- 1Functional Biomolecular Research Group, Biomedical Research Institute, National Institute of Advanced Industrial Science and Technology, Osaka, Japan
- 2DBT-AIST International Laboratory for Advanced Biomedicine, National Institute of Advanced Industrial Science and Technology, Osaka, Japan
Zebrafish have superior regenerative capacity in the central nervous system (CNS) compared to mammals. In contrast, medaka were shown to have low regenerative capacity in the adult heart and larval retina, despite the well-documented high tissue regenerative ability of teleosts. Nevertheless, medaka and zebrafish share similar brain structures and biological features to those of mammals. Hence, this study aimed to compare the neural stem cell (NSC) responses and regenerative capacity in the optic tectum of adult medaka and zebrafish after stab wound injury. Limited neuronal differentiation was observed in the injured medaka, though the proliferation of radial glia (RG) was induced in response to tectum injury. Moreover, the expression of the pro-regenerative transcriptional factors ascl1a and oct4 was not enhanced in the injured medaka, unlike in zebrafish, whereas expression of sox2 and stat3 was upregulated in both fish models. Of note, glial scar-like structures composed of GFAP+ radial fibers were observed in the injured area of medaka at 14 days post injury (dpi). Altogether, these findings suggest that the adult medaka brain has low regenerative capacity with limited neuronal generation and scar formation. Hence, medaka represent an attractive model for investigating and evaluating critical factors for brain regeneration.
Introduction
Zebrafish have a superior ability to regenerate various tissues, including the central nervous system (CNS) and heart, compared with mammals (Becker et al., 1997; Poss et al., 2002; Raymond et al., 2006; März et al., 2011). Recently, to better understand the molecular mechanisms underlying the high regenerative capacity of zebrafish, comparative analyses of tissue regeneration in the retina and heart between zebrafish and mice have been performed, given their similarities in cell type and tissue structure (Kang et al., 2016; Hoang et al., 2020; Simões et al., 2020). Comparative studies using next-generation sequencing technology have revealed differences in the immune response or expression of transcriptional factors associated with tissue regeneration (Hoang et al., 2020; Simões et al., 2020). In contrast, the brain structure and cell types between zebrafish and mice are quite different (Kizil et al., 2012; Alunni and Bally-Cuif, 2016; Diotel et al., 2020; Labusch et al., 2020). Despite the efforts made to explore and compare the brain regeneration mechanisms in zebrafish and mice, comparative studies with omics approaches have not been well examined (Llorens-Bobadilla et al., 2015; Zhong et al., 2016; Arneson et al., 2018; Yu and He, 2019; Demirci et al., 2020). To investigate the mechanisms that contribute to the high regenerative capacity of the zebrafish brain, non-regenerative animal models with similar brain structures and biological features are warranted.
Medaka (Oryzias latipes) is a popular experimental model among freshwater teleosts that has been extensively used for tissue regeneration analysis. Despite its high regenerative capacity in the fin and pancreas (Akimenko et al., 1995; Katogi et al., 2004; Moss et al., 2009; Otsuka and Takeda, 2017), similar to zebrafish, medaka have a low capacity for heart and retina regeneration (Ito et al., 2014; Lai et al., 2017; Lust and Wittbrodt, 2018). Comparative analysis of heart regeneration between adult medaka and zebrafish, cardiac cryoinjury results in less cardiomyocyte proliferation and scar formation in medaka (Ito et al., 2014; Lai et al., 2017), whereas zebrafish show induced cardiomyocyte proliferation and injured tissues are filled with newborn cardiomyocytes, with little or no scar tissue formation (Poss et al., 2002; Kikuchi and Poss, 2012). Regenerative capacity in the retina has also been compared between larval medaka and zebrafish, indicating that retinal injury induces Müller glia proliferation in both models; however, Müller glia in medaka have less multipotency, with photoreceptors being generated, but not retinal ganglion cells (Lust and Wittbrodt, 2018). Moreover, overexpression of sox2 in Müller glia was found to promote the regenerative potential of these cells in the medaka retina. However, the CNS regenerative capacity in the adult medaka remains unclear.
Medaka and zebrafish have similar brain structures and niches of adult neural stem cells (NSCs) (Adolf et al., 2006; Grandel et al., 2006; Alunni et al., 2010; Kuroyanagi et al., 2010). Stab wound injury models affecting various regions of the adult zebrafish brain, including the optic tectum, have been developed to investigate brain regeneration (Kroehne et al., 2011; März et al., 2011; Kishimoto et al., 2012; Kaslin et al., 2017; Shimizu et al., 2018; Lindsey et al., 2019; Yu and He, 2019). The optic tectum of both zebrafish and medaka harbors two types of NSCs—neuroepithelial-like stem (NE) and radial glia (RG) cells—that express stem cell markers, such as sox2 and msi1. NE cells are proliferative cells that produce neurons, RG, and oligodendrocytes, whereas most of RG are quiescent (Alunni et al., 2010; Ito et al., 2010; Takeuchi and Okubo, 2013; Galant et al., 2016; Dambroise et al., 2017). Previous studies showed that RG proliferation and differentiation into newborn neurons are induced in response to injury in young adult zebrafish (2–4 months old) (Shimizu et al., 2018; Ueda et al., 2018; Yu and He, 2019; Kiyooka et al., 2020). In contrast, the regenerative responses in the medaka tectum remain to be elucidated.
Herein, the proliferation and differentiation of RG and NE in injured medaka and zebrafish were examined to evaluate the regenerative capacity of the medaka brain. The present study highlights the potential of medaka as a useful experimental non-regenerative model to investigate and identify pro-regenerative factors that mediate CNS regeneration.
Materials and Methods
Animals
Medaka (O. latipes) and zebrafish (Danio rerio), specifically the Kyoto-Cab and RIKEN Wako wild-type strains, respectively, were maintained at 27.0 ± 1°C under a 14/10 h light/dark cycle. All experimental protocols were approved by the Institutional Animal Care and Use Committee of the National Institute of Advanced Industrial Science and Technology (2021-0276). Animals with 3–7 months old were used for all experiments, except for the analysis of newborn neurons after tectum injury, which 3–5-months-old medaka and zebrafish were used.
Stab Wound Injury Protocol
To induce a stab wound injury in the adult optic tectum, medaka and zebrafish were anesthetized with 0.02% tricaine (pH 7.0; Nacalai Tesque, Kyoto, Japan) and a 30 G needle was vertically inserted into the medial region of the right hemisphere, as previously described (Shimizu et al., 2018). The contralateral uninjured hemisphere was used as internal control for each animal. For quantitative real-time polymerase chain reaction (PCR) analysis, both hemispheres were injured.
5-Bromo-2-Deoxyuridine (BrdU) Administration
To label proliferating cells, injured medaka and zebrafish were kept in 5 mM BrdU (Wako, Osaka, Japan). Injured medaka and zebrafish were treated with BrdU for 48 h, from 1 to 3 days post injury (dpi).
