- 1Department of Medicine, Columbia Center for Human Development, Columbia University Medical Center, New York, NY, United States
- 2Department of Surgery, Johns Hopkins University, Baltimore, MD, United States
Millions of people suffer from end-stage refractory diseases. The ideal treatment option for terminally ill patients is organ transplantation. However, donor organs are in absolute shortage, and sadly, most patients die while waiting for a donor organ. To date, no technology has achieved long-term sustainable patient-derived organ generation. In this regard, emerging technologies of chimeric human organ production via blastocyst complementation (BC) holds great promise. To take human organ generation via BC and transplantation to the next step, we reviewed current emerging organ generation technologies and the associated efficiency of chimera formation in human cells from the standpoint of developmental biology.
1 Introduction
Organ transplantation is the ultimate treatment option for patients suffering from refractory diseases. However, there has been an absolute scarcity of donor organs, and the gap between available donors vs. waitlisted recipients continues to expand (Giwa et al., 2017). In 2017, approximately 114,000 patients in the United States waited for an organ transplant (Sykes and Sachs, 2019). Presently, in the United States, another person is added to an organ transplant list every 10 min, 17 people die each day while waiting for donor organs, and approximately 105,800 patients are waitlisted for an organ transplant according to the health resources and services administration (HRSA). To overcome this significant crisis, researchers are investigating various approaches involving direct xenotransplantation, organoids, decellularization, and recellularization, and more recently, organ bioengineering using blastocyst complementation (BC). Depending on the patient’s medical condition, a refractory disease patient also requires an on-time selective option, such as less invasive cellular therapy options or curative organ transplantation that can function immediately after transplantation. In this regard, whole organ generation via the BC approach holds great promise with a ready resource (livestock) for cellular therapies and as a radical treatment option for most terminal diseases. However, though BC is emerging as a potential organ transplant option, challenges regarding organ size scalability, immune system incompatibilities, long-term maintenance, potential evolutionary distance, or unveiled mechanisms between donor and host cells remain. These challenges can be overcome by a multifaceted approach, especially by filling in the knowledge gaps on the mechanisms of interspecies chimera formation. In this review, we summarize the history of interspecies chimerism in various animal models to find hints for BC application and describe the challenges and prospects of utilizing BC for human organ generation.
2 Hybrids vs. chimeras
In ancient history, humans used the term “chimera” to describe mythical creatures and hybrids. Animals sexually derived from the fusion of gametes from two different organisms, such as mules, are considered “hybrids.” On the other hand, a chimera is defined as an organism in which cells from two or more different organisms have contributed. In nature, hybrids and chimeras are rare due to incompatibilities and developmental divergence during evolution. At the organismal level, evolutionary distance is defined by genome-wide sequence homology between species. Humans, and other species, such as mice, swine, birds, etc., have diverged from one another. A molecular program that causes the interspecific barrier might be independent of genome-wide evolution. Instead, it may depend on the host and donor molecular similarities or distinctions critical for the organogenesis program. For instance, human LIF protein can signal via LIF receptors to help maintain a high degree of stemness in mice and rats, but the converse is ineffective (Dahéron et al., 2004). How evolutionary distance affects the formation of hybrids and chimeras is an open question that may vary from situation to situation.
3 Interspecies barriers in clone technologies via somatic cell nuclear transfer (SCNT)
In 1997, a breakthrough discovery was made: Dolly the sheep. Dolly was the first mammal cloned from an adult cell using somatic cell nuclear transfer (SCNT) (Wilmut et al., 1997). This study proved that a differentiated cell has the ability to produce exact copies of its source animals. SCNT has been successfully applied to clone 24 mammalian species, including mice, cows, pigs, cats, rats, dogs, and monkeys (Rodriguez-Osorio et al., 2012). However, SCNT was not successful in interspecies attempts. The process had been tested through electro-fusion using donor somatic cell nucleus replacement into a host’s enucleated interspecies egg cytoplasm, but failed. The failure appears to be due to reprogramming elimination of the donor mitochondria by the host mitochondria, and inability to activate the donor embryogenesis program after fusion (Chang et al., 2003; Chen et al., 2003; St John and Lovell-Badge, 2007; Jiang et al., 2011). The interspecific SNCT studies imply that there are critical interspecies barriers at the subcellular level of totipotent zygotes, distinct from interspecies chimera that are at an intercellular level (Figure 1A). The study of interspecies SCNT, focusing on intracellular compartments, such as the interspecific reprogramming process, mitochondrial competition between donor and host, and genome-stability post-electrical fusion, may open new avenues to better understand subcellular interspecies barriers in SCNT.
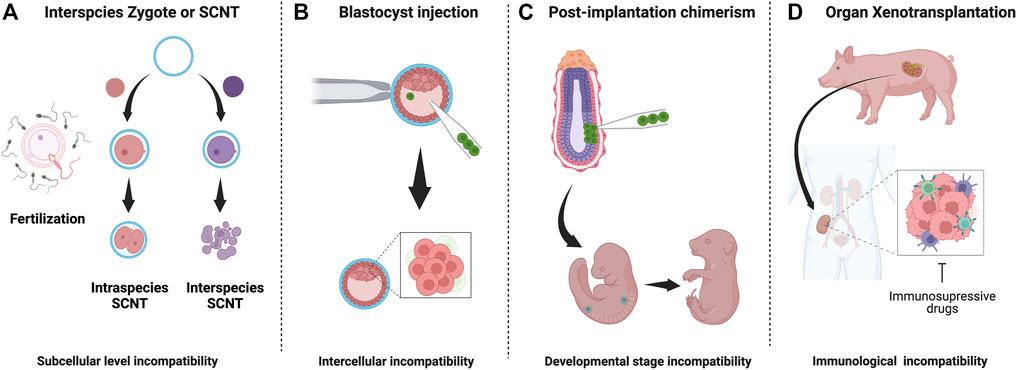
FIGURE 1. Interspecies embryo incompatibilities and barriers in interspecies chimerism: (A) Interspecies zygotes cannot reprogram and fail to develop due to subcellular level incompatibilities (B) Cell competition eliminates less fit (donor) cells donor cells in host blastocyst (C) Stage-matching of donor cells is challenging and unknown factor contribute to apoptosis of donor cells (D) Recipient immune system reacts strongly against graft tissue/organ. Despite long-term immunosuppression, organ failure occurs.
4 Interspecies barriers in chimeras
The field of chimera research emerged nearly 50 years ago, with pioneering experiments using a mixture of pluripotent stem cells (PSCs) in an intra- or interspecies manner. Briefly, cells from the Ryukyu mouse (Mus caroli), when transplanted into blastocysts of the house mouse (Mus musculus), showed very high chimerism compared to cells derived from rat blastocysts (Stanny et al., 1980). On the other hand, cells derived from the bank vole (Clethrionomys glareolus), a more evolutionary distant rodent from mice, showed no detectable chimerism even during the late embryonic stages (Mystkowska, 1975). Similar studies were also performed within closely related mammalian species such as sheep and goats. Composite blastocysts from goats and sheep could successfully generate goat-sheep chimeras (Fehilly et al., 1984). Likewise, chimeric calves produced from two closely related cow species—Bos indicus and Bos taurus, were also viable (Williams et al., 1990). These early experiments made a clear distinction between interspecies and intraspecies chimerism. In other words, the evolutionary distance between two species may be inversely correlated with the extent of chimerism (de Los Angeles and Wu, 2022).
This section reviews various interspecies chimera formation rates and chimerism during development. Chimera formation rate refers to the percentage of host animals with donor cells. Assessment of chimera formation requires checking the markers of donor cell survival, proliferation, and differentiation and proof that the donor cells are responsive to the host animal’s developmental program. Chimerism, on the other hand, indicates the % of donor cell contribution to a particular developmental niche or organism. Overall, the efficacy of intraspecies chimerism and chimera formation is far superior to that of interspecies (Table 1). Elucidation of the interspecies barrier incompatibility between host animals and human cells during development is a breakthrough in the field of human organ generation in a host animal.
4.1 Human chimeras in chick
Chicken models offer significant advantages in the study of developmental biology and chimerism. The developmental stages can be accurately determined, and embryos can be manipulated for experiments such as tissue transplantation, resection, and genetic modification (Hamburger and Hamilton, 1951; Spurlin and Lwigale, 2013; Cloney and Franz-Odendaal, 2015). Early experiments with chick embryos used intraspecific chimeras to test the vertebrate “organizer” concept and its role in gastrulation, known as embryonic differentiation. When transplanted into another, a portion of the anterior streak cells from one embryo gave rise to an ectopic neural plate comprising a neural tube, notochord, and somites (Addington, 1932; Waddington and Schmidt, 1933; Waddington, 1934). Decades later, the first evidence of avian interspecies chimerism was demonstrated by Nicole le Douarin and colleagues by engrafting quail-derived neural crest cells into the neural plate boundary of chick embryos (Couly and le Douarin, 1985). Human embryonic stem cells (hESC) transplanted into the trunk of a chick embryo were also found to differentiate into β-III tubulin-positive neurons in the host microenvironment (Goldstein, 2010). Furthermore, the neurogenic potential of hESCs was tested in mutant chick models of open neural tube defects (ONTDs). ONTDs were significantly rescued in chick embryos by the transplanted hESCs, compared to control groups, as early as post-operative Day3 (Lee et al., 2004). In another study, inoculated donor hESCs adequately contributed to the hindbrain and regions of the spinal cord within host chick embryos (Boulland et al., 2010). Similar results were also observed when hESCs were injected intra-amnionically into neural-tube defective chick embryos after 24 h of lesion induction, miming a clinical situation of human ONTDs (Lee et al., 2006). Recently, in a pioneering effort, human RUES-GLR (germ layer reporter) cells treated with Wnt3a and Activin were transplanted into chick embryos to assess their ability to serve as “organizers”. This transplantation resulted in the generation of an ectopic secondary axis within the chick embryo capable of inducing neural tissue (marked by Sox2/Sox3 expression) in the adjacent regions (Martyn et al., 2018).
These chick studies showed relatively higher chimerism than rodent-human or pig-human chimera, although the exact extent of chimerism was not described. Although the injection timing differed from BC, host chicken embryos were rescued by human PSC-derived cells. They showed differentiation markers with high chimerism beyond the blastocyst stage at the donor cell injection site. Intriguingly, early embryos of humans are discoidal shaped, closely resembling that of chicken, while mouse embryos are more cylindrical (Akhlaghpour et al., 2021). It suggests that the molecular program of early embryos may be similar between humans and chickens, probably related to the polarity and the body plan, allowing human PSCs to differentiate into neuronal lineages. On the other hand, the evolutionary distance between birds and humans is more than 300 million years, while the differences between rodents and humans are about 96 million years old (Nanda et al., 1999; Nei et al., 2001). The implanted chicken niche may have a unique niche character that secretes or expresses proteins or other molecules to overcome interspecific barriers beyond evolution. Suppose these potential mechanisms of human cell compatibility are common in all bird species. In that case, the human chimera studies with giant birds, such as ostrich (Struthio camelus), are an intriguing option for studying organ generation. However, birds harbor completely distinct anatomy and circulation systems from humans, which must be considered well. A mouse-avian chimerism examination also would be fascinating to clarify the significance of such physical constraints toward interspecific incompatibility mechanisms in early embryogenesis.
4.2 Human chimeras in mice
Chimera efficiency depends on donor cell preparation and host genetic background. Various group attempts have made dramatic progress in maintaining the pluripotency of naïve, primed, intermediated, and extended PSCs under different cell culture conditions. A naïve pluripotent state can be best described by cells within the inner cell mass of a mouse blastocyst. Cells in the morulae or 16-cell stage are considered to have totipotency or expanded potential pluripotency because they can contribute to both embryonic and extra-embryonic lineages. The concept of primed-type pluripotency is typically associated with cells within the post-implantation epiblast of mouse embryos having very low chimeric potential when injected in a host blastocyst. Lastly, the newly established concept of intermediate pluripotency refers to many possible cell states that overlap between naïve-state markers and primed-state markers. In the following section, we discuss how the chimeric potential of PSCs in various pluripotent states varies within host mouse embryos.
4.2.1 Naïve type pluripotency
Regarding donor cell preparation, scientists successfully maintained embryonic stem (ES) cells from mouse blastocysts and recognized their ability to undergo various lineage differentiation over 4 decades ago (Evans and Kaufman, 1981; Martin, 1981; Martello and Smith, 2014). In subsequent years, with refinement in culture conditions, reagents, and specific inhibitors, using a “2i” medium, the combination of a MEK inhibitor and a GK3β inhibitor, mouse PSCs can be maintained and propagated in a state of naïve pluripotency (Ying et al., 2008). For the intraspecies blastocyst injection, a donor cell naïve pluripotent state is fundamentally necessary to achieve a high degree of chimerism (Silva and Smith, 2008). Gene-targeted mouse ES cells have been successfully used in numerous studies for their ability to form germ-line chimeras (Mansour et al., 1990; Bradley, 1991; Robertson, 1991). However, the prolonged culture of PSCs in the 2i medium caused irreversible epigenetic changes leading to defects in developmental potential (Choi et al., 2017; Yagi et al., 2017). To overcome these problems, we have developed a defined media cocktail named a2i VPA LIF, which allows us to maintain PSCs with the highest chimera-forming capacity capable of forming functional lungs (Mori et al., 2019). To generate human naïve PSCs, 5iLAF, ReST, and t2iLGoY mediums have been developed (Theunissen et al., 2014; Pastor et al., 2016; Liu et al., 2017). Further comparative single-cell transcriptome analysis of the induced pluripotent stem cells (iPSCs) cultured in those media showed distinct naïve pluripotency.
