- GReD Institut - UMR CNRS 6293 / INSERM U1103 University of Clermont-Auvergne, Clermont-Ferrand, France
To ensure locomotion and body stability, the active role of muscle contractions relies on a stereotyped muscle pattern set in place during development. This muscle patterning requires a precise assembly of the muscle fibers with the skeleton via a specialized connective tissue, the tendon. Like in vertebrate limbs, Drosophila leg muscles make connections with specific long tendons that extend through different segments. During the leg disc development, cell precursors of long tendons rearrange and collectively migrate to form a tube-shaped structure. A specific developmental program underlies this unique feature of tendon-like cells in the Drosophila model. We provide for the first time a transcriptomic profile of leg tendon precursors through fluorescence-based cell sorting. From promising candidates, we identified the Krüppel-like factor Dar1 as a critical actor of leg tendon development. Specifically expressed in the leg tendon precursors, loss of dar1 disrupts actin-rich filopodia formation and tendon elongation. Our findings show that Dar1 acts downstream of Stripe and is required to set up the correct number of tendon progenitors.
Introduction
The musculoskeletal system comprises numerous cellular components including muscles and tendons. The assembly of these components is tightly controlled to achieve a stereotyped functional architecture. Acquisition of muscle shape and pattern thus directly relies on where the muscle fibers are anchored to the skeleton via specialized structures, the tendons. Tendons are required not only to transmit the muscle contraction force to the skeleton but also to set up a functional musculoskeletal system. The importance of coordinated development of tendons and more generally of connective tissues (CT) and muscles into an integrated system is well documented in different model organisms (Kardon et al., 2003; Schweitzer et al., 2010; Hasson, 2011; Kardon, 2011; Nassari et al., 2017; Felsenthal et al., 2018). Research on different models indicates that final muscle patterning relies on several extrinsic elements (Schnorrer and Dickson, 2004; Hasson, 2011; Valdivia et al., 2017; Laurichesse and Soler, 2020). In Drosophila embryo, inducing ectopic muscle attachment sites interferes with myotube guidance (Vorbrüggen and Jäckle, 1997) and several secreted or membrane-associated proteins have been described as mediating muscle migration toward the tendon cells (Callahan et al., 1996; Prokop et al., 1998; Kramer et al., 2001; Swan et al., 2004; Schnorrer et al., 2007; Ordan et al., 2015). In avian models, transplantation studies showed that myoblast patterning was dependent on the surrounding connective tissues with Tcf-4-expressing CT cells establishing the muscle prepattern (Kardon et al., 2002, 2003; Rinon et al., 2007; Mathew et al., 2011). These observations support the hypothesis that the correct patterning of musculo-skeletal system depends not only on the features of specific muscles but also on specific CT features. Yet, in contrast to muscles, the genetic program that controls the formation of morphologically and functionally distinct tendons has not been investigated.
Like vertebrates, Drosophila shows a variety of morphologically distinct tendons. Larval monofiber muscles are linked to the exoskeleton (Volk and VijayRaghavan, 1994; Frommer et al., 1996) through a single cell attachment site, whereas large clusters of tendon cells specified in the wing disc epithelium anchor the massive flight muscles in the adult thorax (Fernandes et al., 1996). In the fly leg, the appendicular movements are ensured by the connection between muscles and long internal tendons (Miller, 1950; Soler et al., 2004). In Drosophila, Stripe (Sr)/Egr-like is the key transcription factor of tendon differentiation and a hallmark of all tendon cells (Volk and VijayRaghavan, 1994; Fernandes et al., 1996; Frommer et al., 1996; Vorbrüggen and Jäckle, 1997; Ghazi et al., 2003; Soler et al., 2004). Although a few other transcription factors are specifically expressed in tendon sub-populations such as apterous in wing disc-associated tendons or GCM/Glide in embryos, their contribution to the acquisition of characteristic properties of specific tendons remains elusive.
Amongst the various tendons observed in Drosophila, the leg tendons show morphological and developmental similarities with the long tendons of the autopod in the mouse. They extend from the most distal part of the leg and elongate through the leg segments, where they are associated with extrinsic muscles (Huang, 2017; Huang et al., 2015; Soler et al., 2004; Watson et al., 2009). Strikingly, the morphogenesis of these leg long tendons in Drosophila shares many similarities with the tubulogenesis that occurs during tracheal or salivary gland formation (Girdler and Röper, 2014; Hayashi and Kondo, 2018; Maruyama and Andrew, 2012). First, the tendon cells undergo apical constriction followed by invagination without migration (Laddada et al., 2019). The cells then collectively migrate with the extension of basal protrusions, leading to the elongation of a tube-shaped tendon (Figure 1). It has also been suggested that like for the developing salivary glands (Vining et al., 2005), elongating tendons could interact with surrounding tissues, especially with the myoblasts (Soler et al., 2016, 2004). We have already shown that long tendons originate from tendon precursors that are selected among the cells of the leg segmental joints expressing odd-skipped (Laddada et al., 2019). While Odd is sufficient to induce the primary invagination of the epithelial cells (Hao et al., 2003; Ibeas and Bray, 2003), Stripe is required to make these cells competent to migrate and form a long tube-shaped tendon (Laddada et al., 2019).
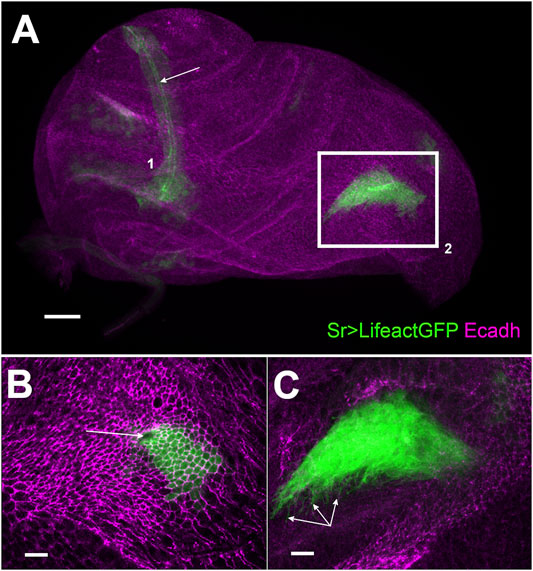
FIGURE 1. Development of long tendons from 0 h APF leg disc visualized using sr-gal4 driven Lifeact.GFP expression (A) z-projection of selected optical sections of 0 h APF leg disc. Long tendons form from clusters of epithelial cells (stained with E-cadherin in magenta) expressing sr-gal4>UAS-Lifeact.GFP (green), long tendon (lt) of the tarsi (1) has elongated from the joint between tarsus T5 and pretarsus. Apical accumulation of Lifeact.GFP outlines the tube lumen (arrow). In the dorsal femur (box 2), the tibia levator long tendon (tilt) has started to invaginate and elongate. Scale bar 30 μm (B) and (C) High magnification of selected confocal planes (from bo×2). Scale bar 10 μm (B) Surface view: we can observe apical constriction of Lifeact.GFP cells, revealed by E-cadherin staining, around the invaginating pit (arrow) forming the tube lumen (C) deeper Z planes reveal dense arborization of actin-based filopodia at the basal side of the invaginating tendon (arrows).
To gain a better understanding of the developmental mechanisms of appendicular tendon formation, we undertook a transcriptomic analysis of the long tendon precursors. RNA sequencing of these cells at the onset of long tendon formation enabled us to identify the associated expression of tube morphogenesis-related genes, the downregulation of which recapitulated phenotypes observed in other models of tubulogenesis. This finding functionally validates Drosophila leg tendon development as a new model to study tube morphogenesis. We subsequently performed a genetic screen to identify key transcription factors involved in the developmental program of these specific tendons. Among the positive candidates, we identified dar1 (dendritic arbor reduction 1), a gene coding for a DNA-binding protein belonging to the Krüppel-like transcription factor (KLF) family. In Drosophila, Dar1 had previously been shown to determine the multipolar morphology of post-mitotic neurons (Ye et al., 2011; Wang et al., 2015) and to downregulate the proliferation of intestinal stem cells (Wu et al., 2018). Here, we show that dar1 is not expressed in the tendon precursors of the flight and larval muscles but that its expression is restricted to leg tendon precursors. Our functional analysis revealed that long tendon development was severely impaired after dar1 knockdown. The number of cytoplasmic protrusions was drastically reduced, and the elongation, but not the invagination of tendon cell precursors was compromised. Importantly, our results show that in the absence of Dar1, the initial expression of stripe (sr) in subsets of leg disc epithelial cells is not compromised but that the final number of Stripe-positive tendon cells is strongly reduced. In view of its critical effect on tendon development, we describe the role of Dar1 as a key component of tube-shaped tendon morphogenesis, which is also required to set up the correct number of leg tendon cells.
Results
Transcriptomic Profiling of Appendicular Long Tendon Precursors Reveals a Gene Expression Signature of Tubulogenesis
We had previously shown that precursors of the long tendons are clusters of epithelial cells selected among the cells of leg segmental joints. The Notch pathway initiates stripe expression, the key factor in tendon cell differentiation, in the leg tendon precursors (Soler et al., 2004; Laddada et al., 2019).
These Sr-positive cells invaginate and migrate to form the tube-shaped long tendon between the end of the larval stages and the first hours of pupal formation. To gain a better understanding of the molecular mechanisms underlying these dramatic cell morphological changes, we undertook a whole transcriptome RNA-seq analysis of these cells at the onset of pupation (0 h APF). Total RNAs were prepared from FACS-isolated sr-gal4>UAS-GFP cells from 0 h APF leg discs and processed for whole-transcriptome RNA-seq (GSE169313), followed by bioinformatic analysis (for cell-sorting and RNAseq detailed protocols and validations see Materials and Methods and Supplementary Material). With the FPKM cut-off at 10 for reliable detection of gene expression, we found that a wide array of genes 5,479) were significantly expressed in sr-gal4>UAS-GFP cells, probably reflecting the developmental plasticity exhibited by Drosophila imaginal disc cells (McClure and Schubiger, 2007). However, gene ontology (GO) analysis of these genes showed an over-representation of the GO terms related to “locomotion” and “muscle structure development” with numerous genes known to be involved in muscle attachment site development and/or the formation of myotendinous junctions (Table 1). Considering GOs related to pathways, we found an over-representation of Notch, Wnt and MAPK pathways, consistent with our previous results and those of others, showing that these pathways play a key role in tendon development in Drosophila (Yarnitzky et al., 1997; Soler et al., 2004; Lahaye et al., 2012; Laddada et al., 2019). Finally, this analysis also pointed to GO terms relative to tube morphogenesis such as “epithelial tube morphogenesis” or “regulation of tube size”, supporting our earlier assertion that long tendon development shared common features with salivary gland or tracheal tube morphogenesis (Girdler and Röper, 2014; Hayashi and Kondo, 2018; Laddada et al., 2019). In order to functionally validate this point, we crossed the sr-gal4 line with UAS-RNAi or UAS-Dominant Negative lines directed against genes known to regulate many aspects of cell adhesion, migration or maintenance of tissue integrity during morphogenetic processes such as tubulogenesis (Supplementary Table S1). Expression of most of these RNAi or dominant negative transgenes strongly impacted the normal development at larval or pupal stages and RNAi against genes such as the βPS integrin totally disrupted the long tendon formation (data not shown). Interestingly, knocking down the expression of genes controlling the length of developing tubes (Luschnig et al., 2006; Kerman et al., 2008) such as serpentine, vermiform or lolal, led to an excessive elongation of the long tendons in the mature leg (Supplementary Figure S1). Altogether, these results validate our approach to identifying new regulators of appendicular long tendons and support a pivotal role of tubulogenesis in their development.