Histological and Immunohistochemical Analysis
Medaka and zebrafish were anesthetized using 0.02% tricaine and intracardially perfused with phosphate-buffered saline. Brains were dissected and stored in 4% paraformaldehyde (Wako) solution overnight at 4°C. The fixed brains were stored in 30% sucrose solution overnight at 4°C, and whole brains were then embedded in a 2:1 mixture of 30% sucrose and Tissue-Tek O.C.T. compound (Sakura Finetek Japan, Tokyo, Japan). For fluorescence immunohistochemistry, 14 μm cryosections were prepared using a Leica CM1960 cryostat (Leica Biosystems, Wetzlar, Germany). Fluorescence immunohistochemistry was performed as described previously, using the following primary antibodies: mouse anti-HuC (1:100 dilution, A21271; Invitrogen, Waltham, MA, United States) as a pan-neuronal marker, mouse anti-proliferating cell nuclear antigen (PCNA) (1:200, sc-56; Santa Cruz Biotechnology, Dallas, TX, United States) as a proliferating cell marker, mouse anti-glial fibrillary acid protein (GFAP) (1:500, G3893; Sigma-Aldrich, St. Louis, MO, United States), and rabbit anti-brain lipid binding protein (BLBP) (1:500, ABN14; Millipore, Burlington, MA, United States) as RG cell markers, and sheep anti-BrdU (1:500, ab1893; Abcam, Cambridge, United Kingdom). Alexa Fluor 488- and 546-conjugated subclass-specific antibodies (1:500, Invitrogen) were used as secondary antibodies. For PCNA antigen retrieval, sections were incubated with 10 mM sodium citrate for 30 min at 85°C prior to primary antibody incubation. For BrdU antigen retrieval, sections were incubated with 2N HCl (Wako) for 30 min at 37°C. For nuclear staining, the sections were incubated with Hoechst 33258 (1:500; Dojindo, Kumamoto, Japan) for 30 min following immunohistochemistry.
Quantitative Real-Time PCR (qRT-PCR)
For qRT-PCR, both hemispheres of the optic tectum were injured. After anesthesia with 0.02% tricaine, both hemispheres of the optic tectum were dissected from one fish and homogenized in TRIzol reagent (Invitrogen). Total RNA was purified using the Directozol RNA Miniprep (Zymo Research, Irvine, CA, United States), and cDNA was synthesized using RevaTra Ace (Toyobo, Osaka, Japan). The gene-specific primers used for ascl1a, oct4, sox2, stat3, and tbp are listed in Supplementary Table 1. The expression of tbp was used as endogenous control.
Cell Quantification
To quantify proliferating RG after the stab injury, the number of BLBP+PCNA+ cells was counted in 5–10 sections, including the center of the injury. To quantify NE proliferation, the number of PCNA+ cells located in the tectal marginal zone was counted in 5–10 sections, including the center of the injury. To quantify the number of newborn neurons after the stab injury, the number of BrdU+HuC+ cells in five sections, including the center of the injury, was counted. The number of BrdU+HuC+ cells in the tectal marginal zone was also counted in five sections after the tectum injury. The corresponding contralateral regions were examined as internal controls.
Statistical Analysis
All data are expressed as the mean ± standard error of the mean (SEM), and sample numbers are indicated in each figure legend. Statistical analysis in two experimental groups was performed using paired and unpaired Student’s t-tests. In three or more groups, one-way analysis of variance was performed, followed by Tukey’s post hoc test. P-values were calculated using Prism software (GraphPad Software, San Diego, CA, United States) and statistical significance was defined as ∗∗∗ and ††† if P < 0.001; ∗∗ and †† if P < 0.01; ∗ and † if P < 0.05.
Results
Increase in the Proliferation of Radial Glia in Response to Stab Injury
In the adult zebrafish optic tectum, most RG are quiescent under physiological conditions, but stab wound injury can induce their proliferation (Shimizu et al., 2018; Lindsey et al., 2019; Yu and He, 2019). To examine that this regenerative mechanism was also present in medaka, stab wound injury was induced in the right hemisphere of the optic tectum of medaka and RG proliferation was quantified by counting BLBP (RG marker), and PCNA (proliferating cell marker) double-positive cells. At 2 dpi, the number of proliferative RG cells (BLBP+PCNA+ cells) was significantly increased in the injured hemisphere than in the contralateral (internal control) uninjured side (Figures 1A,B). Additional analysis between 6 h post injury (hpi) to 7 dpi (Figures 1C–F) further revealed that the number of proliferative RG significantly increased from 1 dpi and peaking at around 2 dpi, with no significant difference being observed at 7 dpi (Figure 1G), which follows the same response trend observed in the injured zebrafish (Shimizu et al., 2018; Yu and He, 2019). Moreover, we quantified BLBP–PCNA+ cells except for proliferative NE known as PCNA+ cells located in the tectal marginal zone to analyze the cell proliferation of another type of cell. These BLBP–PCNA+ cells which may include oligodendrocytes, microglia, neutrophils, and endothelial cells also significantly increased in response to the injury (Figure 1H). Although the contribution of NE to tectum regeneration is controversial (Shimizu et al., 2018; Lindsey et al., 2019), NE proliferation after stab wound injury was also evaluated by counting the PCNA+ cells in the tectal marginal zone (Supplementary Figures 1A–L). This analysis confirmed that the stab wound injury had no significant effect on the proliferation of NE (Supplementary Figure 1M), which is consistent with previous injured zebrafish (Shimizu et al., 2018). Taken together, these results suggest that RG in the medaka and zebrafish tectum have similar proliferative potential after injury.
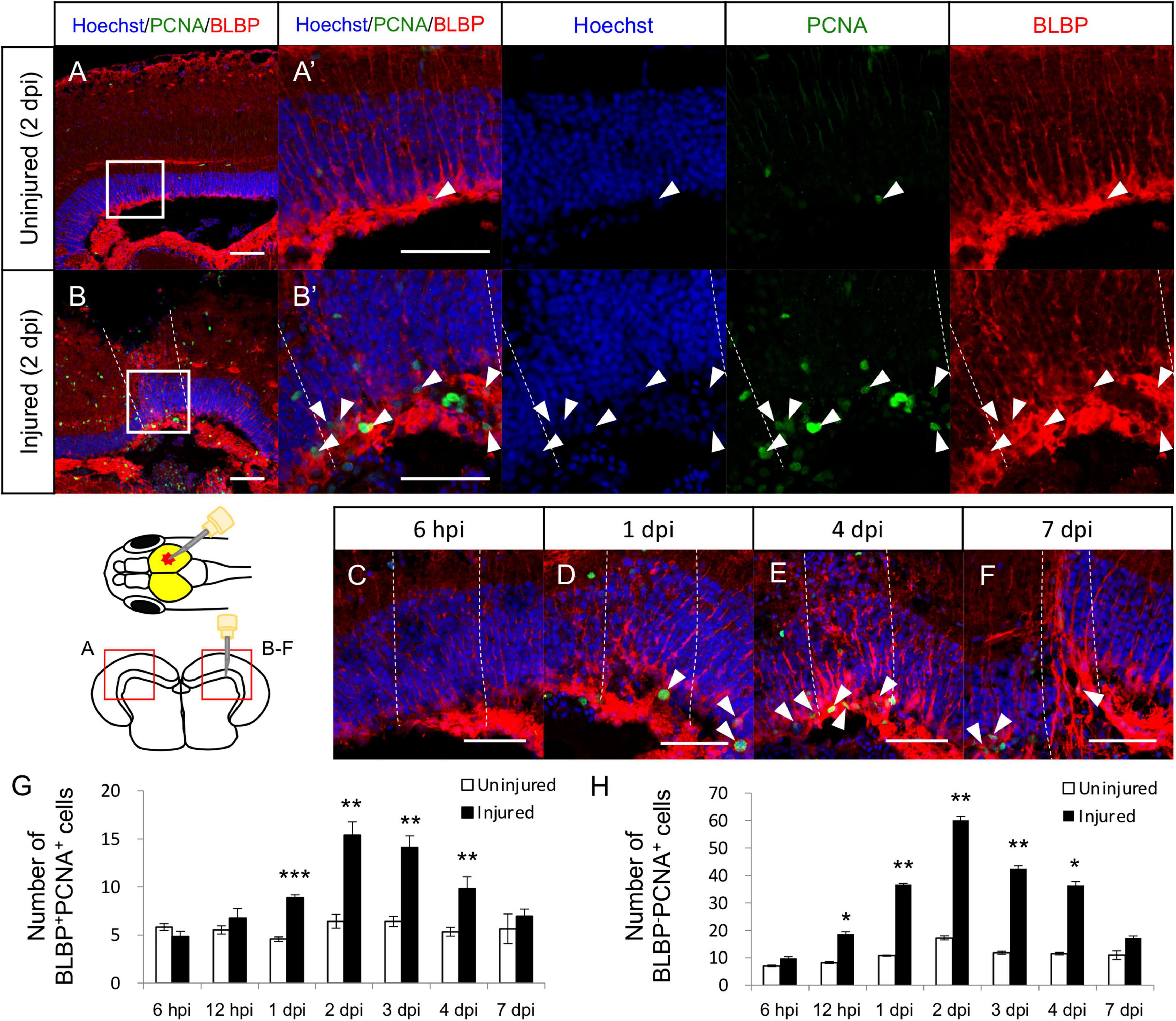
Figure 1. Proliferation of radial glia (RG) is increased in response to stab wound injury. Representative images of proliferative RG (BLBP+PCNA+ cells) in the uninjured (A) and injured (B) hemispheres at 2 days post injury (dpi). (A’,B’) Magnified images of the boxed area in (A,B). (C–F) Representative images of proliferative RG in the injured hemisphere at 6 h post injury (hpi) and at 1, 4, and 7 dpi. White arrowheads indicate BLBP+PCNA+ cells, and dashed lines indicate injured areas. Scale bar: 50 μm in (A–F) and (A’,B’). Schematic drawing of the stab injury in the right hemisphere of the optic tectum and cross-section. (G) Quantification of proliferative RG in both uninjured and injured hemispheres at 6 (n = 5) and 12 (n = 3) hpi, and 1 (n = 5), 2 (n = 5), 3 (n = 4), 4 (n = 5), and 7 (n = 4) dpi. (H) Quantification of proliferative cells (BLBP–PCNA+ cells) except NE in both uninjured and injured hemispheres at 6 (n = 5) and 12 (n = 3) hpi, and 1 (n = 5), 2 (n = 5), 3 (n = 4), 4 (n = 5), and 7 (n = 4) dpi. Statistical analyses between uninjured and injured hemispheres at each time point were evaluated using paired Student’s t-tests. Statistical significance was defined as *P < 0.05; **P < 0.01; ***P < 0.001.
