Arhgap21 Deficiency Results in Increase of Osteoblastic Lineage Cells in the Murine Bone Marrow Microenvironment
- 1Hematology and Hemotherapy Center, University of Campinas, São Paulo, Brazil
- 2Institute of Environmental, Chemical and Pharmaceutical Sciences—Federal University of São Paulo, São Paulo, Brazil
ARHGAP21 is a member of the RhoGAP family of proteins involved in cell growth, differentiation, and adhesion. We have previously shown that the heterozygous Arhgap21 knockout mouse model (Arhgap21+/−) presents several alterations in the hematopoietic compartment, including increased frequency of hematopoietic stem and progenitor cells (HSPC) with impaired adhesion in vitro, increased mobilization to peripheral blood, and decreased engraftment after bone marrow transplantation. Although these HSPC functions strongly depend on their interactions with the components of the bone marrow (BM) niche, the role of ARHGAP21 in the marrow microenvironment has not yet been explored. In this study, we investigated the composition and function of the BM microenvironment in Arhgap21+/− mice. The BM of Arhgap21+/− mice presented a significant increase in the frequency of phenotypic osteoblastic lineage cells, with no differences in the frequencies of multipotent stromal cells or endothelial cells when compared to the BM of wild type mice. Arhgap21+/− BM cells had increased capacity of generating osteogenic colony-forming units (CFU-OB) in vitro and higher levels of osteocalcin were detected in the Arhgap21+/− BM supernatant. Increased expression of Col1a1, Ocn and decreased expression of Trap1 were observed after osteogenic differentiation of Arhgap21+/− BM cells. In addition, Arhgap21+/− mice recipients of normal BM cells showed decreased leucocyte numbers during transplantation recovery. Our data suggest participation of ARHGAP21 in the balanced composition of the BM microenvironment through the regulation of osteogenic differentiation.
Introduction
Normal hematopoiesis is controlled by the dynamic interactions between hematopoietic stem cells (HSC) and specialized microenvironments (niches) composed of different cell types within the bone marrow (BM) (Galán-Díez and Kousteni 2017; Méndez-Ferrer et al., 2020). Endothelial cells, mesenchymal cells (MSCs), and osteoblasts are key components of BM niches, acting as regulators of hematopoiesis (Méndez-Ferrer et al., 2010, 2020; Pinho and Frenette 2019). Disruptions in BM niches have been found in hematological malignancies (Krevvata et al., 2014; Wang et al., 2016; Galán-Díez and Kousteni 2017; Méndez-Ferrer et al., 2020) and there is increasing evidence that hematopoietic malignant cells actively remodel their local microenvironment to subserve their physiological demands. For instance, in myeloproliferative neoplasia, BM-MSCs were stimulated by malignant cells to produce altered osteoblasts, transforming the BM microenvironment into a leukemic niche (Schepers et al., 2013). Conversely, changes in specific components of the BM niche may result in hematological malignancies (Man et al., 2021). Osteoblasts have been directly implicated in the development of myeloid disorders in mice models of myelodysplasia and myeloproliferative neoplasms leading to acute myeloid leukemia (AML) (Krevvata et al., 2014). Thus, identifying new molecules involved in the regulation of the BM niche presents an unexploited opportunity for therapeutic targeting.
Members of the Rho family of GTPases participate in the regulation of normal and malignant hematopoiesis, including interactions between HSC and the BM microenvironment (Yang et al., 2007; Cancelas and Williams 2009). Rho GTPases comprise small G-proteins well known for their functions in controlling cytoskeletal rearrangements. Most Rho GTPases cycle between active and inactive conformations (GTP/GDP-bound) aided by three types of proteins: guanine exchange factors, GDP dissociation inhibitors and GTPase-activating proteins (GAPs) (McMichael et al., 2017). RhoGAPs stimulate the conversion from the GTP-bound form to the GDP-bound form, acting as negative regulators of Rho GTPases.
Rho GTPase deregulation found in abnormal hematopoiesis frequently results from changes in RhoGAPs. Heterozygous knockout mouse model for p190 RhoGAP exhibit altered constitution of the BM microenvironment, with an increased number of adipocytes and decreased number and functionality of osteoblasts (Raman et al., 2013). Knockdown of the RhoGAP ARHGAP18 in murine MSCs increases RhoA activity with concomitant suppression of adipogenesis and increase in osteogenic commitment (Thompson et al., 2018). The Arhgap28 knockout mice showed normal bone phenotype, but with decreased expression of genes that encode extracellular matrix proteins (Yeung et al., 2014).
Our group has been studying the role of the RhoGAP protein ARHGAP21 in hematopoietic and non-hematopoietic cells (Sanchez Bassères et al., 2002; Barcellos et al., 2013; Lazarini et al., 2013; Xavier-Ferrucio et al., 2018; Bernusso et al., 2021). ARHGAP21 presents RhoGAP activity for the Rho GTPases RhoA, RhoC and Cdc42 (Dubois et al., 2005; Lazarini et al., 2013) and interacts with FAK, α-tubulin and β-arrestin (Bigarella et al., 2009; Anthony et al., 2011; Bernusso et al., 2021). Upregulation of ARHGAP21 was detected during erythrocytic, granulocytic (Sanchez Bassères et al., 2002) and megakaryocytic differentiation (Bernusso et al., 2021), and its silencing in human hematopoietic progenitor cells decreased erythroid commitment (Xavier-Ferrucio et al., 2018). Heterozygous mouse model with reduced Arhgap21 expression (Arhgap21+/−) showed increased frequency of hematopoietic stem and progenitor cells, but with impaired functionality. Arhgap21+/− hematopoietic progenitor cells also presented defective adhesion and enhanced mobilization (Xavier-Ferrucio et al., 2018). Accelerated hemostatic response was another feature of Arhgap21+/− mice (Bernusso et al., 2021). Despite all these hematological changes, the impact of ARHGAP21 reduction in the BM microenvironment had not been investigated. In this study, we characterized the cellular composition of Arhgap21+/− mice BM microenvironment and the impact of Arhgap21 reduction on osteoblast differentiation of BM MSCs.
Materials and Methods
Heterozygous Arhgap21 Mouse (Arhgap21+/−)
Arhgap21 heterozygous mice (Arhgap21+/−) were obtained by Arhgap21+/− versus wild type (WT) crossed mating pairs, according to Mendelian distribution. All animals were bred and maintained at the University of Campinas (UNICAMP) and housed four per cage. Environmental conditions were temperature-controlled (21 ± 2°C), 55 ± 5% humidity and a 12 h/12 h light-dark circadian cycle with access to food and water ad libitum. Arhgap21+/− mice present an average of approximately 50% reduction of Arhgap21 mRNA and protein levels compared to WT, and their lifespan is similar to that of WT mice (Xavier-Ferrucio et al., 2018). Arhgap21+/− mice and WT littermates (control) at 8–12-weeks of age were used in all experiments. All procedures were approved by the Institutional Animal Experimentation Ethics Committee (CEUA 4894-1/2018/UNICAMP) and conducted according to National Institutes of Health Guide for the Care and Use of Laboratory Animals.
Obtention of BM Cells and Supernatant
BM cells were collected from the femur and tibiae of Arhgap21+/− and WT mice by flushing. The flushing method consists of cutting the ends of the bones and removing the bone marrow cells using a syringe with 25-gauge needle and 200 µl of phosphate-buffered saline (PBS) (Huang et al., 2015). Cells were centrifuged at 1,500 rpm for 5 min and BM supernatant was then collected and stocked at −20°C for protein analysis.
Immunophenotyping of Endothelial, Multipotent Stromal and Osteoblastic Lineage Cells
A total of 1 × 107 BM cells collected as described above were resuspended in 100 µl of PBS containing specific anti-mouse antibodies (Lin/APC-BD Biosciences, CD45/PerCP-Cy5.5-Biolegend, CD31/FITC-Biolegend, Sca-1/PE-Cy7-Biolegend and CD51/PE-Biolegend) and incubated for 30 min at room temperature in the dark. Frequency of each cell subset was determined using a FacsAria II (BD Biosciences) with the following antibodies: Multipotent Stromal Cells—MSCs (CD45-/Lin-/CD31-/Sca1+/CD51+); Osteoblastic Lineage Cells—OBCs (CD45-/Lin-/CD31-/Sca1-/CD51+); Arteriolar Endothelial Cells—AEC (CD45-/Lin-/CD31+/Sca1+) and Sinusoidal Endothelial Cells—SEC (CD45-/Lin-/CD31+/Sca1-). Analysis was performed with FlowJo software (TreeStar Inc.).
