- 1Institute for Stem Cell Research, Helmholtz Zentrum München, Munich, Germany
- 2Physiological Genomics, Biomedical Center, Ludwig-Maximilians-Universität, Munich, Germany
- 3Graduate School of Systemic Neurosciences, Ludwig-Maximilians-Universität, Munich, Germany
- 4SyNergy, Munich Cluster for Systems Neurology, Ludwig-Maximilians-Universität, Munich, Germany
In this perspective article, we briefly review tools for stable gain-of-function expression to explore key fate determinants in embryonic brain development. As the piggyBac transposon system has the highest insert size, a seamless integration of the transposed sequence into the host genome, and can be delivered by transfection avoiding viral vectors causing an immune response, we explored its use in the murine developing forebrain. The original piggyBac transposase PBase or the mouse codon-optimized version mPB and the construct to insert, contained in the piggyBac transposon, were introduced by in utero electroporation at embryonic day 13 into radial glia, the neural stem cells, in the developing dorsal telencephalon, and analyzed 3 or 5 days later. When using PBase, we observed an increase in basal progenitor cells, often accompanied by folding aberrations. These effects were considerably ameliorated when using the piggyBac plasmid together with mPB. While size and strength of the electroporated region was not correlated to the aberrations, integration was essential and the positive correlation to the insert size implicates the frequency of transposition as a possible mechanism. We discuss this in light of the increase in transposing endogenous viral vectors during mammalian phylogeny and their role in neurogenesis and radial glial cells. Most importantly, we aim to alert the users of this system to the phenotypes caused by non-codon optimized PBase application in vivo.
Introduction
Much has been learnt about fate determinants in development during neurogenesis and gliogenesis from candidate approaches, natural gene mutations and genome-wide expression analyses. To elucidate their function, and subsequently use them e.g., for direct neuronal reprogramming or therapeutic approaches, we need reliable tools for the long-term manipulation of gene expression, e.g., by seamless genomic integration. Tools for this are still relatively limited and mostly rely on viral vectors. The piggyBac transposon system allows genomic integration and long-term expression of genes of interest, providing a viable alternative as described below. These approaches are now timely to review and consider for specific pitfalls, due to the immense power of their potential for therapeutic use, e.g., by direct neuronal reprogramming in vivo (Götz and Bocchi, 2021). This applies especially as the CRISPR/Cas technology now facilitates multiplexing, targeting double-digit numbers of genes without problem (Breunig et al., 2018, 2021). This will help understanding the role of transcriptional networks in development, or to manipulate cell fate in a fine-tuned manner—e.g., to achieve correct subtype specification of glia or neurons in reprogramming—but needs to be delivered and integrated in a safe, yet reliable manner.
An important consideration in the use of integrating constructs is the similarity to endogenous transposable elements (TE), which play an important role in development. In humans, these make up the majority of the genome, with up to 69% suggested to be TE (de Koning et al., 2011; Ferrari et al., 2021). In the developing brain, the mobilization and (re-)integration of TE, especially of the endogenous retroviral LINE-1 elements, is linked to the development of neuronal subtype diversity and genomic mosaicism, and dysregulation may lead to developmental abnormalities (see e.g., Bodea et al., 2018; Misiak et al., 2019).
We will therefore review specific approaches to genome editing with an emphasis on gain-of-function and highlight a not yet reported and hitherto not yet fully explained artifact using the piggyBac transposon system in vivo.
Tools for Stable Expression of Genes of Interest
Viral Vectors for Long-Term Gene Expression
Viral vectors are a classical tool for the long-term manipulation of gene expression. Especially Retrovirus (RV) and Lentivirus (LV) are often used both in vitro and in vivo, e.g., to elucidate the function of candidate genes in embryonic development (Artegiani and Calegari, 2013), or in direct neuronal reprogramming approaches (Gascón et al., 2016; Herrero-Navarro et al., 2021; Russo et al., 2021). A big advantage of viral vectors is the ability to target specific cell types of the brain by pseudotyping the capsid (Buffo et al., 2008; Mattugini et al., 2019). Both virus types achieve long-term gene expression by integrating their reverse-transcribed RNA genome into the host genome as DNA (Figure 1A) at semi-random sites, LV preferring active transcription units, while RV favor enhancers and regulatory sites (Schröder et al., 2002). This semi-random integration has the disadvantage of potential off-target effects and the introduction of mutations. While expression systems have been optimized to avoid gene silencing (Pfeifer et al., 2002) and reduce the immune response (Piras et al., 2017; Russo et al., 2021), the limited packaging capacity and the immune reactions still elicited by these viral vectors and their pseudotypes (Mattugini et al., 2019) remain disadvantageous.
