- Centro de Biología Molecular Severo Ochoa (C.S.I.C.-U.A.M.), Universidad Autónoma de Madrid, Madrid, Spain
Appendage development requires the coordinated function of signaling pathways and transcription factors to pattern the leg along the three main axes: the antero-posterior (AP), proximo-distal (PD), and dorso-ventral (DV). The Drosophila leg DV axis is organized by two morphogens, Decapentaplegic (Dpp), and Wingless (Wg), which direct dorsal and ventral cell fates, respectively. However, how these signals regulate the differential expression of its target genes is mostly unknown. In this work, we found that two members of the Drosophila forkhead family of transcription factors, Fd4 and Fd5 (also known as fd96Ca and fd96Cb), are identically expressed in the ventro-lateral domain of the leg imaginal disc in response to Dpp signaling. Here, we analyze the expression regulation and function of these genes during leg development. We have generated specific mutant alleles for each gene and a double fd4/fd5 mutant chromosome to study their function during development. We highlight the redundant role of the fd4/fd5 genes during the formation of the sex comb, a male specific structure that appears in the ventro-lateral domain of the prothoracic leg.
Introduction
Territorial specification depends on the ability of cells to activate a specific developmental program depending on their position within a tissue. The positional information, often provided by extrinsic signaling molecules, is integrated at the cis-regulatory modules (CRMs) of genes that encode for transcription factors that instruct cells with a unique developmental fate. Appendage development is a great model to study pattern formation, as it requires the specification of different cell fates along three main axes: the antero-posterior (AP), the dorso-ventral (DV), and the proximo-distal (PD) (Estella et al., 2012; Ruiz-Losada et al., 2018). More than 30 years of studies in Drosophila have identified many of the signals and transcription factors that pattern these axes, numerous of them conserved in vertebrates (Shubin et al., 1997; Tabin et al., 1999; Pueyo and Couso, 2005). An important, and yet not fully understood question is how the different signaling pathways regulate the restricted expression of the pattering genes along the three main appendage axes.
Appendages in Drosophila are derived from specialized epithelial sacs, named imaginal discs, which are specified in the embryo and grow and pattern during larva development (reviewed in Ruiz-Losada et al., 2018). The leg imaginal disc is divided into an anterior and posterior compartment by the expression of the selector gene engrailed (en) in the posterior compartment. En activates the expression of the short-range morphogene Hedgehog (Hh) in posterior compartment cells. Hh signals to anterior cells where it induces the transcription of two signaling molecules: Decapentaplegic (Dpp) in dorsal anterior cells and Wingless (Wg) in ventral anterior cells (Basler and Struhl, 1994). The dorsal and ventral domains of dpp and wg expression are maintained by a mutual repression, where Dpp prevents the activation of wg in dorsal cells and vice versa (Brook and Cohen, 1996; Jiang and Struhl, 1996; Johnston and Schubiger, 1996; Morimura et al., 1996; Penton and Hoffmann, 1996; Theisen et al., 1996). However, low levels of dpp expression could still be observed in the ventral domain of the leg disc (Figure 1D). Both, Dpp and Wg are required for the initiation and patterning of the PD and DV axes (Diaz-Benjumea et al., 1994; Brook and Cohen, 1996; Lecuit and Cohen, 1997; Campbell and Tomlinson, 1998). The juxtaposition of cells expressing high levels of Wg and Dpp in the center of the leg disc leads to the formation of the PD axis by activating a regulatory cascade of transcription factors that divide the leg in different domains of gene expression (Estella et al., 2012). In addition to initiating the PD axis formation, Wg and Dpp play an instructive role in the distinction between dorsal and ventral fates (Brook and Cohen, 1996; Jiang and Struhl, 1996; Johnston and Schubiger, 1996; Morimura et al., 1996; Penton and Hoffmann, 1996; Theisen et al., 1996). Dpp specifies dorsal fates and represses ventral ones, while Wg specifies ventral identities and represses dorsal fates. Therefore, hypomorphic mutations of wg show strong derepression of Dpp in the ventral domain and formation of ectopic dorsal structures in place of the corresponding ventral ones in the adult leg (Held et al., 1994). Similar phenotypes, but in the opposite direction, were observed in dpp mutants (Held et al., 1994). Interestingly, lateral fates are recovered in double hypomorphic mutants for dpp and wg, suggesting that the lateral fate is the default DV state (Held et al., 1994). The Dpp and Wg signaling molecules depend on the activation of a specific set of transcription factors that promote the DV fate of these cells. The family of T-box transcription factors plays an important role in the specification of DV identities (Brook, 2010). In the ventral domain of the leg, Wg activates while Dpp represses the expression of the redundant genes H15 and midline (mid) that act as selector genes for ventral fates, promoting the acquisition of ventral identity. Accordingly, mutants for H15/mid lack ventral leg structures or these are transformed to dorsal, whereas when ectopically expressed in the dorsal domain, H15/mid induce ventral fates (Svendsen et al., 2009, 2019). In the dorsal domain, the expression of the T-box genes optomotor-blind (omb) and the Dorsocross (Doc) 1, 2, and 3 are able to repress ventral genes (Maves and Schubiger, 1998; Reim et al., 2003; Svendsen et al., 2015). However, whether omb and the Doc genes are required to specify dorsal fates is mostly unknown. Gene expression analysis and loss of function studies suggested that the function of the PD and DV patterning genes is generally conserved across arthropods (Maves and Schubiger, 1998; Abzhanov and Kaufman, 2000; Prpic et al., 2001; Inoue et al., 2002; Angelini and Kaufman, 2004; Ober and Jockusch, 2006; Janssen et al., 2008; Grossmann et al., 2009).
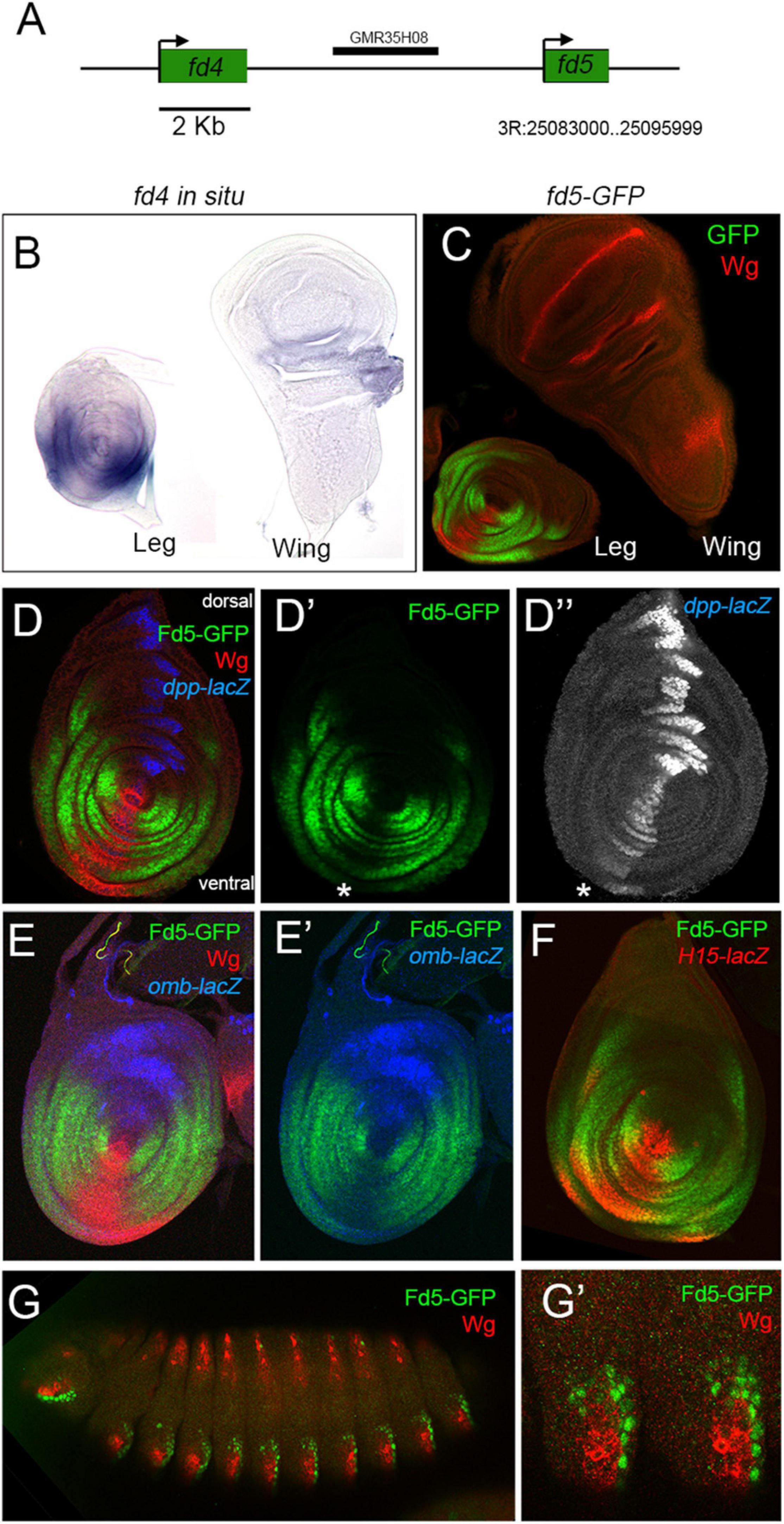
Figure 1. fd4 and fd5 expression during development. (A) Schematic representation of the fd4 and fd5 genomic locus. (B) fd4 expression pattern by in situ hybridization. (C) fd5 expression visualized by a fd5-GFP tagged (green) line. Wg (red) staining is also shown. (D) Expression pattern of fd5 (green), dpp-lacZ (blue), and wg (red) in the leg disc. Separate channels for fd5 (D′) and dpp (D″) are shown. The asterisk indicates the ventral most region of the disc where low Fd5-GFP levels are detected. (E) Leg imaginal disc stained for Fd5-GFP (green), omb-lacZ (blue), and Wg (red). Separate channels for Fd5-GFP (green) and omb-lacZ (blue) are shown in panel (E′). (F) Leg imaginal disc stained for Fd5-GFP (green) and H15-lacZ (red). (G) Stage 14 embryo stained for Fd5-GFP (green) and Wg (red). (G′) A close up view of the ventro-lateral domain of two abdominal segments. In all confocal images, dorsal is up and anterior is left.
In this study we address the role of the sister genes fd4 and fd5 (also known as fd96Ca and fd696cb), members of the forkhead family of transcription factors, during appendage development (Hacker et al., 1992). We found that fd4/fd5 are expressed exclusively in the ventral imaginal discs and more specifically in the ventro-lateral domain of the leg disc. We identified a minimal CRM that directs the expression of these genes and characterized its regulation in the leg disc. Furthermore, using specific mutations generated for each gene we found that fd4/fd5 play redundant roles during the formation of the sex comb, a characteristic ventro-lateral structure of the prothoracic male leg. Our results highlight the function of the fd4/fd5 genes during leg development.
