- 1The State Key Laboratory Breeding Base of Basic Science of Stomatology and Key Laboratory for Oral Biomedicine of Ministry of Education, School and Hospital of Stomatology, Wuhan University, Wuhan, China
- 2Fujian Key Laboratory of Developmental and Neuro Biology, College of Life Science, Fujian Normal University, Fuzhou, China
- 3Division of Rheumatology, Department of Medicine, University of Massachusetts Medical School, Worcester, MA, United States
- 4Department of Periodontology, School of Stomatology, Wuhan University, Wuhan, China
Embryonic development and stem cell differentiation are orchestrated by changes in sequential binding of regulatory transcriptional factors to their motifs. These processes are invariably accompanied by the alternations in chromatin accessibility, conformation, and histone modification. Odontoblast lineage originates from cranial neural crest cells and is crucial in dentinogenesis. Our previous work revealed several transcription factors (TFs) that promote odontoblast differentiation. However, it remains elusive as to whether chromatin accessibility affects odontoblast terminal differentiation. Herein, integration of single-cell RNA-seq and bulk RNA-seq revealed that in vitro odontoblast differentiation using dental papilla cells at E18.5 was comparable to the crown odontoblast differentiation trajectory of OC (osteocalcin)-positive odontogenic lineage. Before in vitro odontoblast differentiation, ATAC-seq and H3K27Ac CUT and Tag experiments demonstrated high accessibility of chromatin regions adjacent to genes associated with odontogenic potential. However, following odontoblastic induction, regions near mineralization-related genes became accessible. Integration of RNA-seq and ATAC-seq results further revealed that the expression levels of these genes were correlated with the accessibility of nearby chromatin. Time-course ATAC-seq experiments further demonstrated that odontoblast terminal differentiation was correlated with the occupation of the basic region/leucine zipper motif (bZIP) TF family, whereby we validated the positive role of ATF5 in vitro. Collectively, this study reports a global mapping of open chromatin regulatory elements during dentinogenesis and illustrates how these regions are regulated via dynamic binding of different TF families, resulting in odontoblast terminal differentiation. The findings also shed light on understanding the genetic regulation of dentin regeneration using dental mesenchymal stem cells.
Introduction
Cell fate specification is achieved through spatiotemporal gene expression during embryonic development, tissue regeneration, or cell reprogramming (Spitz and Furlong, 2012). A set of tissue-specific transcription factors (TFs) regulate these genes at the transcriptional level. They potentially recognize and interact with their specific DNA-binding motifs in the genome to drive lineage-specific gene expression at different developmental stages (Lee and Young, 2013). Moreover, TFs are master regulators in gene regulatory networks (GRNs) to establish competency for different cell fates (Davidson, 2010). However, a majority of potential DNA-binding sites are inaccessible because the genomic DNA in eukaryotic cells is occluded by higher-order chromatin structures (Luger et al., 1997). Within this context, gene regulation occurs at gene regulatory regions in the opened chromatin, which allows for the binding of TFs and functioning of RNA polymerase (Calo and Wysocka, 2013). These types of opened chromatin regions, such as active promoters or enhancers, are characterized by histone modifications that flank nucleosome-free regions, including H3K4 methylation and H3K27 acetylation (Creyghton et al., 2010). The mechanism by which TFs recognize their binding motifs or influence the chromatin accessibility to initiate different biological processes remains largely unknown.
Dental papilla cells are cranial neural crest-derived mesenchymal populations, which form odontoblasts and dentin. Numerous TFs play crucial roles in odontoblastic differentiation via the regulation of gene expression programs, such as RUNX2, DLX3, SOX9, SOX2, and KLF6 (Li et al., 2011; Wang et al., 2014; Yang G. et al., 2017; Yang Y. et al., 2017; Chen et al., 2021). In our recent studies, three zinc-finger TFs, KLF4, SALL1, and ZEB1, have been revealed to regulate odontoblastic differentiation via different mechanisms. KLF4 regulates Dmp1 and Sp7 transcription through modulation of histone acetylation. Interaction of SALL1 with RUNX2 directly activates TGF-β2 to regulate the commitment of odontoblast lineages. Besides, ZEB1 alters the chromatin accessibility of cis-elements adjacent to genes including Runx2 in the early stage and directly promotes Dspp transcription in the late stage (Tao et al., 2019; Xiao et al., 2021b; Lin et al., 2021). The previous findings affirm that chromatin accessibility is associated with odontoblast terminal differentiation. However, a global view of the interaction of lineage-determining TFs with dynamic changes in chromatin accessibility during odontoblast cell fate specification is elusive.
To fill this knowledge gap, we validated that postnatal day 0 (PN0) OC (osteocalcin)-positive odontogenic lineage in the first lower molar tooth germ mainly contributes to the crown odontoblast layer, which served as a potential in vivo odontoblast differentiation model. We employed this in vivo model and the frequently adopted in vitro odontoblast differentiation model to comprehensively analyze the mechanism of chromatin accessibility in odontoblast terminal differentiation.
Materials and methods
Animal maintenance
All C57BL/6 mouse experiments used for mDPC culture and subsequent ATAC-seq and RNA-seq were performed under the guideline and approval of the Institutional Animal Care and Use Committees at the School and Hospital of Stomatology attached to Wuhan University (protocol no.S07920070I). The OC-Cre (Zhang et al., 2013) and Rosa26-mTmG (Muzumdar et al., 2007) alleles used in this study were described. All experiments involving these two lines were performed with the approval of the Institutional Animal Care and Use Committees at the School of Life Science in Fujian Normal University (protocol no. 20210007). All animal experiments were performed in accordance to the ARRIVE guidelines 2.0.
Cell culture
We isolated primary dental papilla mesenchymal cells (mDPCs) from embryonic day 18.5 (E18.5) first molar tooth germ. A dissection needle was employed to remove the dental epithelium after digestion using 0.75 mg/ml of dispase (Becton, Dickinson and Co., Franklin Lakes, NJ, USA). The tooth germ was isolated from the mandible using forceps, dispersed, and digested with 0.25% trypsin-EDTA (Life Technologies, Carlsbad, CA, USA) at 37°C for 15 min. Cells were cultured in Dulbecco’s modified Eagle medium (DMEM; Hyclone, Pittsburgh, PA, USA). To induce mineralization, mDPCs between passage 2 and 4 were cultured in an induction medium supplemented with 50 μg/ml of ascorbic acid (Sigma, St. Louis, MO, USA), 10 mmol/L sodium-glycerophosphate, and 10 nmol/L dexamethasone (Sigma). All the cells used in this study were maintained in 5% CO2 at 37°C, and the medium was replenished every 2 days.
Plasmid construction dual-luciferase assay, and transfection
For enhancer activity assay, DNA fragments synthesized by Sangon Biotech (Shanghai, China) were inserted into the pGL3-promoter vector. Phusion® polymerase (NEB, USA) was used to clone the full-length of open-reading frame of ATF5, and subcloned into pcDNA3.1 (+). For dual-luciferase assay, we cotransfected mDPCs with pGL3 vector along with the pRL-TK plasmid and ATF5-OE plasmid or pcDNA3.1 (+) using Lipofectamine 2000 (Life Technologies). Four days posttransfection, the dual-luciferase assay was performed using the luciferase Assay System (Promega) following the protocol of the manufacturer. Triplicate wells were analyzed. Firefly luciferase activity from the whole-cell lysates was normalized using Renila activity internal control.
Lentivirus-expressing short-hairpin RNA (shRNA) against Atf5 (shAtf5) (top strand: GATCCGCGGGAGATCCAGTACGTGAATTCAAGAGATTCACGTACTGGATCTCCCGCTTTTTTG; bottom strand: AATTCAAAAAAGCGGGAGATCCAGTACGTGAATCTCTTGAATTCACGTACTGGATCTCCCGCG) and empty control (control) were generated from HanBio (HanBio, China). mDPC at passage was infected with lentivirus, 4 days after which knockdown efficiency was validated using qRT-PCR.
Single-cell RNA-Seq and downstream analysis
Single-cell RNA-seq was performed on the 10x Chromium platform using Chromium Single Cell 3′ Library and Gel Bead Kit v3 (10x Genomics; Annoroad Genomics, China). Following the quality check, the DNA library was sequenced on the Illumina Novaseq 6000 (Illumina, Annoroad Genomics, China). Filtered reads were mapped to mm10 transcriptome using Cell Ranger v3.0 (10x Genomics) (Zheng et al., 2017). The Seurat package (v3.0) (Stuart et al., 2019) was employed in R for downstream analysis. Briefly, raw count matrices were filtered to remove barcodes with less than 500 genes expressed; more than 8,000 genes expressed, and a there was a high percentage of UMIs from the mitochondria (>10%), leaving 6,720 cells for the first round of clustering. Counts were normalized and scaled using the SCTransform function (Hafemeister and Satija, 2019). The first round of dimension reduction and clustering was performed using “dim = 1:30.” Dmp1+/GFP+ (GFP >1) served as a terminal odontoblast cluster for the OC-positive population. Considering the distance between other clusters and this cluster, we subset all clusters with “nearby” as odontogenic lineage for further analysis. Finally, 975 cells were picked as OC-positive odontogenic lineage. Dimension reduction and clustering were performed for the second round, and clusters were visualized using tSNE plots. We reconstructed cell differentiation trajectories using Monocle (Trapnell et al., 2014) (v3.0) from the Seurat object above. All cluster information was inherited from Seurat. Based on counts of genes in each cell, cell trajectories were imputed using the “order_cells” function. Distribution of regulons was generated in SCENIC packages (v 1.1.2) (Aibar et al., 2017) in R.