4.2.2 Primed type pluripotency
There have been many efforts to generate and maintain various types of PSCs in human subjects. Human ES cells were first characterized as a primed state (Thomson et al., 1998). Unlike mice, human PSCs cannot be sustained in a naïve pluripotent state in the 2i-culture condition (Chan et al., 2013; Gafni et al., 2013; Takashima et al., 2014; Theunissen et al., 2014; Ware et al., 2014; Wu et al., 2015). This fundamental difference is likely a contributing factor behind the low chimerism of human ES in mouse embryos, examples of which are discussed below (Theunissen et al., 2014; Weinberger et al., 2016). The first evidence of human-mouse chimeras came from a study where RUES1 cells were transplanted into mouse blastocysts and examined for chimeric contribution in-vivo. Although the extent of chimerism was low, cells of human origin contributed to the prospective foregut epithelium and neuroepithelium at the E8.5 developmental stage (James et al., 2006). In a subsequent study, Hanna and colleagues reported a special 2i-LIF media supplemented with ligands FGF2 and TGFβ1, as well as inhibitors for p38 and JNK, that could confer naïve pluripotency in human ESCs and iPSCs. Additionally, they reported that cells grown in culture conditions robustly contributed to craniofacial tissues and embryonic neural folds within mouse-human chimeras (Gafni et al., 2013). Although this result was acclaimed to be a defining functional read-out for naive human pluripotency, other groups that tested this method failed to generate chimeras (Takashima et al., 2014; Theunissen et al., 2014).
4.2.3 Intermediate type pluripotency
During embryogenesis, cell competition is one of the significant interspecific incompatibility barriers that cause regional elimination of low-fitness cells, accounting for almost 35% of all cells produced via the balance between proliferation and apoptosis (Figure 1B) (Clavería and Torres, 2016; Merino et al., 2016; Bowling et al., 2019; Hashimoto and Sasaki, 2020). This mechanism, initially identified in Drosophila, involves relatively higher-MYC expressing “winner” cells to dominate the developing embryo by forcing adjacent, relatively lower-MYC “loser” cells to undergo apoptosis (de La Cova et al., 2004; Moreno and Basler, 2004; Clavería et al., 2013; Sancho et al., 2013; Díaz-Díaz et al., 2017). To avoid cell-competition-based elimination of donor cells in a chimeric epiblast, Belmonte and colleagues targeted E7.5 post-implantation mouse embryos for engrafting human PSCs and accessed chimeric efficiency (Figure 1C). By conditioning human ESCs into a region-selective primed state characterized by a transcriptome that closely matches that of a late-streak/no-bud stage mouse embryo, the authors reported efficient chimerism into endoderm, ectoderm, and mesoderm tissues (Wu et al., 2015). Mascetti and Pedersen further illustrated the idea of stage-matching hPSCs with the corresponding mouse embryonic state to enhance chimeric efficiency. However, in contrast to Belmonte’s study, there was no requirement of special pre-treatment for human PSCs before their injection into early and late gastrulation-stage embryos. Yet, primed-type cells could differentiate very efficiently into tissues derived from all three germ layers (Mascetti and Pedersen, 2016). Such disparity in experimental results highlights the extent of technical challenges and the requirement of a reliable genetic or epigenetic landmark for evaluating human PSC lines that form chimera efficiently before injection.
The state of pluripotency can have a profound influence on chimeric efficiency. For instance, primed-type PSCs corresponding to epiblast stem cells cannot form chimeras even in intraspecies contexts (Nichols and Smith, 2009). On the other hand, naïve-type cells show gene expression profiles similar to a blastocyst’s inner cell mass and can contribute to interspecies chimeras (Kobayashi et al., 2010). Although this distinction is well grounded for rodent models, the concept is not well defined in context of large animals (Rashid et al., 2014; Graham, 2017). Wu and colleagues examined all available culture conditions for primed and naïve states of human iPSCs (Wu et al., 2017). In addition to primed and naïve, human iPSCs were also characterized in an intermediate state of pluripotency, previously shown to have chimeric potential in mouse germline (Tsukiyama and Ohinata, 2014). Reporter human iPSCs were amenable to this state of pluripotency and showed significantly higher chimeric contribution in swine embryos compared to cells in other naïve or naïve-like pluripotent conditions (Wu et al., 2017). A recent study also showed that human iPSCs with intermediate pluripotency contribute to primordial germ cell specification in chimeric embryos (Yu et al., 2021).
4.2.4 Extended type pluripotency
In 2017, Deng and colleagues introduced the concept of extended pluripotency, a state of stemness that is transcriptionally distinct from conventional naïve and primed cells. An extended potential state exists between a totipotent 1-cell embryo and a blastocyst in a developing embryo. Both mouse and human extended pluripotent stem cells (EPSCs) were found to have very high chimeric potential, supported by the fact that a single mouse EPS cell could generate both embryonic and extraembryonic lineages in vivo (Yang Y. et al., 2017). Further developing this concept, human EPSCs were shown to be approximately 20 times more chimerically efficient than naïve hPSCs when injected into preimplantation mouse blastocysts (Yang Y. et al., 2017). The most recent evidence highlighted the importance of mTOR pathway inhibition in donor human PSCs enabling them to achieve a high degree of chimerism in mouse embryos (Hu et al., 2020; Zhang et al., 2021). In many of the examples discussed above, modified hPSCs were transplanted into a wild-type mouse host, and not surprisingly, the extent of chimerism was low (Freedman, 2018). Based on these results, the human donor cell naïve pluripotency requirement for achieving high chimerism in the host organism is debatable in the human-mouse developmental chimera context. This is because it is difficult to maintain the chimera-competent naïve quality of human iPSCs for the long term even in naïve maintenance medium (5iLAF, ReST, and t2iLGoY) or 2i-medium and the naïve pluripotency is profoundly distinct across the species (Silva and Smith, 2008). Intermediate or extended-type PSCs are attractive; however, substantial efforts are required for efficient chimerism (Yang J. et al., 2017; Gao et al., 2019). How to maintain an organogenic competency in human iPSC, that is, the ability to form functional organs post-injection of cells in the host animals, remains a question, and it may depend on both pluripotency and the host animal developmental program.
4.3 Human chimeras in rats
Rats have also been used to study interspecies chimerism in various contexts for developing humanized rat models, although not to the same extent as mice. Early studies in the 1980s utilized the nude rat model to study the engraftment of human skin and immune cells (Vos et al., 1980). These rats exhibited moderate immunodeficiency characterized by loss of lymphocyte counts with age but sustained high counts for neutrophils, eosinophils, and monocytes. Therefore, this model could only support adult human skin grafts for a short time before host-mediated rejection (Brüngger et al., 1984; Gilhar et al., 1986; 1990). Human CD45+ cells were detected in various rat tissues, such as the liver, thymus, kidney, spleen, etc., up to 6 months post-in-utero cord blood transplantation (Sun et al., 2007). The success of this model primarily relies on the permissive nature of the immune-deficient fetal state of rats. Recent studies have further perpetuated this concept by showing high engraftment efficiency of human iPSCs and human cancer cells in two rat models of severe combined immunodeficiency (Yang et al., 2018; He et al., 2019; Noto et al., 2020). The degree of immune surveillance for a given tissue also contributes to chimeric efficiency. Regions of the rat brain, such as the corpus striatum, when transplanted by human marrow stromal cells, show a remarkable 20% engraftment efficacy over 72 days with a lack of inflammatory responses (Azizi et al., 1998). In addition to immune aversion, various pathological conditions or tissue injury forms enhance chimerism’s chances. Neural precursors cells derived from human ES cells differentiate into neurons and glial cells in the regions of adult rat brains and spinal cords (Tabar et al., 2005; Yan et al., 2007). Human neural stem cells exert neuroprotective function in rat models of Parkinson’s disease and conditions of stroke-induced ischemia (Yasuhara et al., 2006; Bliss et al., 2007; Andres et al., 2011). Other human stem cell types, such as mesenchymal stem cells, also exhibit localized chimerism in rat organs such as the liver, heart, and pancreas (Grinnemo et al., 2004; Sato et al., 2005; Laporte et al., 2019). These studies primarily attempt to generate humanized rat models of various pathological conditions and tissue damage without using BC methods. With the emergence of new humanized rat models, the future of functional human-rat interspecies chimerism holds excellent promise, while the permissiveness of non-cancerous, normal human epithelial chimerism needs to be addressed well (Agarwal et al., 2020).
4.4 Human chimeras in monkeys
Close evolutionary relationships between monkeys and humans make them a suitable model for studying the long-term effects of interspecies organ chimerism (Gibbs et al., 2007; Stevens et al., 2013). Despite their relatively large size compared to small rodents, extended gestation period, and logistical difficulties such as labor and farming costs, monkeys can be valuable models for studying interspecies chimerism in various contexts. Takahashi and colleagues performed human ES stem cell-derived retinal tissue transplantation in two monkey models of injury-induced retinal degeneration (Shirai et al., 2016). It is well known that the odds of immune rejection decrease significantly in human-leucocyte antigen-matched iPSC transplantations (Sugita et al., 2016; Morizane et al., 2017). From this observation, Mandai and colleagues used human iPSCs to generate long-term functioning chimeric retinas in the same primate models of retinal degeneration with lower immunosuppression. In another pre-clinical study, human iPS cell-derived dopaminergic neurons showed functional engraftment in a neurotoxin-induced model of Parkinson’s disease (Kikuchi et al., 2017).
Furthermore, when used in the order of billions, human ES cell-derived cardiomyocytes can regenerate hearts in a non-human primate model of myocardial ischemia (Chong et al., 2014). Despite such success, certain aspects of monkey-human chimerism remain poorly understood. For instance, human ES cell-derived cardiovascular progenitors failed to induce remuscularization in infracted primate hearts (Zhu et al., 2018). From the developmental standpoint, Belmonte and colleagues showed the ability of human EPSCs to differentiate into hypoblast and epiblast lineages in monkey-human chimeras ex vivo (Tan et al., 2021). Attempts have also been made to generate monkeys genetically predisposed to immunodeficiency (Niu et al., 2014). Such models can be beneficial in achieving a high chimeric success of engrafted donor cells. However, using monkey-human chimeras for studying complex human brain disorders will require more cautious exploration and robust ethical modalities for crossing the interspecies barrier (Greely and Farahany, 2021). The organ size distinction between humans and monkeys also can be another significant xenobarrier for monkey-human chimeric organ generation, particularly for the solid organs that require anatomically and physiologically functional size for transplantation, such as eyes, bones, kidneys, hearts, lungs, and gastrointestinal tracts.
4.5 Human chimeras in pigs
Previously, large animals such as cattle and pigs were mainly used for developing human/animal xenograft models. The main objective was to gain mechanistic insights into graft vs. host tissue interactions that could be leveraged to achieve long-term clinical success of organ transplantation. Modeling such events in smaller animals was not feasible owing to shorter life spans and anatomical disproportionalities. Flake and colleagues showed site-specific differentiation of engrafted human mesenchymal stem cells upon transplantation into sheep embryos (Liechty et al., 2000; Almeida-Porada et al., 2004). Among large animals, pigs have been proposed as an ideal host for studying interspecies chimerism, primarily due to very close anatomical and physiological similarities to humans (Cooper et al., 2016; Nagashima and Matsunari, 2016). The following section summarizes currently available pig models and the prospects of using gene-modified pigs for xenotransplantation (Figure 1D).
5 Blastocyst complementation
“Blastocyst complementation (BC)” is a significant concept for organ generation as a regenerative approach. BC utilizes the host developmental program to incorporate donor PSCs. Donor PSCs, when injected into recipient morula or blastocysts lacking critical genes for organogenesis, can replace the defective organ niches and compensate for the functional defects of recipient cells.
Alt and colleagues first illustrated intraspecies BC when they injected wild-type mouse ES cells into RAG2-deficient blastocysts. Mature B and T cells derived from donor PSCs rescued adaptive immune responses in host mice that were niche depleted in terms of lymphocyte diversity (Chen et al., 1993). Approximately two decades later, Nakauchi and colleagues showed the first evidence of interspecies pancreatic organ generation in mice using rat PSCs (Kobayashi et al., 2010). Remarkably, they also showed that the rat pancreatic tissues generated in mice via BC were transplantable into a recipient rat disease model and rescued its phenotype (Yamaguchi et al., 2017).
Recently, this concept has been extended to large-animal interspecies, yet other barriers such as donor cell pluripotency, cell-cell competition, and other potential xenogeneic incompatibility barriers result in low chimeric efficacies (Wu et al., 2017; Garry and Garry, 2019; de Los Angeles and Wu, 2022; Roodgar et al., 2022). Chimerism during and beyond the post-implantation stage of development presents a separate set of challenges (Figure 1). Firstly, the stage-matching of donor cells into a particular tissue niche requires extensive optimization to ensure the proliferation of engrafted cells or tissues (Cohen et al., 2018; Thomas et al., 2021). Secondly, donor cells require well-fitness to survive cell competition (Wu and Barbaric, 2021). Finally, tissues derived from donor cells need to avoid immune rejection within transplant recipients even under treatment with immune-suppressive drugs (Lu et al., 2019). Critical barriers in interspecies chimerism corresponding to its developmental stage have been summarized in Figure 1.