Deciphering the Core Genetic Program of Leg Tendon Development
Because transcription factors are fundamental in controlling the developmental program to build any structure during development, we carried out a lethality and climbing-based in vivo RNAi screen targeting the genes that encode for TFs that we found specifically enriched in our RNAseq data. Remarkably, of the 5,479 genes with FPKM>10, transcripts of 31 genes encoding for predicted transcription factors (Rhee et al., 2014) exhibited more than 1.5-fold higher levels of expression in the GFP + cells than in the whole leg disc cells (input), strongly suggesting that these TFs have a relevant specific role in the development of the leg long tendon (Supplementary Table S2). We performed this RNAi screen by crossing tendon-specific UAS-Dicer2; sr-gal4 line with UAS-RNAi lines targeting these 31 candidates from two independent libraries: TRiP (Bloomington Stock Center) and VDRC (Vienna Stock Center), when available. To limit the number of false positives due to off-target effects, we did not include UAS-line RNAi from the VDRC stock center, for which more than two off-targets were predicted (this was the case for three genes). In this way, we crossed 53 UAS-RNAi lines to target the 31 selected genes, and we could test two different lines for 71% of them (22/31) (Supplementary Table S2). F1 generation were screened for developmental lethality and/or climbing defect (for the detailed screen protocol, see Materials and Methods). Out of the 31 candidates analyzed, seven showed a significant embryonic (or early larval stage) lethality, with more than 20% of embryos unable to hatch or dying before their third larval stage, 12 displayed a pupal lethality score higher than 20%, and 14 showed a climbing defect. Thus, nearly 65% (20/31, including stripe itself) of the candidate genes were positive for at least one readout and for eight of them this result was obtained with two different RNAi lines (Table 2). Within these positive hits, we found odd and drm both members of the odd-skipped gene family that act downstream of the Notch pathway to promote leg tendon growth (Laddada et al., 2019). We also identified esg, which interferes with the Notch pathway during stem cell maintenance/differentiation (Korzelius et al., 2014), and which is required during tracheal morphogenesis (Miao and Hayashi, 2016). Moreover, it has been shown that esg misexpression in embryonic epidermal cells (including muscle attachment sites) can interfere with the development of muscle attachment sites (Staudt et al., 2005). These positive genes strengthen our strategy to identify new regulatory factors of leg long tendon development. Other candidates, including six uncharacterized predicted transcription factors (CG numbers), have never been reported or investigated in tendon development.
We then considered the vertebrate orthologs of these newly-highlighted TFs in order to identify functional evolutionary conservation. We note that of the 14 conserved genes, the orthologs of dar1 (KLF5 and KLF4) and CG9650 (BCL11 A) were shown to be differentially regulated in limb tendon cells during mouse development in two independent studies (Havis et al., 2014; Liu et al., 2015). Strikingly, for each of these genes, the expression of two different RNAi lines gave significant adult climbing defect and/or metamorphosis but no embryonic lethality (Table 2). These observations strongly suggest that these two genes are likely candidates for the specific development of appendicular long tendons. Below we analyze the expression and function of dar1 characterized by high transcript enrichment in our RNAseq datasets.
dar1 Is Expressed in Leg Tendons but Not in Embryonic and Flight Muscle Attachment Sites
To confirm the expression of dar1 in the leg disc tendon, we performed immunostaining on sr-gal4>UASmcherryNLS leg discs from third larval instar to 5 h after pupal formation (APF) using Dar1 antibody (Ye et al., 2011). dar1 expression colocalizes with stripe expression domains, within the cells corresponding to the specified long tendons of the leg (Figure 2). dar1 was first found in the two earliest clusters of Sr-positive cells that would form the long tendon of the tarsi (lt) and in tibia levator tendon (tilt) in the dorsal femur (Figures 2A,B). Subsequently, during early pupation this expression was maintained in lt and tilt and new Sr-positive clusters corresponding to other long tendons in different leg segments started to be specified (Figures 2C–F). Importantly, dar1 expression was observed in all these clusters and correlated with our RNA-sequencing data generated from tendon precursors at early pupal stage (0,2 h APF), which showed a high specific enrichment of dar1 expression in sr-gal4>GFP cells compared to IP (FC = 4.7), strengthening the reliability of our data. To determine whether dar1 was a hallmark of all tendon cells in Drosophila, we also analyzed its expression in embryos and in wing discs (Supplementary Figure S2). In embryos, we could not detect Dar1 in sr-gal4>UASmcherryNLS positive tendon cells (Supplementary Figure S2A,B). Likewise, tendon precursors of flight muscles in the wing disc did not show any dar1 expression (Supplementary Figure. S2C,D).
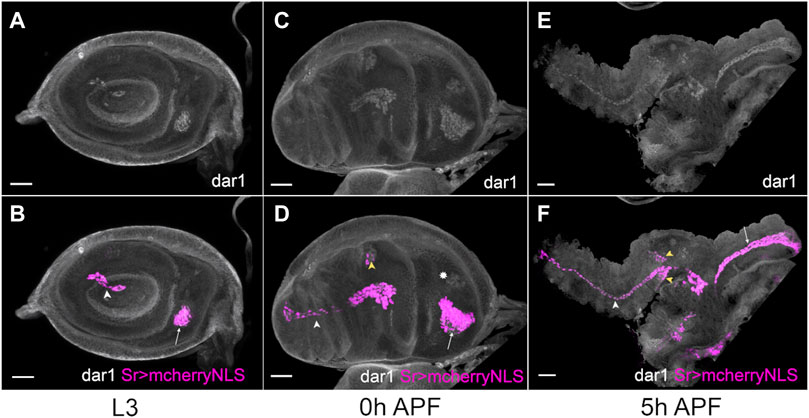
FIGURE. 2. dar1 expression pattern co-localizes with stripe expression in Drosophila leg disc. Selected optical sections of sr-gal4>UAS-mcherryNLS (magenta) leg discs immunostained with anti-Dar1 (gray) at different steps of development (A,B) In L3 leg disc, dar1 and mcherryNLS expressions colocalize in cells prefiguring the future lt in the tarsi (white arrowhead) and the future tilt in the dorsal femur (arrow). This co-expression is maintained at 0 h APF (C,D) and 5 h APF (E,F) in elongating tendons, whereas newly-specified sr > mcherry-positive cells, in tibia segment notably, also start to express dar1 (yellow arrowheads). Note that dar1 is also expressed at a low level in the apparent chordotonal organ at 0 h APF (asterisk). Scale bar 30 μm.
All in all, these results strongly suggest that Dar1 plays a specific role in the establishment of these unique long tendons in Drosophila.
Correct Adult Muscle Patterning Required dar1 Tendon Expression
To determine whether the adult locomotion defects observed in tendon-specific dar1 KD could be explained by an abnormal development of the appendicular musculotendinous system, we analyzed adult leg myotendinous architecture in dar1 KD flies. In sr-gal4>UAS-Lifeact.GFP, UAS-dar1RNAi flies, we observed heterogenous results with only a few tendons partially affected per fly (data not shown). This observation is likely due to a residual expression of the targeted gene, which is expected when using RNA interference. We therefore decided to use the sr-gal4>UAS-Lifeact.GFP, UAS-dar1RNAi flies associated with a heterozygous null dar13010 allele to potentialize dar1RNAi efficiency and facilitate the subsequent analysis. As shown by longitudinal cryosections along the proximo-distal axis of the sr-gal4,dar13010/+>UAS-Lifeact.GFP, UAS dar1RNAi legs, long tendons in all segments were often shortened or even missing, and so muscle pattern was strongly affected (Figure 3). In control legs, major muscles labeled by phalloidin, displayed a feather-like pattern with each fiber attached on one side to a long tendon and on the other one to an individual cuticle muscle attachment site (cMAS). By contrast, when long internal tendons were affected, both ends of a muscle fiber were anchored to a cMAS, generating a transverse fiber. This is clearly visible in the ventral tibia where the long tendon appears much shorter in the visualized cryosections (Figure 3E). Thus, in the proximal region of this segment, both ends of the muscle fibers are attached to cMAS, whereas in the distal part, the fibers still connect to the shortened remaining ventral long tendon (Figure 3F). We could also observe a highly disorganized muscle pattern in the dorsal femur that coincided with no visible tilt in this cryosection. Our observations thus indicate that when dar1 expression is specifically lowered in leg tendon precursors, resulting long tendons are severely affected, leading to abnormal muscle patterning. Moreover, even in the absence of long tendons, the muscle fibers can still attach to cuticle via cMAS, suggesting that the latter, like those of flight and embryonic muscles, do not required Dar1 activity.
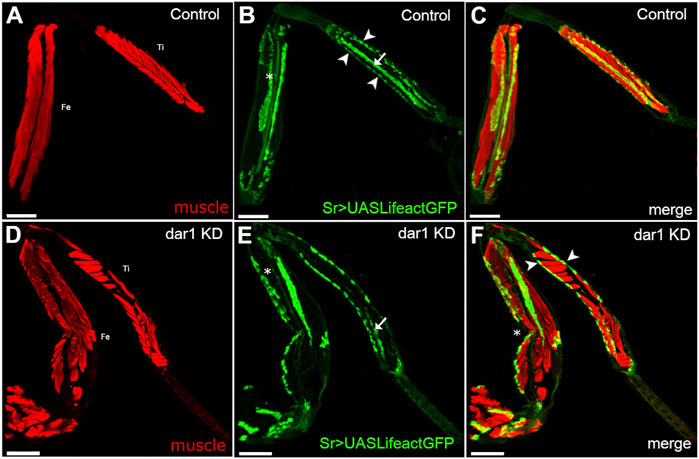
FIGURE 3. Knockdown of dar1 expression in long tendons alters myotendinous architecture of adult leg. Combination of confocal images from adult leg cryosections (Tibia: Ti, Femur: Fe); muscles are stained with stained phalloidin (red). Tendons are visualized with Lifeact.GFP (green) (A–C) In each segment of control sr-gal4,dar13010/+>UAS-Lifeact.GFP legs, major muscles display a feather-like pattern. For instance, fibers of the muscle in the ventral tibia are attached on one side to the tarsi depressor long tendon (arrow in B) and on the other to an individual cuticle muscle attachment site (arrowheads in B) (D–F) on this cryosection of sr-gal4, dar13010/+>UAS-Lifeact.GFP,UAS-dar1RNAi leg, the tilt in the dorsal femur is absent (asterisk in E compared to B) and the long ventral tendon appears shorter in the tibia (arrow in B and E). Alteration or absence of these long tendons leads to the misattachments of muscle fibers with both ends attached to cuticular attachment sites (arrowheads in F) leading to the formation of transversal fibers. Note that disruption of myotendinous architecture seems to affect the morphology of the leg, with a pinch in the femur segment (asterisk in F). number of leg segment observed after cryosection (control n = 5, dar1 KD n = 6). Scale bar 100 μm.
