 2018), from muscles. Exercise and physical activity have been demonstrated to augment the release of muscle EVs into circulation.
Skeletal Muscle EVs
Both myoblasts and non-proliferating myotube forms of skeletal tissue are capable of secreting EVs, in vitro (Forterre et al., 2014a; Choi et al., 2016). Skeletal muscle-derived EVs have been demonstrated to be key players in muscle physiology and systemic homeostasis via paracrine actions (Rome et al., 2019). The release of small EVs into circulation is particularly enhanced by physical exercise, inflammation/stress, and several muscle-related conditions (Frühbeis et al., 2015; Barone et al., 2016). Skeletal muscle is a major contributor of exercise-induced secreted molecules. Some of these secreted molecules were identified in EVs harvested from conditioned medium (CM) of myotube cultures (Forterre et al., 2014b; Deshmukh et al., 2015). Skeletal muscle-derived EVs play vital roles in the differentiation and regeneration of muscle tissue by triggering cues for myogenic processes and myofiber regeneration. Exosomes derived from human skeletal myoblasts were found to contain various myogenic factors, such as insulin-like growth factors (IGFs), fibroblast growth factor-2 (FGF2), and hepatocyte growth factor (HGF) that enhanced terminal myogenic differentiation of stem cells, in vitro (Choi et al., 2016). Conversely, skeletal muscle-derived exosomes may be implicated in suppressing myogenic events. During inflammatory conditions or tissue damage, inflammatory cells and the milieu of pro-inflammatory mediators, such as chemokine ligand 2 (CCL2) induce the production of muscle EVs which package more myostatin (negative regulator of myoblast proliferation and differentiation) and less decorin (myostatin antagonist) (Tidball, 2017; Kim S. et al., 2018).
Crosstalk between the skeletal system and the peripheral nervous system have been demonstrated to be at least in part, mediated by skeletal muscle-derived exosomes. Motor neuron regeneration and survival has been found to be positively impacted by EVs released from muscle cells, in vitro (Madison et al., 2014). Additionally, skeletal muscle denervation induced the release of muscle EVs that preferentially support motor neuron regeneration accuracy. Muscle-derived EVs (containing muscle specific markers, such as α-sarcoglycan) were taken up by denervated, but not naïve nerve tissue around the neuro-muscular junction and significantly induced the exclusive projection of motor neurons to muscle branch, thus, ensuring anatomically accurate motor neuron regeneration in rats (Madison and Robinson, 2019). However, mechanisms underlying exactly how muscle-derived EVs are taken up and localized within the denervated nerve remain a knowledge gap incompletely bridged.
MicroRNAs, carried by muscle EVs have been implicated in the crosstalk between muscle and other cell types (Jalabert et al., 2016; Nie et al., 2019), particularly between muscle and bone in the events of bone remodeling. Skeletal muscle-derived exosomes enriched in miR-27a-3p positively impacted osteogenesis by delivering and increasing miR-27a-3p to target adenomatous polyposis coli (APC), thereby, activating the Wnt/β-catenin pathway to promote osteogenic differentiation of MC3T3-E1 pre-osteoblasts (Xu Q. et al., 2018). Another area of evidence for muscle-bone crosstalk mediated by muscle EVs is osteoclast biogenesis in osteoporosis. Mouse muscle cell line (C2C12 myoblasts)-derived EVs suppressed osteoclast formation and mitochondrial energy metabolism in bone marrow cells (Takafuji et al., 2020a). This group demonstrated that Myo-EVs were successfully taken up by bone cells in culture and suppressed the expression of RANKL-induced osteogenic factors, such as cathepsin K (CTSK), nuclear factor of activated T-cells 1 (NFATc1) and dendrocyte expressed seven transmembrane protein (DCSTAMP). Evidently, Myo-EVs suppressed the oxygen consumption rate and expressions of peroxisome proliferator-activated receptor co-activator 1 beta (PGC1β), NADH-ubiquinone oxidoreductase chain 4 (ND4) and cytochrome c in mouse bone cells (Takafuji et al., 2020a), possibly by an miRNA-mediated inhibition of CREB/PGC1β pathway in osteoclast precursors (Takafuji et al., 2020b). Oxidative stress has been shown to modify miRNA contents of Myo-EVs; miR-34a is elevated in C2C12 derived EVs following oxidative stress. Muscle EVs enriched in miR-34a home to the bone and induce senescence of bone marrow-derived stem cells by decreasing Sirtuin1 expression on primary bone marrow cells (Fulzele et al., 2019).
In metabolic abnormalities like diabetes and insulin resistance, paracrine and/or endocrine effects of Myo-EVs have been reported. A study revealed that the pancreas takes up muscle EVs in vivo. Such EVs transferred muscle specific miRNAs to pancreatic beta cells and islets, thereby, modulating gene expression and proliferation (demonstrated in vitro with MIN6B1 pancreatic cell line). In this study, muscle EVs derived from insulin resistant skeletal muscle were particularly enriched in miR-16 cargo that could be transferred to pancreatic cells and regulate PTCH1, potentially contributing to the pathogenesis of type-2 diabetes (Jalabert et al., 2016).
As positive regulators of metabolic function, exercise-induced skeletal muscle exosomes potentially promote adipocyte lipolysis. IL-15 is known to be highly expressed in skeletal muscle, and acts to decrease fat depots in adipose tissue (Quinn et al., 2005). Additionally, myonectin, a recently established myokine involved in lipid metabolism has been identified as a link between skeletal muscle and systemic lipid metabolism (Seldin and Wong, 2012). Considering the abundance of IL-15 mRNA in the contracting skeletal muscle (Nielsen et al., 2007) and the exercise-induced release of muscle IL-6 in vesicular structures (Lauritzen et al., 2013), it is not far-fetched to speculate the packaging and cargo of this lipolysis-influencing myokine in skeletal muscle derived exosomes. Alterations in skeletal muscle (e.g., muscle activity and injury) have been shown to influence the release of key microRNAs (e.g., miR-21, miR-148b, and miR-486 in tissues, plasma and exosomes) that regulate insulin responsiveness (D'Souza et al., 2018).
Cardiomyocyte Derived EVs
Although not classically described as typical secretory cells, cardiac muscle cells can be induced to release EVs, in vitro (Gupta and Knowlton, 2007; Chistiakov et al., 2016). Cardiomyocytes, which constitute the prime contractile cell type in cardiac tissue, display augmented secretions of EVs under several cellular stress conditions like inflammation, injury, hypoxia, alcohol exposure and glucose starvation (Garcia et al., 2015; Yu and Wang, 2019). Similar to skeletal muscles, cardiac muscle EVs cargo nucleic acids, including miRNAs that can regulate gene expression in recipient cells (Waldenström et al., 2012).
Oxygen and nutrient supply are imperative for the efficient function of the myocardium. As such, blood vessels feeding the myocardium play a key role in cardiac function. The crosstalk between cardiac myocytes and intra-cardiac endothelial cells has been established. This crosstalk is vital to meet the metabolic needs for efficient heart function (Garcia et al., 2016) and has been found to be mediated by paracrine signal molecules, direct cell-cell communication, and EVs (Colliva et al., 2020). In the light of cardiomyocyte-endothelial cell communication, cardiomyocyte-derived EVs effect both anti-angiogenic and pro-angiogenic regulatory functions (Ribeiro et al., 2013; Wang et al., 2014; Chistiakov et al., 2016). Heat shock proteins (Hsps) well-known to protect cardiomyocytes from stresses (Willis and Patterson, 2010; Edwards et al., 2011; Yu and Wang, 2019; Yu et al., 2019) are highly expressed in muscle cells and secreted from cardiomyocytes via exosomes. Particularly, cardiomyocyte Hsp20 released through exosomes by the non-classical endoplasmic pathway promote angiogenesis; enhanced proliferation, migration, and tube formation of endothelial cells by Hsp20 via the activation of vascular endothelial growth factor receptor (VEGFR) signaling cascade (Zhang et al., 2012). The crucial role of cardiac microvascular endothelial (CME) cells in protecting against myocardial injury by way of activating endothelial nitric oxide synthase (eNOS) has been evidently linked to signals from cardiomyocytes. A study reported that, cardiomyocytes regulated CME cells via EV transfer of long intergenic non-protein coding RNA, Regulator of Reprogramming (Linc-ROR) to target miR-145-5p, ultimately resulting in the activation of eNOS pathway (Chen et al., 2020). Conversely, anti-angiogenic pathways are mediated by cardiomyocyte-derived EVs and contribute to endothelial dysfunction, insufficient myocardial angiogenesis and cardiovascular complications (Wang et al., 2014). Myocyte-derived exosomes from type-2 diabetic rats but not wild type inhibited proliferation, migration, and tube formation of cardiac endothelial cells in culture through miRNA regulation. It was observed that diabetes induced the release and transfer of exosomes harboring high levels of miR-320 (while being less enriched in miR-126) that target angiogenic factors (Hsp20 and insulin-like growth factor-1) in endothelial cells (Wang et al., 2009, 2014), potentially contributing to diabetes-related vascular dysfunction.