Transposase-accessible chromatin assay with high-throughput sequencing
We cultured mDPCs in a mineralization medium and normal culture medium for 0, 3, 5, 7, and 9 days. OC-positive and OC-negative tooth germ cells were harvested as follows: The mandible of PN0 OC-Cre and Rosa26-mTmG was treated with dispase to allow for the removal of dental epithelium using dissection needles. Fine forceps were then applied to isolate the tooth germ. To obtain a single-cell suspension, tooth germ was disassociated with trypsin and subjected to FACS sorting to acquire 105 OC-positive and 105 OC-negative cells. ATAC-seq libraries were prepared as described by Buenrostro et al. (2013) and indexed using a TruePrep DNA Library Prep Kit (TD501, Vazyme, Nanjing, China). Approximately 50,000 cells in each biological replicate were harvested and dissociated using a cell strainer via centrifugation (750 × g for 5 min) at room temperature. The cell pellets were resuspended in lysis buffer (10 mM Tris-HCl, pH 7.5, 10 mM NaCl, 3 mM MgCl2, 0.1% NP-40), then centrifuged at 500 × g for 15 min at 4°C. The supernatant was discarded. The pelleted nuclei were immediately submitted to tagmentation reaction using Tn5 transposase (TTE Mix V50) for 30 min at 37°C. DNA purified using the Qiagen PCR purification MinElute Kit (Qiagen, Valencia, CA, USA) was eluted in 10 μl of elusion buffer, indexed, and amplified. All libraries were cleaned using VAHTS DNA Clean Beads and sequenced on the Illumina Novaseq 6000 (Illumina, provided by Annoroad Genomics, China). Three independent biological replicates were performed for each mDPC-D0 and D9, whereas two independent biological replicates were performed for each time point in the time-course ATAC-seq. However, one replicate was performed for OC-positive and OC-negative cells ATAC-seq.
Cleavage under targets and tagmentation library preparation
We cultured mDPCs in a mineralization medium and normal culture medium for 0 and 9 days. Cleavage under targets and tagmentation (CUT and Tag) libraries were prepared as previously described by Kaya-Okur et al. (2019) and indexed using an In-Situ ChIP Library Prep Kit (TD902, Vazyme, Nanjing, China). Approximately 10,000 mDPCs in each biological replicate were harvested and centrifuged (600 × g) at room temperature for 3 min. The supernatant was washed and resuspended in Wash Buffer supplemented with protease inhibitors (Roche Complete Protease Inhibitor EDTA-Free Tablet, Sigma-Aldrich, St. Louis, MO, USA). Concanavalin A-coated magnetic beads in each sample were washed and resuspended in binding buffer. Then beads were added to the cells, gently vortexed, and incubated in a shaker for 10 min at room temperature. The unbound supernatant was removed. The bead-bound cells were resuspended in precooling antibody buffer [2 mM EDTA, 0.1% BSA in DIG Wash Buffer (0.05% digitonin in wash buffer)] and incubated with (1:50 dilution) primary antibody against H3K27ac (ab4729, Abcam, Cambridge, MA, USA) in a shaker overnight at 4°C. The primary antibody on the magnet stand was removed, and then a secondary antibody (Guinea Pig Anti-Rabbit IgG antibody, 611-201-122, Rockland Immunochemicals, PA, USA) was diluted (1:100) in DIG Wash buffer and incubated with cells at room temperature for 1 h. Cells were washed in DIG Wash buffer using the magnet stand to remove unbound antibodies. A dilution of hyperactive pA-Tn5 transposon complex (0.04 µM) was prepared in DIG-300 buffer supplemented with 0.01% digitonin and protease inhibitors. Then cells were incubated with pA-Tn5 transposon complex in a shaker at room temperature for 1 h. Subsequently, cells were resuspended in tagmentation buffer (10 mM MgCl2 in DIG-300 buffer) and incubated at 37°C for 1 h. To terminate tagmentation, we added 10 µl of 0.5 M EDTA, 3 µl of 10% SDS, and 2.5 µl of 20 mg/ml proteinase K to each sample, followed by overnight incubation at 37°C. Purified DNA was amplified and indexed. The libraries were cleaned using VAHTS DNA Clean Beads (N411, Vazyme, Nanjing, China) and sequenced on the Illumina Novaseq 6000 (Illumina, provided by Annoroad Genomics, China). We applied 150-bp pair-end sequencing with a sequencing depth of 6G base pair raw data (generated approximately 20 million mapped paired reads). Three independent biological replicates were performed for each mDPC-D0 and D9.
Cleavage under targets and tagmentation and Transposase-accessible chromatin assay with high-throughput sequencing library analyses
Raw reads of CUT and Tag and ATAC-seq were first subjected to trimmomatic (v.0.38) (Bolger et al., 2014) for adaptor trimming. We did a quality check using FastQC (https://www.bioinformatics.babraham.ac.uk/projects/fastqc/) before alignment to ensure proportionate quality libraries. Then the paired-end sequencing reads were aligned to the mouse genome (mm10) using Bowtie 2 (Langmead and Salzberg, 2012). SAMtools (Li et al., 2009) was applied to eliminate the PCR duplicates. DeepTools2 (Ramírez et al., 2016) was used to generate bigwig files. MACS2 (v.2.1.1) (Zhang et al., 2008) was applied for peak calling. Comparison of differentially accessible NFRs between different time points was achieved using DiffBind (DESeq2 v.1.26.0) (Love et al., 2014). We further applied the Homer package (Heinz et al., 2010) to identify the de novo motifs enriched in the NFRs of different mineralization time points. Genomic Regions Enrichment of Annotations Tool (GREAT) (McLean et al., 2010) was adopted to annotate differentially accessible NFRs and perform GO enrichment assay. Eventually, coverage plots for CUT and Tag and ATAC-seq results were generated using DeepTools2 and uploaded to the UCSC genome browser. All correlative graphs were plotted using R scripts in RStudio (v.February 1, 5001). For footprint, mapped reads at the groups were concatenated and subjected to CENTIPEDE (Pique-Regi et al., 2011), and cutting frequency near Dmp1 3′UTR was then visualized. Example scripts were uploaded to Github (https://github.com/Badgerliu/mDPC_epi_paper). All scripts are available upon request.
Integration of transposase-accessible chromatin assay with high-throughput sequencing and H3K27Ac cleavage under targets and tagmentation data
To identify odontoblast stage-specific active enhancers, we integrated H3K27Ac CUT and Tag peaks to ATAC-seq at the same time point following our previously published strategy (Liu et al., 2020). The regions flanked by two adjacent H3K27Ac peaks less than 1,500 bp were defined as “H3K27Ac-flanked” regions. D0- or D9-enriched NFRs were “intersected” with D0- or D9-enriched H3K27Ac peaks or “H3K27Ac flanked” regions using bedtools. The overlapped NFRs were termed as D0- or D9-enriched active enhancers.
Immunohistochemistry
The isolated mandibles of wild-type C57BL/6 mouse at PN2 (for anti-ATF5) and OC-Cre, and Rosa26-mTmG (for OC-positive lineage tracing) were fixed in 4% paraformaldehyde at 4°C for 24 h. The samples were then treated in 10% ethylenediaminetetraacetic acid (EDTA) for 1–2 days, dehydrated, and embedded in paraffin. Sagittal sections (5-µm thick) were dewaxed and rehydrated. After being boiled in 1 mM citrate buffer (pH = 6.0) for 15 min, slides were cooled down to room temperature. Subsequently, histological sections were blocked in bovine serum albumin (BSA) (Biosharp, China) and incubated with antibodies against GFP (1:100, Cat. No. ab13970, Lot No. GR89472–15; Abcam, MA, USA) or ATF5 (1:100; Cat. No.ab184923, Lot No. GR282324-13; Abcam, MA, USA) at 4°C overnight. For regular immune chemical color reaction, samples were reacted with polymer Helper and poly-HRP-anti-rabbit IgG or poly-HRP-anti-goat IgG at room temperature for 15 min after they were washed with phosphate-buffered saline (PBS). The samples were detected using a diaminobenzidine (DAB) reagent kit (Maixin) and counterstained with hematoxylin. For immunofluorescent analysis, we performed anti-GFP staining in Alexa 633 (anti-Chicken lgY, 1:500, Cat. No. A21103, Lot No. 2079359; Thermo Fisher Scientific, MA, USA) and counterstained with DAPI (Life Technologies, USA). Pseudocolor was performed using Fiji (Schindelin et al., 2012) to convert color of Alexa633 to GFP.
Quantitative reverse transcriptase PCR analysis
Total RNA from cells was extracted using HP Total RNA Kit (Omega biotech, Norcross, GA, USA), then reverse transcribed into cDNA using Reverse Transcription System (Life Technologies). qPCR was performed in CFX Connect Real-Time PCR system (Bio-RAD) using ChamQ SYBR qPCR Master Mix (Vazyme). Gapdh, Atf5, Alp, and Dmp1 were quantified with Gapdh as the internal normalization control. The RNA expression ratio was denoted as “mean ± standard deviation” from three independent biological replicates.
Western blot analysis
After different treatments, mDPCs were lysed in lysis buffer (Feiyi Technology, China) and centrifuged at 13,000 rpm for 10 min at 4°C. Total protein was quantified. After that, Western blot was performed with the following antibodies.
DMP1 (1:1,000; Cat. No. ab103203, Lot No. GR3212251-2; Abcam, MA, USA), DSP (1:1,000; Cat. No. NBP1-91612, Lot No. QC6694; NOVUSBIO, CO, USA), ATF5 (1:2,000; Cat. No. ab184923, Lot No. GR282324-13; Abcam, MA, USA), and β-ACTIN (1:8,000; BioPM, Beijing, China).