CFU-F and CFU-OB Assay
Colony Forming Unit-Fibroblast (CFU-F) and Colony Forming Unit-Osteoblast (CFU-OB) assays were performed according to Balderman et al., 2016, using three mice per group. Briefly, for CFU-F assays, 106 total BM cells/well were cultured in a six-well dish in Minimum Essential Medium Eagle, alpha modification (alpha MEM) media containing 20% fetal bovine serum (FBS) for 7 days, when fresh media was added. After 14 days, adherent cells were fixed in formalin 10% buffer and stained for crystal violet (0.5% in methanol) or alkaline phosphatase activity (0.005% weight/volume naphthol AS MX-PO4, and 0.03% weight/volume fast red violet LB salt in 100 mM Tris-HCl). For CFU-OB assays, 4 × 106 total BM cells/well were cultured in a six-well dish in alpha MEM media containing 10% FBS for 5–6 days, when non-adherent cells were removed and new mineralizing alpha MEM media (containing 10% FBS, 50 ug/ml L-ascorbic acid 2-phosphate and 10 mM glycerol2-phosphate disodium salt hydrate) was added and replenished every 2–3 days. On the 17th day, cells were fixed with 10% neutral buffered formalin and stained for alkaline phosphatase activity or for von Kossa positive bone nodules (2.5%weight/volume AgNO3). Reagents were purchased from Sigma-Aldrich.
RhoA and Cdc42 Activation Assays
Arhgap21+/− and WT BM cells were induced for osteogenic differentiation as described above and total protein was extracted on days 0, 7 and 16. Three pools of BM cells containing three mice each were evaluated per group. Day 0 denotes the addition of mineralizing media. RhoA and Cdc42 activities were determined in protein extracts using G-Lisa Activation Assays (Cytoskeleton, Inc.).
Cytokine Quantification
Cytokine levels were determined in peripheral blood (PB) and BM supernatant of Arhgap21+/− and WT mice. Osteocalcin levels were measured using Mouse OC/BGP (Osteocalcin) ELISA Kit, 96T (Elabscience Biotechnology Co.). Levels of G-CSF, M-CSF, IFN-γ, IL-1α, IL-1β, IL-4, IL-10, IL-17, and VEGF-A were measured using the customized Miliplex MAP Mouse Cytokine Magnetic Kit (Milipore, MCYTMAG-70K-PX32). Levels of TGF-β1 were measured by Multi-species TGFb-Singleplex (Milipore, TGFBMAG-64k-01).
Bone Marrow Transplants
Bone marrow transplantation was performed by injecting normal hematopoietic cells in Arhgap21+/− or WT mice. Briefly, 106 bone marrow cells from B6.SJL/BoyJ (PEP; CD45.1+, Jackson Labs) mice were transplanted into 9.5Gy sub-lethally irradiated WT (n = 5) or Arhgap21+/− (n = 8) (CD45.2+) recipient mice. All mice were 10-weeks old when transplanted. Donor reconstitution (CD45.1+) and hematological parameters (hemoglobin, platelets, and WBC) were evaluated every 4 weeks after transplant until 16 weeks post-transplantation, when animals were terminated. PB was collected by ocular cavity and hematological parameters were analyzed using a CELL-DYN Emerald Hematology System counter (Abbott Laboratories). Chimerism was evaluated by FACScalibur flow cytometer using mAb CD45.1-PE (BD Bioscience).
Immunophenotyping of BM and PB Cells From Recipient Mice
BM cells from recipient mice were characterized 16 weeks post-transplant by flow cytometry using the mAbs CD45-PE (pan leucocyte marker); Lineage-APC/Sca-1-PerCP/c-Kit-FITC (LSKs); Gr-1-PerCP/Mac-1-FITC (myeloid cells). PB cells were characterized using CD45-PE (pan leucocyte marker); CD3-FITC (T cells); B220-APC (B cells); CD11b-FITC (monocytes/granulocytes). All antibodies were from BD Biosciences. At least 1,000,000 events were acquired using a FACScalibur flow cytometer. Gated cells were analyzed using the FlowJo software (TreeStar Inc.). Absolute numbers of WBC subpopulations were calculated by multiplying their frequencies per total leucocyte number obtained with complete blood count test.
Patient Samples
ARHGAP21 gene expression was analyzed in BM-MSCs cultures from myelodysplastic syndromes (MDS, n = 21) and acute myeloid leukemia (AML, n = 18) patients. BM-MSCs cultures obtained from heathy donors (HD) were used as control (n = 7). Briefly, BM mononuclear cells were isolated by density gradient centrifugation and cultured at 37°C, 5% CO2 in Dulbecco’s Modified Eagle Medium (DMEM) media containing 1% penicillin/streptomycin, 1% L-glutamine and 10% FBS for 3–4 days, when non-adherent cells were removed. Monolayers were sub-cultured at approximately 80% confluence. To minimize the possible modifications that these cells may have acquired when cultured, all cell cultures were maintained under the same conditions and expanded only until the fourth passage. MSC cultures were previously characterized by our group (Lopes et al., 2017) and RNA samples were stored at −80°C. ARHGAP21 expression was also evaluated in total BM cells from HD (n = 9) and patients with MDS (n = 48) and AML (n = 50). MDS patients were classified according to the World Health Organization (WHO-2016) and AML patients were classified into AML with myelodysplasia-related changes (AML-MRC) and de novo AML. All patients were untreated when samples were collected, and their characteristics are described in Supplementary Table 1. Participants provided their informed written consent, and the study was approved by the Ethics Committee of UNICAMP and was adherent to the Declaration of Helsinki.
Quantitative PCR
Total RNA was extracted from patient samples using TRIzol Reagent (Thermo Fisher Scientific). RNeasy Micro Kit (Qiagen) was used to isolate total RNA from BM cells under osteoblastic differentiation. RevertAid First Strand cDNA synthesis kit (Thermo Scientific) was used for the reverse transcription reaction of all samples. Gene expression was analyzed by quantitative PCR using Maxima SYBR Green/ROX qPCR Master Mix (2X) (Thermo Scientific) and ABI-7500 Sequence Detection System (Applied Biosystems). A negative “no template control” was included for each pair of primers. Primer sequences are described in Supplementary Table 2. Relative expression was calculated using the 2−ΔΔCT equation (Livak and Schmittgen 2001).
Statistical Analysis
Statistical analysis was performed using GraphPad Prism 9 software (GraphPad Software). ANOVA or Student t-test was used to analyze the results obtained with Arhgap21+/− and WT mice. Mann-Whitney test was used for comparisons of gene expression from patient samples. Data represent mean ± SD. A p < 0.05 was considered statistically significant.
Results
Arhgap21+/− Mice Present Increased Osteoblastic Lineage Cells (OBCs) and Osteocalcin Levels in the Bone Marrow
Homozygous Arhgap21 knockout mice (Arhgap21−/−) were not detected 8 days post-conception. Therefore, we aimed to characterize the BM microenvironment of heterozygous Arhgap21+/− mice, which present an average of approximately 50% reduction of Arhgap21 expression in the BM (Xavier-Ferrucio et al., 2018). We first evaluated the frequency of BM multipotent stromal cell population, osteoblasts, and endothelial cells by immunophenotyping with flow cytometry analysis (Figure 1A). BM cells from Arhgap21+/− mice (n = 12) showed no difference in the frequency of MSCs compared to WT (n = 11) (Figure 1B). However, Arhgap21+/− BM presented increased phenotypic OBCs (Figure 1C). Similar frequencies of AEC and SEC were found in both mice groups (Figures 1D,E).