Cardiac remodeling is crucial in the pathophysiology of cardiac injury and hence, should be appropriately regulated to prevent irreversible changes to the structure and function of the myocardium (Tracy et al., 2020). Cardiac muscle exosomes play a key role in regulating myocardial remodeling by the transfer of encapsulated miRNAs to target fibrotic factors (Chaturvedi et al., 2015; Chistiakov et al., 2016). A study revealed that miR-133a from cardiomyocyte exosomes targeted type IA1 collagen (Col1A1) and connective tissue growth factor, resulting in decreased myocardial fibrosis in hypertensive rats (Castoldi et al., 2012). Other cardiomyocyte-derived exosome miRNAs that have been identified to promote fibrosis and fibroblast differentiation in animal models include miR-208a (Yang et al., 2018), miR-92a (Wang et al., 2020) and miR-195 (Morelli et al., 2019). While a number of exosome-derived miRNAs contribute to myocardial fibrosis, others, such as miR-378 tend to exhibit a protective effect by targeting mitogen-activated protein kinase kinase 6 (MKK6) to suppress p38 mitogen-activated protein kinase phosphorylation in cardiac fibroblasts (Yuan et al., 2018). Other miRNAs implicated in regulating the fibrotic cascades in this regard include miR-29b (Chaturvedi et al., 2015) and miR-373 (Xuan et al., 2019).
Recently, the roles of cardiomyocyte-derived exosomes have expanded to encompass a role in mechanisms that underlie the therapeutic efficacy of stem cells in myocardial infarction (Hu et al., 2018). Cardiomyocyte exosomes obtained from culture conditioned medium under stress microenvironment (peroxide-induced oxidative stress) hastened stress-induced injury of bone marrow-derived stem cells (Hu et al., 2018).
Smooth Muscle–Derived EVs
Vascular smooth muscles in a synthetic or proliferative, non-contractile state exhibit an increased production of extracellular vesicles (Kapustin and Shanahan, 2016; Schurgers et al., 2018), demonstrated by the inverse relationship between the expression of contractile vascular smooth muscle cell markers and exosome secretion (Kapustin et al., 2015). Inflammatory cytokines and growth factors like tumor necrosis factor-α (TNF-α) and platelet-derived growth factor (PDGF), respectively stimulate the phenotypic transition and exosome secretion by vascular smooth muscle cells (Kapustin and Shanahan, 2016). The activation of vascular smooth muscle cells (SMC) form one of the key events in atherogenesis and the onset of vascular complications, such as stroke and myocardial infarction (Bennett et al., 2016). Overexpression of Krüppel-like factor 5 (KLF5), a transcription factor requisite for mediating SMC proliferation and migration in vascular remodeling events, mediates the secretion of exosomes enriched on miR-155 from human smooth muscle cells. The transfer and uptake of SMC-derived exosomal miR-155 by endothelial cells have been shown to impair endothelial integrity by suppressing their proliferation, migration, tube formation (angiogenesis), and expression of tight junction proteins (Zheng et al., 2017).
Recently, smooth muscles of the pulmonary vasculature have been shown to release EVs that package a myriad of RNA transcripts transferrable to pulmonary arterial endothelial cells (de la Cuesta et al., 2019). Migration and apoptosis of pulmonary arterial smooth muscle cells account for the modulation of miR-143. As a result, exosomes derived from these cells selectively pack abundant miR-143-3p that exhibit pro-angiogenic and pro-migratory effects on recipient pulmonary arterial epithelial cells (Deng et al., 2015). In another recent finding, pulmonary arterial epithelial cells cultured with exosomes derived from platelet-derived growth factor-stimulated smooth muscle cells showed an enhanced migratory but not proliferative ability via the effect of exosomal miRNAs. It was observed that exosomes from PDGF-stimulated smooth muscle cells were deficient in miR-182, miR-1246, and miR-486 and this alteration proved critical for the enhanced migratory phenotype of endothelial cells in pathologic states (Heo et al., 2020).
The microenvironment or matrix of the vasculature is to a large extent regulated by microvesicles secreted from muscle cells, endothelial cells, and infiltrating immune cells of blood vessels (Reynolds et al., 2004; New et al., 2013). Small EVs secreted by vascular smooth muscle cells normally cargo various factors like the high affinity calcium ion-binding matrix Gla protein (MGP) and fetuin-A that function to inhibit calcification, thus protecting against vascular mineralization and the formation of atherosclerotic plagues (Reynolds et al., 2005). However, inflammation and mineral imbalance influence this protective role by inducing the release of EVs enriched in calcification promoters and depleted of inhibitors, thus, conditioning an environment conducive for vascular mineralization and stiffening (Reynolds et al., 2005; Kapustin et al., 2011).
Emergence of Irisin Signaling and Potential Implications for Microenvironment Remodeling
Irisin, a recent discovery to the family of adipomyokines (exerting its effect on both adipose and muscle tissues) is a thermogenic protein thought to be vital in energy metabolism (Boström et al., 2012; Rodríguez et al., 2017). Irisin has been proposed to bridge the communication between the muscle and other tissues of the body, and therefore has gained much attention in research relating to metabolism and tissue crosstalk (Pukajło et al., 2015). Irisin is a fragment of the fibronectin type III domain-containing protein 5 (FNDC5) on the cell membrane of myocytes, adipocytes, and other cell types (liver, brain, stomach, etc.) (Aydin et al., 2014). The FNDC5 protein has a cytoplasmic C-terminal portion and an N-terminal extracellular portion that is proteolytically cleaved and released into circulation as irisin (Panati et al., 2018). Physical activity, especially high intensity exercise and resistance training have been shown to increase the levels of irisin in circulation (Tsuchiya et al., 2014, 2015). Although the specific receptor for irisin was yet to be identified until recently, the pivotal study by Spiegelman and colleagues has demonstrated that irisin binds to integrins (Kim H. et al., 2018). Integrin-ligand interactions activate several downstream signaling pathways that regulate cellular processes (Figures 2, 3). We have previously reviewed and discussed the emergence of irisin and integrin-ligand interactions in the context of cancer, metabolic disorders, and inflammation (Park et al., 2020). Irisin binds to several integrins, such as αVβ5 and α5β1 on bone and fat cells, however, binding affinity seems to be higher with the αV family of integrins (Kim H. et al., 2018). Irisin increases the expression of thermogenin (UPC-1, a mitochondrial protein in adipose tissue lipid droplets) in matured fat cells and facilitates browning of white adipose tissue (WAT), leading to the formation of beige-adipose tissue. Although not directly inducing browning, irisin inhibits the formation of new adipose cells, and thus, negatively regulates adipogenesis via irisin-induced phosphorylation of mitogen-activated protein kinases (Zhang et al., 2014; Korta et al., 2019). In vitro studies suggest that irisin plays a protective anti-inflammatory role by suppressing the production of pro-inflammatory cytokines in fat cells and immune cells, and therefore alleviates obesity-induced inflammation. Adipocytes are sensitive to irisin and tend to release relatively low levels of IL-6, IL-1β, monocyte chemotactic protein 1 (MCP 1), and TNF-α under the effect of irisin in vitro, potentially via the regulation of downstream signaling of Toll-like receptor 4/myeloid differentiation primary response 88 (TLR4/MyD88) (Mazur-Bialy et al., 2017a,b) (Figure 2).
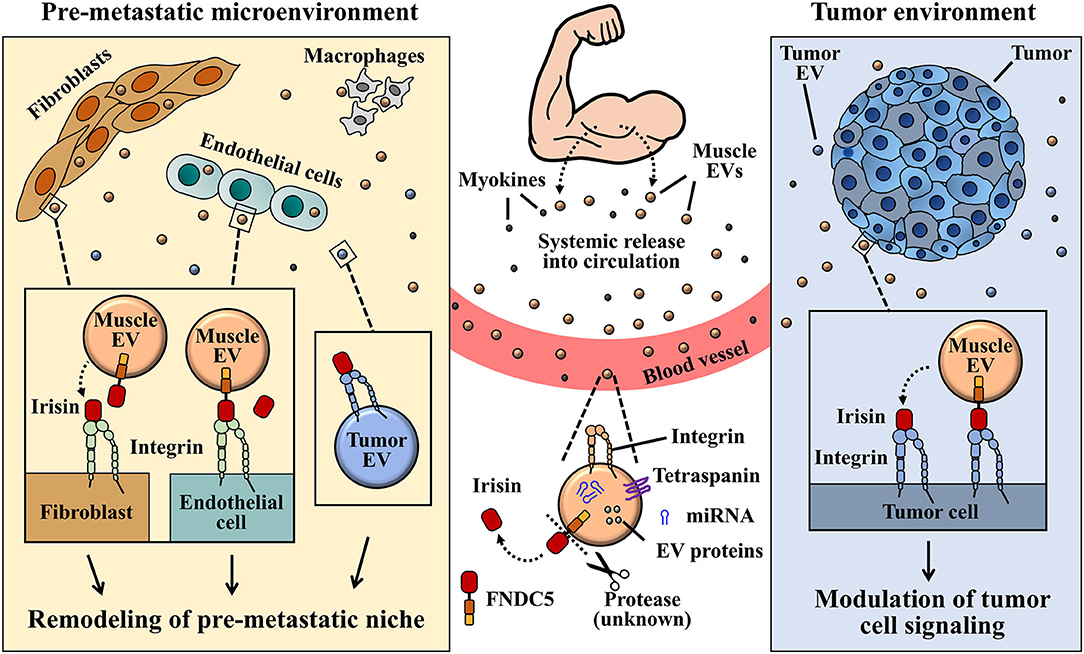
Figure 3. A graphical illustration proposed for the interaction between muscle EV irisin and integrin, and its role in pre-metastatic tumor environment remodeling. Circulatory or EV-bound irisin derived from muscle cells can potentially bind to integrin receptors expressed on several tumor cells or tumor-derived EVs. EVs and irisin released by muscles into circulation, upon reaching pre-metastatic microenvironment, may engage tumor-derived EVs via EV-bound irisin/EV integrin and/or free-irisin/EV integrin interactions. Signaling from integrin-irisin interaction in tumor microenvironment could mediate functional regulation of tumor-derived exosomes in the remodeling of pre-metastatic microenvironment.