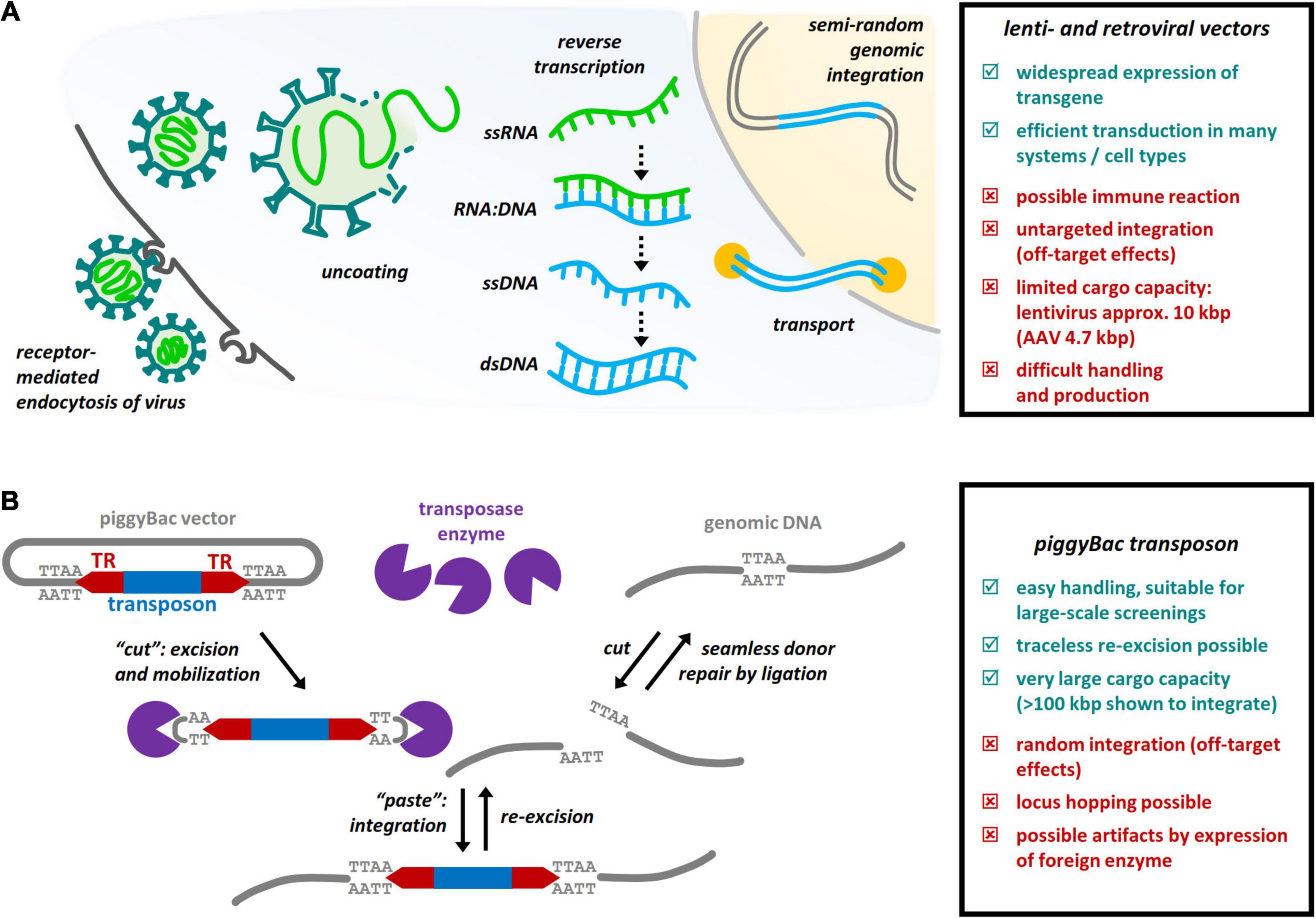
Figure 1. Common methods of genome editing in neural stem cells in vivo. (A) Retro- or lentiviral transduction. The virus is introduced by receptor-mediated endocytosis depending on the pseudotype of the capsid, which is then degraded to uncoat the viral genome. This is retrotranscribed from ssRNA to dsDNA, transported to the cell’s nucleus and integrated into the genome, with preferred integration sites dependent on the type of virus. (B) Integration of the piggyBac transposon. In the presence of its specific transposase, the piggyBac transposon can be integrated to the genome in a “cut-and-paste” manner. TTAA motifs in the terminal repeats (TR) regions are recognized by the transposase which excises and mobilizes the transposon. The genomic DNA is cut at TTAA sites and the transposon is integrated by ligation. In the same way, the transposon can be re-excised, with a seamless re-ligation repairing the host’s donor site.
For translational therapeutic approaches, adeno-associated virus (AAV) is better suited due to the limited immune response (see e.g., Mattugini et al., 2019; Rittiner et al., 2020). A multitude of serotypes allow targeting specific cell types even via non-invasive, e.g., intravenous, application routes (Haggerty et al., 2020; Nectow and Nestler, 2020; Rittiner et al., 2020). While allowing long-term stable gene expression depending on the cell type, these vectors stay episomal, i.e., do not integrate into the host genome, but may be used to deliver constructs that are themselves able to integrate (such as transposon systems) or to permanently edit the host genome (e.g., CRISPR/Cas9). However, their biggest disadvantage is the small packaging size of less than 5 kBp (Rittiner et al., 2020), and although it can be overcome by splitting some proteins, e.g., Cas9, into two parts (Truong et al., 2015), it generally limits the applicability of these vectors.
Transposable Elements for Widespread Genomic Integration
The piggyBac system has been discovered as a transposable element decades ago, initially in insect cells (Cary et al., 1989), and was used to generate transgenic vertebrate models with stable inheritance (Ding et al., 2005). A huge advantage is the ability to accommodate large constructs, with sequences of 100 kBp reported to successfully integrate in the genome (Li et al., 2011). There seems to be no bias for integration into specific chromosomes, but a strong preference to integrate into accessible chromatin and highly transcribed genes (Elick et al., 1996; Ding et al., 2005; Wang et al., 2008; Li et al., 2013; Yoshida et al., 2017).
The piggyBac transposon is characterized by terminal repeats ending in TTAA that are necessary for successful transposition (Figure 1B; Cary et al., 1989; Li et al., 2005). The construct to insert, i.e., the transposon, by itself shows little integration, but when paired with its specific transposase even in low amounts, integration is very efficient (Ding et al., 2005; Cadinanos and Bradley, 2007; Wang et al., 2008). In mammalian cells, as in its native system, the piggyBac transposon integrates into the host genome exclusively at TTAA sites (Figure 1B), with the specific transposase enzyme nicking the DNA and inducing transient double strand breaks which are repaired not by DNA synthesis, but rather ligation of the ends. This leads to seamless integration and traceless excision of the transposon (Mitra et al., 2008; Chen et al., 2020).
Due to efficient cloning strategies, the piggyBac system has readily lent itself for screenings in vivo (Xu et al., 2017), and the possibility of removing the transgene without a footprint by transposase-mediated excision (Woltjen et al., 2009; Behringer et al., 2017) is promising for translational approaches. The application of the piggyBac transposon for in vivo studies has been further encouraged by the development of improved transposase enzymes that, based on the original PBase, have been optimized for their use e.g., in mammalian systems. A mouse-codon optimized version of the enzyme, mPB, has been shown to elicit a 20-fold higher transposition activity in mouse ES cells as compared to the non-optimized PBase, likely due to higher expression levels following the facilitation of the translation process (Cadinanos and Bradley, 2007). Further optimization yielded an even more efficient enzyme, the hyperactive transposase hyPBase, by introducing point mutations in the sequence of mPB (Yusa et al., 2011).