Results
fd4 and fd5 Expression During Leg Development
To identify genes with a potential role in DV patterning we searched the Flylight database for non-coding DNA elements that have a restricted DV activity pattern in the leg disc (Jory et al., 2012). We identified a DNA fragment that activates the reporter gene in the ventro-lateral domain of the leg disc (named GMR35H08 at Flylight database). This fragment is located between the fd4 and fd5 genes, two members of the forkhead family of transcription factors (Hacker et al., 1992; Lee and Frasch, 2004; Figure 1A). The Fd4 and Fd5 proteins share a 49% of aa sequence identity, suggesting they could play similar functions. In order to investigate the expression pattern of these genes we used an in situ hybridization probe for fd4 and GFP-tagged versions for fd4 and fd5. Both genes are identically expressed in the three leg imaginal discs, the antenna and the genital imaginal discs and show no expression in the wing or haltere discs (Figures 1B,C and Supplementary Figure 1; Heingard et al., 2019). When compared to the dorsal and ventral determinants, dpp and wg, fd4 and fd5 expression is restricted to the ventro-lateral domain of the leg disc with faint expression in the ventral most region that coincides with the highest levels of Wg and low Dpp (Figure 1D and Supplementary Figure 1; Heingard et al., 2019). Comparison of fd5 expression with that of the Dpp and Wg targets, omb and H15, confirmed that fd5 expression is complementary to omb and extends more laterally than H15 (Estella and Mann, 2008; Brook, 2010; Ruiz-Losada et al., 2018; Figures 1E,F). As previously reported, these genes are also expressed in the embryo (Archbold et al., 2014), and at least for fd5, its expression is restricted to ectodermal segmental ventral stripes surrounding wg expression (Figure 1G).
Fd4 and Fd5 Act Redundantly in the Formation of the Sex Comb Structure
Next, we investigated the role of fd4 and fd5 during leg development. To this end, we generated specific mutant alleles for each gene and a double fd4/fd5 mutant using CRISPR/Cas9 (Supplementary Figure 2) (see details in Materials and Methods). The fd45nt mutant allele has a five nucleotide (nt) deletion at the beginning of the coding region that changes the open reading frame. fd45nt homozygous mutant flies are viable and have normal patterned legs with the exception of a slight reduction in the number of sex comb bristles (∼10.6 in the control vs. ∼8.7 in fd45nt mutants) (Figures 2A,E). The sex comb is a male specific structure present on the prothoracic leg (T1 leg) that develops from modified bristles of the most distal transverse row (TRs) on the first tarsal segment that rotate approximately 90° (Tokunaga, 1962; Tanaka et al., 2009; Kopp, 2011). The fd51nt mutant has a reading frame shift due to a single nt deletion at the start of the gene that completely changes the amino acid sequence (Supplementary Figure 2). No defects were observed in the legs of fd51nt mutant animals (Figures 2A,E). As both genes have identical expression patterns, and likely similar functions, we generated a double fd4, fd5 mutant chromosome by mutating the fd5 gene over the fd45nt allele (Supplementary Figure 2). The new fd5stop mutant has a nine nt sequence change and a three nt deletion that generate a premature stop codon at the beginning of the coding region (Supplementary Figure 2). fd45nt, fd5stop homozygous mutant animals reach adulthood, though they get caught in the food where they die soon afterwards.
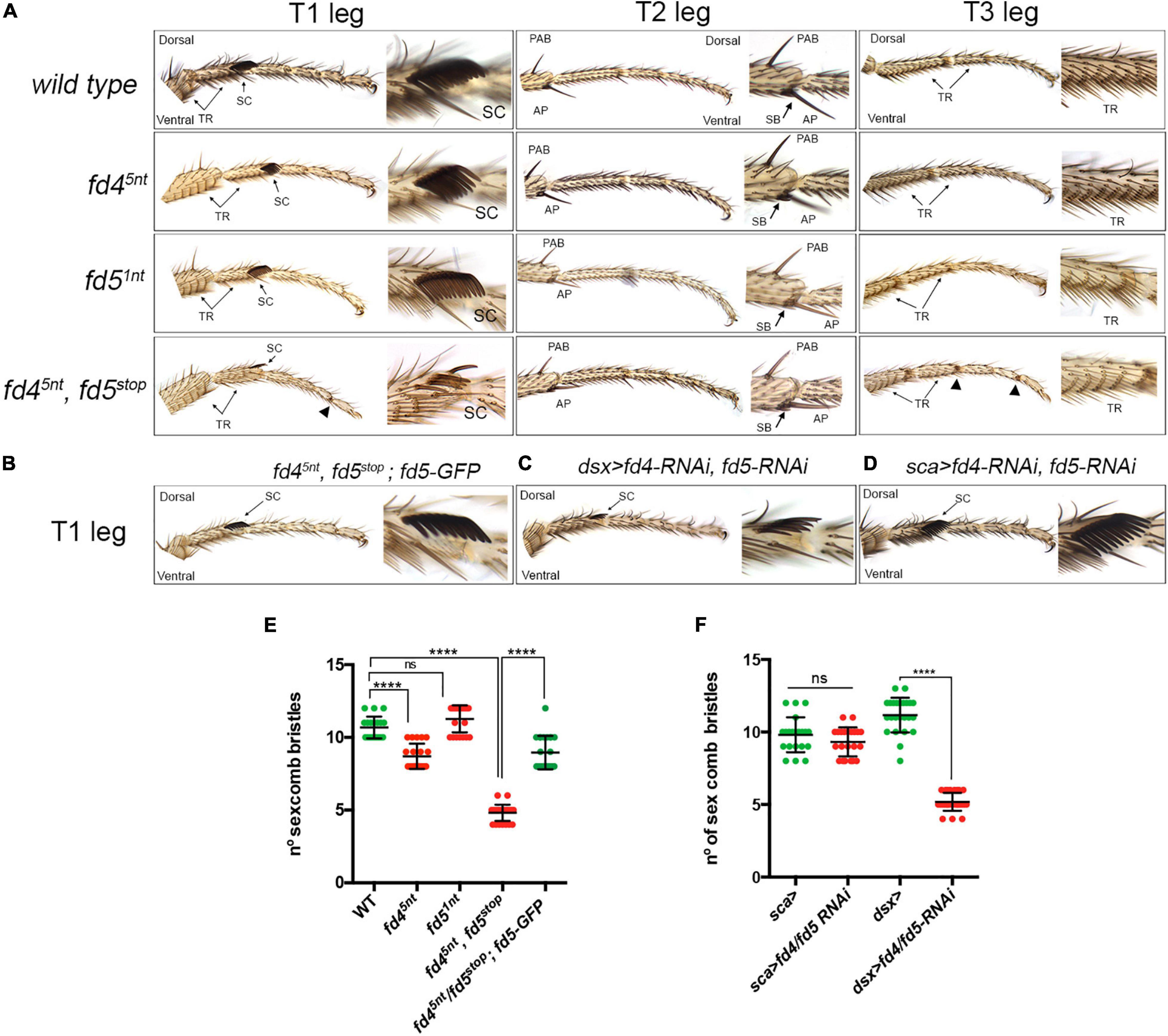
Figure 2. Phenotypic analysis of fd4 and fd5 mutants. (A) Male adult leg phenotypes of a wild type, fd45nt, fd51nt and the double fd45nt, fd5stop mutants. Representative examples of the three legs (T1–T3) are shown. Close up views of the sex comb in T1, the distal tibia in T2, and the first tarsus in T3 are shown. TR, transverse row; SC, sex comb; PAB, pre-apical bristle; AP, apical bristle and SB, spur bristles. Arrowheads indicate defective joints. Characteristic ventro-lateral structures are the transverse rows (TRs), the sex combs (SCs), and the apical bristles (ABs). (B–D) Male first thoracic legs of the genotypes indicated. Note that the sex comb phenotype of the double fd45nt, fd5stop mutant is largely rescued by one copy of the fd5-GFP transgene (B). Knockdown of Fd4 and Fd5 levels with the dsx-Gal4 (C), but not with the sca-Gal4 (D), reduced the number of sex comb bristles. (E,F) Quantification of sex comb bristles in the genotypes indicated and presented in panels (A,B). n > 19 sex combs per genotype were counted. Error bars indicate standard deviation (SD). Statistically significant differences based on Student’s t test are indicated: ****P < 0.0001 and not significant (ns).
A detailed leg phenotypic analysis of the different DV landmarks present in the three legs from fd45nt, fd5stop double mutant animals revealed defects in the formation of the sex comb and some necrotic tissue in few joints (Hannah-Alava, 1958). We also found that the pattern of transverse row bristles is slightly altered in these mutants (Figure 2A and Supplementary Figure 3). The number of sex comb bristles is strongly reduced in the double mutant fd45nt, fd5stop when compared to each single mutant or the control (∼10.6 in the control vs. ∼4.8 in fd45nt, fd5stop mutants), suggesting a redundant role of these genes in the formation of this male specific structure (Figures 2A,E). The orientation of the remaining sex comb teeth is longitudinal as in the control, suggesting that the 90° rotation of precursor distal transverse row bristles have occurred properly in the mutants. In females, we detected approximately the same number of ta1 transverse rows in the control and in the double fd45nt, fd5stop mutant (Supplementary Figure 3). Importantly, the number of sex combs bristles of fd45nt, fd5stop mutant animals was almost completely rescued when a wild type copy of the fd5 gene (BAC-fd5-GFP) was provided in the mutant background (∼4.8 in fd45nt, fd5stop mutants vs. ∼9 in the fd45nt, fd5stop; fd5-GFP rescue), confirming that these phenotypes are specific of the fd4, fd5 mutations (Figures 2B,E).
In addition, we used specific RNAi lines for each gene that efficiently reduced Fd4 and Fd5 protein levels (Supplementary Figure 4). When these RNAi lines were expressed in the distal domain of the leg with the Dll-Gal4 driver (Dll>) we obtained identical phenotypes that with the mutants (Supplementary Figure 4). These results corroborate the redundant roles of the fd4 and fd5 genes in the formation of the sex comb.
Next, we investigated whether the requirement of the fd4/fd5 genes is restricted to the bristles precursors that will form the sex comb teeth or to the epidermal cells of the leg imaginal disc. To this end, we used the scabrous (sca)-Gal4 line to knock down simultaneously Fd4 and Fd5 levels in nascent bristle cells (Shroff et al., 2007). In addition, both RNAi lines were simultaneously expressed with the doublesex (dsx)-Gal4 that is expressed both in the epidermis and sensory organ precursors (SOPs) of the sex comb (Robinett et al., 2010). While no defects were observed in sca>fd4-RNAi, fd5-RNAi animals, a strong reduction in the number of sex comb bristles was found in dsx>fd4-RNAi, fd5-RNAi flies (∼11 in dsx> control animals vs. ∼5.2 in dsx>fd4/fd5-RNAi mutant animals) (Figures 2C,D,F). These results suggest that Fd4 and Fd5 function is not restricted to late sex comb SOPs but instead required in leg epithelium cells including those that will be re-specified as SOPs.
In order to study the function of the fd4/fd5 genes in gain of function experiments we have generated specific UAS lines for each gene and ectopically expressed them in the dorsal region (dpp-gal4) or in the entire distal domain (Dll-Gal4) of the leg imaginal disc (Figure 3). Importantly, the ectopic expression of fd4 or fd5 did not increase the number of sex comb bristles in any of these conditions (Figures 3A,B,D). Nevertheless, we observed the appearance of an extra dorsal pre-apical bristle in 50% of T2 legs and a distal truncation that deletes the claw in the Dll>fd5 genotype (Figure 3B). When both genes were expressed together with the Dll-Gal4 line, a more severe distal truncation phenotype was detected, however the number on sex comb bristles remained close to the control (∼11.4 in Dll> vs. ∼10 in Dll>fd4/fd5 animals) (Figures 3C,D).