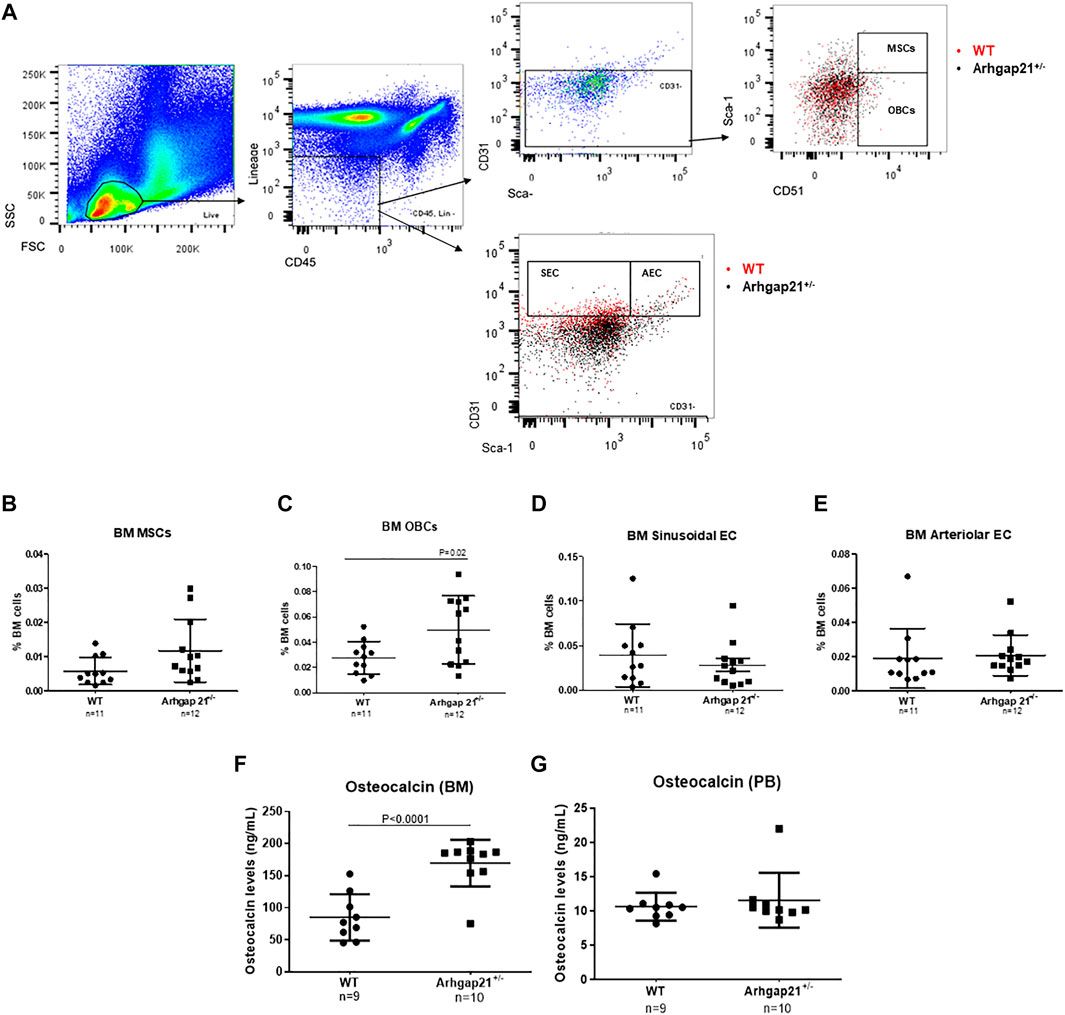
FIGURE 1. Increased osteoblastic cell population in Arhgap21+/− mice. (A) Flow cytometry gating strategy used to identify mesenchymal stromal cells (MSCs), osteoblasts (OBCs), sinusoidal endothelial cells (SEC) and arteriolar endothelial cells (AEC) in murine bone marrow (BM). Last plots show the overlay of wild type (WT in red) and Arhgap21+/− (in black) cellular populations. (B) Frequency of MSCs among total bone marrow cells did not significantly differ between WT and Arhgap21+/− mice. (C) Arhgap21+/− mice presented increased percentage of BM OBCs compared with WT mice (p = 0.02). (D) Similar frequencies of BM SEC and (E) AEC populations were detected in WT and Arhgap21+/− mice. (F) Osteocalcin levels were increased in bone marrow supernatant, (G) but not in peripheral blood (PB), from Arhgap21+/− mice compared with WT (p < 0.0001). In all graphs, each dot represents an individual mouse; mean and standard error of the mean are shown; 2-way Student’s t-test.
Serum level of osteocalcin, which is exclusively synthesized by osteoblasts, is used as a marker of bone formation (Zoch et al., 2016). Osteocalcin levels were then analyzed in the BM supernatant and PB plasma of Arhgap21+/− (n = 10) and WT (n = 9) mice. BM supernatant of Arhgap21+/− mice presented increased levels of osteocalcin compared to WT (p < 0.0001) (Figure 1F), but no difference was observed in PB (Figure 1G).
Osteoblasts synthesize and secrete other signaling molecules in addition to osteocalcin, such as growth factors, cytokines and chemokines to support bone architecture and remodeling (Han et al., 2018). We evaluated the levels of G-CSF, M-CSF, IFN-γ, IL-1α, IL-1β, IL-4, IL-10, IL-17, VEGF-A and TGF-β1 in the BM supernatant of the same mice, but no differences were observed between Arhgap21+/− and WT (Supplementary Figure 1). Among these cytokines, only G-CSF, IL-1α, IL-17 and TGF-β1 were detected in the murine PB with high variation and no statistically significant difference between Arhgap21+/− (n = 3) and WT (n = 3) (data not shown).
Expression of Osteogenic Markers is Increased in Arhgap21+/− BM Stromal Cells Submitted to Osteogenic Differentiation In Vitro
We next investigated whether the increased frequency of OBC in the Arhgap21+/− BM microenvironment could be caused by enhanced osteogenic differentiation of BM cells. Therefore, we evaluated their ability to form colonies of fibroblasts (CFU-F) and osteoblasts (CFU-OB) in vitro. No differences were observed in the numbers of CFU-F (stained positive for alkaline phosphatase or crystal violet) obtained from BM cells of Arhgap21+/− and WT mice (Figures 2A,B). According to the immunophenotyping findings, the number of Arhgap21+/− CFU-OB stained for alkaline phosphatase activity was significantly increased compared to controls (p < 0.05) (Figure 2C). An elevated number of von Kossa-positive CFU-OB was also obtained from Arhgap21+/− mice compared to WT, although not statistically significant (Figure 2D).
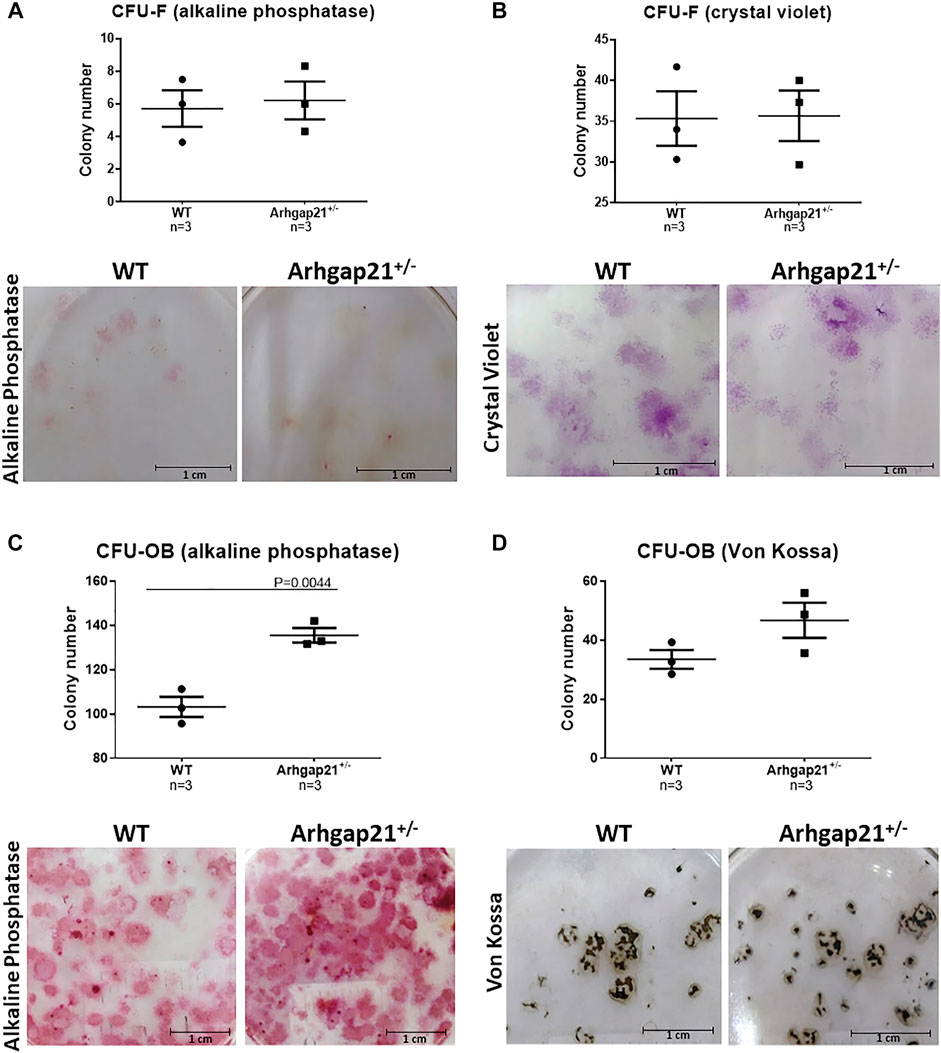
FIGURE 2. Arhgap21+/− BM cells have a higher potential to form CFU-OB with activity for alkaline phosphatase. (A) Number of total CFU fibroblasts (CFU-F) positive stained for alkaline phosphatase or (B) crystal violet obtained from WT (n = 3) and Arhgap21+/− (n = 3) BM cells cultured for a period of 10–14 days. Representative photographs of the stained colonies are shown in the lower panels. (C) Number of alkaline phosphatase–positive CFU-OB and (D) Von Kossa–positive mineralized nodules formed from BM cells after 17 days of culture under osteoblast conditions. Representative photographs of the stained colonies are shown in the lower panels. We detected a significantly higher number of colonies with alkaline phosphatase activity formed from Arhgap21+/− BM cells compared with WT (p = 0.0044). Three independent biological experiments were performed in triplicate for each assay. (In all graphs, each dot represents an individual mouse; mean and standard error of the mean are shown; 2-way Student’s t-test.