Aside adipocytes, bone cells (osteocytes) have been identified to be sensitive to irisin, and thus, irisin partly plays a role in bone remodeling. Upon irisin treatment, osteocytes under oxidative stress conditions show a reduced ratio of apoptotic cells in culture (Kim H. et al., 2018). Irisin directly regulates osteoclast function and differentiation by stimulating the release of osteoclastogenic promoters like RANKL and sclerostin, even at normal circulatory concentrations (Estell et al., 2020). Earlier studies had also identified osteoblasts as direct targets of irisin; an enhancer of osteoblast differentiation (Colaianni et al., 2014). Although irisin dually targets osteocytes and osteoblasts, the effect of irisin on different bone cell types greatly depends on the circulatory concentrations of the protein, since varying concentrations of irisin treatment have demonstrated contrasting effects in in vitro studies (Colaianni et al., 2014; Estell et al., 2020). Further studies in this area are needed to clearly understand the mechanisms by which irisin mediates bone metabolism.
Recently, the involvement of irisin in inflammatory disease has been demonstrated, particularly with intestinal inflammation. Binding of irisin to αVβ5 integrin units on intestinal epithelial cells have been shown to alleviate epithelial barrier dysfunction during gut injury. Irisin restored epithelial barrier function via the activation of αVβ5/AMPK-UCP 2 signaling pathway, while reducing oxidative stress and apoptosis in enterocytes (Bi et al., 2020). Taken together, irisin is a physiologically beneficial adipomyokine that possess properties contributing to oxidative stress alleviation (Bi et al., 2020), anti-inflammation (Mazur-Bialy et al., 2017a,b; Xiong et al., 2018), and anti-metastatic effects (Rabiee et al., 2020). It is worth mentioning that, there are a few conditions in which irisin has been demonstrated to promote inflammation. This is particularly shown in hepatocellular carcinoma (HCC) whereby, the increased hepatic mRNA levels of FNDC5/irisin in HCC patients correlated with increased expression of proinflammatory markers, such as IL-6 and TNF-α. However, mechanisms underlying the irisin-induced inflammation in hepatic carcinoma remains to be completely understood (Gaggini et al., 2017).
Exosomal Integrin-Irisin Signaling (Potential Implication for Cancer Metastasis)
The discovery of integrins belonging to the αV family as receptors for irisin signaling (Kim H. et al., 2018) has been a great leap toward understanding how irisin could regulate metabolism of both host and tumor cells, considering that, integrins are ubiquitously expressed. Although irisin has not yet been identified as a cargo content of exosomes released by muscle and adipose cells, circulating irisin may engage integrin receptors on exosomes to mediate various niche pre-conditioning (Figure 3). Taking into consideration that exosomes express and transport integrins (identified receptor for irisin) on their surfaces, the potential “cargoing” of irisin in muscle-derived exosomes can be proposed in a few ways. One of such possibilities lies in the endocytosis of surface proteins on muscle cells during EV biogenesis, leading to the formation of MVBs that may package membrane-bound integrin-irisin complexes (Figure 1). This mechanism has been proposed for the exosomal packaging of ECM proteins, such as fibronectin (Sung et al., 2015).
The expression of αV integrins is highly upregulated in several cancers and tends to augment the metastatic phenotype of tumor cells, thus promoting their migration (Teti et al., 2002; McCabe et al., 2007; Haeger et al., 2020). Expression of integrins is not confined to cancer cells only; exosomes obtained from tumors contain a myriad of functional tumor-derived integrins that precondition microenvironments for organ-specific metastasis (Hoshino et al., 2015). Tumor-derived exosomes are capable of transferring exosomal integrins to tumorigenic and non-tumorigenic cells. For example, Fedele et al. demonstrated that αVβ6 which is highly expressed on prostate cancer cells was transferable among different subsets of prostate cancer cell lines via their exosomes. Prostate cancer cell-derived exosomes which expressed functional integrin αVβ6 were taken up by recipient prostate cancer cells that subsequently re-expressed the integrin on their surfaces (Fedele et al., 2015). This group also showed that another member of the αV integrin family, αVβ3, was transferred from tumorigenic to non-tumorigenic prostate epithelial cells via exosomes and induced functional changes like enhanced migration and adhesion in recipient cells (Singh et al., 2016). Other studies confirm the in vitro transfer of exosomal αVβ3 integrin to β3-negative cells resulting in the acquisition of ligand binding activity (Krishn et al., 2019). Indeed, integrins expressed on the surface of exosomes are able to bind and adhere to cell surface, or ECM proteins, such as collagen and fibronectin, thus, support integrin binding-mediated cell adhesion (Park et al., 2019b; Altei et al., 2020).
Although the particular role of irisin in cancer progression still remains to be elucidated, serum levels of irisin is markedly altered in several cancers (Zhang et al., 2018). However, there are contrasting reports on the effects of irisin in association with cells. For example, irisin was found to suppress the viability, proliferation and migration of some malignant breast cancer cells (MCF-7 and MDA-MB-231, by way of induced apoptosis) but not others like MCF-10A breast epithelial cells (Gannon et al., 2015). Additionally, irisin signaling has been found to inhibit the proliferation, migration, and invasion of lung cancer (Shao et al., 2017). This effect results from the irisin-induced inhibition of Snail transcription factors via the PI3K/AKT pathway, resulting in the reduced expression of epithelial-mesenchymal transition (EMT) markers (Shao et al., 2017) (Figure 2). Nonetheless, these findings point to the potentially protective and organ specific role played by irisin in cancer progression and metastasis (Zhang et al., 2018).
Taken together, the evidence supporting exosomal carriage of functional and transferable integrin proteins, such as those belonging to the αV family (Fedele et al., 2015; Singh et al., 2016; Krishn et al., 2019), and the identification of integrins as signaling receptors (Kim H. et al., 2018) propose irisin-exosome interactions as potential players in niche regulation especially in cancer metastasis (Figures 1–3). Irisin may negatively impact cancer progression and metastasis indirectly via their interaction with tumor-derived exosomes. Extracellular vesicles designed to precondition distant microenvironment for organotropic metastasis could potentially be altered by the activation of integrin-irisin signaling cascades (Figures 2, 3). Further studies in the area of irisin-exosomal integrin interaction is warranted to ascertain molecular mechanisms that underlie the remodeling of microenvironment and tumor metastasis. Muscle-derived myokines and EVs may hold promising contributions toward therapeutic breakthroughs in cancer management.
Perspective
With the growing evidence of their involvement in the autocrine/paracrine/endocrine regulation of metabolic pathways (Seldin and Wong, 2012) and inflammation (Febbraio and Pedersen, 2005), muscle cells and muscle cell-derived EVs deserve attention in our quest to demystify pathophysiological processes underlying various health condition, including cancers. Muscle-derived EVs that cargo myokines and other biologic modulators (nucleic acids, growth factors, etc.) could potentially modulate the remodeling of niches in vital sites, such as liver and adipose tissues. The anti-inflammatory effect of skeletal muscle activity mediated by myokines (e.g., IL-6) could negatively contribute to the establishment of favorable inflammatory niche conditions, necessary for cancer metastasis. Although more studies to support exosomal packaging and release of important myokines like irisin, IL-6, and myonectin are desired, the growing evidence of muscle EV secretion and remarkable encapsulation of muscle-specific biologic contents (transferable to various cell types and also implicated in cancer progression) (Singh et al., 2016; Wang et al., 2016; Li et al., 2018; Xu X. et al., 2018; Krishn et al., 2019) provide substantial cues for further investigations. Metabolic syndrome (including obesity, hyperlipidemia and diabetes) burden organs with pro-tumorigenic and metastatic cascades associated with chronic inflammation, increased oxidative stress, and deregulated cellular signals (Harvey et al., 2011), and therefore, potentially render tissues niche-ready for cancer metastasis. On one end, because muscle and muscle-derived EVs pack mediators that restitute the effects of metabolic dysregulation, their indirect attribution to the regulation of microenvironment remodeling and the establishment of pre-metastatic homing niches can be hypothesized. Little evidence exists to explain the relationship between muscle-derived EVs and tumor metastasis, however, findings from other pathologies, such as inflammation and metabolic disorders discussed in this review point out a plausible connection that may potentially benefit homeostatic regulation of homing niches against tumor progression metastasis. Further studies are imperative to better understand the role of muscle-derived exosomes in regulating the events of pre-metastatic niches and cancer progression. This may provide novel insights to the development of therapeutic interventions for the management of cancers.
Author Contributions
SD and MS contributed to the conceptualization, scope, and outline of this review. SD, EJP, PKM, AI, MGA, GO, EK, and MS analyzed the referenced manuscripts in this manuscript and participated in preparing the manuscript. All authors read and approved the final version.
Funding
This work was supported by the JSPS KAKENHI Grants (EJP, 19K09392; AI, 19K18210; EK, 18K08917 and 19KK0224; and MS, 18H02622 and 19KK0196).
Conflict of Interest
The authors declare that the research was conducted in the absence of any commercial or financial relationships that could be construed as a potential conflict of interest.