Statistical analysis
All results were presented as “mean ± standard deviation (SD).” One-way ANOVA was performed for multiple group comparisons, whereas a two-tailed t-test was performed for two groups. Values of p < 0.05 were considered statistically significant.
Results
In vitro odontoblastic differentiation resembles crown odontoblast differentiation trajectory of OC-positive odontogenic cells.
Previous studies on odontoblast differentiation mainly employed an in vitro model using mouse dental papilla cells (mDPCs) induced by a mineralization medium. They reported significant upregulation of Dmp1, Dspp (Chen et al., 2009), and Nestin (Nes) (Kaukua et al., 2014), which are marker genes mainly expressed in the odontoblast layer [Nes is also expressed in the pericytes in dental pulp (Yianni and Sharpe, 2018)]. However, the same medium could also potentially induce osteogenic differentiation when bone marrow-derived mesenchymal cells (BMSCs) are used (Yu et al., 2021). Thus, whether this model can mimic in vivo odontoblast differentiation remains unclear. To clarify this, we first attempted to compare the transcriptomes of in vitro with in vivo odontoblast differentiation models (Figure 1A). Inspired by the specific Cre activity in the odontoblast layer of OC-Cre mice (Yun et al., 2016), we generated OC-Cre and Rosa26-mTmG mice to assess odontoblast differentiation in vivo. GFP activity was mainly localized at the odontoblast layer (Supplementary Figures 2A, D) and dental follicle cells in developing tooth root at postnatal day 0 (PN0) in the first lower molar. Also, some blood vessels were GFP positive (Supplementary Figure 2C). We isolated GFP-positive cells from PN0 first lower molar tooth germ of OC-Cre and Rosa26-mTmG mice, and performed single-cell RNA-seq. Considering the initial cluster and expression of Dmp1 and Cd34, we isolated 975 cells associated with Dmp1, which was termed as “OC-positive odontogenic lineage.” Six clusters were revealed (Figure 1B). Following the assessment of the significant differential marker genes (minimal percentage as 25% and p < 0.01; Supplementary Table 1), the cell distribution was revealed to resemble a subset of population single-cell RNA-seq reported from the apical halves of molar at PN7.5 (Wen et al., 2020). Clusters 1 and 2 were identified as dental papilla cells, expressing high levels of Slc20a2, Osr2, and Dlx3 (Figures 1C, E; Supplementary Figures 3, 4) (Yamashiro et al., 2003). Cluster 5 was termed as odontoblast with explicitly high expression of Dmp1, Nes, and Dspp (Figures 1C, F; Supplementary Figures 3 and 4). Runx2, Mmp13, and Bmp3 were highly enriched in cluster 6 (Figures 1C, G; Supplementary Figures 3 and 4); they were previously identified to be highly associated with dental follicle cells (Wen et al., 2020). Clusters 3 and 4 were regarded as transient status from OC-positive dental papilla cells to odontoblasts or dental follicle cells; they exhibited gradually low expression of Slc20a2 and Msx2. Differentiation trajectory of these OC-positive odontogenic lineages based on gene expression changes was inferred (Figure 1D). Dental papilla cells (clusters 1 and 2) were the start point, whereas two destinations were crown odontoblasts (cluster 5) and dental follicle cells (cluster 6). These results demonstrated that OC-positive odontogenic lineage contributed to crown odontoblasts and dental follicle cells.
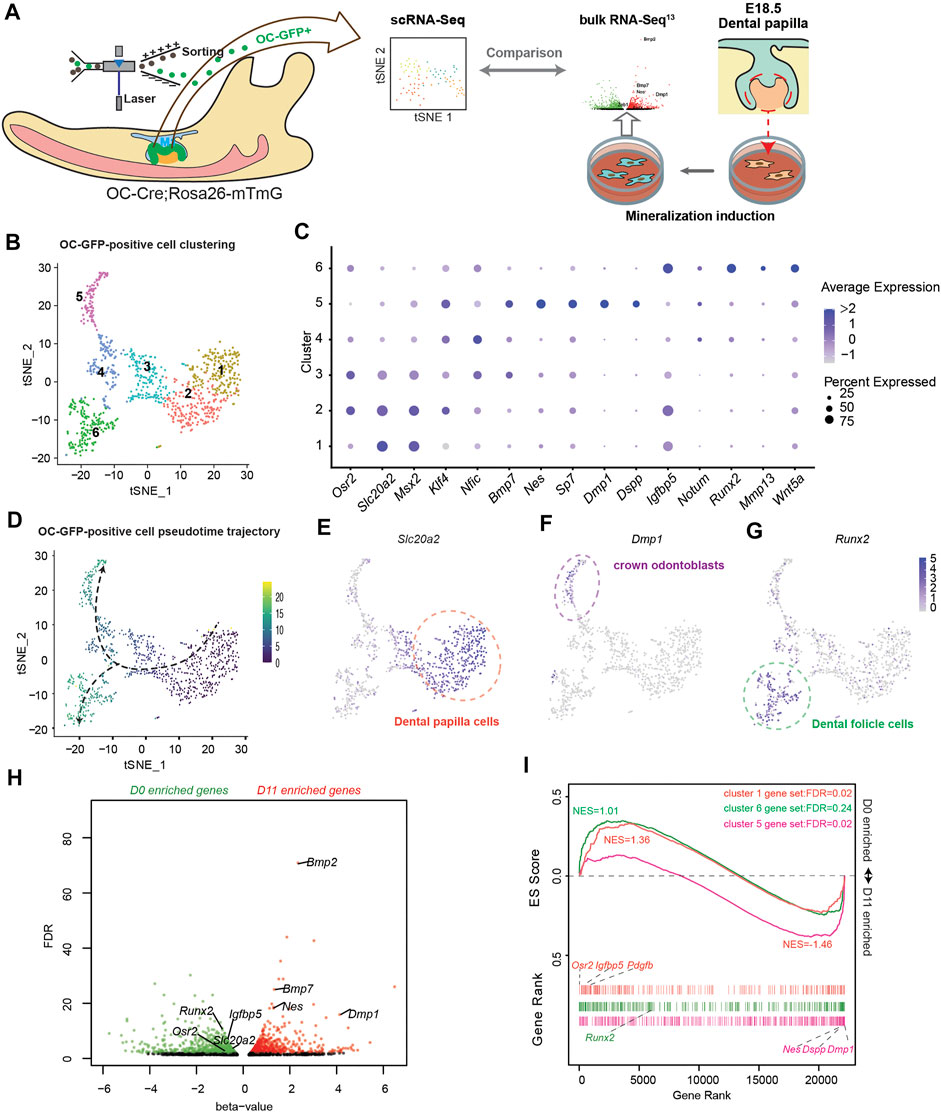
FIGURE 1. Comparison between in vitro odontoblastic differentiation and in vivo osteocalcin (OC)-positive odontogenic lineage differentiation. (A) Study design comparing single-cell RNA-seq (scRNA-seq) using OC + odontogenic lineage from postnatal day 0 (PN0) first lower molar tooth germ and bulk RNA-seq of dental papilla cells before and after mineralization induction. (B) t-distributed stochastic neighbor embedding (tSNE) depicting the clustering of 975 single-cell transcriptional profiles obtained from FACS-sorted GFP-positive cells from OC-Cre and Rosa26-mTmG PN0 first lower molar tooth germ. (C) Dot-plot showing expression and enrichment of selected top genes identified in each cluster. The size of dot indicate the percentage of cells per cluster. (D) Pseudotime-trajectory of OC-GFP-positive scRNA-seq. (E) Slc20a2 marks pre-odontoblast population in OC + odontogenic lineage. (F) Dmp1 marks crown odontoblast population in OC + odontogenic lineage. (G) Runx2 marks dental follicle cell population in OC + odontogenic lineage. (H) Volcano scatter plot showing the differentially expressed genes revealed in bulk RNA-seq from dental papilla cells before (D0) and after (D11) mineralization induction. Red dots indicate genes significantly enriched in D11, and green dots indicate genes significantly enriched in D0. (I) Gene set enrichment analysis (GSEA) using marker genes from cluster 1 (orange), cluster 6 (green), and cluster 5 (violet) as gene sets comparing expression profiles from D0 and D11 bulk-RNA-seq. NES, normalized enrichment score; FDR, false discovery rate, as generated in GSEA.
To make a transcriptome-wide comparison between in vivo and in vitro odontoblast differentiation, we adopted our previously published bulk RNA-seq profile using mDPCs treated with mineralization medium for 0 days (D0) and 11 days (D11) (Lin et al., 2021) (Figure 1H). Zeb1 (Xiao et al., 2021a) and Sall1 (Lin et al., 2021) were upregulated on day 11 and promoted odontoblastic differentiation. Also, the enrichment of Osr2, Slc20a2, and Runx2 on D0 was not found in the crown odontoblast population (cluster 5) in the scRNA-seq profile. We applied gene set enrichment analysis (GSEA) with marker genes in clusters 1, 5, or 6 as three independent gene sets and found that genes enriched on D11 were positively correlated with cluster 5 gene set (NES = −1.46, FDR = 0.02, no. of permutations = 10,000), whereas D0 was positively correlated with cluster 1 gene set (NES = 1.36, FDR = 0.02). However, genes in the cluster 6 set exhibited an even distribution in both D0 and D11 (NES = 1.01) (Figure 1I) indicating it was not like the in vitro differentiation. These findings suggested that in vitro odontoblast differentiation using mDPCs from E18.5 concurs with in vivo odontoblast differentiation of OC-positive odontogenic lineage.