Dar1 Is Required for Tubulogenesis-like Morphogenesis of Leg Tendons
To better characterize the role of Dar1 in long tendon development, we focused our analysis on the time when tendon precursors started to express dar1, from the L3 larval stage to the early hours of pupation. We specifically analyzed the tarsal lt, extending from pre-tarsus to femur, and the tilt in the dorsal femur. These two tendons form earliest: they are specified at the early third larval instar and then invaginate and elongate until the first hours of metamorphosis (Laddada et al., 2019; Soler et al., 2004). Tendon specific depletion of dar1 led to apparent shortening of these elongated structures in early metamorphosis (Figures 4A–F). To quantify this phenotype, we measured the relative size of the lt and tilt (for measurement details see Materials and Methods) at three developmental time points (late L3, 2 and 4 h APF) in control (sr-gal4,dar13010/+>UASLifeact.GFP) and dar1 KD (sr-gal4,dar13010/+>UAS-Lifeact.GFP, UASdar1RNAi) leg discs. For each of these developing tendons, we observed a significant reduction of their length at the three different time points when dar1 expression was lowered (Figures 4G,H). The tarsal tendon was very significantly shortened as early as late L3, whereas the difference in size of the tilt in dorsal femur was more pronounced at later stages (4 h APF). This is probably because tarsal lt is specified and starts to elongate slightly earlier than the one in the dorsal femur (Soler et al., 2004) and is therefore longer at a given time point. Thus, the length difference compared with control was greater in earlier stages for the tarsal lt than for the tilt in dorsal femur. We infer that the apparent smaller size of the tendons after dar1 KD becomes more and more significant as the tendons elongate, supporting a potential function of Dar1 in this process. Likewise, 2 h APF leg discs from homozygote dar13010 rare escapers (less than 1% of the larva reach the pupal stage and none of them survive for more than a few hours APF), stained against discs large (dlg) septate junction marker, showed no visible elongating structure (Figures 5A,B). Remarkably, in the location of the most distal tarsal segment where lt normally starts to develop, we could still see an accumulation of dlg protein prefiguring the local folding of the epithelium and the lumen formation (Figures 5A,B). These observations indicate that Dar1 is not needed for initial steps of epithelium invagination but is then required for the tube-like tendon elongation.
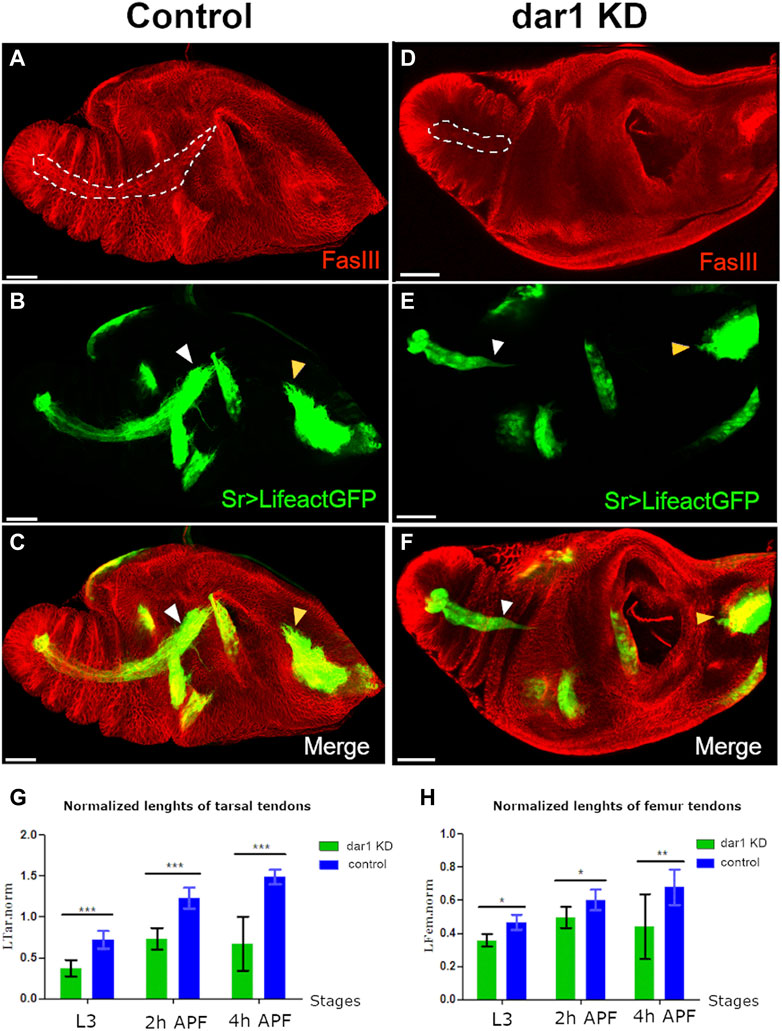
FIGURE. 4. Dar1 is required for the elongation of long tendons. Confocal images of sr-gal4,dar13010/+>UAS-Lifeact.GFP (A–C) and sr-gal4,dar13010/+>UAS-Lifeact.GFP,UAS-dar1RNAi (D–F) leg discs at 3 h APF immunostained with anti-fasIII. On this optical section, the long elongating tendon of the tarsi is revealed by UAS-Lifeact.GFP and FasIII (dashed line A and D). In dar1 KD leg disc (D–F), the tarsal lt appears much shorter than in the control (white arrowheads in B,C and E,F). At this stage of development, other tendons are less elongated than the one in the tarsi and therefore their difference in length between control and dar1 KD is not as obvious, except for the tilt in the dorsal femur (yellow arrowhead in B,C and E,F), which also appears shorter (G,H) Graphs showing the lengths of the lt in tarsi (G) and tilt femur (H) in control leg discs versus and dar1 KD leg disc at three time points: late L3 (control n = 11, dar1 KD n = 11), 2 h APF (control n = 10, dar1 KD n = 17) and 4hAPF (control n = 5, dar1 KD n = 5). Error bars represent s.d.; *p < 0.05, **p < 0.01 and ***p < 0.001 (Bonferroni test). Scale bar 40 μm.
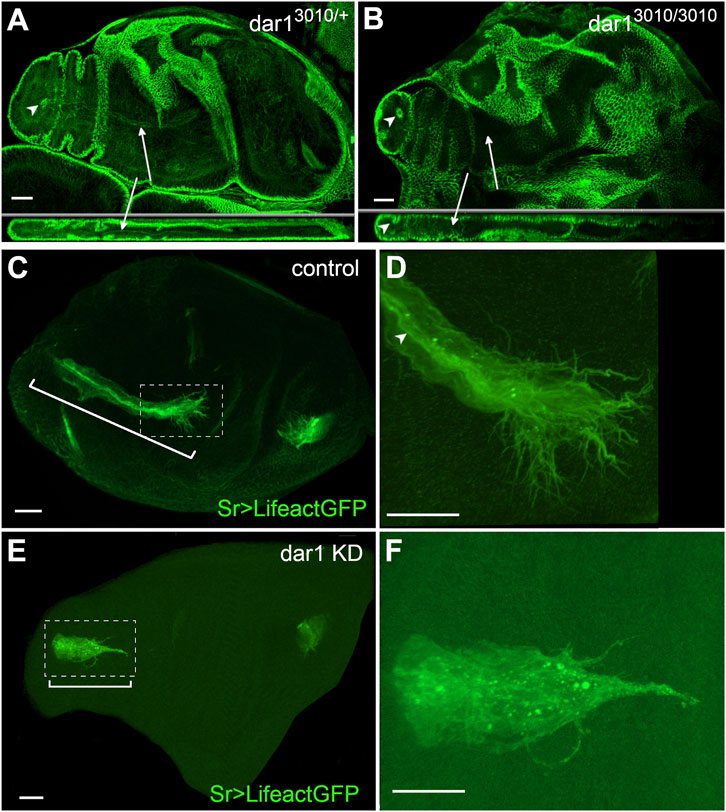
FIGURE 5. Dar1 is not required for the epithelium invagination but is needed for actin-rich filopodia arborization of long tendons (A,B) Confocal sections of 2 h APF leg discs immunostained with anti-dlg (A) selected optical sections of control dar13010/+ heterozygous leg disc. dlg accumulation reveals lumen aperture on the most distal tarsal segment (arrowhead) and also stained a long internal structure corresponding to tarsal lt (arrow) (B) In all observed leg discs from rare dar13010/3010 homozygous mutant escapers, local constriction of the epithelium still underlines the local invagination of the epithelial cells, but no elongating internal structure is formed (arrows) (number of samples, control dar13010/+ n = 10, dar 3010/3010 n = 5) (C–F) Confocal sections of 0 h APF leg discs from sr-gal4,dar13010/+>UAS-Lifeact.GFP and sr-gal4,dar13010/+>UAS-Lifeact.GFP,UAS-dar1RNAi 2 h APF pupae. On these selected optical sections, in dar1 KD condition tarsal lt (bracket in E) appears much shorter than in the control (bracket in C) (D) and (F) are higher magnifications from (C) and (E) respectively (D) In the control leg disc, leading cells at the distal part of the elongating tendon display numerous actin-rich protrusions at their basal membrane and apical accumulation of actin underlines the tube lumen (arrowhead) (F) In the dar1 KD leg disc, filopodia arborization is systematically affected with a marked decrease in protrusion number (number of samples, control n = 10, dar1 KD n = 17). Overall Lifeact.GFP distribution is strongly affected and tube lumen cannot be distinguished in dar1 KD. Scale bar 20 μm.
Besides this elongation defect, dar1 RNAi expression in long tendon precursors results in a severe depletion of filopodia as revealed by UAS-Lifeact.GFP expression (Figures 5C–F). For instance, the total number of filopodia at the tip of the tarsal lt in control 2 h APF disc was systematically greater than 30 (n = 10), whereas in dar1 KD leg discs, tarsal lt systematically displayed fewer than five remaining protrusions (n = 17). This result indicates that the reduction of dar1 expression directly or indirectly affects the formation of actin-rich filopodia. Moreover, while the tendon lumen in control leg discs is underlined by apical Lifeact.GFP accumulation, in dar1 KD leg disc, we observed a fluorescent dotty pattern reflecting a general actin disorganization or mislocalization.
Taken together, our results show that Dar1 participates in the cellular events required for actin cytoskeleton organization and that dar1 KD reduces the number of cytoplasmic protrusions.