Expression of osteogenic-associated genes was evaluated before (day 0), and during early (day 7) and late (day 16) stages of osteogenic differentiation. Culturing in osteogenic media induced the expression of collagen type 1 alpha 1 (Col1a1), osteocalcin (Ocn) and osteoprotegerin (Opn). Ocn, a marker of late osteogenic differentiation (Amin et al., 2012, 2013), was not detected on day 0, whereas Opn, a marker of early osteogenic differentiation (Amin et al., 2012, 2013), was upregulated only on day 7 (Figures 3A–C). Expression of the negative regulators of osteogenic differentiation, tyrosine-rich amelogenin peptide (Trap) and nuclear factor κ B (Rank) were also analyzed (Figures 3D,E).
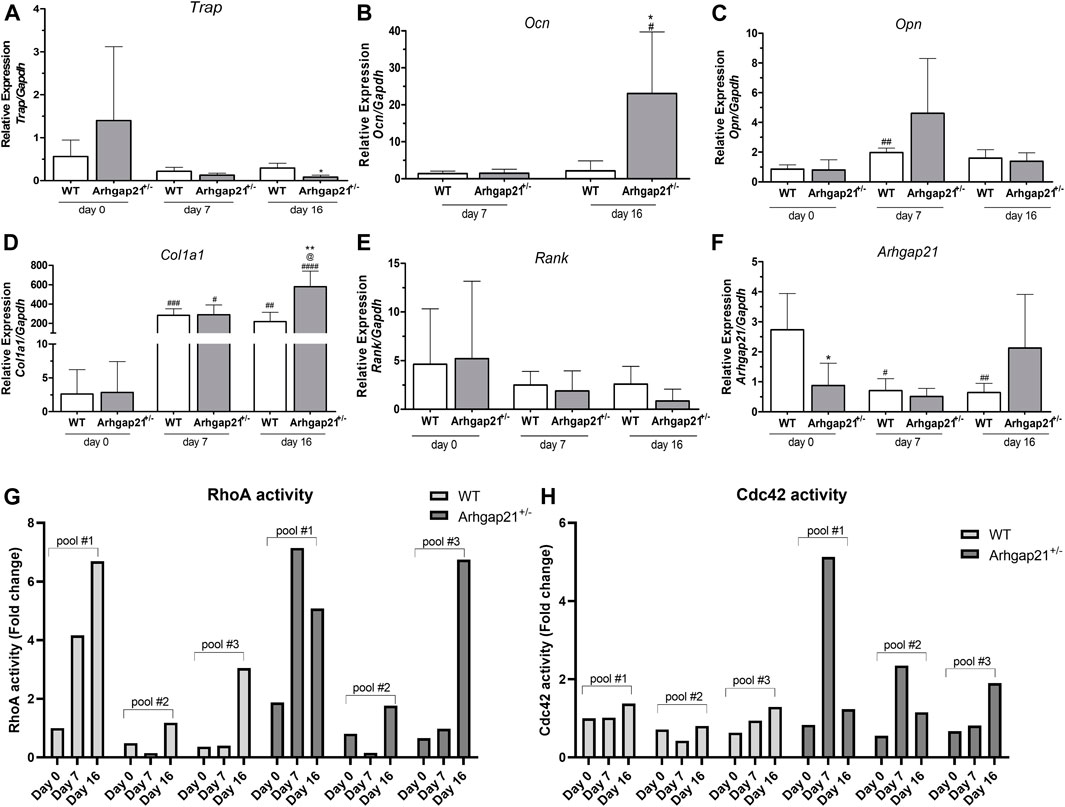
FIGURE 3. Arhgap21+/− BM cells present increased expression of osteogenic markers when induced for osteoblast differentiation. (A–F) Analysis of the mRNA expression of Col1a1, Ocn, Opn, Trap, Rank and Arhgap21 before (day 0) and on days 7 and 16 after induction of differentiation (mean ± S.D., n = 4). (A) Expression of Col1a1 and (B) Ocn (both positive regulators of osteoblastic differentiation) was increased in Arhgap21+/− cells compared to WT cells on day 16 of differentiation. Ocn expression was not detected on day 0 in both groups. (D) Athgap21+/− cultures also showed decreased levels of Trap on day 16. Expression of (C) Opn and (E) Rank did not significantly differ between Arhgap21+/− and WT during the differentiation, (F) whereas Arhgap21 expression was reduced only during differentiation of WT cells. 2-way Student’s t-test. (G) RhoA and (H) Cdc42 activities were also evaluated during osteogenic differentiation of Ahgap21+/− and WT BM cells. RhoA activity seems to increase through the induction of differentiation of both Ahgap21+/− and WT BM cells, whereas Cdc42 activity appears to increase only in Arhgap21+/− cells. RhoA and Cdc42 activities were analyzed in three pools of Arhgap21+/− or WT BM cells containing three animals each.
In accordance with CFU-OB counts, Arhgap21+/− cultures showed increased mRNA levels of Col1a1 (mean 580.51 ± SD 159.56 in Arhgap21+/− versus 218.75 ± 96.63 in WT, p < 0.01) and Ocn (23.06 ± 16.62 in Arhgap21+/− versus 2.16 ± 2.68 in WT, p < 0.05) on day 16 (Figures 3A,B). Expression of Trap1 was decreased in Arhgap21+/− cultures (0.08 ± 0.04 in Arhgap21+/− versus 0.29 ± 0.10 in WT, p < 0.05) on day 16 (Figure 3D). No significant differences were observed in the expression of Opn (Figure 3C) and Rank (Figure 3E) between Arhgap21+/− and WT cultures.
ARHGAP21 was previously described as upregulated during erythroid (Sanchez Bassères et al., 2002) and megakaryocyte (Bernusso et al., 2021) differentiation of human hematopoietic cells. Therefore, we also evaluated whether Arhgap21 mRNA levels would be altered during osteogenic differentiation. As expected, Arhgap21 expression was reduced in Arhgap21+/− BM cells compared to WT cells on day 0 of differentiation. Culturing in osteogenic media induced a decrease in Arhgap21 expression in WT cells (1.73 ± 1.21 on day 0 versus 0.70 ± 0.40 on day 7 and 0.64 ± 0.3 on day 16, p < 0.01) but not in Arhgap21+/− cells (Figure 3F).
RhoA and Cdc42 signaling pathways act as positive regulators of MSC osteogenesis (Mcbeath et al., 2004; Gao et al., 2011; Wang et al., 2012; Wang et al., 2012; John et al., 2018). We then investigated the activity of these Rho GTPases during osteogenic differentiation. RhoA activity was increased during differentiation, especially in late differentiation (day 16) in both Arhgap21+/− and WT cultures. Interestingly, Cdc42 activity seemed to increase only during differentiation of Arhgap21+/− cells, whereas no changes were observed during differentiation of WT BM cells.