Identification of odontoblast-specific active enhancers
Activation and deactivation of cis-regulatory elements have been directly associated with transcriptional regulation (Heinz et al., 2010). We explored whether there are such elements that regulate transcription during odontoblast differentiation. The above in vitro and in vivo models were employed for ATAC-seq to identify open chromatin regions before and after odontoblast differentiation. The open chromatin regions in the in vitro models were annotated using H3K27Ac CUT and Tag to reveal active enhancers (Figure 2A). We found 27,484 and 26,215 nucleosome-free regions (NFRs) enriched in OC-positive and OC-negative cells (Figure 2B). We compared the ATAC-seq reads from in vitro odontoblast differentiation and found no significant difference between D0 and D11 ATAC-seq in the OC-positive enriched NFRs. However, the overall D0 and D11 ATAC-seq signals in the OC-positive enriched NFRs were significantly higher than those in OC-negative ones (p < 0.001, by Kolmogorov Smirnov test, ks-test) (Supplementary Figure 6A). Considering the transcriptome comparison between in vitro and in vivo differentiation, this is reasonable given that OC-positive odontogenic lineage covers the transition from D0 to D11. Besides, the shift in chromatin accessibility from D0 to D11 reflected detailed changes of cis-regulatory elements during odontoblast terminal differentiation. We, therefore, chose this in vitro model for further analysis.
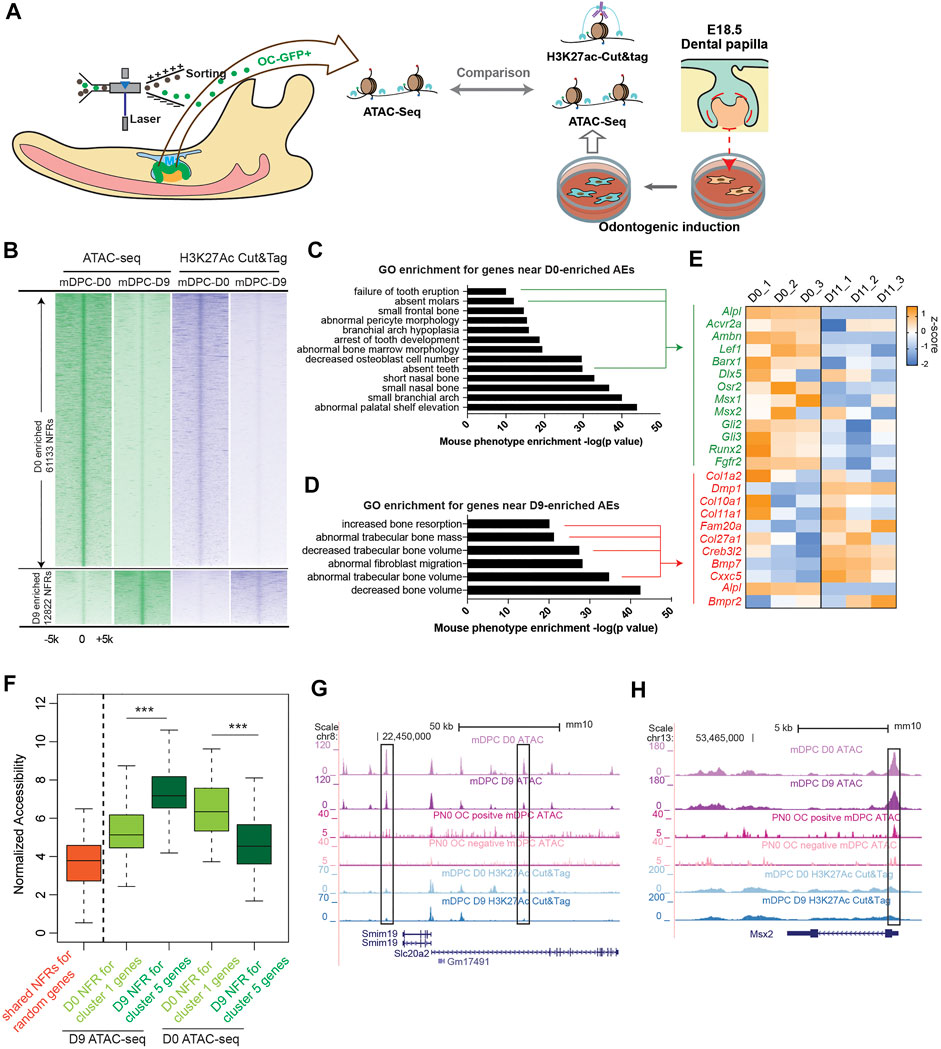
FIGURE 2. Transposase-accessible chromatin assay with high-throughput sequencingATAC-seq) and H3K27Ac cleavage under targets and tagmentation (CUT and Tag) from both in vitro and in vivo odontoblast differentiation revealed chromatin early shaping predetermined dentinogenesis potential of dental papilla cells. (A) Study design comparing ATAC-seq from in vitro and in vivo odontoblast differentiation and annotation for active enhancers using H3K27Ac CUT and Tag. (B) Density plot of aligned ATAC-seq and H3K27Ac CUT and Tag peaks differentially enriched in D0 and D9 during mineralization of E18.5 dental papilla cells. Each line is centered on the nucleosome-free region (NFR) with significantly more reads in D0 or D9 ATAC-seq. Reads in H3K27Ac CUT and Tag are aligned to the same NFR. Gene ontology enrichment using “Mouse Phenotype Single KO” for D0- (C) and D9- (D) enriched active enhancers (AEs). (E) Expression changes of the overlapped genes from bulk RNA-seq profile in indicated GO terms visualized in heatmap. (F) Plot of accessibility scores (generated in DiffBind) of elements with differential accessibility associated with genes differentially expressed in cluster 1 and cluster 5, showing elements with increased accessibility in D9-in-vitro-differentiated odontoblast tend to be associated with genes enriched in cluster 5, and vice versa. ***p < 0.001, by Kolmogorov–Smirnov test. (G, H) UCSC genome browser view showing ATAC-seq and CUT and Tag peaks near the Slc20a2 and Msx2 loci.
As for the ATAC-seq from in vitro odontoblast differentiation model, there were 61,133 NFRs enriched in D0 (before mineralization induction) and 12,822 enriched in D9 [log2 (fold change) >0.5 or <0.5, FDR <0.01, by DESEQ2; Supplementary Tables 2 and 3]. The enrichment of ATAC-seq reads correlated with H3K27Ac CUT and Tag (Figure 2B and Supplementary Figures 7A, B). The NFRs overlapped or flanked by H3K27Ac signals at the same stage were defined as active enhancers (AEs); also, we found 12,221 AEs on D0 and 2,461 on D9 (Supplementary Figure 7C and Tables 4 and 5). In several regions near Dmp1 locus (Supplementary Figure 7D), the accessibility remained unchanged or decreased post odontoblast differentiation. However, the H3K27Ac CUT and Tag signals were significantly elevated. This is common during the establishment of enhancer activity: H3K27Ac modification follows the “opening” of chromatin regions (Calo and Wysocka, 2013). We analyzed the GO enrichment for the associated genes of D0- and D9-enriched NFRs in “Mouse phenotype single KO.” Genes associated with D0-enriched NFRs were more enriched in odontogenic-related GO terms such as “failure of tooth eruption” and “absent teeth” (Figure 2C), including Acvr2a, Lef1, and Msx1. The expressions of these genes were low in D11 according to the RNA-seq profile. Transient transfection-based dual-luciferase assay in mDPCs confirmed elements near Msx1 (Msx1-0.6; mm10 chr5: 37,824,275–37,824,941) and Fgfr2 (Fgfr2+96; mm10 chr7: 130,167,270–130,167,658; and Fgfr2-34; chr7: 130,298,850–130,299,791) exhibited higher enhancer activity on D0 compared with D11, despite the possible loss of plasmids during odontoblast differentiation (Supplementary Figure 8). Moreover, genes associated with D9-enriched NFRs were highly associated with mineralization GO terms, such as “increased bone resorption” and “decreased trabecular bone volume/mass” (Figure 2D). RNA-seq profile demonstrated that the genes, Dmp1 and Creb3l2, were also significantly upregulated on D11 (Figure 2E). We performed in vitro validation and found that two elements targeting these genes (Col1a2-8.3; chr6: 4,497,230–4,497,895; and Bmp7+67; chr2: 172,872,129–172,872,743) exhibited high enhancer activity (Supplementary Figure 9).
We also found that differentially accessible NFRs associated with cluster 5 genes (in scRNA-seq) had significantly higher average accessibility from mDPCs D9 than those from mDPCs D0, and vice versa (Figure 2F). Particularly, Msx2 and Slc20a2 were highly expressed in cluster 1, and there were more intense signals of ATAC-seq along with H3K27Ac in the mDPC D0 group (Figures 2G,H). Collectively, this analysis suggested that chromatin accessibility was associated with the terminal differentiation of odontoblast.
Time-course ATAC-seq reveals that dynamic changes in open chromatin regions predetermine terminal differentiation of odontoblast.