The Use of piggyBac Transposon in the Developing Brain in vivo—A Call for Codon Optimization
Given the advantages of the piggyBac transposon system, we tested different transposases for gene manipulation in the developing cortex. For plasmid DNA, such as the piggyBac transposon, a favored delivery method is in utero electroporation (IUE), often performed during mid-gestation between E12 and E15 (Saito, 2006; Meyer-Dilhet and Courchet, 2020).
With the aim of later activating endogenous gene expression, we first administered the original transposase enzyme PBase (Supplementary Figures 1A,B) and a piggyBac transposon containing a fusion protein of enzymatically deactivated Cas9 and GFP (dCas9-GFP, Supplementary Figure 1B) into radial glia by IUE during mid-neurogenesis at E13 (Figure 2A). After 3 days, all of the brains showed ectopia of cells expressing the stem cell marker PAX6 and the basal progenitor (BP) marker TBR2 (Figure 2C). These markers are normally found in a distinctive band in the ventricular and subventricular zone, respectively (Götz et al., 1998; Englund et al., 2005).
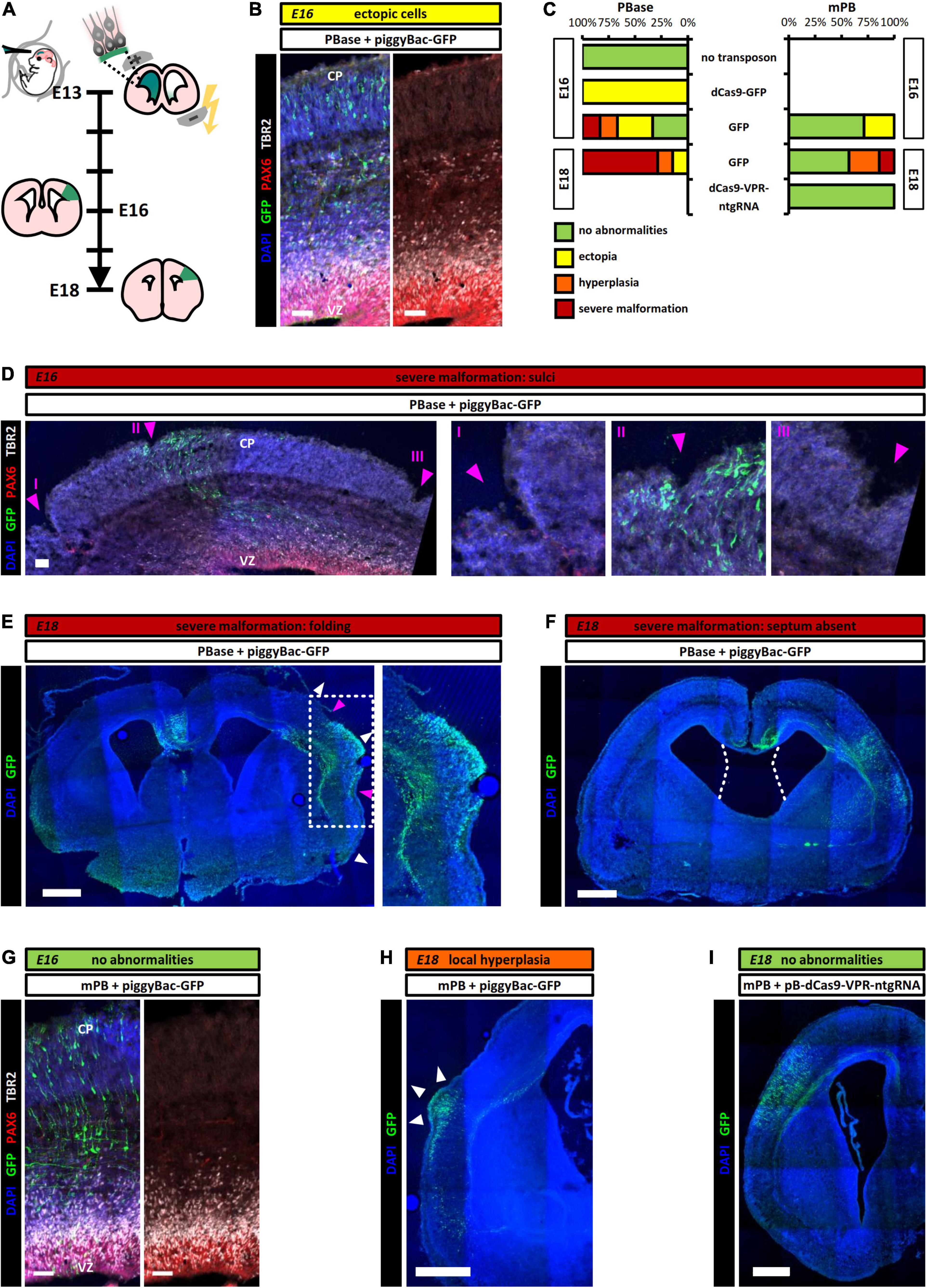
Figure 2. Unexpected abnormalities in cortex development upon electroporation of PBase, but not mPB. (A) Experimental schedule. piggyBac transposon plasmids as indicated in Supplementary Figure 1 were delivered into the cortex by IUE at E13 and brains were analyzed at E16 or E18. (B) At E16 (3 days post-IUE), transposition of piggyBac-GFP by the original piggyBac transposase PBase leads to different phenotypes, such as an accumulation of ectopic PAX6+/TBR2+ cells in the intermediate zone of the cerebral cortex (VZ = ventricular zone, CP = cortical plate; scale bar: 50 μm). (C) Quantification of the different phenotypes at time points indicated in the graphs. mPB leads to a lower penetrance and milder phenotypes, while PBase causes strong developmental aberrations depending on the co-electroporated transposon. Shorter transposons lead to an exacerbated effect, no abnormalities develop in the absence of a transposon. n = 6 for PBase/no transposon (E16), PBase + dCas9-GFP (E16), and PBase + GFP (E16); n = 7 for mPB + GFP (E16), PBase + GFP (E18), and mPB + GFP (E18); n = 4 for mPB + dCas9-VPR-ntgRNA (E18). (D) Example of severe developmental aberration caused by PBase transposition at E16, here leading to the formation of sulcus-like structures at and further away from the electroporation site (Scale bar: 50 μm). (E) At E18 (5 days post-IUE), the phenotype of PBase-mediated transposition of piggyBac-GFP is exacerbated, with severe malformation mostly manifesting in folding of the cortex at and further away from the electroporation site (Scale bar: 500 μm). (F) Another example of severe malformation by PBase IUE is lack of the medial region (the septum) normally separating the lateral ventricles (Scale bar: 500 μm). (G) At E16, IUE of mPB mostly led to a normal phenotype with no ectopic cells (Scale bar: 50 μm). (H) Some animals showed developmental abnormalities at E18 after mPB IUE, e.g., local hyperplasia with increased cortical thickness at the electroporation site (Scale bar: 500 μm). (I) With mPB-mediated integration of the largest transposon (piggyBac-dCas9-VPR-ntgRNA), no phenotypes were observed (Scale bar: 500 μm).