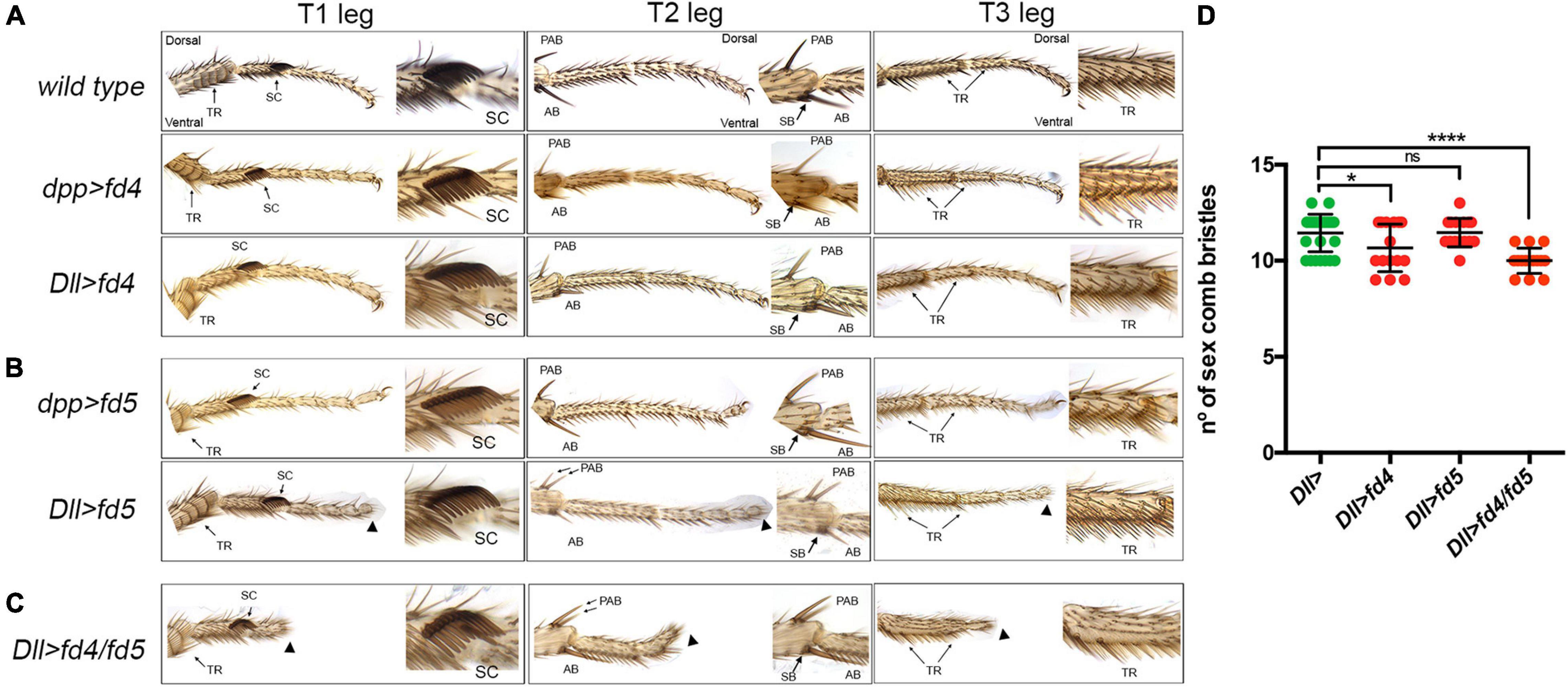
Figure 3. Phenotypic analysis of fd4 and fd5 gain of function. (A,B) Male adult legs phenotypes of wild type control animals and of flies misexpressing fd4 (A) or the fd5 (B) in the distal (Dll-Gal4) or dorsal (dpp-Gal4) domain of the legs. Representative examples of the three legs (T1–T3) are presented. Close up views of the sex comb in T1, the distal tibia in T2, and the first tarsus in T3 are shown. Arrowheads indicate distal truncations. Arrows indicate the presence of duplicated PABs. (C) Male adult legs of flies misexpressing fd4 and fd5 under the Dll-Gal4 driver. Note the severe truncation of the distal leg (arrowheads) and the presence of two PABs. (D) Quantification of sex comb bristles in the genotypes indicated and presented in panels (A–C). n = 15 sex combs per genotype were counted. Error bars indicate standard deviation (SD). Statistically significant differences based on Student’s t test are indicated: *P < 0.05, ****P < 0.0001, and not significant (ns).
In summary, all these results suggest that fd4/fd5 act redundantly in the formation of the sex comb, however these genes are not sufficient to generate ectopic sex comb teeth when ectopically expressed.
Analysis of fd4/fd5 Role in the Sex Comb Regulatory Network
The formation of the sex comb is directed by a gene regulatory network that precisely localizes this structure in the anterior ventro-lateral domain of the first tarsal segment of prothoracic male legs (Kopp, 2011). PD patterning genes such as Dll, dachsound (dac), and bric à brac (bab), in combination with H15/mid, wg, and en regulate the prominent expression of the Hox gene Sex comb reduced (Scr) in the tibia (ti) and first tarsal segment (ta1) of the prothoracic leg (Tokunaga, 1961; Struhl, 1982; Couderc et al., 2002; Svendsen et al., 2009; Eksi et al., 2018; Figures 4A,I). Scr, together with PD transcription factors, regulate doublesex (dsx) expression in two anterior distal crescents (ta1 and ta2). Dsx is a sex-specific transcription factor that exists in two isoforms. The male isoform promotes male-specific structures while the female isoform dictates the corresponding female ones (Burtis and Baker, 1989). Once activated, Dsx modulates the sexual dimorphic male-specific expression of scr in ta1 segment (Kopp, 2011; Tanaka et al., 2011; Figures 4B,I).
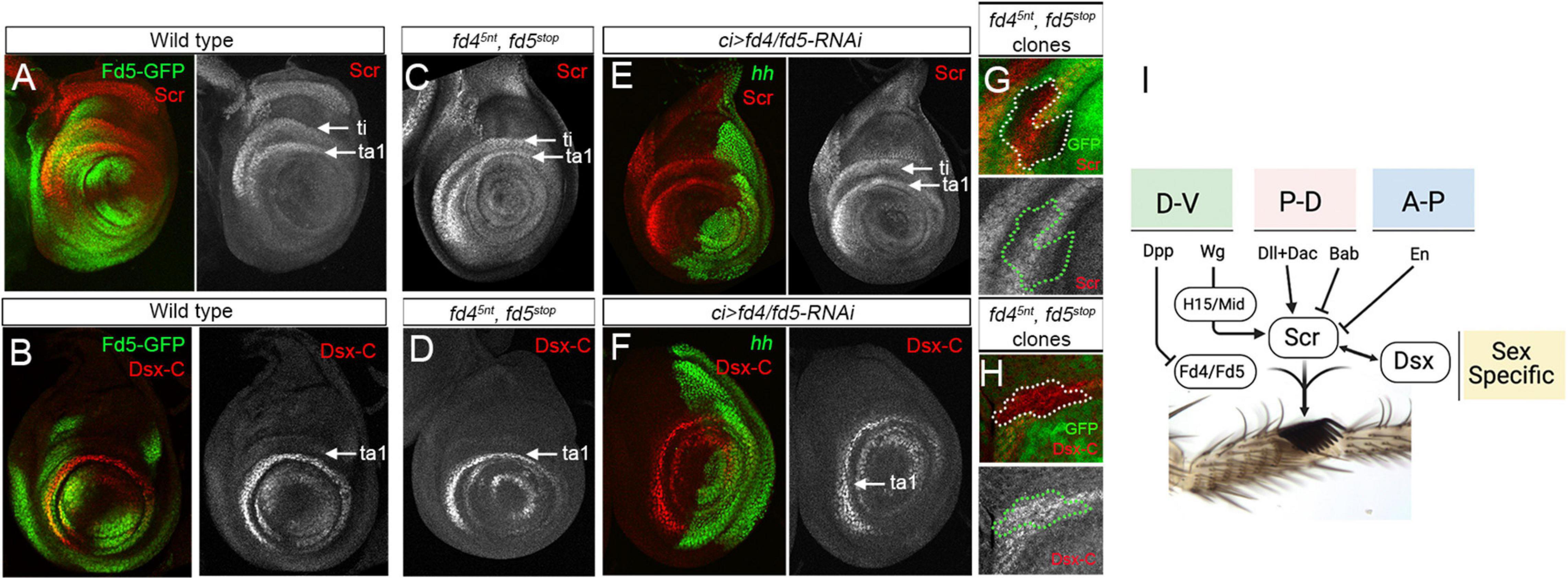
Figure 4. Fd4/Fd5 are not requiered for Scr and dsx expression. (A,B) Expression of fd5-GFP (green) and Scr (red, A) or dsx-C (red, B) in a male third instar prothoracic leg imaginal disc. Single channels for Scr and Dsx-C stainings are shown. White arrows point to the tibia and first tarsal segment. (C,D) Scr (C) and Dsx-C (D) antibody stainings in male prothoracic leg imaginal discs of fd45nt, fd5stop mutant animals. (E,F) Male prothoracic leg imaginal discs stained for Hh (green) and Scr (red, E) and Dsx-C (red, F). The knockdown of Fd4/Fd5 levels in the anterior compartment by the expression of the fd4 and fd5 RNAi lines under the ci-Gal4 driver does not affect Scr or Dsx-C. Single channels for Scr and Dsx-C staining are shown. (G,H) fd45nt, fd5stop mitotic mutant clones visualized by the absence of GFP (green) and by a dotted white and green line. Scr (G) and Dsx-C (H) levels (red) are not affected in fd45nt, fd5stop mutant cells. Single channels for Scr and Dsx-C staining are shown. (I) The sex comb integrate multiple regulatory inputs form the three axes (D-V, P-D, and A-P) and sex-specific cues to develop in a precise location in the first tarsal segment of the male prothoracic leg. A simplified version of the gene regulatory network that directs the formation of the sex comb is depicted. In all confocal images, dorsal is up and anterior is left.
Initially, we analyzed the expression of wg and its target genes H15 and mid in fd4/fd5 loss of function conditions. No changes were observed in the expression of any of these genes (Supplementary Figure 5). Next, as both Scr and Dsx direct the morphogenesis of the sex comb structure, we decided to study the functional relationship between these genes and fd4/fd5. To monitor dsx expression we used an antibody against the common domain shared by the male and female Dsx protein isoforms (Sanders and Arbeitman, 2008). First, we compared the expression of fd5-GFP with Scr and Dsx and confirmed that both genes overlap with Fd5 in the ventro-lateral domain of the presumptive ta1 in male prothoracic imaginal disc, the region that will form the transverse row bristles and the sex comb (Figures 4A,B). Second, we analyzed Scr and dsx expression in leg discs from fd45nt, fd5stop double mutant animals. No visible changes were observed in the expression of these genes in mutants as compared to controls, or when we knockdown Fd4/Fd5 levels in the anterior compartment using the RNAi lines (Figures 4C–F). To confirm that the fd4/fd5 genes are not modulating Scr or Dsx levels, we generated mosaic mitotic fd45nt, fd5stop mutant clones and monitored Scr and Dsx levels in mutant and wild type adjacent cells of the same leg imaginal disc (Figures 4G,H). We did not detect any change on Scr and Dsx levels in fd45nt, fd5stop mutant clones. As fd4 and fd5 expression is neither sexually dimorphic nor restricted to the prothoracic legs, it is very unlikely that these genes are downstream of Scr and Dsx regulation.
These results indicate that the fd4/fd5 genes may work in parallel with Scr and Dsx in the regulatory network that controls the formation of the sex comb (Figure 4I).