Because NFRs enriched in mDPC-D9 groups are associated with odontoblast terminal differentiation, we explored whether these regions were regulated by any specific TFs. We performed a more detailed time-course ATAC-seq during in vitro odontoblast differentiation at 2-day intervals (Figure 3A). Following the analysis of differentially enriched NFRS in each group, we found that the accessibility of NFRs changed dramatically from D0 to D3 (Figure 3B), much earlier than the upregulation of odontoblast-specific genes, Dmp1 and Dspp, which are always, in most cases, increased around day 5 or day 7 post-induction (Tao et al., 2019). There were 5,526 NFRs differentially enriched between the D0 and D3 group, 48 between D3 and D5, 131 between D5 and D7, and no different NFRs between D7 and D9 (Supplementary Figure 10). High-resolution analysis across all the merged consensus peaks from D0 to D9 using k-means clustering revealed four clusters of elements with two major trends of chromatin accessibility, with the NFRs in clusters 1, 2, and 4 gradually “closed”, and cluster 3 gradually “open” (Figure 3C). The promoter of Gli1 (Supplementary Figure 11A) and an element near Klf4 (Supplementary Figure 11B) gradually lost accessibility during differentiation. GO enrichment assay for NFRs revealed that genes near cluster 1 (including Gli1) were associated with “incisor/tooth morphology” indicating their odontogenic function, whereas genes near cluster 3 were associated with “bone mass” (Supplementary Figure 12). These underpinned cluster 3 are the very NFRs associated with odontoblast terminal differentiation, given by mineralization as the major biological process.
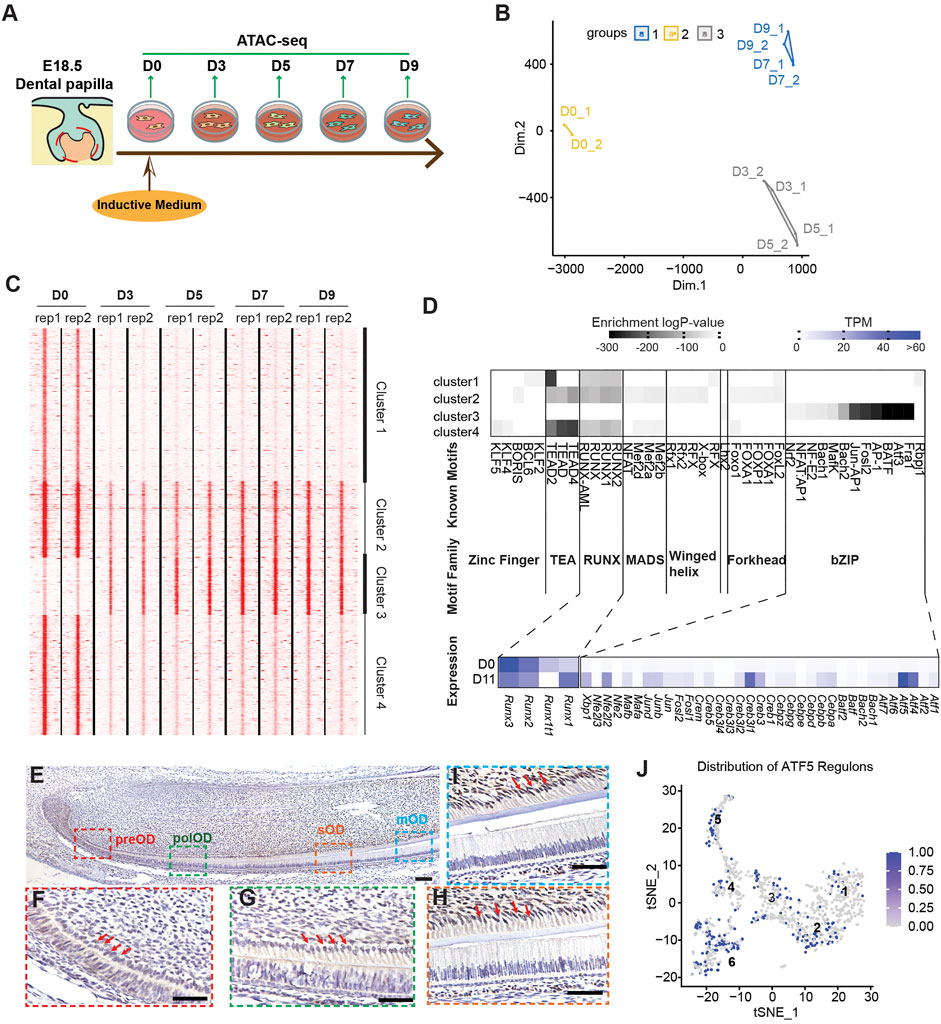
FIGURE 3. Time–course ATAC-seq integrated with RNA-seq identified transcription factors (TFs) enriched in open chromatin regions associated with odontoblast terminal differentiation. (A) Study design for time-course ATAC-seq using in vitro odontoblast differentiation. (B) A multidimensional scaling (MDS) plot showing the difference between differential-enriched NFRs among D0, D3, D5, D7, and D9 ATAC-seq. (C) k-mean clustering of differential-enriched NFRs across replicates and stages, highlighting peak density profile. Each line is centered in NFRs enriched in different stages, with expansion from −5,000 to +5,000 bp. (D) Top enriched known motifs in each cluster as predicted via Homer analysis. The color gradient indicates the log10 (p-value) of enrichment analysis over the total background peaks using binomial testing. Hierarchy clustering of TF distribution based on log10 (p-value) was depicted in the heatmap. Coincidently, most of the clustered TFs belong to a specific TF family with a similar structure, such as zinc-finger, RUNX, forkhead, or bZIP. Of note, the bZIP TFs family was specifically enriched in cluster 3-enriched NFRs. The average expression of each member in the bZIP family on D0 and D11 bulk RNA-seq profiles was exhibited in the heatmap. (E–I) Immunohistochemistry of the expression pattern of ATF5 in PN2 murine lower incisor. ATF5 expression in the pre-odontoblasts (preOD) (F), polarized odontoblasts (polOD) (G), secretory odontoblast (sOD) (H), and mature odontoblast (mOD) (I) were gradually increasing. Scale bar: 100 µm. Red arrows point to the odontoblast layer. (J) tSNE plot showing the distribution of cells with abundant ATF5-targeting genes (regulons) in OC-positive scRNA-seq profile.
We applied Homer to identify the known TFs motifs enriched in the four clusters, which allowed us to find the specific TFs that regulate NFR activity. We found a well-separated pattern of the TF family in the four clusters of NFRs. Zinc Finger, TEA, RUNX, MADS, and Forkhead TF families were exclusively enriched in clusters 1, 2, and 4, whereas the basic region/leucine zipper (bZIP) family members were enriched in cluster 3. To identify the possible missing TF members in the bZIP family attributed to the incomplete database, we examined the changes in expression level and abundances of all the recorded bZIP TF (from UniProtKB database) (UniProt, 2019) referring to our bulk RNA-seq data. Most of the bZIP family members detected in bulk RNA-seq were upregulated post odontoblast differentiation. In contrast, RUNX family members, enriched only in clusters 1, 2, and 4 were downregulated (Figure 3D). Of the bZIP family members, we selected Atf5 for further validation. IHC results revealed that ATF5 was highly expressed in the secretary odontoblasts and mature odontoblasts, but weakly expressed in the pre-odontoblast in the odontoblast layer of the lower incisor from PN0 mouse (Figures 3E–H and Supplementary Figure 14). Additionally, SECNIC imputation demonstrated that the regulons of ATF5 in the scRNA-seq profile were mainly distributed in clusters 2, 3, 4, 5, and 6, which depicted its positive role in the differentiation of odontoblast and dental follicle cells (Figure 3J). Furthermore, we scanned all the target NFRs of ATF5 motifs in cluster 3 and found that the genes associated with these NFRs mediated “ossification” and “bone development” (Supplementary Figure 13). Taken together, our time-course ATAC-seq experiments with motif analyses revealed that the dynamic changes in chromatin accessibility occur earlier than the transcriptional changes and predetermined odontoblast-related gene transcription.
ATF5 promotes odontoblastic differentiation partially by binding to a Dmp1 enhancer
We further characterized the function of ATF5 in the in vitro odontoblast differentiation model. We applied lentivirus-mediated shAtf5 transduction with scrambled plasmid as a control. qPCR and Western blot demonstrated that Atf5 knockdown significantly reduced RNA levels of Dmp1 and Alp on day-9 post induction. A significantly lower protein level of DSPP was reported in the shAtf5 group on day 9 (Figure 4A and Supplementary Figure 15). Alizarin red results demonstrated that mineralization was significantly inhibited in the Atf5-knockdown group (Figure 4B). Based on our previous analysis of the target genes of the ATF5 motif in cluster 3 NFRs, Dmp1 was selected as a target of ATF5 for further exploration. We found an element in the downstream of Dmp1 (Dmp1+13; chr5: 104,216,600–104,216,700) with ATF5 motif (Figure 4C), accessibility of which was significantly increased. CENTIPEDE-based Tn5 transposase footprinting assay (Pique-Regi et al., 2011) was performed using two concatenated replicates from mDPC D0 and D9 ATAC-seq. Despite the fact that there were more mapped reads in D0 than D9, footprint revealed a higher cut frequency of Tn5 flanking this motif on D9 mDPC ATAC-seq (Figure 4D), an implication that this site was protected by protein. Furthermore, ATF5 overexpression significantly increased the enhancer activity of Dmp1+13 (Figure 4E). Collectively, these findings affirmed that ATF5, a major TF enriched in odontoblast-related NFRs, potentially promoted odontoblast terminal differentiation partially via the induction of Dmp1-related enhancer activity.
