- 1German Consortium for Translational Cancer Research (DKTK), Heidelberg, Germany
- 2Department of Hematology and Oncology, University Medical Center Mainz, Mainz, Germany
- 3German Cancer Research Center (DKFZ), Heidelberg, Germany
Mesenchymal stromal cells (MSCs) are a heterogenous cell population found in a wide range of tissues in the body, known for their nutrient-producing and immunomodulatory functions. In the bone marrow (BM), these MSCs are critical for the regulation of hematopoietic stem cells (HSC) that are responsible for daily blood production and functional immunity throughout an entire organism’s lifespan. Alongside other stromal cells, MSCs form a specialized microenvironment BM tissue called “niche” that tightly controls HSC self-renewal and differentiation. In addition, MSCs are crucial players in maintaining bone integrity and supply of hormonal nutrients due to their capacity to differentiate into osteoblasts and adipocytes which also contribute to cellular composition of the BM niche. However, MSCs are known to encompass a large heterogenous cell population that remains elusive and poorly defined. In this review, we focus on deciphering the BM-MSC biology through recent advances in single-cell identification of hierarchical subsets with distinct functionalities and transcriptional profiles. We also discuss the contribution of MSCs and their osteo-adipo progeny in modulating the complex direct cell-to-cell or indirect soluble factors-mediated interactions of the BM HSC niche during homeostasis, aging and myeloid malignancies. Lastly, we examine the therapeutic potential of MSCs for rejuvenation and anti-tumor remedy in clinical settings.
Introduction
Located within specific anatomical zones of the skeleton, the bone marrow (BM) is a specialized microenvironment or “niche” that lodges cells of hematopoietic and mesenchymal origins in various hierarchical committed states. The main role of the BM niche is the tight control of cell-fate decisions of the hematopoietic stem cells (HSCs) and their progeny to sustain the daily supply in functional blood and immune cells throughout life. These environmental cues are produced by a variety of stromal cells that constitute the BM niche which mainly include neurons, endothelial cells and mesenchymal stromal cells (MSCs) (Pinho and Frenette, 2019). The latter are considered a versatile stem cell population due to their capacity to differentiate into bone (osteoblasts), cartilage (chondrocytes) and fat cells (adipocytes), thus playing a central role in HSCs maintenance, BM niche composition and life-long turnover and bone growth (Bianco and Robey, 2015). Due to their fibroblastic nature and heterogenous origin, MSCs have been referred to in the literature under different names which were accounted for in this review. In addition, prominent gene reporter-mouse models that helped investigate the role of stromal populations in the BM led to synonymous use of the reporter strains themselves as putative markers for MSC populations, which are different from their human counterparts (see Table 1). However, current consensus divided MSCs into subgroups based on their anatomical location which influence both their functional and phenotypic potentialities. Therefore, within the scope of this review, we refer to the nomenclature proposed by Matsuzaki et al. (2014) and revised by Ambrosi et al. (2019); according to which MSCs are defined as bone marrow stromal cells bearing trilineage potential and expressing both Leptin receptor (LEPR) and PDGF-receptor α (PDGFR-α) in human and mouse (see Figure 1). Acknowledging the presence of further heterogeneity within the MSCs compartment, we will review major niche factors contributed by the MSCs and their osteo-adipo progeny in sustaining hematopoiesis. We will also present the most recent advances in identifying MSCs subset heterogeneity and cellular hierarchy by single cell technologies and their impact on remodeling the BM during aging and myeloid leukemias. Consequently, we will highlight possible therapeutic options in targeting MSCs in clinical settings.
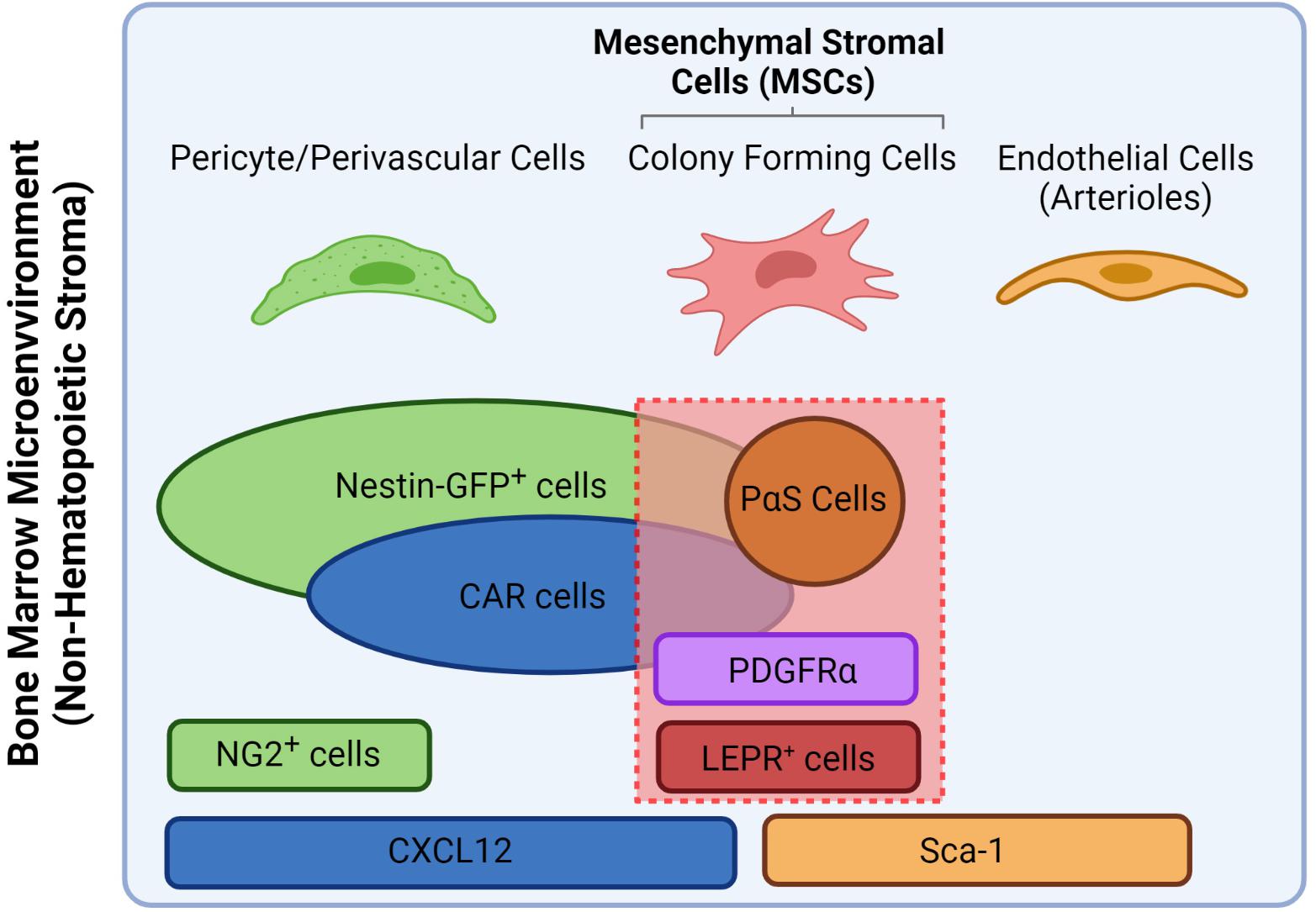
Figure 1. Nomenclature overview of different stromal populations including putative and gene markers and how they relate to MSCs. For the scope of this review, MSCs are defined as all colony forming cells that express both PDGFR-α and LEPR (Matsuzaki et al., 2014). PαS stand for PDGFR-α+/Sca-1+. Figure was generated using Biorender.com.
Functional MSC Heterogeneity: Location and Progeny Matters
The BM niche can be divided into two distinct regions based on the location of the cells, vascular flow and oxygen conditions they are exposed to which consequently define functional differences between MSCs within these distinct niche sites (see Figure 2):
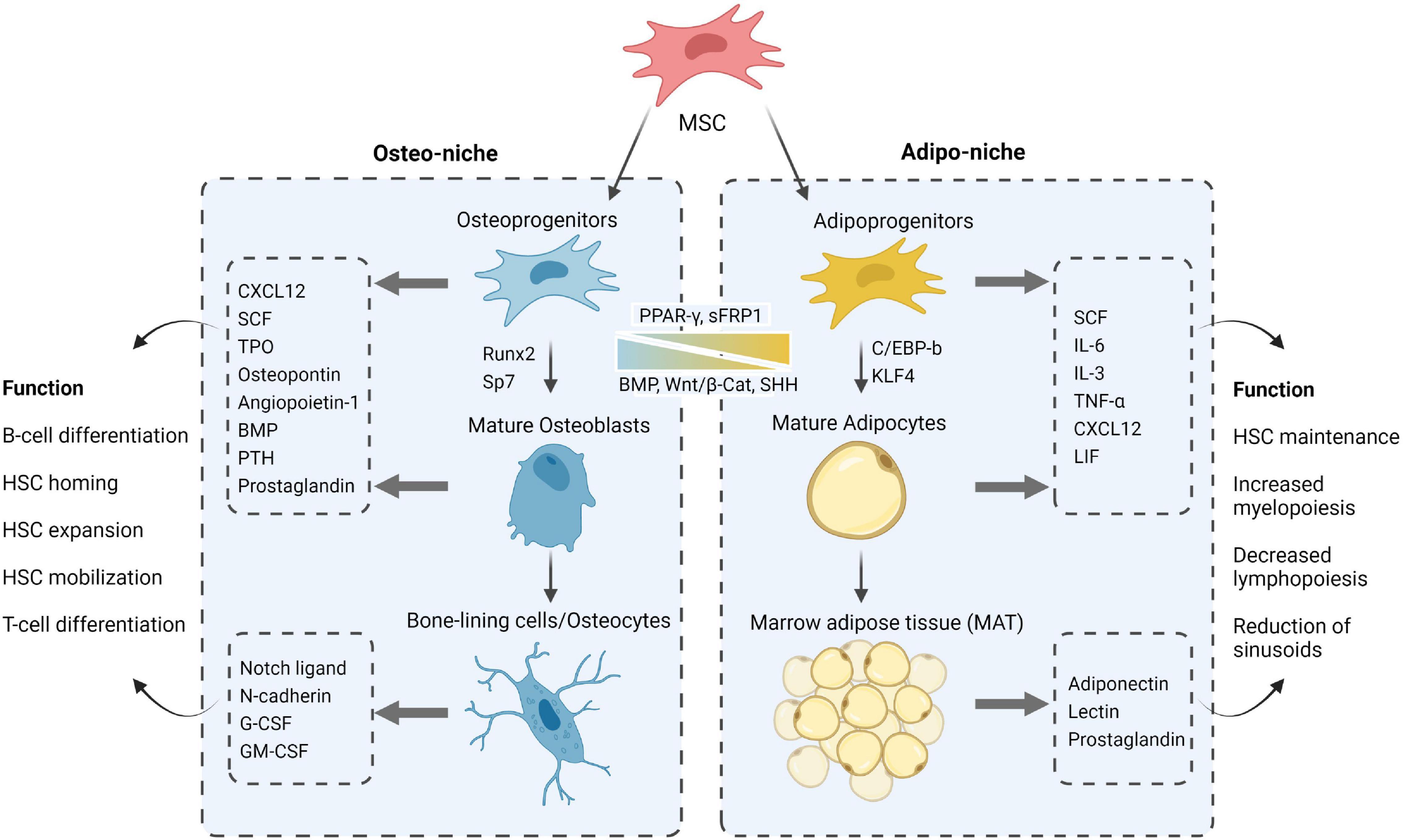
Figure 2. A closer look at the MSC progeny, their constituting roles via secreted factors and differentiation cues toward adipo- and osteogenesis in the respective niches. Figure was generated using Biorender.com.
The endosteal bone marrow niche represents 10% of total BM volume and comprises the MSCs with high osteolineage capacity including osteoprogenitors, osteoblasts, and osteocytes, which populate the inner surface of the bone along small arterioles and capillary vessels (Méndez-Ferrer et al., 2020). NG2+ pericytes and MSCs along with their osteo-progeny were shown to promote HSC quiescence through secretion of pro-survival and homing factors such as C-X-C Motif Chemokine Ligand 12 (CXCL12) (Wei and Frenette, 2018), Angiopoietin-1 (Ang-1) (Arai et al., 2004), thrombopoietin (TPO) (Yoshihara et al., 2007), and Notch ligands (Calvi et al., 2003; Guezguez et al., 2013); thereby reinforcing their tight contact with osteoblasts and maintaining the HSCs in a long-term non-cycling status (Qian et al., 2007; Loeffler and Schroeder, 2021). In accordance, the osteocalcin+ osteoblasts have been identified as a supportive “layer” niche due to their organization in follicle-like structures which surround HSCs and bind to them via N-cadherin- and Notch/Jagged1 mediated cell-cell interactions (Calvi et al., 2003; Zhang et al., 2003; Lawal et al., 2017). More recent reports indicate that the regulation of hematopoiesis by the osteolineage may also depend on it differentiation state (Sacchetti et al., 2007; Méndez-Ferrer et al., 2010; Calvi et al., 2012; He et al., 2017), as well as the close spatial localization of HSCs with the bone-lining cells of the endosteal niche (Lo Celso et al., 2009; Xie et al., 2009; Guezguez et al., 2013; Kim et al., 2017). These physical osteoblastic niche interactions controlling HSC fate are extensively influenced by a profusion of autocrine, paracrine, and endocrine factors such as bone morphogenetic proteins (Jung et al., 2008; Goldman et al., 2009; Khurana et al., 2014; Guo et al., 2018), growth factors (Yoon et al., 2012, 2017; Caselli et al., 2013), prostaglandins (Frisch et al., 2009; Hoggatt et al., 2009, 2013), shared cytokines/chemokines (Sugiyama et al., 2006; Ding and Morrison, 2013; Brylka and Schinke, 2019) and hormones such as the parathyroid hormone (PTH) (Calvi et al., 2001, 2003; Kuznetsov et al., 2004; Li et al., 2012). Although all of these molecules appear to be essential cornerstones for the preservation of bone microarchitecture and stem/progenitor cell homeostatic features within the BM, PTH has been identified as a key osteo-niche element linking MSCs and HSCs activities functionally and spatially (Adams et al., 2007; Li et al., 2012; Yu et al., 2012; Yao et al., 2014; Wein and Kronenberg, 2018). Additionally, osteoprogenitors were shown to be indispensable for B-cell differentiation by the release of Interleukin-7 (IL-7) and Insulin Growth Factor (IGF-1) which are critical for the maturation steps of B-cell progenitors (Wu et al., 2008; Yu et al., 2016). On the other hand, osteocytes were shown to restrict myelopoiesis by secreting granulocyte colony-stimulating factor (G-CSF) an important factor in HSC mobilization (Fulzele et al., 2013). The interdependence of endosteal BM niche inhabitants and the multifaceted signaling of MSCs and their osteo-lineage progeny in controlling HSC functions continue to be the object of intense investigation.