Dar1 Is Required to Set up the Correct Number of Sr-Positive Cells
dar1 Depletion Induces Loss of Sr-Positive Progenitors Without Affecting Number of Mitotic Cells nor Inducing Apoptosis
Although it has been shown that the number of cells that make up a tube is not closely correlated to tube length (Beitel and Krasnow, 2000), the question arose of whether the total number of sr-gal4 positive cell was affected after dar1 KD. To find out, we counted the number of cells constituting both tarsal and dorsal femur tendons in sr-gal4,dar3010/+>UASmcherryNLS control pupae, and in sr-gal4,dar3010/+>UASmcherryNLS, UASdar1RNAi pupae at early stages of metamorphosis (Figures 6A–C). First, in control leg disc we saw a significant increasing number of sr-gal4>mcherryNLS cells from 0 to 5 h APF for both tendons, indicating that during the elongation process new Sr-positive cells were recruited. Next, we found that the number of sr-gal4>mcherryNLS cells was significantly reduced in dar1 KD leg discs at the two different time points compared to the control leg disc. (Figures 6A–C). Lastly, in dar1 depleted tarsal tendon, the number of sr-gal4>mcherryNLS cells was not statistically different between 0hAPF and 5hAPF, suggesting that no further tendon cells were recruited between these two time points, unlike in controls. Our results thus show that the failing of long tendon elongation due to dar1 downregulation is associated to a defect of sr-gal4>mcherryNLS cell numbers. As a decrease in the number of cells may be due to a defect in cell proliferation and/or to an increase in cell death, we tested these two variables in sr-gal4,dar3010/+>UASmcherryNLS, UASdar1RNAi leg discs. Because Dar1 has been shown to restrict the proliferation of intestinal stem cells (Wu et al., 2018), we did not favor a defect of proliferation, still we immunostained dar1 KD and control leg discs using phospho-histone three antibody. Although numerous mitotic cells can be observed in leg disc from mid-L3 stage (beginning of tendon cell induction) to early metamorphosis, only rare mitotic events could be observed among sr-gal4>mcherryNLS cells in this time window in both dar1 KD and control conditions (Supplementary Figure S3).
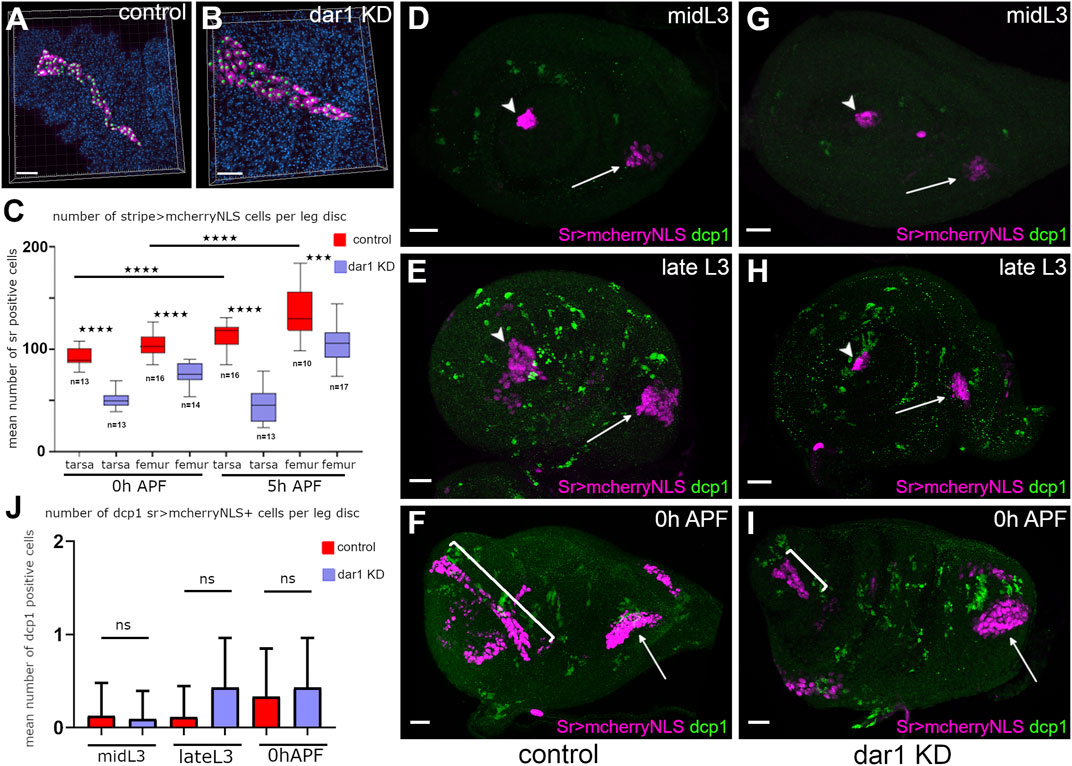
FIGURE 6. Reduction of tendon cell number in dar1 KD leg discs is not due to ectopic apoptosis (A,B) examples of semi-automated counting tendon cells (magenta) from Z-stack projections of confocal images of lt from sr-gal4,dar13010/+>UAS-mcherryNLS (A) and sr-gal4,dar13010/+>UAS-mcherryNLS, UAS-dar1RNAi (B) 5 h APF leg discs, stained with DAPI (blue). Each green spot corresponds to a single cell (C) Box-plot diagram comparing number of mcherry-positive cells in lt and in the tilt, in control and in dar1 KD leg discs at two different time points. Box boundaries and horizontal bar indicate 25/75 percentile and mean value, respectively. ***p < 0.001 and ****p < 0.0001 (Bonferroni or Welch test) (D–I) Confocal images of sr-gal4,dar13010/+>UAS-mcherryNLS (magenta) (D–F) and sr-gal4,dar13010/+>UAS-mcherryNLS,UAS-dar1RNAi (G–I) leg discs immunostained with anti-dcp1 (green) at different times of development. Arrowheads and arrows point to tarsal lt and tilt in femur, respectively. In both control (D) and dar1 KD (G) L3 leg discs, cell death cannot be detected in lt or tilt. At late L3, several apoptotic cells are observed throughout the leg discs (E,H) but none of them are tendon cells. As stated previously, at this time there are already fewer tendon cells in the dar1 KD leg disc (H) than in the control (E). At early metamorphosis, lt (bracket) appears much shorter in the dar1 KD leg disc (I) than in the control (F), but no specific cell death is observed in this tendon (J) Graphs showing the mean number of sr > mcherryNLS cells that are dcp1positive per disc at different stages of development in control and dar1 KD leg discs (total number of analysed discs, control n = 23, dar1 KD n = 25). Scale bar 20 μm.
To determine whether loss of sr-gal4>UASmcherryNLS cells could result from cell death increase, we performed immunostaining against caspase-activated dcp1 (Figures 6D–I). Between the time of induction of first Sr-positive cells and the early step of metamorphosis, we could barely detect sr-gal4>mcherryNLS apoptotic cells in dar1 KD leg discs Figures 6M–O), while the defect in cell number was evident as early as the late L3 stage (compare Figures 6E,H). We confirmed this result by expressing GC3Ai fluorescent apoptotic sensor, which efficiently follows apoptotic cell dynamics tissue-specifically (Schott et al., 2017). Thus, when GC3Ai apoptotic sensor was specifically expressed in sr-gal4 cells, rare events of apoptosis were occasionally observed in both control and dar1 KD leg discs (Supplementary Figure S4). We concluded that the up to 50% fewer tendon cells in dar1 KD context could not be explained by any marked increase in cell death or a lower proliferation rate.
Epistatic Relationship Between dar1 and Stripe Expressions
One obvious cause of missing tendon cells in the dar1 KD leg disc would be a positive requirement of Dar1 to induce and/or maintain stripe expression (and so sr-gal4 expression). However, a direct requirement of Dar1 in inducing stripe expression is very unlikely because sr-gal4 driver comes from a Gal4 insertion in the stripe gene (sr-gal4md710) that is known to fairly recapitulate stripe expression (Soler et al., 2004; Usui et al., 2004), Indeed, all the phenotypes that we observed were obtained using sr-gal4 driver to induce UAS-dar1RNAi. So, if Dar1 was inducing stripe expression, we should expect a limited effect of dar1RNAi expression using this driver. Still, we decided to test this possibility by monitoring stripe expression in dar13010 homozygous null-mutant escapers at the time when initial pools of stripe expressing cells were set up in tarsi and dorsal femur (between early and mid-L3 larval stage). As expected, Stripe was still clearly detected at the L3 stage in both clusters in dar13010 heterozygous or homozygous mutants, indicating that stripe initial induction was very likely dar1-independent (Figures 7A–D). Conversely, expression of dar1 was completely abolished when a dominant negative form of stripe was expressed in the leg disc epithelium (Supplementary Figure S5). These results strongly suggest that Stripe acts upstream to regulate dar1 expression in the developing tendon, and that Dar1 is then needed to correctly pattern long tendons with the right number of Sr-positive cells. Finally, to determine whether the lack of Sr-positive cells in sr-gal4,dar3010/+>UASdar1RNAi context could be explained by a role for Dar1 in long-term maintenance of stripe expression, we performed a G-TRACE experimental analysis (Evans et al., 2009). This technique is used to determine the expression of a gal4 driver (here sr-gal4) at a current time point by inducing UAS-RFP expression (Figures 7F,I), and its historical expression by inducing UAS-Flippase expression. Flp enzyme recognizes FRT sites and removes the STOP cassette in the Act-FRT-STOP-FRT-GFP construct, allowing a permanent expression of GFP. Accordingly, if any cell has been committed as a sr-gal4 positive cell at any time during development and then loses this expression, it should still be detectable through sustainable GFP expression. In other words, RFP expression reveals real-time gal4 expression and GFP expression its past expression. In this way, we could see in both dar1 KD and control 5 h APF leg disc, cells that currently expressed sr-gal4 (RFP+) are also GFP+ (Figures 7E,H). This result means that missing tendon cells in dar1 KD disc are not cells that have lost their sr-gal4-positive fate. We therefore conclude that Dar1 is not required to maintain stripe expression in developing tendon cells.