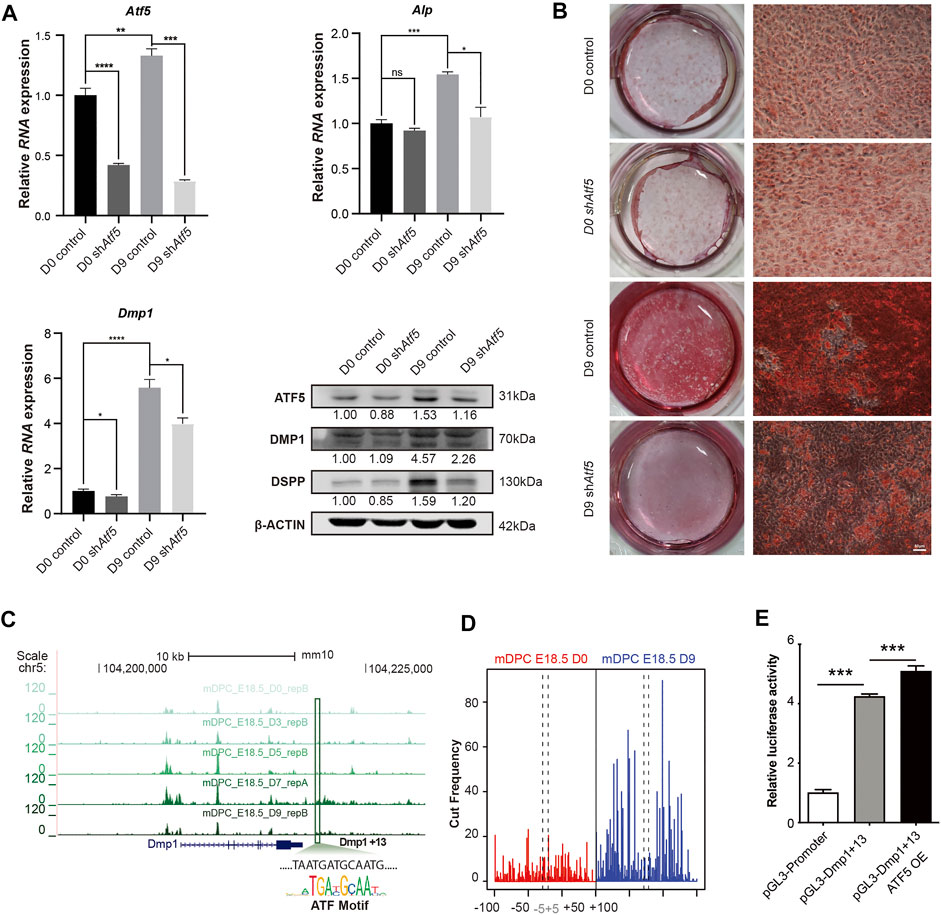
FIGURE 4. ATF5 promotes odontoblast terminal differentiation by activating a Dmp1-related enhancer. Primary mDPCs of E18.5 mice cultured in mineralization induction medium for 0 days (D0) and 9 days (D9) after infection with lentivirus-expressing shRNA against Atf5 and empty control. (A) qRT-PCR and Western blot showing expression of related odontoblast markers in different conditions. (B) Alizarin red staining showing fewer mineralization nodules on D9 in shAtf5 group compared with control. (C) A chromatin region in the downstream of Dmp1 (Dmp1+13) gradually gained accessibility (belonged to cluster 3 in Figure 3) in time–course ATAC-seq with a typical binding motif for the ATF family. (D) Tn5-transposase footprint based on CENTIPEDE revealed the ATF-binding motif in (C) “protected” on D9 ATAC-seq. (E) Dual-luciferase assay showing the enhancer activity of Dmp1+13 could be promoted by the overexpression of ATF5 in mDPC cells. *p < 0.05, **p < 0.01, ***p < 0.001.
Discussion
The mechanism by which TFs interact with chromatin to regulate gene expression and influence cell fates is one of the leading questions in the genome and developmental biology. A previous study combining in vivo genetic lineage tracing and bulk RNA-seq along with ChIP-seq against histone modifications in postnatal dental pulp perivascular-derived mesenchymal stem cells illustrated that odontoblast-specific genes, such as Dspp and Dmp1, were in a transcriptionally permissive state inhibiting by RING1B (Yianni and Sharpe, 2018). However, during embryonic development, how the fate of odontoblast lineage is initiated remained unclear. The variation in regional accessibility is the first step for chromatin landscape alternation (Calo and Wysocka, 2013). In this study, we described the transcriptome changes during odontoblast terminal differentiation via single-cell RNA-seq from OC-positive odontogenic lineage combined with bulk RNA-seq from mDPC induced by mineralization medium. Following the identification of marker genes, we found that the increase in chromatin accessibility of these markers, such as Dmp1 (Balic and Mina, 2011) and Dspp (Chen et al., 2004), occurred much earlier than the initiation of transcriptional upregulation. This integrated analysis underpinned the predecisive roles of chromatin accessibility during odontoblast terminal differentiation (Figure 5).
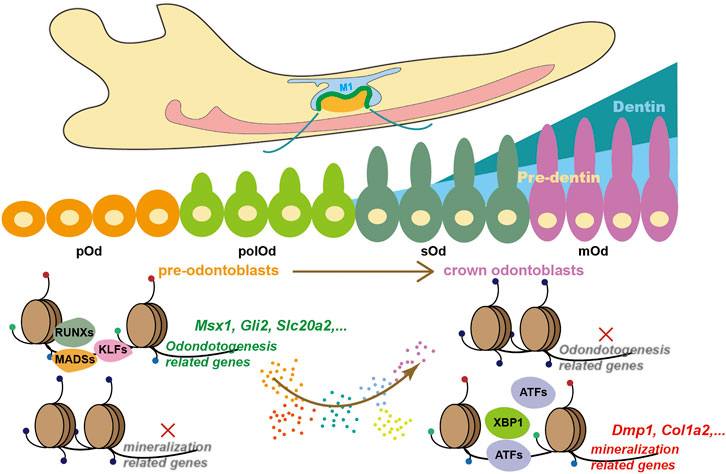
FIGURE 5. A proposed working model describing how chromatin accessibility predetermines odontoblast terminal differentiation.
At the end of differentiation, marker genes upregulated along with the enriched NFRs were associated with the major biological process of odontoblast, including GO terms such as “bone mass” and “bone volume.” Because several odontoblast markers and the same induction medium are shared with osteoblast, it is unclear whether the in vitro induction is via odontoblast or osteoblast differentiation. Dmp1 and Dspp are two marker genes for odontoblast differentiation; however, loss of either of them causes bone defect (Verdelis et al., 2008; Sun et al., 2010). Our integrated analysis between single-cell RNA-seq from odontogenic lineage and bulk RNA-seq from in vitro odontoblast induction revealed that mineralization using mDPC isolated from E18.5 lower molar is comparable with crown odontoblast differentiation at the transcriptional level. Runx2 is a positive regulator of osteoblast differentiation but is nearly undetectable after the bell stage of a tooth (Chen et al., 2009) and was significantly down-regulated during in vitro differentiation. Also, NFRs with Runx2 gradually lost accessibility during this biological process. These results imply that “downregulation of Runx2” may be a crucial aspect to differentiate between odontoblast and osteoblast differentiation. We also noticed that there were more open chromatin regions in the odontoblast in vitro at D0 than OC-positive and -negative cells. This may be due to the batch effects in sequencing and culture condition. Nevertheless, the in vivo model is the best validation method for whether a gene potentially promotes odontoblast differentiation.
Other than terminal differentiation, we revealed that NFRs near odontogenic genes, including Gli2, Msx1, and Runx2, were gradually “closed.” These genes have been extensively explored for their roles in early odontogenesis (Alappat et al., 2003), and any defect would lead to hereditary tooth abnormality (Hardcastle et al., 1999) or tooth agenesis (Mundlos et al., 1997). The loss of these odontogenic potential may be attributed by the long-term in vitro culture or the differentiation, whereby the differentiation of dental papilla cells becomes irreversible. Compelling evidence shows that dental mesenchymal cells (Yoshikawa and Kollar, 1981) or dental pulp stem cells (Hu et al., 2014) after PN0 cannot form tooth when recombined with dental epithelium before E11.5. In our recent work, we analyzed the role of Zeb1 and Sall1 in the regulation of odontoblast lineage and found that these 2 TFs can only alter odontogenic-related NFRs in mDPCs from E16.5 but not PN0 lower molars. These results suggested that despite the effect of culture, mDPCs at late embryonic stages (i.e., PN0) lose their odontogenic potential as depicted by the loss of accessibility of chromatin regions.
Assessment of transcription factor motifs enriched in ATAC-seq peaks can yield insights into the transcriptional regulatory network (Miraldi et al., 2019). Previously, we made a direct imputation on the relationship between the most possible transcription factors based on the highest expression (Liu et al., 2020). Herein, we surprisingly found that the enrichment and expression of TFs in the same family (with similar domains) exhibited a distinct stage-dependent pattern. For instance, KLF4 and KLF5, and other zinc finger TFs were enriched in clusters 1, 2, and 4 NFRs associated with the expression of odontogenic genes. We had previously revealed that other zinc-finger TFs not enriched also contribute to early chromatin accessibility maintenance, such as ZEB1 (Xiao et al., 2021a) and SALL1 (Lin et al., 2021). Given the similar binding motifs of all the transcription factors belonging to the same family, it is reasonable to hypothesize that the functional redundancy among zinc-finger TF robustly maintains the odontogenic potential. Such redundancy is common in other tissues, for instance, the function of tfap2a and tfap2c in melanocyte differentiation (Li and Cornell, 2007).
In summary, the present study outlined the landscape of chromatin accessibility during odontoblast terminal differentiation, broadening our understanding of how chromatin associates and predetermine the fate of odontoblast lineage. Also, our findings could serve as a consensus for understanding dentinogenesis using dental mesenchymal stem cells. Moreover, several TF families have been revealed and are associated with chromatin accessibility in a stage-dependent manner. ATF5, for instance, promotes this process. However, a detailed functional analysis of the interaction between a specific TF or TF family and chromatin regions (such as enhancers) needs more in vivo validation.