Identification of the Leg Disc fd4/fd5 Minimal Cis-Regulatory Module
To identify the CRMs that direct fd4/fd5 expression in the leg imaginal disc, we searched for differentially open chromatin regions between the leg and the wing disc in the fd4/fd5 genomic locus (McKay and Lieb, 2013). Two regions, A and C, show a clear open chromatin state in the leg as opposed to the wing disc (Figure 5A). Both regions and an additional sequence (B fragment) that has been previously shown to reproduce fd4/fd5 expression in the embryo were cloned in a GFP or lacZ reporter vector (Figures 5A,B; Archbold et al., 2014). Of these three elements, only fragment C, which is contained within the GMR35H08 element, is active in the leg disc in a similar pattern as fd4/fd5 expression (Figure 5B). However, unlike the fd4/fd5 genes, this element is also active in the wing disc (Supplementary Figure 6). We further subdivided the C element in two non-overlapping halves (C1 and C2) and a fragment that encompass the peak of open chromatin in the leg (C3) (Figure 5A). As both C2 and C3 elements reproduce fd4/fd5 expression, we tested the activity of the 200 bp overlapping region (Cs) in the leg disc. No reporter activity was observed for the Cs fragment, suggesting that the C3 element contains the minimal information to drive fd4/fd5 expression in the leg disc (Figure 5B).
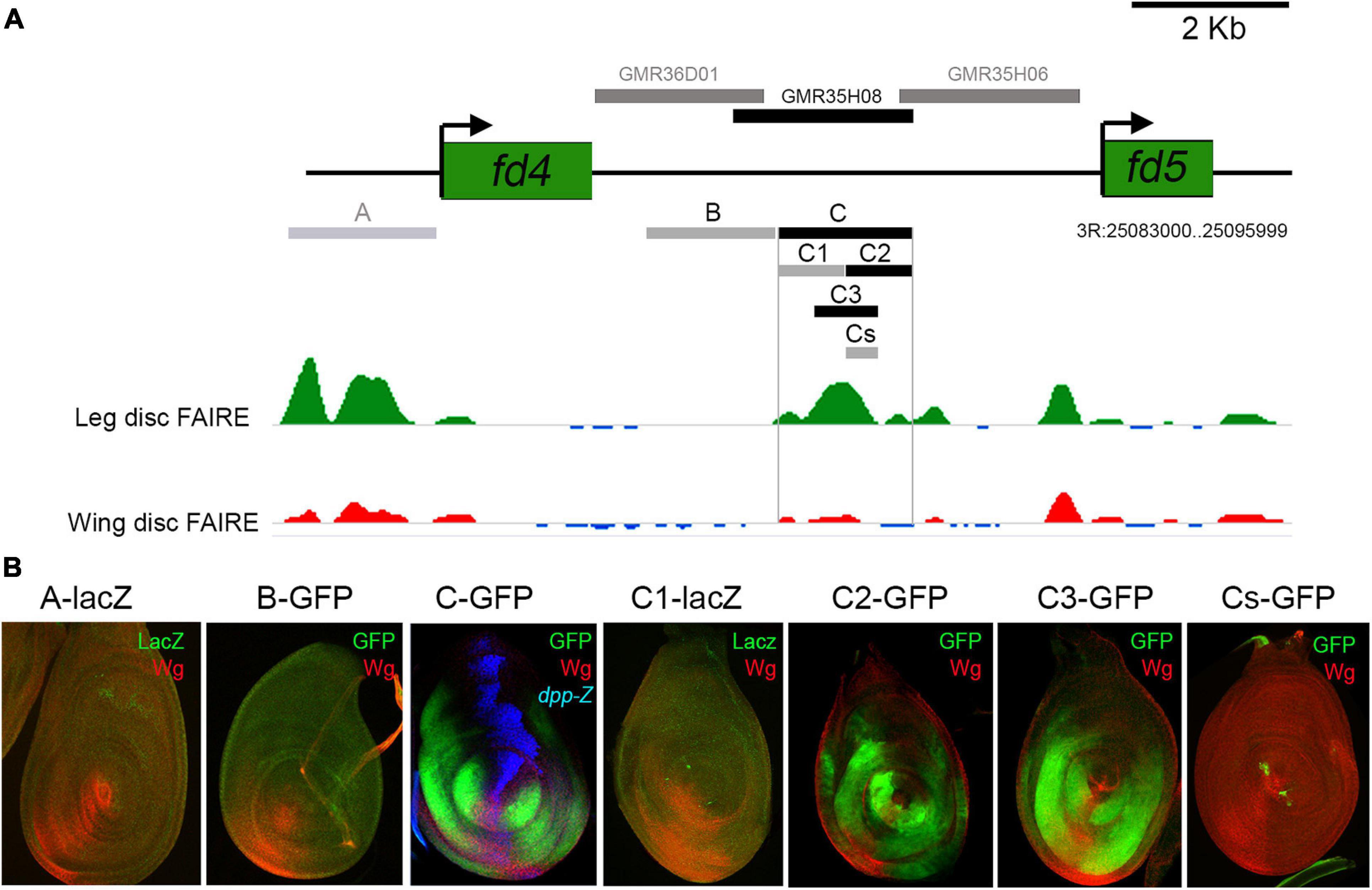
Figure 5. Identification of the fd4/fd5 CRM. (A) Schematic representation of the fd4 and fd5 genomic locus in which open chromatin regions, identified by FAIRE seq for leg and wing imaginal discs are indicated by green and red peaks, respectively. Data obtained from McKay and Lieb (2013). In the upper part of the panel, horizontal bars represent the DNA elements for which Gal4 drivers were generated by the Janelia Farm consortium (gray bars). Only the GMR35H08 line (black bar) reproduced fd4/fd5 expression in the leg disc. Below the genomic locus are drawn the different DNA elements cloned in this work into a reporter GFP or lacZ construct. Only the C fragment and the C2 and C3 subfragments faithfully reproduced the expression of fd4/fd5 in the leg imaginal disc. Note that the A and C fragments were selected because of the different chromatin accessibility profiles between the leg and wing imaginal discs. Gray bars indicate no activity and black bars indicate activity in leg imaginal discs. (B) Leg imaginal disc activity of the different fragments cloned in this work and shown in panel (A). All elements were cloned in a GFP or lacZ reporter vector and inserted in the same attP site. GFP (green), Wg (red), and dpp-lacz (blue).
Regulation of fd4/fd5 Expression in the Leg Imaginal Disc
As the expression of the fd4/fd5 genes is restricted to the ventro-lateral domain of the leg disc, we investigated the role of the Dpp and Wg pathways in their regulation. We used the fd5-GFP line or the fd4/fd5 C-CRM as readouts for fd4/fd5 expression depending on the experimental setup. To test the idea that the fd4/fd5 genes integrate the Wg and Dpp inputs, we generated clones expressing a constitutive activated form of the β-catenin homolog Armadillo (Arm∗) to activate the Wg pathway, or an activated form of the Dpp receptor Thickveins (TkvQD), respectively (Figures 6A–C). Most of dorsally located arm∗ expressing clones show a cell autonomous upregulation of fd5-GFP and C-GFP CRM expression (Figures 6A,B). However, arm∗ clones close to the dpp domain failed to induce the expression of these reporters (Figures 6A,B). In contrast, activation of the Dpp pathway by tkvQD expressing clones strongly repressed fd5-GFP expression (Figure 6C). These results suggest that the Wg pathway activates, while the Dpp pathway represses fd4/fd5 expression. To test for the requirement of these pathways in the regulation of the fd4/fd5 genes, we generated mitotic loss of function clones for the transcriptional effectors of the Dpp pathway, Mad and for the Wg co-receptor Arrow (Arr). C-GFP was strongly upregulated in all dorsally located mad12 clones, while ventral arr2 mutant clones exhibit different behaviors of C-CRM activity depending on their localization (Figures 6D–F). For example, ventral anterior clones close to the AP compartment border downregulate C-lacZ activity while ventral posterior clones have no effect on it (Figures 6E,F, respectively). The regulation of fd4/fd5 expression by the Dpp and Wg pathways is not mediated by their target genes omb and H15/mid, as mutant clones for these genes have no effect on fd5-GFP expression or C activity (Supplementary Figure 6).
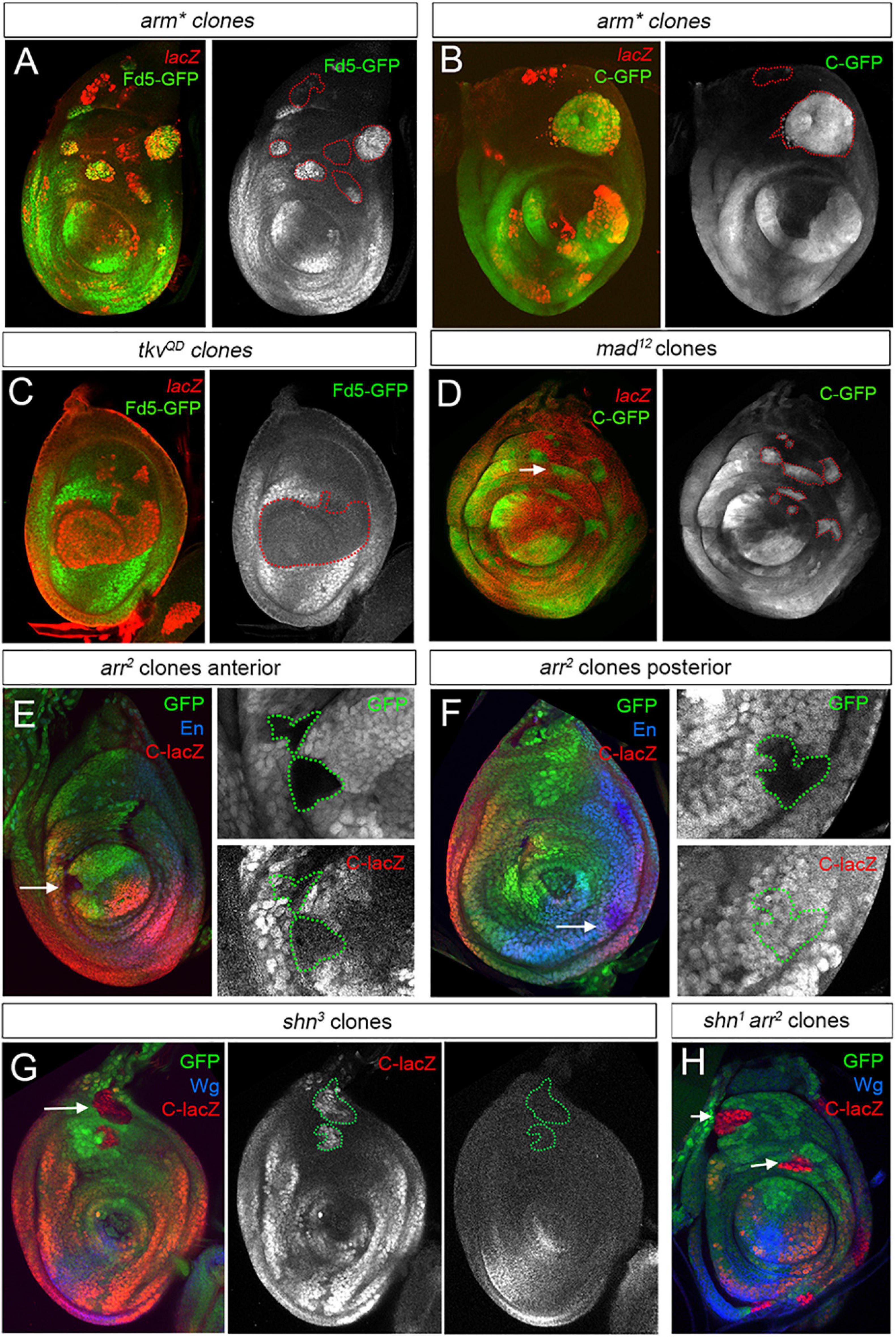
Figure 6. Wg and Dpp contribution to fd4/fd5 regulation in the leg disc. (A,B) Clones expressing arm* marked by lacZ (red) activates fd5-GFP (green, A) or C-GFP (green, B) expression in dorso-lateral regions of the disc. Note that dorsal-most clones failed to activate fd5 or C activity. Single channels for Fd5-GFP and C-GFP are shown separately and arm* clones are outlined with red dots. (C) Clones expressing tkvQD marked by lacZ (red) repress fd5-GFP (green) expression. Single channel for Fd5-GFP is shown and tkvQD clones are outlined with red dots. (D) mad12 clones marked by absence of lacZ (red), show C-GFP (green) derepression in the dorso-lateral domain of the leg (white arrow). Single channel for C-GFP is shown and clones are outlined with red dots. (E,F) arr2 clones marked by absence of GFP stained for C-lacZ (red) and En (blue) to mark the posterior compartment. In panel (E), an anterior arr2 mutant clones in the ventro-lateral domain of the leg show downregulation of C-lacZ (arrow). In panel (F), the same clones located in the posterior compartment have no effect on C-lacZ activity (arrow). Single channels for GFP and C-lacZ are shown and clones are outlined with green dots. (G) shn3 mutant clones marked by the absence of GFP (green) derepress C-lacZ activity (red) in the dorsal domain of the leg disc (arrow). Note that in these clones, wg expression (blue) is not activated. Single channels for C-lacZ and Wg are shown and clones are outlined with green dots. (H) shn1, arr2 double mutant clones marked by the absence of GFP (green) derepress C-lacZ activity (red) in the dorsal domain of the leg disc (arrows). Wg staining is in blue. All clones were generated 48–72 h AEL.