Limited Generation of Newborn Neurons After Stab Injury of Optic Tectum
Previous studies showed that newborn neurons around the injured site after the tectum injury in young adult zebrafish are mainly derived from RG (Shimizu et al., 2018; Yu and He, 2019). To analyze whether newborn neurons were similarly generated in tectum injured medaka, BrdU-labeled proliferative cells (including RG and NE) in the injured zebrafish and medaka were evaluated at 7 dpi (Figure 2A). We confirmed that RG incorporated BrdU at 3 dpi (Supplementary Figures 2A–E). Then, the number of newborn neurons (BrdU+HuC+ cells) at 7 dpi was quantified (Figures 2B,C), revealing that were not significantly increased in the injured hemisphere in the medaka unlike in the zebrafish (Figure 2D). BrdU+ cells around the injured periventricular gray zone (PGZ) in the medaka optic tectum are BLBP+ (Supplementary Figures 2F–I). Moreover, the number of BrdU+ cells observed in PGZ was not significantly different in both fish models (Figure 2E). These results suggest that post-proliferating RG in injured medaka have limited capacity for neuronal differentiation. As NE can also generate neuronal cells in the optic tectum, the differentiation potential of BrdU+ cells in the tectal marginal zone after tectum injury was also evaluated (Supplementary Figure 3A). However, no significant differences were observed in the BrdU+HuC+ cells around the tectal marginal zone between injured and uninjured hemispheres in both medaka and zebrafish (Supplementary Figures 3B–G). Overall, these results suggest that post-proliferating RG in the injured medaka tectum have limited neuronal differentiation, whereas stab wound injury in the optic tectum does not affect NE differentiation into neurons.
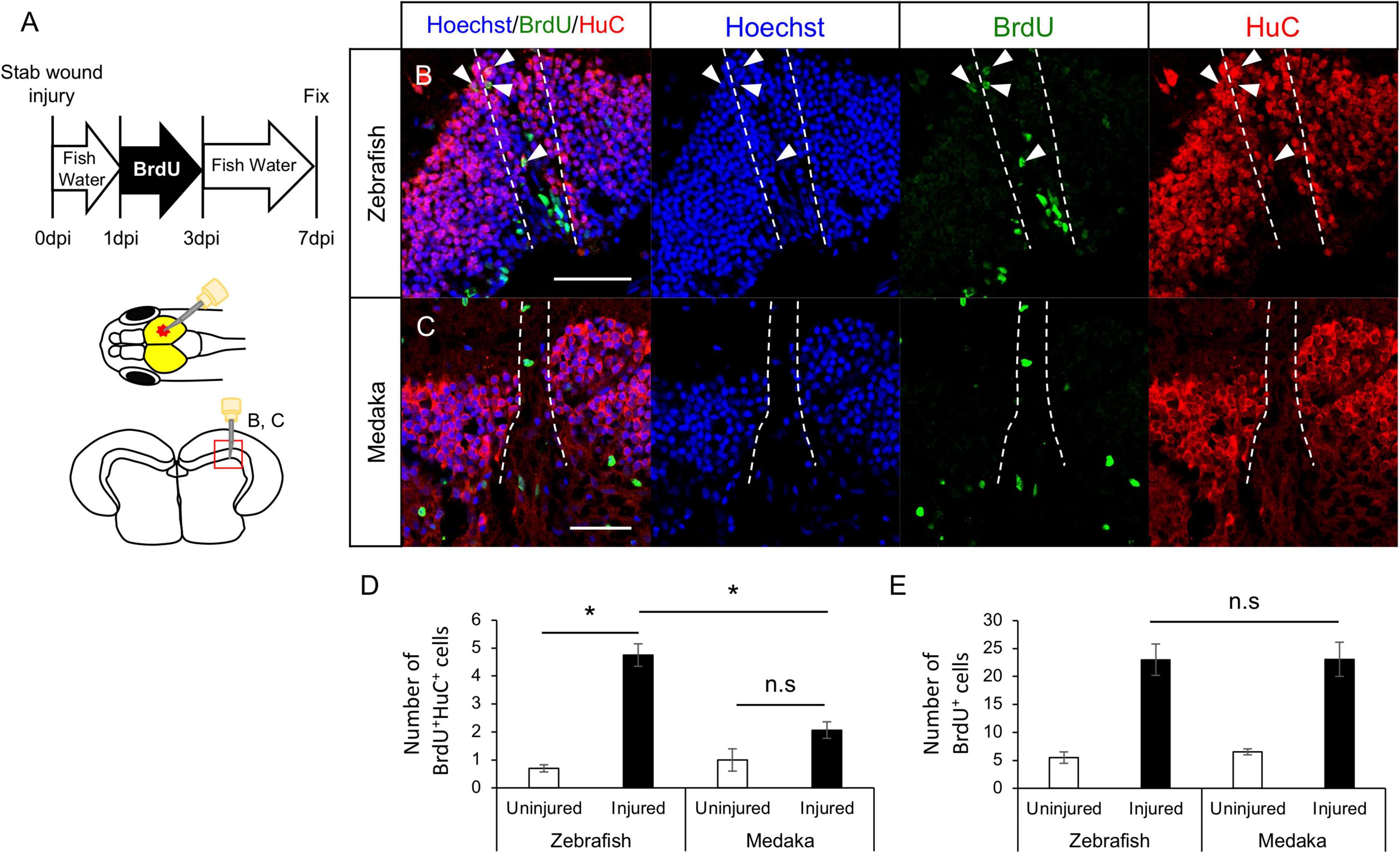
Figure 2. Generation of newborn neurons in the injured medaka is limited compared with zebrafish. (A) Schematic drawing of bromodeoxyuridine (BrdU)-labeling and stab injury in the right hemisphere of the optic tectum. Representative images of newborn neurons (BrdU+HuC+ cells) in both injured zebrafish (B) and medaka (C). White arrowheads indicate BrdU+HuC+ cells, and dashed lines indicate the injured areas. Scale bar: 50 μm. (D) Quantification of BrdU+HuC+ cells in the uninjured and injured hemispheres in zebrafish (n = 4) and medaka (n = 4). (E) Quantification of total BrdU+ cells in both uninjured and injured zebrafish (n = 4) and medaka (n = 4). Statistical analyses were evaluated using one-way analysis of variance followed by Tukey’s post hoc test. Statistical significance was defined as *P < 0.05.