To test if the observed phenotype may be caused by the expression of the foreign protein dCas9, we repeated the experiment with a simpler piggyBac transposon containing only a GFP reporter (Supplementary Figure 1B). We again observed developmental abnormalities in the electroporated brains after 3 days (Figures 2B,C). However, the variability here was much higher, ranging from some brains exhibiting no abnormalities, over the previously observed PAX6+/TBR2+ ectopia, to one embryo exhibiting severe malformations, where the cortex showed several sulcus-like indentations. We noted also a non-cell autonomous phenotype, as the folds were found not only at the electroporation site, but also at more distant positions (Figure 2D).
We then introduced a plasmid only carrying the GFP reporter, lacking the terminal repeats recognized by the transposase enzyme and thus without the possibility to integrate genomically (pCAG-IRES-GFP, Supplementary Figure 1B). In this case, no aberrant phenotype was observed (Figure 2C); a significant difference from the effects elicited by both piggyBac constructs (padj = 0.026 for piggyBac-GFP and padj = 0.005 for piggyBac-dCas9-GFP; Supplementary Table 1). Thus, the observed aberrations are dependent on the integration and transposition activity of the piggyBac system.
To determine if the phenotypes may be transient, we examined the brains later at E18, 5 days after IUE. Now all PBase-expressing brains showed an aberrant and in most cases exacerbated phenotype with anatomical malformations (Figure 2C). These could be distinguished into two levels of severity, with some cortices only showing localized hyperplasia at the electroporation site, whereas others exhibited more severe and widespread malformations, such as folded gyrus- and sulcus-like structures (Figure 2E), and, in one case, no septum with fusion of the lateral ventricles (Figure 2F).
As the PBase enzyme originates from insect cells (Cary et al., 1989), the sequence and codon usage is not adapted to the translation machinery in murine cells. The use of rare codons may influence the ribosomal translation speed and lead to ribosome collisions, a ribotoxic response (RTR) (Wu et al., 2020), and subsequently an unfolded protein stress response (UPR) (Cao and Kaufman, 2012). We thus exchanged the transposase PBase for mPB (Supplementary Figures 1A,B), the mouse codon optimized version of the same enzyme (Cadinanos and Bradley, 2007). This encodes the same amino acid sequence, generating an identical enzyme, but has an altered nucleotide sequence better suited to the murine translation machinery. When expressed together with piggyBac-GFP, only few brains exhibited PAX6+/TBR2+ ectopia at E16 and most brains did not show any abnormalities (Figures 2C,G).
At E18, we again observed a striking difference between the two versions of the transposase enzyme: Upon IUE of PBase, all brains showed aberrations, in most cases severe, while the phenotype of mPB was considerably milder and observed less frequently (Figures 2C,H), showing a significantly different overall phenotype severity between both enzymes (p = 0.017, Supplementary Table 1). Indeed, a piggyBac plasmid containing a dCas9-VPR fusion protein as well as a non-targeted gRNA (ntgRNA) and a GFP reporter (Supplementary Figure 1B), did not elicit any abnormalities 5 days after IUE, when combined with mPB (Figures 2C,I). Thus, we strongly recommend to use the codon-optimized mPB to avoid developmental aberrations.
Exploring the Cause of Developmental Defects Caused by PBase Application
The above results imply a striking difference between the effects of the codon optimized and the non-codon optimized version of the same enzyme. Both proteins have the same amino acid sequence, but mPB has been found to enable an integration and transposition activity up to 20 times higher than PBase, likely due to a higher expression level in otherwise identical systems (Cadinanos and Bradley, 2007). To test if this is also the case in the developing cortex, we measured the size and intensity of IUE at E18 for the mPB and PBase constructs combined with piggyBac-GFP. There was no detectable difference in the size (Supplementary Figure 2A) or the GFP intensity (Supplementary Figure 2B) of the IUEs between the different transposases, and also no correlation between larger or stronger IUEs and phenotypic alterations.
Next, we asked if the difference in the translation of the transposase enzymes, which is optimized for mPB and suboptimal for PBase due to different codon usage (Cadinanos and Bradley, 2007), may be relevant and elicit differences in the RTR, UPR or DNA damage and double-strand breaks (Ciccia and Elledge, 2010). To explore this, we transfected the different transposase constructs with different piggyBac transposons into adherent neural progenitor cells derived from embryonic cortex (Pollard et al., 2006), but found no upregulation in marker genes for either of these pathways using qRT-PCR (Supplementary Figure 3). Despite PBase being expressed under a stronger promoter (pCAG vs. pCMV for mPB; Supplementary Figure 1B), when measuring transposase mRNA levels, we found a considerably higher expression of mPB than PBase (Supplementary Figure 3B) as suggested previously for expression under the same promoters (Cadinanos and Bradley, 2007). Thus, the promoter seems to have little effect on expression, and despite higher expression levels, mPB causes fewer developmental aberrations.
Discussion
After reviewing different methods for stable gain-of-function expression systems, we show here an unexpected artifact of the piggyBac transposon system in the developing cortex. Adverse developmental effects clearly depended on the integration of the constructs, suggesting that integration and/or transposition is important. In this regard it is interesting to note that there seems to be a connection between the size of the transposon, ranging between 3.0 and 9.6 kBp, and the severity of the phenotype, as smaller transposons elicited a more severe phenotype than larger ones (Figure 2C and Supplementary Figure 1B). It has been shown that the size of the transposon influences the rate of transposition, which starts decreasing considerably around 9 kBp (Ding et al., 2005). This may suggest that smaller constructs with potentially higher transposition rate are more prone to cause the observed abnormalities. Notably, the expression level of the transposase enzymes (Supplementary Figure 3B) seems not to affect the phenotype severity, possibly due to a saturated ratio of constantly expressed transposase enzyme to the limited number of available transposons. Once bound to the enzyme, only the length of the transposon may influence the speed or efficiency (and thereby the frequency) of the transposition process.