References
Abels, E. R., and Breakefield, X. O. (2016). Introduction to extracellular vesicles: biogenesis, RNA cargo selection, content, release, and uptake. Cell Mol. Neurobiol. 36, 301–312. doi: 10.1007/s10571-016-0366-z
Akers, J. C., Gonda, D., Kim, R., Carter, B. S., and Chen, C. C. (2013). Biogenesis of extracellular vesicles (EV): exosomes, microvesicles, retrovirus-like vesicles, and apoptotic bodies. J. Neurooncol. 113, 1–11. doi: 10.1007/s11060-013-1084-8
Alberti, K. G., Eckel, R. H., Grundy, S. M., Zimmet, P. Z., Cleeman, J. I., Donato, K. A., et al. (2009). Harmonizing the metabolic syndrome: a joint interim statement of the International Diabetes Federation Task Force on Epidemiology and Prevention; National Heart, Lung, and Blood Institute; American Heart Association; World Heart Federation; International Atherosclerosis Society; and International Association for the Study of Obesity. Circulation 120, 1640–1645. doi: 10.1161/CIRCULATIONAHA.109.192644
Altei, W. F., Pachane, B. C., Dos Santos, P. K., Ribeiro, L. N. M., Sung, B. H., Weaver, A. M., et al. (2020). Inhibition of αvβ3 integrin impairs adhesion and uptake of tumor-derived small extracellular vesicles. Cell Commun Signal. 18:158. doi: 10.1186/s12964-020-00630-w
Aydin, S., Kuloglu, T., Kalayci, M., Yilmaz, M., Cakmak, T., Albayrak, S., et al. (2014). A comprehensive immunohistochemical examination of the distribution of the fat-burning protein irisin in biological tissues. Peptides 61, 130–136. doi: 10.1016/j.peptides.2014.09.014
Barone, R., Macaluso, F., Sangiorgi, C., Campanella, C., Marino Gammazza, A., Moresi, V., et al. (2016). Skeletal muscle Heat shock protein 60 increases after endurance training and induces peroxisome proliferator-activated receptor gamma coactivator 1 α1 expression. Sci Rep. 6:19781. doi: 10.1038/srep19781
Bennett, M. R., Sinha, S., and Owens, G. K. (2016). Vascular smooth muscle cells in atherosclerosis. Circ Res. 118, 692–702. doi: 10.1161/CIRCRESAHA.115.306361
Bergsmedh, A., Szeles, A., Henriksson, M., Bratt, A., Folkman, M. J., Spetz, A. L., et al. (2001). Horizontal transfer of oncogenes by uptake of apoptotic bodies. Proc. Natl. Acad. Sci. U.S.A. 98, 6407–6411. doi: 10.1073/pnas.101129998
Berlin, C., Bargatze, R. F., Campbell, J. J., von Andrian, U. H., Szabo, M. C., Hasslen, S. R., et al. (1995). alpha 4 integrins mediate lymphocyte attachment and rolling under physiologic flow. Cell 80, 413–422. doi: 10.1016/0092-8674(95)90491-3
Bi, J., Zhang, J., Ren, Y., Du, Z., Li, T., Wang, T., et al. (2020). Irisin reverses intestinal epithelial barrier dysfunction during intestinal injury via binding to the integrin αVβ5 receptor. J. Cell. Mol. Med. 24, 996–1009. doi: 10.1111/jcmm.14811
Boström, P., Wu, J., Jedrychowski, M. P., Korde, A., Ye, L., Lo, J. C., et al. (2012). A PGC1-α-dependent myokine that drives brown-fat-like development of white fat and thermogenesis. Nature 481, 463–468. doi: 10.1038/nature10777
Castoldi, G., Di Gioia, C. R., Bombardi, C., Catalucci, D., Corradi, B., Gualazzi, M. G., et al. (2012). MiR-133a regulates collagen 1A1: potential role of miR-133a in myocardial fibrosis in angiotensin II-dependent hypertension. J. Cell Physiol. 227, 850–856. doi: 10.1002/jcp.22939
Chaturvedi, P., Kalani, A., Medina, I., Familtseva, A., and Tyagi, S. C. (2015). Cardiosome mediated regulation of MMP9 in diabetic heart: role of mir29b and mir455 in exercise. J. Cell. Mol. Med. 19, 2153–2161. doi: 10.1111/jcmm.12589
Chen, G., Xu, C., Gillette, T. G., Huang, T., Huang, P., Li, Q., et al. (2020). Cardiomyocyte-derived small extracellular vesicles can signal eNOS activation in cardiac microvascular endothelial cells to protect against ischemia/reperfusion injury. Theranostics 10, 11754–11774. doi: 10.7150/thno.43163
Chistiakov, D. A., Orekhov, A. N., and Bobryshev, Y. V. (2016). Cardiac extracellular vesicles in normal and infarcted heart. Int. J. Mol. Sci. 17:63. doi: 10.3390/ijms17010063
Choi, J. S., Yoon, H. I., Lee, K. S., Choi, Y. C., Yang, S. H., Kim, I. S., et al. (2016). Exosomes from differentiating human skeletal muscle cells trigger myogenesis of stem cells and provide biochemical cues for skeletal muscle regeneration. J. Control Release 222, 107–115. doi: 10.1016/j.jconrel.2015.12.018
Cocucci, E., Racchetti, G., and Meldolesi, J. (2009). Shedding microvesicles: artefacts no more. Trends Cell Biol. 19, 43–51. doi: 10.1016/j.tcb.2008.11.003
Colaianni, G., Cuscito, C., Mongelli, T., Oranger, A., Mori, G., Brunetti, G., et al. (2014). Irisin enhances osteoblast differentiation in vitro. Int. J. Endocrinol. 2014:902186. doi: 10.1155/2014/902186
Colliva, A., Braga, L., Giacca, M., and Zacchigna, S. (2020). Endothelial cell-cardiomyocyte crosstalk in heart development and disease. J. Physiol. 598, 2923–2939. doi: 10.1113/JP276758
Colombo, M., Moita, C., van Niel, G., Kowal, J., Vigneron, J., Benaroch, P., et al. (2013). Analysis of ESCRT functions in exosome biogenesis, composition and secretion highlights the heterogeneity of extracellular vesicles. J. Cell Sci. 126, 5553–5565. doi: 10.1242/jcs.128868
Corti, S., Locatelli, F., Papadimitriou, D., Donadoni, C., Del Bo, R., Fortunato, F., et al. (2005). Multipotentiality, homing properties, and pyramidal neurogenesis of CNS-derived LeX(ssea-1)+/CXCR4+ stem cells. FASEB J. 19, 1860–1862. doi: 10.1096/fj.05-4170fje
De Angel, R. E., Conti, C. J., Wheatley, K. E., Brenner, A. J., Otto, G., Degraffenried, L. A., et al. (2013). The enhancing effects of obesity on mammary tumor growth and Akt/mTOR pathway activation persist after weight loss and are reversed by RAD001. Mol. Carcinog. 52, 446–458. doi: 10.1002/mc.21878
de la Cuesta, F., Passalacqua, I., Rodor, J., Bhushan, R., Denby, L., and Baker, A. H. (2019). Extracellular vesicle cross-talk between pulmonary artery smooth muscle cells and endothelium during excessive TGF-β signalling: implications for PAH vascular remodelling. Cell Commun. Signal. 17:143. doi: 10.1186/s12964-019-0449-9
Deng, L., Blanco, F. J., Stevens, H., Lu, R., Caudrillier, A., McBride, M., et al. (2015). MicroRNA-143 activation regulates smooth muscle and endothelial cell crosstalk in pulmonary arterial hypertension. Circ. Res. 117, 870–883. doi: 10.1161/CIRCRESAHA.115.306806
Deshmukh, A. S., Murgia, M., Nagaraj, N., Treebak, J. T., Cox, J., and Mann, M. (2015). Deep proteomics of mouse skeletal muscle enables quantitation of protein isoforms, metabolic pathways, and transcription factors. Mol. Cell Proteomics 14, 841–853. doi: 10.1074/mcp.M114.044222
D'Souza, R. F., Woodhead, J. S. T., Zeng, N., Blenkiron, C., Merry, T. L., Cameron-Smith, D., et al. (2018). Circulatory exosomal miRNA following intense exercise is unrelated to muscle and plasma miRNA abundances. Am. J. Physiol. Endocrinol. Metab. 315, E723–E733. doi: 10.1152/ajpendo.00138.2018
Edwards, H. V., Cameron, R. T., and Baillie, G. S. (2011). The emerging role of HSP20 as a multifunctional protective agent. Cell Signal. 23, 1447–1454. doi: 10.1016/j.cellsig.2011.05.009
Esposito, K., Chiodini, P., Colao, A., Lenzi, A., and Giugliano, D. (2012). Metabolic syndrome and risk of cancer: a systematic review and meta-analysis. Diabetes Care 35, 2402–2411. doi: 10.2337/dc12-0336
Estell, E. G., Le, P. T., Vegting, Y., Kim, H., Wrann, C., Bouxsein, M. L., et al. (2020). Irisin directly stimulates osteoclastogenesis and bone resorption in vitro and in vivo. Elife 9:e58172. doi: 10.7554/eLife.58172