The central/perivascular bone marrow niche delineates 90% of total BM volume and englobes most of the vasculature that is enveloped with a variety of cells, including MSCs, pericytes, neurons along with adipocytes, which populate the central region of the bone shaft (Méndez-Ferrer et al., 2020). The BM vasculature in this region is enriched with arterioles that branch with thin-walled and fenestrated blood vessels called sinusoids. This endothelial architecture allows for the tight balance in the retention and activation of HSCs as well as the trafficking of their progenitors and mature immune cells back and forth the BM (Itkin et al., 2016). Along secretion of CXCL12, the LEPR+-MSCs enveloping the sinusoids are shown to produce stem cell factor (SCF, also known as KITL) that is required for long-term preservation of HSCs in the BM (Ding et al., 2012). Adipocytes, known to be a rich source in nutrients for the BM, also produce a variety of cytokines and factors involved in HSCs maintenance (SCF, IL-3, IL-6, CXCL12) (Kumar and Geiger, 2017) as well as inhibitors of hematopoiesis such as TGF-β1, a mediator of cell-cycle arrest (Scandura et al., 2004; Brenet et al., 2013) and lipocalin 2 (LCN2) that inhibits erythroid differentiation (Miharada et al., 2008). More intriguingly, accumulation of adipocytes as marrow adipose tissue (MAT) was also shown to reduce blood flow and suppress hematopoiesis through reduction of sinusoid caliber and microvasculature pruning (Scheller et al., 2016).
Overall, accumulating evidence has demonstrated a balance of MSCs differentiation commitment between osteoblastic and adipocytic lineages; as well as mutual dependency to ensure homeostasis that can be derailed during aging, chronic stress or cancer (Rendina-Ruedy and Rosen, 2017). However, possible feedback signals between osteo-adipo lineage and their parental MSCs as well as their impact on BM niche biology remains to be elucidated.
Single-Cell MSC Heterogeneity: Lesson From Single Cell RNA Sequencing
MSC Heterogeneity in the Murine Bone Marrow
With the advance of single-cell RNA sequencing technologies (scRNA-seq), traditionally homogenous cell populations reveal functionally different subclasses. The same is true for MSCs; recent well-designed scRNA-seq studies from different stromal gene-reporter mice shed some light on the murine bone marrow and help us to identify subclasses of MSCs. However, results from these studies varied greatly in number of identified MSC subsets due to different methods of BM extraction, cell sorting and sequencing depth (see Table 2). In summary, both “adipogenic” and “osteogenic” clusters can be identified regardless of the gene-reporter or surface MSC marker (LEPR+, CD51-/Sca1+, PDGFR- α+, Col2+) (Tikhonova et al., 2019; Wolock et al., 2019; Baccin et al., 2020; Zhong et al., 2020). Depending on gene set signatures, MSC can be subdivided into subsets with less differentiated and more stem-like features that are defined as mesenchymal progenitors or mesenchymal stem cells (Tikhonova et al., 2019; Zhong et al., 2020). Additionally, some of these studies also discerned “intermediate” MSC populations, suggesting that adipogenic and osteogenic differentiation is a continuous process with little definite cell states in-between (Tikhonova et al., 2019; Wolock et al., 2019; Leimkühler et al., 2021), as shown recently for the HSC compartment (Liggett and Sankaran, 2020).
MSC Heterogeneity in the Human Bone Marrow
There are few comparable scRNA-seq studies of the MSC heterogeneity in human. This is in parts due to the scarcity of material and the difficulties in getting consistent cell content from BM aspirates. Compared to full mouse bones, human BM aspirates contain very few MSCs within the range of 0.001–0.01% of total cellularity (Pittenger et al., 1999; Qin et al., 2021). In addition, the donors’ age and sex also influences MSCs phenotype and content (Siegel et al., 2013), adding another layer of heterogeneity to the analyzed samples. Further approaches to increase MSCs content from human material require enrichment applications by cell sorting strategies and in-vitro expansion, inevitably leading to a loss of subpopulations and altered gene expression while affecting resolution capacity of scRNA-seq (Ghazanfari et al., 2017; Liu et al., 2019). The current high cost of single cell-sequencing and the low MSCs content typically result in scRNA-seq experiments with fewer than 100 MSCs, resulting in difficulty for sub-clustering analysis. In consequence, these experiments translate BM-derived MSCs as a single “homogenous” population that is compared to other MSC sources (Barrett et al., 2019; Zhou et al., 2019). In a recent scRNA-seq mapping experiment of large BM hematopoietic cell populations, a small amount of heterogeneous MSCs were captured, with one subset expressing high levels of the key bone marrow-homing cytokine CXCL12. This MSC subclass was later validated by high enrichment of CXCL12 and other key MSC signature genes from FACS-based isolation of CD13+CD11a− cells (Triana et al., 2021). Another notable exception is a study done by Wang et al. (2020), where a total of 14.494 CD271+ BM-MNCs were analyzed. This study led to similar findings compared to the murine experiments, revealing adipo-, osteo-, and chondrogenic clusters as well as two terminal clusters that could represent senescent cells (see Table 3).
Recent advances in species transfer learning methods allowed the harmonization of single cell-sequencing data from mouse to human, finding equivalent clusters of cells in BM of both species (Stumpf et al., 2020). While this approach is useful to generalize findings across species, it is also limited in several ways, e.g., only orthologous genes are transferred. Even within the same cluster of cells of each respective species, there are significant transcriptional profile differences, for instance in GO terms (Wang et al., 2020), posing the question whether these cells truly play the same role in mouse and man. With all these factors in mind, we propose the following hierarchy of the MSCs and their progeny in the BM that is validated in both mouse and human (see Figure 3), with the outlook that future studies will reconcile the missing phylogenic gaps for a unified cellular portrait of MSCs.
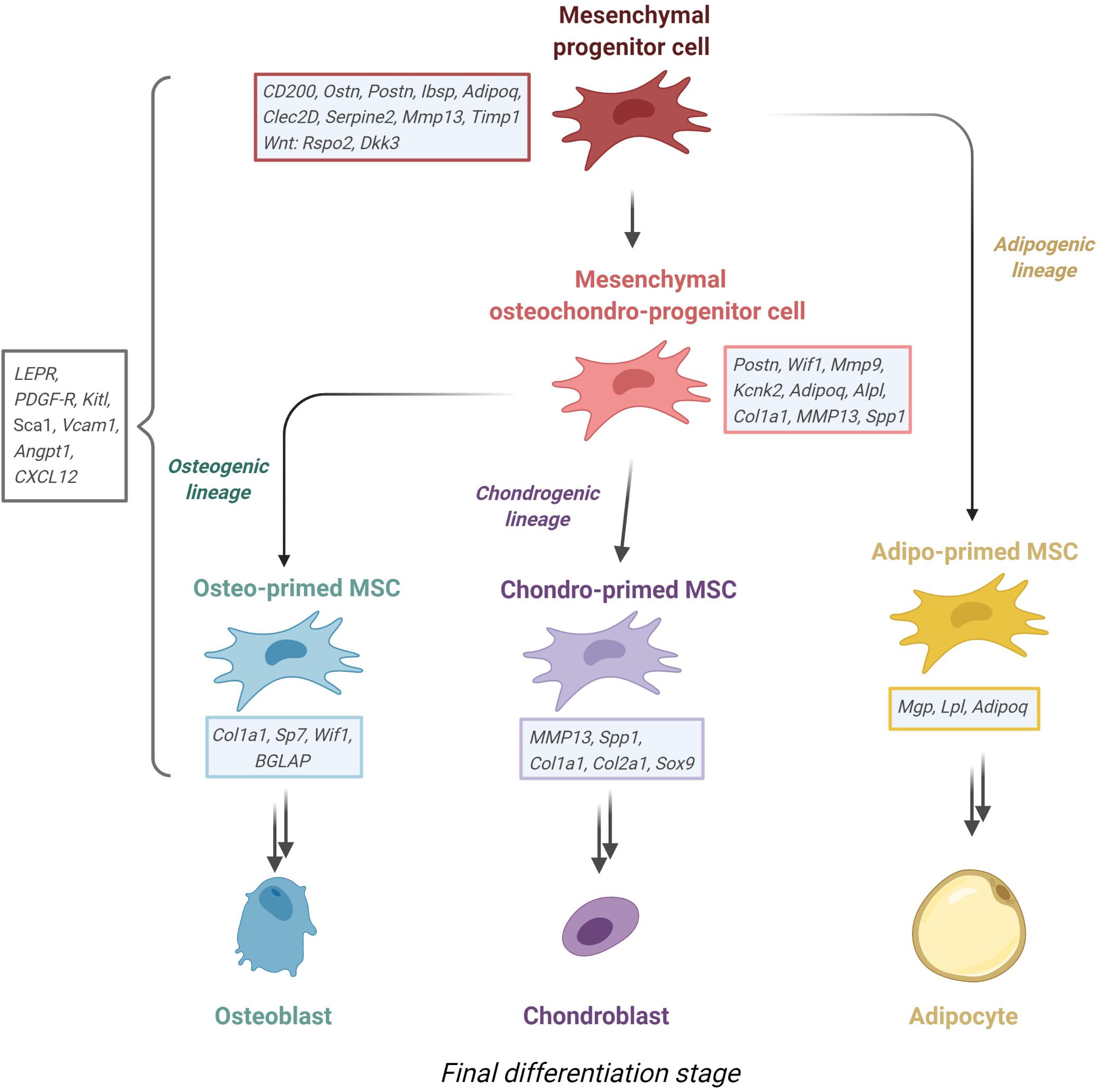
Figure 3. Proposed MSC lineage tree derived from recent sc-RNA sequencing experiments from murine stroma. Mesenchymal progenitor cells give rise to either adipo-primed MSC or an osteochondro-progenitor, which in turn gives rise to osteo-primed or chondro-primed MSC. Noted beside each entity are the most defining upregulated genes. Figure was generated using Biorender.com.
MSC Changes in Aging Bone Marrow
During aging, the BM undergoes drastic changes with loss in osteoblasts and increase in adipocytes content leading to a change in overall cellularity, bone density and a shift in anatomical distribution from “red” to “yellow” marrow (reviewed in detail by Goltzman, 2019). In recent years, focus has been set on MSCs as the main source of these changes with the hope of ameliorating age-related alterations such as osteoporosis. In accordance with age-shift toward an adipogenic phenotype, recent scRNA-seq studies in old mice found that MSC subsets with adipogenic potential (AdipoCAR) increase excessively alongside with a depletion of mature osteoblasts (Zhong et al., 2020; Dolgalev and Tikhonova, 2021). However, there are conflicting reports about the overall number of MSCs during BM aging, with some studies indicating no changes (Aguilar-Navarro et al., 2020; Meza-León et al., 2021) while a majority of reports indicates an increase in some subsets of MSCs (Maryanovich et al., 2018; Frisch et al., 2019; Singh et al., 2019). These discrepancies can be explained due to different methodological approaches and is further underlined by pathological observations demonstrating divergent cellular BM changes between mouse and human during aging (Meza-León et al., 2021). However, common mammalian features of functional deregulation have been described in deciphering the age-related changes of MSCs:
Direct Deregulation
The observed hypocellularity in aged individuals can be attributed to altered MSCs differentiation capacity toward expansion of adipocytes and increased risk of osteoporosis. Indeed, MSC show an age-dependent lineage switch between the osteogenic and adipogenic fate. Under normal conditions, MSCs homeostasis is regulated by transcription factors PPARγ and C/EBPs toward the adipogenic lineage and Runx2 and Osterix for the osteogenic lineage. These in turn are controlled by cell adhesion toward extracellular matrix (ECM)-Integrins and molecular signaling from Wnt, Notch, BMP, Hedgehog and FGF pathways (Figure 2 and reviewed in detail by Chen et al., 2016). In consequence, these pathways are of special interest to identify aging effects. Clinical data demonstrated that patients with osteoporosis or age-dependent bone loss display low activity of Wnt/β-Catenin signaling in MSC while RhoA-Rock activity is inversely correlated with β-Catenin signaling in BM-MSCs from elderly human subjects (Stevens et al., 2010; Shi et al., 2021). The decrease of Wnt-signaling can be attributed in parts to a decrease in Yes-associated protein (YAP) in MSCs during aging, a co-transcription factor that was identified recently as an interaction partner of the β-Catenin complex (Pan et al., 2018). Recent studies revealed additional transcriptional regulatory mechanisms of the Wnt pathway by different classes of non-coding RNAs, such as microRNA miR-146a, whose levels increased in patients suffering from bone fragility (Saferding et al., 2020). Other circular (Ji et al., 2021) and long (Li et al., 2018) non-coding RNA were also found to play a role in lineage commitment by inhibiting the Runx2 transcriptional complex needed for osteoblastic differentiation. The delicate balance between osteo- and adipogenesis via the different transcriptional programs can also be influenced by Bmi1, a polycomb group protein that restricts adipogenic differentiation (Kato et al., 2019) and is downregulated in aged mice (Zheng et al., 2021). Similar to Wnt pathway, Indian Hedgehog-(IHH) signaling, which induces chondrogenesis in human MSCs (Steinert et al., 2012), was shown to be decreased in peroxide-induced senescent MSCs and MSCs from older donors (Al-Azab et al., 2020). Furthermore, adipogenesis and osteoclastogenesis is promoted indirectly by Sirtuin 3 (Sirt-3), a metabolic regulator of cellular senescence driven by the mTOR-pathway, that is found to be elevated in aged male mice and resulting in cortical bone loss (Ho et al., 2017).