“Locus hopping” in the continued presence of the transposase enzyme may cause problems due to its preference for transcriptionally active sites (Ding et al., 2005; Li et al., 2013), which may disrupt their expression and alter transcription networks, the likelihood of which increases with the transposition activity and each excision and re-integration event. We would therefore suggest to develop mPB constructs that self-inactivate after integration of the transposon has been achieved, to avoid continued transposition events. Additionally, the link to endogenous transposition events has to be considered: While it has been shown that piggyBac transposase is unable to mobilize endogenous, PB-like transposons in mouse or human cells (Yusa et al., 2011; Saha et al., 2015), an endogenous human transposase highly expressed in neurons, PGDB5, has been suggested to potentially mobilize piggyBac transposons (Henssen et al., 2015).
It is intriguing that specifically the generation of PAX6+ and/or TBR2+ BPs and folding of the brain were affected, as these cells comprise an important glial subtype, the basal radial glial cells, and are particularly frequent in species with folded cortices (Borrell and Götz, 2014). Interestingly, in the developing gyrified cortex, they occur in a blockwise manner, with folds formed at the sites of expanded basal subventricular zone where basal radial glial cells are most frequent (Borrell and Götz, 2014). This organization can be mimicked even in the normally smooth murine cortex e.g., by locally lowering the levels of TRNP1, a protein involved in nuclear phase transition (Esgleas et al., 2020). Depletion of the protein, which in murine cortex is normally expressed in a salt-and-pepper fashion, in a specific region by IUE artificially causes blockwise expression, generating a region of expanded subventricular zone and basal radial glial cells, and accordingly induces cortical folding (Stahl et al., 2013; Borrell and Götz, 2014). We do not know if the ectopic BPs and folding that we observed upon PBase-mediated transposition after IUE activate a natural endogenous program, but it is intriguing to see how readily BP number and position may be influenced.
As noted above, the phenotype we observed was not strictly cell autonomous, as was also the case in the TRNP1 manipulations (Stahl et al., 2013; Esgleas et al., 2020) and other means to expand BP numbers (Rash et al., 2013; Xie et al., 2019). It will thus be very important to understand, if—and which—cell surface molecules or secreted signals may be regulated by these manipulations. Given the important communication via secreted vesicles in the cerebrospinal fluid (Marzesco et al., 2005; Morton and Feliciano, 2016), these may be involved in non-cell autonomous communication within the cortex tissue.
While the human cortex is naturally gyrified, the developmental disorder polymicrogyria (PMG) is characterized by the generation of additional, aberrant gyri in the neocortex. While PMG is commonly considered a neuronal migration disorder (Romero et al., 2018), several studies have also suggested causative progenitor and proliferation aberrations. Mutations in cyclin D2 were linked to a cortical malformation syndrome including PMG in patients, and IUE of these mutated genes caused an increased proliferation of progenitors and impaired cell-cycle exit in the developing mouse cortex (Mirzaa et al., 2014). In addition, PMG patients with mutations in the Pax6 as well as Tbr2 genes have been identified (Mitchell et al., 2003; Baala et al., 2007), which may relate to the data presented here with ectopic PAX6+ or TBR2+ cells and additional folds in this study.
While it is not immediately evident why the transposition would cause an increase in BPs, it is intriguing to consider that the naturally occurring transposition by recently integrated endogenous retroviruses (ERVs) may play a similar role, as their proportion hugely increases in the mammalian and especially the primate genome, correlating to cortex size and gyrification (Linker et al., 2017). Indeed, ERVs can regulate the expression of neighboring genes (Fasching et al., 2015; Brattas et al., 2017; Jonsson et al., 2019, 2020; Petri et al., 2019) and may thus have evolved as a novel gene regulatory machinery involved in enlarging and folding brain regions (Ferrari et al., 2021).
It may thus be interesting to follow up the artifact caused by the piggyBac transposon system mechanistically, by controlling transposition events and the generation of BPs. For the use of the piggyBac transposon system it is important to use codon optimized mPB and develop transposase removal strategies to minimize transposition after initial integration. Foremost, however, it is important to be aware of this pitfall, which has so far not been reported, and how to optimize the system as detailed here.
Data Availability Statement
The raw data supporting the conclusions of this article will be made available by the authors, without undue reservation.
Ethics Statement
The animal study was reviewed and approved by the Government of Upper Bavaria.
Author Contributions
MG and FV conceived, conceptualized the project, and wrote the perspective. FV performed all experiments and analyses shown. MK aided in preliminary experiments revealing the phenotype difference between transposase enzymes. All authors contributed to the article and approved the submitted version.
Funding
This work was funded by the advanced ERC grants ChroNeuroRepair and NeuroCentro to MG.
Conflict of Interest
The authors declare that the research was conducted in the absence of any commercial or financial relationships that could be construed as a potential conflict of interest.
Publisher’s Note
All claims expressed in this article are solely those of the authors and do not necessarily represent those of their affiliated organizations, or those of the publisher, the editors and the reviewers. Any product that may be evaluated in this article, or claim that may be made by its manufacturer, is not guaranteed or endorsed by the publisher.
Acknowledgments
We thank Rebeca Sánchez González, Germán Camargo Ortega, Anna Köferle, Miriam Esgleas, and Christopher Breunig for providing plasmid constructs and Stefan Stricker, Maximilian Wiesbeck, and Danny Nedialkova for excellent discussions and suggestions during the project. We are very grateful for excellent technical support from Andrea Steiner-Mezzadri and Gabi Horn. Data in this perspective, Figure 1B and Supplementary Figures 2, 3 were previously published in the dissertation of Vierl (2021).