Data Availability Statement
The datasets presented in this study can be found in online repositories. The names of the repository/repositories and accession number(s) can be found below: National Genomic Data Center, ngdc.cncb.ac.cn, CRA004359.
Ethics Statement
The animal study was reviewed and approved by the Institutional Animal Care and Use Committees at the School and Hospital of Stomatology attached to Wuhan University (protocol no. S07920070I) and the Institutional Animal Care and Use Committees at the School of Life Science in Fujian Normal University (protocol no. 20210007).
Author Contributions
QZ contributed to the conception of the study, data acquisition, and critically revised the manuscript. ZH contributed to the conception and design of the study, data acquisition, analysis and interpretation of the data, and critically revised the manuscript. HZ contributed to the conception, data acquisition, drafting the manuscript and critical revision of the manuscript. CL, YL, YX, YC, FP, and ZC contributed to the study design and critically revised the manuscript. HL contributed to the study design, conception, data acquisition, analysis and interpretation, and drafted and critically revised the manuscript.
Funding
This work was supported by grants from the National Key R and D Project of China (2018YFC1105103) to ZC and “the Fundamental Research Funds for the Central Universities” (no. 2042021kf0197) to HL, and the National Natural Science Foundation of China (grant nos.: 81771066, 82071110, 81771057, 82071077, and 82170918). This work was also supported by the scientific research innovation program “Xiyuanjiang River Scholarship” of the College of Life Sciences, Fujian Normal University, to ZH. The numerical calculations in this paper have been done on the supercomputing system in the Supercomputing Center of Wuhan University.
Conflict of Interest
The authors declare that the research was conducted in the absence of any commercial or financial relationships that could be construed as a potential conflict of interest.
Publisher’s Note
All claims expressed in this article are solely those of the authors and do not necessarily represent those of their affiliated organizations, or those of the publisher, the editors, and the reviewers. Any product that may be evaluated in this article, or claim that may be made by its manufacturer, is not guaranteed or endorsed by the publisher.
Acknowledgments
We appreciate Xiao Yang (Academy of Military Medical Sciences) for kindly providing the OC-Cre mice.
Supplementary Material
The Supplementary Material for this article can be found online at: https://www.frontiersin.org/articles/10.3389/fcell.2021.769193/full#supplementary-material
References
Aibar, S., González-Blas, C. B., Moerman, T., Huynh-Thu, V. A., Imrichova, H., Hulselmans, G., et al. (2017). SCENIC: Single-Cell Regulatory Network Inference and Clustering. Nat. Methods 14 (11), 1083–1086. doi:10.1038/nmeth.4463
Alappat, S., Zhang, Z. Y., and Chen, Y. P. (2003). Msx Homeobox Gene Family and Craniofacial Development. Cell Res 13 (6), 429–442. doi:10.1038/sj.cr.7290185
Balic, A., and Mina, M. (2011). Identification of Secretory Odontoblasts Using DMP1-GFP Transgenic Mice. Bone 48 (4), 927–937. doi:10.1016/j.bone.2010.12.008
Bolger, A. M., Lohse, M., and Usadel, B. (2014). Trimmomatic: a Flexible Trimmer for Illumina Sequence Data. Bioinformatics 30 (15), 2114–2120. doi:10.1093/bioinformatics/btu170
Buenrostro, J. D., Giresi, P. G., Zaba, L. C., Chang, H. Y., and Greenleaf, W. J. (2013). Transposition of Native Chromatin for Fast and Sensitive Epigenomic Profiling of Open Chromatin, DNA-Binding Proteins and Nucleosome Position. Nat. Methods 10 (12), 1213–1218. doi:10.1038/nmeth.2688
Calo, E., and Wysocka, J. (2013). Modification of Enhancer Chromatin: what, How, and Why? Mol. Cel 49 (5), 825–837. doi:10.1016/j.molcel.2013.01.038
Chen, S., Gluhak-Heinrich, J., Wang, Y. H., Wu, Y. M., Chuang, H. H., Chen, L., et al. (2009). Runx2, Osx, andDsppin Tooth Development. J. Dent Res. 88 (10), 904–909. doi:10.1177/0022034509342873
Chen, S., Unterbrink, A., Kadapakkam, S., Dong, J., Gu, T. T., Dickson, J., et al. (2004). Regulation of the Cell Type-specific Dentin Sialophosphoprotein Gene Expression in Mouse Odontoblasts by a Novel Transcription Repressor and an Activator CCAAT-Binding Factor. J. Biol. Chem. 279 (40), 42182–42191. doi:10.1074/jbc.M402476200
Chen, Z., Xie, H., Yuan, J., Lan, Y., and Xie, Z. (2021). Krüppel‐like Factor 6 Promotes Odontoblastic Differentiation through Regulating the Expression of Dentine Sialophosphoprotein and Dentine Matrix Protein 1 Genes. Int. Endod. J. 54 (4), 572–584. doi:10.1111/iej.13447
Creyghton, M. P., Cheng, A. W., Welstead, G. G., Kooistra, T., Carey, B. W., Steine, E. J., et al. (2010). Histone H3K27ac Separates Active from Poised Enhancers and Predicts Developmental State. Proc. Natl. Acad. Sci. 107 (50), 21931–21936. doi:10.1073/pnas.1016071107
Davidson, E. H. (2010). Emerging Properties of Animal Gene Regulatory Networks. Nature 468 (7326), 911–920. doi:10.1038/nature09645
Hafemeister, C., and Satija, R. (2019). Normalization and Variance Stabilization of Single-Cell RNA-Seq Data Using Regularized Negative Binomial Regression. Genome Biol. 20 (1), 296. doi:10.1186/s13059-019-1874-1
Hardcastle, Z., Hui, C. C., and Sharpe, P. T. (1999). The Shh Signalling Pathway in Early Tooth Development. Cel Mol Biol (Noisy-le-grand) 45 (5), 567–578.
Heinz, S., Benner, C., Spann, N., Bertolino, E., Lin, Y. C., Laslo, P., et al. (2010). Simple Combinations of Lineage-Determining Transcription Factors Prime Cis-Regulatory Elements Required for Macrophage and B Cell Identities. Mol. Cel 38 (4), 576–589. doi:10.1016/j.molcel.2010.05.004
Hu, X., Lin, C., Shen, B., Ruan, N., Guan, Z., Chen, Y., et al. (2014). Conserved Odontogenic Potential in Embryonic Dental Tissues. J. Dent Res. 93 (5), 490–495. doi:10.1177/0022034514523988
Kaukua, N., Shahidi, M. K., Konstantinidou, C., Dyachuk, V., Kaucka, M., Furlan, A., et al. (2014). Glial Origin of Mesenchymal Stem Cells in a Tooth Model System. Nature 513 (7519), 551–554. doi:10.1038/nature13536
Kaya-Okur, H. S., Wu, S. J., Codomo, C. A., Pledger, E. S., Bryson, T. D., Henikoff, J. G., et al. (2019). CUT&Tag for Efficient Epigenomic Profiling of Small Samples and Single Cells. Nat. Commun. 10 (1), 1930. doi:10.1038/s41467-019-09982-5
Langmead, B., and Salzberg, S. L. (2012). Fast Gapped-Read Alignment with Bowtie 2. Nat. Methods 9 (4), 357–359. doi:10.1038/nmeth.1923
Lee, T. I., and Young, R. A. (2013). Transcriptional Regulation and its Misregulation in Disease. Cell 152 (6), 1237–1251. doi:10.1016/j.cell.2013.02.014
Li, H., Handsaker, B., Wysoker, A., Fennell, T., Ruan, J., Homer, N., et al. (2009). The Sequence Alignment/Map Format and SAMtools. Bioinformatics 25 (16), 2078–2079. doi:10.1093/bioinformatics/btp352
Li, S., Kong, H., Yao, N., Yu, Q., Wang, P., Lin, Y., et al. (2011). The Role of Runt-Related Transcription Factor 2 (Runx2) in the Late Stage of Odontoblast Differentiation and Dentin Formation. Biochem. Biophysical Res. Commun. 410 (3), 698–704. doi:10.1016/j.bbrc.2011.06.065
Li, W., and Cornell, R. A. (2007). Redundant Activities of Tfap2a and Tfap2c Are Required for Neural Crest Induction and Development of Other Non-neural Ectoderm Derivatives in Zebrafish Embryos. Dev. Biol. 304 (1), 338–354. doi:10.1016/j.ydbio.2006.12.042
Lin, Y., Xiao, Y., Lin, C., Zhang, Q., Zhang, S., Pei, F., et al. (2021). SALL1 Regulates Commitment of Odontoblast Lineages by Interacting with RUNX2 to Remodel Open Chromatin Regions. Stem Cells 39 (2), 196–209. doi:10.1002/stem.3298
Liu, H., Duncan, K., Helverson, A., Kumari, P., Mumm, C., Xiao, Y., et al. (2020). Analysis of Zebrafish Periderm Enhancers Facilitates Identification of a Regulatory Variant Near Human KRT8/18. eLife 9, e51325. doi:10.7554/eLife.51325