Senescence
Beside an apparent increase in MSCs content during aging, there is also a substantial increase in their senescence contributing to a decrease in the osteoblastic lineage and accelerated bone loss. A possible reason for this might be the development of aging-dependent inflammatory niche signaling, leading to noticeably increased IL-1α levels (which induces senescence via Bmi-1 downregulation) as well as IL-6 and TGF-β (Valletta et al., 2020; Zheng et al., 2021). A wide range of non-coding RNA have also been shown to regulate senescence both in mice and human (reviewed in Cai et al., 2021). In addition, aged MSCs produce high amounts of CXCL2 and CXCL5 chemokines, which contribute to the senescence-associated secretory phenotype (SASP) (Helbling et al., 2019). RANKL, an osteoclastogenic cytokine, has been shown to be increasingly secreted by MSCs in aged mice (Lin et al., 2017), leading to bone loss (Kim et al., 2020). Cellular senescence also leads to a decrease in Optineurin (OPTN), an autophagy receptor therefore contributing to osteoporosis alongside with accumulation of the OPTN substrate fatty acid binding protein 3 (FABP3) (Liu et al., 2020).
Indirect Deregulation
A possible mechanism for the observed increase in MSCs might be driven by sensory adrenergic denervation that occurs in the aging microenvironment (Neuropathy), which in turn leads to reduced negative regulation of MSCs pool size and to the expansion of certain subsets holding adipogenic potential (Maryanovich et al., 2018; Ho et al., 2019). These shifts in BM content are further exacerbated by an increase in endothelial cell numbers and a regression of arteriolar structures (Kusumbe et al., 2014). Such BM stromal transformations increases the risk toward a myeloid-skewing differentiation of HSCs and can potentially lead to clonal hematopoiesis and subsequent hematological neoplasia (Steensma and Ebert, 2020).
The aforementioned changes in the MSC niche are summarized in Figure 4.
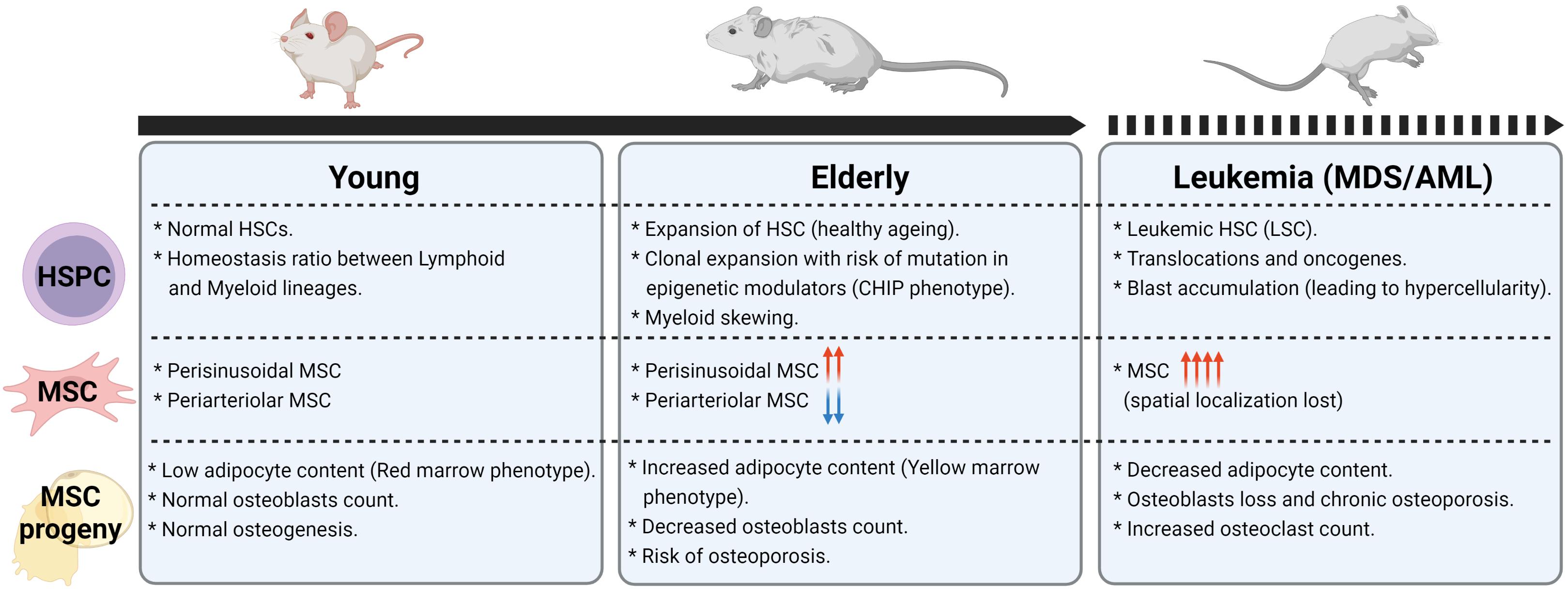
Figure 4. Changes in HSPC, MSC, and MSC progeny frequencies in aging and leukemia. Figure was generated using Biorender.com.
Addressing Age-Related Changes in the Niche
In recent years, focus on reverting cellular senescence became of major interest in addressing the aging-associated changes of MSCs. These approaches involve targeting the metabolic regulators Sirtuins 1 and 3 (Ma et al., 2017, 2020), pro-longevity growth factors such as fibroblast growth factor 21 (FGF-21) (Li et al., 2019) and downstream targets of HIF1α such as macrophage migration inhibitory factor (MIF) (Xia et al., 2015). A recent promising target is the hormone Lipocalin-2 (LCN2) that was previously shown to have a beneficial role in the regulation of various aspects of energy metabolism, especially in promoting fatty acid oxidation (Guo et al., 2010; Paton et al., 2013; Zhang et al., 2014). Further studies demonstrated that overexpression of LCN2 protect MSCs against stress-induced senescence and improve their paracrine and regenerative potentialities (Halabian et al., 2013; Bahmani et al., 2014). Furthermore, an LCN2 transgenic mouse model driven by bone-specific type 1 collagen, an osteolineage-specific promoter, showed expansion of long-term HSCs with higher clonogenic capacity due to elevated levels of CXCL12, SCF and matrix metalloproteinase inhibitors released by the BM niche (Costa et al., 2017). It has also been shown that osteoblasts, which decrease during aging, are the major source for blood circulating LCN2 in the body (Mosialou et al., 2020). Taken together, these findings suggest a beneficial effect of LCN2 supplementation on promoting hematopoieisis and stabilizing the aging BM microenvironment that would require further investigation for potential therapeutic applications.
In parallel, rewiring the MSC differentiation balance, originally explored as a rejuvenation strategy for treating osteoporosis, is currently under investigation as potential regenerative therapy to restore healthy hematopoiesis. One major example is the intermittent treatment with PTH or PTH-related peptide (PTHrP), shown to exert a well-known anabolic effect on the skeleton (Osagie-Clouard et al., 2017) and induction of HSC expansion (Calvi et al., 2003; Adams et al., 2007). Further studies demonstrated that Nestin+ MSCs isolated from PTH-treated mice displays enhanced proliferation and differentiation into osteoblasts in culture (Méndez-Ferrer et al., 2010; Ding et al., 2012); as well as increased osteogenic differentiation capacity in vivo (Fan et al., 2017). Other studies based on drug screening of natural senolytic substances such as Celastrol and Quercetin 3-O-β-D-galactopyranoside was also shown to promote osteogenesis and inhibit adipogenesis in vitro through PGC-1α signaling (Li et al., 2020; Oh et al., 2020). On a similar note, inhibition of the mTOR-pathway was shown to extensively prolong life-span in mice (Papadopoli et al., 2019), including revitalized pluripotency of human MSCs in vitro (Antonioli et al., 2019). Epigenetic modifiers were also recently proposed to revert the fat-bone-imbalance in skeletal aging, especially Lysine Demethylase 4B, which was shown to regulate β-catenin/Smad1 signaling toward MSC rejuvenation (Deng et al., 2021). Lastly, rejuvenated MSCs could also be interesting for ex vivo HSCs expansion in the context of stem cell transplantation therapies. As such, a recent and elegant co-culture study of HSCs with MSCs allowed to identify a set of “rejuvenating” transcription factors (Klf7, Ostf1, Xbp1, Irf3, and Irf7), that when over-expressed in MSC induces expansion of HSCs with enhanced regenerative and engraftment capacity while preventing accumulation of DNA damage (Nakahara et al., 2019).
In summary, most of these anti-aging approaches will require further validation prior possible translation toward clinical applications and other stromal targets not cited in this review are also currently under investigation (reviewed in more detail by Meng et al., 2020).
MSC Heterogeneity in Myeloid Malignancies
Myeloid malignancies are clonal blood diseases arising from HSCs or subsequent progenitor cells that acquired oncogenic mutations and/or chromosomal translocations over a period of several years. Depending on the etiology of the disease, myeloid malignancies comprise chronic stages (including myelodysplastic syndromes: MDS, myeloproliferative neoplasms: MPN and chronic myelomonocytic leukemia: CMML) and acute stages encompassing different subtypes of Acute Myeloid Leukemia (AML) (Arber et al., 2016; Sperling et al., 2017; Vetrie et al., 2020; Witkowski et al., 2020). A large body of work demonstrated direct and indirect involvement of the BM niche in supporting neoplastic and leukemic cells during the development of myeloid malignancies. These tumorigenic features include advantageous release of pro-survival factors, competition in niche space with healthy HSCs, stromal reprogramming and physical protection against therapy (Méndez-Ferrer et al., 2020; Witkowski et al., 2020).
MSC Niche-Driven Hematological Malignancies
Genetic mutation in mouse models affecting MSCs or their osteolineage progeny can induce different types of myeloid malignancies. For instance, activating-mutations in Nestin+ MSCs of the protein tyrosine phosphatase SHP2 (a positive regulator of the RAS signaling pathway) can lead to the development of childhood-like MPN by hyperactivating HSCs via overproduction of the CC-chemokine CCL3 and IL-1β (Dong et al., 2016). By contrast, deletion of the microRNA regulator DICER-1 in the Osterix+ osteolineage cells, prompt a pre-leukemia disease that mirrors human MDS and can evolve into secondary AML (Raaijmakers et al., 2010). Similarly, induction of Shwachman-Diamond syndrome mutation in Osterix+ stromal cells was shown to drive MDS evolution through the S100A8/9-TLR inflammatory signaling axis as a common driving mechanism of genotoxic stress that predicts AML progression in human patients (Zambetti et al., 2016). More recently, osteoblasts have also emerged as critical drivers of MDS via activating mutations in β-catenin signaling that can lead to progression to overt AML in mice (Kode et al., 2014; Stoddart et al., 2017). This aberrant activation of β-catenin signaling is also found in stromal cells of MDS patients along with DICER-1 dysregulation (Santamaría et al., 2012; Ozdogan et al., 2017) correlating with adverse prognosis (Bhagat et al., 2017).
MSC Niche Reprogramming by Leukemia
Neoplastic and malignant cells can further remodel the MSC niche by specifically targeting the osteoblastic progeny during the stepwise disease progression from pre-leukemia stage (MDS/MPN) to overt AML (Yamaguchi et al., 2021). Specifically, it was shown that both MDS and MPN cells secrete inflammatory mediators such as CCL3 and TPO, thereby driving transformation of the MSC niche toward a highly supportive milieu for leukemic cell expansion at the expense of normal hematopoiesis (Schepers et al., 2013; Medyouf et al., 2014). This is consistent with xenograft studies suggesting that the MSC niche also provides a chemo-resistant niche for leukemic blasts (Ishikawa et al., 2007; Duan et al., 2014; Bertoli et al., 2018; Boutin et al., 2020).
Healthy Nestin+ MSCs and osteoblasts can also be indirect targets of sympathetic neuropathy (through β2-adrenergic signaling) in models of myeloid malignancies, leading either to aberrant expansion or loss of Nestin+ MSCs while restricting the numbers of mature osteoblasts in both MLL-AF9-AML (Hanoun et al., 2014) and JAK2V617F-MPN mouse models (Arranz et al., 2014). As a result, the impaired MSC niche promotes expansion of mutant HSCs and facilitates disease progression by loss of expression of HSC-retention factors, including CXCL12, SCF, ANG1, and VCAM1 (Arranz et al., 2014; Hanoun et al., 2014). Collectively, this is in agreement with clinical observations of stromal cells from MDS/AML patients, where expression of cell-surface molecules involved in interaction with HSCs is decreased (Geyh et al., 2013), whereas the population of human MSCs is increased, favoring blast expansion (Kim et al., 2015). In addition, osteogenic differentiation is significantly impaired by remodeling of the vasculature leading to reduced osteocalcin serum levels and deficiency in bone growth (Geyh et al., 2016; Duarte et al., 2018; Kumar et al., 2018), which is in line with reports of osteopenia or osteoporosis observed in newly diagnosed children or adults with acute Leukemia (Datzmann et al., 2018; Ruchlemer et al., 2018; Ahn and Suh, 2020).