Arhgap21+/− Recipient Mouse Showed Reduced Numbers of WBC After BM Transplant
Osteoblasts have been shown to regulate the number and localization of transplanted HSC in the BM (Calvi et al., 2003; Zhang et al., 2003; Ho and Méndez-Ferrer 2020). Therefore, we investigated whether the increased population of OBCs in the Arhgap21+/− BM niche would interfere in hematopoietic reconstitution. Accordingly, we performed BM transplants with wild-type CD45.1 hematopoietic stem and progenitor cells (HSPC) into conditioned Arhgap21+/− or wild-type recipient mice (both CD45.2) (Figure 4A). Transplant efficiency was tracked every 4 weeks post-transplantation until 16 weeks, when mice were terminated. No difference was observed in the chimerism of Arhgap21+/− and WT recipients (Figure 4B). Arhgap21+/− recipient mice showed significantly reduced levels of WBC compared to WT after 8 (p < 0.01) and 16 (p < 0.05) weeks post-transplant (Figure 4C). Hemoglobin levels and platelet counts did not differ between Arhgap21+/− and WT recipient mice either (Figures 4D,E). Sixteen weeks post-transplant, the BM from Arhgap21+/− and WT recipients was analyzed by flow cytometry and no statistically significant differences were observed in the frequencies of LSK (Figure 4F), GR-1+Mac-1+ cells (Figure 4G). Absolute counts of CD11b+ and B220+ cells were decreased in the PB of Arhgap21+/− recipients compared to WT group (p < 0.05), whereas no differences were detected in the numbers of CD3+ cells (Figure 4H).
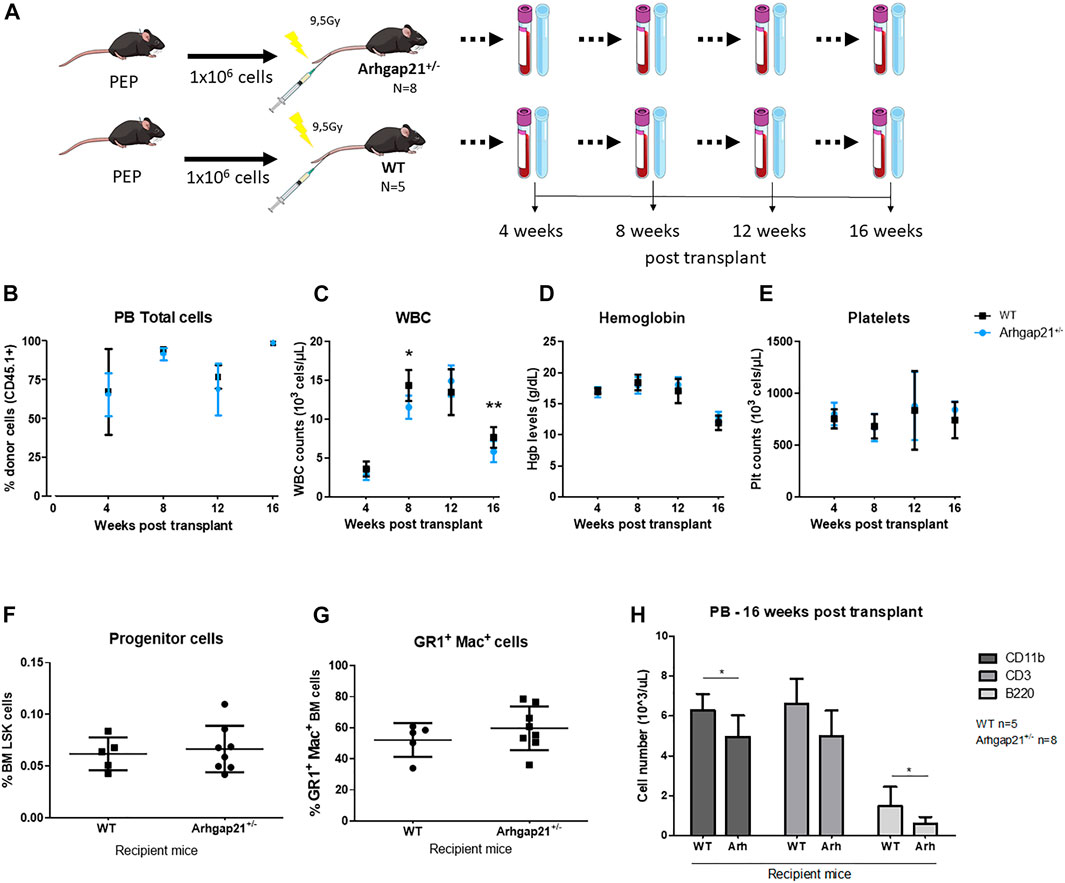
FIGURE 4. Decreased WBC reconstitution in Arhgap21+/− recipients of bone marrow transplantation. (A) Schematic figure of 1 × 106 PEP BM cells transplanted into irradiated (9.5 Gy) Arhgap21+/− recipients (N = 8) and WT recipients (N = 5). Recipient mice were maintained for 16 weeks after transplantation and chimerism (CD45.1+), WBC count, hemoglobin levels (Hgb) and platelet count (Plt) were assessed every 4 weeks post-transplant. (B) Engraftment of PEP marrow cells CD45.1+ were similar in WT and Arhgap21+/− recipients. (C) WBC counts were decreased in Arhgap21+/− recipients compared with WT recipients at weeks 8 and 16 following the transplant. (D) Hemoglobin levels and (E) platelet counts did not differ between WT and Arhgap21+/− recipients. (F) Frequency of immature LSK and (G) GR1+ Mac+ cellular populations were also similar between the two groups. (H) Absolute counts of CD11b+ and B220 + cells (but not CD3+ cells) were decreased in the PB of Arhgap21+/− recipients compared with WT recipients. Mean and standard error of the mean are shown in all graphs; 2-way Student’s t-test; *p < 0.05.
ARHGAP21 Gene Expression Is Increased in Mesenchymal Stromal Cells From AML Patients
Besides playing an important role in regulating normal HSCs, the osteogenic niche has been shown to participate in the pathogenesis of MDS and AML. Genetic depletion of osteoblasts in AML mouse models increased tumor engraftment and blast numbers leading to shorter survival. Conversely, maintenance of the osteoblast pool resulted in reduced tumor burden and longer survival (Krevvata et al., 2014). AML patients present inhibition of osteogenesis associated with impaired expression of genes related to bone growth and mineralization in the BM, concomitant with decreased levels of osteocalcin in PB (Chen et al., 2020). Therefore, we wondered whether ARHGAP21 gene expression would be altered in MSCs from MDS and AML patients.
MSCs from de novo AML patients [median 1.50 (range 0.43–3.69)] presented higher ARHGAP21 expression compared to MSCs from HD [0.13 (0.07–1)], p = 0.0035. ARHGAP21 expression was also increased in MSCs from de novo AML when compared to MDS [0.20 (0.00–1.65)], p = 0.0001, and AML-MRC patients [0.22 (0.43–0.32)], p = 0.0009. No statistical differences in ARHGAP21 expression were observed between the two groups of MDS patients classified according to the WHO-2016 [RS-SLD/RS-MLD/del(5q)/SLD/MLD versus EB-1/EB-2] (Supplementary Figure 2A). Correspondingly, ARHGAP21 expression was increased in total bone marrow samples from de novo AML patients compared to MDS patients classified according to WHO classification [RS-SLD/RS-MLD/del(5q)/SLD/MLD (p = 0.0036) and EB-1/EB-2 (p = 0.0093)] (Supplementary Figure 2B).
Discussion
Rho GTPases control several aspects of hematopoiesis, including the interactions between HSPC and other components of the BM microenvironment (Jansen et al., 2005; Yang et al., 2007; Cancelas and Williams 2009; Jaganathan et al., 2013). However, little is known about the participation of Rho GTPase regulators in the hematopoietic niche. Results from this study indicate that the RhoGAP Arhgap21 is important for a proper composition of the BM microenvironment.