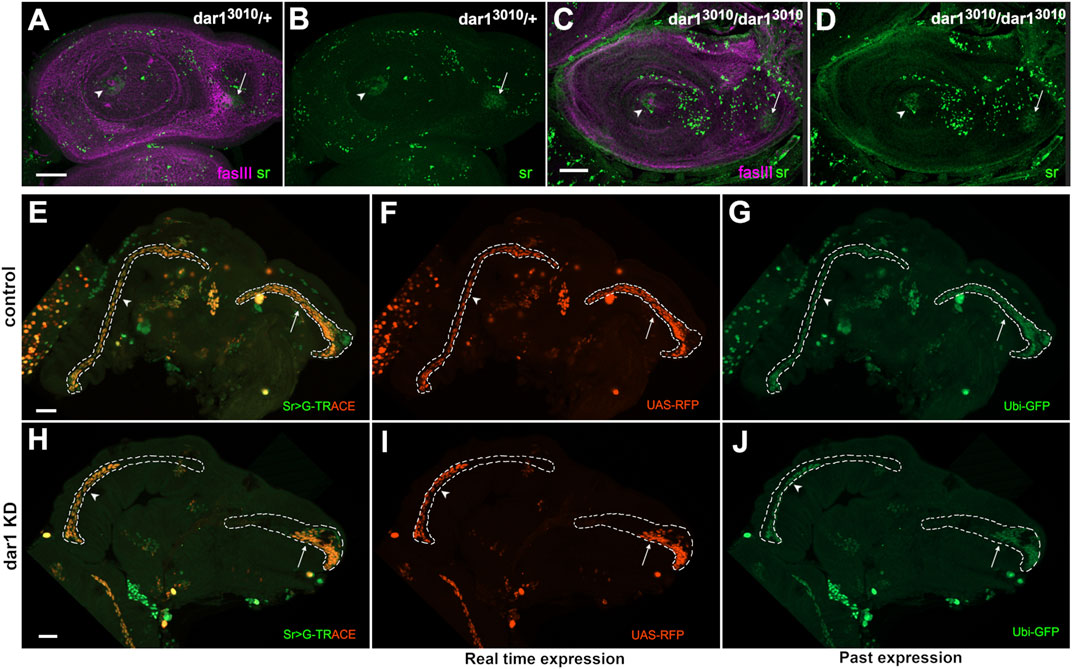
FIGURE 7. Dar1 is not required to initiate or maintain stripe expression (A–D) Confocal sections of L3 leg discs immunostained with anti-sr (green) and anti-fasIII (magenta) (A–B) In dar13010/+ heterozygous leg discs, stripe expression reveals tendon cell precursors in tarsal segments (arrowhead) and in dorsal femur (arrow) (C–D) In the leg disc of rare dar13010/3010 homozygous mutant escapers, stripe expression is still observed in tarsal segments (arrowhead) and dorsal femur (arrow) (total number of analysed discs, control dar13010/+ = 15, dar13010/3010 n = 12) (E–J) G-TRACE in sr-gal4,dar13010/+ (E–G) and sr-gal4,dar13010/+>UAS-dar1RNAi (H–J) leg discs at 5 h APF. RFP (red) staining shows current sr-gal4 expression (F, I) and GFP (green) reveals past expression (G, J) (E,H) merged G-TRACE expression. Dashed lines outline tarsal lt (arrowheads) and tilt in dorsal femur (arrows). At this stage, lt and tilt have deeply invaginated into the developing leg disc in the control (E–G), with most of the tendon cells having maintained sr-gal4 expression (red and green staining). By contrast, in dar1 KD leg discs (H–I), the same tendons appear much shorter with both current sr-gal4 expression (red) and past sr-gal4 expression (green), indicating that shortening of those tendons is not due to premature loss of sr-gal4 expression in “missing” cells (extension of dashed lines in dar1 KD prefigures the position where GFP positive cells should have been detected if the apparent tendon shortening was due to a premature loss of sr-gal4 expression). Scale bar 30 μm.
Altogether, our results show that Dar1 is required for a proper morphogenesis of the long internal leg tendons. Thus, length shortening of the tendons in dar1 KD context is associated to a dramatic decrease in Sr-positive cell numbers, which cannot be explained by cell death, proliferation defect or loss of stripe expression.
Discussion and Perspectives
In Drosophila during metamorphosis, epithelium-derived progenitors of tendon legs undergo critical morphogenetic changes to adopt a unique tube-like structure for muscle attachment sites. Long tendon morphogenesis is characterized by several steps considered hallmarks of canonical tubulogenesis, such as cell apical constriction and tissue invagination, followed by collective cell migration (Maruyama and Andrew, 2012; Girdler and Röper, 2014; Hayashi and Kondo, 2018). Another mark of tubulogenesis is that developing long tendons form an apical lumen and display F-actin-rich protrusions at the basal membrane of migrating cells (Laddada et al., 2019). Our newly-generated transcriptomic data, specific to leg tendon precursors, are consistent with these observations. These data highlight the expression of genes related to cell-shape rearrangement and cytoskeleton regulation, echoing the deep cell rearrangement of these epithelium-derived cells. Corroborating the importance of the tubulogenic process in leg tendon morphogenesis, GO analysis highlighted GO terms such as tube size regulation and morphogenesis. We also validated the involvement of the tubulogenic process by recapitulating the phenotypes observed in other systems, such as the tube length defects observed when serpentine and vermiform expressions are knocked down in the tracheal system (Luschnig et al., 2006).
Tendons are crucial components of the musculoskeletal system but still our knowledge on the developmental program that controls tenogenesis remains poorly investigated, even though tendon injuries are very common. Identifying transcription factors and their roles in regulating this developmental program is crucial to develop new effective treatments for tendon repair. For instance, known transcription factors, such as Scleraxis, Mohwak and EGR1, associated with tendon development in vertebrates are also activated upon tendon injury (reviewed in Nourissat et al., 2015). So, to pinpoint critical transcriptional regulators of leg tendon development, we performed a short lethality and climbing RNAi screen. Our gene preselection, based on our transcriptomic data, was especially helpful: nearly 65% of these candidates induced locomotion defect and/or lethality when downregulated in tendon cells, even though RNAi knockdown efficiency is often limited by residual gene expression (Perkins et al., 2015). In comparison, a previous unbiased RNAi screen targeting 1,384 genes to uncover genes involved in flight muscle attachment sites produced only about 1.5% positive candidates (Tiwari et al., 2015). Thus, the efficiency of our RNAi screen also emphasizes our RNA-seq data reliability.
Among the newly-identified candidates, we found that tendon expression of dar1 was restricted to the appendicular long tendon and was not found in other tendon precursors that do not form long internal structures (tendons of flight muscles or larval muscle tendon), making this gene an excellent candidate for the specific development of long tendons. Correlating climbing defects, the LOF of this gene impaired the formation of the leg long tendon. dar1 KD led to a clear-cut tendon phenotype by the end of larval stages and early metamorphosis when long tendons are specified and when they elongate. Our further analysis of dar1 function shows that Dar1 participates in the cellular events required for actin cytoskeleton organization and specifically filopodia formation. It also highlights a critical role for this Krüppel-like factor in the elongation of the long tendons. Although there is no clear evidence for a direct role of filopodia in the tubulogenesis process, migrating cells form two types of actin-based protrusions, the lamellipodia, involved in cell motility, and the filopodia, which may sense and interact with the surrounding environment (Svitkina et al., 2003; Girdler and Röper, 2014; Okenve-Ramos and Llimargas, 2014). Thus, although our work does not show that cell tendon shortening is a direct consequence of the decrease in filopodia numbers, it makes of Dar1 an interesting potential link between actin-rich protrusion formation and tube elongation. Interestingly, dar1 has been shown to regulate the dendritic microtubule cytoskeleton by suppressing the expression of the microtubule-severing protein spastin (Ye et al., 2011), which we found depleted in our RNAseq data. So, we have tried to phenocopy dar1 KD by overexpressing spastin in tendon cells, but it systematically and rapidly induced death of the larvae, which unabled us to clearly elucidate whether manipulating spastin activity could indirectly affect filopodia formation in developing tendons. Nevertheless, reciprocal interactions between MT and actin networks is a well-established paradigm (Rodriguez et al., 2003; Mohan and John, 2015). For instance, the growth cone in neuronal cells depends on coordinated interactions between MTs and actin filaments (Schaefer et al., 2002; Geraldo and Gordon-Weeks, 2009; Bearce et al., 2015). We therefore cannot exclude the possibility that defects in actin organization observed upon dar1 downregulation are due to alterations of MT assembly and/or MT-actin network coordination. Supporting this last hypothesis, comparison of gene expression profiles between midgut cells expressing dar1 RNAi and overexpressing dar1 showed a deregulation of DAAM expression (Wu et al., 2018). DAAM encodes a formin known to link and coordinate actin and microtubule network dynamics (Szikora et al., 2017). It would thus be of interest to unravel a potential regulation of Dar1 on DAAM, or more broadly, to perform a Dar1 genetic screen interaction with actin and microtubule modulators.
Concomitantly to the defect in long tendon growth and actin cytoskeleton organization, we demonstrated Dar1 involvement in recruitment of new Sr-positive cells. First, we found that additional tendon progenitors were recruited, after initial expression of stripe in L3, contributing to the development of long tendons during their elongation in a normal context. Secondly, our finding showed that dar1 KD led to a reduction of the number of Sr-positive tendon progenitors without apparent effect on apoptosis and the number of mitotic cells suggesting that dar1 might control stripe expression (by inducing or maintaining its expression). However, our lineage and epistasis experiments indicate instead that Dar1 acts downstream of Stripe and that Stripe is necessary for inducing dar1 expression. We can thus infer that Stripe regulates tendon morphogenesis through dar1 activation, which is in turn responsible for tendon elongation but also necessary to recruit new Sr-positive cells in an undetermined mechanism. Organ morphogenesis often correlates with cell-fate induction, and numerous studies in the last decade have shown how multicellular morphogenetic events can feed back into gene regulatory pathways to specify cell fate (Chan et al., 2017). Thus, during the 3D changes of a pseudo-stratified epithelium, the polarity and cytoskeleton rearrangements of the engaged cells are sensed by the neighboring cells through mechano-transduction. For instance, tissue deformations caused by germ-band extension upregulate the expression of the transcription factor twist via nuclear translocation of β-catenin (Desprat et al., 2008). We had previously shown that the Notch pathway is required for the initial expression of stripe in L3 stages (Laddada et al., 2019). However, conditional depletion of Notch activity at a later time point (early metamorphosis) did not appear to alter dorsal femur long tendon formation, whereas we show here that recruitment of additional Sr-positive tendon precursors is still ongoing at this time. It is tempting to speculate that morphological changes, partly driven by dar1, could be mechano-transduced to the following cells and activate stripe expression in an alternative Notch-independent way. Alternatively, local reorganization of the leg disc epithelium by the primary invagination of first specified Sr-positive cells could also play a role by exposing the surrounding cells to local signals. Kesavan et al. have demonstrated the role of CDC42-mediated tubulogenesis as a driver of non-cell-autonomous cell fate specification in pancreatic tubulogenesis (Kesavan et al., 2009). They showed how this small RhoGTPase, through its role in tube formation as an actin nucleator, provided the correct microenvironment for proper cell specification. Our own results indicate dar1 KD impacts both tendon elongation and number of tendon cells. Further work is required to determine whether cellular rearrangement during tube formation can have an impact on the recruitment of new tendon progenitors. For instance, it could help determining whether Dar1 transcriptional activity regulates major actors of the cytoskeleton organization in order to trigger a spatial rearrangement of the epithelial cells. Such reorganization could provide a permissive microenvironment for a specifying signal that remains to be identified.
Although animal appendages have long been seen as non-homologous structures, there is growing molecular evidence that limbs of vertebrates and insects could arise from an ancestral appendage developmental program (Pueyo and Couso, 2005). Recently, Tarazona et al. reported that genes and signaling pathways that guide the development of both vertebrates and arthropods also control the development of cephalopod mollusk arms and tentacles (Tarazona et al., 2019). Their results thus strongly suggest that bilaterian appendages evolved by parallel activation of a genetic program that was present in a common ancestor. Whether the development of limb internal structures follows a similar conserved path is less well-documented. We have previously shown that the key regulator of vertebrate appendicular myogenesis, the lb/Lbx1 gene, is also required for proper leg muscle identity in Drosophila (Maqbool et al., 2006). Strikingly, Huang et al. have shown that long tendons in the mouse form by a rapid elongation of the tendon in parallel with skeletal growth, and that this elongation is fueled by the recruitment of new mesenchymal progenitors (Huang et al., 2019). This recruitment is dependent on the transcription factor Scleraxis (Scx), which appears to be unnecessary for first tendon anchoring but is subsequently needed for the recruitment of new progenitors during tendon elongation. Although the Scx ortholog has not yet been identified in Drosophila, our study identified transcription factor Dar1 as a specific factor of elongating tendons in Drosophila. Interestingly, its mammalian counterparts KLF5 and KLF4 have also been identified in two independent transcriptomic analysis of mouse limb tendon cells (Havis et al., 2014; Liu et al., 2015) and expression of KLFs have been reported in connective tissue surrounding tendon cells in the chicken (Orgeur et al., 2018). More strikingly, a recent study (Kult et al., 2021) showed that tendon-to-bone attachment cells have a bi-fated origin, with the ability to activate a combination of chondrogenic and tenogenic transcriptomes, and those authors identified KLF2/4 as central regulators of these unique bi-fated cells in vertebrates. This work and our previous results (Laddada et al., 2019) indicate that Dar1/KLF-positive cells, which shape the leg long tendons, originate from tendon precursors (Sr-positive cells) that are selected among the cells of leg segmental joints expressing odd-skipped. Thus, our most recent findings and those of others suggest an evolutionary conserved function of KLFs in the complex integration of the musculoskeletal system of the limb.