Differential Expression of Transcriptional Factors Between Medaka and Zebrafish After Tectum Injury
Molecular mechanisms related to ascl1a during zebrafish retina regeneration have been well studied (Fausett et al., 2008; Ramachandran et al., 2010). In particular, the expression of this pro-regenerative transcriptional factor was shown to be induced the optic tectum of zebrafish. Moreover, induction of sox2, stat3, and oct4 expression was also shown to be required for NSC proliferation and differentiation into neurons (Fausett et al., 2008; Ramachandran et al., 2010; Nelson et al., 2012; Zhao et al., 2014; Gorsuch et al., 2017; Sharma et al., 2019). Herein, the expression of these transcriptional factors was also evaluated to assess potential changes induced in response to the tectum injury. Thus, ascl1a, oct4 (pou5f1 in medaka and pou5f3 in zebrafish), sox2, and stat3 were evaluated at 6, 24, 96, and 168 hpi. Expression changes of sox2 and stat3 showed similar patterns, significantly increasing from 6 hpi (Figures 3A,B). At 168 hpi, stat3 expression in zebrafish remained significantly elevated though stat3 expression in medaka returned to baseline. Interestingly, upregulation of ascl1a and oct4 was observed in the injured zebrafish (Figures 3C,D), whereas it was not induced in the injured medaka. Expression of oct4 was decreased at 6 hpi in both injured medaka and zebrafish, subsequently increasing in the injured zebrafish at 24 and 96 hpi, but not in the injured medaka (Figure 3D). These results suggest that differential expression of pro-regenerative factors may contribute for the limited neuronal differentiation potential of RG in medaka during tectum regeneration.
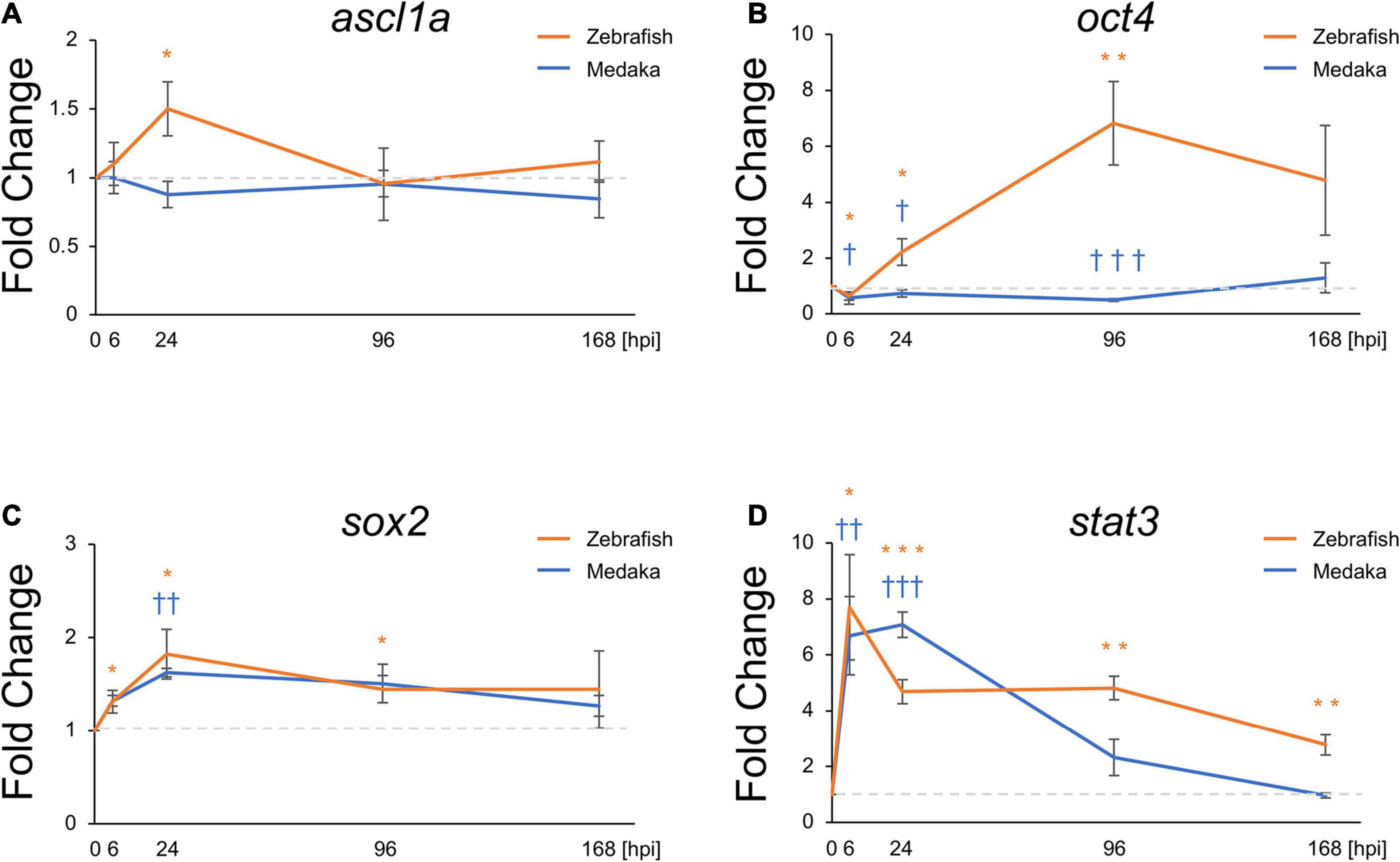
Figure 3. Pro-regenerative transcriptional factors are differentially expressed between the injured medaka and zebrafish. Quantitative polymerase chain reaction analysis of the pro-regenerative transcriptional factors ascl1a (A), oct4 (B), sox2 (C), and stat3 (D). Graphs indicate the relative gene expression in the injured tectum from 6 to 168 h post injury (hpi) compared to the uninjured tectum (n = 4). Statistical analyses between the uninjured and injured hemispheres at each time point were evaluated by unpaired Student’s t-tests. We used * for zebrafish and † for medaka to indicate significant difference. Statistical significance was defined as * and †P < 0.05; ** and ††P < 0.01; *** and ††† P < 0.001.
Glial Scar-Like Structures Persist in the Injured Medaka Tectum
In the adult mammalian brain, stab wound injury increases GFAP immunoreactivity in astrocytes, called reactive gliosis, and these reactive astrocytes are shown to contribute to the GFAP+ scar formation, called glial scar (Feeney et al., 1981; Hozumi et al., 1990; Smith et al., 1995; Xiong et al., 2013; Burda et al., 2016). Although stab wound injury in the zebrafish telencephalon also increases GFAP immunoreactivity in the injured hemisphere, scar formation has not been observed (Kroehne et al., 2011; März et al., 2011; Baumgart et al., 2012; Kishimoto et al., 2012). Hence, the reactive gliosis after the tectum injury was herein assessed by comparing GFAP immunoreactivity in injured medaka and zebrafish at 7, 14, and 30 dpi (Figures 4A–H). At 7 dpi, GFAP expression increased in both injured fishes (Figures 4B,F). In particular, the GFAP immunoreactivity remained activated in the injured zebrafish at 14 dpi (Figures 4I–P), compared with the uninjured tectum (Figure 4C); however, its levels were relatively weak and no obvious scar-like structure was observed at 30 dpi (Figure 4D). Surprisingly, GFAP+ scar-like structures were formed in the injured medaka at 14 dpi (Figure 4G), which persisted at 30 dpi (Figure 4H). Moreover, at 14 dpi (Figures 4I–P), the injured medaka lacked cell layer in the injured PGZ indicated by dashed lines (Figure 4N), and GFAP+ fibers covered the area of this missing cell layer (Figure 4O). This GFAP+ scar-like structure elongated from the basal layer of the PGZ to the apical side (Figure 4O) and this injury-induced GFAP+ structures were co-expressed with BLBP (Figures 4O,P), suggesting that RG in the medaka optic tectum could form this scar-like structure in response to injury. Of note, in the injured zebrafish optic tectum, a disturbed cell layer due to the injury was also observed (Figure 4J), but no obvious lack of layer and no accumulation of GFAP+ or BLBP+ radial fibers around the injured area were noted (Figures 4J–L). These results suggest that RG with GFAP+ scar-like structures in the injured medaka tectum have reactive astrocytic characteristics.