Febbraio, M. A., and Pedersen, B. K. (2005). Contraction-induced myokine production and release: is skeletal muscle an endocrine organ? Exerc. Sport Sci. Rev. 33, 114–119. doi: 10.1097/00003677-200507000-00003
Fedele, C., Singh, A., Zerlanko, B. J., Iozzo, R. V., and Languino, L. R. (2015). The αvβ6 integrin is transferred intercellularly via exosomes. J. Biol. Chem. 290, 4545–4551. doi: 10.1074/jbc.C114.617662
Festa, A., D'Agostino, R., Howard, G., Mykkänen, L., Tracy, R. P., and Haffner, S. M. (2000). Chronic subclinical inflammation as part of the insulin resistance syndrome: the Insulin Resistance Atherosclerosis Study (IRAS). Circulation 102, 42–47. doi: 10.1161/01.CIR.102.1.42
Forterre, A., Jalabert, A., Berger, E., Baudet, M., Chikh, K., Errazuriz, E., et al. (2014b). Proteomic analysis of C2C12 myoblast and myotube exosome-like vesicles: a new paradigm for myoblast-myotube cross talk? PLoS ONE 9:e84153. doi: 10.1371/journal.pone.0084153
Forterre, A., Jalabert, A., Chikh, K., Pesenti, S., Euthine, V., Granjon, A., et al. (2014a). Myotube-derived exosomal miRNAs downregulate Sirtuin1 in myoblasts during muscle cell differentiation. Cell Cycle 13, 78–89. doi: 10.4161/cc.26808
Frenette, P. S., Mayadas, T. N., Rayburn, H., Hynes, R. O., and Wagner, D. D. (1996). Susceptibility to infection and altered hematopoiesis in mice deficient in both P- and E-selectins. Cell 84, 563–574. doi: 10.1016/S0092-8674(00)81032-6
Frühbeis, C., Fröhlich, D., Kuo, W. P., Amphornrat, J., Thilemann, S., Saab, A. S., et al. (2013). Neurotransmitter-triggered transfer of exosomes mediates oligodendrocyte-neuron communication. PLoS Biol. 11:e1001604. doi: 10.1371/journal.pbio.1001604
Frühbeis, C., Helmig, S., Tug, S., Simon, P., and Krämer-Albers, E. M. (2015). Physical exercise induces rapid release of small extracellular vesicles into the circulation. J. Extracell. Vesicles 4:28239. doi: 10.3402/jev.v4.28239
Fulzele, S., Mendhe, B., Khayrullin, A., Johnson, M., Kaiser, H., Liu, Y., et al. (2019). Muscle-derived miR-34a increases with age in circulating extracellular vesicles and induces senescence of bone marrow stem cells. Aging 11, 1791–1803. doi: 10.18632/aging.101874
Gaggini, M., Cabiati, M., Del Turco, S., Navarra, T., De Simone, P., Filipponi, F., et al. (2017). Increased FNDC5/Irisin expression in human hepatocellular carcinoma. Peptides 88, 62–66. doi: 10.1016/j.peptides.2016.12.014
Gannon, N. P., Vaughan, R. A., Garcia-Smith, R., Bisoffi, M., and Trujillo, K. A. (2015). Effects of the exercise-inducible myokine irisin on malignant and non-malignant breast epithelial cell behavior in vitro. Int. J. Cancer. 136, E197–E202. doi: 10.1002/ijc.29142
Garcia, N. A., Moncayo-Arlandi, J., Sepulveda, P., and Diez-Juan, A. (2016). Cardiomyocyte exosomes regulate glycolytic flux in endothelium by direct transfer of GLUT transporters and glycolytic enzymes. Cardiovasc. Res. 109, 397–408. doi: 10.1093/cvr/cvv260
Garcia, N. A., Ontoria-Oviedo, I., González-King, H., Diez-Juan, A., and Sepúlveda, P. (2015). Glucose starvation in cardiomyocytes enhances exosome secretion and promotes angiogenesis in endothelial cells. PLoS ONE 10:e0138849. doi: 10.1371/journal.pone.0138849
Garzón-Muvdi, T., and Quiñones-Hinojosa, A. (2009). Neural stem cell niches and homing: recruitment and integration into functional tissues. ILAR J. 51, 3–23. doi: 10.1093/ilar.51.1.3
Golbidi, S., Mesdaghinia, A., and Laher, I. (2012). Exercise in the metabolic syndrome. Oxid. Med. Cell Longev. 2012:349710. doi: 10.1155/2012/349710
Gupta, S., and Knowlton, A. A. (2007). HSP60 trafficking in adult cardiac myocytes: role of the exosomal pathway. Am. J. Physiol. Heart Circ. Physiol. 292, H3052–H3056. doi: 10.1152/ajpheart.01355.2006
Gustat, J., Srinivasan, S. R., Elkasabany, A., and Berenson, G. S. (2002). Relation of self-rated measures of physical activity to multiple risk factors of insulin resistance syndrome in young adults: the Bogalusa Heart Study. J. Clin. Epidemiol. 55, 997–1006. doi: 10.1016/S0895-4356(02)00427-4
Haeger, A., Alexander, S., Vullings, M., Kaiser, F. M. P., Veelken, C., Flucke, U., et al. (2020). Collective cancer invasion forms an integrin-dependent radioresistant niche. J. Exp. Med. 217:e20181184. doi: 10.1084/jem.20181184
Hagiwara, K., Ochiya, T., and Kosaka, N. (2014). A paradigm shift for extracellular vesicles as small RNA carriers: from cellular waste elimination to therapeutic applications. Drug Deliv. Transl. Res. 4, 31–37. doi: 10.1007/s13346-013-0180-9
Harvey, A. E., Lashinger, L. M., and Hursting, S. D. (2011). The growing challenge of obesity and cancer: an inflammatory issue. Ann. N. Y. Acad. Sci. 1229, 45–52. doi: 10.1111/j.1749-6632.2011.06096.x
Henne, W. M., Stenmark, H., and Emr, S. D. (2013). Molecular mechanisms of the membrane sculpting ESCRT pathway. Cold Spring Harb. Perspect. Biol. 5:a016766. doi: 10.1101/cshperspect.a016766
Heo, J., Yang, H. C., Rhee, W. J., and Kang, H. (2020). Vascular smooth muscle cell-derived exosomal microRNAs regulate endothelial cell migration under PDGF stimulation. Cells 9:639. doi: 10.3390/cells9030639
Hirata, Y., Furuhashi, K., Ishii, H., Li, H. W., Pinho, S., Ding, L., et al. (2018). CD150(high) bone marrow tregs maintain hematopoietic stem cell quiescence and immune privilege via adenosine. Cell Stem Cell 22, 445–453 e5. doi: 10.1016/j.stem.2018.01.017
Hoshino, A., Costa-Silva, B., Shen, T. L., Rodrigues, G., Hashimoto, A., Tesic Mark, M., et al. (2015). Tumour exosome integrins determine organotropic metastasis. Nature 527, 329–335. doi: 10.1038/nature15756
Hu, M., Guo, G., Huang, Q., Cheng, C., Xu, R., Li, A., et al. (2018). The harsh microenvironment in infarcted heart accelerates transplanted bone marrow mesenchymal stem cells injury: the role of injured cardiomyocytes-derived exosomes. Cell Death Dis. 9:357. doi: 10.1038/s41419-018-0392-5
Hugel, B., Martínez, M. C., Kunzelmann, C., and Freyssinet, J. M. (2005). Membrane microparticles: two sides of the coin. Physiology 20, 22–27. doi: 10.1152/physiol.00029.2004
Huttenlocher, A., and Horwitz, A. R. (2011). Integrins in cell migration. Cold Spring Harb. Perspect. Biol. 3:a005074. doi: 10.1101/cshperspect.a005074
Imitola, J., Raddassi, K., Park, K. I., Mueller, F. J., Nieto, M., Teng, Y. D., et al. (2004). Directed migration of neural stem cells to sites of CNS injury by the stromal cell-derived factor 1alpha/CXC chemokine receptor 4 pathway. Proc. Natl. Acad. Sci. U.S.A. 101, 18117–18122. doi: 10.1073/pnas.0408258102
Isles, H. M., Herman, K. D., Robertson, A. L., Loynes, C. A., Prince, L. R., Elks, P. M., et al. (2019). The CXCL12/CXCR4 signaling axis retains neutrophils at inflammatory sites in zebrafish. Front. Immunol. 10:1784. doi: 10.3389/fimmu.2019.01784
Jalabert, A., Vial, G., Guay, C., Wiklander, O. P., Nordin, J. Z., Aswad, H., et al. (2016). Exosome-like vesicles released from lipid-induced insulin-resistant muscles modulate gene expression and proliferation of beta recipient cells in mice. Diabetologia 59, 1049–1058. doi: 10.1007/s00125-016-3882-y
Kalluri, R., and LeBleu, V. S. (2020). The biology, function, and biomedical applications of exosomes. Science 367:eaau6977. doi: 10.1126/science.aau6977
Kalra, H., Drummen, G. P., and Mathivanan, S. (2016). Focus on extracellular vesicles: introducing the next small big thing. Int. J. Mol. Sci. 17:170. doi: 10.3390/ijms17020170
Kapustin, A. N., Chatrou, M. L., Drozdov, I., Zheng, Y., Davidson, S. M., Soong, D., et al. (2015). Vascular smooth muscle cell calcification is mediated by regulated exosome secretion. Circ. Res. 116, 1312–1323. doi: 10.1161/CIRCRESAHA.116.305012