Decapentaplegic and Wg transcriptionally repress each other in the leg disc, and therefore the downregulation of one pathway allows the activation by Hh of the other in anterior cells (Brook and Cohen, 1996; Jiang and Struhl, 1996; Johnston and Schubiger, 1996; Morimura et al., 1996; Penton and Hoffmann, 1996; Theisen et al., 1996). Our arr2 mutant clone analysis points to an indirect regulation of fd4/fd5 expression through the derepression of the Dpp pathway instead of a direct requirement of the Wg pathway for its expression. To further test this possibility, we first generated mutant clones for Schnurri (Shn), a transcriptional repressor downstream of Dpp activity. Shn is a zinc finger protein that together with Mad/Med forms a complex that regulates Dpp target genes such as brinker (brk) (Arora et al., 1995; Grieder et al., 1995; Marty et al., 2000). Dorsally located shn3 mutant clones activated the expression of the C-lacZ reporter cell autonomously and, importantly, they do so without derepression of wg (Figure 6G). Next, we induced shn1 mutant clones that are also mutant for arr, and therefore cannot transduce the Wg pathway. Consistently with our previous results, these shn1 arr2 mutant clones still derepressed C-lacZ activity in dorsal leg regions (Figure 6H).
Taken together, these results demonstrate that fd4/fd5 expression is repressed dorsally by the Dpp effectors Shn and Mad and is activated independently of Wg.
fd4/fd5 are expressed in ventral (legs and antenna) but not dorsal (wing and haltere) imaginal discs, suggesting that their expression could be regulated by a positive input from a ventral selector gene. The sister genes, buttonhead (btd) and Sp1 could fulfill this role: in one hand, forced expression of btd or Sp1 in the wing disc induces ectopic leg development and in the other, removing btd and Sp1 completely abolish leg formation (Estella et al., 2003; Estella and Mann, 2010). To test this idea, we analyzed fd5-GFP expression in wing discs where btd is ectopically induced (Figures 7A–C). Misexpression of btd in the wing imaginal disc with the dpp-Gal4 line activates fd5-GFP in the wing pouch but not in the notum (Figures 7A–C). In contrast, clones for a deficiency that deletes the btd and Sp1 genes (Df(btd,Sp1)) in the leg disc has no effect on fd5-GFP expression, even when these clones lose the ability to activate Dll (Figure 7D). These results suggest that although btd is sufficient to ectopically induce fd5-GFP expression in the wing disc, btd and Sp1 are not necessary for endogenous fd5 expression in the leg disc.
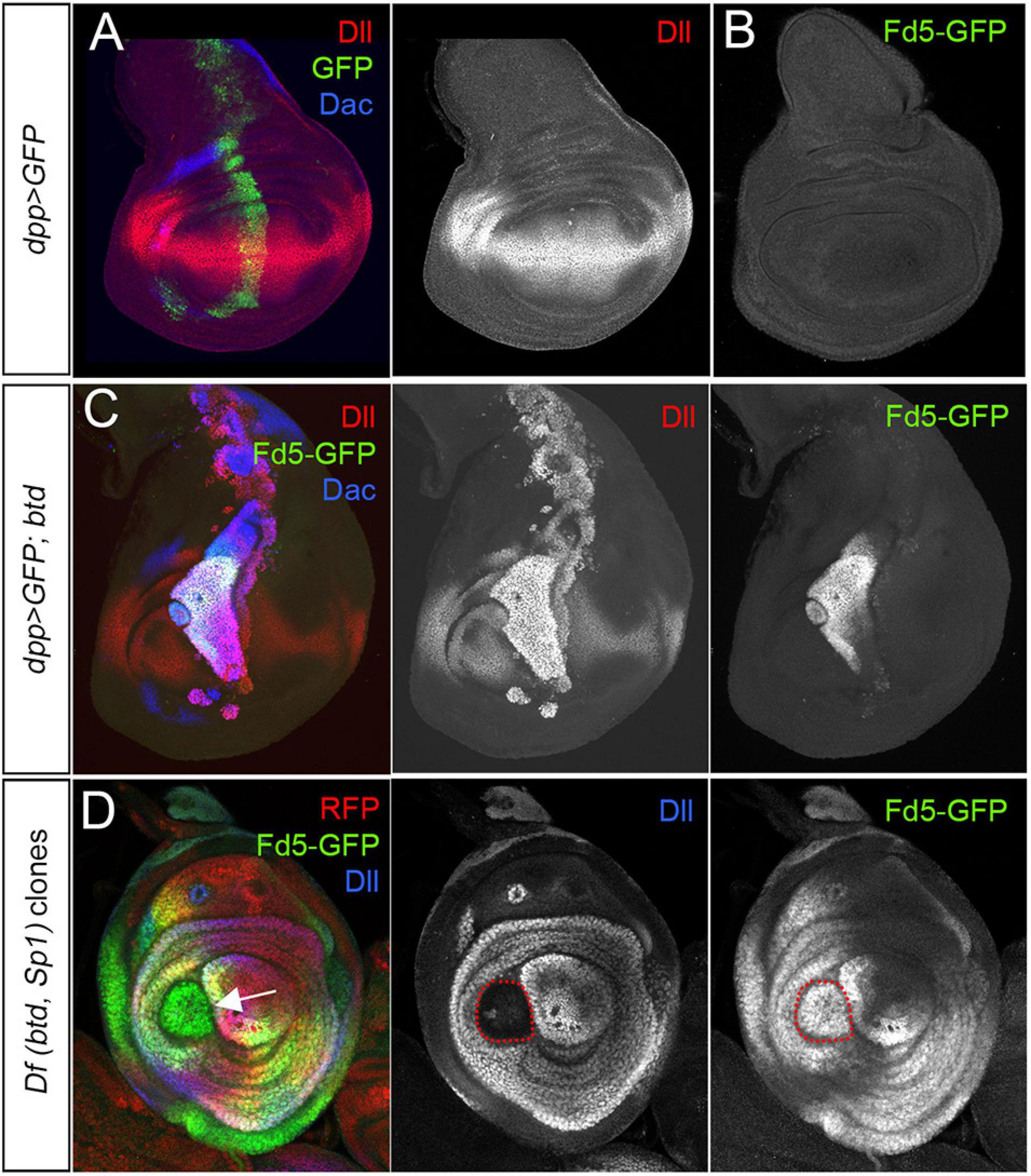
Figure 7. fd5 regulation by btd and Sp1. (A) Control wing imaginal disc expressing GFP (green) under the dpp-Gal4 driver and stained for Dll (red) and Dac (blue). Separate channel for Dll is shown. (B) Wing imaginal disc from a fd5-GFP line. Note the absence of fd5 expression (green) in the wing disc. (C) The expression of btd under the control of the dpp-Gal4 line ectopically activates fd5-GFP (green) expression in the wing disc. Dll (red) and Dac (blue). Separate channels for Dll and Fd5-GFP are shown. (D) Df(btd, Sp1) mutant clones generated 48–72 h before dissection marked by the absence of RFP (red and arrow) and stained for Fd5-GFP (green) and Dll (blue). Single channels for Dll and Fd5-GFP are shown. Clones are outlined with red dots.
Discussion
In this work we studied the expression and function of the forkhead family members fd4 and fd5 during leg development in Drosophila. We found that these genes play redundant roles during sex comb formation.
Subdivision of the DV territories is regulated in a different manner between wing and leg appendages. For example, in the wing imaginal disc the expression of the selector gene apterous (ap) is activated in response to the epidermal growth factor receptor (EGFR) pathway and it is required for the specification of dorsal cell fates (Diaz-Benjumea and Cohen, 1993; Blair et al., 1994; Wang et al., 2000; Bieli et al., 2015). In the leg imaginal disc, DV subdivision is controlled by Dpp and Wg signaling pathways that direct dorsal and ventral fates, respectively (Brook and Cohen, 1996; Jiang and Struhl, 1996; Johnston and Schubiger, 1996; Morimura et al., 1996; Penton and Hoffmann, 1996; Theisen et al., 1996). As Dpp and Wg form gradients, an interesting problem is to understand how cells in the leg disc, which are exposed to different levels of these morphogens are able to integrate this information and assume dorsal, ventral, and lateral fates. This is of special interest as the leg is a circular appendage with no clear morphological DV distinction and no lineage restriction as opposed to the wing. Moreover, in contrast to the PD axis, we have very limited information of the downstream Wg and Dpp targets that controls DV patterning in the leg.
In a search for genes with DV expression patterns in the leg, we identified the forkhead family members fd4 and fd5 to be restricted to the ventro-lateral domain of the ventral imaginal discs. In contrast to other known DV leg patterning genes, the fd4/fd5 expression is extended more laterally than the Wg target genes H15/mid. These genes are not activated in the dorsal domain of the leg, where high levels of Dpp are present. In addition, we identified a minimal CRM that faithfully recapitulates fd4/fd5 expression in the leg imaginal disc. Detailed analysis of the C element regulation reveals that this CRM is activated by an unknown factor and repressed by the Dpp pathway, more specifically by the transcriptional repressor Shn. Interestingly, we found that the C element is similarly expressed as brk, another Shn target gene (Marty et al., 2000; Estella and Mann, 2008). However, Brk is not required for C element activity in the leg as the C-GFP CRM is normally expressed in brk mutant clones (Supplementary Figure 6). No consensus binding site for the Mad/Med/Shn complex was found in the C-CRM sequence, suggesting that either this complex is binding a non-consensus site or that fd4/fd5 regulation by Shn is indirect (Pyrowolakis et al., 2004). Importantly, we found that the Wg pathway is indirectly required for C element activity through the repression of Dpp expression.
Unlike the endogenous expression of the fd4/fd5 genes, the C element is not restricted to the ventral imaginal discs (legs and antenna), as activity of this CRM is also observed in the wing disc. This result suggests that sequences outside the C and GMR35H08 elements restrict fd4/fd5 expression to the ventral imaginal discs. We studied the potential role of the ventral selector genes Sp1 and Btd as regulators of fd4/fd5 ventral specific expression. However, btd/Sp1 loss of function clones still display fd5 expression in the leg. It is possible that wing disc specific genes are required for repressing fd4/fd5 expression in dorsal imaginal discs and restricting its activity to ventral ones.