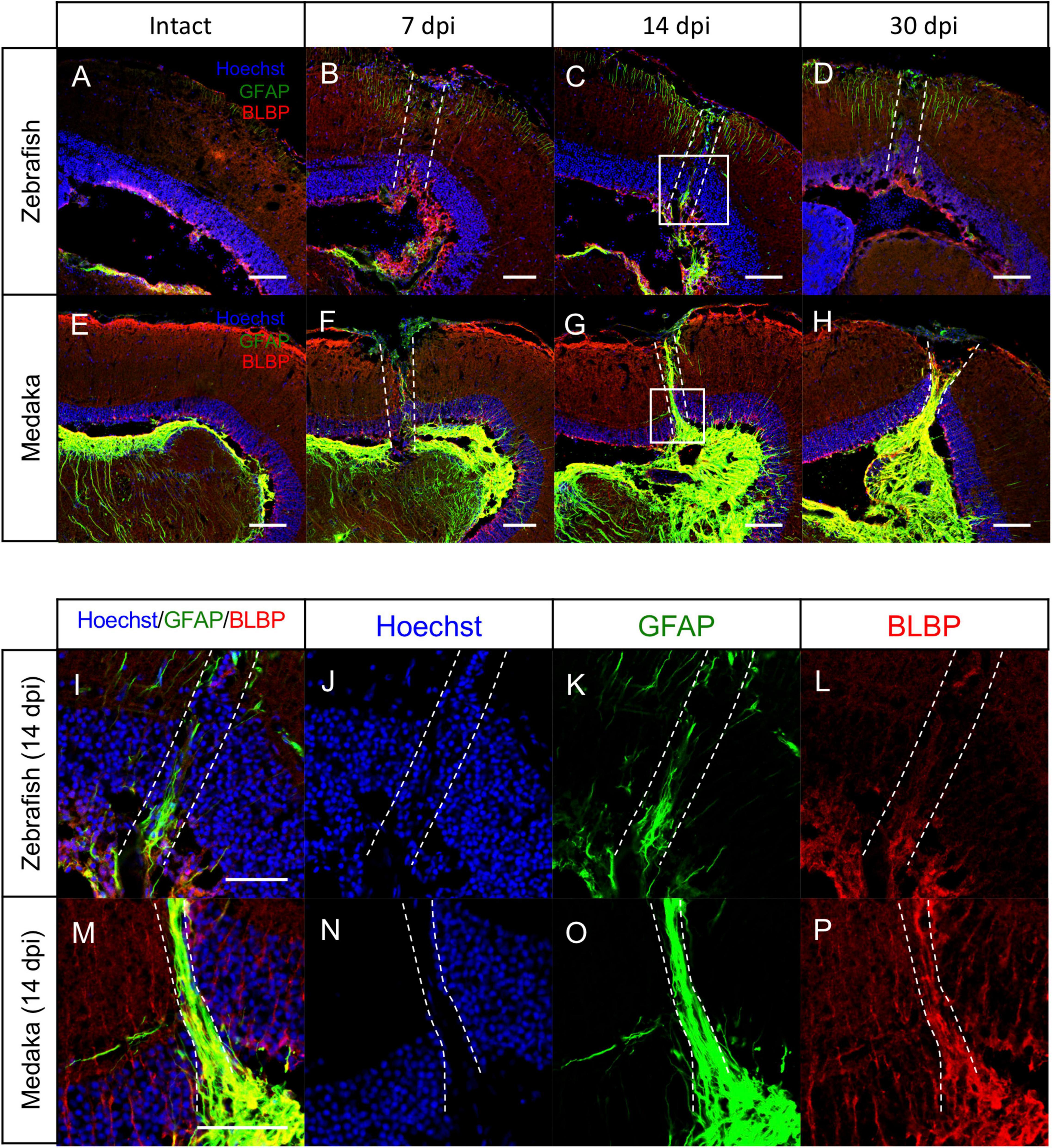
Figure 4. Persistent glial scar-like structure is observed in the injured medaka tectum. (A–H) Representative images of immunostaining with anti-GFAP and anti-BLBP antibodies on the uninjured (A: zebrafish and B: medaka) and injured hemisphere at 7, 14, and 30 days post injury (dpi) [(B–D): zebrafish and (E–H): medaka]. (I–P) Magnified images of the boxed area at 14 dpi [(I–L): zebrafish and (M–P): medaka]. The dashed lines indicate the injured areas. Scale bar: 100 (A–H) and 50 (I,M) μm.
Discussion
Zebrafish have higher CNS regeneration capacity, including of the brain, retina, and spinal cord, compared with mammals (Kizil et al., 2012; Alunni and Bally-Cuif, 2016). Medaka and zebrafish share similar biological features, such as brain structure, body size, and lifespan; nevertheless, medaka have different regenerative capacities in heart and retina (Ito et al., 2014; Lai et al., 2017; Lust and Wittbrodt, 2018). The present study showed that stab wound injury could induce the proliferation of RG in the medaka, but with limited generation of newborn neurons in the injured site compared with the response observed in similarly injured zebrafish. Therefore, this is the first report indicating the limited capacity of neuronal regeneration in the teleost young adult brain. We also confirmed that there was no induction of transcriptional factors, ascl1a and oct4 in the injured medaka. Moreover, we observed injury-induced GFAP+ radial fibers from RG at 14 dpi and found that this glial scar-like structure covered the injured area with lack of cell layer in medaka. Taken together, our findings suggest that medaka have low regenerative ability in the tectum compared to zebrafish because RG in the injured medaka tectum may have reactive astrocytic characteristics rather than neurogenic NSCs.
In the adult zebrafish CNS, the optic tectum and retina share similar features regarding NSCs. For example, RG in the optic tectum and Müller glia in the retina are quiescent under physiological conditions, whereas proliferation and differentiation of these NSCs are activated upon injury (Raymond et al., 2006; Ito et al., 2010; Shimizu et al., 2018). The optic tectum and retina also have NE cells that continuously proliferate and generate newborn neurons throughout life (Raymond et al., 2006; Ito et al., 2010). Comparative analyses of retinal regeneration showed that Müller glia in the larval medaka have limited neuronal differentiation compared with larval zebrafish despite the proliferative response induced by retinal injury (Lust and Wittbrodt, 2018), which is consistent with here observed limited capacity of RG in the medaka tectum. Furthermore, although Müller glia in the medaka only contribute for the generation of photoreceptors, induction of sox2 expression in Müller glia after retinal injury can restore their multi-potency. Although sox2 and stat3 expression increased in both medaka and zebrafish after the tectum injury, that of ascl1a and oct4 did not increase in the injured medaka. In the zebrafish, the transcriptional factors ascl1a (also known as Ascl1/Mash1 in mammals) and oct4 are known to play important roles in retinal regeneration (Fausett et al., 2008; Ramachandran et al., 2010; Sharma et al., 2019). Furthermore, during zebrafish retinal regeneration from light damage, stat3 expression may precede ascl1a expression (Nelson et al., 2012; Goldman, 2014), whereas N-methyl-d-asparate-injured mouse retina showed the lack of Ascl1 expression (Karl et al., 2008) despite the upregulation of phosphorylated Stat3 (Jorstad et al., 2020). These findings suggest that upregulation of STAT3-mediated signaling is a shared feature in both injured medaka and zebrafish, but that lack of ascl1a expression in the injured medaka may result in low neurogenic capacity of RG in the medaka tectum.