Love, M. I., Huber, W., and Anders, S. (2014). Moderated Estimation of Fold Change and Dispersion for RNA-Seq Data with DESeq2. Genome Biol. 15 (12), 550. doi:10.1186/s13059-014-0550-8
Luger, K., Mäder, A. W., Richmond, R. K., Sargent, D. F., and Richmond, T. J. (1997). Crystal Structure of the Nucleosome Core Particle at 2.8 Å Resolution. Nature 389 (6648), 251–260. doi:10.1038/38444
McLean, C. Y., Bristor, D., Hiller, M., Clarke, S. L., Schaar, B. T., Lowe, C. B., et al. (2010). GREAT Improves Functional Interpretation of Cis-Regulatory Regions. Nat. Biotechnol. 28 (5), 495–501. doi:10.1038/nbt.1630
Miraldi, E. R., Pokrovskii, M., Watters, A., Castro, D. M., De Veaux, N., Hall, J. A., et al. (2019). Leveraging Chromatin Accessibility for Transcriptional Regulatory Network Inference in T Helper 17 Cells. Genome Res. 29 (3), 449–463. doi:10.1101/gr.238253.118
Mundlos, S., Otto, F., Mundlos, C., Mulliken, J. B., Aylsworth, A. S., Albright, S., et al. (1997). Mutations Involving the Transcription Factor CBFA1 Cause Cleidocranial Dysplasia. Cell 89 (5), 773–779. doi:10.1016/s0092-8674(00)80260-3
Muzumdar, M. D., Tasic, B., Miyamichi, K., Li, L., and Luo, L. (2007). A Global Double-Fluorescent Cre Reporter Mouse. Genesis 45 (9), 593–605. doi:10.1002/dvg.20335
Pique-Regi, R., Degner, J. F., Pai, A. A., Gaffney, D. J., Gilad, Y., and Pritchard, J. K. (2011). Accurate Inference of Transcription Factor Binding from DNA Sequence and Chromatin Accessibility Data. Genome Res. 21 (3), 447–455. doi:10.1101/gr.112623.110
Ramírez, F., Ryan, D. P., Grüning, B., Bhardwaj, V., Kilpert, F., Richter, A. S., et al. (2016). deepTools2: a Next Generation Web Server for Deep-Sequencing Data Analysis. Nucleic Acids Res. 44 (W1), W160–W165. doi:10.1093/nar/gkw257
Schindelin, J., Arganda-Carreras, I., Frise, E., Kaynig, V., Longair, M., Pietzsch, T., et al. (2012). Fiji: an Open-Source Platform for Biological-Image Analysis. Nat. Methods 9 (7), 676–682. doi:10.1038/nmeth.2019
Spitz, F., and Furlong, E. E. M. (2012). Transcription Factors: from Enhancer Binding to Developmental Control. Nat. Rev. Genet. 13 (9), 613–626. doi:10.1038/nrg3207
Stuart, T., Butler, A., Hoffman, P., Hafemeister, C., Papalexi, E., Mauck, W. M., et al. (2019). Comprehensive Integration of Single-Cell Data. Cell 177 (7), 1888–1902. doi:10.1016/j.cell.2019.05.031
Sun, Y., Prasad, M., Gao, T., Wang, X., Zhu, Q., D'Souza, R., et al. (2010). Failure to Process Dentin Matrix Protein 1 (DMP1) into Fragments Leads to its Loss of Function in Osteogenesis. J. Biol. Chem. 285 (41), 31713–31722. doi:10.1074/jbc.M110.137059
Tao, H., Lin, H., Sun, Z., Pei, F., Zhang, J., Chen, S., et al. (2019). Klf4 Promotes Dentinogenesis and Odontoblastic Differentiation via Modulation of TGF‐β Signaling Pathway and Interaction with Histone Acetylation. J. Bone Miner Res. 34 (8), 1502–1516. doi:10.1002/jbmr.3716
Trapnell, C., Cacchiarelli, D., Grimsby, J., Pokharel, P., Li, S., Morse, M., et al. (2014). The Dynamics and Regulators of Cell Fate Decisions Are Revealed by Pseudotemporal Ordering of Single Cells. Nat. Biotechnol. 32 (4), 381–386. doi:10.1038/nbt.2859
UniProt, C. (2019). UniProt: a Worldwide Hub of Protein Knowledge. Nucleic Acids Res. 47 (D1), D506–D515. doi:10.1093/nar/gky1049
Verdelis, K., Ling, Y., Sreenath, T., Haruyama, N., MacDougall, M., van der Meulen, M. C. H., et al. (2008). DSPP Effects on In Vivo Bone Mineralization. Bone 43 (6), 983–990. doi:10.1016/j.bone.2008.08.110
Wang, J., Zhang, H., Zhang, W., Huang, E., Wang, N., Wu, N., et al. (2014). Bone Morphogenetic Protein-9 Effectively Induces Osteo/odontoblastic Differentiation of the Reversibly Immortalized Stem Cells of Dental Apical Papilla. Stem Cell Dev. 23 (12), 1405–1416. doi:10.1089/scd.2013.0580
Wen, Q., Jing, J., Han, X., Feng, J., Yuan, Y., Ma, Y., et al. (2020). Runx2 Regulates Mouse Tooth Root Development via Activation of WNT Inhibitor NOTUM. J. Bone Miner Res. 35 (11), 2252–2264. doi:10.1002/jbmr.4120
Xiao, Y., Lin, Y. X., Cui, Y., Zhang, Q., Pei, F., Zuo, H. Y., et al. (2021a). Zeb1 Promotes Odontoblast Differentiation in a Stage-dependent Manner. J. Dent Res. 100 (6), 648–657. doi:10.1177/0022034520982249
Xiao, Y., Lin, Y. X., Cui, Y., Zhang, Q., Pei, F., Zuo, H. Y., et al. (2021b). Zeb1 Promotes Odontoblast Differentiation in a Stage-dependent Manner. J. Dent Res. 100, 648–657. doi:10.1177/0022034520982249
Yamashiro, T., Tummers, M., and Thesleff, I. (2003). Expression of Bone Morphogenetic Proteins and Msx Genes during Root Formation. J. Dent Res. 82 (3), 172–176. doi:10.1177/154405910308200305
Yang, G., Yuan, G., MacDougall, M., Zhi, C., and Chen, S. (2017a). BMP-2 Induced Dspp Transcription Is Mediated by Dlx3/Osx Signaling Pathway in Odontoblasts. Sci. Rep. 7 (1), 10775. doi:10.1038/s41598-017-10908-8
Yang, Y., Zhao, Y., Liu, X., Chen, Y., Liu, P., and Zhao, L. (2017b). Effect of SOX2 on Odontoblast Differentiation of Dental Pulp Stem Cells. Mol. Med. Rep. 16 (6), 9659–9663. doi:10.3892/mmr.2017.7812
Yianni, V., and Sharpe, P. T. (2018). Molecular Programming of Perivascular Stem Cell Precursors. Stem Cells 36 (12), 1890–1904. doi:10.1002/stem.2895
Yoshikawa, D. K., and Kollar, E. J. (1981). Recombination Experiments on the Odontogenic Roles of Mouse Dental Papilla and Dental Sac Tissues in Ocular Grafts. Arch. Oral Biol. 26 (4), 303–307. doi:10.1016/0003-9969(81)90051-0
Yu, S., Guo, J., Sun, Z., Lin, C., Tao, H., Zhang, Q., et al. (2021). BMP2-dependent Gene Regulatory Network Analysis Reveals Klf4 as a Novel Transcription Factor of Osteoblast Differentiation. Cell Death Dis 12 (2), 197. doi:10.1038/s41419-021-03480-7
Yun, C.-Y., Choi, H., You, Y.-J., Yang, J.-Y., Baek, J.-A., and Cho, E.-S. (2016). Requirement of Smad4-Mediated Signaling in Odontoblast Differentiation and Dentin Matrix Formation. Anat. Cel Biol 49 (3), 199–205. doi:10.5115/acb.2016.49.3.199
Zhang, R., Yang, G., Wu, X., Xie, J., Yang, X., and Li, T. (2013). Disruption of Wnt/β-Catenin Signaling in Odontoblasts and Cementoblasts Arrests Tooth Root Development in Postnatal Mouse Teeth. Int. J. Biol. Sci. 9 (3), 228–236. doi:10.7150/ijbs.5476
Zhang, Y., Liu, T., Meyer, C. A., Eeckhoute, J., Johnson, D. S., Bernstein, B. E., et al. (2008). Model-based Analysis of ChIP-Seq (MACS). Genome Biol. 9 (9), R137. doi:10.1186/gb-2008-9-9-r137
Keywords: tooth development, odontogenesis, dental mesenchymal stem cells, transcription factors, epigenetics, H3K27ac, chromatin immunoprecipitation sequencing, RNA-seq
Citation: Zhang Q, Huang Z, Zuo H, Lin Y, Xiao Y, Yan Y, Cui Y, Lin C, Pei F, Chen Z and Liu H (2021) Chromatin Accessibility Predetermines Odontoblast Terminal Differentiation. Front. Cell Dev. Biol. 9:769193. doi: 10.3389/fcell.2021.769193
Received: 01 September 2021; Accepted: 29 October 2021;
Published: 25 November 2021.
Edited by:
Anne George, University of Illinois at Chicago, United StatesReviewed by:
Pierfrancesco Pagella, Linköping University, SwedenHaiting Ma, Whitehead Institute for Biomedical Research, United States
Copyright © 2021 Zhang, Huang, Zuo, Lin, Xiao, Yan, Cui, Lin, Pei, Chen and Liu. This is an open-access article distributed under the terms of the Creative Commons Attribution License (CC BY). The use, distribution or reproduction in other forums is permitted, provided the original author(s) and the copyright owner(s) are credited and that the original publication in this journal is cited, in accordance with accepted academic practice. No use, distribution or reproduction is permitted which does not comply with these terms.
*Correspondence: Zhi Chen, emhpY2hlbkB3aHUuZWR1LmNu; Huan Liu, bGl1Lmh1YW5Ad2h1LmVkdS5jbg==
†These authors have contributed equally to this work