The bone marrow of Arhgap21 heterozygous knockout mouse (Arhgap21+/−) presented expansion of osteoblastic lineage cells and increased levels of osteocalcin, pointing out a role of Arhgap21 in osteogenic niche. Notably, these changes are not homogeneous within the Arhgap21+/− mice and phenotypic variation was already reported for other hematological parameters in Arhgap21+/− individuals (Xavier-Ferrucio et al., 2018). High phenotypic heterogeneity is frequently observed in other transgenic mice, including heterozygous knockout mouse models for other RhoGAPs and mouse models for hematological malignancies (Raman et al., 2013; Balderman et al., 2016; McMichael et al., 2017; Lovat et al., 2018; Almosailleakh and Schwaller 2019). Osteoblast ablation in mice decreased the overall BM cellularity and altered the number of long-term HSC (LT-HSC) subtypes. HSC quiescence and long-term engraftment were also reduced by osteoblast depletion (Bowers et al., 2015). Our previous work revealed that Arhgap21+/− mice present increased frequency of phenotypic HSC with decreased ability to form colonies in vitro and in vivo and to engraft during serial transplantation (Xavier-Ferrucio et al., 2018). These alterations in Arhgap21+/− HSCs possibly result from a combination of intrinsic and extrinsic changes triggered by Arhgap21 reduction, such as modifications in the local microenvironment. Development of mouse models with cell type conditional Arhgap21 deletion will be important in assessing to what extent the HSPC alterations are due to the unbalanced microenvironment or the inverse. Notably, we herein observed a decreased WBC reconstitution when normal hematopoietic cells were transplanted into Arhgap21+/− recipients, possibly due to a decrease in monocytes/granulocytes and B lymphocytes. No differences were detected in the percentage of BM progenitor cells (LSK cells) neither in BM GR1+Mac + cells between Arhgap21+/− and WT recipients, suggesting that homing to the Arhgap21+/− BM niche was not altered and that the reduction in PB WBC is related to the differentiation process.
The higher frequency of osteoblasts may be caused by increased capacity of Arhgap21+/− BM cells to form functional osteoprogenitors (CFU-OB positive for alkaline phosphatase). Arhgap21+/− BM cells also presented enhanced expression of Col1a1 and Ocn when submitted to osteogenic differentiation. Type I collagen is the most abundant protein of the bone extracellular matrix and expression of Col1a1 is detected at early periods of osteogenic differentiation (Dacic et al., 2001). Ocn is exclusively produced by osteoblast lineage cells and is detected at late stages of differentiation (Dacic et al., 2001). Increased mRNA levels of both Col1a1 and Ocn were detected on day 16 of differentiation, indicating that Arhgap21 deficiency affects the late stages of differentiation. Arhgap21+/− cells also showed decreased mRNA levels of Trap (Acp5), a marker of osteoclast activity, in the end of differentiation possibly as a result the of fate decision towards osteoblast lineage.
The activity of Cdc42 seems to increase during osteogenic differentiation of Arhgap21+/−, but not in WT cells, which is in accordance with previous studies that showed increased Cdc42 upon ARHGAP21 inhibition (Dubois et al., 2005; Bigarella et al., 2009). Activation of Cdc42 signaling has been shown to enhance MSCs osteogenesis through the control of cell shape and cytoskeletal rearrangements required for differentiation (Gao et al., 2011; John et al., 2018). Therefore, the increased activity of Cdc42 in Arhgap21+/− cells may be a possible mechanism triggering higher osteoblast differentiation. RhoA activity was increased during osteogenic differentiation of Arhgap21+/− and WT cells, which is in agreement with previous studies (Arnsdorf et al., 2009; Zhang et al., 2019). However, no differences in RhoA activity were found between Arhgap21+/− and WT cells during differentiation.
There is increasing evidence that alterations in the BM microenvironment may facilitate the development of hematological neoplasia, which can originate in the niche itself (Kode et al., 2014; Méndez-Ferrer et al., 2020). We have previously demonstrated that MSCs derived from de novo AML patients present reduced growth rate and impaired immunosuppressive ability with a distinct cytokine expression profile (Lopes et al., 2017). Moreover, the osteoblast niche supposedly hides quiescent leukemia stem cells, cooperating for chemoresistance and relapse (Méndez-Ferrer et al., 2020). Interesting, we found increased ARHGAP21 gene expression in MSCs derived from BM cells of de novo AML patients in comparison with HD and patients with MDS or AML developed after myelodysplasia. Recent findings showed that AML MSCs present decreased expression of osteogenesis-related genes and impaired osteogenic differentiation capacity (Geyh et al., 2016; Xu et al., 2020). Lower levels of osteocalcin were also found in the PB of AML patients compared to non-leukemic subjects and predicted poorer clinical outcome (Chen et al., 2020). The elucidation of the effects of ARHGAP21 overexpression in AML niche is still pending, but we speculate that it is related to decreased osteogenesis reported in these patients.
In conclusion, our study revealed a novel function of Arhgap21 in the BM microenvironment. Using a heterozygous Arhgap21 knockout mouse model, we showed that decreased expression of this RhoGAP led to the expansion of BM osteoblastic niche, which may be associated with several hematological defects previously reported. ARHGAP21 also may participate in the deregulation of the leukemic microenvironment and could be investigated as a possible therapeutic target.
Data Availability Statement
The original contributions presented in the study are included in the article/Supplementary Material, further inquiries can be directed to the corresponding authors.
Ethics Statement
The studies involving human participants were reviewed and approved by Research Ethics Committee at the Medical Faculty of University of Campinas (FCM/UNICAMP). The patients/participants provided their written informed consent to participate in this study. The animal study was reviewed and approved by Ethics Committee for the Use of Animals at University of Campinas.
Author Contributions
MP designed and performed the experiments, analyzed, and interpreted data, and wrote the manuscript. CT, RS, IS, KF, RG, MRL, and PF contributed with experiments. SS and ML provided financial support. MP, SS, and ML conceived the study. SS and ML directed the research, and wrote the manuscript. All authors have read and approved the final version of the manuscript.
Funding
This study was supported by Sao Paulo Research Foundation (FAPESP processes 17/21801-2, 2017/19674-2, 2021/12922-6), National Council for Scientific and Technological Development (CNPq) and Coordination for the Improvement of Higher Education Personnel (CAPES Financial code 001).
Conflict of Interest
The authors declare that the research was conducted in the absence of any commercial or financial relationships that could be construed as a potential conflict of interest.
Publisher’s Note
All claims expressed in this article are solely those of the authors and do not necessarily represent those of their affiliated organizations, or those of the publisher, the editors and the reviewers. Any product that may be evaluated in this article, or claim that may be made by its manufacturer, is not guaranteed or endorsed by the publisher.
Acknowledgments
The authors would like to thank Fernanda Soares Niemann, Vanessa Aline Bernusso and Márcio Alves da Cruz for their valuable technical assistance. Part of the results from this manuscript was included in a Masters dissertation.