According to their identical expression and sequence homology, we found that these genes play redundant roles during leg development. Using newly generated alleles for each gene and a double fd4/fd5 mutant, we describe a redundant role for these genes in sex comb formation. In contrast to their wide lateral expression in all three legs, we only found defects on the development of this specific male structure of the first thoracic leg. In males, the distal most transverse row of the first tarsal segment is transformed into a sex comb. We found that in fd4/fd5 double mutants the number of sex comb bristles is strongly reduced but not eliminated, suggesting that these genes contribute to sex comb development but are not completely required. As the sex comb is formed from cells with ventral and lateral fates (Held et al., 1994), the remaining sex comb bristles observed in the fd4/fd5 mutants would only be formed from cells with ventral identity.
The sex comb gene regulatory network integrates information from the three axes (AP, DV, and PD) and sex- and segment-specific cues by the dsx and Scr genes, respectively (Kopp, 2011). No defects on the expression of H15/mid, Scr, or dsx were observed when fd4/fd5 levels were knock down, suggesting that the fd4/fd5 act in parallel to these genes in the regulatory network that controls sex comb formation. The sex comb is a great model to study how the precise combination of positional and sex specific patterning cues promote the formation of morphological structures. Understanding the genetics of sex comb development could help understand the origin and diversification of this recently evolved structure.
A recent study has analyzed the expression and function of the forkhead transcription factor FoxB in the common house spider Parasteatoda tepidariorum (Heingard et al., 2019). This gene is the ortholog of fd4/fd5 and the only family member in the spider. Similar to Drosophila fd4/fd5, pt-FoxB expression is restricted to the ventral domain of developing appendages and it is required for DV patterning. In pt-Foxb-RNAi animals the expression of the ventral determinants wg and H15 is almost lost while the corresponding ventral expansion for the dorsal determinants, dpp and omb, is described. It is remarkable the different mutant phenotypes observed in the spider and the fly after the knockdown of these genes. One possibility that could explain these differences is that the double fd45nt, fd5stop mutant chromosome generated in our study is not a true null for fd5 but a strong hypomorph due to stop codon readthrough mechanisms (Palma and Lejeune, 2021). Either way, the leg phenotypes described here using the double fd45nt, fd5stop mutant and the RNAi lines are almost identical. Also, in the Parasteatoda study the authors used RNAi techniques to downregulate the function of the Foxb gene. Another possibility is that the function and requirement of the FoxB forkhead transcription factors have been modified during the evolution of arthropods. Thanks to the development of new technologies such as CRISPR/Cas9 the function of the FoxB forkhead transcription factors could be easily investigated in other arthropods to study how the DV gene regulatory network has been modified in related species.
Materials and Methods
Drosophila Strains
fd4-GFP (P{fd96Ca-GFP.FPTB}attP40), fd5-GFP (P{fd96Cb-GFP.FPTB}attP40), dpp10368-lacZ (p10368), omb-lacZ, H15-lacZ, mid-lacZ, dpp-Gal4, UAS-GFP, Dll212-Gal4, dsx-Gal4, sca-Gal4, UAS-fd4-RNAi, UAS-fd5-RNAi and hh-dsred, Ci-Gal4 are described in FlyBase.
Loss-of-function clonal analysis the following genotypes were used:
yw122; FRT82B fd45nt, fd5stop/yw122; FRT82B-ubi-GFP.
yw122; FRT42D arr2/w122; FRT42D ubi-GFP; C-lacZ
yw122; Mad1–2 FRT40A/arm-lacZ FRT40A; C-GFP
yw122; FRT42D shn3; C-lacZ/yw122; FRT42D ubi-GFP.
yw122; FRT42D arr2 shn1/yw122; FRT42D ubi-GFP; C-lacZ
Df(btd,Sp1) FRT19A/yw122 ubi-RFP FRT19A, fd5-GFP
omb282 FRT19A/yw122 ubi-RFP FRT19A, fd5-GFP
yw122; H15X4 mid1a5 FRT40A/ubi-GFP FRT40A; C-lacZ
brkM68 FRT19A/yw122 ubi-RFP FRT19A, C-GFP
Clones were induced by heat shocking 48–72 h AEL (after egg laying) larvae for 1 h at 37°C.
Gain-of-function clonal analysis:
yw122; act>STOP>Gal4, UAS-lacZ; fd5-GFP/UAS-tkvQD
yw122; act>STOP>Gal4, UAS-lacZ; fd5-GFP/UAS-arm∗
yw122; act>STOP>Gal4, UAS-lacZ; C-GFP/UAS-arm∗
Clones were induced by heat shocking 48–72 h AEL larvae for 10 min at 37°C.
At least three clones were scored for each experiment.
All fly lines listed above are described in FlyBase.
Generation of the fd45nt, fd51nt and fd45nt, fd5stop Mutant Flies
The fd45nt, fd51nt and fd45nt, fd5stop mutant flies were generated by CRISPR-Cas9 technique. Briefly, fd4 and fd5 gRNAs were cloned in the pBFv-U6.2 and injected in y1v1 P{y[ + t7.7] = nos-phiC31\int.NLS}X;P{y[ + t7.7] = CaryP}attP40 flies. These flies were then crossed with flies expressing Cas9 in their germinal line cells. Candidate mutants were confirmed by sequencing. gRNAs sequences were selected as the produced mutations (insertions and deletions) at the beginning of the respective gene. The following sequences were used as primers to clone the gRNAs in the pBFv-U6.2 vector following FlyCas9 protocols1 :
Sense fd4 5′-CTTCGTAGGATTCTCGCGAGGGCC-3′
Asense fd4 5′-AAACGGCCCTCGCGAGAATCCTAC-3′
Sense fd5 5′-CTTCGCTGAGCGGCAGCAATCTTTG-3′
Asense fd5 5′-AAACCAAAGATTGCTGCCGCTCAGC-3
To generate the fd45nt, fd5stop double mutant we introduced the fd5 gRNA into fd45nt flies. The candidate mutant flies were sequenced to verify the new mutations produced in the fd5 gene.
Generation of UAS-fd4 and UAS-fd5 Lines
Wild type fd4 and fd5 DNA was amplified from genomic DNA and cloned in the pUAST attB vector using the following primers (restriction sites are underlined and restriction enzyme used is noted in brackets):
For UAS-fd4:
5′-CAGTGAATTCGTAATAATGCCCCGGCCCTC GCGAGAATCC-3′ (EcoRI)
5′-CAGTCTCGAGCCCTGCTTGTTGCCACTTATCTAT ATCGTACGC-3′(XhoI)
For UAS-fd5:
5′ CAGTAGATCTCTTCGCAATGCCACGCCC ATTGAAAATGAG 3′ (BglII)
5′ CAGTGCGGCCGCTCAAAAGACGGGCAACGGGCCG 3′ (NotI)
Both UAS constructs were inserted into the same attP site (86Fb).
Generation of GFP and lacZ Reporter Transgenic Lines
To generate the A, B, C, C1, C2, C3, and Cs reporter constructs, DNA from the fd4/fd5 locus was amplified by PCR from genomic DNA. For the GFP reporter lines, the DNA fragments were first cloned into the pEntry/D-TOPO vector and then swapped into the attB-pHPdesteGFP vector (Boy et al., 2010), using the LR Clonase Enzyme Mix (Thermo Fisher Scientific). For the lacZ reporter lines, DNA fragments were amplified with primers containing restriction enzyme sites as overhangs, and subsequently cloned into plasmid attB-hs43-nuc-lacZ (Giorgianni and Mann, 2011).
We cloned all the sequences in the GFP reporter vector except for the C1 and A fragments that were cloned in the lacZ vector and the C-CRM that was cloned in both vectors.
To carry out these experiments, we used the following primers (restriction sites are underlined and restriction enzyme used is noted in brackets)
A fragment in attB-hs43-nuc-lacZ vector:
5′-CAGTGCGGCCGCCCGTGGCCATATTCATATGTCCAC-3′ (NotI)
5′-CAGTGCGGCCGCAGGATTCTCGCGAGGGCCGGGG-3′(NotI)
B fragment in attB-pHPdesteGFP vector:
5′CACCGCGCACCAGGCCACGCCCACCCCCG-3′
5′CAGTAGATCTGGAGCCGCAGGGGCCAGATA-3′
C fragment in attB-hs43-nuc-lacZ vector:
5′-CAGTGCGGCCGCGTATCTGGCCCCTGCGGCTCC-3′(NotI)
5′CAGTAGGCCTGCCCAGAGGCGGATTCGGATTCGG-3′(StuI)
C fragment in attB-pHPdesteGFP vector:
5′-CACCGTATCTGGCCCCTGCGGCTCC-3′
5′-GCCCAGAGGCGGATTCGGATTCGG-3′
C2 fragment in attB-pHPdesteGFP vector:
5′-CACCCAGATCTCAGTTTCTCGGTTCG-3′
5′-GCCCAGAGGCGGATTCGGATTCGG-3′
C3 fragment in attB-pHPdesteGFP vector:
5′-CACCAGATCTCGGCTCGGGTGTTGATGC-3′
5′-CAGTGCGGCCGCGCCGCTAGTTGCCGGCAC-3′
Cs fragment in attB-pHPdesteGFP vector:
5′-CACCCAGATCTCAGTTTCTCGG-3′
5′-CAGTGCCGCTAGTTGCCGGCAC-3′
The C1-lacZ fragment was cloned from the C sequence included in the attB-hs43-nuc-lacZ vector, using the restriction site of NotI enzyme that had been included at the beginning of C sequence, and BglII that digests in the middle of the C fragment. The primers to clone the C2 fragment were designed to clone from BglII.
All these constructs were inserted into the same attP site (86Fb).
Immunostaining
Embryos and larval leg imaginal discs were fixed and stained following standard procedures described in Requena et al. (2017). We used the following primary antibodies: rabbit anti-βGal (1/1000; Promega), mouse anti-βGal (1/1000; MP Biomedicals), guinea pig anti-Dll (1/2000) (Estella et al., 2008), rabbit anti-GFP (1/1000; Thermo Fisher Scientific), mouse anti-Dac (1/50; DSHB #mAbdac1-1), mouse anti-Wg (1/50; DSHB #4D4), rat-anti-Dsx-C (1/200) (Sanders and Arbeitman, 2008), mouse-anti-Scr (1/50; DSHB #6H4.1) and mouse anti-En (1/50; DSHB #4D9).
In situ Hybridization
The fd4 RNA probe used in this experiment was generated by PCR from genomic DNA using primers with the recognition sequences of the RNA polymerase T7 and T3:
Forward T7:
5′-TAATACGACTCACTATAGGGGGGACT GACCAATCTGCCCGCGC-3′
Reverse: T3:
5′-ATTAACCCTCACTAAAGGGACGGGGCTCCG ATATTGCTGCGCC-3′
The transcription to generate antisense probes was done using the RNA polymerase T3 in the presence of DIG (DIG RNA labeling mix, Roche) at 15°C for 2 h, and the probes were precipitated and suspended in H2O with DEPC (Sigma).
Imaginal discs were dissected in PBS 1× and fixed in 4% formaldehyde for 30 min at room temperature. Then, we washed in PBS-0.1% Tween (PBT) three times, and refixed for 20 min at room temperature in 4% formaldehyde, 0.1% Tween. The samples were washed again in PBT.
After three washes in PBT, discs were incubated overnight at –20°C in hybridization solution (SH; 50% formamide, SSC 5×, 100 μg/ml salmon sperm DNA, 50 μg/ml heparin, and 0.1% Tween).