Kapustin, A. N., Davies, J. D., Reynolds, J. L., McNair, R., Jones, G. T., Sidibe, A., et al. (2011). Calcium regulates key components of vascular smooth muscle cell-derived matrix vesicles to enhance mineralization. Circ. Res. 109, e1–e12. doi: 10.1161/CIRCRESAHA.110.238808
Kapustin, A. N., and Shanahan, C. M. (2016). Emerging roles for vascular smooth muscle cell exosomes in calcification and coagulation. J. Physiol. 594, 2905–2914. doi: 10.1113/JP271340
Kawai, T., and Malech, H. L. (2009). WHIM syndrome: congenital immune deficiency disease. Curr. Opin. Hematol. 16, 20–26. doi: 10.1097/MOH.0b013e32831ac557
Kim, H., Wrann, C. D., Jedrychowski, M., Vidoni, S., Kitase, Y., Nagano, K., et al. (2018). Irisin mediates effects on bone and fat via αV integrin receptors. Cell 175, 1756–1768.e17. doi: 10.1016/j.cell.2018.10.025
Kim, S., Lee, M. J., Choi, J. Y., Park, D. H., Kwak, H. B., Moon, S., et al. (2018). Roles of exosome-like vesicles released from inflammatory C2C12 myotubes: regulation of myocyte differentiation and myokine expression. Cell Physiol. Biochem. 48, 1829–1842. doi: 10.1159/000492505
Kong, G., Jiang, Y., Sun, X., Cao, Z., Zhang, G., Zhao, Z., et al. (2017). Irisin reverses the IL-6 induced epithelial-mesenchymal transition in osteosarcoma cell migration and invasion through the STAT3/Snail signaling pathway. Oncol. Rep. 38, 2647–2656. doi: 10.3892/or.2017.5973
Korta, P., Pocheć, E., and Mazur-Biały, A. (2019). Irisin as a multifunctional protein: implications for health and certain diseases. Medicina 55:485. doi: 10.3390/medicina55080485
Krishn, S. R., Singh, A., Bowler, N., Duffy, A. N., Friedman, A., Fedele, C., et al. (2019). Prostate cancer sheds the αvβ3 integrin in vivo through exosomes. Matrix Biol. 77, 41–57. doi: 10.1016/j.matbio.2018.08.004
Lauritzen, H. P., Brandauer, J., Schjerling, P., Koh, H. J., Treebak, J. T., Hirshman, M. F., et al. (2013). Contraction and AICAR stimulate IL-6 vesicle depletion from skeletal muscle fibers in vivo. Diabetes 62, 3081–3092. doi: 10.2337/db12-1261
Leal, L. G., Lopes, M. A., and Batista, M. L. (2018). Physical exercise-induced myokines and muscle-adipose tissue crosstalk: a review of current knowledge and the implications for health and metabolic diseases. Front. Physiol. 9:1307. doi: 10.3389/fphys.2018.01307
Lee, Y., El Andaloussi, S., and Wood, M. J. (2012). Exosomes and microvesicles: extracellular vesicles for genetic information transfer and gene therapy. Hum. Mol. Genet. 21, R125–R134. doi: 10.1093/hmg/dds317
Li, C., Zheng, X., Li, W., Bai, F., Lyu, J., and Meng, Q. H. (2018). Serum miR-486-5p as a diagnostic marker in cervical cancer: with investigation of potential mechanisms. BMC Cancer 18:61. doi: 10.1186/s12885-017-3753-z
Liesveld, J. L., Sharma, N., and Aljitawi, O. S. (2020). Stem cell homing: from physiology to therapeutics. Stem Cells 38, 1241–1253. doi: 10.1002/stem.3242
Lucas, D. (2019). Leukocyte trafficking and regulation of murine hematopoietic stem cells and their niches. Front. Immunol. 10:387. doi: 10.3389/fimmu.2019.00387
Madison, R. D., McGee, C., Rawson, R., and Robinson, G. A. (2014). Extracellular vesicles from a muscle cell line (C2C12) enhance cell survival and neurite outgrowth of a motor neuron cell line (NSC-34). J. Extracell. Vesicles 3:22865. doi: 10.3402/jev.v3.22865
Madison, R. D., and Robinson, G. A. (2019). Muscle-derived extracellular vesicles influence motor neuron regeneration accuracy. Neuroscience 419, 46–59. doi: 10.1016/j.neuroscience.2019.08.028
Mazur-Bialy, A. I., Bilski, J., Pochec, E., and Brzozowski, T. (2017a). New insight into the direct anti-inflammatory activity of a myokine irisin against proinflammatory activation of adipocytes. Implication for exercise in obesity. J. Physiol. Pharmacol. 68, 243–251. Available online at: http://www.jpp.krakow.pl/journal/archive/04_17/pdf/243_04_17
Mazur-Bialy, A. I., Pocheć, E., and Zarawski, M. (2017b). Anti-inflammatory properties of irisin, mediator of physical activity, are connected with TLR4/MyD88 signaling pathway activation. Int. J. Mol. Sci. 18:701. doi: 10.3390/ijms18040701
McCabe, N. P., De, S., Vasanji, A., Brainard, J., and Byzova, T. V. (2007). Prostate cancer specific integrin alphavbeta3 modulates bone metastatic growth and tissue remodeling. Oncogene 26, 6238–6243. doi: 10.1038/sj.onc.1210429
McCullough, J., Fisher, R. D., Whitby, F. G., Sundquist, W. I., and Hill, C. P. (2008). ALIX-CHMP4 interactions in the human ESCRT pathway. Proc. Natl. Acad. Sci. U.S.A. 105, 7687–7691. doi: 10.1073/pnas.0801567105
Mika, A., Macaluso, F., Barone, R., Di Felice, V., and Sledzinski, T. (2019). Effect of exercise on fatty acid metabolism and adipokine secretion in adipose tissue. Front. Physiol. 10:26. doi: 10.3389/fphys.2019.00026
Moore, K. A., and Lemischka, I. R. (2006). Stem cells and their niches. Science 311, 1880–1885. doi: 10.1126/science.1110542
Morelli, M. B., Shu, J., Sardu, C., Matarese, A., and Santulli, G. (2019). Cardiosomal microRNAs are essential in post-infarction myofibroblast phenoconversion. Int. J. Mol. Sci. 21:201. doi: 10.3390/ijms21010201
Muralidharan-Chari, V., Clancy, J., Plou, C., Romao, M., Chavrier, P., Raposo, G., et al. (2009). ARF6-regulated shedding of tumor cell-derived plasma membrane microvesicles. Curr. Biol. 19, 1875–1885. doi: 10.1016/j.cub.2009.09.059
Myint, P. K., Park, E. J., Gaowa, A., Kawamoto, E., and Shimaoka, M. (2020). Targeted remodeling of breast cancer and immune cell homing niches by exosomal integrins. Diagn Pathol. 15:38. doi: 10.1186/s13000-020-00959-3
Neven, K. Y., Nawrot, T. S., and Bollati, V. (2017). Extracellular vesicles: how the external and internal environment can shape cell-to-cell communication. Curr. Environ. Health Rep. 4, 30–37. doi: 10.1007/s40572-017-0130-7
New, S. E., Goettsch, C., Aikawa, M., Marchini, J. F., Shibasaki, M., Yabusaki, K., et al. (2013). Macrophage-derived matrix vesicles: an alternative novel mechanism for microcalcification in atherosclerotic plaques. Circ. Res. 113, 72–77. doi: 10.1161/CIRCRESAHA.113.301036
Nie, Y., Sato, Y., Garner, R. T., Kargl, C., Wang, C., Kuang, S., et al. (2019). Skeletal muscle-derived exosomes regulate endothelial cell functions via reactive oxygen species-activated nuclear factor-κB signalling. Exp. Physiol. 104, 1262–1273. doi: 10.1113/EP087396
Nielsen, A. R., Mounier, R., Plomgaard, P., Mortensen, O. H., Penkowa, M., Speerschneider, T., et al. (2007). Expression of interleukin-15 in human skeletal muscle effect of exercise and muscle fibre type composition. J. Physiol. 584, 305–312. doi: 10.1113/jphysiol.2007.139618
Oguri, Y., Shinoda, K., Kim, H., Alba, D. L., Bolus, W. R., Wang, Q., et al. (2020). CD81 controls beige fat progenitor cell growth and energy balance via FAK signaling. Cell 182, 563–577.e20. doi: 10.1016/j.cell.2020.06.021
Okada, T., Ngo, V. N., Ekland, E. H., Förster, R., Lipp, M., Littman, D. R., et al. (2002). Chemokine requirements for B cell entry to lymph nodes and Peyer's patches. J. Exp. Med. 196, 65–75. doi: 10.1084/jem.20020201
Olsen, R. H., Krogh-Madsen, R., Thomsen, C., Booth, F. W., and Pedersen, B. K. (2008). Metabolic responses to reduced daily steps in healthy nonexercising men. JAMA 299, 1261–1263. doi: 10.1001/jama.299.11.1259
Ostrowski, M., Carmo, N. B., Krumeich, S., Fanget, I., Raposo, G., Savina, A., et al. (2010). Rab27a and Rab27b control different steps of the exosome secretion pathway. Nat. Cell Biol. 12, 19–30. doi: 10.1038/ncb2000