Materials and Methods
The following Drosophila stocks were used: R10H12-gal4 (Pfeiffer et al., 2008; BDSC 48278), UAS-mCherryCAAX (BDSC 59021), UAS-mCherryNLS (BDSC 38425), enhancer trap lines sr-gal4md710 (Usui et al., 2004, BDSC 2663), UAS-Lifeact.GFP (BDSC 35544), UAS-dar1RNAi (BDCS 31987), dar13010 (BDSC 65269), UAS-GC3Ai (Schott et al., 2017, BDSC 84346), UAS-serpRNAi (BDSC 63556), UAS-vermRNAi (BDSC 57188), UAS-lolalRNAi (BDSC 35722), UAS-Dicer2 (BDSC 24650), UAS-SrDN (gift from Vorbrüggen and Jäckle, 1997) and G-TRACE line (Evans et al., 2009, BDSC 28280).
Cell Sorting and RNA Extraction
The fluorescence-activated cell sorting (FACS) protocol was adapted from (Harzer et al., 2013). Briefly, approximately 50 UAS-Lifeactin.GFP; sr-gal4/TM6b, tb white pupae (0 h APF) were dissected on ice to collect 250–300 leg imaginal discs in M3 (S3652 Sigma Aldrich) complemented medium. Leg disc cells were dissociated in collagenase (P4762-Sigma Aldrich) and papain (C267-Sigma Aldrich) solution for 1 h at 30°C, 300 rpm (Thermomixer Eppendorf), with additional mechanical stirring. After filtering, we used a FACSAriaTM device (4 °C, 20 psi, nozzle ∅ 100 μm) to first sort a batch of cells (roughly 50,000 cells per sample) independently of fluorescence, which we used as input (IP) and we then sorted the tendon cell (GFP+) population based on GFP fluorescence. Samples were directly collected in Trizol reagent (Invitrogen, 15596026). RNA extraction was performed with Zymo Quick-RNA microprep kit (R1051), sample RNA quality and quantity were estimated with QuBit (ThermoFisher, Qubit RNA HS Assay Kit, Q32852) and BioAnalyzer (Agilent, Agilent RNA 6000 Nano Kit 5067-1511), and sample specificity was analyzed by qPCR. Samples were stored at−80°C. For the detailed protocol, see Supplementary Material S1.
RNAseq and Transcriptomic Data Analysis
Extracted total RNA from GFP+ and IP cells from three different replicates were sent to Heidelberg genomic platform EMBL for high-throughput mRNA sequencing (NextSeq 500/Illumina). They generated mRNA libraries with NEB RNA Ultra kit (New England Biolabs E7770 L) and dToligos probe were used to target mRNA for cDNA synthesis. Single-end multiplex was performed. Bioinformatic analysis was performed by Dr Yoan Renaud using FastQC and Bowtie2 software (reference genome Dm6). FPKM and differential expression between IP and GFP + samples were determined using R script DEseq2 software. Sample correlations are given in Supplementary Material. Differential expression was performed using R-package DEseq2. Fold change (FC) between GFP+ and IP samples was computed using their normalized raw counts. GEO accession number: GSE169313.
In Vivo RNAi Screen
53 UAS-RNAi lines (see Supplementary Table S2) from two separate stock centres, VDRC and BDSC (Dietzl et al., 2007; Ni et al., 2009) were crossed with UAS-dicer2, UAS-CAAXmcherry; sr-gal4/TM6b,tb, hu line and screened for lethality and/or climbing defect. 5–10 males carrying UAS-RNAi were mated with 15–20 females. Crosses and egg laying were performed at 25°C; 48 h after egg laying, larvae were transferred at 28°C.
Embryonic or early larval stage lethality rate was measured by counting the number of non-tubby over tubby third instar larvae. Percentage of lethality was calculated by applying formula (1 − (number of non-tubby larvae)/(number of tubby larvae)) × 100. Crosses were considered as positive for embryonic (early larval stage) lethality when lethality was higher than the 20% arbitrarily chosen threshold. To assess the percentage of lethality during metamorphosis, we applied formula (1 − (the number of non-tubby, non-humeral emerging adult flies)/(total number of initial non tubby pupae)) × 100. Crosses with a higher score than 20% were considered positive candidates for metamorphosis lethality.
When sr-gal4>UAS-RNAi adult flies survived, we performed a climbing test adapted from the RING (Rapid Iterative Negative Geotaxis) assay published by Gargano et al. (Gargano et al., 2005). Up to ten young non-balanced flies (48 h old) were transferred to an empty vial and allowed to recover at room temperature for at least 2 h. The vial was then rapped sharply on a table three times in rapid succession to initiate negative geotaxis responses. The flies’ positions in the vial were digitally captured after 5 s and we calculated the percentage of flies remaining in the bottom third of the vial. For each cross, two replicates (two vials of ten flies) were measured three times. We calculated the mean of these six trials. When this mean was equal to or greater than 30%, the corresponding cross was scored as “climbing defect”.
RNAis directed against stripe (100% embryonic lethality) and mcherry (0% embryonic lethality, 5% metamorphosis lethality, 15% climbing defect) were considered as positive and negative controls, respectively.
Immuno-Histochemistry, Cryosections and Confocal Microscopy
The following primary antibodies were used: guinea pig anti-Dar1 (1:250, courtesy of B. Ye), guinea pig anti-Stripe (1:500, from T. Volk), mouse anti-Fas III (1:500, Developmental Studies Hybridoma Bank [DSHB]), rabbit anti-Twist (1:500, our lab), mouse anti-Dlg (1:500, DSHB), chicken anti-GFP (1:500, Abcam), rat anti-DE-cadherin (1:500, DHSB), rabbit anti-Dcp1 (1:100, Cell Signaling), rabbit anti-pH3 (1:1000, Invitrogen), and anti-mcherry (rabbit, Abcam). Muscle fibers were visualized using cy3 or cy5-conjugated phalloidin (1:1000, Invitrogen) and nuclei using DAPI (ThermoFisher D1306). Secondary antibodies (Jackson) anti-rabbit, anti-guinea pig, anti-chicken and anti-mouse conjugated to Alexa488, cy3 or cy5 fluorochromes were used (1:500). Immunohistochemistry experiments were performed on samples fixed in 4% paraformaldehyde (PFA) for 20 min, rinsed in 0.1% PBS-Triton and blocked in 10% horse serum before immunostaining with primary antibodies (at 4°C, overnight). The samples were then rinsed and incubated with appropriate secondary antibody (at room temperature, 1 h). For cryosections, we dissected adult leg and fixed them from 40 min to 1 h in 4% PFA. Fixed samples were then incubated in sucrose solution (30%) at 4 °C overnight. Adult legs were then laid on the bottom of a plastic well and carefully covered with Neg-50TM gel (Richard-Allan Scientific). The preparation was frozen at −80°C to set the gel. Sections were cut with a cryostat at 4°C (thickness 18–20 um). The sliced samples were stained with phalloidin before imaging. Immunostaining was visualized on an inverted SP8 Leica confocal microscope, and images were analyzed with Imaris 7.6.5 software.
Tendon Length Measurement
Tendon size was determined by normalizing the length of the tendon over the whole size of the imaginal disc. For this purpose, we used the bounding-box function of the Imaris software to semi-automatically generate a 3D mesh of the imaginal disc (using FasIII staining). The disc was viewed as an ellipse with major axis (length) X and minor axis (width) Y. Discs were considered as flat objects, since at the developmental stages of analysis the thickness (Z) dimension has no significant impact on the measurement. From the ellipse-disc we computed the radius R of each corresponding disc (R = √X × Y⁄2). The lengths of tendons were measured using the function MeasurementPoint by manually following their pathways. Finally, we normalized the tendon length by dividing the length by the disc radius R. Statistical analyses were carried out using Prism software with an analysis of variance (ANOVA) between the different conditions and the different stages. Statistical significance between control and knockdown conditions was estimated using the Bonferroni test.
Counting Cell Number in Developing Tendon
0 and 5 h APF leg discs from sr-gal4,dar13010/+>UAS-mcherryNLS and sr-gal4,dar13010/+>UAS-mcherryNLS,UAS-dar1RNAi pupae were dissected and stained with DAPI for 1 h. 0.5 μm confocal sections of long tendon of tarsi and dorsal tendon in femur (tilt) were performed. Images were processed with Imaris software and numbers of mcherryNLS and DAPI-positive cells per tendon were determined automatically using the function Spots. The automatic cell counting was subject to manual adjustement.
Statistical significance was estimated using the Bonferroni or Welch test.
Data Availability Statement
The datasets presented in this study can be found in online repositories. The names of the repository/repositories and accession number(s) can be found below: https://www.ncbi.nlm.nih.gov/geo/, GSE169313.
Author Contributions
CS, QL, BM, and LL contributed to conception and design of the study YR organized the database. QL performed the statistical analysis CS and QL wrote the first draft of the manuscript CS, QL, and BM wrote sections of the manuscript All authors contributed to manuscript revision, read, and approved the submitted version.
Funding
This work was supported by the iGReD institute (INSERM U1103, CNRS 6293, University of Clermont-Auvergne), AFM-Téléthon (MyoNeurAlp Strategic Program), Fondation pour la Recherche Médicale (Equipe FRM Award), Agence Nationale pour la Recherche (ANR LIMBCT) and the iSITE CAP2025 Grant. QL. was supported by a PhD fellowship from CPER (Epicure 2015).
Conflict of Interest
The authors declare that the research was conducted in the absence of any commercial or financial relationships that could be construed as a potential conflict of interest.
Publisher’s Note
All claims expressed in this article are solely those of the authors and do not necessarily represent those of their affiliated organizations, or those of the publisher, the editors and the reviewers. Any product that may be evaluated in this article, or claim that may be made by its manufacturer, is not guaranteed or endorsed by the publisher.
Acknowledgments
We are grateful to G. Vorbrüggen, B. Ye, T. Volk for their gifts of antibodies and flies. We thank Bloomington Drosophila Stock Center (BDSC) and Vienna Drosophila Resource Center (VDRC) for keeping and sending Drosophila stocks. We thank Nicolas Allègre, Stéphanie Maupetit-Méhouas, Pierre Pouchin and Caroline Vachias from the Single-Cell (S3C) and CLIC imaging facilities at the iGReD (Clermont-Ferrand) and the EMBL Genomics Core Facilities (Heidelberg) for their technical expertise and support.