Supplementary Material
The Supplementary Material for this article can be found online at: https://www.frontiersin.org/articles/10.3389/fcell.2021.718560/full#supplementary-material
References
Almosailleakh, M., and Schwaller, J. (2019). Murine Models of Acute Myeloid Leukaemia. Int. J. Mol. Sci. 20, 453. doi:10.3390/ijms20020453
Amin, H. D., Olsen, I., Knowles, J. C., and Donos, N. (2012). Differential Effect of Amelogenin Peptides on Osteogenic Differentiation In Vitro: Identification of Possible New Drugs for Bone Repair and Regeneration. Tissue Eng. Part. A. 18 (11–12), 1193–1202. doi:10.1089/ten.TEA.2011.0375
Amin, H. D., Olsen, I., Knowles, J. C., Dard, M., and Donos, N. (2013). Effects of Enamel Matrix Proteins on Multi-Lineage Differentiation of Periodontal Ligament Cells In Vitro. Acta Biomater. 9 (1), 4796–4805. doi:10.1016/j.actbio.2012.09.008
Anthony, D. F., Sin, Y. Y., Vadrevu, S., Advant, N., Day, J. P., Byrne, A. M., et al. (2011). β-Arrestin 1 Inhibits the GTPase-Activating Protein Function of ARHGAP21, Promoting Activation of RhoA Following Angiotensin II Type 1A Receptor Stimulation. Mol. Cell Biol 31 (5), 1066–1075. doi:10.1128/mcb.00883-10
Arnsdorf, E. J., Tummala, P., Kwon, R. Y., and Jacobs, C. R. (2009). Mechanically Induced Osteogenic Differentiation - the Role of RhoA, ROCKII and Cytoskeletal Dynamics. J. Cell Sci. 122 (4), 546–553. doi:10.1242/jcs.036293
Balderman, S. R., Li, A. J., Hoffman, C. M., Frisch, B. J., Goodman, A. N., Lamere, M. W., et al. (2016). Targeting of the Bone Marrow Microenvironment Improves Outcome in a Murine Model of Myelodysplastic Syndrome. Blood 127 (5), 616–625. doi:10.1182/blood-2015-06-653113
Barcellos, K. S. A., Bigarella, C. L., Wagner, M. V., Vieira, K. P., Lazarini, M., Langford, P. R., et al. (2013). ARHGAP21 Protein, a New Partner of α-Tubulin Involved in Cell-Cell Adhesion Formation and Essential for Epithelial-Mesenchymal Transition. J. Biol. Chem. 288 (4), 2179–2189. doi:10.1074/jbc.m112.432716
Bernusso, V. A., Vieira, K. P., Duarte, A. S. S., Lescano, C. H., Mónica, F. Z., Vicente, C. P., et al. (2021). Deficiency of ARHGAP21 Alters Megakaryocytic Cell Lineage Responses and Enhances Platelet Hemostatic Function. Biochim. Biophys. Acta (BBA) - Mol. Cell Res. 1868 (6), 119012. doi:10.1016/j.bbamcr.2021.119012
Bigarella, C. L., Borges, L., Costa, F. F., and Saad, S. T. O. (2009). ARHGAP21 Modulates FAK Activity and Impairs Glioblastoma Cell Migration. Biochim. Biophys. Acta (BBA) - Mol. Cell Res. 1793 (5), 806–816. doi:10.1016/j.bbamcr.2009.02.010
Bowers, M., Zhang, B., Ho, Y., Agarwal, P., Chen, C.-C., and Bhatia, R. (2015). Osteoblast Ablation Reduces normal Long-Term Hematopoietic Stem Cell Self-Renewal but Accelerates Leukemia Development. Blood 125 (17), 2678–2688. doi:10.1182/blood-2014-06-582924
Calvi, L. M., Adams, G. B., Weibrecht, K. W., Weber, J. M., Olson, D. P., Knight, M. C., et al. (2003). Osteoblastic Cells Regulate the Haematopoietic Stem Cell Niche. Nature 425 (6960), 841–846. doi:10.1038/nature02040
Cancelas, J. A., and Williams, D. A. (2009). Rho GTPases in Hematopoietic Stem Cell Functions. Curr. Opin. Hematol. 16, 249–254. doi:10.1097/moh.0b013e32832c4b80
Chen, Y., Hoffmeister, L. M., Zaun, Y., Arnold, L., Schmid, K. W., Giebel, B., et al. (2020). Acute Myeloid Leukemia-Induced Remodeling of the Human Bone Marrow Niche Predicts Clinical Outcome. Blood Adv. 4 (20), 5257–5268. doi:10.1182/bloodadvances.2020001808
Cho, E., Chen, Z., Lee, J., Lee, S., and Lee, T.-H. (2019). PSTP-3,5-Me Inhibits Osteoclast Differentiation and Bone Resorption. Molecules 24 (18), 3346. doi:10.3390/molecules24183346
Dacic, S., Kalajzic, I., Visnjic, D., Lichtler, A. C., and Rowe, D. W. (2001). Col1a1-driven Transgenic Markers of Osteoblast Lineage Progression. J. Bone Miner Res. 16 (7), 1228–1236. doi:10.1359/jbmr.2001.16.7.1228
Dubois, T., Paléotti, O., Mironov, A. A., Fraisier, V., Stradal, T. E. B., De Matteis, M. A., et al. (2005). Golgi-localized GAP for Cdc42 Functions Downstream of ARF1 to Control Arp2/3 Complex and F-Actin Dynamics. Nat. Cell Biol 7 (4), 353–364. doi:10.1038/ncb1244
Frisch, B. J., Ashton, J. M., Xing, L., Becker, M. W., Jordan, C. T., and Calvi, L. M. (2012). Functional Inhibition of Osteoblastic Cells in an In Vivo Mouse Model of Myeloid Leukemia. Blood 119 (2), 540–550. doi:10.1182/blood-2011-04-348151
Galán-Díez, M., and Kousteni, S. (2017). The Osteoblastic Niche in Hematopoiesis and Hematological Myeloid Malignancies. Curr. Mol. Bio Rep. 3 (2), 53–62. doi:10.1007/s40610-017-0055-9
Gao, L., Gorski, J. L., and Chen, C. S. (2011). The Cdc42 Guanine Nucleotide Exchange Factor FGD1 Regulates Osteogenesis in Human Mesenchymal Stem Cells. Am. J. Pathol. 178 (3), 969–974. doi:10.1016/j.ajpath.2010.11.051
Geyh, S., Rodríguez-Paredes, M., Jäger, P., Khandanpour, C., Cadeddu, R.-P., Gutekunst, J., et al. (2016). Functional Inhibition of Mesenchymal Stromal Cells in Acute Myeloid Leukemia. Leukemia 30 (3), 683–691. doi:10.1038/leu.2015.325
Han, Y., You, X., Xing, W., Zhang, Z., and Zou, W. (2018). Paracrine and Endocrine Actions of Bone - the Functions of Secretory Proteins from Osteoblasts, Osteocytes, and Osteoclasts. Bone Res. 6, 16. doi:10.1038/s41413-018-0019-6
Ho, Y.-H., and Méndez-Ferrer, S. (2020). Microenvironmental Contributions to Hematopoietic Stem Cell Aging. Haematologica 105 (1), 38–46. doi:10.3324/haematol.2018.211334
Huang, S., Xu, L., Sun, Y., Wu, T., Wang, K., and Li, G. (2015). An Improved Protocol for Isolation and Culture of Mesenchymal Stem Cells from Mouse Bone Marrow. J. Orthopaedic Translation 3, 26–33. doi:10.1016/j.jot.2014.07.005
Jaganathan, B., Anjos-Afonso, F., Kumar, A., and Bonnet, D. (2013). Active RHOA Favors Retention of Human Hematopoietic Stem/progenitor Cells in Their Niche. J. Biomed. Sci. 20 (1), 66. doi:10.1186/1423-0127-20-66
Jansen, M., Yang, F.-C., Cancelas, J. A., Bailey, J. R., and Williams, D. A. (2005). Rac2-Deficient Hematopoietic Stem Cells Show Defective Interaction with the Hematopoietic Microenvironment and Long-Term Engraftment Failure. Stem Cells 23 (3), 335–346. doi:10.1634/stemcells.2004-0216
John, A. A., Prakash, R., Kureel, J., and Singh, D. (2018). Identification of Novel microRNA Inhibiting Actin Cytoskeletal Rearrangement Thereby Suppressing Osteoblast Differentiation. J. Mol. Med. 96 (5), 427–444. doi:10.1007/s00109-018-1624-y
Kode, A., Manavalan, J. S., Mosialou, I., Bhagat, G., Rathinam, C. V., Luo, N., et al. (2014). Leukaemogenesis Induced by an Activating β-catenin Mutation in Osteoblasts. Nature 506 (7487), 240–244. doi:10.1038/nature12883
Krause, D. S., Lazarides, K., Lewis, J. B., Von Andrian, U. H., and Van Etten, R. A. (2014). Selectins and Their Ligands Are Required for Homing and Engraftment of BCR-ABL1+ Leukemic Stem Cells in the Bone Marrow Niche. Blood 123 (9), 1361–1371. doi:10.1182/blood-2013-11-538694
Krevvata, M., Silva, B. C., Manavalan, J. S., Galan-Diez, M., Kode, A., Matthews, B. G., et al. (2014). Inhibition of Leukemia Cell Engraftment and Disease Progression in Mice by Osteoblasts. Blood 124 (18), 2834–2846. doi:10.1182/blood-2013-07-517219