Mapping MSC Niche Heterogeneity in Leukemia
Despite the multiple functional studies investigating the role of the BM niche, little is known on the extent of transcriptional reshape of the MSC populations in myeloid malignancies, but recent scRNA-seq studies led to a better understanding of lineage shift and disease specificity. In AML context, single cell data revealed a concomitant decrease in committed osteolineage LepR+-MSCs in an MLL-AF9 mouse model along with an increase in pre-osteoblasts, suggesting a block in osteolineage maturation (Baryawno et al., 2019). This osteogenic differentiation blockade was further accompanied by a loss of transcriptional expression of multiple HSC niche factors (Vcam-1, CXCL-12, SCF, Angpt, Il-7, CSF1) and gene expression changes were also observed in endothelial cells and adipocytic populations (Baryawno et al., 2019). In a similar manner, RNA-seq studies on BM stroma from both mouse and human MPN shed light on the functional contributions of individual cellular components of the MSC population to myelofibrosis (Leimkühler et al., 2021). ScRNA-seq analysis demonstrated a fate switch between distinct precursor cells and MSC populations during stress-injury induced by malignant MPN clones. Two distinct MSC populations were shown to be the main drivers of BM fibrosis in mouse and human MPN. These two MSC populations are of LepR+ origin and are either adipogenic or osteogenic-biased progenitor populations. During MPN disease evolution, these MSC populations were demonstrated to be functionally reprogrammed into Collagen-producing myofibroblasts, reminiscent of Gli-1+ fibrosis-driving cells (Schneider et al., 2017) and leading to the excess deposition of ECM in BM which is considered one of the hallmarks of overt myelofibrosis (Barbui et al., 2018). Interestingly, all other MSC subsets were also shown to be reprogrammed into the production of non-collagenous ECM with scaffolding function for collagen fibrosis. This aberrant lineage shift was due to increased stromal expression of chronic inflammatory signals, especially TGF-β and S100A8/S100A9, leading toward a loss of hematopoiesis support (Vogl et al., 2018; Ribezzo et al., 2019).
Although more effort is necessary to unravel the MSC changes in different myeloid malignancies stages, all functional and genetic data indicate a shift toward an accumulation of MSC with adipogenic potential (Figure 4) that might be instrumental in disease evolution and should be explored further to specify therapeutic targeting.
Development of MSC Therapies for Myeloid Malignancies
Given the central role of MSCs in the maintenance of both HSC and leukemic blasts, numerous studies investigated their potential direct therapeutic use in hematopoietic malignancies such as MDS and AML (reviewed in Fathi et al., 2019; Lee et al., 2019). Early co-culture studies of MSC and leukemia cells displayed contradictory results: either increased blast survival (Garrido et al., 2001) or anti-leukemic effects through the induction of apoptosis and cell cycle arrest (Liang et al., 2008; Tian et al., 2010). More broadly, a direct use of MSCs as a cellular anti-cancer therapy also proved to be difficult since the cells do not survive long enough to exhibit any beneficial effects (Levy et al., 2020) and were even shown to promote tumor growth in mouse models of MLL-AF9 AML and metastasic solid cancers (Okumura et al., 2009; Spaeth et al., 2009; Xu et al., 2009; Hanoun et al., 2014).
Acknowledging this functional duality of MSCs in leukemia growth, further research was directed in developing antibodies or compounds that target specifically the supportive malignant cues, more prominently toward the inhibition of the CXCL12-CXCR4 axis (Zhang et al., 2012; Kuhne et al., 2013) and IL6 signaling (Stevens et al., 2017). These promising compounds are currently being tested in combination with standard chemotherapy or allogenic transplantation settings in clinical trials of high-risk MDS and refractory AML patients (Martínez-Cuadrón et al., 2018; Roboz et al., 2018; Michelis et al., 2019; Bose et al., 2020). On the other hand, the anti-tumoral effects displayed by MSCs were attributed to small secreted factors (Maguire, 2013; Moll et al., 2020; Wu et al., 2020) and led to increased interest in the use of MSC secretome for anti-leukemic therapy as well as for a wide array of other diseases, such as ischemic, neuroinflammatory and pulmonary malignancies (reviewed in Harman et al., 2021). Collective proteomic studies demonstrated that the MSC secretome consists of trophic factors (e.g., FGF, HGF, VEGF), cytokines (e.g., IL-6, TGFβ-1…), hormones, small peptides (e.g., SCF, PTG, Leptin) and extracellular vesicles (EVs) containing miRNA, mRNA and biologically active proteins (Chulpanova et al., 2018). In consequence, cell-free therapy options are considered more promising for clinical applications (Hmadcha et al., 2020). However, it was shown that EVs from MSC can also contribute to tumor cell migration and growth by activation of Wnt, Erk or Akt pathways (Lin et al., 2013; Gu et al., 2016; Shi et al., 2016). EV content is dependent on many factors, such as MSC source (adipose tissue, umbilical cord, bone marrow), donor age, individual donor-specific influences, sampling method and other factors (Costa et al., 2021). This high variance in EV content hinders consistent therapeutic results, pushing the focus toward developing well-defined, standardized EVs (Lener et al., 2015); as well as engineering MSC-derived EVs that are loaded with anti-tumoral drugs or siRNA (Current clinical trials NCT03608631 and NCT01294072). Therefore, future studies are crucial to decipher the real potential of MSC-derived secretome and EVs for anti-leukemic therapies.
Using a holistic view, recent bioengineering advances were made in recreating in situ BM stroma through organ-on-a-chip devices that would allow to investigate MSC-mediated chemo-resistance mechanisms and assess therapy efficacy of new anti-tumor compounds (reviewed in Santos Rosalem et al., 2020). Similar approaches using biomimetic scaffolds capable of mimicking bone extracellular-matrix were also used to study MSC transcriptional and immunomodulatory alterations by MDS/AML blast cells (Abarrategi et al., 2017; Mian et al., 2021) and allowed recently for the discovery of a novel AML-MSCs selective CaV1.2 channel blocker drug, Lercanidipine, that is able to impair leukemia progression when administered in vivo (Borella et al., 2021). Collectively, although promising targets and drugs are currently further characterized toward translational applications, a careful development of MSC-based cell therapies will be primordial to boost ant-cancer properties while eliminating tumor-promoting effects.
Concluding Remarks
MSCs represent a key component of the BM microenvironment, exerting multiple functions that are fundamental for tissue homeostasis, the support of the hematopoietic niche and the modulation of the immune system response during injury or infection. These activities are carried out through the secretion of a wide variety of factors, such as growth factors, cytokines and EVs. The aging process imposes profound modifications of both the morphology and functions of MSCs, leading to the development of a proinflammatory environment. Increasing evidence demonstrate that this reshape of the MSC niche is exacerbated during disease progression in hematologic malignancies by protecting cancer cells from apoptosis and inducing chemoresistance. Although our understanding of MSC niche contributions to aging and Leukemia has hugely increased over the last decades, more knowledge is required to harness the depth of complex MSC interactions with the highly polyclonal nature of aberrant HSCs or leukemic cells driving disease heterogeneity in MDS/AML. Moreover, many questions remain unresolved; in particular, whether the phenotypes and molecular mechanisms identified in vitro or in mouse models are maintained and therapeutically relevant in the human disease. In addition, the use of human leukemia samples in understanding aberrant MSC niche biology is currently hindered as clinical standard diagnoses are made on BM aspirates that disrupt BM architecture. Recent developments in single-cell sequencing and imaging technologies have made it possible to assess the heterogenous composition and diverse cellular and biochemical interactions present throughout complex tissue. Future integrative single-cell studies aimed at identifying the diverse network of cellular and biochemical interactions underlying the MSC niche may uncover unappreciated regulators or pathways controlling the BM aging process and cancer reprogramming and could lead to the development of novel therapeutic strategies aimed at improving health of the aging population or tackle chemoresistance in hematological malignancies.
Author Contributions
BG and KW designed and edited the figures and tables. Both authors contributed to the manuscript.
Funding
This work was funded by the support of German Cancer Consortium (DKTK) through the joint funding project CHOICE. This work was further supported by the German Cancer Research Center (DKFZ).
Conflict of Interest
The authors declare that the research was conducted in the absence of any commercial or financial relationships that could be construed as a potential conflict of interest.
Publisher’s Note
All claims expressed in this article are solely those of the authors and do not necessarily represent those of their affiliated organizations, or those of the publisher, the editors and the reviewers. Any product that may be evaluated in this article, or claim that may be made by its manufacturer, is not guaranteed or endorsed by the publisher.
Acknowledgments
We are grateful to the steady support of German Cancer Research Center (DKFZ) along with the German Cancer Consortium (DKTK). We extend our gratitude to all collaborators and the José Carreras Leukemia Foundation (DJCLS) for supporting this work.
Abbreviations
AML, Acute Myeloid Leukemia; B-ALL, B-cell Acute Lymphoblastic Leukemia; BM, Bone Marrow; CAR-cells, CXCL12-Abundant Reticular Cells; CML, Chronic Myeloid Leukemia; CMML, Chronic Myelomonocytic Leukemia; ECM, Extracellular Matrix; HSC, Hematopoietic Stem Cell; IHH, Indian Hedgehog; LEPR, Leptin Receptor; MDS, Myelodysplastic Syndrome; MF, Myelofibrosis; MIF, Macrophage Migration Inhibitory Factor; MNC, Mononuclear Cells; MPN, Myeloproliferative Neoplasm; MSC, Mesenchymal Stromal Cell; mTOR, mechanistic Target Of Rapamycin; NG2, Neural/glial antigen 2; PDGF-R, Platelet-Derived Growth Factor-Receptor; PGC-1 α, Peroxisome proliferator-activated receptor gamma coactivator 1-alpha; PTH, Parathyroid Hormone; P α S, PDGF-R-a+/Sca-1+; SASP, Senescence-Associated Secretory Phenotype; SCF, Stem Cell Factor; TPO, Thrombopoietin; YAP, Yes-Associated Protein.
References
Abarrategi, A., Foster, K., Hamilton, A., Mian, S. A., Passaro, D., Gribben, J., et al. (2017). Versatile humanized niche model enables study of normal and malignant human hematopoiesis. J. Clin. Invest. 127, 543–548. doi: 10.1172/JCI89364
Abdallah, B. M., Jensen, C. H., Gutierrez, G., Leslie, R. G. Q., Jensen, T. G., and Kassem, M. (2004). Regulation of human skeletal stem cells differentiation by Dlk1/Pref-1. J. Bone Miner. Res. 19, 841–852. doi: 10.1359/JBMR.040118
Adams, G. B., Martin, R. P., Alley, I. R., Chabner, K. T., Cohen, K. S., Calvi, L. M., et al. (2007). Therapeutic targeting of a stem cell niche. Nat. Biotechnol. 25, 238–243. doi: 10.1038/nbt1281
Aguilar-Navarro, A. G., Meza-León, B., Gratzinger, D., Juárez-Aguilar, F. G., Chang, Q., Ornatsky, O., et al. (2020). Human aging alters the spatial organization between CD34+ hematopoietic cells and adipocytes in bone marrow. Stem Cell Rep. 15, 317–325. doi: 10.1016/j.stemcr.2020.06.011
Ahn, M. B., and Suh, B.-K. (2020). Bone morbidity in pediatric acute lymphoblastic leukemia. Ann. Pediatr. Endocrinol. Metab. 25, 1215–1221. doi: 10.6065/apem.2020.25.1.1
Al-Azab, M., Wang, B., Elkhider, A., Walana, W., Li, W., Yuan, B., et al. (2020). Indian Hedgehog regulates senescence in bone marrow-derived mesenchymal stem cell through modulation of ROS/mTOR/4EBP1, p70S6K1/2 pathway. Aging (Albany N. Y.) 12, 5693–5715. doi: 10.18632/aging.102958
Ambrosi, T. H., Longaker, M. T., and Chan, C. K. F. (2019). A revised perspective of skeletal stem cell biology. Front. Cell Dev. Biol. 7:189. doi: 10.3389/fcell.2019.00189
Antonioli, E., Torres, N., Ferretti, M., Piccinato, C. d. A., and Sertie, A. L. (2019). Individual response to mTOR inhibition in delaying replicative senescence of mesenchymal stromal cells. PLoS One 14:e0204784. doi: 10.1371/journal.pone.0204784
Aoki, K., Kurashige, M., Ichii, M., Higaki, K., Sugiyama, T., Kaito, T., et al. (2021). Identification of CXCL12-abundant reticular cells in human adult bone marrow. Br. J. Haematol. 193, 659–668. doi: 10.1111/bjh.17396
Arai, F., Hirao, A., Ohmura, M., Sato, H., Matsuoka, S., Takubo, K., et al. (2004). Tie2/angiopoietin-1 signaling regulates hematopoietic stem cell quiescence in the bone marrow niche. Cell 118, 149–161. doi: 10.1016/j.cell.2004.07.004
Arber, D. A., Orazi, A., Hasserjian, R., Thiele, J., Borowitz, M. J., Le Beau, M. M., et al. (2016). The 2016 revision to the World Health Organization classification of myeloid neoplasms and acute leukemia. Blood 127, 2391–2405. doi: 10.1182/blood-2016-03-643544
Arranz, L., Sánchez-Aguilera, A., Martín-Pérez, D., Isern, J., Langa, X., Tzankov, A., et al. (2014). Neuropathy of haematopoietic stem cell niche is essential for myeloproliferative neoplasms. Nature 512, 78–81. doi: 10.1038/nature13383
Baccin, C., Al-Sabah, J., Velten, L., Helbling, P. M., Grünschläger, F., Hernández-Malmierca, P., et al. (2020). Combined single-cell and spatial transcriptomics reveal the molecular, cellular and spatial bone marrow niche organization. Nat. Cell Biol. 22, 38–48. doi: 10.1038/s41556-019-0439-6
Bahmani, B., Roudkenar, M. H., Halabian, R., Jahanian-Najafabadi, A., Amiri, F., and Jalili, M. A. (2014). Lipocalin 2 decreases senescence of bone marrow-derived mesenchymal stem cells under sub-lethal doses of oxidative stress. Cell Stress Chaperones 19, 685–693. doi: 10.1007/s12192-014-0496-5
Barbui, T., Thiele, J., Gisslinger, H., Kvasnicka, H. M., Vannucchi, A. M., Guglielmelli, P., et al. (2018). The 2016 WHO classification and diagnostic criteria for myeloproliferative neoplasms: document summary and in-depth discussion. Blood Cancer J. 8:15. doi: 10.1038/s41408-018-0054-y
Barrett, A. N., Fong, C.-Y., Subramanian, A., Liu, W., Feng, Y., Choolani, M., et al. (2019). Human Wharton’s jelly mesenchymal stem cells show unique gene expression compared with bone marrow mesenchymal stem cells using single-cell RNA-sequencing. Stem Cells Dev. 28, 196–211. doi: 10.1089/scd.2018.0132
Baryawno, N., Przybylski, D., Kowalczyk, M. S., Kfoury, Y., Severe, N., Gustafsson, K., et al. (2019). A cellular taxonomy of the bone marrow stroma in homeostasis and leukemia. Cell 177, 1915.e–1932.e. doi: 10.1016/j.cell.2019.04.040
Bertoli, S., Picard, M., Bérard, E., Griessinger, E., Larrue, C., Mouchel, P. L., et al. (2018). Dexamethasone in hyperleukocytic acute myeloid leukemia. Haematologica 103, 988–998. doi: 10.3324/haematol.2017.184267
Bhagat, T. D., Chen, S., Bartenstein, M., Barlowe, A. T., von Ahrens, D., Choudhary, G. S., et al. (2017). Epigenetically aberrant stroma in MDS propagates disease via Wnt/β-catenin activation. Cancer Res. 77, 4846–4857. doi: 10.1158/0008-5472.CAN-17-0282
Bianco, P., and Robey, P. G. (2015). Skeletal stem cells. Development 142, 1023–1027. doi: 10.1242/dev.102210
Borella, G., Da Ros, A., Borile, G., Porcù, E., Tregnago, C., Benetton, M., et al. (2021). Targeting mesenchymal stromal cells plasticity to reroute acute myeloid leukemia course. Blood doi: 10.1182/blood.2020009845 (in press).