Supplementary Material
The Supplementary Material for this article can be found online at: https://www.frontiersin.org/articles/10.3389/fcell.2021.747563/full#supplementary-material
References
Bearce, E. A., Erdogan, B., and Lowery, L. A. (2015). TIPsy Tour Guides: How Microtubule Plus-End Tracking Proteins (+TIPs) Facilitate Axon Guidance. Front. Cel. Neurosci. 9, 241. doi:10.3389/fncel.2015.00241
Beitel, G. J., and Krasnow, M. A. (2000). Genetic Control of Epithelial Tube Size in the Drosophila Tracheal System. Development 127, 3271–3282. doi:10.1242/dev.127.15.3271
Callahan, C. A., Bonkovsky, J. L., Scully, A. L., and Thomas, J. B. (1996). Derailed Is Required for Muscle Attachment Site Selection in Drosophila. Development 122, 2761–2767. doi:10.1242/dev.122.9.2761
Chan, C. J., Heisenberg, C.-P., and Hiiragi, T. (2017). Coordination of Morphogenesis and Cell-Fate Specification in Development. Curr. Biol. 27, R1024–R1035. doi:10.1016/j.cub.2017.07.010
de Celis Ibeas, J. M., and Bray, S. J. (2003). Bowl Is Required Downstream of Notch for Elaboration of Distal Limb Patterning. Development 130, 5943–5952. doi:10.1242/dev.00833
Desprat, N., Supatto, W., Pouille, P.-A., Beaurepaire, E., and Farge, E. (2008). Tissue Deformation Modulates Twist Expression to Determine Anterior Midgut Differentiation in Drosophila Embryos. Develop. Cel 15, 470–477. doi:10.1016/j.devcel.2008.07.009
Dietzl, G., Chen, D., Schnorrer, F., Su, K.-C., Barinova, Y., Fellner, M., et al. (2007). A Genome-wide Transgenic RNAi Library for Conditional Gene Inactivation in Drosophila. Nature 448, 151–156. doi:10.1038/nature05954
Evans, C. J., Olson, J. M., Ngo, K. T., Kim, E., Lee, N. E., Kuoy, E., et al. (2009). G-TRACE: Rapid Gal4-Based Cell Lineage Analysis in Drosophila. Nat. Methods 6, 603–605. doi:10.1038/nmeth.1356
Felsenthal, N., Rubin, S., Stern, T., Krief, S., Pal, D., Pryce, B. A., et al. (2018). Development of Migrating Tendon-Bone Attachments Involves Replacement of Progenitor Populations. Development 145, dev165381. doi:10.1242/dev.165381
Fernandes, J. J., Celniker, S. E., and VijayRaghavan, K. (1996). Development of the Indirect Flight Muscle Attachment Sites inDrosophila:Role of the PS Integrins and thestripeGene. Develop. Biol. 176, 166–184. doi:10.1006/dbio.1996.0125
Frommer, G., Vorbrüggen, G., Pasca, G., Jäckle, H., and Volk, T. (1996). Epidermal Egr-like Zinc finger Protein of Drosophila Participates in Myotube Guidance. EMBO J. 15, 1642–1649. doi:10.1002/j.1460-2075.1996.tb00509.x
Gargano, J., Martin, I., Bhandari, P., and Grotewiel, M. (2005). Rapid Iterative Negative Geotaxis (RING): a New Method for Assessing Age-Related Locomotor Decline in. Exp. Gerontol. 40, 386–395. doi:10.1016/j.exger.2005.02.005
Geraldo, S., and Gordon-Weeks, P. R. (2009). Cytoskeletal Dynamics in Growth-Cone Steering. J. Cel Sci 122, 3595–3604. doi:10.1242/jcs.042309
Ghazi, A., Paul, L., and VijayRaghavan, K. (2003). Prepattern Genes and Signaling Molecules Regulate Stripe Expression to Specify Drosophila Flight Muscle Attachment Sites. Mech. Develop. 120, 519–528. doi:10.1016/s0925-4773(03)00042-x
Girdler, G. C., and Röper, K. (2014). Controlling Cell Shape Changes during Salivary Gland Tube Formation in Drosophila. Semin. Cel Develop. Biol. 31, 74–81. doi:10.1016/j.semcdb.2014.03.020
Hao, I., Green, R. B., Dunaevsky, O., Lengyel, J. A., and Rauskolb, C. (2003). The Odd-Skipped Family of Zinc finger Genes Promotes Drosophila Leg Segmentation. Develop. Biol. 263, 282–295. doi:10.1016/j.ydbio.2003.07.011
Harzer, H., Berger, C., Conder, R., Schmauss, G., and Knoblich, J. A. (2013). FACS Purification of Drosophila Larval Neuroblasts for Next-Generation Sequencing. Nat. Protoc. 8, 1088–1099. doi:10.1038/nprot.2013.062
Hasson, P. (2011). “Soft” Tissue Patterning: Muscles and Tendons of the Limb Take Their Form. Dev. Dyn. 240, 1100–1107. doi:10.1002/dvdy.22608
Havis, E., Bonnin, M.-A., Olivera-Martinez, I., Nazaret, N., Ruggiu, M., Weibel, J., et al. (2014). Transcriptomic Analysis of Mouse Limb Tendon Cells during Development. Development 141, 3683–3696. doi:10.1242/dev.108654
Hayashi, S., and Kondo, T. (2018). Development and Function of the Drosophila Tracheal System. Genetics 209, 367–380. doi:10.1534/genetics.117.300167
Huang, A. H. (2017). Coordinated Development of the Limb Musculoskeletal System: Tendon and Muscle Patterning and Integration with the Skeleton. Develop. Biol. 429, 420–428. doi:10.1016/j.ydbio.2017.03.028
Huang, A. H., Riordan, T. J., Pryce, B., Weibel, J. L., Watson, S. S., Long, F., et al. (2015). Musculoskeletal Integration at the Wrist Underlies Modular Development of Limb Tendons. Development 142, 2431–2441. doi:10.1242/dev.122374
Huang, A. H., Watson, S. S., Wang, L., Baker, B., Akiyama, H., Brigande, J. V., et al. (2019). Requirement for Scleraxis in the Recruitment of Mesenchymal Progenitors during Embryonic Tendon Elongation. Development 146, dev182782. doi:10.1242/dev.182782
Kardon, G., Campbell, J. K., and Tabin, C. J. (2002). Local Extrinsic Signals Determine Muscle and Endothelial Cell Fate and Patterning in the Vertebrate Limb. Develop. Cel 3, 533–545. doi:10.1016/S1534-5807(02)00291-5
Kardon, G. (2011). Development of the Musculoskeletal System: Meeting the Neighbors. Development 138, 2855–2859. doi:10.1242/dev.067181
Kardon, G., Harfe, B. D., and Tabin, C. J. (2003). A Tcf4-Positive Mesodermal Population Provides a Prepattern for Vertebrate Limb Muscle Patterning. Develop. Cel 5, 937–944. doi:10.1016/S1534-5807(03)00360-5
Kerman, B. E., Cheshire, A. M., Myat, M. M., and Andrew, D. J. (2008). Ribbon Modulates Apical Membrane during Tube Elongation through Crumbs and Moesin. Develop. Biol. 320, 278–288. doi:10.1016/j.ydbio.2008.05.541
Kesavan, G., Sand, F. W., Greiner, T. U., Johansson, J. K., Kobberup, S., Wu, X., et al. (2009). Cdc42-mediated Tubulogenesis Controls Cell Specification. Cell 139, 791–801. doi:10.1016/j.cell.2009.08.049
Korzelius, J., Naumann, S. K., Loza-Coll, M. A., Chan, J. S., Dutta, D., Oberheim, J., et al. (2014). Escargot Maintains Stemness and Suppresses Differentiation in Drosophila Intestinal Stem Cells. EMBO J. 33, 2967–2982. doi:10.15252/embj.201489072
Kramer, S. G., Kidd, T., Simpson, J. H., and Goodman, C. S. (2001). Switching Repulsion to Attraction: Changing Responses to Slit during Transition in Mesoderm Migration. Science 292, 737–740. doi:10.1126/science.1058766
Kult, S., Olender, T., Osterwalder, M., Markman, S., Leshkowitz, D., Krief, S., et al. (2021). Bi-fated Tendon-To-Bone Attachment Cells Are Regulated by Shared Enhancers and KLF Transcription Factors. eLife 10, e55361. doi:10.7554/eLife.55361
Laddada, L., Jagla, K., and Soler, C. (2019). Odd-skipped and Stripe Act Downstream of Notch to Promote the Morphogenesis of Long Appendicular Tendons in Drosophila. Biol. Open 8, bio038760. doi:10.1242/bio.038760
Lahaye, L. L., Wouda, R. R., de Jong, A. W. M., Fradkin, L. G., and Noordermeer, J. N. (2012). WNT5 Interacts with the Ryk Receptors Doughnut and Derailed to Mediate Muscle Attachment Site Selection in Drosophila melanogaster. PLOS ONE 7, e32297. doi:10.1371/journal.pone.0032297
Laurichesse, Q., and Soler, C. (2020). Muscle Development : a View from Adult Myogenesis in Drosophila. Semin. Cel Develop. Biol. 104, 39–50. doi:10.1016/j.semcdb.2020.02.009
Liu, H., Xu, J., Liu, C.-F., Lan, Y., Wylie, C., and Jiang, R. (2015). Whole Transcriptome Expression Profiling of Mouse Limb Tendon Development by Using RNA-Seq. J. Orthop. Res. 33, 840–848. doi:10.1002/jor.22886
Luschnig, S., Bätz, T., Armbruster, K., and Krasnow, M. A. (2006). Serpentine and Vermiform Encode Matrix Proteins with Chitin Binding and Deacetylation Domains that Limit Tracheal Tube Length in Drosophila. Curr. Biol. 16, 186–194. doi:10.1016/j.cub.2005.11.072
Maqbool, T., Soler, C., Jagla, T., Daczewska, M., Lodha, N., Palliyil, S., et al. (2006). Shaping Leg Muscles in Drosophila: Role of Ladybird, a Conserved Regulator of Appendicular Myogenesis. PLoS ONE 1, e122. doi:10.1371/journal.pone.0000122
Maruyama, R., and Andrew, D. J. (2012). Drosophila as a Model for Epithelial Tube Formation. Dev. Dyn. 241, 119–135. doi:10.1002/dvdy.22775
Mathew, S. J., Hansen, J. M., Merrell, A. J., Murphy, M. M., Lawson, J. A., Hutcheson, D. A., et al. (2011). Connective Tissue Fibroblasts and Tcf4 Regulate Myogenesis. Development 138, 371–384. doi:10.1242/dev.057463
McClure, K. D., and Schubiger, G. (2007). Transdetermination: Drosophila Imaginal Disc Cells Exhibit Stem Cell-like Potency. Int. J. Biochem. Cel Biol. 39, 1105–1118. doi:10.1016/j.biocel.2007.01.007
Miao, G., and Hayashi, S. (2016). Escargot Controls the Sequential Specification of Two Tracheal Tip Cell Types by Suppressing FGF Signaling in Drosophila. Development 143, 4261–4271. doi:10.1242/dev.133322
Miller, A. (1950). “The Internal Anatomy and Histology of the Imago of Drosophila melanogaster,” in Biology of Drosophila (New York: John Wiley & Sons), 420–531.