Lazarini, M., Traina, F., Machado-Neto, J. A., Barcellos, K. S. A., Moreira, Y. B., Brandão, M. M., et al. (2013). ARHGAP21 Is a RhoGAP for RhoA and RhoC with a Role in Proliferation and Migration of Prostate Adenocarcinoma Cells. Biochim. Biophys. Acta (BBA) - Mol. Basis Dis. 1832 (2), 365–374. doi:10.1016/j.bbadis.2012.11.010
Livak, K. J., and Schmittgen, T. D. (2001). Analysis of Relative Gene Expression Data Using Real-Time Quantitative PCR and the 2−ΔΔCT Method. Methods 25 (4), 402–408. doi:10.1006/meth.2001.1262
Lopes, M. R., Pereira, J. K., de Melo Campos, P., Machado-Neto, J. A., Traina, F., Saad, S. T., et al. (2017). De Novo AML Exhibits Greater Microenvironment Dysregulation Compared to AML with Myelodysplasia-Related Changes. Sci. Rep. 7, 40707. doi:10.1038/srep40707
Lovat, F., Fassan, M., Sacchi, D., Ranganathan, P., Palamarchuk, A., Bill, M., et al. (2018). Knockout of Both miR-15/16 Loci Induces Acute Myeloid Leukemia. Proc. Natl. Acad. Sci. USA 115 (51), 13069–13074. doi:10.1073/pnas.1814980115
Man, Y., Yao, X., Yang, T., and Wang, Y. (2021). Hematopoietic Stem Cell Niche during Homeostasis, Malignancy, and Bone Marrow Transplantation. Front. Cell Developmental Biol. 9 (January), 1–11. doi:10.3389/fcell.2021.621214
Mcbeath, R., Pirone, D. M., Nelson, C. M., Bhadriraju, K., and Chen, C. S. (2004). Cell Shape, Cytoskeletal Tension, and RhoA Regulate Stem Cell Lineage Commitment. Developmental Cell 6, 483–495. doi:10.1016/s1534-5807(04)00075-9
McMichael, B. K., Jeong, Y.-H., Auerbach, J. A., Han, C.-M., Sedlar, R., Shettigar, V., et al. (2017). The RhoGAP Myo9b Promotes Bone Growth by Mediating Osteoblastic Responsiveness to IGF-1. J. Bone Miner Res. 32 (10), 2103–2115. doi:10.1002/jbmr.3192
Méndez-Ferrer, S., Bonnet, D., Steensma, D. P., Hasserjian, R. P., Ghobrial, I. M., Gribben, J. G., et al. (2020). Bone Marrow Niches in Haematological Malignancies. Nat. Rev. Cancer 20 (5), 285–298. doi:10.1038/s41568-020-0245-2
Méndez-Ferrer, S., Michurina, T. V., Ferraro, F., Mazloom, A. R., MacArthur, B. D., Lira, S. A., et al. (2010). Mesenchymal and Haematopoietic Stem Cells Form a Unique Bone Marrow Niche. Nature 466 (7308), 829–834. doi:10.1038/nature09262
Pinho, S., and Frenette, P. S. (2019). Haematopoietic Stem Cell Activity and Interactions with the Niche. Nat. Rev. Mol. Cell Biol 20, 303–320. doi:10.1038/s41580-019-0103-9
Raman, R., Kumar, R. S., Hinge, A., Kumar, S., Nayak, R., Xu, J., et al. (2013). P190-B RhoGAP Regulates the Functional Composition of the Mesenchymal Microenvironment. Leukemia 27 (11), 2209–2219. doi:10.1038/leu.2013.103
Sanchez Bassères, D., Vedelago Tizzei, E., Duarte, A. A., Costa, F. F., and Teresinha Olalla Saad, S. (2002). ARHGAP10, a Novel Human Gene Coding for a Potentially Cytoskeletal Rho-GTPase Activating Protein. Biochem. Biophysical Res. Commun. 294 (3), 579–585. doi:10.1016/s0006-291x(02)00514-4
Schepers, K., Pietras, E. M., Reynaud, D., Flach, J., Binnewies, M., Garg, T., et al. (2013). Myeloproliferative Neoplasia Remodels the Endosteal Bone Marrow Niche into a Self-Reinforcing Leukemic Niche. Cell Stem Cell 13 (3), 285–299. doi:10.1016/j.stem.2013.06.009
Thompson, W. R., Yen, S. S., Uzer, G., Xie, Z., Sen, B., Styner, M., et al. (2018). LARG GEF and ARHGAP18 Orchestrate RhoA Activity to Control Mesenchymal Stem Cell Lineage. Bone 107, 172–180. doi:10.1016/j.bone.2017.12.001
Visnjic, D., Kalajzic, Z., Rowe, D. W., Katavic, V., Lorenzo, J., and Aguila, H. L. (2004). Hematopoiesis Is Severely Altered in Mice with an Induced Osteoblast Deficiency. Blood 103 (9), 3258–3264. doi:10.1182/blood-2003-11-4011
Wang, W., Zimmerman, G., Huang, X., Yu, S., Myers, J., Wang, Y., et al. (2016). Aberrant Notch Signaling in the Bone Marrow Microenvironment of Acute Lymphoid Leukemia Suppresses Osteoblast-Mediated Support of Hematopoietic Niche Function. Cancer Res. 76 (6), 1641–1652. doi:10.1158/0008-5472.can-15-2092
Wang, Y.-K., Yu, X., Cohen, D. M., Wozniak, M. A., Yang, M. T., Gao, L., et al. (2012). Bone Morphogenetic Protein-2-Induced Signaling and Osteogenesis Is Regulated by Cell Shape, RhoA/ROCK, and Cytoskeletal Tension. Stem Cell Development 21 (7), 1176–1186. doi:10.1089/scd.2011.0293
Weber, J. M., Forsythe, S. R., Christianson, C. A., Frisch, B. J., Gigliotti, B. J., Jordan, C. T., et al. (2006). Parathyroid Hormone Stimulates Expression of the Notch Ligand Jagged1 in Osteoblastic Cells. Bone 39 (3), 485–493. doi:10.1016/j.bone.2006.03.002
Xavier-Ferrucio, J., Ricon, L., Vieira, K., Longhini, A. L., Lazarini, M., Bigarella, C. L., et al. (2018). Hematopoietic Defects in Response to Reduced Arhgap21. Stem Cell Res 26, 17–27. doi:10.1016/j.scr.2017.11.014
Xu, Z., Sun, Y., Wei, Z., Jiang, J., Xu, J., and Liu, P. (2020). Suppression of CXCL-1 Could Restore Necroptotic Pathway in Chronic Lymphocytic Leukemia. Ott 13, 6917–6925. doi:10.2147/ott.s256993
Yang, L., Wang, L., Geiger, H., Cancelas, J. A., Mo, J., and Zheng, Y. (2007). Rho GTPase Cdc42 Coordinates Hematopoietic Stem Cell Quiescence and Niche Interaction in the Bone Marrow. Proc. Natl. Acad. Sci. 104 (12), 5091–5096. doi:10.1073/pnas.0610819104
Yeung, C. Y., Taylor, S. H., Garva, R., Holmes, D. F., Zeef, L. A., Soininen, R., et al. (2014). Arhgap28 Is a rhoGAP that Inactivates rhoA and Downregulates Stress Fibers. PLoS ONE 9 (9), e107036. doi:10.1371/journal.pone.0107036
Zhang, J., Niu, C., Ye, L., Huang, H., He, X., Tong, W.-G., et al. (2003). Identification of the Haematopoietic Stem Cell Niche and Control of the Niche Size. Nature 425 (6960), 836–841. doi:10.1038/nature02041
Zhang, Y., Shen, S., Li, P., Fan, Y., Zhang, L., Li, W., et al. (2019). PLEXIN-B2 Promotes the Osteogenic Differentiation of Human Bone Marrow Mesenchymal Stem Cells via Activation of the RhoA Signaling Pathway. Cell Signal 62, 109343. doi:10.1016/j.cellsig.2019.06.008
Keywords: osteocalcin, myelodysplastic syndromes, acute myeloid leukemia, RhoGAP, Rho GTPase, RhoA, Cdc42 (cell division cycle 42 GTP-binding protein)
Citation: Pissarra MF, Torello CO, Gomes RGB, Shiraishi RN, Santos I, Vieira Ferro KP, Lopes MR, Bergamo Favaro PM, Olalla Saad ST and Lazarini M (2021) Arhgap21 Deficiency Results in Increase of Osteoblastic Lineage Cells in the Murine Bone Marrow Microenvironment. Front. Cell Dev. Biol. 9:718560. doi: 10.3389/fcell.2021.718560
Received: 01 June 2021; Accepted: 08 November 2021;
Published: 30 November 2021.
Edited by:
Aldo Roccaro, Civil Hospital of Brescia, ItalyCopyright © 2021 Pissarra, Torello, Gomes, Shiraishi, Santos, Vieira Ferro, Lopes, Bergamo Favaro, Olalla Saad and Lazarini. This is an open-access article distributed under the terms of the Creative Commons Attribution License (CC BY). The use, distribution or reproduction in other forums is permitted, provided the original author(s) and the copyright owner(s) are credited and that the original publication in this journal is cited, in accordance with accepted academic practice. No use, distribution or reproduction is permitted which does not comply with these terms.
*Correspondence: Sara Teresinha Olalla Saad, sara@unicamp.br; Mariana Lazarini, lazarini@unifesp.br
†Present address: Matheus Rodrigues Lopes, Federal University of Vale do São Francisco, Paulo Afonso, Brazil