5.1 Blastocyst complementation in pigs
BC to date has been performed mainly in mice. This is because it is relatively easy to create an organ niche in mice due to the well-established method of creating knockout mice and from the viewpoints of experimental time and cost efficiency. However, the clinical application of BC requires the generation of organs of a size that can be transplanted into humans. BC, with livestock, has attempted to overcome this problem. With newer developments in humanized animal models and an increasingly better understanding of pluripotency across various species, interspecies BC is one of the most promising bioengineering approaches for generating transplantable human organs (Figure 2A) (Garry and Garry, 2021).
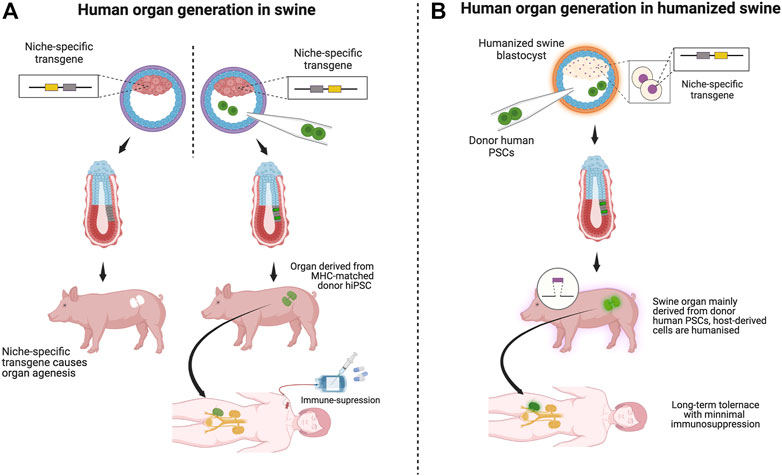
FIGURE 2. Scenarios of organ transplantation derived from various swine models: Schematic: [(A), left] In BC, vacant organ niche formation by a niche-specific transgene depletion through genome editing is essential. [(A), right] Injection of human patient-derived (or MHC-matched human) iPS cells (green) into a blastocyst of a host animal with an organ failure phenotype. [(A), right, and (B)]. (A) Transplantation of human organs by BC is unlikely to fail because most cells are of human origin. Immunosuppression may be necessary if the porcine host origin remains in the graft component. (B) Organs transplanted from human-derived cells created in humanized pigs are assumed to function long-term in recipients with minimal or no immunosuppression because humanized pigs are designed to minimize immune rejection. Using humanized swine as host animals for the BC will work as a safety guard system for immune rejection.
Before the advent of CRISPR-Cas9 genome editing technology, pigs had been mass-edited over several decades by classical genome editing methods to accommodate immune systems similar to humans. With the success of CRISPR-Cas9 and its related genome editing technologies, pig-human chimera formation and intraspecies conditional BC approaches, the potential to grow human-size organs in swine is no longer a distant dream (Matsunari et al., 2013; Chang et al., 2018; Hamanaka et al., 2018; Mori et al., 2019; Kitahara A. et al., 2020; Kobayashi et al., 2021; Ruiz-Estevez et al., 2021; Wen et al., 2021). Yet, this will require a three-pronged approach—1) establishment of high chimera competent-donor human ESCs/iPSCs that can survive and propagate within the pig embryo, and 2) generation of a vacant organ niche in swine using CRISPR-Cas9 genome editing. When the pig embryo can genetically be engineered to form a defective organ niche in the targeted organs, cells of human origin can show localized and targeted chimerism to form the respective organ (Rashid et al., 2014; Kano et al., 2022).
Nakauchi and colleagues have successfully created donor-derived pancreas in PDX1 knockout (KO) apancreatic pigs by BC (Matsunari et al., 2013). This article showed the generation of host pig embryos harboring apancreatic phenotype via SCNT, and Kusabira Orange+ pig blastomeres complemented the vacant niche. In another study, Nagashima and colleagues generated various organogenesis-disabled phenotypes in organs such as kidneys, liver, and blood vessels by targeting genes like KDR (FLK1), SALL1, and HHEX1, respectively (Matsunari et al., 2020). Recently, Zhou and colleagues successfully complemented the eye using wild-type blastomeres in pig eye deficiency by the mutation of the MITF gene in the host swine (Yao et al., 2021). Garry and colleagues injected human iPSCs into ETV2 KO pig embryos (Das et al., 2020). Although human cells can incorporate into porcine host blastocysts, many embryos initially rejected human iPSCs. They overcame this issue by overexpressing BCL2, an anti-apoptotic gene, in donor human iPSCs. Remarkably, all TIE2+ endothelial cells were derived from human PSCs in chimeric pig embryos at gestation days 17–18 (Das et al., 2020). This group also reported efficient complementation of TP53 KO human iPSCs in skeletal muscles of MYF5, MYOD, and MYF6 KO pig embryos at two distinct developmental stages—Gestation days 20 and 27 (Maeng et al., 2021). However, the degree of chimerism in these embryos was highly variable, suggesting that interspecies BC needs further optimization and technical consistency.
5.2 Blastocyst complementation in large animals
BC methodology in large animals includes swine and cattle. Sendai, Aoyagi, and colleagues generated NANOS3 KO Wagyu bovines that lack germ cells in ovaries using SCNT. They also succeeded in creating chimeric bovine ovaries capable of producing germ cells derived from donor (NANOS3+/+) Holstein blastomeres (Ideta et al., 2016). The advantage of using cattle is the similar gestation period to humans and the potential lower xenogeneic incompatibility via interspecific cell competition. Cattle also intrinsically harbor unique tissues identical to humans. For example, limb bones and muscles have better size compatibility with humans than swine. Large internal organ size may also benefit transplantation. However, cattle have lower prolific features for robust applications worldwide than swine, and long-term high-cost performance is the central issue.
In ex-vivo culture experimental systems, Belmonte and colleagues reported that human EPSCs cultured in their original medium could contribute to both embryonic and extra-embryonic lineages in ex-vivo-cultured monkey embryos. However, the contribution of human iPSCs is, at best, 7.08% for Epiblast and 4.96% for Hypoblast (Tan et al., 2021). Attempts have been made to form interspecies chimeras among large animals other than humans. Snyder and colleagues recently reported that over-expression of BCL2 enhances the proliferation of chimpanzees and pig-tailed macaque iPSCs in host rhesus blastocysts. Two days post-injection, most control iPSCs that lacked BCL2 overexpression did not survive (33.3%, chimpanzee; 54.5%, pig-tailed macaque).
In contrast, almost 100% of BCL2 overexpressing pig-tailed macaque and chimpanzee iPSCs survived upon injection (Roodgar et al., 2022). These results demonstrate the feasibility of BC as a method to create human organs in large animal organ niches or non-human primates. In contrast, the efficiency of chimera formation with the versatility of genetic modifications in large animals remains low compared to attempts in rodents. Most current studies limit our understanding of chimerism to early developmental stages. Hence, it would be interesting to study interspecies chimerism over various developmental stages—from blastocysts to post-implantation- to validate the effectiveness of host models and donor cells.
Although very promising, successful BC doesn’t guarantee functional organ generation. Until recently, only three organs—the brain, the lung, and the pancreas, have been successfully complemented in a way that has produced viable adult animals phenotypically indistinguishable from that of a WT littermate (Kobayashi et al., 2010; Chang et al., 2018; Mori et al., 2019). The barrier of functional organ generation is even higher in the case of interspecies chimeras. The only functional organs generated via interspecies chimerism are the pancreas and thymus (Kobayashi et al., 2010; Isotani et al., 2011). Generation of functional organs by intra- or interspecies BC requires not only compatible donor cells but also appropriate host models in which the function of each target organ can be assessed in live adult animals (Kobayashi et al., 2010; Chang et al., 2018; Mori et al., 2019).
6 Swine as a model for xenotransplantation
In 1964, Dr. James D. Hardy performed one of the first xenotransplantation surgeries in modern medicine by replacing a 68-year-old patient’s heart with that of a chimpanzee. Although that first patient did not survive beyond 2 h and succumbed to a hyperacute immune response, there has been tremendous progress in the field of xenotransplantation over the last sixty years: earlier this year, the first porcine-to-human heart transplantation was performed in a patient who was not a candidate for conventional allogeneic heart transplantation, and the patient survived for 2 months (Griffith et al., 2022). Much progress may be attributed to a deeper understanding of the immune system and the relative ease of gene editing (Ekser et al., 2017; Niu et al., 2017). Although many large animals have been considered, pigs are more favored for xenotransplantation mainly because of ease of breeding (with large and frequent litters) and physiologic organ and size similarity to humans. The grafts of porcine origin survived for months and, in some cases, even years upon transplantation into non-human primates. However, xeno-organ transplantation to monkeys, such as lungs, islets, heart, and kidneys, requires systemic immune suppressive drugs for the long term, and most organs are ultimately rejected (Sykes and Sachs, 2019). Strikingly, the thymokidney approach can induce tolerance across allogeneic barriers, prolong the life of the xenograft when transplanted across xenogeneic barriers, and may ultimately be used to reduce the intensity of immunosuppression for xenotransplanted organs (Yamada et al., 1999; 2000; Shimizu et al., 2005; Rivard et al., 2018).
If an organ from a wild-type pig is transplanted directly into a human patient or a non-human primate, the organ will be rejected within minutes. This form of rejection, also known as a hyperacute immune reaction, results from interactions between host-preformed natural antibodies (Nabs) with the galactose-α1,3-galactose (Gal) antigens expressed on cells of pig grafts (Platt et al., 1991). Using antibody absorption techniques, acute rejection can be delayed to a few hours, but eventually, the graft is rejected (Kozlowski et al., 1997). To overcome innate responses and non-Gal preformed antibodies in addition to T Cell dependent responses against graft, researchers have continued to modify the pig genome to 1) target non-Gal natural antibodies and 2) correct species incompatibilities between swine and primate coagulation, phagocytosis and complement regulatory proteins. These include coagulation inhibition by overexpression of anti-coagulation factors hCD39 and hCD141, that express on the surface of endothelial cells, as well as the addition of complement regulatory protein human transgenes (Lee et al., 2018; Singh et al., 2019; Kemter et al., 2020). Using serial SCNT, additional mutations have been accommodated in pigs to avoid anti-inflammatory and anti-apoptotic responses (Fischer et al., 2016). For details on various engineering strategies of an ideal humanized pig, please refer to the latest reviews by Qin and colleagues (Lu et al., 2020).
If permissive, the humanized swine model would be an ideal background for generating niche-depleted host models using the BC approach (Figure 2B). Upon complementation by donor human PSCs, the resulting organ would be suitable for humanized organs, the mixture of human cells and humanized host cells, into MHC-matched patients awaiting organ transplantation. Suppose the entire targeted organ niche that involves endoderm, mesoderm, and ectoderm derivatives would be complemented by human iPSC-derived cells. It is an ideal treatment for MHC-compatible patients awaiting organ transplantation, with minimal or no immunosuppressive approaches.
Transplanted organs should theoretically be superior to humanized porcine-derived organs in function and means of evading short- and long-term immune responses since all cells are derived from each individual patient or MHC-matched donor (Figure 2B).
7 Other efforts for organ generation
With the introduction of iPSC cell technology, the field of organoid biology expanded to provide simple yet powerful insights underlying human tissue development and, more importantly, disease modeling of pathophysiological conditions (Rossi et al., 2018; Kim et al., 2020). Various organs, such as the liver, lung, kidney, brain, intestine, and even specialized tissues such as the endometrium, have been modeled using human iPSC-derived organoids, histologically similar to human organs (Lancaster et al., 2013; Takasato et al., 2015; Turco et al., 2017; Fujii et al., 2018; Hu et al., 2018; Sachs et al., 2019). With the increasing success of human iPSC-derived organoid grafting studies, exploring organoid engraftment in a non-human host such as a mouse is exciting. Liver and kidney organoids derived from human iPSCs induced vascularization from host mouse tissue and support host tissue function (Takebe et al., 2013; van den Berg et al., 2018). Likewise, human intestinal organoids showed robust viability when implanted into mouse mesenteric tissues (Cortez et al., 2018). In another set of studies, human iPSC and ESC cell-derived brain organoids established subcortical projections and functionally integrated them into the mouse pre-existing neural (Dong et al., 2020). Human neural crest cells differentiate into melanocytes and produce pigmented hair in a c-kit mutant mouse background that lacks melanoblasts (Cohen et al., 2016). Engraftment of human iPSCs-derived neurospheres recover motor function characterized by synapse formation and increased local myelination in a nude mice model of spinal cord injury (Nori et al., 2011). In two models of acute liver failure, human liver organoids derived from hiPSCs rescued hepatic functions and improved survival rates in mice (Nagamoto et al., 2016; Nie et al., 2018). Human ES cell-derived cerebral organoids, when transplanted into injured mouse cerebral cortices, showed high survival efficiency, robust vascularization from host tissues, and recovery of axonal projections along corticospinal tracts (Daviaud et al., 2018; Kitahara T. et al., 2020). Transplantation of human iPSC-derived endothelial-like cells in a mouse model of hindlimb ischemia caused significant improvement in postnatal vascularization (Cho et al., 2007). The engineered human iPSC-derived organoid transplantation into the defective tissue niche, particularly in the immune-privilege niche such as subrenal regions, testes, eyes, liver, and brain, holds great promise for evaluating stemness and mouse disease modeling as a next-generation personalized medicine (Harrison et al., 2014; Paquet et al., 2016; Driehuis and Clevers, 2017; Artegiani et al., 2020). Overall, human iPSC-derived organoids are compelling disease models in vitro and in vivo. However, the main challenges of organoid.