Mohan, R., and John, A. (2015). Microtubule-associated Proteins as Direct Crosslinkers of Actin Filaments and Microtubules. IUBMB Life 67, 395–403. doi:10.1002/iub.1384
Nassari, S., Duprez, D., and Fournier-Thibault, C. (2017). Non-myogenic Contribution to Muscle Development and Homeostasis: The Role of Connective Tissues. Front. Cel Dev. Biol. 5, 22. doi:10.3389/fcell.2017.00022
Ni, J.-Q., Liu, L.-P., Binari, R., Hardy, R., Shim, H.-S., Cavallaro, A., et al. (2009). A Drosophila Resource of Transgenic RNAi Lines for Neurogenetics. Genetics 182, 1089–1100. doi:10.1534/genetics.109.103630
Nourissat, G., Berenbaum, F., and Duprez, D. (2015). Tendon Injury: from Biology to Tendon Repair. Nat. Rev. Rheumatol. 11, 223–233. doi:10.1038/nrrheum.2015.26
Okenve-Ramos, P., and Llimargas, M. (2014). A Role for Fascin in Preventing Filopodia Breakage in Drosophila Tracheal Cells. Communicative Integr. Biol. 7, e972846. doi:10.4161/cib.29741
Ordan, E., Brankatschk, M., Dickson, B., Schnorrer, F., and Volk, T. (2015). Slit Cleavage Is Essential for Producing an Active, Stable, Non-diffusible Short-Range Signal that Guides Muscle Migration. Development 142, 1431–1436. doi:10.1242/dev.119131
Orgeur, M., Martens, M., Leonte, G., Nassari, S., Bonnin, M.-A., Börno, S. T., et al. (2018). Genome-wide Strategies Identify Downstream Target Genes of Connective Tissue-Associated Transcription Factors. Development 145, dev161208. doi:10.1242/dev.161208
Perkins, L. A., Holderbaum, L., Tao, R., Hu, Y., Sopko, R., McCall, K., et al. (2015). The Transgenic RNAi Project at Harvard Medical School: Resources and Validation. Genetics 201, 843–852. doi:10.1534/genetics.115.180208
Pfeiffer, B. D., Jenett, A., Hammonds, A. S., Ngo, T.-T. B., Misra, S., Murphy, C., et al. (2008). Tools for Neuroanatomy and Neurogenetics in Drosophila. Proc. Natl. Acad. Sci. 105, 9715–9720. doi:10.1073/pnas.0803697105
Prokop, A., Martı́n-Bermudo, M. D., Bate, M., and Brown, N. H. (1998). Absence of PS Integrins or Laminin A Affects Extracellular Adhesion, but Not Intracellular Assembly, of Hemiadherens and Neuromuscular Junctions inDrosophilaEmbryos. Develop. Biol. 196, 58–76. doi:10.1006/dbio.1997.8830
Pueyo, J. I., and Couso, J. P. (2005). Parallels between the Proximal-Distal Development of Vertebrate and Arthropod Appendages: Homology without an Ancestor. Curr. Opin. Genet. Develop. 15, 439–446. doi:10.1016/j.gde.2005.06.007
Rhee, D. Y., Cho, D.-Y., Zhai, B., Slattery, M., Ma, L., Mintseris, J., et al. (2014). Transcription Factor Networks in Drosophila melanogaster. Cel Rep. 8, 2031–2043. doi:10.1016/j.celrep.2014.08.038
Rinon, A., Lazar, S., Marshall, H., Büchmann-Møller, S., Neufeld, A., Elhanany-Tamir, H., et al. (2007). Cranial Neural Crest Cells Regulate Head Muscle Patterning and Differentiation during Vertebrate Embryogenesis. Development 134, 3065–3075. doi:10.1242/dev.002501
Rodriguez, O. C., Schaefer, A. W., Mandato, C. A., Forscher, P., Bement, W. M., and Waterman-Storer, C. M. (2003). Conserved Microtubule-Actin Interactions in Cell Movement and Morphogenesis. Nat. Cel Biol 5, 599–609. doi:10.1038/ncb0703-599
Schaefer, A. W., Kabir, N., and Forscher, P. (2002). Filopodia and Actin Arcs Guide the Assembly and Transport of Two Populations of Microtubules with Unique Dynamic Parameters in Neuronal Growth Cones. J. Cel Biol 158, 139–152. doi:10.1083/jcb.200203038
Schnorrer, F., and Dickson, B. J. (2004). Muscle Building. Develop. Cel 7, 9–20. doi:10.1016/j.devcel.2004.06.010
Schnorrer, F., Kalchhauser, I., and Dickson, B. J. (2007). The Transmembrane Protein Kon-Tiki Couples to Dgrip to Mediate Myotube Targeting in Drosophila. Develop. Cel 12, 751–766. doi:10.1016/j.devcel.2007.02.017
Schott, S., Ambrosini, A., Barbaste, A., Benassayag, C., Gracia, M., Proag, A., et al. (2017). A Fluorescent Toolkit for Spatiotemporal Tracking of Apoptotic Cells in Living Drosophila Tissues. Development 144, 3840–3846. doi:10.1242/dev.149807
Schweitzer, R., Zelzer, E., and Volk, T. (2010). Connecting Muscles to Tendons: Tendons and Musculoskeletal Development in Flies and Vertebrates. Development 137, 2807–2817. doi:10.1242/dev.047498
Soler, C., Daczewska, M., Da Ponte, J. P., Dastugue, B., and Jagla, K. (2004). Coordinated Development of Muscles and Tendons of the Drosophilaleg. Development 131, 6041–6051. doi:10.1242/dev.01527
Soler, C., Laddada, L., and Jagla, K. (2016). Coordinated Development of Muscles and Tendon-like Structures: Early Interactions in the Drosophila Leg. Front. Physiol. 7, 22. doi:10.3389/fphys.2016.00022
Staudt, N., Molitor, A., Somogyi, K., Mata, J., Curado, S., Eulenberg, K., et al. (2005). Gain-of-Function Screen for Genes that Affect Drosophila Muscle Pattern Formation. Plos Genet. 1, e55. doi:10.1371/journal.pgen.0010055
Svitkina, T. M., Bulanova, E. A., Chaga, O. Y., Vignjevic, D. M., Kojima, S.-i., Vasiliev, J. M., et al. (2003). Mechanism of Filopodia Initiation by Reorganization of a Dendritic Network. J. Cel Biol 160, 409–421. doi:10.1083/jcb.200210174
Swan, L. E., Wichmann, C., Prange, U., Schmid, A., Schmidt, M., Schwarz, T., et al. (2004). A Glutamate Receptor-Interacting Protein Homolog Organizes Muscle Guidance in Drosophila. Genes Dev. 18, 223–237. doi:10.1101/gad.287604
Szikora, S., Földi, I., Tóth, K., Migh, E., Vig, A., Bugyi, B., et al. (2017). The Formin DAAM Is Required for Coordination of the Actin and Microtubule Cytoskeleton in Axonal Growth Cones. J. Cel Sci 130, 2506–2519. doi:10.1242/jcs.203455
Tarazona, O. A., Lopez, D. H., Slota, L. A., and Cohn, M. J. (2019). Evolution of Limb Development in Cephalopod Mollusks. Elife 8, e43828. doi:10.7554/eLife.43828
Tiwari, P., Kumar, A., Das, R. N., Malhotra, V., and VijayRaghavan, K. (2015). A Tendon Cell Specific RNAi Screen Reveals Novel Candidates Essential for Muscle Tendon Interaction. PLoS ONE 10, e0140976. doi:10.1371/journal.pone.0140976
Usui, K., Pistillo, D., and Simpson, P. (2004). Mutual Exclusion of Sensory Bristles and Tendons on the Notum of Dipteran Flies. Curr. Biol. 14, 1047–1055. doi:10.1016/j.cub.2004.06.026
Valdivia, M., Vega-Macaya, F., and Olguín, P. (2017). Mechanical Control of Myotendinous Junction Formation and Tendon Differentiation during Development. Front. Cel Dev. Biol. 5, 26. doi:10.3389/fcell.2017.00026
Vining, M. S., Bradley, P. L., Comeaux, C. A., and Andrew, D. J. (2005). Organ Positioning in Drosophila Requires Complex Tissue-Tissue Interactions. Develop. Biol. 287, 19–34. doi:10.1016/j.ydbio.2005.08.017
Volk, T., and VijayRaghavan, K. (1994). A central Role for Epidermal Segment Border Cells in the Induction of Muscle Patterning in the Drosophila Embryo. Development 120, 59–70. doi:10.1242/dev.120.1.59
Vorbrüggen, G., and Jäckle, H. (1997). Epidermal Muscle Attachment Site-specific Target Gene Expression and Interference with Myotube Guidance in Response to Ectopic Stripe Expression in the Developing Drosophila Epidermis. Proc. Natl. Acad. Sci. 94, 8606–8611. doi:10.1073/pnas.94.16.8606
Wang, X., Zhang, M. W., Kim, J. H., Macara, A. M., Sterne, G., Yang, T., et al. (2015). The Kruppel-like Factor Dar1 Determines Multipolar Neuron Morphology. J. Neurosci. 35, 14251–14259. doi:10.1523/JNEUROSCI.1610-15.2015
Watson, S. S., Riordan, T. J., Pryce, B. A., and Schweitzer, R. (2009). Tendons and Muscles of the Mouse Forelimb during Embryonic Development. Dev. Dyn. 238, 693–700. doi:10.1002/dvdy.21866
Wu, X., Chen, Z., Gao, Y., Wang, L., Sun, X., Jin, Y., et al. (2018). The Krüppel-like Factor Dar1 Restricts the Proliferation of Drosophila Intestinal Stem Cells. Febs J. 285, 3945–3958. doi:10.1111/febs.14652
Yarnitzky, T., Min, L., and Volk, T. (1997). The Drosophila Neuregulin Homolog Vein Mediates Inductive Interactions between Myotubes and Their Epidermal Attachment Cells. Genes Dev. 11, 2691–2700. doi:10.1101/gad.11.20.2691
Keywords: tubulogenesis, leg disc, tendon, myogenesis, krüppel-like factor, dar1, cytoskeleton, drosophila
Citation: Laurichesse Q, Moucaud B, Laddada L, Renaud Y, Jagla K and Soler C (2021) Transcriptomic and Genetic Analyses Identify the Krüppel-Like Factor Dar1 as a New Regulator of Tube-Shaped Long Tendon Development. Front. Cell Dev. Biol. 9:747563. doi: 10.3389/fcell.2021.747563
Received: 26 July 2021; Accepted: 29 November 2021;
Published: 16 December 2021.
Edited by:
Enrique Martin-Blanco, Institute of Molecular Biology of BarcelonaSpainCopyright © 2021 Laurichesse, Moucaud, Laddada, Renaud, Jagla and Soler. This is an open-access article distributed under the terms of the Creative Commons Attribution License (CC BY). The use, distribution or reproduction in other forums is permitted, provided the original author(s) and the copyright owner(s) are credited and that the original publication in this journal is cited, in accordance with accepted academic practice. No use, distribution or reproduction is permitted which does not comply with these terms.
*Correspondence: Cédric Soler, Y2VkcmljLnNvbGVyQHVkYW1haWwuZnI=