Bose, P., Verstovsek, S., Cortes, J. E., Tse, S., Gasior, Y., Jain, N., et al. (2020). A phase 1/2 study of ruxolitinib and decitabine in patients with post-myeloproliferative neoplasm acute myeloid leukemia. Leukemia 34, 2489–2492. doi: 10.1038/s41375-020-0778-0
Boutin, L., Arnautou, P., Trignol, A., Ségot, A., Farge, T., Desterke, C., et al. (2020). Mesenchymal stromal cells confer chemoresistance to myeloid leukemia blasts through side population functionality and ABC transporter activation. Haematologica 105, 987–9998. doi: 10.3324/haematol.2018.214379
Brenet, F., Kermani, P., Spektor, R., Rafii, S., and Scandura, J. M. (2013). TGFβ restores hematopoietic homeostasis after myelosuppressive chemotherapy. J. Exp. Med. 210, 623–639. doi: 10.1084/jem.20121610
Brylka, L. J., and Schinke, T. (2019). Chemokines in physiological and pathological bone remodeling. Front. Immunol. 10:2182. doi: 10.3389/fimmu.2019.02182
Cai, J., Qi, H., Yao, K., Yao, Y., Jing, D., Liao, W., et al. (2021). Non-coding RNAs steering the senescence-related progress, properties, and application of mesenchymal stem cells. Front. Cell Dev. Biol. 9:650431. doi: 10.3389/fcell.2021.650431
Calvi, L. M., Adams, G. B., Weibrecht, K. W., Weber, J. M., Olson, D. P., Knight, M. C., et al. (2003). Osteoblastic cells regulate the haematopoietic stem cell niche. Nature 425, 841–846. doi: 10.1038/nature02040
Calvi, L. M., Bromberg, O., Rhee, Y., Weber, J. M., Smith, J. N. P., Basil, M. J., et al. (2012). Osteoblastic expansion induced by parathyroid hormone receptor signaling in murine osteocytes is not sufficient to increase hematopoietic stem cells. Blood 119, 2489–2499. doi: 10.1182/blood-2011-06-360933
Calvi, L. M., Sims, N. A., Hunzelman, J. L., Knight, M. C., Giovannetti, A., Saxton, J. M., et al. (2001). Activated parathyroid hormone/parathyroid hormone-related protein receptor in osteoblastic cells differentially affects cortical and trabecular bone. J. Clin. Invest. 107, 277–286. doi: 10.1172/JCI11296
Caselli, A., Olson, T. S., Otsuru, S., Chen, X., Hofmann, T. J., Nah, H.-D., et al. (2013). IGF-1-mediated osteoblastic niche expansion enhances long-term hematopoietic stem cell engraftment after murine bone marrow transplantation. Stem Cells 31, 2193–2204. doi: 10.1002/stem.1463
Chen, Q., Shou, P., Zheng, C., Jiang, M., Cao, G., Yang, Q., et al. (2016). Fate decision of mesenchymal stem cells: adipocytes or osteoblasts? Cell Death Differ. 23, 1128–1139. doi: 10.1038/cdd.2015.168
Chulpanova, D. S., Kitaeva, K. V., Tazetdinova, L. G., James, V., Rizvanov, A. A., and Solovyeva, V. V. (2018). Application of mesenchymal stem cells for therapeutic agent delivery in anti-tumor treatment. Front. Pharmacol. 9:259. doi: 10.3389/fphar.2018.00259
Costa, D., Principi, E., Lazzarini, E., Descalzi, F., Cancedda, R., Castagnola, P., et al. (2017). LCN2 overexpression in bone enhances the hematopoietic compartment via modulation of the bone marrow microenvironment. J. Cell. Physiol. 232, 3077–3087. doi: 10.1002/jcp.25755
Costa, L. A., Eiro, N., Fraile, M., Gonzalez, L. O., Saá, J., Garcia-Portabella, P., et al. (2021). Functional heterogeneity of mesenchymal stem cells from natural niches to culture conditions: implications for further clinical uses. Cell. Mol. Life Sci. 78, 447–467. doi: 10.1007/s00018-020-03600-0
Datzmann, T., Trautmann, F., Tesch, F., Mies, A., Hofbauer, L. C., Platzbecker, U., et al. (2018). Associations of myeloid hematological diseases of the elderly with osteoporosis: a longitudinal analysis of routine health care data. Leuk. Res. 69, 81–86. doi: 10.1016/j.leukres.2018.04.010
Deng, P., Yuan, Q., Cheng, Y., Li, J., Liu, Z., Liu, Y., et al. (2021). Loss of KDM4B exacerbates bone-fat imbalance and mesenchymal stromal cell exhaustion in skeletal aging. Cell Stem Cell 28, 1057.e–1073.e. doi: 10.1016/j.stem.2021.01.010
Ding, L., and Morrison, S. J. (2013). Haematopoietic stem cells and early lymphoid progenitors occupy distinct bone marrow niches. Nature 495, 231–235. doi: 10.1038/nature11885
Dolgalev, I., and Tikhonova, A. N. (2021) Connecting the dots: Resolving the bone marrow niche heterogeneity. In Frontiers in cell and developmental biology 9:622519. doi: 10.3389/fcell.2021.622519
Ding, L., Saunders, T. L., Enikolopov, G., and Morrison, S. J. (2012). Endothelial and perivascular cells maintain haematopoietic stem cells. Nature 481, 457–462. doi: 10.1038/nature10783
Dominici, M., Le Blanc, K., Mueller, I., Slaper-Cortenbach, I., Marini, F., Krause, D., et al. (2006). Minimal criteria for defining multipotent mesenchymal stromal cells. The International Society for Cellular Therapy position statement. Cytotherapy 8, 315–317. doi: 10.1080/14653240600855905
Dong, L., Yu, W.-M., Zheng, H., Loh, M. L., Bunting, S. T., Pauly, M., et al. (2016). Leukaemogenic effects of Ptpn11 activating mutations in the stem cell microenvironment. Nature 539, 304–308. doi: 10.1038/nature20131
Duan, C.-W., Shi, J., Chen, J., Wang, B., Yu, Y.-H., Qin, X., et al. (2014). Leukemia propagating cells rebuild an evolving niche in response to therapy. Cancer Cell 25, 778–793. doi: 10.1016/j.ccr.2014.04.015
Duarte, D., Hawkins, E. D., Akinduro, O., Ang, H., de Filippo, K., Kong, I. Y., et al. (2018). Inhibition of endosteal vascular niche remodeling rescues hematopoietic stem cell loss in AML. Cell Stem Cell 22, 64.e–77.e. doi: 10.1016/j.stem.2017.11.006
Fan, Y., Hanai, J.-I., Le, P. T., Bi, R., Maridas, D., DeMambro, V., et al. (2017). Parathyroid hormone directs bone marrow mesenchymal cell fate. Cell Metab. 25, 661–672. doi: 10.1016/j.cmet.2017.01.001
Fathi, E., Sanaat, Z., and Farahzadi, R. (2019). Mesenchymal stem cells in acute myeloid leukemia: a focus on mechanisms involved and therapeutic concepts. Blood Res. 54, 165–174. doi: 10.5045/br.2019.54.3.165
Frisch, B. J., Hoffman, C. M., Latchney, S. E., LaMere, M. W., Myers, J., Ashton, J., et al. (2019). Aged marrow macrophages expand platelet-biased hematopoietic stem cells via Interleukin1B. JCI Insight 5:e124213. doi: 10.1172/jci.insight.124213
Frisch, B. J., Porter, R. L., Gigliotti, B. J., Olm-Shipman, A. J., Weber, J. M., O’Keefe, R. J., et al. (2009). In vivo prostaglandin E2 treatment alters the bone marrow microenvironment and preferentially expands short-term hematopoietic stem cells. Blood 114, 4054–4063. doi: 10.1182/blood-2009-03-205823
Fulzele, K., Krause, D. S., Panaroni, C., Saini, V., Barry, K. J., Liu, X., et al. (2013). Myelopoiesis is regulated by osteocytes through Gsα-dependent signaling. Blood 121, 930–939. doi: 10.1182/blood-2012-06-437160
Garrido, S. M., Appelbaum, F. R., Willman, C. L., and Banker, D. E. (2001). Acute myeloid leukemia cells are protected from spontaneous and drug-induced apoptosis by direct contact with a human bone marrow stromal cell line (HS-5). Exp. Hematol. 29, 448–457. doi: 10.1016/S0301-472X(01)00612-9
Geyh, S., Oz, S., Cadeddu, R.-P., Fröbel, J., Brückner, B., Kündgen, A., et al. (2013). Insufficient stromal support in MDS results from molecular and functional deficits of mesenchymal stromal cells. Leukemia 27, 1841–1851. doi: 10.1038/leu.2013.193
Geyh, S., Rodríguez-Paredes, M., Jäger, P., Khandanpour, C., Cadeddu, R.-P., Gutekunst, J., et al. (2016). Functional inhibition of mesenchymal stromal cells in acute myeloid leukemia. Leukemia 30, 683–691. doi: 10.1038/leu.2015.325
Ghazanfari, R., Zacharaki, D., Li, H., Ching Lim, H., Soneji, S., and Scheding, S. (2017). Human primary bone marrow mesenchymal stromal cells and their in vitro progenies display distinct transcriptional profile signatures. Sci. Rep. 7:10338. doi: 10.1038/s41598-017-09449-x
Goldman, D. C., Bailey, A. S., Pfaffle, D. L., Al Masri, A., Christian, J. L., and Fleming, W. H. (2009). BMP4 regulates the hematopoietic stem cell niche. Blood 114, 4393–4401. doi: 10.1182/blood-2009-02-206433
Goltzman, D. (2019). The aging skeleton. Adv. Exp. Med. Biol. 1164, 153–160. doi: 10.1007/978-3-030-22254-3_12
Gu, H., Ji, R., Zhang, X., Wang, M., Zhu, W., Qian, H., et al. (2016). Exosomes derived from human mesenchymal stem cells promote gastric cancer cell growth and migration via the activation of the Akt pathway. Mol. Med. Rep. 14, 3452–3458. doi: 10.3892/mmr.2016.5625
Guezguez, B., Campbell, C. J. V., Boyd, A. L., Karanu, F., Casado, F. L., Di Cresce, C., et al. (2013). Regional localization within the bone marrow influences the functional capacity of human HSCs. Cell Stem Cell 13, 175–189. doi: 10.1016/j.stem.2013.06.015
Guo, H., Jin, D., Zhang, Y., Wright, W., Bazuine, M., Brockman, D. A., et al. (2010). Lipocalin-2 deficiency impairs thermogenesis and potentiates diet-induced insulin resistance in mice. Diabetes 59, 1376–1385. doi: 10.2337/db09-1735
Guo, Y., Yuan, Y., Wu, L., Ho, T.-V., Jing, J., Sugii, H., et al. (2018). BMP-IHH-mediated interplay between mesenchymal stem cells and osteoclasts supports calvarial bone homeostasis and repair. Bone Res. 6:30. doi: 10.1038/s41413-018-0031-x
Halabian, R., Tehrani, H. A., Jahanian-Najafabadi, A., and Habibi Roudkenar, M. (2013). Lipocalin-2-mediated upregulation of various antioxidants and growth factors protects bone marrow-derived mesenchymal stem cells against unfavorable microenvironments. Cell Stress Chaperones 18, 785–800. doi: 10.1007/s12192-013-0430-2
Hanoun, M., Zhang, D., Mizoguchi, T., Pinho, S., Pierce, H., Kunisaki, Y., et al. (2014). Acute myelogenous leukemia-induced sympathetic neuropathy promotes malignancy in an altered hematopoietic stem cell niche. Cell Stem Cell 15, 365–375. doi: 10.1016/j.stem.2014.06.020
Harman, R. M., Marx, C., and van de Walle, G. R. (2021). Translational animal models provide insight into mesenchymal stromal cell (MSC) secretome therapy. Front. Cell Dev. Biol. 9:654885. doi: 10.3389/fcell.2021.654885