-based approaches are the lack of transplantation methods, scalability to generate whole organs, and reproducibility to generate all cell types to replicate organ function. Unless these issues are resolved, the feasibility of organoids for organ transplantation is low and will depend largely on the stage of the disease; the earlier, the better, but not likely, at the terminal stage.
Tissue engineering is another approach to generating transplantable organs. In such an approach, the goal is to grow an entire or partial organ in vitro. If an engineered organ could be derived from autologous cells of the recipient, the chances of rejection would be close to zero. Creating mechanically, anatomically, physiologically, and biologically compatible organs similar to native organs is ideal. In this regard, decellularization of the extracellular matrix preserves the vascular network and structural framework (Crapo and Wang, 2011). In theory, a decellularized organ scaffold would function as a barcode of cellular repopulation. After an optimal number of cells have populated an organ scaffold, a bioreactor is used to facilitate organ generation. Bioreactors are devices where biological and/or biochemical processes develop under closely monitored and tightly controlled environmental conditions (Martin et al., 2004). Decellularized scaffolds have been made for various organs, including the lung, heart, kidney, liver, intestine, bladder, corneas, limbs, pancreas, and vasculature. (Ott et al., 2008; Hashimoto et al., 2010; Petersen et al., 2010; Yang et al., 2010; Quint et al., 2011; Totonelli et al., 2012; Song et al., 2013a; Gerli et al., 2018; Goh et al., 2019).
For lung tissue engineering, Niklason and colleagues repopulated acellular rat lung scaffolds with neonatal rat lung epithelial cells and microvascular endothelium. They generated viable lung tissue for up to 1 week under in-vitro conditions (Petersen et al., 2010). Ott and colleagues reported that perfusion decellularization of cadaveric lungs yield intact scaffolds seeded with cells to generate bioartificial lung grafts. Upon transplantation of such bioartificial lungs into rats, the grafts maintained respiratory function for up to 7 days (Song et al., 2011). Large animal trials have also been conducted with the hope of pre-clinical applications. Cortiella and colleagues generated porcine lung scaffolds with autologous cells from porcine recipients. Within 2 weeks of transplantation, the lung grafts generated alveolar tissues with supporting vasculature without any sign of immune rejection (Nichols et al., 2018). Ott and colleagues seeded porcine decellularized lung scaffolds with human airway epithelial progenitor cells derived from human donor lungs and banked human umbilical vein endothelial cells. By repopulating porcine extracellular matrix scaffolds with human endothelial cells, they generated pulmonary vasculature with mature endothelial lining supplemented with anti-thrombotic function to enable blood perfusion. They created a functioning gas exchange graft by repopulating the epithelial surface with human epithelial progenitor cells. This graft could withstand physiological blood flow from the recipient’s pulmonary circulation and exchanged gases upon ventilation during the 1-h after transplantation (Zhou et al., 2018).
Initial studies on kidney tissue engineering focused on tissue decellularization methods. Soker and colleagues decellularized porcine kidney scaffolds using a technique that sustained blood pressure up to 2 weeks post-implantation into recipient swine (Orlando et al., 2012). Decellularized monkey kidneys were also functionally effective and biocompatible (Nakayama et al., 2010). Like the lung, kidney tissue engineering also requires the recellularization of decellularized scaffolds, as shown by various studies using swine, monkey, rat, and human organs. Upon transplantation, bioengineered kidney perfused well after orthotopic transplantation into anephric rats and showed urine production (Song et al., 2013b).
Taylor and colleagues reported perfusion decellularization of the whole rat heart, thus setting the stage for other groups to examine this for large animals such as swine (Ott et al., 2008; Wainwright et al., 2010; Weymann et al., 2011; Momtahan 2016). Human-sized decellularized porcine hearts were also developed and showed recellularization of coronary vasculature and myocardium with measurable electrical activity in the decellularized scaffold (Weymann et al., 2014). Shimizu and colleagues reported heterotopic transplantation of a decellularized porcine heart scaffold with mesenchymal stem cells into recipient pigs. The scaffolds could endure surgical procedures and perform short-term coronary artery perfusion by angiography (Kitahara et al., 2016).
Bioengineering techniques of organ generation involving decellularization-recellularization approaches can be challenging in many ways. For instance, existing protocols cannot guarantee the complete removal of residual toxic products within a decellularized tissue. Furthermore, upon transplanting bioengineered tissue into a recipient organism, the degradation rate of decellularized scaffolds should be synchronous with connective tissue remodeling to prevent transplantation failure. Yet, despite its limitations, bioengineering holds excellent promise for organ generation. Further efforts for optimizing recellularization coupled with drug-or genome-editing-mediated approaches for long-term organ maintenance, particularly endothelial components, are essential for organ transplantation into patients suffering from refractory diseases.
8 Future perspectives
Various chimeric, hybrid, and bioengineering studies are being attempted in many initiatives that could be applied to the generation of human organs. Still, most fields are immature, but the successful impact is enormous. Among them, BC is one of the most promising technologies, especially for generating whole organs for transplantation. One of the critical elements for successful entire organ generation using BC is a better understanding of the mechanism of interspecies chimerism between human and host cells during the host developmental program. However, the studies of human-animal chimeras are limited by a lack of resources and ethical concerns. In particular, the contribution of human PSCs to neurogenesis and reproductive tissues within chimeric animals can have profound ethical implications (Bourret et al., 2016; Kwisda et al., 2020). These concerns can be mitigated by engineering cells to be incompetent of ethical-related lineage differentiation.
Another essential step for BC’s success is preparing human iPS cells with organogenic potential. Comparative genome-wide and epigenome-wide analysis of host animal pluripotency with human iPS cells can provide critical guidance. Besides human pluripotency maintenance in human iPSCs, ES cells have also recently been derived from large animals such as pigs, cows, and sheep (Bogliotti et al., 2018; Choi et al., 2020; Vilarino et al., 2020; Kumar et al., 2021). However, except for one study involving pig blastomeres, it has been technically challenging to accurately define naïve state pluripotency and germline competence of large-animal stem cells (Matsunari et al., 2013). To overcome the incompatibilities at the molecular level for efficient interspecies chimerism and subsequent xenotransplantation, humanized large-animal models need to be established, and attempts are already beginning to emerge (Yang et al., 2016; Boettcher et al., 2020). Most recently, Wu and colleagues showed further evidence for the involvement of interspecific cell competition during early embryogenesis. This paper demonstrated the inflammatory-like pathway activation of Myd88-p65-NFkB signaling in donor human iPS cells. The loss of function of Myd88 resulted in overcoming cell competition in early embryogenesis (Zheng et al., 2021). The common feature of outcompeting donor intra- and interspecies PSCs, including ES and iPSC cells that form functional organs in host large animals, is still an open question.
Bioengineered organ transplantation is another option to solve the shortage of donors. Toward human transplantation, the generation of scaffolds based on swine adapted to human organ sizes or human iPSC-derived organoids is promising. However, the fabrication of whole organs that can withstand clinical application has not been realized. Further conceptual and technological breakthroughs are needed beyond the scaffold barcodes of decellularized organs and the self-assembling function of organoids. In this regard, the BC method has perspective advantages over the organoid and decell-recell approaches in terms of scalability, livestock, and a surgical indication of organ transplantation applicable to most end-stage refractory diseases. The advantage of decell-recell-based or organoid systems over the BC approach is the potential for a xeno-free environment and less invasiveness during transplantation. In the future, depending on the recipient’s refractory disease status, the advantages and disadvantages of each technology should complement each other to provide us with multiple beneficial options.
Author contributions
HS, AS, YH, AM, YS, JT, KY and MM conceived the study. HS, AS, KY, and MM, wrote the manuscript. YH, AM, YS, JT, KY, MM revised the manuscript. All authors contributed to the article and approved the submitted version.
Funding
This work was funded by NIH-NHLBI 1R01 HL148223-01, DoD PR190557, PR191133 to MM.
Acknowledgments
We sincerely appreciate the considerate support and scientific input from Dr. Wellington Cardoso and Dr. Jianwen Que at the Columbia Center for Human Development (CCHD). We thank Kathryn K. Kennedy for her grammatical checking of the manuscript.
Conflict of interest
The authors declare that the research was conducted in the absence of any commercial or financial relationships that could be construed as a potential conflict of interest.
Publisher’s note
All claims expressed in this article are solely those of the authors and do not necessarily represent those of their affiliated organizations, or those of the publisher, the editors and the reviewers. Any product that may be evaluated in this article, or claim that may be made by its manufacturer, is not guaranteed or endorsed by the publisher.
References
Addington, C. H. W. (1932). III. Experiments on the development of chick and duck embryos cultivated in vitro. Philoso. Trans. Roy. Soc. Lond. Ser. B 221, 179–230. doi:10.1098/RSTB.1932.0003
Agarwal, Y., Beatty, C., Ho, S., Thurlow, L., Das, A., Kelly, S., et al. (2020). Development of humanized mouse and rat models with full-thickness human skin and autologous immune cells. Sci. Rep. 10 (1), 14598–14611. doi:10.1038/s41598-020-71548-z
Akhlaghpour, A., Taei, A., Ghadami, S. A., Bahadori, Z., Yakhkeshi, S., Molamohammadi, S., et al. (2021). Chicken interspecies chimerism unveils human pluripotency. Stem Cell Rep. 16, 39–55. doi:10.1016/J.STEMCR.2020.11.014
Almeida-Porada, G., Porada, C., and Zanjani, E. D. (2004). The fetal sheep: A unique model system for assessing the full differentiative potential of human stem cells. Yonsei Med. J. 45, 7–14. doi:10.3349/YMJ.2004.45.SUPPL.7
Andres, R. H., Horie, N., Slikker, W., Keren-Gill, H., Zhan, K., Sun, G., et al. (2011). Human neural stem cells enhance structural plasticity and axonal transport in the ischaemic brain. Brain 134, 1777–1789. doi:10.1093/BRAIN/AWR094