Pabst, O., Förster, R., Lipp, M., Engel, H., and Arnold, H. H. (2000). NKX2.3 is required for MAdCAM-1 expression and homing of lymphocytes in spleen and mucosa-associated lymphoid tissue. EMBO J. 19, 2015–2023. doi: 10.1093/emboj/19.9.2015
Panati, K., Narala, V. R., Narasimha, V. R., Derangula, M., Arva Tatireddigari, V. R. R., and Yeguvapalli, S. (2018). Expression, purification and biological characterisation of recombinant human irisin (12.5 kDa). J. Genet. Eng. Biotechnol. 16, 459–466. doi: 10.1016/j.jgeb.2018.06.007
Park, E. J., Appiah, M. G., Myint, P. K., Gaowa, A., Kawamoto, E., and Shimaoka, M. (2019a). Exosomes in sepsis and inflammatory tissue injury. Curr. Pharm. Des. 25, 4486–4495. doi: 10.2174/1381612825666191116125525
Park, E. J., Myint, P. K., Ito, A., Appiah, M. G., Darkwah, S., Kawamoto, E., et al. (2020). Integrin-ligand interactions in inflammation, cancer, and metabolic disease: insights into the multifaceted roles of an emerging ligand irisin. Front. Cell Dev. Biol. 8:588066. doi: 10.3389/fcell.2020.588066
Park, E. J., Prajuabjinda, O., Soe, Z. Y., Darkwah, S., Appiah, M. G., Kawamoto, E., et al. (2019b). Exosomal regulation of lymphocyte homing to the gut. Blood Adv. 3, 1–11. doi: 10.1182/bloodadvances.2018024877
Pollak, M. (2008). Insulin and insulin-like growth factor signalling in neoplasia. Nat. Rev. Cancer 8, 915–928. doi: 10.1038/nrc2536
Pols, M. S., and Klumperman, J. (2009). Trafficking and function of the tetraspanin CD63. Exp. Cell Res. 315, 1584–1592. doi: 10.1016/j.yexcr.2008.09.020
Prestoz, L., Relvas, J. B., Hopkins, K., Patel, S., Sowinski, P., Price, J., et al. (2001). Association between integrin-dependent migration capacity of neural stem cells in vitro and anatomical repair following transplantation. Mol. Cell Neurosci. 18, 473–484. doi: 10.1006/mcne.2001.1037
Pukajło, K., Kolackov, K., and Łaczmański, Ł., Daroszewski, J. (2015). Irisin–a new mediator of energy homeostasis. Postepy Hig. Med. Dosw. 69, 233–242. doi: 10.5604/17322693.1141097
Quinn, L. S., Strait-Bodey, L., Anderson, B. G., Argilés, J. M., and Havel, P. J. (2005). Interleukin-15 stimulates adiponectin secretion by 3T3-L1 adipocytes: evidence for a skeletal muscle-to-fat signaling pathway. Cell Biol. Int. 29, 449–457. doi: 10.1016/j.cellbi.2005.02.005
Rabiee, F., Lachinani, L., Ghaedi, S., Nasr-Esfahani, M. H., Megraw, T. L., and Ghaedi, K. (2020). New insights into the cellular activities of Fndc5/Irisin and its signaling pathways. Cell Biosci. 10:51. doi: 10.1186/s13578-020-00413-3
Regev-Rudzki, N., Wilson, D. W., Carvalho, T. G., Sisquella, X., Coleman, B. M., Rug, M., et al. (2013). Cell-cell communication between malaria-infected red blood cells via exosome-like vesicles. Cell 153, 1120–1133. doi: 10.1016/j.cell.2013.04.029
Reynolds, J. L., Joannides, A. J., Skepper, J. N., McNair, R., Schurgers, L. J., Proudfoot, D., et al. (2004). Human vascular smooth muscle cells undergo vesicle-mediated calcification in response to changes in extracellular calcium and phosphate concentrations: a potential mechanism for accelerated vascular calcification in ESRD. J. Am. Soc. Nephrol. 15, 2857–2867. doi: 10.1097/01.ASN.0000141960.01035.28
Reynolds, J. L., Skepper, J. N., McNair, R., Kasama, T., Gupta, K., Weissberg, P. L., et al. (2005). Multifunctional roles for serum protein fetuin-a in inhibition of human vascular smooth muscle cell calcification. J. Am. Soc. Nephrol. 16, 2920–2930. doi: 10.1681/ASN.2004100895
Ribeiro, M. F., Zhu, H., Millard, R. W., and Fan, G. C. (2013). Exosomes function in pro- and anti-angiogenesis. Curr. Angiogenes 2, 54–59. doi: 10.2174/22115528113020020001
Rodríguez, A., Becerril, S., Ezquerro, S., Méndez-Giménez, L., and Frühbeck, G. (2017). Crosstalk between adipokines and myokines in fat browning. Acta Physiol. 219, 362–381. doi: 10.1111/apha.12686
Rome, S., Forterre, A., Mizgier, M. L., and Bouzakri, K. (2019). Skeletal muscle-released extracellular vesicles: state of the art. Front. Physiol. 10:929. doi: 10.3389/fphys.2019.00929
Sahin, A. O., and Buitenhuis, M. (2012). Molecular mechanisms underlying adhesion and migration of hematopoietic stem cells. Cell Adh. Migr. 6, 39–48. doi: 10.4161/cam.18975
Schippers, A., Kochut, A., Pabst, O., Frischmann, U., Clahsen, T., Tenbrock, K., et al. (2012). β7 integrin controls immunogenic and tolerogenic mucosal B cell responses. Clin. Immunol. 144, 87–97. doi: 10.1016/j.clim.2012.05.008
Schön, M. P., Arya, A., Murphy, E. A., Adams, C. M., Strauch, U. G., Agace, W. W., et al. (1999). Mucosal T lymphocyte numbers are selectively reduced in integrin alpha E (CD103)-deficient mice. J. Immunol. 162, 6641–6649.
Schöneberg, J., Lee, I. H., Iwasa, J. H., and Hurley, J. H. (2017). Reverse-topology membrane scission by the ESCRT proteins. Nat. Rev. Mol. Cell Biol. 18, 5–17. doi: 10.1038/nrm.2016.121
Schurgers, L. J., Akbulut, A. C., Kaczor, D. M., Halder, M., Koenen, R. R., and Kramann, R. (2018). Initiation and propagation of vascular calcification is regulated by a concert of platelet- and smooth muscle cell-derived extracellular vesicles. Front. Cardiovasc. Med. 5:36. doi: 10.3389/fcvm.2018.00036
Schweitzer, K. M., Dräger, A. M., van der Valk, P., Thijsen, S. F., Zevenbergen, A., Theijsmeijer, A. P., et al. (1996). Constitutive expression of E-selectin and vascular cell adhesion molecule-1 on endothelial cells of hematopoietic tissues. Am. J. Pathol. 148, 165–175.
Seldin, M. M., and Wong, G. W. (2012). Regulation of tissue crosstalk by skeletal muscle-derived myonectin and other myokines. Adipocyte 1, 200–202. doi: 10.4161/adip.20877
Shao, L., Li, H., Chen, J., Song, H., Zhang, Y., Wu, F., et al. (2017). Irisin suppresses the migration, proliferation, and invasion of lung cancer cells via inhibition of epithelial-to-mesenchymal transition. Biochem. Biophys. Res. Commun. 485, 598–605. doi: 10.1016/j.bbrc.2016.12.084
Singh, A., Fedele, C., Lu, H., Nevalainen, M. T., Keen, J. H., and Languino, L. R. (2016). Exosome-mediated transfer of αvβ3 integrin from tumorigenic to nontumorigenic cells promotes a migratory phenotype. Mol. Cancer Res. 14, 1136–1146. doi: 10.1158/1541-7786.MCR-16-0058
Stahl, P. D., and Raposo, G. (2018). Exosomes and extracellular vesicles: the path forward. Essays Biochem. 62, 119–124. doi: 10.1042/EBC20170088
Streeter, P. R., Berg, E. L., Rouse, B. T., Bargatze, R. F., and Butcher, E. C. (1988). A tissue-specific endothelial cell molecule involved in lymphocyte homing. Nature 331, 41–46. doi: 10.1038/331041a0
Stuffers, S., Sem Wegner, C., Stenmark, H., and Brech, A. (2009). Multivesicular endosome biogenesis in the absence of ESCRTs. Traffic 10, 925–937. doi: 10.1111/j.1600-0854.2009.00920.x
Sung, B. H., Ketova, T., Hoshino, D., Zijlstra, A., and Weaver, A. M. (2015). Directional cell movement through tissues is controlled by exosome secretion. Nat. Commun. 6:7164. doi: 10.1038/ncomms8164
Takafuji, Y., Tatsumi, K., Ishida, M., Kawao, N., Okada, K., and Kaji, H. (2020a). Extracellular vesicles secreted from mouse muscle cells suppress osteoclast formation: roles of mitochondrial energy metabolism. Bone 134:115298. doi: 10.1016/j.bone.2020.115298
Takafuji, Y., Tatsumi, K., Kawao, N., Okada, K., Muratani, M., and Kaji, H. (2020b). MicroRNA-196a-5p in extracellular vesicles secreted from myoblasts suppresses osteoclast-like cell formation in mouse cells. Calcif. Tissue Int. doi: 10.1007/s00223-020-00772-6. [Epub ahead of print].