In addition to limited neuronal generation after medaka tectum injury, persistent GFAP+BLBP+ scar-like structures were clearly observed from 14 to 30 dpi. In contrast, in the zebrafish adult brain, stab wound injury in the telencephalon induced reactive gliosis with upregulation of GFAP immunoreactivity, but no scar formation was observed (März et al., 2011; Baumgart et al., 2012; Kishimoto et al., 2012). In the injured zebrafish optic tectum, although upregulation of GFAP immunoreactivity was also observed, obvious scar formation like medaka has not been observed. These findings suggest that scar-like structures with radial fibers in the injured medaka tectum are similar to glial scar formed by reactive astrocytes in the damaged mammalian CNS (Burda et al., 2016). Glial scar in the injured rodent CNS includes GFAP and other extracellular matrices, such as chondroitin sulfate proteoglycan and collagen IV (McKeon et al., 1991). The role of glial scar in the tissue regeneration is well investigated, but the findings remain inconclusive (Anderson et al., 2016; Adams and Gallo, 2018; Yang et al., 2020). Glial scar is shown to prevent acute inflammation spreading; however, large scar is an obstacle for neuronal and axonal regeneration. Whether glial scar-like structure in the injured medaka shares these features remain to be explored. Furthermore, Stat3 activation in astrocytes is involved in glial scar formation after spinal cord injury in mice (Herrmann et al., 2008; Anderson et al., 2016), suggesting that the activated stat3 signaling in the medaka RG may contribute to scar formation rather than neuronal generation unlike zebrafish.
Teleost species are shown to have a high regenerative capacity of various tissues, including the CNS. In addition to zebrafish, goldfish (Carassius auratus) and brown ghost knifefish (Apteronotus leptorhynchus) are known to have high CNS regeneration potential (Bernstein, 1964; Stevenson and Yoon, 1978; Zupanc, 1999; Sîrbulescu et al., 2009). In addition to these teleosts, recently, various other species including salmonoids (masu and chum salmon) (Pushchina et al., 2017, 2020) and killifish (mummichog, Aphaniops hormuzensis, and Nothobranchius furzeri) (Bisese et al., 2019; Soltani et al., 2020; Van houcke et al., 2021) have been explored as models to assess the mechanisms regulating the CNS regenerative potential. Previous studies showed that only medaka have low CNS regenerative potential, regardless of age and health condition (Lust and Wittbrodt, 2018). For comparative analyses of tissue regeneration, compatible injury models and similar biological properties, except regenerative capacity, are important. Hence, medaka represent an attractive non-regenerative model to investigate and identify pro-regenerative factors that mediate CNS regeneration.
Data Availability Statement
The original contributions presented in the study are included in the article/Supplementary Material. Further inquiries can be directed to the corresponding author/s.
Ethics Statement
The animal study was reviewed and approved by the Institutional Animal Care and Use Committee at the National Institute of Advanced Industrial Science and Technology.
Author Contributions
YS and TK designed the experiments and wrote and revised the manuscript. YS performed histological and molecular experiments. Both authors approved the submitted version of the manuscript.
Funding
This work was supported by JSPS KAKENHI Grant Numbers 18K14824 and 21K15195, and internal grant of AIST, Japan.
Conflict of Interest
The authors declare that the research was conducted in the absence of any commercial or financial relationships that could be construed as a potential conflict of interest.
Supplementary Material
The Supplementary Material for this article can be found online at: https://www.frontiersin.org/articles/10.3389/fcell.2021.686755/full#supplementary-material
References
Adams, K. L., and Gallo, V. (2018). The diversity and disparity of the glial scar. Nat. Neurosci. 21, 9–15. doi: 10.1038/s41593-017-0033-9
Adolf, B., Chapouton, P., Lam, C. S., Topp, S., Tannhauser, B., Strahle, U., et al. (2006). Conserved and acquired features of adult neurogenesis in the zebrafish telencephalon. Dev. Biol. 295, 278–293. doi: 10.1016/j.ydbio.2006.03.023
Akimenko, M. A., Johnson, S. L., Westerfield, M., and Ekker, M. (1995). Differential induction of four msx homeobox genes during fin development and regeneration in zebrafish. Development 121, 347–357.
Alunni, A., and Bally-Cuif, L. (2016). A comparative view of regenerative neurogenesis in vertebrates. Development 143, 741–753. doi: 10.1242/dev.122796
Alunni, A., Hermel, J. M., Heuzé, A., Bourrat, F., Jamen, F., and Joly, J. S. (2010). Evidence for neural stem cells in the medaka optic tectum proliferation zones. Dev. Neurobiol. 70, 693–713. doi: 10.1002/dneu.20799
Anderson, M. A., Burda, J. E., Ren, Y., Ao, Y., O’Shea, T. M., Kawaguchi, R., et al. (2016). Astrocyte scar formation aids central nervous system axon regeneration. Nature 532, 195–200. doi: 10.1038/nature17623
Arneson, D., Zhang, G., Ying, Z., Zhuang, Y., Byun, H. R., Ahn, I. S., et al. (2018). Single cell molecular alterations reveal target cells and pathways of concussive brain injury. Nat. Commun. 9:3894. doi: 10.1038/s41467-018-06222-0
Baumgart, E. V., Barbosa, J. S., Bally-Cuif, L., Götz, M., and Ninkovic, J. (2012). Stab wound injury of the zebrafish telencephalon: a model for comparative analysis of reactive gliosis. Glia 60, 343–357. doi: 10.1002/glia.22269
Becker, T., Wullimann, M. F., Becker, C. G., Bernhardt, R. R., and Schachner, M. (1997). Axonal regrowth after spinal cord transection in adult zebrafish. J. Comp. Neurol. 377, 577–595.