Artegiani, B., Hendriks, D., Beumer, J., Kok, R., Zheng, X., Joore, I., et al. (2020). Fast and efficient generation of knock-in human organoids using homology-independent CRISPR–Cas9 precision genome editing. Nat. Cell Biol. 22 (3), 321–331. doi:10.1038/s41556-020-0472-5
Azizi, S. A., Stokes, D., Augelli, B. J., DiGirolamo, C., and Prockop, D. J. (1998). Engraftment and migration of human bone marrow stromal cells implanted in the brains of albino rats - similarities to astrocyte grafts. Proc. Natl. Acad. Sci. U. S. A. 95, 3908–3913. doi:10.1073/pnas.95.7.3908
Bliss, T., Guzman, R., Daadi, M., and Steinberg, G. K. (2007). Cell transplantation therapy for stroke. Stroke 38, 817–826. doi:10.1161/01.STR.0000247888.25985.62
Boettcher, A. N., Li, Y., Ahrens, A. P., Kiupel, M., Byrne, K. A., Loving, C. L., et al. (2020). Novel engraftment and T cell differentiation of human hematopoietic cells in ART−/− IL2RG−/Y SCID pigs. Front. Immunol. 11, 100. doi:10.3389/fimmu.2020.00100
Bogliotti, Y. S., Wu, J., Vilarino, M., Okamura, D., Soto, D. A., Zhong, C., et al. (2018). Efficient derivation of stable primed pluripotent embryonic stem cells from bovine blastocysts. Proc. Natl. Acad. Sci. U. S. A. 115, 2090–2095. doi:10.1073/pnas.1716161115
Boulland, J. L., Halasi, G., Kasumacic, N., and Glover, J. C. (2010). Xenotransplantation of human stem cells into the chicken embryo. J. Vis. Exp., 2071. doi:10.3791/2071
Bourret, R., Martinez, E., Vialla, F., Giquel, C., Thonnat-Marin, A., and de Vos, J. (2016). Human–animal chimeras: Ethical issues about farming chimeric animals bearing human organs. Stem Cell Res. Ther. 7, 87. doi:10.1186/S13287-016-0345-9
Bowling, S., Lawlor, K., and Rodríguez, T. A. (2019). Cell competition: The winners and losers of fitness selection. Dev. Camb. 146, dev167486. doi:10.1242/dev.167486
Bradley, A. (1991). Modifying the mammalian genome by gene targeting. Curr. Opin. Biotechnol. 2, 823–829. doi:10.1016/S0958-1669(05)80114-4
Brüngger, A., Hubler, M., Rohr, H. P., and Brungger, A. (1984). Human skin grafts on athymic nude rats. An experimental model for dermatological research. Exp. Cell Biol. 52, 122–124. doi:10.1159/000163246
Chan, Y. S., Göke, J., Ng, J. H., Lu, X., Gonzales, K. A. U., Tan, C. P., et al. (2013). Induction of a human pluripotent state with distinct regulatory circuitry that resembles preimplantation epiblast. Cell Stem Cell 13, 663–675. doi:10.1016/j.stem.2013.11.015
Chang, A. N., Liang, Z., Dai, H. Q., Chapdelaine-Williams, A. M., Andrews, N., Bronson, R. T., et al. (2018). Neural blastocyst complementation enables mouse forebrain organogenesis. Nature 563, 126–130. doi:10.1038/S41586-018-0586-0
Chang, K. H., Lim, J. M., Kang, S. K., Lee, B. C., Moon, S. Y., and Hwang, W. S. (2003). Blastocyst formation, karyotype, and mitochondrial DNA of interspecies embryos derived from nuclear transfer of human cord fibroblasts into enucleated bovine oocytes. Fertil. Steril. 80, 1380–1387. doi:10.1016/j.fertnstert.2003.07.006
Chen, J., Lansford, R., Stewart, V., Young, F., and Alt, F. W. (1993). RAG-2-deficient blastocyst complementation: An assay of gene function in lymphocyte development. Proc. Natl. Acad. Sci. U. S. A. 90, 4528–4532. doi:10.1073/PNAS.90.10.4528
Chen, Y., He, Z. X., Liu, A., Wang, K., Mao, W. W., Chu, J. X., et al. (2003). Embryonic stem cells generated by nuclear transfer of human somatic nuclei into rabbit oocytes. Cell Res. 13 (4), 251–263. doi:10.1038/sj.cr.7290170
Cho, S. W., Moon, S. H., Lee, S. H., Kang, S. W., Kim, J., Lim, J. M., et al. (2007). Improvement of postnatal neovascularization by human embryonic stem cell–derived endothelial-like cell transplantation in a mouse model of hindlimb ischemia. Circulation 116, 2409–2419. doi:10.1161/CIRCULATIONAHA.106.687038
Choi, J., Huebner, A. J., Clement, K., Walsh, R. M., Savol, A., Lin, K., et al. (2017). Prolonged Mek1/2 suppression impairs the developmental potential of embryonic stem cells. Nature 548, 219–223. doi:10.1038/NATURE23274
Choi, K. H., Lee, D. K., Oh, J. N., Kim, S. H., Lee, M., Woo, S. H., et al. (2020). Pluripotent pig embryonic stem cell lines originating from in vitro-fertilized and parthenogenetic embryos. Stem Cell Res. 49, 102093. doi:10.1016/J.SCR.2020.102093
Chong, J. J. H., Yang, X., Don, C. W., Minami, E., Liu, Y. W., Weyers, J. J., et al. (2014). Human embryonic-stem-cell-derived cardiomyocytes regenerate non-human primate hearts. Nature 510, 7504273–7504277. doi:10.1038/nature13233
Clavería, C., Giovinazzo, G., Sierra, R., and Torres, M. (2013). Myc-driven endogenous cell competition in the early mammalian embryo. Nature 500, 746039–746044. doi:10.1038/nature12389
Clavería, C., and Torres, M. (2016). Cell competition: Mechanisms and physiological roles. Annu. Rev. Cell Dev. Biol. 32, 411–439. doi:10.1146/ANNUREV-CELLBIO-111315-125142
Cloney, K., and Franz-Odendaal, T. A. (2015). Optimized <em>Ex-ovo</em> culturing of chick embryos to advanced stages of development. J. Vis. Exp., 52129. doi:10.3791/52129
Cohen, M. A., Markoulaki, S., and Jaenisch, R. (2018). Matched developmental timing of donor cells with the host is crucial for chimera formation. Stem Cell Rep. 10, 1445–1452. doi:10.1016/J.STEMCR.2018.03.004
Cohen, M. A., Wert, K. J., Goldmann, J., Markoulaki, S., Buganim, Y., Fu, D., et al. (2016). Human neural crest cells contribute to coat pigmentation in interspecies chimeras after in utero injection into mouse embryos. Proc. Natl. Acad. Sci. U. S. A. 113, 1570–1575. doi:10.1073/pnas.1525518113
Cooper, D. K. C., Ekser, B., Ramsoondar, J., Phelps, C., and Ayares, D. (2016). The role of genetically-engineered pigs in xenotransplantation research. J. Pathol. 238, 288–299. doi:10.1002/PATH.4635
Coppiello, G. P. B., Moya-Jodar, M., Abizanda, G., Barreda, C., Iglesias, E., Linares, J., et al. (2022). In vivo generation of heart and vascular system by blastocyst complementation. BioRxiv, 2022.10.04.510637. doi:10.1101/2022.10.04.510637
Cortez, A. R., Poling, H. M., Brown, N. E., Singh, A., Mahe, M. M., and Helmrath, M. A. (2018). Transplantation of human intestinal organoids into the mouse mesentery: A more physiologic and anatomic engraftment site. Surgery 164, 643–650. doi:10.1016/J.SURG.2018.04.048
Couly, G. F., and le Douarin, N. M. (1985). Mapping of the early neural primordium in quail-chick chimeras: I. Developmental relationships between placodes, facial ectoderm, and prosencephalon. Dev. Biol. 110, 422–439. doi:10.1016/0012-1606(85)90101-0
Crapo, P. M., and Wang, Y. (2011). Hydrostatic pressure independently increases elastin and collagen co-expression in small-diameter engineered arterial constructs. J. Biomed. Mater Res. A 96 A, 673–681. doi:10.1002/jbm.a.33019
Dahéron, L., Opitz, S. L., Zaehres, H., Lensch, W. M., Andrews, P. W., Itskovitz-Eldor, J., et al. (2004). LIF/STAT3 signaling fails to maintain self-renewal of human embryonic stem cells. Stem Cells 22, 770–778. doi:10.1634/STEMCELLS.22-5-770
Das, S., Koyano-Nakagawa, N., Gafni, O., Maeng, G., Singh, B. N., Rasmussen, T., et al. (2020). Generation of human endothelium in pig embryos deficient in ETV2. Nat. Biotechnol. 38, 297–302. doi:10.1038/s41587-019-0373-y
Daviaud, N., Friedel, R. H., and Zou, H. (2018). Vascularization and engraftment of transplanted human cerebral organoids in mouse cortex. eNeuro 5, ENEURO.0219–18.2018. doi:10.1523/ENEURO.0219-18.2018
de La Cova, C., Abril, M., Bellosta, P., Gallant, P., and Johnston, L. A. (2004). Drosophila myc regulates organ size by inducing cell competition. Cell 117, 107–116. doi:10.1016/S0092-8674(04)00214-4
de Los Angeles, A., and Wu, J. (2022). New concepts for generating interspecies chimeras using human pluripotent stem cells. Protein Cell 13, 234–238. doi:10.1007/s13238-021-00880-5
Díaz-Díaz, C., Fernandez de Manuel, L., Jimenez-Carretero, D., Montoya, M. C., Clavería, C., and Torres, M. (2017). Pluripotency surveillance by myc-driven competitive elimination of differentiating cells. Dev. Cell 42, 585–599.e4. doi:10.1016/J.DEVCEL.2017.08.011
Dong, X., Xu, S. B., Chen, X., Tao, M., Tang, X. Y., Fang, K. H., et al. (2020). Human cerebral organoids establish subcortical projections in the mouse brain after transplantation. Mol. Psychiatry 26, 2964–2976. doi:10.1038/s41380-020-00910-4
Driehuis, E., and Clevers, H. (2017). CRISPR/Cas 9 genome editing and its applications in organoids. Am. J. Physiol. Gastrointest. Liver Physiol. 312, G257–G265. doi:10.1152/ajpgi.00410.2016
Ekser, B., Li, P., and Cooper, D. K. C. (2017). Xenotransplantation: PAST, present, and future. Curr. Opin. Organ Transpl. 22, 513–521. doi:10.1097/MOT.0000000000000463
Evans, M. J., and Kaufman, M. H. (1981). Establishment in culture of pluripotential cells from mouse embryos. Nature 292, 154–156. doi:10.1038/292154A0
Fehilly, C. B., Willadsen, S. M., and Tucker, E. M. (1984). Interspecific chimaerism between sheep and goat. Nature 307, 634–636. doi:10.1038/307634a0
Fischer, K., Kraner-Scheiber, S., Petersen, B., Rieblinger, B., Buermann, A., Flisikowska, T., et al. (2016). Efficient production of multi-modified pigs for xenotransplantation by ‘combineering’, gene stacking and gene editing. Sci. Rep. 6, 29081. doi:10.1038/SREP29081
Freedman, B. S. (2018). Hopes and difficulties for blastocyst complementation. Nephron 138, 42–47. doi:10.1159/000480370
Fu, R., Yu, D., Ren, J., Li, C., Wang, J., Feng, G., et al. (2020). Domesticated cynomolgus monkey embryonic stem cells allow the generation of neonatal interspecies chimeric pigs. Protein Cell 11 (2), 97–107. doi:10.1007/S13238-019-00676-8/TABLES/2
Fujii, M., Matano, M., Toshimitsu, K., Takano, A., Mikami, Y., Nishikori, S., et al. (2018). Human intestinal organoids maintain self-renewal capacity and cellular diversity in niche-inspired culture condition. Cell Stem Cell 23, 787–793.e6. doi:10.1016/J.STEM.2018.11.016
Gafni, O., Weinberger, L., Mansour, A. A., Manor, Y. S., Chomsky, E., Ben-Yosef, D., et al. (2013). Derivation of novel human ground state naive pluripotent stem cells. Nature 504, 282–286. doi:10.1038/nature12745
Gao, X., Nowak-Imialek, M., Chen, X., Chen, D., Herrmann, D., Ruan, D., et al. (2019). Establishment of porcine and human expanded potential stem cells. Nat. Cell Biol. 21, 687–699. doi:10.1038/s41556-019-0333-2
Garry, D. J., and Garry, M. G. (2019). Interspecies chimeras and the generation of humanized organs. Circ. Res. 124, 23–25. doi:10.1161/CIRCRESAHA.118.314189
Garry, D. J., and Garry, M. G. (2021). Interspecies chimeras as a platform for exogenic organ production and transplantation. Exp. Biol. Med. 246, 1838–1844. doi:10.1177/15353702211024948
Gerli, M. F. M., Guyette, J. P., Evangelista-Leite, D., Ghoshhajra, B. B., and Ott, H. C. (2018). Perfusion decellularization of a human limb: A novel platform for composite tissue engineering and reconstructive surgery. PLoS One 13, e0191497. doi:10.1371/JOURNAL.PONE.0191497
Gibbs, R. A., Rogers, J., Katze, M. G., Bumgarner, R., Weinstock, G. M., Mardis, E. R., et al. (2007). Evolutionary and biomedical insights from the rhesus macaque genome. Science 316, 222–234. doi:10.1126/SCIENCE.1139247/SUPPL_FILE/GIBBS.SOM.PDF
Gilhar, A., Etzioni, A., and Krueger, G. G. (1990). Hair growth in human split-thickness skin grafts transplanted onto nude rats: The role of cyclosporin. Dermatologica 181, 117–121. doi:10.1159/000247898
Gilhar, A., Wojciechowski, Z. J., Piepkorn, M. W., Spangrude, G. J., Roberts, L. K., and Krueger, D. G. G. (1986). Description of and treatment to inhibit the rejection of human split-thickness skin grafts by congenitally athymic (nude) rats. Exp. Cell Biol. 54, 263–274. doi:10.1159/000163365