He, Q., Scott Swindle, C., Wan, C., Flynn, R. J., Oster, R. A., Chen, D., et al. (2017). Enhanced hematopoietic stem cell self-renewal-promoting ability of clonal primary mesenchymal stromal/stem cells versus their osteogenic progeny. Stem Cells 35, 473–484. doi: 10.1002/stem.2481
Helbling, P. M., Piñeiro-Yáñez, E., Gerosa, R., Boettcher, S., Al-Shahrour, F., Manz, M. G., et al. (2019). Global transcriptomic profiling of the bone marrow stromal microenvironment during postnatal development, aging, and inflammation. Cell Rep. 29, 3313.e–3330.e. doi: 10.1016/j.celrep.2019.11.004
Hmadcha, A., Martin-Montalvo, A., Gauthier, B. R., Soria, B., and Capilla-Gonzalez, V. (2020). Therapeutic potential of mesenchymal stem cells for cancer therapy. Front. Bioeng. Biotechnol. 8:43. doi: 10.3389/fbioe.2020.00043
Ho, L., Wang, L., Roth, T. M., Pan, Y., Verdin, E. M., Hsiao, E. C., et al. (2017). Sirtuin-3 promotes adipogenesis, osteoclastogenesis, and bone loss in aging male mice. Endocrinology 158, 2741–2753. doi: 10.1210/en.2016-1739
Ho, Y.-H., Del Toro, R., Rivera-Torres, J., Rak, J., Korn, C., García-García, A., et al. (2019). Remodeling of bone marrow hematopoietic stem cell niches promotes myeloid cell expansion during premature or physiological aging. Cell Stem Cell 25, 407.e–418.e. doi: 10.1016/j.stem.2019.06.007
Hoggatt, J., Mohammad, K. S., Singh, P., Hoggatt, A. F., Chitteti, B. R., Speth, J. M., et al. (2013). Differential stem- and progenitor-cell trafficking by prostaglandin E2. Nature 495, 365–369. doi: 10.1038/nature11929
Hoggatt, J., Singh, P., Sampath, J., and Pelus, L. M. (2009). Prostaglandin E2 enhances hematopoietic stem cell homing, survival, and proliferation. Blood 113, 5444–5455. doi: 10.1182/blood-2009-01-201335
Ishikawa, F., Yoshida, S., Saito, Y., Hijikata, A., Kitamura, H., Tanaka, S., et al. (2007). Chemotherapy-resistant human AML stem cells home to and engraft within the bone-marrow endosteal region. Nat. Biotechnol. 25, 1315–1321. doi: 10.1038/nbt1350
Itkin, T., Gur-Cohen, S., Spencer, J. A., Schajnovitz, A., Ramasamy, S. K., Kusumbe, A. P., et al. (2016). Distinct bone marrow blood vessels differentially regulate haematopoiesis. Nature 532, 323–328. doi: 10.1038/nature17624
Jessop, H. L., Noble, B. S., and Cryer, A. (1994). The differentiation of a potential mesenchymal stem cell population within ovine bone marrow. Biochem. Soc. Trans. 22:248S. doi: 10.1042/bst022248s
Ji, H., Cui, X., Yang, Y., and Zhou, X. (2021). CircRNA hsa_circ_0006215 promotes osteogenic differentiation of BMSCs and enhances osteogenesis-angiogenesis coupling by competitively binding to miR-942-5p and regulating RUNX2 and VEGF. Aging (Albany N. Y.) 13, 10275–10288. doi: 10.18632/aging.202791
Jung, Y., Song, J., Shiozawa, Y., Wang, J., Wang, Z., Williams, B., et al. (2008). Hematopoietic stem cells regulate mesenchymal stromal cell induction into osteoblasts thereby participating in the formation of the stem cell niche. Stem Cells 26, 2042–2051. doi: 10.1634/stemcells.2008-0149
Kato, Y., Hou, L.-B., Miyagi, S., Nitta, E., Aoyama, K., Shinoda, D., et al. (2019). Bmi1 restricts the adipogenic differentiation of bone marrow stromal cells to maintain the integrity of the hematopoietic stem cell niche. Exp. Hematol. 76, 24–37. doi: 10.1016/j.exphem.2019.07.006
Khurana, S., Melacarne, A., Yadak, R., Schouteden, S., Notelaers, T., Pistoni, M., et al. (2014). SMAD signaling regulates CXCL12 expression in the bone marrow niche, affecting homing and mobilization of hematopoietic progenitors. Stem Cells 32, 3012–3022. doi: 10.1002/stem.1794
Kim, H.-N., Xiong, J., MacLeod, R. S., Iyer, S., Fujiwara, Y., Cawley, K. M., et al. (2020). Osteocyte RANKL is required for cortical bone loss with age and is induced by senescence. JCI Insight 5:e138815. doi: 10.1172/jci.insight.138815
Kim, J.-A., Shim, J.-S., Lee, G.-Y., Yim, H. W., Kim, T.-M., Kim, M., et al. (2015). Microenvironmental remodeling as a parameter and prognostic factor of heterogeneous leukemogenesis in acute myelogenous leukemia. Cancer Res. 75, 2222–2231. doi: 10.1158/0008-5472.CAN-14-3379
Kim, S., Lin, L., Brown, G. A. J., Hosaka, K., and Scott, E. W. (2017). Extended time-lapse in vivo imaging of tibia bone marrow to visualize dynamic hematopoietic stem cell engraftment. Leukemia 31, 1582–1592. doi: 10.1038/leu.2016.354
Kode, A., Manavalan, J. S., Mosialou, I., Bhagat, G., Rathinam, C. V., Luo, N., et al. (2014). Leukaemogenesis induced by an activating β-catenin mutation in osteoblasts. Nature 506, 240–244. doi: 10.1038/nature12883
Kozanoglu, I., Boga, C., Ozdogu, H., Sozer, O., Maytalman, E., Yazici, A. C., et al. (2009). Human bone marrow mesenchymal cells express NG2: possible increase in discriminative ability of flow cytometry during mesenchymal stromal cell identification. Cytotherapy 11, 527–533. doi: 10.1080/14653240902923153
Kuhne, M. R., Mulvey, T., Belanger, B., Chen, S., Pan, C., Chong, C., et al. (2013). BMS-936564/MDX-1338: a fully human anti-CXCR4 antibody induces apoptosis in vitro and shows antitumor activity in vivo in hematologic malignancies. Clin. Cancer Res. 19, 357–366. doi: 10.1158/1078-0432.ccr-12-2333
Kusumbe, A. P., Ramasamy, S. K., and Adams, R. H. (2014) Coupling of angiogenesis and osteogenesis by a specific vessel subtype in bone. Nature 507, 323–328. doi: 10.1038/nature13145
Kumar, B., Garcia, M., Weng, L., Jung, X., Murakami, J. L., Hu, X., et al. (2018). Acute myeloid leukemia transforms the bone marrow niche into a leukemia-permissive microenvironment through exosome secretion. Leukemia 32, 575–587. doi: 10.1038/leu.2017.259
Kumar, S., and Geiger, H. (2017). HSC niche biology and HSC expansion Ex Vivo. Trends Mol. Med. 23, 799–819. doi: 10.1016/j.molmed.2017.07.003
Kunisaki, Y., Bruns, I., Scheiermann, C., Ahmed, J., Pinho, S., Zhang, D., et al. (2013). Arteriolar niches maintain haematopoietic stem cell quiescence. Nature 502, 637–643. doi: 10.1038/nature12612
Kuznetsov, S. A., Riminucci, M., Ziran, N., Tsutsui, T. W., Corsi, A., Calvi, L., et al. (2004). The interplay of osteogenesis and hematopoiesis: expression of a constitutively active PTH/PTHrP receptor in osteogenic cells perturbs the establishment of hematopoiesis in bone and of skeletal stem cells in the bone marrow. J. Cell Biol. 167, 1113–1122. doi: 10.1083/jcb.200408079
Lawal, R. A., Zhou, X., Batey, K., Hoffman, C. M., Georger, M. A., Radtke, F., et al. (2017). The notch ligand jagged1 regulates the osteoblastic lineage by maintaining the osteoprogenitor pool. J. Bone Miner. Res. 32, 1320–1331. doi: 10.1002/jbmr.3106
Lee, M. W., Ryu, S., Kim, D. S., Lee, J. W., Sung, K. W., Koo, H. H., et al. (2019). Mesenchymal stem cells in suppression or progression of hematologic malignancy: current status and challenges. Leukemia 33, 597–611. doi: 10.1038/s41375-018-0373-9
Leimkühler, N. B., Gleitz, H. F. E., Ronghui, L., Snoeren, I. A. M., Fuchs, S. N. R., Nagai, J. S., et al. (2021). Heterogeneous bone-marrow stromal progenitors drive myelofibrosis via a druggable alarmin axis. Cell Stem Cell 28, 637.e–652.e. doi: 10.1016/j.stem.2020.11.004
Lener, T., Gimona, M., Aigner, L., Börger, V., Buzas, E., Camussi, G., et al. (2015). Applying extracellular vesicles based therapeutics in clinical trials–an ISEV position paper. J. Extracell. Vesicles 4:30087. doi: 10.3402/jev.v4.30087
Levy, O., Kuai, R., Siren, E. M. J., Bhere, D., Milton, Y., Nissar, N., et al. (2020). Shattering barriers toward clinically meaningful MSC therapies. Sci. Adv. 6:eaba6884. doi: 10.1126/sciadv.aba6884
Li, C.-J., Xiao, Y., Yang, M., Su, T., Sun, X., Guo, Q., et al. (2018). Long noncoding RNA Bmncr regulates mesenchymal stem cell fate during skeletal aging. J. Clin. Invest. 128, 5251–5266. doi: 10.1172/JCI99044
Li, J.-Y., Adams, J., Calvi, L. M., Lane, T. F., DiPaolo, R., Weitzmann, M. N., et al. (2012). PTH expands short-term murine hemopoietic stem cells through T cells. Blood 120, 4352–4362. doi: 10.1182/blood-2012-06-438531
Li, L., Wang, B., Li, Y., Li, L., Dai, Y., Lv, G., et al. (2020). Celastrol regulates bone marrow mesenchymal stem cell fate and bone-fat balance in osteoporosis and skeletal aging by inducing PGC-1α signaling. Aging (Albany N. Y.) 12, 16887–16898. doi: 10.18632/aging.103590
Li, X., Hong, Y., He, H., Jiang, G., You, W., Liang, X., et al. (2019). FGF21 mediates mesenchymal stem cell senescence via regulation of mitochondrial dynamics. Oxid. Med. Cell. Longev. 2019:4915149. doi: 10.1155/2019/4915149
Liang, R., Huang, G.-S., Wang, Z., Chen, X.-Q., Bai, Q.-X., Zhang, Y.-Q., et al. (2008). Effects of human bone marrow stromal cell line (HFCL) on the proliferation, differentiation and apoptosis of acute myeloid leukemia cell lines U937, HL-60 and HL-60/VCR. Int. J. Hematol. 87, 152–166. doi: 10.1007/s12185-008-0030-6
Liggett, L. A., and Sankaran, V. G. (2020). Unraveling hematopoiesis through the lens of genomics. Cell 182, 1384–1400. doi: 10.1016/j.cell.2020.08.030
Lin, R., Wang, S., and Zhao, R. C. (2013). Exosomes from human adipose-derived mesenchymal stem cells promote migration through Wnt signaling pathway in a breast cancer cell model. Mol. Cell. Biochem. 383, 13–20. doi: 10.1007/s11010-013-1746-z
Lin, T.-H., Gibon, E., Loi, F., Pajarinen, J., Córdova, L. A., Nabeshima, A., et al. (2017). Decreased osteogenesis in mesenchymal stem cells derived from the aged mouse is associated with enhanced NF-κB activity. J. Orthop. Res. 35, 281–288. doi: 10.1002/jor.23270
Liu, S., Stroncek, D. F., Zhao, Y., Chen, V., Shi, R., Chen, J., et al. (2019). Single cell sequencing reveals gene expression signatures associated with bone marrow stromal cell subpopulations and time in culture. J. Transl. Med. 17:23. doi: 10.1186/s12967-018-1766-2
Liu, Z.-Z., Hong, C.-G., Hu, W.-B., Chen, M.-L., Duan, R., Li, H.-M., et al. (2020). Autophagy receptor OPTN (optineurin) regulates mesenchymal stem cell fate and bone-fat balance during aging by clearing FABP3. Autophagy 1–17. doi: 10.1080/15548627.2020.1839286 (in press).