Bernstein, J. J. (1964). Relation of spinal cord regeneration to age in adult goldfish. Exp. Neurol. 1964, 161–174. doi: 10.1016/0014-4886(64)90014-7
Bisese, E. C., Ciuba, C. M., Davidson, A. L., Kaushik, A., Mullen, S. M., Barth, J. L., et al. (2019). The acute transcriptome response of the midbrain/diencephalon to injury in the adult mummichog (Fundulus heteroclitus). Mol. Brain 12:119. doi: 10.1186/s13041-019-0542-4
Burda, J. E., Bernstein, A. M., and Sofroniew, M. V. (2016). Astrocyte roles in traumatic brain injury. Exp. Neurol. 275, 305–315. doi: 10.1016/j.expneurol.2015.03.020
Dambroise, E., Simion, M., Bourquard, T., Bouffard, S., Rizzi, B., Jaszczyszyn, Y., et al. (2017). Postembryonic fish brain proliferation zones exhibit neuroepithelial-type gene expression profile. Stem Cells 35, 1505–1518. doi: 10.1002/stem.2588
Demirci, Y., Cucun, G., Poyraz, Y. K., Mohammed, S., Heger, G., Papatheodorou, I., et al. (2020). Comparative transcriptome analysis of the regenerating zebrafish telencephalon unravels a resource with key pathways during two early stages and activation of wnt/β-catenin signaling at the early wound healing stage. Front. Cell Dev. Biol. 8:584604. doi: 10.3389/fcell.2020.584604
Diotel, N., Lübke, L., Strähle, U., and Rastegar, S. (2020). Common and distinct features of adult neurogenesis and regeneration in the telencephalon of zebrafish and mammals. Front. Neurosci. 14:568930. doi: 10.3389/fnins.2020.568930
Fausett, B. V., Gumerson, J. D., and Goldman, D. (2008). The proneural basic helix-loop-helix gene ascl1a is required for retina regeneration. J. Neurosci. 28, 1109–1117. doi: 10.1523/JNEUROSCI.4853-07.2008
Feeney, D. M., Boyeson, M. G., Linn, R. T., Murray, H. M., and Dail, W. G. (1981). Responses to cortical injury: I. Methodology and local effects of contusions in the rat. Brain Res. 211, 67–77. doi: 10.1016/0006-8993(81)90067-6
Galant, S., Furlan, G., Coolen, M., Dirian, L., Foucher, I., and Bally-Cuif, L. (2016). Embryonic origin and lineage hierarchies of the neural progenitor subtypes building the zebrafish adult midbrain. Dev. Biol. 420, 120–135. doi: 10.1016/j.ydbio.2016.09.022
Goldman, D. (2014). Müller glial cell reprogramming and retina regeneration. Nat. Rev. Neurosci. 15, 431–442. doi: 10.1038/nrn3723
Gorsuch, R. A., Lahne, M., Yarka, C. E., Petravick, M. E., Li, J., and Hyde, D. R. (2017). Sox2 regulates Muller glia reprogramming and proliferation in the regenerating zebrafish retina via Lin28 and Ascl1a. Exp. Eye Res. 161, 174–192. doi: 10.1016/j.exer.2017.05.012
Grandel, H., Kaslin, J., Ganz, J., Wenzel, I., and Brand, M. (2006). Neural stem cells and neurogenesis in the adult zebrafish brain: origin, proliferation dynamics, migration and cell fate. Dev. Biol. 295, 263–277. doi: 10.1016/j.ydbio.2006.03.040
Herrmann, J. E., Imura, T., Song, B., Qi, J., Ao, Y., Nguyen, T. K., et al. (2008). STAT3 is a critical regulator of astrogliosis and scar formation after spinal cord injury. J. Neurosci. 28, 7231–7243. doi: 10.1523/JNEUROSCI.1709-08.2008
Hoang, T., Wang, J., Boyd, P., Wang, F., Santiago, C., Jiang, L., et al. (2020). Gene regulatory networks controlling vertebrate retinal regeneration. Science 370:eabb8598. doi: 10.1126/science.abb8598
Hozumi, I., Chiu, F. C., and Norton, W. T. (1990). Biochemical and immunocytochemical changes in glial fibrillary acidic protein after stab wounds. Brain Res. 524, 64–71. doi: 10.1016/0006-8993(90)90492-t
Ito, K., Morioka, M., Kimura, S., Tasaki, M., Inohaya, K., and Kudo, A. (2014). Differential reparative phenotypes between zebrafish and medaka after cardiac injury. Dev. Dyn. 243, 1106–1115. doi: 10.1002/dvdy.24154
Ito, Y., Tanaka, H., Okamoto, H., and Ohshima, T. (2010). Characterization of neural stem cells and their progeny in the adult zebrafish optic tectum. Dev. Biol. 342, 26–38. doi: 10.1016/j.ydbio.2010.03.008
Jorstad, N. L., Wilken, M. S., Todd, L., Finkbeiner, C., Nakamura, P., Radulovich, N., et al. (2020). STAT signaling modifies Ascl1 chromatin binding and limits neural regeneration from muller glia in adult mouse retina. Cell Rep. 30, 2195–2208. doi: 10.1016/j.celrep.2020.01.075
Kang, J., Hu, J., Karra, R., Dickson, A. L., Tornini, V. A., Nachtrab, G., et al. (2016). Modulation of tissue repair by regeneration enhancer elements. Nature 532, 201–206. doi: 10.1038/nature17644
Karl, M. O., Hayes, S., Nelson, B. R., Tan, K., Buckingham, B., and Reh, T. A. (2008). Stimulation of neural regeneration in the mouse retina. Proc. Natl. Acad. Sci. U.S.A. 105, 19508–19513. doi: 10.1073/pnas.0807453105
Kaslin, J., Kroehne, V., Ganz, J., Hans, S., and Brand, M. (2017). Distinct roles of neuroepithelial-like and radial glia-like progenitor cells in cerebellar regeneration. Development 144, 1462–1471. doi: 10.1242/dev.144907
Katogi, R., Nakatani, Y., Shin-i, T., Kohara, Y., Inohaya, K., and Kudo, A. (2004). Large-scale analysis of the genes involved in fin regeneration and blastema formation in the medaka Oryzias latipes. Mech. Dev. 121, 861–872. doi: 10.1016/j.mod.2004.03.015
Kikuchi, K., and Poss, K. D. (2012). Cardiac regenerative capacity and mechanisms. Annu. Rev. Cell Dev. Biol. 28, 719–741. doi: 10.1146/annurev-cellbio-101011-155739
Kishimoto, N., Shimizu, K., and Sawamoto, K. (2012). Neuronal regeneration in a zebrafish model of adult brain injury. Dis. Model Mech. 5, 200–209. doi: 10.1242/dmm.007336
Kiyooka, M., Shimizu, Y., and Ohshima, T. (2020). Histone deacetylase inhibition promotes regenerative neurogenesis after stab wound injury in the adult zebrafish optic tectum. Biochem. Biophys. Res. Commun. 529, 366–371. doi: 10.1016/j.bbrc.2020.06.025
Kizil, C., Kaslin, J., Kroehne, V., and Brand, M. (2012). Adult neurogenesis and brain regeneration in zebrafish. Dev. Neurobiol. 72, 429–461. doi: 10.1002/dneu.20918
Kroehne, V., Freudenreich, D., Hans, S., Kaslin, J., and Brand, M. (2011). Regeneration of the adult zebrafish brain from neurogenic radial glia-type progenitors. Development 138, 4831–4841. doi: 10.1242/dev.072587
Kuroyanagi, Y., Okuyama, T., Suehiro, Y., Imada, H., Shimada, A., Naruse, K., et al. (2010). Proliferation zones in adult medaka (Oryzias latipes) brain. Brain Res. 1323, 33–40. doi: 10.1016/j.brainres.2010.01.045
Labusch, M., Mancini, L., Morizet, D., and Bally-Cuif, L. (2020). Conserved and divergent features of adult neurogenesis in zebrafish. Front. Cell Dev. Biol. 8:525. doi: 10.3389/fcell.2020.00525
Lai, S. L., Marín-Juez, R., Moura, P. L., Kuenne, C., Lai, J. K. H., Tsedeke, A. T., et al. (2017). Reciprocal analyses in zebrafish and medaka reveal that harnessing the immune response promotes cardiac regeneration. eLife 6:e25605. doi: 10.7554/eLife.25605
Lindsey, B. W., Aitken, G. E., Tang, J. K., Khabooshan, M., Douek, A. M., Vandestadt, C., et al. (2019). Midbrain tectal stem cells display diverse regenerative capacities in zebrafish. Sci. Rep. 9:4420. doi: 10.1038/s41598-019-40734-z
Llorens-Bobadilla, E., Zhao, S., Baser, A., Saiz-Castro, G., Zwadlo, K., and Martin-Villalba, A. (2015). Single-cell transcriptomics reveals a population of dormant neural stem cells that become activated upon brain injury. Cell Stem Cell 17, 329–340. doi: 10.1016/j.stem.2015.07.002
Lust, K., and Wittbrodt, J. (2018). Activating the regenerative potential of Müller glia cells in a regeneration-deficient retina. eLife 7:e32319. doi: 10.7554/eLife.32319
März, M., Schmidt, R., Rastegar, S., and Strähle, U. (2011). Regenerative response following stab injury in the adult zebrafish telencephalon. Dev. Dyn. 240, 2221–2231. doi: 10.1002/dvdy.22710
McKeon, R. J., Schreiber, R. C., Rudge, J. S., and Silver, J. (1991). Reduction of neurite outgrowth in a model of glial scarring following CNS injury is correlated with the expression of inhibitory molecules on reactive astrocytes. J. Neurosci. 11, 3398–3411. doi: 10.1523/JNEUROSCI.11-11-03398.1991
Moss, J. B., Koustubhan, P., Greenman, M., Parsons, M. J., Walter, I., and Moss, L. G. (2009). Regeneration of the pancreas in adult zebrafish. Diabetes 58, 1844–1851. doi: 10.2337/db08-0628
Nelson, C. M., Gorsuch, R. A., Bailey, T. J., Ackerman, K. M., Kassen, S. C., and Hyde, D. R. (2012). Stat3 defines three populations of Muller glia and is required for initiating maximal muller glia proliferation in the regenerating zebrafish retina. J. Comp. Neurol. 520, 4294–4311. doi: 10.1002/cne.23213
Otsuka, T., and Takeda, H. (2017). Targeted ablation of pancreatic beta cells in medaka. Zoolog. Sci. 34, 179–184. doi: 10.2108/zs170004
Poss, K. D., Wilson, L. G., and Keating, M. T. (2002). Heart regeneration in zebrafish. Science 298, 2188–2190. doi: 10.1126/science.1077857
Pushchina, E. V., Kapustyanov, I. A., and Varaksin, A. A. (2020). Neural Stem cells/neuronal precursor cells and postmitotic neuroblasts in constitutive neurogenesis and after, traumatic injury to the mesencephalic tegmentum of juvenile chum salmon, oncorhynchus keta. Brain Sci. 10:65. doi: 10.3390/brainsci10020065
Pushchina, E. V., Zharikova, E. I., and Varaksin, A. A. (2017). Persistent and reparative neurogenesis in the juvenile masu salmon Oncorhynchus masou telencephalon after mechanical injury. Russ. J. Dev. Biol. 48, 307–320.