Supplementary Material
The Supplementary Material for this article can be found online at: https://www.frontiersin.org/articles/10.3389/fcell.2021.698002/full#supplementary-material
References
Artegiani, B., and Calegari, F. (2013). Lentiviruses allow widespread and conditional manipulation of gene expression in the developing mouse brain. Development 140, 2818–2822. doi: 10.1242/dev.093823
Baala, L., Briault, S., Etchevers, H. C., Laumonnier, F., Natiq, A., Amiel, J., et al. (2007). Homozygous silencing of T-box transcription factor EOMES leads to microcephaly with polymicrogyria and corpus callosum agenesis. Nat. Genet. 39, 454–456. doi: 10.1038/ng1993
Behringer, R., Gertsenstein, M., Nagy, K. V., and Nagy, A. (2017). Reprogramming Mouse Fibroblasts with piggyBac Transposons. Cold Spring Harb. Protoc. 2017:dbrot092627. doi: 10.1101/pdb.prot092627
Bodea, G. O., McKelvey, E. G. Z., and Faulkner, G. J. (2018). Retrotransposon-induced mosaicism in the neural genome. Open Biol. 8:180074. doi: 10.1098/rsob.180074
Borrell, V., and Götz, M. (2014). Role of radial glial cells in cerebral cortex folding. Curr. Opin. Neurobiol. 27, 39–46. doi: 10.1016/j.conb.2014.02.007
Brattas, P. L., Jonsson, M. E., Fasching, L., Nelander Wahlestedt, J., Shahsavani, M., Falk, R., et al. (2017). TRIM28 Controls a Gene Regulatory Network Based on Endogenous Retroviruses in Human Neural Progenitor Cells. Cell Rep. 18, 1–11. doi: 10.1016/j.celrep.2016.12.010
Breunig, C. T., Durovic, T., Neuner, A. M., Baumann, V., Wiesbeck, M. F., Koferle, A., et al. (2018). One step generation of customizable gRNA vectors for multiplex CRISPR approaches through string assembly gRNA cloning (STAgR). PLoS One 13:e0196015. doi: 10.1371/journal.pone.0196015
Breunig, C. T., Koferle, A., Neuner, A. M., Wiesbeck, M. F., Baumann, V., and Stricker, S. H. (2021). CRISPR Tools for Physiology and Cell State Changes: potential of Transcriptional Engineering and Epigenome Editing. Physiol. Rev. 101, 177–211. doi: 10.1152/physrev.00034.2019
Buffo, A., Rite, I., Tripathi, P., Lepier, A., Colak, D., Horn, A. P., et al. (2008). Origin and progeny of reactive gliosis: a source of multipotent cells in the injured brain. Proc. Natl. Acad. Sci. U. S. A. 105, 3581–3586. doi: 10.1073/pnas.0709002105
Cadinanos, J., and Bradley, A. (2007). Generation of an inducible and optimized piggyBac transposon system. Nucleic Acids Res. 35:e87. doi: 10.1093/nar/gkm446
Cao, S. S., and Kaufman, R. J. (2012). Unfolded protein response. Curr. Biol. 22, R622–R626. doi: 10.1016/j.cub.2012.07.004
Cary, L. C., Goebel, M., Corsaro, B. G., Wang, H.-G., Rosen, E., and Fraser, M. J. (1989). Transposon mutagenesis of baculoviruses: analysis of Trichoplusia ni transposon IFP2 insertions within the FP-locus of nuclear polyhedrosis viruses. Virology 172, 156–169. doi: 10.1016/0042-6822(89)90117-7
Chen, Q., Luo, W., Veach, R. A., Hickman, A. B., Wilson, M. H., and Dyda, F. (2020). Structural basis of seamless excision and specific targeting by piggyBac transposase. Nat. Commun. 11:3446. doi: 10.1038/s41467-020-17128-1
Ciccia, A., and Elledge, S. J. (2010). The DNA damage response: making it safe to play with knives. Mol. Cell 40, 179–204. doi: 10.1016/j.molcel.2010.09.019
de Koning, A. P., Gu, W., Castoe, T. A., Batzer, M. A., and Pollock, D. D. (2011). Repetitive elements may comprise over two-thirds of the human genome. PLoS Genet. 7:e1002384. doi: 10.1371/journal.pgen.1002384
Ding, S., Wu, X., Li, G., Han, M., Zhuang, Y., and Xu, T. (2005). Efficient transposition of the piggyBac (PB) transposon in mammalian cells and mice. Cell 122, 473–483. doi: 10.1016/j.cell.2005.07.013
Elick, T. A., Bauser, C. A., and Fraser, M. J. (1996). Excision of the piggyBac transposable element in vitro is a precise event that is enhanced by the expression of its encoded transposase. Genetica 98, 33–41. doi: 10.1007/BF00120216
Englund, C., Fink, A., Lau, C., Pham, D., Daza, R. A., Bulfone, A., et al. (2005). Pax6, Tbr2, and Tbr1 are expressed sequentially by radial glia, intermediate progenitor cells, and postmitotic neurons in developing neocortex. J. Neurosci. 25, 247–251. doi: 10.1523/JNEUROSCI.2899-04.2005
Esgleas, M., Falk, S., Forne, I., Thiry, M., Najas, S., Zhang, S., et al. (2020). Trnp1 organizes diverse nuclear membrane-less compartments in neural stem cells. EMBO J. 39:e103373. doi: 10.15252/embj.2019103373
Fasching, L., Kapopoulou, A., Sachdeva, R., Petri, R., Jonsson, M. E., Manne, C., et al. (2015). TRIM28 represses transcription of endogenous retroviruses in neural progenitor cells. Cell Rep. 10, 20–28. doi: 10.1016/j.celrep.2014.12.004
Ferrari, R., Grandi, N., Tramontano, E., and Dieci, G. (2021). Retrotransposons as Drivers of Mammalian Brain Evolution. Life 11:376. doi: 10.3390/life11050376
Gascón, S., Murenu, E., Masserdotti, G., Ortega, F., Russo, G. L., Petrik, D., et al. (2016). Identification and Successful Negotiation of a Metabolic Checkpoint in Direct Neuronal Reprogramming. Cell Stem Cell 18, 396–409. doi: 10.1016/j.stem.2015.12.003