The next day, we washed the disc in SH for 10 min at room temperature. The discs were prehybridized for 2 h at 55°C in SH and hybridized with digoxigenin (DIG)-labeled RNA probes at 55°C. The probes were previously denatured at 80°C for 10 min. All the solutions used before hybridization were treated with DEPC (Sigma). After hybridization, discs were washed in SH and PBT and incubated for 2 h at room temperature in a 1:4000 dilution of anti-DIG antibody (Roche). After incubation, the discs were washed in PBT and the detection of probes was performed with 4-Nitro blue tetrazolium chloride (NBT) and 5-Bromo-4-chloro-3-indolyl-phosphate (BCIP) solution (Roche). The discs were mounted in 70% glycerol.
Data Availability Statement
The raw data supporting the conclusions of this article will be made available by the authors, without undue reservation.
Author Contributions
MR-L, CP-R, and CE contributed to the conception and design of the study, performed the experiments, and analyzed the data. CE wrote the manuscript. All authors contributed to manuscript revision, read, and approved the submitted version.
Funding
This study was supported by grants from: the Programa Estatal de Generación de conocimiento y fortalecimiento científico y tecnológico del sistema de I + D + I (Ministerio de Ciencia, Innovación y Universidades) [No. PGC2018-095144-B-I00] to CE.
Conflict of Interest
The authors declare that the research was conducted in the absence of any commercial or financial relationships that could be construed as a potential conflict of interest.
Publisher’s Note
All claims expressed in this article are solely those of the authors and do not necessarily represent those of their affiliated organizations, or those of the publisher, the editors and the reviewers. Any product that may be evaluated in this article, or claim that may be made by its manufacturer, is not guaranteed or endorsed by the publisher.
Acknowledgments
We thank Michelle N. Arbeitman, William J. Brook, the Bloomington Stock Center, the Vienna Drosophila Resource Center, and the Developmental Studies Hybridoma Bank at The University of Iowa for fly stocks and reagents. We specially thank the Confocal microscopy service at CBMSO, Sergio Córdoba for comments on the manuscript, and Eva Caminero and Mar Casado for fly injections.
Supplementary Material
The Supplementary Material for this article can be found online at: https://www.frontiersin.org/articles/10.3389/fcell.2021.723927/full#supplementary-material
Supplementary Figure 1 | fd4 and fd5 expression in ventral imaginal discs. Third instar eye-antenna, genital and leg imaginal discs stained for Fd4-GFP (green) or Fd5-GFP (green), dpp-lacZ (blue), and Wg (red).
Supplementary Figure 2 | Generation of fd4, fd5 and fd4, fd5 double mutant alleles. Schematic representation of the different mutants generated for this study. gRNA target sites are in red. Chromatograms, as determined by Sanger sequencing, for the evaluation of the different mutants are presented. All sequences were obtained from homozygous flies at the exception of the fd51nt mutant. Insertions are indicated in blue. Asterisks indicate the first stop codon generated. Gray boxes represent alterations in the protein sequence due to stop codons and open reading frame changes. The corresponding amino acid sequences are shown below.
Supplementary Figure 3 | fd4 and fd5 mutant’s leg phenotypes. Adult male and female prothoracic legs of wild type, fd45nt, fd51nt and the double fd45nt, fd5stop mutants. The tibia (tib) and the first tarsal (ta1) segment are shown. Sex comb (SC) are indicated, and numbers mark the transverse rows in the ta1.
Supplementary Figure 4 | Validation of fd4 and fd5 RNAi lines. (A–D) Third instar leg imaginal discs stained for GFP (green) and hh-dsRED (red) of the following genotypes: (A) ci-Gal4, hh-dsRED; Fd4-GFP, (B) ci-Gal4, hh-dsRED; UAS-fd4-RNAi; Fd4-GFP, (C) ci-Gal4, hh-dsRED; Fd5-GFP, and (D) ci-Gal4, hh-dsRED; UAS-fd5-RNAi; Fd5-GFP. Separate channels for GFP and Hh are shown. Note the downregulation of Fd4 and Fd5 levels in the anterior compartment in panels (B,D). The compartment boundary is marked by white dots. (E) Male prothoracic adult legs of the indicated genotypes. Close up views of the sex comb are shown. (F) Quantification of sex comb bristles in the genotypes indicated and presented in panel (E). n > 15 sex combs per genotype were counted. Error bars indicate standard deviation (SD). Statistically significant differences based on Student’s t test are indicated: ****P < 0.0001 and not significant (ns).
Supplementary Figure 5 | Fd4/Fd5 are not required for H15 and mid expression. (A) Leg imaginal disc stained for Fd5-GFP (green), Wg (blue), and mid-lacZ (red). (B) Expression of the fd4 and fd5 RNAi lines under the control of the Dll-Gal4 line to knockdown Fd4/Fd5 levels. Leg imaginal disc is stained for mid-lacZ (red) and Wg (blue). The domain of Dll-Gal4 is marked by green dots. (C) The knockdown of Fd4/Fd5 levels in the anterior compartment by the expression of the fd4 and fd5 RNAi lines under the ci-Gal4 has no effect on H15-lacZ expression (green) or Wg protein (blue). hh expression is in red and the compartment boundary is marked by red dots. In panels (A–C), separate channels for mid-lacZ, H15-lacZ, and Wg are shown.
Supplementary Figure 6 | brk, omb, and mid/H15 mutant clones have no effect on fd4/fd5 expression. (A) C-GFP activity is observed in the wing imaginal disc in a complementary pattern to dpp expression and similar to brk (not shown). (B) brkM68 mutant clones in the leg disc marked by the absence of RFP (red) and stained for C-GFP (green). Separate channels for RFP and C-GFP are shown and the brkM68 mutant clones are outlined by white dots. (C) Leg imaginal disc with a dorsal omb282 mutant clone marked by the absence of RFP (red, arrow) and stained for Fd5-GFP (green) and Wg (blue). Separate channel for Fd5-GFP is shown and the clone is outlined by a red dotted line. (D) H15X4 mid1a5 mutant clones marked by the absence of GFP (green) in a third instar leg imaginal disc stained for C-lacZ (red) and Wg (blue). An arrow marks a ventro-lateral anterior compartment clone and a close up view is shown. All clones were generated 48–72 h before dissection.
Footnotes
References
Abzhanov, A., and Kaufman, T. C. (2000). Homologs of Drosophila appendage genes in the patterning of arthropod limbs. Dev. Biol. 227, 673–689. doi: 10.1006/dbio.2000.9904
Angelini, D. R., and Kaufman, T. C. (2004). Functional analyses in the hemipteran Oncopeltus fasciatus reveal conserved and derived aspects of appendage patterning in insects. Dev. Biol. 271, 306–321. doi: 10.1016/j.ydbio.2004.04.005
Archbold, H. C., Broussard, C., Chang, M. V., and Cadigan, K. M. (2014). Bipartite recognition of DNA by TCF/Pangolin is remarkably flexible and contributes to transcriptional responsiveness and tissue specificity of wingless signaling. PLoS Genet. 10:e1004591. doi: 10.1371/journal.pgen.1004591
Arora, K., Dai, H., Kazuko, S. G., Jamal, J., O’Connor, M. B., Letsou, A., et al. (1995). The Drosophila schnurri gene acts in the Dpp/TGF beta signaling pathway and encodes a transcription factor homologous to the human MBP family. Cell 81, 781–790. doi: 10.1016/0092-8674(95)90539-1
Basler, K., and Struhl, G. (1994). Compartment boundaries and the control of Drosophila limb pattern by hedgehog protein. Nature 368, 208–214. doi: 10.1038/368208a0
Bieli, D., Kanca, O., Requena, D., Hamaratoglu, F., Gohl, D., Schedl, P., et al. (2015). Establishment of a developmental compartment requires interactions between three synergistic cis-regulatory modules. PLoS Genet. 11:e1005376. doi: 10.1371/journal.pgen.1005376
Blair, S. S., Brower, D. L., Thomas, J. B., and Zavortink, M. (1994). The role of apterous in the control of dorsoventral compartmentalization and PS integrin gene expression in the developing wing of Drosophila. Development 120, 1805–1815. doi: 10.1242/dev.120.7.1805
Boy, A. L., Zhai, Z., Habring-Muller, A., Kussler-Schneider, Y., Kaspar, P., and Lohmann, I. (2010). Vectors for efficient and high-throughput construction of fluorescent Drosophila reporters using the PhiC31 site-specific integration system. Genesis 48, 452–456. doi: 10.1002/dvg.20637
Brook, W. J. (2010). T-box genes organize the dorsal ventral leg axis in Drosophila melanogaster. Fly 4, 159–162. doi: 10.4161/fly.4.2.11251
Brook, W. J., and Cohen, S. M. (1996). Antagonistic interactions between wingless and decapentaplegic responsible for dorsal-ventral pattern in the Drosophila Leg. Science 273, 1373–1377. doi: 10.1126/science.273.5280.1373
Burtis, K. C., and Baker, B. S. (1989). Drosophila doublesex gene controls somatic sexual differentiation by producing alternatively spliced mRNAs encoding related sex-specific polypeptides. Cell 56, 997–1010. doi: 10.1016/0092-8674(89)90633-8
Campbell, G., and Tomlinson, A. (1998). The roles of the homeobox genes aristaless and distal-less in patterning the legs and wings of Drosophila. Development 125, 4483–4493. doi: 10.1242/dev.125.22.4483
Couderc, J. L., Godt, D., Zollman, S., Chen, J., Li, M., Tiong, S., et al. (2002). The bric a brac locus consists of two paralogous genes encoding BTB/POZ domain proteins and acts as a homeotic and morphogenetic regulator of imaginal development in Drosophila. Development 129, 2419–2433. doi: 10.1242/dev.129.10.2419
Diaz-Benjumea, F. J., and Cohen, S. M. (1993). Interaction between dorsal and ventral cells in the imaginal disc directs wing development in Drosophila. Cell 75, 741–752. doi: 10.1016/0092-8674(93)90494-b
Diaz-Benjumea, F. J., Cohen, B., and Cohen, S. M. (1994). Cell interaction between compartments establishes the proximal-distal axis of Drosophila legs. Nature 372, 175–179. doi: 10.1038/372175a0
Eksi, S. E., Barmina, O., McCallough, C. L., Kopp, A., and Orenic, T. V. (2018). A Distalless-responsive enhancer of the Hox gene Sex combs reduced is required for segment- and sex-specific sensory organ development in Drosophila. PLoS Genet. 14:e1007320. doi: 10.1371/journal.pgen.1007320
Estella, C., and Mann, R. S. (2008). Logic of Wg and Dpp induction of distal and medial fates in the Drosophila leg. Development 135, 627–636. doi: 10.1242/dev.014670
Estella, C., and Mann, R. S. (2010). Non-redundant selector and growth-promoting functions of two sister genes, buttonhead and Sp1, in Drosophila leg development. PLoS Genet. 6:e1001001. doi: 10.1371/journal.pgen.1001001