Giwa, S., Lewis, J. K., Alvarez, L., Langer, R., Roth, A. E., Church, G. M., et al. (2017). The promise of organ and tissue preservation to transform medicine. Nat. Biotechnol. 35 (6), 530–542. doi:10.1038/nbt.3889
Goh, S.-K., Bertera, S., Vaidya, V., Dumpe, S., Barner, S., Mathew, S., et al. (2019). Development of perfusion bioreactor for whole organ engineering — A culture system that enhances cellular engraftment, survival and phenotype of repopulated pancreas. World Sci. Technol. 6, 118–134. doi:10.1142/S2339547818500085.06
Goldstein, R. S. (2010). Transplantation of human embryonic stem cells and derivatives to the chick embryo. Methods Mol. Biol. 584, 367–385. doi:10.1007/978-1-60761-369-5_20
Graham, D. M. (2017). Beasts of burden: Large animal chimeras using human pluripotent stem cells. Lab. Anim. 46 (4), 77. doi:10.1038/laban.1241
Greely, H. T., and Farahany, N. A. (2021). Advancing the ethical dialogue about monkey/human chimeric embryos. Cell 184, 1962–1963. doi:10.1016/J.CELL.2021.03.044
Griffith, B. P., Goerlich, C. E., Singh, A. K., Rothblatt, M., Lau, C. L., Shah, A., et al. (2022). Genetically modified porcine-to-human cardiac xenotransplantation. N. Engl. J. Med. 387, 35–44. doi:10.1056/NEJMoa2201422
Grinnemo, K. H., Månsson, A., Dellgren, G., Klingberg, D., Wardell, E., Drvota, V., et al. (2004). Xenoreactivity and engraftment of human mesenchymal stem cells transplanted into infarcted rat myocardium. J. Thorac. Cardiovasc. Surg. 127, 1293–1300. doi:10.1016/j.jtcvs.2003.07.037
Hamanaka, S., Umino, A., Sato, H., Hayama, T., Yanagida, A., Mizuno, N., et al. (2018). Generation of vascular endothelial cells and hematopoietic cells by blastocyst complementation. Stem Cell Rep. 11, 988–997. doi:10.1016/J.STEMCR.2018.08.015
Hamburger, V., and Hamilton, H. L. (1951). A series of normal stages in the development of the chick embryo. J. Morphol. 88, 49–92. doi:10.1002/JMOR.1050880104
Harrison, M. M., Jenkins, B. v., O’Connor-Giles, K. M., and Wildonger, J. (2014). A CRISPR view of development. Genes Dev. 28, 1859–1872. doi:10.1101/GAD.248252.114
Hashimoto, M., and Sasaki, H. (2020). Cell competition controls differentiation in mouse embryos and stem cells. Curr. Opin. Cell Biol. 67, 1–8. doi:10.1016/J.CEB.2020.07.001
Hashimoto, Y., Funamoto, S., Sasaki, S., Honda, T., Hattori, S., Nam, K., et al. (2010). Preparation and characterization of decellularized cornea using high-hydrostatic pressurization for corneal tissue engineering. Biomaterials 31, 3941–3948. doi:10.1016/J.BIOMATERIALS.2010.01.122
He, D., Zhang, J., Wu, W., Yi, N., He, W., Lu, P., et al. (2019). A novel immunodeficient rat model supports human lung cancer xenografts. FASEB J. 33, 140–150. doi:10.1096/FJ.201800102RR
Hu, H., Gehart, H., Artegiani, B., Löpez-Iglesias, C., Dekkers, F., Basak, O., et al. (2018). Long-term expansion of functional mouse and human hepatocytes as 3D organoids. Cell 175, 1591–1606.e19. doi:10.1016/J.CELL.2018.11.013
Hu, Z., Li, H., Jiang, H., Ren, Y., Yu, X., Qiu, J., et al. (2020). Transient inhibition of mTOR in human pluripotent stem cells enables robust formation of mouse-human chimeric embryos. Sci. Adv. 6, eaaz0298–311. doi:10.1126/sciadv.aaz0298
Ideta, A., Yamashita, S., Seki-Soma, M., Yamaguchi, R., Chiba, S., Komaki, H., et al. (2016). Generation of exogenous germ cells in the ovaries of sterile NANOS3-null beef cattle. Sci. Rep. 6, 24983. doi:10.1038/srep24983
Isotani, A., Hatayama, H., Kaseda, K., Ikawa, M., and Okabe, M. (2011). Formation of a thymus from rat ES cells in xenogeneic nude mouse↔rat ES chimeras. Genes Cells 16, 397–405. doi:10.1111/J.1365-2443.2011.01495.X
James, D., Noggle, S. A., Swigut, T., and Brivanlou, A. H. (2006). Contribution of human embryonic stem cells to mouse blastocysts. Dev. Biol. 295, 90–102. doi:10.1016/J.YDBIO.2006.03.026
Jiang, Y., Kelly, R., Peters, A., Fulka, H., Dickinson, A., Mitchell, D. A., et al. (2011). Interspecies somatic cell nuclear transfer is dependent on compatible mitochondrial DNA and reprogramming factors. PLoS One 6, 14805. doi:10.1371/JOURNAL.PONE.0014805
Kano, M., Mizutani, E., Homma, S., Masaki, H., and Nakauchi, H. (2022). Xenotransplantation and interspecies organogenesis: Current status and issues. Front. Endocrinol. (Lausanne) 13, 963282. doi:10.3389/FENDO.2022.963282
Kemter, E., Schnieke, A., Fischer, K., Cowan, P. J., and Wolf, E. (2020). Xeno-organ donor pigs with multiple genetic modifications - the more the better? Curr. Opin. Genet. Dev. 64, 60–65. doi:10.1016/J.GDE.2020.05.034
Kikuchi, T., Morizane, A., Doi, D., Magotani, H., Onoe, H., Hayashi, T., et al. (2017). Human iPS cell-derived dopaminergic neurons function in a primate Parkinson’s disease model. Nature 548 (548), 7669592–7669596. doi:10.1038/nature23664
Kim, J., Koo, B. K., and Knoblich, J. A. (2020). Human organoids: Model systems for human biology and medicine. Nat. Rev. Mol. Cell Biol. 21 (10 21), 571–584. doi:10.1038/s41580-020-0259-3
Kitahara, A., Ran, Q., Oda, K., Yasue, A., Abe, M., Ye, X., et al. (2020). Generation of lungs by blastocyst complementation in apneumic fgf10-deficient mice. Cell Rep. 31, 107626. doi:10.1016/J.CELREP.2020.107626
Kitahara, H., Yagi, H., Tajima, K., Okamoto, K., Yoshitake, A., Aeba, R., et al. (2016). Heterotopic transplantation of a decellularized and recellularized whole porcine heart. Interact. Cardiovasc Thorac. Surg. 22, 571–579. doi:10.1093/ICVTS/IVW022
Kitahara, T., Sakaguchi, H., Morizane, A., Kikuchi, T., Miyamoto, S., and Takahashi, J. (2020). Axonal extensions along corticospinal tracts from transplanted human cerebral organoids. Stem Cell Rep. 15, 467–481. doi:10.1016/J.STEMCR.2020.06.016
Kobayashi, T., Goto, T., Oikawa, M., Sanbo, M., Yoshida, F., Terada, R., et al. (2021). Blastocyst complementation using Prdm14-deficient rats enables efficient germline transmission and generation of functional mouse spermatids in rats. Nat. Commun. 12 (1), 1328. doi:10.1038/s41467-021-21557-x
Kobayashi, T., Yamaguchi, T., Hamanaka, S., Kato-Itoh, M., Yamazaki, Y., Ibata, M., et al. (2010). Generation of rat pancreas in mouse by interspecific blastocyst injection of pluripotent stem cells. Cell 142, 787–799. doi:10.1016/j.cell.2010.07.039
Kozlowski, T., Fuchimoto, Y., Monroy, R., Bailin, M., Martinez-Ruiz, R., Foley, A., et al. (1997). Apheresis and column absorption for specific removal of Gal-alpha-1,3 Gal natural antibodies in a pig-to-baboon model. Transpl. Proc. 29, 961. doi:10.1016/S0041-1345(96)00299-0
Kumar, D., Talluri, T. R., Selokar, N. L., Hyder, I., and Kues, W. A. (2021). Perspectives of pluripotent stem cells in livestock. World J. Stem Cells 13, 1–29. doi:10.4252/WJSC.V13.I1.1
Kwisda, K., White, L., and Hübner, D. (2020). Ethical arguments concerning human-animal chimera research: A systematic review. BMC Med. Ethics 21, 24–14. doi:10.1186/s12910-020-00465-7
Lancaster, M. A., Renner, M., Martin, C. A., Wenzel, D., Bicknell, L. S., Hurles, M. E., et al. (2013). Cerebral organoids model human brain development and microcephaly. Nature 501, 373–379. doi:10.1038/NATURE12517
Laporte, C., Tubbs, E., Cristante, J., Gauchez, A. S., Pesenti, S., Lamarche, F., et al. (2019). Human mesenchymal stem cells improve rat islet functionality under cytokine stress with combined upregulation of heme oxygenase-1 and ferritin. Stem Cell Res. Ther. 10, 85–12. doi:10.1186/s13287-019-1190-4
Lee, D. H., Eun, Y. K., Park, S., Ji, H. P., Kim, S. K., Cho, B. K., et al. (2006). Reclosure of surgically induced spinal open neural tube defects by the intraamniotic injection of human embryonic stem cells in chick embryos 24 hours after lesion induction. J. Neurosurg. 105, 127–133. doi:10.3171/PED.2006.105.2.127
Lee, D. H., Park, S., Kim, E. Y., Kim, S. K., Chung, Y. N., Cho, B. K., et al. (2004). Enhancement of re-closure capacity by the intra-amniotic injection of human embryonic stem cells in surgically induced spinal open neural tube defects in chick embryos. Neurosci. Lett. 364, 98–100. doi:10.1016/j.neulet.2004.04.033
Lee, S. J., Kim, J. S., Chee, H. K., Yun, I. J., Park, K. S., Yang, H. S., et al. (2018). Seven years of experiences of preclinical experiments of xeno-heart transplantation of pig to non-human primate (cynomolgus monkey). Transpl. Proc. 50, 1167–1171. doi:10.1016/J.TRANSPROCEED.2018.01.041
Liechty, K. W., Mackenzie, T. C., Shaaban, A. F., Radu, A., Moseley, A. M. B., Deans, R., et al. (2000). Human mesenchymal stem cells engraft and demonstrate site-specific differentiation after in utero transplantation in sheep. Nat. Med. 6, 1282–1286. doi:10.1038/81395
Liu, X., Nefzger, C. M., Rossello, F. J., Chen, J., Knaupp, A. S., Firas, J., et al. (2017). Comprehensive characterization of distinct states of human naive pluripotency generated by reprogramming. Nat. Methods 14, 1055–1062. doi:10.1038/nmeth.4436
Lu, T., Yang, B., Wang, R., and Qin, C. (2020). Xenotransplantation: Current status in preclinical research. Front. Immunol. 10, 3060. doi:10.3389/fimmu.2019.03060
Lu, Y., Zhou, Y., Ju, R., and Chen, J. (2019). Human-animal chimeras for autologous organ transplantation: Technological advances and future perspectives. Ann. Transl. Med. 7, 576. doi:10.21037/ATM.2019.10.13
Maeng, G., Das, S., Greising, S. M., Gong, W., Singh, B. N., Kren, S., et al. (2021). Humanized skeletal muscle in MYF5/MYOD/MYF6-null pig embryos. Nat. Biomed. Eng. 5, 805–814. doi:10.1038/s41551-021-00693-1
Mansour, S. L., Thomas, K. R., Deng, C., and Capecchi, M. R. (1990). Introduction of a lacZ reporter gene into the mouse int-2 locus by homologous recombination. Proc. Natl. Acad. Sci. U. S. A. 87, 7688–7692. doi:10.1073/PNAS.87.19.7688
Martello, G., and Smith, A. (2014). The nature of embryonic stem cells. Annu. Rev. Cell Dev. Biol. 30, 647–675. doi:10.1146/ANNUREV-CELLBIO-100913-013116
Martin, G. R. (1981). Isolation of a pluripotent cell line from early mouse embryos cultured in medium conditioned by teratocarcinoma stem cells. Proc. Natl. Acad. Sci. 78, 7634–7638. doi:10.1073/PNAS.78.12.7634
Martin, I., Wendt, D., and Heberer, M. (2004). The role of bioreactors in tissue engineering. Trends Biotechnol. 22, 80–86. doi:10.1016/j.tibtech.2003.12.001
Martyn, I., Kanno, T. Y., Ruzo, A., Siggia, E. D., and Brivanlou, A. H. (2018). Self-organization of a human organizer by combined Wnt and Nodal signalling. Nature 558, 132–135. doi:10.1038/s41586-018-0150-y
Mascetti, V. L., and Pedersen, R. A. (2016). Human-mouse chimerism validates human stem cell pluripotency. Cell Stem Cell 18, 67–72. doi:10.1016/J.STEM.2015.11.017
Matsunari, H., Nagashima, H., Watanabe, M., Umeyama, K., Nakano, K., Nagaya, M., et al. (2013). Blastocyst complementation generates exogenic pancreas in vivo in apancreatic cloned pigs. Proc. Natl. Acad. Sci. U. S. A. 110, 4557–4562. doi:10.1073/pnas.1222902110
Matsunari, H., Watanabe, M., Hasegawa, K., Uchikura, A., Nakano, K., Umeyama, K., et al. (2020). Compensation of disabled organogeneses in genetically modified pig fetuses by blastocyst complementation. Stem Cell Rep. 14, 21–33. doi:10.1016/j.stemcr.2019.11.008
Merino, M. M., Levayer, R., and Moreno, E. (2016). Survival of the fittest: Essential roles of cell competition in development, aging, and cancer. Trends Cell Biol. 26, 776–788. doi:10.1016/j.tcb.2016.05.009
Momtahan, N. (2016). Extracellular matrix from whole porcine heart decellularization for cardiac tissue engineering. Theses and Dissertations. Available at: https://scholarsarchive.byu.edu/etd/6225 (Accessed October 11, 2022).