Teti, A., Migliaccio, S., and Baron, R. (2002). The role of the alphaVbeta3 integrin in the development of osteolytic bone metastases: a pharmacological target for alternative therapy? Calcif. Tissue Int. 71, 293–299. doi: 10.1007/s00223-001-2071-1
Théry, C., Witwer, K. W., Aikawa, E., Alcaraz, M. J., Anderson, J. D., Andriantsitohaina, R., et al. (2018). Minimal information for studies of extracellular vesicles 2018 (MISEV2018): a position statement of the International Society for Extracellular Vesicles and update of the MISEV2014 guidelines. J. Extracell. Vesicles 7:1535750. doi: 10.1080/20013078.2018.1535750
Tidball, J. G. (2017). Regulation of muscle growth and regeneration by the immune system. Nat. Rev. Immunol. 17, 165–178. doi: 10.1038/nri.2016.150
Tracy, E., Rowe, G., and LeBlanc, A. J. (2020). Cardiac tissue remodeling in healthy aging: the road to pathology. Am. J. Physiol. Cell Physiol. 319, C166–C182. doi: 10.1152/ajpcell.00021.2020
Tsuchiya, Y., Ando, D., Goto, K., Kiuchi, M., Yamakita, M., and Koyama, K. (2014). High-intensity exercise causes greater irisin response compared with low-intensity exercise under similar energy consumption. Tohoku J. Exp. Med. 233, 135–140. doi: 10.1620/tjem.233.135
Tsuchiya, Y., Ando, D., Takamatsu, K., and Goto, K. (2015). Resistance exercise induces a greater irisin response than endurance exercise. Metabolism 64, 1042–1050. doi: 10.1016/j.metabol.2015.05.010
Viaud, S., Théry, C., Ploix, S., Tursz, T., Lapierre, V., Lantz, O., et al. (2010). Dendritic cell-derived exosomes for cancer immunotherapy: what's next? Cancer Res. 70, 1281–1285. doi: 10.1158/0008-5472.CAN-09-3276
Waldenström, A., Gennebäck, N., Hellman, U., and Ronquist, G. (2012). Cardiomyocyte microvesicles contain DNA/RNA and convey biological messages to target cells. PLoS ONE 7:e34653. doi: 10.1371/journal.pone.0034653
Wang, S., Zeng, Y., Zhou, J. M., Nie, S. L., Peng, Q., Gong, J., et al. (2016). MicroRNA-1246 promotes growth and metastasis of colorectal cancer cells involving CCNG2 reduction. Mol. Med. Rep. 13, 273–280. doi: 10.3892/mmr.2015.4557
Wang, X., Huang, W., Liu, G., Cai, W., Millard, R. W., Wang, Y., et al. (2014). Cardiomyocytes mediate anti-angiogenesis in type 2 diabetic rats through the exosomal transfer of miR-320 into endothelial cells. J. Mol. Cell Cardiol. 74, 139–150. doi: 10.1016/j.yjmcc.2014.05.001
Wang, X., Morelli, M. B., Matarese, A., Sardu, C., and Santulli, G. (2020). Cardiomyocyte-derived exosomal microRNA-92a mediates post-ischemic myofibroblast activation both in vitro and ex vivo. ESC Heart Fail. 7, 284–288. doi: 10.1002/ehf2.12584
Wang, X. H., Qian, R. Z., Zhang, W., Chen, S. F., Jin, H. M., and Hu, R. M. (2009). MicroRNA-320 expression in myocardial microvascular endothelial cells and its relationship with insulin-like growth factor-1 in type 2 diabetic rats. Clin. Exp. Pharmacol. Physiol. 36, 181–188. doi: 10.1111/j.1440-1681.2008.05057.x
Whitham, M., Parker, B. L., Friedrichsen, M., Hingst, J. R., Hjorth, M., Hughes, W. E., et al. (2018). Extracellular vesicles provide a means for tissue crosstalk during exercise. Cell Metab. 27, 237–251.e4. doi: 10.1016/j.cmet.2017.12.001
Wiklander, O. P. B., Brennan, M., Lötvall, J., Breakefield, X. O., and El Andaloussi, S. (2019). Advances in therapeutic applications of extracellular vesicles. Sci. Transl. Med. 11:eaav8521. doi: 10.1126/scitranslmed.aav8521
Willis, M. S., and Patterson, C. (2010). Hold me tight: role of the heat shock protein family of chaperones in cardiac disease. Circulation 122, 1740–1751. doi: 10.1161/CIRCULATIONAHA.110.942250
Wong, K. K., Engelman, J. A., and Cantley, L. C. (2010). Targeting the PI3K signaling pathway in cancer. Curr. Opin. Genet. Dev. 20, 87–90. doi: 10.1016/j.gde.2009.11.002
Wortzel, I., Dror, S., Kenific, C. M., and Lyden, D. (2019). Exosome-mediated metastasis: communication from a distance. Dev. Cell 49, 347–360. doi: 10.1016/j.devcel.2019.04.011
Xiong, X. Q., Geng, Z., Zhou, B., Zhang, F., Han, Y., Zhou, Y. B., et al. (2018). FNDC5 attenuates adipose tissue inflammation and insulin resistance via AMPK-mediated macrophage polarization in obesity. Metabolism 83, 31–41. doi: 10.1016/j.metabol.2018.01.013
Xu, Q., Cui, Y., Luan, J., Zhou, X., Li, H., and Han, J. (2018). Exosomes from C2C12 myoblasts enhance osteogenic differentiation of MC3T3-E1 pre-osteoblasts by delivering miR-27a-3p. Biochem. Biophys. Res. Commun. 498, 32–37. doi: 10.1016/j.bbrc.2018.02.144
Xu, X., Cao, L., Zhang, Y., Lian, H., Sun, Z., and Cui, Y. (2018). MicroRNA-1246 inhibits cell invasion and epithelial mesenchymal transition process by targeting CXCR4 in lung cancer cells. Cancer Biomark. 21, 251–260. doi: 10.3233/CBM-170317
Xuan, W., Wang, L., Xu, M., Weintraub, N. L., and Ashraf, M. (2019). miRNAs in extracellular vesicles from iPS-derived cardiac progenitor cells effectively reduce fibrosis and promote angiogenesis in infarcted heart. Stem Cells Int. 2019:3726392. doi: 10.1155/2019/3726392
Yamada, M., Kubo, H., Kobayashi, S., Ishizawa, K., He, M., Suzuki, T., et al. (2011). The increase in surface CXCR4 expression on lung extravascular neutrophils and its effects on neutrophils during endotoxin-induced lung injury. Cell Mol. Immunol. 8, 305–314. doi: 10.1038/cmi.2011.8
Yang, J., Yu, X., Xue, F., Li, Y., Liu, W., and Zhang, S. (2018). Exosomes derived from cardiomyocytes promote cardiac fibrosis via myocyte-fibroblast cross-talk. Am. J. Transl. Res. 10, 4350–4366.
Yu, D. W., Ge, P. P., Liu, A. L., Yu, X. Y., and Liu, T. T. (2019). HSP20-mediated cardiomyocyte exosomes improve cardiac function in mice with myocardial infarction by activating Akt signaling pathway. Eur. Rev. Med. Pharmacol. Sci. 23, 4873–4881. doi: 10.26355/eurrev_201906_18075
Yu, H., and Wang, Z. (2019). Cardiomyocyte-derived exosomes: biological functions and potential therapeutic implications. Front. Physiol. 10:1049. doi: 10.3389/fphys.2019.01049
Yuan, J., Liu, H., Gao, W., Zhang, L., Ye, Y., Yuan, L., et al. (2018). MicroRNA-378 suppresses myocardial fibrosis through a paracrine mechanism at the early stage of cardiac hypertrophy following mechanical stress. Theranostics 8, 2565–2582. doi: 10.7150/thno.22878
Yue, B., Yang, H., Wang, J., Ru, W., Wu, J., Huang, Y., et al. (2020). Exosome biogenesis, secretion and function of exosomal miRNAs in skeletal muscle myogenesis. Cell Prolif. 53:e12857. doi: 10.1111/cpr.12857
Zhang, X., Wang, X., Zhu, H., Kranias, E. G., Tang, Y., Peng, T., et al. (2012). Hsp20 functions as a novel cardiokine in promoting angiogenesis via activation of VEGFR2. PLoS ONE 7:e32765. doi: 10.1371/journal.pone.0032765
Zhang, Y., Li, R., Meng, Y., Li, S., Donelan, W., Zhao, Y., et al. (2014). Irisin stimulates browning of white adipocytes through mitogen-activated protein kinase p38 MAP kinase and ERK MAP kinase signaling. Diabetes 63, 514–525. doi: 10.2337/db13-1106
Zhang, Z. P., Zhang, X. F., Li, H., Liu, T. J., Zhao, Q. P., Huang, L. H., et al. (2018). Serum irisin associates with breast cancer to spinal metastasis. Medicine 97:e0524. doi: 10.1097/MD.0000000000010524
Zheng, B., Yin, W. N., Suzuki, T., Zhang, X. H., Zhang, Y., Song, L. L., et al. (2017). Exosome-mediated miR-155 transfer from smooth muscle cells to endothelial cells induces endothelial injury and promotes atherosclerosis. Mol. Ther. 25, 1279–1294. doi: 10.1016/j.ymthe.2017.03.031
Keywords: extracellular vesicles, muscle, integrins, myokines, tumor metastasis, tissue microenvironment, homing niche, irisin
Citation: Darkwah S, Park EJ, Myint PK, Ito A, Appiah MG, Obeng G, Kawamoto E and Shimaoka M (2021) Potential Roles of Muscle-Derived Extracellular Vesicles in Remodeling Cellular Microenvironment: Proposed Implications of the Exercise-Induced Myokine, Irisin. Front. Cell Dev. Biol. 9:634853. doi: 10.3389/fcell.2021.634853
Received: 29 November 2020; Accepted: 14 January 2021;
Published: 05 February 2021.
Edited by:
Lucas Treps, VIB KU Leuven Center for Cancer Biology, BelgiumReviewed by:
Valentina Di Felice, University of Palermo, ItalyMichele Guescini, University of Urbino Carlo Bo, Italy
Alexis Forterre, Stanford University, United States
Copyright © 2021 Darkwah, Park, Myint, Ito, Appiah, Obeng, Kawamoto and Shimaoka. This is an open-access article distributed under the terms of the Creative Commons Attribution License (CC BY). The use, distribution or reproduction in other forums is permitted, provided the original author(s) and the copyright owner(s) are credited and that the original publication in this journal is cited, in accordance with accepted academic practice. No use, distribution or reproduction is permitted which does not comply with these terms.
*Correspondence: Samuel Darkwah, a3dla3VhZGFya3dhaEBnbWFpbC5jb20=; Motomu Shimaoka, bW90b211c2hpbWFva2FAZ21haWwuY29t