Lo Celso, C., Fleming, H. E., Wu, J. W., Zhao, C. X., Miake-Lye, S., Fujisaki, J., et al. (2009). Live-animal tracking of individual haematopoietic stem/progenitor cells in their niche. Nature 457, 92–96. doi: 10.1038/nature07434
Loeffler, D., and Schroeder, T. (2021). Symmetric and asymmetric activation of hematopoietic stem cells. Curr. Opin. Hematol. 28, 262–268. doi: 10.1097/MOH.0000000000000644
Ma, C., Pi, C., Yang, Y., Lin, L., Shi, Y., Li, Y., et al. (2017). Nampt expression decreases age-related senescence in rat bone marrow mesenchymal stem cells by targeting Sirt1. PLoS One 12:e0170930. doi: 10.1371/journal.pone.0170930
Ma, C., Sun, Y., Pi, C., Wang, H., Sun, H., Yu, X., et al. (2020). Sirt3 attenuates oxidative stress damage and rescues cellular senescence in rat bone marrow mesenchymal stem cells by targeting superoxide dismutase 2. Front. Cell Dev. Biol. 8:599376. doi: 10.3389/fcell.2020.599376
Maguire, G. (2013). Stem cell therapy without the cells. Commun. Integr. Biol. 6:e26631. doi: 10.4161/cib.26631
Martínez-Cuadrón, D., Boluda, B., Martínez, P., Bergua, J., Rodríguez-Veiga, R., Esteve, J., et al. (2018). A phase I-II study of plerixafor in combination with fludarabine, idarubicin, cytarabine, and G-CSF (PLERIFLAG regimen) for the treatment of patients with the first early-relapsed or refractory acute myeloid leukemia. Ann. Hematol. 97, 763–772. doi: 10.1007/s00277-018-3229-5
Maryanovich, M., Zahalka, A. H., Pierce, H., Pinho, S., Nakahara, F., Asada, N., et al. (2018). Adrenergic nerve degeneration in bone marrow drives aging of the hematopoietic stem cell niche. Nat. Med. 24, 782–791. doi: 10.1038/s41591-018-0030-x
Matsuzaki, Y., Mabuchi, Y., and Okano, H. (2014). Leptin receptor makes its mark on MSCs. Cell Stem Cell 15, 112–114. doi: 10.1016/j.stem.2014.07.001
Medyouf, H., Mossner, M., Jann, J.-C., Nolte, F., Raffel, S., Herrmann, C., et al. (2014). Myelodysplastic cells in patients reprogram mesenchymal stromal cells to establish a transplantable stem cell niche disease unit. Cell Stem Cell 14, 824–837. doi: 10.1016/j.stem.2014.02.014
Méndez-Ferrer, S., Bonnet, D., Steensma, D. P., Hasserjian, R. P., Ghobrial, I. M., Gribben, J. G., et al. (2020). Bone marrow niches in haematological malignancies. Nat. Rev. Cancer 20, 285–298. doi: 10.1038/s41568-020-0245-2
Méndez-Ferrer, S., Michurina, T. V., Ferraro, F., Mazloom, A. R., MacArthur, B. D., Lira, S. A., et al. (2010). Mesenchymal and haematopoietic stem cells form a unique bone marrow niche. Nature 466, 829–834. doi: 10.1038/nature09262
Meng, Q.-S., Liu, J., Wei, L., Fan, H.-M., Zhou, X.-H., and Liang, X.-T. (2020). Senescent mesenchymal stem/stromal cells and restoring their cellular functions. World J. Stem Cells 12, 966–985. doi: 10.4252/wjsc.v12.i9.966
Meza-León, B., Gratzinger, D., Aguilar-Navarro, A. G., Juárez-Aguilar, F. G., Rebel, V. I., Torlakovic, E., et al. (2021). Human, mouse and dog bone marrow show similar mesenchymal stromal cells within a distinctive microenvironment. Exp. Hematol. doi: 10.1016/j.exphem.2021.06.006
Mian, S. A., Abarrategi, A., Kong, K. L., Rouault-Pierre, K., Wood, H., Oedekoven, C. A., et al. (2021). Ectopic humanized mesenchymal niche in mice enables robust engraftment of myelodysplastic stem cells. Blood Cancer Discov. 2, 135–145. doi: 10.1158/2643-3230.BCD-20-0161
Michelis, F. V., Hedley, D. W., Malhotra, S., Chow, S., Loach, D., Gupta, V., et al. (2019). Mobilization of leukemic cells using plerixafor as part of a myeloablative preparative regimen for patients with acute myelogenous leukemia undergoing allografting: assessment of safety and tolerability. Biol. Blood Marrow Transplant. 25, 1158–1163. doi: 10.1016/j.bbmt.2019.01.014
Miharada, K., Hiroyama, T., Sudo, K., Danjo, I., Nagasawa, T., and Nakamura, Y. (2008). Lipocalin 2-mediated growth suppression is evident in human erythroid and monocyte/macrophage lineage cells. J. Cell. Physiol. 215, 526–537. doi: 10.1002/jcp.21334
Moll, G., Hoogduijn, M. J., and Ankrum, J. A. (2020). Editorial: safety, efficacy and mechanisms of action of mesenchymal stem cell therapies. Front. Immunol. 11:243. doi: 10.3389/fimmu.2020.00243
Morikawa, S., Mabuchi, Y., Kubota, Y., Nagai, Y., Niibe, K., Hiratsu, E., et al. (2009). Prospective identification, isolation, and systemic transplantation of multipotent mesenchymal stem cells in murine bone marrow. J. Exp. Med. 206, 2483–2496. doi: 10.1084/jem.20091046
Mosialou, I., Shikhel, S., Luo, N., Petropoulou, P. I., Panitsas, K., Bisikirska, B., et al. (2020). Lipocalin-2 counteracts metabolic dysregulation in obesity and diabetes. J. Exp. Med. 217:e20191261. doi: 10.1084/jem.20191261
Nakahara, F., Borger, D. K., Wei, Q., Pinho, S., Maryanovich, M., Zahalka, A. H., et al. (2019). Engineering a haematopoietic stem cell niche by revitalizing mesenchymal stromal cells. Nat. Cell Biol. 21, 560–567. doi: 10.1038/s41556-019-0308-3
Oh, J. H., Karadeniz, F., Seo, Y., and Kong, C.-S. (2020). Effect of quercetin 3-O-β-D-galactopyranoside on the adipogenic and osteoblastogenic differentiation of human bone marrow-derived mesenchymal stromal cells. Int. J. Mol. Sci. 21:8044. doi: 10.3390/ijms21218044
Okumura, T., Wang, S. S. W., Takaishi, S., Tu, S. P., Ng, V., Ericksen, R. E., et al. (2009). Identification of a bone marrow-derived mesenchymal progenitor cell subset that can contribute to the gastric epithelium. Lab. Invest. 89, 1410–1422. doi: 10.1038/labinvest.2009.88
Osagie-Clouard, L., Sanghani, A., Coathup, M., Briggs, T., Bostrom, M., and Blunn, G. (2017). Parathyroid hormone 1-34 and skeletal anabolic action: the use of parathyroid hormone in bone formation. Bone Joint Res. 6, 14–21. doi: 10.1302/2046-3758.61.BJR-2016-0085.R1
Ozdogan, H., Gur Dedeoglu, B., Oztemur Islakoglu, Y., Aydos, A., Kose, S., Atalay, A., et al. (2017). DICER1 gene and miRNA dysregulation in mesenchymal stem cells of patients with myelodysplastic syndrome and acute myeloblastic leukemia. Leuk. Res. 63, 62–71. doi: 10.1016/j.leukres.2017.10.006
Pan, J.-X., Xiong, L., Zhao, K., Zeng, P., Wang, B., Tang, F.-L., et al. (2018). YAP promotes osteogenesis and suppresses adipogenic differentiation by regulating β-catenin signaling. Bone research 6:18. doi: 10.1038/s41413-018-0018-7
Papadopoli, D., Boulay, K., Kazak, L., Pollak, M., Mallette, F., Topisirovic, I., et al. (2019). mTOR as a central regulator of lifespan and aging. F1000Res 8:F1000FacultyRev–998. doi: 10.12688/f1000research.17196.1
Paton, C. M., Rogowski, M. P., Kozimor, A. L., Stevenson, J. L., Chang, H., and Cooper, J. A. (2013). Lipocalin-2 increases fat oxidation in vitro and is correlated with energy expenditure in normal weight but not obese women. Obesity (Silver Spring) 21, E640–E648. doi: 10.1002/oby.20507
Pinho, S., and Frenette, P. S. (2019). Haematopoietic stem cell activity and interactions with the niche. Nat. Rev. Mol. Cell Biol. 20, 303–320. doi: 10.1038/s41580-019-0103-9
Pinho, S., Lacombe, J., Hanoun, M., Mizoguchi, T., Bruns, I., Kunisaki, Y., et al. (2013). PDGFRα and CD51 mark human nestin+ sphere-forming mesenchymal stem cells capable of hematopoietic progenitor cell expansion. J. Exp. Med. 210, 1351–1367. doi: 10.1084/jem.20122252
Pittenger, M. F., Mackay, A. M., Beck, S. C., Jaiswal, R. K., Douglas, R., Mosca, J. D., et al. (1999). Multilineage potential of adult human mesenchymal stem cells. Science 284, 143–147. doi: 10.1126/science.284.5411.143
Qian, H., Buza-Vidas, N., Hyland, C. D., Jensen, C. T., Antonchuk, J., Månsson, R., et al. (2007). Critical role of thrombopoietin in maintaining adult quiescent hematopoietic stem cells. Cell Stem Cell 1, 671–684. doi: 10.1016/j.stem.2007.10.008
Qin, P., Pang, Y., Hou, W., Fu, R., Zhang, Y., Wang, X., et al. (2021). Integrated decoding hematopoiesis and leukemogenesis using single-cell sequencing and its medical implication. Cell Discov. 7:2. doi: 10.1038/s41421-020-00223-4
Raaijmakers, M. H. G. P., Mukherjee, S., Guo, S., Zhang, S., Kobayashi, T., Schoonmaker, J. A., et al. (2010). Bone progenitor dysfunction induces myelodysplasia and secondary leukaemia. Nature 464, 852–857. doi: 10.1038/nature08851
Rendina-Ruedy, E., and Rosen, C. J. (2017). Bone-fat interaction. Endocrinol. Metab. Clin. North Am. 46, 41–50. doi: 10.1016/j.ecl.2016.09.004
Ribezzo, F., Snoeren, I. A. M., Ziegler, S., Stoelben, J., Olofsen, P. A., Henic, A., et al. (2019). Rps14, Csnk1a1 and miRNA145/miRNA146a deficiency cooperate in the clinical phenotype and activation of the innate immune system in the 5q- syndrome. Leukemia 33, 1759–1772. doi: 10.1038/s41375-018-0350-3
Roboz, G. J., Ritchie, E. K., Dault, Y., Lam, L., Marshall, D. C., Cruz, N. M., et al. (2018). Phase I trial of plerixafor combined with decitabine in newly diagnosed older patients with acute myeloid leukemia. Haematologica 103, 1308–1316. doi: 10.3324/haematol.2017.183418
Ruchlemer, R., Amit-Kohn, M., Tvito, A., Sindelovsky, I., Zimran, A., and Raveh-Brawer, D. (2018). Bone loss and hematological malignancies in adults: a pilot study. Support Care Cancer 26, 3013–3020. doi: 10.1007/s00520-018-4143-z
Sacchetti, B., Funari, A., Michienzi, S., Di Cesare, S., Piersanti, S., Saggio, I., et al. (2007). Self-renewing osteoprogenitors in bone marrow sinusoids can organize a hematopoietic microenvironment. Cell 131, 324–336. doi: 10.1016/j.cell.2007.08.025
Saferding, V., Hofmann, M., Brunner, J. S., Niederreiter, B., Timmen, M., Magilnick, N., et al. (2020). microRNA-146a controls age-related bone loss. Aging Cell 19:e13244. doi: 10.1111/acel.13244
Santamaría, C., Muntión, S., Rosón, B., Blanco, B., López-Villar, O., Carrancio, S., et al. (2012). Impaired expression of DICER, DROSHA, SBDS and some microRNAs in mesenchymal stromal cells from myelodysplastic syndrome patients. Haematologica 97, 1218–1224. doi: 10.3324/haematol.2011.054437
Santos Rosalem, G., Gonzáles Torres, L. A., de Las Casas, E. B., Mathias, F. A. S., Ruiz, J. C., and Carvalho, M. G. R. (2020). Microfluidics and organ-on-a-chip technologies: a systematic review of the methods used to mimic bone marrow. PLoS One 15:e0243840. doi: 10.1371/journal.pone.0243840
Scandura, J. M., Boccuni, P., Massagué, J., and Nimer, S. D. (2004). Transforming growth factor beta-induced cell cycle arrest of human hematopoietic cells requires p57KIP2 up-regulation. Proc. Natl. Acad. Sci. U.S.A. 101, 15231–15236. doi: 10.1073/pnas.0406771101
Scheller, E. L., Cawthorn, W. P., Burr, A. A., Horowitz, M. C., and MacDougald, O. A. (2016). Marrow adipose tissue: trimming the fat. Trends Endocrinol. Metab. 27, 392–403. doi: 10.1016/j.tem.2016.03.016
Schepers, K., Pietras, E. M., Reynaud, D., Flach, J., Binnewies, M., Garg, T., et al. (2013). Myeloproliferative neoplasia remodels the endosteal bone marrow niche into a self-reinforcing leukemic niche. Cell Stem Cell 13, 285–299. doi: 10.1016/j.stem.2013.06.009
Schneider, R. K., Mullally, A., Dugourd, A., Peisker, F., Hoogenboezem, R., van Strien, P. M. H., et al. (2017). Gli1+ mesenchymal stromal cells are a key driver of bone marrow fibrosis and an important cellular therapeutic target. Cell Stem Cell 20, 785.e–800.e. doi: 10.1016/j.stem.2017.03.008
Shi, S., Zhang, Q., Xia, Y., You, B., Shan, Y., Bao, L., et al. (2016). Mesenchymal stem cell-derived exosomes facilitate nasopharyngeal carcinoma progression. Am. J. Cancer Res. 6, 459–472.