Götz, M., and Bocchi, R. (2021). Neuronal replacement: concepts, achievements, and call for caution. Curr. Opin. Neurobiol. 69, 185–192. doi: 10.1016/j.conb.2021.03.014
Götz, M., Stoykova, A., and Gruss, P. (1998). Pax6 Controls Radial Glia Differentiation in the Cerebral Cortex. Neuron 21, 1031–1044. doi: 10.1016/s0896-6273(00)80621-2
Haggerty, D. L., Grecco, G. G., Reeves, K. C., and Atwood, B. (2020). Adeno-Associated Viral Vectors in Neuroscience Research. Mol. Ther. Methods Clin. Dev. 17, 69–82. doi: 10.1016/j.omtm.2019.11.012
Henssen, A. G., Henaff, E., Jiang, E., Eisenberg, A. R., Carson, J. R., Villasante, C. M., et al. (2015). Genomic DNA transposition induced by human PGBD5. Elife 4:e10565. doi: 10.7554/eLife.10565
Herrero-Navarro, A., Puche-Aroca, L., Moreno-Juan, V., Sempere-Ferrandez, A., Espinosa, A., Susin, R., et al. (2021). Astrocytes and neurons share region-specific transcriptional signatures that confer regional identity to neuronal reprogramming. Sci. Adv. 7:eabe8978. doi: 10.1126/sciadv.abe8978
Jonsson, M. E., Garza, R., Johansson, P. A., and Jakobsson, J. (2020). Transposable Elements: a Common Feature of Neurodevelopmental and Neurodegenerative Disorders. Trends Genet. 36, 610–623. doi: 10.1016/j.tig.2020.05.004
Jonsson, M. E., Ludvik Brattas, P., Gustafsson, C., Petri, R., Yudovich, D., Pircs, K., et al. (2019). Activation of neuronal genes via LINE-1 elements upon global DNA demethylation in human neural progenitors. Nat. Commun. 10:3182. doi: 10.1038/s41467-019-11150-8
Li, M. A., Pettitt, S. J., Eckert, S., Ning, Z., Rice, S., Cadinanos, J., et al. (2013). The piggyBac transposon displays local and distant reintegration preferences and can cause mutations at noncanonical integration sites. Mol. Cell Biol. 33, 1317–1330. doi: 10.1128/MCB.00670-12
Li, M. A., Turner, D. J., Ning, Z., Yusa, K., Liang, Q., Eckert, S., et al. (2011). Mobilization of giant piggyBac transposons in the mouse genome. Nucleic Acids Res. 39:e148. doi: 10.1093/nar/gkr764
Li, X., Harrell, R. A., Handler, A. M., Beam, T., Hennessy, K., and Fraser, M. J. Jr. (2005). piggyBac internal sequences are necessary for efficient transformation of target genomes. Insect Mol. Biol. 14, 17–30. doi: 10.1111/j.1365-2583.2004.00525.x
Linker, S. B., Marchetto, M. C., Narvaiza, I., Denli, A. M., and Gage, F. H. (2017). Examining non-LTR retrotransposons in the context of the evolving primate brain. BMC Biol. 15:68. doi: 10.1186/s12915-017-0409-z
Marzesco, A. M., Janich, P., Wilsch-Brauninger, M., Dubreuil, V., Langenfeld, K., Corbeil, D., et al. (2005). Release of extracellular membrane particles carrying the stem cell marker prominin-1 (CD133) from neural progenitors and other epithelial cells. J. Cell Sci. 118, 2849–2858. doi: 10.1242/jcs.02439
Mattugini, N., Bocchi, R., Scheuss, V., Russo, G. L., Torper, O., Lao, C. L., et al. (2019). Inducing Different Neuronal Subtypes from Astrocytes in the Injured Mouse Cerebral Cortex. Neuron 103, 1086–1095.e5. doi: 10.1016/j.neuron.2019.08.009
Meyer-Dilhet, G., and Courchet, J. (2020). In Utero Cortical Electroporation of Plasmids in the Mouse Embryo. STAR Protoc. 1:100027. doi: 10.1016/j.xpro.2020.100027
Mirzaa, G., Parry, D. A., Fry, A. E., Giamanco, K. A., Schwartzentruber, J., Vanstone, M., et al. (2014). De novo CCND2 mutations leading to stabilization of cyclin D2 cause megalencephaly-polymicrogyria-polydactyly-hydrocephalus syndrome. Nat. Genet. 46, 510–515. doi: 10.1038/ng.2948
Misiak, B., Ricceri, L., and Sasiadek, M. M. (2019). Transposable Elements and Their Epigenetic Regulation in Mental Disorders: current Evidence in the Field. Front. Genet. 10:580. doi: 10.3389/fgene.2019.00580
Mitchell, T. N., Free, S. L., Williamson, K. A., Stevens, J. M., Churchill, A. J., Hanson, I. M., et al. (2003). Polymicrogyria and absence of pineal gland due to PAX6 mutation. Ann. Neurol. 53, 658–663. doi: 10.1002/ana.10576
Mitra, R., Fain-Thornton, J., and Craig, N. L. (2008). piggyBac can bypass DNA synthesis during cut and paste transposition. EMBO J. 27, 1097–1109. doi: 10.1038/emboj.2008.41
Morton, M. C., and Feliciano, D. M. (2016). Neurovesicles in Brain Development. Cell. Mol. Neurobiol. 36, 409–416. doi: 10.1007/s10571-015-0297-0
Nectow, A. R., and Nestler, E. J. (2020). Viral tools for neuroscience. Nat. Rev. Neurosci. 21, 669–681. doi: 10.1038/s41583-020-00382-z
Petri, R., Brattas, P. L., Sharma, Y., Jonsson, M. E., Pircs, K., Bengzon, J., et al. (2019). LINE-2 transposable elements are a source of functional human microRNAs and target sites. PLoS Genet. 15:e1008036. doi: 10.1371/journal.pgen.1008036
Pfeifer, A., Ikawa, M., Dayn, Y., and Verma, I. M. (2002). Transgenesis by lentiviral vectors: lack of gene silencing in mammalian embryonic stem cells and preimplantation embryos. Proc. Natl. Acad. Sci. U. S. A. 99, 2140–2145. doi: 10.1073/pnas.251682798
Piras, F., Riba, M., Petrillo, C., Lazarevic, D., Cuccovillo, I., Bartolaccini, S., et al. (2017). Lentiviral vectors escape innate sensing but trigger p53 in human hematopoietic stem and progenitor cells. EMBO Mol. Med. 9, 1198–1211. doi: 10.15252/emmm.201707922