Moreno, E., and Basler, K. (2004). dMyc transforms cells into super-competitors. Cell 117, 117–129. doi:10.1016/S0092-8674(04)00262-4
Mori, M., Furuhashi, K., Danielsson, J. A., Hirata, Y., Kakiuchi, M., Lin, C. S., et al. (2019). Generation of functional lungs via conditional blastocyst complementation using pluripotent stem cells. Nat. Med. 25 (11), 1691–1698. doi:10.1038/s41591-019-0635-8
Morizane, A., Kikuchi, T., Hayashi, T., Mizuma, H., Takara, S., Doi, H., et al. (2017). MHC matching improves engraftment of iPSC-derived neurons in non-human primates. Nat. Commun. 8 (1), 385–412. doi:10.1038/s41467-017-00926-5
Mystkowska, E. T. (1975). Development of mouse—Bank vole interspecific chimaeric embryos. Development 33, 731–744. doi:10.1242/DEV.33.3.731
Nagamoto, Y., Takayama, K., Ohashi, K., Okamoto, R., Sakurai, F., Tachibana, M., et al. (2016). Transplantation of a human iPSC-derived hepatocyte sheet increases survival in mice with acute liver failure. J. Hepatol. 64, 1068–1075. doi:10.1016/J.JHEP.2016.01.004
Nagashima, H., and Matsunari, H. (2016). Growing human organs in pigs-A dream or reality? Theriogenology 86, 422–426. doi:10.1016/J.THERIOGENOLOGY.2016.04.056
Nakayama, K. H., Batchelder, C. A., Lee, C. I., and Tarantal, A. F. (2010). Decellularized rhesus monkey kidney as a three-dimensional scaffold for renal tissue engineering. Tissue Eng. Part A 16, 2207–2216. doi:10.1089/TEN.TEA.2009.0602
Nanda, I., Shan, Z., Schartl, M., Burt, D. W., Koehler, M., Nothwang, G., et al. (1999). 300 million years of conserved synteny between chicken Z and human chromosome 9. Nat. Genet. 21 (3), 258–259. doi:10.1038/6769
Nei, M., Xu, P., and Glazko, G. (2001). Estimation of divergence times from multiprotein sequences for a few mammalian species and several distantly related organisms. Proc. Natl. Acad. Sci. U. S. A. 98, 2497–2502. doi:10.1073/pnas.051611498
Nichols, J. E., la Francesca, S., Niles, J. A., Vega, S. P., Argueta, L. B., Frank, L., et al. (2018). Production and transplantation of bioengineered lung into a large-animal model. Sci. Transl. Med. 10, eaao3926. doi:10.1126/SCITRANSLMED.AAO3926
Nichols, J., and Smith, A. (2009). Naive and primed pluripotent states. Cell Stem Cell 4, 487–492. doi:10.1016/j.stem.2009.05.015
Nie, Y. Z., Zheng, Y. W., Ogawa, M., Miyagi, E., and Taniguchi, H. (2018). Human liver organoids generated with single donor-derived multiple cells rescue mice from acute liver failure. Stem Cell Res. Ther. 9, 5–12. doi:10.1186/s13287-017-0749-1
Niu, D., Wei, H. J., Lin, L., George, H., Wang, T., Lee, I. H., et al. (2017). Inactivation of porcine endogenous retrovirus in pigs using CRISPR-Cas9. Science 357, 1303–1307. doi:10.1126/science.aan4187
Niu, Y., Shen, B., Cui, Y., Chen, Y., Wang, J., Wang, L., et al. (2014). Generation of gene-modified cynomolgus monkey via Cas9/RNA-mediated gene targeting in one-cell embryos. Cell 156, 836–843. doi:10.1016/j.cell.2014.01.027
Nori, S., Okada, Y., Yasuda, A., Tsuji, O., Takahashi, Y., Kobayashi, Y., et al. (2011). Grafted human-induced pluripotent stem-cell-derived neurospheres promote motor functional recovery after spinal cord injury in mice. Proc. Natl. Acad. Sci. U. S. A. 108, 16825–16830. doi:10.1073/pnas.1108077108
Noto, F. K., Sangodkar, J., Adedeji, B. T., Moody, S., McClain, C. B., Tong, M., et al. (2020). The SRG rat, a Sprague-Dawley Rag2/Il2rg double-knockout validated for human tumor oncology studies. PLoS One 15, e0240169. doi:10.1371/JOURNAL.PONE.0240169
Orlando, G., Farney, A. C., Iskandar, S. S., Mirmalek-Sani, S. H., Sullivan, D. C., Moran, E., et al. (2012). Production and implantation of renal extracellular matrix scaffolds from porcine kidneys as a platform for renal bioengineering investigations. Ann. Surg. 256, 363–370. doi:10.1097/SLA.0B013E31825A02AB
Ott, H. C., Matthiesen, T. S., Goh, S. K., Black, L. D., Kren, S. M., Netoff, T. I., et al. (2008). Perfusion-decellularized matrix: Using nature’s platform to engineer a bioartificial heart. Nat. Med. 14, 213–221. doi:10.1038/nm1684
Paquet, D., Kwart, D., Chen, A., Sproul, A., Jacob, S., Teo, S., et al. (2016). Efficient introduction of specific homozygous and heterozygous mutations using CRISPR/Cas9. Nature 533, 125–129. doi:10.1038/NATURE17664
Pastor, W. A., Chen, D., Liu, W., Kim, R., Sahakyan, A., Lukianchikov, A., et al. (2016). Naïve human pluripotent cells feature a methylation landscape devoid of blastocyst or germline memory. Cell Stem Cell 18, 323–329. doi:10.1016/J.STEM.2016.01.019
Petersen, T. H., Calle, E. A., Zhao, L., Lee, E. J., Gui, L., Raredon, M. S. B., et al. (2010). Tissue-engineered lungs for in vivo implantation. Science 329, 538–541. doi:10.1126/SCIENCE.1189345
Platt, J. L., Lindman, B. J., Geller, R. L., Noreen, H. J., Swanson, J. L., Dalmasso, A. P., et al. (1991). The role of natural antibodies in the activation of xenogenic endothelial cells. Transplantation 52, 1037–1043. doi:10.1097/00007890-199112000-00019
Quint, C., Kondo, Y., Manson, R. J., Lawson, J. H., Dardik, A., and Niklason, L. E. (2011). Decellularized tissue-engineered blood vessel as an arterial conduit. Proc. Natl. Acad. Sci. U. S. A. 108, 9214–9219. doi:10.1073/pnas.1019506108
Rashid, T., Kobayashi, T., and Nakauchi, H. (2014). Revisiting the flight of icarus: Making human organs from PSCs with large animal chimeras. Cell Stem Cell 15, 406–409. doi:10.1016/J.STEM.2014.09.013
Rivard, C. J., Tanabe, T., Lanaspa, M. A., Watanabe, H., Nomura, S., Andres-Hernando, A., et al. (2018). Upregulation of CD80 on glomerular podocytes plays an important role in development of proteinuria following pig-to-baboon xeno-renal transplantation - an experimental study. Transpl. Int. 31, 1164–1177. doi:10.1111/TRI.13273
Robertson, E. J. (1991). Using embryonic stem cells to introduce mutations into the mouse germ line. Biol. Reprod. 44, 238–245. doi:10.1095/BIOLREPROD44.2.238
Rodriguez-Osorio, N., Urrego, R., Cibelli, J. B., Eilertsen, K., and Memili, E. (2012). Reprogramming mammalian somatic cells. Theriogenology 78, 1869–1886. doi:10.1016/J.THERIOGENOLOGY.2012.05.030
Roodgar, M., Suchy, F. P., Nguyen, L. H., Bajpai, V. K., Sinha, R., Vilches-Moure, J. G., et al. (2022). Chimpanzee and pig-tailed macaque iPSCs: Improved culture and generation of primate cross-species embryos. Cell Rep. 40, 111264. doi:10.1016/J.CELREP.2022.111264
Rossi, G., Manfrin, A., and Lutolf, M. P. (2018). Progress and potential in organoid research. Nat. Rev. Genet. 19, 671–687. doi:10.1038/S41576-018-0051-9
Ruiz-Estevez, M., Crane, A. T., Rodriguez-Villamil, P., Ongaratto, F. L., You, Y., Steevens, A. R., et al. (2021). Liver development is restored by blastocyst complementation of HHEX knockout in mice and pigs. Stem Cell Res. Ther. 12, 292–313. doi:10.1186/s13287-021-02348-z
Sachs, N., Papaspyropoulos, A., Ommen, D. D. Z., Heo, I., Böttinger, L., Klay, D., et al. (2019). Long-term expanding human airway organoids for disease modeling. EMBO J. 38, e100300. doi:10.15252/EMBJ.2018100300
Sancho, M., Di-Gregorio, A., George, N., Pozzi, S., Sánchez, J. M., Pernaute, B., et al. (2013). Competitive interactions eliminate unfit embryonic stem cells at the onset of differentiation. Dev. Cell 26, 19–30. doi:10.1016/J.DEVCEL.2013.06.012
Sato, Y., Araki, H., Kato, J., Nakamura, K., Kawano, Y., Kobune, M., et al. (2005). Human mesenchymal stem cells xenografted directly to rat liver are differentiated into human hepatocytes without fusion. Blood 106, 756–763. doi:10.1182/BLOOD-2005-02-0572
Shimizu, A., Yamada, K., Yamamoto, S., Lavelle, J. M., Barth, R. N., Robson, S. C., et al. (2005). Thrombotic microangiopathic glomerulopathy in human decay accelerating factor-transgenic swine-to-baboon kidney xenografts. J. Am. Soc. Nephrol. 16, 2732–2745. doi:10.1681/ASN.2004121148
Shirai, H., Mandai, M., Matsushita, K., Kuwahara, A., Yonemura, S., Nakano, T., et al. (2016). Transplantation of human embryonic stem cell-derived retinal tissue in two primate models of retinal degeneration. Proc. Natl. Acad. Sci. U. S. A. 113, E81–E90. doi:10.1073/pnas.1512590113
Silva, J., and Smith, A. (2008). Capturing pluripotency. Cell 132, 532–536. doi:10.1016/j.cell.2008.02.006
Singh, A. K., Chan, J. L., DiChiacchio, L., Hardy, N. L., Corcoran, P. C., Lewis, B. G. T., et al. (2019). Cardiac xenografts show reduced survival in the absence of transgenic human thrombomodulin expression in donor pigs. Xenotransplantation 26, e12465. doi:10.1111/XEN.12465
Song, J. J., Guyette, J. P., Gilpin, S. E., Gonzalez, G., Vacanti, J. P., and Ott, H. C. (2013a). Regeneration and experimental orthotopic transplantation of a bioengineered kidney. Nat. Med. 19, 646–651. doi:10.1038/nm.3154
Song, J. J., Guyette, J. P., Gilpin, S. E., Gonzalez, G., Vacanti, J. P., and Ott, H. C. (2013b). Regeneration and experimental orthotopic transplantation of a bioengineered kidney. Nat. Med. 19, 646–651. doi:10.1038/NM.3154
Song, J. J., Kim, S. S., Liu, Z., Madsen, J. C., Mathisen, D. J., Vacanti, J. P., et al. (2011). Enhanced in vivo function of bioartificial lungs in rats. Ann. Thorac. Surg. 92, 998–1005. doi:10.1016/J.ATHORACSUR.2011.05.018
Spurlin, J., and Lwigale, P. (2013). A technique to increase accessibility to late-stage chick embryos for in ovo manipulations. Dev. Dyn. 242, 148–154. doi:10.1002/DVDY.23907
St John, J., and Lovell-Badge, R. (2007). Human-animal cytoplasmic hybrid embryos, mitochondria, and an energetic debate. Nat. Cell Biol. 9, 988–992. doi:10.1038/NCB436
Stanny, L. F., Elfner, R. R., Zwicker, E., Terhardt, E., and Springer-, E. (1980). Interspecific chimeras in mammals: Successful production of live chimeras between Mus musculus and Mus caroli. Science 208, 419–421. doi:10.1126/SCIENCE.7367871
Stevens, N. J., Seiffert, E. R., O’Connor, P. M., Roberts, E. M., Schmitz, M. D., Krause, C., et al. (2013). Palaeontological evidence for an Oligocene divergence between Old World monkeys and apes. Nature 497, 611–614. doi:10.1038/nature12161
Sugita, S., Iwasaki, Y., Makabe, K., Kimura, T., Futagami, T., Suegami, S., et al. (2016). Lack of T Cell response to iPSC-derived retinal pigment epithelial cells from HLA homozygous donors. Stem Cell Rep. 7, 619–634. doi:10.1016/J.STEMCR.2016.08.011
Sun, Y., Xiao, D., Pan, X. H., Zhang, R. S., Cui, G. H., and Chen, X. G. (2007). Generation of human/rat xenograft animal model for the study of human donor stem cell behaviors in vivo. World J. Gastroenterology WJG 13, 2707–2716. doi:10.3748/WJG.V13.I19.2707
Sykes, M., and Sachs, D. H. (2019). Transplanting organs from pigs to humans. Sci. Immunol. 4, eaau6298. doi:10.1126/SCIIMMUNOL.AAU6298
Tabar, V., Panagiotakos, G., Greenberg, E. D., Chan, B. K., Sadelain, M., Gutin, P. H., et al. (2005). Migration and differentiation of neural precursors derived from human embryonic stem cells in the rat brain. Nat. Biotechnol. 23, 601–606. doi:10.1038/nbt1088
Takasato, M., Er, P. X., Chiu, H. S., Maier, B., Baillie, G. J., Ferguson, C., et al. (2015). Kidney organoids from human iPS cells contain multiple lineages and model human nephrogenesis. Nature 526, 564–568. doi:10.1038/NATURE15695