Shi, W., Xu, C., Gong, Y., Wang, J., Ren, Q., Yan, Z., et al. (2021). RhoA/Rock activation represents a new mechanism for inactivating Wnt/β-catenin signaling in the aging-associated bone loss. Cell Regen. 10:8. doi: 10.1186/s13619-020-00071-3
Siegel, G., Kluba, T., Hermanutz-Klein, U., Bieback, K., Northoff, H., and Schäfer, R. (2013). Phenotype, donor age and gender affect function of human bone marrow-derived mesenchymal stromal cells. BMC Med. 11:146. doi: 10.1186/1741-7015-11-146
Singh, A., Veeriah, V., Xi, P., Labella, R., Chen, J., Romeo, S. G., et al. (2019). Angiocrine signals regulate quiescence and therapy resistance in bone metastasis. JCI Insight 4:e125679. doi: 10.1172/jci.insight.125679
Spaeth, E. L., Dembinski, J. L., Sasser, A. K., Watson, K., Klopp, A., Hall, B., et al. (2009). Mesenchymal stem cell transition to tumor-associated fibroblasts contributes to fibrovascular network expansion and tumor progression. PLoS One 4:e4992. doi: 10.1371/journal.pone.0004992
Sperling, A. S., Gibson, C. J., and Ebert, B. L. (2017). The genetics of myelodysplastic syndrome: from clonal haematopoiesis to secondary leukaemia. Nat. Rev. Cancer 17, 5–19. doi: 10.1038/nrc.2016.112
Steensma, D. P., and Ebert, B. L. (2020). Clonal hematopoiesis as a model for premalignant changes during aging. In Experimental hematology 83, 48–56. doi: 10.1016/j.exphem.2019.12.001
Steinert, A. F., Weissenberger, M., Kunz, M., Gilbert, F., Ghivizzani, S. C., Göbel, S., et al. (2012). Indian hedgehog gene transfer is a chondrogenic inducer of human mesenchymal stem cells. Arthritis Res. Ther. 14:R168. doi: 10.1186/ar3921
Stevens, A. M., Miller, J. M., Munoz, J. O., Gaikwad, A. S., and Redell, M. S. (2017). Interleukin-6 levels predict event-free survival in pediatric AML and suggest a mechanism of chemotherapy resistance. Blood Adv. 1, 1387–1397. doi: 10.1182/bloodadvances.2017007856
Stevens, J. R., Miranda-Carboni, G. A., Singer, M. A., Brugger, S. M., Lyons, K. M., and Lane, T. F. (2010). Wnt10b deficiency results in age-dependent loss of bone mass and progressive reduction of mesenchymal progenitor cells. J. Bone Miner. Res. 25, 2138–2147. doi: 10.1002/jbmr.118
Stoddart, A., Wang, J., Hu, C., Fernald, A. A., Davis, E. M., Cheng, J. X., et al. (2017). Inhibition of WNT signaling in the bone marrow niche prevents the development of MDS in the Apcdel/+ MDS mouse model. Blood 129, 2959–2970. doi: 10.1182/blood-2016-08-736454
Stumpf, P. S., Du, X., Imanishi, H., Kunisaki, Y., Semba, Y., Noble, T., et al. (2020). Transfer learning efficiently maps bone marrow cell types from mouse to human using single-cell RNA sequencing. Commun. Biol. 3:736. doi: 10.1038/s42003-020-01463-6
Sugiyama, T., Kohara, H., Noda, M., and Nagasawa, T. (2006). Maintenance of the hematopoietic stem cell pool by CXCL12-CXCR4 chemokine signaling in bone marrow stromal cell niches. Immunity 25, 977–988. doi: 10.1016/j.immuni.2006.10.016
Tian, K., Yang, S., Ren, Q., Han, Z., Lu, S., Ma, F., et al. (2010). p38 MAPK contributes to the growth inhibition of leukemic tumor cells mediated by human umbilical cord mesenchymal stem cells. Cell Physiol. Biochem. 26, 799–808. doi: 10.1159/000323973
Tikhonova, A. N., Dolgalev, I., Hu, H., Sivaraj, K. K., Hoxha, E., Cuesta-Domínguez, Á, et al. (2019). The bone marrow microenvironment at single-cell resolution. Nature 569, 222–228. doi: 10.1038/s41586-019-1104-8
Triana, S. H., Vonficht, D., Jopp-Saile, L., Raffel, S., Lutz, R., Leonce, D., et al. (2021). Single-cell proteo-genomic reference maps of the hematopoietic system enable the purification and massive profiling of precisely defined cell states. bioRxiv [Preprint] doi: 10.1101/2021.03.18.435922
Valletta, S., Thomas, A., Meng, Y., Ren, X., Drissen, R., Sengül, H., et al. (2020). Micro-environmental sensing by bone marrow stroma identifies IL-6 and TGFβ1 as regulators of hematopoietic ageing. Nat. Commun. 11:4075. doi: 10.1038/s41467-020-17942-7
Vetrie, D., Helgason, G. V., and Copland, M. (2020). The leukaemia stem cell: similarities, differences and clinical prospects in CML and AML. Nat. Rev. Cancer 20, 158–173. doi: 10.1038/s41568-019-0230-9
Vogl, T., Stratis, A., Wixler, V., Völler, T., Thurainayagam, S., Jorch, S. K., et al. (2018). Autoinhibitory regulation of S100A8/S100A9 alarmin activity locally restricts sterile inflammation. J. Clin. Invest. 128, 1852–1866. doi: 10.1172/JCI89867
Wang, Z., Li, X., Yang, J., Gong, Y., Zhang, H., Qiu, X., et al. (2020). Single-cell RNA sequencing deconvolutes the in vivo heterogeneity of human bone marrow-derived mesenchymal stem cells. bioRxiv [Preprint] doi: 10.1101/2020.04.06.027904
Wei, Q., and Frenette, P. S. (2018). Niches for hematopoietic stem cells and their progeny. Immunity 48, 632–648. doi: 10.1016/j.immuni.2018.03.024
Wein, M. N., and Kronenberg, H. M. (2018). Regulation of bone remodeling by parathyroid hormone. Cold Spring Harb. Perspect. Med. 8:a031237. doi: 10.1101/cshperspect.a031237
Weitz, D. A., Klein, A. M., Akartuna, I., Mazutis, L., and Kirschner, M. W. (2015). Systems and Methods for Barcoding Nucleic Acids. WO2015164212A1. Vilnius: Vilnius University.
Witkowski, M. T., Kousteni, S., and Aifantis, I. (2020). Mapping and targeting of the leukemic microenvironment. J. Exp. Med. 217:e20190589. doi: 10.1084/jem.20190589
Wolock, S. L., Krishnan, I., Tenen, D. E., Matkins, V., Camacho, V., Patel, S., et al. (2019). Mapping distinct bone marrow niche populations and their differentiation paths. Cell Rep. 28, 302.e–311.e. doi: 10.1016/j.celrep.2019.06.031
Wu, J. Y., Purton, L. E., Rodda, S. J., Chen, M., Weinstein, L. S., McMahon, A. P., et al. (2008). Osteoblastic regulation of B lymphopoiesis is mediated by Gs{alpha}-dependent signaling pathways. Proc. Natl. Acad. Sci. U.S.A. 105, 16976–16981. doi: 10.1073/pnas.0802898105
Wu, X., Jiang, J., Gu, Z., Zhang, J., Chen, Y., and Liu, X. (2020). Mesenchymal stromal cell therapies: immunomodulatory properties and clinical progress. Stem Cell Res. Ther. 11:345. doi: 10.1186/s13287-020-01855-9
Xia, W., Zhang, F., Xie, C., Jiang, M., and Hou, M. (2015). Macrophage migration inhibitory factor confers resistance to senescence through CD74-dependent AMPK-FOXO3a signaling in mesenchymal stem cells. Stem Cell Res. Ther. 6:82. doi: 10.1186/s13287-015-0076-3
Xie, Y., Yin, T., Wiegraebe, W., He, X. C., Miller, D., Stark, D., et al. (2009). Detection of functional haematopoietic stem cell niche using real-time imaging. Nature 457, 97–101. doi: 10.1038/nature07639
Xu, W., Bian, Z., Fan, Q., Li, G., and Tang, T. (2009). Human mesenchymal stem cells (hMSCs) target osteosarcoma and promote its growth and pulmonary metastasis. Cancer Lett. 281, 32–41. doi: 10.1016/j.canlet.2009.02.022
Yamaguchi, T., Kawamoto, E., Gaowa, A., Park, E. J., and Shimaoka, M. (2021). Remodeling of bone marrow niches and roles of exosomes in leukemia. Int. J. Mol. Sci. 22:1881. doi: 10.3390/ijms22041881
Yao, H., Miura, Y., Yoshioka, S., Miura, M., Hayashi, Y., Tamura, A., et al. (2014). Parathyroid hormone enhances hematopoietic expansion via upregulation of cadherin-11 in bone marrow mesenchymal stromal cells. Stem Cells 32, 2245–2255. doi: 10.1002/stem.1701
Yoon, K.-A., Cho, H.-S., Shin, H.-I., and Cho, J.-Y. (2012). Differential regulation of CXCL5 by FGF2 in osteoblastic and endothelial niche cells supports hematopoietic stem cell migration. Stem Cells Dev 21, 3391–3402. doi: 10.1089/scd.2012.0128
Yoon, K.-A., Son, Y., Choi, Y.-J., Kim, J.-H., and Cho, J.-Y. (2017). Fibroblast growth factor 2 supports osteoblastic niche cells during hematopoietic homeostasis recovery after bone marrow suppression. Cell Commun. Signal. 15:25. doi: 10.1186/s12964-017-0181-2
Yoshihara, H., Arai, F., Hosokawa, K., Hagiwara, T., Takubo, K., Nakamura, Y., et al. (2007). Thrombopoietin/MPL signaling regulates hematopoietic stem cell quiescence and interaction with the osteoblastic niche. Cell Stem Cell 1, 685–697. doi: 10.1016/j.stem.2007.10.020
Yu, B., Zhao, X., Yang, C., Crane, J., Xian, L., Lu, W., et al. (2012). Parathyroid hormone induces differentiation of mesenchymal stromal/stem cells by enhancing bone morphogenetic protein signaling. J. Bone Miner. Res. 27, 2001–2014. doi: 10.1002/jbmr.1663
Yu, V. W. C., Lymperi, S., Oki, T., Jones, A., Swiatek, P., Vasic, R., et al. (2016). Distinctive mesenchymal-parenchymal cell pairings govern B cell differentiation in the bone marrow. Stem Cell Rep. 7, 220–235. doi: 10.1016/j.stemcr.2016.06.009
Zambetti, N. A., Ping, Z., Chen, S., Kenswil, K. J. G., Mylona, M. A., Sanders, M. A., et al. (2016). Mesenchymal inflammation drives genotoxic stress in hematopoietic stem cells and predicts disease evolution in human pre-leukemia. Cell Stem Cell 19, 613–627. doi: 10.1016/j.stem.2016.08.021
Zhang, J., Niu, C., Ye, L., Huang, H., He, X., Tong, W.-G., et al. (2003). Identification of the haematopoietic stem cell niche and control of the niche size. Nature 425, 836–841. doi: 10.1038/nature02041
Zhang, Y., Guo, H., Deis, J. A., Mashek, M. G., Zhao, M., Ariyakumar, D., et al. (2014). Lipocalin 2 regulates brown fat activation via a nonadrenergic activation mechanism. J. Biol. Chem. 289, 22063–22077. doi: 10.1074/jbc.M114.559104
Zhang, Y., Patel, S., Abdelouahab, H., Wittner, M., Willekens, C., Shen, S., et al. (2012). CXCR4 inhibitors selectively eliminate CXCR4-expressing human acute myeloid leukemia cells in NOG mouse model. Cell Death Dis. 3:e396. doi: 10.1038/cddis.2012.137
Zheng, X., Wang, Q., Xie, Z., and Li, J. (2021). The elevated level of IL-1α in the bone marrow of aged mice leads to MSC senescence partly by down-regulating Bmi-1. Exp. Gerontol. 148:111313. doi: 10.1016/j.exger.2021.111313
Zhong, L., Yao, L., Tower, R. J., Wei, Y., Miao, Z., Park, J., et al. (2020). Single cell transcriptomics identifies a unique adipose lineage cell population that regulates bone marrow environment. Elife 9:e54695. doi: 10.7554/eLife.54695
Keywords: mesenchymal stromal cells, bone marrow niche, aging, leukemia, adipocyte, osteoblast
Citation: Woods K and Guezguez B (2021) Dynamic Changes of the Bone Marrow Niche: Mesenchymal Stromal Cells and Their Progeny During Aging and Leukemia. Front. Cell Dev. Biol. 9:714716. doi: 10.3389/fcell.2021.714716
Received: 25 May 2021; Accepted: 22 July 2021;
Published: 10 August 2021.
Edited by:
Aldo Roccaro, Civil Hospital of Brescia, ItalyReviewed by:
Annamaria Gulla, Dana–Farber Cancer Institute, United StatesMarco Ponzetti, University of L’Aquila, Italy
Copyright © 2021 Woods and Guezguez. This is an open-access article distributed under the terms of the Creative Commons Attribution License (CC BY). The use, distribution or reproduction in other forums is permitted, provided the original author(s) and the copyright owner(s) are credited and that the original publication in this journal is cited, in accordance with accepted academic practice. No use, distribution or reproduction is permitted which does not comply with these terms.
*Correspondence: Borhane Guezguez, Yi5ndWV6Z3VlekBka2Z6LmRl