Ramachandran, R., Fausett, B. V., and Goldman, D. (2010). Ascl1a regulates Müller glia dedifferentiation and retinal regeneration through a Lin-28-dependent, let-7 microRNA signalling pathway. Nat. Cell Biol. 2010, 1101–1107. doi: 10.1038/ncb2115
Raymond, P. A., Barthel, L. K., Bernardos, R. L., and Perkowski, J. J. (2006). Molecular characterization of retinal stem cells and their niches in adult zebrafish. BMC Dev. Biol. 6:36. doi: 10.1186/1471-213X-6-36
Sharma, P., Gupta, S., Chaudhary, M., Mitra, S., Chawla, B., Khursheed, M. A., et al. (2019). Oct4 mediates Muller glia reprogramming and cell cycle exit during retina regeneration in zebrafish. Life Sci. Alliance 2:e201900548. doi: 10.26508/lsa.201900548
Shimizu, Y., Ueda, Y., and Ohshima, T. (2018). Wnt signaling regulates proliferation and differentiation of radial glia in regenerative processes after stab injury in the optic tectum of adult zebrafish. Glia 66, 1382–1394. doi: 10.1002/glia.23311
Simões, F. C., Cahill, T. J., Kenyon, A., Gavriouchkina, D., Vieira, J. M., Sun, X., et al. (2020). Macrophages directly contribute collagen to scar formation during zebrafish heart regeneration and mouse heart repair. Nat. Commun. 11:600. doi: 10.1038/s41467-019-14263-2
Sîrbulescu, R. F., Iliȩ, I., and Zupanc, G. K. (2009). Structural and functional regeneration after spinal cord injury in the weakly electric teleost fish, Apteronotus leptorhynchus. J. Comp. Physiol. Neuroethol. Sens. Neural Behav. Physiol. 195, 699–714. doi: 10.1007/s00359-009-0445-4
Smith, D. H., Soares, H. D., Pierce, J. S., Perlman, K. G., Saatman, K. E., Meaney, D. F., et al. (1995). A model of parasagittal controlled cortical impact in the mouse: cognitive and histopathologic effects. J. Neurotrauma 12, 169–178. doi: 10.1089/neu.1995.12.169
Soltani, A. R., Motamedi, M., and Teimori, A. (2020). Adult neuronal regeneration in the telencephalon of the killifish Aphaniops hormuzensis. J. Exp. Zool. B Mol. Dev. Evol. 334, 350–361. doi: 10.1002/jez.b.23008
Stevenson, J. A., and Yoon, M. G. (1978). Regeneration of optic nerve fibers enhances cell proliferation in the goldfish optic tectum. Brain Res. 153, 345–351. doi: 10.1016/0006-8993(78)90413-4
Takeuchi, A., and Okubo, K. (2013). Post-proliferative immature radial glial cells female-specifically express aromatase in the medaka optic tectum. PLoS One 8:e73663. doi: 10.1371/journal.pone.0073663
Ueda, Y., Shimizu, Y., Shimizu, N., Ishitani, T., and Ohshima, T. (2018). Involvement of sonic hedgehog and notch signaling in regenerative neurogenesis in adult zebrafish optic tectum after stab injury. J. Comp. Neurol. 526, 2360–2372. doi: 10.1002/cne.24489
Van houcke, J., Mariën, V., Zandecki, C., Vanhunsel, S., Moons, L., Ayana, R., et al. (2021). Aging impairs the essential contributions of non-glial progenitors to neurorepair in the dorsal telencephalon of the Killifish N. furzeri. Biorxiv [Preprint] doi: 10.1101/2021.02.26.433041
Xiong, Y., Mahmood, A., and Chopp, M. (2013). Animal models of traumatic brain injury. Nat. Rev. Neurosci. 14, 128–142. doi: 10.1038/nrn3407
Yang, T., Dai, Y., Chen, G., and Cui, S. (2020). Dissecting the dual role of the glial scar and scar-forming astrocytes in spinal cord injury. Front. Cell Neurosci. 14:78. doi: 10.3389/fncel.2020.00078
Yu, S., and He, J. (2019). Stochastic cell-cycle entry and cell-state-dependent fate outputs of injury-reactivated tectal radial glia in zebrafish. eLife 8:e48660. doi: 10.7554/eLife.48660
Zhao, X. F., Wan, J., Powell, C., Ramachandran, R., Myers, M. G. Jr., and Goldman, D. (2014). Leptin and IL-6 family cytokines synergize to stimulate Muller glia reprogramming and retina regeneration. Cell Rep. 9, 272–284. doi: 10.1016/j.celrep.2014.08.047
Zhong, J., Jiang, L., Cheng, C., Huang, Z., Zhang, H., Liu, H., et al. (2016). Altered expression of long non-coding RNA and mRNA in mouse cortex after traumatic brain injury. Brain Res. 1646, 589–600. doi: 10.1016/j.brainres.2016.07.002
Keywords: radial glia, stab wound injury, optic tectum, neuronal differentiation, reactive gliosis, zebrafish, medaka
Citation: Shimizu Y and Kawasaki T (2021) Differential Regenerative Capacity of the Optic Tectum of Adult Medaka and Zebrafish. Front. Cell Dev. Biol. 9:686755. doi: 10.3389/fcell.2021.686755
Received: 27 March 2021; Accepted: 01 June 2021;
Published: 29 June 2021.
Edited by:
Igor Schneider, Federal University of Pará, BrazilReviewed by:
Katharina Lust, Research Institute of Molecular Pathology (IMP), AustriaSepand Rastegar, Karlsruhe Institute of Technology (KIT), Germany
Karen Mruk, University of Wyoming, United States
Copyright © 2021 Shimizu and Kawasaki. This is an open-access article distributed under the terms of the Creative Commons Attribution License (CC BY). The use, distribution or reproduction in other forums is permitted, provided the original author(s) and the copyright owner(s) are credited and that the original publication in this journal is cited, in accordance with accepted academic practice. No use, distribution or reproduction is permitted which does not comply with these terms.
*Correspondence: Yuki Shimizu, eXVraS5zaGltaXp1QGFpc3QuZ28uanA=