Takashima, Y., Guo, G., Loos, R., Nichols, J., Ficz, G., Krueger, F., et al. (2014). Resetting transcription factor control circuitry toward ground-state pluripotency in human. Cell 158, 1254–1269. doi:10.1016/j.cell.2014.08.029
Takebe, T., Sekine, K., Enomura, M., Koike, H., Kimura, M., Ogaeri, T., et al. (2013). Vascularized and functional human liver from an iPSC-derived organ bud transplant. Nature 499, 481–484. doi:10.1038/nature12271
Tan, T., Wu, J., Si, C., Dai, S., Zhang, Y., Sun, N., et al. (2021). Chimeric contribution of human extended pluripotent stem cells to monkey embryos ex vivo. Cell 184, 2020–2032.e14. doi:10.1016/j.cell.2021.03.020
Theunissen, T. W., Powell, B. E., Wang, H., Mitalipova, M., Faddah, D. A., Reddy, J., et al. (2014). Systematic identification of culture conditions for induction and maintenance of naive human pluripotency. Cell Stem Cell 15, 471–487. doi:10.1016/j.stem.2014.07.002
Thomas, J., Zimmerlin, L., Huo, J. S., Considine, M., Cope, L., and Zambidis, E. T. (2021). Running the full human developmental clock in interspecies chimeras using alternative human stem cells with expanded embryonic potential. npj Regen. Med. 6, 25–13. doi:10.1038/s41536-021-00135-1
Thomson, J. A., Itskovitz-Eldor, J., Shapiro, S. S., Waknitz, M. A., Swiergiel, J. J., Marshall, V. S., et al. (1998). Embryonic stem cell lines derived from human blastocysts. Science 282, 1145–1147. doi:10.1126/SCIENCE.282.5391.1145
Totonelli, G., Maghsoudlou, P., Garriboli, M., Riegler, J., Orlando, G., Burns, A. J., et al. (2012). A rat decellularized small bowel scaffold that preserves villus-crypt architecture for intestinal regeneration. Biomaterials 33, 3401–3410. doi:10.1016/j.biomaterials.2012.01.012
Tsukiyama, T., and Ohinata, Y. (2014). A modified EpiSC culture condition containing a GSK3 inhibitor can support germline-competent pluripotency in mice. PLoS One 9, e95329. doi:10.1371/JOURNAL.PONE.0095329
Turco, M. Y., Gardner, L., Hughes, J., Cindrova-Davies, T., Gomez, M. J., Farrell, L., et al. (2017). Long-term, hormone-responsive organoid cultures of human endometrium in a chemically defined medium. Nat. Cell Biol. 19, 568–577. doi:10.1038/NCB3516
van den Berg, C. W., Ritsma, L., Avramut, M. C., Wiersma, L. E., van den Berg, B. M., Leuning, D. G., et al. (2018). Renal subcapsular transplantation of PSC-derived kidney organoids induces neo-vasculogenesis and significant glomerular and tubular maturation in vivo. Stem Cell Rep. 10, 751–765. doi:10.1016/J.STEMCR.2018.01.041
Vilarino, M., Soto, D. A., Bogliotti, Y. S., Yu, L., Zhang, Y., Wang, C., et al. (2020). Derivation of sheep embryonic stem cells under optimized conditions. Reproduction 160, 761–772. doi:10.1530/REP-19-0606
Vos, J. G., Kreeftenberg, J. G., Kruijt, B. C., Kruizinga, W., and Steerenberg, P. (1980). The athymic nude rat: II. Immunological characteristics. Clin. Immunol. Immunopathol. 15, 229–237. doi:10.1016/0090-1229(80)90033-1
Waddington, C. H. (1934). Experiments on embryonic induction. J. Exp. Biol. 11, 224–227. doi:10.1242/JEB.11.3.224
Waddington, C. H., and Schmidt, G. A. (1933). Induction by heteroplastic grafts of the primitive streak in birds. Wilhelm Roux’ Arch. für Entwicklungsmechanik Org. 128, 522–563. doi:10.1007/BF00649863
Wainwright, J. M., Czajka, C. A., Patel, U. B., Freytes, D. O., Tobita, K., Gilbert, T. W., et al. (2010). Preparation of cardiac extracellular matrix from an intact porcine heart. Tissue Eng. Part C Methods 16, 525–532. doi:10.1089/TEN.TEC.2009.0392
Ware, C. B., Nelson, A. M., Mecham, B., Hesson, J., Zhou, W., Jonlin, E. C., et al. (2014). Derivation of naïve human embryonic stem cells. Proc. Natl. Acad. Sci. U. S. A. 111, 4484–4489. doi:10.1073/pnas.1319738111
Weinberger, L., Ayyash, M., Novershtern, N., and Hanna, J. H. (2016). Dynamic stem cell states: Naive to primed pluripotency in rodents and humans. Nat. Rev. Mol. Cell Biol. 17, 155–169. doi:10.1038/nrm.2015.28
Wen, B., Li, E., Ustiyan, V., Wang, G., Guo, M., Na, C. L., et al. (2021). In vivo generation of lung and thyroid tissues from embryonic stem cells using blastocyst complementation. Am. J. Respir. Crit. Care Med. 203, 471–483. doi:10.1164/rccm.201909-1836OC
Weymann, A., Loganathan, S., Takahashi, H., Schies, C., Claus, B., Hirschberg, K., et al. (2011). Development and evaluation of a perfusion decellularization porcine heart model--generation of 3-dimensional myocardial neoscaffolds. Circ. J. 75, 852–860. doi:10.1253/CIRCJ.CJ-10-0717
Weymann, A., Patil, N. P., Sabashnikov, A., Jungebluth, P., Korkmaz, S., Li, S., et al. (2014). Bioartificial heart: A human-sized porcine model--the way ahead. PLoS One 9, e111591. doi:10.1371/JOURNAL.PONE.0111591
Williams, T. J., Munro, R. K., and Shelton, J. N. (1990). Production of interspecies chimeric calves by aggregation of Bos indicus and Bos taurus demi-embryos. Reprod. Fertil. Dev. 2, 385–394. doi:10.1071/RD9900385
Wilmut, I., Schnieke, A. E., McWhir, J., Kind, A. J., and Campbell, K. H. S. (1997). Viable offspring derived from fetal and adult mammalian cells. Nature 385, 6619810–6619813. doi:10.1038/385810a0
Wu, J., and Barbaric, I. (2021). Fitness selection in human pluripotent stem cells and interspecies chimeras: Implications for human development and regenerative medicine. Dev. Biol. 476, 209–217. doi:10.1016/J.YDBIO.2021.03.025
Wu, J., Okamura, D., Li, M., Suzuki, K., Luo, C., Ma, L., et al. (2015). An alternative pluripotent state confers interspecies chimaeric competency. Nature 521, 316–321. doi:10.1038/nature14413
Wu, J., Platero-Luengo, A., Sakurai, M., Sugawara, A., Gil, M. A., Yamauchi, T., et al. (2017). Interspecies chimerism with mammalian pluripotent stem cells. Cell 168, 473–486.e15. doi:10.1016/j.cell.2016.12.036
Yagi, M., Kishigami, S., Tanaka, A., Semi, K., Mizutani, E., Wakayama, S., et al. (2017). Derivation of ground-state female ES cells maintaining gamete-derived DNA methylation. Nature 548, 224–227. doi:10.1038/nature23286
Yamada, K., Shimizu, A., Ierino, F. L., Utsugi, R., Barth, R. N., Esnaola, N., et al. (1999). Thymic transplantation in miniature swine. I. Development and function of the “thymokidney. Transplantation 68, 1684–1692. doi:10.1097/00007890-199912150-00011
Yamada, K., Shimizu, A., Utsugi, R., Ierino, F. L., Gargollo, P., Haller, G. W., et al. (2000). Thymic transplantation in miniature swine. II. Induction of tolerance by transplantation of composite thymokidneys to thymectomized recipients. J. Immunol. 164, 3079–3086. doi:10.4049/JIMMUNOL.164.6.3079
Yamaguchi, T., Sato, H., Kato-Itoh, M., Goto, T., Hara, H., Sanbo, M., et al. (2017). Interspecies organogenesis generates autologous functional islets. Nature 542, 191–196. doi:10.1038/nature21070
Yan, J., Xu, L., Welsh, A. M., Hatfield, G., Hazel, T., Johe, K., et al. (2007). Extensive neuronal differentiation of human neural stem cell grafts in adult rat spinal cord. PLoS Med. 4, e39. doi:10.1371/JOURNAL.PMED.0040039
Yang, B., Zhang, Y., Zhou, L., Sun, Z., Zheng, J., Chen, Y., et al. (2010). Development of a porcine bladder acellular matrix with well-preserved extracellular bioactive factors for tissue engineering. Tissue Eng. Part C Methods 16, 1201–1211. doi:10.1089/TEN.TEC.2009.0311
Yang, J., Ryan, D. J., Wang, W., Tsang, J. C. H., Lan, G., Masaki, H., et al. (2017). Establishment of mouse expanded potential stem cells. Nature 550, 393–397. doi:10.1038/nature24052
Yang, X., Zhou, J., He, J., Liu, J., Wang, H., Liu, Y., et al. (2018). An immune system-modified rat model for human stem cell transplantation research. Stem Cell Rep. 11, 514–521. doi:10.1016/J.STEMCR.2018.06.004
Yang, Y., Liu, B., Xu, J., Wang, J., Wu, J., Shi, C., et al. (2017). Derivation of pluripotent stem cells with in vivo embryonic and extraembryonic potency. Cell 169, 243–257.e25. doi:10.1016/j.cell.2017.02.005
Yang, Y., Wang, K., Wu, H., Jin, Q., Ruan, D., Ouyang, Z., et al. (2016). Genetically humanized pigs exclusively expressing human insulin are generated through custom endonuclease-mediated seamless engineering. J. Mol. Cell Biol. 8, 174–177. doi:10.1093/JMCB/MJW008
Yao, J., Wang, Y., Cao, C., Song, R., Bi, D., Zhang, H., et al. (2021). CRISPR/Cas9-mediated correction of MITF homozygous point mutation in a Waardenburg syndrome 2A pig model. Mol. Ther. Nucleic Acids 24, 986–999. doi:10.1016/J.OMTN.2021.04.009
Yasuhara, T., Matsukawa, N., Hara, K., Yu, G., Xu, L., Maki, M., et al. (2006). Transplantation of human neural stem cells exerts neuroprotection in a rat model of Parkinson’s disease. J. Neurosci. 26, 12497–12511. doi:10.1523/JNEUROSCI.3719-06.2006
Ying, Q. L., Wray, J., Nichols, J., Batlle-Morera, L., Doble, B., Woodgett, J., et al. (2008). The ground state of embryonic stem cell self-renewal. Nature 453, 519–523. doi:10.1038/nature06968
Yu, L., Wei, Y., Sun, H. X., Mahdi, A. K., Pinzon Arteaga, C. A., Sakurai, M., et al. (2021). Derivation of intermediate pluripotent stem cells amenable to primordial germ cell specification. Cell Stem Cell 28, 550–567.e12. doi:10.1016/j.stem.2020.11.003
Zhang, B., Li, H., Hu, Z., Jiang, H., Stablewski, A. B., Marzullo, B. J., et al. (2021). Generation of mouse–human chimeric embryos. Nat. Protoc. 16 (8), 3954–3980. doi:10.1038/s41596-021-00565-7
Zhang, H., Huang, J., Li, Z., Qin, G., Zhang, N., Hai, T., et al. (2018). Rescuing ocular development in an anophthalmic pig by blastocyst complementation. EMBO Mol. Med. 10 (12). doi:10.15252/emmm.201808861
Zheng, C., Hu, Y., Sakurai, M., Pinzon-Arteaga, C. A., Li, J., Wei, Y., et al. (2021). Cell competition constitutes a barrier for interspecies chimerism. Nature 592, 272–276. doi:10.1038/S41586-021-03273-0
Zhou, H., Kitano, K., Ren, X., Rajab, T. K., Wu, M., Gilpin, S. E., et al. (2018). Bioengineering human lung grafts on porcine matrix. Ann. Surg. 267, 590–598. doi:10.1097/SLA.0000000000002129
Keywords: interspecies blastocyst complementation, xenotransplantation, bioengineering, human organ generation, swine model, human pluripotent stem cell, chimera
Citation: Sarmah H, Sawada A, Hwang Y, Miura A, Shimamura Y, Tanaka J, Yamada K and Mori M (2023) Towards human organ generation using interspecies blastocyst complementation: Challenges and perspectives for therapy. Front. Cell Dev. Biol. 11:1070560. doi: 10.3389/fcell.2023.1070560
Received: 15 October 2022; Accepted: 05 January 2023;
Published: 19 January 2023.
Edited by:
Jun Wu, University of Texas Southwestern Medical Center, United StatesReviewed by:
Fabian Suchy, Stanford University, United StatesDaniel Garry, University of Minnesota Twin Cities, United States
Copyright © 2023 Sarmah, Sawada, Hwang, Miura, Shimamura, Tanaka, Yamada and Mori. This is an open-access article distributed under the terms of the Creative Commons Attribution License (CC BY). The use, distribution or reproduction in other forums is permitted, provided the original author(s) and the copyright owner(s) are credited and that the original publication in this journal is cited, in accordance with accepted academic practice. No use, distribution or reproduction is permitted which does not comply with these terms.
*Correspondence: Munemasa Mori, bW00NDUyQGN1bWMuY29sdW1iaWEuZWR1