Pollard, S. M., Conti, L., Sun, Y., Goffredo, D., and Smith, A. (2006). Adherent neural stem (NS) cells from fetal and adult forebrain. Cereb. Cortex 16, i112–i120. doi: 10.1093/cercor/bhj167
Rash, B. G., Tomasi, S., Lim, H. D., Suh, C. Y., and Vaccarino, F. M. (2013). Cortical gyrification induced by fibroblast growth factor 2 in the mouse brain. J. Neurosci. 33, 10802–10814. doi: 10.1523/JNEUROSCI.3621-12.2013
Rittiner, J. E., Moncalvo, M., Chiba-Falek, O., and Kantor, B. (2020). Gene-Editing Technologies Paired With Viral Vectors for Translational Research Into Neurodegenerative Diseases. Front. Mol. Neurosci. 13:148. doi: 10.3389/fnmol.2020.00148
Romero, D. M., Bahi-Buisson, N., and Francis, F. (2018). Genetics and mechanisms leading to human cortical malformations. Semin. Cell Dev. Biol. 76, 33–75. doi: 10.1016/j.semcdb.2017.09.031
Russo, G. L., Sonsalla, G., Natarajan, P., Breunig, C. T., Bulli, G., Merl-Pham, J., et al. (2021). CRISPR-Mediated Induction of Neuron-Enriched Mitochondrial Proteins Boosts Direct Glia-to-Neuron Conversion. Cell Stem Cell 28, 524–534.e7. doi: 10.1016/j.stem.2020.10.015
Saha, S., Woodard, L. E., Charron, E. M., Welch, R. C., Rooney, C. M., and Wilson, M. H. (2015). Evaluating the potential for undesired genomic effects of the piggyBac transposon system in human cells. Nucleic Acids Res. 43, 1770–1782. doi: 10.1093/nar/gkv017
Saito, T. (2006). In vivo electroporation in the embryonic mouse central nervous system. Nat. Protoc. 1, 1552–1558. doi: 10.1038/nprot.2006.276
Schröder, A. R. W., Shinn, P., Chen, H., Berry, C., Ecker, J. R., and Bushman, F. (2002). HIV-1 Integration in the Human Genome Favors Active Genes and Local Hotspots. Cell 110, 521–529. doi: 10.1016/s0092-8674(02)00864-4
Stahl, R., Walcher, T., De Juan Romero, C., Pilz, G. A., Cappello, S., Irmler, M., et al. (2013). Trnp1 regulates expansion and folding of the mammalian cerebral cortex by control of radial glial fate. Cell 153, 535–549. doi: 10.1016/j.cell.2013.03.027
Truong, D. J., Kuhner, K., Kuhn, R., Werfel, S., Engelhardt, S., Wurst, W., et al. (2015). Development of an intein-mediated split-Cas9 system for gene therapy. Nucleic Acids Res. 43, 6450–6458. doi: 10.1093/nar/gkv601
Vierl, F. (2021). Identification of Neurogenic Fate Determinants Aiming to Prolong Cortical Neurogenesis. Ph.D. thesis, Ludwig-Maximilians-Universität München, Germany.
Wang, W., Lin, C., Lu, D., Ning, Z., Cox, T., Melvin, D., et al. (2008). Chromosomal transposition of PiggyBac in mouse embryonic stem cells. Proc. Natl. Acad. Sci. U. S. A. 105, 9290–9295. doi: 10.1073/pnas.0801017105
Woltjen, K., Michael, I. P., Mohseni, P., Desai, R., Mileikovsky, M., Hamalainen, R., et al. (2009). piggyBac transposition reprograms fibroblasts to induced pluripotent stem cells. Nature 458, 766–770. doi: 10.1038/nature07863
Wu, C. C., Peterson, A., Zinshteyn, B., Regot, S., and Green, R. (2020). Ribosome Collisions Trigger General Stress Responses to Regulate Cell Fate. Cell 182, 404–416.e14. doi: 10.1016/j.cell.2020.06.006
Xie, Y., Castro-Hernandez, R., Sokpor, G., Pham, L., Narayanan, R., Rosenbusch, J., et al. (2019). RBM15 Modulates the Function of Chromatin Remodeling Factor BAF155 Through RNA Methylation in Developing Cortex. Mol. Neurobiol. 56, 7305–7320. doi: 10.1007/s12035-019-1595-1
Xu, C., Qi, X., Du, X., Zou, H., Gao, F., Feng, T., et al. (2017). piggyBac mediates efficient in vivo CRISPR library screening for tumorigenesis in mice. Proc. Natl. Acad. Sci. U. S. A. 114, 722–727. doi: 10.1073/pnas.1615735114
Yoshida, J., Akagi, K., Misawa, R., Kokubu, C., Takeda, J., and Horie, K. (2017). Chromatin states shape insertion profiles of the piggyBac, Tol2 and Sleeping Beauty transposons and murine leukemia virus. Sci. Rep. 7:43613. doi: 10.1038/srep43613
Keywords: genome integration, transposon, viral vectors, neural stem cells, cortex development, gyrification
Citation: Vierl F, Kaur M and Götz M (2021) Non-codon Optimized PiggyBac Transposase Induces Developmental Brain Aberrations: A Call for in vivo Analysis. Front. Cell Dev. Biol. 9:698002. doi: 10.3389/fcell.2021.698002
Received: 20 April 2021; Accepted: 14 July 2021;
Published: 03 August 2021.
Edited by:
Stefanie Robel, Virginia Tech, United StatesReviewed by:
Wei-Hsiang Huang, McGill University, CanadaAshley Cooney, The University of Iowa, United States
Thomas Pratt, University of Edinburgh, United Kingdom
Copyright © 2021 Vierl, Kaur and Götz. This is an open-access article distributed under the terms of the Creative Commons Attribution License (CC BY). The use, distribution or reproduction in other forums is permitted, provided the original author(s) and the copyright owner(s) are credited and that the original publication in this journal is cited, in accordance with accepted academic practice. No use, distribution or reproduction is permitted which does not comply with these terms.
*Correspondence: Magdalena Götz, bWFnZGFsZW5hLmdvZXR6QGhlbG1ob2x0ei1tdWVuY2hlbi5kZQ==