Estella, C., McKay, D. J., and Mann, R. S. (2008). Molecular integration of wingless, decapentaplegic, and autoregulatory inputs into Distalless during Drosophila leg development. Dev. Cell 14, 86–96. doi: 10.1016/j.devcel.2007.11.002
Estella, C., Rieckhof, G., Calleja, M., and Morata, G. (2003). The role of buttonhead and Sp1 in the development of the ventral imaginal discs of Drosophila. Development 130, 5929–5941. doi: 10.1242/dev.00832
Estella, C., Voutev, R., and Mann, R. S. (2012). A dynamic network of morphogens and transcription factors patterns the fly leg. Curr. Top. Dev. Biol. 98, 173–198. doi: 10.1016/B978-0-12-386499-4.00007-0
Giorgianni, M. W., and Mann, R. S. (2011). Establishment of medial fates along the proximodistal axis of the Drosophila leg through direct activation of dachshund by Distalless. Dev. Cell 20, 455–468. doi: 10.1016/j.devcel.2011.03.017
Grieder, N. C., Nellen, D., Burke, R., Basler, K., and Affolter, M. (1995). Schnurri is required for Drosophila Dpp signaling and encodes a zinc finger protein similar to the mammalian transcription factor PRDII-BF1. Cell 81, 791–800. doi: 10.1016/0092-8674(95)90540-5
Grossmann, D., Scholten, J., and Prpic, N. M. (2009). Separable functions of wingless in distal and ventral patterning of the Tribolium leg. Dev. Genes Evol. 219, 469–479. doi: 10.1007/s00427-009-0310-z
Hacker, U., Grossniklaus, U., Gehring, W. J., and Jackle, H. (1992). Developmentally regulated Drosophila gene family encoding the fork head domain. Proc. Natl. Acad. Sci. U.S.A. 89, 8754–8758. doi: 10.1073/pnas.89.18.8754
Hannah-Alava, A. (1958). Morphology and chaetotaxy of the legs of Drosophila melanogaster. J. Morphol. 103, 281–310. doi: 10.1002/jmor.1051030205
Heingard, M., Turetzek, N., Prpic, N. M., and Janssen, R. (2019). FoxB, a new and highly conserved key factor in arthropod dorsal-ventral (DV) limb patterning. EvoDevo 10:28. doi: 10.1186/s13227-019-0141-6
Held, L. I. Jr., Heup, M. A., Sappington, J. M., and Peters, S. D. (1994). Interactions of decapentaplegic, wingless, and Distal-less in the Drosophila leg. Rouxs Arch. Dev. Biol. 203, 310–319. doi: 10.1007/BF00457802
Inoue, Y., Mito, T., Miyawaki, K., Matsushima, K., Shinmyo, Y., Heanue, T. A., et al. (2002). Correlation of expression patterns of homothorax, dachshund, and Distal-less with the proximodistal segmentation of the cricket leg bud. Mech. Dev. 113, 141–148. doi: 10.1016/s0925-4773(02)00017-5
Janssen, R., Feitosa, N. M., Damen, W. G., and Prpic, N. M. (2008). The T-box genes H15 and optomotor-blind in the spiders Cupiennius salei, Tegenaria atrica and Achaearanea tepidariorum and the dorsoventral axis of arthropod appendages. Evol. Dev. 10, 143–154. doi: 10.1111/j.1525-142X.2008.00222.x
Jiang, J., and Struhl, G. (1996). Complementary and mutually exclusive activities of decapentaplegic and wingless organize axial patterning during Drosophila leg development. Cell 86, 401–409. doi: 10.1016/s0092-8674(00)80113-0
Johnston, L. A., and Schubiger, G. (1996). Ectopic expression of wingless in imaginal discs interferes with decapentaplegic expression and alters cell determination. Development 122, 3519–3529. doi: 10.1242/dev.122.11.3519
Jory, A., Estella, C., Giorgianni, M. W., Slattery, M., Laverty, T. R., Rubin, G. M., et al. (2012). A survey of 6,300 genomic fragments for cis-regulatory activity in the imaginal discs of Drosophila melanogaster. Cell Rep. 2, 1014–1024. doi: 10.1016/j.celrep.2012.09.010
Kopp, A. (2011). Drosophila sex combs as a model of evolutionary innovations. Evol. Dev. 13, 504–522. doi: 10.1111/j.1525-142X.2011.00507.x
Lecuit, T., and Cohen, S. M. (1997). Proximal-distal axis formation in the Drosophila leg. Nature 388, 139–145. doi: 10.1038/40563
Lee, H. H., and Frasch, M. (2004). Survey of forkhead domain encoding genes in the Drosophila genome: classification and embryonic expression patterns. Dev. Dyn. 229, 357–366. doi: 10.1002/dvdy.10443
Marty, T., Muller, B., Basler, K., and Affolter, M. (2000). Schnurri mediates Dpp-dependent repression of brinker transcription. Nat. Cell Biol. 2, 745–749. doi: 10.1038/35036383
Maves, L., and Schubiger, G. (1998). A molecular basis for transdetermination in Drosophila imaginal discs: interactions between wingless and decapentaplegic signaling. Development 125, 115–124. doi: 10.1242/dev.125.1.115
McKay, D. J., and Lieb, J. D. (2013). A common set of DNA regulatory elements shapes Drosophila appendages. Dev. Cell 27, 306–318. doi: 10.1016/j.devcel.2013.10.009
Morimura, S., Maves, L., Chen, Y., and Hoffmann, F. M. (1996). decapentaplegic overexpression affects Drosophila wing and leg imaginal disc development and wingless expression. Dev. Biol. 177, 136–151. doi: 10.1006/dbio.1996.0151
Ober, K. A., and Jockusch, E. L. (2006). The roles of wingless and decapentaplegic in axis and appendage development in the red flour beetle, Tribolium castaneum. Dev. Biol. 294, 391–405. doi: 10.1016/j.ydbio.2006.02.053
Palma, M., and Lejeune, F. (2021). Deciphering the molecular mechanism of stop codon readthrough. Biol. Rev. Camb. Philos. Soc. 96, 310–329. doi: 10.1111/brv.12657
Penton, A., and Hoffmann, F. M. (1996). Decapentaplegic restricts the domain of wingless during Drosophila limb patterning. Nature 382, 162–164. doi: 10.1038/382162a0
Prpic, N. M., Wigand, B., Damen, W. G., and Klingler, M. (2001). Expression of dachshund in wild-type and Distal-less mutant Tribolium corroborates serial homologies in insect appendages. Dev. Genes Evol. 211, 467–477. doi: 10.1007/s004270100178
Pueyo, J. I., and Couso, J. P. (2005). Parallels between the proximal-distal development of vertebrate and arthropod appendages: homology without an ancestor? Curr. Opin. Genet. Dev. 15, 439–446. doi: 10.1016/j.gde.2005.06.007
Pyrowolakis, G., Hartmann, B., Muller, B., Basler, K., and Affolter, M. (2004). A simple molecular complex mediates widespread BMP-induced repression during Drosophila development. Dev. Cell 7, 229–240. doi: 10.1016/j.devcel.2004.07.008
Reim, I., Lee, H. H., and Frasch, M. (2003). The T-box-encoding Dorsocross genes function in amnioserosa development and the patterning of the dorsolateral germ band downstream of Dpp. Development 130, 3187–3204. doi: 10.1242/dev.00548
Requena, D., Alvarez, J. A., Gabilondo, H., Loker, R., Mann, R. S., and Estella, C. (2017). Origins and specification of the Drosophila wing. Curr. Biol. 27, 3826–3836.e5. doi: 10.1016/j.cub.2017.11.023
Robinett, C. C., Vaughan, A. G., Knapp, J. M., and Baker, B. S. (2010). Sex and the single cell. II. There is a time and place for sex. PLoS Biol. 8:e1000365. doi: 10.1371/journal.pbio.1000365
Ruiz-Losada, M., Blom-Dahl, D., Cordoba, S., and Estella, C. (2018). Specification and patterning of Drosophila appendages. J. Dev. Biol. 6:17. doi: 10.3390/jdb6030017
Sanders, L. E., and Arbeitman, M. N. (2008). Doublesex establishes sexual dimorphism in the Drosophila central nervous system in an isoform-dependent manner by directing cell number. Dev. Biol. 320, 378–390. doi: 10.1016/j.ydbio.2008.05.543
Shroff, S., Joshi, M., and Orenic, T. V. (2007). Differential delta expression underlies the diversity of sensory organ patterns among the legs of the Drosophila adult. Mech. Dev. 124, 43–58. doi: 10.1016/j.mod.2006.09.004
Shubin, N., Tabin, C., and Carroll, S. (1997). Fossils, genes and the evolution of animal limbs. Nature 388, 639–648. doi: 10.1038/41710
Struhl, G. (1982). Genes controlling segmental specification in the Drosophila thorax. Proc. Natl. Acad. Sci. U.S.A. 79, 7380–7384. doi: 10.1073/pnas.79.23.7380
Svendsen, P. C., Formaz-Preston, A., Leal, S. M., and Brook, W. J. (2009). The Tbx20 homologs midline and H15 specify ventral fate in the Drosophila melanogaster leg. Development 136, 2689–2693. doi: 10.1242/dev.037911
Svendsen, P. C., Phillips, L. A., Deshwar, A. R., Ryu, J. R., Najand, N., and Brook, W. J. (2019). The selector genes midline and H15 control ventral leg pattern by both inhibiting Dpp signaling and specifying ventral fate. Dev. Biol. 455, 19–31. doi: 10.1016/j.ydbio.2019.05.012
Svendsen, P. C., Ryu, J. R., and Brook, W. J. (2015). The expression of the T-box selector gene midline in the leg imaginal disc is controlled by both transcriptional regulation and cell lineage. Biol. Open 4, 1707–1714. doi: 10.1242/bio.013565
Tabin, C., Carroll, S., and Panganiban, G. (1999). Out on a limb: parallels in vertebrate and invertebrate limb patterning and the origin of appendages. Am. Zool. 39, 650–663. doi: 10.1093/icb/39.3.650
Tanaka, K., Barmina, O., and Kopp, A. (2009). Distinct developmental mechanisms underlie the evolutionary diversification of Drosophila sex combs. Proc. Natl. Acad. Sci. U.S.A. 106, 4764–4769. doi: 10.1073/pnas.0807875106
Tanaka, K., Barmina, O., Sanders, L. E., Arbeitman, M. N., and Kopp, A. (2011). Evolution of sex-specific traits through changes in HOX-dependent doublesex expression. PLoS Biol. 9:e1001131. doi: 10.1371/journal.pbio.1001131
Theisen, H., Haerry, T. E., O’Connor, M. B., and Marsh, J. L. (1996). Developmental territories created by mutual antagonism between Wingless and Decapentaplegic. Development 122, 3939–3948. doi: 10.1242/dev.122.12.3939
Tokunaga, C. (1961). The differentiation of a secondary sex comb under the influence of the gene engrailed in Drosophila melanogaster. Genetics 46, 157–176. doi: 10.1093/genetics/46.2.157
Tokunaga, C. (1962). Cell lineage and differentiation on the male foreleg of Drosophila melanogaster. Dev. Biol. 4, 489–516. doi: 10.1016/0012-1606(62)90054-4
Keywords: Fd4/Fd5, fd96Ca/fd96Cb, forkhead transcription factors, leg development, dorso-ventral axis, sex comb, Drosophila
Citation: Ruiz-Losada M, Pérez-Reyes C and Estella C (2021) Role of the Forkhead Transcription Factors Fd4 and Fd5 During Drosophila Leg Development. Front. Cell Dev. Biol. 9:723927. doi: 10.3389/fcell.2021.723927
Received: 11 June 2021; Accepted: 12 July 2021;
Published: 02 August 2021.
Edited by:
Rosa Barrio, CIC bioGUNE, SpainReviewed by:
Artyom Kopp, UC Davis Genome Center, United StatesWilliam Brook, University of Calgary, Canada
Copyright © 2021 Ruiz-Losada, Pérez-Reyes and Estella. This is an open-access article distributed under the terms of the Creative Commons Attribution License (CC BY). The use, distribution or reproduction in other forums is permitted, provided the original author(s) and the copyright owner(s) are credited and that the original publication in this journal is cited, in accordance with accepted academic practice. No use, distribution or reproduction is permitted which does not comply with these terms.
*Correspondence: Carlos Estella, Y2VzdGVsbGFAY2JtLmNzaWMuZXM=